- 1Departamento de Ciências Biológicas, Universidade Estadual de Santa Cruz—UESC, Ilhéus, Brazil
- 2Laboratório de Produtos Naturais, Universidade Estadual Do Sudoeste da Bahia—UESB, Jequié, Brazil
- 3Laboratório de Inovações em Terapias, Ensino e Bioprodutos, Instituto Oswaldo Cruz, Fundação Oswaldo Cruz-FIOCRUZ, Rio de Janeiro, Brazil
- 4Laboratório de Biologia Celular e Parasitos Intracelulares, Universidade Federal de Minas Gerais—UFMG, Belo Horizonte, Brazil
The Trichoderma genus comprises several species of fungi whose diversity of secondary metabolites represents a source of potential molecules with medical application. Because of increased pathogen resistance and demand for lower production costs, the search for new pharmacologically active molecules effective against pathogens has become more intense. This is particularly evident in the case of American cutaneous leishmaniasis due to the high toxicity of current treatments, parenteral administration, and increasing rate of refractory cases. We have previously shown that a fungus from genus Trichoderma can be used for treating cerebral malaria in mouse models and inhibit biofilm formation. Here, we evaluated the effect of the ethanolic extract of Trichoderma asperelloides (Ext-Ta) and its fractions on promastigotes and amastigotes of Leishmania amazonensis, a major causative agent of cutaneous leishmaniasis in the New World. Ext-Ta displayed leishmanicidal action on L. amazonensis parasites, and its pharmacological activity was associated with the low-molecular-weight fraction (LMWF) of Ext-Ta. Ultrastructural analysis demonstrated morphological alterations in the mitochondria and the flagellar pocket of promastigotes, with increased lipid body and acidocalcisome formation, microtubule disorganization of the cytoplasm, and intense vacuolization of the cytoplasm when amastigotes were present. We suggest the antiparasitic activity of Trichoderma fungi as a promising tool for developing chemotherapeutic leishmanicidal agents.
Introduction
Fungi of the genus Trichoderma are endophytes, well-known as pathogens for other fungi (Atanasova et al., 2013). Because of their high reproductive rate, their ability to secrete antibiotics and biomass-degrading enzymes as well as to colonize crop species, they play an important role in agriculture and biotechnology (Schuster and Schmoll, 2010).
The Trichoderma metabolites with antibiotic activity are distinguished by molecular weight and polarity: (a) high molecular weight and polar molecules, such as peptaibols, have direct activity on their antagonist membrane; (b) low-molecular-weight molecules and volatile compounds, including polyketides, such as butenolides and pyrones, have important pharmacological activity (Cardoza et al., 2005).
Reports on the antiprotozoal activity of Trichoderma fungi are scarce. It was demonstrated that hirsutellone, an alkaloid dimer obtained from Trichoderma sp. and trichothecenes from soil-derived Trichoderma brevicompactum exhibited interesting antimalarial activity upon Plasmodium falciparum (Isaka et al., 2006; Klaiklay et al., 2019). Interestingly, (Podder and Ghosh, 2019) showed a possible indirect control of malaria when observing the efficacy of the fungus Trichoderma asperellum against Anopheles spp. larvae, demonstrating a novel application of fungal constituents as anopheline malaria vector control agents. Iwatsuki et al. (2010) reported that trichosporin B-VIIa and trichosporin B-VIIb peptaibiotics, produced by Trichoderma polysporum, exerted antitrypanosomal activity against Trypanosoma brucei, suggesting that this compound interacts in the protozoan membrane. However, there are no reports of the activity of Trichoderma metabolites against other neglected diseases such as leishmaniasis, which comprises a serious public health problem.
There are few therapeutic alternatives for neglected tropical diseases largely due to the pharmaceutical industry's lack of interest in developing new drugs. Thus, new chemotherapeutic agents are urgently required (Rajasekaran and Chen, 2015). Natural products have been an important focus in research for new drugs, mainly aiming for more efficient treatments for infectious diseases caused by parasitic protozoa such as leishmaniasis (Tenguria et al., 2011).
Around 700,000 to 1 million new leishmaniasis cases occur annually in 98 countries, and 350 million estimated people are under infection risk in endemic areas (World Health Organization, 2020). Infection begins with the blood meal of Phlebotomus (Old World) and Lutzomyia (New World) sand flies. The parasites have a digenetic life cycle, requiring the phlebotomine vectors that harbor the promastigote, flagellar form in its gut and the vertebrate mammalian host in which the non-motile amastigote form (Vannier-Santos et al., 2002). The promastigote forms infect macrophages, and monocytes differentiate into amastigotes that proliferate intracellularly and endure the host immune response causing the disease. These disease clinical manifestations range from asymptomatic lesions to death, depending on the species and the host's immunity (Scorza et al., 2017).
Currently, pentavalent antimonials, paromomycin, pentamidine, amphotericin B, and miltefosine are some of the medications used in the treatment of leishmaniasis (Akbari et al., 2017; Burza et al., 2018). However, these drugs are administered for a prolonged period and have high toxicity, which normally lead to interruptions in the treatment, thus contributing to parasite resistance and the parasite favoring pathogenicity (Maltezou, 2010; Olliaro, 2010; Matrangolo et al., 2013). These drugs may inhibit both glycolysis and β-oxidation of fatty acids, alter membrane permeability, and inhibit protein synthesis (Rajasekaran and Chen, 2015). In the present work, we have shown the effects of the treatment with the ethanolic extract of Trichoderma asperelloides on Leishmania amazonensis parasites, which makes this fungus an innovative alternative to the development of new leishmanicidal drugs.
Materials and Methods
Trichoderma asperelloides Cultures and Preparation of the Ethanolic Extract
The T. asperelloides strain LIBASP02 was cultivated in potato dextrose agar (PDA) in Petri plates at room temperature for 10 days. The crude ethanolic extract from mycelium and spores was obtained from washing cultures with 95% ethanol (5 ml/plate) (Fukuzawa et al., 2008). The ethanolic solution was incubated under gentle shaking on a TE143® shaker table for 24 h, after which it was placed in SPEEDVARS AG 22331 for drying (Hamburg Concentrator 5301®). The final crude ethanolic extract (Ext-Ta) was solubilized in RPMI 1640 medium (Sigma).
The Ext-Ta was centrifuged at 4°C for 20 min, 2,370 × g, and the supernatant was centrifuged using a Centricon membrane (10-kDa pore) at 4°C for 15 min, 2,370 × g until reduction to 1 ml to obtain a high-molecular-weight fraction (HMWF). The eluate was separately collected to obtain a low-molecular-weight fraction below (LMWF) 10 kDa, and then the volume of the two fractions was adjusted to perform the bioassay.
Parasites
Leishmania amazonensis (IFLA/BR/1967/PH8) were cultivated at 25°C and maintained in RPMI 1640 medium (Sigma) supplemented with 10% fetal bovine serum (FBS, Invitrogen-Gibco®) and 1 mg/ml of penicillin/streptomycin (Schering-Plow, Rio de Janeiro, Brazil). The promastigote forms of the protozoan were used in the stationary phase of growth.
Macrophages
The macrophages J774 were cultured in RPMI 1640 medium (Invitrogen-Gibco®) supplemented with 10% of FBS and 1 mg/ml penicillin/streptomycin (Schering-Plow, Rio de Janeiro, Brazil), at 37°C with 5% of CO2 saturation. After cell confluence and monolayer formation, the cells were removed from the bottles using trypsin (Gibco®) and counted on a Neubauer hemocytometer chamber for the biological assays.
Treatment With Ext-Ta, HMWF, and LMWF in L. amazonensis Promastigotes and Macrophages
The MTT cell viability assay was performed to investigate the cytotoxic effects of Ext-Ta, HMWF, LMWF, and pentamidine on L. amazonensis promastigotes and HMWF and LMWF on J774 macrophages. Parasites of 5 × 105 per well in 96-well tissue plates were treated with different concentrations (0.31–4.95 ng/μl) of Ext-Ta and pentamidine (positive control) or HMWF and LMWF fractions (2.5–40 ng/μl) for 24 and 48 h. The non-infected J774 macrophages (1 × 105) were seeded in 96-well tissue plates and treated with different fraction concentrations ranging from 2.5 to 40 ng/μl for 24 h. After that time, the samples were incubated with 5 mg/ml MTT for 4 h under the same culture conditions as described (Mosmann, 1983). After the reaction, DMSO (Sigma-Aldrich) was added to dissolve the salt crystals formed by mitochondrial metabolism, and the absorbance was determined at 570 nm in an ELISA reader (Molecular Devices, Sunnyvale, California). The obtained values were used to determine IC50.
Phagocytosis Assays
The J774 macrophages (5 × 105 cells per well) were cultivated in 24-well plates containing 13-mm round coverslips. The cells were incubated with 200 μl of RPMI 1640 medium supplemented with 10% FBS for 2 h, for adherence. The macrophage monolayer was washed with sterile phosphate-buffered saline (PBS) to remove non-adherent cells and was infected with promastigote forms of L. amazonensis (10:1) for 4 h at 37°C and 5% CO2. After the interaction, the plate was washed using RPMI medium to remove free parasites. The infected macrophages were treated with 2.5 ng/μl of the LMWF and incubated for 24 h. The cells were stained with hematoxylin–eosin in order to establish the percentage of infected macrophages by direct counting of 100 cells per slide under a light microscope, according to the following formula:
Transmission Electron Microscopy (TEM)
Promastigotes of L. amazonensis (5 × 105 parasites per well) were treated for 24 h with different concentrations of LMWF ranging from 2.5 to 20 ng/μl. Macrophages (1 × 106 cells per well) were infected with L. amazonensis (1:10 ratio) and treated for 24 h with 2.5 ng/μl LMWF. After washing with sterile PBS, the wells were fixed with 2.5% glutaraldehyde (SIGMA) in 0.1 mol sodium cacodylate buffer, at pH 7.2 for 1 h and postfixed in 1% osmium tetroxide and 0.8% potassium ferrocyanide (SIGMA). The samples were washed and dehydrated in an acetone series and embedded in a Polybed resin, which was polymerized at 60°C for 72 h (Vannier-Santos and Lins, 2001). Ultrathin sections were contrasted with 7% uranyl acetate and 4% lead nitrate aqueous solutions and observed on an FEI Morgagni 268 TEM.
Statistical Analyses
The obtained data were expressed as mean ± standard deviation and analyzed in GraphPad Prism software version 5.0. The tests used included one-way ANOVA followed by Tukey posttest and the Student t test. IC50 was assayed by non-linear regression analysis. Values of p < 0.05 were considered significant. All experiments were performed in at least three independent assays in triplicate.
Results
Trichoderma asperelloides Ethanolic Extract and Its Fractions Inhibit the Proliferation of L. amazonensis Promastigotes
The antileishmanial action of Ext-Ta and its HMWF and LMWF fractions in L. amazonensis promastigotes was dose dependent (p < 0.05). In the treatment with Ext-Ta, for 24 h, the parasite viability was reduced by 30–90% at concentrations ranging 0.31–4.95 ng/μl (Figure 1A), similarly to that in treatment for 48 h (Supplementary Figure 1A). The IC50 values of Ext-Ta were 1.09 and 1.53 ng/μl for 24 and 48 h, respectively. The viability of pentamidine-treated promastigotes was reduced by about 50%, in all concentrations tested, presenting the calculated IC50 value of 0.77 ng/μl (Figure 1A). The HMWF showed a significant reduction (p < 0.05) in the viability of the parasites only at the 40 ng/μl concentration as compared to the control (Figure 1B). LMWF produced a reduction of the parasite viability at the concentration of 10 ng/μl, which was significant (p < 0.05) in comparison to the control samples (Figure 1B), similarly to that of treatment for 48 h (Supplementary Figure 1B). The IC50 values observed for HMWF were 22.89 and 20.76 ng/μl, for 24 and 48 h, respectively, and those for LMWF were 18.16 and 12.85 ng/μl for 24 and 48 h, respectively.
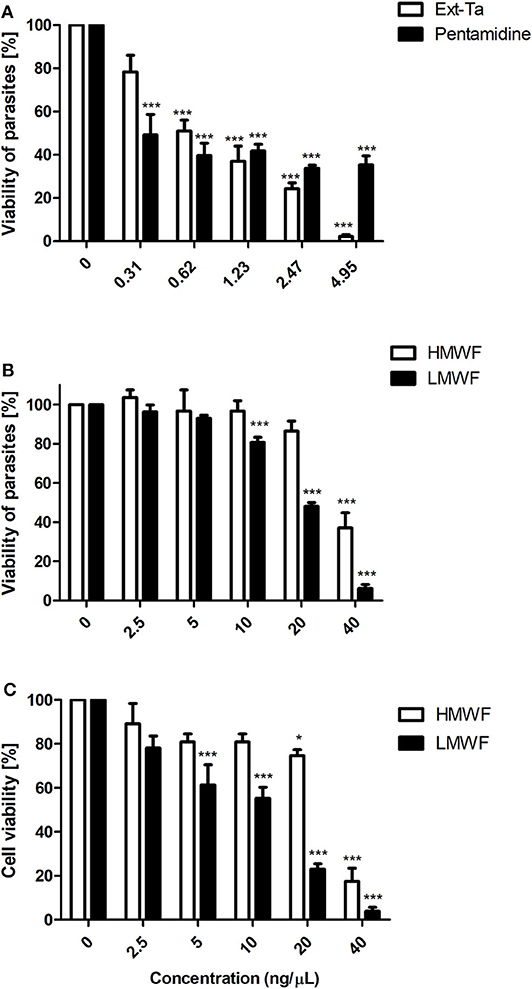
Figure 1. Ext-Ta treatment decreased L. amazonensis promastigote viability. (A) Promastigotes of L. amazonensis were treated with crescent concentrations of Ext-Ta and pentamidine and (B) different concentrations of HMWF and LMWF for 24 h. (C) J774 macrophages were treated with crescent concentrations of HMWF and LMWF for 24 h. The cell viability was performed by MTT assay. A value of p < 0.05 was considered for statistical significance. One-way ANOVA followed by Tukey post-test was performed to establish the statistical significance between the treatments in relation to the control. Ext-Ta: ethanolic extract of T. asperelloides; HMWF: high-molecular-weight fraction; LMWF: low-molecular-weight fraction; 0: control. *p < 0.05; ***p < 0.001.
The higher concentrations of HMWF and LMWF provoked around 80% and 90% of inviability in macrophages, respectively. LMWF showed a significant dose-dependent effect from 5 ng/μl in macrophages. The apparent IC50 value of HMWF was 23.28 ng/μl, and that of LMWF was 12.57 ng/μl (Figure 1C). LMWF was not toxic to macrophages or to L. amazonensis promastigotes at the concentration of 2.5 ng/μl. However, in the light microscopy analysis, it was observed that this concentration decreased promastigote motility (data not shown). This observation encouraged us to investigate whether LMWF interferes in the ultrastructure and proliferation of both evolutive forms of L. amazonensis.
LMWF Alters the Ultrastructure of L. amazonensis Promastigotes and Intracellular Amastigotes
We observed that promastigotes treated with increasing LMWF concentrations exhibited significant ultrastructural changes as compared to the control (Figure 2A). An increase in contractile vacuole was observed, as well as a dilatation of the flagellar pocket presenting luminal exosomes displaying cytoplasmic contents (Figure 2B). Some LMWF-treated parasites presented nuclear disruption or karyorrhexis (Figures 2C,D), with dense peripheral chromatin (C) and nuclear pores (D) in direct contact with the cytoplasm. Large autophagosome (Figure 2D) and enhanced formation of acidocalcisomes and lipid bodies were also observed (Figure 2E) along with mitochondrial destruction (Figure 2F).
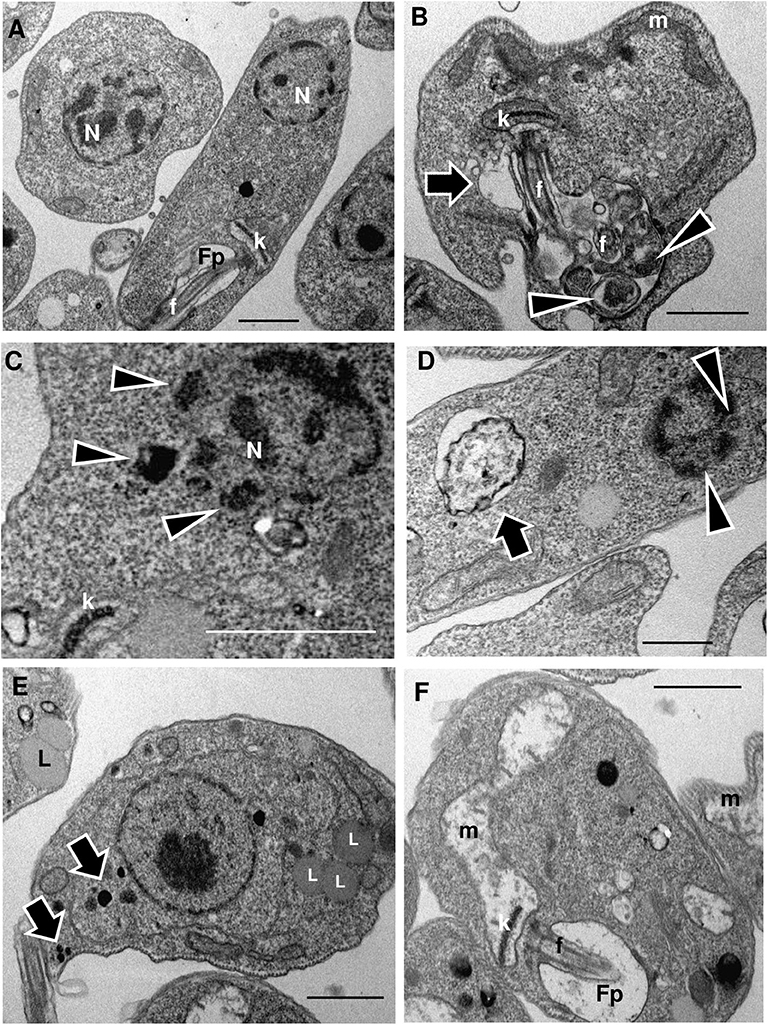
Figure 2. LMWF of Ext-Ta induces ultrastructural changes on L. amazonensis promastigotes. Promastigotes were treated with different concentrations of LMWF of Ext-Ta for 24 h, and the ultrastructure was analyzed by transmission electron microscopy. (A) Untreated control protozoan displaying the normal organization of the parasite cells; (B) promastigotes treated with 2.5 ng/μl, (C) 5 ng/μl, (D) 10 ng/μl, and (E,F) 20 ng/μl of LMWF. (B) Flagellar pocket presenting exosomes containing cytoplasmic material (arrowheads) enlarged contractile vacuole (arrow); (C) karyorrhexis (nuclear disruptor) with dense peripheral chromatin in direct contact with the cytoplasm (arrowheads); (D) parasite showing large autophagosome (arrow) and karyorrhectic nucleus displaying nuclear pores in direct contact with the cytoplasm (arrowheads); (E) Ext-Ta-treated parasites usually displayed numerous lipid bodies (L) and acidocalcisomes (arrows); (F) parasites presenting destroyed mitochondria (m). Fp, flagellar pocket; k, kinetoplast; L, lipid bodies; m, mitochondria; n, nucleus.
The phagocytic index obtained by counting infected cells and the number of parasites inside the macrophages was significantly different between untreated J774 cells (1.61) and cells treated with 2.5 ng/μl of LMWF (1.38) (Figure 3A). To investigate if LMWF also affects the ultrastructure of L. amazonensis amastigotes, infected macrophages were treated with 2.5 ng/μl of LMWF and compared to amastigotes grown on untreated macrophages (Figure 3B). LMWF caused the biogenesis of numerous lipid bodies (Figure 3C), which coalesced in a cumulative manner, eventually reaching the parasite cell surface (Figure 3D) and dead intracellular parasites apparently by necrosis (Figure 3D).
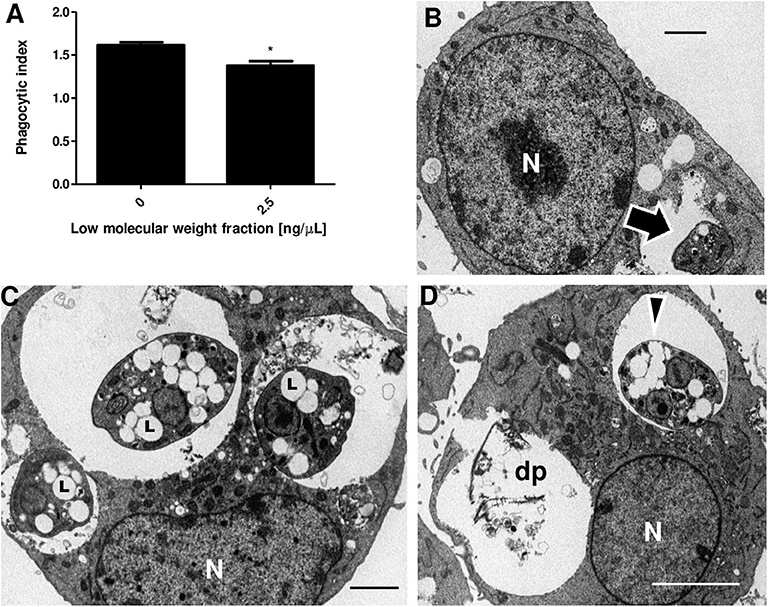
Figure 3. LMWF of Ext-Ta reduces parasite survival and causes ultrastructural changes on L. amazonensis amastigotes. (A) Phagocytic index of macrophages infected with L. amazonensis promastigotes and treated or not with 2.5 ng/μl of LMWF for 24 h. 0: control (untreated parasites). A value of p < 0.05 was considered for statistical significance; *p < 0.05 by comparison with Student t test. (B) Untreated culture of macrophages infected with L. amazonensis promastigotes (10:1 ratio) presenting intact amastigote inside a parasitophorous vacuole (arrow). (C,D) Macrophages infected and treated with 2.5 ng/μl of LMWF for 24 h showed parasites with numerous lipid bodies (C, L) which eventually coalesced, reaching the parasite cell surface (D, arrowhead). (D) The necrosis of the parasites culminated in the complete destruction of parasites (dp). N, macrophage nucleus.
Discussion
The bioactive compounds of endophytic fungi such as Trichoderma sp. have a potential industrial application with antimicrobial, antiviral, antioxidant, and immunomodulatory activities. These compounds include alkaloids, glycosides, flavonoids, phenolic acid, quinones, xanthones, terpenoids, and steroids, among others (Shukla et al., 2014). The discovery of new compounds and strategies against pathogenic microorganisms can be a hope to treat a range of diseases, especially neglected diseases in undeveloped countries.
We have previously shown that an in vivo treatment of cerebral malaria with the ethanolic extract of Trichoderma stromaticum has promising effects, including reduction of parasitemia and inflammation, increased survival, and prevention of neurological signs of cerebral malaria in C57BL/6 mice (Cariaco et al., 2018). In a similar way, the use of crude ethanolic extracts of T. asperelloides and its fraction can efficiently inhibit Staphylococcus growth and biofilm formation, representing a candidate for the development of new antibiotics against gram-positive bacteria (Santos et al., 2018).
The data obtained in this study have shown for the first time that the T. asperelloides fungus has a leishmanicidal action against L. amazonensis. We observed that the ultrastructure of the promastigotes and amastigotes was affected by the treatment with LMWF and that both the Ext-Ta and LMWF obtained from the extract have potent toxic activity against promastigote forms of L amazonensis.
The treatment for leishmaniasis is currently toxic, limited, and long. New perspectives for treatment need to be made. The IC50 of the ethanolic extract of T. asperelloides for promastigotes was shown to be 6-fold lower than that observed in studies using the endophytic fungus Cochliobolus sp., the plant Vernonia polyanthes, and secondary metabolites of the fungus Eurotium repens (Campos et al., 2008; Gao et al., 2012; do Nascimento et al., 2015), demonstrating that products of this fungus have technological potential for developing novel leishmanicidal agents.
TEM has been frequently used to elucidate ultrastructural changes in protozoa submitted to compounds with leishmanicidal action (Rodrigues and de Souza, 2008; Vannier-Santos and De Castro, 2009). Here, we observed important changes in the parasite organization which may shed light on the mechanisms of action of the Ext-Ta fraction.
The triggering of karyorrhexis or nuclear disruption is associated with both apoptosis and necrosis cell death pathways (Cheville, 2009). Karyorrhexis was reported in late apoptosis of human carcinoma cells treated with nanoparticles presenting fungal filtrates of Pestalotiopsis microspora (Netala et al., 2016). Karyorrhexis was also associated with necrosis induced by acetaminophen (Jacob et al., 2007).
Exosomes with cytoplasmic core within the flagellar pocket and autophagosome formation were previously reported in L. amazonensis promastigotes treated with 22,26-azasterol (Rodrigues et al., 2002), and multivesicular bodies were also detected in this site following starvation-induced autophagy in Leishmania major (Basmaciyan et al., 2018).
Interestingly, the formation of acidocalcisomes and lipid bodies was also associated with ergosterol biosynthesis inhibitor-induced autophagy (Vannier-Santos et al., 1995, 1999; Rodrigues et al., 2002). Lipid body accumulation is also related to autophagy (Singh et al., 2009). Furthermore, lipid accumulation plays different roles in the non-apoptotic regulated cell death pathways of necroptosis and ferroptosis (Magtanong et al., 2016).
Nowadays, acidocalcisomes are seen as lysosome-related organelles, required for autophagy in T. brucei (Li and He, 2014) and proposed to act cooperatively with contractile vacuoles in protozoan parasite osmoregulation (Docampo et al., 2010). In this regard, trypanocidal compounds such as squalene synthase inhibitors may also cause contractile vacuole enlargement (Braga et al., 2004). It remains to be determined whether the osmoregulatory mechanism could be involved in cell death mechanism triggered by fungal derivatives, but calcium homeostasis is involved in the mode of action of antiparasitic agents on Leishmania donovani (Pinto-Martinez et al., 2018) and Trypanosoma cruzi (Benaim et al., 2020). Lipid body formation may comprise an escape mechanism as this compartment is an intracellular site of synthesis of prostaglandins, such as PGF2a, inhibiting different facets of the immune response (Vallochi et al., 2018).
The mitochondrial swelling and total extraction of the matrix content were observed in the L. amazonensis promastigotes treated with the LMWF. Based on ultrastructural observations, we found that a higher concentration of the compound affected the mitochondrial ultrastructure through extensive swelling and severe damage to internal membranes (Fonseca-Silva et al., 2015), similar to what has been observed for L. amazonensis promastigotes treated with different concentrations of LMWF.
The most frequent effect of chemotherapeutic agents on trypanosomatid mitochondria is mitochondrial dilation, indicative of a dysfunction in membrane potential. Studies indicate that this organelle undergoes a change in its lipid organization and ultrastructure, in trypanosomatids like T. cruzi and Leishmania spp., when the parasites are incubated with sterol biosynthesis inhibitors, considering that ergosterol is an important structural element present in mitochondrial membranes (Adade and Souto-Padrón, 2010; Medina et al., 2012), possibly modulating the organelle membrane stability and fusogenicity (Vannier-Santos et al., 1995). It was demonstrated that alterations or damage to the mitochondria of L. amazonensis may be involved in the death of the parasite by apoptosis due to changes in the mitochondrial membrane potential, associated with an increase in mitochondrial reactive oxygen species (ROS) production and decreased detoxification by the parasite (Garcia et al., 2013).
The crude extract of T. asperelloides was shown to be toxic to L. amazonensis. The impairment in parasite survival appears to be associated with the ultrastructural changes caused by the LMWF in both developmental forms. The amastigote forms of L. amazonensis appear to be more sensitive than promastigote ones due to the lower concentration of the LMWF and significant ultrastructural changes including nuclear disruption, leading to parasite cell death.
Due to the toxic and often inefficient therapy of leishmaniasis, the discovery of new effective, safe, and low-cost bioactive compounds is urgently required. Thus, Trichoderma spp. may comprise a promising fungus with the potential to develop novel chemotherapy tools for this disease. Preliminary data from our group demonstrated the presence of alkaloids as one of the major components of the LMWF of T. asperelloides (Supplementary Figure 2).
According to Scotti et al. (2016), alkaloids are secondary metabolites with several useful pharmacological properties for the treatment of malaria and bacterial infections, in addition to having an excellent antileishmanial activity, with effects on the biology of the parasite (Mishra et al., 2009). Previous studies report that natural products such as alkaloids have an inhibitory activity against the enzyme arginase of Leishmania sp. (Manjolin et al., 2013; Lacerda et al., 2018). This enzyme has its importance both in the polyamine biosynthesis, essential for the biology of the parasite (Vannier-Santos and Suarez-Fontes, 2017), and in the evasion of the host's immune response against the parasite (D'Antonio et al., 2013). In this regard, inhibition of polyamine metabolism can cause mitochondrial destruction and formation of flagellar pocket exosomes in L. amazonensis (Vannier-Santos et al., 2008). Thus, this polycation metabolism may comprise a promising drug target for the development of leishmanicidal compounds (Cruz et al., 2013; Maquiaveli et al., 2016; Roberts and Ullman, 2017).
Regardless of the cell toxicity observed at certain concentrations, the leishmanicidal activity found in this fungus could be used for the development of new drugs for local treatment of lesions, thus avoiding systemic side effects. The development of an affordable simple drug with easy administration would be an asset for the local treatment of cutaneous leishmaniasis. Future plans involve a further study of the active components of this fungus, with improved identification techniques, in addition to understanding the role of these compounds, especially alkaloids, in the biology of the parasite.
Data Availability Statement
All datasets generated for this study are included in the article/Supplementary Material.
Author Contributions
DL, JL-S, and CP conceived and designed the experiments. DL, DA, LS, VP, TC-G, JL-S, and CP generated and analyzed the data. IS-J and CP contributed reagents. MV-S analyzed the TEM. DL, US, DA, IS-J, TC-G, and MV-S drafted the manuscript. All authors contributed to manuscript revision, read, and approved the submitted version.
Funding
This study was sponsored in part by the Coordenação de Aperfeiçoamento de Pessoal de Nível Superior—Brazil (CAPES)—Finance Code 001 and also by the Fundação de Amparo à Pesquisa do Estado da Bahia (FAPESB) by grants DCR0009/2015, RED 011/2012, and RED 008/2014. CP and MV-S are CNPq Research Fellows.
Conflict of Interest
The authors declare that the research was conducted in the absence of any commercial or financial relationships that could be construed as a potential conflict of interest.
Acknowledgments
The authors are indebted to the Center of Electron Microscopy of the State University of Santa Cruz (CME/UESC) and Electron Microscopy Service of the Gonçalo Moniz Institute (IGM/Fiocruz), and we also appreciate the technical assistance provided by Lucas Ribeiro dos Santos and Eliete da Cruz Souza.
Supplementary Material
The Supplementary Material for this article can be found online at: https://www.frontiersin.org/articles/10.3389/fcimb.2020.00306/full#supplementary-material
References
Adade, C. M., and Souto-Padrón, T. (2010). Contributions of ultrastructural studies to the cell biology of trypanosmatids: targets for anti-parasitic drugs. Open Parasitol. J. 4, 178–187. doi: 10.2174/1874421401004010178
Akbari, M., Oryan, A., and Hatam, G. (2017). Application of nanotechnology in treatment of leishmaniasis: a review. Acta Trop. 172, 86–90. doi: 10.1016/j.actatropica.2017.04.029
Atanasova, L., Druzhinina, I., and Jaklitsch, W. (2013). “Two hundred Trichoderma species recognized on the basis of molecular phylogeny,” in Trichoderma: Biology and Applications, eds P. K. Mukherjee, B. A. Horwitz, U. S. Singh, M. Mukherjee, and M. Schmoll (London: CAB International), 10–42. doi: 10.1079/9781780642475.0010
Basmaciyan, L., Berry, L., Gros, J., Azas, N., and Casanova, M. (2018). Temporal analysis of the autophagic and apoptotic phenotypes in Leishmania parasites. Microb. Cell 5, 404–417. doi: 10.15698/mic2018.09.646
Benaim, G., Paniz-Mondolfi, A. E., Sordillo, E. M., and Martinez-Sotillo, N. (2020). Disruption of intracellular calcium homeostasis as a therapeutic target against Trypanosoma cruzi. Front. Cell. Infect. Microbiol. 1–15. doi: 10.3389/fcimb.2020.00046.
Braga, M. V., Urbina, J. A., and De Souza, W. (2004). Effects of squalene synthase inhibitors on the growth and ultrastructure of Trypanosoma cruzi. Int. J. Antimicrob. Agents 24, 72–78. doi: 10.1016/j.ijantimicag.2003.12.009
Burza, S., Croft, S. L., and Boelaert, M. (2018). Seminar leishmaniasis. Lancet 392, 951–970. doi: 10.1016/S0140-6736(18)31204-2
Campos, F. F., Rosa, L. H., Cota, B. B., Caligiorne, R. B., Teles Rabello, A. L., Alves, T. M. A., et al. (2008). Leishmanicidal metabolites from Cochliobolus sp., an endophytic fungus isolated from Piptadenia adiantoides (Fabaceae). PLoS Negl. Trop. Dis. 2, 1–11. doi: 10.1371/journal.pntd.0000348
Cardoza, R., Hermosa, R., Vizcaino, J., Sanz, L., Monte, E., and Gutiérrez, S. (2005). Secondary metabolites produced by Trichoderma and their importance in the biocontrol process. Microorg. Ind. Enzym. Biocontrol. 37/661, 1–22.
Cariaco, Y., Lima, W. R., Sousa, R., Alencar, L., Nasciment, C., Briceño, M. P., et al. (2018). Ethanolic extract of the fungus Trichoderma stromaticum decreases inflammation and ameliorates experimental cerebral malaria in C57BL/6 mice. Sci. Rep. 8:1547. doi: 10.1038/s41598-018-19840-x
Cheville, N. F. (2009). Ultrastructural Pathology: The Comparative Cellular Basis of Disease, second ed. Ames, IA: Wiley-Blackwell.
Cruz, E. D. M., Da Silva, E. R., Maquiaveli, C. D. C., Alves, E. S. S., Lucon, J. F., Reis, M. B. G., et al. (2013). Leishmanicidal activity of Cecropia pachystachya flavonoids: arginase inhibition and altered mitochondrial DNA arrangement. Phytochemistry 89, 71–77. doi: 10.1016/j.phytochem.2013.01.014
D'Antonio, E. L., Ullman, B., Roberts, S, C., and Dixit, U. G. (2013). Crystal structure of arginase from Leishmania mexicana and implications for the inhibition of polyamine biosynthesis in parasitic infections. Arch. Biochem. Biophys. 535, 163–176. doi: 10.1016/j.abb.2013.03.015
do Nascimento, A. M., Soares, M. G., da Silva Torchelsen, F. K. V., de Araujo, J. A. V., Lage, P. S., Duarte, M. C., et al. (2015). Antileishmanial activity of compounds produced by endophytic fungi derived from medicinal plant Vernonia polyanthes and their potential as source of bioactive substances. World J. Microbiol. Biotechnol. 31, 1793–1800. doi: 10.1007/s11274-015-1932-0
Docampo, R., Ulrich, P., and Moreno, S. N. J. (2010). Evolution of acidocalcisomes and their role in polyphosphate storage and osmoregulation in eukaryotic microbes. Philos. Trans. R. Soc. B Biol. Sci. 365, 775–784. doi: 10.1098/rstb.2009.0179
Fonseca-Silva, F., Canto-Cavalheiro, M. M., Menna-Barreto, R. F. S., and Almeida-Amaral, E. E. (2015). Effect of apigenin on leishmania amazonensis is associated with reactive oxygen species production followed by mitochondrial dysfunction. J. Nat. Prod. 78, 880–884. doi: 10.1021/acs.jnatprod.5b00011
Fukuzawa, M., Yamaguchi, R., Hide, I., Chen, Z., Hirai, Y., Sugimoto, A., et al. (2008). Possible involvement of long chain fatty acids in the spores of Ganoderma lucidum (Reishi Houshi) to its anti-tumor activity. Biol. Pharm. Bull. 31, 1933–1937. doi: 10.1248/bpb.31.1933
Gao, J., Radwan, M. M., León, F., Wang, X., Jacob, M. R., Tekwani, B. L., et al. (2012). Antimicrobial and antiprotozoal activities of secondary metabolites from the fungus Eurotium repens. Med. Chem. Res. 21, 3080–3086. doi: 10.1007/s00044-011-9798-7
Garcia, F. P., Lazarin-Bidóia, D., Ueda-Nakamura, T., Silva, S. D. O., and Nakamura, C. V. (2013). Eupomatenoid-5 isolated from leaves of piper regnellii induces apoptosis in leishmania amazonensis. Evidence-based complement. Altern. Med. 2013:940531. doi: 10.1155/2013/940531
Isaka, M., Prathumpai, W., Wongsa, P., and Tanticharoen, M. (2006). Hirsutellone F, a dimer of antitubercular alkaloids from the seed fungus trichoderma species BCC 7579. Org. Lett. 8, 2815–2817. doi: 10.1021/ol060926x
Iwatsuki, M., Kinoshita, Y., Niitsuma, M., Hashida, J., and Mori, M., Ishiyama, A., et al. (2010). Antitrypanosomal peptaibiotics, trichosporins B-VIIa and B-VIIb, produced by Trichoderma polysporum FKI-4452. J. Antibiot. 63, 331–333; doi: 10.1038/ja.2010.41
Jacob, M., Mannherz, H. G., and Napirei, M. (2007). Chromatin breakdown by deoxyribonuclease1 promotes acetaminophen-induced liver necrosis: an ultrastructural and histochemical study on male CD-1 mice. Histochem. Cell Biol. 128, 19–33. doi: 10.1007/s00418-007-0289-3
Klaiklay, S., Rukachaisirikul, V., Saithong, S., Phongpaichit, S., and Sakayaroj, J. (2019). Trichothecenes from a soil-derived Trichoderma brevicompactum. J. Nat. Prod. 82, 687–693. doi: 10.1021/acs.jnatprod.8b00205
Lacerda, R., B, M., Freitas, T. R., Martins, M, M., and Teixeira, T. (2018). Isolation, leishmanicidal evaluation and molecular docking simulations of piperidine alkaloids from Senna spectabilis. Bioorgan Med Chem. 26, 5816–5823. doi: 10.1016/j.bmc.2018.10.032
Li, F. J., and He, C. Y. (2014). Acidocalcisome is required for autophagy in Trypanosoma brucei. Autophagy 10, 1978–1988. doi: 10.4161/auto.36183
Magtanong, L., Ko, P. J., and Dixon, S. J. (2016). Emerging roles for lipids in non-apoptotic cell death. Cell Death Differ. 23, 1099–1109. doi: 10.1038/cdd.2016.25
Maltezou, H. C. (2010). Drug resistance in visceral leishmaniasis. J. Biomed. Biotechnol. 2010:617521. doi: 10.1155/2010/617521
Manjolin, L. C., dos Reis, M., B, G., and Maquiaveli, C. C.. (2013). Dietary flavonoids fisetin, luteolin and their derived compounds inhibit arginase, a central enzyme in Leishmania (Leishmania) amazonensis infection. Food Chem. 2253–2262. doi: 10.1016/j.foodchem.2013.05.025
Maquiaveli, C. C., Lucon-Júnior, J, F., Brogi, S., Campiani, G., Gemma, S., Vieira, et al. (2016). Verbascoside inhibits promastigote growth and arginase activity of Leishmania amazonensis. J. Nat. Prod. 79, 1459–1463. doi: 10.1021/acs.jnatprod.5b00875
Matrangolo, F. S. V., Liarte, D. B., Andrade, L. C., De Melo, M. F., Andrade, J. M., Ferreira, R. F., et al. (2013). Comparative proteomic analysis of antimony-resistant and-susceptible Leishmania braziliensis and Leishmania infantum chagasi lines. Mol. Biochem. Parasitol. 190, 63–75. doi: 10.1016/j.molbiopara.2013.06.006
Medina, J. M., Rodrigues, J. C. F., De Souza, W., Atella, G. C., and Barrabin, H. (2012). Tomatidine promotes the inhibition of 24-alkylated sterol biosynthesis and mitochondrial dysfunction in Leishmania amazonensis promastigotes. Parasitology 139, 1253–1265. doi: 10.1017/S0031182012000522
Mishra, B. B., Kale, R, R., Singh, R. K., and Tiwari, V, D. (2009). Alkaloids: future prospective to combat leishmaniasis. Fitoterapia 80, 81–90. doi: 10.1016/j.fitote.2008.10.009
Mosmann, T. (1983). Rapid colorimetric assay for cellular growth and survival: application to proliferation and cytotoxicity assays. J. Immunol. Methods 65, 55–63. doi: 10.1016/0022-1759(83)90303-4
Netala, V. R., Bethu, M. S., Pushpalatha, B., Baki, V. B., Aishwarya, S., and Rao, J. V. (2016). Biogenesis of silver nanoparticles using endophytic fungus Pestalotiopsis microspora and evaluation of their antioxidant and anticancer activities. Int. J. Nanomed. 11, 5683–5696. doi: 10.2147/IJN.S112857
Olliaro, P. L. (2010). Drug combinations for visceral leishmaniasis. Curr. Opin. Infect. Dis. 23, 595–602. doi: 10.1097/QCO.0b013e32833fca9d
Pinto-Martinez, A. K., Rodriguez-Durán, J., Serrano-Martin, X., Hernandez-Rodriguez, V., and Benaim, G. (2018). Mechanism of action of miltefosine on Leishmania donovani involves the impairment of acidocalcisome function and the activation of the sphingosine-dependent plasma membrane Ca 2+ channel. Antimicrob. Agents Chemother. 62, 1–10. doi: 10.1128/AAC.01614-17
Podder, D., and Ghosh, S. (2019). A new application of Trichoderma asperellum as an anopheline larvicide for ecofriendly management in medical Science. Sci Rep. 9:1108. doi: 10.1038/s41598-018-37108-2
Rajasekaran, R., and Chen, Y. P. P. (2015). Potential therapeutic targets and the role of technology in developing novel antileishmanial drugs. Drug Discov. Today 20, 958–968. doi: 10.1016/j.drudis.2015.04.006
Roberts, S., and Ullman, B. (2017). Parasite polyamines as pharmaceutical targets. Curr. Pharm Des. 23, 3325–3341. doi: 10.2174/1381612823666170601101644
Rodrigues, J. C. F., Attias, M., Rodriguez, C., Urbina, J. A., and Souza, W. de (2002). Ultrastructural and Biochemical alterations induced by 22,26-Azasterol, a Δ24(25)-sterol methyltransferase inhibitor, on promastigote and amastigote forms of Leishmania amazonensis. Antimicrob. Agents Chemother. 46, 487–499. doi: 10.1128/AAC.46.2.487-499.2002
Rodrigues, J. C. F., and de Souza, W. (2008). Ultrastructural alterations in organelles of parasitic protozoa induced by different classes of metabolic inhibitors. Curr. Pharm. Des. 14, 925–938. doi: 10.2174/138161208784041033
Santos, S. S., Alves, A. C., Pereira, J. S., Augusto, D. G., Duarte, L. M. B., Melo, P. C., et al. (2018). Trichoderma asperelloides ethanolic extracts efficiently inhibit Staphylococcus growth and biofilm formation. PLoS ONE 13:e0202828. doi: 10.1371/journal.pone.0202828
Schuster, A., and Schmoll, M. (2010). Biology and biotechnology of Trichoderma. Appl. Microbiol. Biotechnol. 87, 787–799. doi: 10.1007/s00253-010-2632-1
Scorza, B. M., Carvalho, E. M., and Wilson, M. E. (2017). Cutaneous manifestations of human and murine leishmaniasis. Int. J. Mol. Sci. 18:1296. doi: 10.3390/ijms18061296
Scotti, M. T., Scotti, L., Ishikib, H., Ribeiro, F, F., and da Cruza, R. (2016). Natural products as a source for antileishmanial and antitrypanosomal agents. Comb. Chem. High Throughput Screen. 19, 537–553. doi: 10.2174/1386207319666160506123921
Shukla, S. T., Habbu, P. V., Kulkarni, V. H., and Sutariya, V. (2014). Endophytic microbes: a novel source for biologically/pharmacologically active secondary metabolites. Asian J. Pharmacol. Toxicol. 2, 1–16.
Singh, R., Kaushik, S., Wang, Y., Xiang, Y., Novak, I., Komatsu, M., et al. (2009). Autophagy regulates lipid metabolismo. Nature 458, 1131–1135. doi: 10.1038/nature07976
Tenguria, R. K., Khan, F. N., and Quereshi, S. (2011). Endophytes- mines of pharmacological therapeutics. World J. Sci. Technol. 1, 127–149.
Vallochi, A. L., Teixeira, L., Oliveira, K., da, S., Maya-Monteiro, C. M., and Bozza, P. T. (2018). Lipid droplet, a key player in host-parasite interactions. Front. Immunol. 9:1022. doi: 10.3389/fimmu.2018.01022
Vannier-Santos, M., and De Castro, S. (2009). Electron microscopy in antiparasitic chemotherapy: a (Close) view to a kill. Curr. Drug Targets 10, 246–260. doi: 10.2174/138945009787581168
Vannier-Santos, M., Martiny, A., and Souza, W. (2002). Cell biology of leishmania spp.: invading and evading. Curr. Pharm. Des. 8, 297–318. doi: 10.2174/1381612023396230
Vannier-Santos, M. A., and Lins, U. (2001). Cytochemical techniques and energy-filtering transmission electron microscopy applied to the study of parasitic protozoa. Biol. Proced. Online 3, 8–18. doi: 10.1251/bpo19
Vannier-Santos, M. A., Martiny, A., Lins, U., Urbina, J. A., Borges, V. M., and De Souza, W. (1999). Impairment of sterol biosynthesis leads to phosphorus and calcium accumulation in Leishmania acidocalcisomes. Microbiology 145, 3213–3220. doi: 10.1099/00221287-145-11-3213
Vannier-Santos, M. A., Menezes, D., Oliveira, M. F., and de Mello, F. G. (2008). The putrescine analogue 1,4-diamino-2-butanone affects polyamine synthesis, transport, ultrastructure and intracellular survival in Leishmania amazonensis. Microbiology 154, 3104–3111. doi: 10.1099/mic.0.2007/013896-0
Vannier-Santos, M. A., and Suarez-Fontes, A. M. (2017). Role of polyamines in parasite cell architecture and function. Curr. Pharm. Des. 23. doi: 10.2174/1381612823666170703163458
Vannier-Santos, M. A., Urbina, J. A., Martiny, A., Neves, A., and De Souza, W. (1995). Alterations induced by the antifungal compounds ketoconazole and terbinafine in Leishmania. J. Eukaryot. Microbiol. 42, 337–346. doi: 10.1111/j.1550-7408.1995.tb01591.x
World Health Organization (2020). Leishmaniasis. Available online at: https://www.who.int/en/news-room/fact-sheets/detail/leishmaniasis (accessed April 16, 2020).
Keywords: Trichoderma, chemotherapy, leishmanicidal, leishmaniasis, Leishmania amazonensis
Citation: Lopes DS, Santos UR, Dos Anjos DO, Silva Júnior LJC, Paula VF, Vannier-Santos MA, Silva-Jardim I, Castro-Gomes T, Pirovani CP and Lima-Santos J (2020) Ethanolic Extract of the Fungus Trichoderma asperelloides Induces Ultrastructural Effects and Death on Leishmania amazonensis. Front. Cell. Infect. Microbiol. 10:306. doi: 10.3389/fcimb.2020.00306
Received: 14 January 2020; Accepted: 20 May 2020;
Published: 15 July 2020.
Edited by:
Javier Moreno, Instituto de Salud Carlos III (ISCIII), SpainReviewed by:
Laurence A. Marchat, National Polytechnic Institute of Mexico (IPN), MexicoGaladriel Hovel-Miner, George Washington University, United States
Copyright © 2020 Lopes, Santos, Dos Anjos, Silva Júnior, Paula, Vannier-Santos, Silva-Jardim, Castro-Gomes, Pirovani and Lima-Santos. This is an open-access article distributed under the terms of the Creative Commons Attribution License (CC BY). The use, distribution or reproduction in other forums is permitted, provided the original author(s) and the copyright owner(s) are credited and that the original publication in this journal is cited, in accordance with accepted academic practice. No use, distribution or reproduction is permitted which does not comply with these terms.
*Correspondence: Jane Lima-Santos, amxzYW50b3MmI3gwMDA0MDt1ZXNjLmJy