- Microbiology Division, CSIR-Central Drug Research Institute, Lucknow, India
Mycobacterium tuberculosis (Mtb) has the remarkable ability to persist with a modified metabolic status and phenotypic drug tolerance for long periods in the host without producing symptoms of active tuberculosis. These persisters may reactivate to cause active disease when the immune system becomes disrupted or compromised. Thus, the infected hosts with the persisters serve as natural reservoir of the deadly pathogen. Understanding the host and bacterial factors contributing to Mtb persistence is important to devise strategies to tackle the Mtb persisters. Host lipids act as the major source of carbon and energy for Mtb. Fatty acids derived from the host cells are converted to triacylglycerols (triglycerides or TAG) and stored in the bacterial cytoplasm. TAG serves as a dependable, long-term energy source of lesser molecular mass than other storage molecules like glycogen. TAG are found in substantial amounts in the mycobacterial cell wall. This review discusses the production, accumulation and possible roles of TAG in mycobacteria, pointing out the aspects that remain to be explored. Finally, the essentiality of TAG synthesis for Mtb is discussed with implications for identification of intervention strategies.
Introduction
A recent global tuberculosis (TB) report by the World Health Organization reveals that the TB epidemic is larger than previously estimated despite a global fall in the number of TB deaths and the TB incidence rate [World Health Organization (WHO), 2016]. Also, “The End TB strategy”approved in 2014 calls for a 90% reduction in TB deaths and 80% reduction in TB incidence rates by the year 2030, compared to 2015. The objectives of current anti-tuberculosis therapy are to (1) decrease the severity of the disease by rapidly reducing the number of actively growing Mycobacterium tuberculosis (Mtb) in the patient, thereby, preventing death and halting bacterial transmission (2) prevent relapse by eliminating all populations of persisting bacilli; and (3) prevent development of drug resistance (Nahid et al., 2016). Persistence of Mtb is often described as a condition in which multiple growth limiting factors within the host tissues force a certain population of the bacilli to undergo a state of suboptimal growth or complete growth arrest accompanied by phenotypic drug tolerance (Gold and Nathan, 2017). Persisters exhibit a modified metabolic status in which aerobic respiration and ribosomal function are decreased while lipid utilization is increased (Garton et al., 2008). Persisters, under circumstances of dysregulated immunity may lead to reactivation TB. In fact, the lengthy treatment regimen for TB is necessary to eliminate the persisting population of Mtb. Hence understanding how Mtb persists in the face of adverse conditions in the host is crucial to devise strategies to reduce the duration of treatment and minimize post-treatment relapse.
M. tuberculosis Accumulating Triacylglycerols Is Slow/Non-replicating and Drug Tolerant
Triacylglycerol (TAG), a triester of glycerol with fatty acids, is the major energy depot of all eukaryotes and bacteria of the actinomycetes group. Actinomycetes that accumulate large amounts of TAG include Mycobacterium, Rhodococcus, Nocardia, and Streptomyces (Olukoshi and Packter, 1994; Alvarez et al., 2000; Alvarez and Steinbüchel, 2002). The energy derived from the complete oxidation of the fatty acyl chains of TAG is estimated to be more than twice the same weight of carbohydrate or protein (Sturley and Hussain, 2012). Being nonpolar, triacylglycerols can store nearly six times more energy than the same amount of hydrated glycogen and also provide energy for longer time than the fast catabolising glycogen (Berg et al., 2002).
Evidences suggest that Mtb depends on host nutrients especially lipids to survive in the host (Bloch and Segal, 1956). Intracellular inclusions were detected in mycobacterial species by several studies since 1946 (reviewed in Garton et al., 2002). The use of fluorescent lipid probes showed presence of intracellular lipid inclusions (ILIs) in M. smegmatis and Mtb (Christensen et al., 1999; Garton et al., 2002). Thin layer chromatography, NMR and GC-MS analyses of nonpolar lipid extracts from the ILI-rich M. smegmatis cultures showed TAG containing variable fatty acids as the primary lipid component of ILIs (Garton et al., 2002). The term ILIs is used in this article to describe the lipid bodies in the mycobacterial cytoplasm. Mtb with ILIs has been detected in patient sputa as well as in in vitro cultures modeling non-replicating persistence (Garton et al., 2008; Rodríguez et al., 2014; Vijay et al., 2017) (Figure 1, inset). Interestingly, it has been observed that the ILI-positive bacilli counts correlated significantly with time to detect Mtb growth in diagnostic liquid cultures, which indicated that being non-replicating, bacilli with ILIs took more time to initiate growth (Garton et al., 2008). It is important to note that various in vitro studies have shown that Mtb and other mycobacteria exhibit phenotypic drug tolerance subsequent to accumulation of ILIs (Deb et al., 2009; Rodríguez et al., 2014; Hammond et al., 2015). A Mtb H37Rv mutant with reduced TAG synthesis has been found to be more sensitive to antibiotics during mice infection than the wild type and complemented mutant (Baek et al., 2011).
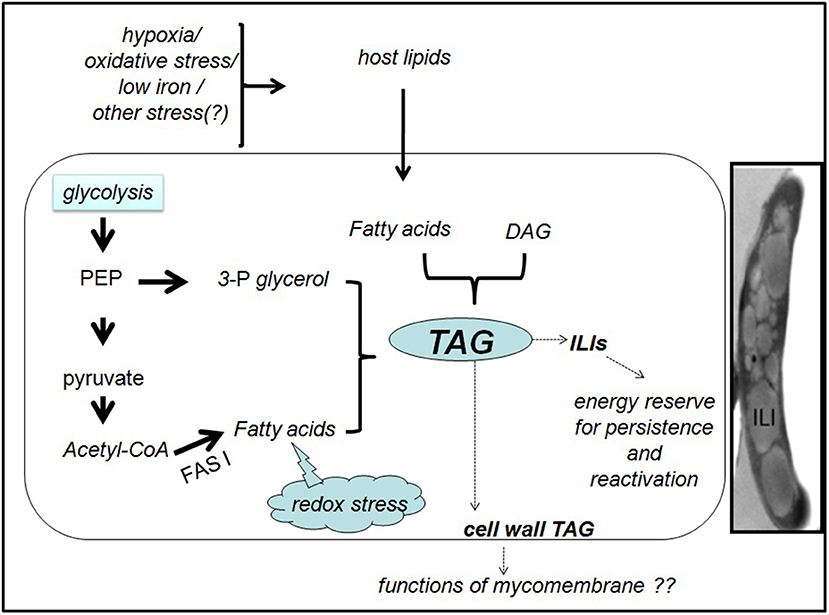
Figure 1. Generation and fate of TAG in M. tuberculosis inside the host. In presence of a lipid-rich diet and/ or various indicated stresses, M. tuberculosis synthesizes TAG, either de novo from fatty acids and 3-phosphoglycerol (3-P glycerol) or from pre-existing DAG. Phosphoenol pyruvate (PEP) and pyruvate may provide the precursors for de novo TAG synthesis. Reductive stress generated due to excess metabolic degradation of fatty acids may also induce the conversion of excess fatty acids to TAG. The cytoplasmic TAG in the ILIs may promote the pathogen survival during dormancy and reactivation. Functions of the cell wall associated TAG are unknown. Inset: Transmission electron microscopy image of Mtb in TB patient sputum showing the intracellular lipid inclusion (ILI)s. The image is reproduced with permission from Vijay et al. (2017).
Biosynthesis of TAG in Mycobacteria
Potential Pathways Leading to TAG Biosynthesis
Garton et al demonstrated that M. smegmatis made ILIs during stationary phase from simple carbon sources in low-nitrogen conditions, but the formation was rapid if fatty acids were provided (Garton et al., 2002). Transcript analysis suggests that Mtb entering growth arrest due to in vitro or in vivo stress undergo metabolic adaptation for long-term persistence (Shi et al., 2010). Shi et al., induced in vitro growth arrest in Mtb either by hypoxia or by nitric oxide treatment while culturing in standard media containing fatty acids or media with defined carbon sources but no fatty acids. The transcript data along with flux balance analysis using an in silico metabolic network proposed a metabolic model for Mtb persisters where carbon flow is redistributed from providing energy and biosynthetic precursors for bacterial growth to accumulating storage compounds like TAG and glutamate (Shi et al., 2010). According to this model, in non replicating Mtb, carbon is rerouted to TAG synthesis in at least two ways. First, due to the downregulation of glycolysis, TCA cycle and pentose phosphate pathway, pyruvate and phosphoenol pyruvate are preferentially used for glyceroneogenesis and TAG synthesis. Second, uncoupling of FASI and FASII (fatty acid synthase I and II systems sequentially involved in mycobacterial fatty acid biosynthesis), FASI products are rerouted to TAG synthesis. An extensive system biology study of dormant Mtb under in vitro hypoxia proposed that metabolites upstream of diacylglycerol (DAG) decrease in production while TAG accumulation may result from conversion of existing DAGs to TAGs by triacylglyceride synthases (Galagan et al., 2013). Figure 1 summarizes the potential routes of TAG synthesis in Mtb.
In more direct experimental approaches, pulsing the macrophages with fluorescent labeled fatty acid has shown that intracellular Mtb imports fatty acids from the macrophage to synthesize its own TAG (Daniel et al., 2011; Nazarova et al., 2017). Fatty acid uptake by Mtb takes place through the Mce1 transporter complex (Nazarova et al., 2017). While Mtb has been shown to derive fatty acids from TAG accumulating inside lipid loaded macrophages (Daniel et al., 2011), a recent study shows that the bacilli were able to accumulate ILIs even in the absence of macrophage TAG and hence suggests that host TAG may not be the primary source of fatty acids for Mtb (Knight et al., 2018).
Molecular Variety of TAG in Mycobacteria
Detection of TAG species showing variable TLC mobilities in M. smegmatis suggests the ability of the mycobacteria to synthesize a large variety of TAG (Garton et al., 2002). In addition to the conventional TAG, pathogenic as well as non-pathogenic mycobacteria contain unusual TAGs bearing a meromycolate substituent called monomeromycolyl-diacylglycerol or MMDAG (Kremer et al., 2005). Mass spectrometry analysis of M. smegmatis biofilm revealed a complex mixture of TAG and MMDAG (Purdy et al., 2013). Various species of TAG and MMDAG consisted of the common structure in which Δ918:1- and 16:0-fatty acyl substituents are exclusively located at sn-1 and sn-2, respectively. The sn-3 position of TAGs contained saturated or mono-unsaturated fatty acyl chains of chain lengths varying from C10 to C26. MMDAG species varied with respect to the meromycolylic fatty acyl chain (C43-C57) at sn-3 (Purdy et al., 2013), Eventhough the structures of Mtb TAGs and MMDAGs are not yet known, at least 32 species of TAG have been detected by mass spectrometry in MtbH37Rv (Martinot et al., 2016).
TAG Synthases of Mycobacteria
Experimental evidence of TAG synthesis in mycobacteria came from a study in which M. avium incorporated 14-C-palmitic acid into TAG within minutes of exposure to the fatty acid (McCarthy, 1971). Bacteria pulsed with 14-C-palmitic acid for 30 min released higher levels of 14-CO2, when supplemented with both glycerol and unlabelled palmitic acid rather than palmitic acid alone, demonstrating the utilization of the newly synthesized TAG. Subsequently, diglyceride acyltransferase [EC 2.3.1.20] (triacylglycerol synthase) activity was detected in a cell-free extract from M. smegmatis (Nakagawa et al., 1976). This class of enzymes are solely responsible for the final acylation of diacylglycerols (DAG).
Daniel et al identified 15 genes in Mtb H37Rv genome, which code for putative triacylglycerol synthase (Tgs)s (Daniel et al., 2004). When expressed in the E. coli host, all the 15 gene products showed Tgs activity with diolein and oleoyl-CoA as substrates. While Rv3130c (tgs1) showed the highest activity among all, the remaining proteins showed moderate to very low activity with diolein and oleoyl-CoA. Some of the proteins also showed mild wax ester synthase activity, which was however not correlated to their Tgs activity (Daniel et al., 2004). In Mtb, the expression of some of these genes, particularly Rv3130c/tgs1, was up-regulated under hypoxia/nitric oxide –induced in vitro non-replicating persistence, along with a corresponding increase in TAG accumulation. In addition to these putative Tgs, TAGs in Mtb could also be synthesized by the mycolyl transferase Ag85A (Rv3804c) by transesterification of DAG (Elamin et al., 2011). Table 1 lists the confirmed and putative TAG synthases in Mtb along with some basic information. The Tgs members may differ from each other with respect to substrate specificity and inducing microenvironment.
Mutagenesis studies suggest that none of the confirmed/putative Tgs are essential for in vitro growth of Mtb H37Rv (Sassetti et al., 2001; Griffin et al., 2011; DeJesus et al., 2017). Since H37Rv strain does not accumulate TAG during in vitro growth unlike the clinical Mtb isolates, the tgs gene essentiality data for H37Rv cannot be extended to clinical strains. tgs1 disruption inhibits most of the TAG accumulation under acidic, static or hypoxic growth conditions in vitro (Sirakova et al., 2006). tgs1 deletion mutant was also found unable to arrest its growth under in vitro hypoxia, low pH and low iron (Baek et al., 2011). TAG synthesis was drastically reduced in a tgs1- deletion mutant of Mtb inside foamy macrophages (Daniel et al., 2011). Mtb- Tgs 1 preferably esterifies C26:0 fatty acyl chains to DAG (Sirakova et al., 2006). The residual TAG detected in the tgs1deletion mutant did not have C26:0 fatty acyl chains, indicating the substrate specificity of the multiple Tgs. A Tgs of M. abscessus with reasonable homology to MtbTgs 1, has been reported to be responsible for most of the TAG synthesized during exponential phase in M. abscessus (Viljoen et al., 2016).
Two of the putative Tgs- Rv3087 and Rv3371 were found essential for in vivo growth of H37Rv in C57BL/6J mouse spleen, by transposon site hybridization (Sassetti and Rubin, 2003). We have reported that an unmarked deletion mutant of Rv3371 continued replication and thus failed to enter persistence under in vitro hypoxia, low iron or nitrosative stress (Rastogi et al., 2017). Moreover, transposon mutants of tgs1 and Rv3371 were among the “over-represented” mutants under low oxygen environment, which suggested their inability to arrest cell division (Baek et al., 2011). Rv3371 has the closest aminoacid sequence (43%) similarity to Tgs1 than any other Tgs in MtbH37Rv (Daniel et al., 2004). Two other putative Tgs- Rv0895 and Rv3480c were found essential for the survival of H37Rv in primary murine macrophages, by transposon site hybridization (Rengarajan et al., 2005). We used an adipocyte-Mtb infection model to mimic the nutritional status inside foamy macrophages.Transcript analysis by quantitative RT-PCR showed that tgs1, tgs2, Rv3371, and Rv3804c are the predominant tgs genes upregulated in Mtb H37Rv during survival inside adipocytes (Rastogi et al., 2016). All these studies point out that expression of tgs genes is regulated and their expression is induced or down regulated by specific signals. Hence, validation of individual Tgs proteins as drug target requires testing of their essentiality for in vivo growth or persistence.
TAG Utilization by Mycobacteria
TAGs are hydrolysed to provide free fatty acids to enter β-oxidation, which in turn can provide energy and acetyl Co-A. This acetyl Co-A is thought to be used for both lipid synthesis via FAS I and anapleurosis of the TCA cycle (Shi et al., 2010). There are at least 24 genes in the Mtb H37Rv genome that code for lipase/esterase proteins (Deb et al., 2006). Of these only one protein Rv3097c (LipY), a hormone-sensitive lipase was found to hydrolyze TAG with long chain fatty acids. A lipY- deficient Mtb H37Rv mutant, showed drastically decreased TAG utilization under nutrient deprived condition (Deb et al., 2006). A recent study using BCG infected foamy macrophages revealed that LipY functions in two forms- as an extracellular lipase that hydrolyses the host TAG and as a cytoplasmic lipase that hydrolyses the TAG in bacterial ILIs (Santucci et al., 2018). This study not only supports the earlier studies that LipY is the major TAG lipase of Mtb, but also suggests the additional involvement of other mycobacterial lipases in ILI formation and utilization. We used 3T3L1 adipocytes as an alternative to faomy macrophages and found intra-adipocyte Mtb to significantly upregulate the expression of lipY and a few other lip genes (Rastogi et al., 2016).
Location of TAG in Mycobacteria
TAG is accumulated in mycobacteria as two forms- peripheral deposits associated with the cell envelope and inclusion bodies in the cytoplasm (ILIs) (Christensen et al., 1999). DAGs and TAGs have been found in substantial amounts in the the outer membrane (mycomembrane) of M. smegmatis (Bansal-Mutalik and Nikaido, 2014). Much earlier, Ortalo-Magne'et al detected TAGs deep inside Mtb capsule and also surface-exposed TAG in M. smegmatis, M. avium, and M. aurum (Ortalo-Magné et al., 1996). TAG production predominantly through a Tgs1 homolog, during exponential phase has also been reported for M. abscessus. Interestingly, electron microscopy images revealed that there was no accumulation of ILIs, which suggests the possible accumulation of TAG in the cell wall (Viljoen et al., 2016).
In Mtb, an efflux pump Rv1410 and lipoprotein LprG which are encoded by the Rv1410c-Rv1411c operon, were found to transport TAG from cytoplasm to the outermembrane. Over expression of these proteins in Mtb also led to release of TAG into the culture medium (Martinot et al., 2016). It was observed that LprG-Rv1410 were important to protect the bacilli from toxic byproducts of cholesterol through their disposal via TAG from the cytoplasm. It remains to be seen if the export of TAG from cytoplasm is just a regulatory mechanism of intracellular TAG accumulation or the cell wall associated TAG has other unknown functions. The observation in M. abscessus of increased TAG synthesis without corresponding formation of ILIs (Viljoen et al., 2016) suggests that accumulation of TAG in the cell wall is not to get rid of the excess cytoplasmic TAG.
Structure and Formation of ILIs in Mycobacteria
Lipid bodies in eukaryotes consist of a hydrophobic core of neutral lipids, mostly TAGs and steryl esters, or wax esters surrounded by a phospholipid (PL) hemimembrane with a few proteins bound to the surface of the particles (Murphy, 2001; Tauchi-Sato et al., 2002). A few proteins have been identified to be associated to ILIs in mycobacteria. They include TAG synthesizing and degrading enzymes (Low et al., 2010) and stress responsive proteins (Low et al., 2010; Armstrong et al., 2016). Proteins with amphipathic helix motifs are thought to associate with ILIs in mycobacteria (Armstrong et al., 2018). Low et al isolated ILI- associated proteins from dormant M. bovis BCG in hypoxic culture and identified BCG1721, tgs1, tgs2, BCG1489c, BCG1169c, and hspX (α-crystallin) (Low et al., 2010). The proteome of ILIs may differ with the condition that induces their formation. MPER-1 is a protein in Mtb that has been identified to be essential for TAG accumulation in ILIs during dormancy induced by a combination of in vitro stresses (Daniel et al., 2016). This protein has weak amino acid similarity to mammalian perilipin1, which is exclusively associated to lipid droplets in adipocytes and regulates lipolysis (Brasaemle et al., 2009).
TAG and other neutral lipids in eukaryotes are synthesized in the lumen of endoplasmic reticulum (ER) and are rapidly deposited into cytoplasmic lipid droplets that bud out of the ER (Murphy and Vance, 1999; Sturley and Hussain, 2012). Murphy and Vance proposed the plasma membrane as the site of origin of ILIs in bacteria (Murphy and Vance, 1999). Later, studies on Acinetobacter calcoaceticus and Rhodococcus opacus by Wältermann et al demonstrated a model for ILI formation in bacteria (Wältermann et al., 2005). According to this model, wax esters and TAG are synthesized at the bacterial plasma membrane by respective enzymes which are docked to the membrane. The newly synthesized lipids form small lipid droplets get coated by a monolayer of phospholipids. Small lipid droplets subsequently conglomerate to membrane-bound lipid-prebodies which are then released into the cytoplasm. The lipid prebodies mature as the small lipid droplets inside them coalesce.
Most of the Tgs proteins of Mtb H37Rv have been detected in the cell membrane fraction (Table 1). Likewise, the Tgs proteins of M. abscessus have been found exclusively in cell wall and cell membrane fractions (Viljoen et al., 2016). In addition to the predominant presence in the cell membrane fraction, minor amounts of Tgs proteins have been found in cytosolic fraction (Stöveken et al., 2005; Rastogi et al., 2017). Since these proteins do not have transmembrane domain, their membrane localization is thought to be mediated by ionic or hydrophobic interactions.
Factors That Induce Formation of ILIs in Mycobacteria
TAG accumulation during exponential growth has been reported in hypervirulent Mtb strains of the W-Beijing Lineage (Reed et al., 2007). The study also showed that Rv3130c/tgs1, which belongs to the DosR regulon, was responsible for the TAG synthesis. Since all the DosR controlled genes were up-regulated, it was presumed that it “pre- adaptated” these hyper virulent strains to the stress-filled microenvironments in vivo. However, this study used only lipid analysis by TLC and did not do ultrastructural study to confirm the accumulation of ILIs. Accumulation of ILIs during exponential growth in vitro by clinical isolates of Mtb, but not by laboratory adapted strain H37Rv, has been reported recently (Vijay et al., 2017). Hence, it can be speculated that stress encountered in vivo might stimulate TAG accumulation in Mtb. The same strains when grown in vitro under optimal conditions may initially retain the property, but gradually lose it as they get adapted to the in vitro growth. Thereafter, TAG accumulation might be induced only under stress conditions, including stationary phase (Hammond et al., 2015).
Fatty Acids
Lipid loaded macrophages known as foamy macrophages in the tubercular granuloma play a key role in persistence of pathogenic mycobacteria (Peyron et al., 2008; Cáceres et al., 2009; Russell et al., 2009; Caire-Brändli et al., 2014). Eventhough hypoxic culture conditions greatly enhance the accumulation of TAG containing lipid bodies by infected macrophages, macrophages under normoxic conditions also accumulate lipid bodies (Daniel et al., 2011). M. marinum inside the host Dictyostelium acquires host fatty acids after the host lipid bodies fuse with the Mycobacterium-containing vesicle (Barisch et al., 2015; Barisch and Soldati, 2017). Mtb inside adipocytes also accumulate ILIs (Neyrolles et al., 2006; Agarwal et al., 2014). M. canettii has been shown to replicate and accumulate ILIs inside brown and white preadipocytes (Bouzid et al., 2017). However, a more recent study demonstrated that macrophage lipid body formation during Mtb infection is part of an adaptive immune response activated by IFN-γ (Knight et al., 2018). The study also found that in the absence of macrophage lipid bodies the bacilli were able to accumulate ILIs, but the presence of lipid bodies induced by IFN-γ impaired the accumulation.
Mtb grown in presence of long chain fatty acids rather than dextrose as sole carbon source accumulated ILIs, while acquiring a slowed-growth and drug-tolerant phenotype (Rodríguez et al., 2014). Too much metabolic degradation of fatty acids can increase the cytoplasmic pool of reducing equivalents leading to a redox imbalance. Thus, the reductive stress is thought to activate conversion of excess free fatty acids to TAG and complex lipids.
Hypoxia and Other Stresses
Both laboratory and clinical strains of Mtb, when exposed to in vitro hypoxic condition were found to accumulate ILIs (Garton et al., 2008). ILIs were observed in bacilli in both NRP (non-replicating persistence) 1 and NRP 2 stages corresponding to 1 and 0.06 % oxygen saturation, respectively. M. bovis BCG accumulates TAG during entry into hypoxia-induced dormancy and enhances its TAG hydrolytic activity up on exit from dormancy, suggesting the significance of TAG for dormancy and reactivation of mycobacteria (Low et al., 2009).
Mtb, when subjected to combined stresses in vitro consisting of low oxygen, high CO2, low nutrient and acidic pH, accumulated TAG and wax ester (Deb et al., 2009). The bacilli also stopped replicating, lost acid-fastness, and showed phenotypic drug tolerance. Oxidative stress by H2O2, and sublethal concentrations of isoniazid in the culture medium induced ILIs in clinical Mtb strains but not in the laboratory strain H37Rv. Too low iron in the medium induced ILIs in clinical strains as well as H37Rv (Vijay et al., 2017). Iron depletion in the medium led to increased abundance of DAG, TAG and wax esters in Mtb (Bacon et al., 2007). Mycobactin containing microvescicles secreted by Mtb grown in low iron medium were enriched in DAG and TAG as well as phosphatidylethanolamines (Prados-Rosales et al., 2014). We have reported decreased release of nile red staining microvesicles from a deletion mutant of Rv3371, a possible tgs, grown in low iron medium (Rastogi et al., 2017). An increased accumulation of DAG and TAG in the mycomembrane could occur prior to budding of microvesicles, which are induced by low iron and other yet unidentified conditions. Actual role of these acylglycerols in the mycomembrane is worth investigating.
Potential factors inducing TAG synthesis, possible pathways leading to TAG formation and the fate of TAG in mycobacteria are summarized in Figure 1.
Implications of Inhibiting TAG Synthesis in Pathogenic Mycobacteria
TAG synthesis is likely important to Mtb in at least three ways: (1) storage of carbon and energy for use during persistence and reactivation (Low et al., 2009; Galagan et al., 2013), (2) reduce the toxic burden of free fatty acids causing the reductive stress (McCarthy, 1971; Weir et al., 1972), and (3) reduce the carbon flux through tricarboxylic acid cycle and thereby arrest growth and acquire antibiotic tolerance (Baek et al., 2011). Hence ideally inhibiting TAG synthesis should interfere with dormancy, persistence, survival in a lipid-rich microenvironment, and antibiotic tolerance. Another unexplored role of TAG for mycobacteria is linked to its presence in the mycomembrane (Ortalo-Magné et al., 1996; Bansal-Mutalik and Nikaido, 2014). Interrupting TAG transport from cytoplasm to mycomembrane led to reduced virulence of the Mtb strain in mouse (Martinot et al., 2016).
Conclusions
Triacylglycerols are associated with the long-term persistence of Mtb. Evidences suggest that they are an important source of energy for the persisting, slow metabolizing population of the bacilli in the host (Low et al., 2009; Shi et al., 2010; Galagan et al., 2013). The presence of TAG in the mycomembrane is intriguing, especially in the absence of ILIs and indicates additional roles for TAG in the pathogen. Although there are multiple genes coding for triacylglycerol synthases in the Mtb genome, only one (tgs1) has been explored so far. It is interesting to note that the members of this family are differentially regulated at the transcription level and also the fact that some of the members are exclusively present in pathogenic mycobacteria. Presence of more than a dozen Tgs, the extreme diversity of TAG species synthesized by them, their differential expression induced by multiple stress conditions and the TAG distribution between cell envelope and cytoplasm urge for investigations on the various members of the Mtb-Tgs family. The proteome of ILIs may be dynamic, changing with the condition inducing ILIs and is likely to include proteins that regulate the TAG content.
Author Contributions
RKM, SB, and MK collected the references, prepared the figure and wrote the manuscript.
Funding
This work was supported by Department of Biotechnology, Government of India Grant-in-Aid project No. BT/PR14876/MED/29/981/2015. This is CDRI communication number 9786.
Conflict of Interest Statement
The authors declare that the research was conducted in the absence of any commercial or financial relationships that could be construed as a potential conflict of interest.
References
Agarwal, P., Khan, S. R., Verma, S. C., Beg, M., Singh, K., Mitra, K., et al. (2014). Mycobacterium tuberculosis persistence in various adipose depots of infected mice and the effect of anti-tubercular therapy. Microbes Infect. 16, 571–580. doi: 10.1016/j.micinf.2014.04.006
Alvarez, H. M., Kalscheuer, R., and Steinbüchel, A. (2000). Accumulation and mobilization of storage lipids by Rhodococcus opacus PD630 and Rhodococcus ruber NCIMB 40126. Appl. Microbiol. Biotechnol. 54, 218–223. doi: 10.1007/s002530000395
Alvarez, H. M., and Steinbüchel, A. (2002). Triacylglycerols in prokaryotic microorganisms. Appl. Microbiol. Biotechnol. 60, 367–376. doi: 10.1007/s00253-002-1135-0
Armstrong, R. M., Adams, K. L., Zilisch, J. E., Bretl, D. J., Sato, H., Anderson, D. M., et al. (2016). Rv2744c is a PspA ortholog that regulates lipid droplet homeostasis and nonreplicating persistence in Mycobacterium tuberculosis. J. Bacteriol. 198, 1645–1661. doi: 10.1128/JB.01001-15
Armstrong, R. M., Carter, D. C., Atkinson, S. N., Terhune, S. S., and Zahrt, T. C. (2018). Association of Mycobacterium Proteins with lipid droplets. J. Bacteriol. 200, e00240-18. doi: 10.1128/JB.00240-18
Bacon, J., Dover, L. G., Hatch, K. A., Zhang, Y., Gomes, J. M., Kendall, S., et al. (2007). Lipid composition and transcriptional response of Mycobacterium tuberculosis grown under iron-limitation in continuous culture: identification of a novel wax ester. Microbiology 153, 1435–1444. doi: 10.1099/mic.0.2006/004317-0
Baek, S. H., Li, A. H., and Sassetti, C. M. (2011). Metabolic regulation of mycobacterial growth and antibiotic sensitivity. PLoS Biol. 9:e1001065. doi: 10.1371/journal.pbio.1001065
Bansal-Mutalik, R., and Nikaido, H. (2014). Mycobacterial outer membrane is a lipid bilayer and the inner membrane is unusually rich in diacyl phosphatidylinositol dimannosides. Proc. Natl. Acad. Sci. U.S.A. 111, 4958–4963. doi: 10.1073/pnas.1403078111
Barisch, C., Paschke, P., Hagedorn, M., Maniak, M., and Soldati, T. (2015). Lipid droplet dynamics at early stages of Mycobacterium marinum infection in Dictyostelium. Cell Microbiol. 17, 1332–1349. doi: 10.1111/cmi.12437
Barisch, C., and Soldati, T. (2017). Mycobacterium marinum Degrades both triacylglycerols and phospholipids from its dictyostelium host to synthesise its own triacylglycerols and generate lipid inclusions. PLoS Pathog. 13:e1006095. doi: 10.1371/journal.ppat.1006095
Berg, J. M., Tymoczko, J. L., and Stryer, L. (2002). Biochemistry, 5th Edn. New York, NY: W H Freeman; Section 22.1, Triacylglycerols Are Highly Concentrated Energy Stores. Available online at: https://www.ncbi.nlm.nih.gov/books/NBK22369/
Betts, J. C., Lukey, P. T., Robb, L. C., McAdam, R. A., and Duncan, K. (2002). Evaluation of a nutrient starvation model of Mycobacterium tuberculosis persistence by gene and protein expression profiling. Mol. Microbiol. 43, 717–731. doi: 10.1046/j.1365-2958.2002.02779.x
Bloch, H., and Segal, W. (1956). Biochemical differentiation of Mycobacterium tuberculosis grown in vivo and in vitro. J. Bacteriol. 72, 132–141.
Bouzid, F., Brégeon, F., Poncin, I., Weber, P., Drancourt, M., and Canaan, S. (2017). Infection of adipose tissues. Front. Cell. Infect. Microbiol. 7:189. doi: 10.3389/fcimb.2017.00189
Brasaemle, D. L., Subramanian, V., Garcia, A., Marcinkiewicz, A., and Rothenberg, A. (2009). Perilipin A and the control of triacylglycerol metabolism. Mol. Cell. Biochem. 326, 15–21. doi: 10.1007/s11010-008-9998-8
Cáceres, N., Tapia, G., Ojanguren, I., Altare, F., Gil, O., Pinto, S., et al. (2009). Evolution of foamy macrophages in the pulmonary granulomas of experimental tuberculosis models. Tuberculosis 89, 175–182. doi: 10.1016/j.tube.2008.11.001
Caire-Brändli, I., Papadopoulos, A., Malaga, W., Marais, D., Canaan, S., Thilo, L., et al. (2014). Reversible lipid accumulation and associated division arrest of Mycobacterium avium in lipoprotein-induced foamy macrophages may resemble key events during latency and reactivation of tuberculosis. Infect. Immun. 82, 476–490. doi: 10.1128/IAI.01196-13
Christensen, H., Garton, N. J., Horobin, R. W., Minnikin, D. E., and Barer, M. R. (1999). Lipid domains of mycobacteria studied with fluorescent molecular probes. Mol. Microbiol. 31, 1561–1572. doi: 10.1046/j.1365-2958.1999.01304.x
Daniel, J., Deb, C., Dubey, V. S., Sirakova, T. D., Abomoelak, B., Morbidoni, H. R., et al. (2004). Induction of a novel class of diacylglycerol acyltransferases and triacylglycerol accumulation in Mycobacterium tuberculosis as it goes into a dormancy-like state in culture. J. Bacteriol. 186, 5017–5030. doi: 10.1128/JB.186.15.5017-5030.2004
Daniel, J., Kapoor, N., Sirakova, T., Sinha, R., and Kolattukudy, P. (2016). The perilipin-like PPE15 protein in Mycobacterium tuberculosis is required for triacylglycerol accumulation under dormancy-inducing conditions. Mol. Microbiol. 101, 784–794. doi: 10.1111/mmi.13422
Daniel, J., Maamar, H., Deb, C., Sirakova, T. D., and Kolattukudy, P. E. (2011). Mycobacterium tuberculosis uses host triacylglycerol to accumulate lipid droplets and acquires a dormancy-like phenotype in lipid-loaded macrophages. PLoS Pathog. 7:e1002093. doi: 10.1371/journal.ppat.1002093
Deb, C., Daniel, J., Sirakova, T. D., Abomoelak, B., Dubey, V. S., and Kolattukudy, P. E. (2006). A novel lipase belonging to the hormone-sensitive lipase family induced under starvation to utilize stored triacylglycerol in Mycobacterium tuberculosis. J. Biol. Chem. 281, 3866–3875. doi: 10.1074/jbc.M505556200
Deb, C., Lee, C. M., Dubey, V. S., Daniel, J., Abomoelak, B., Sirakova, T. D., et al. (2009). A novel in vitro multiple-stress dormancy model for Mycobacterium tuberculosis generates a lipid-loaded, drug-tolerant, dormant pathogen. PLoS ONE 4:e6077. doi: 10.1371/journal.pone.0006077
DeJesus, M. A., Gerrick, E. R., Xu, W., Park, S. W., Long, J. E., Boutte, C. C., et al. (2017). Comprehensive essentiality analysis of the Mycobacterium tuberculosis genome via saturating transposon mutagenesis. MBio 8, e02133-16. doi: 10.1128/mBio.02133-16
Elamin, A. A., Stehr, M., Spallek, R., Rohde, M., and Singh, M. (2011). The Mycobacterium tuberculosis Ag85A is a novel diacylglycerol acyltransferase involved in lipid body formation. Mol. Microbiol. 81, 1577–1592. doi: 10.1111/j.1365-2958.2011.07792.x
Galagan, J. E., Minch, K., Peterson, M., Lyubetskaya, A., Azizi, E., Sweet, L., et al. (2013). The Mycobacterium tuberculosis regulatory network and hypoxia. Nature 499, 178–183. doi: 10.1038/nature12337
Garton, N. J., Christensen, H., Minnikin, D. E., Adegbola, R. A., and Barer, M. R. (2002). Intracellular lipophilic inclusions of mycobacteria in vitro and in sputum. Microbiology 148, 2951–2958. doi: 10.1099/00221287-148-10-2951
Garton, N. J., Waddell, S. J., Sherratt, A. L., Lee, S. M., Smith, R. J., Senner, C., et al. (2008). Cytological and transcript analyses reveal fat and lazy persister-like bacilli in tuberculous sputum. PLoS Med. 5:e75. doi: 10.1371/journal.pmed.0050075
Gold, B., and Nathan, C. (2017). Targeting phenotypically tolerant Mycobacterium tuberculosis. Microbiol. Spectr. 5. doi: 10.1128/microbiolspec.TBTB2-0031-2016
Griffin, J. E., Gawronski, J. D., Dejesus, M. A., Ioerger, T. R., Akerley, B. J., and Sassetti, C. M. (2011). High-resolution phenotypic profiling defines genes essential for mycobacterial growth and cholesterol catabolism. PLoS Pathog. 7:e1002251. doi: 10.1371/journal.ppat.1002251
Hammond, R. J., Baron, V. O., Oravcova, K., Lipworth, S., and Gillespie, S. H. (2015). Phenotypic resistance in mycobacteria: is it because I am old or fat that I resist you? J. Antimicrob Chemother. 70, 2823–2827. doi: 10.1093/jac/dkv178
Knight, M., Braverman, J., Asfaha, K., Gronert, K., and Stanley, S. (2018). Lipid droplet formation in Mycobacterium tuberculosis infected macrophages requires IFN-γ/HIF-1α signaling and supports host defense. PLoS Pathog. 14:e1006874. doi: 10.1371/journal.ppat.1006874
Kremer, L., de Chastellier, C., Dobson, G., Gibson, K. J., Bifani, P., Balor, S., et al. (2005). Identification and structural characterization of an unusual mycobacterial monomeromycolyl-diacylglycerol. Mol. Microbiol. 57, 1113–1126. doi: 10.1111/j.1365-2958.2005.04717.x
Low, K. L., Rao, P. S., Shui, G., Bendt, A. K., Pethe, K., Dick, T., et al. (2009). Triacylglycerol utilization is required for regrowth of in vitro hypoxic nonreplicating Mycobacterium bovis bacillus Calmette-Guerin. J. Bacteriol. 191, 5037–5043. doi: 10.1128/JB.00530-09
Low, K. L., Shui, G., Natter, K., Yeo, W. K., Kohlwein, S. D., Dick, T., et al. (2010). Lipid droplet-associated proteins are involved in the biosynthesis and hydrolysis of triacylglycerol in Mycobacterium bovis bacillus Calmette-Guerin. J. Biol. Chem. 285, 21662–21670. doi: 10.1074/jbc.M110.135731
Martinot, A. J., Farrow, M., Bai, L., Layre, E., Cheng, T. Y., Tsai, J. H., et al. (2016). Mycobacterial metabolic syndrome: LprG and Rv1410 regulate triacylglyceride levels, growth rate and virulence in Mycobacterium tuberculosis. PLoS Pathog. 12:e1005351. doi: 10.1371/journal.ppat.1005351
Mawuenyega, K. G., Forst, C. V., Dobos, K. M., Belisle, J. T., Chen, J., Bradbury, E. M., et al. (2005). Mycobacterium tuberculosis functional network analysis by global subcellular protein profiling. Mol. Biol. Cell. 16, 396–404. doi: 10.1091/mbc.e04-04-0329
McCarthy, C. (1971). Utilization of palmitic acid by Mycobacterium avium. Infect. Immun. 4, 199–204.
Murphy, D. J. (2001). The biogenesis and functions of lipid bodies in animals, plants and microorganisms. Prog. Lipid. Res. 40, 325–438. doi: 10.1016/S0163-7827(01)00013-3
Murphy, D. J., and Vance, J. (1999). Mechanisms of lipid-body formation. Trends Biochem. Sci. 24, 109–115. doi: 10.1016/S0968-0004(98)01349-8
Nahid, P., Dorman, S. E., Alipanah, N., Barry, P. M., Brozek, J. L., Cattamanchi, A., et al. (2016). Official american thoracic society/centers for disease control and prevention/infectious diseases society of america clinical practice guidelines: treatment of drug-susceptible tuberculosis. Clin. Infect. Dis. 63, e147–e195. doi: 10.1093/cid/ciw566
Nakagawa, H., Kashiwabara, Y., and Matsuki, G. (1976). Metabolism of triacylglycerol in Mycobacterium smegmatis. J. Biochem. 80, 923–928. doi: 10.1093/oxfordjournals.jbchem.a131378
Nazarova, E. V., Montague, C. R., La, T., Wilburn, K. M., Sukumar, N., Lee, W., et al. (2017). Rv3723/LucA coordinates fatty acid and cholesterol uptake in. Elife 6:e26969. doi: 10.7554/eLife.26969
Neyrolles, O., Hernández-Pando, R., Pietri-Rouxel, F., Fornès, P., Tailleux, L., Barrios Payán, J. A., et al. (2006). Is adipose tissue a place for Mycobacterium tuberculosis persistence? PLoS ONE 1:e43. doi: 10.1371/journal.pone.0000043
Olukoshi, E. R., and Packter, N. M. (1994). Importance of stored triacylglycerols in Streptomyces: possible carbon source for antibiotics. Microbiology 140 (Pt 4), 931–943. doi: 10.1099/00221287-140-4-931
Ortalo-Magné, A., Lemassu, A., Lanéelle, M. A., Bardou, F., Silve, G., Gounon, P., et al. (1996). Identification of the surface-exposed lipids on the cell envelopes of Mycobacterium tuberculosis and other mycobacterial species. J. Bacteriol. 178, 456–461. doi: 10.1128/jb.178.2.456-461.1996
Peyron, P., Vaubourgeix, J., Poquet, Y., Levillain, F., Botanch, C., Bardou, F., et al. (2008). Foamy macrophages from tuberculous patients' granulomas constitute a nutrient-rich reservoir for M. tuberculosis persistence. PLoS Pathog. 4:e1000204. doi: 10.1371/journal.ppat.1000204
Prados-Rosales, R., Weinrick, B. C., Piqué, D. G., Jacobs, W. R., Casadevall, A., and Rodriguez, G. M. (2014). Role for Mycobacterium tuberculosis membrane vesicles in iron acquisition. J. Bacteriol. 196, 1250–1256. doi: 10.1128/JB.01090-13
Purdy, G. E., Pacheco, S., Turk, J., and Hsu, F. F. (2013). Characterization of mycobacterial triacylglycerols and monomeromycolyl diacylglycerols from Mycobacterium smegmatis biofilm by electrospray ionization multiple-stage and high-resolution mass spectrometry. Anal. Bioanal. Chem. 405, 7415–7426. doi: 10.1007/s00216-013-7179-4
Rastogi, S., Agarwal, P., and Krishnan, M. Y. (2016). Use of an adipocyte model to study the transcriptional adaptation of Mycobacterium tuberculosis to store and degrade host fat. Int. J. Mycobacteriol. 5, 92–98. doi: 10.1016/j.ijmyco.2015.10.003
Rastogi, S., Singh, A. K., Chandra, G., Kushwaha, P., Pant, G., Singh, K., et al. (2017). The diacylglycerol acyltransferase Rv3371 of Mycobacterium tuberculosis is required for growth arrest and involved in stress-induced cell wall alterations. Tuberculosis 104, 8–19. doi: 10.1016/j.tube.2017.02.001
Reed, M. B., Gagneux, S., Deriemer, K., Small, P. M., and Barry, C. E. (2007). The W-Beijing lineage of Mycobacterium tuberculosis overproduces triglycerides and has the DosR dormancy regulon constitutively upregulated. J. Bacteriol. 189, 2583–2589. doi: 10.1128/JB.01670-06
Rengarajan, J., Bloom, B. R., and Rubin, E. J. (2005). Genome-wide requirements for Mycobacterium tuberculosis adaptation and survival in macrophages. Proc. Natl. Acad. Sci. U.S.A. 102, 8327–8332. doi: 10.1073/pnas.0503272102
Rodríguez, J. G., Hernández, A. C., Helguera-Repetto, C., Aguilar Ayala, D., Guadarrama-Medina, R., Anzóla, J. M., et al. (2014). Global adaptation to a lipid environment triggers the dormancy-related phenotype of Mycobacterium tuberculosis. MBio 5, e01125–e01114. doi: 10.1128/mBio.01125-14
Russell, D. G., Cardona, P. J., Kim, M. J., Allain, S., and Altare, F. (2009). Foamy macrophages and the progression of the human tuberculosis granuloma. Nat. Immunol. 10, 943–948. doi: 10.1038/ni.1781
Santucci, P., Diomandé, S., Poncin, I., Alibaud, L., Viljoen, A., Kremer, L., et al. (2018). Delineating the physiological roles of the PE and catalytic domains of LipY in lipid consumption in Mycobacterium-infected foamy macrophages. Infect. Immun. 86:e00394–e00318. doi: 10.1128/IAI.00394-18
Sassetti, C. M., Boyd, D. H., and Rubin, E. J. (2001). Comprehensive identification of conditionally essential genes in mycobacteria. Proc. Natl. Acad. Sci. U.S.A. 98, 12712–12717. doi: 10.1073/pnas.231275498
Sassetti, C. M., Boyd, D. H., and Rubin, E. J. (2003). Genes required for mycobacterial growth defined by high density mutagenesis. Mol. Microbiol. 48, 77–84. doi: 10.1046/j.1365-2958.2003.03425.x
Sassetti, C. M., and Rubin, E. J. (2003). Genetic requirements for mycobacterial survival during infection. Proc. Natl. Acad. Sci. U.S.A. 100, 12989–12994. doi: 10.1073/pnas.2134250100
Shi, L., Sohaskey, C. D., Pheiffer, C., Datta, P., Parks, M., and McFadden, J. (2010). Carbon flux rerouting during Mycobacterium tuberculosis growth arrest. Mol. Microbiol. 78, 1199–1215. doi: 10.1111/j.1365-2958.2010.07399.x
Singh, R., Singh, A., and Tyagi, A. K. (2005). Deciphering the genes involved in pathogenesis of Mycobacterium tuberculosis. Tuberculosis 85, 325–335. doi: 10.1016/j.tube.2005.08.015
Sirakova, T. D., Dubey, V. S., Deb, C., Daniel, J., Korotkova, T. A., Abomoelak, B., et al. (2006). Identification of a diacylglycerol acyltransferase gene involved in accumulation of triacylglycerol in Mycobacterium tuberculosis under stress. Microbiology 152, 2717–2725. doi: 10.1099/mic.0.28993-0
Stöveken, T., Kalscheuer, R., Malkus, U., Reichelt, R., and Steinbüchel, A. (2005). The wax ester synthase/acyl coenzyme A:diacylglycerol acyltransferase from Acinetobacter sp. strain ADP1: characterization of a novel type of acyltransferase. J. Bacteriol. 187, 1369–1376. doi: 10.1128/JB.187.4.1369-1376.2005
Sturley, S. L., and Hussain, M. M. (2012). Lipid droplet formation on opposing sides of the endoplasmic reticulum. J. Lipid. Res. 53, 1800–1810. doi: 10.1194/jlr.R028290
Tauchi-Sato, K., Ozeki, S., Houjou, T., Taguchi, R., and Fujimoto, T. (2002). The surface of lipid droplets is a phospholipid monolayer with a unique Fatty Acid composition. J. Biol. Chem. 277, 44507–44512. doi: 10.1074/jbc.M207712200
Vijay, S., Hai, H. T., Thu, D. D. A., Johnson, E., Pielach, A., Phu, N. H., et al. (2017). Ultrastructural analysis of cell envelope and accumulation of lipid inclusions in clinical. Front. Microbiol. 8:2681. doi: 10.3389/fmicb.2017.02681
Viljoen, A., Blaise, M., de Chastellier, C., and Kremer, L. (2016). MAB_3551c encodes the primary triacylglycerol synthase involved in lipid accumulation in Mycobacterium abscessus. Mol. Microbiol. 102, 611–627. doi: 10.1111/mmi.13482
Wältermann, M., Hinz, A., Robenek, H., Troyer, D., Reichelt, R., Malkus, U., et al. (2005). Mechanism of lipid-body formation in prokaryotes: how bacteria fatten up. Mol. Microbiol. 55, 750–763. doi: 10.1111/j.1365-2958.2004.04441.x
Keywords: Mycobacterium, persistence, lipid inclusions, triacylglycerol, lipid bodies
Citation: Maurya RK, Bharti S and Krishnan MY (2019) Triacylglycerols: Fuelling the Hibernating Mycobacterium tuberculosis. Front. Cell. Infect. Microbiol. 8:450. doi: 10.3389/fcimb.2018.00450
Received: 07 September 2018; Accepted: 18 December 2018;
Published: 09 January 2019.
Edited by:
Stephane Canaan, Center for the National Scientific Research (CNRS), FranceReviewed by:
Evgeniya V. Nazarova, Cornell University, United StatesJaiyanth Daniel, Purdue University Fort Wayne, United States
Copyright © 2019 Maurya, Bharti and Krishnan. This is an open-access article distributed under the terms of the Creative Commons Attribution License (CC BY). The use, distribution or reproduction in other forums is permitted, provided the original author(s) and the copyright owner(s) are credited and that the original publication in this journal is cited, in accordance with accepted academic practice. No use, distribution or reproduction is permitted which does not comply with these terms.
*Correspondence: Manju Y. Krishnan, bWFuanVAY2RyaS5yZXMuaW4=