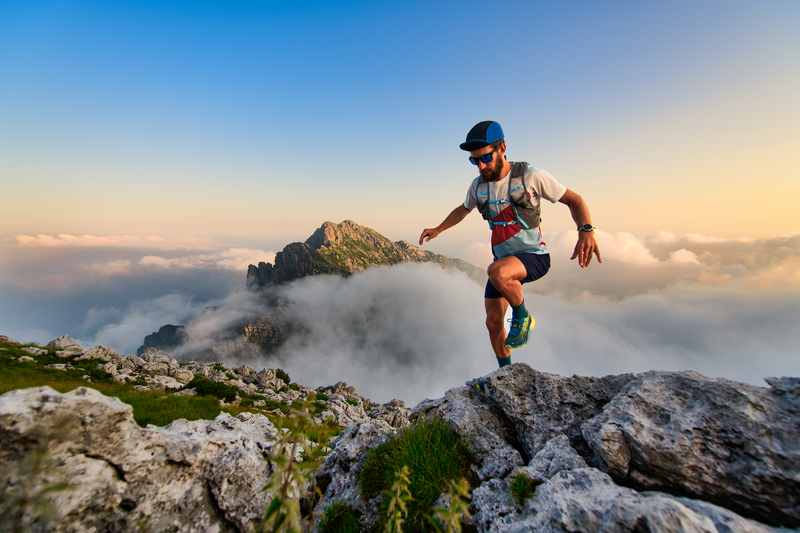
94% of researchers rate our articles as excellent or good
Learn more about the work of our research integrity team to safeguard the quality of each article we publish.
Find out more
REVIEW article
Front. Cell. Infect. Microbiol. , 21 September 2021
Sec. Microbiome in Health and Disease
Volume 11 - 2021 | https://doi.org/10.3389/fcimb.2021.711055
Fecal microbiota transplantation (FMT) has been widely recognized as an approach to determine the microbiome’s causal role in gut dysbiosis-related disease models and as a novel disease-modifying therapy. Despite potential beneficial FMT results in various disease models, there is a variation and complexity in procedural agreement among research groups for performing FMT. The viability of the microbiome in feces and its successful transfer depends on various aspects of donors, recipients, and lab settings. This review focuses on the technical practices of FMT in animal studies. We first document crucial factors required for collecting, handling, and processing donor fecal microbiota for FMT. Then, we detail the description of gut microbiota depletion methods, FMT dosages, and routes of FMT administrations in recipients. In the end, we describe assessments of success rates of FMT with sustainability. It is critical to work under the anaerobic condition to preserve as much of the viability of bacteria. Utilization of germ- free mice or depletion of recipient gut microbiota by antibiotics or polyethylene glycol are two common recipient preparation approaches to achieve better engraftment. Oral-gastric gavage preferred by most researchers for fast and effective administration of FMT in mice. Overall, this review highlights various methods that may lead to developing the standard and reproducible protocol for FMT.
The human microbiota harbors an extensive reservoir of genetic information encoded within ~10 to 100 trillion microbial cells present throughout the human body. Most of this genetic information is present within the human gastrointestinal tract and is commonly referred to as our “second genome”. The ~ 1100 gut microbial species are central to the proper development and function of numerous physiological processes such as sustaining homeostasis in gut barrier integrity (Shi et al., 2017), nutrition metabolism (Nicholson et al., 2012), host immunity (Olszak et al., 2012) and the regulation of neuropsychological behaviors (Diaz Heijtz et al., 2011).
Microbiome research has made tremendous strides in the past decade. After the initial characterization of the microbiome in the healthy population from the Human Microbiome Project (Human Microbiome Project, 2012a; Human Microbiome Project, 2012b), accumulating data from animal and human studies have advocated that gut microbiome dysbiosis (alteration) is associated with onset, development, and progression of gastrointestinal (Chang and Lin, 2016), metabolic (Dabke et al., 2019), autoimmune (Xu et al., 2019), neurologic (Felice and O’Mahony, 2017), and psychiatric diseases (Cenit et al., 2017). Research is rapidly moving from association to causation by experimentally manipulating the gut microbiome to precisely understand the contribution and underlying mechanisms of microbiome in disease. Fecal microbiota transplantation (FMT) is one of the most commonly used approaches for investigating the causal connection between the gut microbiome and diseases in animal models. FMT has shown that the transplanted microbiome can influence physiological functions and alleviate a wide range of diseases that include Clostridium Difficile infection (CDI), inflammatory bowel diseases (IBD), irritable bowel syndrome (IBS), obesity, diabetes, aging, cancer, autism, multiple sclerosis (Evrensel and Ceylan, 2016), Parkinson’s disease (Sun et al., 2018), anorexia nervosa, food allergies and neurological disorders (Bajaj et al., 2017; Kang et al., 2017; Wang et al., 2018a) in animal models. These findings have spearheaded the advancement of targeted microbiome therapeutic using FMT for the treatment of human diseases.
Although FMTs are widely used for studying causality in disease models, standard protocols for relevant FMT procedures do not exist for even basic procedures such as donor stool preparation (selection, storage and processing), delivery mode, dosage, duration of administration and recipient preparation. Such experimental variation complicates the interpretation of results across studies. In this review, we provide a detailed summary of the techniques currently utilized for FMTs in animal studies including FMT from mouse to mouse and human to mouse) (Figure 1) and call for standardizing protocols to increase rigor and reproducibility of critical approach for microbiome research and therapeutics.
Figure 1 General procedures for FMT in murine. Step-wised procedures of FMT from human or mice donors to mice recipients. This includes preparation of donor stool (A) and preparation of recipients (B). Preparation of donor stool is recommended in anaerobic environment. Raw stools will be diluted, homogenized, filtered, followed by centrifuge to remove large particles. Processed stool can be stored in long term in -800C. Recipient mice will treated with antibiotics or PEG to deplete the majority of the gut microbiota, providing an ecological niche for colonization of transplanted stool microbiota.
Several animal studies have demonstrated the benefits of FMT in preventing and treating diseases. Lawley’s research group reported that FMT from a healthy donor mouse into recipient mouse with CDI led to resolution of illness and infection (Lawley et al., 2012). A study by Palma et al. reported altered gastrointestinal function, activation of innate immunity, and anxiety-like behavior in germ-free (GF) mice treated with feces matter of diarrheal IBS patients (De Palma et al., 2017). FMT improves colitis symptoms in the mouse model by upregulating aryl hydrocarbon receptors (Wei et al., 2018). Ferrere and his colleagues reported the prevention of alcoholic liver disease in mice when treated with the fecal transplant of alcohol-fed donor mice resistant to alcoholic liver disease (Ferrere et al., 2017). A recent study reported decrement or limitation in the accumulation of p-cresyl sulfate and uremic toxins after FMT from healthy mice into mice with chronic kidney disease (Barba et al., 2020). FMT also reverses the course of lethal sepsis by improving pathogen clearance through the restoration of host immunity in an interferon regulatory factor 3-dependent method (Kim et al., 2020). FMT of resveratrol-fed mice enhanced glucose homeostasis and cardiovascular symptoms and reduced the high blood pressure in a mouse model of hypertension (Kim et al., 2018b). FMT also alleviates high-fat diet-induced steatohepatitis in mice via restoring the beneficial regulation of gut microbiota (Zhou et al., 2017). FMT might lessen obesity and digestion in both humans and mice (Ridaura et al., 2013). Bárcena’s research group found that FMT from wild-type mice improved health span and life expectancy in progeroid mice. The re-establishment of secondary bile acids is a potential mechanism for restoring a healthy microbiome (Barcena et al., 2019). A number of reports support a protecting role of specific FMT against development and progression of melanoma (Le Bastard et al., 2018; Wang et al., 2019) possibly enhancing anti-tumor immune responses mediated by increased dendritic cells activity, and improved priming of CD8 T cells in and around the tumor microenvironment.
FMT also shows encouraging results in neuropsychological diseases. FMT from resilient rats significantly reduced the pain and depression in antibiotic administered pseudo-germ-free mice (Yang et al., 2019). A recent report advocated FMT of youth gut microbiome in aged mice to reverse the weak stroke recovery (Lee et al., 2020). Remarkably, FMT inhibited the TLR4/TBK1/NF-κB/TNF-a signaling pathway, reduced gut microbial dysbiosis, decreased the activation of brain microglia and astrocytes, and alleviated physical impairment in Parkinson’s disease mice (Sun et al., 2018; Sun et al., 2019). Multiple sclerosis derived gut microbiome contains factors that precipitate numerous sclerosis in a humanized mouse model (Berer et al., 2017). FMT also contains unidentified microbes can be potentially pathogenic (Elinav et al., 2011; Food and Drug Administration, 2020) that exacerbate illness or even be fatal for recipients. Further, FMT might induce obesity as metabolic syndrome in recipients as a long-term effect (Ridaura et al., 2013). FMT can also be the risk of infection or overt immune reaction in immunocompromised recipients (Carlson, 2020). These reports suggest that FMT also has some limitations that need to be evaluated for its continued development.
Collection timing of stool is imperative given reports signifying the circadian rhythms of the abundance of gut microbiota. Diurnal rhythmicity reported in 20-83% of mice gut microbiota (Zarrinpar et al., 2014). Around 20% of human gut microbiota displayed diurnal fluctuations in average abundance (Thaiss et al., 2014). Clostridiales, Lactobacillales, and Bacteroidales, which comprise 60% of total gut microbiota, displayed acrophase and bathyphase in abundance throughout the 24-hour cycle. Noticeably, the bacterial rhythmicity was independent of cage or housing conditions (Thaiss et al., 2014).
Liang and his research group found that bacterial load was highest during night time (11:00 PM), and the lowest load was reported early morning (7:00 AM). Bacterial load steadily increased from the early light phase and decreased toward the late-night dark stage (midnight). The average higher richness (60-66%) of Bacteroidetes was noted at 11:00 PM and 11:00 AM and lower at other time points. The average higher richness (45%) of Firmicutes was noted at 3:00 AM and 7:00 AM, and the lowest richness (29%) was recorded at 11:00 PM. They also reported male mice had higher bacterial load than females, though females had higher bacterial rhythms (Liang et al., 2015). Some reports endorsed to collect mice fecal samples in the early morning between 7:00 AM to 11:00 AM (Ericsson et al., 2018) or afternoon between 3:00 PM to 5:00 PM to reduce potential circadian rhythm effects (Wang et al., 2018b). Due to mice nocturnal feeding actions, it will take less time to excrete the mice and collect the feces in the morning. For human donors, individuals delivered fresh stool one month after health screening (Bakken et al., 2011), however no specific rationale for stool collection timing was provided.
Ericsson and his colleagues conducted an FMT study in mice with frozen and thawed stool in contrast with fresh stool to ensure microbes’ viability after freezing. They stated that frozen feces and fresh cecal contents both performed comparably to fresh feces as the model source for FMT (Ericsson et al., 2017). Using frozen stool permit researchers to freeze and bank multiple samples for later use. However, the number of freeze-and-thaw cycles has been described to influence microbial populations (Sergeant et al., 2012). It will be a standard lab practice to make aliquots of feces to prevent multiple freezes and thaw cycles (Thomas et al., 2015).
Fecal samples kept at room temperature for up to twenty-four hours hold a microbiota comparable in composition to a fresh sample. Storage of feces at room temperature over 24 hours increases the abundance of Actinobacteria and decreases the abundance of Firmicutes (Choo et al., 2015). Feces sample cooled at 4°C did not vary significantly in the microbial community of the control feces samples stored at −80°C for over twenty-four hours period (Tedjo et al., 2015). However, metabolic disease feces or IBS fecal samples are less stable throughout storage at room temperature (Roka et al., 2007; Tana et al., 2010). It is tempting to speculate that higher levels of proteases and acids may result in the rapid degradation of fecal DNA. Short‐term storage of fecal material at −20°C for one month exhibited similar enteric colonization ability frozen at −80°C (Lauber et al., 2010). Long‐span storage of transplanted fecal material at −20°C for more than one month can result in uncertainty of the clinical outcome following FMT (Takahashi et al., 2019). During long-term storage at -80°C, fecal materials kept at up to six months retain a microbiota comparable in composition to a fresh sample (Carroll et al., 2012). There were reports showing that feces can maintain a steady microbial population for up to 2 years when stored at -80°C (Shaw et al., 2016). Freezing of feces at −20°C for around two months has been shown to increase the ratio of Firmicutes to Bacteroidetes (Bahl et al., 2012). Collectively, storing of stool samples streamlines the practical aspects of FMT with effectiveness or safety.
Conservation of fecal transplants is essential to give rapid access to fecal material whose safety has been tested upstream in an allogenic context. Cryoprotection before freezing is a crucial step to preserve bacterial cell viability and integrity (Fadda, 2020). The currently recommended protocol advocates 10% glycerol for the conservation of frozen fecal samples (Cammarota et al., 2014). Glycerol is selected due to its low toxicity to bacterial cells intended for FMT (Hamilton et al., 2012). Storage of stool samples in normal saline causes a decline in all cultured microbiota compared to 10% glycerol solution except coliforms and Lactobacilli. A 10% glycerol is not appropriate for downstream lyophilization as it leads to a dry and sticky product. Cryoprotectants such as maltodextrin and trehalose can be good alternatives of glycerol+ dimethyl sulfoxide as they increase medium viscosity and limit ice crystallization and osmotic disparity during freezing. Cocktail recipes in the ratios of 3:1 maltodextrin and 1:3 trehalose in NaCl retained > 60% microbial viability in stored stool over a three-month period, a proportion which is little less to freshly prepared stool (Béal and Fonseca, 2015; Burz et al., 2019). It advised to include feces preservation method to prevent drastic change in viability when stored.
The human gut consists mostly of anaerobic bacteria and sensitive to oxygen exposure. Anaerobic bacteria outnumber aerobic bacteria by a factor of 1000:1 (Finegold, 1995). Improper handling of the stool may lose the variability of many anaerobic bacteria, thus affects the effect of FMT. Approximately 61.69% of cells were alive when samples were exposed to oxygen <2 min, and cell viability decreased to 55.52% after exposure to oxygen for 90 minutes (Bellali et al., 2019). Under exposure to oxygen for < 2 minutes viability was reduced to 49% (Ben-Amor et al., 2005). Another report stated anaerobic microbes viability ~50% when the human stool was exposed to oxygen for 4-5 minutes, falling to 0.1% post 40 minutes, and no cell was stayed alive after 2 hours (Brusa et al., 1989). Papanicolas and his colleagues found around 50% bacterial viability under stringent anaerobic conditions (Papanicolas et al., 2019). Homogenization in ambient air or freeze-thaw sequence reduced microbial viability to 19% and 23%, correspondingly. Processing of fecal samples in ambient air can be a cause of multi-fold declines in the abundance of Faecalibacterium prausnitzii (12 fold decline), Subdoligranulum variable (8 fold decline), Eubacterium hallii (5 fold decline), and ~3 fold decline in Ruminococcus, Roseburia, lachnospira, and Dorea abundance (Papanicolas et al., 2019). Processing of fecal slurries in ambient air significantly reduced the production of short-chain fatty acids such as butyrate and acetate and the capacity for biosynthesis of important anti-inflammatory metabolites (Papanicolas et al., 2019). However, when the stool sample preserved in maltodextrin and trehalose was rapidly processed with oxygen did not affect the bacterial viability, especially for oxygen-sensitive Firmicutes phylum (Burz et al., 2019). Stool preserved in medium (patent no. N°1H53316 CAS 25 FR) containing antioxidants showed 58.51% cell viability when exposed to oxygen for 90 minutes (Bellali et al., 2019). The understanding of this notion is vital for the improvement of techniques for maintaining the viability of bacteria.
There is frequently some discrepancy in FMT preparation across institutes and labs. However, the overall process is similar and includes mixing feces with a bacteriostatic fluid, eliminating particulate substance, and transporting feces to the recipient. Depending on the dosage and number of FMT, a large quantity of stool can be used for FMT preparation (Thaiss et al., 2014; Barcena et al., 2019; Stebegg et al., 2019; Sun et al., 2019). On the day of FMT, either fresh feces can be diluted in the sterilized phosphate-buffered saline (PBS) to get an estimated fecal suspension (Sivan et al., 2015; Sun et al., 2019). Frozen aliquot fecal suspension can be defrosted for around 10-15 minutes in a water bath at 37°C and appended with L-cysteine amino acid. L-Cysteine is used as a medium, reducing agent to preserve anaerobes. Mixing of feces can also be done with filtered autoclaved water (Ericsson et al., 2017), 200 proof ethanol (Lin et al., 2019) and NaCl as alternatives for PBS. It is recommended FMT should be executed within 6 hours after defrosting. Besides, it is recommended that the dilution ratio of the feces could be adjusted in FMT preparation (Rasmussen et al., 2019). The feces can be diluted approximately 3 times (Stebegg et al., 2019) to 6 times (Barcena et al., 2019) with PBS. As more than half of the stool bacteria are non-cultivable, it is hard to know the number of live bacteria in the donor samples. Vigorously shaking or homogenization of the feces suspension is endorsed to confirm proper mixing (Ericsson et al., 2017). The fecal suspension should be filtered with a filter or gauze to clear away sizeable particulate matter. Further, centrifugation can be performed to pelleting out undissolved solids matter (Liu et al., 2020). Centrifugation speed varies for FMT preparation such as 500 × g for 5 min, (Stebegg et al., 2019) 800 × g for 3 min, (Lai et al., 2018) 2000 ×g for 5 min (Liu et al., 2020) and 5000 × g for 20 min at 20°C. (Zhou et al., 2019) Low-speed centrifugation was probably performed to remove particulate matter, and high-speed centrifugation to concentrate bacteria.
Although trivial differences depend on the specific condition, most organizations prepare the stool centered on a similar protocol. Varieties of stool diluents, such as sterile NaCl, PBS, and skim milk + BHI media (Gough et al., 2011; Brandt and Aroniadis, 2013; Wrzosek et al., 2018) are used to mix feces. The fecal materials’ dilution ratio could be adjusted, considering heterogeneity in the fecal microbes between different individuals or donors (Smits et al., 2013) and advised to be diluted 3–5 times with PBS (Cammarota et al., 2017). Fresh feces should be transported on an icebox to a particular laboratory within 2 hours after excretion (Lee et al., 2016). Feces should be filtered three times through gauze (Mattila et al., 2012), cell strainer, or 0.25 mm stainless steel mesh to remove the undigested and small solid particles in the fecal suspension (Owens et al., 2013). The fecal suspension could be centrifuged 6000 × g for 15 min to remove insolubilized material (Hamilton et al., 2012; Staley et al., 2017).
Notably, all apparatus and solutions used in the fecal suspension preparation should be strictly sterilized. All fecal material preparation processes should be carried out at a room temperature of 20–30°C, preferably in an anaerobic chamber (Rossen et al., 2015).
The best FMT dose up strategy depends on the disease and the type of bacteria to be studied. Staley and his colleagues quantified the donor’s microbial load for FMT preparations using a Petroff-Hauser counting chamber under a microscope (Staley et al., 2017). However, the attainment of FMT mostly depends on the recipient bacterial richness rather than the composition of the donor’s fecal samples. Low microbial load in the recipient can help in improved colonizing of donor microbiota (Sarrabayrouse et al., 2020). The dose-volume and duration of fecal microbiota administration range from a single dose to twice a week for numerous weeks (Hintze et al., 2014; Wrzosek et al., 2018). The scheme using only one dose of FMT was not recommended as there is a cage-dependent shift of the gut microbiota over time, potentially due to the low stability of the transplanted microbiome (Wrzosek et al., 2018). In comparison, FMT twice a week for several weeks can affect the engraftment and trouble the strength of the newly formed microbial ecosystem. FMT twice during the first week allows engraftment of sub-dominant microbes, such as Bifidobacterium (Wrzosek et al., 2018). FMT of the human stool to mice once a week for four weeks may be the best settlement, as it allowed the engraftment of dominant bacteria, such as Faecalibacterium, which was not identified without repeated FMT (Wrzosek et al., 2018). The suggested maximum volume for the administration of FMT is 2% of body weight, especially for the mouse. The appropriate amount of fecal infusion is challenging to define. Agreement varies such as 100 µl per dose, (Sivan et al., 2015) 200 µl per dose (Sun et al., 2019) 300 µl per dose (Ericsson et al., 2017) and 400 µl per dose (Lin et al., 2019). FMT performed via gastric gavage is advised to infuse feces on three consecutive days, beginning immediately after discontinuation of antibiotics and placement on untreated drinking water (Ericsson et al., 2017) corrected disturbed the gut microbiota (Le Bastard et al., 2018). Overall, it is suggested that repeated gavages once a week for 12 weeks can be a good approach to maintain the human donor microbiota population for 12 weeks (Hintze et al., 2014). The human gut microbiota was reported in recipient mice four weeks post-FMT, irrespective of the FMT approach (Wrzosek et al., 2018). Presently, it is not clear how many FMT dosages are essential to sustain the donor microbiota in the recipient in the long-term or if any association exist between FMT dosages and the donor’s sustainability microbiota. Therefore, upcoming work is desired to conclude the optimal dose. It also not fully defined whether a specific microbial community composition affects the efficiency of FMT. Forthcoming studies might also check this parameter to evaluate the efficacy of FMT at numerous time points and dosages.
There is no general agreement on the best approach for delivering fecal microbiota or on the optimal volume. However, oral gastric gavage is a common and preferred method of defined oral dosing for FMT experiments in mice. Fecal suspension may be administered straight into the stomach of mice via a technique called oral-gastric gavage (Sivan et al., 2015). In this method, a stainless-steel bulb tilted gavage needle or a flexible cannula or tube is attached to a syringe and used to deliver the fecal suspension into the stomach. The suggested needle or vessel dimension should be equivalent to the distance from the mouth to exactly after the last rib of the abdominal. Before oral gastric gavage, any anesthesia is not recommended, as this will surge the threat of aspiration pneumonia in mice (Turner et al., 2011). Simple oral-gastric gavage administration of mouse donor microbiota effectively remodels gut microbiota in mouse recipients (Zhang et al., 2015; Rausch et al., 2016). This technique remains the most extensively used method for FMT. It also allows for a quicker transfer of feces. Obstacles linked with this method include unintentional administration into the trachea, bronchial pneumonia, esophageal injury or trauma, stomach rupture, and weight loss (de Meijer et al., 2010; Arantes-Rodrigues et al., 2012; Kinder et al., 2014). Stress-induced by this method (Balcombe et al., 2004) can enhance morbidity and mortality in mice.
Per rectum administration of fecal materials by enema is less common in animals than in humans. FMT by enema may be a practical approach to reduce necrotizing enterocolitis progression. Enema reduces intestinal inflammation and increases intestinal barrier function in mice (Liu et al., 2020). For performing enema, a 4% chloral hydrate/kg body weight solution should be injected intraperitoneal to induce mild anesthesia. The mice should be positioned into the horizontal situation, followed by placement of a plastic tube of 2 mm inner diameter. After applying paraffin oil onto the tube surface, the tube inserts into the colon through the anus approximately 4 cm. The fecal suspension solution should be injected slowly (Zhou et al., 2019). FMT administered via oral gavage can be nasty in taste and can also cause gastric irritation. Rectum favors a reproducible absorption course. Rectum has little enzymatic actions as compared to other parts of the gut. Further, it is anatomically relatively easy for rectally administered FMT to spread the distal portion of the colon than the proximal part of the colon. The disadvantage of enema is associated with leakage of the rectum, unpredictable absorption, and risk of injury to the rectum’s inner lining that could lead to infection.
One of the most straightforward approaches to evaluating an involved gut microbiome’s effect on a documented phenotype is the cohousing of affected and unaffected animals already harboring complex microbial populations. Cohousing works on the hypothesis that the gut microbiome of affected animals will be transferred to cagemates, probably via stochastic contact to gut microbiota-associated microbes in the environment or entire coprophagy (Ivanov et al., 2008). Cohousing can also be achieved using groupings of colonized and GF mice as a means of assessing the ability of microbial communities to colonize the GF mice (Seedorf et al., 2014). As with FMT, GF mice can be co-housed with colonized mice at various ages to determine the effects of early life events in the adult host (Hansen et al., 2012). Cohousing offers numerous logistical benefits to other methods comprising negligible cost and expertise. However, it is not recommended when donor and recipient are fed different diets consist either high-fat high-sucrose or crude extract of camu camu (Anhe et al., 2019). Cohousing also cannot be executed for human microbiota transfer to mice (Llopis et al., 2016). It also depends on passive, partial, and selective exchanges of microbes between co-housed mice. As a result, a lack of phenotype transfer between co-housed mice does not necessarily remove the involvement of the gut microbiota to the phenotype. By contrast, the positive transmission of specific microbiota directly through oral gavage or other techniques to recipient mice offers direct and clear evidence of a microbial influence (Bel et al., 2014).
The age of the recipient determines significant engraftment of donor bacteria (Le Roy et al., 2018). Donor microbiota engrafted better in 3-week old mice compared to 8-week old mice. FMT is more competent if the recipient’s microbiota displays lower richness (Ericsson et al., 2017). Gut microbiota has been less diverse at weaning, probably associated with diet transition from mother milk to a solid diet (Schloss et al., 2012). Microbial diversity surges with age (Zhang et al., 2015) and reaches stability around 8-week of age (Laukens et al., 2016). The immune system is not much prone to alteration by the bacterial influx, perhaps post 8 weeks of age, because of improved stability of the immune system and gut microbiome homeostasis (Laukens et al., 2016). Bowel cleansing is reported more effective in weaning mice (3 weeks old) than in adults (8 weeks old) (Le Roy et al., 2018). Further, the antibiotic treatment causes a long-term alteration in the gut microbiome at the weaning than adult stage (Cox et al., 2014). Considering the provided reports, weaning might be a window of opportunity for FMT.
The gut microbiota acts as a barricade against the invasion of external microorganisms. The basis of the antibiotic treatment is to drop the gut microbiome load in the recipient to ease competition for the repopulating gut microbiome from the donor. The success of FMT is more reliant on the recipient’s microbial load than the composition of the donor’s feces. Low bacterial load in the recipient can help in better colonizing of donor microbiota (Sarrabayrouse et al., 2020). Antibiotics administration decreases the microbial load in the recipient that would favor better engraftment of donor microbiota (Sarrabayrouse et al., 2020). FMT effectiveness in mice could be higher in the antibiotic treated gut than bowel cleansing treated gut and untreated gut (Ji et al., 2017). Antibiotics have a profound effect on the gut microbiota of recipients; therefore, various concoctions of antibiotics, as well as routes of administration, may demonstrate variable efficacy (Johnson et al., 2004). Treatment with broad-spectrum antibiotics is commonly used to deplete the gut microbiome of mice before FMT. Due to variances in the mechanism of action, antibiotics can selectively diminish different microbes. Multiple alternating antibiotic concoctions courses may help allow stable engraftment of the human gut microbiome in recipient mice (Hintze et al., 2014). The specific antibiotic can change the composition of the gut microbiome to recognize bacterial classes related to different phenotypes (Schubert et al., 2015; Zackular et al., 2016). Some antibiotic treatment protocols additionally include antifungals to avoid fungal overgrowth (Grasa et al., 2015; Zakostelska et al., 2016). Investigators have used numerous antibiotic régimes, which differ in the recipe, dosage, and treatment length (Table 1). Often, antibiotics are diluted in drinking water, and mice are allowed to drink during treatment; therefore, actually delivered doses can differ. Water solutions are endorsed to make freshly and change once or twice a week (Smith et al., 2013). Many also supplement sweeteners such as sugar or Kool-aid drink mix to disguise any antibiotics’ bitterness (Baldridge et al., 2015; Emal et al., 2017). Still, studies of mice avoided drinking water and becoming dehydrated when provided antibiotics with sweeteners or sugar (Reikvam et al., 2011; Zakostelska et al., 2016). Oral gavage can stop dehydration and permit the delivery of antibiotics. The oral gastric gavage method is occasionally used alone or mixed with delivery in drinking water (Reikvam et al., 2011). Broad-spectrum antibiotics deplete intestinal microbiota. However, leftover antibiotics might persist in recipients and might affect the engraftment of donor bacteria; thus, FMT is often performed one or two days after the last day of antibiotic treatment. Depending upon the starting bacterial diversity, antibiotic concoction, and length of treatment, resistance to antibiotics may confound results in FMT research.
Bowel cleansing with laxative based methods such as PEG is an alternative approach for removing gut microbiota before implantation of the donor gut microbiome. The PEG increases the volume of fluids via osmotic flow and washes out the luminal bacteria. It also introduces oxygen into the colon, which generally harbors anaerobic bacteria ecosystem and declines the nutrition for them (Strocchi et al., 1990). The higher dose of PEG through oral gastric gavage perhaps permitted a more efficient bowel clean. Recent findings suggested 170 mg of PEG for 4 times at 20 minutes intervals (Wrzosek et al., 2018) and 93 mg of PEG for 5 times at 30 minutes intervals (Le Roy et al., 2018) for clearing the gut and reducing the bacterial load in adult and young mice, respectively. PEG alone can lead to a similar decrease in the quantity of a combination of antibiotics. The PEG method is easy and straightforward to use for diminishing resident bacteria from the gut. The PEG solution causes electrolytes disturbance, dehydration (Yamano et al., 2016; Tropini et al., 2018), and change in the protective mucus lining of the colon, which leads to diarrhea in recipients (Johansson et al., 2014; Jalanka et al., 2015).
In contrast with antibiotics, laxatives temporarily decrease the abundance of gut bacteria ecosystem in humans (Jalanka et al., 2015) and mice (Ji et al., 2017). Bowel cleansing also maintained indigenous microbiota post-treatment compared to antibiotics (Ji et al., 2017).
Germ-free (GF) animals provide a valuable investigational tool for inspecting connections between a host and its GM. GF animals free from bacteria, viruses, fungi, protozoa, and parasites, throughout their lifespan (Al-Asmakh and Zadjali, 2015). The physiological conditions in the GF gut are different from wild type and specific pathogen-free mice gut. Precisely, increased mucin, pH, urea and O2, and low or lack of short-chain fatty acids (SCFAs) (Al-Asmakh and Zadjali, 2015) are among the many typical variances that can have a substantial impact on gut microbiota engraftment (Gillilland et al., 2012; Tomas et al., 2013). Along with stabilization in colonization, GF mice’s innate and adaptive immune system requires 30 days to be functional (El Aidy et al., 2013). GF mice must often be examined for contamination using a combination of bacterial culture, microscopy, serology, gross morphology, and sequencing-based detection techniques (Fontaine et al., 2015; Nicklas et al., 2015).
Additionally, the preservation of GF mice in isolators are quite expensive and limit wide application. The cost and management of isolators may make it impracticable or challenging to conduct behavioral testing or pathological study using GF mice. Overall, these parameters should be considered for planning experimental designs and interpreting results related to microbiota composition analysis and phenotype transmission (Le Roy et al., 2018).
The engraftment of the gut microbiome can be examined by alpha and beta diversity. The extent of donor engraftment can be tracked using a Bayesian Source Tracker algorithm, an OTU-based approach (Knights et al., 2011; Staley et al., 2016; Staley et al., 2017). Several studies reported success in the donor microbiota colonization post-FMT with a varied range of engraftment (Table 2). Transfer of human gut microbiota into GF mice by FMT results in the successful transfer of 85-88% of genera after seven days (Turnbaugh et al., 2009; Staley et al., 2017). Approximately 52-58% of donor mice microbiota successfully transferred in recipient mice post one week of the last dose of FMT and engraftment was maintained for the next three weeks (Ericsson et al., 2017). Further, 57-68% human donor microbial population could be attained post weekly gavage for 12 weeks. Staley and his research group reported engraftment ranging from 63.4% to 87.9% to specific pathogen-free mice at day three post single dose of antibiotics cocktail. 76 While engraftment fell marginally post day 3, high levels of donor similarity were retained for the three weeks. Multiple doses of antibiotics cocktail increase the percentage of engraftment compared to a single dose of antibiotics, but it did not increase significantly. The microbiota in control mice without antibiotics cocktail treatment displayed higher similarity (89.4% to 95.0%) to their microbiota post-FMT rather than donor microbiota, suggesting antibiotic treatment is required for an effective FMT (Turnbaugh et al., 2009; Staley et al., 2017).
Interestingly, not all the bacteria in the donor stool have equal colonization capacity. Bacteriodetes phylum was more successful in colonization compared to Firmicutes phylum (Hintze et al., 2014). It is also important to note that at least some microbiota interactions with the host immune system can only be mediated by host-specific microbiota. The colonization of mice with human microbiota does not fully restore microbiota-associated colonization resistance against some pathogens (Chung et al., 2012; Le Roy et al., 2018).
The gut microbiota alterations post-FMT employ an intense effect on the immune status of the recipient. It can be a crucial parameter in the success of FMT-based therapeutics. A study has shown that mice without CD4+ Foxp3+ T-regulatory cells were unable to resolve CDI post-FMT and exacerbated inflammation with failure of engraftment of donor microbiota (Littmann et al., 2021). However, removing B-cells, CD8-positive T cells, Th17, and Th1 cells did not affect the success of FMT in CDI mice (Littmann et al., 2021). Success of FMT might involve other immune cells such as innate lymphoid cells and mucosal-associated invariant T cells that regulate host gut immune homeostasis (Fan et al., 2019; Ioannidis et al., 2020). Host-derived inflammatory byproducts such as reactive oxygen and nitrogen species promote the growth of inflammation-tolerant pathogens (Winter et al., 2013). Further, inflammation-induced metabolites ethanolamine, nitric acid, formic acid, and lactic acid also simultaneously support the growth of inflammation- tolerant bacteria (Thiennimitr et al., 2011; Spees et al., 2013; Hughes and Winter, 2016; Gillis et al., 2018) and may prevent engraftment of microbiota (Littmann et al., 2021). Transplantation studies demonstrated that outcome of grafts is driven by the compatibility of major histocompatibility complex [MHC, called human leukocyte antigen (HLA) in human] within and between species (Trivedi et al., 2007; Ayala Garcia et al., 2012). Interestingly, MHC class II and HLA-DQ2 expression influences gut microbiota composition in mice (Kubinak et al., 2015; Khan et al., 2019) and human (Olivares et al., 2015) respectively. Therefore, it is probable that incompatibilities with human microbiota transplantation into mice are also influenced by MHC when introducing foreign microbes. Though this is not the only contributing factor, it can be an important consideration in FMT studies. Overall, these reports suggested that the recipient’s specific immune status can exploit the effectiveness or success of FMT. A more extensive characterization of host immune status is needed to understand its role in the engraftment of donor microbiota.
Most studies on FMT have focused explicitly on the bacteria component of the microbiota. Even though this is a crucial feature, FMT comprises the transfer of more than bacteria. Viruses, fungi, and microbial metabolites are also components of feces and may affect the recipient (Bojanova and Bordenstein, 2016). Ott et al. demonstrated that fecal suspension of the donor was influential in the treatment of recurrent CDI patients. They reported similarity in phage community six weeks post-FMT in recipient compared to donor (Ott et al., 2017). Gavage of phages of a high-fat diet-fed donor helped in reducing small intestinal bacterial overgrowth in high-fat diet-fed recipient mice (Lin et al., 2019). Patients with recurrent CDI displayed fungal dysbiosis. Improvement after FMT was related to a higher abundance of donor-derived Saccharomyces and Aspergillus in recipients (Zuo et al., 2018). Microbial metabolites such as short-chain fatty acids (SCFAs) have been shown to noticeable anti-inflammatory and T cell–inducing functions in the host (Arpaia et al., 2013; Smith et al., 2013). Further, FMT rich in SCFAs supplemented with butyric acid effectively managed ischemic stroke (Chen et al., 2019). Understanding link between donor non-bacterial components and host-microbiota throughout the intestine is a crucial next footstep in discovering the prospective of FMT based treatments for numerous forms of gut dysbiosis.
Despite the many studies using FMT to test the causal link of the microbiome in diseases, a multitude of variables of FMT procedures differ between research labs and institutions, and there is no scientific harmony on the standard methodology. An evidence-based approach and routine assessment of stool and FMT preparation protocol are required to maximize FMT effects and ensure the reproducible outcome.
YZ and SB conceived the study, SB wrote the manuscript. YZ, YD, and HP assisted in writing the manuscript. All authors contributed to the article and approved the submitted version.
This work was supported by the NINDS through R01 NS102633-04.
The authors declare that the research was conducted in the absence of any commercial or financial relationships that could be construed as a potential conflict of interest.
All claims expressed in this article are solely those of the authors and do not necessarily represent those of their affiliated organizations, or those of the publisher, the editors and the reviewers. Any product that may be evaluated in this article, or claim that may be made by its manufacturer, is not guaranteed or endorsed by the publisher.
Al-Asmakh, M., Zadjali, F. (2015). Use of Germ-Free Animal Models in Microbiota-Related Research. J. Microbiol. Biotechnol. 25 (10), 1583–1588. doi: 10.4014/jmb.1501.01039
Anhe, F. F., Nachbar, R. T., Varin, T. V., Trottier, J., Dudonne, S., Le Barz, M., et al. (2019). Treatment With Camu Camu (Myrciaria Dubia) Prevents Obesity by Altering the Gut Microbiota and Increasing Energy Expenditure in Diet-Induced Obese Mice. Gut 68 (3), 453–464. doi: 10.1136/gutjnl-2017-315565
Arantes-Rodrigues, R., Henriques, A., Pinto-Leite, R., Faustino-Rocha, A., Pinho-Oliveira, J., Teixeira-Guedes, C., et al. (2012). The Effects of Repeated Oral Gavage on the Health of Male CD-1 Mice. Lab. Anim. (NY) 41 (5), 129–134. doi: 10.1038/laban0512-129
Arpaia, N., Campbell, C., Fan, X., Dikiy, S., van der Veeken, J., deRoos, P., et al. (2013). Metabolites Produced by Commensal Bacteria Promote Peripheral Regulatory T-Cell Generation. Nature 504 (7480), 451–455. doi: 10.1038/nature12726
Atarashi, K., Tanoue, T., Shima, T., Imaoka, A., Kuwahara, T., Momose, Y., et al. (2011). Induction of Colonic Regulatory T Cells by Indigenous Clostridium Species. Science 331 (6015), 337–341. doi: 10.1126/science.1198469
Ayala Garcia, M. A., Gonzalez Yebra, B., Lopez Flores, A. L., Guani Guerra, E. (2012). The Major Histocompatibility Complex in Transplantation. J. Transplant. 2012, 842141. doi: 10.1155/2012/842141
Bahl, M. I., Bergstrom, A., Licht, T. R. (2012). Freezing Fecal Samples Prior to DNA Extraction Affects the Firmicutes to Bacteroidetes Ratio Determined by Downstream Quantitative PCR Analysis. FEMS Microbiol. Lett. 329 (2), 193–197. doi: 10.1111/j.1574-6968.2012.02523.x
Bajaj, J. S., Kassam, Z., Fagan, A., Gavis, E. A., Liu, E., Cox, I. J., et al. (2017). Fecal Microbiota Transplant From a Rational Stool Donor Improves Hepatic Encephalopathy: A Randomized Clinical Trial. Hepatol. 66 (6), 1727–1738. doi: 10.1002/hep.29306
Bakken, J. S., Borody, T., Brandt, L. J., Brill, J. V., Demarco, D. C., Franzos, M. A., et al. (2011). Treating Clostridium Difficile Infection With Fecal Microbiota Transplantation. Clin. Gastroenterol. Hepatol. 9 (12), 1044–1049. doi: 10.1016/j.cgh.2011.08.014
Balcombe, J. P., Barnard, N. D., Sandusky, C. (2004). Laboratory Routines Cause Animal Stress. Contemp. Top. Lab. Anim. Sci. 43 (6), 42–51.
Baldridge, M. T., Nice, T. J., McCune, B. T., Yokoyama, C. C., Kambal, A., Wheadon, M., et al. (2015). Commensal Microbes and Interferon-Lambda Determine Persistence of Enteric Murine Norovirus Infection. Science 347 (6219), 266–269. doi: 10.1126/science.1258025
Barba, C., Soulage, C. O., Caggiano, G., Glorieux, G., Fouque, D., Koppe, L. (2020). Effects of Fecal Microbiota Transplantation on Composition in Mice With CKD. Toxins (Basel) 12 (12), 741. doi: 10.3390/toxins12120741
Barcena, C., Valdes-Mas, R., Mayoral, P., Garabaya, C., Durand, S., Rodriguez, F., et al. (2019). Healthspan and Lifespan Extension by Fecal Microbiota Transplantation Into Progeroid Mice. Nat. Med. 25 (8), 1234–1242. doi: 10.1038/s41591-019-0504-5
Béal, C., Fonseca, F. (2015). “Freezing of Probiotic Bacteria”. in Advances in Probiotic Technology. Eds. Foerst, P., Santivarangkna, C. (Boca Raton: CRC Press) 179–212. doi: 10.1201/b18807-14
Bel, S., Elkis, Y., Elifantz, H., Koren, O., Ben-Hamo, R., Lerer-Goldshtein, T., et al. (2014). Reprogrammed and Transmissible Intestinal Microbiota Confer Diminished Susceptibility to Induced Colitis in TMF-/- Mice. Proc. Natl. Acad. Sci. U. S. A. 111 (13), 4964–4969. doi: 10.1073/pnas.1319114111
Bellali, S., Lagier, J. C., Raoult, D., Bou Khalil, J. (2019). Among Live and Dead Bacteria, the Optimization of Sample Collection and Processing Remains Essential in Recovering Gut Microbiota Components. Front. Microbiol. 10, 1606. doi: 10.3389/fmicb.2019.01606
Ben-Amor, K., Heilig, H., Smidt, H., Vaughan, E. E., Abee, T., de Vos, W. M. (2005). Genetic Diversity of Viable, Injured, and Dead Fecal Bacteria Assessed by Fluorescence-Activated Cell Sorting and 16S rRNA Gene Analysis. Appl. Environ. Microbiol. 71 (8), 4679–4689. doi: 10.1128/AEM.71.8.4679-4689.2005
Berer, K., Gerdes, L. A., Cekanaviciute, E., Jia, X., Xiao, L., Xia, Z., et al. (2017). Gut Microbiota From Multiple Sclerosis Patients Enables Spontaneous Autoimmune Encephalomyelitis in Mice. Proc. Natl. Acad. Sci. U. S. A. 114 (40), 10719–10724. doi: 10.1073/pnas.1711233114
Bojanova, D. P., Bordenstein, S. R. (2016). Fecal Transplants: What Is Being Transferred? PLoS Biol. 14 (7), e1002503. doi: 10.1371/journal.pbio.1002503
Brandt, L. J., Aroniadis, O. C. (2013). An Overview of Fecal Microbiota Transplantation: Techniques, Indications, and Outcomes. Gastrointest. Endosc. 78 (2), 240–249. doi: 10.1016/j.gie.2013.03.1329
Brusa, T., Canzi, E., Pacini, N., Zanchi, R., Ferrari, A. (1989). Oxygen Tolerance of Anaerobic Bacteria Isolated From Human Feces. Curr. Microbiol. 19 (1), 39–43. doi: 10.1007/BF01568901
Burrello, C., Garavaglia, F., Cribiu, F. M., Ercoli, G., Bosari, S., Caprioli, F., et al. (2018). Short-Term Oral Antibiotics Treatment Promotes Inflammatory Activation of Colonic Invariant Natural Killer T and Conventional CD4(+) T Cells. Front. Med. (Lausanne) 5, 21. doi: 10.3389/fmed.2018.00021
Burz, S. D., Abraham, A. L., Fonseca, F., David, O., Chapron, A., Beguet-Crespel, F., et al. (2019). A Guide for Ex Vivo Handling and Storage of Stool Samples Intended for Fecal Microbiota Transplantation. Sci. Rep. 9 (1), 8897. doi: 10.1038/s41598-019-45173-4
Cammarota, G., Ianiro, G., Gasbarrini, A. (2014). Fecal Microbiota Transplantation for the Treatment of Clostridium Difficile Infection: A Systematic Review. J. Clin. Gastroenterol. 48 (8), 693–702. doi: 10.1097/MCG.0000000000000046
Cammarota, G., Ianiro, G., Tilg, H., Rajilic-Stojanovic, M., Kump, P., Satokari, R., et al. (2017). European Consensus Conference on Faecal Microbiota Transplantation in Clinical Practice. Gut 66 (4), 569–580. doi: 10.1136/gutjnl-2016-313017
Carlson, P. E., Jr. (2020). Regulatory Considerations for Fecal Microbiota Transplantation Products. Cell Host Microbe 27 (2), 173–175. doi: 10.1016/j.chom.2020.01.018
Carroll, I. M., Ringel-Kulka, T., Siddle, J. P., Klaenhammer, T. R., Ringel, Y. (2012). Characterization of the Fecal Microbiota Using High-Throughput Sequencing Reveals a Stable Microbial Community During Storage. PLoS One 7 (10), e46953. doi: 10.1371/journal.pone.0046953
Cenit, M. C., Sanz, Y., Codoner-Franch, P. (2017). Influence of Gut Microbiota on Neuropsychiatric Disorders. World J. Gastroenterol. 23 (30), 5486–5498. doi: 10.3748/wjg.v23.i30.5486
Chang, C., Lin, H. (2016). Dysbiosis in Gastrointestinal Disorders. Best Pract. Res. Clin. Gastroenterol. 30 (1), 3–15. doi: 10.1016/j.bpg.2016.02.001
Chen, R., Xu, Y., Wu, P., Zhou, H., Lasanajak, Y., Fang, Y., et al. (2019). Transplantation of Fecal Microbiota Rich in Short Chain Fatty Acids and Butyric Acid Treat Cerebral Ischemic Stroke by Regulating Gut Microbiota. Pharmacol. Res. 148, 104403. doi: 10.1016/j.phrs.2019.104403
Choo, J. M., Leong, L. E., Rogers, G. B. (2015). Sample Storage Conditions Significantly Influence Faecal Microbiome Profiles. Sci. Rep. 5, 16350. doi: 10.1038/srep16350
Chung, H., Pamp, S. J., Hill, J. A., Surana, N. K., Edelman, S. M., Troy, E. B., et al. (2012). Gut Immune Maturation Depends on Colonization With a Host-Specific Microbiota. Cell 149 (7), 1578–1593. doi: 10.1016/j.cell.2012.04.037
Cox, L. M., Yamanishi, S., Sohn, J., Alekseyenko, A. V., Leung, J. M., Cho, I., et al. (2014). Altering the Intestinal Microbiota During a Critical Developmental Window has Lasting Metabolic Consequences. Cell 158 (4), 705–721. doi: 10.1016/j.cell.2014.05.052
Dabke, K., Hendrick, G., Devkota, S. (2019). The Gut Microbiome and Metabolic Syndrome. J. Clin. Invest. 129 (10), 4050–4057. doi: 10.1172/JCI129194
de Meijer, V. E., Le, H. D., Meisel, J. A., Puder, M. (2010). Repetitive Orogastric Gavage Affects the Phenotype of Diet-Induced Obese Mice. Physiol. Behav. 100 (4), 387–393. doi: 10.1016/j.physbeh.2010.04.001
De Palma, G., Lynch, M. D., Lu, J., Dang, V. T., Deng, Y., Jury, J., et al. (2017). Transplantation of Fecal Microbiota From Patients With Irritable Bowel Syndrome Alters Gut Function and Behavior in Recipient Mice. Sci. Transl. Med. 9 (379), eaaf6397. doi: 10.1126/scitranslmed.aaf6397
Diaz Heijtz, R., Wang, S., Anuar, F., Qian, Y., Bjorkholm, B., Samuelsson, A., et al. (2011). Normal Gut Microbiota Modulates Brain Development and Behavior. Proc. Natl. Acad. Sci. U. S. A. 108 (7), 3047–3052. doi: 10.1073/pnas.1010529108
Durand, A., Audemard-Verger, A., Guichard, V., Mattiuz, R., Delpoux, A., Hamon, P., et al. (2018). Profiling the Lymphoid-Resident T Cell Pool Reveals Modulation by Age and Microbiota. Nat. Commun. 9 (1), 68. doi: 10.1038/s41467-017-02458-4
El Aidy, S., Hooiveld, G., Tremaroli, V., Backhed, F., Kleerebezem, M. (2013). The Gut Microbiota and Mucosal Homeostasis: Colonized at Birth or at Adulthood, Does It Matter? Gut Microbes 4 (2), 118–124. doi: 10.4161/gmic.23362
Elinav, E., Strowig, T., Kau, A. L., Henao-Mejia, J., Thaiss, C. A., Booth, C. J., et al. (2011). NLRP6 Inflammasome Regulates Colonic Microbial Ecology and Risk for Colitis. Cell 145 (5), 745–757. doi: 10.1016/j.cell.2011.04.022
Emal, D., Rampanelli, E., Stroo, I., Butter, L. M., Teske, G. J., Claessen, N., et al. (2017). Depletion of Gut Microbiota Protects Against Renal Ischemia-Reperfusion Injury. J. Am. Soc. Nephrol. 28 (5), 1450–1461. doi: 10.1681/ASN.2016030255
Ericsson, A. C., Gagliardi, J., Bouhan, D., Spollen, W. G., Givan, S. A., Franklin, C. L. (2018). The Influence of Caging, Bedding, and Diet on the Composition of the Microbiota in Different Regions of the Mouse Gut. Sci. Rep. 8 (1), 4065. doi: 10.1038/s41598-018-21986-7
Ericsson, A. C., Personett, A. R., Turner, G., Dorfmeyer, R. A., Franklin, C. L. (2017). Variable Colonization After Reciprocal Fecal Microbiota Transfer Between Mice With Low and High Richness Microbiota. Front. Microbiol. 8, 196. doi: 10.3389/fmicb.2017.00196
Evrensel, A., Ceylan, M. E. (2016). Fecal Microbiota Transplantation and Its Usage in Neuropsychiatric Disorders. Clin. Psychopharmacol. Neurosci. 14 (3), 231–237. doi: 10.9758/cpn.2016.14.3.231
Fadda, H. M. (2020). The Route to Palatable Fecal Microbiota Transplantation. AAPS Pharm. Sci. Tech. 21 (3), 114. doi: 10.1208/s12249-020-1637-z
Fan, H., Wang, A., Wang, Y., Sun, Y., Han, J., Chen, W., et al. (2019). Innate Lymphoid Cells: Regulators of Gut Barrier Function and Immune Homeostasis. J. Immunol. Res. 2019, 2525984. doi: 10.1155/2019/2525984
Felice, V. D., O’Mahony, S. M. (2017). The Microbiome and Disorders of the Central Nervous System. Pharmacol. Biochem. Behav. 160, 1–13. doi: 10.1016/j.pbb.2017.06.016
Fernandez-Santoscoy, M., Wenzel, U. A., Yrlid, U., Cardell, S., Backhed, F., Wick, M. J. (2015). The Gut Microbiota Reduces Colonization of the Mesenteric Lymph Nodes and IL-12-Independent IFN-Gamma Production During Salmonella Infection. Front. Cell Infect. Microbiol. 5, 93. doi: 10.3389/fcimb.2015.00093
Ferrere, G., Wrzosek, L., Cailleux, F., Turpin, W., Puchois, V., Spatz, M., et al. (2017). Fecal Microbiota Manipulation Prevents Dysbiosis and Alcohol-Induced Liver Injury in Mice. J. Hepatol. 66 (4), 806–815. doi: 10.1016/j.jhep.2016.11.008
Finegold, S. M. (1995). Anaerobic Infections in Humans: An Overview. Anaerobe 1 (1), 3–9. doi: 10.1016/s1075-9964(95)80340-8
Fontaine, C. A., Skorupski, A. M., Vowles, C. J., Anderson, N. E., Poe, S. A., Eaton, K. A. (2015). How Free of Germs Is Germ-Free? Detection of Bacterial Contamination in a Germ Free Mouse Unit. Gut Microbes 6 (4), 225–233. doi: 10.1080/19490976.2015.1054596
Food and Drug Administration. (2020). Safety Alert Regarding Use of Fecal Microbiota for Transplantation and Risk of Serious Adverse Events Likely Due to Transmission of Pathogenic Organisms (Rockville, MD: Food and Drug Administration).
Freitag, T. L., Hartikainen, A., Jouhten, H., Sahl, C., Meri, S., Anttila, V. J., et al. (2019). Minor Effect of Antibiotic Pre-Treatment on the Engraftment of Donor Microbiota in Fecal Transplantation in Mice. Front. Microbiol. 10, 2685. doi: 10.3389/fmicb.2019.02685
Ganal, S. C., Sanos, S. L., Kallfass, C., Oberle, K., Johner, C., Kirschning, C., et al. (2012). Priming of Natural Killer Cells by Nonmucosal Mononuclear Phagocytes Requires Instructive Signals From Commensal Microbiota. Immunity 37 (1), 171–186. doi: 10.1016/j.immuni.2012.05.020
Gillilland, M. G., 3rd, Erb-Downward, J. R., Bassis, C. M., Shen, M. C., Toews, G. B., Young, V. B., et al. (2012). Ecological Succession of Bacterial Communities During Conventionalization of Germ-Free Mice. Appl. Environ. Microbiol. 78 (7), 2359–2366. doi: 10.1128/AEM.05239-11
Gillis, C. C., Hughes, E. R., Spiga, L., Winter, M. G., Zhu, W., Furtado de Carvalho, T., et al. (2018). Dysbiosis-Associated Change in Host Metabolism Generates Lactate to Support Salmonella Growth. Cell Host Microbe 23 (1), 54–64.e6. doi: 10.1016/j.chom.2017.11.006
Gough, E., Shaikh, H., Manges, A. R. (2011). Systematic Review of Intestinal Microbiota Transplantation (Fecal Bacteriotherapy) for Recurrent Clostridium Difficile Infection. Clin. Infect. Dis. 53 (10), 994–1002. doi: 10.1093/cid/cir632
Grasa, L., Abecia, L., Forcen, R., Castro, M., de Jalon, J. A., Latorre, E., et al. (2015). Antibiotic-Induced Depletion of Murine Microbiota Induces Mild Inflammation and Changes in Toll-Like Receptor Patterns and Intestinal Motility. Microb. Ecol. 70 (3), 835–848. doi: 10.1007/s00248-015-0613-8
Gury-BenAri, M., Thaiss, C. A., Serafini, N., Winter, D. R., Giladi, A., Lara-Astiaso, D., et al. (2016). The Spectrum and Regulatory Landscape of Intestinal Innate Lymphoid Cells Are Shaped by the Microbiome. Cell 166 (5), 1231–1246.e13. doi: 10.1016/j.cell.2016.07.043
Hamilton, M. J., Weingarden, A. R., Sadowsky, M. J., Khoruts, A. (2012). Standardized Frozen Preparation for Transplantation of Fecal Microbiota for Recurrent Clostridium Difficile Infection. Am. J. Gastroenterol. 107 (5), 761–767. doi: 10.1038/ajg.2011.482
Hansen, C. H., Nielsen, D. S., Kverka, M., Zakostelska, Z., Klimesova, K., Hudcovic, T., et al. (2012). Patterns of Early Gut Colonization Shape Future Immune Responses of the Host. PLoS One 7 (3), e34043. doi: 10.1371/journal.pone.0034043
Hintze, K. J., Cox, J. E., Rompato, G., Benninghoff, A. D., Ward, R. E., Broadbent, J., et al. (2014). Broad Scope Method for Creating Humanized Animal Models for Animal Health and Disease Research Through Antibiotic Treatment and Human Fecal Transfer. Gut Microbes 5 (2), 183–191. doi: 10.4161/gmic.28403
Hughes, E. R., Winter, S. E. (2016). Enterococcus Faecalis: E. Coli’s Siderophore-Inducing Sidekick. Cell Host Microbe 20 (4), 411–412. doi: 10.1016/j.chom.2016.09.018
Human Microbiome Project (2012a). A Framework for Human Microbiome Research. Nature 486 (7402), 215–221. doi: 10.1038/nature11209
Human Microbiome Project (2012b). Structure, Function and Diversity of the Healthy Human Microbiome. Nature 486 (7402), 207–214. doi: 10.1038/nature11234
Ioannidis, M., Cerundolo, V., Salio, M. (2020). The Immune Modulating Properties of Mucosal-Associated Invariant T Cells. Front. Immunol. 11, 1556. doi: 10.3389/fimmu.2020.01556
Ivanov, I. I., Frutos Rde, L., Manel, N., Yoshinaga, K., Rifkin, D. B., Sartor, R. B., et al. (2008). Specific Microbiota Direct the Differentiation of IL-17-Producing T-Helper Cells in the Mucosa of the Small Intestine. Cell Host Microbe 4 (4), 337–349. doi: 10.1016/j.chom.2008.09.009
Jalanka, J., Salonen, A., Salojarvi, J., Ritari, J., Immonen, O., Marciani, L., et al. (2015). Effects of Bowel Cleansing on the Intestinal Microbiota. Gut 64 (10), 1562–1568. doi: 10.1136/gutjnl-2014-307240
Ji, S. K., Yan, H., Jiang, T., Guo, C. Y., Liu, J. J., Dong, S. Z., et al. (2017). Preparing the Gut With Antibiotics Enhances Gut Microbiota Reprogramming Efficiency by Promoting Xenomicrobiota Colonization. Front. Microbiol. 8, 1208. doi: 10.3389/fmicb.2017.01208
Johansson, M. E., Gustafsson, J. K., Holmen-Larsson, J., Jabbar, K. S., Xia, L., Xu, H., et al. (2014). Bacteria Penetrate the Normally Impenetrable Inner Colon Mucus Layer in Both Murine Colitis Models and Patients With Ulcerative Colitis. Gut 63 (2), 281–291. doi: 10.1136/gutjnl-2012-303207
Johnson, S. A., Nicolson, S. W., Jackson, S. (2004). The Effect of Different Oral Antibiotics on the Gastrointestinal Microflora of a Wild Rodent (Aethomys Namaquensis). Comp. Biochem. Physiol. A Mol. Integr. Physiol. 138 (4), 475–483. doi: 10.1016/j.cbpb.2004.06.010
Josefsdottir, K. S., Baldridge, M. T., Kadmon, C. S., King, K. Y. (2017). Antibiotics Impair Murine Hematopoiesis by Depleting the Intestinal Microbiota. Blood 129 (6), 729–739. doi: 10.1182/blood-2016-03-708594
Kang, D. W., Adams, J. B., Gregory, A. C., Borody, T., Chittick, L., Fasano, A., et al. (2017). Microbiota Transfer Therapy Alters Gut Ecosystem and Improves Gastrointestinal and Autism Symptoms: An Open-Label Study. Microbiome 5 (1), 10. doi: 10.1186/s40168-016-0225-7
Kelly, C. J., Zheng, L., Campbell, E. L., Saeedi, B., Scholz, C. C., Bayless, A. J., et al. (2015). Crosstalk Between Microbiota-Derived Short-Chain Fatty Acids and Intestinal Epithelial HIF Augments Tissue Barrier Function. Cell Host Microbe 17 (5), 662–671. doi: 10.1016/j.chom.2015.03.005
Kernbauer, E., Ding, Y., Cadwell, K. (2014). An Enteric Virus Can Replace the Beneficial Function of Commensal Bacteria. Nature 516 (7529), 94–98. doi: 10.1038/nature13960
Khan, A. A., Yurkovetskiy, L., O’Grady, K., Pickard, J. M., de Pooter, R., Antonopoulos, D. A., et al. (2019). Polymorphic Immune Mechanisms Regulate Commensal Repertoire. Cell Rep. 29 (3), 541–550.e4. doi: 10.1016/j.celrep.2019.09.010
Khosravi, A., Yanez, A., Price, J. G., Chow, A., Merad, M., Goodridge, H. S., et al. (2014). Gut Microbiota Promote Hematopoiesis to Control Bacterial Infection. Cell Host Microbe 15 (3), 374–381. doi: 10.1016/j.chom.2014.02.006
Kim, S. H., Cho, B. H., Kiyono, H., Jang, Y. S. (2017). Microbiota-Derived Butyrate Suppresses Group 3 Innate Lymphoid Cells in Terminal Ileal Peyer’s Patches. Sci. Rep. 7 (1), 3980. doi: 10.1038/s41598-017-02729-6
Kim, S. M., DeFazio, J. R., Hyoju, S. K., Sangani, K., Keskey, R., Krezalek, M. A., et al. (2020). Fecal Microbiota Transplant Rescues Mice From Human Pathogen Mediated Sepsis by Restoring Systemic Immunity. Nat. Commun. 11 (1), 2354 . doi: 10.1038/s41467-020-15545-w
Kim, M., Galan, C., Hill, A. A., Wu, W. J., Fehlner-Peach, H., Song, H. W., et al. (2018a). Critical Role for the Microbiota in CX3CR1(+) Intestinal Mononuclear Phagocyte Regulation of Intestinal T Cell Responses. Immunity 49 (1), 151–163.e5. doi: 10.1016/j.immuni.2018.05.009
Kim, T. T., Parajuli, N., Sung, M. M., Bairwa, S. C., Levasseur, J., Soltys, C. M., et al. (2018b). Fecal Transplant From Resveratrol-Fed Donors Improves Glycaemia and Cardiovascular Features of the Metabolic Syndrome in Mice. Am. J. Physiol. Endocrinol. Metab. 315 (4), E511–E519. doi: 10.1152/ajpendo.00471.2017
Kinder, J. M., Then, J. E., Hansel, P. M., Molinero, L. L., Bruns, H. A. (2014). Long-Term Repeated Daily Use of Intragastric Gavage Hinders Induction of Oral Tolerance to Ovalbumin in Mice. Comp. Med. 64 (5), 369–376.
Kinnebrew, M. A., Ubeda, C., Zenewicz, L. A., Smith, N., Flavell, R. A., Pamer, E. G. (2010). Bacterial Flagellin Stimulates Toll-Like Receptor 5-Dependent Defense Against Vancomycin-Resistant Enterococcus Infection. J. Infect. Dis. 201 (4), 534–543. doi: 10.1086/650203
Knights, D., Kuczynski, J., Charlson, E. S., Zaneveld, J., Mozer, M. C., Collman, R. G., et al. (2011). Bayesian Community-Wide Culture-Independent Microbial Source Tracking. Nat. Methods 8 (9), 761–763. doi: 10.1038/nmeth.1650
Kubinak, J. L., Stephens, W. Z., Soto, R., Petersen, C., Chiaro, T., Gogokhia, L., et al. (2015). MHC Variation Sculpts Individualized Microbial Communities That Control Susceptibility to Enteric Infection. Nat. Commun. 6, 8642. doi: 10.1038/ncomms9642
Kuss, S. K., Best, G. T., Etheredge, C. A., Pruijssers, A. J., Frierson, J. M., Hooper, L. V., et al. (2011). Intestinal Microbiota Promote Enteric Virus Replication and Systemic Pathogenesis. Science 334 (6053), 249–252. doi: 10.1126/science.1211057
Lai, Z. L., Tseng, C. H., Ho, H. J., Cheung, C. K. Y., Lin, J. Y., Chen, Y. J., et al. (2018). Fecal Microbiota Transplantation Confers Beneficial Metabolic Effects of Diet and Exercise on Diet-Induced Obese Mice. Sci. Rep. 8 (1), 15625. doi: 10.1038/s41598-018-33893-y
Lauber, C. L., Zhou, N., Gordon, J. I., Knight, R., Fierer, N. (2010). Effect of Storage Conditions on the Assessment of Bacterial Community Structure in Soil and Human-Associated Samples. FEMS Microbiol. Lett. 307 (1), 80–86. doi: 10.1111/j.1574-6968.2010.01965.x
Laukens, D., Brinkman, B. M., Raes, J., De Vos, M., Vandenabeele, P. (2016). Heterogeneity of the Gut Microbiome in Mice: Guidelines for Optimizing Experimental Design. FEMS Microbiol. Rev. 40 (1), 117–132. doi: 10.1093/femsre/fuv036
Lawley, T. D., Clare, S., Walker, A. W., Stares, M. D., Connor, T. R., Raisen, C., et al. (2012). Targeted Restoration of the Intestinal Microbiota With a Simple, Defined Bacteriotherapy Resolves Relapsing Clostridium Difficile Disease in Mice. PLoS Pathog. 8 (10), e1002995. doi: 10.1371/journal.ppat.1002995
Le Bastard, Q., Ward, T., Sidiropoulos, D., Hillmann, B. M., Chun, C. L., Sadowsky, M. J., et al. (2018). Fecal Microbiota Transplantation Reverses Antibiotic and Chemotherapy-Induced Gut Dysbiosis in Mice. Sci. Rep. 8 (1), 6219. doi: 10.1038/s41598-018-24342-x
Lee, J., d’Aigle, J., Atadja, L., Quaicoe, V., Honarpisheh, P., Ganesh, B. P., et al. (2020). Gut Microbiota-Derived Short-Chain Fatty Acids Promote Post-Stroke Recovery in Aged Mice. Circ. Res 127 (4), 453–465. doi: 10.1161/CIRCRESAHA.119.316448
Lee, C. H., Steiner, T., Petrof, E. O., Smieja, M., Roscoe, D., Nematallah, A., et al. (2016). Frozen vs Fresh Fecal Microbiota Transplantation and Clinical Resolution of Diarrhea in Patients With Recurrent Clostridium Difficile Infection: A Randomized Clinical Trial. JAMA 315 (2), 142–149. doi: 10.1001/jama.2015.18098
Le Roy, T., Debedat, J., Marquet, F., Da-Cunha, C., Ichou, F., Guerre-Millo, M., et al. (2018). Comparative Evaluation of Microbiota Engraftment Following Fecal Microbiota Transfer in Mice Models: Age, Kinetic and Microbial Status Matter. Front. Microbiol. 9, 3289. doi: 10.3389/fmicb.2018.03289
Liang, X., Bushman, F. D., FitzGerald, G. A. (2015). Rhythmicity of the Intestinal Microbiota Is Regulated by Gender and the Host Circadian Clock. Proc. Natl. Acad. Sci. U. S. A. 112 (33), 10479–10484. doi: 10.1073/pnas.1501305112
Li, F., Hao, X., Chen, Y., Bai, L., Gao, X., Lian, Z., et al. (2017). The Microbiota Maintain Homeostasis of Liver-Resident gammadeltaT-17 Cells in a Lipid Antigen/CD1d-Dependent Manner. Nat. Commun. 7, 13839. doi: 10.1038/ncomms13839
Lin, D. M., Koskella, B., Ritz, N. L., Lin, D., Carroll-Portillo, A., Lin, H. C. (2019). Transplanting Fecal Virus-Like Particles Reduces High-Fat Diet-Induced Small Intestinal Bacterial Overgrowth in Mice. Front. Cell Infect. Microbiol. 9, 348. doi: 10.3389/fcimb.2019.00348
Littmann, E. R., Lee, J. J., Denny, J. E., Alam, Z., Maslanka, J. R., Zarin, I., et al. (2021). Host Immunity Modulates the Efficacy of Microbiota Transplantation for Treatment of Clostridioides Difficile Infection. Nat. Commun. 12 (1), 755. doi: 10.1038/s41467-020-20793-x
Liu, J., Miyake, H., Zhu, H., Li, B., Alganabi, M., Lee, C., et al. (2020). Fecal Microbiota Transplantation by Enema Reduces Intestinal Injury in Experimental Necrotizing Enterocolitis. J. Pediatr. Surg. 55 (6), 1094–1098. doi: 10.1016/j.jpedsurg.2020.02.035
Llopis, M., Cassard, A. M., Wrzosek, L., Boschat, L., Bruneau, A., Ferrere, G., et al. (2016). Intestinal Microbiota Contributes to Individual Susceptibility to Alcoholic Liver Disease. Gut 65 (5), 830–839. doi: 10.1136/gutjnl-2015-310585
Mattila, E., Uusitalo-Seppala, R., Wuorela, M., Lehtola, L., Nurmi, H., Ristikankare, M., et al. (2012). Fecal Transplantation, Through Colonoscopy, Is Effective Therapy for Recurrent Clostridium Difficile Infection. Gastroenterology 142 (3), 490–496. doi: 10.1053/j.gastro.2011.11.037
Nazmul Huda, M., Winnike, J. H., Crowell, J. M., O’Connor, A., Bennett, B. J. (2020). Microbial Modulation of Host Body Composition and Plasma Metabolic Profile. Sci. Rep. 10 (1), 6545. doi: 10.1038/s41598-020-63214-1
Nicholson, J. K., Holmes, E., Kinross, J., Burcelin, R., Gibson, G., Jia, W., et al. (2012). Host-Gut Microbiota Metabolic Interactions. Science 336 (6086), 1262–1267. doi: 10.1126/science.1223813
Nicklas, W., Keubler, L., Bleich, A. (2015). Maintaining and Monitoring the Defined Microbiota Status of Gnotobiotic Rodents. ILAR J. 56 (2), 241–249. doi: 10.1093/ilar/ilv029
Ochoa-Reparaz, J., Mielcarz, D. W., Ditrio, L. E., Burroughs, A. R., Foureau, D. M., Haque-Begum, S., et al. (2009). Role of Gut Commensal Microflora in the Development of Experimental Autoimmune Encephalomyelitis. J. Immunol. 183 (10), 6041–6050. doi: 10.4049/jimmunol.0900747
Olivares, M., Neef, A., Castillejo, G., Palma, G. D., Varea, V., Capilla, A., et al. (2015). The HLA-DQ2 Genotype Selects for Early Intestinal Microbiota Composition in Infants at High Risk of Developing Coeliac Disease. Gut 64 (3), 406–417. doi: 10.1136/gutjnl-2014-306931
Olszak, T., An, D., Zeissig, S., Vera, M. P., Richter, J., Franke, A., et al. (2012). Microbial Exposure During Early Life has Persistent Effects on Natural Killer T Cell Function. Science 336 (6080), 489–493. doi: 10.1126/science.1219328
Ott, S. J., Waetzig, G. H., Rehman, A., Moltzau-Anderson, J., Bharti, R., Grasis, J. A., et al. (2017). Efficacy of Sterile Fecal Filtrate Transfer for Treating Patients With Clostridium Difficile Infection. Gastroenterology 152 (4), 2799–2811.e7. doi: 10.1053/j.gastro.2016.11.010
Owens, C., Broussard, E., Surawicz, C. (2013). Fecal Microbiota Transplantation and Donor Standardization. Trends Microbiol. 21 (9), 443–445. doi: 10.1016/j.tim.2013.07.003
Papanicolas, L. E., Choo, J. M., Wang, Y., Leong, L. E. X., Costello, S. P., Gordon, D. L., et al. (2019). Bacterial Viability in Faecal Transplants: Which Bacteria Survive? EBioMedicine 41, 509–516. doi: 10.1016/j.ebiom.2019.02.023
Rasmussen, T. S., de Vries, L., Kot, W., Hansen, L. H., Castro-Mejia, J. L., Vogensen, F. K., et al. (2019). Mouse Vendor Influence on the Bacterial and Viral Gut Composition Exceeds the Effect of Diet. Viruses 11 (5), 435. doi: 10.3390/v11050435
Rausch, P., Basic, M., Batra, A., Bischoff, S. C., Blaut, M., Clavel, T., et al. (2016). Analysis of Factors Contributing to Variation in the C57BL/6J Fecal Microbiota Across German Animal Facilities. Int. J. Med. Microbiol. 306 (5), 343–355. doi: 10.1016/j.ijmm.2016.03.004
Reikvam, D. H., Erofeev, A., Sandvik, A., Grcic, V., Jahnsen, F. L., Gaustad, P., et al. (2011). Depletion of Murine Intestinal Microbiota: Effects on Gut Mucosa and Epithelial Gene Expression. PLoS One 6 (3), e17996. doi: 10.1371/journal.pone.0017996
Ridaura, V. K., Faith, J. J., Rey, F. E., Cheng, J., Duncan, A. E., Kau, A. L., et al. (2013). Gut Microbiota From Twins Discordant for Obesity Modulate Metabolism in Mice. Science 341 (6150), 1241214. doi: 10.1126/science.1241214
Roka, R., Rosztoczy, A., Leveque, M., Izbeki, F., Nagy, F., Molnar, T., et al. (2007). A Pilot Study of Fecal Serine-Protease Activity: A Pathophysiologic Factor in Diarrhea-Predominant Irritable Bowel Syndrome. Clin. Gastroenterol. Hepatol. 5 (5), 550–555. doi: 10.1016/j.cgh.2006.12.004
Rossen, N. G., Fuentes, S., van der Spek, M. J., Tijssen, J. G., Hartman, J. H., Duflou, A., et al. (2015). Findings From a Randomized Controlled Trial of Fecal Transplantation for Patients With Ulcerative Colitis. Gastroenterology 149 (1), 110–118.e114. doi: 10.1053/j.gastro.2015.03.045
Sarrabayrouse, G., Landolfi, S., Pozuelo, M., Willamil, J., Varela, E., Clark, A., et al. (2020). Mucosal Microbial Load in Crohn’s Disease: A Potential Predictor of Response to Faecal Microbiota Transplantation. EBioMedicine 51, 102611. doi: 10.1016/j.ebiom.2019.102611
Sawa, S., Lochner, M., Satoh-Takayama, N., Dulauroy, S., Berard, M., Kleinschek, M., et al. (2011). RORgammat+ Innate Lymphoid Cells Regulate Intestinal Homeostasis by Integrating Negative Signals From the Symbiotic Microbiota. Nat. Immunol. 12 (4), 320–326. doi: 10.1038/ni.2002
Schloss, P. D., Schubert, A. M., Zackular, J. P., Iverson, K. D., Young, V. B., Petrosino, J. F. (2012). Stabilization of the Murine Gut Microbiome Following Weaning. Gut Microbes 3 (4), 383–393. doi: 10.4161/gmic.21008
Schubert, A. M., Sinani, H., Schloss, P. D. (2015). Antibiotic-Induced Alterations of the Murine Gut Microbiota and Subsequent Effects on Colonization Resistance Against Clostridium Difficile. mBio 6 (4), e00974. doi: 10.1128/mBio.00974-15
Seedorf, H., Griffin, N. W., Ridaura, V. K., Reyes, A., Cheng, J., Rey, F. E., et al. (2014). Bacteria From Diverse Habitats Colonize and Compete in the Mouse Gut. Cell 159 (2), 253–266. doi: 10.1016/j.cell.2014.09.008
Sergeant, M. J., Constantinidou, C., Cogan, T., Penn, C. W., Pallen, M. J. (2012). High-Throughput Sequencing of 16S rRNA Gene Amplicons: Effects of Extraction Procedure, Primer Length and Annealing Temperature. PLoS One 7 (5), e38094. doi: 10.1371/journal.pone.0038094
Shaw, A. G., Sim, K., Powell, E., Cornwell, E., Cramer, T., McClure, Z. E., et al. (2016). Latitude in Sample Handling and Storage for Infant Faecal Microbiota Studies: The Elephant in the Room? Microbiome 4 (1), 40. doi: 10.1186/s40168-016-0186-x
Shi, N., Li, N., Duan, X., Niu, H. (2017). Interaction Between the Gut Microbiome and Mucosal Immune System. Mil. Med. Res. 4, 14. doi: 10.1186/s40779-017-0122-9
Sivan, A., Corrales, L., Hubert, N., Williams, J. B., Aquino-Michaels, K., Earley, Z. M., et al. (2015). Commensal Bifidobacterium Promotes Antitumor Immunity and Facilitates Anti-PD-L1 Efficacy. Science 350 (6264), 1084–1089. doi: 10.1126/science.aac4255
Smith, P. M., Howitt, M. R., Panikov, N., Michaud, M., Gallini, C. A., Bohlooly, Y. M., et al. (2013). The Microbial Metabolites, Short-Chain Fatty Acids, Regulate Colonic Treg Cell Homeostasis. Science 341 (6145), 569–573. doi: 10.1126/science.1241165
Smits, L. P., Bouter, K. E., de Vos, W. M., Borody, T. J., Nieuwdorp, M. (2013). Therapeutic Potential of Fecal Microbiota Transplantation. Gastroenterology 145 (5), 946–953. doi: 10.1053/j.gastro.2013.08.058
Spees, A. M., Wangdi, T., Lopez, C. A., Kingsbury, D. D., Xavier, M. N., Winter, S. E., et al. (2013). Streptomycin-Induced Inflammation Enhances Escherichia Coli Gut Colonization Through Nitrate Respiration. mBio 4 (4), e00430–13. doi: 10.1128/mBio.00430-13
Staley, C., Kaiser, T., Beura, L. K., Hamilton, M. J., Weingarden, A. R., Bobr, A., et al. (2017). Stable Engraftment of Human Microbiota Into Mice With a Single Oral Gavage Following Antibiotic Conditioning. Microbiome 5 (1), 87. doi: 10.1186/s40168-017-0306-2
Staley, C., Kelly, C. R., Brandt, L. J., Khoruts, A., Sadowsky, M. J. (2016). Complete Microbiota Engraftment Is Not Essential for Recovery From Recurrent Clostridium Difficile Infection Following Fecal Microbiota Transplantation. mBio 7 (6), e01965–16. doi: 10.1128/mBio.01965-16
Stebegg, M., Silva-Cayetano, A., Innocentin, S., Jenkins, T. P., Cantacessi, C., Gilbert, C., et al. (2019). Heterochronic Faecal Transplantation Boosts Gut Germinal Centres in Aged Mice. Nat. Commun. 10 (1), 2443. doi: 10.1038/s41467-019-10430-7
Stefka, A. T., Feehley, T., Tripathi, P., Qiu, J., McCoy, K., Mazmanian, S. K., et al. (2014). Commensal Bacteria Protect Against Food Allergen Sensitization. Proc. Natl. Acad. Sci. U. S. A. 111 (36), 13145–13150. doi: 10.1073/pnas.1412008111
Strocchi, A., Bond, J. H., Ellis, C., Levitt, M. D. (1990). Colonic Concentrations of Hydrogen and Methane Following Colonoscopic Preparation With an Oral Lavage Solution. Gastrointest. Endosc. 36 (6), 580–582. doi: 10.1016/s0016-5107(90)71168-6
Sun, J., Xu, J., Ling, Y., Wang, F., Gong, T., Yang, C., et al. (2019). Fecal Microbiota Transplantation Alleviated Alzheimer’s Disease-Like Pathogenesis in APP/PS1 Transgenic Mice. Transl. Psychiatry 9 (1), 189. doi: 10.1038/s41398-019-0525-3
Sun, M. F., Zhu, Y. L., Zhou, Z. L., Jia, X. B., Xu, Y. D., Yang, Q., et al. (2018). Neuroprotective Effects of Fecal Microbiota Transplantation on MPTP-Induced Parkinson’s Disease Mice: Gut Microbiota, Glial Reaction and TLR4/TNF-Alpha Signaling Pathway. Brain Behav. Immun. 70, 48–60. doi: 10.1016/j.bbi.2018.02.005
Takahashi, M., Ishikawa, D., Sasaki, T., Lu, Y. J., Kuwahara-Arai, K., Kamei, M., et al. (2019). Faecal Freezing Preservation Period Influences Colonization Ability for Faecal Microbiota Transplantation. J. Appl. Microbiol. 126 (3), 973–984. doi: 10.1111/jam.14167
Tana, C., Umesaki, Y., Imaoka, A., Handa, T., Kanazawa, M., Fukudo, S. (2010). Altered Profiles of Intestinal Microbiota and Organic Acids May Be the Origin of Symptoms in Irritable Bowel Syndrome. Neurogastroenterol. Motil. 22 (5), 512–519, e114–e115. doi: 10.1111/j.1365-2982.2009.01427.x
Tedjo, D. I., Jonkers, D. M., Savelkoul, P. H., Masclee, A. A., van Best, N., Pierik, M. J., et al. (2015). The Effect of Sampling and Storage on the Fecal Microbiota Composition in Healthy and Diseased Subjects. PLoS One 10 (5), e0126685. doi: 10.1371/journal.pone.0126685
Thackray, L. B., Handley, S. A., Gorman, M. J., Poddar, S., Bagadia, P., Briseno, C. G., et al. (2018). Oral Antibiotic Treatment of Mice Exacerbates the Disease Severity of Multiple Flavivirus Infections. Cell Rep. 22 (13), 3440–3453. doi: 10.1016/j.celrep.2018.03.001. e3446.
Thaiss, C. A., Zeevi, D., Levy, M., Zilberman-Schapira, G., Suez, J., Tengeler, A. C., et al. (2014). Transkingdom Control of Microbiota Diurnal Oscillations Promotes Metabolic Homeostasis. Cell 159 (3), 514–529. doi: 10.1016/j.cell.2014.09.048
Thiennimitr, P., Winter, S. E., Winter, M. G., Xavier, M. N., Tolstikov, V., Huseby, D. L., et al. (2011). Intestinal Inflammation Allows Salmonella to Use Ethanolamine to Compete With the Microbiota. Proc. Natl. Acad. Sci. U. S. A. 108 (42), 17480–17485. doi: 10.1073/pnas.1107857108
Thomas, V., Clark, J., Dore, J. (2015). Fecal Microbiota Analysis: An Overview of Sample Collection Methods and Sequencing Strategies. Future Microbiol. 10 (9), 1485–1504. doi: 10.2217/fmb.15.87
Tomas, J., Wrzosek, L., Bouznad, N., Bouet, S., Mayeur, C., Noordine, M. L., et al. (2013). Primocolonization Is Associated With Colonic Epithelial Maturation During Conventionalization. FASEB J. 27 (2), 645–655. doi: 10.1096/fj.12-216861
Trivedi, V. B., Dave, A. P., Dave, J. M., Patel, B. C. (2007). Human Leukocyte Antigen and Its Role in Transplantation Biology. Transplant. Proc. 39 (3), 688–693. doi: 10.1016/j.transproceed.2007.01.066
Tropini, C., Moss, E. L., Merrill, B. D., Ng, K. M., Higginbottom, S. K., Casavant, E. P., et al. (2018). Transient Osmotic Perturbation Causes Long-Term Alteration to the Gut Microbiota. Cell 173 (7), 1742–1754.e1717. doi: 10.1016/j.cell.2018.05.008
Turnbaugh, P. J., Ridaura, V. K., Faith, J. J., Rey, F. E., Knight, R., Gordon, J. I. (2009). The Effect of Diet on the Human Gut Microbiome: A Metagenomic Analysis in Humanized Gnotobiotic Mice. Sci. Transl. Med. 1 (6), 6ra14. doi: 10.1126/scitranslmed.3000322
Turner, P. V., Brabb, T., Pekow, C., Vasbinder, M. A. (2011). Administration of Substances to Laboratory Animals: Routes of Administration and Factors to Consider. J. Am. Assoc. Lab. Anim. Sci. 50 (5), 600–613.
Wang, H., Cui, B., Li, Q., Ding, X., Li, P., Zhang, T., et al. (2018a). The Safety of Fecal Microbiota Transplantation for Crohn’s Disease: Findings From A Long-Term Study. Adv. Ther. 35 (11), 1935–1944. doi: 10.1007/s12325-018-0800-3
Wang, Z., Hua, W., Li, C., Chang, H., Liu, R., Ni, Y., et al. (2019). Protective Role of Fecal Microbiota Transplantation on Colitis and Colitis-Associated Colon Cancer in Mice Is Associated With Treg Cells. Front. Microbiol. 10, 2498. doi: 10.3389/fmicb.2019.02498
Wang, S., Huang, M., You, X., Zhao, J., Chen, L., Wang, L., et al. (2018b). Gut Microbiota Mediates the Anti-Obesity Effect of Calorie Restriction in Mice. Sci. Rep. 8 (1), 1–14. doi: 10.1038/s41598-018-31353-1
Wei, Y. L., Chen, Y. Q., Gong, H., Li, N., Wu, K. Q., Hu, W., et al. (2018). Fecal Microbiota Transplantation Ameliorates Experimentally Induced Colitis in Mice by Upregulating AhR. Front. Microbiol. 9, 1921. doi: 10.3389/fmicb.2018.01921
Winter, S. E., Winter, M. G., Xavier, M. N., Thiennimitr, P., Poon, V., Keestra, A. M., et al. (2013). Host-Derived Nitrate Boosts Growth of E. Coli in the Inflamed Gut. Science 339 (6120), 708–711. doi: 10.1126/science.1232467
Wrzosek, L., Ciocan, D., Borentain, P., Spatz, M., Puchois, V., Hugot, C., et al. (2018). Transplantation of Human Microbiota Into Conventional Mice Durably Reshapes the Gut Microbiota. Sci. Rep. 8 (1), 6854. doi: 10.1038/s41598-018-25300-3
Wu, Y. Y., Hsu, C. M., Chen, P. H., Fung, C. P., Chen, L. W. (2014). Toll-Like Receptor Stimulation Induces Nondefensin Protein Expression and Reverses Antibiotic-Induced Gut Defense Impairment. Infect. Immun. 82 (5), 1994–2005. doi: 10.1128/IAI.01578-14
Xu, H., Liu, M., Cao, J., Li, X., Fan, D., Xia, Y., et al. (2019). The Dynamic Interplay Between the Gut Microbiota and Autoimmune Diseases. J. Immunol. Res. 2019, 7546047. doi: 10.1155/2019/7546047
Yamano, H. O., Matsushita, H. O., Yoshikawa, K., Takagi, R., Harada, E., Tanaka, Y., et al. (2016). Randomised Clinical Study Comparing the Effectiveness and Physiological Effects of Hypertonic and Isotonic Polyethylene Glycol Solutions for Bowel Cleansing. BMJ Open Gastroenterol. 3 (1), e000101. doi: 10.1136/bmjgast-2016-000101
Yang, C., Fang, X., Zhan, G., Huang, N., Li, S., Bi, J., et al. (2019). Key Role of Gut Microbiota in Anhedonia-Like Phenotype in Rodents With Neuropathic Pain. Transl. Psychiatry 9 (1), 57. doi: 10.1038/s41398-019-0379-8
Yan, J., Herzog, J. W., Tsang, K., Brennan, C. A., Bower, M. A., Garrett, W. S., et al. (2016). Gut Microbiota Induce IGF-1 and Promote Bone Formation and Growth. Proc. Natl. Acad. Sci. U. S. A. 113 (47), E7554–E7563. doi: 10.1073/pnas.1607235113
Zackular, J. P., Baxter, N. T., Chen, G. Y., Schloss, P. D. (2016). Manipulation of the Gut Microbiota Reveals Role in Colon Tumorigenesis. mSphere 1 (1), e00001–15. doi: 10.1128/mSphere.00001-15
Zakostelska, Z., Malkova, J., Klimesova, K., Rossmann, P., Hornova, M., Novosadova, I., et al. (2016). Intestinal Microbiota Promotes Psoriasis-Like Skin Inflammation by Enhancing Th17 Response. PLoS One 11 (7), e0159539. doi: 10.1371/journal.pone.0159539
Zarrinpar, A., Chaix, A., Yooseph, S., Panda, S. (2014). Diet and Feeding Pattern Affect the Diurnal Dynamics of the Gut Microbiome. Cell Metab. 20 (6), 1006–1017. doi: 10.1016/j.cmet.2014.11.008
Zhang, H., Sparks, J. B., Karyala, S. V., Settlage, R., Luo, X. M. (2015). Host Adaptive Immunity Alters Gut Microbiota. ISME J. 9 (3), 770–781. doi: 10.1038/ismej.2014.165
Zhou, D., Pan, Q., Shen, F., Cao, H. X., Ding, W. J., Chen, Y. W., et al. (2017). Total Fecal Microbiota Transplantation Alleviates High-Fat Diet-Induced Steatohepatitis in Mice via Beneficial Regulation of Gut Microbiota. Sci. Rep. 7 (1), 1529. doi: 10.1038/s41598-017-01751-y
Zhou, J., Zhou, Z., Ji, P., Ma, M., Guo, J., Jiang, S. (2019). Effect of Fecal Microbiota Transplantation on Experimental Colitis in Mice. Exp. Ther. Med. 17 (4), 2581–2586. doi: 10.3892/etm.2019.7263
Keywords: fecal microbiota transplantation, mice, microbiome, engraftment, procedure
Citation: Bokoliya SC, Dorsett Y, Panier H and Zhou Y (2021) Procedures for Fecal Microbiota Transplantation in Murine Microbiome Studies. Front. Cell. Infect. Microbiol. 11:711055. doi: 10.3389/fcimb.2021.711055
Received: 18 May 2021; Accepted: 24 August 2021;
Published: 21 September 2021.
Edited by:
Felix Broecker, Idorsia Pharmaceuticals Ltd, SwitzerlandReviewed by:
Yuanqiang Zou, Beijing Genomics Institute (BGI), ChinaCopyright © 2021 Bokoliya, Dorsett, Panier and Zhou. This is an open-access article distributed under the terms of the Creative Commons Attribution License (CC BY). The use, distribution or reproduction in other forums is permitted, provided the original author(s) and the copyright owner(s) are credited and that the original publication in this journal is cited, in accordance with accepted academic practice. No use, distribution or reproduction is permitted which does not comply with these terms.
*Correspondence: Yanjiao Zhou, eWF6aG91QHVjaGMuZWR1
Disclaimer: All claims expressed in this article are solely those of the authors and do not necessarily represent those of their affiliated organizations, or those of the publisher, the editors and the reviewers. Any product that may be evaluated in this article or claim that may be made by its manufacturer is not guaranteed or endorsed by the publisher.
Research integrity at Frontiers
Learn more about the work of our research integrity team to safeguard the quality of each article we publish.