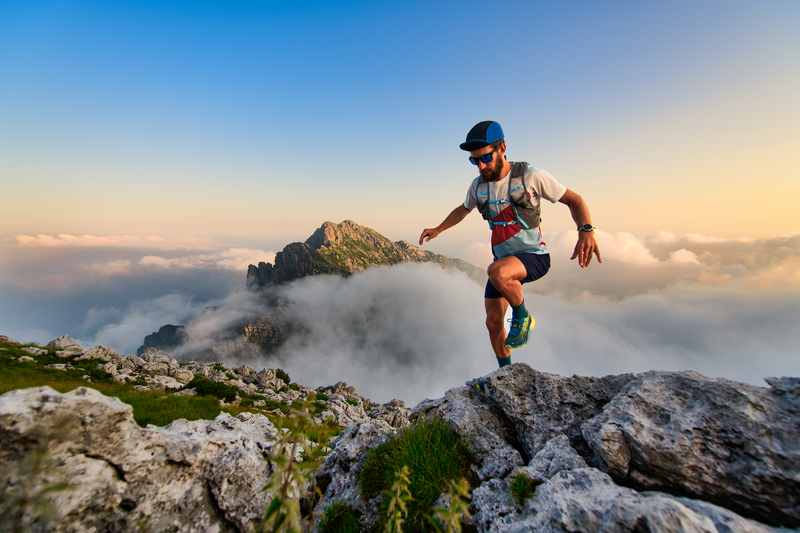
95% of researchers rate our articles as excellent or good
Learn more about the work of our research integrity team to safeguard the quality of each article we publish.
Find out more
ORIGINAL RESEARCH article
Front. Cell. Infect. Microbiol. , 03 September 2021
Sec. Fungal Pathogenesis
Volume 11 - 2021 | https://doi.org/10.3389/fcimb.2021.713092
This article is part of the Research Topic New Antifungal Drugs View all 9 articles
Fungal infections are increasing in prevalence worldwide. The paucity of available antifungal drug classes, combined with the increased occurrence of multidrug resistance in fungi, has led to new clinical challenges in the treatment of fungal infections. Candida auris is a recently emerged multidrug resistant human fungal pathogen that has become a worldwide public health threat. C. auris clinical isolates are often resistant to one or more antifungal drug classes, and thus, there is a high unmet medical need for the development of new therapeutic strategies effective against C. auris. Additionally, C. auris possesses several virulence traits, including the ability to form biofilms, further contributing to its drug resistance, and complicating the treatment of C. auris infections. Here we assessed red, green, and blue visible lights alone and in combination with photosensitizing compounds for their efficacies against C. auris biofilms. We found that (1) blue light inhibited and disrupted C. auris biofilms on its own and that the addition of photosensitizing compounds improved its antibiofilm potential; (2) red light inhibited and disrupted C. auris biofilms, but only in combination with photosensitizing compounds; and (3) green light inhibited C. auris biofilms in combination with photosensitizing compounds, but had no effects on disrupting C. auris biofilms. Taken together, our findings suggest that photodynamic therapy could be an effective non-drug therapeutic strategy against multidrug resistant C. auris biofilm infections.
Fungi are responsible for a wide range of infections in humans, including superficial skin infections as well as life-threatening disseminated infections (Brown et al., 2012). Three major classes of antifungal drugs (the polyenes, azoles, and echinocandins) are the most commonly used therapeutic agents for treating invasive fungal infections in humans (Odds et al., 2003; Prasad et al., 2016). The azoles and polyenes target the fungal cell membrane, while echinocandins target the fungal cell wall; thus, there is a need for new antifungal strategies with distinct mechanisms of action (Odds et al., 2003; Prasad et al., 2016).
Candida auris is a recently emerged human fungal pathogen belonging to the Candida/Clavispora clade that was first isolated from the ear canal of a patient in Japan in 2009, and has since been identified in over 35 countries (Satoh et al., 2009; Saris et al., 2018). C. auris is highly transmissible through surface contact, and has been isolated from the surfaces of windows, floors, curtains, bedrails, monitors, and other surfaces in healthcare settings (Welsh et al., 2017; Adams et al., 2018; Horton and Nett, 2020). In infected patients, C. auris is typically isolated from the skin, nares, wounds, axilla, and urinary tracts, as well as the bloodstream, bones, and cerebrospinal fluids of patients with severe invasive infections (Borman et al., 2016; Calvo et al., 2016; Morales-López et al., 2017; Horton and Nett, 2020). Once C. auris infections become systemic, they are associated with high mortality rates, ranging from 30-72%, with the highest mortality rates reported in patients with histories of extended hospital stays, implanted medical devices, or patients who have previously been treated with antifungal drugs (Cortegiani et al., 2018; Osei Sekyere, 2018; Spivak and Hanson, 2018; Chakrabarti and Singh, 2020; Garcia-Bustos et al., 2020; Shastri et al., 2020).
Since its emergence in 2009, C. auris clinical isolates have been reported to be resistant to one or more of the three major classes of antifungal drugs used to treat invasive fungal infections, with 90% resistant to at least one antifungal drug class, 30% resistant to at least two antifungal drug classes, and a handful displaying pan-resistance to all three major antifungal drug classes (Lockhart et al., 2017; Eyre et al., 2018; Cortegiani et al., 2019; Forsberg et al., 2019; Chakrabarti and Singh, 2020). C. auris resistance mechanisms are multifactorial, and have been reported to include the overexpression of the major facilitator superfamily (MFS) and ATP-binding cassette (ABC) drug efflux pumps, mutations in the ergosterol biosynthesis pathway, such as in the ERG11 gene, and mutations in the FKS1 gene, encoding a glucan synthase (Cortegiani et al., 2018; Kean et al., 2018; Chaabane et al., 2019; Dominguez et al., 2019; Bravo Ruiz and Lorenz, 2021). Given its heightened drug resistance and transmissibility, C. auris has become a serious global health threat (Lockhart et al., 2017; Piedrahita et al., 2017; Chaabane et al., 2019).
In the current coronavirus disease 2019 (COVID-19) pandemic, coinfections of C. auris with severe acute respiratory syndrome coronavirus 2 (SARS-CoV-2), have been increasingly reported, with high mortality rates (~60%), especially for critically ill patients who remain in the hospital for extended periods of time (>20 days) and in patients with implanted medical devices (e.g., catheters and ventilators) (Chowdhary et al., 2020; Steele et al., 2020; Zuo et al., 2020; Villanueva-Lozano et al., 2021). Additionally, high mortality rates (50-60%), have also been reported for C. auris-SARS-CoV-2 coinfections in patients with underlying chronic conditions, such as diabetes mellitus and kidney disease (Chowdhary et al., 2020; Rodriguez et al., 2020; Allaw et al., 2021; De Almeida et al., 2021; Magnasco et al., 2021; Prestel et al., 2021). The increased spread of C. auris infections during the COVID-19 pandemic is likely facilitated, at least in part, by the transformation of intensive care units and other hospital facilities into dedicated COVID-19 units, which foster ideal conditions for C. auris outbreaks (Chowdhary and Sharma, 2020; Villanueva-Lozano et al., 2021).
C. auris possesses multiple virulence traits that contribute to its pathogenicity, including the formation of biofilms (Forsberg et al., 2019; Chakrabarti and Singh, 2020). Biofilms are defined as communities of adherent microbial cells encased in a protective extracellular matrix (Kolter and Greenberg, 2006; López et al., 2010). C. auris biofilms are composed primarily of yeast-form cells interspersed with pseudohyphal cells that are encased in a mannan and glucan extracellular matrix (Sherry et al., 2017; Dominguez et al., 2019; Romera et al., 2019). Although planktonic C. auris cells display antifungal drug resistance on their own, C. auris cells isolated from biofilms are even more resistant to antifungal drugs than their free-floating counterparts (Larkin et al., 2017; Sherry et al., 2017; Cortegiani et al., 2018; Horton and Nett, 2020). C. auris biofilm formation is thought to occur in four stages: adherence, initiation, maturation, and dispersal (Kean et al., 2018; Dominguez et al., 2019) (Figure 1A). In the adherence stage, planktonic C. auris yeast-form cells adhere to biotic surfaces (e.g., skin, and mucosal layers) or abiotic surfaces (e.g., catheters, and prosthetic joints). In the initiation stage, the adhered C. auris yeast-form cells begin to proliferate, and some pseudohyphal cells develop. In the maturation stage, the cells within the C. auris biofilm continue to proliferate and an extracellular matrix that encases the biofilm cells is formed. Finally, in the dispersal stage, some C. auris yeast-form cells exit the biofilm to adhere to and form biofilms on new surfaces or enter the bloodstream to cause systemic infections.
Figure 1 The C. auris biofilm life cycle and the three biofilm assays used in this study to assess the effectiveness of visible lights with and without photosensitizing compounds. (A) The C. auris biofilm life cycle occurs in four stages: adherence, initiation, maturation, and dispersal. During the adherence stage, planktonic C. auris yeast-form cells adhere to a surface. During the initiation stage, the adhered cells proliferate, and some pseudohyphal cells are formed. During the maturation stage, the cells continue to proliferate and an extracellular matrix composed of glucans and mannans encases the biofilm cells. Finally, in the dispersal stage, yeast-form cells leave the biofilm to adhere to and form biofilms on new surfaces, or enter the bloodstream to cause systemic infections. (B) Overview of the adherence inhibition biofilm assay, where the visible light with (+) and without (-) the photosensitizing compound (PS) were present during the 90-min adherence stage of biofilm formation. (C) Overview of the developmental inhibition biofilm assay, where the visible light with (+) and without (-) the PS were present during the 24-h maturation stage of biofilm formation. (D) Overview of the disruption biofilm assay, where the visible light with (+) and without (-) the PS were present for an additional 24 h on a mature (24-h) biofilm. CFUs were measured to determine viable cell counts at the end of each biofilm assay. This figure was created using BioRender.com, and adapted from Bapat et al., 2021.
Given that C. auris clinical isolates are often resistant to one or more antifungal drug classes, there is a high unmet medical need for the development of new therapeutic strategies effective against C. auris. Photodynamic therapy has been used for the past 40 years to treat oncologic skin conditions, and more recently to treat benign inflammatory skin conditions, such as acne vulgaris and viral warts (Agostinis et al., 2011; Kim et al., 2015; Cohen et al., 2016). It has also been gaining scientific interest as a non-drug therapeutic strategy to treat a variety of infections (Cieplik et al., 2018). Photodynamic therapy requires the presence of a light source, a non-toxic photosensitizing compound, and molecular oxygen (Wainwright et al., 1998; Hamblin and Hasan, 2004; Wainwright et al., 2017). Following light exposure and absorption, the photosensitizing compound transfers electrons to molecular oxygen, which acts as an electron acceptor, ultimately leading to the production of cytotoxic reactive oxygen species (ROS), such as singlet oxygen, hydroxyl radicals, and superoxide anions (St. Denis et al., 2011; Lyon et al., 2011; Vatansever et al., 2013; Wainwright et al., 2017). Unlike traditional antimicrobial drugs, photodynamic therapy affects numerous microbial targets simultaneously, making it unlikely for resistance to develop. Given its non-specific mechanisms of action, photodynamic therapy could be a clinically useful therapeutic strategy effective against a multitude of infections, including those caused by multidrug resistant C. auris.
Broadly, the visible light spectrum is divided into red (620-700 nm), green (500-560 nm), and blue (400-490 nm) wavelengths, of which certain discreet wavelengths have been reported to display antimicrobial properties (Bruno and Svoronos, 2006; Denis et al., 2011; Wainwright et al., 2017; Cieplik et al., 2018; Gwynne and Gallagher, 2018). Blue light has been shown to effectively kill several different species of pathogenic bacteria and fungi, including methicillin resistant Staphylococcus aureus, carbapenem resistant Klebsiella pneumoniae, and ß-lactam resistant Escherichia coli (Dai et al., 2013; Murdoch et al., 2013; Cieplik et al., 2014; Teixeira et al., 2015; Halstead et al., 2016; Moorhead et al., 2016; Zhang et al., 2016; Dai, 2017; Guffey et al., 2017; Trzaska et al., 2017; Wang et al., 2017; Pinto et al., 2018; Wang et al., 2018; Ferrer-Espada et al., 2019). Compared to blue light, the antimicrobial properties of red and green lights have been considerably less studied to date (Vural et al., 2008; Huh et al., 2012; Gwynne and Gallagher, 2018; Panariello et al., 2019).
Although it has been shown that visible lights can have antimicrobial effects on their own, likely by generating ROS via the photoexcitation of naturally occurring photosensitizing compounds, such as porphyrins, the antimicrobial effects of visible lights combined with exogenous synthetic photosensitizing compounds have been shown to substantially increase the generation of ROS (Wainwright et al., 1998; Carvalho et al., 2009; Cieplik et al., 2014; Romano et al., 2017). Recently, the antimicrobial effects of visible lights alone and in combination with classic photosensitizing compounds, were comprehensively assessed against Candida albicans biofilms (Bapat et al., 2021). In this study, blue light was found to be highly effective at inhibiting and disrupting C. albicans biofilms on its own and the addition of photosensitizing compounds increased its antibiofilm effectiveness, while red and green lights were found to inhibit C. albicans biofilm formation only in combination with photosensitizing compounds, but were unable to disrupt biofilms. In terms of C. auris, to our knowledge, only one study to date has assessed the effects of photodynamic therapy on C. auris biofilms. In this study, red light combined with the photosensitizing compound methylene blue was found to be highly effective at reducing viable cell counts from C. auris biofilms (Tan et al., 2019).
To better understand the benefits of photodynamic therapy against C. auris infections, here we comprehensively assessed the efficacies of red, green, and blue visible lights alone and in combination with the classic photosensitizing compounds new methylene blue, toluidine blue O, and rose bengal, against C. auris biofilms. We found that blue light inhibited and disrupted C. auris biofilms on its own, and that the addition of photosensitizing compounds improved its antibiofilm effectiveness. We found that red light inhibited and disrupted C. auris biofilms, but only in combination with photosensitizing compounds. Finally, we found that green light inhibited C. auris biofilms in combination with photosensitizing compounds, but had no effects on disrupting C. auris biofilms. In general, the effects we observed on C. auris biofilms were similar across biofilms formed by different C. auris clinical isolates from distinct genetic clades that display different antifungal drug susceptibilities.
Given that the effects of visible lights on C. albicans biofilms have been comprehensively assessed (Bapat et al., 2021), we used the C. albicans clinical isolate SC5314 (Meyers et al., 1968) as a reference strain in this study. We used the following C. auris clinical isolates: Strain #0383 (AR0383; South African clade), Strain #0389 (AR0389; South Asian clade), and Strain #0390 (AR0390; South Asian clade) (Centers for Disease Control and Prevention (CDC) AR Isolate Bank, Drug Resistance Candida species panel; https://wwwn.cdc.gov/ARIsolateBank/; accessed on 02/20/2021). The minimum inhibitory concentrations (MICs) for representative drugs from the three major antifungal drug classes used to treat invasive fungal infections for each C. auris isolate used in this study have been reported previously (Lockhart et al., 2017; https://www.cdc.gov/fungal/candida-auris/c-auris-antifungal.html/; accessed on 05/07/2021), and can be found in Table S1. C. auris and C. albicans cells were recovered from -80°C glycerol stocks for two days at 30°C on yeast extract peptone dextrose (YPD) agar plates [1% yeast extract (Thermo Fisher Scientific, Catalog #211929), 2% Bacto peptone (Gibco, Catalog #211677), 2% dextrose (Fisher Scientific Catalog #D16-3), and 2% agar (Criterion, Catalog #89405-066)]. Overnight cultures were grown for ~15 h at 30°C, shaking at 225 rpm in YPD liquid medium [1% yeast extract (Thermo Fisher Scientific, Catalog #211929), 2% Bacto peptone (Gibco, Catalog #211677), and 2% dextrose (Fisher Scientific Catalog #D16-3)]. All biofilm assays were performed using RPMI-1640 medium with L-glutamine and without sodium bicarbonate (Sigma Aldrich, Catalog #R6504-10X1L), supplemented with 34.5 g/L MOPS (Sigma Aldrich, Catalog #M3183), adjusted to pH 7.2 with sodium hydroxide (Fisher Scientific, Catalog #S318-100), and filter sterilized using a 0.22 µm filter (Corning, Catalog #431098).
A red LED light source (ABI LED lighting, Catalog #GR-PAR38-26W-RED, 26-Watt 620-630 nm, outputting 176 J/cm2), a green LED light source (ABI LED lighting, #GR-PAR38-24W-520nm, 24-Watt 520-530 nm, outputting 204 J/cm2), and a blue LED light source (ABI LED lighting, GR-PAR38-24W-BLU, 24-Watt 450 nm, outputting 240 J/cm2) were placed at a distance of 8 inches directly above the biofilm wells and were used as described previously in the biofilm assays (Bapat et al., 2021). Average LED light intensity measurements at this distance were 6500 lux for red light, 6700 lux for green light, and 5900 lux for blue light.
The photosensitizing compounds new methylene blue (Sigma Aldrich, Catalog #B-4631), toluidine blue O (Sigma Aldrich, Catalog #T3260), and rose bengal (Sigma Aldrich, Catalog #198250) were added alone and in combination with the red, green, and blue visible lights in the biofilm assays. The photosensitizing compounds were dissolved in PBS (HyClone, Catalog #16777-252) at a stock concentration of 10 mM and diluted to a working concentration of 400 μM in RPMI-1640 medium, which was used to grow the biofilms. Stocks of the photosensitizing compounds were prepared fresh every two weeks, filter sterilized using a 0.22 µm filter, and stored at 4°C in the dark.
The adherence inhibition, developmental inhibition, and disruption biofilm assays were performed as described previously (Bapat et al., 2021), where colony forming units (CFUs) were measured at the end of the assays to assess the efficacies of the visible lights with or without photosensitizing compounds at reducing C. auris and C. albicans viable cell counts from the biofilms.
Briefly, biofilms were grown in triplicate on the bottoms of sterile flat-bottomed 12-well non-tissue culture treated polystyrene plates (Corning, Catalog #351143). The 12-well plates were seeded with Candida cells at a final OD600 of 0.5 in a final volume of 2 mL of RPMI-1640 medium and grown for 90 min at 37°C, with shaking at 250 rpm in an ELMI shaker (M2 Scientifics, Catalog #ELMI-TRMS 04). After the 90-min adherence stage, the wells were washed gently with PBS and fresh RPMI-1640 medium was added to each well. The plates were sealed with breathable sealing membranes (Sigma Aldrich, Catalog #Z380059) and grown for 24 h at 37°C, with shaking at 250 rpm in an ELMI shaker. For the adherence inhibition biofilm assay, the biofilms were exposed to red, green, or blue visible lights with or without a photosensitizing compound during the 90-min adherence stage of biofilm formation (Figure 1B). For the developmental inhibition biofilm assay, the biofilms were exposed to red, green, or blue visible lights with or without a photosensitizing compound throughout the first 24 h of biofilm growth, but not during the initial 90-min adherence stage (Figure 1C). For the disruption biofilm assay, biofilms were grown, medium was removed from each well containing mature 24-h biofilms, fresh RPMI-1640 medium was added to each well, the plates were re-sealed, and the mature biofilms were exposed to red, green, or blue visible lights with or without a photosensitizing compound for an additional 24 h (Figure 1D). The 12-well plates were divided such that half of one plate was exposed to the light of interest and the other half was covered with foil and served as a no light control.
CFU determinations from biofilms were performed as previously described (Lohse et al., 2017; Gulati et al., 2018; Bapat et al., 2021). Briefly, biofilms were scraped from the bottoms of the each well of a 12-well plate using a sterile spatula, vigorously vortexed, serially diluted in PBS, and plated onto YPD agar plates. The plates were incubated at 30°C for 2 days and colonies were counted to determine CFUs/mL. Statistical significance was determined using Student’s unpaired two-tailed t-tests assuming unequal variance.
To assess the viability of C. auris biofilm cells, viability staining was performed both on C. auris biofilms directly and on C. auris cells resuspended from biofilms under each light and photosensitizing compound treatment condition using the LIVE/DEAD BacLight viability kit (Invitrogen, Catalog #L7012) as described previously for use on C. albicans biofilms (Jin et al., 2005; Bapat et al., 2021), and according to the manufacturer’s protocol. Briefly, the samples were incubated with 3 μL of SYTO9 and 3 μL of propidium iodide in the dark at 30°C for 20 min. Following incubation, the samples were imaged by fluorescence microscopy at 20X magnification with a green laser (GFP/green channel; 470 nm excitation wavelength) and a red laser (Texas Red/red channel; 585 nm excitation wavelength) using an EVOS Cell Imaging System (Life Technologies, Catalog #EVOS FL Cell Imaging System).
To determine whether red, green, and blue visible lights on their own can affect C. auris biofilm development, we first performed the three biofilm assays in the presence of each of these visible light treatments. We used three C. auris clinical isolates encompassing two different genetic clades (AR0383 from the South African clade, AR0389 from the South Asian clade, and AR0390 from the South Asian clade). We found that red and green visible lights on their own had no effects on C. auris biofilms in any of the three biofilm assays compared to the untreated control (Figures 2A, B). We also found that blue light on its own had no effect at inhibiting C. auris biofilm formation in the adherence inhibition biofilm assay compared to the untreated control (Figure 2C). However, blue light on its own was effective at inhibiting C. auris biofilm formation by 77% (averaging all three C. auris strains) in the developmental inhibition biofilm assay (p=0.0001) (Figure 2C). We also found that blue light on its own was effective at disrupting C. auris biofilms by 57% (averaging all three C. auris strains) in the disruption biofilm assay (p=0.0004) (Figure 2C).
Figure 2 Blue visible light alone is effective against C. auris biofilms. C. albicans (SC5314) and C. auris (AR0383, AR0389, and AR0390) biofilms were exposed to red, green, and blue visible lights individually in the adherence inhibition, developmental inhibition, and disruption biofilm assays. CFUs/mL were counted to determine viable cell counts at the end of each of the biofilm assays. Effects of (A) red light alone (Red Light), (B) green light alone (Green Light), and (C) blue light alone (Blue Light) in the three different biofilm assays compared to an untreated control (Untreated). Standard deviations are shown for each sample (n = 3). The average CFUs/mL of the untreated control samples for each assay were normalized to 1. Significance comparisons are relative to the untreated control and were determined using student’s unpaired two-tailed t-tests assuming unequal variance for p ≤ 0.001 (***).
To determine whether red, green, and blue visible lights combined with classic exogenous photosensitizing compounds can affect C. auris biofilm development, we performed the three biofilm assays in the presence of each of these visible light treatments plus new methylene blue, toluidine blue O, and rose bengal, and assessed the effects of this treatment on C. auris biofilms formed by AR0383, AR0389, and AR0390. Compared to the average of the untreated control, red light on its own, and each photosensitizing compound on its own (i.e., the three negative controls), we found that red light plus any of the photosensitizing compounds had no effect on C. auris biofilm formation in the adherence inhibition biofilm assay (Figure 3A). Compared to the average of the three negative controls, we found that red light plus any of the photosensitizing compounds was effective at inhibiting C. auris biofilm formation by 58% when combined with new methylene blue (p=0.0001), 58% when combined with toluidine blue O (p=0.0002), and 55% when combined with rose bengal (p=0.0001) (averaging all three C. auris strains) in the developmental inhibition biofilm assay (Figure 3B). Compared to the average of the three negative controls, we found that red light plus any of the photosensitizing compounds was effective at disrupting mature C. auris biofilms by 71% when combined with new methylene blue (p=0.0005), 76% when combined with toluidine blue O (p=0.0004), and 32% when combined with rose bengal (p=0.009) (averaging all three C. auris strains) in the disruption biofilm assay (Figure 3C).
Figure 3 Red visible light in combination with photosensitizing compounds is effective against C. auris biofilms. C albicans (SC5314) and C. auris (AR0383, AR0389, and AR0390) biofilms were exposed to red visible light with and without the photosensitizing compound indicated in the (A) adherence inhibition, (B) developmental inhibition, and (C) disruption biofilm assays. Untreated control (Untreated), red light alone (Red Light), photosensitizing compound alone (New Methylene Blue, Toluidine Blue O, and Rose Bengal), and red light in combination with the photosensitizing compound (Red Light + New Methylene Blue, Red Light + Toluidine Blue O, and Red Light + Rose Bengal) are shown. CFUs/mL were measured to determine viable cell counts from the biofilms at the end of each biofilm assay. Standard deviations are shown for each sample (n = 3). The average CFUs/mL of the untreated control samples for each assay were normalized to 1. Significance comparisons are relative to the untreated control unless otherwise noted with significance bars and were determined using student’s unpaired two-tailed t-tests assuming unequal variance for p ≤ 0.05 (*), p ≤ 0.01 (**), and p ≤ 0.001 (***).
Compared to the average of the untreated control, green light on its own, and each photosensitizing compound on its own (i.e., the three negative controls), we found that green light plus any of the photosensitizing compounds had no effect on C. auris biofilm formation in the adherence inhibition biofilm assay (Figure 4A). Compared to the average of the three negative controls, we found that green light plus any of the photosensitizing compounds was effective at inhibiting C. auris biofilm formation by 62% when combined with new methylene blue (p=0.004), 76% when combined with toluidine blue O (p=0.0007), and 74% when combined with rose bengal (p=0.0004) (averaging all three C. auris strains) in the developmental inhibition biofilm assay (Figure 4B). Compared to the average of the three negative controls, we found that green light plus any of the photosensitizing compounds was not effective at disrupting mature C. auris biofilms (averaging all three C. auris strains) in the disruption biofilm assay (Figure 4C).
Figure 4 Green visible light in combination with photosensitizing compounds is effective against C. auris biofilms. C albicans (SC5314) and C. auris (AR0383, AR0389, and AR0390) biofilms were exposed to green visible light with and without the photosensitizing compound indicated in the (A) adherence inhibition, (B) developmental inhibition, and (C) disruption biofilm assays. Untreated control (Untreated), green light alone (Green Light), photosensitizing compound alone (New Methylene Blue, Toluidine Blue O, and Rose Bengal), and green light in combination with the photosensitizing compound (Green Light + New Methylene Blue, Green Light + Toluidine Blue O, and Green Light + Rose Bengal) are shown. CFUs/mL were counted to determine viable cell counts at the end of each of the biofilm assays. Standard deviations are shown for each sample (n = 3). The average CFUs/mL of the untreated control samples for each assay were normalized to 1. Significance comparisons are relative to the untreated control unless otherwise noted with significance bars and were determined using student’s unpaired two-tailed t-tests assuming unequal variance for p ≤ 0.05 (*), and p ≤ 0.01 (**) and p ≤ 0.001 (***).
Compared to the average of the untreated control, blue light on its own, and each photosensitizing compound on its own, we found that blue light plus any of the three photosensitizing compounds had no effect on C. auris biofilm formation in the adherence inhibition biofilm assay (Figure 5A). Since blue light on its own was effective at inhibiting and disrupting C. auris biofilms in the developmental inhibition biofilm assay and the disruption biofilm assay, respectively (Figure 2C), we compared the effects of blue light plus the three photosensitizing compounds to the average of the untreated control and each photosensitizing compound on its own (i.e., the two negative controls) for these biofilm assays. Compared to the average of the two negative controls, we found that blue light plus any of the photosensitizing compounds was effective at inhibiting C. auris biofilm formation by 84% when combined with new methylene blue (p=0.00001), 85% when combined with toluidine blue O (p=0.00001), and 78% when combined with rose bengal (p=0.0001) (averaging all three C. auris strains) in the developmental inhibition biofilm assay (Figure 5B). Compared to the biofilm inhibitory effects of blue light on its own, we found that blue light plus new methylene blue had an additive inhibitory effect of 7% (p=0.01), and blue light plus toluidine blue O had an additive inhibitory effect of 8% (p=0.01) (averaging all three C. auris strains) in the developmental inhibition biofilm assay (Figure 5B). We did not observe an additive inhibitory effect of blue light plus rose bengal against C. auris biofilms in the developmental inhibition biofilm assay (Figure 5B). Compared to the average of the two negative controls, we found that blue light plus any of the photosensitizing compounds was effective at disrupting mature C. auris biofilms by 79% when combined with new methylene blue (p=0.0003), 79% when combined with toluidine blue O (p=0.0002), and 66% when combined with rose bengal (p=0.007) (averaging all three C. auris strains) in the disruption biofilm assay (Figure 5C). Compared to the biofilm disruption effects of blue light on its own, the combination of blue light plus new methylene blue had an additive biofilm disruption effect of 22% (p=0.002), blue light plus toluidine blue O had an additive effect of 22% (p=0.002), and blue light plus rose bengal had an additive effect of 9% (p=0.01) (averaging all three C. auris strains) in the disruption biofilm assay (Figure 5C).
Figure 5 Blue visible light in combination with photosensitizing compounds is effective against C. auris biofilms. C. albicans (SC5314) and C. auris (AR0383, AR0389, and AR0390) biofilms were exposed to blue visible light with and without the photosensitizing compound indicated in the (A) adherence inhibition, (B) developmental inhibition, and (C) disruption biofilm assays. Untreated control (Untreated), blue light alone (Blue Light), photosensitizing compound alone (New Methylene Blue, Toluidine Blue O, and Rose Bengal), and blue light in combination with the photosensitizing compounds (Blue Light + New Methylene Blue, Blue Light + Toluidine Blue O, and Blue Light + Rose Bengal) are shown. CFUs/mL were counted to determine viable cell counts at the end of each of the biofilm assays. Standard deviations are shown for each sample (n = 3). The average CFUs/mL of the untreated control samples for each assay were normalized to 1. Significance comparisons are relative to the untreated control unless otherwise noted with significance bars and were determined using student’s unpaired two-tailed t-tests assuming unequal variance for p ≤ 0.05 (*), p ≤ 0.01 (**), and p ≤ 0.001 (***).
Given that none of the light and photosensitizing compound combination treatments were effective at inhibiting C. auris biofilms in the adherence inhibition biofilm assay (90 min exposure), but were effective at inhibiting C. auris biofilms in the development inhibition biofilm assay (24 hr exposure) and were effective at disrupting C. auris biofilms in the disruption biofilm assay (24 hr exposure), we wondered whether these treatments would have antibiofilm effects on C. auris biofilms in the developmental inhibition and disruption biofilm assays if they were applied for only 90 min. To test this, we used blue light plus toluidine blue O, which was highly effective against C. auris biofilms, and assessed the effects of this treatment for only 90 min on C. auris biofilms formed by AR0383, AR0389, and AR0390 in the developmental inhibition and disruption biofilm assays. In this shortened timeframe (90 min exposure), we observed no significant antibiofilm effects in the developmental inhibition or disruption biofilm assays (Figure S1).
Finally, to independently assess cell viability within C. auris biofilms, we performed LIVE/DEAD staining assays on both C. auris biofilms directly and on C. auris cells resuspended from biofilms after treatment with the different visible lights and photosensitizing compounds. Our cell viability staining results were consistent with our CFU determinations for all treatment conditions (see Figures S2–S6 for representative images from the LIVE/DEAD staining assays performed directly on C. auris biofilms formed by AR0383 and Figures S7–S11 for representative images from the LIVE/DEAD staining assays performed on C. auris cells resuspended from biofilms formed by AR0383).
Photodynamic therapy is used today to treat oncological and inflammatory skin conditions; however, its use as an antimicrobial strategy is only beginning to be realized. Photodynamic therapy relies on the production of ROS that can have cytotoxic effects on targeted cells. To determine the utility of photodynamic therapy for use against C. auris infections, we assessed the antibiofilm effects of visible lights alone and in combination with classic photosensitizing compounds on C. auris biofilms. We found that, of the visible lights tested, blue light was the only visible light that had antibiofilm properties on its own against C. auris biofilms, where it clearly prevented biofilm formation when it was applied throughout biofilm development, as well as clearly disrupted biofilms when it was applied on a mature biofilm. Overall, we found that red, green, and blue visible lights when combined with photosensitizing compounds, prevented C. auris biofilm formation when applied throughout biofilm development; however, only red and blue lights in combination with photosensitizing compounds disrupted mature C. auris biofilms. Interestingly, none of the visible lights and photosensitizing compound combination treatments were effective at inhibiting C. auris biofilms during the 90-min adherence stage of biofilm formation, at inhibiting C. auris biofilm development when the exposure time was shortened to 90 min, or at disrupting mature C. auris biofilms when the exposure time was shortened to 90 min, highlighting the potential importance of exposure time in the antibiofilm efficacy of photodynamic therapy.
Our findings on C. auris biofilms indicate that photosensitizing compounds can sensitize C. auris biofilms to visible lights when applied during biofilm development (i.e., over the course of a 24-hr period). We found that the combination treatments of red and blue lights with the photosensitizing compounds had the most striking antibiofilm effects, where these treatments both prevented C. auris biofilm formation as well as disrupted mature C. auris biofilms, significantly above red and blue light treatments alone. These effects were most notable when red and blue lights were combined with new methylene blue and toluidine blue O, which are structurally similar (phenothiazinium salt) photosensitizing compounds. Although the detailed mechanisms of how photosensitizing compounds sensitize C. auris biofilms to light exposure are not understood, photosensitizing compounds are generally known to enhance the production of ROS (Cieplik et al., 2014; Abrahamse and Hamblin, 2016; Cieplik et al., 2018), which likely leads to cytotoxicity of C. auris biofilm cells. Overall, our findings demonstrate that blue light combined with toluidine blue O, followed by blue light combined with new methylene blue, red light combined with toluidine blue O, and then red light combined with new methylene blue, are the most effective photodynamic therapy treatment combinations against C. auris biofilms.
In general, the majority of our findings on the effects of visible lights combined with photosensitizing compounds on C. auris biofilms are consistent with the effects of these treatments on C. albicans biofilms (Bapat et al., 2021); however, there are two notable species-specific differences that we would like to point out. First, we found that red light in combination with photosensitizing compounds was effective at disrupting mature C. auris biofilms by 60% on average, while this treatment had no effect on C. albicans biofilms. Second, we found that green light in combination with toluidine blue O, and green light in combination with rose bengal, were on average more effective at preventing C. auris biofilm formation by 32% and 42%, respectively, than they were at preventing C. albicans biofilm formation. These observed species-specific differences in treatment efficacies suggest that photodynamic therapy may be overall more effective against C. auris biofilms than against C. albicans biofilms, which may, in part, be due to structural differences between C. auris and C. albicans biofilms. For example, C. auris biofilms are generally thinner than C. albicans biofilms, and are composed of yeast-form cells with occasional pseudohyphal cells that are encased in a glucan and mannan extracellular matrix (Sherry et al., 2017; Dominguez et al., 2019; Romera et al., 2019). C. albicans biofilms, on the other hand, are generally thicker than C. auris biofilms, and are composed of yeast-form cells, pseudohyphal cells, and hyphal cells, encased in an extracellular matrix composed of proteins, lipids, carbohydrates, and nucleic acids (Zarnowski et al., 2014; Mukherjee and Chandra, 2015; Nobile and Johnson, 2015; Gulati and Nobile, 2016). These structural differences between C. auris and C. albicans biofilms could influence the efficacies of photodynamic therapy by affecting the uptake of photosensitizing compounds and the traversal of visible lights throughout the biofilm architecture (Larkin et al., 2017). In addition, differences in cell wall composition between C. auris and C. albicans cells could also impact how visible lights and photosensitizing compounds interact with the cell wall and thus impact the antibiofilm effectiveness of photodynamic therapy. The C. auris cell wall, for example, contains distinct cell surface mannans that are absent from the C. albicans cell wall as well as elevated chitin levels relative to the C. albicans cell wall (Navarro-arias et al., 2019; Zamith-Miranda et al., 2019; Yan et al., 2020).
Since antimicrobial photodynamic therapy relies on the localized production of ROS to cause oxidation of microbial lipids, proteins, and carbohydrates, it is likely to have broad-spectrum antimicrobial activity against many different microorganisms (Jockusch et al., 1996; Hamblin and Hasan, 2004; Maisch, 2015; Carrera et al., 2016). Indeed, there is evidence in the literature to suggest that photodynamic therapy is effective at killing a wide range of microorganisms, including pathogenic gram-positive and gram-negative bacteria, protozoa, fungi, and even viruses (Gardlo et al., 2003; Wainwright, 2004; Marotti et al., 2009; Kharkwal et al., 2011; Lyon et al., 2011; De Lucca et al., 2012; Teixeira et al., 2015). In fact, in the current COVID-19 pandemic, antimicrobial photodynamic therapy has been suggested as a potential therapeutic strategy to use against COVID-19 infections (Wiehe et al., 2019; Almeida et al., 2020; Dias and Bagnato, 2020). Consistent with this idea, one recent study demonstrated that red light in combination with photosensitizing compounds was effective at inhibiting SARS-CoV-2 viral replication within mammalian Vero E6 cells (Svyatchenko et al., 2021). Given that the prevalence of C. auris-SARS-CoV-2 coinfections have been increasing throughout the COVID-19 pandemic and that there is evidence to suggest that photodynamic therapy could be effective against C. auris and SARS-CoV-2 infections individually, photodynamic therapy could be a promising therapeutic strategy to consider for these as well as other coinfections in the clinic.
Recently, pan-resistant clinical isolates of C. auris that are resistant to all three of the major classes of antifungal drugs available to treat invasive fungal infections in humans have been reported in several countries, including the United States (Ostrowsky et al., 2020). Despite the emergence of these pan-resistant isolates, antifungal drugs remain the most commonly used treatment for C. auris infections (Cortegiani et al., 2018; Spivak and Hanson, 2018). Based on our findings as well as numerous findings in the literature on the effectiveness of antimicrobial photodynamic therapies against a multitude of pathogenic microorganisms across phylogenetic kingdoms, we believe that photodynamic therapy could be a valuable therapeutic strategy that should be explored further for use against C. auris infections. In the context of C. auris infections, there are at least three major shortcomings of traditional antifungal drug therapies that could be overcome by using photodynamic therapy. First, the development of antifungal drug resistance after exposure to antifungal drugs has the potential to render antifungal drug treatments completely ineffective against fungal infections. This is frequently observed in the context of C. auris infections, and in fact, the majority of C. auris clinical isolates are resistant to at least one antifungal drug class (Lockhart et al., 2017; Cortegiani et al., 2018; Forsberg et al., 2019). Since photodynamic therapy leads to the production of ROS that broadly affect numerous microbial targets simultaneously, it is unlikely that C. auris resistance to photodynamic therapy could be developed. Second, antifungal drugs, like the polyenes, are known to cause substantial toxicities in humans and are usually administered systemwide (Roemer and Krysan, 2014). The non-toxic photosensitizing compounds and the visible lights used during the administration of photodynamic therapy pose little, if any, toxicity concerns to humans (Hamblin and Hasan, 2004; Wainwright et al., 2017). Additionally, photodynamic therapy can be spatially confined to an area of interest, thus limiting unnecessary exposure of human cells to the treatment. Third, almost all existing antimicrobial drugs function by targeting microbial metabolic processes, and therefore require that the cells are metabolically active (Kuhn et al., 2002; Bojsen et al., 2014; Stokes et al., 2019). This necessity, especially within heterogeneous microbial cell populations, can cause substantial inconsistencies in effectiveness of the antimicrobial drug. This is particularly true in the context of biofilms, where different cell populations are present throughout the biofilm architecture with varying levels of metabolic activity (Wimpenny et al., 2000; Mah and O’Toole, 2001; Taff et al., 2013). Additionally, persister cells, defined as metabolically dormant phenotypic cell variants, are markedly difficult to eradicate within mature biofilms using traditional antimicrobial drugs (Stewart, 2002; Lewis, 2010; Taff et al., 2013; Fox et al., 2015). C. auris biofilms, in particular, are notorious for displaying low susceptibilities to existing antifungal drugs, including caspofungin and amphotericin B, which is likely the result of, at least in part, cell heterogeneity within C. auris biofilms (Sherry et al., 2017; Kean et al., 2018). The effectiveness of photodynamic therapy does not require that microbial cells are metabolically active; indeed, there are studies that have found that photodynamic therapy can be effective against bacterial persister cells (Hamblin and Hasan, 2004; Oppezzo and Forte Giacobone, 2018).
In summary, our results suggest that photodynamic therapy is highly effective at inhibiting C. auris biofilm formation and at disrupting mature C. auris biofilms in vitro. Given that our antifungal drug arsenal is extremely limited and that pan-resistant C. auris isolates have been emerging, new therapeutic strategies effective against C. auris are urgently needed. Our work suggests that photodynamic therapy could be a clinically viable option in combating C. auris infections that should be explored further.
The original contributions presented in the study are included in the article/Supplementary Material. Further inquiries can be directed to the corresponding author.
Conceptualization PB and CN. Methodology PB and CN. Validation PB and CN. Formal Analysis PB. Investigation PB. Resources, CN. Data Curation, PB. Writing – Original Draft Preparation, PB. Writing – Review & Editing, PB and CN. Visualization, PB. Supervision, CN. Project Administration, CN. Funding Acquisition, CN. All authors contributed to the article and approved the submitted version.
This work was supported by the National Institutes of Health (NIH) National Institute of General Medical Sciences (NIGMS) award R35GM124594, and by the Kamangar family in the form of an endowed chair to CN. The funders had no role in the study design, data collection and interpretation, or the decision to submit the work for publication.
CN is a cofounder of BioSynesis, Inc., a company developing diagnostics and therapeutics for biofilm infections.
The remaining author declares that the research was conducted in the absence of any commercial or financial relationships that could be construed as a potential conflict of interest.
All claims expressed in this article are solely those of the authors and do not necessarily represent those of their affiliated organizations, or those of the publisher, the editors and the reviewers. Any product that may be evaluated in this article, or claim that may be made by its manufacturer, is not guaranteed or endorsed by the publisher.
We thank all members of the Nobile lab for helpful discussions on the topic of this manuscript.
The Supplementary Material for this article can be found online at: https://www.frontiersin.org/articles/10.3389/fcimb.2021.713092/full#supplementary-material
Abrahamse, H., Hamblin, M. R. (2016). New Photosensitizers for Photodynamic Therapy. Biochem. J. 473, 347–364. doi: 10.1042/BJ20150942
Adams, E., Quinn, M., Tsay, S., Poirot, E., Chaturvedi, S., Southwick, K., et al. (2018). Candida Auris in Healthcare Facilities, New York, US–2017. Emerg. Infect. Dis. 24, 1816–1824. doi: 10.3201/eid2410.180649
Agostinis, P., Berg, K., Cengel, K. A., Foster, T. H., Girotti, A. W., Gollnick, S. O., et al. (2011). Photodynamic Therapy of Cancer: An Update. CA. Cancer J. Clin. 61, 250–281. doi: 10.3322/caac.20114
Allaw, F., Kara Zahreddine, N., Ibrahim, A., Tannous, J., Taleb, H., Rahman Bizri, A., et al. (2021). First Candida Auris Outbreak During a COVID-19 Pandemic in a Tertiary-Care Center in Lebanon. Pathogens 10, 1–10. doi: 10.3390/pathogens10020157
Almeida, A., Faustino, M. A. F., Neves, M. G. P. M. S. (2020). Antimicrobial Photodynamic Therapy in the Control of COVID-19. Antibiotics 9, 1–10. doi: 10.3390/antibiotics9060320
Bapat, P., Singh, G., Nobile, C. J. (2021). Visible Lights Combined With Photosensitizing Compounds are Effective Against Candida Albicans Biofilms. Microorganisms 9:500. doi: 10.3390/microorganisms9030500
Bojsen, R., Regenberg, B., Folkesson, A. (2014). Saccharomyces Cerevisiae Biofilm Tolerance Towards Systemic Antifungals Depends on Growth Phase. BMC Microbiol. 14:305. doi: 10.1186/s12866-014-0305-4
Borman, A. M., Szekely, A., Johnson, E. M. (2016). Comparative Pathogenicity of United Kingdom Isolates of the Emerging Pathogen Candida Auris and Other Key Pathogenic Candida Species. mSphere 1, 1–8. doi: 10.1128/msphere.00189-16
Bravo Ruiz, G., Lorenz, A. (2021). What do We Know About the Biology of the Emerging Fungal Pathogen of Humans Candida Auris? Microbiol. Res. 242:126621. doi: 10.1016/j.micres.2020.126621
Brown, G. D., Denning, D. W., Gow, N. A. R., Levitz, S. M., Netea, M. G., White, T. C. (2012). Hidden Killers: Human Fungal Infections. Sci. Transl. Med. 4, 165rv13–165rv13. doi: 10.1126/scitranslmed.3004404
Bruno, J. T., Svoronos, P. (2006). CRC Handbook of Fundamental Spectroscopic Correlation Charts (Boca Raton, United States: CRC Press). doi: 10.1201/9781420037685
Calvo, B., Melo, A. S. A., Perozo-Mena, A., Hernandez, M., Francisco, E. C., Hagen, F., et al. (2016). First Report of Candida Auris in America: Clinical and Microbiological Aspects of 18 Episodes of Candidemia. J. Infect. 73, 369–374. doi: 10.1016/j.jinf.2016.07.008
Carrera, E. T., Dias, H. B., Corbi, S. C. T., Marcantonio, R. A. C., Bernardi, A. C. A., Bagnato, V. S., et al. (2016). The Application of Antimicrobial Photodynamic Therapy (Apdt) in Dentistry: A Critical Review. Laser Phys. 26, 1–14. doi: 10.1088/1054-660X/26/12/123001
Carvalho, G. G., Felipe, M. P., Costa, M. S. (2009). The Photodynamic Effect of Methylene Blue and Toluidine Blue on Candida Albicans Is Dependent on Medium Conditions. J. Microbiol. 47, 619–623. doi: 10.1007/s12275-009-0059-0
Chaabane, F., Graf, A., Jequier, L. (2019). Review on Antifungal Resistance Mechanisms in the Emerging Pathogen Candida Auris. Front. Microbiol. 10:2788. doi: 10.3389/fmicb.2019.02788
Chakrabarti, A., Singh, S. (2020). Multidrug-Resistant Candida Auris: An Epidemiological Review. Expert Rev. Anti-infective Ther. 18, 551–562. doi: 10.1080/14787210.2020.1750368
Chowdhary, A., Sharma, A. (2020). The Lurking Scourge of Multidrug Resistant Candida Auris in Times of COVID-19 Pandemic. J. Glob. Antimicrob. Resist. 22, 175–176. doi: 10.1016/j.jgar.2020.06.003
Chowdhary, A., Tarai, B., Singh, A., Sharma, A. (2020). Multidrug-Resistant Candida Auris Infections in Critically Ill Coronavirus Disease Patients, India, April-July 2020. Emerg. Infect. Dis. 26, 2694–2696. doi: 10.1007/s00134-020-05991-x
Cieplik, F., Deng, D., Crielaard, W., Buchalla, W., Hellwig, E., Al-Ahmad, A., et al. (2018). Antimicrobial Photodynamic Therapy – What We Know and What We Don’t. Crit. Rev. Microbiol. 44, 571–589. doi: 10.1080/1040841X.2018.1467876
Cieplik, F., Tabenski, L., Buchalla, W., Maisch, T. (2014). Antimicrobial Photodynamic Therapy for Inactivation of Biofilms Formed by Oral Key Pathogens. Front. Microbiol. 5:405. doi: 10.3389/fmicb.2014.00405
Cohen, D. K., Lee, P. K., Hamblin, M. R. (2016). Photodynamic Therapy for Non-Melanoma Skin Cancers. Cancers (Basel) 88, 1–9. doi: 10.3390/cancers8100090
Cortegiani, A., Misseri, G., Fasciana, T., Giammanco, A., Giarratano, A., Chowdhary, A. (2018). Epidemiology, Clinical Characteristics, Resistance, and Treatment of Infections by Candida Auris. J. Intensive Care 6, 1–13. doi: 10.1186/s40560-018-0342-4
Cortegiani, A., Misseri, G., Giarratano, A., Bassetti, M., Eyre, D. (2019). The Global Challenge of Candida Auris in the Intensive Care Unit. Crit. Care 23, 4–6. doi: 10.1186/s13054-019-2449-y
Dai, T. (2017). The Antimicrobial Effect of Blue Light: What are Behind? Virulence 8 (6), 649–652. doi: 10.1080/21505594.2016.1276691
Dai, T., Gupta, A., Huang, Y. Y., Sherwood, M. E., Murray, C. K., Vrahas, M. S., et al. (2013). Blue Light Eliminates Community-Acquired Methicillin-Resistant Staphylococcus Aureus in Infected Mouse Skin Abrasions. Photomed. Laser Surg. 31, 531–538. doi: 10.1089/pho.2012.3365
De Almeida, J. N., Francisco, E. C., Hagen, F., Brandão, I. B., Pereira, F. M., Dias, P. H. P., et al. (2021). Emergence of Candida Auris in Brazil in a COVID-19 Intensive Care Unit. J. Fungi 7, 1–13. doi: 10.3390/jof7030220
De Lucca, A. J., Carter-Wientjes, C., Williams, K. A., Bhatnagar, D. (2012). Blue Light (470 Nm) Effectively Inhibits Bacterial and Fungal Growth. Lett. Appl. Microbiol. 55, 460–466. doi: 10.1111/lam.12002
Denis, T.G. St., Dai, T., Izikson, L., Astrakas, C., Anderson, R. R., Hamblin, M. R., et al. (2011). All You Need Is Light. Virulence 2, 509–520. doi: 10.4161/viru.2.6.17889
Dias, L. D., Bagnato, V. S. (2020). An Update on Clinical Photodynamic Therapy for Fighting Respiratory Tract Infections: A Promising Tool Against COVID-19 and Its Co-Infections. Laser Phys. Lett. 17, 1–9. doi: 10.1088/1612-202X/ab95a9
Dominguez, E. G., Zarnowski, R., Choy, H. L., Zhao, M., Sanchez, H., Nett, J. E., et al. (2019). Conserved Role for Biofilm Matrix Polysaccharides in Candida Auris Drug Resistance. mSphere 4, e00680–1, e00680-18. doi: 10.1128/mSphereDirect.00680-18
Eyre, D. W., Sheppard, A. E., Madder, H., Moir, I., Moroney, R., Quan, T. P., et al. (2018). A Candida Auris Outbreak and Its Control in an Intensive Care Setting. N. Engl. J. Med. 379, 1322–1331. doi: 10.1056/NEJMoa1714373
Ferrer-Espada, R., Wang, Y., Goh, X. S., Dai, T. (2019). Antimicrobial Blue Light Inactivation of Microbial Isolates in Biofilms. Lasers Surg. Med. 52, 472–478. doi: 10.1002/lsm.23159
Forsberg, K., Woodworth, K., Walters, M., Berkow, E. L., Jackson, B., Chiller, T., et al. (2019). Candida Auris: The Recent Emergence of a Multidrug-Resistant Fungal Pathogen. Med. Mycol. 57, 1–12. doi: 10.1093/mmy/myy054
Fox, E. P., Singh-Babak, S. D., Hartooni, N., Nobile, C. J. (2015). “Biofilms and Antifungal Resistance,” in Antifungals: From Genomics to Resistance and the Development of Novel Agents (Norfolk, United Kingdom: Caister Academic Press), 71–90. doi: 10.21775/9781910190012.04
Garcia-Bustos, V., Salavert, M., Ruiz-Gaitán, A. C., Cabañero-Navalon, M. D., Sigona-Giangreco, I. A., Pemán, J. (2020). A Clinical Predictive Model of Candidaemia by Candida Auris in Previously Colonized Critically Ill Patients. Clin. Microbiol. Infect. 26, 1507–1513. doi: 10.1016/j.cmi.2020.02.001
Gardlo, K., Horska, Z., Enk, C. D., Rauch, L., Megahed, M., Ruzicka, T., et al. (2003). Treatment of Cutaneous Leishmaniasis by Photodynamic Therapy. J. Am. Acad. Dermatol. 48, 893–896. doi: 10.1067/mjd.2003.218
Guffey, J. S., Payne, W., Buchanan, B., Daugherty, J., Meurer, L., Hensley, P. (2017). Susceptibility of Trichophyton Mentagrophytes to Visible Light Wavelengths. Adv. Skin Wound Care 30, 218–222. doi: 10.1097/01.ASW.0000512271.19164.ef
Gulati, M., Lohse, M. B., Ennis, C. L., Gonzalez, R. E., Perry, A. M., Bapat, P., et al. (2018). In Vitro Culturing and Screening of Candida Albicans Biofilms. Curr. Protoc. Microbiol. 50, 1–62. doi: 10.1002/cpmc.60
Gulati, M., Nobile, C. J. (2016). Candida Albicans Biofilms: Development, Regulation, and Molecular Mechanisms. Microbes Infect. 18, 310–321. doi: 10.1016/j.micinf.2016.01.002
Gwynne, P. J., Gallagher, M. P. (2018). Light as a Broad-Spectrum Antimicrobial. Antimicrob. Front. Microbiol. 9, 119. doi: 10.3389/fmicb.2018.00119
Halstead, F. D., Thwaite, J. E., Burt, R., Laws, T. R., Raguse, M., Moeller, R., et al. (2016). Antibacterial Activity of Blue Light Against Nosocomial Wound Pathogens Growing Planktonically and as Mature Biofilms. Appl. Environ. Microbiol. 82, 4006–4016. doi: 10.1128/AEM.00756-16
Hamblin, M. R., Hasan, T. (2004). Photodynamic Therapy: A New Antimicrobial Approach to Infectious Disease? Photochem. Photobiol. Sci. 3, 436–450. doi: 10.1039/b311900a
Horton, M. V., Nett, J. E. (2020). Candida Auris Infection and Biofilm Formation: Going Beyond the Surface. Curr. Clin. Microbiol. Rep. 7, 51–56. doi: 10.1007/s40588-020-00143-7
Huh, S. Y., Na, J. I., Huh, C. H., Park, K. C. (2012). The Effect of Photodynamic Therapy Using Indole-3-Acetic Acid and Green Light on Acne Vulgaris. Ann. Dermatol. 24, 56–60. doi: 10.5021/ad.2012.24.1.56
Jin, Y., Zhang, T., Samaranayake, Y. H., Fang, H. H. P., Yip, H. K., Samaranayake, L. P. (2005). The Use of New Probes and Stains for Improved Assessment of Cell Viability and Extracellular Polymeric Substances in Candida Albicans Biofilms. Mycopathologia 159, 353–360. doi: 10.1007/s11046-004-6987-7
Jockusch, S., Leet, D., Turro, N. J., Leonardt, E. F. (1996). Photo-Induced Inactivation of Viruses: Adsorption of Methylene Blue, Thionine, and Thiopyronine on Qb Bacteriophage. Proc.Natl.Acad.Sci 93, 7446–7451. doi: 10.1073/pnas.93.15.7446
Kean, R., Delaney, C., Sherry, L., Borman, A., Johnson, E. M., Richardson, M. D., et al. (2018). Transcriptome Assembly and Profiling of Candida Auris Reveals Novel Insights Into Biofilm-Mediated Resistance. mSphere 3, 1–14. doi: 10.1128/msphere.00334-18
Kharkwal, G. B., Sharma, S. K., Huang, Y. Y., Dai, T., Hamblin, M. R. (2011). Photodynamic Therapy for Infections: Clinical Applications. Lasers Surg. Med. 43, 755–767. doi: 10.1002/lsm.21080
Kim, M., Jung, Y., Park, H. J. (2015). Topical PDT in the Treatment of Benign Skin Diseases: Principles and New Applications. Int. J. Mol. Sci. 16, 23259–23278. doi: 10.3390/ijms161023259
Kolter, R., Greenberg, E. P. (2006). The Superficial Life of Microbes. Nature 441, 300–302. doi: 10.1038/441300a
Kuhn, D. M., George, T., Chandra, J., Mukherjee, P. K., Ghannoum, M. A. (2002). Antifungal Susceptibility of Candida Biofilms: Unique Efficacy of Amphotericin B Lipid Formulations and Echinocandins. Antimicrob. Agents Chemother. 46, 1773–1780. doi: 10.1128/AAC.46.6.1773-1780.2002
Larkin, E., Hager, C., Chandra, J., Mukherjee, P. K., Retuerto, M., Salem, I., et al. (2017). The Emerging Pathogen Candida Auris: Growth Phenotype, Virulence Factors, Activity of Antifungals, and Effect of SCY-078, a Novel Glucan Synthesis Inhibitor, on Growth Morphology and Biofilm Formation. Antimicrob. Agents Chemother. 61, 1–13. doi: 10.1128/AAC.02396-16
Lewis, K. (2010). Persister Cells. Annu. Rev. Microbiol. 64, 357–372. doi: 10.1146/annurev.micro.112408.134306
Lockhart, S. R., Etienne, K. A., Vallabhaneni, S., Farooqi, J., Chowdhary, A., Govender, N. P., et al. (2017). Simultaneous Emergence of Multidrug-Resistant Candida Auris on 3 Continents Confirmed by Whole-Genome Sequencing and Epidemiological Analyses. Clin. Infect. Dis. 64, 134–140. doi: 10.1093/cid/ciw691
Lohse, M. B., Gulati, M., Valle Arevalo, A., Fishburn, A., Johnson, A. D., Nobile, C. J. (2017). Assessment and Optimizations of Candida Albicans In Vitro Biofilm Assays. Antimicrob. Agents Chemother. 61, e02749–e02716. doi: 10.1128/aac.02749-16
López, D., Vlamakis, H., Kolter, R. (2010). Biofilms. Cold Spring Harb. Perspect. Biol. 2, 1–11. doi: 10.1101/cshperspect.a000398
Lyon, J. P., Moreira, L. M., de Moraes, P. C. G., dos Santos, F. V., de Resende, M. A. (2011). Photodynamic Therapy for Pathogenic Fungi. Mycoses 54, 1–7. doi: 10.1111/j.1439-0507.2010.01966.x
Magnasco, L., Mikulska, M., Giacobbe, D. R., Taramasso, L., Vena, A., Dentone, C., et al. (2021). Spread of Carbapenem-Resistant Gram-Negatives and Candida Auris During the Covid-19 Pandemic in Critically Ill Patients: One Step Back in Antimicrobial Stewardship? Microorganisms 9, 1–10. doi: 10.3390/microorganisms9010095
Mah, T.-F. C., O’Toole, G. A. (2001). Mechanisms of Biofilm Resistance to Antimicrobial Agents. Trends Microbiol. 9, 34–39. doi: 10.1016/S0966-842X(00)01913-2
Maisch, T. (2015). Resistance in Antimicrobial Photodynamic Inactivation of Bacteria. Photochem. Photobiol. Sci. 14, 1518–1526. doi: 10.1039/c5pp00037h
Marotti, J., Aranha, A. C. C., Eduardo, C. D. P., Ribeiro, M. S. (2009). Photodynamic Therapy can be Effective as a Treatment for Herpes Simplex Labialis. Photomed. Laser Surg. 27, 357–363. doi: 10.1089/pho.2008.2268
Meyers, E., Miraglia, G. J., Smith, D. A., Basch, H., Pansy, F. E., Trejo, W. H., et al. (1968). Biological Characterization of Prasinomycin, a Phosphorus-Containing Antibiotic. Appl. Microbiol. 16, 603–608. doi: 10.1128/am.16.4.603-608.1968
Moorhead, S., Hons, B., Maclean, M., Macgregor, S. J., Anderson, J. G. (2016). Comparative Sensitivity of Trichophyton and Aspergillus Conidia to Inactivation by Violet-Blue Light Exposure. Photomed. Laser Surg. 34, 36–41. doi: 10.1089/pho.2015.3922
Morales-López, S. E., Parra-Giraldo, C. M., Ceballos-Garzón, A., Martínez, H. P., Rodríguez, G. J., Álvarez-Moreno, C. A., et al. (2017). Invasive Infections With Multidrug-Resistant Yeast Candida Auris, Colombia. Emerg. Infect. Dis. 23, 162–164. doi: 10.3201/eid2301.161497
Mukherjee, P. K., Chandra, J. (2015). Candida Biofilms: Development, Architecture, and Resistance. Microbiol. Spectr. 3, 1–24. doi: 10.1128/microbiolspec.MB-0020-2015
Murdoch, L. E., McKenzie, K., Maclean, M., MacGregor, S. J., Anderson, J. G. (2013). Lethal Effects of High-Intensity Violet 405-Nm Light on Saccharomyces Cerevisiae, Candida Albicans, and on Dormant and Germinating Spores of Aspergillus Niger. Fungal Biol. 117, 519–527. doi: 10.1016/j.funbio.2013.05.004
Navarro-arias, M. J., Hernández-chávez, M. J., García-carnero, L., Amezcua-hernández, D., Lozoya-Pérez, N., Estrada-Mata, E., et al. (2019). Differential Recognition of Candida Tropicalis, Candida Guilliermondii, Candida Krusei, and Candida Auris by Human Innate Immune Cells. Infect. Drug Resist. 12, 783–794. doi: 10.2147/IDR.S197531
Nobile, C. J., Johnson, A. D. (2015). Candida Albicans Biofilms and Human Disease. Annu. Rev. Microbiol. 69, 71–92. doi: 10.1146/annurev-micro-091014-104330
Odds, F. C., Brown, A. J. P., Gow, N. A. R. (2003). Antifungal Agents: Mechanisms of Action. Trends Microbiol. 11, 272–279. doi: 10.1016/S0966-842X(03)00117-3
Oppezzo, O. J., Forte Giacobone, A. F. (2018). Lethal Effect of Photodynamic Treatment on Persister Bacteria. Photochem. Photobiol. 94, 186–189. doi: 10.1111/php.12843
Osei Sekyere, J. (2018). Candida Auris: A Systematic Review and Meta-Analysis of Current Updates on an Emerging Multidrug-Resistant Pathogen. Microbiol. Open 7, e578. doi: 10.1002/mbo3.578
Ostrowsky, B., Greenko, J., Adams, E., Quinn, M., O’Brien, B., Chaturvedi, V., et al. (2020). Candida Auris Isolates Resistant to Three Classes of Antifungal Medications - New Yor. Morb. Mortal. Wkly. Rep. 69, 6–9. doi: 10.15585/mmwr.mm6901a2
Panariello, B. H. D., Garcia, B. A., Duarte, S. (2019). Daily Phototherapy With Red Light to Regulate Candida Albicans Biofilm Growth. J. Vis. Exp. 146, e59326. doi: 10.3791/59326
Piedrahita, C. T., Cadnum, J. L., Jencson, A. L., Shaikh, A. A., Ghannoum, M. A., Donskey, C. J. (2017). Environmental Surfaces in Healthcare Facilities are a Potential Source for Transmission of Candida Auris and Other Candida Species. Infect. Control Hosp. Epidemiol. 38, 1107–1109. doi: 10.1017/ice.2017.127
Pinto, A. P., Rosseti, I. B., Carvalho, M. L., da Silva, B. G. M., Alberto-Silva, C., Costa, M. S., et al. (2018). Photodynamic Antimicrobial Chemotherapy (PACT), Using Toluidine Blue O Inhibits the Viability of Biofilm Produced by Candida Albicans at Different Stages of Development. Photodiagnosis Photodyn. Ther. 21, 182–189. doi: 10.1016/j.pdpdt.2017.12.001
Prasad, R., Shah, A. H., Rawal, M. K., Prasad, R., Shah, A. H., Rawal, M. K. (2016). “Mechanism of Action and Drug Resistance,” in Yeast Membrane Transport. Adv. Exp. Med. Biol. 14, 327–349. doi: 10.1007/978-3-319-25304-6_14
Prestel, C., Anderson, E., Forsberg, K., Lyman, M., de Perio, M. A., Kuhar, D., et al. (2021). Candida Auris Outbreak in a COVID-19 Specialty Care Unit - Florida, July–August 2020. MMWR. Morb. Mortal. Wkly. Rep. 70, 56–57. doi: 10.15585/mmwr.mm7002e3
Rodriguez, J. Y., Le Pape, P., Lopez, O., Esquea, K., Labiosa, A. L., Moreno-Alvarez, C. (2020). Candida Auris: A Latent Threat to Critically III Patients With Coronavirus Disease 2019. Clin. Infect. Dis. Corresp. 1595, 1–2. doi: 10.1093/cid/ciaa1595/5929667
Roemer, T., Krysan, D. J. (2014). Antifungal Drug Development: Challenges, Unmet Clinical Needs, and New Approaches. Cold Spring Harb. Perspect. Med. 4, 1–15. doi: 10.1101/cshperspect.a019703
Romano, R. A., Pratavieira, S., Silva, A. P., Kurachi, C., Guimarães, F. (2017). Light-Driven Photosensitizer Uptake Increases Candida Albicans Photodynamic Inactivation. J. Biophotonics 10, 1538–1546. doi: 10.1002/jbio.201600309
Romera, D., Aguilera-Correa, J. J., Gadea, I., Viñuela-Sandoval, L., García-Rodríguez, J., Esteban, J. (2019). Candida Auris: A Comparison Between Planktonic and Biofilm Susceptibility to Antifungal Drugs. J. Med. Microbiol. 68, 1353–1358. doi: 10.1099/jmm.0.001036
Saris, K., Meis, J. F., Voss, A. (2018). Candida Auris. Curr. Opin. Infect. Dis. 31, 334–340. doi: 10.1097/QCO.0000000000000469
Satoh, K., Makimura, K., Hasumi, Y., Nishiyama, Y., Uchida, K., Yamaguchi, H. (2009). Candida Auris Sp. Nov., a Novel Ascomycetous Yeast Isolated From the External Ear Canal of an Inpatient in a Japanese Hospital. Microbiol. Immunol. 53, 41–44. doi: 10.1111/j.1348-0421.2008.00083.x
Shastri, P. S., Shankarnarayan, S. A., Oberoi, J., Rudramurthy, S. M., Wattal, C., Chakrabarti, A. (2020). Candida Auris Candidaemia in an Intensive Care Unit – Prospective Observational Study to Evaluate Epidemiology, Risk Factors, and Outcome. J. Crit. Care 57, 42–48. doi: 10.1016/j.jcrc.2020.01.004
Sherry, L., Ramage, G., Kean, R., Borman, A., Johnson, E. M., Richardson, M. D., et al. (2017). Biofilm-Forming Capability of Highly Virulent, Multidrug-Resistant Candida Auris. Emerg. Infect. Dis. 23, 328–331. doi: 10.3201/eid2302.161320
Spivak, E. S., Hanson, K. E. (2018). Candida Auris: An Emerging Fungal Pathogen. J. Clin. Microbiol. 56, e01588–e01517. doi: 10.1128/JCM.01588-17
Steele, E. J., Gorczynski, R. M., Lindley, R. A., Tokoro, G., Temple, R., Chandra Wickramasinghe, N., et al. (2020). Origin of New Emergent Coronavirus and Candida Fungal Diseases-Terrestrial or Cosmic? Adv. Genet. 106, 75–100. doi: 10.1016/bs.adgen.2020.04.002
Stewart, P. S. (2002). Mechanisms of Antibiotic Resistance in Bacterial Biofilms. Int. J. Med. Microbiol. 292, 107–113. doi: 10.1078/1438-4221-00196
Stokes, J. M., Lopatkin, A. J., Lobritz, M. A., Collins, J. J. (2019). Bacterial Metabolism and Antibiotic Efficacy. Cell Metab. 30, 251–259. doi: 10.1016/j.cmet.2019.06.009
Svyatchenko, V. A., Nikonov, S. D., Mayorov, A. P., Gelfond, M. L., Loktev, V. B. (2021). Antiviral Photodynamic Therapy: Inactivation and Inhibition of SARS-CoV-2 In Vitro Using Methylene Blue and Radachlorin. Photodiagnosis Photodyn. Ther. 33, 353–60. doi: 10.1016/j.pdpdt.2020.102112
Taff, H. T., Mitchell, K. F., Edward, J. A., Andes, D. R. (2013). Mechanisms of Candida Albicans Biofilm Drug Resistance. Future Microbiol. 8, 1325–1337. doi: 10.2217/fmb.13.101
Tan, J., Liu, Z., Sun, Y., Yang, L., Gao, L. (2019). Inhibitory Effects of Photodynamic Inactivation on Planktonic Cells and Biofilms of Candida Auris. Mycopathologia 184, 525–531. doi: 10.1007/s11046-019-00352-9
Teixeira, N., De Sousa, A., Santos, M. F., Gomes, R. C., Brandino, H. E., Martinez, R., et al. (2015). Blue Laser Inhibits Bacterial Growth of Staphylococcus Aureus, Escherichia Coli, and Pseudomonas Aeruginosa. Photomed. Laser Ther. 33, 278–282. doi: 10.1089/pho.2014.3854
Trzaska, W. J., Wrigley, H. E., Thwaite, J. E., May, R. C. (2017). Species-Specific Antifungal Activity of Blue Light. Sci. Rep. 7, 1–7. doi: 10.1038/s41598-017-05000-0
Vatansever, F., de Melo, W. C. M. A., Avci, P., Vecchio, D., Sadasivam, M., Gupta, A., et al. (2013). Antimicrobial Strategies Centered Around Reactive Oxygen Species - Bactericidal Antibiotics, Photodynamic Therapy, and Beyond. FEMS Microbiol. Rev. 37, 955–989. doi: 10.1111/1574-6976.12026
Villanueva-Lozano, H., Treviño-Rangel, R., de, J., González, G. M., Ramírez-Elizondo, M. T., Lara-Medrano, R., et al. (2021). Outbreak of Candida Auris Infection in a COVID-19 Hospital in Mexico. Clin. Microbiol. Infect 27, 813–816. doi: 10.1016/j.cmi.2020.12.030
Vural, E., Winfield, H. L., Shingleton, A. W., Horn, T. D., Shafirstein, G. (2008). The Effects of Laser Irradiation on Trichophyton Rubrum Growth. Lasers Med. Sci. 23, 349–353. doi: 10.1007/s10103-007-0492-4
Wainwright, M. (2004). Photoinactivation of Viruses. Photochem. Photobiol. Sci. 3, 406–411. doi: 10.1039/b311903n
Wainwright, M., Maisch, T., Nonell, S., Plaetzer, K., Almeida, A., Tegos, G. P., et al. (2017). Photoantimicrobials—are We Afraid of the Light? Lancet Infect. Dis. 17, e49–e55. doi: 10.1016/S1473-3099(16)30268-7
Wainwright, M., Phoenix, D., Laycock, S., Wareing, D., Wright, P. (1998). Photobactericidal Activity of Phenothiazinium Dyes Against Methicillin-Resistant Strains of Staphylococcus Aureus. FEMS Microbiol. Lett. 160, 177–181. doi: 10.1111/j.1574-6968.1998.tb12908.x
Wang, Y., Wang, Y., Wang, Y., Murray, C. K., Hamblin, M. R., Hooper, D. C., et al. (2017). Antimicrobial Blue Light Inactivation of Pathogenic Microbes: State of the Art. Drug Resist. Update 33–35, 1–22. doi: 10.1016/j.drup.2017.10.002
Wang, C., Yang, Z., Peng, Y., Guo, Y., Yao, M., Dong, J. (2018). Application of 460 Nm Visible Light for the Elimination of Candida Albicans In Vitro and In Vivo. Mol. Med. Rep. 18, 2017–2026. doi: 10.3892/mmr.2018.9196
Welsh, R. M., Bentz, M. L., Shams, A., Houston, H., Lyons, A., Rose, L. J., et al. (2017). Survival, Persistence, and Isolation of the Emerging Multidrug-Resistant Pathogenic Yeast Candida Auris on a Plastic Health Care Surface. J. Clin. Microbiol 55, 2996–3005. doi: 10.1128/JCM.00921-17
Wiehe, A., O’brien, J. M., Senge, M. O. (2019). Trends and Targets in Antiviral Phototherapy. Photochem. Photobiol. Sci. 18, 2565. doi: 10.1039/c9pp00211a
Wimpenny, J., Manz, W., Szewzyk, U. (2000). Heterogeneity in Biofilms. FEMS Microbiol. Rev. 24, 661–671. doi: 10.1111/j.1574-6976.2000.tb00565.x
Yan, L., Xia, K., Yu, Y., Miliakos, A., Chaturvedi, S., Zhang, F., et al. (2020). Unique Cell Surface Mannan of Yeast Pathogen Candida Auris With Selective Binding to Igg. ACS Infect. Dis. 6, 1018–1031. doi: 10.1021/acsinfecdis.9b00450
Zamith-Miranda, D., Heyman, H. M., Cleare, L. G., Couvillion, S. P., Clair, G. C., Bredeweg, E. L., et al. (2019). Multi-Omics Signature of Candida Auris, an Emerging and Multidrug-Resistant Pathogen. mSystems 4, 1–14. doi: 10.1128/msystems.00257-19
Zarnowski, R., Westler, W. M., Lacmbouh, G. A., Marita, J. M., Bothe, J. R., Bernhardt, J., et al. (2014). Novel Entries in a Fungal Biofilm Matrix Encyclopedia. MBio 5, 1–13. doi: 10.1128/mBio.01333-14
Zhang, Y., Zhu, Y., Chen, J., Wang, Y., Sherwood, M. E., Murray, C. K., et al. (2016). Antimicrobial Blue Light Inactivation of Candida Albicans: In Vitro and In Vivo Studies. Virulence 7, 536–545. doi: 10.1080/21505594.2016.1155015
Keywords: Candida auris biofilms, drug resistance, red, green and blue (RGB) visible lights, photodynamic therapy, photosensitizing compounds, reactive oxygen species (ROS), non-drug therapeutic strategies, non-drug antifungal strategies
Citation: Bapat PS and Nobile CJ (2021) Photodynamic Therapy Is Effective Against Candida auris Biofilms. Front. Cell. Infect. Microbiol. 11:713092. doi: 10.3389/fcimb.2021.713092
Received: 21 May 2021; Accepted: 13 August 2021;
Published: 03 September 2021.
Edited by:
Leah Elizabeth Cowen, University of Toronto, CanadaReviewed by:
Matt Anderson, The Ohio State University, United StatesCopyright © 2021 Bapat and Nobile. This is an open-access article distributed under the terms of the Creative Commons Attribution License (CC BY). The use, distribution or reproduction in other forums is permitted, provided the original author(s) and the copyright owner(s) are credited and that the original publication in this journal is cited, in accordance with accepted academic practice. No use, distribution or reproduction is permitted which does not comply with these terms.
*Correspondence: Clarissa J. Nobile, Y25vYmlsZUB1Y21lcmNlZC5lZHU=
Disclaimer: All claims expressed in this article are solely those of the authors and do not necessarily represent those of their affiliated organizations, or those of the publisher, the editors and the reviewers. Any product that may be evaluated in this article or claim that may be made by its manufacturer is not guaranteed or endorsed by the publisher.
Research integrity at Frontiers
Learn more about the work of our research integrity team to safeguard the quality of each article we publish.