- 1Laboratório de Bioquímica de Tripanosomatideos, Instituto Oswaldo Cruz (IOC), Fundação Oswaldo Cruz – FIOCRUZ, Rio de Janeiro, Brazil
- 2Laboratório de Esquistossomose Experimental, Instituto Osvaldo Cruz, Fundação Oswaldo Cruz – FIOCRUZ, Rio de Janeiro, Brazil
- 3i3S—Instituto de Investigação e Inovação em Saúde, Universidade do Porto, Porto, Portugal
- 4ICBAS—Instituto de Ciências Biomédicas Abel Salazar, Universidade do Porto, Porto, Portugal
Leishmania infantum is a protozoan parasite that causes a vector borne infectious disease in humans known as visceral leishmaniasis (VL). This pathology, also caused by L. donovani, presently impacts the health of 500,000 people worldwide, and is treated with outdated anti-parasitic drugs that suffer from poor treatment regimens, severe side effects, high cost and/or emergence of resistant parasites. In previous works we have disclosed the anti-Leishmania activity of (-)-Epigallocatechin 3-O-gallate (EGCG), a flavonoid compound present in green tea leaves. To date, the mechanism of action of EGCG against Leishmania remains unknown. This work aims to shed new light into the leishmanicidal mode of action of EGCG. Towards this goal, we first confirmed that EGCG inhibits L. infantum promastigote proliferation in a concentration-dependent manner. Second, we established that the leishmanicidal effect of EGCG was associated with i) mitochondria depolarization and ii) decreased concentration of intracellular ATP, and iii) increased concentration of intracellular H2O2. Third, we found that the leishmanicidal effect and the elevated H2O2 levels induced by of EGCG can be abolished by PEG-catalase, strongly suggesting that this flavonoid kills L. infantum promastigotes by disturbing their intracellular redox balance. Finally, we gathered in silico and in vitro evidence that EGCG binds to trypanothione reductase (TR), a central enzyme of the redox homeostasis of Leishmania, acting as a competitive inhibitor of its trypanothione substrate.
Introduction
Visceral leishmaniasis (VL) is a neglected tropical disease caused by Leishmania infantum and L. donovani that affects 500,000 people and is fatal in over 95% of cases if left untreated. It is estimated that 50,000 to 90,000 new VL cases occur worldwide each year (World Health Organization, 2018). The disease has a high prevalence in the Americas, particularly in Brazil where 96% of the cases are reported (Pan American Health Organization, 2018). In the absence of a vaccine, VL treatment is largely based on chemotherapy, pentavalent antimonials and amphotericin B being the most used drugs. However, even though these treatments have saved thousands of lives, they can only be administered parenterally, present severe side effects and are expensive (Barral et al., 1991; Grimaldi and Tesh, 1993; Croft and Coombs, 2003; Amato et al., 2008). The introduction of miltefosine in 2014 as another first-line option for VL therapy and the first orally delivered drug, aimed at attending some of those drawbacks (McGwire and Satoskar, 2014). Nevertheless, an increasing number of clinical relapses has meanwhile been reported (Rijal et al., 2013) rendering the development of new, more effective, safer, and easily accessible drugs extremely urgent (Chappuis et al., 2007; Capela et al., 2019).
Flavonoids are natural products that have demonstrated significant antiprotozoal activities (Tasdemir et al., 2006; Gervazoni et al., 2020). Within this chemotype, (-)-Epigallocatechin 3-O-gallate (EGCG), the most abundant flavonoid constituent of green tea, was recently demonstrated to display activity against different species of Leishmania, including visceral leishmaniasis species (L. infantum (Inacio et al., 2019)).
Indeed, we found EGCG to be active in vitro, against intracellular amastigotes with a selectivity index over 100, and in vivo, in a murine model of infection also without evident toxicity towards treated animals (Inacio et al., 2019). These data and the fact that EGCG can be orally administered encourage further studies towards the potential of this compound for VL therapy. In this regard, one important aspect refers to the characterization of the underlying mechanism of activity. Based on the observation that in mice EGCG can modulate two important antioxidant proteins, hepatic glutathione peroxidase and glutathione reductase (Dong et al., 2016), we considered here the possibility that EGCG activity in Leishmania interferes with the parasite antioxidant mechanisms
In Leishmania spp. and related trypanosomatid organisms, redox balance is largely accomplished by the enzyme trypanothione reductase (Leroux and Krauth-Siegel, 2016). This molecule functions as a FAD-dependent disulfide oxidoreductase that catalyzes the reduction of trypanothione [N1,N8-bis-glutathionylspermidine or T(SH)2] at expenses of NADPH. Trypanothione is a dithiol, composed of two molecules of glutathione linked by one spermidine bridge. It is the major thiol in trypanosomatids, where it acts as electron donor for a multitude of biological reactions (including the elimination of hydroperoxides), and thus assumes the functions of glutathione in other organisms. The recycling of trypanothione back to its reduced, active form is carried out by the enzyme trypanothione reductase (TR). This process is absolutely critical for parasite redox balance and overall cell viability, as demonstrated by the essential character of TR (Dumas et al., 1997; Tovar et al., 1998). The nearest mammalian homologue of TR is the enzyme glutathione reductase (GR). Despite their overall similarity, TR and GR differ significantly in their thiol-binding sites, making it feasible to target TR with chemical entities that do not compromise GR activity (Castro et al., 2018). Overall, the essential role played by TR in Leishmania spp. and its absence from the human host render this molecule an attractive target for the development of new potential drugs.
The present study investigates the mechanism of action of EGCG in promastigotes of L. infantum. It confirms that EGCG inhibits promastigote proliferation in a concentration-dependent manner and describes that this phenomenon is associated with mitochondrial depolarization, decreased intracellular ATP concentration, and increased levels of H2O2. Importantly, it establishes that the leishmanicidal effect of EGCG is completely abolished by PEG-catalase, suggesting that this flavonoid induces an oxidative stress status in promastigotes that culminates in their death. Aligned with this hypothesis, this report presents evidence that EGCG interacts with TR in silico and that it acts as a competitive inhibitor for trypanothione binding to TR in in vitro enzymatic assays. Overall, this report contributes to clarify the mode of leishmanicidal action of EGCG.
Materials and Methods
Reagents
(−)-Epigallocatechin 3-O-gallate (molecular formula: C22H18O11; molecular weight: 458.37 g/mol; purity ≥95%), trypanothione, JC-1, horseradish peroxidase (HRP), digitonin, NADP+, NADPH, DTNB, FCCP, HEPES, Schneider’s Drosophila medium, fetal calf serum and RPMI 1640 medium were obtained from Sigma Aldrich (St Louis, MO, USA). Amplex Red, and alamarBlue were obtained from Invitrogen Molecular Probes (Leiden, The Netherlands). Other reagents were purchased from Merck (Sao Paulo, Brazil). Deionized distilled water was obtained using a Milli-Q system of resins (Millipore Corp., Bedford, MA, USA) and was used to prepare all solutions.
Parasites
The MHOM/MA/67/ITMAP263 strain of Leishmania infantum was used throughout this study. Leishmania infantum amastigotes were isolated from BALB/c mice and maintained as promastigotes at 26°C in Schneider’s medium supplemented with 20% fetal bovine serum (v/v), 100 U/ml penicillin and 100 μg/ml streptomycin. Parasite maintenance was achieved by passaging every 3 days. Female BALB/c mice (8−10 weeks) were provided by the Instituto Ciências e Tecnologia em Biomodelos (ICTB/FIOCRUZ). All animals were bred and maintained at the Instituto Oswaldo Cruz according to Guide for the Care and Use of Laboratory Animals of the Brazilian National Council for Control of Animal Experimentation (CONCEA). The protocol was approved by the Committee on the Ethics of Animal Experiments of the Instituto Oswaldo Cruz (CEUA-IOC, License Number: L-11/2017).
Promastigote Proliferation Assay
L. infantum (106/mL) promastigotes were incubated with different concentrations of EGCG (0.015–1 mM) or vehicle (PBS) for 72 h. The cell density was estimated with the addition of alamarBlue (10% v/v) for 3 h at 26°C. The absorbance was measured at 570 nm using a spectrophotometer. The growth curve was initiated with 1 x 106 cells/ml. The 50% inhibitory concentration (IC50) was determined by logarithmic regression analysis using GraphPad Prism 6 (GraphPad Software, La Jolla, CA, USA). The experiments were run three times in triplicate.
Hydrogen Peroxide Measurement
Hydrogen peroxide production was measured using Amplex Red and horseradish peroxidase (HRP) (Fonseca-Silva et al., 2015). Promastigotes were treated for 72 h in the absence or presence of EGCG (125, 250 and 500 µM). Cells were harvested and resuspended in Hanks balanced salt solution (HBSS). The cell number was obtained by counting using a Neubauer chamber. Promastigotes (2x107 cells/mL) were incubated with HBSS containing 10 μM Amplex Red reagent, 64 μM digitonin (to permeabilize parasites) and 10 U/ml HRP for 30 min at 26°C. Fluorescence was monitored at excitation and emission wavelengths of 560 and 590 nm, respectively, in a spectrofluorometer. H2O2 concentrations were calculated from an H2O2 standard curve.
Mitochondrial Membrane Potential (ΔΨm) Assay
The cationic probe JC-1 was used to determine the mitochondrial membrane potential (ΔΨm) as described previously (Fonseca-Silva et al., 2011). Promastigotes (106 cells/ml) were cultured for 72 h in the absence or presence of EGCG (125 – 500 µM). Cells were harvested and resuspended in HBSS. The cell number was obtained by counting in a Neubauer chamber. Promastigotes (107 cells/ml) were incubated with JC-1 (10 μg/ml) for 10 minutes at 37°C. After washing twice with HBSS, fluorescence was measured using a spectrofluorometer at 530 nm and 590 nm using an excitation wavelength of 480 nm. The ratio of the values obtained at 590 nm and 530 nm was plotted as the relative ΔΨm. The mitochondrial uncoupling agent carbonyl cyanide p-trifluoromethoxyphenylhydrazone (FCCP; 20 μM) was used as control.
Determination of Intracellular ATP Concentration
Intracellular ATP concentrations in L. infantum promastigotes were measured using a CellTiter-Glo luminescent assay (Promega), where the signal was proportional to ATP concentration. Briefly, promastigotes were treated for 72 h in the absence or presence of EGCG (125 – 500 μM). Cultures were washed three times, and parasite concentration was adjusted to 107 cells in 200 μl of PBS. A 50-µl aliquot of each sample was transferred to a 96-well plate and mixed with the same volume of CellTiter-Glo. Plates were incubated in the dark for 10 min, and bioluminescence was measured using a GloMax-Multi Microplate Multimode Reader (Promega). ATP concentrations were calculated from the ATP standard curve.
Docking Studies
The crystal structures of oxidized and reduced TR (PDB 2JK6 and 4ADW, respectively) were downloaded from the RCSB Protein DataBank (www.rcsb.org) (Berman et al., 2000). The atomic partial charges were calculated in the PDB2PQR Server (www.nbcr-222.ucsd.edu) using the AMBER forcefield, and the protonation state was determined using PROKA at pH 7.4. All proteins were converted to PDBQT format using Autodock Tools. A cubic grid map with 80 Å of side and spacing of 0.375 Å was built centralized on active site residues (x, y, z coordinates: 23.3, 51.9, -15.6) of TR on both states (oxidized and reduced) using Autodock Tools. (Baiocco et al., 2009a; Baiocco et al., 2009b; Venkatesan et al., 2010; Colotti et al., 2013).
The docking of EGCG to L. infantum TR was performed with AutoDock (Morris et al., 2009). The grid box parameters for both states of TR were center x/y/z, 23.3/51.9/-15.6, dimension x/y/z, 80/80/80 and spacing, 0.375 Å (Ortalli et al., 2018). Grid boxes were set around the active site residues involved in the biological functions of the protein used in this study (Baiocco et al., 2009a; Baiocco et al., 2009b; Venkatesan et al., 2010; Colotti et al., 2013).
The docking procedure was performed using 500 runs of Lamarckian Genetic Algorithm (LGA). Ligand binding position and interaction analysis were performed using PyMOL software and the 2D interaction was visualized with BIOVIA Discovery Studio 2020 (Dassault Systems BIOVIA, Discovery Studio Modeling Environment, Release 2017, San Diego: Dassault Systems, 2016).
Heterologous Expression and Purification of Recombinant LiTR
Full-length LiTR (tritryp ID: LINF_050008500) was PCR-amplified from the genomic DNA of L. infantum and cloned into the NdeI/XhoI sites of the pET23a(+) plasmid. The resulting construct was introduced in Escherichia coli BL21(DE3) for expression of a modified version of LiTR containing a carboxy-terminal six-histidine tag (LiTR.6His), as follows. Bacteria were grown at 37 °C, in Luria Bertani (LB) medium supplemented with 50 μg/ml ampicillin and 0.1% (w/v) glucose, until an OD600nm of 0.6. LiTR.6His expression was induced by addition of 2 mM isopropyl-β-D-thiogalactopyranoside (IPTG) and further incubation at 37 °C, for 4 h. Bacteria were pelleted and suspended in 20 mM Tris-HCl pH7.6, 500 mM NaCl, 10 mM imidazole, at a ratio of 1 g wet weight per 5 ml buffer, followed by disruption by sonication and centrifugation at 30,000 ×g, for 20 min, at 4 °C. LiTR.6His was obtained as a soluble protein in the supernatant of lysed bacteria and purified by passage through a 1 ml HisTrap column (GEHealthcare), followed by elution in a 25-500 mM imidazole gradient, at 4 °C and a flow rate of 0.5 ml/min, using an ÄKTAprime plus equipment (GEHealthcare). Fractions confirmed by SDS-PAGE to contain LiTR.6His were pooled, passed through a PD-10 desalting column packed with Sephadex G-25 resin (GEHealthcare), and recovered in 50 mM HEPES pH 7.6, 1 mM EDTA. LiTR.6His quality and purity was confirmed by SDS-PAGE, and its concentration estimated using the bicinchoninic acid assay (Pierce) with bovine serum albumin as standard.
Trypanothione Reductase Activity Assay
This assay, in which generation of reduced trypanothione [T(SH)2] by TR is coupled with the chemical oxidant 5,5’-dithiobis-(2-nitrobenzoic acid) (DTNB; or Ellman’s reagent) (Hamilton et al., 2003; Richardson et al., 2009), was performed in 96-well plates. Reaction mixtures contained 50 mM HEPES pH 8.0, 1 mM EDTA, 30 µM NADP+, 1 µM oxidized trypanothione (TS2), 0.1 mM DTNB, 0.15 mM NADPH and increasing concentrations of EGCG (7.8 – 500 µM). Reactions were started by addition of 7 nM recombinant LiTR.6His (i.e. C-terminally histidine-tagged L. infantum TR), purified from Escherichia coli by nickel chromatography and quantified by the bicinchoninic acid (BCA) protein assay (Pierce), using bovine serum albumin (BSA) as standard. Upon addition of LiTR.6His, the rate of TNB formation was monitored at 410 nm at 25°C for 5 minutes using a UV-2401PC UV–vis recording spectrophotometer (Shimadzu Scientific).
Determination of Kinetic Parameters
Kinetics assays were performed in 96-well plates, following the DTNB-coupled reduction of trypanothione, as described above. Reactions were carried out under a constant concentration of LiTR.6His (0.7 nM) and different concentrations of EGCG (100 and 200 µM) and of trypanothione (2, 4, 6, 8, 12, 21, 30 and 60 µM). First, a reaction mixture containing trypanothione, EGCG and 15 mM NADPH was prepared. Next, the reaction was started with addition of a mixture containing 50 mM HEPES pH 8.0, 1 mM EDTA, 8.5 µM NADP+, 25 µM DTNB and 0.7 nM TR. The rate of TNB formation was monitored at 410 nm at 25°C for 5 minutes using a UV-2401PC UV–vis recording spectrophotometer (Shimadzu Scientific). The kinetic parameters of Km and Vmax were calculated using a computerized nonlinear regression fit of the data obtained to the Michaelis–Menten equation. The resulting data were also analyzed under Lineweaver–Burk plots.
Statistical Analysis
All experiments were performed in three independent trials performed in triplicate. Data were analyzed using the Mann-Whitney test or one-way analysis of variance (ANOVA) followed by Tukey’s posttest in GraphPad Prism 6 (GraphPad Software). Differences were considered statistically significant at p ≤ 0.05. Data are expressed as means ± standard error.
Results
EGCG Impairs Survival of L. infantum Promastigotes
Prior to this work, EGCG has been acknowledged for its cytotoxicity against Leishmania promastigotes of different species (Inacio et al., 2012; Inacio et al., 2014). Here, we expanded on those findings, towards the calculation of the IC50 of EGCG against promastigotes of L. infantum. To accomplish this, we exposed parasites to different concentrations of the flavonoid (0.015 − 1 mM) for 72 h, after which time we assessed cellular viability by the alamar Blue assay. The results of this analysis, plotted in Figure 1, show that EGCG inhibits promastigote survival in a concentration-dependent manner, reaching 97.5% inhibition at the highest concentration tested (1 mM) (Figure 1). Logarithmic regression (R-square = 0.9806) of this concentration-dependent curve revealed an IC50 of 177.9 µM.
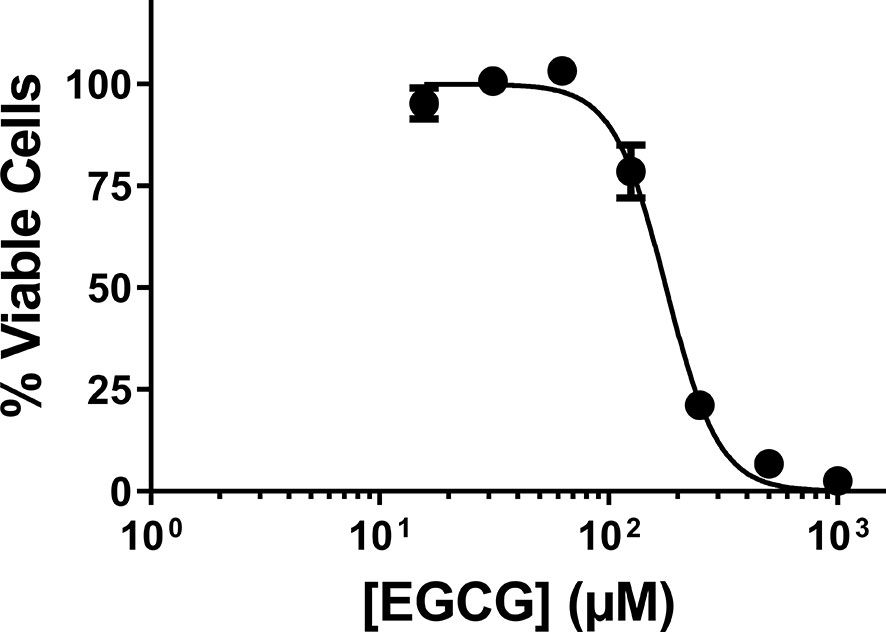
Figure 1 Effect of EGCG on Leishmania infantum. Promastigotes of L. infantum were cultivated in Schneider’s Drosophila medium at 26°C for 72 h in the absence or presence of EGCG (0.015 – 1 mM). Cell viability was measured using the alamar Blue assay. In the control (absence of EGCG), the same volume of PBS (solvent of EGCG) was added to the growth medium of controls. Values represent mean ± standard error of three different experiments.
EGCG Leads to Mitochondria Depolarization and Impairs ATP Production
EGCG induces mitochondrial membrane potential (ΔΨm) depolarization in Leishmania braziliensis and L. amazonensis (Inacio et al., 2012; Inacio et al., 2014). To characterize the deadly phenotype imposed by EGCG on L. infantum promastigotes, we set out to evaluate the mitochondrial membrane potential (ΔΨm) of parasites treated with this flavonoid compound (125, 250 and 500 µM) for 72 h. Resorting to the fluorometric JC-1 probe assay, we observed that EGCG induces a concentration-dependent decrease in the ΔΨm, achieving a reduction of 85.6% upon treatment with 500 µM EGCG (Figure 2A). These results indicate that EGCG treatment impacts on promastigote mitochondrial function.
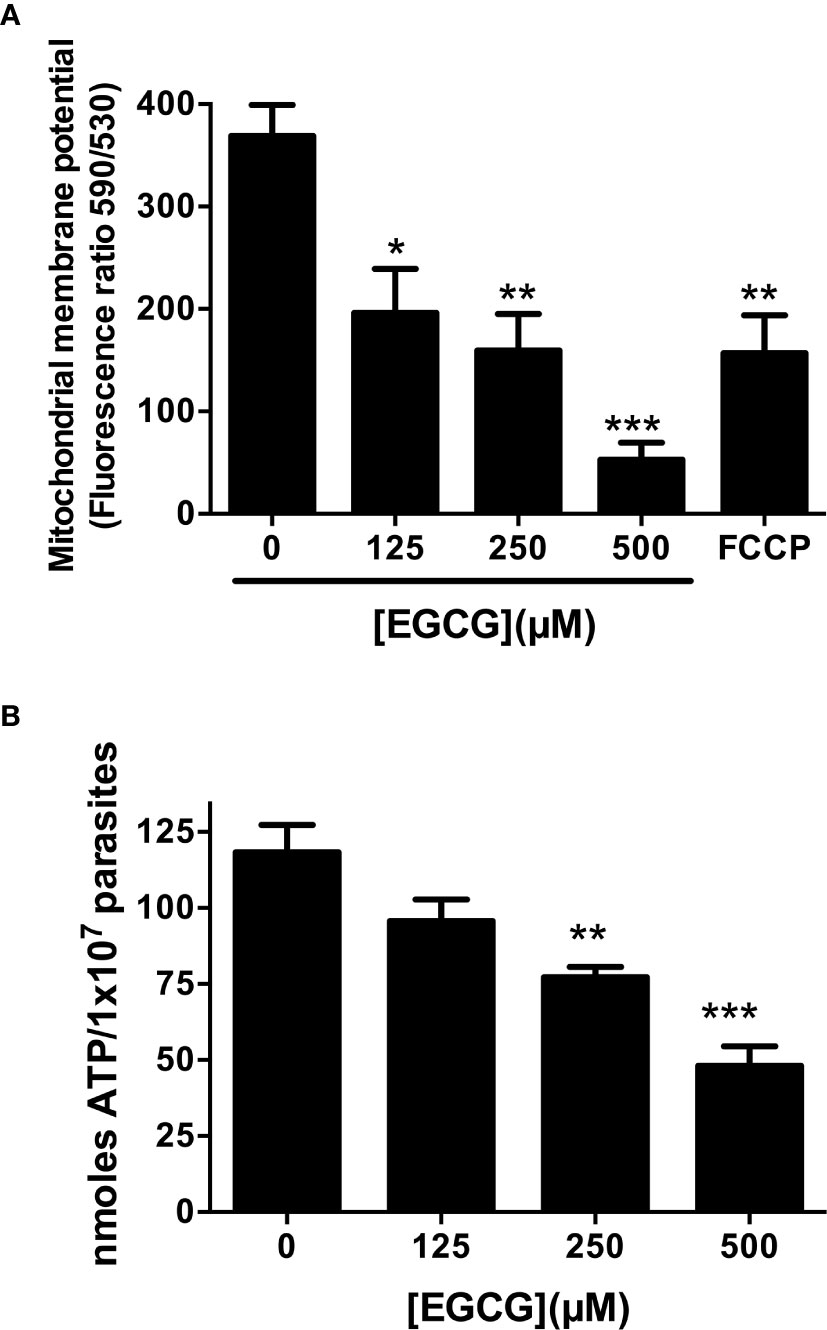
Figure 2 EGCG induces mitochondrial depolarization in L. infantum with concomitant loss of intracellular ATP. Leishmania infantum promastigotes were cultivated in Schneider’s Drosophila medium at 26°C for 72 h in the absence or presence of 125 – 500 µM EGCG. Promastigotes were stained with the potentiometric probe JC-1 (10 µg/ml). The positive control was treated with FCCP (20 µM) for 20 minutes. In the control (absence of EGCG), the same volume of vehicle (PBS) was added to the growth medium. Concentration-dependent alterations in relative mitochondrial membrane potential (ΔΨm) values are expressed as the ratio of the fluorescence measurements at 590 nm (for J-aggregate) versus 530 nm (for J-monomer) (A). Promastigotes were incubated with EGCG (125 - 500 µM) for 72 h. Intracellular ATP concentrations were measured using a bioluminescence assay. The results are expressed as ATP concentration (B). Data represent means ± standard errors of three different experiments run in triplicate. * indicates a significant difference relative to the control group (p < 0.05); ** indicates a significant difference relative to the control group (p < 0.01); *** indicates a significant difference relative to the control group (p < 0.001).
Depolarization of mitochondrial membrane usually leads to a decrease in ATP synthesis, resulting in parasite death (Inacio et al., 2014; Kaplum et al., 2016; Teixeira de Macedo Silva et al., 2018). Accordingly, we evaluated intracellular ATP concentrations in EGCG-treated L. infantum promastigotes. As shown in Figure 2B, EGCG reduces intracellular ATP levels in a concentration-dependent manner, reaching a reduction of 59.3% in promastigotes treated with 500 µM EGCG for 72 h.
A negative correlation was observed between the percent inhibition of L. infantum promastigotes and both ΔΨm depolarization (Pearson correlation r = -0.9025) and reduction of intracellular ATP levels (Pearson correlation r = -0.9506), suggesting that these two phenomena are directly associated to EGCG-induced parasite death.
EGCG Exerts Its Leishmanicidal Action by Means of a H2O2 Boost
EGCG is known to increase hydrogen peroxide (H2O2) concentration in different cell types (Inacio et al., 2014; Kim et al., 2014). To investigate whether synthesis of this oxidant also occurred in promastigotes, we monitored its levels of this oxidant in EGCG-treated parasites (125 – 500 µM) during 72 h resorting to Amplex Red. The outcome of this analysis, depicted in Figure 3, revealed that the amount of the hydroperoxide increases with increasing doses of EGCG. Illustrating this, the concentration of H2O2 increased 5.1-fold in promastigotes treated with 500 µM EGCG, relative to non-treated controls (Figure 3A). In short, these data show that exposure to EGCG leads to H2O2 accumulation in L. infantum promastigotes.
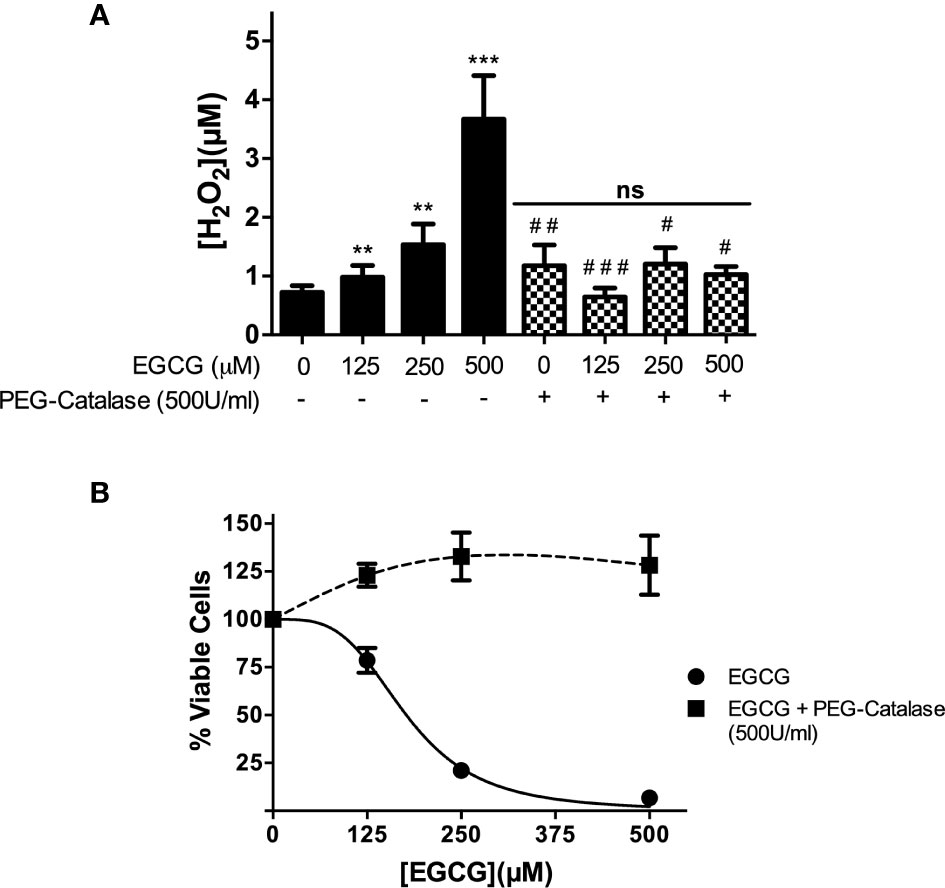
Figure 3 EGCG induces a boost of H2O2 in L. infantum promastigotes that leads to parasite death. Promastigotes of L. infantum were cultivated in Schneider’s Drosophila medium at 26°C for 72 h with EGCG (125–500 µM) in the absence or in the presence of PEG-catalase (500 U/ml). H2O2 was measured with Amplex Red and expressed as the H2O2 concentration (A). Cellular viability was measured using the alamar Blue assay (B). Values represent mean ± standard error of three different experiments. ** indicates a significant difference relative to the control group (p < 0.01); *** indicates a significant difference relative to the control group (p < 0.001); # indicates a significant difference relative to the L. infantum incubated with 500 µM of EGCG (p < 0.05); ## indicates a significant difference relative to the L. infantum incubated with 500 µM of EGCG (p < 0.01); ### indicates a significant difference relative to the L. infantum incubated with 500 µM of EGCG (p < 0.001).
Next, we moved on to investigate whether the leishmanicidal activity of EGCG is mediated by the H2O2 boost. Towards this end, we co-incubated EGCG-treated L. infantum promastigotes with polyethylene glycol (PEG)-catalase (500 U/ml), an enzymatic formulation that catalytically decomposes H2O2. First, we confirmed that PEG-catalase decreases H2O2 levels in promastigotes treated with EGCG (Figure 3A). Second, we observed that PEG-catalase completely abolishes the cytotoxic effect of EGCG towards L. infantum promastigotes (Figure 3B). In short, these results strongly suggest that EGCG mediates death of L. infantum promastigotes by promoting accumulation of H2O2 to levels that are not tolerated by parasites.
In Silico Docking Analysis Sustains EGCG Interaction With Trypanothione Reductase
Our observation that EGCG triggers a H2O2 hike in L. infantum, prompted us to investigate whether this phenomenon occurs via inactivation of the enzymatic machinery responsible for hydroperoxide elimination, which in Leishmania spp. is centralized in trypanothione reductase (TR). We started by performing in silico docking studies between EGCG and TR, looking for theoretical thermodynamic and Ki values characterizing the interaction between these molecules. Docking analysis was conducted using two crystalized forms of TR (TRox oxidized; PDB: 2JK6 and TRred reduced; PDB: 4ADW) which differ in what concerns the oxidation state of trypanothione (Baiocco et al., 2009a; Baiocco et al., 2009b; Colotti et al., 2013). Molecular docking analysis of EGCG with either TRox or TRred yielded a total of 500 poses of potential interactions, which we grouped in clusters according to conformation similarity [root-mean-square deviation (RMSD) < 2 Å]: i) the cluster with lowest energy, and ii) the cluster containing the most prevalent conformation.
Looking at the lowest energy cluster, we could predict binding energy (ΔG) values of -7.39 or -7.09 kcal/mol between EGCG and TRox or TRred, respectively, which translated into theoretical Ki values of 3.85 and 6.40 µM (Table 1). Interestingly, these values changed for EGCG/TRred interaction in the most prevalent cluster – ΔG increased to -6.4 kcal/mol, accompanying the raise in Ki to 20.5 µM (Table 1).
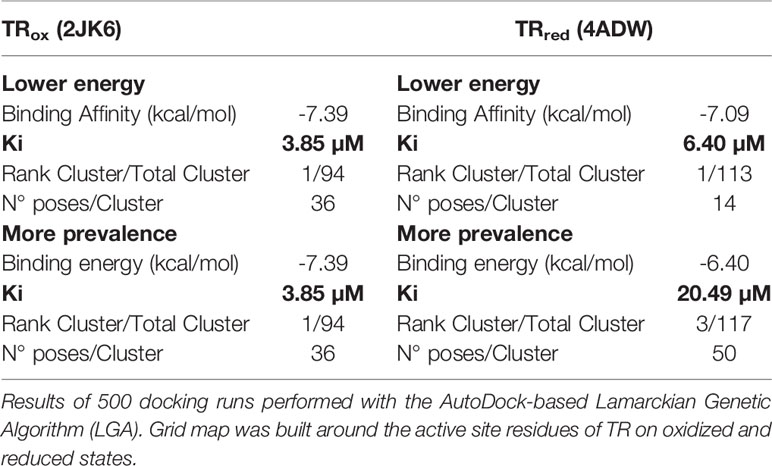
Table 1 Docking calculations of interactions of EGCG with oxidized and reduced states of trypanothione reductase (TR).
From this analysis, we obtained theoretical support to our premise that EGCG interacts with TR. To gain insight into the binding site of EGCG within the TR quaternary structure, we moved on to carry out a detailed structural analysis of EGCG/TR in silico models.
In Silico Structural Analysis Supports EGCG Interaction With the Trypanothione Binding Site of TR
Trypanothione reductase is a homodimeric enzyme with two symmetric active sites, wherein reducing equivalents are conveyed from NADPH to oxidized trypanothione (Baiocco et al., 2009a; Baiocco et al., 2009b; Colotti et al., 2013). Apart from NADPH- and trypanothione-binding pockets, each TR monomer is also comprised of a FAD-binding site and one interface domain. In our in silico structural analysis of EGCG/TR interaction, we wondered which of these TR sites are preferentially targeted by EGCG. To assess this, we looked into high confidence molecular models of EGCG interaction with both TRox and TRred, taking into account both the lowest energy and the most prevalent clusters (Figure 4). In the case of EGCG/TRox, both clusters yielded similar results, therefore similar interactions (Figure 4A).
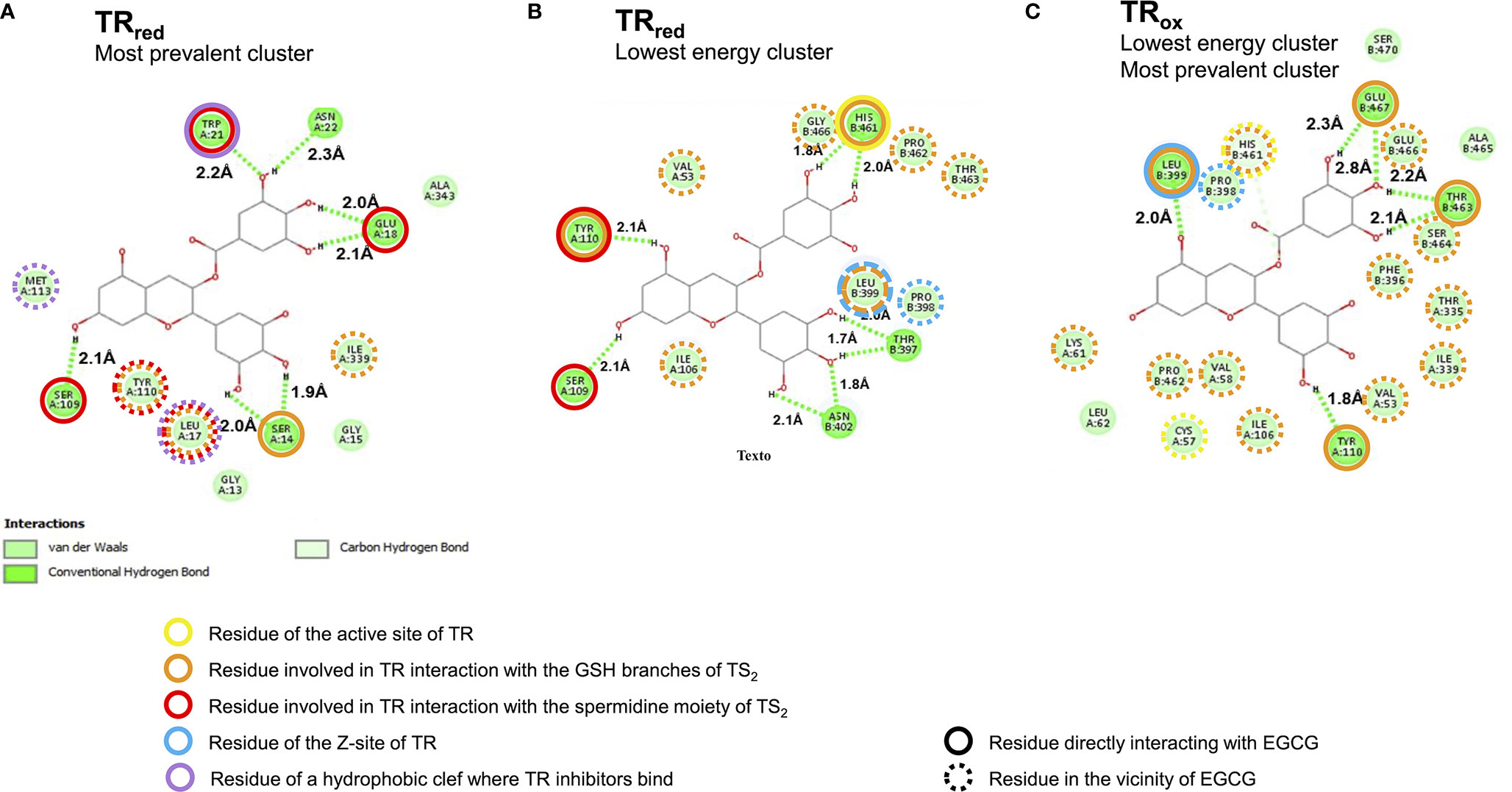
Figure 4 High confidence in silico models predict EGCG binding to the trypanothione binding site of trypanothione reductase (TR). Two dimensional representation of the interaction between EGCG (central carbon structure) and TR residues (green circles with aminoacid numbering; the letters A and B refer to different monomers) in (A) the most prevalent and also lowest energy EGCG/TRox cluster, (B) the lowest energy EGCG/TRred cluster, and (C) the most prevalent EGCG/TRred cluster.
The results of this in silico analysis, depicted in Figure 4, predicted that EGCG accommodates to the trypanothione binding site of TR, regardless the redox state of the enzyme (Figure 4 and Figure S1). Indeed, EGCG can potentially establish hydrogen bonds (Figure 4, dashed green lines) with residues that directly bind the glutathione (GSH) branches of trypanothione (Figure 4, orange circles), and, particularly in the reduced state of TR, also with residues implicated in TR interaction with the spermidine moiety of trypanothione (Figures 4B, C, red circles). The preference of EGCG for spermidine-interacting residues is even more striking in the most prevalent EGCG/TRred cluster (Figure 4C). By interacting with trypanothione-binding residues, EGCG ends up occupying the trypanothione binding pocket of TR, surrounded by residues that build up this site (Figure 4, dashed orange and red circles).
Other EGCG/TR interactions brought into light by this in silico analysis include: i) EGCG interaction with residues of the Z-site (Figures 4B, C, blue circles), an hydrophobic cavity with interest for drug development owing to its accessibility by hydrophobic compounds with TR-inhibitory activity; and ii) EGCG docking near a hydrophobic wall formed by residues Leu17, Trp21 and Met113 (Figure 4C, purple circles), which together form a target region for competitive inhibition by TR inhibitors (Meiering et al., 2005; Baiocco et al., 2013; Colotti et al., 2013; Battista et al., 2020).
From this structural modelling assessment, we conclude that EGCG has the potential to accommodate within the trypanothione binding site of TR and therefore act as a competitor for this substrate.
In Vitro Analyses Confirm That EGCG Is a Competitive Inhibitor of Trypanothione Reductase
To provide experimental demonstration to the in silico prediction that EGCG can interact with and inhibit TR by competing with trypanothione, we set out to perform in vitro enzymatic assays of TR activity in the presence of this flavonoid. As target enzyme we used recombinant LiTR.6His (i.e. L. infantum TR with a C-terminal histidine tag), purified from E. coli. Our assay consisted in monitoring TR activity based on the DTNB-based colorimetric test originally described by Hamilton et al. (Hamilton et al., 2003). Briefly, this assay combines the TR-catalyzed reduction of TS2 to T(SH)2, and re-oxidation of the T(SH)2 product back to TS2 coupled to reduction of DTNB, forming a yellow thionitrobenzoate (TNB) ion. This method maintains a constant substrate concentration, saving on reagents, and enables the linearity of the assay for at least 60 min.
We started by testing the impact that increasing concentrations of EGCG have on TR activity. The results, plotted in Figure 5, evidence that, TR activity is inhibited by the flavonoid in a concentration-dependent manner. Almost full inhibition (95.8%) is achieved at the highest concentration of EGCG tested (500 µM). Under these assay conditions, the estimated IC50 of EGCG against LiTR.6His is 193.8 µM.
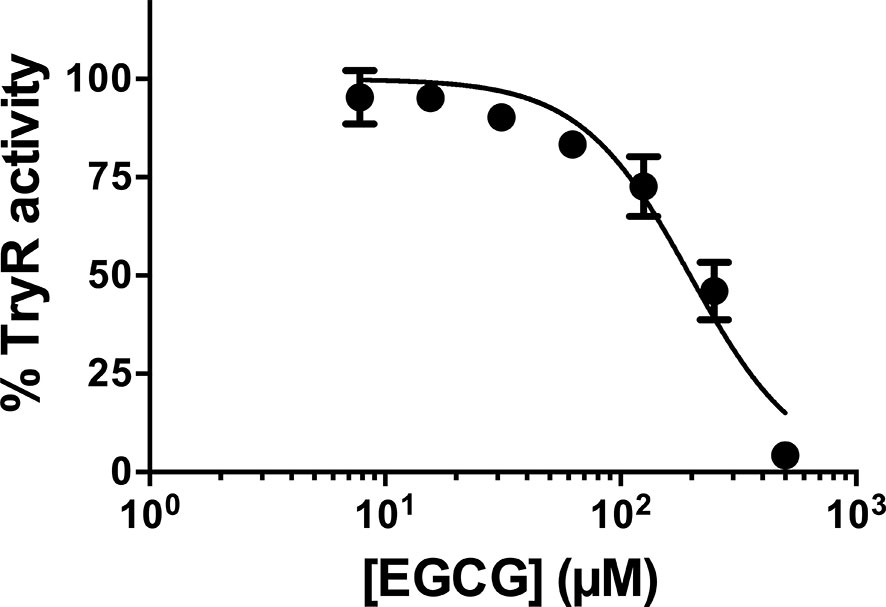
Figure 5 L. infantum recombinant TR activity was inhibited by EGCG. L. infantum recombinant TR activity was assayed at 25°C and pH 7.5 in the presence of increasing concentrations of EGCG (7.8 – 500 µM), and the assays were carried out in triplicate. Control experiments were carried out in the absence of the inhibitor. Values represent mean ± standard error of three different experiments run in triplicate.
Next, we moved on to characterize the type of inhibition that EGCG exerts over TR. This was done by performing a set of reactions with a fixed amount of LiTR.6His and varying concentrations of EGCG (0, 100 and 200 µM) and trypanothione (2 - 60 µM). The velocities estimated for these set of reactions were analyzed in a Lineweaver-Burk plot (1/V vs. 1/[T(SH)2]) across the varying concentrations of the inhibitor (Figure 6). From this analysis, we obtained several pieces of insightful data. First, results obtained in the absence of ECGC (Figure 6, black circles), allowed us to calculate the KM and Vmax values for the trypanothione substrate (36.4 ± 10 µM and 0.11 ± 0.02 nmoles 2TNB.mg-1.min-1, specifically) (Table 2). Second, when the same kinetic parameters were calculated in the presence of 100 and 200 µM EGCG, they revealed invariable Vmax values and increasing KM (60.8 ± 15.9 µM and 66.4 ± 18.5 µM, respectively) (Table 2), a behavior that conforms with the hypothesis that EGCG acts as a competitive inhibitor of trypanothione. The Ki value calculated from the Dixon plot analysis is 293.9 ± 66.3 μM (Fig. S2).
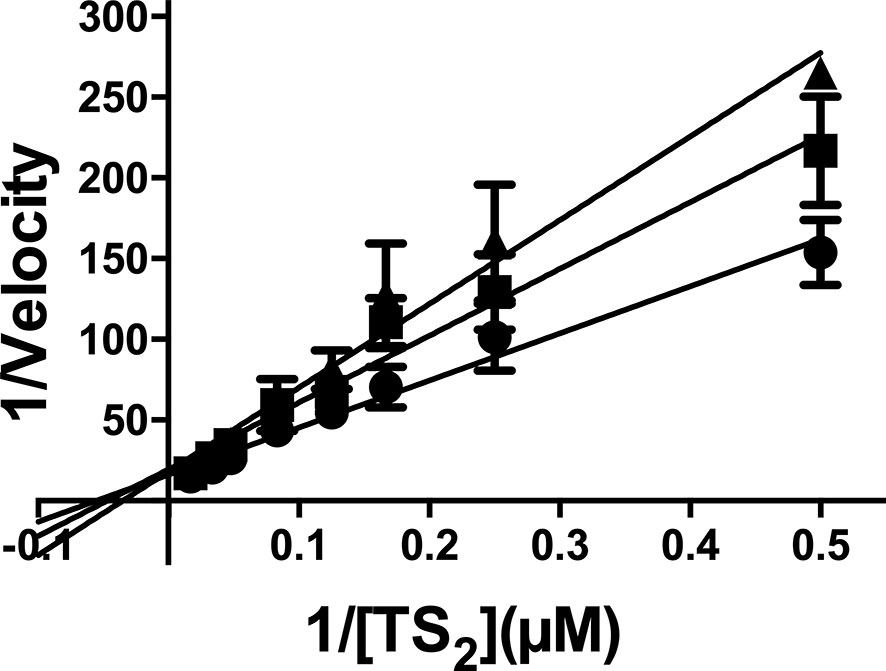
Figure 6 Kinetic analysis of TR activity in the presence of EGCG. The enzyme concentration was maintained as a constant, while the substrate [T(S)2] varied as described in the abscissa, and EGCG varied as follows: no addition (closed circles); 100 µM EGCG (closed squares); 200 µM EGCG (closed triangles). The values are presented as the mean ± standard error of three different experiments run in triplicate.
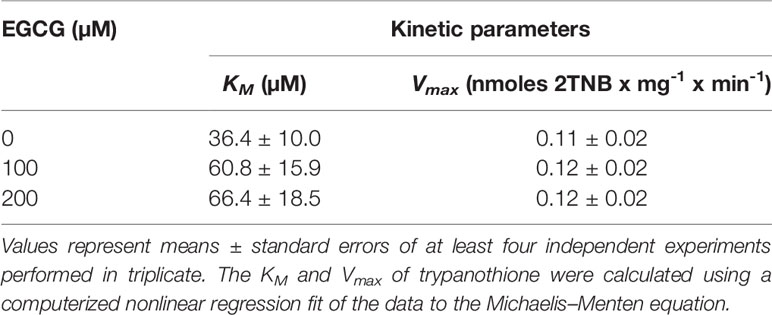
Table 2 Kinetic parameters of L. infantum recombinant trypanothione reductase (TR) in the presence of EGCG.
Discussion
Visceral leishmaniasis is a life-threatening form of infection by protozoan L. infantum and L. donovani parasites, against which new therapeutic formulas are in urgent demand to overcome the limitations of the currently-in-use, leishmanicidal drugs. Natural products, with their enormous chemical diversity, are a major resource for the discovery and development of novel therapeutics (Ioset, 2008; Ndjonka et al., 2013). For parasitic diseases, most of the drugs in use are derived from natural products, often carrying specific modifications to maximize effectiveness (Gervazoni et al., 2020). (−)-Epigallocatechin 3- O-gallate (EGCG), the most abundant flavanol constituent of green tea (Camellia sinensis (L.) Kuntze; Theaceae), is widely studied and has generated considerable interest as a biologically active compound with a wide range of potential therapeutic activities (Khan and Mukhtar, 2018). In a previous study from our group, EGCG was found to exhibit an IC50 of 2.6 µM against intracellular, mammalian-stage L. infantum amastigotes, and the ability to reduce L. infantum loads in the livers of infected mice in a dose-dependent manner, without compromising the overall health condition of the murine host [consistent with its high Selectivity Index (167.8)] (Inacio et al., 2019). The present report expands on these findings and tries to dig into the mechanistic mode of action of EGCG.
In our study, we started by confirming that, as reported for other Leishmania species (Inacio et al., 2012; Inacio et al., 2014), EGCG is active against extracellular, insect stage promastigotes of L. infantum, with an IC50 of 177.9 µM. Nevertheless, this value is much higher than that observed against intramacrophagic amastigotes. Such stage-specific effect of EGCG is not uncommon (Vermeersch et al., 2009; De Muylder et al., 2011; Gervazoni et al., 2018). Promastigotes and amastigotes differ in terms of protein expression (Holzer et al., 2006) and metabolism (Saunders et al., 2014; Jara et al., 2017) and this might translate into disparate drug susceptibilities, as observed for EGCG. Furthermore, the host cell can greatly impact drug effectiveness. This occurs with compounds that act as pro-drugs, requiring conversion to toxic derivatives by host cell factors for activity, and with molecules whose efficacy derives from their immunomodulatory properties. Pentavalent antimonials provide an example of a drug whose activity, like that of EGCG, is much more pronounced against intracellular parasites. These compounds kill parasites via their trivalent form, reduction apparently taking place in both the macrophage (Decuypere et al., 2005; Ponte-Sucre et al., 2017) and the amastigote, but not in promastigotes (Shaked-Mishan et al., 2001). Also contributing to parasite clearance might be their capacity to activate macrophages to produce toxic reactive oxygen and nitrogen species (Mookerjee Basu et al., 2006). Future studies will address whether any of these mechanisms applies to EGCG. Alternatively, macrophages could accumulate high levels of EGCG, as demonstrated for HIV-1 protease inhibitors which also require lower concentrations to eliminate intracellular amastigotes compared to axenic amastigotes (Trudel et al., 2008).
These parasite forms then set the basis for us to characterize the physiological alterations imposed by EGCG on L. infantum. In this regard, we observed that EGCG disturbs the mitochondrial polarization of L. infantum promastigotes, with concomitant decrease of intracellular ATP. Importantly, we also found that EGCG triggers an H2O2 boost in these parasites, whose reversion by the H2O2-eliminating enzyme catalase neutralizes the leishmanicidal effect of the flavonoid. Since trypanothione reductase (TR) is a central molecule of the H2O2 metabolism in Leishmania spp., we hypothesized that the EGCG-triggered boost of H2O2 resulted from TR inhibition. Our hypothesis was validated by 1) in silico analyses, where we found evidence that EGCG can bind to TR with Ki values spanning between 3.85 and 20.49 µM (depending on the cluster under analysis). High confidence models of EGCG/TR interaction predicted that the flavonoid could act as a competitor for the trypanothione substrate of TR by occupation of its binding pocket. Importantly, these predictions were confirmed by 2) in vitro kinetic assays of purified recombinant TR in the presence of EGCG, revealing a Ki of 293.9 ± 66.3 μM, this Ki value is higher when compared with other TR inhibitor such trivalent antimony (Sb+3), clomipramine and chalcone-based compounds (Baiocco et al., 2009a; Baiocco et al., 2009b; Jones et al., 2010; Ortalli et al., 2018). Positive correlations were observed between the inhibition of L. infantum promastigote proliferation and both EGCG-induced TR inhibition (Pearson correlation r = 0.9521) and increased intracellular H2O2 concentration in L. infantum promastigotes (Pearson correlation r = 0.9476)
Altogether, the findings reported in this manuscript add EGCG to a growing list of natural products with TR inhibitory activity (Uliassi et al., 2017; Ortalli et al., 2018). We reckon that, by interfering with this enzyme, central to the redox homeostasis of Leishmania spp. and related trypanosomatids, EGCG impairs the antioxidant response of the parasites, leading to the development of oxidative stress and resulting in the destruction of cellular macromolecular components promoting parasite death. This mechanism is also the basis of various antiprotozoal medications used to combat parasites in infected cells (Pal and Bandyopadhyay, 2012). At this point, we cannot exclude that EGCG also blocks other Leishmania molecules, being the accumulated inhibitory effect of the flavonoid over different targets that leads to parasite death.
In conclusion, the present data provide further insights into the mechanisms of action of EGCG against Leishmania infantum. Our results strongly suggest that EGCG, an effective compound for the treatment of visceral leishmaniasis (Inacio et al., 2019), competitively inhibits TR activity, causing an oxidative imbalance that leads to a decrease in mitochondrial membrane potential, thus reducing the intracellular ATP concentration.
Data Availability Statement
The original contributions presented in the study are included in the article/Supplementary Material. Further inquiries can be directed to the corresponding author.
Ethics Statement
The animal study was reviewed and approved by Committee on the Ethics of Animal Experiments of the Instituto Oswaldo Cruz (CEUA-IOC, License Number: L-11/2017).
Author Contributions
Conceptualization: JI and EA-A. Data curation: JI, MF, GL-S, HC, and EA-A. Formal analysis: JI, MF, GL-S, AT, HC, and EA-A. Investigation: JI, GL-S, AT, HC, and EA-A. Methodology: JI, MF, GL-S, AT, HC, and EA-A. Writing – original draft: JI, AT, HC, and EA-A. Writing – review and editing, JI, GL-S, AT, HC, and EA-A. All authors contributed to the article and approved the submitted version.
Funding
This work was supported by Fundação Carlos Chagas Filho de Amparo a Pesquisa do Estado do Rio de Janeiro (FAPERJ), Conselho Nacional de Desenvolvimento Científico e Tecnológico (CNPq), Programa Estratégico de Apoio a Pesquisa em Saúde (PAPES/FIOCRUZ);, and Fundação Oswaldo Cruz (FIOCRUZ). EA-A is the recipient of a research scholarship from Conselho Nacional de Desenvolvimento Científico e Tecnológico (CNPq). JI was supported by a postdoctoral fellowship from Conselho Nacional de Desenvolvimento Científico e Tecnológico (CNPq). JI is a recipient of a postdoctoral fellowship from Fundação Oswaldo Cruz (FIOCRUZ). HC is funded by Fundação para a Ciência e Tecnologia (FCT, Portugal) through the “Investigador FCT” contract IF/01244/2015.
Conflict of Interest
The authors declare that the research was conducted in the absence of any commercial or financial relationships that could be construed as a potential conflict of interest.
Supplementary Material
The Supplementary Material for this article can be found online at: https://www.frontiersin.org/articles/10.3389/fcimb.2021.640561/full#supplementary-material
References
Amato V. S., Tuon F. F., Bacha H. A., Neto V. A., Nicodemo A. C. (2008). Mucosal leishmaniasis . Current scenario and prospects for treatment. Acta Trop. 105 (1), 1–9. doi: 10.1016/j.actatropica.2007.08.003
Baiocco P., Colotti G., Franceschini S., Ilari A. (2009a). Molecular basis of antimony treatment in leishmaniasis. J. Med. Chem. 52 (8), 2603–2612. doi: 10.1021/jm900185q
Baiocco P., Franceschini S., Ilari A., Colotti G. (2009b). Trypanothione reductase from Leishmania infantum: cloning, expression, purification, crystallization and preliminary X-ray data analysis. Protein Pept. Lett. 16 (2), 196–200. doi: 10.2174/092986609787316306
Baiocco P., Poce G., Alfonso S., Cocozza M., Porretta G. C., Colotti G., et al. (2013). Inhibition of Leishmania infantum trypanothione reductase by azole-based compounds: A comparative analysis with its physiological substrate by x-ray crystallography. ChemMedChem 8, 1175–1183. doi: 10.1002/cmdc.201300176
Barral A., Pedral-Sampaio D., Grimaldi Junior G., Momen H., McMahon-Pratt D., Ribeiro de Jesus A., et al. (1991). Leishmaniasis in Bahia, Brazil: evidence that Leishmania amazonensis produces a wide spectrum of clinical disease. Am. J. Trop. Med. Hyg. 44 (5), 536–546. doi: 10.4269/ajtmh.1991.44.536
Battista T., Colotti G., Ilari A., Fiorillo A. (2020). Targeting Trypanothione Reductase, a Key Enzyme in the Redox Trypanosomatid Metabolism, to Develop New Drugs against Leishmaniasis and Trypanosomiases. Molecules 25 (8). doi: 10.3390/molecules25081924
Berman H. M., Westbrook J., Feng Z., Gilliland G., Bhat T. N., Weissig H., et al. (2000). The Protein Data Bank. Nucleic Acids Res. 28 (1), 235–242. doi: 10.1093/nar/28.1.235
Capela R., Moreira R., Lopes F. (2019). An Overview of Drug Resistance in Protozoal Diseases. Int. J. Mol. Sci. 20 (22). doi: 10.3390/ijms20225748
Castro H., Duarte M., Tomás A. M. (2018). “Chapter 16 The Redox Metabolism and Oxidative Stress in Leishmania as a Crossroads for the Lethal Effect of Drugs,” in Drug Discovery for Leishmaniasis (Croydon, England: The Royal Society of Chemistry), 316–347. doi: 10.1039/9781788010177-00316
Chappuis F., Sundar S., Hailu A., Ghalib H., Rijal S., Peeling R. W., et al. (2007). Visceral leishmaniasis: what are the needs for diagnosis, treatment and control? Nat. Rev. Microbiol. 5 (11), 873–882. doi: 10.1038/nrmicro1748
Colotti G., Baiocco P., Fiorillo A., Boffi A., Poser E., Chiaro F. D., et al. (2013). Structural insights into the enzymes of the trypanothione pathway: Targets for antileishmaniasis drugs. Future Med. Chem. 5, 1861–1875. doi: 10.4155/fmc.13.146
Croft S. L., Coombs G. H. (2003). Leishmaniasis–current chemotherapy and recent advances in the search for novel drugs. Trends Parasitol. 19 (11), 502–508. doi: 10.1016/j.pt.2003.09.008
De Muylder G., Ang K. K., Chen S., Arkin M. R., Engel J. C., McKerrow J. H. (2011). A screen against Leishmania intracellular amastigotes: comparison to a promastigote screen and identification of a host cell-specific hit. PloS Negl. Trop. Dis. 5 (7), e1253. doi: 10.1371/journal.pntd.0001253
Decuypere S., Rijal S., Yardley V., De Doncker S., Laurent T., Khanal B., et al. (2005). Gene expression analysis of the mechanism of natural Sb(V) resistance in Leishmania donovani isolates from Nepal. Antimicrob. Agents Chemother. 49 (11), 4616–4621. doi: 10.1128/AAC.49.11.4616-4621.2005
Dong R., Wang D., Wang X., Zhang K., Chen P., Yang C. S., et al. (2016). Epigallocatechin-3-gallate enhances key enzymatic activities of hepatic thioredoxin and glutathione systems in selenium-optimal mice but activates hepatic Nrf2 responses in selenium-deficient mice. Redox Biol. 10, 221–232. doi: 10.1016/j.redox.2016.10.009
Dumas C., Ouellette M., Tovar J., Cunningham M. L., Fairlamb A. H., Tamar S., et al. (1997). Disruption of the trypanothione reductase gene of Leishmania decreases its ability to survive oxidative stress in macrophages. EMBO J. 16 (10), 2590–2598. doi: 10.1093/emboj/16.10.2590
Fonseca-Silva F., Inacio J. D., Canto-Cavalheiro M. M., Almeida-Amaral E. E. (2011). Reactive oxygen species production and mitochondrial dysfunction contribute to quercetin induced death in Leishmania amazonensis. PloS One 6 (2), e14666. doi: 10.1371/journal.pone.0014666
Fonseca-Silva F., Canto-Cavalheiro M. M., Menna-Barreto R. F., Almeida-Amaral E. E. (2015). Effect of Apigenin on Leishmania amazonensis Is Associated with Reactive Oxygen Species Production Followed by Mitochondrial Dysfunction. J. Nat. Prod. 78 (4), 880–884. doi: 10.1021/acs.jnatprod.5b00011
Gervazoni L. F. O., Goncalves-Ozorio G., Almeida-Amaral E. E. (2018). 2’-Hydroxyflavanone activity in vitro and in vivo against wild-type and antimony-resistant Leishmania amazonensis. PloS Negl. Trop. Dis. 12 (12), e0006930. doi: 10.1371/journal.pntd.0006930
Gervazoni L. F. O., Barcellos G. B., Ferreira-Paes T., Almeida-Amaral E. E. (2020). Use of Natural Products in Leishmaniasis Chemotherapy: An Overview. Front. Chem. 8, 579891 (1031). doi: 10.3389/fchem.2020.579891
Grimaldi G. Jr., Tesh R. B. (1993). Leishmaniases of the New World: current concepts and implications for future research. Clin. Microbiol. Rev. 6 (3), 230–250. doi: 10.1128/CMR.6.3.230
Hamilton C. J., Saravanamuthu A., Eggleston I. M., Fairlamb A. H. (2003). Ellman’s-reagent-mediated regeneration of trypanothione in situ: substrate-economical microplate and time-dependent inhibition assays for trypanothione reductase. Biochem. J. 369 (Pt 3), 529–537. doi: 10.1042/BJ20021298
Holzer T. R., McMaster W. R., Forney J. D. (2006). Expression profiling by whole-genome interspecies microarray hybridization reveals differential gene expression in procyclic promastigotes, lesion-derived amastigotes, and axenic amastigotes in Leishmania mexicana. Mol. Biochem. Parasitol. 146 (2), 198–218. doi: 10.1016/j.molbiopara.2005.12.009
Inacio J. D., Canto-Cavalheiro M. M., Menna-Barreto R. F., Almeida-Amaral E. E. (2012). Mitochondrial damage contribute to epigallocatechin-3-gallate induced death in Leishmania amazonensis. Exp. Parasitol. 132 (2), 151–155. doi: 10.1016/j.exppara.2012.06.008
Inacio J. D., Gervazoni L., Canto-Cavalheiro M. M., Almeida-Amaral E. E. (2014). The effect of (-)-epigallocatechin 3-O–gallate in vitro and in vivo in Leishmania braziliensis: involvement of reactive oxygen species as a mechanism of action. PloS Negl. Trop. Dis. 8 (8), e3093. doi: 10.1371/journal.pntd.0003093
Inacio J. D. F., Fonseca M. S., Almeida-Amaral E. E. (2019). (-)-Epigallocatechin 3-O-Gallate as a New Approach for the Treatment of Visceral Leishmaniasis. J. Nat. Prod. 82 (9), 2664–2667. doi: 10.1021/acs.jnatprod.9b00632
Ioset J. R. (2008). Natural Products for Neglected Diseases: A Review. Curr. Organic Chem. 12 (8), 643–666. doi: 10.2174/138527208784577394
Jara M., Berg M., Caljon G., de Muylder G., Cuypers B., Castillo D., et al. (2017). Macromolecular biosynthetic parameters and metabolic profile in different life stages of Leishmania braziliensis: Amastigotes as a functionally less active stage. PloS One 12 (7), e0180532. doi: 10.1371/journal.pone.0180532
Jones D. C., Ariza A., Chow W. H., Oza S. L., Fairlamb A. H. (2010). Comparative structural, kinetic and inhibitor studies of Trypanosoma brucei trypanothione reductase with T. cruzi. Mol. Biochem. Parasitol. 169 (1), 12–19. doi: 10.1016/j.molbiopara.2009.09.002
Kaplum V., Cogo J., Sangi D. P., Ueda-Nakamura T., Correa A. G., Nakamura C. V. (2016). In Vitro and In Vivo Activities of 2,3-Diarylsubstituted Quinoxaline Derivatives against Leishmania amazonensis. Antimicrob. Agents Chemother. 60 (6), 3433–3444. doi: 10.1128/AAC.02582-15
Khan N., Mukhtar H. (2018). Tea Polyphenols in Promotion of Human Health. Nutrients 11 (1). doi: 10.3390/nu11010039
Kim H. S., Quon M. J., Kim J. A. (2014). New insights into the mechanisms of polyphenols beyond antioxidant properties; lessons from the green tea polyphenol, epigallocatechin 3-gallate. Redox Biol. 2, 187–195. doi: 10.1016/j.redox.2013.12.022
Leroux A. E., Krauth-Siegel R. L. (2016). Thiol redox biology of trypanosomatids and potential targets for chemotherapy. Mol. Biochem. Parasitol. 206 (1-2), 67–74. doi: 10.1016/j.molbiopara.2015.11.003
McGwire B. S., Satoskar A. R. (2014). Leishmaniasis: clinical syndromes and treatment. QJM 107 (1), 7–14. doi: 10.1093/qjmed/hct116
Meiering S., Inhoff O., Mies J., Vincek A., Garcia G., Kramer B., et al. (2005). Inhibitors of Trypanosoma cruzi trypanothione reductase revealed by virtual screening and parallel synthesis. J. Med. Chem. 48 (15), 4793–4802. doi: 10.1021/jm050027z
Mookerjee Basu J., Mookerjee A., Sen P., Bhaumik S., Sen P., Banerjee S., et al. (2006). Sodium antimony gluconate induces generation of reactive oxygen species and nitric oxide via phosphoinositide 3-kinase and mitogen-activated protein kinase activation in Leishmania donovani-infected macrophages. Antimicrob. Agents Chemother. 50 (5), 1788–1797. doi: 10.1128/AAC.50.5.1788-1797.2006
Morris G. M., Huey R., Lindstrom W., Sanner M. F., Belew R. K., Goodsell D. S., et al. (2009). AutoDock4 and AutoDockTools4: Automated docking with selective receptor flexibility. J. Comput. Chem. 30 (16), 2785–2791. doi: 10.1002/jcc.21256
Ndjonka D., Rapado L. N., Silber A. M., Liebau E., Wrenger C. (2013). Natural products as a source for treating neglected parasitic diseases. Int. J. Mol. Sci. 14 (2), 3395–3439. doi: 10.3390/ijms14023395
Ortalli M., Ilari A., Colotti G., De Ionna I., Battista T., Bisi A., et al. (2018). Identification of chalcone-based antileishmanial agents targeting trypanothione reductase. Eur. J. Med. Chem. 152, 527–541. doi: 10.1016/j.ejmech.2018.04.057
Pal C., Bandyopadhyay U. (2012). Redox-active antiparasitic drugs. Antioxid. Redox Signal 17 (4), 555–582. doi: 10.1089/ars.2011.4436
Pan American Health Organization (2018). Leishmaniasis: Epidemiological Report in the Americas (Washington) (Accessed January, 28 2019).
Ponte-Sucre A., Gamarro F., Dujardin J. C., Barrett M. P., Lopez-Velez R., Garcia-Hernandez R., et al. (2017). Drug resistance and treatment failure in leishmaniasis: A 21st century challenge. PloS Negl. Trop. Dis. 11 (12), e0006052. doi: 10.1371/journal.pntd.0006052
Richardson J. L., Nett I. R., Jones D. C., Abdille M. H., Gilbert I. H., Fairlamb A. H. (2009). Improved tricyclic inhibitors of trypanothione reductase by screening and chemical synthesis. ChemMedChem 4 (8), 1333–1340. doi: 10.1002/cmdc.200900097
Rijal S., Ostyn B., Uranw S., Rai K., Bhattarai N. R., Dorlo T. P., et al. (2013). Increasing failure of miltefosine in the treatment of Kala-azar in Nepal and the potential role of parasite drug resistance, reinfection, or noncompliance. Clin. Infect. Dis. 56 (11), 1530–1538. doi: 10.1093/cid/cit102
Saunders E. C., Ng W. W., Kloehn J., Chambers J. M., Ng M., McConville M. J. (2014). Induction of a stringent metabolic response in intracellular stages of Leishmania mexicana leads to increased dependence on mitochondrial metabolism. PloS Pathog. 10 (1), e1003888. doi: 10.1371/journal.ppat.1003888
Shaked-Mishan P., Ulrich N., Ephros M., Zilberstein D. (2001). Novel Intracellular SbV reducing activity correlates with antimony susceptibility in Leishmania donovani. J. Biol. Chem. 276 (6), 3971–3976. doi: 10.1074/jbc.M005423200
Tasdemir D., Kaiser M., Brun R., Yardley V., Schmidt T. J., Tosun F., et al. (2006). Antitrypanosomal and antileishmanial activities of flavonoids and their analogues: in vitro, in vivo, structure-activity relationship, and quantitative structure-activity relationship studies. Antimicrob. Agents Chemother. 50 (4), 1352–1364. doi: 10.1128/AAC.50.4.1352-1364.2006
Teixeira de Macedo Silva S., Visbal G., Lima Prado Godinho J., Urbina J. A., de Souza W., Cola Fernandes Rodrigues J. (2018). In vitro antileishmanial activity of ravuconazole, a triazole antifungal drug, as a potential treatment for leishmaniasis. J. Antimicrob. Chemother. 73 (9), 2360–2373. doi: 10.1093/jac/dky229
Tovar J., Wilkinson S., Mottram J. C., Fairlamb A. H. (1998). Evidence that trypanothione reductase is an essential enzyme in Leishmania by targeted replacement of the tryA gene locus. Mol. Microbiol. 29 (2), 653–660. doi: 10.1046/j.1365-2958.1998.00968.x
Trudel N., Garg R., Messier N., Sundar S., Ouellette M., Tremblay M. J. (2008). Intracellular survival of Leishmania species that cause visceral leishmaniasis is significantly reduced by HIV-1 protease inhibitors. J. Infect. Dis. 198 (9), 1292–1299. doi: 10.1086/592280
Uliassi E., Fiorani G., Krauth-Siegel R. L., Bergamini C., Fato R., Bianchini G., et al. (2017). Crassiflorone derivatives that inhibit Trypanosoma brucei glyceraldehyde-3-phosphate dehydrogenase (TbGAPDH) and Trypanosoma cruzi trypanothione reductase (TcTR) and display trypanocidal activity. Eur. J. Med. Chem. 141, 138–148. doi: 10.1016/j.ejmech.2017.10.005
Venkatesan S. K., Shukla A. K., Dubey V. K. (2010). Molecular docking studies of selected tricyclic and quinone derivatives on trypanothione reductase of Leishmania infantum. J. Comput. Chem. 31 (13), 2463–2475. doi: 10.1002/jcc.21538
Vermeersch M., da Luz R. I., Tote K., Timmermans J. P., Cos P., Maes L. (2009). In vitro susceptibilities of Leishmania donovani promastigote and amastigote stages to antileishmanial reference drugs: practical relevance of stage-specific differences. Antimicrob. Agents Chemother. 53 (9), 3855–3859. doi: 10.1128/AAC.00548-09
Keywords: EGCG, Leishmania infantum, mechanism of action, trypanothione reductase, competitive inhibitor
Citation: Inacio JDF, Fonseca MS, Limaverde-Sousa G, Tomas AM, Castro H and Almeida-Amaral EE (2021) Epigallocathechin-O-3-Gallate Inhibits Trypanothione Reductase of Leishmania infantum, Causing Alterations in Redox Balance and Leading to Parasite Death. Front. Cell. Infect. Microbiol. 11:640561. doi: 10.3389/fcimb.2021.640561
Received: 20 January 2021; Accepted: 09 March 2021;
Published: 25 March 2021.
Edited by:
Brice Rotureau, Institut Pasteur, FranceReviewed by:
Wanderley De Souza, Federal University of Rio de Janeiro, BrazilAndrea Ilari, Italian National Research Council, Italy
Marina Gramiccia, ISS, Italy
Copyright © 2021 Inacio, Fonseca, Limaverde-Sousa, Tomas, Castro and Almeida-Amaral. This is an open-access article distributed under the terms of the Creative Commons Attribution License (CC BY). The use, distribution or reproduction in other forums is permitted, provided the original author(s) and the copyright owner(s) are credited and that the original publication in this journal is cited, in accordance with accepted academic practice. No use, distribution or reproduction is permitted which does not comply with these terms.
*Correspondence: Elmo E. Almeida-Amaral, ZWxtb0Bpb2MuZmlvY3J1ei5icg==