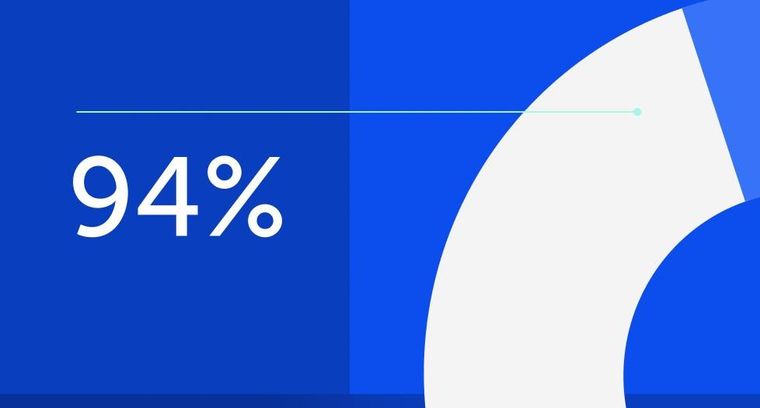
94% of researchers rate our articles as excellent or good
Learn more about the work of our research integrity team to safeguard the quality of each article we publish.
Find out more
REVIEW article
Front. Cell. Infect. Microbiol., 04 August 2021
Sec. Biofilms
Volume 11 - 2021 | https://doi.org/10.3389/fcimb.2021.720742
This article is part of the Research TopicMicrobial Biofilms Interacting with Host Mucosal SurfacesView all 6 articles
Non-typeable Haemophilus influenzae (NTHi) is an ubiquitous commensal-turned-pathogen that colonises the respiratory mucosa in airways diseases including Chronic Obstructive Pulmonary Disease (COPD). COPD is a progressive inflammatory syndrome of the lungs, encompassing chronic bronchitis that is characterised by mucus hypersecretion and impaired mucociliary clearance and creates a static, protective, humid, and nutrient-rich environment, with dysregulated mucosal immunity; a favourable environment for NTHi colonisation. Several recent large COPD cohort studies have reported NTHi as a significant and recurrent aetiological pathogen in acute exacerbations of COPD. NTHi proliferation has been associated with increased hospitalisation, disease severity, morbidity and significant lung microbiome shifts. However, some cohorts with patients at different severities of COPD do not report that NTHi is a significant aetiological pathogen in their COPD patients, indicating other obligate pathogens including Moraxella catarrhalis, Streptococcus pneumoniae and Pseudomonas aeruginosa as the cause. NTHi is an ubiquitous organism across healthy non-smokers, healthy smokers and COPD patients from childhood to adulthood, but it currently remains unclear why NTHi becomes pathogenic in only some cohorts of COPD patients, and what behaviours, interactions and adaptations are driving this susceptibility. There is emerging evidence that biofilm-phase NTHi may play a significant role in COPD. NTHi displays many hallmarks of the biofilm lifestyle and expresses key biofilm formation-promoting genes. These include the autoinducer-mediated quorum sensing system, epithelial- and mucus-binding adhesins and expression of a protective, self-produced polymeric substance matrix. These NTHi biofilms exhibit extreme tolerance to antimicrobial treatments and the immune system as well as expressing synergistic interspecific interactions with other lung pathogens including S. pneumoniae and M. catarrhalis. Whilst the majority of our understanding surrounding NTHi as a biofilm arises from otitis media or in-vitro bacterial monoculture models, the role of NTHi biofilms in the COPD lung is now being studied. This review explores the evidence for the existence of NTHi biofilms and their impact in the COPD lung. Understanding the nature of chronic and recurrent NTHi infections in acute exacerbations of COPD could have important implications for clinical treatment and identification of novel bactericidal targets.
COPD is currently the third leading cause of global mortality affecting an estimated 174 – 384 million people worldwide and is responsible for 3.2 million deaths annually (Rabe and Watz, 2017). COPD is caused by the inhalation of noxious particles and gases and at least 90% of COPD patients live in developing countries where the disease is mainly associated with biomass fuel burning and pollution. The remaining 10% live in developed countries where the predominant cause is cigarette smoking (Rabe and Watz, 2017). COPD is a heterogenous progressive, inflammatory syndrome of the lungs, characterised by the co-existence of predominantly two distinct syndromes; chronic bronchitis and emphysema (Rabe et al., 2007). Together these conditions result in a chronic productive cough, poorly reversible airflow obstruction and alveolar parenchymal destruction (MacNee, 2006), punctuated by episodic acute exacerbations of COPD (AECOPD), that lead to hospitalisation (Wilkinson et al., 2017).
Chronic bronchitis is particularly important with respect to the role of bacteria and chronic infections. An immunological cascade in response to inhaled particles and gases drives airway structural remodelling that leads to hypertrophy and hyperplasia of sub-mucosal bronchial glands and mucus-producing goblet cells (MacNee, 2006), forming a viscous and alkaline mucus layer to trap particles and bacteria. Excess mucus production and damage to the airway epithelium by inflammation and oxidative stress results in ciliary dysfunction, which is also caused by the direct effects of smoking. This impairs mucociliary clearance and leads to the formation of obstructive mucus plugs, changing the biotic and abiotic conditions of the lung (McDaniel et al., 2015; Panchabhai et al., 2016). Static mucus provides a nutrient-rich substrate for the colonisation of bacteria and mucus plugs trapped in bronchioles may form local anaerobic regions (McDaniel et al., 2015). Further immunological responses to bacterial infection include the formation of neutrophil extracellular traps (NETs) in severe COPD patients that has been associated with more frequent exacerbations and a reduction in lung microbiome diversity (Dicker et al., 2018), as well as modulation of the complement system that enhances phagocytotic clearance of bacteria (Ahearn et al., 2017).
Together these conditions may lead to significant microbiome shifts and favour the proliferation of fastidious, facultatively anaerobic pathogens, and drive a biofilm lifestyle.
Contrary to historical views, the lung is not a sterile environment but has a rich and diverse microflora. The role of the lung microbiome and microbial dysbiosis in respiratory diseases is becoming increasingly understood through the advent of 16S rRNA sequencing of sputum, deep lung brushes and biopsy samples (Pragman et al., 2016), where the microbial composition of sputum samples closely resembles that of the low biomass bronchial and peripheral lung samples (Pragman et al., 2018). This has led to the characterisation of microbial patterns of respiratory disease and of COPD sub-types (Mayhew et al., 2018), supporting a patient-specific approach to treatment. However, there is currently no formal curated database of the Human Lung Microbiota, which has not formed part of the Human Microbiome Project (Beck et al., 2012). Therefore, there is disparity across lung microbiome investigations (Table 1) into airways diseases, partly driven by the lack of standardisation of methods of sample acquisition and processing (Pragman et al., 2018).
The general consensus reports that the healthy lung microbiota is largely comprised of Pseudomonas, Streptococcus, Prevotella, Fusobacterium, Veillonella Porphyromonas and Haemophilus spp. (Erb-Downward et al., 2011; Beck et al., 2012) that together represent >85% of the core lung microbiome. These genera have an important commensal role in modulating the immune response mechanisms of the lung and priming of the immune system (Segal et al., 2014). These genera are ubiquitous inhabitants of the lung microenvironment in both health and disease such as COPD, which is associated with an overall reduction in this microbial diversity. However, this microbial community composition remains diverse between healthy, non-smoker individuals, and microbial diversity further varies in healthy-smokers and COPD patients. Microbiota composition changes also occur in COPD patients as a consequence of the chronic infection process, host immune system responses and long-term use of bronchodilators, antibiotics and corticosteroid therapy (Toraldo and Conte, 2019). Previously, significantly different clusters of microbial communities have been reported between severe (GOLD 3/4) and less severe (GOLD 1/2) COPD patients (Mika et al., 2018), with correlations also being drawn between the abundance of Moraxellaceae and Streptococcaceae with the expression of immune system factors including IL-10 and TNF-α (Mika et al., 2018). Lung microbiome comparisons also detected significant increases in Proteobacteria and Haemophilus spp. and reductions in Bacteroidetes, Prevotella and Veillonella (Mayhew et al., 2018) in severe and very severe COPD patients compared to moderate COPD patients (Mayhew et al., 2018). Coupled with a decrease in overall diversity, these studies highlight how the lung microbiota increasingly destabilises throughout COPD progression.
Whilst this core, heterogeneous microbial diversity exists without necessarily causing disease, individually, all of these genera are also associated with microbial diversity shifts, or dysbiosis, that contribute to oral and airways disease states. These include periodontitis, cystic fibrosis, pneumonia, and inflammatory lung diseases (Pragman et al., 2016). The normal lung microbiome is extremely diverse, and some studies report Neisseria (Hilty et al., 2010), Acinetobacter (Cabrera-Rubio et al., 2012), Sphingomonas, Megasphaera and Staphylococcus (Zakharkina et al., 2013) as predominant genera in both health and disease. Additionally, fungi, bacteriophages and viruses, including human rhinovirus (HRV), (Molyneaux et al., 2013) constitute part of this microbiome and have complex interactions with the bacteria, driving dysbiosis such as significantly increasing the proportion of Proteobacteria including Haemophilus spp. in the sputum microbiome of COPD patients (Molyneaux et al., 2013). Therefore, the existence of these bacteria in individuals in both health and disease states suggests that the aetiological factor for disease is not solely due to the presence of pathogens but a change in how those pathogens interact with the host and other bacteria i.e. their behaviour.
Of these genera, there is a substantial amount of evidence that suggests non-typeable Haemophilus influenzae (NTHi) is responsible for a large subset of AECOPD hospitalisations (Nakou et al., 2014; Wilkinson et al., 2017; Jubinville et al., 2018), alongside Streptococcus pneumoniae and Moraxella catarrhalis in several recent studies (Table 1). NTHi is an ubiquitous, non-encapsulated Gram-negative coccobacillus and opportunistic pathogen (Clementi and Murphy, 2011) despite having commensal properties (Hartwig et al., 2016). This commensal-turned-pathogen is the most common cause of Haemophilus spp. infection across all ages (Slack, 2017), is present in 70% COPD patients (Wilkinson et al., 2017) and is associated with 24% of AECOPD hospitalisations (Nakou et al., 2014). Furthermore, higher bacterial loads of NTHi are correlated with more severe airway inflammation, more severe AECOPD and increased disease burden on the patient (King and Sharma, 2015). However, NTHi presence does not explain every incidence of AECOPD across different patient cohorts, raising the question of how the behaviour of NTHi has changed in these cases to cause a disease state. One current hypothesis is that in developed countries, the routine immunisation of infants with the conjugated H. influenzae type B (Hib) vaccine and prescription of COPD maintenance drugs (Van Eldere et al., 2014) (Mayhew et al., 2018) has contributed to lung microbiome dysbiosis that favours NTHi colonisation and provides immunological pressures for the selection of biofilm-forming NTHi strains (Foxwell et al., 1998). For example, selection for NTHi strains expressing different outer-membrane proteins (OMPs) has led to a change in behaviour, favouring attachment and colonisation of the airways epithelia (Foxwell et al., 1998; Post et al., 2014), implicated in COPD infections (Cerquetti and Giufre, 2016). Similarly, antibiotic and maintenance treatments have been shown to be largely ineffective in combating the microbial causes of COPD in the long term. Systematic reviews have revealed that regular, long-term prophylactic antibiotic prescription did not result in reduced hospitalisation or improvement in lung function nor mortality (Herath et al., 2018). In addition, long-term maintenance drugs, including inhalation of corticosteroids, has been found to increase sputum bacterial load (Contoli et al., 2017). Moreover, short-term 3-month treatments with either moxifloxacin, doxycycline or azithromycin antibiotics were all found to not significantly decrease airway bacterial load but instead promoted antibiotic resistance in all treatment groups (Brill et al., 2015). These observations demonstrate how changes in the behaviour of NTHi, driven by disease progression and treatment strategies, may promote the biofilm lifestyle, contributing to disease.
Many severe COPD patients are receiving ‘long-term’ treatment with macrolide antibiotics that exhibit secondary anti-inflammatory properties and immune-modulating effects (Yao et al., 2013; Qiu and Zhong, 2017). Meta-analysis of several COPD cohorts has shown that macrolide antibiotic treatment successfully decreases the frequency of AECOPD when prescribed over 6 – 12 months (Yao et al., 2013) by suppressing bacterial load and reducing inflammation (Kelly et al., 2018). However, macrolides exert no benefit in the short term over 3 months (Yao et al., 2013). These findings have been corroborated using low-dose, long-term macrolide therapy up to 12 months (Cao et al., 2019) and current guidelines indicate optimal usage over 6 – 12 months in patients who experience 3 or more AECOPD per year, are prescribed steroids and are hospitalised due to AECOPD at least once per year (Smith et al., 2020). However, these guidelines do not describe long-term treatment that exceeds 12-months. This presents an issue for patients living with a progressive and currently incurable respiratory disease. Long term macrolide therapy also presents several risks, with studies reporting non-fatal but adverse effects including gastrointestinal reactions, liver injury and ototoxicity (Yao et al., 2013). In addition, due to the increased risk of comorbidities, macrolide therapy has been reported as being unsuitable for the elderly (Cao et al., 2019; Watson and Wilkinson, 2021). Further systematic reviews have reported the increasing risk of these adverse events as well as macrolide antibiotic resistance (Cui et al., 2018). This resistance is achieved by multiple mechanisms including modification of macrolide target sequences and upregulation of efflux pumps that increase with prolonged use of macrolides over 12-months (Djamin et al., 2020). Despite the benefit of macrolides in reducing AECOPD, much of the supporting evidence is attributed to azithromycin and not wider macrolides (Kelly et al., 2018), and ‘long-term’ treatment is limited to only 12 months. Accompanied by the reported risks of serious adverse events and acquisition of antibiotic resistance, macrolide therapy does not represent a substantial solution to controlling the bacterial and inflammatory components of COPD.
It is also important to recognise the limitations of these previous lung microbiome studies. There was previously no standardisation of microbiome analysis, such as the use of different 16S rRNA hypervariable regions (Beck et al., 2012). Many studies contained only small, cross-sectional cohorts (Wang et al., 2016). Furthermore, unprotected specimen brushings or sputum samples have also been widely used, which may introduce oropharyngeal contamination (Pragman et al., 2018). Together, these factors may limit the accuracy with which the healthy lung microbiome is reported and how it differs in disease states over time. However, results highlighting the importance of NTHi are reciprocated across several COPD cohorts by PCR, culture (Nakou et al., 2014; Wilkinson et al., 2017) and 16S rRNA techniques (Mayhew et al., 2018), warranting further investigation of NTHi and this pathogen’s life cycle within the COPD lung.
Different microbiome identification techniques should also be taken into consideration to allow the fair comparative appraisal of results between studies. A major advantage of culture techniques is that media is often selective and enriched with supplements that promote the growth of fastidious organisms, allowing low-abundance organisms to be detected and cultured (Hiergeist et al., 2015), whilst also eliminating contaminants. Furthermore, culture methods can lead to useful downstream experimental models and assays including antimicrobial susceptibility testing. However, whilst culture methods are improving, it is low-throughput and a central dogma is that only around 1% bacteria are culturable (Martiny, 2019), and those that can be cultured are likely altered under the nutrient-rich growth conditions. In addition, culture techniques may also introduce bias, depending on which selective growth media are used, based upon the microorganisms that are expected to be present in the sample. Together, these limitations mean the composition of bacteria detected using culture may not be a valid representation of the lung microbiome. Whilst 16S rRNA sequencing does not detect fungi and viral constituents, its major advantage is its sensitivity to the detection of phenotypically aberrant, rarely isolated or unculturable bacteria, as well as novel pathogens (Clarridge, 2004). Advances in Illumina sequencing and bioinformatic approaches also allow high-throughput detection, analysis and quantification of entire microbiome communities from multiple samples. However, high 16S rRNA sequence similarities may make it difficult to distinguish beyond the genus level in some taxa. The analysis is also dependent on the choice of one of nine bacterial 16S hypervariable regions (V1 – V9) with no single hypervariable region exhibiting a different enough degree of sequence diversity to distinguish amongst all bacteria at the species level (Chakravorty et al., 2007). Whilst not a sterile environment, the lung microbiome does have extremely low biomass which increases its susceptibility to contaminant sequences during sample acquisition, processing and 16S rRNA sequencing (Zubiria-Barrera et al., 2020). This may also explain high variances between studies.
It is clear however, that NTHi presents a significant problem for a large number of COPD patients and their clinicians alike, being detected across culture and 16S rRNA techniques. These patient-cohort focused studies show that, despite widespread use of antibiotics, NTHi persist in the COPD lung and lead to chronic and recurrent infections, exhibiting extreme tolerance to antimicrobial immune system defences and pharmacological treatments as well as displaying synergistic or co-infection interactions with other pathogens. Whilst NTHi intracellular infection is a well-documented mode of persistence in COPD, by becoming internalised and surviving within host cells as a protected reservoir that facilitates recurrent infection (Clementi and Murphy, 2011), NTHi exhibits distinct adaptations that are typical hallmarks of the biofilm lifestyle (Bjarnsholt, 2013). These include biotic surface adherence, extreme tolerance and resistance to antibiotics and antimicrobials, persistence of sub-populations of bacteria following treatment leading to recurrent infection, extreme capacity for evading host antimicrobial immunological defences and complex interspecific or polymicrobial interactions (Bjarnsholt, 2013).
NTHi is well adapted for colonisation of the human airways. This fastidious organism survives in a mucus-rich environment and has a complex nutritional requirement for haemin and nicotinamide adenine dinucleotide, that are both available in the human lungs (Sriram et al., 2018). NTHi expresses an arsenal of proteins that allow it to adhere to and invade respiratory epithelial cells. These proteins include type IV pilus protein and the adhesin OMP P1 that bind to the host cell-surface protein ICAM-1 and glycoprotein CEACAM1 (Novotny and Bakaletz, 2013; Tchoupa et al., 2015), as well as mucin proteins and lactoferrins in human mucus (Kubiet and Ramphal, 1995). These mucus secretions and plugs that coat the airways epithelium may be especially important for the initial attachment stage in the development of bacterial biofilms (Langereis and Hermans, 2013) (Figure 1), which are sessile, three-dimensional multicellular communities of bacteria embedded within a self-produced extracellular polymeric substance (EPS) matrix (Costerton and Lewandowski, 1995). Biofilms exhibit complex strategies that confer persistent or chronic infection and dissemination to secondary sites of infection, characterised by tolerance and resistance to the immune system and antibiotic drugs.
Figure 1 Model of NTHi Biofilm Formation and Lifecycle. (A) The impaired mucociliary clearance system coupled with inflammatory mucus secretion hyperplasia creates a suitable substrate for the initial colonisation and reversible attachment of NTHi to the air-liquid interface of the tracheobronchial respiratory mucosa, a pseudostratified, ciliated, columnar epithelium predominated by ciliated epithelial cells and interspersed by secretory cells (mucus-secreting goblet cells and secretoglobin secreting club cells) and basal cells. In COPD disease states, the mucus layer extends into the periciliary layer. (B) NTHi irreversibly attach to respiratory epithelial cells by adhesins including Type IV Pilus and OMP P1 that bind ICAM-1, CEACAM-1 and CLEC7A. NTHi also binds to the mucus layer itself by having adhesins that bind tracheobronchial mucins and lactoferrins. (C) Irreversibly attached NTHi begin to aggregate and produce an EPS matrix, favouring secondary colonisers. (D) Biofilms exhibit complex intraspecific and interspecific interactions, signalling, metabolism changes, gene expression changes that drive nutrient and oxygen gradients. This leading to differentiation of bacteria and maturation of the biofilm with structurally large microcolonies with nutrient and water channels. (E) Quorum sensing changes drive dispersal of the biofilm as planktonic bacteria and aggregates of biofilm leading to (F) dissemination and colonisation of secondary sites, facilitating persistence. Created with BioRender.com.
The biofilm lifestyle confers several advantages to survival compared to the planktonic or free-living state (Figure 2). Typically, biofilm bacteria exhibit pleiomorphic behaviour, altering their morphology, coordinating gene expression and differentiating metabolomic functions in a heterogeneous manner. These changes occur in response to many factors such as: bacteria population density and quorum sensing, that regulates gene expression cascades; stressful environmental conditions, such as oxygen and nutrient gradients, that develop as the biofilm matures; the immune system; and pharmacological antimicrobial effectors. Additionally, biofilms exhibit physical and chemical strategies against the immune system through the formation of a protective barrier, the EPS matrix, as well as virulence factor modulation, interspecific synergistic interactions with other bacteria and the sharing of antimicrobial-sequestering compounds. The differentiation of metabolically inactive persister cells and dissemination of biofilm to secondary sites are major causes of chronic, recurrent infection following antibiotic treatment of the initial infection, which may be implicated in COPD.
Figure 2 Model of NTHi Biofilm Strategies and Host-Pathogen Interactions Facilitating Chronic Infection in the COPD Airways. (A) The LuxS-RbsB AI-2 quorum sensing system facilitates coordination of intra-specific and inter-specific gene expression that promotes a transition from the planktonic to biofilm lifestyle in response to the local environmental conditions, leading to the downstream transcription of biofilm-associate genes. NTHi also express a QSeB two-component secondary system that may support biofilm gene expression coordination where the LuxS-RbsB system has been impaired. The quorum sensing system drives dissemination of biofilm bacteria to secondary sites, and formation of oxygen, nutrient and water channels towards the centre of the biofilm. (B) NTHi biofilms produce a thick protein- and eDNA-rich EPS matrix that provides a physical and chemical barrier, slowing penetration against pharmacological and immune system antimicrobial agents by blocking phagocytes and macrophages and chelating peptides and antibiotics such as β-defensin and β-lactams. The EPS matrix also provides structural stability and provides a site for mature biofilm structures to form, whilst also limiting metabolic activity, altering microenvironments, and driving persister cells through the development of gradients towards the centre of the biofilm. (C) NTHi express chemical defence strategies that allow for the evasion of the host’s NETosis response through recruitment of NETs into the EPS and expression of catalase that provides oxidative stress tolerance. Recognition by the immune system is also modulated through the differential expression of LOS and OMP P5 and OMP P2 that recruit neutrophils to form NETs but by evading the phagocytosing and cytotoxic mechanisms. NTHi biofilms further undergo a series of surface modifications that promote irreversible attachment, recruit secondary colonisers and impair recognition and/or clearance by immune system factors. These include sialyation, phosphorycholination, and the expression of TfP, OMPs and HMWs. (D) NTHi biofilms exhibit multidrug resistance due to upregulated carbohydrate metabolism and a preference for glycogen metabolism, as well as expressing antibiotic resistance genes including penicillin-binding protein 3 (PBP3) and β-lactamases. Negatively charged eDNA in the EPS matrix also sequesters positively charged antimicrobials. (E) Biofilms are seldom mono-species and exhibit complex inter-specific interactions with other inhabitants of the microbiome. In particular NTHi interacts with other COPD-associated pathogens including S. pneumoniae and M. catarrhalis that selects for hypoxia tolerant NTHi and damages cilia and mucociliary clearance, respectively, favouring NTHi biofilm formation. Tissue shown is the tracheobronchial respiratory mucosa, a pseudostratified, ciliated, columnar epithelium predominated by ciliated epithelial cells and interspersed by secretory cells (mucus-secreting goblet cells and secretoglobin secreting club cells) and basal cells. Created with BioRender.com.
Quorum sensing (QS) is the density-dependent coordination of gene expression (Costerton and Lewandowski, 1995; Langereis and Hermans, 2013) across bacteria within a community that is integral for biofilm formation, development and dispersal. Most biofilms achieve QS through autoinducer-2 (AI-2) chemical signalling (Langereis and Hermans, 2013). Autoinducer expression is encoded by the LuxS homologue (Armbruster et al., 2009) and these signals are detected by the ABC transporter RbsB (Armbruster et al., 2011), leading to further downstream transcription cascades that drive biofilm formation (Figure 2). This signalling mechanism highlights a target for interrupting biofilm development.
Like most other biofilm-forming bacteria, AI expression has been shown to promote NTHi biofilm formation and persistence, although much of this research is in the context of NTHi otitis media (OM) strains grown in-vitro (Armbruster et al., 2009) or the chinchilla middle ear model of NTHi-induced OM (Hong et al., 2009). Scanning confocal laser microscopy (SCLM) of NTHi LuxS mutants grown in continuous flow chambers show significantly reduced biomass, thickness and persistence (Armbruster et al., 2009). Disruption of the NTHi RbsB transporter reciprocated these results (Armbruster et al., 2011) showing that detection of QS signals is just as important as production. Downstream QS cascades result in increased transcription of biofilm-promoting genes including gstA – a glycosyltransferase that increases sialyation of the biofilm matrix (Pang et al., 2018), with gstA mutants producing sialyation deficient and low biomass biofilms.
However, NTHi also express a second, AI-2 independent QS system; the QSeB/C two component system that drives biofilm formation (Unal et al., 2012). However, QSeB/C disruption does not affect the biofilm in the same way as LuxS mutants. Instead, QSeB/C mutants show significant biofilm biomass and surface coverage in continuous flow models over 24 – 48 hours (Unal et al., 2012). Whilst this system is less well understood, the expression of a secondary QS system may explain why NTHi with a disrupted AI-2-RbsB system are still capable of forming reduced biofilms rather than no biofilms at all.
Biofilms exhibit defence strategies against the immune system including survival and persistence within neutrophil extracellular traps (NETs) (Figure 2). NETs are extruding DNA-rich (Hong et al., 2009) webs of decondensed chromatin containing histones, neutrophil elastase and granules that trap bacteria (Dicker et al., 2018). One of the biofilm’s tolerance strategies is the expression of peroxiredoxin-glutaredoxin (pdgx) and catalase that protect bacteria against NET-derived oxidative stress effectors (Juneau et al., 2015) and expression is upregulated in biofilm compared to planktonic strains (Murphy et al., 2005). This NET-survival strategy has been demonstrated in NTHi biofilm OM models that form greater biomass over 14 days (Hong et al., 2009). Immunofluorescence and viability staining techniques demonstrated NTHi biofilms surviving and persisting within the NET lattices themselves. It is hypothesised that NETs can be incorporated into and benefit the structure of the biofilm (Hong et al., 2009). By increasing biomass, biofilms drive metabolism-limiting gradients that induce metabolically inactive persister cells at the centre of the biofilm and provide a physical and chemical barrier to immune system effectors and antimicrobial agents (Langereis and Hermans, 2013).
One of the lung’s primary defences against bacteria is activation of complement that opsonises invading pathogens for phagocytosis by neutrophils and macrophages (Ahearn et al., 2017). However, NTHi have adapted to evade or even ‘hijack’ the complement system, driving collateral damage and host tissue destruction (Su et al., 2018; Santocki and Kolaczkowska, 2020). Several studies report up- and down-regulation of virulence and complement activating factors including lipoligosaccharide (endotoxin) (West-Barnette et al., 2006), sialic acid, outer membrane proteins (OMP P2 and P5) and inflammatory mediators (King and Sharma, 2015) that trigger a local neutrophil response (Figure 2). However, because some NTHi have adapted to hijack complement and are inherently tolerant to NETosis, this increased neutrophil response instead promotes airways inflammation, facilitating intracellular invasion and persistent infection by NTHi.
The EPS matrix is a hallmark of biofilms which confers a protective barrier against the immune system and antimicrobials and is comprised of polysaccharides, proteins, lipids and eDNA (Figure 2), that may constitute 90% of the dry mass of most biofilms (Goodman et al., 2011). Until recently there was little evidence for the production of an EPS matrix (Langereis and Hermans, 2013) as part of the NTHi biomass. Unlike the polysaccharide-rich and alginate-producing biofilms of P. aeruginosa, the aetiological pathogen in chronic cystic fibrosis (CF) infections, NTHi biofilms have a limited carbohydrate or exopolysaccharide component (Domenech et al., 2016), containing only lipooligosaccharide (LOS) endotoxin. The composition of NTHi EPS is largely DNA and protein-rich which may contribute to its success in the hostile lung environment and tolerance to pharmaceuticals through a cascade of host-pathogen interactions (Gunn et al., 2016), despite not being embedded in a thick alginate.
Analysis of 18 biofilm-specific multifunctional proteins has demonstrated that the protein constituent of the EPS is essential for NTHi biofilm formation, maintenance, structural integrity, survival and tolerance to immune-system and antimicrobial effectors (Wu et al., 2014; Domenech et al., 2016). The EPS is structurally stabilised by Nuclear Associated Proteins DNABII that binds bent double stranded extracellular DNA (ds-eDNA), forming a strong nucleoprotein mesh (Goodman et al., 2011). Bent ds-eDNA are curved rather than straight architectures of extracellular DNA for which DNABII family proteins have a higher affinity (Goodman et al., 2011). This eDNA is independent of DNA derived from cell lysis or outer membrane vesicle biogenesis and instead NTHi employs an active mechanism of eDNA release via Tra-dependent inner membrane transit and release through the comE pore (Jurcisek et al., 2017). Bent ds-eDNA-DNABII complexes confer amoxicillin tolerance and increased biomass that is relinquished through antibody degradation of DNABII (Goodman et al., 2011). Immunofluorescent techniques have shown that eDNA provides structural stabilisation, maintenance and expansion of the biofilm to secondary sites (Domenech et al., 2016) and it is arranged in a meshwork of fine strands and thicker, rope-like ds-eDNA structures (Jurcisek and Bakaletz, 2007). Additionally, the negative charge of eDNA sequesters positively charged antimicrobials through a cation chelating interaction, conferring biocide resistance, requiring 1000-fold increases in antibiotic concentration to kill biofilms compared to planktonic phenotypes (Jones et al., 2013). In the chinchilla OM model, eDNA was also found to reduce the activity of the antimicrobial defence peptide (AMP) Beta Defensin-3 (Cavaliere et al., 2014). DNase I mediated degradation of eDNA resulted in reduced biofilm formation and increased susceptibility to ampicillin and ciprofloxacin in-vitro (Izano et al., 2009). Degradation of eDNA also destabilised the strong biofilms formed by clinical NTHi isolates and decreased surface adhesion and biofilm formation in planktonic cultures (Cavaliere et al., 2014).
However, the NTHi biofilm itself has its own eDNA control, mediated by the endogenous nuclease Nuc (Cho et al., 2015). Nuc is thought to be under the control of the QS system, leading to structural remodelling of the biofilm such as the formation of water and nutrient channels (Cho et al., 2015). Low-level expression of endogenous Nuc in NTHi biofilms exerts a similar destructive effect on these discrete areas of the biofilm in a similar way to treatment with DNAse I, but with a 1,400-fold greater activity (Cho et al., 2015). The high expression present in planktonic NTHi likely contributes to maintenance of the planktonic lifestyle. A second mechanism of structural remodelling is expression of the transcription inhibitor ModA2 methyltransferase that decreases eDNA and DNABII expression (Brockman et al., 2018), destabilising the biofilm. Therefore, both Nuc and ModA2 may be considered therapeutic targets of biofilm disruption, by increasing expression to drive NTHi biofilm instability and cause a biofilm to planktonic transition, making the bacteria more susceptible to treatment.
The NTHi outer membrane is particularly protein-rich and the expression of key proteins, including type IV Pilus, OMP P1 and P5 and HMW1/2 adhesins, together facilitate initial attachment directly to the bronchial epithelium and mucin-rich mucus (Kubiet and Ramphal, 1995; Jurcisek and Bakaletz, 2007; Giufre et al., 2008; Novotny and Bakaletz, 2013; Jurcisek et al., 2017), as well as trafficking of the aforementioned eDNA and DNABII components (Figure 2). Whilst NTHi are rich in only one polysaccharide, lipooligosaccharide (LOS), there is a diverse number of LOS and cell-surface modifications expressed in NTHi that are consistent with biofilm-forming bacteria (Swords et al., 2004). These LOS modifications include sialyation and phosphorylcholination that together increase biofilm formation and reduce LOS endotoxin bioactivity, reducing the host’s innate immune response to these substances (Greiner et al., 2004; Swords et al., 2004; West-Barnette et al., 2006). These modifications summarised below (Table 2) may also serve as therapeutic targets as interfering with key biofilm proteins and adhesins shows great potential in disruption of initial biofilm formation and maturation, and thus increases the biofilm’s susceptibility to treatments.
NTHi biofilms exhibit complex chemical multi-drug resistant strategies (Table 3) against a variety of widely used antibiotics (Slinger et al., 2006). Clinically important antibiotics including ciprofloxacin, azithromycin, and amoxicillin were 100% effective in eliminating 28 NTHi OM planktonic isolates whilst only killing 68%, 57% and 4% of NTHi biofilms, respectively (Slinger et al., 2006). Furthermore, 7% of biofilms even survived combined rifampicin - ciprofloxacin treatment. NTHi biofilms are inherently tolerant to high concentrations of gentamycin (Starner et al., 2006) and erythromycin (Starner et al., 2008). This tolerance is achieved partly through upregulated carbohydrate metabolism which drives transformation into the metabolically inactive biofilm phenotype dependent on stored glycogen (Wu et al., 2014). This is a protective, epigenetic response to sub-minimum inhibitory concentrations (sub-MIC) of β-lactam antibiotics (Wu et al., 2014) that target and inhibit the growth of metabolically active bacteria. Other proteomic changes occur acting against reactive oxygen species and promoting a semi-dormant lifestyle, rendering immune system factors and β-lactam antibiotics ineffective (Post et al., 2014). The aforementioned eDNA-rich EPS matrix protects against a range of antimicrobials including chlorhexidine glucoronate (Izano et al., 2009) and antibiotics including ampicillin and ciprofloxacin (Cavaliere et al., 2014). However, NTHi biofilms are, counter-intuitively, susceptible to sub-inhibitory concentrations of the macrolide antibiotic, azithromycin (Starner et al., 2008), which exhibits effective antibiofilm properties not demonstrated by erythromycin or gentamycin (Starner et al., 2008), even against clinical NTHi strains resistant to a wide range of antibiotics. However, the efficacy of azithromycin may not be due to only its antibacterial properties in this context. Recently, azithromycin has proved popular and effective for treatment of lung infections possibly due to its secondary anti-inflammatory properties (Southern and Barker, 2004). The COPD lung is characterised by chronic inflammation, increasing the risk of recurrent infection following treatment. The use of an antibiotic such as azithromycin with secondary anti-inflammatory properties may help decrease the lung’s innate immune system that causes collateral tissue destruction. Additionally, azithromycin has been reported to antagonise the quorum sensing system in P. aeruginosa, leading to decreased biofilm forming capacity, diminished virulence and an impaired oxidative stress response (Nalca et al., 2006), offering one explanation as to why β-lactam–azithromycin combination therapy in a cohort of critical care community-acquired pneumonia (CAP) patients reduced mortality by ~20% (Wise et al., 2010).
Many studies investigate the role of biofilm-phase bacteria in the context of mono-species biofilms. However, because the lung microbiome is polymicrobial (Erb-Downward et al., 2011; Beck et al., 2012; Wilkinson et al., 2017) this diversity may facilitate complex but poorly understood interspecific interactions between bacteria as well as viruses (Molyneaux et al., 2013). These interspecific interactions (Figure 2) may be indirect, through changing of both biotic and abiotic conditions that favour the proliferation of secondary colonisers in airways diseases, analogous to ecological succession (Khanolkar et al., 2020). For example, NTHi infection has been found to upregulate the pro-inflammatory responses that may drive wider microbial shifts and exacerbate COPD (Rotta Detto Loria et al., 2013; Staples et al., 2016) as well as upregulate MUC2 production, increasing airway obstruction and providing a viscous substrate for secondary bacterial colonisers (Zhang et al., 2020) over time. Alternatively these interactions may be more direct through the growth of multi-species biofilms (Kyd et al., 2016) that result in co-operative adhesion and stability of the biofilm structure. NTHi and S. pneumoniae multi-species biofilms, for example, together produce and share a single EPS that is constituted by type IV pilus, eDNA, LOS, QS signals and other proteins, carbohydrates, adhesins and transcription factors (Kyd et al., 2016) from both species. Whilst further research is required to provide direct evidence as to whether NTHi is a primary coloniser of the human respiratory tract in COPD, NTHi does express an arsenal of adhesins and invasins that facilitate initial attachment directly to bronchial epithelia and mucin-rich mucus (Kubiet and Ramphal, 1995; Jurcisek and Bakaletz, 2007; Giufre et al., 2008; Novotny and Bakaletz, 2013; Jurcisek et al., 2017). Additionally, NTHi can express factors that change the environment making subsequent colonisation by other bacteria more favourable. NTHi and S. pneumoniae often co-colonise the respiratory tracts of COPD patients (Jacobs et al., 2018) and interact synergistically, promoting initial attachment, biofilm formation and survival. These interactions include increased expression of virulence genes such as the pilA of type IV Pilus (TfP), a strong and flexible transmembrane filament that has diverse functional roles in pathogenicity. Other interactions include the sharing of β-lactamases and eDNA (Jacobs et al., 2018), and the release of biocidal H2O2 (Tikhomirova and Kidd, 2013) that provides a nutrient and DNA reservoir at the expense of killed bacterial cells and senescent host cells, supporting biofilm survival. Co-culture of NTHi and S. pneumoniae strains was found to significantly increase biofilm formation on cultured respiratory epithelial cells (Krishnamurthy and Kyd, 2014) compared to mono-species biofilms. Whilst these bacteria also interact competitively, competition may not be a negative for NTHi survival in the long term. One such example is that S. pneumoniae expresses bactericidal H2O2 (Bair and Campagnari, 2019) and stimulates neutrophils that together provide a selection pressure for ROS-tolerant and persistent strains of NTHi (Tikhomirova and Kidd, 2013).
M. catarrhalis is a major respiratory pathogen that colonises 5-32% of COPD patients and accounts for 10% of acute exacerbations (Murphy et al., 2005). M. catarrhalis infections usually clear after 40 days but can result in long term biotic changes that favour secondary colonisation of the airways by NTHi (Murphy et al., 2005) following initial M. catarrhalis infection. M. catarrhalis binds to the tips of healthy ciliated epithelia and forms aggregates, significantly reducing cilia beat frequency in bronchial epithelial cultures (Velkova et al., 2018) which results in impairment of the mucociliary clearance pathway and formation of mucus plugs which become colonised by NTHi. In OM models, M. catarrhalis has been demonstrated to stabilise NTHi - S. pneumoniae - M. catarrhalis polymicrobial biofilms by protecting NTHi from the bactericidal properties of S. pneumoniae, further promoting NTHi viability and persistence (Bair and Campagnari, 2019).
A plethora of studies has identified single key genes and transcriptional products associated with biofilm formation and persistence in-vitro and in-vivo in bronchial epithelial and OM models, using clinical NTHi isolates (Table 4). Whilst these studies provide good evidence that NTHi has the capacity to form biofilms under nutrient-rich conditions, it does not confirm whether they actually form stable, persistent biofilms within the human COPD lung. Recently, however, the ferret COPD model, involving chronic long-term exposure to cigarette smoke, has provided immunofluorescent and gene expression evidence for NTHi biofilm formation and persistence (Hunt et al., 2020). Here, smoke-exposed ferret lungs, which presented characteristic histological and immunological hallmarks of COPD, showed increased NTHi aggregation and the expression of four key biofilm-associated genes, pdgX, luxS, dps and hktE, that are together involved in growth, quorum sensing, environmental stress and oxidative stress tolerance (Hunt et al., 2020).
Whilst recent reviews have highlighted several mechanisms of NTHi that favor epithelial cell adherence and biofilm formation in the lower airways (Short et al., 2021), there remains a gap in the research pertaining to NTHi biofilm development in the context of the COPD lung of which there is limited direct evidence (Ahearn et al., 2017). Some of the major limits to our understanding can be attributed to current methods of biofilm detection, which include isolation of NTHi from sputum and BAL samples and culture under nutrient-rich conditions in-vitro or non-lung in-vivo models.
Recently, PCR-based direct gene expression studies have revealed associations between adhesin expression and in-vitro biofilm formation, providing the first set of NTHi biofilm gene biomarkers (Nishi et al., 2006). However, this sub-set of genes is limited as thus far a gene expression profile using a comprehensive array of biofilm genes in NTHi clinical lung-derived samples has not been completed. Moreover, current imaging methods of ex-vivo biofilms are limited to transmission electron microscopy (Starner et al., 2006). Some studies report poor evidence of biofilm formation and in one cohort of COPD patients only 10% of NTHi isolates formed biofilm whilst 80% of these were classified as weak biofilms (Martinez-Resendez et al., 2016). Similarly, in a cohort of pulmonary sarcoidosis patients, another airways disease characterised partly by inflammation, there was a reduction in biofilm-forming Haemophilus spp. isolates in disease compared to health (Kosikowska et al., 2016) using culture methods.
Another study provided evidence for the existence of NTHi biofilm in the human respiratory tract as well as host responses to the biofilm in a cohort of COPD patients (Murphy et al., 2005). NTHi strains isolated from the sputum of six COPD patients grew biofilms expressing significantly more abundant pdgx compared to planktonic cultures and were shown to elicit an immune response in 44.4% of a further 18 COPD patients through detection of pdgx antibodies by ELISA (Murphy et al., 2005). This increased association of pdgx expression with biofilm formation is likely due to the protective properties against reactive oxygen species and survival within NETs (Juneau et al., 2015) which may serve as a biomarker for NTHi biofilm infection.
Overall, this evidence points to the existence of NTHi biofilms in infections across airways diseases. However, at the current time explicit evidence is limited in COPD. Further understanding the role of NTHi biofilms in COPD may elucidate novel, specific, therapeutic targets that may improve the disease management of patients where otherwise COPD maintenance drugs and antibiotics are ineffective in the long term.
The current literature provides strong genetic and phenotypic evidence that NTHi are well-adapted to have the capacity to colonise the human lung and form biofilms on bronchial epithelia with impaired mucociliary clearance, characteristic of COPD amongst other diseases. NTHi isolates are highly heterogeneous and further express an arsenal of strategies that confer immune system evasion, antimicrobial tolerance, persistence and chronic infection, that make it a particularly challenging pathogen to clinically treat. Considering the role of NTHi infections in the context of biofilms and their physical and chemical properties may explain the differences between patient cohorts, and why airways diseases are characterised by chronic, recurring lung infections. However, the presence of NTHi is not synonymous with AECOPD, so perhaps it is not necessarily the presence of a particular pathogen but the phase in which bacteria are present, biofilm or planktonic. Conversely, whilst some studies report NTHi as a non-significant bacteria in AECOPD or hospitalisation, this does not rule out the potential historical role of NTHi colonisation in the airways and host-pathogen interactions leading up to the present time of study. It is also important to consider that NTHi may have commensal benefits for the host’s immune system. For example, intracellular NTHi infection of airway epithelia protects against respiratory syncytial virus (RSV) (Hartwig et al., 2016) through stimulation of immune effectors. Conversely, NTHi can take advantage of compromised immune systems following viral infection (Molyneaux et al., 2013).
Understanding COPD and NTHi infections in the context of biofilms has several clinical implications. Firstly, the potential to improve diagnosis; currently, physical symptoms of chronic bronchitis and emphysema are used in the clinical diagnosis of COPD, despite recent microbiome associations. Whilst bacteria are understood to have a significant impact on the progression of this disease, dysbiosis of the lung microbiome is not currently used but could be a biomarker for predicting early onset COPD. Secondly, improving AECOPD treatment; NTHi isolates are highly heterogeneous in terms of biofilm-forming, antimicrobial tolerance and immune system stimulation or evasion mechanisms so a ‘one-treatment-fits-all’ approach may not be appropriate. Understanding the underlying biofilm mechanisms may reveal several targets to disrupt biofilm integrity, harmful host-pathogen interactions and increase antimicrobial susceptibility. Recently, nitric oxide donors have been successful in triggering biofilm dispersal from β-lactam resistant cystic fibrosis P. aeruginosa isolates and improving the efficacy of antibiotics (Cai and Webb, 2020; Soren et al., 2020). Mucolytic drugs are currently widely prescribed by clinicians as an adjunctive therapy for COPD (Papi et al., 2020) and include erdosteine that has been shown to increase the efficacy of antibiotics against chronic respiratory infections (Dal Negro et al., 2018). The adhesins OMP P5 and TfP that facilitate initial attachment have been targeted for vaccine development to prevent biofilm formation, which was successful in evoking a protective immune response to NTHi in the OM model (Novotny and Bakaletz, 2013). The literature demonstrates that targeting biofilm components are an effective way to reduce biofilm formation and increase antimicrobial susceptibility in-vitro, including antibody-mediated DNABII degradation (Goodman et al., 2011) and DNase I mediated eDNA degradation (Izano et al., 2009) with a plethora of surface modifications and tolerance mechanisms identified as future targets for development. Being able to profile the genotype or transcriptome of clinical isolates for biofilm-genes may reveal strain-specific susceptibilities or drug-targets where airways infections could be treated with greater efficacy using a precision medicine approach.
Despite recent advancements, there is a lack of evidence that NTHi forms biofilms within the human COPD lung. The majority of the research investigating this biofilm concept involves isolated strains cultured under nutrient-rich conditions in-vitro or in OM or bronchial epithelial cell culture models – not within the hostile lung environment with a cascade of immunological responses and potentially nutrient-deprived and anaerobic microenvironments. To our knowledge, there are no current studies that have attempted to detect NTHi biofilm within sputum, BALF samples or tissue biopsies from COPD patients ex-vivo using PCR detection of biofilm-associated genes or immunofluorescence imaging techniques. This would provide direct evidence of biofilms existing within the COPD lung.
Additionally, microbiome studies have a great deal of disparity reporting the core lung microbiome. Different studies take sputum and BAL samples which are different lung niches and may account for some of this disparity in microbiota shifts and diversity changes during AECOPD. Disparity can also be attributed to having no standard methodology, including sampling and sequencing inconsistencies, for example using different 16S hypervariable regions or using Illumina or Pyrosequencing platforms, that may yield different results when quantifying the abundance of bacteria taxa.
Future research should be directed towards clinical COPD NTHi isolates and model development that better represent the lung microenvironment and polymicrobial communities, to understand the behaviour of NTHi during chronic airways infections. Developing a comprehensive list of ‘biotypes’ for NTHi strains by profiling biofilm-gene expression could reveal patient-strain specific biofilm mechanisms, susceptibilities and potential therapeutic targets. Understanding the complex interspecific and host-pathogen interactions and microbiota shifts over time would allow us to model disease progression and design effective, precision-medicine treatment strategies to improve the prognosis of COPD patients and slow down the progression of COPD by targeting the microbial cause.
Understanding the behaviour of NTHi as a biofilm-producing pathogen reveals a growing number of mechanisms that could be potentially targeted by therapeutics. Research in model systems has already shown that disruption of key biofilm systems, including LuxS quorum sensing, the eDNA – DNABII binding complex, expression of key adhesins and cell surface modifications, alters the NTHi biofilm phenotype and increases antimicrobial susceptibility (Giufre et al., 2008; Starner et al., 2008; Armbruster et al., 2009; Jones et al., 2013; Cavaliere et al., 2014). However, the challenge now is to alter these processes within the human lung. Increasing our understanding of how NTHi coordinates the expression of genes that control these biofilm- and survival-promoting processes is essential. Recently, the role of bacterial exosomes or outer membrane vesicles (OMVs) have been implicated in NTHi biofilms in the air-liquid-interface epithelium model (Ren et al., 2012). Gram negative bacteria, including NTHi, produce spherically bilayered OMVs of approximately 100-300nm in size by a process of outward blebbing from the bacteria outer membrane (Jan, 2017) and have multifunctional roles including supporting the biofilm lifestyle (Gunn et al., 2016). Such proposed functions include the shuttling of quorum-sensing molecules (Ren et al., 2012) as well as enrichment with key outer membrane proteins (OMPs) and LOS that have previously been associated with biofilm development (Winter and Barenkamp, 2017) as well as NET recruitment (Sharpe et al., 2011). NTHi OMVs also traffic cargo destined for the EPS matrix (Gunn et al., 2016), a hallmark feature of biofilms that confers physical and chemical survival against the immune system and antimicrobial drugs. As analogous structures of eukaryotic extracellular vesicles that deliver a cargo of DNA, RNA and miRNA (Rodrigues et al., 2018; Guiot et al., 2019), it is possible that NTHi biofilm-derived OMVs represent a novel target for the disruption of gene expression pathways controlling biofilm development and contributing to disease.
This review has highlighted several key questions that need exploring in the field. Firstly, how does the behaviour of biofilm-phase NTHi affect progression of COPD? Specifically, there is a need to conduct research into lung-derived NTHi biofilms and develop valid airways models. Furthering our understanding of host-biofilm interactions, such as the proinflammatory cascade, could lead to slowing the progression of COPD. Next, the NTHi biofilm itself. Are the biofilm-specific strategies of NTHi the reason why disease management drugs and antimicrobials are largely ineffective in the long term? Whilst current treatment strategies do help to alleviate AECOPD and slow the progression of COPD, infections remain recurrent throughout the chronic disease leading to increased hospitalisation and morbidity (Wedzicha and Wilkinson, 2006; Wilkinson T, 2006; Kong and Wilkinson, 2020). This leads us to hypothesise that NTHi biofilms present potential, novel therapeutic targets (Wilkinson et al., 2019). For example, there is potential for biofilm development to be heavily impaired by targeting the aforementioned NTHi biofilm strategies including the eDNA-DNABII binding complex, adhesins, outer membrane proteins, high molecular weight proteins, LOS modifications and biofilm-promoting gene expression pathways.
NTHi is a major aetiological commensal-turned-pathogen implicated in COPD progression and AECOPD across several recent large-cohort patient COPD studies. However, reports differ on the prevalence of this ubiquitous organism across healthy and non-healthy individuals, the reported significance of this pathogen in the onset or exacerbation of disease, as well as treatment efficacy. NTHi are well adapted to colonisation of the respiratory epithelium and express many genes that promote the biofilm lifestyle, as well as proteins that confer immune system evasion, antibiotic tolerance, and metabolomic changes that drive survival, persistence and chronic, recurrent infections. Furthering our understanding of the mechanisms of NTHi colonisation in chronic lung infections in COPD patients in the context of biofilms is now essential and may help to explain these vast differences. Whilst several studies report that clinical NTHi isolates can express the key hallmarks of a biofilm, these are often in the context of in-vivo OM or in-vitro airways epithelia models under nutrient-rich conditions that poorly represent the human lung. Further research is required to characterise the biofilm-forming capacity of NTHi and identify the COPD lung host-pathogen interactions and anti-biofilm therapeutic targets, which could have a significant impact on the diagnosis and treatment of patients living with chronic airways diseases.
JW, conceptualization, investigation, literature searching, analysis, project administration, writing original draft, reviewing and editing, approval of final draft. KS, supervision, conceptualization, reviewing and editing, approval of final draft. CS, supervision, conceptualization, reviewing and editing, approval of final draft. AW, supervision, reviewing and editing, approval of final draft. TW, supervision, conceptualization, reviewing and editing, approval of final draft. All authors contributed to the article and approved the submitted version.
This work was undertaken using a BBSRC iCASE PhD studentship (BB/T508135/1) awarded for JW’s doctoral studies.
KS reports grants from AstraZeneca, outside the conduct of the study; TW reports grants and personal fees from AstraZeneca, outside the conduct of the study; personal fees and other from MMH, grants and personal fees from GSK, grants and personal fees from AZ, personal fees from BI, grants and personal fees from Synairgen, outside the submitted work.
The remaining authors declare that the research was conducted in the absence of any commercial or financial relationships that could be construed as a potential conflict of interest.
All claims expressed in this article are solely those of the authors and do not necessarily represent those of their affiliated organizations, or those of the publisher, the editors and the reviewers. Any product that may be evaluated in this article, or claim that may be made by its manufacturer, is not guaranteed or endorsed by the publisher.
Ahearn, C. P., Gallo, M. C., Murphy, T. F. (2017). Insights on Persistent Airway Infection by Non-Typeable Haemophilus Influenzae in Chronic Obstructive Pulmonary Disease. Pathog. Dis. 75 (4), 1–18. doi: 10.1093/femspd/ftx042
Armbruster, C. E., Hong, W., Pang, B., Dew, K. E., Juneau, R. A., Byrd, M. S., et al (2009). LuxS Promotes Biofilm Maturation and Persistence of Nontypeable Haemophilus Influenzae In Vivo Via Modulation of Lipooligosaccharides on the Bacterial Surface. Infect. Immun. 77 (9), 4081–4091. doi: 10.1128/IAI.00320-09
Armbruster, C. E., Pang, B., Murrah, K., Juneau, R. A., Perez, A. C., Weimer, K. E., et al (2011). RbsB (NTHI_0632) Mediates Quorum Signal Uptake in Nontypeable Haemophilus Influenzae Strain 86-028NP. Mol. Microbiol. 82 (4), 836–850. doi: 10.1111/j.1365-2958.2011.07831.x
Bair, K. L., Campagnari, A. A. (2019). Moraxella Catarrhalis Promotes Stable Polymicrobial Biofilms With the Major Otopathogens. Front. Microbiol. 10, 3006. doi: 10.3389/fmicb.2019.03006
Beck, J. M., Young, V. B., Huffnagle, G. B. (2012). The Microbiome of the Lung. Transl. Res. 160 (4), 258–266. doi: 10.1016/j.trsl.2012.02.005
Bjarnsholt, T. (2013). The Role of Bacterial Biofi Lms in Chronic Infections. APMIS 121, 51. doi: 10.1111/apm.12099
Brill, S. E., Law, M., El-Emir, E., Allinson, J. P., James, P., Maddox, V., et al (2015). Effects of Different Antibiotic Classes on Airway Bacteria in Stable COPD Using Culture and Molecular Techniques: A Randomised Controlled Trial. Thorax 70 (10), 930–938. doi: 10.1136/thoraxjnl-2015-207194
Brockman, K. L., Azzari, P. N., Branstool, M. T., Atack, J. M., Schulz, B. L., Jen, F. E., et al. (2018). Epigenetic Regulation Alters Biofilm Architecture and Composition in Multiple Clinical Isolates of Nontypeable Haemophilus influenzae. mBio 9 (5), 1–15. doi: 10.1128/mBio.01682-18
Cabrera-Rubio, R., Garcia-Nunez, M., Seto, L., Anto, J. M., Moya, A., Monso, E., et al (2012). Microbiome Diversity in the Bronchial Tracts of Patients With Chronic Obstructive Pulmonary Disease. J. Clin. Microbiol. 50 (11), 3562–3568. doi: 10.1128/JCM.00767-12
Cai, Y. M., Webb, J. S. (2020). Optimization of Nitric Oxide Donors for Investigating Biofilm Dispersal Response in Pseudomonas Aeruginosa Clinical Isolates. Appl. Microbiol. Biotechnol. 104 (20), 8859–8869. doi: 10.1007/s00253-020-10859-7
Cao, Y., Xuan, S., Wu, Y., Yao, X. (2019). Effects of Long-Term Macrolide Therapy at Low Doses in Stable COPD. Int. J. Chron. Obstruct. Pulmon. Dis. 14, 1289–1298. doi: 10.2147/COPD.S205075
Cavaliere, R., Ball, J. L., Turnbull, L., Whitchurch, C. B. (2014). The Biofilm Matrix Destabilizers, EDTA and DNaseI, Enhance the Susceptibility of Nontypeable Hemophilus Influenzae Biofilms to Treatment With Ampicillin and Ciprofloxacin. Microbiologyopen 3 (4), 557–567. doi: 10.1002/mbo3.187
Cerquetti, M., Giufre, M. (2016). Why We Need a Vaccine for Non-Typeable Haemophilus Influenzae. Hum. Vaccin. Immunother. 12 (9), 2357–2361. doi: 10.1080/21645515.2016.1174354
Chakravorty, S., Helb, D., Burday, M., Connell, N., Alland, D. (2007). A Detailed Analysis of 16S Ribosomal RNA Gene Segments for the Diagnosis of Pathogenic Bacteria. J. Microbiol. Methods 69 (2), 330–339. doi: 10.1016/j.mimet.2007.02.005
Cho, C., Chande, A., Gakhar, L., Bakaletz, L. O., Jurcisek, J. A., Ketterer, M., et al (2015). Role of the Nuclease of Nontypeable Haemophilus Influenzae in Dispersal of Organisms From Biofilms. Infect. Immun. 83 (3), 950–957. doi: 10.1128/IAI.02601-14
Clarridge, J. E., 3rd. (2004). Impact of 16S rRNA Gene Sequence Analysis for Identification of Bacteria on Clinical Microbiology and Infectious Diseases. Clin. Microbiol. Rev. 17 (4), 840–862. table of contents. doi: 10.1128/CMR.17.4.840-862.2004
Clementi, C. F., Murphy, T. F. (2011). Non-Typeable Haemophilus Influenzae Invasion and Persistence in the Human Respiratory Tract. Front. Cell Infect. Microbiol. 1, 1. doi: 10.3389/fcimb.2011.00001
Contoli, M., Pauletti, A., Rossi, M. R., Spanevello, A., Casolari, P., Marcellini, A., et al (2017). Long-Term Effects of Inhaled Corticosteroids on Sputum Bacterial and Viral Loads in COPD. Eur. Respir. J. 50 (4), 1–10. doi: 10.1183/13993003.00451-2017
Costerton, J. W., Lewandowski, Z. (1995). Microbial Biofilms. Annu. Rev. Microbiol. 49, 34. doi: 10.1146/annurev.mi.49.100195.003431
Cui, Y., Luo, L., Li, C., Chen, P., Chen, Y. (2018). Long-Term Macrolide Treatment for the Prevention of Acute Exacerbations in COPD: A Systematic Review and Meta-Analysis. Int. J. Chron. Obstruct. Pulmon. Dis. 13, 3813–3829. doi: 10.2147/COPD.S181246
Dal Negro, R., Pozzi, E., Cella, S. G. (2018). Erdosteine: Drug Exhibiting Polypharmacy for the Treatment of Respiratory Diseases. Pulm. Pharmacol. Ther. 53, 80–85. doi: 10.1016/j.pupt.2018.10.005
Dicker, A. J., Crichton, M. L., Pumphrey, E. G., Cassidy, A. J., Suarez-Cuartin, G., Sibila, O., et al (2018). Neutrophil Extracellular Traps are Associated With Disease Severity and Microbiota Diversity in Patients With Chronic Obstructive Pulmonary Disease. J. Allergy Clin. Immunol. 141 (1), 117–127. doi: 10.1016/j.jaci.2017.04.022
Djamin, R. S., Talman, S., Schrauwen, E. J. A., von Wintersdorff, C. J. H., Wolffs, P. F., Savelkoul, P. H. M., et al (2020). Prevalence and Abundance of Selected Genes Conferring Macrolide Resistance Genes in COPD Patients During Maintenance Treatment With Azithromycin. Antimicrob. Resist. Infect. Control 9 (1), 116. doi: 10.1186/s13756-020-00783-w
Domenech, M., Pedrero-Vega, E., Prieto, A., Garcia, E. (2016). Evidence of the Presence of Nucleic Acids and Beta-Glucan in the Matrix of Non-Typeable Haemophilus Influenzae In Vitro Biofilms. Sci. Rep. 6, 36424. doi: 10.1038/srep36424
Einarsson, G. G., Comer, D. M., McIlreavey, L., Parkhill, J., Ennis, M., Tunney, M. M., et al (2016). Community Dynamics and the Lower Airway Microbiota in Stable Chronic Obstructive Pulmonary Disease, Smokers and Healthy non-Smokers. Thorax 71 (9), 795–803. doi: 10.1136/thoraxjnl-2015-207235
Erb-Downward, J. R., Thompson, D. L., Han, M. K., Freeman, C. M., McCloskey, L., Schmidt, L. A., et al (2011). Analysis of the Lung Microbiome in the “Healthy” Smoker and in COPD. PLoS One 6 (2), e16384. doi: 10.1371/journal.pone.0016384
Foxwell, A. R., Kyd, J., Cripps, A. W. (1998). Nontypeable Haemophilus Influenzae: Pathogenesis and Prevention. Microbiol. Mol. Biol. Rev. 62 (2), 15. doi: 10.1128/MMBR.62.2.294-308.1998
Giufre, M., Carattoli, A., Cardines, R., Mastrantonio, P., Cerquetti, M. (2008). Variation in Expression of HMW1 and HMW2 Adhesins in Invasive Nontypeable Haemophilus Influenzae Isolates. BMC Microbiol. 8, 83. doi: 10.1186/1471-2180-8-83
Goodman, S. D., Obergfell, K. P., Jurcisek, J. A., Novotny, L. A., Downey, J. S., Ayala, E. A., et al (2011). Biofilms can be Dispersed by Focusing the Immune System on a Common Family of Bacterial Nucleoid-Associated Proteins. Mucosal Immunol. 4 (6), 625–637. doi: 10.1038/mi.2011.27
Greiner, L. L., Watanabe, H., Phillips, N. J., Shao, J., Morgan, A., Zaleski, A., et al (2004). Nontypeable Haemophilus Influenzae Strain 2019 Produces a Biofilm Containing N-Acetylneuraminic Acid That may Mimic Sialylated O-Linked Glycans. Infect. Immun. 72 (7), 4249–4260. doi: 10.1128/IAI.72.7.4249-4260.2004
Guiot, J., Struman, I., Louis, E., Louis, R., Malaise, M., Njock, M. S. (2019). Exosomal miRNAs in Lung Diseases: From Biologic Function to Therapeutic Targets. J. Clin. Med. 8 (9), 1–17. doi: 10.3390/jcm8091345
Gunn, J. S., Bakaletz, L. O., Wozniak, D. J. (2016). What’s on the Outside Matters: The Role of the Extracellular Polymeric Substance of Gram-Negative Biofilms in Evading Host Immunity and as a Target for Therapeutic Intervention. J. Biol. Chem. 291 (24), 12538–12546. doi: 10.1074/jbc.R115.707547
Hartwig, S. M., Ketterer, M., Apicella, M. A., Varga, S. M. (2016). Non-Typeable Haemophilus Influenzae Protects Human Airway Epithelial Cells From a Subsequent Respiratory Syncytial Virus Challenge. Virology 498, 128–135. doi: 10.1016/j.virol.2016.08.020
Herath, S. C., Normansell, R., Maisey, S., Poole, P. (2018). Prophylactic Antibiotic Therapy for Chronic Obstructive Pulmonary Disease (COPD). Cochrane Database Syst. Rev. 10, CD009764. doi: 10.1002/14651858.CD009764.pub3
Hiergeist, A., Glasner, J., Reischl, U., Gessner, A. (2015). Analyses of Intestinal Microbiota: Culture Versus Sequencing. ILAR J. 56 (2), 228–240. doi: 10.1093/ilar/ilv017
Hilty, M., Burke, C., Pedro, H., Cardenas, P., Bush, A., Bossley, C., et al (2010). Disordered Microbial Communities in Asthmatic Airways. PLoS One 5 (1), e8578. doi: 10.1371/journal.pone.0008578
Hong, W., Juneau, R. A., Pang, B., Swords, W. E. (2009). Survival of Bacterial Biofilms Within Neutrophil Extracellular Traps Promotes Nontypeable Haemophilus Influenzae Persistence in the Chinchilla Model for Otitis Media. J. Innate Immun. 1 (3), 215–224. doi: 10.1159/000205937
Hunt, B. C., Stanford, D., Xu, X., Li, J., Gaggar, A., Rowe, S. M., et al (2020). Haemophilus Influenzae Persists in Biofilm Communities in a Smoke-Exposed Ferret Model of COPD. ERJ Open Res. 6 (3), 1–12. doi: 10.1183/23120541.00200-2020
Izano, E. A., Shah, S. M., Kaplan, J. B. (2009). Intercellular Adhesion and Biocide Resistance in Nontypeable Haemophilus Influenzae Biofilms. Microb. Pathog. 46 (4), 207–213. doi: 10.1016/j.micpath.2009.01.004
Jacobs, D. M., Ochs-Balcom, H. M., Zhao, J., Murphy, T. F., Sethi, S. (2018). Lower Airway Bacterial Colonization Patterns and Species-Specific Interactions in Chronic Obstructive Pulmonary Disease. J. Clin. Microbiol. 56 (10), 1–12. doi: 10.1128/JCM.00330-18
Jan, A. T. (2017). Outer Membrane Vesicles (OMVs) of Gram-Negative Bacteria: A Perspective Update. Front. Microbiol. 8, 1–11. doi: 10.3389/fmicb.2017.01053
Jones, E. A., McGillivary, G., Bakaletz, L. O. (2013). Extracellular DNA Within a Nontypeable Haemophilus Influenzae-Induced Biofilm Binds Human Beta Defensin-3 and Reduces its Antimicrobial Activity. J. Innate Immun. 5 (1), 24–38. doi: 10.1159/000339961
Jubinville, E., Veillette, M., Milot, J., Maltais, F., Comeau, A. M., Levesque, R. C., et al (2018). Exacerbation Induces a Microbiota Shift in Sputa of COPD Patients. PLoS One 13 (3), e0194355. doi: 10.1371/journal.pone.0194355
Juneau, R. A., Pang, B., Armbruster, C. E., Murrah, K. A., Perez, A. C., Swords, W. E. (2015). Peroxiredoxin-Glutaredoxin and Catalase Promote Resistance of Nontypeable Haemophilus Influenzae 86-028NP to Oxidants and Survival Within Neutrophil Extracellular Traps. Infect. Immun. 83 (1), 239–246. doi: 10.1128/IAI.02390-14
Jurcisek, J. A., Bakaletz, L. O. (2007). Biofilms Formed by Nontypeable Haemophilus Influenzae In Vivo Contain Both Double-Stranded DNA and Type IV Pilin Protein. J. Bacteriol. 189 (10), 3868–3875. doi: 10.1128/JB.01935-06
Jurcisek, J. A., Brockman, K. L., Novotny, L. A., Goodman, S. D., Bakaletz, L. O. (2017). Nontypeable Haemophilus Influenzae Releases DNA and DNABII Proteins Via a T4SS-Like Complex and ComE of the Type IV Pilus Machinery. Proc. Natl. Acad. Sci. U. S. A. 114 (32), E6632–E6E41. doi: 10.1073/pnas.1705508114
Kelly, C., Chalmers, J. D., Crossingham, I., Relph, N., Felix, L. M., Evans, D. J., et al (2018). Macrolide Antibiotics for Bronchiectasis. Cochrane Database Syst. Rev. 3, CD012406. doi: 10.1002/14651858.CD012406.pub2
Khanolkar, R. A., Clark, S. T., Wang, P. W., Hwang, D. M., Yau, Y. C. W., Waters, V. J., et al (2020). Ecological Succession of Polymicrobial Communities in the Cystic Fibrosis Airways. mSystems 5 (6), 16. doi: 10.1128/mSystems.00809-20
King, P. T., Sharma, R. (2015). The Lung Immune Response to Nontypeable Haemophilus Influenzae (Lung Immunity to NTHi). J. Immunol. Res. 2015, 706376. doi: 10.1155/2015/706376
Kong, C. W., Wilkinson, T. M. A. (2020). Predicting and Preventing Hospital Readmission for Exacerbations of COPD. ERJ Open Res. 6 (2), 1–13. doi: 10.1183/23120541.00325-2019
Kosikowska, U., Rybojad, P., Stepien-Pysniak, D., Zbikowska, A., Malm, A. (2016). Changes in the Prevalence and Biofilm Formation of Haemophilus Influenzae and Haemophilus Parainfluenzae From the Respiratory Microbiota of Patients With Sarcoidosis. BMC Infect. Dis. 16 (1), 449. doi: 10.1186/s12879-016-1793-7
Krishnamurthy, A., Kyd, J. (2014). The Roles of Epithelial Cell Contact, Respiratory Bacterial Interactions and Phosphorylcholine in Promoting Biofilm Formation by Streptococcus Pneumoniae and Nontypeable Haemophilus Influenzae. Microbes Infect. 16 (8), 640–647. doi: 10.1016/j.micinf.2014.06.008
Kubiet, M., Ramphal, R. (1995). Adhesion of Nontypeable Haemophilus Influenzae Biofilms From Blood and Sputum to Human Tracheobronchial Mucins and Lactoferrin. Am. Soc. Microbiol. 63 (3), 4. doi: 10.1128/iai.63.3.899-902.1995
Kyd, J. M., Krishnamurthy, A., Kidd, S. (2016). “Interactions and Mechanisms of Respiratory Tract Biofilms Involving Streptococcus Pneumoniae and Nontypeable Haemophilus Influenzae,” in Microbial Biofilms - Importance and Applications https://www.intechopen.com/chapters/50702.
Langereis, J. D., Hermans, P. W. (2013). Novel Concepts in Nontypeable Haemophilus Influenzae Biofilm Formation. FEMS Microbiol. Lett. 346 (2), 81–89. doi: 10.1111/1574-6968.12203
MacNee, W. (2006). ABC of Chronic Obstructive Pulmonary Disease Pathology, Pathogenesis, and Pathophysiology. BMJ 332, 3. doi: 10.1136/bmj.332.7551.1202
Martinez-Resendez, M. F., Gonzalez-Chavez, J. M., Garza-Gonzalez, E., Castro-Fuentes, L. N., Gutierrez-Ferman, J. L., Echaniz-Aviles, G., et al (2016). Non-Typeable Haemophilus Influenzae Biofilm Production and Severity in Lower Respiratory Tract Infections in a Tertiary Hospital in Mexico. J. Med. Microbiol. 65 (12), 1385–1391. doi: 10.1099/jmm.0.000369
Martiny, A. C. (2019). High Proportions of Bacteria are Culturable Across Major Biomes. ISME J. 13 (8), 2125–2128. doi: 10.1038/s41396-019-0410-3
Mayhew, D., Devos, N., Lambert, C., Brown, J. R., Clarke, S. C., Kim, V. L., et al (2018). Longitudinal Profiling of the Lung Microbiome in the AERIS Study Demonstrates Repeatability of Bacterial and Eosinophilic COPD Exacerbations. Thorax 73 (5), 422–430. doi: 10.1136/thoraxjnl-2017-210408
McDaniel, C. T., Panmanee, W., Hassett, D. J. (2015). “An Overview of Infections in Cystic Fibrosis Airways and the Role of Environmental Conditions on Pseudomonas Aeruginosa Biofilm Formation and Viability,” in Cystic Fibrosis in the Light of New Research. Available at: https://www.intechopen.com/chapters/48663.
Mika, M., Nita, I., Morf, L., Qi, W., Beyeler, S., Bernasconi, E., et al (2018). Microbial and Host Immune Factors as Drivers of COPD. ERJ Open Res. 4 (3), 1–10, 1–10. doi: 10.1183/23120541.00015-2018
Molyneaux, P. L., Mallia, P., Cox, M. J., Footitt, J., Willis-Owen, S. A., Homola, D., et al (2013). Outgrowth of the Bacterial Airway Microbiome After Rhinovirus Exacerbation of Chronic Obstructive Pulmonary Disease. Am. J. Respir. Crit. Care Med. 188 (10), 1224–1231. doi: 10.1164/rccm.201302-0341OC
Murphy, T. F., Brauer, A. L., Grant, B. J., Sethi, S. (2005). Moraxella Catarrhalis in Chronic Obstructive Pulmonary Disease: Burden of Disease and Immune Response. Am. J. Respir. Crit. Care Med. 172 (2), 195–199. doi: 10.1164/rccm.200412-1747OC
Murphy, T. F., Kirkham, C., Sethi, S., Lesse, A. J. (2005). Expression of a Peroxiredoxin-Glutaredoxin by Haemophilus Influenzae in Biofilms and During Human Respiratory Tract Infection. FEMS Immunol. Med. Microbiol. 44 (1), 81–89. doi: 10.1016/j.femsim.2004.12.008
Nakou, A., Papaparaskevas, J., Diamantea, F., Skarmoutsou, N., Polychronopoulos, V., Tsakris, A. (2014). A Prospective Study on Bacterial and Atypical Etiology of Acute Exacerbation in Chronic Obstructive Pulmonary Disease. Future Microbiol. 9 (11), 10. doi: 10.2217/fmb.14.90
Nalca, Y., Jansch, L., Bredenbruch, F., Geffers, R., Buer, J., Haussler, S. (2006). Quorum-Sensing Antagonistic Activities of Azithromycin in Pseudomonas Aeruginosa PAO1: A Global Approach. Antimicrob. Agents Chemother. 50 (5), 1680–1688. doi: 10.1128/AAC.50.5.1680-1688.2006
Nishi, J., Imuta, N., Kamenosono, A., Tokuda, K., Tanaka, S., Manago, K. (2006). Prevalence of Adhesin Genes and Biofilm Formation Among Clinical Isolates of Nontypable Haemophilus Influenzae J-STAGE Japan Science Technology Information Aggregator, Electronic 17, 2, 8. doi: 10.5701/jjpp.17.143
Novotny, L. A., Bakaletz, L. O. (2013). “Peptide Vaccines for Otitis Media,” in Handbook of Biologically Active Peptides. 603–611. Available at: https://reader.elsevier.com/reader/sd/pii/B9780123694423500787?token=10ED78ACB7806C6D64F14D9F428DFC76E024E67DAF72DA84E6C472D8E7D9A77E76070F9809E5F5804BD4DECDE0BDF8E2&originRegion=eu-west-1&originCreation=20210722144721.
Panchabhai, T. S., Mukhopadhyay, S., Sehgal, S., Bandyopadhyay, D., Erzurum, S. C., Mehta, A. C. (2016). Plugs of the Air Passages: A Clinicopathologic Review. Chest 150 (5), 1141–1157. doi: 10.1016/j.chest.2016.07.003
Pang, B., Armbruster, C., Foster, G., Learman, B., Gandi, U., Swords, E. (2018). Autoinducer 2 (AI-2) Production by Nontypeable Haemophilus Influenzae 86-082NP Promotes Expression of a Predicted Glycosyltransferase That Is a Determinant of Biofilm Maturation, Prevention of Dispersal, and Persistence In Vivo. Infect. Immun. 86 (12), 1–16. doi: 10.1128/IAI.00506-18
Papi, A., Avdeev, S., Calverley, P. M. A., Cordeiro, C. R., Jesenak, M., Koblizek, V., et al (2020). Use of Mucolytics in COPD: A Delphi Consensus Study. Respir. Med. 175, 106190. doi: 10.1016/j.rmed.2020.106190
Post, D. M., Held, J. M., Ketterer, M. R., Phillips, N. J., Sahu, A., Apicella, M. A., et al (2014). Comparative Analyses of Proteins From Haemophilus Influenzae Biofilm and Planktonic Populations Using Metabolic Labeling and Mass Spectrometry. BMC Microbiol. 14, 329. doi: 10.1186/s12866-014-0329-9
Pragman, A. A., Berger, J. P., Williams, B. J. (2016). Understanding Persistent Bacterial Lung Infections: Clinical Implications Informed by the Biology of the Microbiota and Biofilms. Clin. Pulm. Med. 23 (2), 57–66. doi: 10.1097/CPM.0000000000000108
Pragman, A. A., Lyu, T., Baller, J. A., Gould, T. J., Kelly, R. F., Reilly, C. S., et al (2018). The Lung Tissue Microbiota of Mild and Moderate Chronic Obstructive Pulmonary Disease. Microbiome 6 (1), 7. doi: 10.1186/s40168-017-0381-4
Qiu, S., Zhong, X. (2017). Macrolides: A Promising Pharmacologic Therapy for Chronic Obstructive Pulmonary Disease. Ther. Adv. Respir. Dis. 11 (3), 147–155. doi: 10.1177/1753465816682677
Rabe, K. F., Hurd, S., Anzueto, A., Barnes, P. J., Buist, S. A., Calverley, P., et al (2007). Global Strategy for the Diagnosis, Management, and Prevention of Chronic Obstructive Pulmonary Disease: GOLD Executive Summary. Am. J. Respir. Crit. Care Med. 176 (6), 532–555. doi: 10.1164/rccm.200703-456SO
Rabe, K. F., Watz, H. (2017). Chronic Obstructive Pulmonary Disease. Lancet 389 (10082), 1931–1940. doi: 10.1016/S0140-6736(17)31222-9
Ren, D., Nelson, K. L., Uchakin, P. N., Smith, A. L., Gu, X. X., Daines, D. A. (2012). Characterization of Extended Co-Culture of non-Typeable Haemophilus Influenzae With Primary Human Respiratory Tissues. Exp. Biol. Med. (Maywood) 237 (5), 540–547. doi: 10.1258/ebm.2012.011377
Rodrigues, M., Fan, J., Lyon, C., Wan, M., Hu, Y. (2018). Role of Extracellular Vesicles in Viral and Bacterial Infections: Pathogenesis, Diagnostics, and Therapeutics. Theranostics 8 (10), 2709–2721. doi: 10.7150/thno.20576
Rotta Detto Loria, J., Rohmann, K., Droemann, D., Kujath, P., Rupp, J., Goldmann, T., et al (2013). Nontypeable Haemophilus Influenzae Infection Upregulates the NLRP3 Inflammasome and Leads to Caspase-1-Dependent Secretion of Interleukin-1beta - A Possible Pathway of Exacerbations in COPD. PLoS One 8 (6), e66818. doi: 10.1371/journal.pone.0066818
Santocki, M., Kolaczkowska, E. (2020). On Neutrophil Extracellular Trap (NET) Removal: What We Know Thus Far and Why So Little. Cells 9 (9), 1–25. doi: 10.3390/cells9092079
Segal, L. N., Rom, W. N., Weiden, M. D. (2014). Lung Microbiome for Clinicians. New Discoveries About Bugs in Healthy and Diseased Lungs. Ann. Am. Thorac. Soc. 11 (1), 108–116. doi: 10.1513/AnnalsATS.201310-339FR
Sharpe, S. W., Kuehn, M. J., Mason, K. M. (2011). Elicitation of Epithelial Cell-Derived Immune Effectors by Outer Membrane Vesicles of Nontypeable Haemophilus Influenzae. Infect. Immun. 79 (11), 4361–4369. doi: 10.1128/IAI.05332-11
Short, B., Carson, S., Devlin, A. C., Reihill, J. A., Crilly, A., MacKay, W., et al (2021). Non-Typeable Haemophilus Influenzae Chronic Colonization in Chronic Obstructive Pulmonary Disease (COPD). Crit. Rev. Microbiol. 47 (2), 192–205. doi: 10.1080/1040841X.2020.1863330
Slack, M. P. E. (2017). The Evidence for non-Typeable Haemophilus Influenzae as a Causative Agent of Childhood Pneumonia. Pneumonia (Nathan) 9, 9. doi: 10.1186/s41479-017-0033-2
Slinger, R., Chan, F., Ferris, W., Yeung, S. W., St Denis, M., Gaboury, I., et al (2006). Multiple Combination Antibiotic Susceptibility Testing of Nontypeable Haemophilus Influenzae Biofilms. Diagn. Microbiol. Infect. Dis. 56 (3), 247–253. doi: 10.1016/j.diagmicrobio.2006.04.012
Smith, D., Du Rand, I., Addy, C. L., Collyns, T., Hart, S. P., Mitchelmore, P. J., et al (2020). British Thoracic Society Guideline for the Use of Long-Term Macrolides in Adults With Respiratory Disease. Thorax 75 (5), 370–404. doi: 10.1136/thoraxjnl-2019-213929
Soren, O., Rineh, A., Silva, D. G., Cai, Y., Howlin, R. P., Allan, R. N., et al (2020). Cephalosporin Nitric Oxide-Donor Prodrug DEA-C3D Disperses Biofilms Formed by Clinical Cystic Fibrosis Isolates of Pseudomonas Aeruginosa. J. Antimicrob. Chemother. 75 (1), 117–125. doi: 10.1093/jac/dkz378
Southern, K. W., Barker, P. M. (2004). Azithromycin for Cystic Fibrosis. Eur. Respir. J. 24 (5), 834–838. doi: 10.1183/09031936.04.00084304
Sriram, K. B., Cox, A. J., Clancy, R. L., Slack, M. P. E., Cripps, A. W. (2018). Nontypeable Haemophilus Influenzae and Chronic Obstructive Pulmonary Disease: A Review for Clinicians. Crit. Rev. Microbiol. 44 (2), 125–142. doi: 10.1080/1040841X.2017.1329274
Staples, K. J., Taylor, S., Thomas, S., Leung, S., Cox, K., Pascal, T. G., et al (2016). Relationships Between Mucosal Antibodies, Non-Typeable Haemophilus Influenzae (NTHi) Infection and Airway Inflammation in COPD. PLoS One 11 (11), e0167250. doi: 10.1371/journal.pone.0167250
Starner, T. D., Shrout, J. D., Parsek, M. R., Appelbaum, P. C., Kim, G. (2008). Subinhibitory Concentrations of Azithromycin Decrease Nontypeable Haemophilus Influenzae Biofilm Formation and Diminish Established Biofilms. Antimicrob. Agents Chemother. 52 (1), 137–145. doi: 10.1128/AAC.00607-07
Starner, T. D., Zhang, N., Kim, G., Apicella, M. A., McCray, P. B., Jr. (2006). Haemophilus Influenzae Forms Biofilms on Airway Epithelia: Implications in Cystic Fibrosis. Am. J. Respir. Crit. Care Med. 174 (2), 213–220. doi: 10.1164/rccm.200509-1459OC
Su, Y. C., Jalalvand, F., Thegerstrom, J., Riesbeck, K. (2018). The Interplay Between Immune Response and Bacterial Infection in COPD: Focus Upon Non-Typeable Haemophilus Influenzae. Front. Immunol. 9, 2530. doi: 10.3389/fimmu.2018.02530
Swords, W. E., Moore, M. L., Godzicki, L., Bukofzer, G., Mitten, M. J., VonCannon, J. (2004). Sialylation of Lipooligosaccharides Promotes Biofilm Formation by Nontypeable Haemophilus Influenzae. Infect. Immun. 72 (1), 106–113. doi: 10.1128/IAI.72.1.106-113.2004
Sze, M. A., Dimitriu, P. A., Hayashi, S., Elliott, W. M., McDonough, J. E., Gosselink, J. V., et al (2012). The Lung Tissue Microbiome in Chronic Obstructive Pulmonary Disease. Am. J. Respir. Crit. Care Med. 185 (10), 1073–1080. doi: 10.1164/rccm.201111-2075OC
Tchoupa, A. K., Lichtenegger, S., Reidl, J., Hauck, C. R. (2015). Outer Membrane Protein P1 Is the CEACAM-Binding Adhesin of Haemophilus Influenzae. Mol. Microbiol. 98 (3), 440–455. doi: 10.1111/mmi.13134
Tikhomirova, A., Kidd, S. P. (2013). Haemophilus Influenzae and Streptococcus Pneumoniae: Living Together in a Biofilm. Pathog. Dis. 69 (2), 114–126. doi: 10.1111/2049-632X.12073
Toraldo, D. M., Conte, L. (2019). Influence of the Lung Microbiota Dysbiosis in Chronic Obstructive Pulmonary Disease Exacerbations: The Controversial Use of Corticosteroid and Antibiotic Treatments and the Role of Eosinophils as a Disease Marker. J. Clin. Med. Res. 11 (10), 667–675. doi: 10.14740/jocmr3875
Tunney, M. M., Einarsson, G. G., Wei, L., Drain, M., Klem, E. R., Cardwell, C., et al (2013). Lung Microbiota and Bacterial Abundance in Patients With Bronchiectasis When Clinically Stable and During Exacerbation. Am. J. Respir. Crit. Care Med. 187 (10), 1118–1126. doi: 10.1164/rccm.201210-1937OC
Unal, C. M., Singh, B., Fleury, C., Singh, K., Chavez de Paz, L., Svensater, G., et al (2012). QseC Controls Biofilm Formation of Non-Typeable Haemophilus Influenzae in Addition to an AI-2-Dependent Mechanism. Int. J. Med. Microbiol. 302 (6), 261–269. doi: 10.1016/j.ijmm.2012.07.013
Van Eldere, J., Slack, M. P. E., Ladhani, S., Cripps, A. W. (2014). Non-Typeable Haemophilus Influenzae, an Under-Recognised Pathogen. Lancet Infect. Dis. 14 (12), 1281–1292. doi: 10.1016/S1473-3099(14)70734-0
Velkova, S., Petris, A., Lee, D., Freestone, P., Williamson, R., Beinke, S., et al (2018). Moraxella Catarrhalis Infection of Healthy and COPD Ciliated Epithelial Cultures. Eur. Respir. J. 52, 1–18. doi: 10.1183/13993003.congress-2018.PA5305
Wang, Z., Bafadhel, M., Haldar, K., Spivak, K., Mayhew, D., Miller, B., et al (2016). Lung Microbiome Dynamics in COPD Exacerbations. Eur. Respir. J. 47 (4), 1034–1036. doi: 10.1183/13993003.01406-2015
Watson, A., Wilkinson, T. M. A. (2021). Respiratory Viral Infections in the Elderly. Ther. Adv. Respir. Dis. 15, 1753466621995050. doi: 10.1177/1753466621995050
Wedzicha, J. A., Wilkinson, T. (2006). Impact of Chronic Obstructive Pulmonary Disease Exacerbations on Patients and Payers. Proc. Am. Thorac. Soc. 3 (3), 218–221. doi: 10.1513/pats.200510-114SF
West-Barnette, S., Rockel, A., Swords, W. E. (2006). Biofilm Growth Increases Phosphorylcholine Content and Decreases Potency of Nontypeable Haemophilus Influenzae Endotoxins. Infect. Immun. 74 (3), 1828–1836. doi: 10.1128/IAI.74.3.1828-1836.2006
Wilkinson, T. M. A., Aris, E., Bourne, S., Clarke, S. C., Peeters, M., Pascal, T. G., et al (2017). A Prospective, Observational Cohort Study of the Seasonal Dynamics of Airway Pathogens in the Aetiology of Exacerbations in COPD. Thorax 72 (10), 919–927. doi: 10.1136/thoraxjnl-2016-209023
Wilkinson, T. M. A., Schembri, S., Brightling, C., Bakerly, N. D., Lewis, K., MacNee, W., et al (2019). Non-Typeable Haemophilus Influenzae Protein Vaccine in Adults With COPD: A Phase 2 Clinical Trial. Vaccine 37 (41), 6102–6111. doi: 10.1016/j.vaccine.2019.07.100
Wilkinson T, W. J. (2006). Strategies for Improving Outcomes of COPD Exacerbations. Int. J. Chron. Obstruct. Pulmon. Dis. 1, 7. doi: 10.2147/copd.2006.1.3.335
Winter, L. E., Barenkamp, S. J. (2017). Immunogenicity of Nontypeable Haemophilus Influenzae Outer Membrane Vesicles and Protective Ability in the Chinchilla Model of Otitis Media. Clin. Vaccine Immunol. 24 (10), 1–12. doi: 10.1128/CVI.00138-17
Wise, M. P., Williams, D. W., Lewis, M. A., Frost, P. J. (2010). Macrolides and Community-Acquired Pneumonia: Is Quorum Sensing the Key? Crit. Care 14 (181), 3. doi: 10.1186/cc9084
Wu, S., Baum, M. M., Kerwin, J., Guerrero, D., Webster, S., Schaudinn, C., et al (2014). Biofilm-Specific Extracellular Matrix Proteins of Nontypeable Haemophilus Influenzae. Pathog. Dis. 72 (3), 143–160. doi: 10.1111/2049-632X.12195
Wu, S., Li, X., Gunawardana, M., Maguire, K., Guerrero-Given, D., Schaudinn, C., et al (2014). Beta- Lactam Antibiotics Stimulate Biofilm Formation in Non-Typeable Haemophilus Influenzae by Up-Regulating Carbohydrate Metabolism. PLoS One 9 (7), e99204. doi: 10.1371/journal.pone.0099204
Yao, G.-Y., Ma, Y.-L., Zhang, M.-Q., Gao, Z.-C. (2013). Macrolide Therapy Decreases Chronic Obstructive Pulmonary Disease Exacerbation: A Meta-Analysis. Respiration 86 (3), 254–260. doi: 10.1159/000350828
Zakharkina, T., Heinzel, E., Koczulla, R. A., Greulich, T., Rentz, K., Pauling, J. K., et al (2013). Analysis of the Airway Microbiota of Healthy Individuals and Patients With Chronic Obstructive Pulmonary Disease by T-RFLP and Clone Sequencing. PLoS One 8 (7), e68302. doi: 10.1371/journal.pone.0068302
Zhang, J., Zhu, Z., Zuo, X., Pan, H., Gu, Y., Yuan, Y., et al (2020). The Role of NTHi Colonization and Infection in the Pathogenesis of Neutrophilic Asthma. Respir. Res. 21 (1), 170. doi: 10.1186/s12931-020-01438-5
Keywords: Non-typeable Haemophilus influenzae (NTHi), biofilms, chronic obstructive pulmonary disease (COPD), airways diseases, lung microbiome, host-pathogen interactions, antimicrobial tolerance
Citation: Weeks JR, Staples KJ, Spalluto CM, Watson A and Wilkinson TMA (2021) The Role of Non-Typeable Haemophilus influenzae Biofilms in Chronic Obstructive Pulmonary Disease. Front. Cell. Infect. Microbiol. 11:720742. doi: 10.3389/fcimb.2021.720742
Received: 04 June 2021; Accepted: 15 July 2021;
Published: 04 August 2021.
Edited by:
Dirk Lange, University of British Columbia, CanadaReviewed by:
Sara Martí, Bellvitge University Hospital, SpainCopyright © 2021 Weeks, Staples, Spalluto, Watson and Wilkinson. This is an open-access article distributed under the terms of the Creative Commons Attribution License (CC BY). The use, distribution or reproduction in other forums is permitted, provided the original author(s) and the copyright owner(s) are credited and that the original publication in this journal is cited, in accordance with accepted academic practice. No use, distribution or reproduction is permitted which does not comply with these terms.
*Correspondence: Jake R. Weeks, Si5SLldlZWtzQHNvdG9uLmFjLnVr
Disclaimer: All claims expressed in this article are solely those of the authors and do not necessarily represent those of their affiliated organizations, or those of the publisher, the editors and the reviewers. Any product that may be evaluated in this article or claim that may be made by its manufacturer is not guaranteed or endorsed by the publisher.
Research integrity at Frontiers
Learn more about the work of our research integrity team to safeguard the quality of each article we publish.