- 1Department of Clinical Microbiology and Laboratory for Molecular Infection Medicine Sweden (MIMS), Umeå University, Umeå, Sweden
- 2Department of Immunoparasitology, Research Institute for Microbial Diseases, Osaka, Japan
- 3CIRI, Centre International de Recherche en Infectiologie, Inserm U1111, Université Claude Bernard Lyon 1, CNRS, UMR5308, ENS de Lyon, Univ Lyon, Lyon, France
Francisella tularensis is a Select Agent that causes the severe disease tularemia in humans and many animal species. The bacterium demonstrates rapid intracellular replication, however, macrophages can control its replication if primed and activation with IFN-γ is known to be essential, although alone not sufficient, to mediate such control. To further investigate the mechanisms that control intracellular F. tularensis replication, an in vitro co-culture system was utilized containing splenocytes obtained from naïve or immunized C57BL/6 mice as effectors and infected bone marrow-derived wild-type or chromosome-3-deficient guanylate-binding protein (GBP)-deficient macrophages. Cells were infected either with the F. tularensis live vaccine strain (LVS), the highly virulent SCHU S4 strain, or the surrogate for F. tularensis, F. novicida. Regardless of strain, significant control of the bacterial replication was observed in co-cultures with wild-type macrophages and immune splenocytes, but not in cultures with immune splenocytes and GBPchr3-deficient macrophages. Supernatants demonstrated very distinct, infectious agent-dependent patterns of 23 cytokines, whereas the cytokine patterns were only marginally affected by the presence or absence of GBPs. Levels of a majority of cytokines were inversely correlated to the degree of control of the SCHU S4 and LVS infections, but this was not the case for the F. novicida infection. Collectively, the co-culture assay based on immune mouse-derived splenocytes identified a dominant role of GBPs for the control of intracellular replication of various F. tularensis strains, regardless of their virulence, whereas the cytokine patterns markedly were dependent on the infectious agents, but less so on GBPs.
Introduction
Francisella tularensis is a facultative intracellular bacterium and the etiological agent of tularemia, a highly infectious disease affecting humans and a wide range of animals. F. tularensis is highly contagious, since its infectious dose is very low and it may be spread via aerosol. These characteristics, together with high virulence, have led to its classification as a Tier 1 Select Agent, along with other potential agents of bioterrorism. Tularemia is an emerging disease in several parts of the world and extensive outbreaks have occurred in some countries in Scandinavia and Eastern Europe and in Turkey. Two subspecies cause human disease, subspecies tularensis, with high mortality resulting if untreated, and subspecies holarctica, which despite lower virulence still can cause serious illness in humans. Currently, no licensed tularemia vaccine is available; however, the live vaccine strain (LVS) of subsp. holarctica has been used as a human vaccine and confers efficacious protection against laboratory-acquired infection (Burke, 1977). The closely related species F. novicida is an often used surrogate for F. tularensis and although a very rare human pathogen, it is virulent for many animal species and highly virulent in the frequently used mouse model of tularemia.
Cell-mediated immunity is critically required to control tularemia in human and animal models of the disease and therefore a comprehensive understanding of the protective cell-mediated mechanisms will be essential as part of the efforts to generate efficacious vaccines. Development of a licensed vaccine is dependent on the identification of correlates of protection; however, such correlates are elusive for infections requiring cell-mediated protection. With regard to tularemia, much work in experimental models have focused on the role of IFN-γ, which has been shown to be essential, although alone not sufficient to control infection. Population-based testing of vaccine candidates cannot be performed, since tularemia generally is an uncommon disease and even in endemic areas, it occurs highly irregularly (Sjöstedt, 2007) and, therefore, assessment of efficacy will not be feasible. Vaccine efficacy was evaluated in the 1950s by challenging volunteers with F. tularensis, however, it is unlikely that such future studies will be deemed ethically acceptable due to the severity of respiratory tularemia. To circumvent these limitations, an option is to utilize the FDA Animal Rule (Snoy, 2010) in order to license a tularemia vaccine. The rule is based on the premise of the exclusive use of relevant animal models to identify correlates of protection pertinent to human infection and thereafter to extrapolate data on efficacy in these models to efficacy in humans.
The existing evidence indicates that protection to F. tularensis is achieved through an intricate interaction of several T cells subsets and multiple cytokines that jointly effectuate control of infection (Cowley and Elkins, 2011; de Pascalis et al., 2012; Eneslätt et al., 2012; Mahawar et al., 2013; de Pascalis et al., 2014; Griffin et al., 2015; Golovliov et al., 2016). Thus, the mechanisms cannot be identified by use of infection assays based on a single cell type, rather assays are required that faithfully mirror the complex interplay that occurs in vivo. Therefore, models are required that allow detailed characterization of mechanisms that control bacterial intracellular replication and that also can be used to validate potential protective cell-mediated correlates, as required by the Animal Rule. Of relevance, a co-culture assay has been widely used for this purpose and is based on a combination of effector cells derived from naïve or immune animals that are added to cultures with infected monocytic cells (Elkins et al., 2011). Thereby, replication of F. tularensis can be followed over time and immune activation carefully assessed. There are numerous examples when the assay has been used to identify potential correlates of protection against F. tularensis (Collazo et al., 2009; Elkins et al., 2011; de Pascalis et al., 2012; Mahawar et al., 2013; de Pascalis et al., 2014; Griffin et al., 2015; Golovliov et al., 2016; de Pascalis et al., 2018; Eneslätt et al., 2018; Lindgren et al., 2020). To further assess the relevance of the findings, validation can be achieved by demonstrating that potential correlates identified contribute to protection in animal models (Kurtz et al., 2013; Melillo et al., 2013; Melillo et al., 2014). There are several animal models that are considered to be highly relevant as experimental models for tularemia, e.g., the mouse, rat, rabbit, and non-human primate models, since the target organs and histo-pathology closely resemble those of humans and all of these species can be naturally infected with F. tularensis (Lyons and Wu, 2007).
Many pathogenic bacteria and parasites have evolved sophisticated means to invade and replicate within host cells and in parallel, eukaryotes have developed counter-measures to effectively detect the invasion by these intracellular microorganisms and rapidly mount an antimicrobial response. One recently identified key mechanism for this detection is mediated by the Guanylate Binding Proteins (GBPs), belonging to a family of interferon-inducible dynamin-like GTPases. In fact, for many pathogenic intracellular bacteria and parasites, the role of GBPs appear essential for the execution of the IFN-γ-induced protective immune response (Tretina et al., 2019). However, how the effectuation of their antimicrobial functions is mediated is not fully understood, but it has been suggested that GBPs directly target and disrupt pathogen-containing vacuoles, as evidenced by findings on Toxoplasma and Salmonella (Man et al., 2017). In the case of Gram-negative bacteria, it has been observed that GBPs destabilize the rigidity of the bacterial outer membrane by binding to LPS and induce LPS clustering through GBP polymerization (Kutsch et al., 2020). There is also evidence for a link between GBPs and the inflammasomes (Meunier and Broz, 2016) and this has been clearly demonstrated to be the case for the AIM2 inflammasome in macrophages infected with F. novicida (Fernandes-Alnemri et al., 2010; Jones et al., 2010). In fact, the GBPs have been suggested to serve as master regulators of numerous inflammasomes (Wallet et al., 2017). After being ingested, Francisella strains rapidly lyse the phagosome, escape into and replicate within the host cytosol. In the murine system, the chromosome 3-encoded GBPs, GBP2 and GBP5, are recruited to cytosolic F. novicida and are required to lyse bacteria and release the bacterial genomic DNA into the host cytosol where it is recognized by AIM2 (Fernandes-Alnemri et al., 2010). However, although GBPs serve a key role for control of certain inflammasomes, control of intracellular F. novicida replication occurs independently of inflammasomes, but strictly dependent on GBPs (Wallet et al., 2017). Notably, GBPchr3-deficient mice showed no control of an F. novicida infection, despite high levels of circulating IFN-γ (Wallet et al., 2017). Whereas control of intracellular replication of F. novicida and the LVS strain was completely reversed in the absence of GBPs, no control of the highly virulent SCHU S4 strain was observed, neither was exacerbation seen in the absence of GBPs (Wallet et al., 2017).
Here, we asked whether a complex co-culture system would provide additional information regarding the role of GBPs for the control of intracellular F. tularensis infection beyond what has already been revealed by the use of intramacrophage assays and the mouse model. As effector cells, ΔclpB-immune splenocytes were used, since they execute very potent control of F. tularensis infection (Golovliov et al., 2016). Cultures also contained bone marrow-derived macrophages (BMDM) from wild-type or GBPchr3-deficient macrophages infected with either F. novicida, the LVS, or the SCHU S4 strain, the latter a highly virulent subspecies tularensis strain. We observed that in the co-culture system, in contrast to what is observed when cultures with BMDM alone, SCHU S4 replication was significantly controlled. Although control of SCHU S4 replication was not as marked as control of LVS or F. novicida replication, it was mostly GBP-dependent. Thus, the co-culture assay with immune mouse-derived cells identified a critical role of GBPs for the control of intracellular replication of various F. tularensis strains, regardless of their virulence.
Materials and Methods
Bacterial Strains
F. tularensis LVS (F. tularensis subsp. holarctica), F. novicida U112, and F. tularensis strain SCHU S4 (F. tularensis subsp. tularensis) were obtained from the American Type Culture Collection, ATCC 29684 and ATCC 15482 and from the Francisella Strain Collection of the Swedish Defense Research Agency, Umeå, Sweden, respectively. Work with the SCHU S4 strain was performed in a biosafety level 3 facility certified by the Swedish Work Environment Authority. The generation of the ΔclpB mutant and its utility as an efficacious vaccine have previously been described (Conlan et al., 2010; Golovliov et al., 2013).
Animals
C57/BL6 mice obtained from Charles River, Germany were used. Immunization was performed with a subcutaneous injection of 5 × 103 CFU of the ΔclpB strain. This results in an infection with no or only slight symptoms during peak replication of bacteria that occurs around day 4–6 of infection. GBPchr3–/– C57BL/6 mice have been previously described (Yamamoto et al., 2012). Ethical approval for the described mouse experiments was obtained from the Ethical Committee on Animal Research, Umeå, Sweden, A67-14 and A36-2019, or the University of Lyon, France (CEC-CAPP) under the protocol no. #ENS_2012_061 in accordance with the European regulations (#2010/63/UE).
Generation of BMDM
Bone-marrow-derived macrophages were prepared by collecting bone marrow from the femurs of mice and then plating the cells in Petri dishes in DMEM (Invitrogen Life Technologies) and supplemented with 10% fetal calf serum (Invitrogen Life Technologies), 10 mM HEPES (Invitrogen Life Technologies), and 30% macrophage colony-stimulating factor (M-CSF)-conditioned medium. The latter was collected from an L929 M-CSF cell line. After incubation at 37°C in 5% CO2 for 6 days, BMDM were harvested and added to 24-well plates, incubated overnight, and then used in the co-culture assay. The viability of the BMDM was determined using staining with trypan blue and enumeration with a Vi-CELL XR cell viability analyzer (Beckman Coulter).
Splenocyte Preparation
After immunization of mice, spleens were removed 4 to 5 weeks later and cells were obtained by squeezing the organ. They were prepared as previously described (Golovliov et al., 2016). The cell suspension was treated with ammonium chloride to lyse erythrocytes, washed with PBS + 2% FBS, and suspended in complete DMEM (cDMEM), DMEM supplemented with fetal calf serum and HEPES.
Infection of the BMDM in the Co-Culture Assay
Bacteria were cultivated overnight on modified Gc-agar plates. After harvesting, they were added to BMDM cultures using an MOI of 0.2 of bacteria per BMDM and after 2 h, medium was removed and the cultures washed twice with DMEM. cDMEM with 20 µg/ml of gentamicin was added and after incubation for 45 min, cultures were washed with PBS. Then, 200 µl of cDMEM with 2.5 × 106 splenocytes was added, resulting in a ratio of five splenocytes per BMDM. This was defined as time 0 h. After lysis of cells at 0 and 72 h, enumeration of bacteria was performed by plating of serial dilutions on Gc-agar plates.
Cytokine Analysis
Supernatants from cell cultures were collected and stored at −80°C. Analysis was performed with a 23-plex kit (#M60009RDPD (BioRad Laboratories Inc, Hercules, CA, USA) using a Bio-Plex 200 system following the instructions of the manufacturer. Preliminary experiments indicated that significant increases of cytokine levels occurred between 24 and 48 h after infection, whereas changes between 48 and 72 h were mostly non-significant, therefore, the 48 h time point was chosen for analysis of cytokines.
Data Analysis and Statistical Methods
All statistical analyses were performed using IBM SPSS statistics version 25. One-way ANOVA with Tukey’s test for multiple comparisons was used to analyze the significance of differences between different groups. A value of P < 0.05 was considered significant. Correlations were estimated using Spearman’s correlation. Twenty-three cytokines measured in co-cultures incubated with immune cells were analyzed by stepwise linear discriminant analysis (LDA) with Wilks’ lambda variable selection method. The F entry value was set to F > 3.84 and F removal value was set to F < 2.71.
Results
Intracellular Replication of F. tularensis Strains
Co-cultures were established by overlaying BMDM monolayers infected with either the F. novicida, LVS, or SCHU S4 strains with splenocytes from naïve or ΔclpB-immune mice and bacterial replication was followed for a period of 72 h. In cultures with wild-type BMDM, the outcome of the F. novicida and LVS infections was rather similar and addition of immune splenocytes resulted in approximately 1.7 log10 CFU lower numbers than in cultures with naïve splenocytes (P < 0.01 for F. novicida and P < 0.001 for LVS; Figures 1A, B). In contrast, control of the SCHU S4 infection was less marked, approximately 0.7 log10 CFU lower in cultures with immune splenocytes (P < 0.01; Figure 1C). Regardless of infecting strain, bacterial replication was similar in cultures with naïve splenocytes and wild-type BMDM or GBPchr3-deficient BMDM (P > 0.05; Figures 1A–C). Compared to cultures with wild-type BMDM, the CFU of the GBPchr3-deficient BMDM cultures showed much smaller differences between immune and naïve splenocytes; 0.5 log10 CFU for F. novicida, 0.6 log10 CFU for LVS, and 0.2 log10 CFU for SCHU S4 (P > 0.05 for all strains; Figures 1A–C). Addition of immune splenocytes resulted in significantly lower bacterial numbers, regardless of infection, in cultures with wild-type BMDM than with GBPchr3-deficient BMDM (P < 0.05 for F. novicida and SCHU S4 and P < 0.01 for LVS; Figures 1A–C).
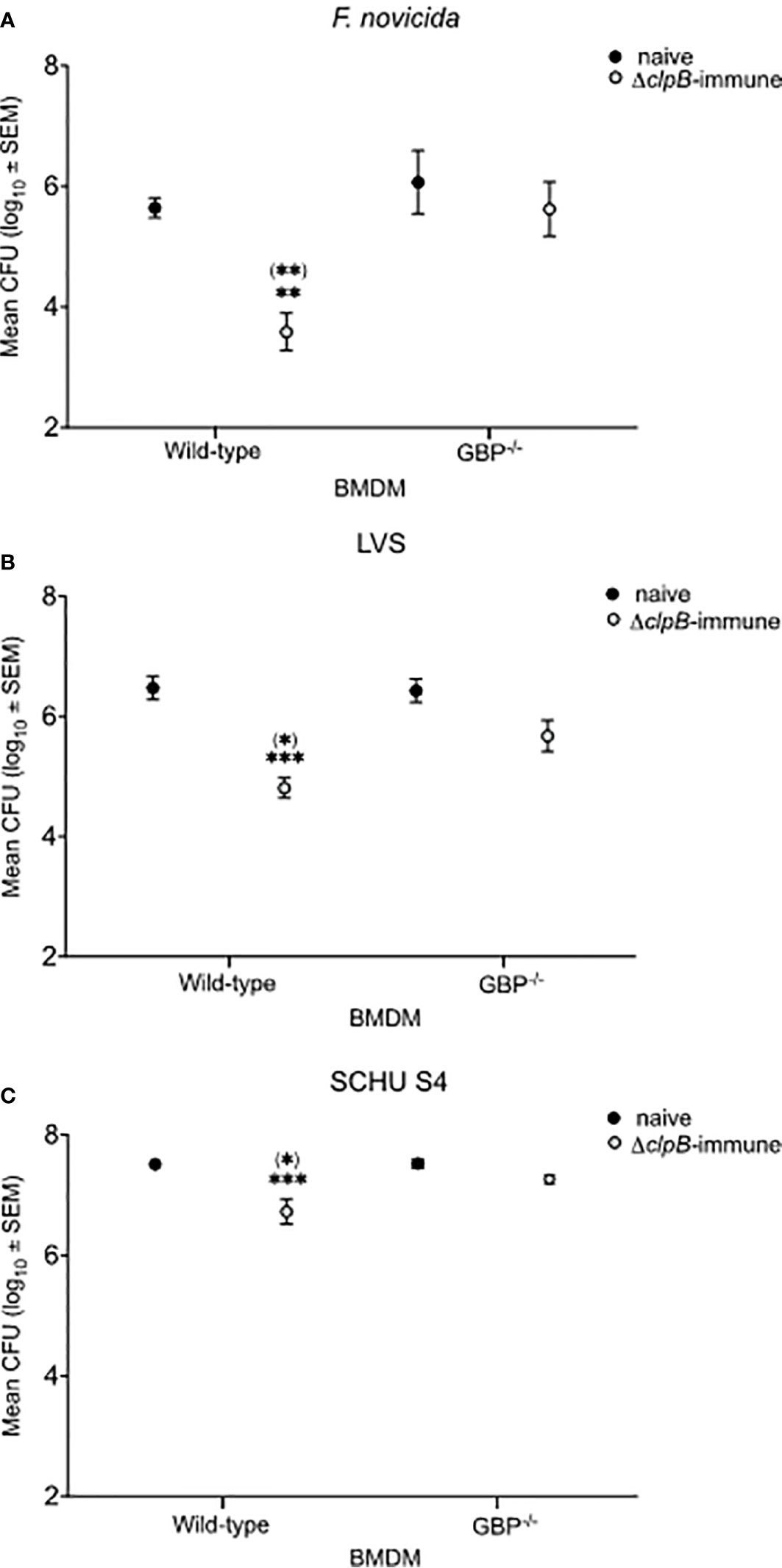
Figure 1 Intracellular replication of F. tularensis strains in the co-culture assay. BMDM monolayers were infected with (A) F. novicida, (B) LVS, or (C) SCHU S4 and thereafter overlaid with splenocytes from either naïve or ΔclpB-immunized mice. Bacterial numbers were recorded after 72 h of infection. The bars represent CFU values from nine cultures generated in three separate experiments. Stars above the bars indicate significant difference in comparison to the values of cultures with wild-type BMDM and naïve splenocytes. Stars in brackets indicate significant differences vs. cultures with GBPchr3−/−-deficient BMDM with ΔclpB-immune splenocytes.
Cytokine Production in Co-Cultures
The levels of 23 cytokines were measured in the culture supernatants after 48 h of infection. A comparative analysis of the levels was performed and the results were expressed as P-values in Tables 1–3, with levels of the cultures infected with F. novicida as the reference, and the absolute values are provided in Figure S1.
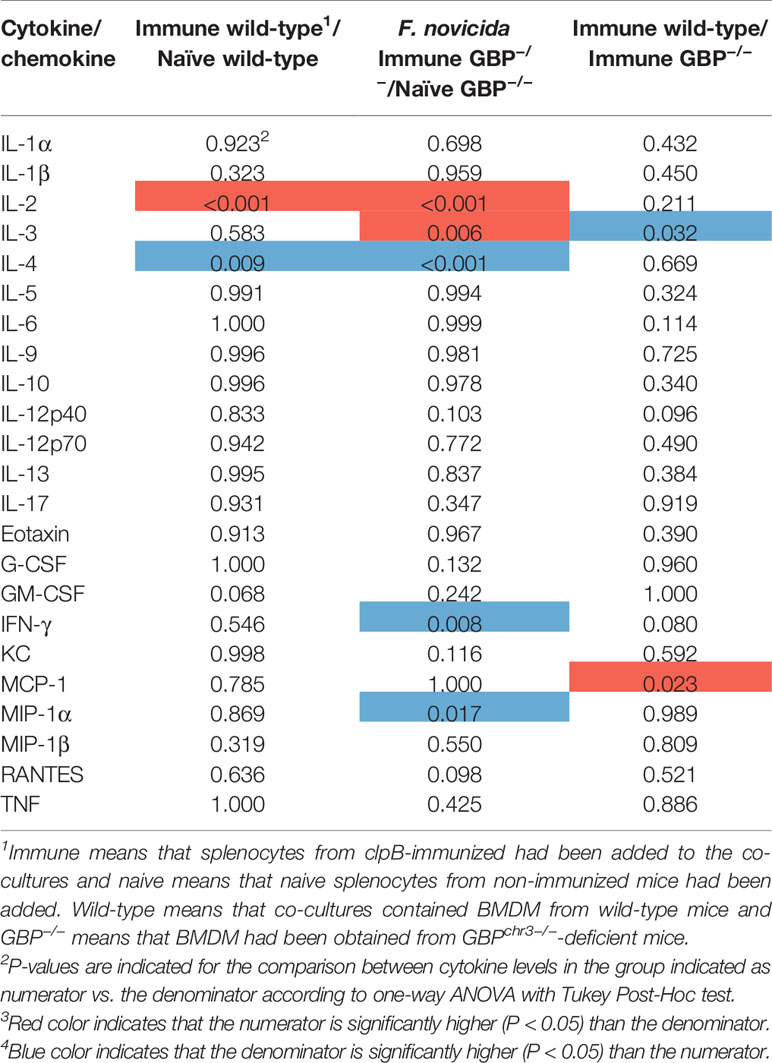
Table 1 Differences in cytokine levels, expressed as P-values, between groups of F. novicida-infected co-cultures.
In cultures infected with F. novicida, IL-2 was the only cytokine expressed at significantly higher levels in wild-type BMDM cultures with immune vs. naïve splenocytes, whereas levels of IL-4 was higher in the latter (Table 1). In cultures with GBPchr3-deficient BMDM, again, IL-2, together with IL-3, were higher in cultures with immune vs. naïve splenocytes and, again, IL-4, as well as MIP-1β and IFN-γ, were higher in the latter cultures (Table 1). When cultures with immune splenocytes were compared, IL-3 was the sole cytokine expressed at higher levels in cultures with wild-type BMDM and MCP-1 the only cytokine expressed at higher levels with GBPchr3-deficient BMDM.
After infection with LVS, six cytokines were significantly higher in wild-type BMDM cultures with immune vs. naïve splenocytes, whereas in cultures with GBP-deficient BMDM IL-2 and IL-3 were higher and IL-4 lower with immune vs. naïve splenocytes (Table 2). When cultures with immune splenocytes were compared, levels of five cytokines, IL-5, eotaxin, MCP-1, MIP-1β, and RANTES, were higher with in cultures with wild-type BMDM vs. GBPchr3-deficient BMDM (Table 2).
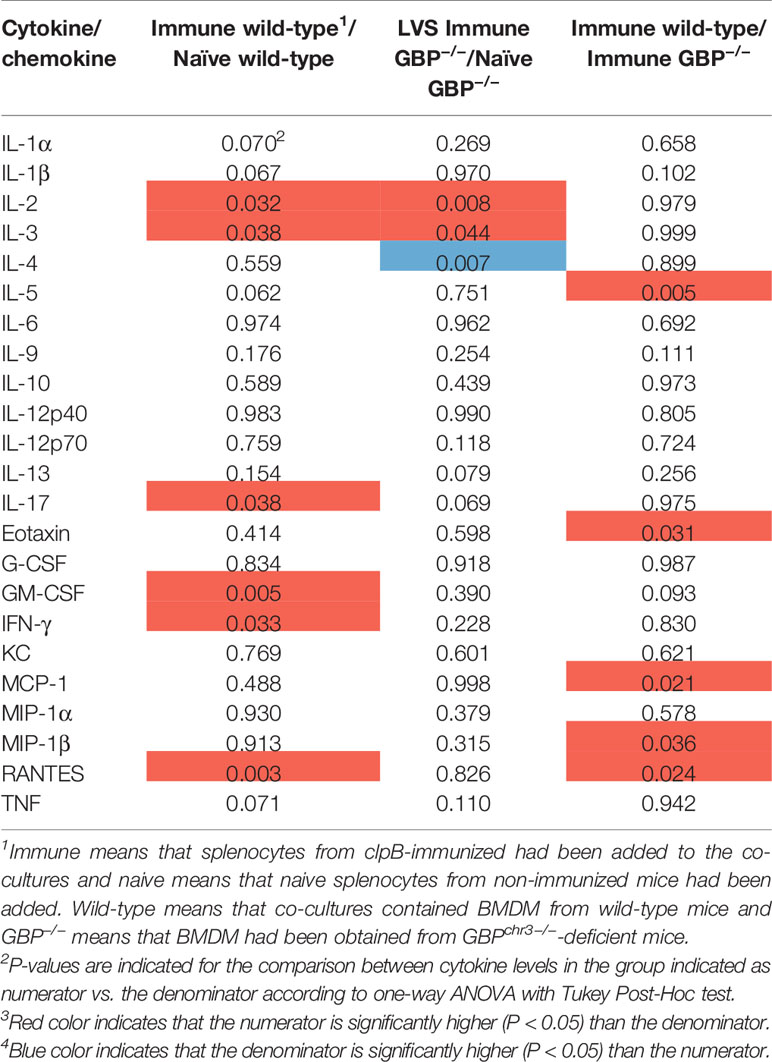
Table 2 Differences, expressed as P-values, in cytokine levels between groups of LVS-infected co-cultures.
After infection with SCHU S4, 18 cytokines were secreted at significantly higher levels in wild-type BMDM cultures with immune vs. naïve splenocytes, whereas in GBP-deficient cultures, 20 cytokines were higher with immune vs. naïve splenocytes (Table 3). Eight cytokines were expressed at higher levels in immune splenocyte co-cultures with wild-type BMDM vs. co-cultures with GBPchr3-deficient BMDM, including IL-1β, MCP-1, MIP-1β, IL-12p40, and RANTES, whereas two, IL-1α and KC, were higher in the latter cultures (Table 3). These results demonstrate that the immune splenocytes induced a pronounced immune activation in co-cultures regardless of the BMDM phenotype and also, in comparison to LVS- or F. novicida-infected cultures, that the differences between cultures with immune vs. naïve splenocytes were much more marked for the SCHU S4-infected cultures.
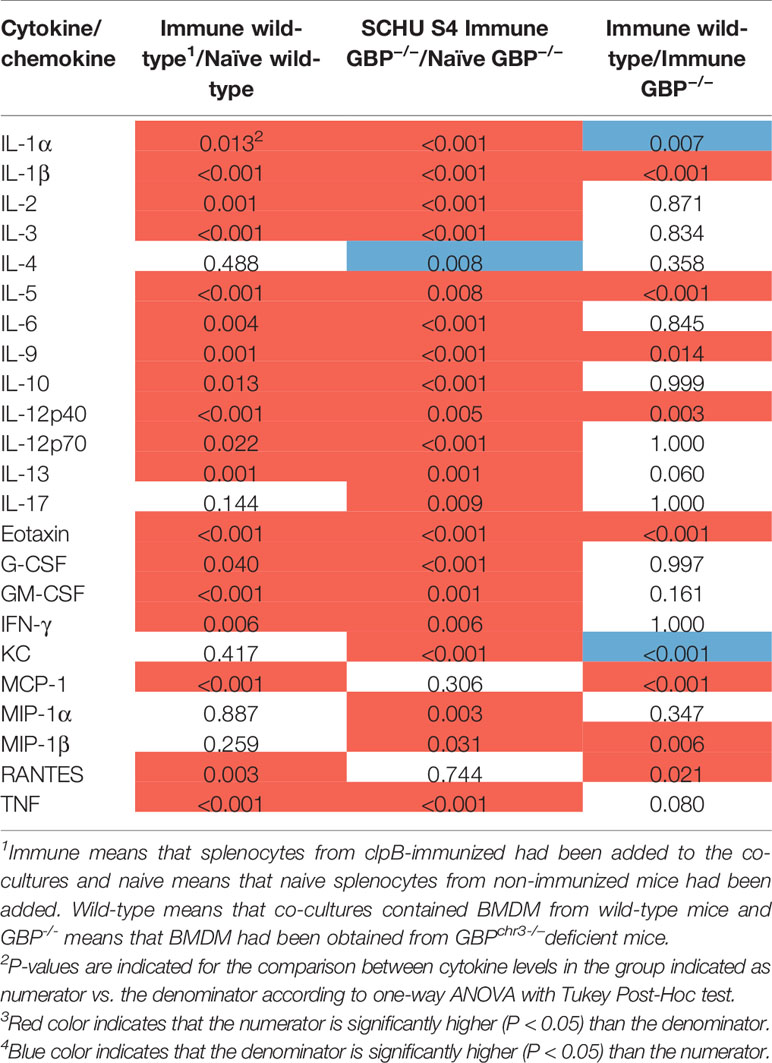
Table 3 Differences, expressed as P-values, in cytokine levels between groups of SCHU S4-infected co-cultures.
Relative cytokine levels in cultures with immune splenocytes are shown for all infections in Tables 4 and 5, with levels of the cultures infected with F. novicida as the reference, and the absolute values are provided in Figure S1. In cultures with immune splenocytes and wild-type macrophages, levels of a majority of cytokines were highest in those infected with SCHU S4. Compared to the F. novicida-infected cultures, levels of 15 cytokines were significantly higher, including IL-2, IFN-γ, TNF, GM-CSF, and IL-17 (Table 4). The cultures with the same cellular composition infected with LVS showed higher levels of eight cytokines compared to the F. novicida-infected cultures, including the aforementioned cytokines. A comparison between SCHU S4 and LVS-infected cultures, demonstrated that the levels were higher for 12 cytokines of the former and one of the latter. In cultures with immune splenocytes and GBPchr3-deficient macrophages, the differences between the infections were not consistently higher in one or the other. When cultures infected with SCHU S4 were compared to those infected with F. novicida, levels were higher for IL-3, the Th2 cytokine IL-4 and the Th1 cytokine GM-CSF of the former and the chemokine RANTES was higher of the latter. The same comparison between LVS- and F. novicida-infected cultures, demonstrated higher levels of IL-1α and KC for the latter (Table 5).
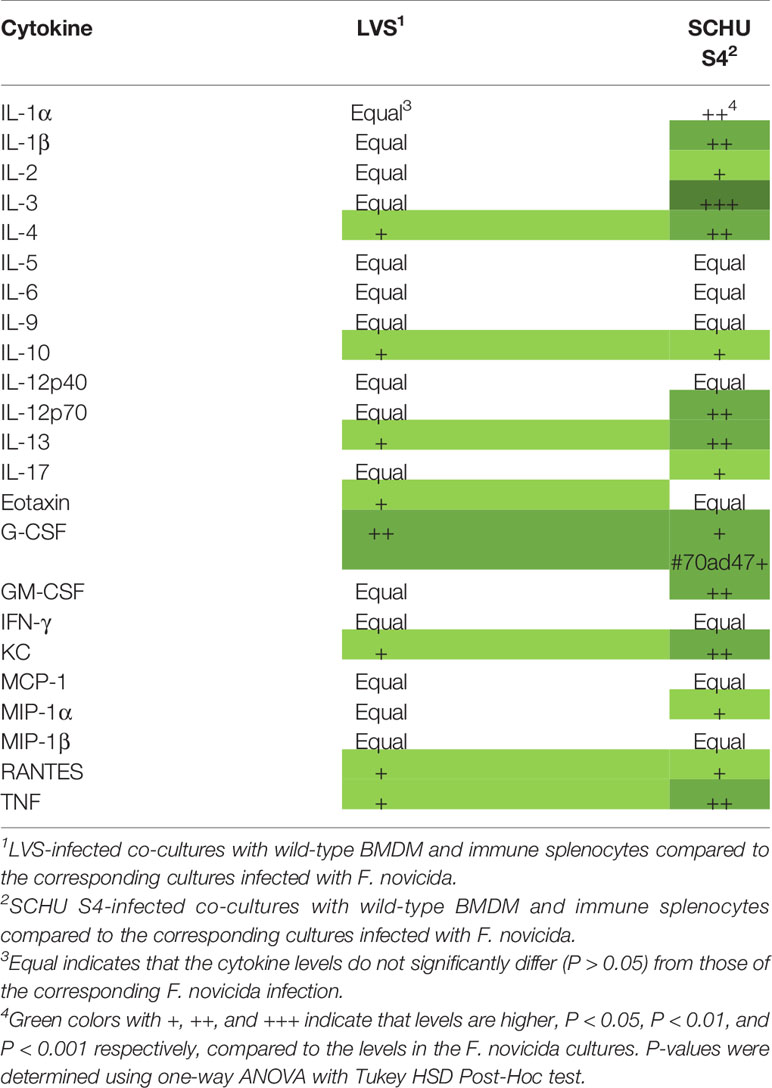
Table 4 Analysis of cytokine levels in F. novicida-infected co-cultures with wild-type BMDM and immune splenocytes compared to the corresponding cultures infected with LVS or SCHU S4.
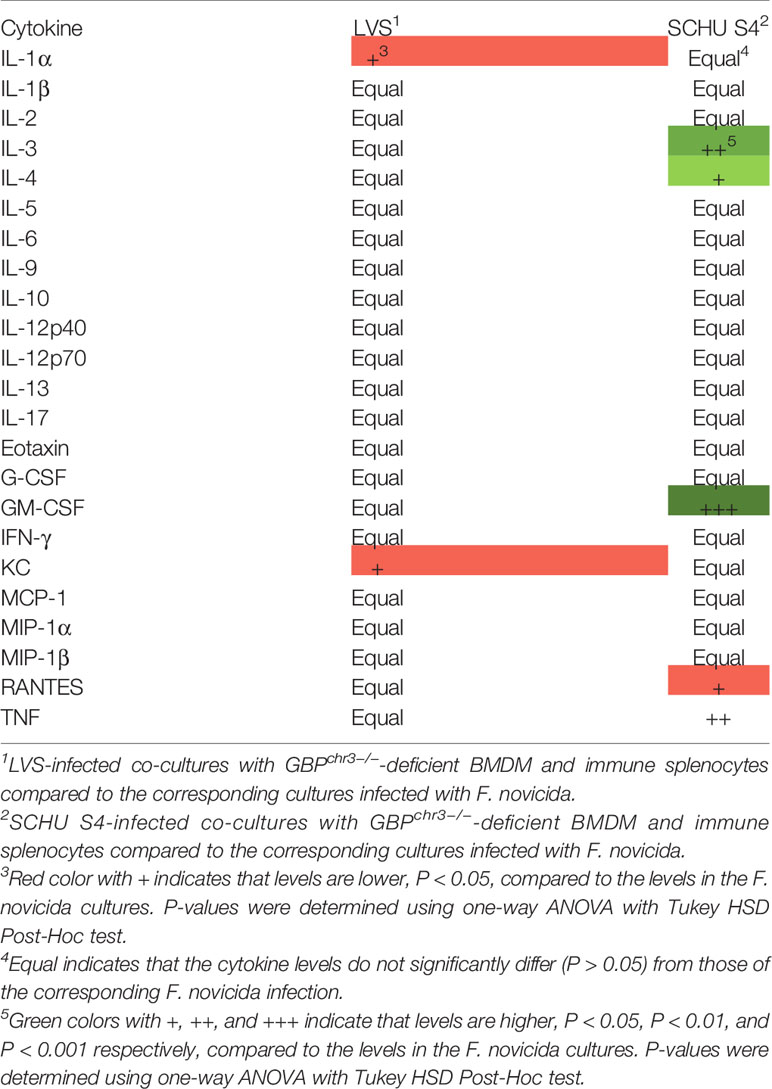
Table 5 Analysis of cytokine levels in F. novicida-infected co-cultures with GBPchr3−/−-deficient BMDM and immune splenocytes compared to the corresponding cultures infected with LVS or SCHU S4.
Correlation Between Cytokines and Control of Bacterial Infection
Levels of individual cytokines were correlated to bacterial numbers after 72 h. A majority of cytokines in the SCHU S4- and LVS-infected cultures, 17 and 15, respectively, was significantly inversely correlated to bacterial numbers with Spearman´s rho of >0.5 (P < 0.05; Table 6). In contrast, none of the 23 cytokines showed an inverse correlation to bacterial numbers in the F. novicida-infected cultures, instead 13 showed positive correlation with bacterial numbers (Table 6). Thus, levels of many individual cytokines were inversely correlated with the bacterial numbers of the SCHU S4- and LVS-infected cultures, whereas a majority of cytokines showed a positive correlation with the numbers of F. novicida. Of the 17 cytokines in the SCHU S4 cultures and 15 cytokines in the LVS cultures that showed significant inverse correlations, several have previously been identified as correlates of protection, e.g., IL-2, IFN-γ, TNF, IL-12p40, GM-CSF, and IL-17 and most of these cytokines are also characteristic of a Th1 T cell response. Notably, IL-2, IFN-γ, IL-12p40, and GM-CSF were not among the 13 cytokines that correlated to bacterial numbers in the F. novicida-infected cultures.
Discrimination of Co-Cultures With Immune Cells Based on Cytokine Patterns
Linear discriminant analysis was used to determine whether individual cytokines, or sets of cytokines, could differentiate between the six groups of co-cultures with immune cells. The cytokines giving the best separation of the groups were GM-CSF, IL-6, IL-10, Eotaxin, and RANTES (Table 7). To further illustrate the discriminative ability of the set of cytokines, the data were plotted using discriminant loading (Figure 2). The results demonstrate that both the infectious agent and BMDM phenotype affected the location of each group (Figure 2). The SCHU S4-infected wild-type and GBPchr3-deficient cultures were relatively distant from each other and also distinct from the other four groups. The LVS-infected cultures demonstrated an intermediate position compared to the other groups, although they were relatively distant to each other. The F. novicida-infected cultures were localized far away from each other and also far from the SCHU S4-infected cultures, in particular the culture with GBPchr3-deficient BMDM (Figure 2). The classification correctness of the model built by the linear discriminate analysis was 100% for three groups and 83% for the remaining groups; LVS-infected co-cultures and the F. novicida-infected co-culture with GBPchr3-deficient BMDM (Figure 2).
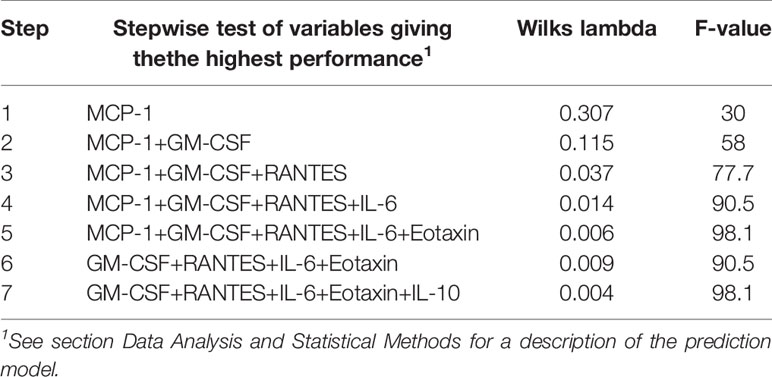
Table 7 Stepwise LDA was used to select variables that best classified the co-cultures according to BMDM phenotype and type of infection.
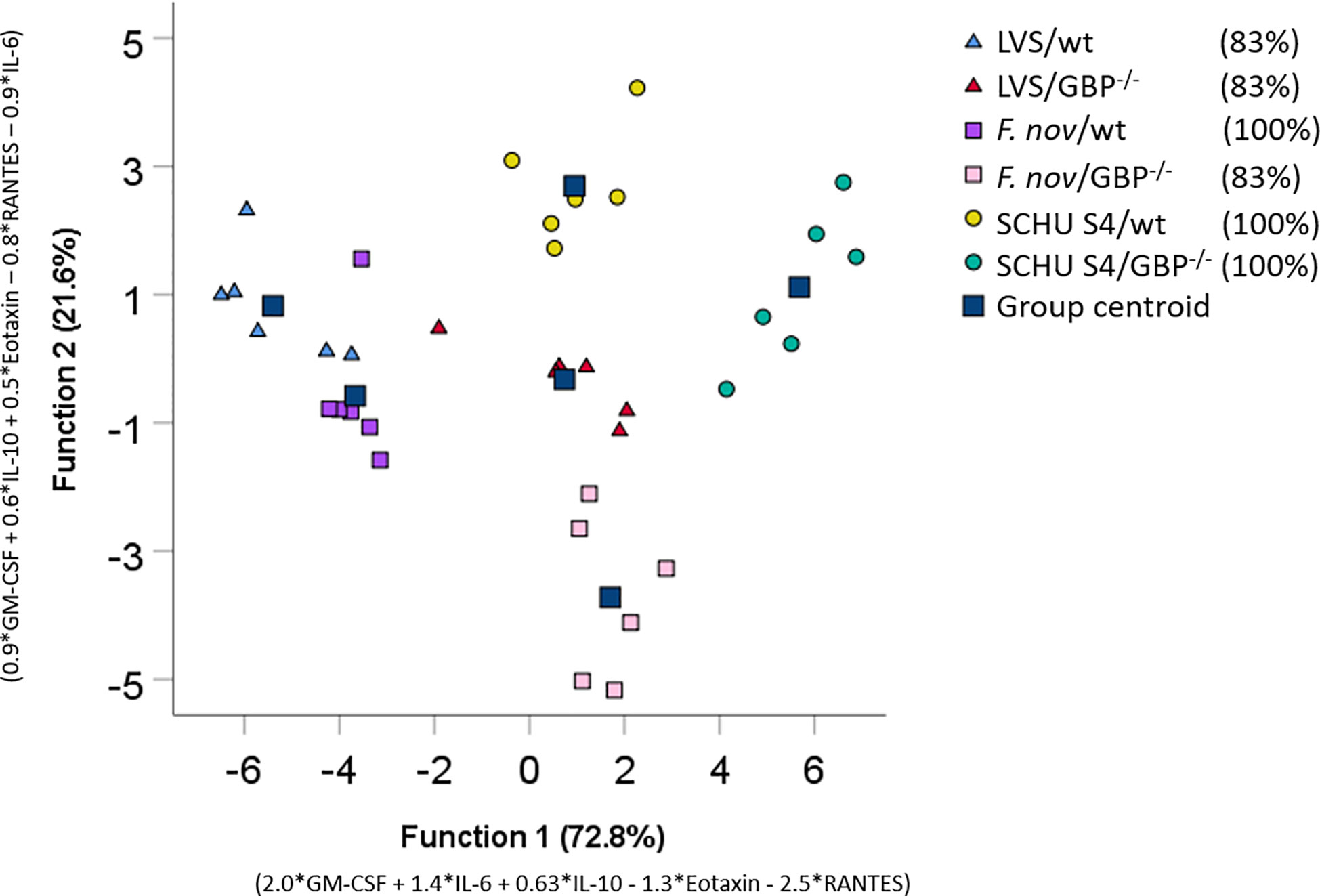
Figure 2 Discriminant loadings of co-cultures. Twenty-three cytokines measured in co-cultures incubated with immune cells were included in a stepwise discriminant function analysis. Function 1 and 2 explains 72.8 and 21.6% of the variance respectively and are depicted on the x- and y-respectively. Each data point corresponds to each replicate individual in a group and the squares represent the group centroid. The percentage values of group classification correctness are presented in brackets.
In summary, using linear discriminant analysis and combining values for five cytokines, a high-resolution identification was achieved for each of the co-culture groups.
Discussion
Cell-mediated immunity is essential for control of a majority of intracellular microorganisms, however, to qualitatively and quantitatively characterize cell-mediated mechanisms executing protection is very challenging, unlike measurements used to describe humoral immune responses. In fact, there are no validated methods for describing cell-mediated correlates of protection and this is an obvious limitation hampering rational vaccine development for infections caused by intracellular microorganisms. With regard to development of tularemia vaccines, another limitation is the fact that tularemia is an infrequent disease in most countries and in other countries occurring very irregularly, therefore, clinical trials to assess vaccine efficacy will not be feasible. Thus, the Animal Rule may be the only possibility to license a tularemia vaccine in the future. In this context, the co-culture described herein will be one of several models that provide important information, since it in many respects reflects the complexity of cell-mediated immune responses in vivo and allows for elaborate measurements of the molecular mechanisms at work.
Previous work based on the use of co-culture methods have identified mechanisms that closely correlate to the degree of protection observed and in this regard we and others have previously demonstrated the critical roles of, e.g., nitric oxide, IFN-γ, IL-17, GM-CSF, and TNF in the mouse model (Elkins et al., 2011; de Pascalis et al., 2012; de Pascalis et al., 2014; Golovliov et al., 2016). All of these cytokines are in several infectious model involved in the development of Th1 immunity, which is critical for the successful control of an F. tularensis infection. Numerous studies have identified the critical role of IFN-γ for the control of intracellular infection with F. tularensis or F. novicida (Anthony et al., 1991; Fortier et al., 1992; Polsinelli et al., 1994; Lindgren et al., 2005; Santic et al., 2005; Lindgren et al., 2007; Edwards et al., 2010). However, the IFN-γ-dependent effector mechanisms have long been elusive and numerous IFN-γ-inducible factors with potent bactericidal activities have been excluded as essential, e.g., reactive oxygen or nitrogen species, tryptophan degradation, autophagy, and various forms of cell death (Edwards et al., 2010). Our understanding of IFN-γ-dependent effector mechanisms has been greatly advanced with the identification of GBPs and their important role for the control of many intracellular microorganisms (Meunier and Broz, 2016). Not the least F. novicida has been a focus of these studies, since it was demonstrated that GBPs are critically required for activation of AIM2 in conjunction with an F. novicida infection (Man et al., 2015; Meunier et al., 2015). In addition to previous demonstrations of their critical role for the protection against vacuolar pathogens, F. novicida became the first example that GBPs also target cytosolic bacteria (Meunier and Broz, 2016). Notably, subsequently it was demonstrated that the IFN-γ-induced control of F. novicida replication also occurred in macrophages deficient for caspase-1 and caspase-11 combined, or for AIM2, and independent of NADPH oxidase and nitric oxide synthase, all of which have been identified as IFN-γ-inducible effectors known to act downstream of GBPs (Wallet et al., 2017). Thereby, these findings identified GBPs as the most critical effector of IFN-γ-mediated killing of F. novicida. It was observed that the prerequisites for control of BMDM infection with the LVS strain was similar to that of F. novicida, whereas replication of the SCHU S4 strain was not affected by IFN-γ activation (Wallet et al., 2017).
To further understand the protective mechanisms controlling F. tularensis and F. novicida in the murine model, we believed that implementation of the co-culture model would be of relevance and may provide additional information beyond that of an intracellular infection model based on a single cell-type. Moreover, in contrast to previous publications investigating the role of GBPs, our study assessed their role for acquired immune responses. Previously, we and others have demonstrated that the use of splenocytes from vaccinated animals in the model is highly relevant, since their protective capacity as effector cells closely mimics the efficacy of the vaccination regime in both mice and rats (Golovliov et al., 2016; de Pascalis et al., 2018; Lindgren et al., 2020). Thus, the model can be assumed to closely reflect the complex acquired cell-mediated immune response occurring in vivo. In view of the previous findings that the requirements for GBPs to control an Francisella infection was distinct between the LVS strain and F. novicida, on one hand, and the SCHU S4 strain on the other hand (Wallet et al., 2017), warranted the inclusion of all three strains in the study.
Our present results are in agreement with those of previous studies using the mouse co-culture assay demonstrating correlation between levels of Th1 cytokines, such as IL-2, GM-CSF, IFN-γ, TNF, and MIP-1β and the degree of protection (Golovliov et al., 2016; de Pascalis et al., 2018; Lindgren et al., 2020). Moreover, we have established a human co-culture assay model and identified IFN-γ, TNF, and MIP-1β as protective correlates (Eneslätt et al., 2018). Thus, many of the cytokines identified in the various in vitro and in vivo model are overlapping and therefore corroborate their relevance for protection. In view of the ability of each of these cytokines to potentiate the ability of macrophages to control intracellular pathogens, the finding is not surprising. The results also demonstrate that control of even highly virulent strains is achievable in the co-culture model, in agreement with previous data (Mahawar et al., 2013; Griffin et al., 2015; Golovliov et al., 2016; Eneslätt et al., 2018; Lindgren et al., 2020), although the degree of control was less marked with the highly virulent SCHU S4 strain as compared to the LVS or F. novicida strains. Thus, the strain with the highest virulence, SCHU S4, demonstrated the most rapid replication and also was the least affected by the presence of immune cells, likely due to its potent immunomodulatory properties (Melillo et al., 2010; Gillette et al., 2014; Ireland et al., 2018).
A focus of the present study was to understand the role of GBPs in the adaptive anti-Francisella immune defense. The findings unequivocally demonstrate their dominant role for the control of infection, regardless of infectious agent, and in their absence, no significant control was observed. However, in cultures with GBPchr3-deficient BMDM, there was consistently lower numbers of all three bacteria in the presence of immune splenocytes vs. naïve splenocytes. It cannot be excluded that these differences, although rather small, are indicative of low-level protection in cultures with immune splenocytes and GBPchr3-deficient BMDM and that a larger data-set would have corroborated this.
The results in the co-culture mirror to some extent the virulence of the infection agents, in as much as the degree of control of bacterial replication effectuated in the cultures was much more prominent for the low virulent strains F. novicida and LVS than for the high virulent strain SCHU S4. Also, the cytokine profiles of the culture supernatants were very much dependent on the infectious agent and in particular, the profiles of the F. novicida-infected cultures were distinct from the other cultures. Notably, the cytokine profiles of the SCHU S4 and LVS-infected cultures showed much similarities in cultures with immune splenocytes and wild-type or GBPchr3-deficient BMDM, although control of the latter infection was much more marked in cultures with wild-type BMDM. Also, the most marked differences with regard to cytokine profiles were found between cultures with immune vs. naïve splenocytes, at least in cultures infected with SCHU S4 and LVS. This implies that the difference between the two infections mostly is dependent on the relative susceptibility of SCHU S4 and LVS to the GBP-mediated killing, rather than the ability of each bacterium to modulate the immune responses. Whereas the SCHU S4 and LVS-infected cultures demonstrated significantly higher levels of a majority of cytokines in immune splenocyte cultures vs. naïve splenocyte cultures, this was not the case for the F. novicida cultures. For both of the former infections, IFN-γ and GM-CSF were increased in cultures with immune splenocytes, which is of relevance since both have been correlated to protection in other studies on Francisella. Our results further demonstrate that the cytokines secreted in the cultures with immune splenocytes were rather independent of GBPs. This is of interest since it has been demonstrated that GBPs serve a very important role for regulation of multiple inflammasomes (Wallet et al., 2017), whereas they in the complex co-culture do not appear to be critically required for cytokine regulation at the time point investigated. In view of their critical role for control of infection, this implicates that the main function for the GBPs in the co-culture system with immune effector cells is to execute a cell-autonomous bactericidal effect.
Few studies have compared the cytokine patterns during infections with bacteria of variable virulence in the co-culture model (Eneslätt et al., 2018), but there are several studies that consistently have reported that the monocytic cells demonstrate much more pronounced inflammatory responses during infection with F. novicida than with LVS (Gavrilin et al., 2006; Bröms et al., 2011). In addition, the immunomodulatory abilities of the LVS and SCHU S4 strains have been extensively studied (Telepnev et al., 2005; Gavrilin et al., 2006; McCaffrey and Allen, 2006; Weiss et al., 2007; Bröms et al., 2011; Lindgren et al., 2013; Rabadi et al., 2016). Specifically, a number of potent immunosuppressive traits of SCHU S4 have been identified during infection of monocytic cells (Melillo et al., 2010; Gillette et al., 2014; Ireland et al., 2018). In view of this background, we find it unsurprising that there were distinct cytokine patterns observed in response to infection with each of the three bacterial strains.
Linear discriminant modeling was performed to identify individual cytokines that correlated to control of infection. The modeling revealed that the identified cytokines to some extent was dependent on the infectious agent, but some cytokines were consistently identified to predict the type of infection, i.e., MCP-1, Eotaxin, RANTES, GM-CSF, IL-6, and IL-10. Since these cytokines represent a diverse set of functions; chemokines, Th1 cytokines, pro- and anti-inflammatory cytokines, the findings illustrate the complexity of the immune responses elicited in the co-culture model. This data together with data obtained previously using various in vitro and in vivo models will make it possible to obtain a comprehensive understanding of the protective immune responses in various animal species as well as in different types of tissues against F. tularensis.
Data Availability Statement
The raw data supporting the conclusions of this article will be made available by the authors, without undue reservation.
Ethics Statement
The animal study was reviewed and approved by Ethical Committee on Animal Research, Umeå, Sweden and the University of Lyon, France (CEC-CAPP).
Author Contributions
NM, HL, IG, and KE performed all experiments. AM prepared GBP chr3-deficient macrophages. AS, NM, and HL designed the study, analyzed the data, and wrote the manuscript. TH wrote and reviewed the manuscript. MY provided the GBP chr3-deficient mice. All authors contributed to the article and approved the submitted version.
Funding
Grant support was obtained from Region Västerbotten (Spjutspetsmedel, VLL-582571, Centrala ALF medel, VLL-463691). MY was supported by the Research Program on Emerging and Re-emerging Infectious Diseases (JP20fk0108137), Japanese Initiative for Progress of Research on Infectious Diseases for global Epidemic (JP20wm0325010) and Strategic International Collaborative Research Program (JP19jm0210067) from Agency for Medical Research and Development (AMED), Grant-in-Aid for Scientific Research on Innovative Areas (Production, function and structure of neo-self; 19H04809), for Scientific Research, 18KK0226, 18H02642, and 19H00970, from Ministry of Education, Culture, Sports, Science and Technology of Japan.
Conflict of Interest
The authors declare that the research was conducted in the absence of any commercial or financial relationships that could be construed as a potential conflict of interest.
Supplementary Material
The Supplementary Material for this article can be found online at: https://www.frontiersin.org/articles/10.3389/fcimb.2020.594063/full#supplementary-material
References
Anthony L. D., Burke R. D., Nano F. E. (1991). Growth of Francisella spp. in rodent macrophages. Infect. Immun. 59, 3291–3296. doi: 10.1128/IAI.59.9.3291-3296.1991
Bröms J. E., Lavander M., Meyer L., Sjöstedt A. (2011). IglG and IglI of the Francisella pathogenicity island are important virulence determinants of Francisella tularensis LVS. Infect. Immun. 79, 3683–3696. doi: 10.1128/IAI.01344-10
Burke D. S. (1977). Immunization against tularemia: analysis of the effectiveness of live Francisella tularensis vaccine in prevention of laboratory-acquired tularemia. J. Infect. Dis. 135, 55–60. doi: 10.1093/infdis/135.1.55
Collazo C. M., Meierovics A. I., De Pascalis R., Wu T. H., Lyons C. R., Elkins K. L. (2009). T cells from lungs and livers of Francisella tularensis-immune mice control the growth of intracellular bacteria. Infect. Immun. 77, 2010–2021. doi: 10.1128/IAI.01322-08
Conlan J. W., Shen H., Golovliov I., Zingmark C., Oyston P. C., Chen W., et al. (2010). Differential ability of novel attenuated targeted deletion mutants of Francisella tularensis subspecies tularensis strain SCHU S4 to protect mice against aerosol challenge with virulent bacteria: Effects of host background and route of immunization. Vaccine 28, 1824–1831. doi: 10.1016/j.vaccine.2009.12.001
Cowley S., Elkins K. (2011). Immunity to Francisella. Front. Microbiol. 2, 26. doi: 10.3389/fmicb.2011.00026
de Pascalis R., Chou A. Y., Bosio C. M., Huang C. Y., Follmann D. A., Elkins K. L. (2012). Development of functional and molecular correlates of vaccine-induced protection for a model intracellular pathogen, F. tularensis LVS. PLoS Pathog. 8, e1002494. doi: 10.1371/journal.ppat.1002494
de Pascalis R., Chou A. Y., Ryden P., Kennett N. J., Sjöstedt A., Elkins K. L. (2014). Models derived from in vitro analyses of spleen, liver, and lung leukocyte functions predict vaccine efficacy against the Francisella tularensis Live Vaccine Strain (LVS). MBio 5, e00936. doi: 10.1128/mBio.00936-13
de Pascalis R., Hahn A., Brook H. M., Ryden P., Donart N., Mittereder L., et al. (2018). A panel of correlates predicts vaccine-induced protection of rats against respiratory challenge with virulent Francisella tularensis. PLoS One 13, e0198140. doi: 10.1371/journal.pone.0198140
Edwards J. A., Rockx-Brouwer D., Nair V., Celli J. (2010). Restricted cytosolic growth of Francisella tularensis subsp. tularensis by IFN-gamma activation of macrophages. Microbiology 156, 327–339. doi: 10.1099/mic.0.031716-0
Elkins K. L., Cowley S. C., Conlan J. W. (2011). Measurement of macrophage-mediated killing of intracellular bacteria, including Francisella and mycobacteria. Curr. Protoc. Immunol. 14, 1–14. Unit14 25. doi: 10.1002/0471142735.im1425s93
Eneslätt K., Normark M., Björk R., Rietz C., Zingmark C., Wolfraim L. A., et al. (2012). Signatures of T cells as correlates of immunity to Francisella tularensis. PLoS One 7, e32367. doi: 10.1371/journal.pone.0032367
Eneslätt K., Golovliov I., Ryden P., Sjöstedt A. (2018). Vaccine-Mediated Mechanisms Controlling Replication of Francisella tularensis in Human Peripheral Blood Mononuclear Cells Using a Co-culture System. Front. Cell Infect. Microbiol. 8, 27. doi: 10.3389/fcimb.2018.00027
Fernandes-Alnemri T., Yu J. W., Juliana C., Solorzano L., Kang S., Wu J., et al. (2010). The AIM2 inflammasome is critical for innate immunity to Francisella tularensis. Nat. Immunol. 11, 385–393. doi: 10.1038/ni.1859
Fortier A. H., Polsinelli T., Green S. J., Nacy C. A. (1992). Activation of macrophages for destruction of Francisella tularensis: identification of cytokines, effector cells, and effector molecules. Infect. Immun. 60, 817–825. doi: 10.1128/IAI.60.3.817-825.1992
Gavrilin M. A., Bouakl I. J., Knatz N. L., Duncan M. D., Hall M. W., Gunn J. S., et al. (2006). Internalization and phagosome escape required for Francisella to induce human monocyte IL-1beta processing and release. Proc. Natl. Acad. Sci. U. S. A. 103, 141–146. doi: 10.1073/pnas.0504271103
Gillette D. D., Curry H. M., Cremer T., Ravneberg D., Fatehchand K., Shah P. A., et al. (2014). Virulent Type A Francisella tularensis actively suppresses cytokine responses in human monocytes. Front. Cell Infect. Microbiol. 4, 45. doi: 10.3389/fcimb.2014.00045
Golovliov I., Twine S. M., Shen H., Sjöstedt A., Conlan W. (2013). A DeltaclpB mutant of Francisella tularensis subspecies holarctica strain, FSC200, is a more effective live vaccine than F. tularensis LVS in a mouse respiratory challenge model of tularemia. PLoS One 8, e78671. doi: 10.1371/journal.pone.0078671
Golovliov I., Lindgren H., Eneslätt K., Conlan W., Mosnier A., Henry T., et al. (2016). An in vitro co-culture mouse model demonstrates efficient vaccine-mediated control of Francisella tularensis SCHU S4 and identifies nitric oxide as a predictor of efficacy. Front. Cell Infect. Microbiol. 6, 152. doi: 10.3389/fcimb.2016.00152
Griffin A. J., Crane D. D., Wehrly T. D., Bosio C. M. (2015). Successful protection against tularemia in C57BL/6 mice is correlated with expansion of Francisella tularensis-specific effector T cells. Clin. Vaccine Immunol. 22, 119–128. doi: 10.1128/CVI.00648-14
Ireland R., Schwarz B., Nardone G., Wehrly T. D., Broeckling C. D., Chiramel A. I., et al. (2018). Unique Francisella phosphatidylethanolamine acts as a potent anti-inflammatory lipid. J. Innate Immun. 10, 291–305. doi: 10.1159/000489504
Jones J. W., Kayagaki N., Broz P., Henry T., Newton K., O’Rourke K., et al. (2010). Absent in melanoma 2 is required for innate immune recognition of Francisella tularensis. Proc. Natl. Acad. Sci. U. S. A. 107, 9771–9776. doi: 10.1073/pnas.1003738107
Kurtz S. L., Foreman O., Bosio C. M., Anver M. R., Elkins K. L. (2013). Interleukin-6 is essential for primary resistance to Francisella tularensis live vaccine strain infection. Infect. Immun. 81, 585–597. doi: 10.1128/IAI.01249-12
Kutsch M., Sistemich L., Lesser C. F., Goldberg M. B., Herrmann C., Coers J. (2020). Direct binding of polymeric GBP1 to LPS disrupts bacterial cell envelope functions. EMBO J. 39, e104926. doi: 10.15252/embj.2020104926
Lindgren H., Stenman L., Tärnvik A., Sjöstedt A. (2005). The contribution of reactive nitrogen and oxygen species to the killing of Francisella tularensis LVS by murine macrophages. Microbes Infect. 7, 467–475. doi: 10.1016/j.micinf.2004.11.020
Lindgren H., Shen H., Zingmark C., Golovliov I., Conlan W., Sjöstedt A. (2007). Resistance of Francisella tularensis strains against reactive nitrogen and oxygen species with special reference to the role of KatG. Infect. Immun. 75, 1303–1309. doi: 10.1128/IAI.01717-06
Lindgren M., Eneslatt K., Bröms J. E., Sjöstedt A. (2013). Importance of PdpC, IglC, IglI, and IglG for modulation of a host cell death pathway induced by Francisella tularensis. Infect. Immun. 81, 2076–2084. doi: 10.1128/IAI.00275-13
Lindgren H., Eneslätt K., Golovliov I., Gelhaus C., Ryden P., Wu T., et al. (2020). Vaccine-mediated mechanisms controlling Francisella tularensis SCHU S4 growth in a rat co-culture system. Pathogens 9, 338–355. doi: 10.3390/pathogens9050338
Lyons R. C., Wu T. H. (2007). Animal models of Francisella tularensis infection. Ann. N Y Acad. Sci. 1105, 238–265. doi: 10.1196/annals.1409.003
Mahawar M., Rabadi S. M., Banik S., Catlett S. V., Metzger D. W., Malik M., et al. (2013). Identification of a live attenuated vaccine candidate for tularemia prophylaxis. PLoS One 8, e61539. doi: 10.1371/journal.pone.0061539
Man S. M., Karki R., Malireddi R. K., Neale G., Vogel P., Yamamoto M., et al. (2015). The transcription factor IRF1 and guanylate-binding proteins target activation of the AIM2 inflammasome by Francisella infection. Nat. Immunol. 16, 467–475. doi: 10.1038/ni.3118
Man S. M., Place D. E., Kuriakose T., Kanneganti T. D. (2017). Interferon-inducible guanylate-binding proteins at the interface of cell-autonomous immunity and inflammasome activation. J. Leukoc. Biol. 101, 143–150. doi: 10.1189/jlb.4MR0516-223R
McCaffrey R. L., Allen L. A. (2006). Francisella tularensis LVS evades killing by human neutrophils via inhibition of the respiratory burst and phagosome escape. J. Leukoc. Biol. 80, 1224–1230. doi: 10.1189/jlb.0406287
Melillo A. A., Bakshi C. S., Melendez J. A. (2010). Francisella tularensis antioxidants harness reactive oxygen species to restrict macrophage signaling and cytokine production. J. Biol. Chem. 285, 27553–27560. doi: 10.1074/jbc.M110.144394
Melillo A. A., Foreman O., Elkins K. L. (2013). IL-12Rbeta2 is critical for survival of primary Francisella tularensis LVS infection. J. Leukoc. Biol. 93, 657–667. doi: 10.1189/jlb.1012485
Melillo A. A., Foreman O., Bosio C. M., Elkins K. L. (2014). T-bet regulates immunity to Francisella tularensis live vaccine strain infection, particularly in lungs. Infect. Immun. 82, 1477–1490. doi: 10.1128/IAI.01545-13
Meunier E., Broz P. (2016). Interferon-inducible GTPases in cell autonomous and innate immunity. Cell Microbiol. 18, 168–180. doi: 10.1111/cmi.12546
Meunier E., Wallet P., Dreier R. F., Costanzo S., Anton L., Ruhl S., et al. (2015). Guanylate-binding proteins promote activation of the AIM2 inflammasome during infection with Francisella novicida. Nat. Immunol. 16, 476–484. doi: 10.1038/ni.3119
Polsinelli T., Meltzer M. S., Fortier A. H. (1994). Nitric oxide-independent killing of Francisella tularensis by IFN-gamma-stimulated murine alveolar macrophages. J. Immunol. 153, 1238–1245.
Rabadi S. M., Sanchez B. C., Varanat M., Ma Z., Catlett S. V., Melendez J. A., et al. (2016). Antioxidant defenses of Francisella tularensis modulate macrophage function and production of proinflammatory cytokines. J. Biol. Chem. 291, 5009–5021. doi: 10.1074/jbc.M115.681478
Santic M., Molmeret M., Abu Kwaik Y. (2005). Modulation of biogenesis of the Francisella tularensis subsp. novicida-containing phagosome in quiescent human macrophages and its maturation into a phagolysosome upon activation by IFN-gamma. Cell Microbiol. 7, 957–967. doi: 10.1111/j.1462-5822.2005.00529.x
Sjöstedt A. (2007). Tularemia: history, epidemiology, pathogen physiology, and clinical manifestations. Ann. N Y Acad. Sci. 1105, 1–29. doi: 10.1196/annals.1409.009
Snoy P. J. (2010). Establishing efficacy of human products using animals: the US food and drug administration’s “animal rule”. Vet. Pathol. 47, 774–778. doi: 10.1177/0300985810372506
Telepnev M., Golovliov I., Sjöstedt A. (2005). Francisella tularensis LVS initially activates but subsequently down-regulates intracellular signaling and cytokine secretion in mouse monocytic and human peripheral blood mononuclear cells. Microb. Pathog. 38, 239–247. doi: 10.1016/j.micpath.2005.02.003
Tretina K., Park E. S., Maminska A., MacMicking J. D. (2019). Interferon-induced guanylate-binding proteins: Guardians of host defense in health and disease. J. Exp. Med. 216, 482–500. doi: 10.1084/jem.20182031
Wallet P., Benaoudia S., Mosnier A., Lagrange B., Martin A., Lindgren H., et al. (2017). A. Sjöstedt, and T. Henry, IFN-gamma extends the immune functions of Guanylate Binding Proteins to inflammasome-independent antibacterial activities during Francisella novicida infection. PLoS Pathog. 13, e1006630. doi: 10.1371/journal.ppat.1006630
Weiss D. S., Henry T., Monack D. M. (2007). Francisella tularensis: activation of the inflammasome. Ann. N Y Acad. Sci. 1105, 219–237. doi: 10.1196/annals.1409.005
Keywords: Francisella tularensis, guanylate-binding proteins, mouse co-culture model, cytokine patterns, correlates of protection
Citation: Mohammadi N, Lindgren H, Golovliov I, Eneslätt K, Yamamoto M, Martin A, Henry T and Sjöstedt A (2020) Guanylate-Binding Proteins Are Critical for Effective Control of Francisella tularensis Strains in a Mouse Co-Culture System of Adaptive Immunity. Front. Cell. Infect. Microbiol. 10:594063. doi: 10.3389/fcimb.2020.594063
Received: 12 August 2020; Accepted: 06 November 2020;
Published: 10 December 2020.
Edited by:
Thomas Rudel, Julius Maximilian University of Würzburg, GermanyReviewed by:
George Liechti, Uniformed Services University of the Health Sciences, United StatesSarah Maddocks, Cardiff Metropolitan University, United Kingdom
Copyright © 2020 Mohammadi, Lindgren, Golovliov, Eneslätt, Yamamoto, Martin, Henry and Sjöstedt. This is an open-access article distributed under the terms of the Creative Commons Attribution License (CC BY). The use, distribution or reproduction in other forums is permitted, provided the original author(s) and the copyright owner(s) are credited and that the original publication in this journal is cited, in accordance with accepted academic practice. No use, distribution or reproduction is permitted which does not comply with these terms.
*Correspondence: Anders Sjöstedt, YW5kZXJzLnNqb3N0ZWR0QHVtdS5zZQ==
†These authors have contributed equally to this work