- 1School of Life Sciences, Northwestern Polytechnical University, Xi'an, China
- 2Department of General Surgery, First Affiliated Hospital of Xi'an Jiaotong University, Xi'an, China
- 3Faculty of Applied Science, University of British Columbia, Kelowna, BC, Canada
- 4Institute of Medical Research, Northwestern Polytechnical University, Xi'an, China
- 5Princess Margaret Cancer Center, University of Health Network, Toronto, ON, Canada
Oral supplemented nutraceuticals derived from food sources are surmised to improve the human health through interaction with the gastrointestinal bacteria. However, the lack of fundamental quality control and authoritative consensus (e.g., formulation, route of administration, dose, and dosage regimen) of these non-medical yet bioactive compounds are one of the main practical issues resulting in inconsistent individual responsiveness and confounded clinical outcomes of consuming nutraceuticals. Herein, we studied the dose effects of widely used food supplement, microalgae spirulina (Arthrospira platensis), on the colonic microbiota and physiological responses in healthy male Balb/c mice. Based on the analysis of 16s rDNA sequencing, compared to the saline-treated group, oral administration of spirulina once daily for 24 consecutive days altered the diversity, structure, and composition of colonic microbial community at the genus level. More importantly, the abundance of microbial taxa was markedly differentiated at the low (1.5 g/kg) and high (3.0 g/kg) dose of spirulina, among which the relative abundance of Clostridium XIVa, Desulfovibrio, Eubacterium, Barnesiella, Bacteroides, and Flavonifractor were modulated at various degrees. Evaluation of serum biomarkers in mice at the end of spirulina intervention showed reduced the oxidative stress and the blood lipid levels and increased the level of appetite controlling hormone leptin in a dose-response manner, which exhibited the significant correlation with differentially abundant microbiota taxa in the cecum. These findings provide direct evidences of dose-related modulation of gut microbiota and physiological states by spirulina, engendering its future mechanistic investigation of spirulina as potential sources of prebiotics for beneficial health effects via the interaction with gut microbiota.
Introduction
The gut microbiota is a complex and functional ecological community and plays an important role in influencing the physiological states, disease susceptibilities, and even therapeutic efficacies (Flint et al., 2012; Lozupone et al., 2012; Geller et al., 2017). The homeostasis of the gut microbial community in terms of its distribution, diversity, species composition and metabolic output contributes to the net benefits of host health (Flint et al., 2012; Sommer and Backhed, 2013). Yet, the gut microbiota is frequently shaped under both host and environmental selective pressures, such as host genotype, intestinal barrier (e.g., mucus layers, IgA, and epithelia-associated immune cells), colonic environments (e.g., intestinal pH and oxygen gradients and bile acids re-absorption), life styles and living conditions (e.g., smoking, geographical location, and surgery) of which diet exerts a large effect on the microbial colonization and its relative abundance (Spor et al., 2011; Wu et al., 2011; Thursby and Juge, 2017). Dysbiosis as a result of the disruption to the overall state of gut microbiota (e.g., antibiotics utilization) has been associated with the pathogenesis of many chronic diseases, such as cancer, inflammatory bowel disease (IBD), cardiovascular diseases, obesity, and diabetes (Guinane and Cotter, 2013; de Clercq et al., 2016; Tang et al., 2017).
To sustain or restore the intestinal bacterial homeostasis in healthy individuals or disease states, several approaches have been implemented, including the oral supplementation of probiotics, prebiotics and synbiotics (Verdu, 2009; Quigley, 2018), fecal microbiota transplantation (Zipursky et al., 2012; Li et al., 2016; Bilinski et al., 2017), bacterial consortium transplantation (Li et al., 2015) as well as bacteriocins and bacteriophage targeted antimicrobial therapies (Mills et al., 2017). Especially, orally supplementing non-medical nutraceuticals derived from the food sources have been widely used for disease prevention and amelioration of disease symptoms due to its potential capacity of promoting the growth of commensal gut microorganisms (Cencic and Chingwaru, 2010; Laparra and Sanz, 2010; Wang et al., 2019). Nevertheless, the meta-analysis of humans and animals studies reveals inconsistent individual responsiveness and clinical outcomes of consuming food supplements or dietary compounds (Gibson et al., 2017; Quigley, 2018). One of the main practical issues in the evaluation of nutraceuticals is lack of fundamental quality control and authoritative consensus (e.g., formulation, route of administration, dose, and dosage regimen) of these non-medical yet bioactive compounds. In addition, there is a large knowledge gap of cause-and-effect relationships between marketed nutraceutical products, the change of microbial population and the physiological benefits.
In the current study, we investigated spirulina, one of the most commonly consumed microalgae as food supplements worldwide (de Jesus Raposo et al., 2016), because of its potential benefits of nutritional values and therapeutic properties in human health (Khan et al., 2005; Nicoletti, 2016). Oral supplementation of spirulina in human studies have shown to potentiate the innate immune system, ameliorate hyperlipidemia, reduce the body mass, improve antioxidant status, and enhance anti-inflammatory and antihypertensive effects (Hirahashi et al., 2002; Khan et al., 2005; Lu et al., 2006; Torres-Duran et al., 2007; Mazokopakis et al., 2014; Ngo-Matip et al., 2014; Yogianti et al., 2014; Szulinska et al., 2017). Although the underlying mechanism of claimed biological functions of spirulina has not yet been fully understood yet, recent studies in healthy and disease animal models have shown that spirulina can modulate the composition of gut microbiota (e.g., Lactaobacilli and Roseburia) that may link to improved health status (Rasmussen et al., 2009; Yusuf et al., 2016; Neyrinck et al., 2017). However, despite of its widespread global use by the general populations, the quantitative characterization of dose-related effects of spirulina on the safety and effectiveness remains unknown.
Thus, to investigate whether the doses of spirulina alter on the gut microorganisms, which in turn affect physiological responses, orally administered spirulina at low and high doses in healthy mice were performed for a short period of intervention (Figure 1). The α- and β-diversity of colonic microbiota from fecal and cecal samples at designed times were evaluated by small subunit ribosomal DNA (16s rDNA) sequencing. Differentially abundant bacteria organisms were identified at the genus-level among different treatment groups using a quantitative computational method. Various health related indicators, including the body weight and biological markers, such as malondialdehyde (MDA), superoxide dismutase (SOD), total cholesterol (TC), total triglycerides (TG), and leptin, were also measured in the serum.
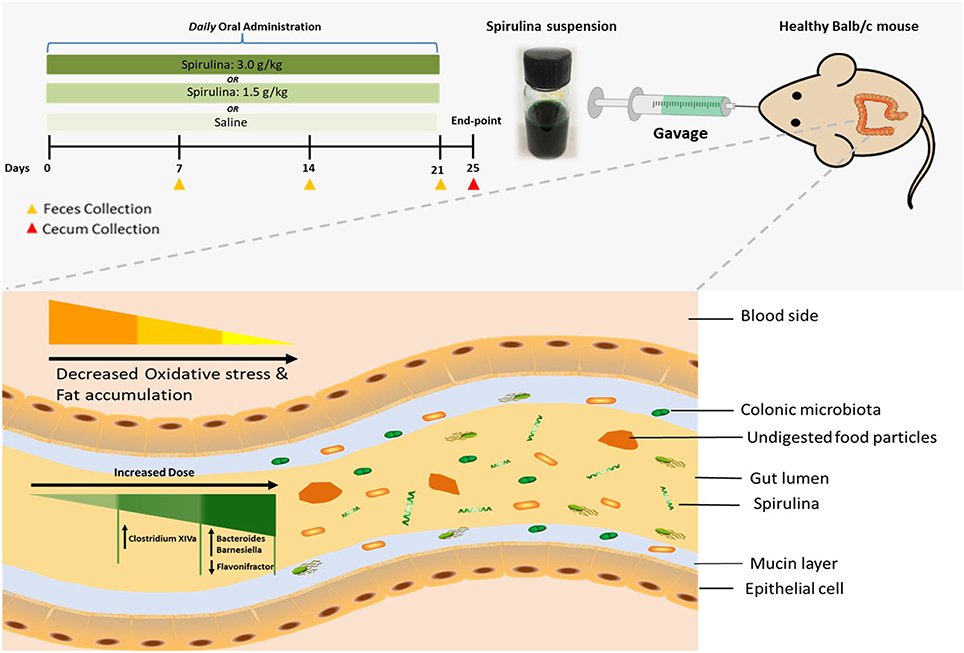
Figure 1. Schematic illustration of dose-dependent modulation of colonic microbiota and physiological responses in healthy mice via oral administration of spirulina suspension. Top panel: aqueous spirulina is orally administered to healthy male Balb/c mice at the low (1.5 g/kg) or high (3.0 g/kg) doses daily for consecutive 24 days. The change of colonic microbiota is identified from their fecal and cecal samples collected on day of 7th, 14th, 21st, and 25th post-treatment using high-throughput 16S rDNA sequencing. The status of oxidative stress and lipid profile are determined using various metabolic blood biomarkers (MDA, SOD, TG, TC, and leptin). Bottom panel: undigested components of spirulina (green color and spiral shape) are transited into the lumen of the distal large intestine where most of the gut microbial species colonize. Orally administered spirulina suspension alters the specific genus of colonic microbiota in a dose-dependent manner (triangle with gradient green color) in mice. At the low dose, strict anaerobes Clostridium Cluster XIVa is increased, whereas the high dose increases the abundance of Bacteroides and Barnesiella and decreases Flavonifractor. Oral intake of spirulina also effectively reduces the oxidative stress and fat accumulation (triangle with gradient yellow color) that is believed to relate to many chronic diseases, such as cancer, inflammatory bowel disease and cardiovascular disease.
Materials and Methods
Animal Maintenance
All animal experiments were approved by the Institutional Animal Care and Use Committee of Northwestern Polytechnical University (Xi'an, China) and performed in accordance with the Institutional Ethical Guideline of Experimental Animals. Healthy 6 weeks old male BALB/c mice, weighting 22.85 ± 1.32 g, were obtained from the Experimental Animal Center of the Fourth Military Medical University (Xi'an, China) and housed individually in a polypropylene cage under standard laboratory conditions of 22 ± 1°C and a 12 h light-dark cycle (lights on from 06:00 a.m. to 18:00 p.m.) in the pathogen-free animal facility. All mice were ad libitum access to sterile water and commercial fodder free of probiotics and antibiotics (Keaoxieli, Beijing, China).
Treatment of Animals With Spirulina
The fresh aqueous spirulina suspension was prepared daily at room temperature by adding 1.05 g or 2.10 g of dark blue-green spray-dried Arthrospira platensis (A. platensis) powder (Templer, Zhongshan, China) into 15 mL 0.9% sterile physiological saline (SCR, Shanghai, China). After 1 week of acclimatization, BALB/c mice were randomly assigned into one of the following treatment groups: (1) saline alone; (2) low dosage 1.5 g/kg of spirulina; and (3) high dosage 3.0 g/kg of spirulina. The saline alone or spirulina suspension was fed into the mice stomach with an oral gavage needle (12 Ga ×55 mm, 1.2 mm tip) (Hengao, Beijing, China) once daily for 24 consecutive days. The overall health of all treated mice was monitored closely, and their body weights were recorded every 3 or 4 days.
Collection of Serum, Feces, and Cecum Samples
Before and during the treatment, every animal was raised separately in a metabolic cage (Suhang, Suzhou, China) and their fresh stools were collected at the following designated days: 0, 7, 14, and 21 days and the cecal contents were obtained at the end-point of treatments on the 25th day. The animals were deprived of food for 12 h and then anesthetized with 1.9% diethyl ether. The whole blood samples were withdrawn by inferior vena cava puncture and collected in polypropylene tubes (Shenggong, Shanghai, China). The serum was obtained by allowing the whole blood to clot and then centrifuging samples at 1,000 × g for 10 min in a refrigerated centrifuge (Thermo Scientific, USA). The resulting supernatant was immediately transferred into a clean polypropylene tube (Sangon Biotech®, Shanghai, China) and stored at −20°C for biomarker analysis. The cecum was excised, and its contents were collected in freezing tubes (IMEC Sunshinebio, Hangzhou, China). All fecal and cecum samples were snap frozen with liquid nitrogen and stored at −80°C for microbiota analysis.
DNA Extraction, PCR Amplification, and Pyrosequencing
Total bacterial genomic DNA from the frozen fecal pellets and cecal specimens were extracted using QIAamp Fast DNA Stool MiniKit (QIAGEN, Germany). The microbial 16S rDNA was amplified with specific primers (341F: ACT CCT ACG GGRSGC AGC AG, 806R: GGA CTA CVV GGG TAT CTA ATC) targeting the V3-4 region by KAPA HiFi Hotstart Ready Mix PCR kit (Kapabiosystems, USA), purified with the AxyPrep DNA Gel Extraction Kit (Axygen, USA) and quantified by NanoDrop 2000 (Thermo Scientific, USA) at wavelengths of 260 and 280 nm. The library was finally fragment-selected and purified by 2% agarose gel electrophoresis. The purified fragments were end-repaired and ligated to the Illumina paired-end sequencing adapters. Amplicon libraries were sequenced on Illumina Miseq PE250 platform (Illumina, USA) for paired-end reads of 250 bp according to the Illumina instructions (Realbio Genomics Institute, Shanghai, China). The original data of high-throughput sequencing was taken to qualify preliminary screening using QIIME software (http://qiime.org/). The assembled long tags using the paired-end reads were quality controlled by removing tags with a length of <220 nt, an average quality score of <20 (low-quality bases), and tags containing >3 ambiguous bases by PANDAse (Li et al., 2016). A total of 3,132,725 clean reads were obtained after quality control. The deep sequencing data are available from the NCBI Sequence Read Archive under accession number PRJNA511783.
Bioinformatics
A total of 51 fecal samples were collected on day of 7th, 14th, and 21st (number of samples and their ID: 5 S-7F, 6 L-7F, 6 H-7F; 5 S-14F, 6 L-14F, 6 H-14F; 5 S-21F, 6 L-21F, 6 H-21F) and 17 cecum samples were collected at the endpoint of the 25th day (number of samples and their ID: 5 S-25C, 6 L-25C, 6 H-25C). The singletons and chimeras from unique sequences were removed by UPARSE algorithm method (Edgar, 2013). After discarding the sequencing and amplification artifacts, the high-quality tags were clustered into operational taxonomic units (OTUs) with a similarity threshold of 97% using Usearch. The OTUs were further subjected to the taxonomy-based analysis by Ribosomal Database Project (RDP) algorithm. A total of 2,853,914 mapped reads were assigned to 416 OTUs, resulting in the classification of 86 taxa at the genus level. Each sample has 244 OTUs and 41,969 reads on average (Table S1 and Figure S1). α-diversity (Chao1, observed species, Shannon and Simpson diversity indexes) and β-diversity [weighted UniFrac, principal coordinate analysis (PCoA)] were analyzed using QIIME1 software. Linear discriminant analysis (LDA) effect size (LEfSe) method was performed with the LEfSe tool (http://huttenhower.sph.harvard.edu/galaxy).
Evaluation of Blood Biomarkers
Serum levels of MDA, SOD, TC, and TG were quantified by biochemical assays of thiobarbituric acid (TBA), water-soluble tetrazolium-1 (WST-1), cholesterol oxidase p-aminophenol (COD-PAP), and glycerol-phosphate oxidase (GPO-PAP), respectively (Jiancheng Bioengineering Institute, Nanjing, China). Serum leptin was measured with Mouse Leptin ELISA Assay Kit (Jiancheng Bioengineering Institute, Nanjing, China). The procedures of each assay are performed according to the manufacturers' instructions (Jiancheng Bioengineering Institute, Nanjing, China). In brief, the oxidative stress was assessed in 20 μL serum by quantifying 1% TBA reactivity with MDA and WST-1 formazan for SOD enzyme activity. The resulting chromogen absorbance was determined at 532 nm for MDA-TBA adducts and at 435 nm for WST-1 formazan, respectively. The lipid contents (TC and TG) were measured directly in 0.25 mL serum by quantifying the formation of red quinone compound from COD-PAP and GPO-PAP at 510 nm. The quantitative measurement of serum leptin was determined by adding 50μL serum into 96-microplate wells containing the antibody complex of pre-coated anti-tag antibody, biotinylated anti-mouse leptin antibody, and the color density of horseradish peroxidase (HRP)-conjugated streptavidin was measured at 450 nm.
Statistical Analyses
Differential analysis of various α-diversity indexes among samples within the same designated day were analyzed by the Wilcoxon signed-rank test (GraphPad Prism®, USA). Differences in the colonic bacterial structure, diversity and relative abundance among individual samples and treatment groups were analyzed by the multiresponse permutation procedure (MRPP) and Kruskal–Wallis tests, respectively, with p < 0.05 considered statistically significant. LEfSe method was used to identify differential bacterial taxa representing between groups at the genus level. The body weight and various blood biomarker levels were presented as mean ± standard deviation (SD) and were compared by One-way factor analysis of variance (ANOVA) following the Tukey post-hoc method with p < 0.05 considered statistically significant (GraphPad Prism®, USA). Spearman correlations between the differentially abundant microbiota and biological markers were computed in R, with the absolute value r > 0.7 and p < 0.033 considered to be statistically correlated with each other.
Results
Spirulina Affected the Diversity of Colonic Microbiota
To assess the diversity and structural differences in the colonic microbiota of healthy mice treated with saline or spirulina at the low and high doses over 25 days of daily intervention, differential significance in α-and β-diversity of collected fecal and cecal samples were analyzed by the Wilcoxon signed-rank test and weighted UniFrac distance matrix based MRPP, respectively (Figures 2, 3). We firstly compared each of the α-diversity indexes (Chao1, Observed species, Shannon and Simpson) between treated samples (saline, low, and high doses of spirulina) on the same designated days (Figure 2). No significant difference in Chao1 (estimated the number of OTU) and observed species (observed the number of OTU) indexes were observed between samples from the treatments of saline, low, and high spirulina at all designated time points (Figures 2A,B), indicating the microbial richness (i.e., the total number of species) within each treatment were not changed. The Shannon and Simpson indexes, which took into account both richness and evenness (i.e., microbial equality) of species within each sample, showed different α-diversity on the 7th, 14th, and 21st days upon various spirulina doses (0 mg/kg, 1.5 mg/kg, 3 mg/kg) (Figures 2C,D). Especially, the Simpson index that weighted more on the dominant species revealed that intake of the low or high doses of spirulina exhibited different alteration patterns of the α-diversity (Figure 2D). Interestingly, the low dose of spirulina reduced the α-diversity on the 14th day and increased back on the 21st day, while the high-dose group exhibited an increase in the diversity over the time frame of 25 days (Figure S2). All of α-diversity indexes were not significantly different from each other in cecal samples on the 25th day (Figure 2). The structural difference in the colonic microbiota community was also observed by the gradual shifts of distance matrix among treatment groups over time using PCoA (Figure 3). At the early 7 and 14th days of spirulina intervention, no significant differences in colonic microbiota structure were observed with p = 0.686 and p = 0.319 (Figures 3A,B). At the later 21st and 25th days, the microbiota structure in fecal and cecal samples was significantly altered by spirulina treatments at different doses (both low and high doses) compared to the saline group with p = 0.016 and p = 0.014, respectively (Figures 3C,D), as demonstrated by the distinguished distances between treatment groups.
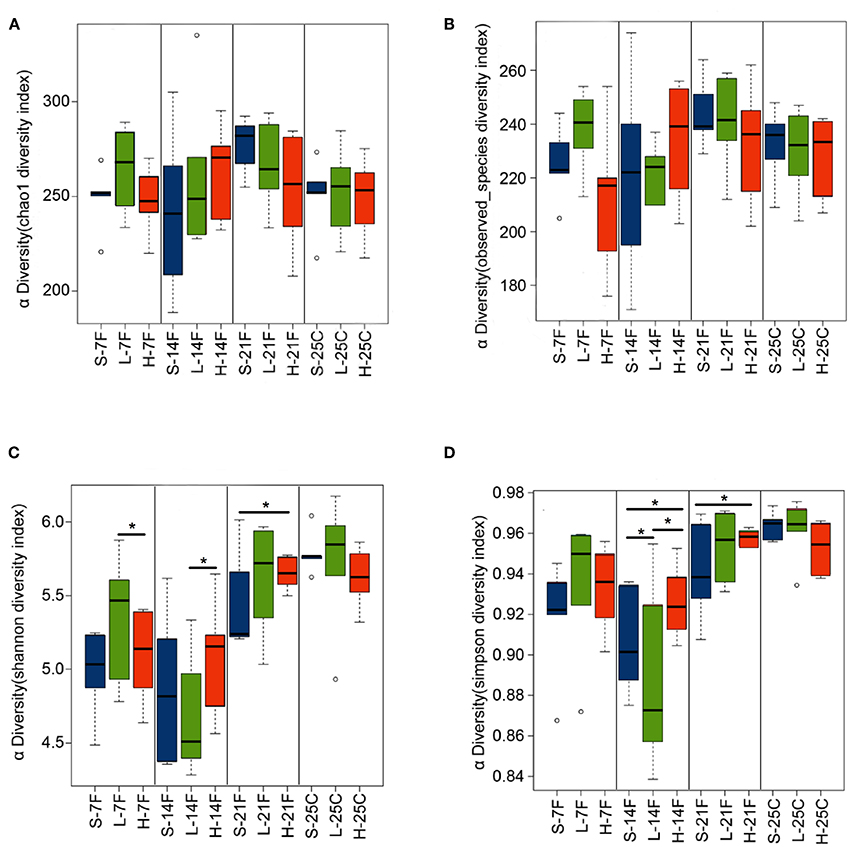
Figure 2. Barplots of α-diversity indexes in the treatment groups of saline, low, and high doses of spirulina at designated time points (day 7th, 14th, 21st, and 25th). (A) Chao1 index; (B) Observed species index; (C) Shannon index; (D) Simpson index. The difference of each α-diversity index was compared between individual treated samples within the same designated day by the Wilcoxon test with *p < 0.05 considered as statistically significant. In the x-axis label, S, L, and H represent the treatment of saline, low, and high doses of spirulina, respectively, and F and C represent feces and cecum, respectively.
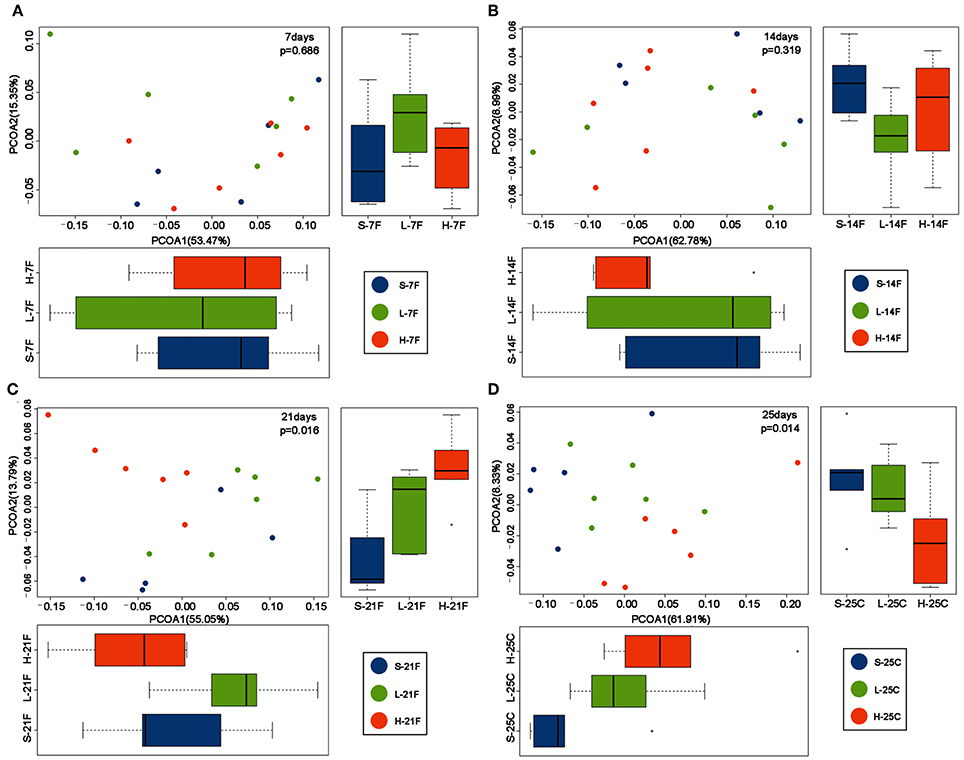
Figure 3. PCoA plots of β-diversity of feces collected on day (A) 7th day; (B) 14th day; (C) 21st; and cecal contents collected on day (D) 25th. Results revealed samples from spirulina treatment clustered separately from the saline group on the 21st and 25th days. The colonic microbial community structures were compared between treatments using the PCoA based on the weighted UniFrac distance matrixes. MRPP analysis was used to determine the statistical significance with p < 0.05. Each time point contains 5 or 6 samples. S (purple), L (green), and H (orange) represent saline, low, and high doses of spirulina, respectively, and F and C represent feces and cecum, respectively. Colored dots indicate the sample from each animal.
Spirulina Modulated the Taxonomic Abundance of Colonic Microbiota
To identify specific bacterial genus in spirulina treated groups at the low and high dose, the abundance of colonic bacteria from fecal pellets on the 21st day and cecal contents on the 25th day in mice were analyzed by the LEfSe method and Kruskal-Wallis test, and the significantly differentiated phylotypes by abundance was visualized using the histogram of LDA scores, heatmap and boxplots (Figures 4, 5). At the genus level of fecal bacteria, Clostridium XIVa, Barnesiella, Desulfovribrio, and Eubacterium were significantly differentiated by abundance among treatment groups (Figure 4A). The Clostridium XIVa genus in particularly was detected by LEfSe with a high LDA score (nearly four orders of magnitude), reflecting marked abundance in the low dose spirulina treatment group, whereas the Barnesiella and Eubacterium genera were found in abundance in the high-dose treated group (Figure 4A). Compared to saline-treated mice, the obvious changes in the colonic microbial composition and abundance was reduced Eubacterium and increased Barnesiella in the low and high doses treated spirulina groups, respectively (Figures 4B,C). At the genus level of cecal bacteria, only feeding high dose spirulina to mice significantly altered the gut microbial abundance and composition with increased the levels of Barnesiella and Bacteroides and decreased the level of Flavonifractor (Figure 5). These three bacteria taxa were consistently detected when comparing the saline group with the combined sample sets of the low- and high- doses spirulina (Figure S3).
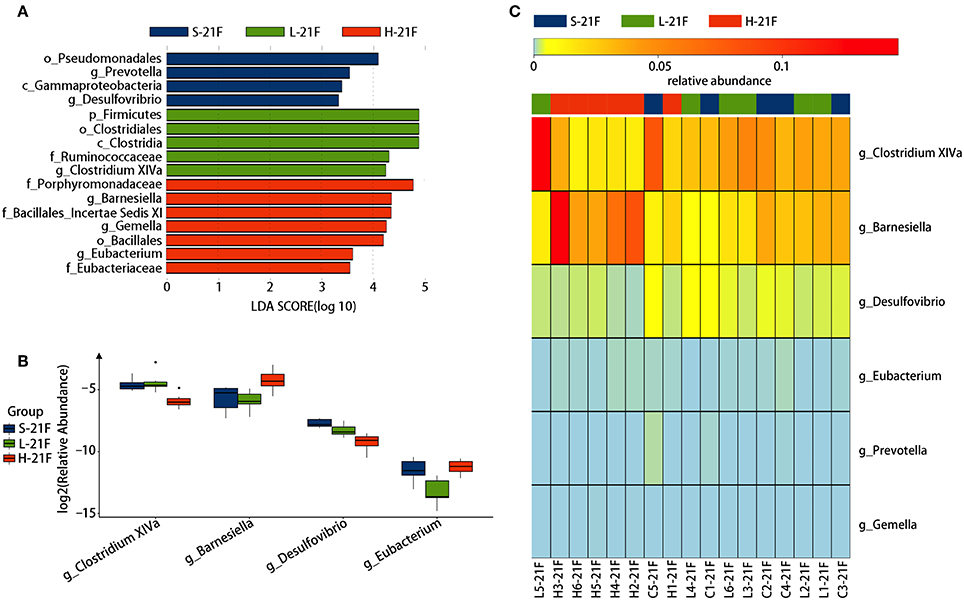
Figure 4. Significant influence of identified fecal microbiota at the genus level on the 21st day post-treatment of spirulina at low and high doses. (A) Histogram of the LDA scores of differential phylotypes abundant among treatment groups according to LEfse analysis; (B) Heatmap and (C) Barplots of the relative abundance of significantly differentiated bacteria among all three treatment groups. S-21F, L-21F, and H-21F represent fecal samples from saline, low, and high dose groups on day 21st, respectively. Differential significant analysis of abundance and composition of gut microbiome was analyzed at the OTUs level by the Kruskal-Wallis test.
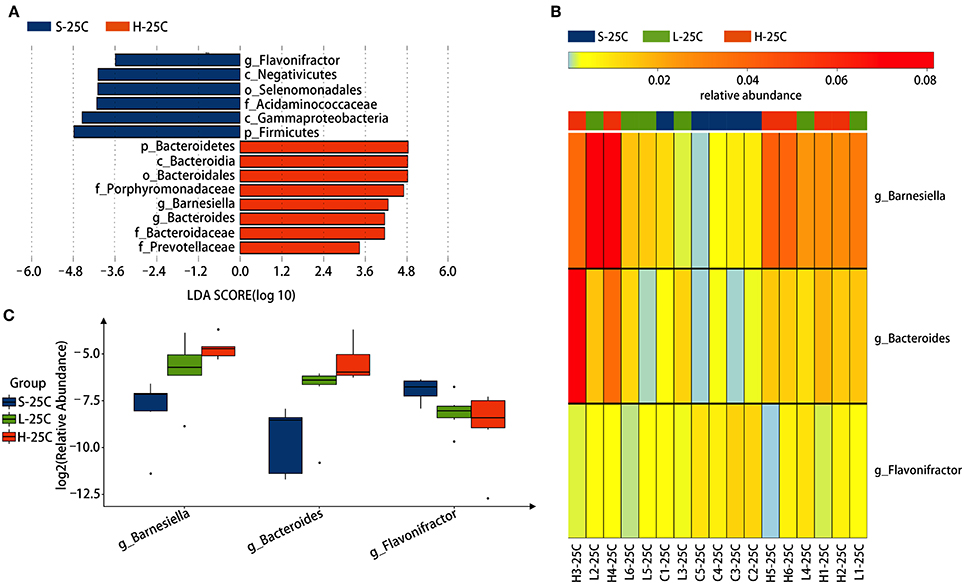
Figure 5. Significant influence of identified cecal microbiota at the genus level on the 25th day post-treatment of spirulina at low and high doses. (A) Histogram of the LDA scores of differential phylotypes abundant among treatment groups according to LEfse analysis; (B) Heatmap and (C) Barplots of the relative abundance of significantly differentiated bacteria among all three treatment groups. S-21C, L-21C, and H-21C represent cecal samples from saline, low, and high dose groups on day 25th, respectively. Differential significant analysis of abundance and composition of gut microbiome was analyzed at the OTUs level by the Kruskal-Wallis test.
Intake of Spirulina Reduced the Oxidative Stress and Lipid Accumulation
The change in body weight of mice treated with the saline, low, and high doses of spirulina at 1.5 g/kg and 3 g/kg, respectively, were determined by comparing them to the initial body weight of the mice prior to the treatment on the day 0. Intake of saline and spirulina showed no significant change in the body weight in healthy mice with p > 0.05. Compared to the saline group, the mice treated with spirulina at both low and high doses showed a similar trend of the body weight change (Figure 6A). The effects of orally administered spirulina on the oxidative stress and fat accumulation were biochemically evaluated by the serum biomarkers. Compared to the saline treatment, mice treated with spirulina showed a significant decline in the level of lipid peroxidation quantified by its end-product MDA formation and the elevation of serum antioxidant enzyme SOD activity in the dose-dependent manner (Figures 6B,C). Moreover, the serum lipid profile, including the level of TC and TG, were significantly attenuated in spirulina treatment groups compared to the one in saline with a nearly 2.1-fold reduction in TG level observed in the high dose (3.0 g/kg) treatment of spirulina (Figures 6D,E). Interestingly, the serum leptin, an appetite-regulating hormone secreted by adipocytes for hunger inhibition, was doubled in concentration along with the dose increment of spirulina from 1.5 to 3 g/kg (Figure 6F).
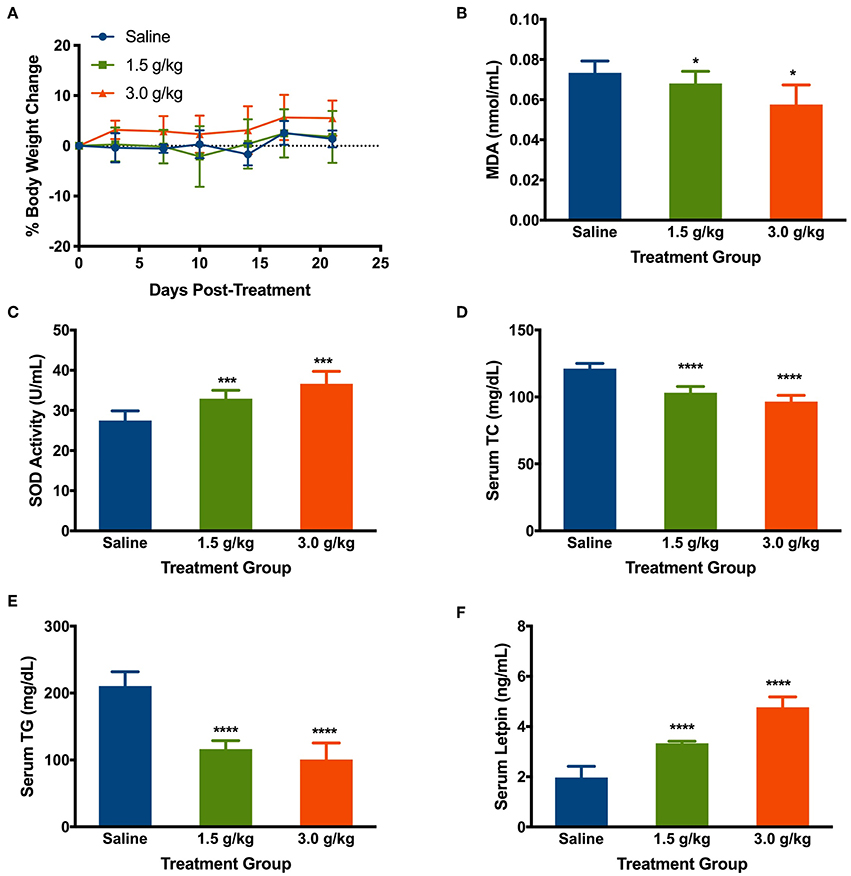
Figure 6. Effects of orally administering spirulina at low (1.5 g/kg) and high (3.0 g/kg) doses on various health factors in healthy mice. (A) The percent of body weight change in mice over 25 days post-treatments. The change of body weight in each mouse was determined by comparing them to the initial weight of the mice before the treatment; (B) Serum level of Malondialdehyde (MDA); (C) Serum level of superoxide dismutase (SOD); (D) Serum level of total cholesterol (TC); (E) Serum level of total triglycerides (TG); and (F) Serum level of Leptin. All mice were gavaged and monitored for 25 consecutive days post-treatment. At the end point of mice, the serum was obtained on the 25th day post-treatment of spirulina. Data are represented as mean ± SD with n = 7 per treatment group, and were analyzed by one-way ANOVA followed by the Tukey post-hoc method with *p < 0.05, ***p < 0.001, and ****p < 0.0001.
Differentially Abundant Cecal Bacteria in High-Dose Spirulina Treatment Were Correlated With the Health Indices
To study the correlation between detected significantly different gut microbiota in cecum and prominent changes of various health biomarkers upon spirulina treatment on the 25th days, a series of spearman correlations were performed using a linear regression model (Figure 7 and Table S2). The relative abundances of Barnesiella and Bacteroides were positively correlated with the increased serum leptin level (Figures 7A,B). Also, the relative abundance of Bacteroides were negatively correlated with the serum lipid concentrations of TC and TG (Figures 7C,D). Moreover, the third identified bacteria, Flavonifractor, was negatively correlated with the activity of SOD, an important antioxidative enzyme providing cellular defense against reactive oxygen species (i.e., ) (Figure 7E).
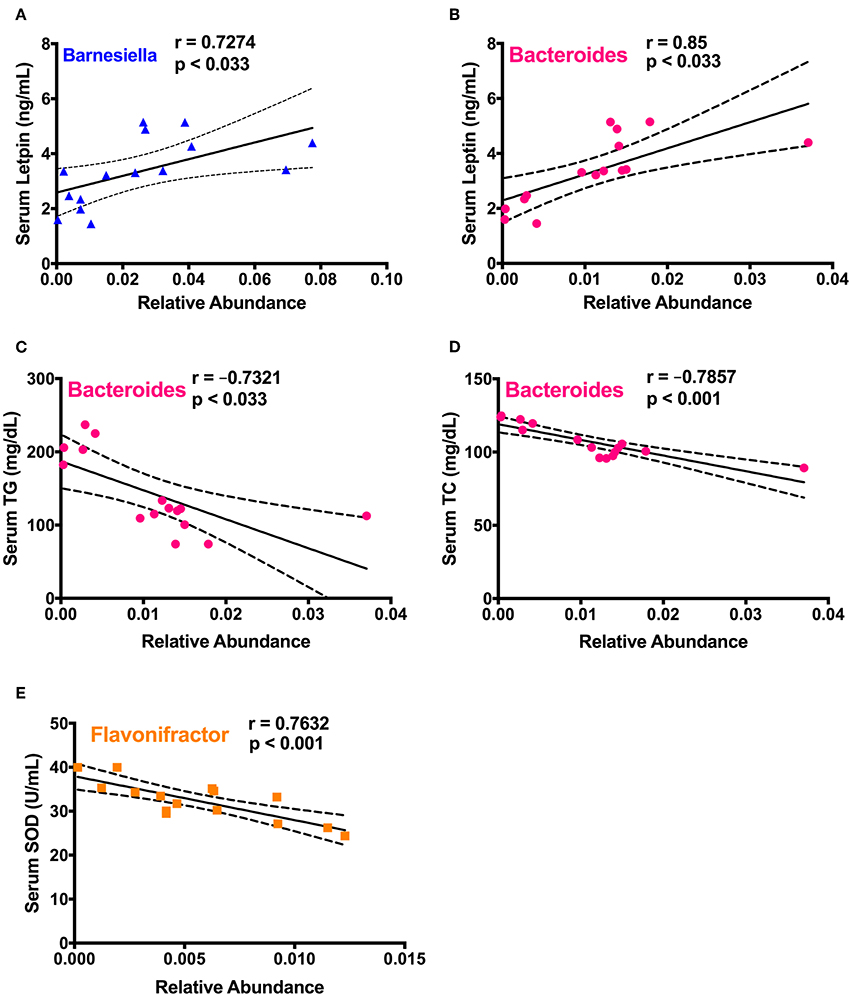
Figure 7. Spearman correlation analysis between the differentially abundant cecal microbial taxa and biological markers in mice. (A) Barnesiella and serum leptin level; (B–D) Bacteroides and the levels of serum leptin, TC, and TG, respectively; (E) Flavonifractor and serum SOD activity. Spearman correlation coefficient (r) and p-value were computed from a linear regression analysis in R, and a regression line with the 95% confidence interval were plotted. The absolute value of r > 0.7 and p < 0.033 were considered to be statistically correlated with each other.
Discussion
The human gut microbiota plays a fundamental role in the well-being of their host, and is generally stable within individuals over time (Clemente et al., 2012). Yet, the intervention of the diet, food supplements and drugs (e.g., antibiotics) can perturb the dynamic of gut microbiota, resulting in a temporal shift of biological responses and sometimes the development of diseases in the host for a long term usage (Jernberg et al., 2007; Wu et al., 2011; Clemente et al., 2012; Pakpour et al., 2017; Quigley, 2018). Particularly, the influence of widely consumed yet not completely characterized nutraceuticals on the gut microbiota and their underlying mechanisms of biological functions remain largely unknown. In the present study, the dose effects of oral administered spirulina on the colonic microbiota community and physiological responses were observed in healthy mice (Figure 1). The microbial (evenness, structure and relative abundance) were significantly altered in groups between various doses of spirulina (Figures 2–5), assuming no difference in microbiome samples in all mice before spirulina treatment (i.e., on the day 0). The mice treated with low dose spirulina showed a short period of significantly decreased microbial α-diversity at the day 14th which were not observed in the high-dose treated group (Figure S2). Such varied patterns of temporal shift in α-diversity between low and high doses could result from intra- and inter-individual heterogeneity of the gut flora in mice even prior to any spirulina treatment (Laukens et al., 2016; Franklin and Ericsson, 2017). Yet, the oral administered low dose of spirulina (i.e., 1.5 g/kg) seem to increase the ratio of Firmicutes and Bacteroidetes from fecal pellets on the 21st day in contrast to the ones treated with the high dose (i.e., 3.0 g/kg) as evidenced by the change in the relative abundance of the two phyla (Figure S4). Such ratio change (Firmicutes/Bacteroidetes) has been shown to associate with obesity, IBD and colorectal cancer and gut physiological barrier structure (Ley et al., 2005; Islam et al., 2011; Devkota et al., 2012; Feng et al., 2015; Hayes et al., 2018). Thus, depending on the therapeutic application, rationally adjusting the dose of spirulina suspension is necessary.
Restoring a specific single bacterial species has been shown to improve therapeutic effects or reduce the disease symptoms (Geller et al., 2017; Roy and Trinchieri, 2017; Tsoi et al., 2017). The examples include the boost of intestinal colonization of Barnesiella for reduction of vancomycin-resistant enterococcus (Ubeda et al., 2013; Crouzet et al., 2015), increased mutualism Bacteroides for the human fitness and carbohydrate fermentation for other intestinal bacteria (Wexler, 2007; Wexler and Goodman, 2017), association of increased Flavonifractor with mental disease bipolar disorder and induction of oxidative stress and inflammation (Coello et al., 2019). However, unlike the definitive prebiotics (e.g., inulin, fructo-oligosaccharides, and galacto-oligosaccharides) for which their structural biochemistry and selectivity of specific bacterial strain, such as Lactobacillus and Biofidobacterium, are well-characterized for conferring health benefits (Gibson et al., 2017; Quigley, 2018), spirulina is a complex organism enriched of potential prebiotics, including carbohydrates, polyphenols, and polyunsaturated fatty acids (Tokuşoglu and Üunal, 2003; Khan et al., 2005; de Jesus Raposo et al., 2016). Knowing the selectivity of spirulina components to particular bacterial taxa may provide insights into the development of novel targeted nutraceutical-based drug delivery systems (Zhang et al., 2017a,b, 2018; Wang et al., 2018). Moreover, to maintain or improve the health benefits of the host, it is important to rationally dose spirulina for the overall homeostasis and holistic interaction of gut microbiota community rather than modulation of single bacterial species.
The changed levels of metabolic parameters (MDA, SOD, TG, TC, and leptin) in the spirulina treated mice indicate potential therapeutic application of spirulina-based therapies in the prevention and treatment of cancer, cardiovascular diseases, and obesity (Figures 6, 7), which are similar to previous reported antioxidative, anti-obesity, and anti-inflammatory effects of spirulina treatment in vivo (Piñero Estrada et al., 2001; Sharma et al., 2007; Yogianti et al., 2014; Yusuf et al., 2016; Neyrinck et al., 2017; Abd El-Hakim et al., 2018; Heo and Choung, 2018; Kata et al., 2018). More importantly, three differentially abundant cecal bacterial genus (Barnesiella, Bacteroides, and Flavonifractor) in the high dose treatment, not the low-dose, were significantly correlated with several health markers. Both Barnesiella and Bacterioides play an important role in the carbohydrate metabolism linking to the obesity (Hooper et al., 2002; Ley et al., 2005; Turnbaugh et al., 2006). The ability of boosting the leptin level and reducing the lipid metabolism by the high-dose spirulina implicates the potential therapeutic application for clinical obesity management (Carlier et al., 2010; Brown et al., 2014; Coello et al., 2019). Yet, the cause-and-effect relationship between the use of spirulina and its components (e.g., polysaccharides), the gut microbiota composition, and the physiological status of the host remains unclear. To assess the microbial functions, in-depth future investigation that utilize metabolomics, metagenomics, and transcriptomics is needed. Other factors such as the dosage regimen (short vs. long duration), sex (male vs. female), and biogeography of gut microbiota (distal vs. proximal colon) also need to be carefully considered in evaluating the therapeutic outcomes of spirulina.
In conclusion, daily orally administering spirulina provided the dose-dependent effects on colonic microbiota community in healthy mice and significantly changed the physiological states of oxidative stress, lipid profiles, and the appetite controlling hormone leptin. These findings shed light on spirulina induced biological functions potentially mediated through the gut microbiota, which in turn may lead to the novel and effective spirulina based pharmaceutical formulation to selectively modulate the gut microbial community in a controllable and precise manner for disease prevention and treatment.
Ethics Statement
This study was carried out in accordance with the recommendations of the Institutional Ethical Guideline of Experimental Animals, the Institutional Animal Care and Use Committee of Northwestern Polytechnical University (Xi'an, China). The protocol was approved by the Institutional Animal Care and Use Committee of Northwestern Polytechnical University (Xi'an, China).
Author Contributions
JH conducted experiments and wrote the first draft of manuscript. YL, ZP, and JL collected and analyzed the data. SP, SW, and QW contributed to analysis of 16S rDNA sequencing of gut microbiota. JS and HC reviewed the manuscript. RXZ proposed the initial idea, designed and supervised the studies, and wrote and proof the manuscript.
Funding
This work was partially supported by the National Natural Science Foundation of China (31700055), the Shaanxi Provincial Natural Science Foundation (2018JQ3019), the China Postdoctoral Science Foundation (2017M610648), the Shaanxi Province Postdoctoral Science Foundation (2017BSHEDZZ102), and the Fundamental Research Funds for the Central Universities (G2018KY0302 and 3102017OQD042).
Conflict of Interest Statement
The authors declare that the research was conducted in the absence of any commercial or financial relationships that could be construed as a potential conflict of interest.
Acknowledgments
Authors gratefully thank Dr. Wan-Ping Lee (The Jackson Laboratory, Farmington, CT) for the valuable suggestions on the diversity analysis.
Supplementary Material
The Supplementary Material for this article can be found online at: https://www.frontiersin.org/articles/10.3389/fcimb.2019.00243/full#supplementary-material
References
Abd El-Hakim, Y. M., Mohamed, W. A., and El-Metwally, A. E. (2018). Spirulina platensis attenuates furan reprotoxicity by regulating oxidative stress, inflammation, and apoptosis in testis of rats. Ecotoxicol. Environ. Saf. 161, 25–33. doi: 10.1016/j.ecoenv.2018.05.073
Bilinski, J., Grzesiowski, P., Sorensen, N., Madry, K., Muszynski, J., Robak, K., et al. (2017). Fecal microbiota transplantation in patients with blood disorders inhibits gut colonization with antibiotic-resistant bacteria: results of a prospective, single-center study. Clin. Infect. Dis. 65, 364–370. doi: 10.1093/cid/cix252
Brown, N. C., Andreazza, A. C., and Young, L. T. (2014). An updated meta-analysis of oxidative stress markers in bipolar disorder. Psychiatry Res. 218, 61–68. doi: 10.1016/j.psychres.2014.04.005
Carlier, J. P., Bedora-Faure, M., K'Ouas, G., Alauzet, C., and Mory, F. (2010). Proposal to unify Clostridium orbiscindens Winter et al. 1991 and Eubacterium plautii (Seguin 1928) Hofstad and Aasjord 1982, with description of Flavonifractor plautii gen. nov., comb. nov, and reassignment of Bacteroides capillosus to Pseudoflavonifractor capillosus gen. nov., comb. nov. Int. J. Syst. Evol. Microbiol. 60, 585–590. doi: 10.1099/ijs.0.016725-0
Cencic, A., and Chingwaru, W. (2010). The role of functional foods, nutraceuticals, and food supplements in intestinal health. Nutrients 2, 611–625. doi: 10.3390/nu2060611
Clemente, J. C., Ursell, L. K., Parfrey, L. W., and Knight, R. (2012). The impact of the gut microbiota on human health: an integrative view. Cell 148, 1258–1270. doi: 10.1016/j.cell.2012.01.035
Coello, K., Hansen, T. H., Sorensen, N., Munkholm, K., Kessing, L. V., Pedersen, O., et al. (2019). Gut microbiota composition in patients with newly diagnosed bipolar disorder and their unaffected first-degree relatives. Brain Behav. Immun. 75, 112–118. doi: 10.1016/j.bbi.2018.09.026
Crouzet, L., Rigottier-Gois, L., and Serror, P. (2015). Potential use of probiotic and commensal bacteria as non-antibiotic strategies against vancomycin-resistant enterococci. FEMS Microbiol. Lett. 362:fnv012. doi: 10.1093/femsle/fnv012
de Clercq, N. C., Groen, A. K., Romijn, J. A., and Nieuwdorp, M. (2016). Gut microbiota in obesity and undernutrition. Adv. Nutr. 7, 1080–1089. doi: 10.3945/an.116.012914
de Jesus Raposo, M. F., de Morais, A. M., and de Morais, R. M. (2016). Emergent sources of prebiotics: seaweeds and microalgae. Mar. Drugs 14:E27. doi: 10.3390/md14020027
Devkota, S., Wang, Y. W., Musch, M. W., Leone, V., Fehlner-Peach, H., Nadimpalli, A., et al. (2012). Dietary-fat-induced taurocholic acid promotes pathobiont expansion and colitis in Il10−/− mice. Nature 487, 104–108. doi: 10.1038/nature11225
Edgar, R. C. (2013). UPARSE: highly accurate OTU sequences from microbial amplicon reads. Nat. Methods 10, 996–998. doi: 10.1038/nmeth.2604
Feng, Q., Liang, S. S., Jia, H. J., Stadlmayr, A., Tang, L. Q., Lan, Z., et al. (2015). Gut microbiome development along the colorectal adenoma-carcinoma sequence. Nat. Commun. 6:6528. doi: 10.1038/ncomms7528
Flint, H. J., Scott, K. P., Louis, P., and Duncan, S. H. (2012). The role of the gut microbiota in nutrition and health. Nat. Rev. Gastroenterol. Hepatol. 9, 577–589. doi: 10.1038/nrgastro.2012.156
Franklin, C. L., and Ericsson, A. C. (2017). Microbiota and reproducibility of rodent models. Lab Anim. 46, 114–122. doi: 10.1038/laban.1222
Geller, L. T., Barzily-Rokni, M., Danino, T., Jonas, O. H., Shental, N., Nejman, D., et al. (2017). Potential role of intratumor bacteria in mediating tumor resistance to the chemotherapeutic drug gemcitabine. Science 357, 1156–1160. doi: 10.1126/science.aah5043
Gibson, G. R., Hutkins, R., Sanders, M. E., Prescott, S. L., Reimer, R. A., Salminen, S. J., et al. (2017). Expert consensus document: The International Scientific Association for Probiotics and Prebiotics (ISAPP) consensus statement on the definition and scope of prebiotics. Nat. Rev. Gastroenterol. Hepatol. 14, 491–502. doi: 10.1038/nrgastro.2017.75
Guinane, C. M., and Cotter, P. D. (2013). Role of the gut microbiota in health and chronic gastrointestinal disease: understanding a hidden metabolic organ. Therap. Adv. Gastroenterol. 6, 295–308. doi: 10.1177/1756283X13482996
Hayes, C. L., Dong, J., Galipeau, H. J., Jury, J., McCarville, J., Huang, X. X., et al. (2018). Commensal microbiota induces colonic barrier structure and functions that contribute to homeostasis. Sci. Rep. 8:14. doi: 10.1038/s41598-018-32366-6
Heo, M. G., and Choung, S. Y. (2018). Anti-obesity effects of Spirulina maxima in high fat diet induced obese rats via the activation of AMPK pathway and SIRT1. Food Funct. 9, 4906–4915. doi: 10.1039/C8FO00986D
Hirahashi, T., Matsumoto, M., Hazeki, K., Saeki, Y., Ui, M., and Seya, T. (2002). Activation of the human innate immune system by Spirulina: augmentation of interferon production and NK cytotoxicity by oral administration of hot water extract of Spirulina platensis. Int. Immunopharmacol. 2, 423–434. doi: 10.1016/S1567-5769(01)00166-7
Hooper, L. V., Midtvedt, T., and Gordon, J. I. (2002). How host-microbial interactions shape the nutrient environment of the mammalian intestine. Annu. Rev. Nutr. 22, 283–307. doi: 10.1146/annurev.nutr.22.011602.092259
Islam, K. B., Fukiya, S., Hagio, M., Fujii, N., Ishizuka, S., Ooka, T., et al. (2011). Bile acid is a host factor that regulates the composition of the cecal microbiota in rats. Gastroenterology 141, 1773–1781. doi: 10.1053/j.gastro.2011.07.046
Jernberg, C., Lofmark, S., Edlund, C., and Jansson, J. K. (2007). Long-term ecological impacts of antibiotic administration on the human intestinal microbiota. ISME J. 1, 56–66. doi: 10.1038/ismej.2007.3
Kata, F. S., Athbi, A. M., Manwar, E. Q., Al-Ashoor, A., Abdel-Daim, M. M., and Aleya, L. (2018). Therapeutic effect of the alkaloid extract of the cyanobacterium Spirulina platensis on the lipid profile of hypercholesterolemic male rabbits. Environ. Sci. Pollut. Res. Int. 25, 19635–42. doi: 10.1007/s11356-018-2170-4
Khan, Z., Bhadouria, P., and Bisen, P. S. (2005). Nutritional and therapeutic potential of Spirulina. Curr. Pharm. Biotechnol. 6, 373–379. doi: 10.2174/138920105774370607
Laparra, J. M., and Sanz, Y. (2010). Interactions of gut microbiota with functional food components and nutraceuticals. Pharmacol. Res. 61, 219–225. doi: 10.1016/j.phrs.2009.11.001
Laukens, D., Brinkman, B. M., Raes, J., De Vos, M., and Vandenabeele, P. (2016). Heterogeneity of the gut microbiome in mice: guidelines for optimizing experimental design. FEMS Microbiol. Rev. 40, 117–132. doi: 10.1093/femsre/fuv036
Ley, R. E., Backhed, F., Turnbaugh, P., Lozupone, C. A., Knight, R. D., and Gordon, J. I. (2005). Obesity alters gut microbial ecology. Proc. Natl. Acad. Sci. U.S.A. 102, 11070–11075. doi: 10.1073/pnas.0504978102
Li, M., Liang, P., Li, Z., Wang, Y., Zhang, G., Gao, H., et al. (2015). Fecal microbiota transplantation and bacterial consortium transplantation have comparable effects on the re-establishment of mucosal barrier function in mice with intestinal dysbiosis. Front. Microbiol. 6:692. doi: 10.3389/fmicb.2015.00692
Li, S. S., Zhu, A., Benes, V., Costea, P. I., Hercog, R., Hildebrand, F., et al. (2016). Durable coexistence of donor and recipient strains after fecal microbiota transplantation. Science 352, 586–589. doi: 10.1126/science.aad8852
Lozupone, C. A., Stombaugh, J. I., Gordon, J. I., Jansson, J. K., and Knight, R. (2012). Diversity, stability and resilience of the human gut microbiota. Nature 489, 220–230. doi: 10.1038/nature11550
Lu, H. K., Hsieh, C. C., Hsu, J. J., Yang, Y. K., and Chou, H. N. (2006). Preventive effects of Spirulina platensis on skeletal muscle damage under exercise-induced oxidative stress. Eur. J. Appl. Physiol. 98, 220–226. doi: 10.1007/s00421-006-0263-0
Mazokopakis, E. E., Starakis, I. K., Papadomanolaki, M. G., Mavroeidi, N. G., and Ganotakis, E. S. (2014). The hypolipidaemic effects of Spirulina (Arthrospira platensis) supplementation in a Cretan population: a prospective study. J. Sci. Food Agric. 94, 432–437. doi: 10.1002/jsfa.6261
Mills, S., Ross, R. P., and Hill, C. (2017). Bacteriocins and bacteriophage; a narrow-minded approach to food and gut microbiology. FEMS Microbiol. Rev. 41, S129–S153. doi: 10.1093/femsre/fux022
Neyrinck, A. M., Taminiau, B., Walgrave, H., Daube, G., Cani, P. D., Bindels, L. B., et al. (2017). Spirulina protects against hepatic inflammation in aging: an effect related to the modulation of the gut microbiota? Nutrients 9:E633. doi: 10.3390/nu9060633
Ngo-Matip, M. E., Pieme, C. A., Azabji-Kenfack, M., Biapa, P. C., Germaine, N., Heike, E., et al. (2014). Effects of Spirulina platensis supplementation on lipid profile in HIV-infected antiretroviral naive patients in Yaounde-Cameroon: a randomized trial study. Lipids Health Dis. 13:191. doi: 10.1186/1476-511X-13-191
Pakpour, S., Bhanvadia, A., Zhu, R., Amarnani, A., Gibbons, S. M., Gurry, T., et al. (2017). Identifying predictive features of Clostridium difficile infection recurrence before, during, and after primary antibiotic treatment. Microbiome 5:148. doi: 10.1186/s40168-017-0368-1
Piñero Estrada, J. E., Bermejo Bescós, P., and Villar del Fresno, A. M. (2001). Antioxidant activity of different fractions of Spirulina platensis protean extract. Farmaco 56, 497–500. doi: 10.1016/S0014-827X(01)01084-9
Quigley, E. M. M. (2018). Prebiotics and probiotics in digestive health. Clin. Gastroenterol. Hepatol. 17, 333–344. doi: 10.1016/j.cgh.2018.09.028
Rasmussen, H. E., Martinez, I., Lee, J. Y., and Walter, J. (2009). Alteration of the gastrointestinal microbiota of mice by edible blue-green algae. J. Appl. Microbiol. 107, 1108–1118. doi: 10.1111/j.1365-2672.2009.04288.x
Roy, S., and Trinchieri, G. (2017). Microbiota: a key orchestrator of cancer therapy. Nat. Rev. Cancer 17, 271–285. doi: 10.1038/nrc.2017.13
Sharma, M. K., Sharma, A., Kumar, A., and Kumar, M. (2007). Spirulina fusiformis provides protection against mercuric chloride induced oxidative stress in Swiss albino mice. Food Chem. Toxicol. 45, 2412–2419. doi: 10.1016/j.fct.2007.06.023
Sommer, F., and Backhed, F. (2013). The gut microbiota–masters of host development and physiology. Nat. Rev. Microbiol. 11, 227–238. doi: 10.1038/nrmicro2974
Spor, A., Koren, O., and Ley, R. (2011). Unravelling the effects of the environment and host genotype on the gut microbiome. Nat. Rev. Microbiol. 9, 279–290. doi: 10.1038/nrmicro2540
Szulinska, M., Gibas-Dorna, M., Miller-Kasprzak, E., Suliburska, J., Miczke, A., Walczak-Galezewska, M., et al. (2017). Spirulina maxima improves insulin sensitivity, lipid profile, and total antioxidant status in obese patients with well-treated hypertension: a randomized double-blind placebo-controlled study. Eur. Rev. Med. Pharmacol. Sci. 21, 2473–2481.
Tang, W. H., Kitai, T., and Hazen, S. L. (2017). Gut microbiota in cardiovascular health and disease. Circ. Res. 120, 1183–1196. doi: 10.1161/CIRCRESAHA.117.309715
Thursby, E., and Juge, N. (2017). Introduction to the human gut microbiota. Biochem. J. 474, 1823–1836. doi: 10.1042/BCJ20160510
Tokuşoglu, Ö., and Üunal, M. (2003). Biomass nutrient profiles of three microalgae: Spirulina platensis, Chlorella vulgaris, and Isochrisis galbana. J. Food Sci. 68, 1144–1148. doi: 10.1111/j.1365-2621.2003.tb09615.x
Torres-Duran, P. V., Ferreira-Hermosillo, A., and Juarez-Oropeza, M. A. (2007). Antihyperlipemic and antihypertensive effects of Spirulina maxima in an open sample of Mexican population: a preliminary report. Lipids Health Dis. 6:33. doi: 10.1186/1476-511X-6-33
Tsoi, H., Chu, E. S. H., Zhang, X., Sheng, J., Nakatsu, G., Ng, S. C., et al. (2017). Peptostreptococcus anaerobius induces intracellular cholesterol biosynthesis in colon cells to induce proliferation and causes dysplasia in mice. Gastroenterology 152, 1419–1433.e1415. doi: 10.1053/j.gastro.2017.01.009
Turnbaugh, P. J., Ley, R. E., Mahowald, M. A., Magrini, V., Mardis, E. R., and Gordon, J. I. (2006). An obesity-associated gut microbiome with increased capacity for energy harvest. Nature 444, 1027–1031. doi: 10.1038/nature05414
Ubeda, C., Bucci, V., Caballero, S., Djukovic, A., Toussaint, N. C., Equinda, M., et al. (2013). Intestinal microbiota containing Barnesiella species cures vancomycin-resistant Enterococcus faecium colonization. Infect. Immun. 81, 965–973. doi: 10.1128/IAI.01197-12
Verdu, E. F. (2009). Probiotics effects on gastrointestinal function: beyond the gut? Neurogastroenterol. Motil. 21, 477–480. doi: 10.1111/j.1365-2982.2009.01297.x
Wang, H., Zhang, X., Wang, S., Li, H., Lu, Z., Shi, J., et al. (2018). Mannan-oligosaccharide modulates the obesity and gut microbiota in high-fat diet-fed mice. Food Funct. 9, 3916–3929. doi: 10.1039/C8FO00209F
Wang, Z. G., Zhang, R. X., Zhang, C., Dai, C. X., Ju, X. R., and He, R. (2019). Fabrication of stable and self-assembling rapeseed protein nanogel for hydrophobic curcumin delivery. J. Agric. Food Chem. 67, 887–894. doi: 10.1021/acs.jafc.8b05572
Wexler, A. G., and Goodman, A. L. (2017). An insider's perspective: Bacteroides as a window into the microbiome. Nat. Microbiol. 2:17026. doi: 10.1038/nmicrobiol.2017.26
Wexler, H. M. (2007). Bacteroides: the good, the bad, and the nitty-gritty. Clin. Microbiol. Rev. 20, 593–621. doi: 10.1128/CMR.00008-07
Wu, G. D., Chen, J., Hoffmann, C., Bittinger, K., Chen, Y. Y., Keilbaugh, S. A., et al. (2011). Linking long-term dietary patterns with gut microbial enterotypes. Science 334, 105–108. doi: 10.1126/science.1208344
Yogianti, F., Kunisada, M., Nakano, E., Ono, R., Sakumi, K., Oka, S., et al. (2014). Inhibitory effects of dietary Spirulina platensis on UVB-induced skin inflammatory responses and carcinogenesis. J. Invest. Dermatol. 134, 2610–2619. doi: 10.1038/jid.2014.188
Yusuf, M. S., Hassan, M. A., Abdel-Daim, M. M., Nabtiti, A. S., Ahmed, A. M., Moawed, S. A., et al. (2016). Value added by Spirulina platensis in two different diets on growth performance, gut microbiota, and meat quality of Japanese quails. Vet. World 9, 1287–1293. doi: 10.14202/vetworld.2016.1287-1293
Zhang, R. X., Ahmed, T., Li, L. Y., Li, J., Abbasi, A. Z., and Wu, X. Y. (2017a). Design of nanocarriers for nanoscale drug delivery to enhance cancer treatment using hybrid polymer and lipid building blocks. Nanoscale 9, 1334–1355. doi: 10.1039/C6NR08486A
Zhang, R. X., Li, J., Zhang, T., Amini, M. A., He, C. S., Lu, B., et al. (2018). Importance of integrating nanotechnology with pharmacology and physiology for innovative drug delivery and therapy - an illustration with firsthand examples. Acta Pharmacol. Sin. 39, 825–844. doi: 10.1038/aps.2018.33
Zhang, R. X., Li, L. Y., Li, J., Xu, Z. S., Abbasi, A. Z., Lin, L., et al. (2017b). Coordinating biointeraction and bioreaction of ananocarrier material and an anticancer drug to overcome membrane rigidity and target mitochondria in multidrug-resistant cancer cells. Adv. Funct. Mater. 27:12. doi: 10.1002/adfm.201700804
Keywords: oral delivery, microalgae, large intestine, prebiotics, microorganisms, prevention, 16s rDNA sequencing
Citation: Hu J, Li Y, Pakpour S, Wang S, Pan Z, Liu J, Wei Q, She J, Cang H and Zhang RX (2019) Dose Effects of Orally Administered Spirulina Suspension on Colonic Microbiota in Healthy Mice. Front. Cell. Infect. Microbiol. 9:243. doi: 10.3389/fcimb.2019.00243
Received: 29 January 2019; Accepted: 21 June 2019;
Published: 05 July 2019.
Edited by:
Hsin-Jung Joyce Wu, University of Arizona, United StatesReviewed by:
César López-Camarillo, Universidad Autónoma de la Ciudad de México, MexicoVanessa L. Hale, The Ohio State University, United States
Copyright © 2019 Hu, Li, Pakpour, Wang, Pan, Liu, Wei, She, Cang and Zhang. This is an open-access article distributed under the terms of the Creative Commons Attribution License (CC BY). The use, distribution or reproduction in other forums is permitted, provided the original author(s) and the copyright owner(s) are credited and that the original publication in this journal is cited, in accordance with accepted academic practice. No use, distribution or reproduction is permitted which does not comply with these terms.
*Correspondence: Huaixing Cang, aHhjYW5nQG53cHUuZWR1LmNu; Rui Xue Zhang, emhhbmdydWl4dWVAbndwdS5lZHUuY24=