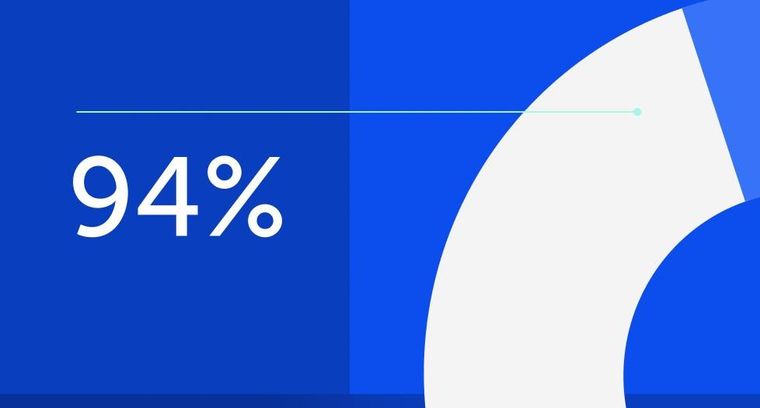
94% of researchers rate our articles as excellent or good
Learn more about the work of our research integrity team to safeguard the quality of each article we publish.
Find out more
PERSPECTIVE article
Front. Cell. Infect. Microbiol., 09 April 2021
Sec. Parasite and Host
Volume 11 - 2021 | https://doi.org/10.3389/fcimb.2021.660679
This article is part of the Research TopicCelebrating Microbial Diversity: The Many Cell Cycles of Eukaryotic MicrobesView all 7 articles
Plasmodium, the unicellular parasite that causes malaria, evolved a highly unusual mode of reproduction. During its complex life cycle, invasive or transmissive stages alternate with proliferating stages, where a single parasite can produce tens of thousands of progeny. In the clinically relevant blood stage of infection, the parasite replicates its genome up to thirty times and forms a multinucleated cell before daughter cells are assembled. Thus, within a single cell cycle, Plasmodium develops from a haploid to a polypoid cell, harboring multiple copies of its genome. Polyploidy creates several biological challenges, such as imbalances in genome output, and cells can respond to this by changing their size and/or alter the production of RNA species and protein to achieve expression homeostasis. However, the effects and possible adaptations of Plasmodium to the massively increasing DNA content are unknown. Here, we revisit and embed current Plasmodium literature in the context of polyploidy and propose potential mechanisms of the parasite to cope with the increasing gene dosage.
Malaria is caused by unicellular eukaryotic parasites of the genus Plasmodium. Several species of this evolutionary very distinct genus cause malaria in humans, with Plasmodium falciparum (P. falciparum) being the most virulent one. Plasmodium spp. display a complex life cycle, alternating between a mosquito and a vertebrate host. Observing the parasite population size throughout its life cycle reveals two striking numerical bottlenecks, which occur each time the parasite changes its host (Cowman et al., 2016; Matthews et al., 2018). To compensate for these losses the parasite proliferates massively. After transmission to the mosquito, one cell cycle produces hundreds to thousands of daughter cells in a stage called oocyst. After transmission to the human host, P. falciparum proliferates first in hepatocytes. Here, one cell cycle of the parasite can give rise to tens of thousands of daughter cells, which leave the liver and infect red blood cells, where they continue to proliferate. This additional proliferation establishes parasite densities in peripheral blood that are sufficiently high to ensure transmission during an ensuing mosquito blood meal. All three described parasite proliferation events result in the formation of a polyploid and multinucleated cell before daughter cells are assembled during a relatively synchronous mass cytokinesis event (Prudêncio et al., 2006; Burda et al., 2017; Spreng et al., 2019; Rudlaff et al., 2020; Simon et al., this issue). Thus, at a given time, the parasite cytoplasm can harbor one to dozens or even thousands of genome copies.
Other examples of cells (and organisms) with a ‘higher-than-usual’ DNA content can be found in many eukaryotes and on all levels of biological organization (Edgar et al., 2014; Fox et al., 2020). The cells of many plants, domesticated or not, are polyploid. In mammals, polyploid cells are found for example in the liver or in the bone marrow (Gillooly et al., 2015) (Figure 1A). Polyploidy increases the size of the cell and can allow for higher genomic output, i.e., RNA species and protein (Osborn et al., 2003; Comai, 2005; Otto, 2007; Parisod et al., 2010; Marshall et al., 2012; Edgar et al., 2014; Frawley and Orr-Weaver, 2015; Schoenfelder and Fox, 2015; Fox et al., 2020). For example, the silk gland cells of the silk worm Bombyx mori increase their genomic DNA content up to 4 x105 times during larval development, enhancing the secretion of macromolecules (Gage, 1974).
Figure 1 Distribution of nuclear DNA content and cell volume in eukaryotic cells. (A) Relative DNA content and cell size of P. falciparum (40 hours post invasion, hpi, of an erythrocyte) and human cells with varying ploidy based on Gillooly et al., 2015. Each point represents the fold increase in cell size and DNA content relative to the smallest cell with the lowest DNA content of each population. (B) Relative DNA content and cell size of 25 species of unicellular eukaryotes in G1 phase based on Table 2 from Shuter et al., 1983. Here, the relative cell size and DNA content was normalized to the Saccharomyces cerevisiae. Note: Values for P. falciparum were derived as follows: estimated DNA content before DNA replication in femtogram reported by Mangold et al., 2005; ploidy level based on Ganter et al., 2017 and Simon et al., this issue; cell volume estimated from Waldecker et al., 2017.
When additional genomic output is not needed, an increased gene dosage can be detrimental, likely as a result of disrupted gene expression homeostasis (Sheltzer and Amon, 2011; Birchler and Veitia, 2012). This imbalance can have severe adverse effects, which can be seen in chromosomal disorders like trisomy. Here, the additional copy of a single chromosome leads to an imbalance in protein concentration, resulting in frequent miscarriages (Herbert et al., 2015). Sex chromosomes also cause an imbalance and compensatory mechanisms have evolved (Wutz and Gribnau, 2007; Lucchesi and Kuroda, 2015; Disteche, 2016). For example, in many female mammals compensation is achieved by the inactivation of one of the two X-chromosomes (Wutz and Gribnau, 2007). Conversely, in male Drosophila cells, transcription from the single X-chromosome is increased two-fold (Lucchesi and Kuroda, 2015).
However, our understanding of the impact of increasing DNA content for Plasmodium is very rudimentary and not discussed in the literature. Yet, investigating the effects of increasing DNA content, and thus gene dosage, is key to our understanding of other aspects of parasite biology. Hence, in this Perspective we discuss potential strategies of Plasmodium to cope with polyploidy and highlight some gaps in our knowledge of Plasmodium biology.
In many cells, polyploidy is associated with an increased cell size. While the causal relationship between the two remains unclear (Sugimoto-Shirasu and Roberts, 2003; Wood and Nurse, 2015), a positive correlation between DNA content and cell size can be observed in bacteria, plants and mammals (Jovtchev et al., 2006; Fomina-Yadlin et al., 2014). Strikingly, this can also be seen in cells of the same type, for example human megakaryocytes vary dramatically in their ploidy level with megakaryocytes of higher ploidy being substantially larger than megakaryocytes of lower ploidy (Gillooly et al., 2015). Thus, increasing the cell size may be a strategy for P. falciparum to cope with the increasing DNA content.
Data to test this hypothesis are scarce, especially for the proliferating parasite stages in the mosquito and the liver. For blood stage parasites, several studies report that the DNA content (or the number of daughter cells as proxy) increases 20-fold on average within one proliferative cycle and individual cells with an up to 30-fold increase can be found occasionally (Ganter et al., 2017; Simon et al., in this issue). But, to our knowledge, only Waldecker et al. quantified the volume occupied by P. falciparum inside the erythrocyte over time (Waldecker et al., 2017). They found that blood stage parasites grow approximately 3-fold during the so-called ring and trophozoite stage, and approximately 7-fold during the complete proliferative cycle. Whether this 7-fold increase in cell size is sufficient to cope with the up to 30-fold increase in DNA content is difficult to gauge. Human cells of the same type but with different ploidy levels resemble somewhat the situation in the parasite, in terms of the change in DNA content over time (in contrast to polyploid species, where the level of polyploidy is stable over time). Plotting the relative cell size versus relative DNA content, P. falciparum clearly segregates from human cells, as the parasite displays a comparatively small increase in size in relation to the DNA content (Gillooly et al., 2015) (Figure 1A). This would argue against increasing cell size as major coping mechanism. Nevertheless, the sizes and DNA contents of the developing P. falciparum blood-stage parasite still fall within the range of size vs. DNA content of other unicellular eukaryotes (Shuter et al., 1983) (Figure 1B). Whether this interspecies comparison is valid remains questionable, since only the pre-DNA replication values were reported for the other unicellular eukaryotes. Another major caveat is the comparison of a relatively stable situation with dynamic events, as both size and DNA content change quickly within one proliferative cycle of the parasite (Ganter et al., 2017; Waldecker et al., 2017). Thus, it remains unclear, whether increasing cell size plays a role for coping with increasing DNA content in the blood stage of infection. High- and super resolution microscopy may allow for the parallel quantification of cell size and DNA content of replicating parasites in the blood, mosquito, and liver stage. These analyses will provide valuable insights to better understand the interplay between cell size and DNA content.
During S-phase of the cell cycle and before cytokinesis, a similar dynamic change of DNA content also occurs: While cells grow continuously, their DNA content doubles abruptly (Bar-Ziv et al., 2016). For bacteria it was shown that the expression of a given gene increases shortly after it is replicated (Chandler and Pritchard, 1975; Schmid and Roth, 1987). Eukaryotic transcription, however, is buffered against increasing gene dosage during S-phase (Killander and Zetterberg, 1965; Elliott and McLaughlin, 1978; Bar-Ziv et al., 2016). It was shown for Saccharomyces cerevisiae that transcription from recently synthetized DNA is reduced, which depends on acetylation of a histone H3 lysine residue at position 56 (Voichek et al., 2016). Thus, we asked whether altering the genome output could also be a mechanism employed by P. falciparum to cope with the increasing DNA content. This hypothesis would predict little or no correlation between DNA and total RNA content of the cell. This notion is supported by a study from the mid 1980’s, which found the rate of total RNA synthesis to peak at approximately 38 hours post invasion (hpi), while the rate of DNA replication peaked at approximately 45 hpi (De Rojas and Wasserman, 1985). Employing nuclear run-on assays Sims et al., 2009 reported highest transcriptional activity in parasites with a 10-fold increased DNA content, but a linear correlation between the number of nuclei per cell and RNA polymerase II-dependent transcriptional activity was not found (Sims et al., 2009). This may indicate a mechanism that balances DNA content and the transcription of messenger RNA (mRNA). To provide more insights into the relationship of total DNA and total RNA content over time during parasite development in the blood stage, we measured the DNA and, indirectly, the total RNA content by flow cytometry. This estimation found that the total RNA content peaked approximately 12 hours before the DNA content reached a maximum (Figure 2, top panel). In addition, similar RNA contents were also reached in parasites where DNA replication was genetically blocked (Figure 2, bottom panel). Together this suggest limited or no scaling of total RNA content and total DNA content and strongly supports the existence of a mechanism that buffers genome output against increasing DNA content in P. falciparum. In such a scenario, the parasite could use shared pools of nucleotide precursors more efficiently. Yet, to be able to draw firm conclusions, more data are needed on the correlation of total RNA and total DNA content over time. In addition, quantifying the relative amount of the different RNA species in relation to the DNA content will not only inform on the relative contribution to the total RNA content but may also shed light on the regulatory mechanism that drive the expression of the different RNA species.
Figure 2 Estimation of total DNA and total RNA content during a proliferative cycle of P. falciparum blood stages. Synchronized cultures of DNA replicating (PfCRK4-DD on Shield-1) and non-DNA replicating (PfCRK4-DD off Shield-1; Ganter et al., 2017) parasites were treated with or without RNase. Subsequently, parasites were stained with SYBR green I, which labels both DNA and RNA (Theron et al., 2010; Ganter et al., 2017) and analyzed by flow cytometry. Total DNA and total RNA contents were estimated by calculating the difference in signal intensity with and without RNase treatment. Total DNA and total RNA contents were normalized by Min-Max scaling using the minimum and maximum values for DNA and RNA regardless of whether parasites replicate DNA or not.
Two other observations support the hypothesis of a reduced genome output in parasites with an increased DNA content. Firstly, the distribution and number of nuclear pore complexes (NPCs) is highly dynamic during one proliferative cycle. The number of NPCs per nucleus peaks in late trophozoites (1 to 2 genome copies) and then decrease gradually as nuclei divide (Weiner et al., 2011; Dahan-Pasternak et al., 2013; Guizetti et al., 2013; Hollin and Le Roch, 2020). It is also at the late trophozoite stage when NPCs show a redistribution from a polarized to a more dispersed localization across the nuclei (Weiner et al., 2011). Interestingly, these phenomena coincide with the peak in total RNA content (Figure 2). Thus it is reasonable to assume that gene gating also occurs in Plasmodium (Blobel, 1985; Cabal et al., 2006; Mendjan et al., 2006; Weiner et al., 2011; Dahan-Pasternak et al., 2013; Raices and D’Angelo, 2017) and that the NPCs are potentially involved in the regulation of gene expression.
Secondly, transcription in P. falciparum was described as not responsive to environmental perturbations (Gunasekera et al., 2007; Ganesan et al., 2008; Young et al., 2008). For cells with a changing DNA content, being not responsive may be beneficial as it intuitively appears difficult to equally integrate an external stimulus in a cell with one or multiple copies of the genome. Nevertheless, the significance of these observations in the context of polyploidy and genome output remains unclear.
Another observation contributes to our picture of transcription in polyploid P. falciparum during the blood stage. Labeling of nascent RNA with 5-bromouridine 5′-triphosphate (BrUTP) reported an uneven staining among the different nuclei of the parasite, suggesting differential transcriptional activity (Moraes et al., 2013). This indicates that there may be unexplored means of transcriptional regulation in multinucleated stages. To further investigate potential qualitative and quantitative transcriptional differences between nuclei of a given cell, more experiments are needed. For example, investigating all three Plasmodium RNA polymerases (I, II and III) and assessing their spatiotemporal localization dynamics in multinucleated cells will be informative (Zhao et al., 2016). Moreover, single-molecule fluorescence in situ hybridization (smFISH) of different RNA transcripts (e.g., ‘housekeeping’ genes and stage specific genes) can provide high-resolution snapshots of the transcription and localization of different RNAs at defined time points (Kramer et al., 2020). Integrating such data with RNA species quantification in blood parasites and also liver and mosquito stages, will shed light on the potential mechanisms that are in play to buffer against the increasing DNA content.
The apparent independence of total RNA content and total DNA content over time could be achieved in different ways, which individually or in concert may help to maintain gene expression homeostasis. The total RNA output could be altered by activating only a subset of genomes or all genomes transcribe RNA, but each produces less. How exactly the parasite responds to the increasing DNA content is unknown but cues may be found in the literature on Plasmodium mRNA transcription. To gain insight, we need to compare temporal information on mRNA transcription and DNA content, which however may originate from independent analysis. Thus, drawing robust conclusions is difficult. This is further complicated by the use of different methods to classify the developmental stage of the parasite, which is sometimes reported as ‘hours post invasion’ and sometimes done by morphology (e.g., ring stage, trophozoite, schizont). In addition, when using transcriptional profiles of mRNAs as a proxy for total genome output, it is imperative to bear in mind that mRNAs may only represent a very small fraction of the total RNA. In other eukaryotic organisms, ribosomal RNAs account for approximately 80% of the total RNA content. Another approximately 15% are transfer RNAs and the remaining 5% are other RNA species, including mRNAs, non-coding RNAs, and micro RNAs (Westermann et al., 2012). But to our knowledge it is unclear how much the different RNA species contribute to the total RNA content of Plasmodium blood stages.
A landmark study that used microarrays to profile the P. falciparum transcriptome reported iconic transcriptional cascades (Bozdech et al., 2003). These cascades are thought to ensure gene expression only at times when the gene product is required. Several studies support these results, including a recent study, which profiled nascent and stabilized mRNA separately. This work reported active transcription throughout the proliferative cycle and showed that both nascent mRNA transcription and mRNA stabilization contribute to the observed transcriptional cascades (Painter et al., 2018). Moreover, several distinct periods of transcriptional bursts were identified. This ‘just-in-time’ transcription could maintain expression homeostasis, e.g., through variable promoter strength. In such a scenario, relatively strong promoters would drive expression of proteins that are needed before the DNA content increases and comparatively weak promoters would drive the expression of proteins needed after the DNA content increased. ‘Just-in-time’ transcription requires sophisticated regulation, which can be achieved by transcription factors. Only one family of 27 transcription factors was identified in the P. falciparum genome, the Apicomplexan AP2 transcription factors, which bind diverse DNA motifs that group functionally-related genes (Campbell et al., 2010). As the P. falciparum genome harbors over 5000 genes, this relatively small repertoire suggests that other regulatory mechanisms, e.g., post-transcriptional or translational, play an important role to ensure a ‘just-in-time’ expression. Indeed, transcripts for almost 90% of the P. falciparum genome can be detected in blood-stage parasites, including genes that are specific for the mosquito- and liver stages of the parasite’s life cycle (e.g. CSP, TRAP, SPECT2, STARP) (Le Roch et al., 2004; Otto et al., 2010; Painter et al., 2018; Toenhake et al., 2018; Chappell et al., 2020), supporting an important role for post-transcriptional regulation of gene expression.
However, a study that used nuclear run-on assays and nascent RNA sequencing to profile mRNA transcription reported that most protein-coding genes were transcribed during a single burst, suggesting an ‘all-at-once’ model of transcription (Lu et al., 2017). As the reported transcriptional activation occurred in a developmental stage that presumably harbors only a single copy of the genome, ‘all-at-once’ transcription represents a conceptually simple way to cope with the increasing DNA content. Indirect support for this model comes from studies that observed a genome-wide drop in nucleosome levels approximately at the time when the transcriptional burst was detected and nucleosomes reassembled in the stages that replicate DNA (Ponts et al., 2010; Bunnik et al., 2014; Batugedara and Le Roch, 2019). In contrast, another study reported highly similar nucleosome occupancy at different developmental stages (Kensche et al., 2015).
In an ‘all-at-once’ scenario, proteins need to be provided long after the transcriptional burst. This could be achieved through stabilized mRNAs and/or translational repression (Vembar et al., 2016). Indeed, Shock et al., 2007 reported that the mRNA half-life is substantially longer in polyploid parasites (Shock et al., 2007). This stabilization is likely accomplished by RNA-binding proteins, which make up approximately 4-10% of the P. falciparum genome and are increasingly transcribed in stages that replicate DNA (Painter et al., 2018). Translational repression plays an important role during other stages of the Plasmodium life cycle (Mair et al., 2006), but data on global and/or gene-specific translational control within one proliferative cycle in the blood stage of P. falciparum remains sparse. Translational repression was shown for the P. falciparum dihydrofolate reductase-thymidylate synthase, which binds the coding region of its own mRNA, thus repressing translation (Zhang and Rathod, 2002). More recently, it was reported that P. falciparum alba1 stabilizes up to 1193 transcripts and prevents their translation (Vembar et al., 2015). Although these reports on mRNA stabilization and translational repression are in line with an ‘all-at-once’ transcription, more data are needed to answer the current and emerging questions regarding transcription in Plasmodium.
Regardless of in favor of one or the other model of transcription, active mRNA transcription in polyploid stages was shown (Bozdech et al., 2003; Le Roch et al., 2004; Sims et al., 2009; Otto et al., 2010; Lu et al., 2017; Painter et al., 2018; Reid et al., 2018) and enzymatically active RNA polymerase II complex was detected in cells with multiple genomes (Rai et al., 2014). Moreover, dynamic histone acetylation and promoter opening can be observed throughout the proliferative cycle, further supporting active transcription in polyploid stages (Bártfai et al., 2010; Gupta et al., 2013; Josling et al., 2015; Kensche et al., 2015; Lu et al., 2017; Toenhake et al., 2018). Many of the corresponding gene products expressed at this stage play a role for erythrocyte invasion or are important to establish the intraerythrocytic compartment, in which the parasite resides. Proteins of the up to 30 daughter cells are likely needed at a relatively high copy number and it is tempting to speculate that an increasing DNA content could be beneficial for this subset of genes. Still, whether the dynamics of mRNA transcription are a valid surrogate for the total genomic output that includes all RNA species remains to be determined.
Plasmodium parasites increase their cellular DNA content massively during one proliferative cycle. In other cells, such changes in the DNA content and, thus, the level of ploidy, are accompanied by an increased cell size and/or altered genome output. But how Plasmodium copes with the increasing DNA content remains elusive. Without experiments specifically designed to address this question, it is difficult to draw firm conclusions. The possible contribution of increasing cell size as a means to cope with the increased DNA content remains to be investigated in detail. The currently available data suggest a temporal separation of total RNA production and DNA replication as coping mechanism, but only quantification of genomic output over time (i.e., all different RNA species and protein) in relation to DNA content and cell volume can inform on this aspect of Plasmodium biology. The emerging single-cell approaches (Poran et al., 2017; Reid et al., 2018; Walzer et al., 2019), probe-independent RNA sequencing with a theoretically infinite dynamic range, and parallel quantification of the DNA content will be critical. Indeed, using spike-in RNA standards and different normalizations approaches (reads per transcriptome, per gene copy number, and per cell) were successful in detecting specific changes in transcript stoichiometry and abundance across polyploid species (Visger et al., 2019; Song et al., 2020). Addressing this aspect of Plasmodium biology and revealing how the parasite keeps genome output in homeostasis is an important step to understand the impact of increasing DNA content on Plasmodium evolution.
The raw data supporting the conclusions of this article will be made available by the authors, without undue reservation.
MM and MG conceived this study. MM and SS reviewed the literature. MM, SS, and MG wrote the manuscript. All authors contributed to the article and approved the submitted version.
This work was funded by the Deutsche Forschungsgemeinschaft (DFG, German Research Foundation)—Project number 240245660—SFB 1129 and the Baden-Württemberg Foundation (ref: 1.16101.17) to MG, and the Fundação para a Ciência e Tecnologia (FCT, Portugal)—PD/BD/128002/2016 to MM.
The authors declare that the research was conducted in the absence of any commercial or financial relationships that could be construed as a potential conflict of interest.
We are very grateful to Manoj Duraisingh, as the data shown in Figure 2 was generated while MG was a post-doc in his laboratory at Harvard T.H. Chan School of Public Health. We are grateful to the Plasmodium genomics resource PlasmoDB, which facilitated this research. We thank Julien Guizetti and Miguel Prudêncio, for critical reading the manuscript.
Bártfai, R., Hoeijmakers, W. A. M., Salcedo-Amaya, A. M., Smits, A. H., Janssen-Megens, E., Kaan, A., et al. (2010). H2A.Z demarcates intergenic regions of the Plasmodium falciparum epigenome that are dynamically marked by H3K9ac and H3K4me3. PloS Pathog. 6, e1001223. doi: 10.1371/journal.ppat.1001223
Bar-Ziv, R., Voichek, Y., Barkai, N. (2016). Chromatin dynamics during DNA replication. Genome Res. 26, 1245–1256. doi: 10.1101/gr.201244.115
Batugedara, G., Le Roch, K. G. (2019). Unraveling the 3D genome of human malaria parasites. Semin. Cell Dev. Biol. 90, 144–153. doi: 10.1016/j.semcdb.2018.07.015
Birchler, J. A., Veitia, R. A. (2012). Gene balance hypothesis: Connecting issues of dosage sensitivity across biological disciplines. Proc. Natl. Acad. Sci. U. S. A. 109, 14746–14753. doi: 10.1073/pnas.1207726109
Blobel, G. (1985). Gene gating: A hypothesis. Proc. Natl. Acad. Sci. U. S. A. 82, 8527–8529. doi: 10.1073/pnas.82.24.8527
Bozdech, Z., Llinás, M., Pulliam, B. L., Wong, E. D., Zhu, J., DeRisi, J. L. (2003). The transcriptome of the intraerythrocytic developmental cycle of Plasmodium falciparum. PLoS Biol. 1, 85–100. doi: 10.1371/journal.pbio.0000005
Bunnik, E. M., Polishko, A., Prudhomme, J., Ponts, N., Gill, S. S., Lonardi, S., et al. (2014). DNA-encoded nucleosome occupancy is associated with transcription levels in the human malaria parasite Plasmodium falciparum. BMC Genomics 15, 1–15. doi: 10.1186/1471-2164-15-347
Burda, P. C., Schaffner, M., Kaiser, G., Roques, M., Zuber, B., Heussler, V. T. (2017). A Plasmodium plasma membrane reporter reveals membrane dynamics by live-cell microscopy. Sci. Rep. 7, 1–14. doi: 10.1038/s41598-017-09569-4
Cabal, G. G., Genovesio, A., Rodriguez-Navarro, S., Zimmer, C., Gadal, O., Lesne, A., et al. (2006). SAGA interacting factors confine sub-diffusion of transcribed genes to the nuclear envelope. Nature 441, 770–773. doi: 10.1038/nature04752
Campbell, T. L., de Silva, E. K., Olszewski, K. L., Elemento, O., Llinás, M. (2010). Identification and Genome-Wide Prediction of DNA Binding Specificities for the ApiAP2 family of regulators from the malaria parasite. PLoS Pathog. 6, e1001165. doi: 10.1371/journal.ppat.1001165
Chandler, M. G., Pritchard, R. H. (1975). The effect of gene concentration and relative gene dosage on gene output in Escherichia coli. Mol. Gen. Genet. 138, 127–141. doi: 10.1007/BF02428117
Chappell, L., Ross, P., Orchard, L., Russell, T. J., Otto, T. D., Berriman, M., et al. (2020). Refining the transcriptome of the human malaria parasite Plasmodium falciparum using amplification-free RNA-seq. BMC Genomics 21, 1–19. doi: 10.1186/s12864-020-06787-5
Comai, L. (2005). The advantages and disadvantages of being polyploid. Nat. Rev. Genet. 6, 836–846. doi: 10.1038/nrg1711
Cowman, A. F., Healer, J., Marapana, D., Marsh, K. (2016). Malaria: Biology and Disease. Cell 167, 610–624. doi: 10.1016/j.cell.2016.07.055
Dahan-Pasternak, N., Nasereddin, A., Kolevzon, N., Pe’er, M., Wong, W., Shinder, V., et al. (2013). Pfsec13 is an unusual chromatin-associated nucleoporin of plasmodium falciparum that is essential for parasite proliferation in human erythrocytes. J. Cell Sci. 126, 3055–3069. doi: 10.1242/jcs.122119
De Rojas, M. O., Wasserman, M. (1985). Temporal relationships on macromolecular synthesis during the asexual cell cycle of plasmodium falciparum. Trans. R. Soc. Trop. Med. Hyg. 79, 792–796. doi: 10.1016/0035-9203(85)90119-1
Disteche, C. M. (2016). Dosage compensation of the sex chromosomes and autosomes. Semin. Cell Dev. Biol. 56, 9–18. doi: 10.1016/j.semcdb.2016.04.013
Edgar, B. A., Zielke, N., Gutierrez, C. (2014). Endocycles: A recurrent evolutionary innovation for post-mitotic cell growth. Nat. Rev. Mol. Cell Biol. 15, 197–210. doi: 10.1038/nrm3756
Elliott, S. G., McLaughlin, C. S. (1978). Rate of macromolecular synthesis through the cell cycle of the yeast Saccharomyces cerevisiae. Proc. Natl. Acad. Sci. U. S. A. 75, 4384–4388. doi: 10.1073/pnas.75.9.4384
Fomina-Yadlin, D., Du, Z., McGrew, J. T. (2014). Gene expression measurements normalized to cell number reveal large scale differences due to cell size changes, transcriptional amplification and transcriptional repression in CHO cells. J. Biotechnol. 189, 58–69. doi: 10.1016/j.jbiotec.2014.08.037
Fox, D. T., Soltis, D. E., Soltis, P. S., Ashman, T. L., Van de Peer, Y. (2020). Polyploidy: A Biological Force From Cells to Ecosystems. Trends Cell Biol. 30, 688–694. doi: 10.1016/j.tcb.2020.06.006
Frawley, L. E., Orr-Weaver, T. L. (2015). Polyploidy. Curr. Biol. 25, 353–358. doi: 10.1016/j.cub.2015.03.037
Gage, L. P. (1974). Polyploidization of the silk gland of Bombyx mori. J. Mol. Biol. 86, 97–108. doi: 10.1016/S0022-2836(74)80010-0
Ganesan, K., Ponmee, N., Jiang, L., Fowble, J. W., White, J., Kamchonwongpaisan, S., et al. (2008). A genetically hard-wired metabolic transcriptome in Plasmodium falciparum fails to mount protective responses to lethal antifolates. PLoS Pathog. 4, e1000214. doi: 10.1371/journal.ppat.1000214
Ganter, M., Goldberg, J. M., Dvorin, J. D., Paulo, J. A., Jonas, G., Tripathi, A. K., et al. (2017). Plasmodium falciparum CRK4 directs continuous rounds of DNA replication during schizogony. Nat. Microbiol. 2, 17017. doi: 10.1038/nmicrobiol.2017.17
Gillooly, J. F., Hein, A., Damiani, R. (2015). Nuclear DNA content varies with cell size across human cell types. Cold Spring Harb. Perspect. Biol. 7, 1–27. doi: 10.1101/cshperspect.a019091
Guizetti, J., Martins, R. M., Guadagnini, S., Claes, A., Scherf, A. (2013). Nuclear pores and perinuclear expression sites of var and ribosomal DNA genes correspond to physically distinct regions in Plasmodium falciparum. Eukaryot. Cell. 12, 697–702. doi: 10.1128/EC.00023-13
Gunasekera, A. M., Myrick, A., Le Roch, K., Winzeler, E., Wirth, D. F. (2007). Plasmodium falciparum: Genome wide perturbations in transcript profiles among mixed stage cultures after chloroquine treatment. Exp. Parasitol. 117, 87–92. doi: 10.1016/j.exppara.2007.03.001
Gupta, A. P., Chin, W. H., Zhu, L., Mok, S., Luah, Y. H., Lim, E. H., et al. (2013). Dynamic Epigenetic Regulation of Gene Expression during the Life Cycle of Malaria Parasite Plasmodium falciparum. PLoS Pathog. 9, e1003170. doi: 10.1371/journal.ppat.1003170
Herbert, M., Kalleas, D., Cooney, D., Lamb, M., Lister, L. (2015). Meiosis and maternal aging: Insights from aneuploid oocytes and trisomy births. Cold Spring Harb. Perspect. Biol. 7, a017970. doi: 10.1101/cshperspect.a017970
Hollin, T., Le Roch, K. G. (2020). From Genes to Transcripts, a Tightly Regulated Journey in Plasmodium. Front. Cell Infect. Microbiol. 10, 618454. doi: 10.3389/fcimb.2020.618454
Josling, G. A., Petter, M., Oehring, S. C., Gupta, A. P., Dietz, O., Wilson, D. W., et al. (2015). A Plasmodium Falciparum Bromodomain Protein Regulates Invasion Gene Expression. Cell Host Microbe 17, 741–751. doi: 10.1016/j.chom.2015.05.009
Jovtchev, G., Schubert, V., Meister, A., Barow, M., Schubert, I. (2006). Nuclear DNA content and nuclear and cell volume are positively correlated in angiosperms. Cytogenet. Genome Res. 114, 77–82. doi: 10.1159/000091932
Kensche, P. R., Hoeijmakers, W. A. M., Toenhake, C. G., Bras, M., Chappell, L., Berriman, M., et al. (2015). The nucleosome landscape of Plasmodium falciparum reveals chromatin architecture and dynamics of regulatory sequences. Nucleic Acids Res. 44, 2110–2124. doi: 10.1093/nar/gkv1214
Killander, D., Zetterberg, A. (1965). Quantitative cytochemical studies on interphase growth. I. Determination of DNA, RNA and mass content of age determined mouse fibroblasts in vitro and of intercellular variation in generation time. Exp. Cell Res. 38, 272–284. doi: 10.1016/0014-4827(65)90403-9
Kramer, S., Meyer-Natus, E., Stigloher, C., Thoma, H., Schnaufer, A., Engstler, M. (2020). Parallel monitoring of RNA abundance, localization and compactness with correlative single molecule FISH on LR White embedded samples. Nucleic Acids Res. 49, e14. doi: 10.1093/nar/gkaa1142
Le Roch, K. G., Johnson, J. R., Florens, L., Zhou, Y., Santrosyan, A., Grainger, M., et al. (2004). Global analysis of transcript and protein levels across the Plasmodium falciparum life cycle. Genome Res. 14, 2308–2318. doi: 10.1101/gr.2523904.7
Lu, X. M., Batugedara, G., Lee, M., Prudhomme, J., Bunnik, M., Le Roch, K. G. (2017). Nascent RNA sequencing reveals mechanisms of gene regulation in the human malaria parasite Plasmodium falciparum. Nucleic Acids Res. 45, 7825–7840. doi: 10.1093/nar/gkx464
Lucchesi, J. C., Kuroda, M. I. (2015). Dosage compensation in drosophila. Cold Spring Harb. Perspect. Biol. 7, 1–21. doi: 10.1101/cshperspect.a019398
Mair, G. R., Braks, J. A., Garver, L. S., Wiegant, J. C., Hall, N., Dirks, R. W., et al. (2006). Regulation of sexual development of Plasmodium by translational repression. Science 313, 667–669. doi: 10.1126/science.1125129
Mangold, K. A., Manson, R. U., Koay, E. S. C., Stephens, L., Regner, M. A., Thomson, R. B., et al. (2005). Real-time PCR for detection and identification of Plasmodium spp. J. Clin. Microbiol. 43, 2435–2440. doi: 10.1128/JCM.43.5.2435-2440.2005
Marshall, W. F., Young, K. D., Swaffer, M., Wood, E., Nurse, P., Kimura, A., et al. (2012). The big question of cell size. BMC Biol. 10:101. doi: 10.1186/1741-7007-10-101
Matthews, H., Duffy, C. W., Merrick, C. J. (2018). Checks and balances? DNA replication and the cell cycle in Plasmodium. Parasit. Vectors 11, 1–13. doi: 10.1186/s13071-018-2800-1
Mendjan, S., Taipale, M., Kind, J., Holz, H., Gebhardt, P., Schelder, M., et al. (2006). Nuclear pore components are involved in the transcriptional regulation of dosage compensation in Drosophila. Mol. Cell. 21, 811–823. doi: 10.1016/j.molcel.2006.02.007
Moraes, C. B., Dorval, T., Contreras-Dominguez, M., Dossin, F., de, M., Hansen, M. A. E., et al. (2013). Transcription Sites Are Developmentally Regulated during the Asexual Cycle of Plasmodium falciparum. PLoS One 8, 1–10. doi: 10.1371/journal.pone.0055539
Osborn, T. C., Chris Pires, J., Birchler, J. A., Auger, D. L., Chen, Z. J., Lee, H. S., et al. (2003). Understanding mechanisms of novel gene expression in polyploids. Trends Genet. 19, 141–147. doi: 10.1016/S0168-9525(03)00015-5
Otto, T. D., Wilinski, D., Assefa, S., Keane, T. M., Sarry, L. R., Böhme, U., et al. (2010). New insights into the blood-stage transcriptome of Plasmodium falciparum using RNA-Seq. Mol. Microbiol. 76, 12–24. doi: 10.1111/j.1365-2958.2009.07026.x
Otto, S. P. (2007). The Evolutionary Consequences of Polyploidy. Cell 131, 452–462. doi: 10.1016/j.cell.2007.10.022
Painter, H. J., Chung, N. C., Sebastian, A., Albert, I., Storey, J. D., Llinás, M. (2018). Genome-wide real-time in vivo transcriptional dynamics during Plasmodium falciparum blood-stage development. Nat. Commun. 9, 1–12. doi: 10.1038/s41467-018-04966-3
Parisod, C., Holderegger, R., Brochmann, C. (2010). Evolutionary consequences of autopolyploidy. N. Phytol. 186, 5–17. doi: 10.1111/j.1469-8137.2009.03142.x
Ponts, N., Harris, E. Y., Prudhomme, J., Wick, I., Eckhardt-Ludka, C., Hicks, G. R., et al. (2010). Nucleosome landscape and control of transcription in the human malaria parasite. Genome Res. 20, 228–238. doi: 10.1101/gr.101063.109
Poran, A., Aly, O., Mencia-trinchant, N., Chantal, T., Guzman, M. L., Hassane, D. C., et al. (2017). Single-cell RNA-seq reveals a signature of sexual commitment in malaria parasites. Nature 551, 95–99. doi: 10.1038/nature24280.Single-cell
Prudêncio, M., Rodriguez, A., Mota, M. M. (2006). The silent path to thousands of merozoites: The Plasmodium liver stage. Nat. Rev. Microbiol. 4, 849–856. doi: 10.1038/nrmicro1529
Rai, R., Zhu, L., Chen, H., Gupta, A. P., Sze, S. K., Zheng, J., et al. (2014). Genome-wide analysis in Plasmodium falciparum reveals early and late phases of RNA polymerase II occupancy during the infectious cycle. BMC Genomics 15, 959. doi: 10.1186/1471-2164-15-959
Raices, M., D’Angelo, M. A. (2017). Nuclear pore complexes and regulation of gene expression. Curr. Opin. Cell Biol. 46, 26–32. doi: 10.1016/j.ceb.2016.12.006
Reid, A. J., Talman, A. M., Bennett, H. M., Gomes, A. R., Sanders, M. J., Illingworth, C. J. R., et al. (2018). Single-cell RNA-seq reveals hidden transcriptional variation in malaria parasites. ELife 7, 1–29. doi: 10.7554/eLife.33105
Rudlaff, R. M., Kraemer, S., Marshman, J., Dvorin, J. D. (2020). Three-dimensional ultrastructure of Plasmodium falciparum throughout cytokinesis. PLoS Pathog. 16, 1–21. doi: 10.1371/journal.ppat.1008587
Schmid, M. B., Roth, J. R. (1987). Gene location affects expression level in salmonella typhimurium. J. Bacteriol. 169, 2872–2875. doi: 10.1128/jb.169.6.2872-2875.1987
Schoenfelder, K. P., Fox, D. T. (2015). The expanding implications of polyploidy. J. Cell Biol. 209, 485–491. doi: 10.1083/jcb.201502016
Sheltzer, M., Amon, A. (2011). The Aneuploidy Paradox: Costs and Benefits of an Incorrect Karyotype. Trends Genet. 27, 446–453. doi: 10.1016/j.tig.2011.07.003
Shock, J. L., Fischer, K. F., deRisi, J. L. (2007). Whole-genome analysis of mRNA decay in Plasmodium falciparum reveals a global lengthening of mRNA half-life during the intra-erythrocytic development cycle. Genome Biol. 8, 1–12. doi: 10.1186/gb-2007-8-7-r134
Shuter, B. J., Thomas, J. E., Zimmerman, A. M. (1983). Phenotypic correlates of genomic DNA content in unicellular eukaryotes and other cells. Am. Nat. 122, 26–44. doi: 10.1086/284116
Sims, J. S., Militello, K. T., Sims, P. A., Patel, V. P., Kasper, J. M., Wirth, D. F. (2009). Patterns of gene-specific and total transcriptional activity during the Plasmodium falciparum intraerythrocytic developmental cycle. Eukaryot. Cell. 8, 327–338. doi: 10.1128/EC.00340-08
Song, Q., Ando, A., Jiang, N., Ikeda, Y., Chen, Z. J. (2020). Single-cell RNA-seq analysis reveals ploidy-dependent and cell-specific transcriptome changes in Arabidopsis female gametophytes. Genome Biol. 21, 1–18. doi: 10.1186/s13059-020-02094-0
Spreng, B., Fleckenstein, H., Kübler, P., Di Biagio, C., Benz, M., Patra, P., et al. (2019). Microtubule number and length determine cellular shape and function in Plasmodium. EMBO J. 38, 1–22. doi: 10.15252/embj.2018100984
Sugimoto-Shirasu, K., Roberts, K. (2003). “Big it up”: Endoreduplication and cell-size control in plants. Curr. Opin. Plant Biol. 6, 544–553. doi: 10.1016/j.pbi.2003.09.009
Theron, M., Hesketh, R. L., Subramanian, S., Rayner, J. C. (2010). An adaptable two-color flow cytometric assay to quantitate the invasion of erythrocytes by Plasmodium falciparum parasites. Cytometry A. 77, 1067–1074. doi: 10.1002/cyto.a.20972
Toenhake, C. G., Fraschka, S. A. K., Vijayabaskar, M. S., Westhead, D. R., van Heeringen, S. J., Bártfai, R. (2018). Chromatin Accessibility-Based Characterization of the Gene Regulatory Network Underlying Plasmodium falciparum Blood-Stage Development. Cell Host Microbe 23, 557–569. doi: 10.1016/j.chom.2018.03.007
Vembar, S. S., Macpherson, C. R., Sismeiro, O., Coppée, J. Y., Scherf, A. (2015). The PfAlba1 RNA-binding protein is an important regulator of translational timing in Plasmodium falciparum blood stages. Genome Biol. 16, 1–18. doi: 10.1186/s13059-015-0771-5
Vembar, S. S., Droll, D., Scherf, A. (2016). Translational regulation in blood stages of the malaria parasite studies pave the way. WIREs RNA 7, 772–792. doi: 10.1002/wrna.1365
Visger, C. J., Wong, G. K. S., Zhang, Y., Soltis, P. S., Soltis, D. E. (2019). Divergent gene expression levels between diploid and autotetraploid Tolmiea relative to the total transcriptome, the cell, and biomass. Am. J. Bot. 106, 280–291. doi: 10.1002/ajb2.1239
Voichek, Y., Bar-Ziv, R., Barkai, N. (2016). Expression homeostasis during DNA replication. Science 351, 1087–1090. doi: 10.1126/science.aad1162
Waldecker, M., Dasanna, A. K., Lansche, C., Linke, M., Srismith, S., Cyrklaff, M., et al. (2017). Differential time-dependent volumetric and surface area changes and delayed induction of new permeation pathways in P. falciparum-infected hemoglobinopathic erythrocytes. Cell Microbiol. 19, 1–13. doi: 10.1111/cmi.12650
Walzer, K. A., Fradin, H., Emerson, L. Y., Corcoran, D. L., Chi, J. T. (2019). Latent transcriptional variations of individual Plasmodium falciparum uncovered by single-cell RNA-seq and fluorescence imaging. PLoS Genet. 15, 1–26. doi: 10.1371/journal.pgen.1008506
Weiner, A., Dahan-Pasternak, N., Shimoni, E., Shinder, V., von Huth, P., Elbaum, M., et al. (2011). 3D nuclear architecture reveals coupled cell cycle dynamics of chromatin and nuclear pores in the malaria parasite Plasmodium falciparum. Cell Microbiol. 13, 967–977. doi: 10.1111/j.1462-5822.2011.01592.x
Westermann, A. J., Gorski, S. A., Vogel, J. (2012). Dual RNA-seq of pathogen and host. Nat. Rev. Microbiol. 10, 618–630. doi: 10.1038/nrmicro2852
Wood, E., Nurse, P. (2015). Sizing up to Divide: Mitotic Cell-Size Control in Fission Yeast. Ann. Rev. Cell Dev. Biol. 31, 11–29. doi: 10.1146/annurev-cellbio-100814-125601
Wutz, A., Gribnau, J. (2007). X inactivation Xplained. Curr. Opin. Genet. Dev. 17, 387–393. doi: 10.1016/j.gde.2007.08.001
Young, J. A., Johnson, J. R., Benner, C., Yan, S. F., Chen, K., Le Roch, K. G., et al. (2008). In silico discovery of transcription regulatory elements in Plasmodium falciparum. BMC Genomics 9, 1–21. doi: 10.1186/1471-2164-9-70
Zhang, K., Rathod, P. K. (2002). Divergent Regulation of Dihydrofolate Reductase between malaria parasite and human host. Science 296. doi: 10.1126/science.1068274
Keywords: malaria, Plasmodium, polyploidy, DNA content, gene dosage, gene expression, transcription regulation
Citation: Machado M, Steinke S and Ganter M (2021) Plasmodium Reproduction, Cell Size, and Transcription: How to Cope With Increasing DNA Content? Front. Cell. Infect. Microbiol. 11:660679. doi: 10.3389/fcimb.2021.660679
Received: 29 January 2021; Accepted: 19 March 2021;
Published: 09 April 2021.
Edited by:
Catherine J. Merrick, University of Cambridge, United KingdomReviewed by:
Richard Bartfai, Radboud University Nijmegen, NetherlandsCopyright © 2021 Machado, Steinke and Ganter. This is an open-access article distributed under the terms of the Creative Commons Attribution License (CC BY). The use, distribution or reproduction in other forums is permitted, provided the original author(s) and the copyright owner(s) are credited and that the original publication in this journal is cited, in accordance with accepted academic practice. No use, distribution or reproduction is permitted which does not comply with these terms.
*Correspondence: Markus Ganter, bWFya3VzLmdhbnRlckBtZWQudW5pLWhlaWRlbGJlcmcuZGU=
Disclaimer: All claims expressed in this article are solely those of the authors and do not necessarily represent those of their affiliated organizations, or those of the publisher, the editors and the reviewers. Any product that may be evaluated in this article or claim that may be made by its manufacturer is not guaranteed or endorsed by the publisher.
Research integrity at Frontiers
Learn more about the work of our research integrity team to safeguard the quality of each article we publish.