- Institute of Infection, Immunity and Inflammation, University of Glasgow, Glasgow, United Kingdom
Cytokinesis, or the division of the cytoplasm, following the end of mitosis or meiosis, is accomplished in animal cells, fungi, and amoebae, by the constriction of an actomyosin contractile ring, comprising filamentous actin, myosin II, and associated proteins. However, despite this being the best-studied mode of cytokinesis, it is restricted to the Opisthokonta and Amoebozoa, since members of other evolutionary supergroups lack myosin II and must, therefore, employ different mechanisms. In particular, parasitic protozoa, many of which cause significant morbidity and mortality in humans and animals as well as considerable economic losses, employ a wide diversity of mechanisms to divide, few, if any, of which involve myosin II. In some cases, cell division is not only myosin II-independent, but actin-independent too. Mechanisms employed range from primitive mechanical cell rupture (cytofission), to motility- and/or microtubule remodeling-dependent mechanisms, to budding involving the constriction of divergent contractile rings, to hijacking host cell division machinery, with some species able to utilize multiple mechanisms. Here, I review current knowledge of cytokinesis mechanisms and their molecular control in mammalian-infective parasitic protozoa from the Excavata, Alveolata, and Amoebozoa supergroups, highlighting their often-underappreciated diversity and complexity. Billions of people and animals across the world are at risk from these pathogens, for which vaccines and/or optimal treatments are often not available. Exploiting the divergent cell division machinery in these parasites may provide new avenues for the treatment of protozoal disease.
Introduction
Cytokinesis is the final stage of cell division, where the mother cell's cytoplasm, following the replication/duplication and segregation of cellular components, is partitioned, resulting in two daughter cells. To date, eukaryotic cell division has mostly been studied in cells of model organisms such as yeast, plants and animals. Cytokinesis is broadly considered to comprise the following key events (Pollard and Wu, 2010; Glotzer, 2017), although modifications occur during asymmetric division (Thieleke-Matos et al., 2017), insect embryogenesis (Xue and Sokac, 2016) and life cycle-related morphogenetic changes (Seiler and Justa-Schuch, 2010):
(i) division site selection (which often occurs much earlier in the cell cycle or even in the previous cell cycle).
(ii) initiation signaling events.
(iii) division machinery assembly.
(iv) daughter cell partitioning by plasma membrane constriction (furrowing) or new membrane and cell wall construction (vesicle fusion).
(v) division machinery disassembly.
(vi) daughter cell separation (abscission).
Within the evolutionary supergroups Opisthokonta (including metazoa and fungi) and Amoebozoa (amoebae), cytokinesis occurs via the assembly and constriction of a contractile actomyosin ring composed of filamentous actin, myosin II, and associated proteins during anaphase, perpendicular to the midpoint of the mitotic spindle (reviewed in Fededa and Gerlich, 2012; Pollard and O'Shaughnessy, 2019 and summarized in Figure 1). Members of all other supergroups (excepting perhaps Naegleria spp.) use different mechanisms to divide since they lack myosin II (Richards and Cavalier-Smith, 2005; Odronitz and Kollmar, 2007; Fritz-Laylin et al., 2010; Sebe-Pedros et al., 2014). Land plants and some green algae, for example, use vesicle delivery to assemble a phragmoplast composed of actin, microtubules, membranes and proteins, which partitions daughter cells (Livanos and Muller, 2019), while other green algae use a microtubule-based phycoplast (Cross and Umen, 2015). Parasitic protozoa use a plethora of alternative and divergent cytokinesis strategies.
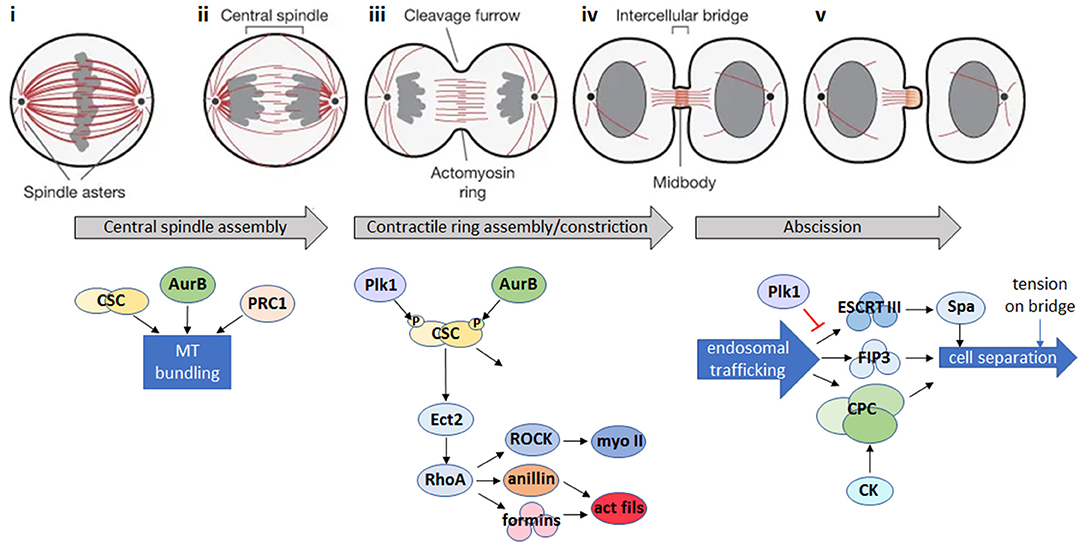
Figure 1. Animal cell cytokinesis. Top: schematic of the major events during cytokinesis in animal cells [gray: DNA; red: microtubules; adapted by permission from Springer Nature: ©(Fededa and Gerlich, 2012)]. Bottom: summary of the main signaling events during cytokinesis in animal cells. (i) During mitotic metaphase, condensed chromosomes align at the metaphase plate. (ii) Bipolar attachment of chromosomes to spindle microtubules releases the spindle attachment checkpoint and activates the anaphase promoting complex/cyclosome (APC/C), which degrades mitotic cyclin B and inactivates the mitotic cyclin-dependent kinase (CDK1). CDK1 inactivation triggers reorganization of the mitotic spindle into an array of antiparallel microtubule bundles (the central spindle) between the separating chromosomes. Microtubule bundling is promoted by Aurora B (AurB), the centralspindlin complex (CSC) and microtubule-bundling protein required for cytokinesis 1 (PRC1). (iii) A cortical contractile ring assembles from long formin-nucleated actin filaments and bipolar filaments of the motor, myosin II, and constricts to cleave the daughter cells. Actomyosin ring assembly is initiated in response to a signaling pathway where Polo-like kinase 1 (Plk1) and AurB phosphorylate the CSC, leading to activation of the Rho GDP-GTP exchange factor, Ect2, and its translocation to the cell cortex where it activates the RhoA GTPase. RhoA activates both myosin II (myo II) via the Rho kinase, ROCK, and formins which nucleate actin filaments (act fils), and recruits the scaffold protein anillin, resulting in the formation of actin and myosin filaments and subsequent assembly of the actomyosin ring. In addition to continued RhoA signaling, constriction of the actomyosin ring is influenced by changes in cortical tension, plasma membrane lipid composition at the site of furrow ingression, and by active force generation by the action of myosin motors (Emoto et al., 2005; Atilla-Gokcumen et al., 2014; Glotzer, 2017). (iv) The central spindle is compacted to form a microtubule-based midbody positioned in the center of a thin intercellular bridge that connects the daughter cells while the contractile ring is converted into a cortical midbody ring. (v) Endosomal trafficking of the Chromosomal Passenger Complex (CPC) and FIP3-endosomes, together with the Endosomal Sorting Complex Required for Transport III (ESCRT-III) filament system, which recruits the microtubule severing enzyme, spastin (Spa), act to remodel the intercellular bridge and bring about abscission, the final topological separation of the two daughter cells (Connell et al., 2009; Carmena et al., 2012; D'Avino and Capalbo, 2016). Additional regulators of abscission include citron kinase (CK), which works together with AurB in the CPC to stabilize the midbody architecture (Watanabe et al., 2013; McKenzie et al., 2016) and Plk1, which inhibits ESCRT-III recruitment to the midbody until late cytokinesis, when Plk1 is degraded (Bastos and Barr, 2010). Abscission is also regulated by tension on the intercellular bridge (Gould, 2016).
The Parasitic Protozoa and Myosin II
The protozoa are a diverse group of unicellular organisms, encompassing both free-living and parasitic species, with mammalian-infective species being found in the Amoebozoa (archamoebae), Excavata (heteroloboseans, parabasalids, diplomonads, and kinetoplastids), and Alveolata (ciliates and apicomplexans) supergroups (Figure 2). Of the myosin II-containing species, Entamoeba spp. are the best studied, yet there is no evidence for an actomyosin ring. Further, while it is possible that myosin II is key to cytokinesis in Naegleria spp., this has not yet been experimentally demonstrated. Most parasitic protozoa, though, lack myosin II, and some (Giardia and Trichomonas) lack functional myosins altogether (Richards and Cavalier-Smith, 2005; Odronitz and Kollmar, 2007; Sebe-Pedros et al., 2014), using e.g., alternative myosins or mechanisms relying on flagellar/ciliary motility and/or microtubule remodeling to divide. Further, some parasitic protozoa display relaxed cytokinesis regulatory mechanisms, with the apparent absence of canonical molecular checkpoints. Knowledge of the molecular regulation of cytokinesis is scant in some species, but substantial in others. Arguably, a greater knowledge of how these organisms divide is not just of interest from an evolutionary perspective given that model organisms are restricted to just three of the seven evolutionary supergroups (Figure 2) (Worden et al., 2015), but, given the significant morbidity and mortality caused by mammalian-infective parasitic protozoa, of great importance for developing novel parasite control strategies.
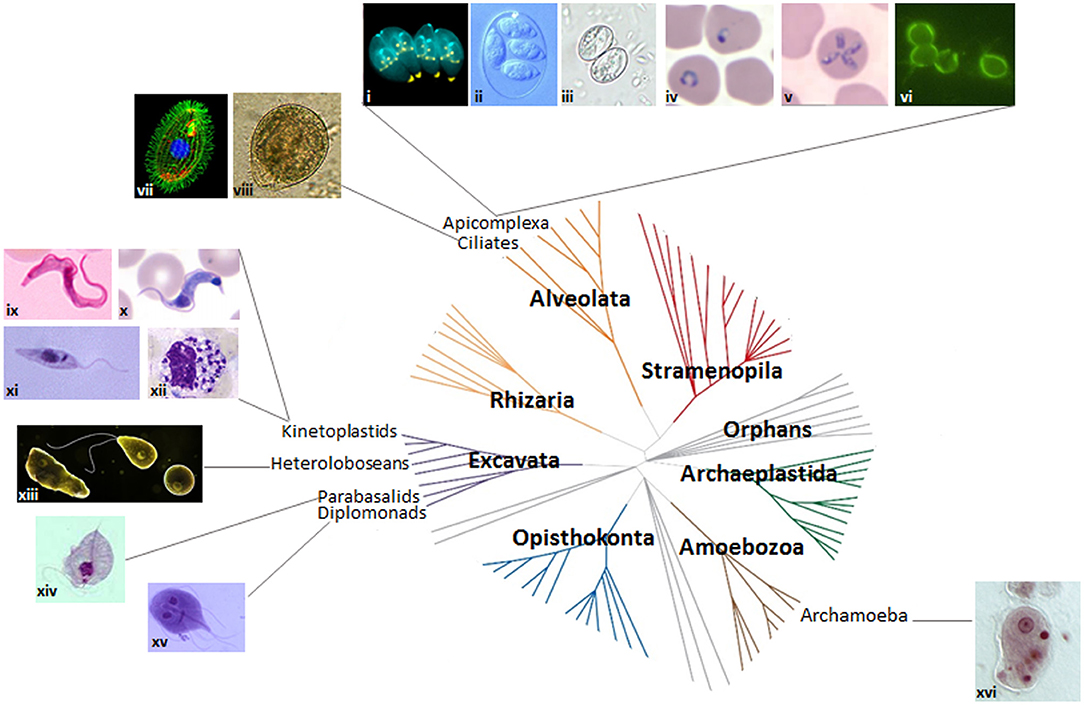
Figure 2. Protozoan parasites and the eukaryotic tree of life. Images of protozoan parasites (not to scale) mapped onto the seven domains of the eukaryotic tree of life. Adapted from Worden et al. (2015). Reprinted with permission from AAAS. (i) dividing Toxoplasma gondii (credit: Ke Hu and John M. Murray); (ii) Eimeria maxima (credit: S.J. Upton); (iii) Sarcocystis sporulated oocyst (credit: DPDx image gallery); (iv) P. falciparum ring stage (credit: DPDx image gallery); (v) Theileria microti (credit: DPDx image gallery); (vi) Cryptosporidium parvum (credit: EPA/H.D.A. Lindquist); (vii) Tetrahymena thermophila (credit: Dr. Muthugapatti Kandasamy, Director of the Biomedical Microscopy Core. University of Georgia bmc.uga.edu/); (viii) Balantidium coli (credit: Euthman); (ix) Trypanosoma brucei bloodstream stage (credit: CDC/Dr. Myron G. Schultz); (x) T. cruzi trypomastigote (credit: DPDx image gallery); (xi) Leishmania promastigote (credit: CDC/Dr. Mae Melvin/Public Health Image Library); (xii) Leishmania amastigotes in white blood cell (credit: DPDx image gallery); (xiii) Naegleria fowleri trophozoite, flagellated form and cyst (https://www.cdc.gov/parasites/naegleria/); (xiv) Trichomonas trophozoite (credit: DPDx image gallery); (xv) Giardia trophozoite (credit: schmidty4112), and (xvi) Entamoeba histolytica trophozoite (credit: Stefan Walkowski).
Cytokinesis in the Amoebozoa
Entamoeba spp. (Infraphylum Archamoebae) Divide by Cytofission
Entamoeba spp., which can cause dysentery and liver abscesses in humans and animals, display extremely plastic cell division. The Entamoeba genome is genetically heterogeneous, with individual trophozoites displaying ploidies of 1–10 n and varying numbers of nuclei. Cytokinesis involves plasma membrane constriction and formation/severing of an intercellular bridge (Figure 3), similar to other amoebae and animal cells. However, cell division is delinked from S phase and mitosis, occurring erratically and often asymmetrically, resulting in cells with no, one or multiple nuclei (Orozco et al., 1988; Lohia, 2003; Lohia et al., 2007; Mukherjee et al., 2009), with cell fusion events also contributing to multinuclear cell formation (Krishnan and Ghosh, 2018). Indeed, genome analysis suggests that E. histolytica encodes orthologs of all essential budding yeast cytokinesis proteins (although these have not been characterized), but that many cell cycle checkpoint proteins are not conserved (Grewal and Lohia, 2015), providing an explanation for the erratic division. Further, no actomyosin ring appears to be formed. Myosin II is present but has not yet been shown to convincingly localize to the constriction site, and actin forms longitudinal cables that run through the intercellular bridge (Majumder and Lohia, 2008). This is in contrast to the non-parasitic amoeba Dictyostelium, which does form a myosin-based contractile ring (De Lozanne and Spudich, 1987; Fukui, 1990; Fukui and Inoue, 1991), although since Dictyostelium cells lack a midbody, they utilize traction or external forces to complete abscission (Taira and Yumura, 2017). The Entamoeba intercellular bridge is severed mechanically as cells pull apart, although ~30% of cell cleavage events are aided by a helper or “midwife” cell migrating through the bridge, providing external mechanical force to ensure its scission (Biron et al., 2001; Mukherjee et al., 2009; Krishnan and Ghosh, 2018) (Figures 3A,B), as also observed in Dictyostelium (Tanaka et al., 2019). 10–20% of initiated cytokinetic events in Entamoeba are unsuccessful; the intercellular bridge retracts and daughter cells re-fuse.
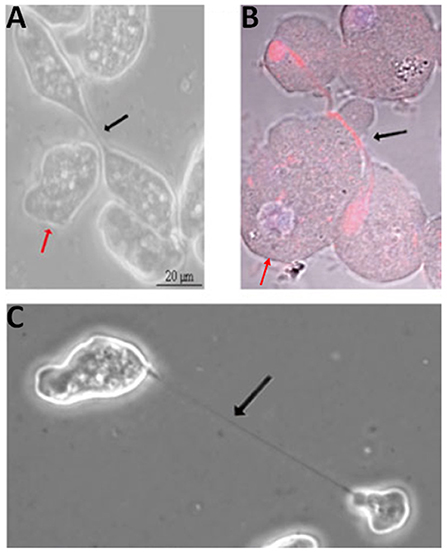
Figure 3. Cytokinesis in Entamoeba histolytica. Helper cells (red arrows) approaching (A) and migrating beneath (B; red: tubulin; blue: DNA) the intercellular bridge (black arrows) in dividing E. histolytica cells. (C) Dividing E. histolytica cells with an extended intercellular bridge. Adapted by permission from Springer Nature© (Grewal and Lohia, 2015).
Mechanical rupture or cytofission, uncoupled to the cell cycle, may be one of the earliest evolutionary mechanisms for cytokinesis, and is considered myosin II-independent, being also employed by Dictyostelium cells lacking myosin II (Taira and Yumura, 2017). However, the myosin II inhibitor, 2,3-butanedione monoxime, inhibits cytofission in E. invadens multinuclear giant cells, suggesting that at least in these cells, cytofission may be dependent on myosin II (Krishnan and Ghosh, 2018). Other likely Entamoeba cytokinesis proteins include actin, Ehformin-1 and Ehformin-2, which localize to the constriction site and the intercellular bridge, along with tubulin and the calcium- and β-tubulin-binding protein, EhCaBP6 (Chavez-Munguia et al., 2006; Majumder and Lohia, 2008; Grewal et al., 2013; Verma et al., 2017). Overexpression of Ehformin-1 [whose activity is regulated by Rho1 GTPase (Bosch et al., 2012)] or Ehformin-2 increases ploidy and formation of binucleate/multinucleate cells (Majumder and Lohia, 2008). Further, overexpression of the kinase domain of p21-activated kinase, EhPAK2 (Arias-Romero et al., 2006), or constitutive activation of EhRACA (Ghosh and Samuelson, 1997) or EhRACG (Guillen et al., 1998) also inhibit cytokinesis in ~10% cells, leading to the formation of multinucleate cells. The EhPC4 transcription factor seems to regulate cytokinesis (in addition to DNA replication), with its overexpression upregulating expression of various cell cycle proteins, e.g., EhNUDC, a nuclear movement protein ortholog, inhibiting cytokinesis and resulting in the formation of multinucleate cells (Hernández De La Cruz et al., 2016). Finally, analysis of the Entamoeba protein kinome suggests that some canonical regulators are present, but unusual and divergent signaling pathways may also operate (Anamika et al., 2008).
Cytokinesis in the Excavata
Parasitic Excavata exhibit a wide variety of division mechanisms. While Naegleria potentially divides via a conventional actomyosin ring, the majority of excavate parasites divide along their long axis, employing mechanisms that heavily rely on flagellar motility and cytoskeletal rearrangements, and are regulated by complex signaling pathways.
Naegleria spp. (Class Heterolobosea): Myosin II Is Present, but Is It Required for Cytokinesis?
Despite not being an Amoebozoa, Naegleria reproduces as an amoeboid form, although it also forms a transient, non-dividing flagellated form and a cyst form. Most Naegleria spp. are free-living protists that feed on bacteria. However, N. fowleri is an opportunistic human pathogen, causing usually fatal primary amoebic meningoencephalitis. Little attention has been paid to Naegleria cytokinesis, despite detailed studies of nuclear division having revealed unusual and divergent features (Schuster, 1975; Gonzalez-Robles et al., 2009; Walsh, 2012). However, dividing cells appear to constrict at the division site with daughter cells linked by an intercellular bridge prior to abscission, similar to animal cells, although it is not known if an actomyosin ring forms (Gonzalez-Robles et al., 2009; Walsh, 2012). N. gruberi genome analysis has revealed the presence of genes encoding myosin II as well as complete actin and microtubule cytoskeletons, although in the amoeboid form, microtubules are only present within the mitotic spindle (Fulton and Simpson, 1976; Chung et al., 2002; Fritz-Laylin et al., 2010, 2011). However, the signaling networks regulating cell division remain to be elucidated, and given that the genome encodes at least 265 protein kinases, 32 protein phosphatases and 182 Ras GTPases, along with 21 RhoGEFs and 25 RhoGAPs, but apparently no Rho GTPase genes, they are likely to be complex and potentially divergent (Fritz-Laylin et al., 2010, 2011).
Giardia (Order Diplomonadida): Flagellar Motility, Vesicle Trafficking, and Cytoskeletal Rearrangements Are Key
Giardia, also known as Giardia lamblia, G. intestinalis, or G. duodenalis, is a waterborne diplomonad that causes the diarrhoeal disease, Giardiasis. It exists as a proliferative flagellated trophozoite and a water-resistant cyst. Trophozoites have two genetically identical diploid nuclei, and four pairs of flagella (anterior, posterolateral, ventral and caudal) (McInally and Dawson, 2016). There are also several other microtubule-based structures including the ventral disc, which mediates surface attachment, and the median body, which lies perpendicular to the caudal axonemes and is present only up until mitosis (Hardin et al., 2017). Cytokinesis proceeds along the long axis of the cell, from the anterior end (Figure 4), and is promoted by remodeling of the parental ventral disc following mitotic spindle disassembly (Hardin et al., 2017). Daughter cells move in opposite directions, receiving two nuclei derived from each of the mother cell nuclei (Sagolla et al., 2006).
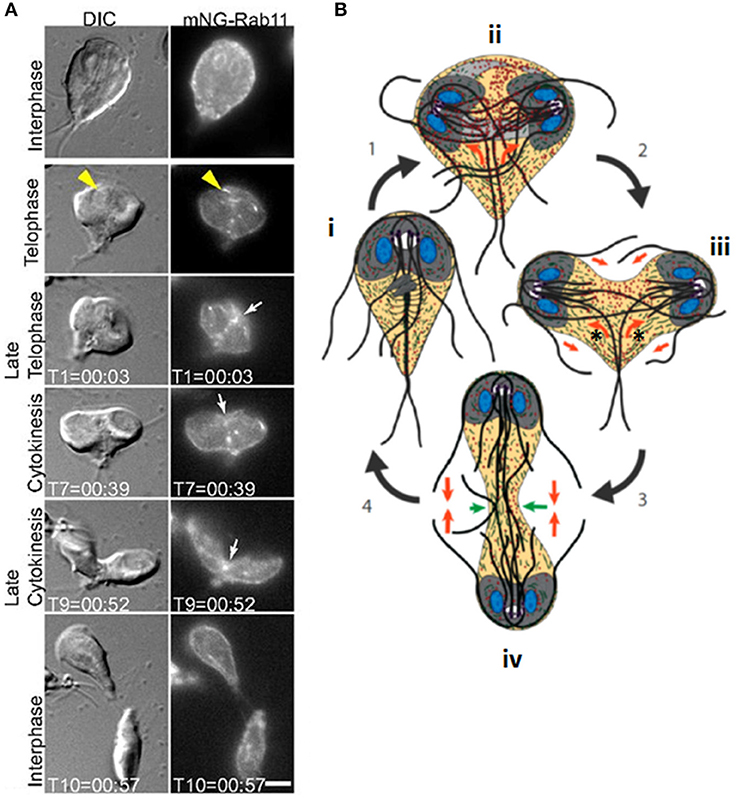
Figure 4. Cytokinesis in Giardia. (A) Stills from time-lapse imaging of an mNeonGreen-Rab11-expressing Giardia trophozoite undergoing cytokinesis. Rab11 is recruited to the intracytoplasmic flagellar axonemes (yellow arrowheads) in telophase and marks the cleavage furrow just prior to and during furrow ingression (white arrows). Scale bar: 5 μm. (B): cartoon of dividing Giardia trophozoite. Nuclei are shown in blue, ventral discs in gray and flagella as dark gray/black lines. An interphase cell (i) initiates mitosis (1). Actin (green) positions the microtubule cytoskeleton (ii). Rab11 (red) labels the intracytoplasmic axonemes; newly-forming axonemes guide Rab11 to the furrow (orange arrows). Furrow ingression (2) is initiated in response to the thinning of overlapping microtubules in the parental ventral disc (light gray), which allows it to open to a C-shaped conformation (new daughter cell ventral discs are in dark gray), and opposing forces (orange arrow) from the intracytoplasmic caudal axonemes (asterisks) (iii). (3) Caudal flagella flexion continues to drive the daughter cells apart and reduced cortical actin at the leading edge of the furrow (green arrows, iv) ensures continued furrow ingression. (4) Flagellar motility and Rab11-mediated trafficking of membrane remodeling proteins results in abscission. Adapted from Hardin et al. (2017).
Flagellar forces appear to be critical for Giardia cytokinesis. Inhibiting flagellar motility via depletion of axonemal central pair protein, PF16, results in a 10-fold increase in cells with four or more nuclei, leading to the proposal that furrowing initiates in response to sustained bending of the caudal flagella, while anterior flagella motility may contribute to abscission (Hardin et al., 2017). This flagellar motility-based division is highly efficient, with mitosis occurring in ~6.5 min, and cytokinesis taking just 50 s, some 30–90 times faster than has been reported in plants, fungi, and mammalian cells. Cytokinesis does not proceed uniformly; furrow ingression occurs at a faster rate than abscission, suggesting sequential action of cell division components. Further, Brefeldin A treatment (which disrupts trafficking from the endoplasmic reticulum) arrests furrowing at a defined point, approximately midway through ingression, indicating that additional membrane/proteins are required to complete cytokinesis (Hardin et al., 2017). Cells that arrest partway through cytokinesis are heart-shaped, and although heart-shaped cells were formerly thought to be a normal cytokinesis stage (Benchimol, 2004a; Sagolla et al., 2006; Paredez et al., 2011), it has recently been proposed that such cells are abnormal cells that are not actively dividing (Hardin et al., 2017).
Actin is also key for Giardia cytokinesis, since its knockdown stalls furrow ingression and delays abscission or blocks cytokinesis completely in a subset of cells. However, actin does not mark the division furrow, and is instead enriched around spindles and developing axonemes, likely helping to position these structures (Paredez et al., 2011). Actin abundance is reduced just ahead of the leading edge of the furrow, which may promote furrow ingression via alterations in cortical tension, as in other organisms (Hardin et al., 2017). Actin is also required for protein trafficking (Paredez et al., 2011) potentially supporting vesicular trafficking required for furrowing/abscission. The GTPase, Rab11, is also key for vesicle trafficking and cytokinesis, and its knockdown extends or prevents cytokinesis (Hardin et al., 2017). Rab11 delineates the cleavage furrow during pre-furrowing (where the cell membrane invaginates, but has not yet cleaved), remaining in the furrow and marking its leading edge during cytokinesis (Figure 4). Further, it marks intracytoplasmic flagellar axonemes late in telophase and the plus ends of growing axonemes, co-localizing with actin, and it has been proposed that developing axonemes are positioned to perform a midbody/phragmoplast-like role in directing trafficking to the furrow (Hardin et al., 2017). Sphingolipid biosynthesis also appears to be important for Giardia cytokinesis since treatment with the glucosylceramide synthase (GCS) inhibitor, DL-threo-1-phenyl-2-palmitoylamino-3-morpholino-1-propanol (PPMP), or increasing ceramide levels, significantly inhibited cytokinesis leading to the appearance of large proportions of doublet (heart-shaped) and triplet cells (Sonda et al., 2008; Stefanic et al., 2010). Further, GCS appears to be important for vesicular trafficking. Another sphingolipid, psychosine, has also been reported to inhibit Giardia cytokinesis (Stefanic et al., 2010).
Several canonical mitotic and/or cytokinesis regulators, including CDK1-like kinases, a Wee1 kinase, an Aurora kinase (AK), and a Polo-like kinase (PLK) are present in Giardia (Manning et al., 2011), although cell cycle protein degradation machinery (APC and SCF complexes) is missing (Gourguechon et al., 2013). Differential expression profiles of cell cycle proteins across the cell cycle have been mapped (Horlock-Roberts et al., 2017), but their cell cycle-dependent degradation is not always mediated via the ubiquitin pathway (Gourguechon et al., 2013). Of the putative cell cycle kinases, only AK has been studied functionally. AK inhibitors block completion of Giardia cytokinesis (Davids et al., 2008). AK co-localizes with its potential substrate, microtubule end-binding protein 1 homolog, GlEB1, at the nuclear membrane and median body during interphase, and at the mitotic spindle during mitosis. GlEB1 is phosphorylated on S148 by AK in vitro, a phosphosite that, when mutated in vivo, leads to defects in cytokinesis (Kim et al., 2017), suggesting AK plays roles in the disassembly/reorganization of the cytoskeleton during mitosis and cytokinesis. Further, GlEB1 also interacts with Glγ-tubulin in vivo; depletion of Glγ-tubulin or two γ-tubulin complex proteins, GlGCP2, and GlGCP3, results in cytoskeletal defects, including defects in median body formation and flagellar structural defects, and inhibition of furrowing (Kim and Park, 2019).
Trichomonas spp. (Parabasalia): Flagellar Motility and Cytoskeletal Rearrangements Are Important
Trichomonads are flagellated protists that cause trichomoniasis in some hosts; the human pathogen, Trichomonas vaginalis, which infects ~3% of the world's population annually (Kusdian et al., 2013), and the cattle and feline pathogen, Tritrichomonas fetus, are among the best studied. In culture, trichomonads have a tear-drop shape during interphase, but transform to multinucleate amoeboid forms when in contact with epithelial cells, and will form pseudocysts under stress (Benchimol, 2004b). Like Giardia, they have a complex cytoskeleton, comprising various microtubule (axostyle, pelta, basal bodies, flagella, and mitotic spindle) and proteinaceous (costa, rootlet, and parabasal filaments) structures (Benchimol, 2004b; Preisner et al., 2016). Actin is also present throughout the cell (Brugerolle et al., 1996; Kusdian et al., 2013). During cell division, basal body migration, signaling the start of mitosis, and flagellar-driven propulsion lead to morphological changes that drive crossing of duplicated axostyles, resulting in constriction of the nucleus during karyokinesis and thinning of the connection between the daughter cells, such that by telophase, daughter cells remain connected only by an axostylar trunk (Ribeiro et al., 2000) (Figure 5). Flagellar motility then rotates the daughters around their axis, likely providing torsional forces to help drive cytokinesis to completion (Ribeiro et al., 2000; Benchimol, 2004b). However, despite detailed ultrastructural studies of mitosis and cytokinesis, very little is known of their molecular regulation (Amador et al., 2017).
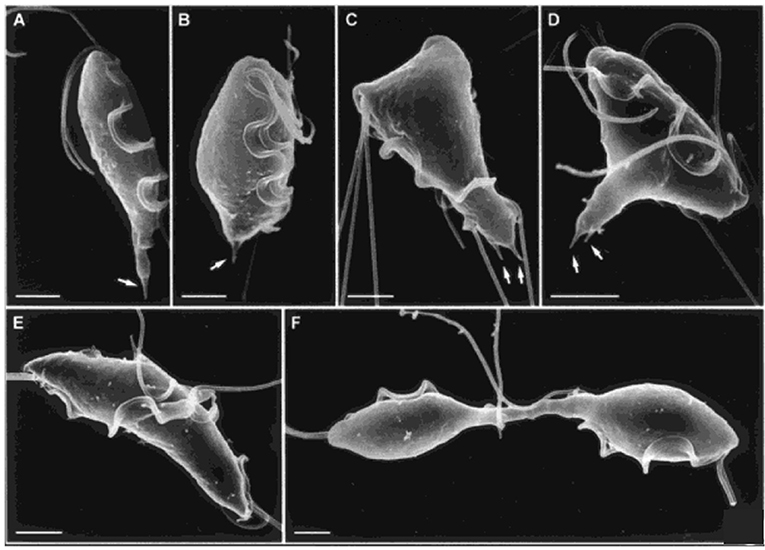
Figure 5. Cytokinesis in Trichomonas. Scanning electron microscopy images of T. fetus at different cell cycle stages. (A) interphase; (B) premitosis; (C) prophase; (D) metaphase; (E) anaphase; (F) telophase with daughter cells connected by an axostylar trunk. Scale bars: 3 μm (A,F); 2 μm (B–E). Arrows: axostyle tips. Adapted by permission from Cambridge University Press© Microscopy Society of America (Benchimol, 2004b).
Trypanosoma and Leishmania spp. (Class Kinetoplastea): Complex Signaling, Microtubule Cytoskeleton Remodeling, and Flagellar Motility Regulate Longitudinal Furrow Ingression
The defining feature of the Kinetoplastea is the kinetoplast, a disc-shaped structure containing the DNA of the single mitochondrion (kDNA), although some Trypanosoma brucei subspecies e.g., T. b. evansi and T. b. equiperdum have lost all or part of their kDNA (Lai et al., 2008). Some kinetoplastids are free-living; others infect animals and plants, often being transmitted between hosts by insect vectors. Among the best studied are the mammalian pathogens, Trypanosoma brucei spp. (and to a lesser extent, T. congolense), the causative agents of human and/or animal African trypanosomiasis (HAT/AAT), Trypanosoma cruzi, the causative agent of Chagas disease, and Leishmania spp., which cause the Leishmaniases. Molecular studies of cytokinesis have mainly been confined to T. b. brucei due to the availability of molecular genetic tools. While there are undoubtedly many similarities between different kinetoplastids, a variety of structural and developmental differences will likely influence cell division and its molecular control. Recent tool development (Duncan et al., 2016; Beneke et al., 2017; Gibson et al., 2017; Costa et al., 2018; Lander et al., 2019) should accelerate progress and shed light on these differences.
T. brucei and Other Animal Trypanosomes
T. brucei, T. congolense, and T. vivax have complex life cycles split between mammalian and tsetse fly hosts, comprising a series of morphologically and metabolically distinct stages. These may be replicative (bloodstream trypomastigotes in the mammal, and procyclic and epimastigote forms in the fly) or cell cycle arrested (Van Den Abbeele et al., 1999; Matthews, 2005; Gluenz et al., 2008; Rotureau et al., 2012; Capewell et al., 2016; Trindade et al., 2016; Peacock et al., 2018). Cell division has been best studied in T. brucei in the procyclic form; more limited studies in bloodstream and epimastigote forms have highlighted similarities alongside key structural/molecular differences. T. brucei is vermiform and flagellated; a corset of parallel subpellicular microtubules confer a helical axis and polarity (Hemphill et al., 1991; Robinson et al., 1995; Gull, 1999), while a single motile flagellum emerges from a flagellar pocket at the posterior of the cell and is linked laterally to the cell body by a flagellum attachment zone (FAZ) (Figure 6A) (Gull, 1999). Following organelle replication, the cytoskeleton is remodeled locally in the vicinity of the furrow site in preparation for cytokinesis, but does not globally break down (Sherwin and Gull, 1989). New subpellicular microtubules are inserted in between the duplicated FAZ microtubule quartets (Wheeler et al., 2013). The plasma membrane then invaginates between the duplicated flagella, forming a cleavage fold (Figures 6B, 7) and microtubules are remodeled at the posterior end to create nascent daughter cell tips (Wheeler et al., 2013). The cleavage furrow/cleft then initiates from the anterior end of the new FAZ (Robinson et al., 1995; Kohl et al., 2003; Zhou et al., 2011) and ingresses posteriorly (Figures 6B, 7). Significant molecular evidence (see below) suggests that there are at least two distinct stages in furrow ingression: separation of the cell bodies up to roughly the midpoint between the segregated daughter cell nuclei, and then continued ingression to cleave the nascent posterior ends. In the procyclic, but not bloodstream form, a flagellar connector holds daughter flagella together at their anterior tips until cleavage furrow/cleft formation is complete (Moreira-Leite et al., 2001; Wheeler et al., 2013). Just before abscission, the flagellar ends dissociate, allowing the cell bodies to move apart, now connected by just a thin cytoplasmic bridge containing microtubules (Figures 6B, 7A). This initially links the posterior tip of the old-flagellum daughter with the side of the new-flagellum daughter, before migrating to connect the daughter posterior tips (Figure 7A). The end of cytokinesis is morphologically distinct in the bloodstream form (Figure 7B); the cytoplasmic bridge is thicker and links the cells only at their posterior tips. In both life cycle stages, it takes a while to resolve the cytoplasmic bridge, and bloodstream form cells may undergo a second cell cycle before abscission is completed (Wheeler et al., 2013).
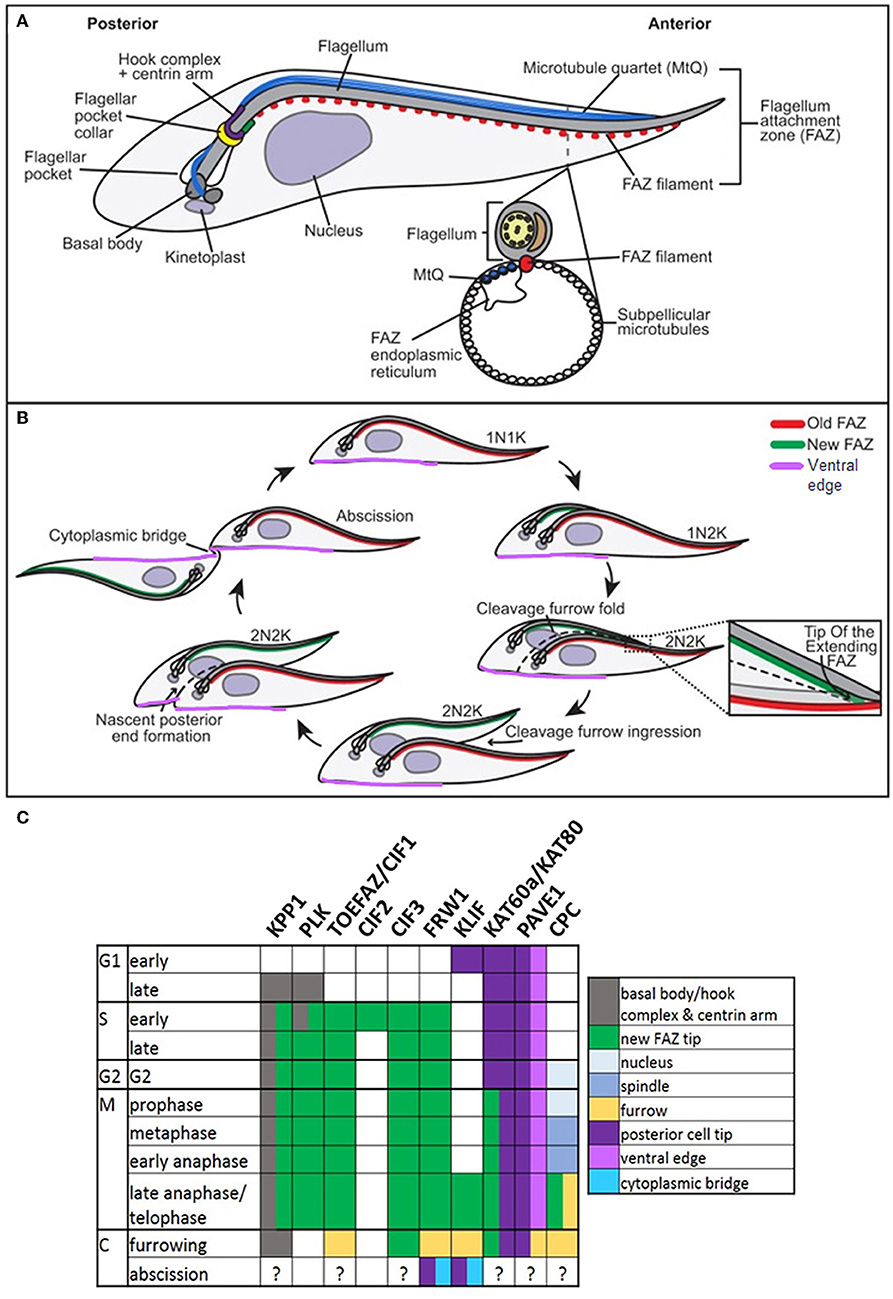
Figure 6. Cytokinesis in Trypanosoma brucei. Cartoons of cytoskeletal structures (A) and cell division (B) in procyclic T. brucei. N: nucleus; K: kinetoplast. Adapted with permission from Journal of Cell Science (Sinclair-Davis et al., 2017). (C) Table indicating the cellular localizations of key cytokinesis proteins. KPP1 and PLK co-localize throughout the cell cycle from late G1, until late anaphase, when PLK becomes undetectable. TOEFAZ1/CIF1 localizes to the Tip Of the Extending FAZ (or new FAZ tip) (highlighted in B), and acts as a scaffold for many other cytokinesis proteins, including KPP1, PLK1, CIF2, CIF3, FRW1, KLIF, KAT60a/KAT80, and PAVE1. The location of PAVE1 at the ventral edge of the old-flagellum daughter is indicated in purple in (B). The localization of the chromosomal passenger complex (CPC) is also indicated (C). Note that the localizations of many of these proteins have not been specifically determined at abscission.
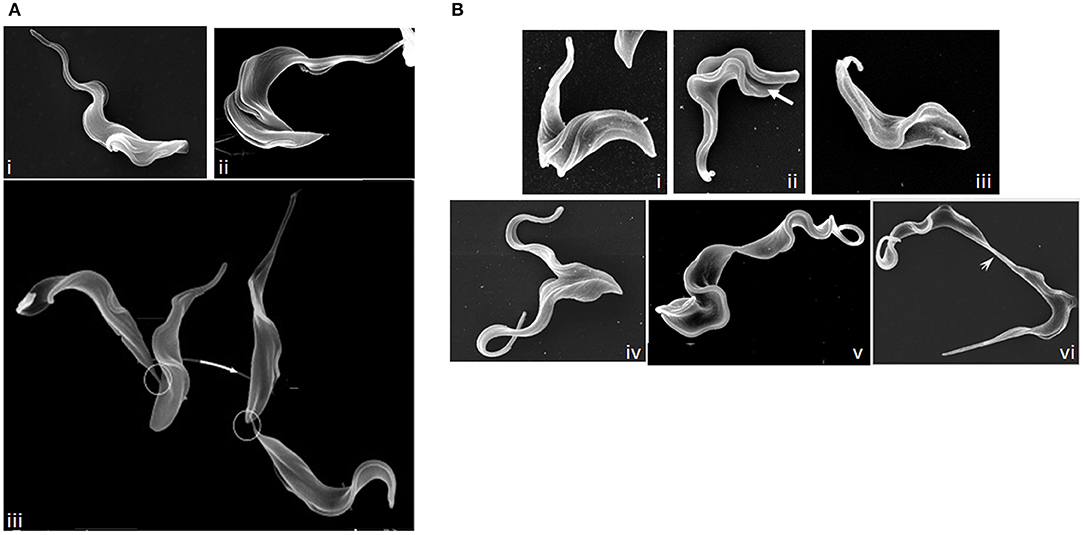
Figure 7. Images of dividing T. brucei. (A) Scanning electron microscopy (SEM) images of procyclic T. brucei undergoing division. (i) cell with two flagella but no furrow (Gull Lab of courtesy of Sue Vaughan); (ii) cell with a cleavage fold (Gull Lab courtesy of Catarina Gadelha), and (iii) cells undergoing abscission (adapted from Wheeler et al., 2013). Note the differing posterior end morphologies of the daughter cells and the migration of the intercellular bridge (circled) as cells progress through abscission. (B) SEM images of dividing bloodstream form T. brucei. (i) cell with two flagella but no furrow; (ii) formation of a cleavage fold (arrow); (iii) remodeling of the posterior ends; (iv) cleavage furrow ingression; (v) abscission; and (vi) late abscission with an extended intercellular bridge (arrowhead). Note that the intercellular bridge is thicker than in procyclic cells and does not migrate. Images (i–v) Tansy Hammarton; image (vi) adapted from Zhang et al. (2019a).
Additional cytokinesis events occur during epimastigote development in the tsetse fly; T. brucei proventricular cells undergo an asymmetric division to produce long and short epimastigote daughters (Van Den Abbeele et al., 1999); in contrast, T. congolense remodels to form epimastigotes (Peacock et al., 2018). Short epimastigotes can divide to produce two epimastigote daughters [T. brucei, T. congolense, T. vivax, and T. lewisi (a rat trypanosome transmitted by fleas)], divide asymmetrically to give epimastigote and pre-metacyclic trypomastigote daughters (T. brucei, T. vivax, and T. congolense) or interconvert to trypomastigotes (T. lewisi) (Van Den Abbeele et al., 1999; Gluenz et al., 2008; Peacock et al., 2012, 2018; Rotureau et al., 2012; Ooi et al., 2016; Zhang et al., 2019a,c). While epimastigote furrow ingression appears to progress from anterior to posterior in all species, as in bloodstream and procyclic T. brucei, there are some notable differences in abscission. In T. congolense (Figure 8A), daughter cells are rarely joined tip to tip; instead, the anterior of the new-flagellum daughter is attached to the side of the old-flagellum daughter (termed the “mother” cell in Peacock et al., 2018). Further, the mother cell rapidly divides again, while still attached to its first daughter, resulting in a rosette of three cells; daughter cell motility is thought to ensure final severing of the link to the mother cell (Peacock et al., 2018). T. lewisi epimastigotes also undergo several rounds of cell division without completing cytokinesis, rapidly forming rosettes with 5–6 cell bodies attached at their posterior ends, from which, periodically, a fully segmented cell is released (Zhang et al., 2019c).
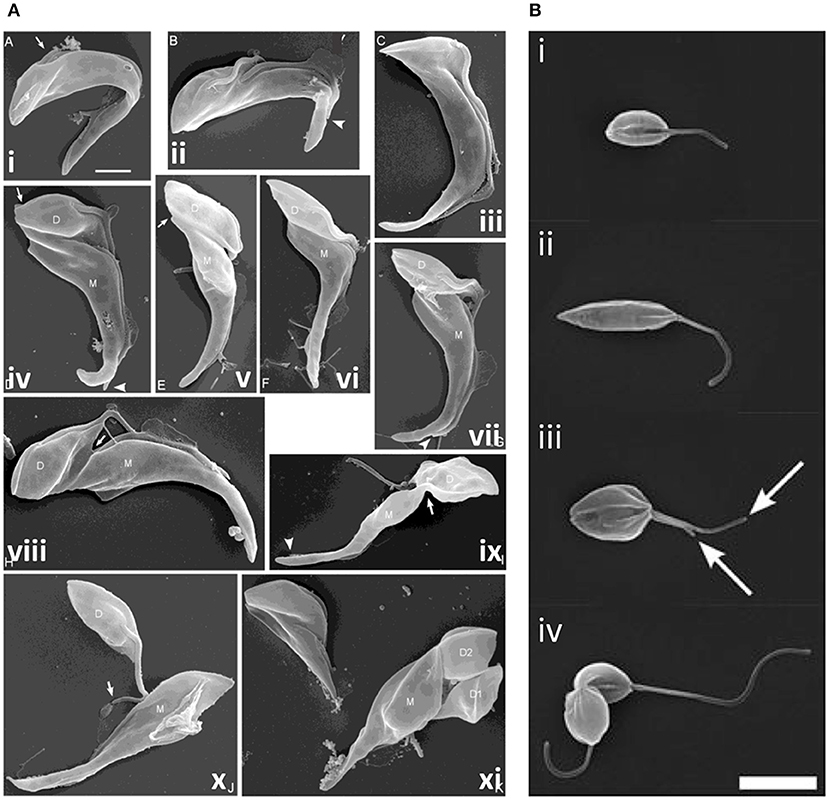
Figure 8. Cytokinesis in Trypanosoma congolense and Leishmania mexicana. (A) Scanning electron microscopy (SEM) images of T. congolense proventricular cells undergoing cell division (adapted from Peacock et al., 2018). (i) emergence of the new flagellum (arrow); (ii) extension of the new flagellum, but not to the tip of the old flagellum (arrowhead); (iii) cleavage fold formation; (iv–vii) cleavage furrow ingression and formation of nascent ends of mother (M) and daughter (D) cells (arrows). Arrowheads indicate distal tip of old flagellum. (viii) late-stage cleavage furrow ingression (arrow), pre-abscission; (ix) formation of cytoplasmic bridge (arrow); (x) completion of abscission; (xi) mother cell (M; left) with two sequentially-produced daughter cells, D1 and D2. Scale bar = 2 μm. (B) SEM images of dividing L. mexicana cells (taken from Wheeler et al., 2011). (i) Cell in early G1 phase with one flagellum; (ii) elongated cell (in later G1/ S phase); (iii) mitotic cell with two flagella (arrows); and (iv) cell in abscission. Scale bar: 5 μm.
Cytokinesis in T. brucei requires the prior duplication and segregation of various single copy organelles and structures, with FAZ duplication being critically important (Hammarton et al., 2003; Kohl et al., 2003; Chanez et al., 2006; Hall et al., 2006; Rodgers et al., 2007; Li and Wang, 2008; Barquilla and Navarro, 2009; Zhou et al., 2010). Further, flagellar motility (Branche et al., 2006; Broadhead et al., 2006; Ralston et al., 2006) and plasma membrane composition (Lillico et al., 2003; Sheader et al., 2005; Rodgers et al., 2007; Fridberg et al., 2008; Schoijet et al., 2018) are also key. Molecular regulation of cytokinesis has probably been more extensively investigated in T. b. brucei (Figure 6C) than in any other protozoan parasite, although studies have been restricted to in vitro-cultured procyclic and bloodstream forms, despite in vitro differentiation of procyclic parasites to epimastigote and metacyclic forms being possible (Kolev et al., 2012). Some significant molecular differences between the life cycle stages have been revealed, including different checkpoints. For example, inhibiting mitosis blocks cytokinesis in the bloodstream form, but not the procyclic form (Hammarton et al., 2003). Further, the anaphase-promoting complex/cyclosome (APC/C) appears to promote the metaphase to anaphase transition in procyclic cells, as in other eukaryotes, but the anaphase to telophase transition in bloodstream form cells (Kumar and Wang, 2005; Bessat et al., 2013; Bessat, 2014).
Additionally, while many canonical cell cycle regulators are present in T. brucei, their function may be divergent and they often act together with trypanosome-specific regulators. For example, the trypanosome ortholog of the multifunctional protein kinase and key mitotic regulator, polo-like kinase (TbPLK), appears to be non-essential for mitosis. Instead, it plays critical roles in basal body segregation, flagellum biogenesis, attachment and segregation, and cytokinesis (Kumar and Wang, 2006; Hammarton et al., 2007; de Graffenried et al., 2008, 2013; Li et al., 2010; Ikeda and de Graffenried, 2012; Lozano-Nunez et al., 2013). Interestingly, several kinetochore proteins contain a divergent polo-box domain suggesting that an ancestral PLK may have duplicated and functionally diverged (Nerusheva and Akiyoshi, 2016). Further, while the SCF (Skp1-Cdc53/Cullin-F-box) protein degradation complex regulates the G1/S transition in metazoans, the trypanosome F-box proteins CFB1 and CFB2, along with TbCDC34, a homolog of the E2 ubiquitin-conjugating enzyme usually associated with the SCF complex, modulate progression through bloodstream form cytokinesis (Benz and Clayton, 2007; Rojas et al., 2017). Additionally, while canonical protein kinase A (or cAMP-dependent kinase) regulates a multitude of signaling pathways in response to cAMP, TbPKA is not activated by cyclic nucleotides and is essential for cytokinesis, potentially via phosphorylation of flagellar and cytoskeletal targets and/or cell cycle proteins such as mitotic cyclin 6, the Nuclear-DBF2-related (NDR) kinase, TbPK50 and TOEFAZ1/CIF1 (see below) (Jones et al., 2014; Bachmaier et al., 2019).
The aurora kinase, TbAUK1, is vital for mitosis and progression into cytokinesis in procyclic and bloodstream T. brucei (Li and Wang, 2006; Tu et al., 2006; Li et al., 2007, 2009) as in other eukaryotes, but acts alongside two kinetoplastid-specific proteins, CPC1 and CPC2, forming a divergent chromosomal passenger complex (CPC) (Li et al., 2008a). The chromosomal passenger complex assembles at the spindle midzone together with two novel nuclear kinesins (TbKINA and TbKINB) and the tousled like kinase, TbTLK1 (Li et al., 2008a,b), translocating at late anaphase/telophase to the anterior tip of the new FAZ before traveling with the leading edge of the ingressing furrow toward the posterior cell tip (Li et al., 2008a, 2009). In the procyclic form, recruitment of the chromosomal passenger complex to the new FAZ tip relies on the scaffold protein TOEFAZ1/CIF1 (tip of extending FAZ protein 1/cytokinesis initiation factor 1). TOEFAZ1/CIF1 first appears (after being phosphorylated by TbPLK at the centrin arm or hook complex) at the new FAZ tip early in S phase, remaining there until cytokinesis, when it tracks the leading edge of the ingressing furrow (Zhou et al., 2016a). TbBOH1 (bait on hook protein 1) is required for TOEFAZ/CIF1 and PLK localization to the new FAZ tip (Pham et al., 2019). Further, TOEFAZ1/CIF1 also requires the FAZ tip localizing Protein Required for Cytokinesis, FPRC, to localize to the new FAZ tip and the cleavage furrow. Interestingly, FPRC is also found at the old FAZ tip and, in turn, requires the cytokinesis initiation factor, CIF4, to localize to the FAZ tips and the furrow (Hu et al., 2019). TOEFAZ1/CIF1 keeps TbPLK at the new FAZ tip from S phase to anaphase (Mcallaster et al., 2015; Zhou et al., 2016a), recruiting TbAUK1 to the new FAZ tip at late anaphase when TbPLK is lost from this site (Zhou et al., 2016a). Interactions with two other cytokinesis initiation factors, CIF2 and CIF3, are also important for maintaining TOEFAZ1/CIF1 localization at the new FAZ tip and for TbAUK1 recruitment (Zhou et al., 2016b; Kurasawa et al., 2018). In bloodstream form trypanosomes, CIF1-3 display similar localizations to those in the procyclic form, but their interactions with TbAUK1 and TbPLK, and the localizations of FPRC and CIF4 have not yet been investigated (Zhang et al., 2019a). Depletion of any of the CIF proteins in procyclic and/or bloodstream T. brucei affects furrow ingression, impairing its accuracy or blocking normal furrowing completely (Zhou et al., 2016a,b; Sinclair-Davis et al., 2017; Kurasawa et al., 2018; Hu et al., 2019; Zhang et al., 2019a). In procyclic, but not bloodstream form trypanosomes, CIF1, CIF2, CIF4, or FPRC depletion has been reported to result in furrow ingression occurring in the opposite direction, from the posterior to the anterior, which was interpreted as indicating a backup cytokinesis pathway was turned on (Zhou et al., 2016a,b; Hu et al., 2019; Zhang et al., 2019a). However, why such a pathway should operate, and in only one life cycle stage, is unclear. An alternative explanation is that the “posterior-anterior furrowing” instead reflects continued normal nascent daughter cell posterior end remodeling (Wheeler et al., 2013) in the absence of furrow ingression, as previously observed following FAZ10 or katanin depletion (Benz et al., 2012; Moreira et al., 2017), with continued flagellar motility acting to pull apart the nascent posterior ends (Sinclair-Davis et al., 2017). Intriguingly, one further difference between bloodstream and procyclic trypanosomes, is that a flagellar axonemal inner-arm dynein complex, comprising dynein heavy chain ortholog TbIAD5-1 and TbCentrin3,is essential for CIF1-3 localization, and therefore, for cytokinesis in the bloodstream form, but not in the procyclic form (Zhang et al., 2019b). However, how an axonemal complex is able to modulate the localization of cytokinesis proteins at the new FAZ tip, and why this only occurs in one life cycle stage, is currently unclear.
In addition to recruiting TbPLK and TbAUK1 to the new FAZ tip, BioID studies in procyclic T. brucei show that TOEFAZ1/CIF1 interacts with, or is a near neighbor of, dozens of other proteins, including FAZ and microtubule–associated proteins (Zhou et al., 2016b, 2018a; Hilton et al., 2018). Many interactors are cytokinesis proteins, and TOEFAZ1/CIF1 is required for at least some of these to localize to the new FAZ tip (Figure 6C). For example, the kinetoplastid-specific protein phosphatase, KPP1, colocalizes with TbPLK throughout the cell cycle and with TOEFAZ1/CIF1 at the new FAZ tip (Zhou et al., 2016b; Hilton et al., 2018). KPP1 depletion substantially depleted TOEFAZ1/CIF1 and TbPLK and/or prevented TbPLK localization to the new FAZ tip (Hilton et al., 2018; Zhou et al., 2018b) and resulted in defects in duplicating and segregating flagellum-associated cytoskeletal structures (Zhou et al., 2018b). Post-mitotic cells with prematurely detached flagellar connectors accumulated, and cytokinesis was delayed or inaccurate. Increased phosphorylation of TbPLK substrate Centrin2 (de Graffenried et al., 2013) was also observed, suggesting that KPP1 opposes TbPLK action, either by dephosphorylating activatory T loop phosphorylation of TbPLK, or by dephosphorylating TbPLK substrates (Zhou et al., 2018a).
TOEFAZ1/CIF1 also localizes the kinesin, KLIF (Kinesin Localized to the Ingressing Furrow) to the FAZ tip and furrow (Hilton et al., 2018; Zhou et al., 2018a; Zhang et al., 2019a). KLIF depletion inhibits the latter stages of procyclic furrowing, but although it slows bloodstream form proliferation, it does not discernibly affect cytokinesis (Zhang et al., 2019a). The coiled coil protein, FRW1, also interacts with TOEFAZ1/CIF1, localizing to the FAZ tip, ingressing furrow, and the posterior cell tips and/or cytoplasmic bridge at abscission in procyclic trypanosomes, but exhibits a punctate localization in the middle of bloodstream form cells (Zhang et al., 2019a). Surprisingly, in the procyclic form, almost total FRW1 depletion did not affect proliferation, suggesting it may be non-essential for cytokinesis (Crozier et al., 2018; Zhou et al., 2018a), while in the bloodstream form, just moderate FRW1 depletion rapidly inhibited cytokinesis initiation (Zhang et al., 2019a).
TOEFAZ1/CIF1 also interacts with cytoskeleton remodeling proteins, including the katanin subunit, KAT80, and a coiled coil protein, PAVE1 (Posterior and Ventral Edge Protein 1). KAT80 and its associated KAT60 subunits are required for furrow ingression in procyclic and bloodstream trypanosomes, most likely due to a role in severing and remodeling microtubules (Casanova et al., 2009; Benz et al., 2012; Zhou et al., 2018a). PAVE1 is found at the posterior end of procyclic cells (and at both daughter cell ends in dividing cells), enriched on the ventral side, and also localizes to the cleavage fold and ingressing furrow (where it colocalizes with TOEFAZ1/CIF1). It is required for the tapering of daughter cell posterior ends (Hilton et al., 2018). Two translationally controlled tumor protein (TCTP) paralogues may also play a role in posterior end remodeling during procyclic form cytokinesis (Jojic et al., 2018a) but appear to be required for cytokinesis initiation in bloodstream form trypanosomes (Jojic et al., 2018b). The cytoskeleton-associated protein, AIR9, and the giant FAZ intermembrane staple protein, FAZ10, are also putative TOEFAZ1/CIF1 interactors (Hilton et al., 2018; Zhou et al., 2018a). AIR9 regulates posterior end microtubule extension and nucleus positioning in procyclic trypanosomes, and controls cleavage furrow placement accuracy in the bloodstream form (May et al., 2012), while FAZ10 plays roles in cell morphogenesis, flagellum attachment, kinetoplast and nucleus positioning, and optimal timing and placement of the cleavage furrow in both forms (Moreira et al., 2017).
TOEFAZ1/CIF1 therefore appears to be a critical scaffolding protein that dynamically interacts with many proteins required to signal and effect cytokinesis, thereby integrating the structural pre-requisites of cytokinesis (e.g., duplicating the flagellum and its associated structures/organelles) with the signaling molecules that coordinate cell division and the cytoskeleton remodeling proteins that effect it.
In addition to TOEFAZ1/CIF1 and its binding partners, most of which localize to structures (new FAZ tip, ingressing furrow, posterior cell tip) consistent with their cytokinesis function, there are various other essential cytokinesis proteins that are cytoplasmic. These seem to play specific and direct roles in cytokinesis, since their depletion and/or overexpression specifically affects cytokinesis without affecting earlier cell cycle events such as basal body segregation, which would have knock-on effects on cytokinesis. However, it is likely that these proteins operate upstream of, or perhaps in different pathways from, TOEFAZ1/CIF1. For example, the essential trypanosome receptor for activated C kinase (TRACK) scaffold protein, is critical for cytokinesis, with its depletion arresting procyclic cells mid-furrow (similar to KLIF depletion) and bloodstream parasites prior to furrow ingression (Rothberg et al., 2006). TRACK interacts (at least in vitro) with Rho-related protein, TbRHP, which is also required for progression through cytokinesis in both procyclic and bloodstream T. brucei (Abbasi et al., 2011). Both proteins mainly localize to the cytosol, where TRACK associates with EF1α in monosomes and polysomes; TRACK depletion reduces translation efficiency and renders trypanosomes hypersensitive to the translational inhibitor, anisomycin, suggesting that ongoing translation is required to complete cytokinesis (Regmi et al., 2008). Further, the cytosolic NDR kinases, PK50 and PK53, appear to regulate furrowing initiation and the latter stages of furrow ingression, respectively, in bloodstream form trypanosomes (Ma et al., 2010). While NDR kinases in other organisms are activated by a MOB (Mps One Binder) binding partner, and trypanosome MOB1 proteins are essential for the accuracy (procyclic) and completion (bloodstream form) of furrowing (Hammarton et al., 2005), PK50 and PK53 are MOB1-independent (Ma et al., 2010). Consistent with this, NDR kinases were reported as potential interactors of TOEFAZ1/CIF1, while the also cytosolic MOB1 proteins were not (Hilton et al., 2018). The cytosolic GTPase, ARL2, which regulates α-tubulin acetylation, and ESAG4/ESAG4-like adenylyl cyclases are also required for the latter stages of furrow ingression in the bloodstream form (Price et al., 2010; Salmon et al., 2012), and phosphodiesterases (PDEs) may promote abscission (de Koning et al., 2012).
T. cruzi
T. cruzi cells differ structurally from T. brucei and Leishmania since they possess an endo/exocytic cytostome-cytopharynx complex, associated with a triplet of microtubules running from beneath the cytostome membrane to the cell posterior and a microtubule quartet linking the cytostome with the flagellar pocket. The cytostome-cytopharynx complex and the microtubule triplet disassemble during G2 phase and reassemble during cytokinesis, guided by the cytostome microtubule quartet (Alcantara et al., 2017). However, while there are additional cytoskeleton components and the order of organelle replication differs in T. cruzi compared to T. brucei (Elias et al., 2007), the overall process of cytokinesis appears similar, with a furrow ingressing from the anterior to the posterior. Little is known about the molecular regulation of T. cruzi cytokinesis, although colchicine, which blocks microtubule polymerization, the protein phosphatase 1 (PP1)-inhibitor, calyculin, and the Casein Kinase 2 (CK2) inhibitor, emodin, inhibit epimastigote cytokinesis (Orr et al., 2000; Potenza and Tellez-Inon, 2015; De Lima et al., 2017). However, T. cruzi AUK1 is apparently not required for cytokinesis (Fassolari and Alonso, 2019).
Leishmania spp.
Leishmania cell division differs from that of T. brucei in several ways. G2 phase is much briefer and the order of nucleus and kinetoplast division varies according to the species; some species use more than one route to divide (Ambit et al., 2011; Minocha et al., 2011; Wheeler et al., 2011). Further, in promastigotes, the flagellum continues to grow from one cell cycle to the next and significant morphological changes occur during the cycle, with cells elongating during G1 phase and then shrinking and broadening during mitosis, prior to cytokinesis. A cleavage cleft forms along the longitudinal axis of the cell, with the furrow progressing from the anterior to the posterior with bilateral symmetry (Figure 8B). Abscission then occurs, but only in 90% cells in L. mexicana; the remaining 10% re-enter the cell cycle, replicating as doublets (Wheeler et al., 2011). Little is known about cytokinesis regulators in Leishmania promastigotes; only Aurora kinase has been linked to a possible role in cytokinesis, in addition to its roles in mitosis (Chhajer et al., 2016). However, actin dynamics are important, influencing flagellar pocket division, basal body/kinetoplast segregation and vesicular movement, with defects in these processes in turn inhibiting cytokinesis furrowing (Tammana et al., 2010). Further, several microtubule-severing agents localize to the cleavage furrow (LmjKAT106 and LmjKAT80) or midbody (LmjFID), suggesting that they sever microtubules during cytokinesis (Casanova et al., 2009). However, Leishmania do not have a spastin ortholog, highlighting differences in microtubule severing compared to T. brucei.
In contrast to promastigotes, Leishmania amastigotes are intracellular and morphologically rounded with only a vestigial flagellum. KHARON, a kinetoplastid-specific protein, involved in trafficking flagellar membrane proteins, is important for cytokinesis specifically in amastigotes grown in macrophages; KHARON null mutants are viable as promastigotes and axenic amastigotes, but exhibit flagellum and morphological aberrations and arrest in cytokinesis in macrophages (Tran et al., 2013, 2015; Santi et al., 2018). Similarly, knockout of a centrin gene in L. donovani, was only lethal in amastigotes but not promastigotes, demonstrating failure of basal body duplication and cytokinesis (Selvapandiyan et al., 2004).
Cytokinesis in the Alveolata
Tetrahymena spp. and Balantidium coli (Phylum Ciliophora): Hippo Pathway Signaling, Microtubule Remodeling, and Ciliary Beating Regulate Tandem Duplication
Ciliates are structurally complex, with micro-and macronuclei, an oral (feeding) apparatus, contractile vacuole pores, a cytoproct (a site of exocytosis and membrane recycling), and an intricate polar array of surface cilia. Cilia align in longitudinal rows alongside longitudinal microtubule bundles, and their basal bodies are associated with transverse and postciliary bands of microtubules. Most ciliates are free-living and non-pathogenic. However, certain Tetrahymena species infect fish, insects or dogs (Jerome et al., 1996; Lynn et al., 2000; Chettri et al., 2009) and Ichthyophthirius multifiliis infects freshwater fish (Coyne et al., 2011), while Balantidium coli, the largest known protozoan (25–200 μm), commonly found in the pig intestine, is the only known human-infective ciliate, causing balantidiasis (Schuster and Ramirez-Avila, 2008). Ciliates divide by transverse binary fission (Figure 9A). Following tandem duplication of the cortex, a furrow forms at the midpoint of the cell's anterior-posterior axis, constricting due to the presence of a divergent contractile ring. The most detailed studies of ciliate cell division have been performed in Tetrahymena spp., although other work indicates that Nassula (Tucker, 1971) and Paramecium (Jurand and Selman, 1969) also use a non-myosin II contractile ring to divide. Despite B. coli being a human pathogen, its cell division has been little studied, perhaps due to difficulties with its laboratory culture (Schuster and Ramirez-Avila, 2008; Nilles-Bije and Rivera, 2010).
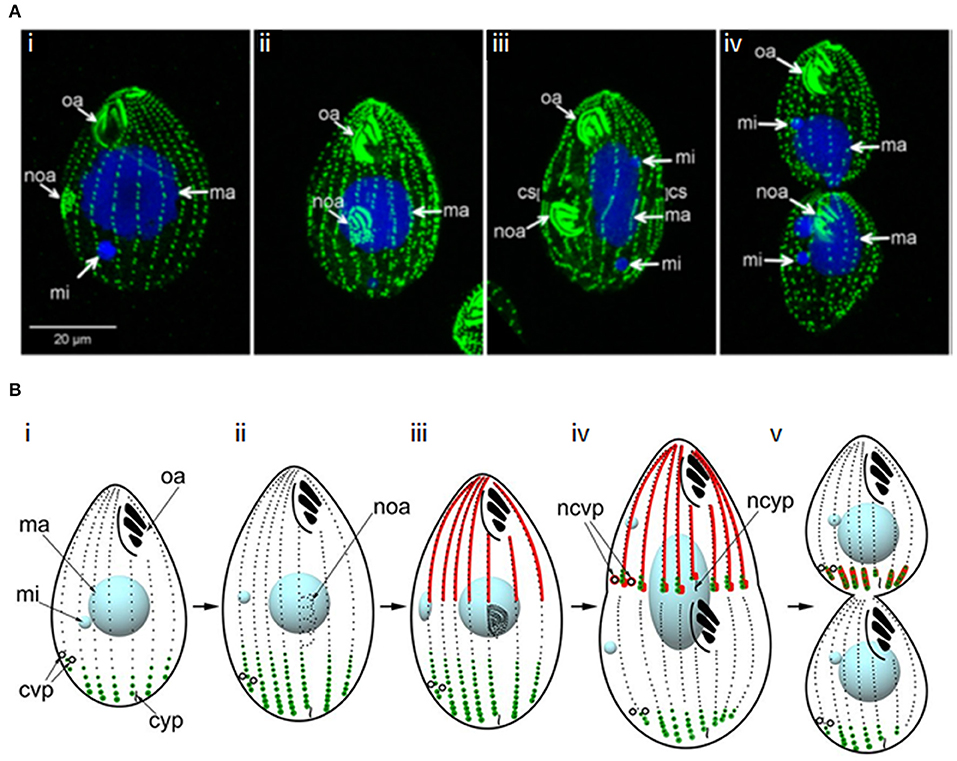
Figure 9. Cytokinesis in Tetrahymena. (A) Immunofluorescence images of a dividing Tetrahymena cell. Basal bodies are labeled with anti-centrin 205H antibody (green); DNA is stained with DAPI (blue); oa: oral apparatus; noa: new oral apparatus; mi: micronucleus; ma: macronucleus; cs: cortical subdivision. (i,ii) early stages of cell division highlighting development of the new oral apparatus; (iii) early stage of amitosis (elongated macronucleus)—the micronuclei have already completed mitosis and the cortical subdivision is visible; (iv) late cytokinesis–amitosis has completed. Adapted and republished with permission of Genetics Society of America from Jiang et al. (2017); permission conveyed through Copyright Clearance Center, Inc. (B) Schematic of the determination of cleavage site positioning by the localisations of two Hippo-kinases, Elo1 (green) and Cda1 (red), which sequentially inhibit segmentation at the posterior and anterior of the dividing cell, respectively. Mob1 shares an identical localization with Elo1, suggesting it might activate Elo1. However, it also colocalizes with Cda1 at the posterior end of the anterior daughter cell, with Mob1 depletion phenocopying Cda1 depletion, suggesting it may operate in both pathways. (i) cell in interphase; (ii, iii) early and late in the development of the new oral apparatus; (iv) early amitosis; (v) late cytokinesis. cvp, contractile vacuole pore; ncvp, new contractile vacuole pore; cyp, cytoproct; ncyp, new cytoproct. Adapted and republished with permission of Genetics Society of America from Jiang et al. (2019); permission conveyed through Copyright Clearance Center, Inc.
Tetrahymena replication begins with basal body duplication and elongation of the longitudinal ciliary rows, following which a new oral apparatus is constructed in the posterior half of the cell. Mitosis of the micronucleus follows and a gap (the cortical subdivision) appears in the ciliary rows just anterior to the new oral apparatus, demarcating the newly-forming daughter cells. This region is free from cilia, basal bodies and their associated microtubules, but initially, longitudinal microtubule bundles remain. The macronucleus then divides amitotically, with the two resultant macronuclei being positioned either side of the cortical subdivision. Furrow ingression follows, with the concomitant severing of longitudinal microtubule bundles, resulting in the formation of new cortical ends; new contractile vacuole pores and cytoproct are constructed at the posterior end of the anterior daughter cell. To complete cytokinesis, a thin residual cytoplasmic bridge between the daughter cells must be cleaved; opposing rotational forces exerted by ciliary beating (“rotokinesis”) may contribute to this (Brown et al., 1999a).
The cortical subdivision is placed at the cell's midpoint by the joint actions of two opposing Hippo kinase signaling pathways (Figure 9B). The Hippo/MST2-like kinase, CDA1, and its putative binding partner and substrate, MOB1, exclude divisional activities in the anterior half of the cell, while a second Hippo kinase, ELO1, prevents division in the posterior half (Tavares et al., 2012; Jiang et al., 2017, 2019). Beneath the cortex at the division site, is a contractile ring containing actin, but no myosin II or septins (Yasuda et al., 1980; Hirono et al., 1987; Wloga et al., 2008). However, actin appears to play an indirect role in cytokinesis. It is found at multiple cellular sites in Tetrahymena and is required for phagocytosis and motility, leading to secondary effects on cytokinesis (Ohba et al., 1992; Brown et al., 1999a,b; Hosein et al., 2003; Williams et al., 2006). Indeed, inhibiting actin polymerization with Lantrunculin A does not prevent cytokinesis (Shimizu et al., 2013) and the actin-modulator, actin-depolymerization factor (ADF)/cofilin is not required for cytokinesis (Shiozaki et al., 2013). However, a number of actin-binding proteins localize to the division site (Edamatsu et al., 1992; Numata et al., 2000; Numata and Gonda, 2001; Shirayama and Numata, 2003), and some have been shown to be important for cytokinesis (Numata and Gonda, 2001; Wilkes and Otto, 2003). Further, repetitive protein p85 localizes to the furrow in a calmodulin/calcium-dependent manner; inhibition of this interaction inhibits contractile ring formation and furrowing (Gonda et al., 1999; Numata et al., 1999).
Tubulin dynamics and modifications also seem to be important for Tetrahymena cytokinesis. Polyglycylated β-tubulin is found in cilia, and at a lower level, in all cortical microtubules. Reduced polyglycylation results in ciliary paralysis (due to absence of the central microtubule pair and other microtubule defects) (Xia et al., 2000; Thazhath et al., 2002) and chains of incompletely separated daughter cells. While a lack of ciliary beat can inhibit cytokinesis completion (e.g., as in kinesin-II null mutants) (Brown et al., 1999b), other defects (disrupted cortical rows, and longitudinal microtubule bundles that continued to grow into the fission area or were not severed) were noted, suggesting an additional physical inhibition of furrowing (Thazhath et al., 2002). Katanin null mutants phenocopy β-tubulin mutations that prevent polyglycylation (Thazhath et al., 2002), suggesting katanin cleaves longitudinal microtubule bundles at the division site (Sharma et al., 2007).
The Apicomplexa–Multiple Modes of Budding and/or Hijacking of Host Cell Mitotic Machinery
Apicomplexans comprise four major clades: the Coccidia, Plasmodia, Piroplasmida, and Cryptosporidia, and encompass a number of important human/animal pathogens. They infect a wide range of host cells, mostly residing within a parasitophorous vacuole. The apicomplexan cell (reviewed in Morrissette and Sibley, 2002a) is surrounded by a pellicle, comprised of the plasma membrane and the inner membrane complex (IMC), with apical and basal complexes at either end. The inner membrane complex consists of flattened alveoli associated with cytoskeletal elements including the actin-myosin motor complex (required for motility and invasion) and subpellicular microtubules (Dubremetz and Elsner, 1979; Adams and Todd, 1984; Kono et al., 2012; Dubey et al., 2017). The apical complex has roles in secretion and invasion, and its apical polar ring acts as an MTOC (Hu et al., 2002b), while the basal complex is a mobile and contractile proteinaceous ring important for mitosis and cytokinesis (Gubbels et al., 2006; Hu et al., 2006; Hu, 2008). Apicomplexans also contain a nucleus surrounded by endoplasmic reticulum, a mitochondrion, a Golgi, an apicoplast (a vestigial, non-photosynthetic plastid unique to this phylum), and two further MTOCs: the centrioles or centriolar plaque, and the basal bodies (Francia et al., 2015). In coccidians, two parallel centrioles form a centrosome, which is associated with the centrocone, a specialized invagination of the nuclear envelope that anchors the intranuclear mitotic spindle and plays key regulatory roles in budding (Sheffield and Melton, 1968). Plasmodia instead have a centriolar plaque within the nuclear envelope that nucleates the spindle microtubules (Francia and Striepen, 2014). Basal bodies are formed solely in male gametes, the only flagellated life cycle stage.
Apicomplexans display great flexibility in cell division (Francia and Striepen, 2014), exhibiting a vast range of proliferative scale, with a single parasite replicating to form just two or up to tens of thousands of daughters, depending on the species, life cycle stage and host. The apicomplexan cell cycle comprises just three main phases: G1, S, and M; G2 phase is very brief or absent (Radke et al., 2001). Some daughter cell components (e.g., centrosome, Golgi, nucleus, apicoplast, mitochondrion) arise through the duplication and division of mother cell organelles, while others (e.g., apical and basal complexes and inner membrane complex/cytoskeleton) are assembled de novo (Nishi et al., 2008). Apicomplexans divide by budding, assembling daughter cells either deep inside or at the plasma membrane of the mother cell. The appearance of the daughter inner membrane complex scaffolds in late S phase marks the start of budding (cytokinesis), and following completion of daughter cell assembly, daughters are cleaved from each other at the basal end via constriction of the basal complex (abscission) (Hu, 2008), and egress from the mother cell, acquiring her plasma membrane on the way (Hu et al., 2002a). The remaining mother cell components either disassemble during cytokinesis or are assimilated into a residual body after abscission (Hu et al., 2002a).
Depending on the species and their host, apicomplexans may suspend cytokinesis (undergoing just a nuclear cycle) and sometimes also mitosis, to achieve rapid successive rounds of DNA replication, before a final (usually synchronous) budding cycle assembles multiple daughters (Figure 10). For example, T. gondii tachyzoites usually replicate by endodyogeny, assembling just two daughter cells inside each mother cell (Figure 10A) (Sheffield and Melton, 1968; Hu et al., 2004), but employ endopolygeny within their definitive host, the cat (Ferguson et al., 2008). Endopolygeny involves multiple cycles of S/M phases in the absence of budding, forming a multinucleate cell, before a single synchronized round of mitosis and budding occurs deep within the mother cell. This is very similar to schizogony that is employed by Eimeria and Plasmodium spp. (Figure 10B); again, repeated rounds of S/M phases occur without budding, followed by a final round of division that ends with synchronous budding, but at the mother cell surface (Ferguson et al., 2008). A variation of endopolygeny occurs in Sarcocystis spp., whereby the nucleus undergoes multiple (five in S. neurona) rounds of S phase without mitosis, generating a large polyploid (32N) nucleus (Figure 10C). Multiple intranuclear mitotic spindles are assembled during S phase, which although always present, shrink back to mini spindles during interphase, allowing chromosome segregation/organization during each round of S phase, but without nuclear division. A final round of division proceeds (64N), culminating in a mass, synchronized karyokinesis and budding event yielding 64 haploid daughter cells (Vaishnava et al., 2005). In contrast to most apicomplexans, which exit their host cell following division, Theileria remains intracellular, exploiting host mitotic machinery to segregate between host cells during host cell division (Figure 10D) (von Schubert et al., 2010).
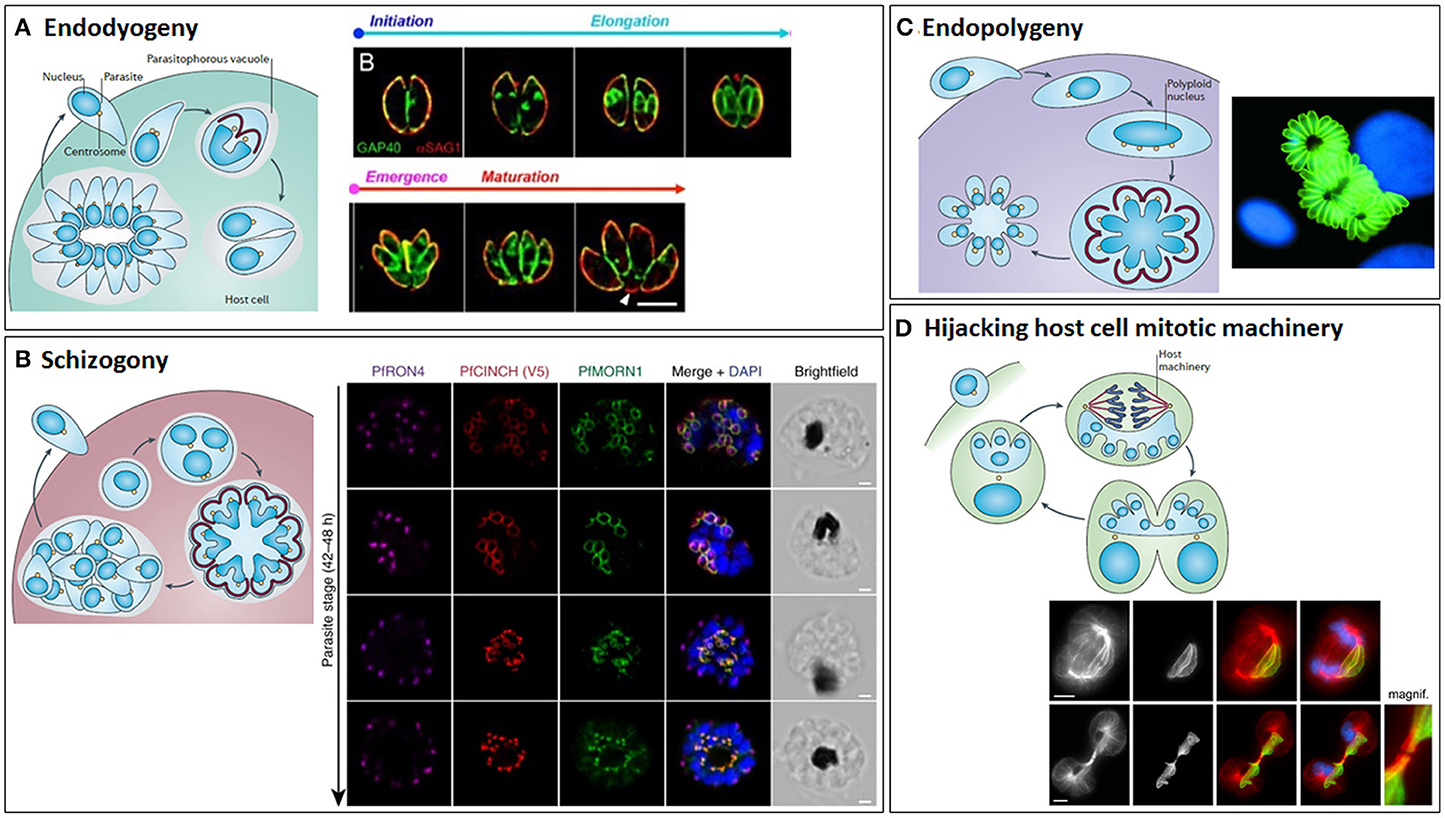
Figure 10. The different modes of cytokinesis in Apicomplexans. Apicomplexans may divide by endodyogeny (A), schizogony (B), endopolygeny (C), or hijacking the host mitotic machinery (D). Schematics of each mode of division [reprinted by permission from Springer Nature© (Francia and Striepen, 2014)] are shown alongside corresponding fluorescent microscope images. (A) Endodyogeny, employed by T. gondii tachyzoites, is the simplest form of cell division, where just two daughter cells are constructed inside each mother cell within the parasitophorous vacuole. Daughter cells egress from the mother cell, but remain within the parasitophorous vacuole and undergo geometric expansion via further synchronous rounds of endodyogeny, generating rosettes of parasites connected by tubules radiating from the residual body. Subsequently, the replicated parasites lyse the parasitophorous vacuole membrane and egress from the host cell. Images show Toxoplasma gondii tachyzoites undergoing endodyogeny. Green: integral membrane protein, GAP40; red: plasma membrane protein, SAG1. The initiation and elongation of the daughter cell membrane can be seen, followed by emergence of the daughter parasites from their mothers and their subsequent maturation. Scale bar: 5 μm. Adapted from (Ouologuem and Roos, 2014). (B) Schizogony (employed by Plasmodium spp. in their mammalian hosts, Eimeria spp. in host intestinal cells and Babesia spp. in nucleated blood cells) involves repeated rounds of DNA replication and mitosis in the absence of budding, followed by a final round of division culminating in a synchronous budding event at the surface of the mother cell. Again, this is followed by lysis of the parasitophorous vacuole and egress from the host cell. Images taken from timecourse of segmentation in Plasmodium falciparum. Airyscan superresolution microscopy of a segmenting schizont showing inner membrane complex (PfMORN1, green) and basal complex assembly (PrCINCH, red) alongside staining for the rhoptry neck protein, PfRON4 (magenta), and DNA (DAPI, blue). Note the widening of the basal complex ring as it extends past the nucleus and then its tapering as it reaches the basal end of the parasite. Scale bars: 1 μm. Taken from Rudlaff et al. (2019). Note that T. gondii utilizes endopolygeny to divide within the cat host, which is similar to schizogony, involving repeated rounds of S/M phases, but followed by a final round of cell division and synchronous budding deep within the mother cell. (C) Sarcocystis spp. divide by a variant of endopolygeny, involving multiple rounds of S phase in the absence of mitosis, generating a giant polyploid nucleus, prior to a final synchronous round of cell division with mitosis and budding. In some cases, the merozoites produced remain within the host cells and undergo additional rounds of division, this time asynchronously. Image shows schizonts of Sarcocystis neurona (green) (Daniel K. Howe). (D) Theileria spp. (and some Eimeria spp.) utilize the host cell's mitotic machinery to divide, with parasite schizonts associating with host centrosomes and spindle microtubules such that they span the metaphase plate and become trapped within the midbody, ensuring cleavage of the schizont and segregation to each host daughter cell at abscission. Theileria schizonts may also undergo merogony (as Babesia spp. and Cryptosporidium also do in red blood cells and epithelial cells, respectively) where they are packaged into individual merozoites that are released into the bloodstream and invade erythrocytes. Images show the segregation of a Theileria annulata schizont, highlighting its association with the host mitotic spindle microtubules (top) and cytoplasmic bridge/midbody (bottom). Left to right: anti-α-tubulin, anti-Theileria annulata surface protein 1 (TaSP1), anti-α-tubulin (red)/anti-TaSP1 (green) merge, anti-α-tubulin/anti-TaSP1/DAPI (blue) merge. Scale bars: 5 μm. Taken from von Schubert et al. (2010).
The Coccidia—Toxoplasma gondii, Sarcocystis neurona, and Eimeria spp.
The majority of apicomplexan cell division studies have been performed in T. gondii tachyzoites, which cause Toxoplasmosis, since they are experimentally tractable and divide by endodyogeny, the simplest form of apicomplexan division. Multiple rounds of daughter cell assembly occur synchronously within a single parasitophorus vacuole, forming rosettes, before the daughters lytically egress from the host cell. During S phase, a TgMORN1 (membrane occupation and recognition nexus repeat protein 1) ring forms around duplicated centrioles to initiate basal complex assembly followed by assembly of the conoids and apical rings, the inner membrane complex and subpellicular microtubules (Hu et al., 2002a, 2006; Gubbels et al., 2006; Ferguson et al., 2008; Hu, 2008; Nishi et al., 2008). Centrosome splitting is crucial for daughter cell construction; if centrosomes are replicated but not segregated, only a single daughter scaffold is assembled (Chen and Gubbels, 2013). A fiber of striated fiber assemblin (SFA) proteins then extends from each centrosome, which is essential to position the daughter apical complexes (Francia et al., 2012). As the inner membrane complex extends during the rest of the cell cycle, the basal complex migrates distally, enveloping the replicated and segregated organelles, widening to migrate over the daughter cell nucleus post-mitosis, and then undergoing centrin-mediated contraction to form the tapered basal end (Hu et al., 2002a; Hu, 2008; Nishi et al., 2008). However, all daughter cells in a rosette remain connected within the parasitophorus vacuole by tubules radiating from the residual body that not only contribute to the spatial organization of the rosette (Muniz-Hernandez et al., 2011), but ensure that daughters share cytoplasm until they egress from the host cell (Frenal et al., 2017). Further constriction of the basal complex occurs post-emergence from the mother cell (Hu, 2008), and this, or alternatively, degradation or recycling of mother cell material (Ouologuem and Roos, 2014) and/or mechanical stress from daughter cell motility as they egress from the mother (Lorestani et al., 2010) has been proposed to lead to completion of abscission. Following emergence, the daughter cell inner membrane complexes continue to mature; proteolytic processing of the TgIMC1 protein confers increased stability to the inner membrane complexes (Mann et al., 2002) and the inner membrane complexes expand with the incorporation of maternal inner membrane complex proteins recycled from the residual body (Ouologuem and Roos, 2014).
The Toxoplasma cytoskeleton is thus critical for cell division. Active microtubule polymerization is essential for mitosis and inner membrane complex elongation during budding (Shaw et al., 2000; Morrissette and Sibley, 2002b). Further, many other cytoskeletal and cytoskeleton-associated proteins display dynamic and hierarchical localizations during inner membrane complex deposition in daughter cells (Beck et al., 2010; Anderson-White et al., 2011; Chen et al., 2015, 2017), although only ISP2 has been shown to be required for cell division (Beck et al., 2010). Actin, Formin-2 (TgFRM2) and various non-myosin II myosins are also important for Toxoplasma cell division, with roles in centrosome positioning, apicoplast segregation, turnover of mother cell organelles during daughter cell budding, and residual body formation (Shaw et al., 2000; Andenmatten et al., 2013; Jacot et al., 2013; Frenal et al., 2017; Stortz et al., 2019). Additionally, actin has been proposed to work in concert with the class XXIII (VI-like) myosin, TgMyoJ, and TgCentrin2 to bring about basal complex constriction, although a direct interaction between these proteins has not yet been demonstrated (Frenal et al., 2017). Another myosin, the class XIV TgMyoC, also localizes to the basal complex, and overexpression of its splice variant, TgMyoB, causes defects in cytokinesis (Delbac et al., 2001). Finally, TgMORN1 localizes to the basal complex (as well as the apical ring and the centrocone) and is important for cytokinesis (Gubbels et al., 2006; Hu et al., 2006), with its role likely conserved across the Apicomplexa (Ferguson et al., 2008). Overexpression of TgMORN1 results in Toxoplasma parasites being unable to duplicate their centrocone, segregate their nucleus or to bud (Gubbels et al., 2006), while TgMORN1 knockout affects basal complex organization, Golgi replication, apicoplast segregation, and cytokinesis (Heaslip et al., 2010; Lorestani et al., 2010). A haloacid dehalogenase (HAD) phosphatase, TgHAD2a, which interacts with TgMORN1 at the basal complex, is also required for correct basal complex organization and its constriction at cytokinesis (Engelberg et al., 2016).
Various Toxoplasma cell cycle regulators have been described. Apicomplexans use a “just in time” approach to gene transcription, with two main waves of transcription: growth and house-keeping genes are transcribed in G1 phase, while daughter cell assembly and cell division genes are transcribed in S phase (Behnke et al., 2010; Gaji et al., 2011). Ten Crks have been identified, along with seven cyclins (Cycs) from the L, P, H, and Y classes, but these are rather atypical, with expression of some Crks (TgCrk4, TgCrk5, and TgCrk6), but only one cyclin (TgCycY) oscillating through the cell cycle (Alvarez and Suvorova, 2017). Only one Crk, TgCrk1, has been shown to date to regulate cytokinesis, although depletion of TgCrk4 or TgCrk6, which regulate centrosome duplication and mitosis, respectively, also has downstream effects on budding (Alvarez and Suvorova, 2017). TgCrk1 is a Cdk11 family member, whose orthologs regulate mRNA synthesis and maturation; it is localized in the nucleus and, along with its partner, CycL, is required for daughter bud formation, suggesting perhaps that this CDK complex regulates the S phase wave of transcription/splicing. Depletion of TgCrk11 or TgCycL results in improperly formed daughter cell TgMORN1 rings, leading to defective basal complexes, disorganized inner membrane complex scaffolds and deformed apical ends (Alvarez and Suvorova, 2017).
Two Aurora family kinases, TgArk1 and TgArk3, also play important roles in Toxoplasma cell division (Suvorova et al., 2015; Berry et al., 2016, 2018). Dominant negative mutants of chromosomal passenger complex kinase TgArk1 result in an early mitotic block and a knock-on effect on cytokinesis. A “Russian doll” phenotype ensues, whereby multiple rounds of centrosome duplication and budding occur, but budding does not complete due to the block in mitosis, and multiple layers of incomplete inner membrane complexes form inside each other (Berry et al., 2018). TgArk3 [confusingly, originally named TgArk1 (Suvorova et al., 2015)] is first expressed in S phase and localizes to the outer centrosome core, and later, during mitosis/cytokinesis, also localizes to one side of the forming daughter cytoskeletons (Suvorova et al., 2015; Berry et al., 2016). TgArk3-depleted parasites undergo karyokinesis, but show an impairment in daughter cell budding, resulting in reduced replication rates, correlating with the known role of the centrosome outer core in controlling budding (see below). TgArk3-depletion also results in shorter daughter parasites, defective rosette formation and impaired invasion and virulence (Berry et al., 2016). Further cell cycle regulators undoubtedly remain to be identified. A forward genetics chemical mutagenesis screen isolated fifty temperature sensitive growth mutants with defects in budding, accompanied or not by defects in karyokinesis (Gubbels et al., 2008). However, only some of the genes affected in the mutants are known and few [e.g., Nek1 (mutant V-A15), see below] have been studied in detail.
The Toxoplasma centrosome is key to cell division, directing mitotic spindle formation via the centrocone and regulating daughter cell bud assembly, and plays a critical regulatory role in determining which division cycle occurs (Suvorova et al., 2015). It is comprised of two proteinaceous cores. The outer core, distal from the nucleus, contains TgCentrin1, the centrin-binding protein, TgSfi1, cartwheel protein TgSas-6, γ-tubulin, TgArk3 and during cytokinesis, also TgCep250. Depletion of TbSfi1 leads to reduced duplication and/or loss of outer cores, accompanied by uncontrolled replication of the inner core and centrocone and inhibition of budding (Suvorova et al., 2015). The inner core, located close to the centrocone, is comprised of orthologs of the CEP250/C-Nap centrosomal protein family, including a proteolytically processed form of TgCep250, present throughout the cell cycle. Depletion of TgCep250 disrupts the connection between inner and outer cores of the daughter centrosome, resulting in inner core loss and disruption of mitosis, without affecting outer core duplication or budding (Suvorova et al., 2015; Chen and Gubbels, 2019). Further, a large coiled-coil protein, TgCep530, localizes to the interface of the inner and outer cores and appears to coordinate nuclear packaging into daughter cells (Courjol and Gissot, 2018). Thus, the centrosome inner and outer cores appear to control mitosis and budding, respectively, with several protein kinases in addition to TgArk3 (discussed above) involved in this regulation. TgNEK1 localizes to the proximal ends of duplicating centrosomes and regulates centrosome splitting and therefore daughter cell scaffold assembly (Chen and Gubbels, 2013), and the calcium-dependent protein kinase, TgCDPK7, regulates centrosome duplication and integrity, and daughter cell orientation, amongst other roles (Morlon-Guyot et al., 2014). Further, the MAP kinase-like kinase, TgMAPK-L1, a candidate pericentriolar matrix protein that surrounds the TgCentrin1 outer core, is proposed to promote entry into the budding cycle and to suppress the nuclear cycle (Suvorova et al., 2015). Its depletion results in over-amplified centrocones and inhibition of basal complex ring formation, resulting in multiple rounds of S/M phases, without cytokinesis or accompanied by defective budding. In the absence of TgMAPK-L1 activity, or if the outer core is faulty or not assembled (e.g., as seems likely in the early division cycles of endopolygeny or schizogony, although this has not yet been demonstrated), the nuclear cycle is the default (Chen and Gubbels, 2015; Suvorova et al., 2015). Further, many integral centrosomal proteins have been identified, which are likely to be important for centrosome function and accurate budding (Morlon-Guyot et al., 2017). Ubiquitination may also play a role in controlling inner/outer core stoichiometry and coupling of mitosis and cytokinesis (Dhara et al., 2017). In addition, a number of other proteins have functions linked to controlling the switch between endodyogeny and endopolygeny. The inner membrane complex components, IMC14, IMC15, and ISP2, as well as the trafficking GTPase, Rab6, and the lipid storage/membrane biosynthesis stimulatory protein, TgNCR1, have been shown to limit the number of T. gondii offspring to two per mother cell (Stedman et al., 2003; Beck et al., 2010; Lige et al., 2011; Dubey et al., 2017); IMC14 depletion also affects synchrony of cell division (Dubey et al., 2017).
Far less is known about cell division in other coccidians, although imaging studies have revealed key differences compared to T. gondii. Sarcocystis neurona, the causative agent of equine protozoal myeloencephalitis in horses, replicates via a variant of endopolygeny, as discussed above, which can occur asynchronously within a single host cell i.e., the resultant merozoites sometimes remain within their original host cell and undergo another round of division (Speer and Dubey, 2001). Little is known about the molecular regulation of Sarcocystis cell division. Analysis of the S. neurona kinome has shown that most kinases discussed above are conserved (Murungi and Kariithi, 2017), but none have been functionally characterized. Further, while budding superficially resembles that in Toxoplasma, some inner membrane complex proteins may have differential functions compared to their T. gondii homologs (Dubey et al., 2017). Eimeria spp., which cause coccidiosis in poultry and ruminants, divide via schizogony within intestinal cells of their host. The first generation of schizogony involves sporozoites transforming to schizonts, while subsequent generations involve merozoites undergoing schizogony. Species-specific differences in schizont size between the first and second generations have been noted (Dubremetz and Elsner, 1979; Gregory et al., 1989; Ferguson et al., 2007). Further, E. vermiformis first generation merozoites bud randomly from the schizont, while second generation merozoites align in parallel before unidirectional budding (Adams and Todd, 1984). In E. crandallis and E. bakuensis, following second generation schizogony, dividing parasites appear to stretch across the host cell mitotic spindle (Gregory et al., 1987, 1989), similar to Theileria (see below). However, as with Sarcocystis, molecular detail is lacking; the CDK inhibitor, flavopiridol, inhibits schizont development (Engels et al., 2010), but functional analyses of CDKs/cyclins have not yet been performed (Kinnaird et al., 2004; Fernandez et al., 2012).
The Plasmodia
In Plasmodium spp., the causative agents of malaria, there are added complexities to cell division, with staggering differences of scale associated with their intricate life cycles (Gerald et al., 2011), where they are spread between animal hosts by mosquito vectors. There are four distinct cytokinesis events within the malaria life cycle. Within the mosquito, microgametocytes undergo three sequential rounds of S/M phase (forming eight basal bodies and flagellar axonemes de novo) in rapid succession (Billker et al., 1998). Chromatin then condenses, axonemes become motile, vesicles containing membranolytic proteins are secreted and a single mass and very rapid (8–12 min) cytokinesis event, known as exflagellation, occurs, whereby eight microgametes are formed as axonemes swim out of the residual gametocyte body, each dragging a condensed haploid genome attached to the basal body with it. Subsequently within the mosquito, sporogony (10–11 rounds of S/M phases over ~10 days) occurs, generating syncytial cells containing thousands of nuclei before a mass synchronous budding event occurs, releasing tens of thousands of infectious haploid sporozoites. Two more mass budding events occur within the mammalian host, at the end of schizogony in hepatocytes and erythrocytes, generating merozoites. Within hepatocytes, syncytia containing tens of thousands of nuclei are formed. The parasite plasma membrane invaginates (cytomere stage), forming spheres within the parasitophorous vacuole, each containing multiple nuclei, with branches of the apicoplast at the periphery and clumps of mitochondrion in the center. Regular constrictions then appear along the apicoplast's length, and mitochondrial protrusions reach out to each nucleus. The apicoplast then divides synchronously, followed by the mitochondrion, and then cytokinesis completes, with further invagination of the plasma membrane to generate individual haploid merozoites, which are released into the bloodstream in parasite-filled vesicles known as merosomes (Sturm et al., 2006). Merosomes then disintegrate, releasing merozoites into the circulation, which then invade red blood cells. Blood stage schizogony (lasting ~48 h), in comparison, comprises just 3–4 rounds of asynchronous S/M phases before a synchronous budding event occurs to yield 16–32 daughter merozoites.
Several proteins are known to be essential for exflagellation in male gametocytes. Calcium dependent protein kinase 4 (CDPK4) activity is required at multiple points during microgamete development; a large myristoylated isoform is required for initiating DNA replication and mitotic spindle assembly while a small non-myristoylated isoform also supports spindle assembly and is essential for axonemal motility just prior to exflagellation (Billker et al., 2004; Fang et al., 2017). The CDPK4 substrate, SOC3, an axoneme-associated protein, appears to be the effector for driving axonemal motility and cytokinesis (Fang et al., 2017). A gametocyte and mosquito-stage-specific actin, PfACT2, is also required for axoneme motility and exflagellation (Deligianni et al., 2011). Further, the MAP kinase, PfMAP-2, an in vitro substrate of CDPK4 and PfNek1, and APC/C components CDC20 and APC3, are required for axonemal motility, although perhaps indirectly as depletion of these proteins also blocks chromatin condensation/karyokinesis (Dorin et al., 2001; Rangarajan et al., 2005; Tewari et al., 2005; Wall et al., 2018). Depletion of a serine/argine-rich (SR) protein kinase, SRPK (predicted to act as a pre-mRNA splicing factor), or of the protein phosphatase, PPM1, also completely blocks exflagellation, although the exact stage of microgamete development affected has not been determined (Tewari et al., 2010; Guttery et al., 2014; Robbins et al., 2017).
Little is known about the regulation of cytokinesis at the end of sporogony, with just the phosphatase, PTPLA, the cyclin, PfCYC3, and LAP (LCCL lectin domain adhesive-like proteins) family proteins having been implicated (Roques et al., 2015; Saeed et al., 2018, 2019). Further, nothing is known of the regulation of schizogony in hepatocytes, so all knowledge of cytokinesis at the end of schizogony comes from studies of the blood stages. A key regulator of schizont cytokinesis is the divergent cyclin PfCYC1, a cyclin that is most similar at the sequence level to cyclin H, yet when expressed in yeast, functions instead as a G1 cyclin (Robbins et al., 2017). PfCYC1 is expressed throughout the blood stage cell cycle, but its knockdown specifically inhibits schizont segmentation, with aberrant formation of daughter cell inner membrane complexes (Robbins et al., 2017). Although PfCYC1 can activate PfPK5 (the CDK1 homolog) in vitro (Le Roch et al., 2000), it does not seem to interact with PfPK5 in vivo, instead forming a complex with the CDK7 homolog, PfMRK, and its accessory protein, PfMAT1, reminiscent of the yeast and metazoan CDK7/Cyclin H/MAT1 complex that comprises transcription factor IIH. TFIIH promotes efficient RNA polymerase II transcription, but CDK7 also acts as a CDK-activating kinase in some organisms, linking transcription and cell cycle control. PfCYC1/MRK/MAT1 phosphorylates the RNA polymerase II C-terminal domain in vitro, and the activity of CYC1/MRK is increased by the presence of MAT1 (Chen et al., 2006; Jirage et al., 2010), but how exactly it regulates cytokinesis is unknown. The kinases PfPK7 and PfCRK5 are required for efficient merozoite generation from schizonts and optimal proliferation rates (Dorin-Semblat et al., 2008, 2013), but their exact role has also not been determined. However, they both localize to the nuclear periphery in schizonts and interact in vitro, suggesting they might operate in the same pathway (Dorin-Semblat et al., 2013).
Several structural proteins are also required for cytokinesis of blood stage parasites. Knockdown of the merozoite organizing protein, PfMOP, results in defective inner membrane complex formation and incomplete segmentation of merozoites. Since PfMOP localizes to the apical end of daughter cells and first appears early in schizogony, it may organize inner membrane complex formation (Absalon et al., 2016). Later, the actin, PfACT1, and its nucleator, Formin-2 (PfFRM2), are required for apicoplast segregation at the end of schizogony, with their disruption resulting in conglomerates of merozoites (Das et al., 2017; Stortz et al., 2019). Actin filaments connect apicoplasts during schizogony and are thought to allow their segregation and promote efficient cell separation at the end of cytokinesis. The coordinator of nascent cell detachment, PfCINCH, a basal complex protein, is also essential for correct segmentation of daughter cells and for daughter cells pinching off from the residual body (Rudlaff et al., 2019). PfCINCH colocalizes with PfMORN1 at the basal complex, and interacts with the Plasmodium-specific proteins, basal complex protein 1 (PfBCP1) and basal complex transmembrane protein 2 (PfBTP2); PfBTP1 also colocalizes with PfMORN1 and may be involved in the interactions between the invaginating parasite plasma membrane and the inner membrane complex during the latter stages of cytokinesis (Kono et al., 2016). Finally, a trafficking protein, PfSortilin, is essential for inner membrane complex and basal complex contractile ring formation and the generation of merozoites, also playing a role in apicoplast segregation (Hallee et al., 2018), although its T. gondii ortholog is not required for pellicle formation (Sloves et al., 2012).
The Piroplasmida—Theileria and Babesia spp.
Piroplasms are intracellular parasites, exclusively transmitted by hard ticks worldwide. Sporozoites from tick saliva are introduced into the vertebrate bloodstream, where they undergo different forms of asexual replication in various blood cells, depending on the species (Jalovecka et al., 2018). Theileria spp. infect cattle, invading and transforming leukocytes, causing pronounced pathology and high mortality rates. Babesia spp. cause Babesiosis, an emerging zoonosis and the most common blood disease of free-living animals, leading to abortions, reduced meat and milk production and even death. Cytauxzoon causes often fatal disease in cats, but has been little studied.
Theileria sporozoites are immotile, with a poorly developed apical complex. They invade monocytes and lymphocytes, shedding their coat and escaping from the host cell membrane surrounding them to replicate free in the cytoplasm by schizogony. Schizonts from many, but not all, Theileria spp. interfere with their host's mitogenic pathways, causing continuous replication and inhibition of apoptosis. Within transformed leukocytes, Theileria and the host replicate their DNA asynchronously, with parasite S phase occurring predominantly during early host cell mitosis (Irvin et al., 1982), but divide in synchrony. To ensure transmission to daughter host cells, schizonts integrate themselves into the host's mitotic machinery, associating with newly formed spindle microtubules (Hulliger et al., 1964; Seitzer et al., 2010; von Schubert et al., 2010), and spanning across the equatorial region of the cell at metaphase. At anaphase, following inactivation of host cell CDK1, the schizont scavenges active host cell polo-like kinase (Plk1) to its surface, allowing the schizont to associate closely with the central spindle (von Schubert et al., 2010). The middle of the schizont is trapped within the midbody during telophase, ensuring schizont cleavage and approximately equal segregation as host cells undergo abscission (von Schubert et al., 2010), which is important as continued parasite presence is required for host cell transformation (Hudson et al., 1985).
Various parasite proteins facilitate host cell microtubule interaction. The T. annulata surface protein, TaSP, interacts with host cell alpha and gamma tubulin, enabling parasite association with host cell centrosomes, mitotic spindle and midbody (Seitzer et al., 2010). A secreted protein, TaSE, also interacts with alpha tubulin, colocalising with the host cell centromere, mitotic spindle and midbody (Schneider et al., 2007). Further, the schizont membrane protein, p104, interacts with host microtubule plus-end-tracking protein (+TIP) End-binding protein 1 (EB1), likely directing growing microtubules to the schizont surface (Woods et al., 2013), and also with the microtubule-stabilizing + TIP protein CLASP1 (Huber et al., 2017). Finally, a novel schizont surface protein, TA03615, immunoprecipitates with EB1 and CLASP1, suggesting that it too might be involved in regulating schizont:microtubule interactions (Huber et al., 2017).
Theileria schizonts also undergo merogony (Shaw and Tilney, 1992), where they are packaged into individual merozoites. Following organelle replication, the forming merozoites bud, apical end first, in two or more synchronous waves, from the schizont surface. The schizont plasma membrane invaginates and a sublamellar basket of tubules extends from the apical to the basal end of each parasite, with a basal ring thought to constrict to separate the merozoite from the schizont. The leukocyte then ruptures, releasing merozoites into the bloodstream where they invade erythrocytes, transform into the piroplasm stage and undergo a limited number of asynchronous and little studied replication cycles, before some transform to gametocytes (Jalovecka et al., 2018). Upon uptake by a tick, gametocytes differentiate to gametes and then motile kinetes, which migrate to the salivary glands (Mehlhorn and Schein, 1976; Schein et al., 1977; Jalovecka et al., 2018) and rapidly replicate to form multinucleated syncitia within structures termed acini, before a poorly understood mass cytokinesis event occurs, giving rise to ~30–50,000 sporozoites per acinus (Fawcett et al., 1982).
In contrast, Babesia spp. multiply exclusively by merogony in red blood cells. Sporozoites injected by a tick bite are internalized into erythrocytes where they develop into ring stages or trophozoites and replicate freely and asynchronously in the cytoplasm, producing merozoites, which are released upon host cell rupture to invade other red blood cells. Little more is known about Babesia merogony or of the molecules that control it, although inhibitor studies suggest that CDKs are required (Nakamura et al., 2007). Indeed, despite piroplasms having the smallest kinomes of the Apicomplexans, with just 35 protein kinases in B. bovis and 37 and 38 in T. annulata and T. parva, respectively (Miranda-Saavedra et al., 2012), none have been functionally characterized. Within the tick, development of Babesia is mostly similar to that of Theileria. However, during sporogony, sporoblast maturation involves cytomere formation in some Babesia spp., which has not been observed in Theileria spp. (Jalovecka et al., 2018), and sporogony is asynchronous in Babesia, resulting in the continued release of sporozoites into tick saliva over several days (Yano et al., 2005).
The Cryptosporidia
Cryptosporidium spp., once thought to be coccidian parasites, are gregarines (Clode et al., 2015) which infect gut epithelial cells, causing diarrhea in humans and ruminants. They structurally differ from other apicomplexans, lacking an apicoplast and mitochondrial DNA (Bouzid et al., 2013). Sporozoites released from ingested sporulated oocysts invade host epithelial cells, becoming encapsulated in a parasite-modified host membrane on top of the epithelial cells, transforming into a trophozoite and replicating by merogony to release eight merozoites (O'Hara and Chen, 2011). However, in axenic culture, differentiation to trophozoites and merogony may occur within sporulated oocysts, with merozoites budding asynchronously from the oocyst membrane, with electron-dense collars at the bud site (Aldeyarbi and Karanis, 2016). Analysis of the Cryptosporidium kinome indicates that it lacks all aurora kinases and several CRKs, and it only possesses one NEK kinase (NEK1), suggesting divergent control. Recent advances in culturing (Wilke et al., 2019) should facilitate further studies of Cryptosporidum cytokinesis.
Summary
Parasitic protozoans display an impressive array of cytokinesis mechanisms. These range from inaccurate and primitive cytofission in Entamoeba spp. to very precise and tightly regulated microtubule rearrangements that lead to furrow ingression in kinetoplastids, intricate assembly and segmentation of new daughter cells during apicomplexan budding, and ingenious hijacking of host mitotic machinery employed by Theileria and some Eimeria spp. However, to date, no protozoan parasite is known to use a contractile actomyosin ring, with most lacking myosin II. Even in Entamoeba, which does possess myosin II, there is no evidence for contractile actomyosin ring formation and only limited data to suggest that myosin II is important for cytokinesis in certain cell types (Majumder and Lohia, 2008; Krishnan and Ghosh, 2018). It remains to be seen whether myosin II-containing Naegleria spp. employ an actomyosin ring to divide, but other aspects of the cell cycle are divergent (Fritz-Laylin et al., 2010, 2011). Ciliates and some apicomplexans, which lack myosin II, however, do employ other types of contractile rings (Tucker, 1971; Yasuda et al., 1980; Hu, 2008). In T. gondii the basal complex ring contains non-myosin II myosins, which aid basal complex constriction (Delbac et al., 2001; Engelberg et al., 2016; Frenal et al., 2017), but Plasmodium spp. lack orthologs of some of these myosins, and the cytokinesis role of others is not conserved (Wall et al., 2019). Hence, myosins may not be involved in cytokinesis across the apicomplexa. Further, Giardia and Trichomonas spp. do not possess any functional myosins, relying on cytoskeletal elements/proteins and flagellar motility to bring about cytokinesis (Ribeiro et al., 2000; Benchimol, 2004b; Hardin et al., 2017). Flagellar/ciliary motility is also important for cytokinesis in T. brucei (Branche et al., 2006; Ralston et al., 2006; Zhang et al., 2019b) and Tetrahymena (Brown et al., 1999a), and during exflagellation in Plasmodium spp. (Billker et al., 1998).
It is not surprising, therefore, that molecular control of cytokinesis across the parasitic protozoans is also divergent. Canonical molecular cell cycle checkpoints are often absent; some key cell cycle regulators are present, but their functions are not always conserved and they may act in conjunction with parasite-specific regulators. The level of molecular control is also variable, from minimal in Entamoeba (Mukherjee et al., 2009; Grewal and Lohia, 2015) to highly intricate with built-in flexibility in the kinetoplastids and apicomplexans. However, despite the physical and molecular differences compared to model organisms, some aspects of cytokinesis are conserved. Vesicle delivery to the furrow, the plasma membrane lipid composition and changes in cortical tension are key for cytokinesis in Giardia and/or T. brucei (Lillico et al., 2003; Sheader et al., 2005; Rodgers et al., 2007; Fridberg et al., 2008; Stefanic et al., 2010; Paredez et al., 2011; Hardin et al., 2017; Schoijet et al., 2018). Further, protozoan parasites must cleave an intercellular cytoplasmic bridge containing cytoskeletal components that still links the daughter cells late in cytokinesis, to complete abscission, although midbodies have not been described in protozoan parasites, and in many protozoans, similar to metazoans, the rate of abscission is different from that of furrowing.
Exploiting Cytokinesis for Drug Discovery
Given the essentiality and uniqueness of cytokinesis in protozoan parasites, key cytokinesis proteins have strong potential as novel drug targets. In particular, protein kinases are of interest, since kinases are druggable molecules (Urbaniak et al., 2012), with an increasing number of kinase inhibitors used clinically to treat various cancers (Klaeger et al., 2017), and the possibility of repurposing human kinase inhibitors. Various kinase inhibitors have been identified for kinetoplastids (Pena et al., 2015; Woodland et al., 2015; Amata et al., 2016; Wyllie et al., 2018) and Plasmodium spp. (Hallyburton et al., 2017; Mathews and Odom John, 2018), with some showing clinical promise. But what about cytokinesis kinases? Repurposing and refinement of human aurora kinase inhibitors has identified effective and selective compounds for T. brucei and P. falciparum in vitro (Ochiana et al., 2013; Patel et al., 2014). Further, PfPKG inhibitory thiazole compounds with additional activity against PfCDPK4, PfCRK5, and PfNEK1 have been identified (Penzo et al., 2019), as well as PfCDPK4 inhibitors that block exflagellation and hence, likely, transmission of P. falciparum (Ojo et al., 2014; Vidadala et al., 2014). TgMAPKL-1, targeted by bumped kinase inhibitors, is reported to have promising drug target potential (Sugi et al., 2013, 2015; Brown et al., 2014), TbPK50 and TbPK53 kinase inhibitors have been identified (Ma et al., 2010; Urbaniak et al., 2012) and the CK2 inhibitor, emodin, inhibits cytokinesis in T. cruzi (De Lima et al., 2017). Aside from protein kinases, sphingosine derivatives and inhibitors of sphingolipid biosynthetic enzymes have potential against Giardia (Sonda et al., 2008; Stefanic et al., 2010) and T. brucei (Fridberg et al., 2008; Jones et al., 2015), while repurposing of human PDE inhibitors shows promise in T. brucei (de Koning et al., 2012; Ochiana et al., 2015). In the future, it may also be possible to repurpose inhibitors of human Plk1 (Gutteridge et al., 2016), Hippo pathway inhibitors (Qiao et al., 2019) or enzymes such as katanin/spastin as they are developed (Pisa et al., 2019). However, currently, further work is required to refine existing cytokinesis inhibitors before they will be clinically useful.
Conclusions
Protozoan parasites cause great morbidity and mortality in humans and animals worldwide, and are therefore of considerable medical and economic concern. They are also of great evolutionary interest, with the multiple and varied modes of cytokinesis highlighting the diversity of cell biology in non-model organisms. Indeed, across the evolutionary tree, only a relatively small proportion of organisms use a canonical actomyosin ring to divide. Increasing our knowledge of the molecular pathways that underpin such diverse biology will aid our understanding of the evolution of cytokinesis and potentially uncover novel drug discovery approaches for protozoan parasites.
Author Contributions
TH conceived, researched, and wrote the article.
Funding
This work was funded by MRC grant GO900239 to TH. Article Processing Charges were funded by UKRI.
Conflict of Interest
The author declares that the research was conducted in the absence of any commercial or financial relationships that could be construed as a potential conflict of interest.
Acknowledgments
I would like to thank Ross Madden for his helpful comments on the manuscript.
References
Abbasi, K., Dubois, K. N., Leung, K. F., Dacks, J. B., and Field, M. C. (2011). A novel Rho-like protein TbRHP is involved in spindle formation and mitosis in trypanosomes. PLoS ONE 6:e26890. doi: 10.1371/annotation/b4b38a99-0b4c-483a-ad21-4dd721974b97
Absalon, S., Robbins, J. A., and Dvorin, J. D. (2016). An essential malaria protein defines the architecture of blood-stage and transmission-stage parasites. Nat. Commun. 7:11449. doi: 10.1038/ncomms11449
Adams, J. H., and Todd, K. S. Jr. (1984). Transmission electron microscopy of meront development of Eimeria vermiformis Ernst, Chobotar and Hammond, 1971 (Apicomplexa, Eucoccidiorida) in the mouse, Mus musculus. J. Protozool. 31, 233–240. doi: 10.1111/j.1550-7408.1984.tb02953.x
Alcantara, C. L., Vidal, J. C., De Souza, W., and Cunha, E. S. N. L. (2017). The cytostome-cytopharynx complex of Trypanosoma cruzi epimastigotes disassembles during cell division. J. Cell Sci. 130, 164–176. doi: 10.1242/jcs.187419
Aldeyarbi, H. M., and Karanis, P. (2016). Electron microscopic observation of the early stages of Cryptosporidium parvum asexual multiplication and development in in vitro axenic culture. Eur. J. Protistol. 52, 36–44. doi: 10.1016/j.ejop.2015.07.002
Alvarez, C. A., and Suvorova, E. S. (2017). Checkpoints of apicomplexan cell division identified in Toxoplasma gondii. PLoS Pathog. 13:e1006483. doi: 10.1371/journal.ppat.1006483
Amador, E., Lopez-Pacheco, K., Morales, N., Coria, R., and Lopez-Villasenor, I. (2017). Characterization of cyclin-dependent kinases and Cdc2/Cdc28 kinase subunits in Trichomonas vaginalis. Parasitology 144, 571–582. doi: 10.1017/S0031182016002195
Amata, E., Xi, H., Colmenarejo, G., Gonzalez-Diaz, R., Cordon-Obras, C., Berlanga, M., et al. (2016). Identification of “preferred” human kinase inhibitors for sleeping sickness lead discovery. Are some kinases better than others for inhibitor repurposing? ACS Infect. Dis. 2, 180–186. doi: 10.1021/acsinfecdis.5b00136
Ambit, A., Woods, K. L., Cull, B., Coombs, G. H., and Mottram, J. C. (2011). Morphological events during the cell cycle of Leishmania major. Eukaryotic Cell 10, 1429–1438. doi: 10.1128/EC.05118-11
Anamika, K., Bhattacharya, A., and Srinivasan, N. (2008). Analysis of the protein kinome of Entamoeba histolytica. Proteins 71, 995–1006. doi: 10.1002/prot.21790
Andenmatten, N., Egarter, S., Jackson, A. J., Jullien, N., Herman, J. P., and Meissner, M. (2013). Conditional genome engineering in Toxoplasma gondii uncovers alternative invasion mechanisms. Nat. Methods 10, 125–127. doi: 10.1038/nmeth.2301
Anderson-White, B. R., Ivey, F. D., Cheng, K., Szatanek, T., Lorestani, A., Beckers, C. J., et al. (2011). A family of intermediate filament-like proteins is sequentially assembled into the cytoskeleton of Toxoplasma gondii. Cell. Microbiol. 13, 18–31. doi: 10.1111/j.1462-5822.2010.01514.x
Arias-Romero, L. E., De Jesus Almaraz-Barrera, M., Diaz-Valencia, J. D., Rojo-Dominguez, A., Hernandez-Rivas, R., and Vargas, M. (2006). EhPAK2, a novel p21-activated kinase, is required for collagen invasion and capping in Entamoeba histolytica. Mol. Biochem. Parasitol. 149, 17–26. doi: 10.1016/j.molbiopara.2006.04.001
Atilla-Gokcumen, G. E., Muro, E., Relat-Goberna, J., Sasse, S., Bedigian, A., Coughlin, M. L., et al. (2014). Dividing cells regulate their lipid composition and localization. Cell 156, 428–439. doi: 10.1016/j.cell.2013.12.015
Bachmaier, S., Volpato Santos, Y., Kramer, S., Githure, G. B., Klockner, T., Pepperl, J., et al. (2019). Nucleoside analogue activators of cyclic AMP-independent protein kinase A of Trypanosoma. Nat. Commun. 10, 1421. doi: 10.1038/s41467-019-09338-z
Barquilla, A., and Navarro, M. (2009). Trypanosome TOR complex 2 functions in cytokinesis. Cell Cycle 8, 697–699. doi: 10.4161/cc.8.5.7808
Bastos, R. N., and Barr, F. A. (2010). Plk1 negatively regulates Cep55 recruitment to the midbody to ensure orderly abscission. J. Cell Biol. 191, 751–760. doi: 10.1083/jcb.201008108
Beck, J. R., Rodriguez-Fernandez, I. A., De Leon, J. C., Huynh, M. H., Carruthers, V. B., Morrissette, N. S., et al. (2010). A novel family of Toxoplasma IMC proteins displays a hierarchical organization and functions in coordinating parasite division. PLoS Pathog. 6:e1001094. doi: 10.1371/journal.ppat.1001094
Behnke, M. S., Wootton, J. C., Lehmann, M. M., Radke, J. B., Lucas, O., Nawas, J., et al. (2010). Coordinated progression through two subtranscriptomes underlies the tachyzoite cycle of Toxoplasma gondii. PLoS ONE 5:e12354. doi: 10.1371/journal.pone.0012354
Benchimol, M. (2004a). Mitosis in Giardia lamblia: multiple modes of cytokinesis. Protist 155, 33–44. doi: 10.1078/1434461000162
Benchimol, M. (2004b). Trichomonads under microscopy. Microsc. Microanal. 10, 528–550. doi: 10.1017/S1431927604040905
Beneke, T., Madden, R., Makin, L., Valli, J., Sunter, J., and Gluenz, E. (2017). A CRISPR Cas9 high-throughput genome editing toolkit for kinetoplastids. R. Soc. Open Sci. 4:170095. doi: 10.1098/rsos.170095
Benz, C., and Clayton, C. E. (2007). The F-box protein CFB2 is required for cytokinesis of bloodstream-form Trypanosoma brucei. Mol. Biochem. Parasitol. 156, 217–224. doi: 10.1016/j.molbiopara.2007.08.005
Benz, C., Clucas, C., Mottram, J. C., and Hammarton, T. C. (2012). Cytokinesis in bloodstream stage Trypanosoma brucei requires a family of katanins and spastin. PLoS ONE 7:e30367. doi: 10.1371/annotation/aa6cd97c-cfb5-4afe-aa25-f5ecfed07980
Berry, L., Chen, C. T., Francia, M. E., Guerin, A., Graindorge, A., Saliou, J. M., et al. (2018). Toxoplasma gondii chromosomal passenger complex is essential for the organization of a functional mitotic spindle: a prerequisite for productive endodyogeny. Cell Mol. Life Sci. 75, 4417–4443. doi: 10.1007/s00018-018-2889-6
Berry, L., Chen, C. T., Reininger, L., Carvalho, T. G., El Hajj, H., Morlon-Guyot, J., et al. (2016). The conserved apicomplexan Aurora kinase TgArk3 is involved in endodyogeny, duplication rate and parasite virulence. Cell. Microbiol. 18, 1106–1120. doi: 10.1111/cmi.12571
Bessat, M. (2014). Knockdown of APC/C-associated genes and its effect on viability and cell cycle of protozoan parasite of Trypanosoma brucei. Parasitol. Res. 113, 1555–1562. doi: 10.1007/s00436-014-3800-5
Bessat, M., Knudsen, G., Burlingame, A. L., and Wang, C. C. (2013). A minimal anaphase promoting complex/cyclosome (APC/C) in Trypanosoma brucei. PLoS ONE 8:e59258. doi: 10.1371/annotation/d048ae1b-6a9b-4353-bdf2-c707925ce37c
Billker, O., Dechamps, S., Tewari, R., Wenig, G., Franke-Fayard, B., and Brinkmann, V. (2004). Calcium and a calcium-dependent protein kinase regulate gamete formation and mosquito transmission in a malaria parasite. Cell 117, 503–514. doi: 10.1016/S0092-8674(04)00449-0
Billker, O., Lindo, V., Panico, M., Etienne, A. E., Paxton, T., Dell, A., et al. (1998). Identification of xanthurenic acid as the putative inducer of malaria development in the mosquito. Nature 392, 289–292. doi: 10.1038/32667
Biron, D., Libros, P., Sagi, D., Mirelman, D., and Moses, E. (2001). Asexual reproduction: 'midwives' assist dividing amoebae. Nature 410:430. doi: 10.1038/35068628
Bosch, D. E., Yang, B., and Siderovski, D. P. (2012). Entamoeba histolytica Rho1 regulates actin polymerization through a divergent, diaphanous-related formin. Biochemistry 51, 8791–8801. doi: 10.1021/bi300954g
Bouzid, M., Hunter, P. R., Chalmers, R. M., and Tyler, K. M. (2013). Cryptosporidium pathogenicity and virulence. Clin. Microbiol. Rev. 26, 115–134. doi: 10.1128/CMR.00076-12
Branche, C., Kohl, L., Toutirais, G., Buisson, J., Cosson, J., and Bastin, P. (2006). Conserved and specific functions of axoneme components in trypanosome motility. J. Cell Sci. 119, 3443–3455. doi: 10.1242/jcs.03078
Broadhead, R., Dawe, H. R., Farr, H., Griffiths, S., Hart, S. R., Portman, N., et al. (2006). Flagellar motility is required for the viability of the bloodstream trypanosome. Nature 440, 224–227. doi: 10.1038/nature04541
Brown, J. M., Hardin, C., and Gaertig, J. (1999a). Rotokinesis, a novel phenomenon of cell locomotion-assisted cytokinesis in the ciliate Tetrahymena thermophila. Cell Biol. Int. 23, 841–848. doi: 10.1006/cbir.1999.0480
Brown, J. M., Marsala, C., Kosoy, R., and Gaertig, J. (1999b). Kinesin-II is preferentially targeted to assembling cilia and is required for ciliogenesis and normal cytokinesis in Tetrahymena. Mol. Biol. Cell 10, 3081–3096. doi: 10.1091/mbc.10.10.3081
Brown, K. M., Suvorova, E., Farrell, A., Mclain, A., Dittmar, A., Wiley, G. B., et al. (2014). Forward genetic screening identifies a small molecule that blocks Toxoplasma gondii growth by inhibiting both host- and parasite-encoded kinases. PLoS Pathog. 10:e1004180. doi: 10.1371/journal.ppat.1004180
Brugerolle, G., Bricheux, G., and Coffe, G. (1996). Actin cytoskeleton demonstration in Trichomonas vaginalis and in other trichomonads. Biol. Cell 88, 29–36. doi: 10.1016/S0248-4900(97)86828-1
Capewell, P., Cren-Travaille, C., Marchesi, F., Johnston, P., Clucas, C., Benson, R. A., et al. (2016). The skin is a significant but overlooked anatomical reservoir for vector-borne African trypanosomes. Elife 5:e17716. doi: 10.7554/eLife.17716
Carmena, M., Wheelock, M., Funabiki, H., and Earnshaw, W. C. (2012). The chromosomal passenger complex (CPC): from easy rider to the godfather of mitosis. Nat. Rev. Mol. Cell Biol. 13, 789–803. doi: 10.1038/nrm3474
Casanova, M., Crobu, L., Blaineau, C., Bourgeois, N., Bastien, P., and Pages, M. (2009). Microtubule-severing proteins are involved in flagellar length control and mitosis in Trypanosomatids. Mol. Microbiol. 71, 1353–1370. doi: 10.1111/j.1365-2958.2009.06594.x
Chanez, A. L., Hehl, A. B., Engstler, M., and Schneider, A. (2006). Ablation of the single dynamin of T-brucei blocks mitochondrial fission and endocytosis and leads to a precise cytokinesis arrest. J. Cell Sci. 119, 2968–2974. doi: 10.1242/jcs.03023
Chavez-Munguia, B., Tsutsumi, V., and Martinez-Palomo, A. (2006). Entamoeba histolytica: ultrastructure of the chromosomes and the mitotic spindle. Exp. Parasitol. 114, 235–239. doi: 10.1016/j.exppara.2006.03.005
Chen, A. L., Kim, E. W., Toh, J. Y., Vashisht, A. A., Rashoff, A. Q., Van, C., et al. (2015). Novel components of the Toxoplasma inner membrane complex revealed by BioID. MBio 6, e02357–e02314. doi: 10.1128/mBio.02357-14
Chen, A. L., Moon, A. S., Bell, H. N., Huang, A. S., Vashisht, A. A., Toh, J. Y., et al. (2017). Novel insights into the composition and function of the Toxoplasma IMC sutures. Cell. Microbiol. 19:12678. doi: 10.1111/cmi.12678
Chen, C. T., and Gubbels, M. J. (2013). The Toxoplasma gondii centrosome is the platform for internal daughter budding as revealed by a Nek1 kinase mutant. J. Cell Sci. 126, 3344–3355. doi: 10.1242/jcs.123364
Chen, C. T., and Gubbels, M. J. (2015). Apicomplexan cell cycle flexibility: centrosome controls the clutch. Trends Parasitol. 31, 229–230. doi: 10.1016/j.pt.2015.04.003
Chen, C. T., and Gubbels, M. J. (2019). TgCep250 is dynamically processed through the division cycle and is essential for structural integrity of the Toxoplasma centrosome. Mol. Biol. Cell 30, 1160–1169. doi: 10.1091/mbc.E18-10-0608
Chen, Y., Jirage, D., Caridha, D., Kathcart, A. K., Cortes, E. A., Dennull, R. A., et al. (2006). Identification of an effector protein and gain-of-function mutants that activate Pfmrk, a malarial cyclin-dependent protein kinase. Mol. Biochem. Parasitol. 149, 48–57. doi: 10.1016/j.molbiopara.2006.04.004
Chettri, J. K., Leibowitz, M. P., Ofir, R., and Zilberg, D. (2009). Protective immunization against Tetrahymena sp. infection in guppies (Poecilia reticulata). Fish Shellfish Immunol. 27, 302–308. doi: 10.1016/j.fsi.2009.05.013
Chhajer, R., Bhattacharyya, A., Didwania, N., Shadab, M., Das, N., Palit, P., et al. (2016). Leishmania donovani Aurora kinase: a promising therapeutic target against visceral Leishmaniasis. Biochim. Biophys. Acta 1860, 1973–1988. doi: 10.1016/j.bbagen.2016.06.005
Chung, S., Cho, J., Cheon, H., Paik, S., and Lee, J. (2002). Cloning and characterization of a divergent alpha-tubulin that is expressed specifically in dividing amebae of Naegleria gruberi. Gene 293, 77–86. doi: 10.1016/S0378-1119(02)00509-7
Clode, P. L., Koh, W. H., and Thompson, R. C. A. (2015). Life without a host cell: what is cryptosporidium? Trends Parasitol. 31, 614–624. doi: 10.1016/j.pt.2015.08.005
Connell, J. W., Lindon, C., Luzio, J. P., and Reid, E. (2009). Spastin couples microtubule severing to membrane traffic in completion of cytokinesis and secretion. Traffic 10, 42–56. doi: 10.1111/j.1600-0854.2008.00847.x
Costa, F. C., Francisco, A. F., Jayawardhana, S., Calderano, S. G., Lewis, M. D., Olmo, F., et al. (2018). Expanding the toolbox for Trypanosoma cruzi: a parasite line incorporating a bioluminescence-fluorescence dual reporter and streamlined CRISPR/Cas9 functionality for rapid in vivo localisation and phenotyping. PLoS Negl. Trop. Dis. 12:e0006388. doi: 10.1371/journal.pntd.0006388
Courjol, F., and Gissot, M. (2018). A coiled-coil protein is required for coordination of karyokinesis and cytokinesis in Toxoplasma gondii. Cell. Microbiol. 20:e12832. doi: 10.1111/cmi.12832
Coyne, R. S., Hannick, L., Shanmugam, D., Hostetler, J. B., Brami, D., Joardar, V. S., et al. (2011). Comparative genomics of the pathogenic ciliate Ichthyophthirius multifiliis, its free-living relatives and a host species provide insights into adoption of a parasitic lifestyle and prospects for disease control. Genome Biol. 12:R100. doi: 10.1186/gb-2011-12-10-r100
Cross, F. R., and Umen, J. G. (2015). The Chlamydomonas cell cycle. Plant J. 82, 370–392. doi: 10.1111/tpj.12795
Crozier, T. W. M., Tinti, M., Wheeler, R. J., Ly, T., Ferguson, M., Lamond, A. I., et al. (2018). Proteomic analysis of the cell cycle of procylic form Trypanosoma brucei. Mol. Cell. Proteomics 17, 1184–1195. doi: 10.1074/mcp.RA118.000650
Das, S., Lemgruber, L., Tay, C. L., Baum, J., and Meissner, M. (2017). Multiple essential functions of Plasmodium falciparum actin-1 during malaria blood-stage development. BMC Biol. 15:70. doi: 10.1186/s12915-017-0406-2
Davids, B. J., Williams, S., Lauwaet, T., Palanca, T., and Gillin, F. D. (2008). Giardia lamblia aurora kinase: a regulator of mitosis in a binucleate parasite. Int. J. Parasitol. 38, 353–369. doi: 10.1016/j.ijpara.2007.08.012
D'Avino, P. P., and Capalbo, L. (2016). Regulation of midbody formation and function by mitotic kinases. Semin. Cell Dev. Biol. 53, 57–63. doi: 10.1016/j.semcdb.2016.01.018
de Graffenried, C. L., Anrather, D., Von Raussendorf, F., and Warren, G. (2013). Polo-like kinase phosphorylation of bilobe-resident TbCentrin2 facilitates flagellar inheritance in Trypanosoma brucei. Mol. Biol. Cell 24, 1947–1963. doi: 10.1091/mbc.e12-12-0911
de Graffenried, C. L., Ho, H. H., and Warren, G. (2008). Polo-like kinase is required for Golgi and bilobe biogenesis in Trypanosoma brucei. J. Cell Biol. 181, 431–438. doi: 10.1083/jcb.200708082
de Koning, H. P., Gould, M. K., Sterk, G. J., Tenor, H., Kunz, S., Luginbuehl, E., et al. (2012). Pharmacological validation of Trypanosoma brucei phosphodiesterases as novel drug targets. J. Infect. Dis. 206, 229–237. doi: 10.1093/infdis/jir857
De Lima, A. R., Noris-Suarez, K., Bretana, A., Contreras, V. T., Navarro, M. C., Perez-Ybarra, L., et al. (2017). Growth arrest and morphological changes triggered by emodin on Trypanosoma cruzi epimastigotes cultivated in axenic medium. Biochimie 142, 31–40. doi: 10.1016/j.biochi.2017.08.005
De Lozanne, A., and Spudich, J. A. (1987). Disruption of the Dictyostelium myosin heavy chain gene by homologous recombination. Science 236, 1086–1091. doi: 10.1126/science.3576222
Delbac, F., Sanger, A., Neuhaus, E. M., Stratmann, R., Ajioka, J. W., Toursel, C., et al. (2001). Toxoplasma gondii myosins B/C: one gene, two tails, two localizations, and a role in parasite division. J. Cell Biol. 155, 613–623. doi: 10.1083/jcb.200012116
Deligianni, E., Morgan, R. N., Bertuccini, L., Kooij, T. W., Laforge, A., Nahar, C., et al. (2011). Critical role for a stage-specific actin in male exflagellation of the malaria parasite. Cell. Microbiol. 13, 1714–1730. doi: 10.1111/j.1462-5822.2011.01652.x
Dhara, A., De Paula Baptista, R., Kissinger, J. C., Snow, E. C., and Sinai, A. P. (2017). Ablation of an ovarian tumor family deubiquitinase exposes the underlying regulation governing the plasticity of cell cycle progression in Toxoplasma gondii. MBio 8:e01846-17. doi: 10.1128/mBio.01846-17
Dorin, D., Le Roch, K., Sallicandro, P., Alano, P., Parzy, D., Poullet, P., et al. (2001). Pfnek-1, a NIMA-related kinase from the human malaria parasite Plasmodium falciparum- biochemical properties and possible involvement in MAPK regulation. Eur. J. Biochem. 268, 2600–2608. doi: 10.1046/j.1432-1327.2001.02151.x
Dorin-Semblat, D., Carvalho, T. G., Nivez, M. P., Halbert, J., Poullet, P., Semblat, J. P., et al. (2013). An atypical cyclin-dependent kinase controls Plasmodium falciparum proliferation rate. Kinome 1, 4–16. doi: 10.2478/kinome-2013-0001
Dorin-Semblat, D., Sicard, A., Doerig, C., Ranford-Cartwright, L., and Doerig, C. (2008). Disruption of the PfPK7 gene impairs schizogony and sporogony in the human malaria parasite Plasmodium falciparum. Eukaryotic Cell 7, 279–285. doi: 10.1128/EC.00245-07
Dubey, R., Harrison, B., Dangoudoubiyam, S., Bandini, G., Cheng, K., Kosber, A., et al. (2017). Differential roles for inner membrane complex proteins across Toxoplasma gondii and Sarcocystis neurona development. mSphere 2, e00409–e00417. doi: 10.1128/mSphere.00409-17
Dubremetz, J. F., and Elsner, Y. Y. (1979). Ultrastructural study of schizogony of Eimeria bovis in cell cultures. J. Protozool. 26, 367–376. doi: 10.1111/j.1550-7408.1979.tb04639.x
Duncan, S. M., Myburgh, E., Philipon, C., Brown, E., Meissner, M., Brewer, J., et al. (2016). Conditional gene deletion with DiCre demonstrates an essential role for CRK3 in Leishmania mexicana cell cycle regulation. Mol. Microbiol. 100, 931–944. doi: 10.1111/mmi.13375
Edamatsu, M., Hirono, M., and Watanabe, Y. (1992). Tetrahymena profilin is localized in the division furrow. J. Biochem. 112, 637–642. doi: 10.1093/oxfordjournals.jbchem.a123952
Elias, M. C., Da Cunha, J. P., De Faria, F. P., Mortara, R. A., Freymuller, E., and Schenkman, S. (2007). Morphological events during the Trypanosoma cruzi cell cycle. Protist 158, 147–157. doi: 10.1016/j.protis.2006.10.002
Emoto, K., Inadome, H., Kanaho, Y., Narumiya, S., and Umeda, M. (2005). Local change in phospholipid composition at the cleavage furrow is essential for completion of cytokinesis. J. Biol. Chem. 280, 37901–37907. doi: 10.1074/jbc.M504282200
Engelberg, K., Ivey, F. D., Lin, A., Kono, M., Lorestani, A., Faugno-Fusci, D., et al. (2016). A MORN1-associated HAD phosphatase in the basal complex is essential for Toxoplasma gondii daughter budding. Cell. Microbiol. 18, 1153–1171. doi: 10.1111/cmi.12574
Engels, K., Beyer, C., Suarez Fernandez, M. L., Bender, F., Gassel, M., Unden, G., et al. (2010). Inhibition of Eimeria tenella CDK-related kinase 2: from target identification to lead compounds. ChemMedChem 5, 1259–1271. doi: 10.1002/cmdc.201000157
Fang, H., Klages, N., Baechler, B., Hillner, E., Yu, L., Pardo, M., et al. (2017). Multiple short windows of calcium-dependent protein kinase 4 activity coordinate distinct cell cycle events during Plasmodium gametogenesis. Elife 6:e26524. doi: 10.7554/eLife.26524
Fassolari, M., and Alonso, G. D. (2019). Aurora kinase protein family in Trypanosoma cruzi: novel role of an AUK-B homologue in kinetoplast replication. PLoS Negl. Trop. Dis. 13:e0007256. doi: 10.1371/journal.pntd.0007256
Fawcett, D. W., Buscher, G., and Doxsey, S. (1982). Salivary gland of the tick vector of East Coast fever. III. The ultrastructure of sporogony in Theileria parva. Tissue Cell 14, 183–206. doi: 10.1016/0040-8166(82)90017-9
Fededa, J. P., and Gerlich, D. W. (2012). Molecular control of animal cell cytokinesis. Nat. Cell Biol. 14, 440–447. doi: 10.1038/ncb2482
Ferguson, D. J., Campbell, S. A., Henriquez, F. L., Phan, L., Mui, E., Richards, T. A., et al. (2007). Enzymes of type II fatty acid synthesis and apicoplast differentiation and division in Eimeria tenella. Int. J. Parasitol. 37, 33–51. doi: 10.1016/j.ijpara.2006.10.003
Ferguson, D. J., Sahoo, N., Pinches, R. A., Bumstead, J. M., Tomley, F. M., and Gubbels, M. J. (2008). MORN1 has a conserved role in asexual and sexual development across the apicomplexa. Eukaryotic Cell 7, 698–711. doi: 10.1128/EC.00021-08
Fernandez, M. L., Engels, K. K., Bender, F., Gassel, M., Marhofer, R. J., Mottram, J. C., et al. (2012). High-throughput screening with the Eimeria tenella CDC2-related kinase2/cyclin complex EtCRK2/EtCYC3a. Microbiology 158, 2262–2271. doi: 10.1099/mic.0.059428-0
Francia, M. E., Dubremetz, J. F., and Morrissette, N. S. (2015). Basal body structure and composition in the apicomplexans Toxoplasma and Plasmodium. Cilia 5:3. doi: 10.1186/s13630-016-0025-5
Francia, M. E., Jordan, C. N., Patel, J. D., Sheiner, L., Demerly, J. L., Fellows, J. D., et al. (2012). Cell division in Apicomplexan parasites is organized by a homolog of the striated rootlet fiber of algal flagella. PLoS Biol. 10:e1001444. doi: 10.1371/journal.pbio.1001444
Francia, M. E., and Striepen, B. (2014). Cell division in apicomplexan parasites. Nat. Rev. Microbiol. 12, 125–136. doi: 10.1038/nrmicro3184
Frenal, K., Jacot, D., Hammoudi, P. M., Graindorge, A., Maco, B., and Soldati-Favre, D. (2017). Myosin-dependent cell-cell communication controls synchronicity of division in acute and chronic stages of Toxoplasma gondii. Nat. Commun. 8:15710. doi: 10.1038/ncomms15710
Fridberg, A., Olson, C. L., Nakayasu, E. S., Tyler, K. M., Almeida, I. C., and Engman, D. M. (2008). Sphingolipid synthesis is necessary for kinetoplast segregation and cytokinesis in Trypanosoma brucei. J. Cell Sci. 121, 522–535. doi: 10.1242/jcs.016741
Fritz-Laylin, L. K., Ginger, M. L., Walsh, C., Dawson, S. C., and Fulton, C. (2011). The Naegleria genome: a free-living microbial eukaryote lends unique insights into core eukaryotic cell biology. Res. Microbiol. 162, 607–618. doi: 10.1016/j.resmic.2011.03.003
Fritz-Laylin, L. K., Prochnik, S. E., Ginger, M. L., Dacks, J. B., Carpenter, M. L., Field, M. C., et al. (2010). The genome of Naegleria gruberi illuminates early eukaryotic versatility. Cell 140, 631–642. doi: 10.1016/j.cell.2010.01.032
Fukui, Y. (1990). Actomyosin organization in mitotic Dictyostelium amoebae. Ann. N. Y. Acad. Sci. 582, 156–165. doi: 10.1111/j.1749-6632.1990.tb21676.x
Fukui, Y., and Inoue, S. (1991). Cell division in Dictyostelium with special emphasis on actomyosin organization in cytokinesis. Cell Motil. Cytoskeleton 18, 41–54. doi: 10.1002/cm.970180105
Fulton, C., and Simpson, P. A. (1976). Selective Synthesis and Utilization of Flagellar tubulin. The Multi-Tubulin Hypothesis. Cold Spring Harbor, NY: Cold Spring Harbor Press.
Gaji, R. Y., Behnke, M. S., Lehmann, M. M., White, M. W., and Carruthers, V. B. (2011). Cell cycle-dependent, intercellular transmission of Toxoplasma gondii is accompanied by marked changes in parasite gene expression. Mol. Microbiol. 79, 192–204. doi: 10.1111/j.1365-2958.2010.07441.x
Gerald, N., Mahajan, B., and Kumar, S. (2011). Mitosis in the human malaria parasite Plasmodium falciparum. Eukaryotic Cell 10, 474–482. doi: 10.1128/EC.00314-10
Ghosh, S. K., and Samuelson, J. (1997). Involvement of p21racA, phosphoinositide 3-kinase, and vacuolar ATPase in phagocytosis of bacteria and erythrocytes by Entamoeba histolytica: suggestive evidence for coincidental evolution of amebic invasiveness. Infect. Immun. 65, 4243–4249.
Gibson, W., Kay, C., and Peacock, L. (2017). Trypanosoma congolense: Molecular toolkit and resources for studying a major livestock pathogen and model trypanosome. Adv. Parasitol. 98, 283–309. doi: 10.1016/bs.apar.2017.03.002
Glotzer, M. (2017). Cytokinesis in Metazoa and Fungi. Cold Spring Harb. Perspect. Biol. 9:a022343. doi: 10.1101/cshperspect.a022343
Gluenz, E., Sharma, R., Carrington, M., and Gull, K. (2008). Functional characterization of cohesin subunit SCC1 in Trypanosoma brucei and dissection of mutant phenotypes in two life cycle stages. Mol. Microbiol. 69, 666–680. doi: 10.1111/j.1365-2958.2008.06320.x
Gonda, K., Katoh, M., Hanyu, K., Watanabe, Y., and Numata, O. (1999). Ca(2+)/calmodulin and p85 cooperatively regulate an initiation of cytokinesis in Tetrahymena. J. Cell Sci. 112 (Pt 21), 3619–3626. doi: 10.1006/bbrc.1999.1354
Gonzalez-Robles, A., Cristobal-Ramos, A. R., Gonzalez-Lazaro, M., Omana-Molina, M., and Martinez-Palomo, A. (2009). Naegleria fowleri: light and electron microscopy study of mitosis. Exp. Parasitol. 122, 212–217. doi: 10.1016/j.exppara.2009.03.016
Gould, G. W. (2016). Animal cell cytokinesis: the role of dynamic changes in the plasma membrane proteome and lipidome. Semin. Cell Dev. Biol. 53, 64–73. doi: 10.1016/j.semcdb.2015.12.012
Gourguechon, S., Holt, L. J., and Cande, W. Z. (2013). The Giardia cell cycle progresses independently of the anaphase-promoting complex. J. Cell Sci. 126, 2246–2255. doi: 10.1242/jcs.121632
Gregory, M. W., Catchpole, J., and Norton, C. C. (1989). Observations on the endogenous stages of Eimeria crandallis in domestic lambs (Ovis aries). Int. J. Parasitol. 19, 907–914. doi: 10.1016/0020-7519(89)90118-5
Gregory, M. W., Catchpole, J., Norton, C. C., and Pittilo, R. M. (1987). Synchronised division of coccidia and their host cells in the ovine intestine. Parasitol. Res. 73, 384–386. doi: 10.1007/BF00531095
Grewal, J. S., and Lohia, A. (2015). “Mechanism of cell division in Entamoeba histolytica,” in Amebiasis, eds T. Nozaki, and A. Bhattacharya (Tokyo: Springer), 263–278. doi: 10.1007/978-4-431-55200-0_16
Grewal, J. S., Padhan, N., Aslam, S., Bhattacharya, A., and Lohia, A. (2013). The calcium binding protein EhCaBP6 is a microtubular-end binding protein in Entamoeba histolytica. Cell. Microbiol. 15, 2020–2033. doi: 10.1111/cmi.12167
Gubbels, M. J., Lehmann, M., Muthalagi, M., Jerome, M. E., Brooks, C. F., Szatanek, T., et al. (2008). Forward genetic analysis of the apicomplexan cell division cycle in Toxoplasma gondii. PLoS Pathog. 4:e36. doi: 10.1371/journal.ppat.0040036
Gubbels, M. J., Vaishnava, S., Boot, N., Dubremetz, J. F., and Striepen, B. (2006). A MORN-repeat protein is a dynamic component of the Toxoplasma gondii cell division apparatus. J. Cell Sci. 119, 2236–2245. doi: 10.1242/jcs.02949
Guillen, N., Boquet, P., and Sansonetti, P. (1998). The small GTP-binding protein RacG regulates uroid formation in the protozoan parasite Entamoeba histolytica. J. Cell Sci. 111, 1729–1739.
Gull, K. (1999). The cytoskeleton of trypanosomatid parasites. Annu. Rev. Microbiol. 53, 629–655. doi: 10.1146/annurev.micro.53.1.629
Gutteridge, R. E., Ndiaye, M. A., Liu, X., and Ahmad, N. (2016). Plk1 inhibitors in cancer therapy: from laboratory to clinics. Mol. Cancer Ther. 15, 1427–1435. doi: 10.1158/1535-7163.MCT-15-0897
Guttery, D. S., Poulin, B., Ramaprasad, A., Wall, R. J., Ferguson, D. J., Brady, D., et al. (2014). Genome-wide functional analysis of Plasmodium protein phosphatases reveals key regulators of parasite development and differentiation. Cell Host Microbe. 16, 128–140. doi: 10.1016/j.chom.2014.05.020
Hall, B. S., Gabernet-Castello, C., Voak, A., Goulding, D., Natesan, S. K., and Field, M. C. (2006). TbVps34, the trypanosome orthologue of Vps34, is required for golgi complex segregation. J. Biol. Chem. 281, 27600–27612. doi: 10.1074/jbc.M602183200
Hallee, S., Counihan, N. A., Matthews, K., De Koning-Ward, T. F., and Richard, D. (2018). The malaria parasite Plasmodium falciparum sortilin is essential for merozoite formation and apical complex biogenesis. Cell. Microbiol. 20:e12844. doi: 10.1111/cmi.12844
Hallyburton, I., Grimaldi, R., Woodland, A., Baragana, B., Luksch, T., Spinks, D., et al. (2017). Screening a protein kinase inhibitor library against Plasmodium falciparum. Malar. J. 16:446. doi: 10.1186/s12936-017-2085-4
Hammarton, T. C., Clark, J., Douglas, F., Boshart, M., and Mottram, J. C. (2003). Stage-specific differences in cell cycle control in Trypanosoma brucei revealed by RNA interference of a mitotic cyclin. J. Biol. Chem 278, 22877–22886. doi: 10.1074/jbc.M300813200
Hammarton, T. C., Kramer, S., Tetley, L., Boshart, M., and Mottram, J. C. (2007). Trypanosoma brucei polo-like kinase is essential for basal body duplication, kDNA segregation and cytokinesis. Mol. Microbiol. 65, 1229–1248. doi: 10.1111/j.1365-2958.2007.05866.x
Hammarton, T. C., Lillico, S. G., Welburn, S. C., and Mottram, J. C. (2005). Trypanosoma brucei MOB1 is required for accurate and efficient cytokinesis but not for exit from mitosis. Mol. Microbiol. 56, 104–116. doi: 10.1111/j.1365-2958.2005.04542.x
Hardin, W. R., Li, R., Xu, J., Shelton, A. M., Alas, G. C. M., Minin, V. N., et al. (2017). Myosin-independent cytokinesis in giardia utilizes flagella to coordinate force generation and direct membrane trafficking. Proc. Natl. Acad. Sci. U.S.A. 114, E5854–E5863. doi: 10.1073/pnas.1705096114
Heaslip, A. T., Dzierszinski, F., Stein, B., and Hu, K. (2010). TgMORN1 is a key organizer for the basal complex of Toxoplasma gondii. PLoS Pathog. 6:e1000754. doi: 10.1371/journal.ppat.1000754
Hemphill, A., Lawson, D., and Seebeck, T. (1991). The cytoskeletal architecture of trypanosoma-brucei. J. Parasitol. 77, 603–612. doi: 10.2307/3283167
Hernández De La Cruz, O., Marchat, L. A., Guillen, N., Weber, C., Lopez Rosas, I., Diaz-Chavez, J., et al. (2016). Multinucleation and polykaryon formation is promoted by the EhPC4 transcription factor in Entamoeba histolytica. Sci. Rep. 6:19611. doi: 10.1038/srep19611
Hilton, N. A., Sladewski, T. E., Perry, J. A., Pataki, Z., Sinclair-Davis, A. N., Muniz, R. S., et al. (2018). Identification of TOEFAZ1-interacting proteins reveals key regulators of Trypanosoma brucei cytokinesis. Mol. Microbiol. 109, 306–326. doi: 10.1111/mmi.13986
Hirono, M., Nakamura, M., Tsunemoto, M., Yasuda, T., Ohba, H., Numata, O., et al. (1987). Tetrahymena actin: localization and possible biological roles of actin in Tetrahymena cells. J. Biochem. 102, 537–545. doi: 10.1093/oxfordjournals.jbchem.a122086
Horlock-Roberts, K., Reaume, C., Dayer, G., Ouellet, C., Cook, N., and Yee, J. (2017). Drug-free approach to study the unusual cell cycle of Giardia intestinalis. mSphere 2, e00384–e00416. doi: 10.1128/mSphere.00384-16
Hosein, R. E., Williams, S. A., Haye, K., and Gavin, R. H. (2003). Expression of GFP-actin leads to failure of nuclear elongation and cytokinesis in Tetrahymena thermophila. J. Eukaryot. Microbiol. 50, 403–408. doi: 10.1111/j.1550-7408.2003.tb00261.x
Hu, H., An, T., Kurasawa, Y., Zhou, Q., and Li, Z. (2019). The trypanosome-specific proteins FPRC and CIF4 regulate cytokinesis initiation by recruiting CIF1 to the cytokinesis initiation site. J. Biol. Chem. 294, 16672–16683. doi: 10.1074/jbc.RA119.010538
Hu, K. (2008). Organizational changes of the daughter basal complex during the parasite replication of Toxoplasma gondii. PLoS Pathog. 4:e10. doi: 10.1371/journal.ppat.0040010
Hu, K., Johnson, J., Florens, L., Fraunholz, M., Suravajjala, S., Dilullo, C., et al. (2006). Cytoskeletal components of an invasion machine–the apical complex of Toxoplasma gondii. PLoS Pathog. 2:e13. doi: 10.1371/journal.ppat.0020013
Hu, K., Mann, T., Striepen, B., Beckers, C. J., Roos, D. S., and Murray, J. M. (2002a). Daughter cell assembly in the protozoan parasite Toxoplasma gondii. Mol. Biol. Cell 13, 593–606. doi: 10.1091/mbc.01-06-0309
Hu, K., Roos, D. S., Angel, S. O., and Murray, J. M. (2004). Variability and heritability of cell division pathways in Toxoplasma gondii. J. Cell Sci. 117, 5697–5705. doi: 10.1242/jcs.01494
Hu, K., Roos, D. S., and Murray, J. M. (2002b). A novel polymer of tubulin forms the conoid of Toxoplasma gondii. J. Cell Biol. 156, 1039–1050. doi: 10.1083/jcb.200112086
Huber, S., Theiler, R., De Quervain, D., Wiens, O., Karangenc, T., Heussler, V., et al. (2017). The Microtubule-stabilizing protein CLASP1 associates with the Theileria annulata schizont surface via its kinetochore-binding domain. mSphere 2, e00215–00217. doi: 10.1128/mSphere.00215-17
Hudson, A. T., Randall, A. W., Fry, M., Ginger, C. D., Hill, B., Latter, V. S., et al. (1985). Novel anti-malarial hydroxynaphthoquinones with potent broad spectrum anti-protozoal activity. Parasitology 90, 45–55. doi: 10.1017/S0031182000049003
Hulliger, L., Wilde, K. H., Brown, C. G., and Turner, L. (1964). Mode of multiplication of Theileria in cultures of bovine lymphocytic cells. Nature 203, 728–730. doi: 10.1038/203728a0
Ikeda, K. N., and de Graffenried, C. L. (2012). Polo-like kinase is necessary for flagellum inheritance in Trypanosoma brucei. J. Cell Sci. 125, 3173–3184. doi: 10.1242/jcs.101162
Irvin, A. D., Ocama, J. G., and Spooner, P. R. (1982). Cycle of bovine lymphoblastoid cells parasitised by Theileria parva. Res. Vet. Sci. 33, 298–304. doi: 10.1016/S0034-5288(18)32305-1
Jacot, D., Daher, W., and Soldati-Favre, D. (2013). Toxoplasma gondii myosin F, an essential motor for centrosomes positioning and apicoplast inheritance. EMBO J. 32, 1702–1716. doi: 10.1038/emboj.2013.113
Jalovecka, M., Hajdusek, O., Sojka, D., Kopacek, P., and Malandrin, L. (2018). The complexity of piroplasms life cycles. Front. Cell. Infect. Microbiol. 8:248. doi: 10.3389/fcimb.2018.00248
Jerome, C. A., Simon, E. M., and Lynn, D. H. (1996). Description of Tetrahymena empidokyrea n sp, a new species in the Tetrahymena pyriformis sibling species complex (Ciliophora, Oligohymenophorea), and an assessment of its phylogenetic position using small-subunit rRNA sequences. Can. J. Microbiol. 74, 1898–1906. doi: 10.1139/z96-214
Jiang, Y. Y., Maier, W., Baumeister, R., Joachimiak, E., Ruan, Z., Kannan, N., et al. (2019). Two antagonistic Hippo signaling circuits set the division plane at the medial position in the ciliate Tetrahymena. Genetics 211, 651–663. doi: 10.1534/genetics.118.301889
Jiang, Y. Y., Maier, W., Baumeister, R., Minevich, G., Joachimiak, E., Ruan, Z., et al. (2017). The Hippo pathway maintains the equatorial division plane in the ciliate Tetrahymena. Genetics 206, 873–888. doi: 10.1534/genetics.117.200766
Jirage, D., Chen, Y., Caridha, D., O'neil, M. T., Eyase, F., Witola, W. H., et al. (2010). The malarial CDK Pfmrk and its effector PfMAT1 phosphorylate DNA replication proteins and co-localize in the nucleus. Mol. Biochem. Parasitol. 172, 9–18. doi: 10.1016/j.molbiopara.2010.03.009
Jojic, B., Amodeo, S., Bregy, I., and Ochsenreiter, T. (2018a). Distinct 3' UTRs regulate the life-cycle-specific expression of two TCTP paralogs in Trypanosoma brucei. J. Cell Sci. 131:jcs206417. doi: 10.1242/jcs.206417
Jojic, B., Amodeo, S., and Ochsenreiter, T. (2018b). The translationally controlled tumor protein TCTP is involved in cell cycle progression and heat stress response in the bloodstream form of Trypanosoma brucei. Microb. Cell 5, 460–468. doi: 10.15698/mic2018.10.652
Jones, A. J., Kaiser, M., and Avery, V. M. (2015). Identification and characterization of FTY720 for the treatment of human African trypanosomiasis. Antimicrob. Agents Chemother. 60, 1859–1861. doi: 10.1128/AAC.02116-15
Jones, N. G., Thomas, E. B., Brown, E., Dickens, N. J., Hammarton, T. C., and Mottram, J. C. (2014). Regulators of Trypanosoma brucei cell cycle progression and differentiation identified using a kinome-wide RNAi screen. PLoS Pathog. 10:e1003886. doi: 10.1371/journal.ppat.1003886
Kim, J., Lee, H. Y., Lee, K. H., and Park, S. J. (2017). Phosphorylation of serine 148 in Giardia lamblia end-binding 1 protein is important for cell division. J. Eukaryot. Microbiol. 64, 464–480. doi: 10.1111/jeu.12384
Kim, J., and Park, S. J. (2019). Roles of end-binding 1 protein and gamma-tubulin small complex in cytokinesis and flagella formation of Giardia lamblia. Microbiologyopen 8:e00748. doi: 10.1002/mbo3.748
Kinnaird, J. H., Bumstead, J. M., Mann, D. J., Ryan, R., Shirley, M. W., Shiels, B. R., et al. (2004). EtCRK2, a cyclin-dependent kinase gene expressed during the sexual and asexual phases of the Eimeria tenella life cycle. Int. J. Parasitol. 34, 683–692. doi: 10.1016/j.ijpara.2004.01.003
Klaeger, S., Heinzlmeir, S., Wilhelm, M., Polzer, H., Vick, B., Koenig, P. A., et al. (2017). The target landscape of clinical kinase drugs. Science 358:eaan4368. doi: 10.1126/science.aan4368
Kohl, L., Robinson, D., and Bastin, P. (2003). Novel roles for the flagellum in cell morphogenesis and cytokinesis of trypanosomes. EMBO J. 22, 5336–5346. doi: 10.1093/emboj/cdg518
Kolev, N. G., Ramey-Butler, K., Cross, G. A., Ullu, E., and Tschudi, C. (2012). Developmental progression to infectivity in Trypanosoma brucei triggered by an RNA-binding protein. Science 338, 1352–1353. doi: 10.1126/science.1229641
Kono, M., Heincke, D., Wilcke, L., Wong, T. W., Bruns, C., Herrmann, S., et al. (2016). Pellicle formation in the malaria parasite. J. Cell Sci. 129, 673–680. doi: 10.1242/jcs.181230
Kono, M., Herrmann, S., Loughran, N. B., Cabrera, A., Engelberg, K., Lehmann, C., et al. (2012). Evolution and architecture of the inner membrane complex in asexual and sexual stages of the malaria parasite. Mol. Biol. Evol. 29, 2113–2132. doi: 10.1093/molbev/mss081
Krishnan, D., and Ghosh, S. K. (2018). Cellular events of multinucleated giant cells formation during the encystation of Entamoeba invadens. Front. Cell. Infect. Microbiol. 8:262. doi: 10.3389/fcimb.2018.00262
Kumar, P., and Wang, C. C. (2005). Depletion of APC/C subunit homolog APC1 or CDC27 of Trypanosoma brucei arrests the procyclic form in metaphase but the bloodstream form in anaphase. J. Biol. Chem. 280, 31783–31791. doi: 10.1074/jbc.M504326200
Kumar, P., and Wang, C. C. (2006). Dissociation of cytokinesis initiation from mitotic control in a eukaryote. Eukaryotic Cell 5, 92–102. doi: 10.1128/EC.5.1.92-102.2006
Kurasawa, Y., Hu, H., Zhou, Q., and Li, Z. (2018). The trypanosome-specific protein CIF3 cooperates with the CIF1 protein to promote cytokinesis in Trypanosoma brucei. J. Biol. Chem. 293, 10275–10286. doi: 10.1074/jbc.RA118.003113
Kusdian, G., Woehle, C., Martin, W. F., and Gould, S. B. (2013). The actin-based machinery of Trichomonas vaginalis mediates flagellate-amoeboid transition and migration across host tissue. Cell. Microbiol. 15, 1707–1721. doi: 10.1111/cmi.12144
Lai, D. H., Hashimi, H., Lun, Z. R., Ayala, F. J., and Lukes, J. (2008). Adaptations of Trypanosoma brucei to gradual loss of kinetoplast DNA: Trypanosoma equiperdum and Trypanosoma evansi are petite mutants of T. brucei. Proc. Natl. Acad. Sci. U.S.A. 105, 1999–2004. doi: 10.1073/pnas.0711799105
Lander, N., Chiurillo, M. A., and Docampo, R. (2019). Genome editing by CRISPR/Cas9 in Trypanosoma cruzi. Methods Mol. Biol. 1955, 61–76. doi: 10.1007/978-1-4939-9148-8_5
Le Roch, K., Sestier, C., Dorin, D., Waters, N., Kappes, B., Chakrabarti, D., et al. (2000). Activation of a Plasmodium falciparum cdc2-related kinase by heterologous p25 and cyclin H - Functional characterization of a P. falciparum cyclin homologue. J. Biol. Chem. 275, 8952–8958. doi: 10.1074/jbc.275.12.8952
Li, Z., Gourguechon, S., and Wang, C. C. (2007). Tousled-like kinase in a microbial eukaryote regulates spindle assembly and S-phase progression by interacting with Aurora kinase and chromatin assembly factors. J. Cell Sci. 120, 3883–3894. doi: 10.1242/jcs.007955
Li, Z., Lee, J. H., Chu, F., Burlingame, A. L., Gunzl, A., and Wang, C. C. (2008a). Identification of a novel chromosomal passenger complex and its unique localization during cytokinesis in Trypanosoma brucei. PLoS ONE 3:e2354. doi: 10.1371/journal.pone.0002354
Li, Z., Umeyama, T., Li, Z., and Wang, C. C. (2010). Polo-like kinase guides cytokinesis in Trypanosoma brucei through an indirect means. Eukaryotic Cell 9, 705–716. doi: 10.1128/EC.00330-09
Li, Z., Umeyama, T., and Wang, C. C. (2008b). The chromosomal passenger complex and a mitotic kinesin interact with the Tousled-like kinase in trypanosomes to regulate mitosis and cytokinesis. PLoS ONE 3:e3814. doi: 10.1371/journal.pone.0003814
Li, Z., Umeyama, T., and Wang, C. C. (2009). The Aurora kinase in Trypanosoma brucei plays distinctive roles in metaphase-anaphase transition and cytokinetic initiation. PLoS Pathog. 5:e1000575. doi: 10.1371/journal.ppat.1000575
Li, Z., and Wang, C. C. (2008). KMP-11, a basal body and flagellar protein, is required for cell division in Trypanosoma brucei. Eukaryotic Cell 7, 1941–1950. doi: 10.1128/EC.00249-08
Li, Z. Y., and Wang, C. C. (2006). Changing roles of aurora-B kinase in two life cycle stages of Trypanosoma brucei. Eukaryotic Cell 5, 1026–1035. doi: 10.1128/EC.00129-06
Lige, B., Romano, J. D., Bandaru, V. V., Ehrenman, K., Levitskaya, J., Sampels, V., et al. (2011). Deficiency of a Niemann-Pick, type C1-related protein in Toxoplasma is associated with multiple lipidoses and increased pathogenicity. PLoS Pathog. 7:e1002410. doi: 10.1371/journal.ppat.1002410
Lillico, S. G., Field, M. C., Blundell, P., Coombs, G. H., and Mottram, J. C. (2003). Essential roles for GPI-anchored proteins in African trypanosomes revealed using mutants deficient in GPI8. Mol. Biol. Cell 14, 1182–1194. doi: 10.1091/mbc.e02-03-0167
Livanos, P., and Muller, S. (2019). Division plane establishment and cytokinesis. Annu. Rev. Plant Biol. 70, 239–267. doi: 10.1146/annurev-arplant-050718-100444
Lohia, A. (2003). The cell cycle of Entamoeba histolytica. Mol. Cell. Biochem. 253, 217–222. doi: 10.1023/A:1026055631421
Lohia, A., Mukherjee, C., Majumder, S., and Dastidar, P. G. (2007). Genome re-duplication and irregular segregation occur during the cell cycle of Entamoeba histolytica. Biosci. Rep. 27, 373–384. doi: 10.1007/s10540-007-9058-8
Lorestani, A., Sheiner, L., Yang, K., Robertson, S. D., Sahoo, N., Brooks, C. F., et al. (2010). A Toxoplasma MORN1 null mutant undergoes repeated divisions but is defective in basal assembly, apicoplast division and cytokinesis. PLoS ONE 5:e12302. doi: 10.1371/journal.pone.0012302
Lozano-Nunez, A., Ikeda, K. N., Sauer, T., and De Graffenried, C. L. (2013). An analogue-sensitive approach identifies basal body rotation and flagellum attachment zone elongation as key functions of PLK in Trypanosoma brucei. Mol. Biol. Cell 24, 1321–1333. doi: 10.1091/mbc.e12-12-0846
Lynn, D. H., Gransden, S. G., Wright, A. D. G., and Josephson, G. (2000). Characterization of a new species of the ciliate Tetrahymena (Ciliophora: Oligohymenophorea) isolated from the urine of a dog: first report of Tetrahymena from a mammal. Acta Protozool. 39, 289–294.
Ma, J., Benz, C., Grimaldi, R., Stockdale, C., Wyatt, P., Frearson, J., et al. (2010). Nuclear DBF-2-related kinases are essential regulators of cytokinesis in bloodstream stage Trypanosoma brucei. J. Biol. Chem. 285, 15356–15368. doi: 10.1074/jbc.M109.074591
Majumder, S., and Lohia, A. (2008). Entamoeba histolytica encodes unique formins, a subset of which regulates DNA content and cell division. Infect. Immun. 76, 2368–2378. doi: 10.1128/IAI.01449-07
Mann, T., Gaskins, E., and Beckers, C. (2002). Proteolytic processing of TgIMC1 during maturation of the membrane skeleton of Toxoplasma gondii. J. Biol. Chem. 277, 41240–41246. doi: 10.1074/jbc.M205056200
Manning, G., Reiner, D. S., Lauwaet, T., Dacre, M., Smith, A., Zhai, Y., et al. (2011). The minimal kinome of Giardia lamblia illuminates early kinase evolution and unique parasite biology. Genome Biol. 12:R66. doi: 10.1186/gb-2011-12-7-r66
Mathews, E. S., and Odom John, A. R. (2018). Tackling resistance: emerging antimalarials and new parasite targets in the era of elimination. F1000Res 7:1170. doi: 10.12688/f1000research.14874.1
Matthews, K. R. (2005). The developmental cell biology of Trypanosoma brucei. J. Cell Sci. 118, 283–290. doi: 10.1242/jcs.01649
May, S. F., Peacock, L., Almeida Costa, C. I., Gibson, W. C., Tetley, L., Robinson, D. R., et al. (2012). The Trypanosoma brucei AIR9-like protein is cytoskeleton-associated and is required for nucleus positioning and accurate cleavage furrow placement. Mol. Microbiol. 84, 77–92. doi: 10.1111/j.1365-2958.2012.08008.x
Mcallaster, M. R., Ikeda, K. N., Lozano-Nunez, A., Anrather, D., Unterwurzacher, V., Gossenreiter, T., et al. (2015). Proteomic identification of novel cytoskeletal proteins associated with TbPLK, an essential regulator of cell morphogenesis in Trypanosoma brucei. Mol. Biol. Cell 26, 3013–3029. doi: 10.1091/mbc.E15-04-0219
McInally, S. G., and Dawson, S. C. (2016). Eight unique basal bodies in the multi-flagellated diplomonad Giardia lamblia. Cilia 5:21. doi: 10.1186/s13630-016-0042-4
McKenzie, C., Bassi, Z. I., Debski, J., Gottardo, M., Callaini, G., Dadlez, M., et al. (2016). Cross-regulation between Aurora B and Citron kinase controls midbody architecture in cytokinesis. Open Biol. 6:160019. doi: 10.1098/rsob.160019
Mehlhorn, H., and Schein, E. (1976). [Electron microscope studies on developmental stages of Theileria parva (Theiler, 1904) in the intestine of the tick Hyalomma anatolicum excavatum (Koch, 1844) (author's transl)]. Tropenmed. Parasitol. 27, 182–191.
Minocha, N., Kumar, D., Rajanala, K., and Saha, S. (2011). Kinetoplast morphology and segregation pattern as a marker for cell cycle progression in Leishmania donovani. J. Eukaryot. Microbiol. 58, 249–253. doi: 10.1111/j.1550-7408.2011.00539.x
Miranda-Saavedra, D., Gabaldon, T., Barton, G. J., Langsley, G., and Doerig, C. (2012). The kinomes of apicomplexan parasites. Microbes. Infect. 14, 796–810. doi: 10.1016/j.micinf.2012.04.007
Moreira, B. P., Fonseca, C. K., Hammarton, T. C., and Baqui, M. M. (2017). Giant FAZ10 is required for flagellum attachment zone stabilization and furrow positioning in Trypanosoma brucei. J. Cell Sci. 130, 1179–1193. doi: 10.1242/jcs.194308
Moreira-Leite, F. F., Sherwin, T., Kohl, L., and Gull, K. (2001). A trypanosome structure involved in transmitting cytoplasmic information during cell division. Science 294, 610–612. doi: 10.1126/science.1063775
Morlon-Guyot, J., Berry, L., Chen, C. T., Gubbels, M. J., Lebrun, M., and Daher, W. (2014). The Toxoplasma gondii calcium-dependent protein kinase 7 is involved in early steps of parasite division and is crucial for parasite survival. Cell. Microbiol. 16, 95–114. doi: 10.1111/cmi.12186
Morlon-Guyot, J., Francia, M. E., Dubremetz, J. F., and Daher, W. (2017). Towards a molecular architecture of the centrosome in Toxoplasma gondii. Cytoskeleton (Hoboken). 74, 55–71. doi: 10.1002/cm.21353
Morrissette, N. S., and Sibley, L. D. (2002a). Cytoskeleton of apicomplexan parasites. Microbiol. Mol. Biol. Rev. 66, 21–38. doi: 10.1128/MMBR.66.1.21-38.2002
Morrissette, N. S., and Sibley, L. D. (2002b). Disruption of microtubules uncouples budding and nuclear division in Toxoplasma gondii. J. Cell Sci. 115, 1017–1025.
Mukherjee, C., Majumder, S., and Lohia, A. (2009). Inter-cellular variation in DNA content of Entamoeba histolytica originates from temporal and spatial uncoupling of cytokinesis from the nuclear cycle. PLoS Negl. Trop. Dis. 3:e409. doi: 10.1371/journal.pntd.0000409
Muniz-Hernandez, S., Carmen, M. G., Mondragon, M., Mercier, C., Cesbron, M. F., Mondragon-Gonzalez, S. L., et al. (2011). Contribution of the residual body in the spatial organization of Toxoplasma gondii tachyzoites within the parasitophorous vacuole. J. Biomed. Biotechnol. 2011:473983. doi: 10.1155/2011/473983
Murungi, E. K., and Kariithi, H. M. (2017). Genome-wide identification and evolutionary analysis of Sarcocystis neurona protein kinases. Pathogens 6:E12. doi: 10.3390/pathogens6010012
Nakamura, K., Yokoyama, N., and Igarashi, I. (2007). Cyclin-dependent kinase inhibitors block erythrocyte invasion and intraerythrocytic development of Babesia bovis in vitro. Parasitology 134, 1347–1353. doi: 10.1017/S0031182007002831
Nerusheva, O. O., and Akiyoshi, B. (2016). Divergent polo box domains underpin the unique kinetoplastid kinetochore. Open Biol. 6:150206. doi: 10.1098/rsob.150206
Nilles-Bije, M. L., and Rivera, W. L. (2010). Ultrastructural and molecular characterization of Balantidium coli isolated in the Philippines. Parasitol. Res. 106, 387–394. doi: 10.1007/s00436-009-1673-9
Nishi, M., Hu, K., Murray, J. M., and Roos, D. S. (2008). Organellar dynamics during the cell cycle of Toxoplasma gondii. J. Cell Sci. 121, 1559–1568. doi: 10.1242/jcs.021089
Numata, O., Fujiu, K., and Gonda, K. (1999). Macronuclear division and cytokinesis in Tetrahymena. Cell Biol. Int. 23, 849–857. doi: 10.1006/cbir.1999.0481
Numata, O., and Gonda, K. (2001). Determination of division plane and organization of contractile ring in Tetrahymena. Cell Struct. Funct. 26, 593–601. doi: 10.1247/csf.26.593
Numata, O., Kurasawa, Y., Gonda, K., and Watanabe, Y. (2000). Tetrahymena elongation factor-1 alpha is localized with calmodulin in the division furrow. J. Biochem. 127, 51–56. doi: 10.1093/oxfordjournals.jbchem.a022583
Ochiana, S. O., Bland, N. D., Settimo, L., Campbell, R. K., and Pollastri, M. P. (2015). Repurposing human PDE4 inhibitors for neglected tropical diseases. Evaluation of analogs of the human PDE4 inhibitor GSK-256066 as inhibitors of PDEB1 of Trypanosoma brucei. Chem. Biol. Drug Des. 85, 549–564. doi: 10.1111/cbdd.12443
Ochiana, S. O., Pandarinath, V., Wang, Z., Kapoor, R., Ondrechen, M. J., Ruben, L., et al. (2013). The human Aurora kinase inhibitor danusertib is a lead compound for anti-trypanosomal drug discovery via target repurposing. Eur. J. Med. Chem. 62, 777–784. doi: 10.1016/j.ejmech.2012.07.038
Odronitz, F., and Kollmar, M. (2007). Drawing the tree of eukaryotic life based on the analysis of 2,269 manually annotated myosins from 328 species. Genome Biol. 8:R196. doi: 10.1186/gb-2007-8-9-r196
O'Hara, S. P., and Chen, X. M. (2011). The cell biology of cryptosporidium infection. Microbes. Infect. 13, 721–730. doi: 10.1016/j.micinf.2011.03.008
Ohba, H., Hirono, M., Edamatsu, M., and Watanabe, Y. (1992). Timing of formation of Tetrahymena contractile ring microfilaments investigated by inhibition with skeletal-muscle actin. Dev. Genet. 13, 210–215. doi: 10.1002/dvg.1020130306
Ojo, K. K., Eastman, R. T., Vidadala, R., Zhang, Z., Rivas, K. L., Choi, R., et al. (2014). A specific inhibitor of PfCDPK4 blocks malaria transmission: chemical-genetic validation. J. Infect. Dis. 209, 275–284. doi: 10.1093/infdis/jit522
Ooi, C. P., Schuster, S., Cren-Travaille, C., Bertiaux, E., Cosson, A., Goyard, S., et al. (2016). The cyclical development of Trypanosoma vivax in the tsetse fly involves an asymmetric division. Front. Cell. Infect. Microbiol. 6, 115. doi: 10.3389/fcimb.2016.00115
Orozco, E., Solis, F. J., Dominguez, J., Chavez, B., and Hernandez, F. (1988). Entamoeba histolytica- cell cycle and nuclear division. Exp. Parasitol. 67, 85–95. doi: 10.1016/0014-4894(88)90011-2
Orr, G. A., Werner, C., Xu, J., Bennett, M., Weiss, L. M., Takvorkan, P., et al. (2000). Identification of novel serine/threonine protein phosphatases in Trypanosoma cruzi: a potential role in control of cytokinesis and morphology. Infect. Immun. 68, 1350–1358. doi: 10.1128/IAI.68.3.1350-1358.2000
Ouologuem, D. T., and Roos, D. S. (2014). Dynamics of the Toxoplasma gondii inner membrane complex. J. Cell Sci. 127, 3320–3330. doi: 10.1242/jcs.147736
Paredez, A. R., Assaf, Z. J., Sept, D., Timofejeva, L., Dawson, S. C., Wang, C. J., et al. (2011). An actin cytoskeleton with evolutionarily conserved functions in the absence of canonical actin-binding proteins. Proc. Natl. Acad. Sci. U.S.A. 108, 6151–6156. doi: 10.1073/pnas.1018593108
Patel, G., Roncal, N. E., Lee, P. J., Leed, S. E., Erath, J., Rodriguez, A., et al. (2014). Repurposing human Aurora kinase inhibitors as leads for anti-protozoan drug discovery. Medchemcomm 5, 655–658. doi: 10.1039/C4MD00045E
Peacock, L., Cook, S., Ferris, V., Bailey, M., and Gibson, W. (2012). The life cycle of Trypanosoma (Nannomonas) congolense in the tsetse fly. Parasites Vectors 5:109. doi: 10.1186/1756-3305-5-109
Peacock, L., Kay, C., Bailey, M., and Gibson, W. (2018). Shape-shifting trypanosomes: flagellar shortening followed by asymmetric division in Trypanosoma congolense from the tsetse proventriculus. PLoS Pathog. 14:e1007043. doi: 10.1371/journal.ppat.1007043
Pena, I., Pilar Manzano, M., Cantizani, J., Kessler, A., Alonso-Padilla, J., Bardera, A. I., et al. (2015). New compound sets identified from high throughput phenotypic screening against three kinetoplastid parasites: an open resource. Sci. Rep. 5:8771. doi: 10.1038/srep08771
Penzo, M., De Las Heras-Duena, L., Mata-Cantero, L., Diaz-Hernandez, B., Vazquez-Muniz, M. J., Ghidelli-Disse, S., et al. (2019). High-throughput screening of the Plasmodium falciparum cGMP-dependent protein kinase identified a thiazole scaffold which kills erythrocytic and sexual stage parasites. Sci. Rep. 9:7005. doi: 10.1038/s41598-019-42801-x
Pham, K. T. M., Zhou, Q., Kurasawa, Y., and Li, Z. (2019). BOH1 cooperates with Polo-like kinase to regulate flagellum inheritance and cytokinesis initiation in Trypanosoma brucei. J. Cell Sci. 132:jcs230581. doi: 10.1242/jcs.230581
Pisa, R., Cupido, T., and Kapoor, T. M. (2019). Designing allele-specific inhibitors of spastin, a microtubule-severing AAA protein. J. Am. Chem. Soc. 141, 5602–5606. doi: 10.1021/jacs.8b13257
Pollard, T. D., and O'Shaughnessy, B. (2019). Molecular mechanism of cytokinesis. Annu. Rev. Biochem. 88, 661–689. doi: 10.1146/annurev-biochem-062917-012530
Pollard, T. D., and Wu, J. Q. (2010). Understanding cytokinesis: lessons from fission yeast. Nat. Rev. Mol. Cell Biol. 11, 149–155. doi: 10.1038/nrm2834
Potenza, M., and Tellez-Inon, M. T. (2015). Colchicine treatment reversibly blocks cytokinesis but not mitosis in Trypanosoma cruzi epimastigotes. Parasitol. Res. 114, 641–649. doi: 10.1007/s00436-014-4227-8
Preisner, H., Karin, E. L., Poschmann, G., Stuhler, K., Pupko, T., and Gould, S. B. (2016). The cytoskeleton of parabasalian parasites comprises proteins that share properties common to intermediate filament proteins. Protist 167, 526–543. doi: 10.1016/j.protis.2016.09.001
Price, H. P., Peltan, A., Stark, M., and Smith, D. F. (2010). The small GTPase ARL2 is required for cytokinesis in Trypanosoma brucei. Mol. Biochem. Parasitol. 173, 123–131. doi: 10.1016/j.molbiopara.2010.05.016
Qiao, J., Lin, G., Xia, A., Xiang, Z., Chen, P., Zhang, G., et al. (2019). Discovery of 1,8-disubstituted-[1,2,3]triazolo[4,5-c]quinoline derivatives as a new class of Hippo signaling pathway inhibitors. Bioorg. Med. Chem. Lett. 29, 2595–2603. doi: 10.1016/j.bmcl.2019.08.001
Radke, J. R., Striepen, B., Guerini, M. N., Jerome, M. E., Roos, D. S., and White, M. W. (2001). Defining the cell cycle for the tachyzoite stage of Toxoplasma gondii. Mol. Biochem. Parasitol. 115, 165–175. doi: 10.1016/S0166-6851(01)00284-5
Ralston, K. S., Lerner, A. G., Diener, D. R., and Hill, K. L. (2006). Flagellar motility contributes to cytokinesis in Trypanosoma brucei and is modulated by an evolutionarily conserved dynein regulatory system. Eukaryotic Cell 5, 696–711. doi: 10.1128/EC.5.4.696-711.2006
Rangarajan, R., Bei, A. K., Jethwaney, D., Maldonado, P., Dorin, D., Sultan, A. A., et al. (2005). A mitogen-activated protein kinase regulates male gametogenesis and transmission of the malaria parasite Plasmodium berghei. EMBO Rep. 6, 464–469. doi: 10.1038/sj.embor.7400404
Regmi, S., Rothberg, K., Hubbard, J. G., and Ruben, L. (2008). The RACK1 signal anchor protein from Trypanosoma brucei associates with eukaryotic elongation factor 1A: a role for translational control in cytokinesis. Mol. Microbiol. 70, 724–745. doi: 10.1111/j.1365-2958.2008.06443.x
Ribeiro, K. C., Monteiro-Leal, L. H., and Benchimol, M. (2000). Contributions of the axostyle and flagella to closed mitosis in the protists Tritrichomonas foetus and Trichomonas vaginalis. J. Eukaryot. Microbiol. 47, 481–492. doi: 10.1111/j.1550-7408.2000.tb00077.x
Richards, T. A., and Cavalier-Smith, T. (2005). Myosin domain evolution and the primary divergence of eukaryotes. Nature 436, 1113–1118. doi: 10.1038/nature03949
Robbins, J. A., Absalon, S., Streva, V. A., and Dvorin, J. D. (2017). The malaria parasite cyclin H homolog PfCyc1 is required for efficient cytokinesis in blood-stage Plasmodium falciparum. MBio 8, e00605–e00617. doi: 10.1128/mBio.00605-17
Robinson, D. R., Sherwin, T., Ploubidou, A., Byard, E. H., and Gull, K. (1995). Microtubule polarity and dynamics in the control of organelle positioning, segregation, and cytokinesis in the trypanosome cell cycle. J. Cell Biol. 128, 1163–1172. doi: 10.1083/jcb.128.6.1163
Rodgers, M. J., Albanesi, J. P., and Phillips, M. A. (2007). Phosphatidylinositol 4-kinase III-beta is required for Golgi maintenance and cytokinesis in Trypanosoma brucei. Eukaryotic Cell 6, 1108–1118. doi: 10.1128/EC.00107-07
Rojas, F., Koszela, J., Bua, J., Llorente, B., Burchmore, R., Auer, M., et al. (2017). The ubiquitin-conjugating enzyme CDC34 is essential for cytokinesis in contrast to putative subunits of a SCF complex in Trypanosoma brucei. PLoS Negl. Trop. Dis. 11:e0005626. doi: 10.1371/journal.pntd.0005626
Roques, M., Wall, R. J., Douglass, A. P., Ramaprasad, A., Ferguson, D. J., Kaindama, M. L., et al. (2015). Plasmodium P-type cyclin CYC3 modulates endomitotic growth during oocyst development in mosquitoes. PLoS Pathog. 11:e1005273. doi: 10.1371/journal.ppat.1005273
Rothberg, K. G., Burdette, D. L., Pfannstiel, J., Jetton, N., Singh, R., and Ruben, L. (2006). The RACK1 homologue from Trypanosoma brucei is required for the onset and progression of cytokinesis. J. Biol. Chem. 281, 9781–9790. doi: 10.1074/jbc.M600133200
Rotureau, B., Subota, I., Buisson, J., and Bastin, P. (2012). A new asymmetric division contributes to the continuous production of infective trypanosomes in the tsetse fly. Development 139, 1842–1850. doi: 10.1242/dev.072611
Rudlaff, R. M., Kraemer, S., Streva, V. A., and Dvorin, J. D. (2019). An essential contractile ring protein controls cell division in Plasmodium falciparum. Nat. Commun. 10:2181. doi: 10.1038/s41467-019-10214-z
Saeed, S., Lau, C. I., Tremp, A. Z., Crompton, T., and Dessens, J. T. (2019). Dysregulated gene expression in oocysts of Plasmodium berghei LAP mutants. Mol. Biochem. Parasitol. 229, 1–5. doi: 10.1016/j.molbiopara.2019.02.001
Saeed, S., Tremp, A. Z., and Dessens, J. T. (2018). The Plasmodium LAP complex affects crystalloid biogenesis and oocyst cell division. Int. J. Parasitol. 48, 1073–1078. doi: 10.1016/j.ijpara.2018.09.002
Sagolla, M. S., Dawson, S. C., Mancuso, J. J., and Cande, W. Z. (2006). Three-dimensional analysis of mitosis and cytokinesis in the binucleate parasite Giardia intestinalis. J. Cell Sci. 119, 4889–4900. doi: 10.1242/jcs.03276
Salmon, D., Bachmaier, S., Krumbholz, C., Kador, M., Gossmann, J. A., Uzureau, P., et al. (2012). Cytokinesis of Trypanosoma brucei bloodstream forms depends on expression of adenylyl cyclases of the ESAG4 or ESAG4-like subfamily. Mol. Microbiol. 84, 225–242. doi: 10.1111/j.1365-2958.2012.08013.x
Santi, A. M. M., Lanza, J. S., Tunes, L. G., Fiuza, J. A., Roy, G., Orfano, A. D. S., et al. (2018). Growth arrested live-attenuated Leishmania infantum KHARON1 null mutants display cytokinesis defect and protective immunity in mice. Sci. Rep. 8:11627. doi: 10.1038/s41598-018-30076-7
Schein, E., Mehlhorn, H., and Warnecke, M. (1977). [Fine structural study on the erythrocytic stages of Theileria annulata (Dschunkowsky, Luhs, 1904) (author's transl)]. Tropenmed. Parasitol. 28, 349–360.
Schneider, I., Haller, D., Kullmann, B., Beyer, D., Ahmed, J. S., and Seitzer, U. (2007). Identification, molecular characterization and subcellular localization of a Theileria annulata parasite protein secreted into the host cell cytoplasm. Parasitol. Res. 101, 1471–1482. doi: 10.1007/s00436-007-0663-z
Schoijet, A. C., Miranda, K., Sternlieb, T., Barrera, N. M., Girard-Dias, W., De Souza, W., et al. (2018). TbVps15 is required for vesicular transport and cytokinesis in Trypanosoma brucei. Mol. Biochem. Parasitol. 219, 33–41. doi: 10.1016/j.molbiopara.2017.11.004
Schuster, F. L. (1975). Ultrastructure of mitosis in ameboflagellate Naegleria-Gruberi. Tissue Cell 7, 1–11. doi: 10.1016/S0040-8166(75)80003-6
Schuster, F. L., and Ramirez-Avila, L. (2008). Current world status of Balantidium coli. Clin. Microbiol. Rev. 21, 626–638. doi: 10.1128/CMR.00021-08
Sebe-Pedros, A., Grau-Bove, X., Richards, T. A., and Ruiz-Trillo, I. (2014). Evolution and classification of myosins, a paneukaryotic whole-genome approach. Genome Biol. Evol. 6, 290–305. doi: 10.1093/gbe/evu013
Seiler, S., and Justa-Schuch, D. (2010). Conserved components, but distinct mechanisms for the placement and assembly of the cell division machinery in unicellular and filamentous ascomycetes. Mol. Microbiol. 78, 1058–1076. doi: 10.1111/j.1365-2958.2010.07392.x
Seitzer, U., Gerber, S., Beyer, D., Dobschanski, J., Kullmann, B., Haller, D., et al. (2010). Schizonts of Theileria annulata interact with the microtubuli network of their host cell via the membrane protein TaSP. Parasitol. Res. 106, 1085–1102. doi: 10.1007/s00436-010-1747-8
Selvapandiyan, A., Debrabant, A., Duncan, R., Muller, J., Salotra, P., Sreenivas, G., et al. (2004). Centrin gene disruption impairs stage-specific basal body duplication and cell cycle progression in Leishmania. J. Biol. Chem. 279, 25703–25710. doi: 10.1074/jbc.M402794200
Sharma, N., Bryant, J., Wloga, D., Donaldson, R., Davis, R. C., Jerka-Dziadosz, M., et al. (2007). Katanin regulates dynamics of microtubules and biogenesis of motile cilia. J. Cell Biol. 178, 1065–1079. doi: 10.1083/jcb.200704021
Shaw, M. K., Compton, H. L., Roos, D. S., and Tilney, L. G. (2000). Microtubules, but not actin filaments, drive daughter cell budding and cell division in Toxoplasma gondii. J. Cell Sci. 113, 1241–1254.
Shaw, M. K., and Tilney, L. G. (1992). How individual cells develop from a syncytium: merogony in Theileria parva (Apicomplexa). J. Cell Sci. 101, 109–123.
Sheader, K., Vaughan, S., Minchin, J., Hughes, K., Gull, K., and Rudenko, G. (2005). Variant surface glycoprotein RNA interference triggers a precytokinesis cell cycle arrest in African trypanosomes. Proc. Natl. Acad. Sci. U. S. A 102, 8716–8721. doi: 10.1073/pnas.0501886102
Sheffield, H. G., and Melton, M. L. (1968). The fine structure and reproduction of Toxoplasma gondii. J. Parasitol. 54, 209–226. doi: 10.2307/3276925
Sherwin, T., and Gull, K. (1989). Visualization of detyrosination along single microtubules reveals novel mechanisms of assembly during cytoskeletal duplication in trypanosomes. Cell 57, 211–221. doi: 10.1016/0092-8674(89)90959-8
Shimizu, Y., Kushida, Y., Kiriyama, S., Nakano, K., and Numata, O. (2013). Formation and ingression of division furrow can progress under the inhibitory condition of actin polymerization in ciliate Tetrahymena pyriformis. Zool. Sci. 30, 1044–1049. doi: 10.2108/zsj.30.1044
Shiozaki, N., Nakano, K., Kushida, Y., Noguchi, T. Q., Uyeda, T. Q., Wloga, D., et al. (2013). ADF/cofilin is not essential but is critically important for actin activities during phagocytosis in Tetrahymena thermophila. Eukaryotic Cell 12, 1080–1086. doi: 10.1128/EC.00074-13
Shirayama, S., and Numata, O. (2003). Tetrahymena fimbrin localized in the division furrow bundles actin filaments in a calcium-independent manner. J. Biochem. 134, 591–598. doi: 10.1093/jb/mvg183
Sinclair-Davis, A. N., Mcallaster, M. R., and De Graffenried, C. L. (2017). A functional analysis of TOEFAZ1 uncovers protein domains essential for cytokinesis in Trypanosoma brucei. J. Cell Sci. 130, 3918–3932. doi: 10.1242/jcs.207209
Sloves, P. J., Delhaye, S., Mouveaux, T., Werkmeister, E., Slomianny, C., Hovasse, A., et al. (2012). Toxoplasma sortilin-like receptor regulates protein transport and is essential for apical secretory organelle biogenesis and host infection. Cell Host Microbe 11, 515–527. doi: 10.1016/j.chom.2012.03.006
Sonda, S., Stefanic, S., and Hehl, A. B. (2008). A sphingolipid inhibitor induces a cytokinesis arrest and blocks stage differentiation in Giardia lamblia. Antimicrob. Agents Chemother. 52, 563–569. doi: 10.1128/AAC.01105-07
Speer, C. A., and Dubey, J. P. (2001). Ultrastructure of schizonts and merozoites of Sarcocystis neurona. Vet. Parasitol. 95, 263–271. doi: 10.1016/S0304-4017(00)00392-7
Stedman, T. T., Sussmann, A. R., and Joiner, K. A. (2003). Toxoplasma gondii Rab6 mediates a retrograde pathway for sorting of constitutively secreted proteins to the Golgi complex. J. Biol. Chem. 278, 5433–5443. doi: 10.1074/jbc.M209390200
Stefanic, S., Spycher, C., Morf, L., Fabrias, G., Casas, J., Schraner, E., et al. (2010). Glucosylceramide synthesis inhibition affects cell cycle progression, membrane trafficking, and stage differentiation in Giardia lamblia. J. Lipid Res. 51, 2527–2545. doi: 10.1194/jlr.M003392
Stortz, J. F., Del Rosario, M., Singer, M., Wilkes, J. M., Meissner, M., and Das, S. (2019). Formin-2 drives polymerisation of actin filaments enabling segregation of apicoplasts and cytokinesis in Plasmodium falciparum. Elife 8:e49030. doi: 10.7554/eLife.49030
Sturm, A., Amino, R., Van De Sand, C., Regen, T., Retzlaff, S., Rennenberg, A., et al. (2006). Manipulation of host hepatocytes by the malaria parasite for delivery into liver sinusoids. Science 313, 1287–1290. doi: 10.1126/science.1129720
Sugi, T., Kawazu, S., Horimoto, T., and Kato, K. (2015). A single mutation in the gatekeeper residue in TgMAPKL-1 restores the inhibitory effect of a bumped kinase inhibitor on the cell cycle. Int. J. Parasitol. Drugs Drug Resist. 5, 1–8. doi: 10.1016/j.ijpddr.2014.12.001
Sugi, T., Kobayashi, K., Takemae, H., Gong, H., Ishiwa, A., Murakoshi, F., et al. (2013). Identification of mutations in TgMAPK1 of Toxoplasma gondii conferring resistance to 1NM-PP1. Int. J. Parasitol. Drugs Drug Resist. 3, 93–101. doi: 10.1016/j.ijpddr.2013.04.001
Suvorova, E. S., Francia, M., Striepen, B., and White, M. W. (2015). A novel bipartite centrosome coordinates the apicomplexan cell cycle. PLoS Biol. 13:e1002093. doi: 10.1371/journal.pbio.1002093
Taira, R., and Yumura, S. (2017). A novel mode of cytokinesis without cell-substratum adhesion. Sci. Rep. 7:17694. doi: 10.1038/s41598-017-17477-w
Tammana, T. V., Sahasrabuddhe, A. A., Bajpai, V. K., and Gupta, C. M. (2010). ADF/cofilin-driven actin dynamics in early events of Leishmania cell division. J. Cell Sci. 123, 1894–1901. doi: 10.1242/jcs.068494
Tanaka, Y., Jahan, M. G. S., Kondo, T., Nakano, M., and Yumura, S. (2019). Cytokinesis D is mediated by cortical flow of dividing cells instead of chemotaxis. Cells 8:473. doi: 10.3390/cells8050473
Tavares, A., Goncalves, J., Florindo, C., Tavares, A. A., and Soares, H. (2012). Mob1: defining cell polarity for proper cell division. J. Cell Sci. 125, 516–527. doi: 10.1242/jcs.096610
Tewari, R., Dorin, D., Moon, R., Doerig, C., and Billker, O. (2005). An atypical mitogen-activated protein kinase controls cytokinesis and flagellar motility during male gamete formation in a malaria parasite. Mol. Microbiol. 58, 1253–1263. doi: 10.1111/j.1365-2958.2005.04793.x
Tewari, R., Straschil, U., Bateman, A., Bohme, U., Cherevach, I., Gong, P., et al. (2010). The systematic functional analysis of Plasmodium protein kinases identifies essential regulators of mosquito transmission. Cell Host Microbe 8, 377–387. doi: 10.1016/j.chom.2010.09.006
Thazhath, R., Liu, C., and Gaertig, J. (2002). Polyglycylation domain of beta-tubulin maintains axonemal architecture and affects cytokinesis in Tetrahymena. Nat. Cell Biol. 4, 256–259. doi: 10.1038/ncb764
Thieleke-Matos, C., Osorio, D. S., Carvalho, A. X., and Morais-De-Sa, E. (2017). Emerging mechanisms and roles for asymmetric cytokinesis. Int. Rev. Cell Mol. Biol. 332, 297–345. doi: 10.1016/bs.ircmb.2017.01.004
Tran, K. D., Rodriguez-Contreras, D., Vieira, D. P., Yates, P. A., David, L., Beatty, W., et al. (2013). KHARON1 mediates flagellar targeting of a glucose transporter in Leishmania mexicana and is critical for viability of infectious intracellular amastigotes. J. Biol. Chem. 288, 22721–22733. doi: 10.1074/jbc.M113.483461
Tran, K. D., Vieira, D. P., Sanchez, M. A., Valli, J., Gluenz, E., and Landfear, S. M. (2015). Kharon1 null mutants of Leishmania mexicana are avirulent in mice and exhibit a cytokinesis defect within macrophages. PLoS ONE 10:e0134432. doi: 10.1371/journal.pone.0134432
Trindade, S., Rijo-Ferreira, F., Carvalho, T., Pinto-Neves, D., Guegan, F., Aresta-Branco, F., et al. (2016). Trypanosoma brucei parasites occupy and functionally adapt to the adipose tissue in mice. Cell Host Microbe 19, 837–848. doi: 10.1016/j.chom.2016.05.002
Tu, X., Kumar, P., Li, Z., and Wang, C. C. (2006). An aurora kinase homologue is involved in regulating both mitosis and cytokinesis in Trypanosoma brucei. J. Biol. Chem 281, 9677–9687. doi: 10.1074/jbc.M511504200
Tucker, J. B. (1971). Microtubules and a contractile ring of microfilaments associated with a cleavage furrow. J. Cell Sci. 8, 557–571.
Urbaniak, M. D., Mathieson, T., Bantscheff, M., Eberhard, D., Grimaldi, R., Miranda-Saavedra, D., et al. (2012). Chemical proteomic analysis reveals the drugability of the kinome of Trypanosoma brucei. ACS Chem. Biol. 7, 1858–1865. doi: 10.1021/cb300326z
Vaishnava, S., Morrison, D. P., Gaji, R. Y., Murray, J. M., Entzeroth, R., Howe, D. K., et al. (2005). Plastid segregation and cell division in the apicomplexan parasite Sarcocystis neurona. J. Cell Sci. 118, 3397–3407. doi: 10.1242/jcs.02458
Van Den Abbeele, J., Claes, Y., Van Bockstaele, D., Le Ray, D., and Coosemans, M. (1999). Trypanosoma brucei spp. development in the tsetse fly: characterization of the post-mesocyclic stages in the foregut and proboscis. Parasitology 118, 469–478. doi: 10.1017/S0031182099004217
Verma, D., Murmu, A., Gourinath, S., Bhattacharya, A., and Chary, K. V. R. (2017). Structure of Ca2+-binding protein-6 from Entamoeba histolytica and its involvement in trophozoite proliferation regulation. PLoS Pathog. 13, e1006332. doi: 10.1371/journal.ppat.1006332
Vidadala, R. S., Ojo, K. K., Johnson, S. M., Zhang, Z., Leonard, S. E., Mitra, A., et al. (2014). Development of potent and selective Plasmodium falciparum calcium-dependent protein kinase 4 (PfCDPK4) inhibitors that block the transmission of malaria to mosquitoes. Eur. J. Med. Chem. 74, 562–573. doi: 10.1016/j.ejmech.2013.12.048
von Schubert, C., Xue, G., Schmuckli-Maurer, J., Woods, K. L., Nigg, E. A., and Dobbelaere, D. A. (2010). The transforming parasite Theileria co-opts host cell mitotic and central spindles to persist in continuously dividing cells. PLoS Biol. 8:e1000499. doi: 10.1371/journal.pbio.1000499
Wall, R. J., Ferguson, D. J. P., Freville, A., Franke-Fayard, B., Brady, D., Zeeshan, M., et al. (2018). Plasmodium APC3 mediates chromosome condensation and cytokinesis during atypical mitosis in male gametogenesis. Sci. Rep. 8:5610. doi: 10.1038/s41598-018-23871-9
Wall, R. J., Zeeshan, M., Katris, N. J., Limenitakis, R., Rea, E., Stock, J., et al. (2019). Systematic analysis of Plasmodium myosins reveals differential expression, localisation, and function in invasive and proliferative parasite stages. Cell Microbiol. 21:e13082. doi: 10.1111/cmi.13082
Walsh, C. J. (2012). The structure of the mitotic spindle and nucleolus during mitosis in the amebo-flagellate Naegleria. PLoS ONE 7:e34763. doi: 10.1371/journal.pone.0034763
Watanabe, S., De Zan, T., Ishizaki, T., and Narumiya, S. (2013). Citron kinase mediates transition from constriction to abscission through its coiled-coil domain. J. Cell Sci. 126, 1773–1784. doi: 10.1242/jcs.116608
Wheeler, R. J., Gluenz, E., and Gull, K. (2011). The cell cycle of Leishmania: morphogenetic events and their implications for parasite biology. Mol. Microbiol. 79, 647–662. doi: 10.1111/j.1365-2958.2010.07479.x
Wheeler, R. J., Scheumann, N., Wickstead, B., Gull, K., and Vaughan, S. (2013). Cytokinesis in Trypanosoma brucei differs between bloodstream and tsetse trypomastigote forms: implications for microtubule-based morphogenesis and mutant analysis. Mol. Microbiol. 90, 1339–1355. doi: 10.1111/mmi.12436
Wilke, G., Funkhouser-Jones, L. J., Wang, Y., Ravindran, S., Wang, Q., Beatty, W. L., et al. (2019). A stem-cell-derived platform enables complete Cryptosporidium development in vitro and genetic tractability. Cell Host Microbe 26, 123–134.e128. doi: 10.1016/j.chom.2019.05.007
Wilkes, D. E., and Otto, J. J. (2003). Profilin functions in cytokinesis, nuclear positioning, and stomatogenesis in Tetrahymena thermophila. J. Eukaryot. Microbiol. 50, 252–262. doi: 10.1111/j.1550-7408.2003.tb00130.x
Williams, N. E., Tsao, C. C., Bowen, J., Hehman, G. L., Williams, R. J., and Frankel, J. (2006). The actin gene ACT1 is required for phagocytosis, motility, and cell separation of Tetrahymena thermophila. Eukaryotic Cell 5, 555–567. doi: 10.1128/EC.5.3.555-567.2006
Wloga, D., Strzyzewska-Jowko, I., Gaertig, J., and Jerka-Dziadosz, M. (2008). Septins stabilize mitochondria in Tetrahymena thermophila. Eukaryotic Cell 7, 1373–1386. doi: 10.1128/EC.00085-08
Woodland, A., Thompson, S., Cleghorn, L. A., Norcross, N., De Rycker, M., Grimaldi, R., et al. (2015). Discovery of inhibitors of Trypanosoma brucei by phenotypic screening of a focused protein kinase library. ChemMedChem 10, 1809–1820. doi: 10.1002/cmdc.201500300
Woods, K. L., Theiler, R., Muhlemann, M., Segiser, A., Huber, S., Ansari, H. R., et al. (2013). Recruitment of EB1, a master regulator of microtubule dynamics, to the surface of the Theileria annulata schizont. PLoS Pathog. 9:e1003346. doi: 10.1371/journal.ppat.1003346
Worden, A. Z., Follows, M. J., Giovannoni, S. J., Wilken, S., Zimmerman, A. E., and Keeling, P. J. (2015). Environmental science. Rethinking the marine carbon cycle: factoring in the multifarious lifestyles of microbes. Science 347:1257594. doi: 10.1126/science.1257594
Wyllie, S., Thomas, M., Patterson, S., Crouch, S., De Rycker, M., Lowe, R., et al. (2018). Cyclin-dependent kinase 12 is a drug target for visceral Leishmaniasis. Nature 560, 192–197. doi: 10.1038/s41586-018-0356-z
Xia, L., Hai, B., Gao, Y., Burnette, D., Thazhath, R., Duan, J., et al. (2000). Polyglycylation of tubulin is essential and affects cell motility and division in Tetrahymena thermophila. J. Cell Biol. 149, 1097–1106. doi: 10.1083/jcb.149.5.1097
Xue, Z., and Sokac, A. M. (2016). -Back-to-back mechanisms drive actomyosin ring closure during Drosophila embryo cleavage. J. Cell Biol. 215, 335–344. doi: 10.1083/jcb.201608025
Yano, Y., Saito-Ito, A., Anchalee, D., and Takada, N. (2005). Japanese Babesia microti cytologically detected in salivary glands of naturally infected tick Ixodes ovatus. Microbiol. Immunol. 49, 891–897. doi: 10.1111/j.1348-0421.2005.tb03680.x
Yasuda, T., Numata, O., Ohnishi, K., and Watanabe, Y. (1980). A contractile ring and cortical changes found in the dividing Tetrahymena pyriformis. Exp. Cell Res. 128, 407–417. doi: 10.1016/0014-4827(80)90076-2
Zhang, X., An, T., Pham, K. T. M., Lun, Z. R., and Li, Z. (2019a). Functional analyses of cytokinesis regulators in bloodstream stage Trypanosoma brucei parasites identify functions and regulations specific to the life cycle stage. mSphere 4, e00199–e00119. doi: 10.1128/mSphere.00199-19
Zhang, X., Hu, H., Lun, Z. R., and Li, Z. (2019b). Functional analyses of an axonemal inner-arm dynein complex in the bloodstream form of Trypanosoma brucei uncover its essential role in cytokinesis initiation. Mol. Microbiol. doi: 10.1111/mmi.14385. [Epub ahead of print].
Zhang, X., Li, S. J., Li, Z., He, C. Y., Hide, G., Lai, D. H., et al. (2019c). Cell cycle and cleavage events during in vitro cultivation of bloodstream forms of Trypanosoma lewisi, a zoonotic pathogen. Cell Cycle 18, 552–567. doi: 10.1080/15384101.2019.1577651
Zhou, Q., An, T., Pham, K. T. M., Hu, H., and Li, Z. (2018a). The CIF1 protein is a master orchestrator of trypanosome cytokinesis that recruits several cytokinesis regulators to the cytokinesis initiation site. J. Biol. Chem. 293, 16177–16192. doi: 10.1074/jbc.RA118.004888
Zhou, Q., Dong, G., and Li, Z. (2018b). Flagellum inheritance in Trypanosoma brucei requires a kinetoplastid-specific protein phosphatase. J. Biol. Chem. 293, 8508–8520. doi: 10.1074/jbc.RA118.002106
Zhou, Q., Gheiratmand, L., Chen, Y., Lim, T. K., Zhang, J., Li, S., et al. (2010). A comparative proteomic analysis reveals a new bi-lobe protein required for bi-lobe duplication and cell division in Trypanosoma brucei. PLoS ONE 5:e9660. doi: 10.1371/journal.pone.0009660
Zhou, Q., Gu, J., Lun, Z. R., Ayala, F. J., and Li, Z. (2016a). Two distinct cytokinesis pathways drive trypanosome cell division initiation from opposite cell ends. Proc. Natl. Acad. Sci. U.S.A. 113, 3287–3292. doi: 10.1073/pnas.1601596113
Zhou, Q., Hu, H., and Li, Z. (2016b). An EF-hand-containing protein in Trypanosoma brucei regulates cytokinesis initiation by maintaining the stability of the cytokinesis initiation factor CIF1. J. Biol. Chem. 291, 14395–14409. doi: 10.1074/jbc.M116.726133
Keywords: cell division, cytokinesis, protozoan parasite, actomyosin ring-independent cell division, budding, furrow ingression, abscission, cytofission
Citation: Hammarton TC (2019) Who Needs a Contractile Actomyosin Ring? The Plethora of Alternative Ways to Divide a Protozoan Parasite. Front. Cell. Infect. Microbiol. 9:397. doi: 10.3389/fcimb.2019.00397
Received: 03 September 2019; Accepted: 06 November 2019;
Published: 21 November 2019.
Edited by:
Brice Rotureau, Institut Pasteur, FranceReviewed by:
Anna Frappaolo, Institute of Molecular Biology and Pathology (CNR), ItalyKaren Oegema, University of California, San Diego, United States
Sabrina Absalon, Boston Children's Hospital and Harvard Medical School, United States
Copyright © 2019 Hammarton. This is an open-access article distributed under the terms of the Creative Commons Attribution License (CC BY). The use, distribution or reproduction in other forums is permitted, provided the original author(s) and the copyright owner(s) are credited and that the original publication in this journal is cited, in accordance with accepted academic practice. No use, distribution or reproduction is permitted which does not comply with these terms.
*Correspondence: Tansy C. Hammarton, VGFuc3kuSGFtbWFydG9uQGdsYXNnb3cuYWMudWs=