- 1Department of Microbiology and Immunology, University of Louisville, Louisville, KY, United States
- 2Center for Predictive Medicine, College of Medicine, University of Louisville, Louisville, KY, United States
While most bacterial species taken up by macrophages are degraded through processing of the bacteria-containing vacuole through the endosomal-lysosomal degradation pathway, intravacuolar pathogens have evolved to evade degradation through the endosomal-lysosomal pathway. All intra-vacuolar pathogens possess specialized secretion systems (T3SS-T7SS) that inject effector proteins into the host cell cytosol to modulate myriad of host cell processes and remodel their vacuoles into proliferative niches. Although intravacuolar pathogens utilize similar secretion systems to interfere with their vacuole biogenesis, each pathogen has evolved a unique toolbox of protein effectors injected into the host cell to interact with, and modulate, distinct host cell targets. Thus, intravacuolar pathogens have evolved clear idiosyncrasies in their interference with their vacuole biogenesis to generate a unique intravacuolar niche suitable for their own proliferation. While there has been a quantum leap in our knowledge of modulation of phagosome biogenesis by intravacuolar pathogens, the detailed biochemical and cellular processes affected remain to be deciphered. Here we discuss how the intravacuolar bacterial pathogens Salmonella, Chlamydia, Mycobacteria, Legionella, Brucella, Coxiella, and Anaplasma utilize their unique set of effectors injected into the host cell to interfere with endocytic, exocytic, and ER-to-Golgi vesicle traffic. However, Coxiella is the main exception for a bacterial pathogen that proliferates within the hydrolytic lysosomal compartment, but its T4SS is essential for adaptation and proliferation within the lysosomal-like vacuole.
Introduction
Immune cells are equipped with a variety of receptors that recognize foreign material and particles permitting them to internalize the particles into a plasma-membrane derived vacuole that follows a maturation process that ultimately yields a phagolysosome (Vieira et al., 2002). Additionally, non-immune cells are equipped with receptors utilized by bacterial pathogens such as Chlamydia to enter by receptor-mediated endocytosis (Hybiske and Stephens, 2007a). Phagocytosis is a form of endocytosis that refers to cellular uptake of large particles and is initiated by the interaction of surface receptors with their cognate ligand (Vieira et al., 2002; Kinchen and Ravichandran, 2008). Upon phagocytosis, the nascent phagosome undergoes a complex sequence of maturation events governed by regulators of the endosomal-lysosomal degradation pathway that yields an acidic degradative compartment designated as the phagolysosome (Pitt et al., 1992; Vieira et al., 2002; Rama et al., 2015). Therefore, successful evolution of intra-vacuolar pathogens is dependent on their capacity to evade innate immune pathways and modulate biogenesis of their vacuole and adaptation to the unique micro-environment within the vacuole (Mnich et al., 2020). Depending on the phagocytosed bacterial pathogen, the default maturation of the phagosome along the endosomal-lysosomal pathway is overridden by specific pathogenic factors that interfere with the fate of the pathogen-containing vacuole (Do et al., 2016). The interference and divergence of the pathogen-containing vacuole from the default endosomal-lysosomal pathway is at the crux of the successful evolution of many intra-vacuolar pathogens to adapt to the intra-vacuolar microenvironment and inflict pathology and disease (Çakır et al., 2020; Leseigneur et al., 2020; Sachdeva and Sundaramurthy, 2020). Despite being an accidental human pathogen Legionella has evolved similar lysosomal evasion mechanisms to avoid killing by its natural amoeba hosts and human macrophages.
Intra-vacuolar bacterial pathogens have evolved to manipulate interactions between the pathogen-containing vacuole and endocytic vesicles to interfere with the progressive transfer of endo/lysosomal membrane and luminal constituents to the pathogen-containing vacuole (Vieira et al., 2002; Mitchell et al., 2016). There are several important regulators that govern endosomal maturation that have been identified including the Rab5, Rab7, and Rab 9 GTPases (Rama et al., 2015; Kucera et al., 2016; Szulc-Dabrowska et al., 2020). Pathogens also undergo various aspects of gene regulation to adapt to the intracellular environment (Chakravarty and Massé, 2019; Leipheimer et al., 2019).
Bacterial pathogens that have adopted an intracellular lifestyle have evolved different strategies to avoid trafficking to the degradative phagolysosome (Duclos et al., 2000). A distinct group of bacterial pathogens, such as Listeria, Shigella, and Rickettsia, escape from the phagosome to the cytosol (Ray et al., 2009; Curto et al., 2019a; Curto et al., 2019b; Green et al., 2020; Wang et al., 2020b). The cytosolic pathogens have evolved mechanisms to evade innate immune mechanisms of macrophages such as autophagy (Thomas et al., 2020), activation of the inflammasomes (Snaka and Fasel, 2020), and other host innate sensing of foreign material within the cytosol, and these are reviewed elsewhere (Kubelkova and Macela, 2019; Thakur et al., 2019). In contrast, we focus this review on the group of bacterial pathogens that reside and proliferate within a modified vacuole whose altered biogenesis is governed by the interaction of injected pathogenic effectors with cellular targets leading to its diversion from the default endosomal-lysosomal pathway. These intra-vacuolar pathogens have evolved to alter their initial phagosomal compartment in order to halt its maturation or divert it from the endocytic pathway to avoid the fatal fate of degradation within the phagolysosome (Garin et al., 2001). The intra-vacuolar niche within the host cell benefits intracellular pathogens as there is limited competition with other bacteria, the intracellular environment can provide vital nutrients, and the pathogen is no longer susceptible to complement or neutralizing antibodies (Casadevall, 2008; Casadevall and Pirofski, 2011). However, although most intra-vacuolar pathogens proliferate within a phagosome that is stalled from maturing into a phagolysosome, the pathogenic factors and biochemical mechanisms involved in this interference are all idiosyncratic and there is no common theme among intra-vacuolar pathogens in their evolution and interference with biogenesis of their replicative vacuoles (Figure 1).
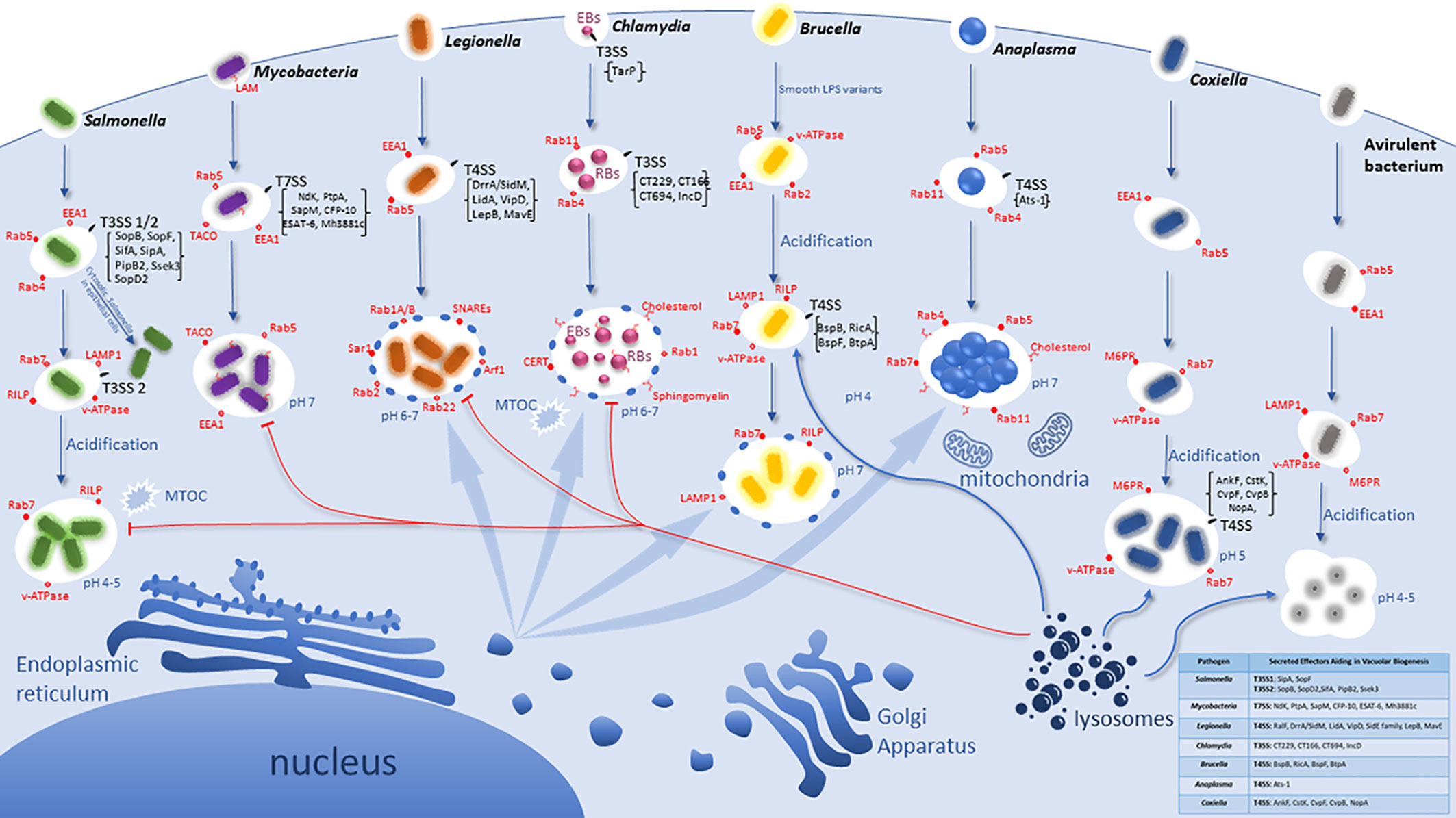
Figure 1 Idiosyncratic Biogenesis of the Vacuoles harboring Intracellular bacterial Pathogens. Pathogens have evolved distinct pathogenic factors and biochemical mechanisms to evade degradation upon entering host cells and enable their proliferation within modified vacuoles. All the listed pathogens have evolved specialized secretion machineries (Type 3 - Type 7) that inject effector proteins into the host cytosol to interact and modulate specific host cell proteins and/or processes. However, the T4SS of Coxiella is not functional until vacuolar acidification and is specifically used in replication but may not be involved in vacuole biogenesis. These seven intra-vacuolar bacterial pathogens, Salmonella, Mycobacteria, Legionella, Chlamydia, Brucella, Anaplasma, and Coxiella, interfere with endocytic, exocytic and/or ER-to-Golgi vesicle trafficking to evade lysosomal degradation. Salmonella and Coxiella proliferate within an acidified late endosome/lysosomal-like vacuole whereas Legionella, Chlamydia, Anaplasma as well as Brucella intercept and modulate ER-to Golgi vesicles for their vacuole biogenesis.
The abundant series of steps, molecules, and checkpoints that must take place for proper endosomal maturation allow for many possible host targets to be interfered with and modulated by pathogens, and thus potential evolution and adaptation of the pathogen to exploit the endosomal pathway to the pathogen advantage (Rama et al., 2015). Intracellular bacteria have evolutionarily gained the capacity to subvert signaling and trafficking pathways by employing T3SS-T7SS (Weber et al., 2009; Green and Mecsas, 2016; Monjarás Feria and Valvano, 2020). These secretion systems are multiprotein complexes that span both bacterial membranes and inject a variety of effector proteins directly into the host cytoplasm via a molecular nano-syringe (Ninio and Roy, 2007; Schroeder and Hilbi, 2008; Steele-Mortimer, 2008; Weber et al., 2009; Green and Mecsas, 2016). Bacterial effector proteins modulate a myriad of host cell processes and play an essential role in mediating vesicle trafficking pathways controlled by Rab GTPases and V-ATPases involved in phagosome biogenesis (Weber et al., 2009; Rama et al., 2015). Each of the following Gram-negative pathogens has evolved to be equipped with a unique toolbox of a set of effectors that are injected into the host cell, with a myriad of effects on cell biology. Here, we focus on the intra-vacuolar pathogens Salmonella, Mycobacteria, Legionella, Chlamydia, Brucella, Anaplasma, and Coxiella to discuss their evolution and adaptation to modulate and exploit host endocytic and exocytic trafficking pathways to create an idiosyncratic vacuolar niche suitable for intra-vacuolar proliferation (Figure 1).
The Late Endosome-Like Vacuole of Salmonella enterica
Salmonella enterica serovar Typhimurium is a major cause of food‐borne enterocolitis in humans and a systemic, typhoid-like disease in genetically susceptible mice (Tsolis et al., 1999; Brumell and Scidmore, 2007). These bacteria are facultative intracellular pathogens that initially interact with intestinal epithelial cells at the onset of infection primarily through the M cells of Peyer’s patches, however they infect a wide variety of cell types in their host during systemic infection (Gordon et al., 2008). S. enterica serovar Typhimurium invades and replicates within host cells using two different T3SS and interferes with the host cell cycle to enhance invasion (Galan and Wolf-Watz, 2006; Brumell and Scidmore, 2007; Mambu et al., 2020). The first injected group of effectors is delivered across the epithelial plasma membrane by the T3SS1 (Lou et al., 2019) to modulate host signal transduction pathways, including the activation of Rho family GTPases, to induce actin rearrangements that drive the ruffling of the cell surface, IAM formation (fluid-filled infection associated macropinosomes), and the uptake of the bacteria into a Salmonella-containing vacuole (SCV) (Schlumberger and Hardt, 2006; Ramos-Morales, 2012; Stevenin et al., 2019). This is an essential pathogenic adaptation to infect non-phagocytic cells, since the recognition of the T3SS by macrophages induces activation of the inflammasome, resulting in caspase-1-mediated pyroptosis (Brennan and Cookson, 2000; Wang et al., 2020a). The T3SS2 of S. enterica is expressed within the SCV in response to acidic pH and Mg2+ limitations (Ramos-Morales, 2012). The SCV is a pleomorphic organelle characterized by filamentous projections termed Salmonella-induced filaments (SIFs) (Garcia-del Portillo et al., 1993; Kumar and Valdivia, 2009). Some late endosome-lysosomes markers accumulate on the SCV and enable its traffic along microtubules to the microtubule-organizing center (Bakowski et al., 2008; Kumar and Valdivia, 2009; Gao et al., 2018). Thus, the modification of the SCV is governed by T3SS2-secreted effector proteins located on Salmonella pathogenicity island 2 (SPI-2), allowing the bacteria to sense the acidic vacuolar environment, modulate vesicle traffic, and ensue replication (Kenney, 2019).
SCV biogenesis can be divided into three stages (Figure 1): early (10 min–1 h post infection), intermediate (1 h–4 h), and late (>4 h) (Ramos-Morales, 2012). Initially in epithelial cells, the SCV interacts with early endosomes and acquires the markers Rab4, Rab5, and EEA1 (Steele-Mortimer et al., 1999; Smith et al., 2005). Overexpression of Rab5 causes the retention of the early endosomal marker EEA1 and promotes homotypic early endosome fusion (Baldeon et al., 2001; Brumell and Scidmore, 2007). Approximately 15 to 60 minutes post infection, lysosomal glycoprotein (Lgp) LAMP-1 is recruited to the SCV membrane. The SCVs are thought to acquire Lgp by interacting with a Rab7-positive/Lgp-positive/manose-6-phosphate (M6PR)-negative/Cathepsin D-negative vesicles (Meresse et al., 1999). During this stage of SCV maturation, active Rab7 recruits its effector RILP and promotes centripetal SCV movement to the microtubule organizing center (Harrison et al., 2004). This is characteristic of the intermediate state in SCV development (Ramos-Morales, 2012). The vATPase is acquired by the SCV leading to its acidification with a pH of 4.0-5.0, which is required for the expression of various pathogen genes required for adaptation and proliferation within the SCV (Buchmeier and Heffron, 1991; Mills and Finlay, 1998; Garvis et al., 2001; Cuellar-Mata et al., 2002; Brumell and Scidmore, 2007; Smith et al., 2007; Lahiri et al., 2010).
During the late stage of SCV maturation a set of T3SS effectors generate different types of tubular perinuclear networks, SIFs, Salmonella-induced secretory carrier membrane protein 3 (SCAMP3) tubules (SISTs), and LAMP1-negative tubules (LNTs) (Ramos-Morales, 2012). However, because SCVs do not fuse with lysosomes, it is suggested that S. enterica can modify the Rab network associated with phagosome maturation and the Lgp acquisition that contributes to the control of SCV during infection is promoted by the positioning of Rab7 (Brumell and Scidmore, 2007). Additionally, the SopB translocated effector manipulates the SCV surface charge by dissociating several host-cell endocytic trafficking proteins from the SCV: inhibiting lysosomal fusion (Bakowski et al., 2010; Kaur and Jain, 2012) (Figure 1). SopB has also been found to promote IAM formation and also trigger the formation of SVATs (spacious vacuole-associated tubules), resulting in the shrinkage of the vacuole (Stevenin et al., 2019). To evade the innate immune response of macrophages, the injected SopF effector is involved in blocking autophagy (Xu et al., 2019).
Upon its maturation, the SCV becomes a replicative niche for Salmonella, although there are a small number of WT cytosolic bacteria that routinely escape from the SCV, Salmonella-induced filament A (sifA) mutants readily escape into the cytoplasm (Beuzon et al., 2000; Kumar and Valdivia, 2009). This indicates a role for SifA in maintaining integrity for the SCV and SifA is likely to play a role in systemic disease as sifA mutants fail to establish infection in mice (Stein et al., 1996; Kumar and Valdivia, 2009; Liss et al., 2017). Salmonella injects the SopD2 effector, which modulates Rab7 activity leading to interference with endosome-to-lysosome trafficking (D'Costa et al., 2015). In addition to SCV escape in epithelial cells, a subset of SCVs follow retrograde vesicle traffic from the Golgi back to the endoplasmic reticulum, which is also dependent on the T3SS2 effector PipB2 associated with a decrease in the T3SS1 effector SipA (Ramos-Morales, 2012). Kinesin is involved in maintaining the structure and position of the Golgi apparatus along with transport of vesicles (Goldstein and Philp, 1999; Allan et al., 2002). Work performed by Boucrot et al. determined SifA targeted SKIP, a host protein that down-regulates the recruitment of kinesin on the SCV (Boucrot et al., 2005). Recently, the SifA/SKIP complex has been shown to be necessary for the activation of the kinesin-1 recruited by PipB2, revealing a functional interaction important for formation of tubules in membrane exchange and nutrient supply (Alberdi et al., 2020). The SseK3 effector targets various host small GTPases (Gan et al., 2020).
In epithelial cells, roughly 10% of intra-vacuolar bacteria can escape the SCV and reside in the host cytoplasm, where the bacteria can proliferate at high rates to reach a progeny of >100 cfu’s per infected cell (Kumar and Valdivia, 2009; Knodler, 2015; Castanheira and Garcia-Del Portillo, 2017). Interestingly, S. Typhimurium does not escape into the cytosol in fibroblasts, allowing endosomal and lysosomal membranes to accumulate around the SCV forming large aggregates that engage the autophagy machinery in an ATG9A-independent manner (Kageyama et al., 2011; Lopez-Montero et al., 2016). Furthermore, a recent study concluded Rab7-positive vesicles are more acidic as compared to the Arl8b-positive vesicles (Johnson et al., 2016). Arl8b, a small lysosomal GTPase, promotes membrane localization of Vps41, a HOmotypic fusion and Protein Sorting complex (HOPS). This may suggest that Salmonella recruits late endosomal and lysosomal host membrane proteins for access to host nutrients to ensure its intracellular survival and replication (Sindhwani et al., 2017). More recently, Eswarappa et al. concluded that a majority of SCVs only have one bacterium per vacuole, ultimately increasing the vacuolar load per cell, the host would have to target each SCV separately with lysosomes and other microbicidal agents potentially affecting the susceptibility of the host cell to other pathogens (Eswarappa et al., 2010). Consistent with the IAM and/or SVAT formation causing the SCV to undergo size modifications, the number of bacteria present in the SCV causes vacuole size increase and decrease.
Both SCV escape and retrograde movement of the SCV have been associated with the ability of Salmonella to achieve cell-to-cell transfer in order to repeat the intracellular cycle (Knodler et al., 2010; Ramos-Morales, 2012). Thus, Salmonella has evolved multiple mechanisms for adaptation and survival within an idiosyncratic acidified late endosome-like vacuole. Ultimately, its successful pathogenic evolution and adaptation to the intra-vacuolar microenvironment is dependent of its ability of membrane remodeling, interactions with the endosomal/lysosomal pathway, actin rearrangements and microtubule-based movement.
The Early Endosome-Like Vacuole of Mycobacterium tuberculosis
Mycobacterium tuberculosis is the causative agent of Tuberculosis, with an estimated 10 million infected last year (Clemens and Horwitz, 1995; Kumar and Valdivia, 2009). While the majority of infected people are asymptomatic, 1-2 million people die annually of the disease. The slow-growing and chronic nature of the infection and the difficulty in adherence to the long-term therapy by patients has potentially contributed to the rise of hyper-virulent multidrug-resistant strains (Clemens and Horwitz, 1995; Kumar and Valdivia, 2009; Matteelli et al., 2014). M. tuberculosis in most cases induces irreversible necrosis of lung tissue as a result of exacerbated inflammation and enhanced recruitment of myeloid cells with progression to chronic disease associated with a reduction in inflammatory mediators (Almeida et al., 2017).
M. tuberculosis is a highly aerobic bacteria that primarily infects macrophages and monocytes (Clemens and Horwitz, 1995). However, outside the lungs M. tuberculosis can disseminate to any organ via the lymphatic system (Bussi and Gutierrez, 2019; Moule and Cirillo, 2020). Infected alveolar macrophages elicit recruitment of more monocytes to the infection site creating a granuloma within the infected lung to be the sites of chronic inflammation (Arora et al., 2020) and a growing niche for replication where the pathogen utilize lipids for nutrition (Cambier et al., 2014; Warsinske et al., 2017; Del Portillo et al., 2019; Maurya et al., 2019) and targets host mitochondria to trigger metabolic changes (Mohareer et al., 2020).
A prominent mannose-containing lipoglycan of the M. tuberculosis cell wall, the terminal mannose-capped lipoarabinomannan (ManLAM) is a high molecular mass amphipathic lipoglycan that plays a crucial role in mycobacterial survival. ManLAM facilitates M. tuberculosis entry into phagocytes, regulates the intracellular trafficking network as well as the immune responses of infected host cells (Kang et al., 2005; Turner and Torrelles, 2018). After entry into macrophages, M. tuberculosis resides in a vacuole that is arrested from maturation at the early endosomal stage where early endosomal markers (EEA1, Rab5) accumulate on the pathogen-containing vacuole (Figure 1) (Clemens and Horwitz, 1995; Kumar and Valdivia, 2009). The M. tuberculosis-containing vacuole has a near-neutral pH and retains its ability to interact with early endosomes. In addition to ManLAM, it is suggested that the M. tuberculosis glycosylated phosphatidylinositol lipoarabinomannan (LAM) excludes V-ATPase from the vacuole and limits the induction of host autophagy (Vergne et al., 2004; Augenstreich and Briken, 2020). Ferrari et al. has reported that 90% of M. tuberculosis vacuoles retain Tryptophan Aspartate- Containing Coat protein/Coronin 1 (TACO) and macrophages incubated with dead bacteria shortly release TACO, suggesting long-term retention of TACO on the Mycobacteria containing vacuole (MCV) contribute to inhibition of phagosomal maturation (Ferrari et al., 1999).
In addition to the role of LAM and ManLAM, M. tuberculosis injects protein effectors through its ESX T7SS (Guo et al., 2019), to maintain the early endosomal characteristics of the vacuole (Crosskey et al., 2020) and modulate autophagosome formation (Garg et al., 2020). Mutants of Mycobacteria that lack the ESX-1 secretion system are less efficient in surviving inside macrophages (MacGurn and Cox, 2007). Phosphoinositides are low-abundance lipid constituents on eukaryotic membranes that determine vesicle trafficking/organelle identity within eukaryotic cells (Di Paolo and De Camilli, 2006; Sasaki et al., 2009). Mycobacteria are able to disrupt the host cell phosphoinositide pattern and other processes through secretory phosphatases (Koliwer-Brandl et al., 2019). ManLAM co-operates with the secreted acid phosphatase SapM to block phagosome fusion with late endosomes by inhibiting PI3P deposition on the phagosome surface (Deretic et al., 2006; Wong et al., 2018). Specifically, SapM inhibits PI3P deposition on the phagosome surface via PI3P hydrolysis, contributing to inhibition of phagosomal maturation (Vergne et al., 2005) The PtpA T7SS-injected effector protein is a low molecular weight tyrosine phosphatase (Cowley et al., 2002) essential for growth in human macrophages (Bach et al., 2008). PtpA binds to the host H subunit of V-ATPase rendering it unable to bind to the M. tuberculosis containing vacuole and impedes acidification of the M. tuberculosis-containing vacuole (Wong et al., 2011; Saha et al., 2020). PtpA colocalizes with a regulator of membrane fusion, VPS33B, in the host cytosol, resulting in dephosphorylation of the VPS33B subunit of the Homotypic Fusion and Vacuole Protein Sorting (HOPS) complex. In infected macrophages, this process prevents anchoring of the HOPS complex to SNARE molecules (Bach et al., 2008). PtpA interaction with host V-ATPase is required for the dephosphorylation of VPS33B and subsequent exclusion of V-ATPase from the phagosome, inhibiting phagosome-lysosome fusion during M. tuberculosis infection (Wong et al., 2011). Protein kinase A (PtkA), encoded on the same operon as PtpA, increases PtpA activity via phosphorylation, thereby enhancing PtpA phosphatase activity (Zhou et al., 2015; Wong et al., 2018). The NdK effector, a nucleoside diphosphate kinase, dephosphorylates cellular Rab7-GTP and Rab5-GTP (Sun et al., 2010). This prevents “Rab switching” and Rab7- dependent fusion of the vacuole which halts endosomal maturation from lysosomal fusion. The eukaryotic-type protein kinase G (PknG) is a member of the STPK family in M. tuberculosis and is associated with mycobacterial survival within macrophages, metabolic regulation, and inhibits phagosome lysosome fusion (Av-Gay and Everett, 2000; Cowley et al., 2004; Scherr et al., 2007; O'Hare et al., 2008; Ge et al., 2021). PknG inhibits autophagosome maturation by phosphorylating TBC1D4/AS160 (TBC1 domain family member 4) to suppress its GTPase-activating protein (GAP) activity toward RAB14 and also by binding to host small GTPase RAB14 to block RAB14-GTP hydrolysis (Ge et al., 2021).
Recently, M. tuberculosis has been found to rapidly escape from phagosomes within infected macrophages via the activation of host cytosolic phospholipase A2 and establish residence in the cytoplasm of the host cell (Jamwal et al., 2016). Individual M. tuberculosis strains display a strain-specific capacity to escape from phagosomes, with the predominant population of JAL2287, JAL2549, and MYC431 bacteria strains localizing to the cytosol (Jamwal et al., 2016). Previously, a related species Mycobacterium marinum, has shown to escape phagosomes and can recruit host cell cytoskeletal factors to induce actin polymerization leading to direct cell to cell infection (Stamm et al., 2003). Also, virulent strains of M. tuberculosis have been shown to translocate from the phagosome into the cytosol of dendritic cells by interfering with the TLR-2-MyD88 signaling pathway (Rahman et al., 2014). The effectors PtpA and SapM are also translocated M. marinum phosphatases, which dephosphorylate phosphatidylinositol-3-phosphate (PtdIns3P) on the cytoplasmic side of MCV, modulating the MCV PI pattern (Koliwer-Brandl et al., 2019). PtdIns3P is required for phagolysosome progression along the endosomal pathway and a reduction of PtdIns3P on MCV likely impairs lysosomal fusion thus promoting intracellular replication of M. marinum and escape into the cytoplasm (Murray et al., 2002; Koliwer-Brandl et al., 2019). Additionally, Mh3881c, an ESX-1 protein, has been found to co-secrete with CFP-10/ESAT-6 and this co-dependent secretion is required for M. marium intracellular growth in macrophages and correlates with its role in inhibiting phagosome maturation (Xu et al., 2007). This shows plasticity in the adaptation of M. tuberculosis and M. marinum for survival and growth in susceptible hosts. Thus, M. tuberculosis have evolved to arrest phagosome maturation at an early stage of biogenesis through exclusion of the V-ATPase and directly alters various host signaling pathways through the secretion of effector proteins that alter phagosomal maturation to generate an idiosyncratic early endosome-like replicative niche (Bussi and Gutierrez, 2019).
The ER-Derived Vacuole of Legionella pneumophila
Legionella pneumophila is a Gram-negative, aquatic, facultative intracellular bacterium that is the causative agent of Legionnaires’ disease (Fraser et al., 1977; McDade et al., 1977). It naturally infects a wide variety of freshwater amoebas and other protists, but the organism can also accidentally infect humans upon the inhalation of contaminated aerosols (Albert-Weissenberger et al., 2007; Best and Abu Kwaik, 2018). Once inhaled, the pathogen is able to invade and replicate in alveolar macrophages (Aridor et al., 1995; Albert-Weissenberger et al., 2007).
The Dot/Icm-encoded T4SS of L. pneumophila delivers effector proteins into the mammals and protists hosts (Segal et al., 2005). The Dot/Icm-encoded T4SS of L. pneumophila injects ~350 effector proteins into the host cytosol to target specific host processes and pathways in order to modulate biogenesis of the Legionella-containing vacuole (LCV) (Roy et al., 1998; Coers et al., 1999; Segal et al., 2005; Li et al., 2020). Within alveolar macrophages and the amoeba hosts, the LCV evades fusion with vesicles of the endosome-lysosomal pathway (Swanson and Isberg, 1995), and intercepts ER-to-Golgi vesicle traffic to become an ER-derived vacuole associated with polyubiquitinated proteins (Figure 1) (Horwitz, 1983; Swanson and Isberg, 1995; Kitao et al., 2020). The ER-to-Golgi vesicle traffic is regulated by Rab1A/Rab1B (Nuoffer et al., 1994; Wilson et al., 1994), Rab2 (Tisdale et al., 1992), and at least one SNARE complex composed of one v-SNARE (Sec22b) and three cognate t-SNAREs (syntaxin 5, membrin, and Bet1) (Hay et al., 1997; Xu et al., 2000). Both Rab1 and Arf1 have been found to be localized to the LCV by 30 min post infection (Nagai et al., 2002; Derre and Isberg, 2004; Kagan et al., 2004). In addition, Sar1 and Arf1, two small GTPases, regulate the formation of COPII and COPI-coated vesicles and are required for the production of early secretory vesicles (Aridor et al., 1995; Scales et al., 1997; Duden, 2003). The expression of dominant interfering mutants in the three small GTPases (Sar1, Rab1, and Arf1) inhibits the formation of LCV and decreases intracellular survival of the organism (Derre and Isberg, 2004; Kagan et al., 2004; Robinson and Roy, 2006).
The function of many Dot/Icm-injected effectors is still to be determined. However, among the characterized effectors involved in the LCV biogenesis, RalF functions as a GEF to recruit the ADP-ribosylation factor 1 (Arf1) to the LCV (Nagai et al., 2002). This enables the GEF-like RalF effector to modulate membrane transport in the secretory pathway (Alix et al., 2012). Additionally, the DrrA/SidM effector functions to recruit and regulate Rab1 activity at the LCV. DrrA/SidM preferentially recruits Rab1 and tethers ER-derived vesicles to the LCV, mediated by another L. pneumophila effector, LidA (Ninio and Roy, 2007). DrrA/SidM may compete with endogenous guanine nucleotide exchange factors (GEFs) to redirect Rab1 from its normal secretory intracellular localization to plasma membrane-derived vesicles (Murata et al., 2006). The LepB effector accumulates on the LCV as DrrA/SidM and Rab1 cycle off and has been shown to function as a GTPase activating protein (GAP) for Rab1 (Ingmundson et al., 2007; Mishra et al., 2013). While LidA binds several Rab GTPases including Rab1, it may sequester Rab proteins or tether ER-derived vesicles with the LCV to facilitate SNARE-mediated fusion (Mishra et al., 2013). The VipD effector of L. pneumophila belongs to a family of bacterial effectors that contain the N-terminal lipase domain and a C-terminal domain (Ku et al., 2012). VipD localizes to early endosomes via the C-terminal domain and interferes with endosomal trafficking through blocking interaction with Rab5 and Rab22 with EEA1 (Ku et al., 2012). VipD binds to the endosomal regulator Rab5 and triggers the hydrolytic phospholipase A1 activity in VipD, causing the removal of the lipid phosphatidylinositol 3-phosphate facilitating endosomal lysosomal avoidance by L. pneumophilia (Gaspar and Machner, 2014). The SidE family of effector proteins (SidE, SdeA, SdeB, and SdeC) have been found to catalyze the non-canonical ubiquitination of Rab small GTPases, leading to a potential role in vesicular trafficking (Wang et al., 2018).
Recently, the MavE translocated effector has been shown to be indispensable for diverting the LCV from the endosomal-lysosomal pathway and is essential for intracellular replication in human macrophages and amoeba (Vaughn et al., 2021). MavE has an interaction with another effector protein, a metaeffector, YlfA/LegC7 that along with two other effectors (LegC2 & LegC3), assembles in a complex on the LCV and interacts with ER-derived vesicles to initiate membrane fusion (Jahn and Scheller, 2006; Huang et al., 2011; O'Brien et al., 2015; Shi et al., 2016; Urbanus et al., 2016). Importantly, the crystal structure of MavE shows a eukaryotic NPxY motif that interacts with phosphotyrosine-containing proteins, and this motif is essential for the function of MavE in lysosomal evasion (Vaughn et al., 2021). However, the role of the LegC7/LegC2/LegC3 complex and its potential interaction with MavE in the biological functions of MavE is still to be determined.
A key virulence determinant of L. pneumophila is the ability of the LCV to be remodeled into an ER-derived vacuole diverted from traffic through the endosomal-lysosomal pathway (Isberg et al., 2009; Richards et al., 2013; Kotewicz et al., 2017). While the mechanisms L. pneumophilia employs to evade lysosomal fusion is still unclear, the T4SS effector proteins DrrA/SidM, LidA, VipD, LepB and MavE have been shown to play roles in diversion of the LCV from the endosomal-lysosomal pathway. The long-term evolution of L. pneumophila with various amoeba as the natural hosts, and inter-kingdom as well as inter-bacterial horizontal gene transfer has most likely shaped the evolution of the pathogen to adapt to the intra-vacuolar environment of eukaryotic cells.
Intercepting the Exocytic Pathway by Chlamydia trachomatis
Chlamydia trachomatis is an obligate, Gram-negative, intracellular bacteria that is responsible for the most reported sexually transmitted bacterial infection in the United States. In developing countries, it is known to cause a blinding disease known as trachoma. The pathogen replicates in the host cell in a vacuole termed the Chlamydia inclusion (van Ooij et al., 1997; Johnson et al., 2014), and has a biphasic developmental intracellular life cycle (Figure 1) (Birkelund, 1992; Brumell and Scidmore, 2007; Kunz et al., 2019). The first phase is an infectious, extracellular form termed the elementary body (EB) that is metabolically inert but exhibiting metabolic activity under axenic conditions (Omsland et al., 2014). The second is an intracellular, non-infectious, and metabolically active reticulate body (RB) (Moulder, 1991). To induce uptake into host cells, the EB utilizes a T3SS to inject an effector designated as translocated actin-recruiting phosphoprotein (TARP) across the host cell membrane (Clifton et al., 2004; Clifton et al., 2005; Jewett et al., 2006). TARP remodels the host actin at the bacterial entry site and activates a master regulator of lamellipodium formation (Rac1) (Marei and Malliri, 2017; Tolchard et al., 2018). The EB then exploits the host cytoskeleton to facilitate entry into the host cell and form the inclusion (Hybiske and Stephens, 2007b). Within the inclusion, the EBs rapidly differentiate into RBs and the pathogen begins to replicate by binary fission within the inclusion (Figure 1) (Hybiske and Stephens, 2007b). The infection cycle lasts 48 -72 hours, when the RBs then differentiate back into infectious EBs and are released from the host cell by extrusion or lysis (Hybiske and Stephens, 2007b). While Chlamydiae and chlamydiae-related organisms share a strictly intracellular biphasic developmental cycle, depending on the species, the cycle can last up to 10 days (Derre, 2015). This review focuses on intracellular trafficking of C. trachomatis and the establishment of other Chlamydia species to manipulate the host cellular environment has been shown to have effector-dependent subversion from the endocytic pathway, interaction with the mitochondria and/or the ER (Croxatto and Greub, 2010; Collingro et al., 2011; Mehlitz et al., 2014).
Early in the infection cycle, Chlamydia trachomatis relies on the modification of the inclusion membrane to disguise the vacuole as a host organelle that is separate from the endocytic pathway. The bacteria actively modify the inclusion by inserting bacterial inclusion effector proteins (Incs) and host lipids are acquired into its membrane early in infection (Rzomp et al., 2003; Hybiske and Stephens, 2007b). Inc are commonly thought to interact with host cell components to facilitate the organisms’ growth, survival and subversion throughout the host cell (Gauliard et al., 2015). The Chlamydia inclusion recruits Rab GTPases to the inclusion (Rzomp et al., 2003), and rapidly exits the endocytic pathway and associates with Rabs 1, 4 and 11 (Rzomp et al., 2003). A few chlamydial Incs effectors have been shown to bind Rabs and specifically, Inc CT229 interacts directly with Rab4-GTP (Rzomp et al., 2006). The association with specific Rabs and the inclusion membrane helps identify the bacterial vacuole apart from the endocytic pathway.
Interestingly, Chlamydia also diverts its inclusion from the endocytic pathway by employing the host’s microtubule network for the duration of its infectious cycle (Mital et al., 2010). Chlamydia expresses two potential effectors that regulate actin depolymerization, CT166- an inhibitor of Rac1 and CT694- results in AHNAK- dependent loss of actin stress-fibers (Belland et al., 2001; Hower et al., 2009; Thalmann et al., 2010). The inclusion is trafficked to the peri-Golgi region of the host cell, maintaining close association with the microtubule organizing center (MTOC) (Brumell and Scidmore, 2007; Kumar and Valdivia, 2008; Mital et al., 2010). While the actin cytoskeleton may provide a protective framework for the inclusion, the lipid content of the inclusion is thought to help to identify it as an organelle separate from the endocytic pathway (Elwell and Engel, 2012). Chlamydia intercepts sphingomyelin (SM) trafficking from the Golgi to the plasma membrane and from basolateral vesicle traffic and incorporates it not only into the inclusion membrane but its own bacterial membrane (Moore et al., 2008). Chlamydia has also been shown to exploit the host protein CERT which regulates ceramide traffic from the ER to the Golgi (Derre et al., 2011; Elwell et al., 2011). CERT binds to the IncD effector to regulate and acquire SM incorporation into the inclusion (Derre et al., 2011; Elwell et al., 2011; Olson et al., 2019). Additional host lipids including lipids of the Golgi apparatus have been detected in the inclusion membrane including cholesterol, phosphatidic acid, and PtdIns(4)P (Carabeo et al., 2003; Beatty, 2008; Moorhead et al., 2010). Chlamydiae likely acquire cholesterol from the host cells as the pathogen lacks the enzymatic capacity for the lipid’s synthesis (Carabeo et al., 2003). Cholesterol and sphingomyelin are intercepted from the Golgi-derived vesicles and rerouted to the inclusion (Walpole et al., 2018). Cholesterol containing domains of the inclusion aid in recruiting Src-family kinases that also help maintain close association of the inclusion with the MTOC (Walpole et al., 2018). Ultimately, both cholesterol and sphingomyelin are important for the pathogen nutrition and growth but may also contribute to fusion of the cholesterol containing inclusion by “organelle mimicry” (Walpole et al., 2018).
Thus, the Chlamydia inclusion is modified through the secretion of chlamydia-specific effectors and is diverted from the endocytic pathway, and intercepts vesicles along the exocytic pathway to incorporate lipids and cholesterol into the inclusion (Brumell and Scidmore, 2007; Walpole et al., 2018). The development and dissemination of C. trachomatis is dependent upon the delivery of an arsenal of effector proteins that interfere with a wide diversity of host cell processes that influence vesicular trafficking, allowing the pathogen to establish an intracellular niche (Elwell et al., 2016; Bugalhao and Mota, 2019).
Trafficking of Brucella-Containing Vacuole Along the Endosomal Pathway
Brucella are Gram-negative zoonotic pathogens known to infect a wide range of host species, but the three most common incidentally infecting humans are Brucella melitensis (primarily associated with goats and sheep), Brucella suis (primarily infecting swine) and Brucella abortus (primarily infecting cattle), (Boschiroli et al., 2002b; Xavier et al., 2010). Brucella is highly infectious, only requiring 10-100 bacteria to establish infection via inhalation (Hoover, 1997; Bossi et al., 2004) however, it can also be transmitted by consuming undercooked meat or unpasteurized dairy products (Bossi et al., 2004). Human infection with Brucella can result in a debilitating and chronic illness manifested as undulant fever (Young, 200; Roop et al., 2004). Brucella passes through the mucus membranes to infect phagocytic cells and when left untreated can disseminate throughout the body’s tissues and organs (Fernandez-Prada et al., 2003; Paixao et al., 2009).
Within macrophages, Brucella is trafficked within a vacuole through the endocytic pathway and this Brucella containing vacuole (BCV) is remodeled by the ER. The smooth LPS of Brucella with a O-side chain is crucial for entry into host cells, for vacuole biogenesis and for adaptation to the acidic pH and tolerance of the reactive oxygen intermediates (Roop et al., 2004; Haag et al., 2010). Rough LPS mutants of Brucella that lack O-side chain enter cells independently of lipid rafts and are rapidly killed by macrophages (Porte et al., 2003; Rittig et al., 2003; Pei and Ficht, 2004). However, less than 10% of internalized Brucella survive to establish a replicative BCV (He et al., 2006; von Bargen et al., 2012).
Once Brucella is internalized, the BCV traffics along the endocytic pathway, acquiring both early and late endosomal markers (Figure 1) (Pizarro-Cerda et al., 1998; Starr et al., 2012). Endosomal trafficking of the BCV proceeds with the acquisition of EEA1 and Rab5 (Pizarro-Cerda et al., 1998; Celli et al., 2003), these early endosomal markers are rapidly exchanged for the late endosomal markers Rab7, RILP, LAMP1, and vATPase. These events begin the conversion of the BCV into a more acidic compartment, which is essential for pathogenesis of this organism (Porte et al., 1999). The pH of the BCVs decreases to 4.0 within 1hr of bacterial entry and this pH persists for at least 5 hrs following infection (Porte et al., 1999). Importantly, the acidic pH seems to be involved in bacterial adaptation to the BCV, since inhibition of the vATPase results in a decreased bacterial viability (Porte et al., 1999).
However, the limited lysosomal fusion of the BCV is well controlled by the bacteria that have developed adaptations to resist degradation within the lysosomal microenvironment (Starr et al., 2008; Starr et al., 2012). The acidic pH micro-environment in the BCV is an activation signal for virulence genes as it activates the expression of the VirB T4SS that directs diversion of the BCV from the endocytic pathway into an ER-associated BCV (Figure 1) (Comerci et al., 2001; Boschiroli et al., 2002a; Celli et al., 2003). A critical virulence factor of Brucella, Cyclic-β-1,2-glucan, has a role in decreasing lysosomal fusion by depletion of BCV membrane cholesterol (Arellano-Reynoso et al., 2005). Since the BCV lumen is acidified and contains degradative enzymes, the pathogen mitigates the risk of degradation through expression of several proteins (HdeA and CydB) to resist the drop in pH, nitrosative damage, and antimicrobial peptides (Endley et al., 2001; Valderas et al., 2005; Roop et al., 2009). The cydB mutant strain has shown a diminished capacity for survival during stationary phase reflected by an inability to offset the effects of oxidative stress (Endley et al., 2001). The HdeA protein, which is conserved in several Gram-negative bacterial species, functions as chaperone protein in the periplasmic space to resist acid stress (Wu et al., 2008; Hong et al., 2012). The Brucella homolog of HdeA is expressed during stationary phase cultures (acidic conditions), which depends on the expression of the small RNA binding protein Hfq (HF-1), since deletion of the hfq gene significantly reduced levels of HdeA protein production (Valderas et al., 2005). HF-1 is required for entrance into stationary-phase physiology (Robertson and Roop, 1999). This post-transcriptional regulator of global gene expression promotes base pairing interactions of small RNAs with their target mRNAs to regulate target gene expression (Robertson and Roop, 1999; Valentin-Hansen et al., 2004). The pathogen antioxidant SodC, a Cu/Zn superoxide dismutase equip Brucella with the proper tools to counteract reactive oxygen species (ROS) (Gee et al., 2005). Gee et al, presented evidence supporting Hfq-dependent optimal stationary-phase sodC expression in B. abortus and showed decreased SodC production in the hfq mutant Hfq3 (Gee et al., 2005). Additionally, there are two other known defense mechanisms, the production of nitric oxide reductase to detoxify NO within macrophages and overexpressed by the bacterium early during infection and AhpC, a peroxiredoxin that scavenges endogenous ROS generated by Brucella metabolism produced in defense against H2O2 -mediated toxicity (Gross et al., 1998; Haine et al., 2006; Loisel-Meyer et al., 2006; Steele et al., 2010; Poncin et al., 2019). The progressive exclusion of LAMP1 from the vacuolar membrane of BCVs (80% loss at 6 - 12 hrs post infection), allows for the capture vesicles from ER exit sites creating a subcellular compartment with neutral pH that is permissive for replication (Starr et al., 2008). Recently it has been demonstrated by electron microscopy that at any given time, some of the replicative BCVs share a continuous lumen with the ER and speculate that the snapshots most likely reflect tethering events between these compartments (Sedzicki et al., 2018).
BCV-ER vesicle fusion is carefully coordinated by translocated effectors and requires the activity of the small GTPase, Rab2a (Fugier et al., 2009). The importance of Rab2a activity on rBCV development is shown by the fact that inhibition of Rab2 expression by small interfering RNA results in LAMP1 retention on the BCV, hindering ER vesicle fusion and ultimately inhibiting replication (Fugier et al., 2009; de Barsy et al., 2011). The effector, BspB, contributes to Brucella’s replicative niche by interacting with the conserved oligomeric Golgi (COG) tethering complex, a major coordinator of Golgi vesicular trafficking, thus remodeling Golgi membrane traffic and redirecting Golgi-derived vesicles to the BCV, and if BspB is deleted, Brucella replication in macrophages is attenuated (Miller et al., 2017). Interestingly however, the intracellular growth defect of a ΔbspB mutant in macrophages is reversed when host Rab2a protein levels are depleted by siRNA (Miller et al., 2017), with the mutant bacteria now able to grow. The T4SS effector RicA directly interacts with Rab2a and promotes Rab2a recruitment to the BCV, but deletion of ricA does not impact rBCV development in macrophages (de Barsy et al., 2011). However, deletion of ricA in a bspB mutant background suppresses the growth defect of the bspB mutant in macrophages, indicating an epistatic relationship between the functional roles of BspB and RicA (Myeni et al., 2013; Smith et al., 2020). This epistatic interplay between these two effectors allows Brucella to fine tune the modulation of host cell processes to allow development of the rBCV and promote intracellular bacterial proliferation. Another effector, BspF, promotes intracellular replication within rBCVs (Borghesan et al., 2021). BspF hijacks vesicular transport between the trans-Golgi network (TGN) and the recycling endosome pathway, and this results in the accumulation of TGN-associated vesicles to the rBCV (Borghesan et al., 2021). Therefore it is clear Brucella targets the host vesicular pathways by using multiple translocated effector proteins to develop a replicative niche.
Maturation of the BCV into replicative organelles is dependent upon BCV acidification and interaction with late endosomes/lysosomes allowing for biogenesis of the ER-derived replicative BCV (Figure 1) (Starr et al., 2008). The O-side chain of smooth LPS plays an important role in initiating and maintaining the early stages of development of the BCV while the T4SS secreted effectors are responsible for maintaining the interactions of the BCV with the ER to allow remodeling of the BCV into a replicative niche (Roop et al., 2004). Following replication, a subpopulation of Brucella traffic to a new subcellular compartment resembling an autophagosome that may aid in spreading to neighboring cells for a second round of infection (Starr et al., 2012). More experiments are needed to determine the intermediate steps of BCV trafficking and maturation. For example, in addition to BCV trafficking, microtubule manipulation by either depolymerization or polymerization events interfere with BCV maturation and replication of B. abortus (Alves-Silva et al., 2017). The effector, TcpB (also known as BtpA), possesses a TIR domain and localizes to microtubules, and in turn increases nucleation and polymerization of microtubules and acts as a stabilization factor for microtubules (Radhakrishnan et al., 2011; Alves-Silva et al., 2017). It is possible other effectors contribute to this process, to generate the rBCV. Additionally, further analysis with markers of different endosomal, Golgi- and ER-derived compartments are necessary to further characterize the trafficking of the Brucella-containing vacuole along the endosomal pathway and its ER-mediated remodeling (Piersanti et al., 2015). For further reading on the survival cycle in host cells by the facultative intracellular bacteria, Brucella, please refer to the recent review by Jiao, et al. (Jiao et al., 2021).
Interaction of Anaplasma-Containing Vacuoles With Endocytic and ER-to-Golgi Vesicle Traffic
Anaplasma spp. are Gram-negative obligate, ixodid tick-transfected intracellular bacteria that infect neutrophils and endothelial cells of vertebrate hosts (Alberdi et al., 2016). Within the tick vector, Anaplasma spp. enter the tick midgut epithelium. After initial replication in tick gut cells, Anaplasma reach the tick salivary glands where a second round of replication occurs. Following second replication, Anaplasma migrate to the saliva allowing bacterial transmission to the next vertebrate host (Ueti et al., 2009). Anaplasma is also transmitted by biting flies or blood-contaminated fomites including needles, tattooing instruments, or nose tongs (Kocan et al., 2010; Scherler et al., 2018). Of all the ixodid tick-transmitted bacteria, Anaplasma marginale and Anaplasma phagocytophilum pose major veterinary and public health significance. In humans, the species A. phagocytophilum is the causative agent of human granulocytic anaplasmosis, with symptoms including high fever and leukopenia implicating both granulocytes and lymphocytes (Woldehiwet, 2006; Scherler et al., 2018). In a small number of human infections (<3%), patients can experience acute respiratory distress syndrome that can lead to death in 1% of total cases (Bakken and Dumler, 2015; Scherler et al., 2018). During infection in cattle and other ruminants, Anaplasma marginale replicates in erythrocytes leading to bovine anaplasmosis principally and this infection persists up to 7 weeks ultimately resulting in death (Kocan et al., 2010). In contrast to infection of A. marginale, A. phagocytophilum does not replicate in erythrocytes but within the vacuoles of neutrophils (Stuen et al., 2013; Scherler et al., 2018).
As an obligate intracellular pathogen, A. phagocytophilum has evolved the ability to inhibit host cell apoptosis through the extrinsic (the death receptor pathway) or the intrinsic pathway (the mitochondrial pathway) (Ge et al., 2005; Ge and Rikihisa, 2006; Elmore, 2007; Niu et al., 2010; Niu and Rikihisa, 2013; Alberdi et al., 2016; de la Fuente et al., 2016). The Anaplasma developmental cycle exhibits two distinct forms, the noninfectious replicative form, termed reticulate cell. This is followed by the infectious “dense-cored cell” form, forming microcolonies called morulae (Figure 1) (Troese et al., 2011; Alberdi et al., 2016). Anaplasma spp. have evolved diverse strategies to persistently facilitate the transmission of the pathogen between ticks and host species. Immunodominant outer membrane proteins (MSPs) present in the Anaplasmataceae family generate new antigenic variants in order to evade host immune response (Scherler et al., 2018). Additionally, members of Anaplasma genus show no evidence of peptidoglycan (PG) layer or lipopolysaccharide (LPS) biosynthesis, thus allowing Anaplasma to infect host cells without activating the innate immune response (Lin and Rikihisa, 2003a; Scherler et al., 2018).
Similar to Chlamydia, A. phagocytophilum intercepts host cholesterol for intracellular growth and survival. Anaplasma also uses cholesterol to enter host cells through caveolae or lipid rafts (Toledo and Benach, 2015). Throughout infection, the phagosome membrane maintains caveolin-1 indicating that there is a need for continuing acquisition of cholesterol (Lin and Rikihisa, 2003b). To acquire cholesterol to enhance its growth, A. phagocytophilum hijacks the host low-density lipoprotein (LDL) uptake pathway to obtain cholesterol from outside the host cell, rather than relying on de novo production of this lipid by the host cell, to bring cholesterol to its phagosome (Xiong et al., 2009). Infection by A. phagocytophilum increases transcription and stabilization of the host LDL receptor mRNA and also expression of the protein in host cells (Xiong et al., 2009). Additionally, the Niemann-Pick type C1 (NPC1) protein, which plays a key role in intracellular cholesterol transport, and the lipid raft protein, flotillin, are hijacked by A. phagocytophilum to transport cholesterol to the A. phagocytophilum vacuole (Huang et al., 2021), though the mechanism is not yet known. The acquisition of cholesterol by upregulating the expression of low-density lipoprotein receptors is a unique evolutionary adaptation of A. phagocytophilum, occupying a distinctive niche.
Alternatively, the A. marginale-containing vacuoles have shown to accumulate and retain the early endosomal compartment marker, Rab5, the recycling endosomal compartment markers Rab4 and Rab11, and the late endosomal compartment marker Rab7 (Figure 1) (Magunda et al., 2016). The association of the A. marginale-containing vacuoles with the ER and Golgi apparatus during early in infection and the maintained association throughout the course of infection, allows for nutrient acquisition required to establish this vacuolar replicative niche (Magunda et al., 2016). A. marginale’s ability to inhibit apoptosis, maintain association with the ER and Golgi apparatus during infection, and acquire cholesterol by upregulating low-density receptors allows the bacteria to continue to occupy this distinctive niche. The mechanisms utilized by Anaplasma for modulation of host phagosome biogenesis and to adapt to the maintain intra-vacuolar microenvironment are still to be deciphered. However, an Anaplasma-derived protein, APH_0032, was found to localize to the A. phagocytophilum-occupied vacuolar membrane (AVM) during infection of human myeloid cells, human microvascular endothelial cells, and murine neutrophils and might play a role in its phagosome biogenesis (Huang et al., 2010). Additionally, a known T4SS effector of A. phagocytophilum, Ats-1, manipulates the host autophagy degradation pathway by binding directly to Beclin 1, a central regulator of autophagy (Niu et al., 2012). The progress in our understanding of Anaplasma-host interactions is hampered by the obligate nature of the pathogen, the lack of an efficient genetic system, and the difficultly in bridging the similarities and/or differences between two tick-transmitted pathogens of Anaplasmataceae family.
The Lysosomal-Like Coxiella-Containing Vacuole
Coxiella burnetii is an intracellular pathogen that initially targets alveolar macrophages, systematically manifesting as a severe flu-like illness known as Q fever (Miller et al., 2019). Coxiella is the only documented bacterial pathogen known to survive and proliferate within a phagolysosome. However, in specific pathological conditions, such as Crohn’s disease, adherent-invasive Escherichia coli replicates in phagolysosomes within macrophages (Bringer et al., 2006). The Coxiella-containing vacuole (CCV) undergoes endocytic trafficking within the endosomal-lysosomal pathway to mature into what is considered a bactericidal acidic phagolysosome, which contains lysosomal hydrolases that degrade most macromolecules and most pathogens (Baxt et al., 2013; Miller et al., 2019; Newton et al., 2020). Because C. burnetii utilizes host machinery for uptake and travels along the default endosomal-lysosomal pathway, the bacteria do not require a functional Dot/Icm T4SS system until the bacteria have established residence in an acidified lysosome-derived vacuole (Newton and Roy, 2011). The Dot/Icm system with its > 60 translocated effector proteins identified to date is crucial for replication and virulence of C. burnetii (Newton and Roy, 2011; Pechstein et al., 2020). This is supported by the observations that C. burnetii mutants defective in the Dot/Icm translocation apparatus travel to a lysosome-like vacuole, similar to the wild type strain, but yet are incapable of intracellular replication (Newton et al., 2013).
This acidic environment of the CCV triggers the delivery of the translocated T4SS system effectors into the host cytosol that likely modulate the intracellular environment to provide specific nutritional requirements conducive to its replicative niche (Newton and Roy, 2011). Studies have shown that siRNA silencing of the host Rab5 or Rab7 GTPases, or blocking acidification of the CCV, results in a significant reduction in effector translocation by C. burnetii (Newton et al., 2013; Newton et al., 2016; Newton et al., 2020). For example, the translocated effector AnkF, interacts with the host cytoskeletal component, vimentin, but this interaction appears to be important for establishing the CCV to become a replicative compartment, rather than directing the intracellular trafficking of the Coxiella vacuole (Pechstein et al., 2020). The injected serine/threonine protein kinase, CstK, influences the development of the acidified CCV through interacting with the host protein TBC1D5, a Rab7 GTPase-activating protein (Martinez et al., 2020). Hijacking TBC1D5 activity appears to be important for intracellular replication of Coxiella within the acidified CCV (Martinez et al., 2020). Additionally, at least two effectors, CvpF and CvpB manipulate autophagy to modify the acidified CCV into a more replicative compartment. CvpF interacts with the host Rab26, resulting in recruitment of MAP1LC3B/LC3B to the CCV (Siadous et al., 2021), a marker of autophagosomes. CvpB binds to phosphatidylinositol 3-phosphate to enhance association of the autophagosomal components to the CCV (Martinez et al., 2016). Another effector, AnkG plays a key role in anti-apoptosis, which is distinct from maturation of the CCV, though it is important in progression of disease in a Galleria mellonella infection model (Schafer et al., 2020). NopA is injected into host cells and impacts the innate immune response to Coxiella infection, but does not impact trafficking of this pathogen to a replicative CCV (Burette et al., 2020). Thus, the translocated effector proteins enable proliferation of C. burnetii in a spacious CCV but may not be required for progression of the CCV through the endocytic pathway (Newton et al., 2013).
Although all other intra-vacuolar pathogens have evolved and adapted to evade or subvert the endosomal/lysosomal pathway, the CCV becomes acidified, and bacteria replicate within this acidified phagolysosome (Miller et al., 2019). It is likely that there is an evolutionary advantage for delaying effector translocation until the CCV has matured into a lysosome-like vacuole. Coxiella remains unique as the main intra-vacuolar bacterial pathogen that proliferates within a phagolysosome. Recent technologies of in vivo genetic studies on obligate intracellular bacteria should move the field forward (Bekebrede et al., 2020).
Conclusions
The main goal of default phagosome formation and maturation through a series of fission and fusion events with early endosomes, late endosomes and lysosomes is to eliminate foreign pathogens and apoptotic cells. However, despite the common objective of avoiding lysosomal fusion, intracellular bacterial pathogens including Salmonella, Mycobacteria, Legionella, Chlamydia, Brucella, Coxiella, and Anaplasma have evolved a diverse range of different strategies to evade or subvert the default endosomal-lysosomal pathway, generating a favorable intracellular environment for survival and replication. Coxiella is unique as its vacuole matures through the default endosomal-lysosomal pathway and promotes fusogenic events with lysosomes to create a lysosome-like vacuole that enables proliferation. Similar to Coxiella, the Salmonella containing vacuole has evolved to accumulate some late endosome-lysosomes markers and multiple mechanisms to allow for its adaptation and survival within an idiosyncratic acidified late endosome-like vacuole. Mycobacteria and Anaplasma retain early endosomal compartment markers halting phagosomal maturation by secreting various effector proteins that alters host signaling pathways, generating an early endosome-like replicative niche. Legionella also modulates host signaling pathways by way of remodeling the LCV into an ER-derived vacuole which diverts traffic away from the endosomal-lysosomal pathway. Chlamydia and Anaplasma can divert their bacteria containing vacuoles from the endosomal-lysosomal pathways and intercept vesicles to incorporate lipids and cholesterol into their membranes. While Legionella, Chlamydia, Brucella and Anaplasma all exhibit ER vesicle fusion, this is carefully coordinated by specific secreted effectors that function with different mechanisms. The Brucella containing vacuole also initially fuses lysosomal compartments but then is remodeled by ER-derived vesicles to enable proliferation. Therefore, this clearly demonstrates the unique strategies evolved by diverse intracellular pathogens to overcome the host defensive strategy of phagosome/lysosomal fusion.
Understanding these idiosyncratic mechanisms has provided novel insights into the mechanisms of host-pathogen interactions and will continue to unravel new strategies for the control and prevention of infectious diseases. Furthermore, the ability of intracellular bacterial pathogens to modulate their phagosome has provided knowledge on how the default endosomal-lysosomal pathway functions in eukaryotes, which has a broad importance in the understanding of eukaryotic cell biology. The bacterial effectors involved in host cell modulation through interaction with specific host targets in these pathways are potential biotechnology tools that can be utilized to decipher cell biology. Future studies will reveal how intra-vacuolar pathogens have evolved and adapted with such idiosyncratic mechanisms employing a unique set of injected effectors to modulate the default endosomal-lysosomal and as well as the exocytic pathways and will further increase the understanding of these essential pathways in eukaryotes. While there has been a quantum leap in our knowledge of modulation of phagosome biogenesis by intra-vacuolar pathogens, the detailed biochemical and cellular processes affected remain to be deciphered for most intra-vacuolar pathogens. Therefore, future studies should be aimed at expanding the mechanisms of how bacterial effectors used by intra-vacuolar pathogens biochemically and/or physiologically modulate phagosome biogenesis. Furthermore, research has yet to elucidate the temporal and hierarchical delivery of effectors involved in phagosome biogenesis. The new era of single-cell biology of deciphering host-microbe interaction should facilitate future studies (Hayward et al., 2019; Sharma and Thaiss, 2020).
Author Contributions
BV collected and assembled the information and drafted the article. BV and YK critically revised the article. All authors contributed to the article and approved the submitted version.
Funding
The YK lab is supported by Public Health Service Awards R01AI120244, R01AI140195 and R21AI142727 from the NIAID and by the Commonwealth of Kentucky Research Challenge Trust Fund.
Conflict of Interest
The authors declare that the research was conducted in the absence of any commercial or financial relationships that could be construed as a potential conflict of interest.
Publisher’s Note
All claims expressed in this article are solely those of the authors and do not necessarily represent those of their affiliated organizations, or those of the publisher, the editors and the reviewers. Any product that may be evaluated in this article, or claim that may be made by its manufacturer, is not guaranteed or endorsed by the publisher.
Acknowledgments
We thank Dr. Christopher T. Price for help in revising the manuscript.
References
Alberdi, P., Espinosa, P. J., Cabezas-Cruz, A., de la Fuente, J. (2016). Anaplasma Phagocytophilum Manipulates Host Cell Apoptosis by Different Mechanisms to Establish Infection. Vet. Sci. 3 (3), 1–11. doi: 10.3390/vetsci3030015
Alberdi, L., Vergnes, A., Manneville, J. B., Tembo, D. L., Fang, Z., Zhao, Y., et al. (2020). Regulation of Kinesin-1 Activity by the Salmonella Enterica Effectors PipB2 and SifA. J. Cell Sci. 133 (9), 1–12. doi: 10.1242/jcs.239863
Albert-Weissenberger, C., Cazalet, C., Buchrieser, C. (2007). Legionella Pneumophila - a Human Pathogen That Co-Evolved With Fresh Water Protozoa. Cell Mol. Life Sci. 64 (4), 432–448. doi: 10.1007/s00018-006-6391-1
Alix, E., Chesnel, L., Bowzard, B. J., Tucker, A. M., Delprato, A., Cherfils, J., et al. (2012). The Capping Domain in RalF Regulates Effector Functions. PloS Pathog. 8 (11), e1003012. doi: 10.1371/journal.ppat.1003012
Allan, V. J., Thompson, H. M., McNiven, M. A. (2002). Motoring Around the Golgi. Nat. Cell Biol. 4 (10), E236–E242. doi: 10.1038/ncb1002-e236
Almeida, F. M., Ventura, T. L., Amaral, E. P., Ribeiro, S. C., Calixto, S. D., Manhaes, M. R., et al. (2017). Hypervirulent Mycobacterium Tuberculosis Strain Triggers Necrotic Lung Pathology Associated With Enhanced Recruitment of Neutrophils in Resistant C57BL/6 Mice. PloS One 12 (3), e0173715. doi: 10.1371/journal.pone.0173715
Alves-Silva, J., Tavares, I. P., Guimaraes, E. S., Costa Franco, M. M., Figueiredo, B. C., Marques, J. T., et al. (2017). Modulation of Microtubule Dynamics Affects Brucella Abortus Intracellular Survival, Pathogen-Containing Vacuole Maturation, and Pro-Inflammatory Cytokine Production in Infected Macrophages. Front. Microbiol. 8, 2217. doi: 10.3389/fmicb.2017.02217
Arellano-Reynoso, B., Lapaque, N., Salcedo, S., Briones, G., Ciocchini, A. E., Ugalde, R., et al. (2005). Cyclic Beta-1,2-Glucan Is a Brucella Virulence Factor Required for Intracellular Survival. Nat. Immunol. 6 (6), 618–625. doi: 10.1038/ni1202
Aridor, M., Bannykh, S. I., Rowe, T., Balch, W. E. (1995). Sequential Coupling Between COPII and COPI Vesicle Coats in Endoplasmic Reticulum to Golgi Transport. J. Cell Biol. 131 (4), 875–893. doi: 10.1083/jcb.131.4.875
Arora, S. K., Naqvi, N., Alam, A., Ahmad, J., Alsati, B. S., Sheikh, J. A., et al. (2020). Mycobacterium Smegmatis Bacteria Expressing Mycobacterium Tuberculosis-Specific Rv1954A Induce Macrophage Activation and Modulate the Immune Response. Front. Cell. Infect. Microbiol. 10:564565 (543). doi: 10.3389/fcimb.2020.564565
Augenstreich, J., Briken, V. (2020). Host Cell Targets of Released Lipid and Secreted Protein Effectors of Mycobacterium Tuberculosis. Front. Cell. Infect. Microbiol. 10:595029 (618). doi: 10.3389/fcimb.2020.595029
Av-Gay, Y., Everett, M. (2000). The Eukaryotic-Like Ser/Thr Protein Kinases of Mycobacterium Tuberculosis. Trends Microbiol. 8 (5), 238–244. doi: 10.1016/s0966-842x(00)01734-0
Bach, H., Papavinasasundaram, K. G., Wong, D., Hmama, Z., Av-Gay, Y. (2008). Mycobacterium Tuberculosis Virulence Is Mediated by PtpA Dephosphorylation of Human Vacuolar Protein Sorting 33B. Cell Host Microbe 3 (5), 316–322. doi: 10.1016/j.chom.2008.03.008
Bakken, J. S., Dumler, J. S. (2015). Human Granulocytic Anaplasmosis. Infect. Dis. Clin. North Am. 29 (2), 341–355. doi: 10.1016/j.idc.2015.02.007
Bakowski, M. A., Braun, V., Brumell, J. H. (2008). Salmonella-Containing Vacuoles: Directing Traffic and Nesting to Grow. Traffic 9 (12), 2022–2031. doi: 10.1111/j.1600-0854.2008.00827.x
Bakowski, M. A., Braun, V., Lam, G. Y., Yeung, T., Heo, W. D., Meyer, T., et al. (2010). The Phosphoinositide Phosphatase SopB Manipulates Membrane Surface Charge and Trafficking of the Salmonella-Containing Vacuole. Cell Host Microbe 7 (6), 453–462. doi: 10.1016/j.chom.2010.05.011
Baldeon, M. E., Ceresa, B. P., Casanova, J. E. (2001). Expression of Constitutively Active Rab5 Uncouples Maturation of the Salmonella-Containing Vacuole From Intracellular Replication. Cell Microbiol. 3 (7), 473–486. doi: 10.1046/j.1462-5822.2001.00130.x
Baxt, L. A., Garza-Mayers, A. C., Goldberg, M. B. (2013). Bacterial Subversion of Host Innate Immune Pathways. Science 340 (6133), 697–701. doi: 10.1126/science.1235771
Beatty, W. L. (2008). Late Endocytic Multivesicular Bodies Intersect the Chlamydial Inclusion in the Absence of CD63. Infect. Immun. 76 (7), 2872–2881. doi: 10.1128/IAI.00129-08
Bekebrede, H., Lin, M., Teymournejad, O., Rikihisa, Y. (2020). Discovery of In Vivo Virulence Genes of Obligatory Intracellular Bacteria by Random Mutagenesis. Front. Cell Infect. Microbiol. 10:2. doi: 10.3389/fcimb.2020.00002
Belland, R. J., Scidmore, M. A., Crane, D. D., Hogan, D. M., Whitmire, W., McClarty, G., et al. (2001). Chlamydia Trachomatis Cytotoxicity Associated With Complete and Partial Cytotoxin Genes. Proc. Natl. Acad. Sci. U.S.A. 98 (24), 13984–13989. doi: 10.1073/pnas.241377698
Best, A., Abu Kwaik, Y. (2018). Evolution of the Arsenal of Legionella Pneumophila Effectors To Modulate Protist Hosts. mBio 9 (5), 1–16. doi: 10.1128/mBio.01313-18
Beuzon, C. R., Meresse, S., Unsworth, K. E., Ruiz-Albert, J., Garvis, S., Waterman, S. R., et al. (2000). Salmonella Maintains the Integrity of its Intracellular Vacuole Through the Action of SifA. EMBO J. 19 (13), 3235–3249. doi: 10.1093/emboj/19.13.3235
Birkelund, S. (1992). The Molecular Biology and Diagnostics of Chlamydia Trachomatis. Dan Med. Bull. 39 (4), 304–320.
Borghesan, E., Smith, E. P., Myeni, S., Binder, K., Knodler, L. A., Celli, J. (2021). A Brucella Effector Modulates the Arf6-Rab8a GTPase Cascade to Promote Intravacuolar Replication. EMBO J. 40 (19), e107664. doi: 10.15252/embj.2021107664
Boschiroli, M. L., Ouahrani-Bettache, S., Foulongne, V., Michaux-Charachon, S., Bourg, G., Allardet-Servent, A., et al. (2002a). Type IV Secretion and Brucella Virulence. Vet. Microbiol. 90 (1-4), 341–348. doi: 10.1016/s0378-1135(02)00219-5
Boschiroli, M. L., Ouahrani-Bettache, S., Foulongne, V., Michaux-Charachon, S., Bourg, G., Allardet-Servent, A., et al. (2002b). The Brucella Suis virB Operon is Induced Intracellularly in Macrophages. Proc. Natl. Acad. Sci. U.S.A. 99 (3), 1544–1549. doi: 10.1073/pnas.032514299
Bossi, P., Tegnell, A., Baka, A., van Loock, F., Hendriks, J., Werner, A., et al. (2004). Bichat Guidelines for the Clinical Management of Brucellosis and Bioterrorism-Related Brucellosis. Euro. Surveill. 9 (12), 33–34. doi: 10.2807/esm.09.12.00506-en
Boucrot, E., Henry, T., Borg, J. P., Gorvel, J. P., Meresse, S. (2005). The Intracellular Fate of Salmonella Depends on the Recruitment of Kinesin. Science 308 (5725), 1174–1178. doi: 10.1126/science.1110225
Brennan, M. A., Cookson, B. T. (2000). Salmonella Induces Macrophage Death by Caspase-1-Dependent Necrosis. Mol. Microbiol. 38 (1), 31–40. doi: 10.1046/j.1365-2958.2000.02103.x
Bringer, M. A., Glasser, A. L., Tung, C. H., Meresse, S., Darfeuille-Michaud, A. (2006). The Crohn's Disease-Associated Adherent-Invasive Escherichia Coli Strain LF82 Replicates in Mature Phagolysosomes Within J774 Macrophages. Cell Microbiol. 8 (3), 471–484. doi: 10.1111/j.1462-5822.2005.00639.x
Brumell, J. H., Scidmore, M. A. (2007). Manipulation of Rab GTPase Function by Intracellular Bacterial Pathogens. Microbiol. Mol. Biol. Rev. 71 (4), 636–652. doi: 10.1128/mmbr.00023-07
Buchmeier, N. A., Heffron, F. (1991). Inhibition of Macrophage Phagosome-Lysosome Fusion by Salmonella Typhimurium. Infect. Immun. 59 (7), 2232–2238. doi: 10.1128/iai.59.7.2232-2238.1991
Bugalhao, J. N., Mota, L. J. (2019). The Multiple Functions of the Numerous Chlamydia Trachomatis Secreted Proteins: The Tip of the Iceberg. Microb. Cell 6 (9), 414–449. doi: 10.15698/mic2019.09.691
Burette, M., Allombert, J., Lambou, K., Maarifi, G., Nisole, S., Di Russo Case, E., et al. (2020). Modulation of Innate Immune Signaling by a Coxiella Burnetii Eukaryotic-Like Effector Protein. Proc. Natl. Acad. Sci. U.S.A. 117 (24), 13708–13718. doi: 10.1073/pnas.1914892117
Bussi, C., Gutierrez, M. G. (2019). Mycobacterium Tuberculosis Infection of Host Cells in Space and Time. FEMS Microbiol. Rev. 43 (4), 341–361. doi: 10.1093/femsre/fuz006
Çakır, T., Panagiotou, G., Uddin, R., Durmuş, S. (2020). Novel Approaches for Systems Biology of Metabolism-Oriented Pathogen-Human Interactions: A Mini-Review. Front. Cell. Infect. Microbiol. 10, 52 (52). doi: 10.3389/fcimb.2020.00052
Cambier, C. J., Falkow, S., Ramakrishnan, L. (2014). Host Evasion and Exploitation Schemes of Mycobacterium Tuberculosis. Cell 159 (7), 1497–1509. doi: 10.1016/j.cell.2014.11.024
Carabeo, R. A., Mead, D. J., Hackstadt, T. (2003). Golgi-Dependent Transport of Cholesterol to the Chlamydia Trachomatis Inclusion. Proc. Natl. Acad. Sci. U.S.A. 100 (11), 6771–6776. doi: 10.1073/pnas.1131289100
Casadevall, A. (2008). Evolution of Intracellular Pathogens. Annu. Rev. Microbiol. 62, 19–33. doi: 10.1146/annurev.micro.61.080706.093305
Casadevall, A., Pirofski, L. A. (2011). A New Synthesis for Antibody-Mediated Immunity. Nat. Immunol. 13 (1), 21–28. doi: 10.1038/ni.2184
Castanheira, S., Garcia-Del Portillo, F. (2017). Salmonella Populations Inside Host Cells. Front. Cell Infect. Microbiol. 7:432. doi: 10.3389/fcimb.2017.00432
Celli, J., de Chastellier, C., Franchini, D. M., Pizarro-Cerda, J., Moreno, E., Gorvel, J. P. (2003). Brucella Evades Macrophage Killing via VirB-Dependent Sustained Interactions With the Endoplasmic Reticulum. J. Exp. Med. 198 (4), 545–556. doi: 10.1084/jem.20030088
Chakravarty, S., Massé, E. (2019). RNA-Dependent Regulation of Virulence in Pathogenic Bacteria. Front. Cell. Infect. Microbiol. 9, 337 (337). doi: 10.3389/fcimb.2019.00337
Clemens, D. L., Horwitz, M. A. (1995). Characterization of the Mycobacterium Tuberculosis Phagosome and Evidence That Phagosomal Maturation is Inhibited. J. Exp. Med. 181 (1), 257–270. doi: 10.1084/jem.181.1.257
Clifton, D. R., Dooley, C. A., Grieshaber, S. S., Carabeo, R. A., Fields, K. A., Hackstadt, T. (2005). Tyrosine Phosphorylation of the Chlamydial Effector Protein Tarp is Species Specific and Not Required for Recruitment of Actin. Infect. Immun. 73 (7), 3860–3868. doi: 10.1128/iai.73.7.3860-3868.2005
Clifton, D. R., Fields, K. A., Grieshaber, S. S., Dooley, C. A., Fischer, E. R., Mead, D. J., et al. (2004). A Chlamydial Type III Translocated Protein is Tyrosine-Phosphorylated at the Site of Entry and Associated With Recruitment of Actin. Proc. Natl. Acad. Sci. U.S.A. 101 (27), 10166–10171. doi: 10.1073/pnas.0402829101
Coers, J., Monahan, C., Roy, C. R. (1999). Modulation of Phagosome Biogenesis by Legionella Pneumophila Creates an Organelle Permissive for Intracellular Growth. Nat. Cell Biol. 1 (7), 451–453. doi: 10.1038/15687
Collingro, A., Tischler, P., Weinmaier, T., Penz, T., Heinz, E., Brunham, R. C., et al. (2011). Unity in Variety–the Pan-Genome of the Chlamydiae. Mol. Biol. Evol. 28 (12), 3253–3270. doi: 10.1093/molbev/msr161
Comerci, D. J., Martinez-Lorenzo, M. J., Sieira, R., Gorvel, J. P., Ugalde, R. A. (2001). Essential Role of the VirB Machinery in the Maturation of the Brucella Abortus-Containing Vacuole. Cell Microbiol. 3 (3), 159–168. doi: 10.1046/j.1462-5822.2001.00102.x
Cowley, S. C., Babakaiff, R., Av-Gay, Y. (2002). Expression and Localization of the Mycobacterium Tuberculosis Protein Tyrosine Phosphatase PtpA. Res. Microbiol. 153 (4), 233–241. doi: 10.1016/s0923-2508(02)01309-8
Cowley, S., Ko, M., Pick, N., Chow, R., Downing, K. J., Gordhan, B. G., et al. (2004). The Mycobacterium Tuberculosis Protein Serine/Threonine Kinase PknG is Linked to Cellular Glutamate/Glutamine Levels and is Important for Growth In Vivo. Mol. Microbiol. 52 (6), 1691–1702. doi: 10.1111/j.1365-2958.2004.04085.x
Crosskey, T. D., Beckham, K. S. H., Wilmanns, M. (2020). The ATPases of the Mycobacterial Type VII Secretion System: Structural and Mechanistic Insights Into Secretion. Prog. Biophys. Mol. Biol. 152, 25–34. doi: 10.1016/j.pbiomolbio.2019.11.008
Croxatto, A., Greub, G. (2010). Early Intracellular Trafficking of Waddlia Chondrophila in Human Macrophages. Microbiol. (Reading). 156 (Pt 2), 340–355. doi: 10.1099/mic.0.034546-0
Cuellar-Mata, P., Jabado, N., Liu, J., Furuya, W., Finlay, B. B., Gros, P., et al. (2002). Nramp1 Modifies the Fusion of Salmonella Typhimurium-Containing Vacuoles With Cellular Endomembranes in Macrophages. J. Biol. Chem. 277 (3), 2258–2265. doi: 10.1074/jbc.M105508200
Curto, P., Riley, S. P., Simões, I., Martinez, J. J. (2019a). Macrophages Infected by a Pathogen and a Non-Pathogen Spotted Fever Group Rickettsia Reveal Differential Reprogramming Signatures Early in Infection. Front. Cell. Infect. Microbiol. 9:97 (97). doi: 10.3389/fcimb.2019.00097
Curto, P., Santa, C., Allen, P., Manadas, B., Simões, I., Martinez, J. J. (2019b). A Pathogen and a Non-Pathogen Spotted Fever Group Rickettsia Trigger Differential Proteome Signatures in Macrophages. Front. Cell. Infect. Microbiol. 9, 43 (43). doi: 10.3389/fcimb.2019.00043
D'Costa, V. M., Braun, V., Landekic, M., Shi, R., Proteau, A., McDonald, L., et al. (2015). Salmonella Disrupts Host Endocytic Trafficking by SopD2-Mediated Inhibition of Rab7. Cell Rep. 12 (9), 1508–1518. doi: 10.1016/j.celrep.2015.07.063
de Barsy, M., Jamet, A., Filopon, D., Nicolas, C., Laloux, G., Rual, J. F., et al. (2011). Identification of a Brucella Spp. Secreted Effector Specifically Interacting With Human Small GTPase Rab2. Cell Microbiol. 13 (7), 1044–1058. doi: 10.1111/j.1462-5822.2011.01601.x
de la Fuente, J., Estrada-Pena, A., Cabezas-Cruz, A., Kocan, K. M. (2016). Anaplasma Phagocytophilum Uses Common Strategies for Infection of Ticks and Vertebrate Hosts. Trends Microbiol. 24 (3), 173–180. doi: 10.1016/j.tim.2015.12.001
Del Portillo, P., García-Morales, L., Menéndez, M. C., Anzola, J. M., Rodríguez, J. G., Helguera-Repetto, A. C., et al. (2019). Hypoxia Is Not a Main Stress When Mycobacterium Tuberculosis Is in a Dormancy-Like Long-Chain Fatty Acid Environment. Front. Cell. Infect. Microbiol. 8, 449 (449). doi: 10.3389/fcimb.2018.00449
Deretic, V., Singh, S., Master, S., Harris, J., Roberts, E., Kyei, G., et al. (2006). Mycobacterium Tuberculosis Inhibition of Phagolysosome Biogenesis and Autophagy as a Host Defence Mechanism. Cell Microbiol. 8 (5), 719–727. doi: 10.1111/j.1462-5822.2006.00705.x
Derre, I. (2015). Chlamydiae Interaction With the Endoplasmic Reticulum: Contact, Function and Consequences. Cell Microbiol. 17 (7), 959–966. doi: 10.1111/cmi.12455
Derre, I., Isberg, R. R. (2004). Legionella Pneumophila Replication Vacuole Formation Involves Rapid Recruitment of Proteins of the Early Secretory System. Infect. Immun. 72 (5), 3048–3053. doi: 10.1128/iai.72.5.3048-3053.2004
Derre, I., Swiss, R., Agaisse, H. (2011). The Lipid Transfer Protein CERT Interacts With the Chlamydia Inclusion Protein IncD and Participates to ER-Chlamydia Inclusion Membrane Contact Sites. PloS Pathog. 7 (6), e1002092. doi: 10.1371/journal.ppat.1002092
Di Paolo, G., De Camilli, P. (2006). Phosphoinositides in cell regulation and membrane dynamics. Nature 443(7112), 651–657. doi: 10.1038/nature05185
Do, H. T., Santos, J. A., Trieu, K., Petersen, K., Le, M. B., Lai, D. T., et al. (2016). Effectiveness of a Communication for Behavioral Impact (COMBI) Intervention to Reduce Salt Intake in a Vietnamese Province Based on Estimations From Spot Urine Samples. J. Clin. Hypertens. (Greenwich). 18 (11), 1135–1142. doi: 10.1111/jch.12884
Duclos, S., Diez, R., Garin, J., Papadopoulou, B., Descoteaux, A., Stenmark, H., et al. (2000). Rab5 Regulates the Kiss and Run Fusion Between Phagosomes and Endosomes and the Acquisition of Phagosome Leishmanicidal Properties in RAW 264.7 Macrophages. J. Cell Sci. 113 Pt 19, 3531–3541. doi: 10.1242/jcs.113.19.3531
Duden, R. (2003). ER-To-Golgi Transport: COP I and COP II Function (Review). Mol. Membr. Biol. 20 (3), 197–207. doi: 10.1080/0968768031000122548
Elmore, S. (2007). Apoptosis: A Review of Programmed Cell Death. Toxicol. Pathol. 35 (4), 495–516. doi: 10.1080/01926230701320337
Elwell, C. A., Engel, J. N. (2012). Lipid Acquisition by Intracellular Chlamydiae. Cell Microbiol. 14 (7), 1010–1018. doi: 10.1111/j.1462-5822.2012.01794.x
Elwell, C. A., Jiang, S., Kim, J. H., Lee, A., Wittmann, T., Hanada, K., et al. (2011). Chlamydia Trachomatis Co-Opts GBF1 and CERT to Acquire Host Sphingomyelin for Distinct Roles During Intracellular Development. PloS Pathog. 7 (9), e1002198. doi: 10.1371/journal.ppat.1002198
Elwell, C., Mirrashidi, K., Engel, J. (2016). Chlamydia Cell Biology and Pathogenesis. Nat. Rev. Microbiol. 14 (6), 385–400. doi: 10.1038/nrmicro.2016.30
Endley, S., McMurray, D., Ficht, T. A. (2001). Interruption of the cydB Locus in Brucella Abortus Attenuates Intracellular Survival and Virulence in the Mouse Model of Infection. J. Bacteriol. 183 (8), 2454–2462. doi: 10.1128/jb.183.8.2454-2462.2001
Eswarappa, S. M., Negi, V. D., Chakraborty, S., Chandrasekhar Sagar, B. K., Chakravortty, D. (2010). Division of the Salmonella-Containing Vacuole and Depletion of Acidic Lysosomes in Salmonella-Infected Host Cells Are Novel Strategies of Salmonella Enterica to Avoid Lysosomes. Infect. Immun. 78 (1), 68–79. doi: 10.1128/IAI.00668-09
Fernandez-Prada, C. M., Zelazowska, E. B., Nikolich, M., Hadfield, T. L., Roop, R. M., Robertson, G. L., et al. (2003). Interactions Between Brucella Melitensis and Human Phagocytes: Bacterial Surface O-Polysaccharide Inhibits Phagocytosis, Bacterial Killing, and Subsequent Host Cell Apoptosis. Infect. Immun. 71 (4), 2110–2119. doi: 10.1128/iai.71.4.2110-2119.2003
Ferrari, G., Langen, H., Naito, M., Pieters, J. (1999). A Coat Protein on Phagosomes Involved in the Intracellular Survival of Mycobacteria. Cell 97 (4), 435–447. doi: 10.1016/s0092-8674(00)80754-0
Fraser, D. W., Tsai, T. R., Orenstein, W., Parkin, W. E., Beecham, H. J., Sharrar, R. G., et al. (1977). Legionnaires' Disease: Description of an Epidemic of Pneumonia. N. Engl. J. Med. 297 (22), 1189–1197. doi: 10.1056/nejm197712012972201
Fugier, E., Salcedo, S. P., de Chastellier, C., Pophillat, M., Muller, A., Arce-Gorvel, V., et al. (2009). The Glyceraldehyde-3-Phosphate Dehydrogenase and the Small GTPase Rab 2 Are Crucial for Brucella Replication. PloS Pathog. 5 (6), e1000487. doi: 10.1371/journal.ppat.1000487
Galan, J. E., Wolf-Watz, H. (2006). Protein Delivery Into Eukaryotic Cells by Type III Secretion Machines. Nature 444 (7119), 567–573. doi: 10.1038/nature05272
Gan, J., Scott, N. E., Newson, J. P. M., Wibawa, R. R., Wong Fok Lung, T., Pollock, G. L., et al. (2020). The Salmonella Effector SseK3 Targets Small Rab GTPases. Front. Cell. Infect. Microbiol. 10:419 (419). doi: 10.3389/fcimb.2020.00419
Gao, Y., Spahn, C., Heilemann, M., Kenney, L. J. (2018). The Pearling Transition Provides Evidence of Force-Driven Endosomal Tubulation During Salmonella Infection. mBio 9 (3), 1–10. doi: 10.1128/mBio.01083-18
Garcia-del Portillo, F., Zwick, M. B., Leung, K. Y., Finlay, B. B. (1993). Salmonella Induces the Formation of Filamentous Structures Containing Lysosomal Membrane Glycoproteins in Epithelial Cells. Proc. Natl. Acad. Sci. U.S.A. 90 (22), 10544–10548. doi: 10.1073/pnas.90.22.10544
Garg, R., Borbora, S. M., Bansia, H., Rao, S., Singh, P., Verma, R., et al. (2020). Mycobacterium Tuberculosis Calcium Pump CtpF Modulates the Autophagosome in an mTOR-Dependent Manner. Front. Cell. Infect. Microbiol. 10, 461 (461). doi: 10.3389/fcimb.2020.00461
Garin, J., Diez, R., Kieffer, S., Dermine, J. F., Duclos, S., Gagnon, E., et al. (2001). The Phagosome Proteome: Insight Into Phagosome Functions. J. Cell Biol. 152 (1), 165–180. doi: 10.1083/jcb.152.1.165
Garvis, S. G., Beuzon, C. R., Holden, D. W. (2001). A Role for the PhoP/Q Regulon in Inhibition of Fusion Between Lysosomes and Salmonella-Containing Vacuoles in Macrophages. Cell Microbiol. 3 (11), 731–744. doi: 10.1046/j.1462-5822.2001.00153.x
Gaspar, A. H., Machner, M. P. (2014). VipD is a Rab5-Activated Phospholipase A1 That Protects Legionella Pneumophila From Endosomal Fusion. Proc. Natl. Acad. Sci. U.S.A. 111 (12), 4560–4565. doi: 10.1073/pnas.1316376111
Gauliard, E., Ouellette, S. P., Rueden, K. J., Ladant, D. (2015). Characterization of Interactions Between Inclusion Membrane Proteins From Chlamydia Trachomatis. Front. Cell Infect. Microbiol. 5:13. doi: 10.3389/fcimb.2015.00013
Gee, J. M., Valderas, M. W., Kovach, M. E., Grippe, V. K., Robertson, G. T., Ng, W. L., et al. (2005). The Brucella Abortus Cu,Zn Superoxide Dismutase is Required for Optimal Resistance to Oxidative Killing by Murine Macrophages and Wild-Type Virulence in Experimentally Infected Mice. Infect. Immun. 73 (5), 2873–2880. doi: 10.1128/iai.73.5.2873-2880.2005
Ge, P., Lei, Z., Yu, Y., Lu, Z., Qiang, L., Chai, Q., et al. (2021). M. Tuberculosis PknG Manipulates Host Autophagy Flux to Promote Pathogen Intracellular Survival. Autophagy 17, 1–19. doi: 10.1080/15548627.2021.1938912
Ge, Y., Rikihisa, Y. (2006). Anaplasma Phagocytophilum Delays Spontaneous Human Neutrophil Apoptosis by Modulation of Multiple Apoptotic Pathways. Cell Microbiol. 8 (9), 1406–1416. doi: 10.1111/j.1462-5822.2006.00720.x
Ge, Y., Yoshiie, K., Kuribayashi, F., Lin, M., Rikihisa, Y. (2005). Anaplasma Phagocytophilum Inhibits Human Neutrophil Apoptosis via Upregulation of Bfl-1, Maintenance of Mitochondrial Membrane Potential and Prevention of Caspase 3 Activation. Cell Microbiol. 7 (1), 29–38. doi: 10.1111/j.1462-5822.2004.00427.x
Goldstein, L. S., Philp, A. V. (1999). The Road Less Traveled: Emerging Principles of Kinesin Motor Utilization. Annu. Rev. Cell Dev. Biol. 15, 141–183. doi: 10.1146/annurev.cellbio.15.1.141
Gordon, M. A., Graham, S. M., Walsh, A. L., Wilson, L., Phiri, A., Molyneux, E., et al. (2008). Epidemics of Invasive Salmonella Enterica Serovar Enteritidis and S. Enterica Serovar Typhimurium Infection Associated With Multidrug Resistance Among Adults and Children in Malawi. Clin. Infect. Dis. 46 (7), 963–969. doi: 10.1086/529146
Green, R. S., Izac, J. R., Naimi, W. A., O'Bier, N., Breitschwerdt, E. B., Marconi, R. T., et al. (2020). Ehrlichia Chaffeensis EplA Interaction With Host Cell Protein Disulfide Isomerase Promotes Infection. Front. Cell. Infect. Microbiol. 10:500 (500). doi: 10.3389/fcimb.2020.00500
Green, E. R., Mecsas, J. (2016). Bacterial Secretion Systems: An Overview. Microbiol. Spectr. 4 (1), 1–32. doi: 10.1128/microbiolspec.VMBF-0012-2015
Gross, A., Spiesser, S., Terraza, A., Rouot, B., Caron, E., Dornand, J. (1998). Expression and Bactericidal Activity of Nitric Oxide Synthase in Brucella Suis-Infected Murine Macrophages. Infect. Immun. 66 (4), 1309–1316. doi: 10.1128/IAI.66.4.1309-1316.1998
Guo, Q., Bi, J., Li, M., Ge, W., Xu, Y., Fan, W., et al. (2019). ESX Secretion-Associated Protein C From Mycobacterium Tuberculosis Induces Macrophage Activation Through the Toll-Like Receptor-4/Mitogen-Activated Protein Kinase Signaling Pathway. Front. Cell Infect. Microbiol. 9:158. doi: 10.3389/fcimb.2019.00158
Haag, A. F., Myka, K. K., Arnold, M. F., Caro-Hernandez, P. (2010). And Ferguson, G.P). Importance of Lipopolysaccharide and Cyclic Beta-1,2-Glucans in Brucella-Mammalian Infections. Int. J. Microbiol. 2010, 124509. doi: 10.1155/2010/124509
Haine, V., Dozot, M., Dornand, J., Letesson, J. J., De Bolle, X. (2006). NnrA is Required for Full Virulence and Regulates Several Brucella Melitensis Denitrification Genes. J. Bacteriol. 188 (4), 1615–1619. doi: 10.1128/jb.188.4.1615-1619.2006
Harrison, R. E., Brumell, J. H., Khandani, A., Bucci, C., Scott, C. C., Jiang, X., et al. (2004). Salmonella Impairs RILP Recruitment to Rab7 During Maturation of Invasion Vacuoles. Mol. Biol. Cell 15 (7), 3146–3154. doi: 10.1091/mbc.e04-02-0092
Hay, J. C., Chao, D. S., Kuo, C. S., Scheller, R. H. (1997). Protein Interactions Regulating Vesicle Transport Between the Endoplasmic Reticulum and Golgi Apparatus in Mammalian Cells. Cell 89 (1), 149–158. doi: 10.1016/s0092-8674(00)80191-9
Hayward, R. J., Marsh, J. W., Humphrys, M. S., Huston, W. M., Myers, G. S. A. (2019). Early Transcriptional Landscapes of Chlamydia Trachomatis-Infected Epithelial Cells at Single Cell Resolution. Front. Cell. Infect. Microbiol. 9, 392 (392). doi: 10.3389/fcimb.2019.00392
He, Y., Reichow, S., Ramamoorthy, S., Ding, X., Lathigra, R., Craig, J. C., et al. (2006). Brucella Melitensis Triggers Time-Dependent Modulation of Apoptosis and Down-Regulation of Mitochondrion-Associated Gene Expression in Mouse Macrophages. Infect. Immun. 74 (9), 5035–5046. doi: 10.1128/IAI.01998-05
Hong, W., Wu, Y. E., Fu, X., Chang, Z. (2012). Chaperone-Dependent Mechanisms for Acid Resistance in Enteric Bacteria. Trends Microbiol. 20 (7), 328–335. doi: 10.1016/j.tim.2012.03.001
Hoover, D. ,. F. A. (1997). Textbook of Military Medicine: Medical Aspects of Chemical and Biological Warfare. Ed. Zajtchuk, R. (Washington, DC: US Department of the Army, Surgeon General, and the Borden Institute), 513–521.
Horwitz, M. A. (1983). Formation of a Novel Phagosome by the Legionnaires' Disease Bacterium (Legionella Pneumophila) in Human Monocytes. J. Exp. Med. 158 (4), 1319–1331. doi: 10.1084/jem.158.4.1319
Hower, S., Wolf, K., Fields, K. A. (2009). Evidence That CT694 is a Novel Chlamydia Trachomatis T3S Substrate Capable of Functioning During Invasion or Early Cycle Development. Mol. Microbiol. 72 (6), 1423–1437. doi: 10.1111/j.1365-2958.2009.06732.x
Huang, L., Boyd, D., Amyot, W. M., Hempstead, A. D., Luo, Z. Q., O'Connor, T. J., et al. (2011). The E Block Motif is Associated With Legionella Pneumophila Translocated Substrates. Cell Microbiol. 13 (2), 227–245. doi: 10.1111/j.1462-5822.2010.01531.x
Huang, B., Troese, M. J., Howe, D., Ye, S., Sims, J. T., Heinzen, R. A., et al. (2010). Anaplasma Phagocytophilum APH_0032 Is Expressed Late During Infection and Localizes to the Pathogen-Occupied Vacuolar Membrane. Microb. Pathog. 49 (5), 273–284. doi: 10.1016/j.micpath.2010.06.009
Huang, W., Xiong, Q., Lin, M., Rikihisa, Y. (2021). Anaplasma Phagocytophilum Hijacks Flotillin and NPC1 Complex To Acquire Intracellular Cholesterol for Proliferation, Which Can Be Inhibited With Ezetimibe. mBio 12 (5), e0229921. doi: 10.1128/mBio.02299-21
Hybiske, K., Stephens, R. S. (2007a). Mechanisms of Chlamydia Trachomatis Entry Into Nonphagocytic Cells. Infect. Immun. 75 (8), 3925–3934. doi: 10.1128/IAI.00106-07
Hybiske, K., Stephens, R. S. (2007b). Mechanisms of Host Cell Exit by the Intracellular Bacterium Chlamydia. Proc. Natl. Acad. Sci. U.S.A. 104 (27), 11430–11435. doi: 10.1073/pnas.0703218104
Ingmundson, A., Delprato, A., Lambright, D. G., Roy, C. R. (2007). Legionella Pneumophila Proteins That Regulate Rab1 Membrane Cycling. Nature 450 (7168), 365–369. doi: 10.1038/nature06336
Isberg, R. R., O'Connor, T. J., Heidtman, M. (2009). The Legionella Pneumophila Replication Vacuole: Making a Cosy Niche Inside Host Cells. Nat. Rev. Microbiol. 7 (1), 13–24. doi: 10.1038/nrmicro1967
Jahn, R., Scheller, R. H. (2006). SNAREs–engines for Membrane Fusion. Nat. Rev. Mol. Cell Biol. 7 (9), 631–643. doi: 10.1038/nrm2002
Jamwal, S. V., Mehrotra, P., Singh, A., Siddiqui, Z., Basu, A., Rao, K. V. (2016). Mycobacterial Escape From Macrophage Phagosomes to the Cytoplasm Represents an Alternate Adaptation Mechanism. Sci. Rep. 6, 23089. doi: 10.1038/srep23089
Jewett, T. J., Fischer, E. R., Mead, D. J., Hackstadt, T. (2006). Chlamydial TARP is a Bacterial Nucleator of Actin. Proc. Natl. Acad. Sci. U.S.A. 103 (42), 15599–15604. doi: 10.1073/pnas.0603044103
Jiao, H., Zhou, Z., Li, B., Xiao, Y., Li, M., Zeng, H., et al. (2021). The Mechanism of Facultative Intracellular Parasitism of Brucella. Int. J. Mol. Sci. 22 (7), 1–13. doi: 10.3390/ijms22073673
Johnson, N. B., Hayes, L. D., Brown, K., Hoo, E. C., Ethier, K. A., Centers for Disease, C., et al. (2014). CDC National Health Report: Leading Causes of Morbidity and Mortality and Associated Behavioral Risk and Protective Factors–United State-2013. MMWR. Suppl. 63 (4), 3–27.
Johnson, D. E., Ostrowski, P., Jaumouille, V., Grinstein, S. (2016). The Position of Lysosomes Within the Cell Determines Their Luminal pH. J. Cell Biol. 212 (6), 677–692. doi: 10.1083/jcb.201507112
Kagan, J. C., Stein, M. P., Pypaert, M., Roy, C. R. (2004). Legionella Subvert the Functions of Rab1 and Sec22b to Create a Replicative Organelle. J. Exp. Med. 199 (9), 1201–1211. doi: 10.1084/jem.20031706
Kageyama, S., Omori, H., Saitoh, T., Sone, T., Guan, J. L., Akira, S., et al. (2011). The LC3 Recruitment Mechanism is Separate From Atg9L1-Dependent Membrane Formation in the Autophagic Response Against Salmonella. Mol. Biol. Cell 22 (13), 2290–2300. doi: 10.1091/mbc.E10-11-0893
Kang, P. B., Azad, A. K., Torrelles, J. B., Kaufman, T. M., Beharka, A., Tibesar, E., et al. (2005). The Human Macrophage Mannose Receptor Directs Mycobacterium Tuberculosis Lipoarabinomannan-Mediated Phagosome Biogenesis. J. Exp. Med. 202 (7), 987–999. doi: 10.1084/jem.20051239
Kaur, J., Jain, S. K. (2012). Role of Antigens and Virulence Factors of Salmonella Enterica Serovar Typhi in its Pathogenesis. Microbiol. Res. 167 (4), 199–210. doi: 10.1016/j.micres.2011.08.001
Kenney, L. J. (2019). The Role of Acid Stress in Salmonella Pathogenesis. Curr. Opin. Microbiol. 47, 45–51. doi: 10.1016/j.mib.2018.11.006
Kinchen, J. M., Ravichandran, K. S. (2008). Phagosome Maturation: Going Through the Acid Test. Nat. Rev. Mol. Cell Biol. 9 (10), 781–795. doi: 10.1038/nrm2515
Kitao, T., Nagai, H., Kubori, T. (2020). Divergence of Legionella Effectors Reversing Conventional and Unconventional Ubiquitination. Front. Cell. Infect. Microbiol. 10:448 (448). doi: 10.3389/fcimb.2020.00448
Knodler, L. A. (2015). Salmonella Enterica: Living a Double Life in Epithelial Cells. Curr. Opin. Microbiol. 23, 23–31. doi: 10.1016/j.mib.2014.10.010
Knodler, L. A., Vallance, B. A., Celli, J., Winfree, S., Hansen, B., Montero, M., et al. (2010). Dissemination of Invasive Salmonella via Bacterial-Induced Extrusion of Mucosal Epithelia. Proc. Natl. Acad. Sci. U.S.A. 107 (41), 17733–17738. doi: 10.1073/pnas.1006098107
Kocan, K. M., de la Fuente, J., Blouin, E. F., Coetzee, J. F., Ewing, S. A. (2010). The Natural History of Anaplasma Marginale. Vet. Parasitol. 167 (2-4), 95–107. doi: 10.1016/j.vetpar.2009.09.012
Koliwer-Brandl, H., Knobloch, P., Barisch, C., Welin, A., Hanna, N., Soldati, T., et al. (2019). Distinct Mycobacterium Marinum Phosphatases Determine Pathogen Vacuole Phosphoinositide Pattern, Phagosome Maturation, and Escape to the Cytosol. Cell Microbiol. 21 (6), e13008. doi: 10.1111/cmi.13008
Kotewicz, K. M., Ramabhadran, V., Sjoblom, N., Vogel, J. P., Haenssler, E., Zhang, M., et al. (2017). A Single Legionella Effector Catalyzes a Multistep Ubiquitination Pathway to Rearrange Tubular Endoplasmic Reticulum for Replication. Cell Host Microbe 21 (2), 169–181. doi: 10.1016/j.chom.2016.12.007
Kubelkova, K., Macela, A. (2019). Innate Immune Recognition: An Issue More Complex Than Expected. Front. Cell. Infect. Microbiol. 9, 241 (241). doi: 10.3389/fcimb.2019.00241
Kucera, A., Bakke, O., Progida, C. (2016). The Multiple Roles of Rab9 in the Endolysosomal System. Commun. Integr. Biol. 9 (4), e1204498. doi: 10.1080/19420889.2016.1204498
Ku, B., Lee, K. H., Park, W. S., Yang, C. S., Ge, J., Lee, S. G., et al. (2012). VipD of Legionella Pneumophila Targets Activated Rab5 and Rab22 to Interfere With Endosomal Trafficking in Macrophages. PloS Pathog. 8 (12), e1003082. doi: 10.1371/journal.ppat.1003082
Kumar, Y., Valdivia, R. H. (2008). Actin and Intermediate Filaments Stabilize the Chlamydia Trachomatis Vacuole by Forming Dynamic Structural Scaffolds. Cell Host Microbe 4 (2), 159–169. doi: 10.1016/j.chom.2008.05.018
Kumar, Y., Valdivia, R. H. (2009). Leading a Sheltered Life: Intracellular Pathogens and Maintenance of Vacuolar Compartments. Cell Host Microbe 5 (6), 593–601. doi: 10.1016/j.chom.2009.05.014
Kunz, T. C., Götz, R., Sauer, M., Rudel, T. (2019). Detection of Chlamydia Developmental Forms and Secreted Effectors by Expansion Microscopy. Front. Cell. Infect. Microbiol. 9, 276 (276). doi: 10.3389/fcimb.2019.00276
Lahiri, A., Eswarappa, S. M., Das, P., Chakravortty, D. (2010). Altering the Balance Between Pathogen Containing Vacuoles and Lysosomes: A Lesson From Salmonella. Virulence 1 (4), 325–329. doi: 10.4161/viru.1.4.12361
Leipheimer, J., Bloom, A. L. M., Panepinto, J. C. (2019). Protein Kinases at the Intersection of Translation and Virulence. Front. Cell. Infect. Microbiol. 9, 318 (318). doi: 10.3389/fcimb.2019.00318
Leseigneur, C., Le-Bury, P., Pizarro-Cerda, J., Dussurget, O. (2020). Emerging Evasion Mechanisms of Macrophage Defenses by Pathogenic Bacteria. Front. Cell Infect. Microbiol. 10:577559. doi: 10.3389/fcimb.2020.577559
Lin, M., Rikihisa, Y. (2003a). Ehrlichia Chaffeensis and Anaplasma Phagocytophilum Lack Genes for Lipid A Biosynthesis and Incorporate Cholesterol for Their Survival. Infect. Immun. 71 (9), 5324–5331. doi: 10.1128/iai.71.9.5324-5331.2003
Lin, M., Rikihisa, Y. (2003b). Obligatory Intracellular Parasitism by Ehrlichia Chaffeensis and Anaplasma Phagocytophilum Involves Caveolae and Glycosylphosphatidylinositol-Anchored Proteins. Cell Microbiol. 5 (11), 809–820. doi: 10.1046/j.1462-5822.2003.00322.x
Liss, V., Swart, A. L., Kehl, A., Hermanns, N., Zhang, Y., Chikkaballi, D., et al. (2017). Salmonella Enterica Remodels the Host Cell Endosomal System for Efficient Intravacuolar Nutrition. Cell Host Microbe 21 (3), 390–402. doi: 10.1016/j.chom.2017.02.005
Li, P., Vassiliadis, D., Ong, S. Y., Bennett-Wood, V., Sugimoto, C., Yamagishi, J., et al. (2020). Legionella Pneumophila Infection Rewires the Acanthamoeba Castellanii Transcriptome, Highlighting a Class of Sirtuin Genes. Front. Cell. Infect. Microbiol. 10, 428 (428). doi: 10.3389/fcimb.2020.00428
Loisel-Meyer, S., Jimenez de Bagues, M. P., Basseres, E., Dornand, J., Kohler, S., Liautard, J. P., et al. (2006). Requirement of norD for Brucella Suis Virulence in a Murine Model of In Vitro and In Vivo Infection. Infect. Immun. 74 (3), 1973–1976. doi: 10.1128/iai.74.3.1973-1976.2006
Lopez-Montero, N., Ramos-Marques, E., Risco, C., Garcia-Del Portillo, F. (2016). Intracellular Salmonella Induces Aggrephagy of Host Endomembranes in Persistent Infections. Autophagy 12 (10), 1886–1901. doi: 10.1080/15548627.2016.1208888
Lou, L., Zhang, P., Piao, R., Wang, Y. (2019). Salmonella Pathogenicity Island 1 (SPI-1) and Its Complex Regulatory Network. Front. Cell. Infect. Microbiol. 9:270 (270). doi: 10.3389/fcimb.2019.00270
MacGurn, J. A., Cox, J. S. (2007). A Genetic Screen for Mycobacterium Tuberculosis Mutants Defective for Phagosome Maturation Arrest Identifies Components of the ESX-1 Secretion System. Infect. Immun. 75 (6), 2668–2678. doi: 10.1128/IAI.01872-06
Magunda, F., Thompson, C. W., Schneider, D. A., Noh, S. M. (2016). Anaplasma Marginale Actively Modulates Vacuolar Maturation During Intracellular Infection of Its Tick Vector, Dermacentor Andersoni. Appl. Environ. Microbiol. 82 (15), 4715–4731. doi: 10.1128/AEM.01030-16
Mambu, J., Barilleau, E., Fragnet-Trapp, L., Le Vern, Y., Olivier, M., Sadrin, G., et al. (2020). Rck of Salmonella Typhimurium Delays the Host Cell Cycle to Facilitate Bacterial Invasion. Front. Cell. Infect. Microbiol. 10:586934 (656). doi: 10.3389/fcimb.2020.586934
Marei, H., Malliri, A. (2017). GEFs: Dual Regulation of Rac1 Signaling. Small. GTPases. 8 (2), 90–99. doi: 10.1080/21541248.2016.1202635
Martinez, E., Allombert, J., Cantet, F., Lakhani, A., Yandrapalli, N., Neyret, A., et al. (2016). Coxiella Burnetii Effector CvpB Modulates Phosphoinositide Metabolism for Optimal Vacuole Development. Proc. Natl. Acad. Sci. U.S.A. 113 (23), E3260–E3269. doi: 10.1073/pnas.1522811113
Martinez, E., Huc-Brandt, S., Brelle, S., Allombert, J., Cantet, F., Gannoun-Zaki, L., et al. (2020). The Secreted Protein Kinase CstK From Coxiella Burnetii Influences Vacuole Development and Interacts With the GTPase-Activating Host Protein TBC1D5. J. Biol. Chem. 295 (21), 7391–7403. doi: 10.1074/jbc.RA119.010112
Matteelli, A., Roggi, A., Carvalho, A. C. (2014). Extensively Drug-Resistant Tuberculosis: Epidemiology and Management. Clin. Epidemiol. 6, 111–118. doi: 10.2147/CLEP.S35839
Maurya, R. K., Bharti, S., Krishnan, M. Y. (2019). Triacylglycerols: Fuelling the Hibernating Mycobacterium Tuberculosis. Front. Cell. Infect. Microbiol. 8, 450 (450). doi: 10.3389/fcimb.2018.00450
McDade, J. E., Shepard, C. C., Fraser, D. W., Tsai, T. R., Redus, M. A., Dowdle, W. R. (1977). Legionnaires' Disease: Isolation of a Bacterium and Demonstration of its Role in Other Respiratory Disease. N. Engl. J. Med. 297 (22), 1197–1203. doi: 10.1056/nejm197712012972202
Mehlitz, A., Karunakaran, K., Herweg, J. A., Krohne, G., van de Linde, S., Rieck, E., et al. (2014). The Chlamydial Organism Simkania Negevensis Forms ER Vacuole Contact Sites and Inhibits ER-Stress. Cell Microbiol. 16 (8), 1224–1243. doi: 10.1111/cmi.12278
Meresse, S., Steele-Mortimer, O., Finlay, B. B., Gorvel, J. P. (1999). The Rab7 GTPase Controls the Maturation of Salmonella Typhimurium-Containing Vacuoles in HeLa Cells. EMBO J. 18 (16), 4394–4403. doi: 10.1093/emboj/18.16.4394
Miller, H. E., Hoyt, F. H., Heinzen, R. A. (2019). Replication of Coxiella Burnetii in a Lysosome-Like Vacuole Does Not Require Lysosomal Hydrolases. Infect. Immun. 87 (11), 1–13. doi: 10.1128/IAI.00493-19
Miller, C. N., Smith, E. P., Cundiff, J. A., Knodler, L. A., Bailey Blackburn, J., Lupashin, V., et al. (2017). A Brucella Type IV Effector Targets the COG Tethering Complex to Remodel Host Secretory Traffic and Promote Intracellular Replication. Cell Host Microbe 22 (3), 317–329 e317. doi: 10.1016/j.chom.2017.07.017
Mills, S. D., Finlay, B. B. (1998). Isolation and Characterization of Salmonella Typhimurium and Yersinia Pseudotuberculosis-Containing Phagosomes From Infected Mouse Macrophages: Y. Pseudotuberculosis Traffics to Terminal Lysosomes Where They Are Degraded. Eur. J. Cell Biol. 77 (1), 35–47. doi: 10.1016/s0171-9335(98)80100-3
Mishra, A. K., Del Campo, C. M., Collins, R. E., Roy, C. R., Lambright, D. G. (2013). The Legionella Pneumophila GTPase Activating Protein LepB Accelerates Rab1 Deactivation by a non-Canonical Hydrolytic Mechanism. J. Biol. Chem. 288 (33), 24000–24011. doi: 10.1074/jbc.M113.470625
Mital, J., Miller, N. J., Fischer, E. R., Hackstadt, T. (2010). Specific Chlamydial Inclusion Membrane Proteins Associate With Active Src Family Kinases in Microdomains That Interact With the Host Microtubule Network. Cell Microbiol. 12 (9), 1235–1249. doi: 10.1111/j.1462-5822.2010.01465.x
Mitchell, G., Chen, C., Portnoy, D. A. (2016). Strategies Used by Bacteria to Grow in Macrophages. Microbiol. Spectr. 4 (3), 1–38. doi: 10.1128/microbiolspec.MCHD-0012-2015
Mnich, M. E., van Dalen, R., van Sorge, N. M. (2020). C-Type Lectin Receptors in Host Defense Against Bacterial Pathogens. Front. Cell. Infect. Microbiol. 10:309 (309). doi: 10.3389/fcimb.2020.00309
Mohareer, K., Medikonda, J., Vadankula, G. R., Banerjee, S. (2020). Mycobacterial Control of Host Mitochondria: Bioenergetic and Metabolic Changes Shaping Cell Fate and Infection Outcome. Front. Cell. Infect. Microbiol. 10:457 (457). doi: 10.3389/fcimb.2020.00457
Monjarás Feria, J., Valvano, M. A. (2020). An Overview of Anti-Eukaryotic T6SS Effectors. Front. Cell. Infect. Microbiol. 10:584751 (617). doi: 10.3389/fcimb.2020.584751
Moore, E. R., Fischer, E. R., Mead, D. J., Hackstadt, T. (2008). The Chlamydial Inclusion Preferentially Intercepts Basolaterally Directed Sphingomyelin-Containing Exocytic Vacuoles. Traffic 9 (12), 2130–2140. doi: 10.1111/j.1600-0854.2008.00828.x
Moorhead, A. M., Jung, J. Y., Smirnov, A., Kaufer, S., Scidmore, M. A. (2010). Multiple Host Proteins That Function in Phosphatidylinositol-4-Phosphate Metabolism are Recruited to the Chlamydial Inclusion. Infect. Immun. 78 (5), 1990–2007. doi: 10.1128/IAI.01340-09
Moulder, J. W. (1991). Interaction of Chlamydiae and Host Cells. Vitro. Microbiol. Rev. 55 (1), 143–190. doi: 10.1128/mr.55.1.143-190.1991
Moule, M. G., Cirillo, J. D. (2020). Mycobacterium Tuberculosis Dissemination Plays a Critical Role in Pathogenesis. Front. Cell Infect. Microbiol. 10, 65. doi: 10.3389/fcimb.2020.00065
Murata, T., Delprato, A., Ingmundson, A., Toomre, D. K., Lambright, D. G., Roy, C. R. (2006). The Legionella Pneumophila Effector Protein DrrA Is a Rab1 Guanine Nucleotide-Exchange Factor. Nat. Cell Biol. 8 (9), 971–977. doi: 10.1038/ncb1463
Murray, J. T., Panaretou, C., Stenmark, H., Miaczynska, M., Backer, J. M. (2002). Role of Rab5 in the Recruitment of Hvps34/P150 to the Early Endosome. Traffic 3 (6), 416–427. doi: 10.1034/j.1600-0854.2002.30605.x
Myeni, S., Child, R., Ng, T. W., Kupko, J. J., 3rd, Wehrly, T. D., Porcella, S. F., et al. (2013). Brucella Modulates Secretory Trafficking via Multiple Type IV Secretion Effector Proteins. PloS Pathog. 9 (8), e1003556. doi: 10.1371/journal.ppat.1003556
Nagai, H., Kagan, J. C., Zhu, X., Kahn, R. A., Roy, C. R. (2002). A Bacterial Guanine Nucleotide Exchange Factor Activates ARF on Legionella Phagosomes. Science 295 (5555), 679–682. doi: 10.1126/science.1067025
Newton, P., Latomanski, E. A., Newton, H. J. (2016). Applying Fluorescence Resonance Energy Transfer (FRET) to Examine Effector Translocation Efficiency by Coxiella Burnetii During siRNA Silencing. J. Vis. Exp. 113, 1–12. doi: 10.3791/54210
Newton, H. J., McDonough, J. A., Roy, C. R. (2013). Effector Protein Translocation by the Coxiella Burnetii Dot/Icm Type IV Secretion System Requires Endocytic Maturation of the Pathogen-Occupied Vacuole. PloS One 8 (1), e54566. doi: 10.1371/journal.pone.0054566
Newton, H. J., Roy, C. R. (2011). The Coxiella Burnetii Dot/Icm System Creates a Comfortable Home Through Lysosomal Renovation. mBio 2 (5), 1–3. doi: 10.1128/mBio.00226-11
Newton, P., Thomas, D. R., Reed, S. C. O., Lau, N., Xu, B., Ong, S. Y., et al. (2020). Lysosomal Degradation Products Induce Coxiella Burnetii Virulence. Proc. Natl. Acad. Sci. U.S.A. 117 (12), 6801–6810. doi: 10.1073/pnas.1921344117
Ninio, S., Roy, C. R. (2007). Effector Proteins Translocated by Legionella Pneumophila: Strength in Numbers. Trends Microbiol. 15 (8), 372–380. doi: 10.1016/j.tim.2007.06.006
Niu, H., Kozjak-Pavlovic, V., Rudel, T., Rikihisa, Y. (2010). Anaplasma Phagocytophilum Ats-1 Is Imported Into Host Cell Mitochondria and Interferes With Apoptosis Induction. PloS Pathog. 6 (2), e1000774. doi: 10.1371/journal.ppat.1000774
Niu, H., Rikihisa, Y. (2013). Ats-1: A Novel Bacterial Molecule That Links Autophagy to Bacterial Nutrition. Autophagy 9 (5), 787–788. doi: 10.4161/auto.23693
Niu, H., Xiong, Q., Yamamoto, A., Hayashi-Nishino, M., Rikihisa, Y. (2012). Autophagosomes Induced by a Bacterial Beclin 1 Binding Protein Facilitate Obligatory Intracellular Infection. Proc. Natl. Acad. Sci. U.S.A. 109 (51), 20800–20807. doi: 10.1073/pnas.1218674109
Nuoffer, C., Davidson, H. W., Matteson, J., Meinkoth, J., Balch, W. E. (1994). A GDP-Bound of Rab1 Inhibits Protein Export From the Endoplasmic Reticulum and Transport Between Golgi Compartments. J. Cell Biol. 125 (2), 225–237. doi: 10.1083/jcb.125.2.225
O'Brien, K. M., Lindsay, E. L., Starai, V. J. (2015). The Legionella Pneumophila Effector Protein, LegC7, Alters Yeast Endosomal Trafficking. PloS One 10 (2), e0116824. doi: 10.1371/journal.pone.0116824
O'Hare, H. M., Duran, R., Cervenansky, C., Bellinzoni, M., Wehenkel, A. M., Pritsch, O., et al. (2008). Regulation of Glutamate Metabolism by Protein Kinases in Mycobacteria. Mol. Microbiol. 70 (6), 1408–1423. doi: 10.1111/j.1365-2958.2008.06489.x
Olson, M. G., Widner, R. E., Jorgenson, L. M., Lawrence, A., Lagundzin, D., Woods, N. T., et al. (2019). Proximity Labeling To Map Host-Pathogen Interactions at the Membrane of a Bacterium-Containing Vacuole in Chlamydia Trachomatis-Infected Human Cells. Infect. Immun. 87 (11), 1–31. doi: 10.1128/IAI.00537-19
Omsland, A., Sixt, B. S., Horn, M., Hackstadt, T. (2014). Chlamydial Metabolism Revisited: Interspecies Metabolic Variability and Developmental Stage-Specific Physiologic Activities. FEMS Microbiol. Rev. 38 (4), 779–801. doi: 10.1111/1574-6976.12059
Paixao, T. A., Roux, C. M., den Hartigh, A. B., Sankaran-Walters, S., Dandekar, S., Santos, R. L., et al. (2009). Establishment of Systemic Brucella Melitensis Infection Through the Digestive Tract Requires Urease, the Type IV Secretion System, and Lipopolysaccharide O Antigen. Infect. Immun. 77 (10), 4197–4208. doi: 10.1128/IAI.00417-09
Pechstein, J., Schulze-Luehrmann, J., Bisle, S., Cantet, F., Beare, P. A., Ölke, M., et al. (2020). The Coxiella Burnetii T4SS Effector AnkF Is Important for Intracellular Replication. Front. Cell. Infect. Microbiol. 10, 559915 (695). doi: 10.3389/fcimb.2020.559915
Pei, J., Ficht, T. A. (2004). Brucella Abortus Rough Mutants are Cytopathic for Macrophages in Culture. Infect. Immun. 72 (1), 440–450. doi: 10.1128/iai.72.1.440-450.2004
Piersanti, S., Burla, R., Licursi, V., Brito, C., La Torre, M., Alves, P. M., et al. (2015). Transcriptional Response of Human Neurospheres to Helper-Dependent CAV-2 Vectors Involves the Modulation of DNA Damage Response, Microtubule and Centromere Gene Groups. PloS One 10 (7), e0133607. doi: 10.1371/journal.pone.0133607
Pitt, A., Mayorga, L. S., Stahl, P. D., Schwartz, A. L. (1992). Alterations in the Protein Composition of Maturing Phagosomes. J. Clin. Invest. 90 (5), 1978–1983. doi: 10.1172/jci116077
Pizarro-Cerda, J., Meresse, S., Parton, R. G., van der Goot, G., Sola-Landa, A., Lopez-Goni, I., et al. (1998). Brucella Abortus Transits Through the Autophagic Pathway and Replicates in the Endoplasmic Reticulum of Nonprofessional Phagocytes. Infect. Immun. 66 (12), 5711–5724. doi: 10.1128/IAI.66.12.5711-5724.1998
Poncin, K., Roba, A., Jimmidi, R., Potemberg, G., Fioravanti, A., Francis, N., et al. (2019). Occurrence and Repair of Alkylating Stress in the Intracellular Pathogen Brucella Abortus. Nat. Commun. 10 (1), 4847. doi: 10.1038/s41467-019-12516-8
Porte, F., Liautard, J. P., Kohler, S. (1999). Early Acidification of Phagosomes Containing Brucella Suis Is Essential for Intracellular Survival in Murine Macrophages. Infect. Immun. 67 (8), 4041–4047. doi: 10.1128/IAI.67.8.4041-4047.1999
Porte, F., Naroeni, A., Ouahrani-Bettache, S., Liautard, J. P. (2003). Role of the Brucella Suis Lipopolysaccharide O Antigen in Phagosomal Genesis and in Inhibition of Phagosome-Lysosome Fusion in Murine Macrophages. Infect. Immun. 71 (3), 1481–1490. doi: 10.1128/iai.71.3.1481-1490.2003
Radhakrishnan, G. K., Harms, J. S., Splitter, G. A. (2011). Modulation of Microtubule Dynamics by a TIR Domain Protein From the Intracellular Pathogen Brucella Melitensis. Biochem. J. 439 (1), 79–83. doi: 10.1042/BJ20110577
Rahman, A., Sobia, P., Gupta, N., Kaer, L. V., Das, G. (2014). Mycobacterium Tuberculosis Subverts the TLR-2-MyD88 Pathway to Facilitate Its Translocation Into the Cytosol. PloS One 9 (1), e86886. doi: 10.1371/journal.pone.0086886
Rama, F., Pranadinata, S. R. P., Soumita, D. (2015). The Hijacking of Host Endocytic Trafficking by the Bacterial Pathogen. MOJ. Immunol. 2 (1- 2015), 1–2. doi: 10.15406/moji.2015.02.00037
Ramos-Morales, F. (2012). Impact of Salmonella Enterica Type III Secretion System Effectors on the Eukaryotic Host Cell. Int. Scholarly. Res. Notices. 2012 1–37. doi: 10.5402/2012/787934
Ray, K., Marteyn, B., Sansonetti, P. J., Tang, C. M. (2009). Life on the Inside: The Intracellular Lifestyle of Cytosolic Bacteria. Nat. Rev. Microbiol. 7 (5), 333–340. doi: 10.1038/nrmicro2112
Richards, A. M., Von Dwingelo, J. E., Price, C. T., Abu Kwaik, Y. (2013). Cellular Microbiology and Molecular Ecology of Legionella-Amoeba Interaction. Virulence 4 (4), 307–314. doi: 10.4161/viru.24290
Rittig, M. G., Kaufmann, A., Robins, A., Shaw, B., Sprenger, H., Gemsa, D., et al. (2003). Smooth and Rough Lipopolysaccharide Phenotypes of Brucella Induce Different Intracellular Trafficking and Cytokine/Chemokine Release in Human Monocytes. J. Leukoc. Biol. 74 (6), 1045–1055. doi: 10.1189/jlb.0103015
Robertson, G. T., Roop, R. M., Jr. (1999). The Brucella Abortus Host Factor I (HF-I) Protein Contributes to Stress Resistance During Stationary Phase and is a Major Determinant of Virulence in Mice. Mol. Microbiol. 34 (4), 690–700. doi: 10.1046/j.1365-2958.1999.01629.x
Robinson, C. G., Roy, C. R. (2006). Attachment and Fusion of Endoplasmic Reticulum With Vacuoles Containing Legionella Pneumophila. Cell Microbiol. 8 (5), 793–805. doi: 10.1111/j.1462-5822.2005.00666.x
Roop, R. M., 2nd, Bellaire, B. H., Valderas, M. W., Cardelli, J. A. (2004). Adaptation of the Brucellae to Their Intracellular Niche. Mol. Microbiol. 52 (3), 621–630. doi: 10.1111/j.1365-2958.2004.04017.x
Roop, R. M., Gaines, J. M., Anderson, E. S., Caswell, C. C., Martin, D. W. (2009). Survival of the Fittest: How Brucella Strains Adapt to Their Intracellular Niche in the Host. Med. Microbiol. Immunol. 198 (4), 221–238. doi: 10.1007/s00430-009-0123-8
Roy, C. R., Berger, K. H., Isberg, R. R. (1998). Legionella Pneumophila DotA Protein is Required for Early Phagosome Trafficking Decisions That Occur Within Minutes of Bacterial Uptake. Mol. Microbiol. 28 (3), 663–674. doi: 10.1046/j.1365-2958.1998.00841.x
Rzomp, K. A., Moorhead, A. R., Scidmore, M. A. (2006). The GTPase Rab4 Interacts With Chlamydia Trachomatis Inclusion Membrane Protein CT229. Infect. Immun. 74 (9), 5362–5373. doi: 10.1128/iai.00539-06
Rzomp, K. A., Scholtes, L. D., Briggs, B. J., Whittaker, G. R., Scidmore, M. A. (2003). Rab GTPases are Recruited to Chlamydial Inclusions in Both a Species-Dependent and Species-Independent Manner. Infect. Immun. 71 (10), 5855–5870. doi: 10.1128/iai.71.10.5855-5870.2003
Sachdeva, K., Sundaramurthy, V. (2020). The Interplay of Host Lysosomes and Intracellular Pathogens. Front. Cell. Infect. Microbiol. 10, 595502 (713). doi: 10.3389/fcimb.2020.595502
Saha, S., Das, P., BoseDasgupta, S. (2020). “It Takes Two to Tango”: Role of Neglected Macrophage Manipulators Coronin 1 and Protein Kinase G in Mycobacterial Pathogenesis. Front. Cell. Infect. Microbiol. 10:582563 (546). doi: 10.3389/fcimb.2020.582563
Sasaki, T., Takasuga, S., Sasaki, J., Kofuji, S., Eguchi, S., Yamazaki, M., et al. (2009). Mammalian Phosphoinositide Kinases and Phosphatases. Prog. Lipid Res. 48 (6), 307–343. doi: 10.1016/j.plipres.2009.06.001
Scales, S. J., Pepperkok, R., Kreis, T. E. (1997). Visualization of ER-To-Golgi Transport in Living Cells Reveals a Sequential Mode of Action for COPII and COPI. Cell 90 (6), 1137–1148. doi: 10.1016/s0092-8674(00)80379-7
Schafer, W., Schmidt, T., Cordsmeier, A., Borges, V., Beare, P. A., Pechstein, J., et al. (2020). The Anti-Apoptotic Coxiella Burnetii Effector Protein AnkG Is a Strain Specific Virulence Factor. Sci. Rep. 10 (1), 15396. doi: 10.1038/s41598-020-72340-9
Scherler, A., Jacquier, N., Greub, G. (2018). Chlamydiales, Anaplasma and Bartonella: Persistence and Immune Escape of Intracellular Bacteria. Microbes Infect. 20 (7-8), 416–423. doi: 10.1016/j.micinf.2017.11.002
Scherr, N., Honnappa, S., Kunz, G., Mueller, P., Jayachandran, R., Winkler, F., et al. (2007). Structural Basis for the Specific Inhibition of Protein Kinase G, a Virulence Factor of Mycobacterium Tuberculosis. Proc. Natl. Acad. Sci. U.S.A. 104 (29), 12151–12156. doi: 10.1073/pnas.0702842104
Schlumberger, M. C., Hardt, W. D. (2006). Salmonella Type III Secretion Effectors: Pulling the Host Cell's Strings. Curr. Opin. Microbiol. 9 (1), 46–54. doi: 10.1016/j.mib.2005.12.006
Schroeder, G. N., Hilbi, H. (2008). Molecular Pathogenesis of Shigella Spp.: Controlling Host Cell Signaling, Invasion, and Death by Type III Secretion. Clin. Microbiol. Rev. 21 (1), 134–156. doi: 10.1128/CMR.00032-07
Sedzicki, J., Tschon, T., Low, S. H., Willemart, K., Goldie, K. N., Letesson, J. J., et al. (2018). 3D Correlative Electron Microscopy Reveals Continuity of Brucella-Containing Vacuoles With the Endoplasmic Reticulum. J. Cell Sci. 131 (4), 1–11. doi: 10.1242/jcs.210799
Segal, G., Feldman, M., Zusman, T. (2005). The Icm/Dot Type-IV Secretion Systems of Legionella Pneumophila and Coxiella Burnetii. FEMS Microbiol. Rev. 29 (1), 65–81. doi: 10.1016/j.femsre.2004.07.001
Sharma, P. V., Thaiss, C. A. (2020). Host-Microbiome Interactions in the Era of Single-Cell Biology. Front. Cell Infect. Microbiol. 10, 569070. doi: 10.3389/fcimb.2020.569070
Shi, X., Halder, P., Yavuz, H., Jahn, R., Shuman, H. A. (2016). Direct Targeting of Membrane Fusion by SNARE Mimicry: Convergent Evolution of Legionella Effectors. Proc. Natl. Acad. Sci. U.S.A. 113 (31), 8807–8812. doi: 10.1073/pnas.1608755113
Siadous, F. A., Cantet, F., Van Schaik, E., Burette, M., Allombert, J., Lakhani, A., et al. (2021). Coxiella Effector Protein CvpF Subverts RAB26-Dependent Autophagy to Promote Vacuole Biogenesis and Virulence. Autophagy 17 (3), 706–722. doi: 10.1080/15548627.2020.1728098
Sindhwani, A., Arya, S. B., Kaur, H., Jagga, D., Tuli, A., Sharma, M. (2017). Salmonella Exploits the Host Endolysosomal Tethering Factor HOPS Complex to Promote its Intravacuolar Replication. PloS Pathog. 13 (10), e1006700. doi: 10.1371/journal.ppat.1006700
Smith, A. C., Cirulis, J. T., Casanova, J. E., Scidmore, M. A., Brumell, J. H. (2005). Interaction of the Salmonella-Containing Vacuole With the Endocytic Recycling System. J. Biol. Chem. 280 (26), 24634–24641. doi: 10.1074/jbc.M500358200
Smith, E. P., Cotto-Rosario, A., Borghesan, E., Held, K., Miller, C. N., Celli, J. (2020). Epistatic Interplay Between Type IV Secretion Effectors Engages the Small GTPase Rab2 in the Brucella Intracellular Cycle. mBio 11 (2), 1–14. doi: 10.1128/mBio.03350-19
Smith, A. C., Heo, W. D., Braun, V., Jiang, X., Macrae, C., Casanova, J. E., et al. (2007). A Network of Rab GTPases Controls Phagosome Maturation and is Modulated by Salmonella Enterica Serovar Typhimurium. J. Cell Biol. 176 (3), 263–268. doi: 10.1083/jcb.200611056
Snaka, T., Fasel, N. (2020). Behind the Scenes: Nod-Like Receptor X1 Controls Inflammation and Metabolism. Front. Cell Infect. Microbiol. 10, 609812. doi: 10.3389/fcimb.2020.609812
Stamm, L. M., Morisaki, J. H., Gao, L. Y., Jeng, R. L., McDonald, K. L., Roth, R., et al. (2003). Mycobacterium Marinum Escapes From Phagosomes and is Propelled by Actin-Based Motility. J. Exp. Med. 198 (9), 1361–1368. doi: 10.1084/jem.20031072
Starr, T., Child, R., Wehrly, T. D., Hansen, B., Hwang, S., Lopez-Otin, C., et al. (2012). Selective Subversion of Autophagy Complexes Facilitates Completion of the Brucella Intracellular Cycle. Cell Host Microbe 11 (1), 33–45. doi: 10.1016/j.chom.2011.12.002
Starr, T., Ng, T. W., Wehrly, T. D., Knodler, L. A., Celli, J. (2008). Brucella Intracellular Replication Requires Trafficking Through the Late Endosomal/Lysosomal Compartment. Traffic 9 (5), 678–694. doi: 10.1111/j.1600-0854.2008.00718.x
Steele, K. H., Baumgartner, J. E., Valderas, M. W., Roop, R. M., 2nd (2010). Comparative Study of the Roles of AhpC and KatE as Respiratory Antioxidants in Brucella Abortus 2308. J. Bacteriol. 192 (19), 4912–4922. doi: 10.1128/jb.00231-10
Steele-Mortimer, O. (2008). The Salmonella-Containing Vacuole: Moving With the Times. Curr. Opin. Microbiol. 11 (1), 38–45. doi: 10.1016/j.mib.2008.01.002
Steele-Mortimer, O., Meresse, S., Gorvel, J. P., Toh, B. H., Finlay, B. B. (1999). Biogenesis of Salmonella Typhimurium-Containing Vacuoles in Epithelial Cells Involves Interactions With the Early Endocytic Pathway. Cell Microbiol. 1 (1), 33–49. doi: 10.1046/j.1462-5822.1999.00003.x
Stein, M. A., Leung, K. Y., Zwick, M., Garcia-del Portillo, F., Finlay, B. B. (1996). Identification of a Salmonella Virulence Gene Required for Formation of Filamentous Structures Containing Lysosomal Membrane Glycoproteins Within Epithelial Cells. Mol. Microbiol. 20 (1), 151–164. doi: 10.1111/j.1365-2958.1996.tb02497.x
Stevenin, V., Chang, Y. Y., Le Toquin, Y., Duchateau, M., Gianetto, Q. G., Luk, C. H., et al. (2019). Dynamic Growth and Shrinkage of the Salmonella-Containing Vacuole Determines the Intracellular Pathogen Niche. Cell Rep. 29 (12), 3958–3973 e3957. doi: 10.1016/j.celrep.2019.11.049
Stuen, S., Granquist, E. G., Silaghi, C. (2013). Anaplasma Phagocytophilum–a Widespread Multi-Host Pathogen With Highly Adaptive Strategies. Front. Cell Infect. Microbiol. 3, 31. doi: 10.3389/fcimb.2013.00031
Sun, J., Wang, X., Lau, A., Liao, T. Y., Bucci, C., Hmama, Z. (2010). Mycobacterial Nucleoside Diphosphate Kinase Blocks Phagosome Maturation in Murine RAW 264.7 Macrophages. PloS One 5 (1), e8769. doi: 10.1371/journal.pone.0008769
Swanson, M. S., Isberg, R. R. (1995). Association of Legionella Pneumophila With the Macrophage Endoplasmic Reticulum. Infect. Immun. 63 (9), 3609–3620. doi: 10.1128/iai.63.9.3609-3620.1995
Szulc-Dabrowska, L., Bossowska-Nowicka, M., Struzik, J., Toka, F. N. (2020). Cathepsins in Bacteria-Macrophage Interaction: Defenders or Victims of Circumstance? Front. Cell Infect. Microbiol. 10, 601072. doi: 10.3389/fcimb.2020.601072
Thakur, A., Mikkelsen, H., Jungersen, G. (2019). Intracellular Pathogens: Host Immunity and Microbial Persistence Strategies. J. Immunol. Res. 2019:1356540. doi: 10.1155/2019/1356540
Thalmann, J., Janik, K., May, M., Sommer, K., Ebeling, J., Hofmann, F., et al. (2010). Actin Re-Organization Induced by Chlamydia Trachomatis Serovar D–evidence for a Critical Role of the Effector Protein CT166 Targeting Rac. PloS One 5 (3), e9887. doi: 10.1371/journal.pone.0009887
Thomas, D. R., Newton, P., Lau, N., Newton, H. J. (2020). Interfering With Autophagy: The Opposing Strategies Deployed by Legionella Pneumophila and Coxiella Burnetii Effector Proteins. Front. Cell. Infect. Microbiol. 10, 599762 (685). doi: 10.3389/fcimb.2020.599762
Tisdale, E. J., Bourne, J. R., Khosravi-Far, R., Der, C. J., Balch, W. E. (1992). GTP-Binding Mutants of Rab1 and Rab2 are Potent Inhibitors of Vesicular Transport From the Endoplasmic Reticulum to the Golgi Complex. J. Cell Biol. 119 (4), 749–761. doi: 10.1083/jcb.119.4.749
Tolchard, J., Walpole, S. J., Miles, A. J., Maytum, R., Eaglen, L. A., Hackstadt, T., et al. (2018). The Intrinsically Disordered Tarp Protein From Chlamydia Binds Actin With a Partially Preformed Helix. Sci. Rep. 8 (1), 1960. doi: 10.1038/s41598-018-20290-8
Toledo, A., Benach, J. L. (2015). Hijacking and Use of Host Lipids by Intracellular Pathogens. Microbiol. Spectr. 3 (6), 1–37. doi: 10.1128/microbiolspec.VMBF-0001-2014
Troese, M. J., Kahlon, A., Ragland, S. A., Ottens, A. K., Ojogun, N., Nelson, K. T., et al. (2011). Proteomic Analysis of Anaplasma Phagocytophilum During Infection of Human Myeloid Cells Identifies a Protein That is Pronouncedly Upregulated on the Infectious Dense-Cored Cell. Infect. Immun. 79 (11), 4696–4707. doi: 10.1128/iai.05658-11
Tsolis, R. M., Kingsley, R. A., Townsend, S. M., Ficht, T. A., Adams, L. G., Baumler, A. J. (1999). Of Mice, Calves, and Men. Comparison of the Mouse Typhoid Model With Other Salmonella Infections. Adv. Exp. Med. Biol. 473, 261–274. doi: 10.1007/978-1-4615-4143-1_28
Turner, J., Torrelles, J. B. (2018). Mannose-Capped Lipoarabinomannan in Mycobacterium Tuberculosis Pathogenesis. Pathog. Dis. 76 (4), 1–15. doi: 10.1093/femspd/fty026
Ueti, M. W., Knowles, D. P., Davitt, C. M., Scoles, G. A., Baszler, T. V., Palmer, G. H. (2009). Quantitative Differences in Salivary Pathogen Load During Tick Transmission Underlie Strain-Specific Variation in Transmission Efficiency of Anaplasma Marginale. Infect. Immun. 77 (1), 70–75. doi: 10.1128/iai.01164-08
Urbanus, M. L., Quaile, A. T., Stogios, P. J., Morar, M., Rao, C., Di Leo, R., et al. (2016). Diverse Mechanisms of Metaeffector Activity in an Intracellular Bacterial Pathogen, Legionella Pneumophila. Mol. Syst. Biol. 12 (12), 893. doi: 10.15252/msb.20167381
Valderas, M. W., Alcantara, R. B., Baumgartner, J. E., Bellaire, B. H., Robertson, G. T., Ng, W. L., et al. (2005). Role of HdeA in Acid Resistance and Virulence in Brucella Abortus 2308. Vet. Microbiol. 107 (3-4), 307–312. doi: 10.1016/j.vetmic.2005.01.018
Valentin-Hansen, P., Eriksen, M., Udesen, C. (2004). The Bacterial Sm-Like Protein Hfq: A Key Player in RNA Transactions. Mol. Microbiol. 51 (6), 1525–1533. doi: 10.1111/j.1365-2958.2003.03935.x
van Ooij, C., Apodaca, G., Engel, J. (1997). Characterization of the Chlamydia Trachomatis Vacuole and its Interaction With the Host Endocytic Pathway in HeLa Cells. Infect. Immun. 65 (2), 758–766. doi: 10.1128/IAI.65.2.758-766.1997
Vaughn, B., Voth, K., Price, C. T., Jones, S., Ozanic, M., Santic, M., et al. (2021). An Indispensable Role for the MavE Effector of Legionella Pneumophila in Lysosomal Evasion. mBio 12 (1), 1–21. doi: 10.1128/mBio.03458-20
Vergne, I., Chua, J., Lee, H. H., Lucas, M., Belisle, J., Deretic, V. (2005). Mechanism of Phagolysosome Biogenesis Block by Viable Mycobacterium Tuberculosis. Proc. Natl. Acad. Sci. U.S.A. 102 (11), 4033–4038. doi: 10.1073/pnas.0409716102
Vergne, I., Chua, J., Singh, S. B., Deretic, V. (2004). Cell Biology of Mycobacterium Tuberculosis Phagosome. Annu. Rev. Cell Dev. Biol. 20, 367–394. doi: 10.1146/annurev.cellbio.20.010403.114015
Vieira, O. V., Botelho, R. J., Grinstein, S. (2002). Phagosome Maturation: Aging Gracefully. Biochem. J. 366 (Pt 3), 689–704. doi: 10.1042/BJ20020691
von Bargen, K., Gorvel, J. P., Salcedo, S. P. (2012). Internal Affairs: Investigating the Brucella Intracellular Lifestyle. FEMS Microbiol. Rev. 36 (3), 533–562. doi: 10.1111/j.1574-6976.2012.00334.x
Walpole, G. F. W., Grinstein, S., Westman, J. (2018). The Role of Lipids in Host-Pathogen Interactions. IUBMB Life 70 (5), 384–392. doi: 10.1002/iub.1737
Wang, M., Qazi, I. H., Wang, L., Zhou, G., Han, H. (2020a). Salmonella Virulence and Immune Escape. Microorganisms 8 (3), 1–25. doi: 10.3390/microorganisms8030407
Wang, Y., Shi, M., Feng, H., Zhu, Y., Liu, S., Gao, A., et al. (2018). Structural Insights Into Non-Canonical Ubiquitination Catalyzed by SidE. Cell 173 (5), 1231–1243.e1216. doi: 10.1016/j.cell.2018.04.023
Wang, X., Sun, J., Wan, L., Yang, X., Lin, H., Zhang, Y., et al. (2020b). The Shigella Type III Secretion Effector IpaH4.5 Targets NLRP3 to Activate Inflammasome Signaling. Front. Cell. Infect. Microbiol. 10, 511798 (523). doi: 10.3389/fcimb.2020.511798
Warsinske, H. C., DiFazio, R. M., Linderman, J. J., Flynn, J. L., Kirschner, D. E. (2017). Identifying Mechanisms Driving Formation of Granuloma-Associated Fibrosis During Mycobacterium Tuberculosis Infection. J. Theor. Biol. 429, 1–17. doi: 10.1016/j.jtbi.2017.06.017
Weber, S. S., Ragaz, C., Hilbi, H. (2009). Pathogen Trafficking Pathways and Host Phosphoinositide Metabolism. Mol. Microbiol. 71 (6), 1341–1352. doi: 10.1111/j.1365-2958.2009.06608.x
Wilson, B. S., Nuoffer, C., Meinkoth, J. L., McCaffery, M., Feramisco, J. R., Balch, W. E., et al. (1994). A Rab1 Mutant Affecting Guanine Nucleotide Exchange Promotes Disassembly of the Golgi Apparatus. J. Cell Biol. 125 (3), 557–571. doi: 10.1083/jcb.125.3.557
Woldehiwet, Z. (2006). Anaplasma Phagocytophilum in Ruminants in Europe. Ann. N. Y. Acad. Sci. 1078, 446–460. doi: 10.1196/annals.1374.084
Wong, D., Bach, H., Sun, J., Hmama, Z., Av-Gay, Y. (2011). Mycobacterium Tuberculosis Protein Tyrosine Phosphatase (PtpA) Excludes Host Vacuolar-H+-ATPase to Inhibit Phagosome Acidification. Proc. Natl. Acad. Sci. U.S.A. 108 (48), 19371–19376. doi: 10.1073/pnas.1109201108
Wong, D., Li, W., Chao, J. D., Zhou, P., Narula, G., Tsui, C., et al. (2018). Protein Tyrosine Kinase, PtkA, is Required for Mycobacterium Tuberculosis Growth in Macrophages. Sci. Rep. 8 (1), 155. doi: 10.1038/s41598-017-18547-9
Wu, Y. E., Hong, W., Liu, C., Zhang, L., Chang, Z. (2008). Conserved Amphiphilic Feature is Essential for Periplasmic Chaperone HdeA to Support Acid Resistance in Enteric Bacteria. Biochem. J. 412 (2), 389–397. doi: 10.1042/BJ20071682
Xavier, M. N., T, A. P., den Hartigh, A. B., Tsolis, R. M., Santos, R. L. (2010). Pathogenesis of Brucella Spp. Open Vet. Sci. J. 4, 109–118. doi: 10.2174/1874318801004010109
Xiong, Q., Lin, M., Rikihisa, Y. (2009). Cholesterol-Dependent Anaplasma Phagocytophilum Exploits the Low-Density Lipoprotein Uptake Pathway. PloS Pathog. 5 (3), e1000329. doi: 10.1371/journal.ppat.1000329
Xu, D., Joglekar, A. P., Williams, A. L., Hay, J. C. (2000). Subunit Structure of a Mammalian ER/Golgi SNARE Complex. J. Biol. Chem. 275 (50), 39631–39639. doi: 10.1074/jbc.M007684200
Xu, J., Laine, O., Masciocchi, M., Manoranjan, J., Smith, J., Du, S. J., et al. (2007). A Unique Mycobacterium ESX-1 Protein Co-Secretes With CFP-10/ESAT-6 and is Necessary for Inhibiting Phagosome Maturation. Mol. Microbiol. 66 (3), 787–800. doi: 10.1111/j.1365-2958.2007.05959.x
Xu, Y., Zhou, P., Cheng, S., Lu, Q., Nowak, K., Hopp, A. K., et al. (2019). A Bacterial Effector Reveals the V-ATPase-ATG16L1 Axis That Initiates Xenophagy. Cell 178 (3), 552–566.e520. doi: 10.1016/j.cell.2019.06.007
Young, E. J. (200). “Brucella Species” in Douglas and Bennet's Principles and Practice of Infectious Disease. Eds. Mandell, G. L., Bennet, J. E., Dolin, R. (Philadelphia, PA: Churchill Livingstone), 2386–2393.
Keywords: vacuoles, intracellular pathogens, biogenesis, lysosomes, trafficking
Citation: Vaughn B and Abu Kwaik Y (2021) Idiosyncratic Biogenesis of Intracellular Pathogens-Containing Vacuoles. Front. Cell. Infect. Microbiol. 11:722433. doi: 10.3389/fcimb.2021.722433
Received: 08 June 2021; Accepted: 25 October 2021;
Published: 11 November 2021.
Edited by:
Annette C. Vergunst, U1047, Bacterial Virulence and Chronic Infections (INSERM), FranceReviewed by:
Yasuko Rikihisa, The Ohio State University, United StatesMatteo Bonazzi, Centre National de la Recherche Scientifique (CNRS), France
Jean Louis Herrmann, Université de Versailles Saint-Quentin-en-Yvelines, France
Copyright © 2021 Vaughn and Abu Kwaik. This is an open-access article distributed under the terms of the Creative Commons Attribution License (CC BY). The use, distribution or reproduction in other forums is permitted, provided the original author(s) and the copyright owner(s) are credited and that the original publication in this journal is cited, in accordance with accepted academic practice. No use, distribution or reproduction is permitted which does not comply with these terms.
*Correspondence: Yousef Abu Kwaik, YWJ1a3dhaWtAbG91aXN2aWxsZS5lZHU=