- 1Department of Biomolecular Systems, Max Planck Institute of Colloids and Interfaces, Potsdam, Germany
- 2Institute for Chemistry and Biochemistry, Freie Universität Berlin, Berlin, Germany
Vaccination is an efficient means of combating infectious disease burden globally. However, routine vaccines for the world's major human parasitic diseases do not yet exist. Vaccines based on carbohydrate antigens are a viable option for parasite vaccine development, given the proven success of carbohydrate vaccines to combat bacterial infections. We will review the key components of carbohydrate vaccines that have remained largely consistent since their inception, and the success of bacterial carbohydrate vaccines. We will then explore the latest developments for both traditional and non-traditional carbohydrate vaccine approaches for three of the world's major protozoan parasitic diseases—malaria, toxoplasmosis, and leishmaniasis. The traditional prophylactic carbohydrate vaccine strategy is being explored for malaria. However, given that parasite disease biology is complex and often arises from host immune responses to parasite antigens, carbohydrate vaccines against deleterious immune responses in host-parasite interactions are also being explored. In particular, the highly abundant glycosylphosphatidylinositol molecules specific for Plasmodium, Toxoplasma, and Leishmania spp. are considered exploitable antigens for this non-traditional vaccine approach. Discussion will revolve around the application of these protozoan carbohydrate antigens for vaccines currently in preclinical development.
Introduction
The first vaccine was the smallpox inoculation introduced in 1796 by Jenner that used whole, attenuated organisms to generate a protective immune response. Our ever-increasing understanding of the underlying immune response that drives vaccination has led to modern subunit vaccines that are formulated to exacting standards. These subunit vaccines use defined protein or carbohydrate antigens implicated in virulence and disease to generate a more directed, nuanced immune response against the offending pathogen.
Over two centuries of vaccinology has seen significant progress in protection from many viral and bacterial diseases affecting humans such as polio, Haemophilus influenza type B, Streptococcus pneumoniae, and Neisseria meningitis, saving millions of lives each year. And yet, it is disheartening to consider that no vaccine exists for human parasitic infections that continue to cause suffering in many parts of the world (Nyame et al., 2004; Astronomo and Burton, 2010; Hoffman et al., 2015).
More than a million people die each year from diseases like malaria and leishmaniasis, with lifelong disability, disfigurement, and suffering for those that are living with disease (Hotez et al., 2014). As it stands, diseases caused by protozoan parasites are a leading cause of death the world over yet vaccine strategies for global parasitic diseases such as malaria (John et al., 2008; Seder et al., 2013; Tinto et al., 2015; Gosling and von Seidlein, 2016; WHO, 2016b) and toxoplasmosis (Jongert et al., 2009) have been pursued for decades. The failure in developing an effective vaccine against human parasite infection lies in part to the complexity of parasite biology compared to other microbes (Astronomo and Burton, 2010; Hoffman et al., 2015). Another failure is our relatively poor understanding of the protein and carbohydrate antigens relevant to parasitic virulence. Given that parasite disease pathology often arises from complex host immune responses to parasite antigens, ongoing research to better understand host-parasite interactions in disease is vital to parasite vaccine development (Schofield and Grau, 2005).
Carbohydrates are considered compelling, exploitable targets for vaccination to overcome the challenges that have prevented the realization of a human parasite vaccine (Nyame et al., 2004; Rodrigues et al., 2015). They are abundantly present on the surface of parasites and play a key role in host-parasite interactions (Rodrigues et al., 2015). Unique carbohydrate antigens characterize multiple developmental stages and tend to be immunoreactive for both protozoan and helminth parasites (Nyame et al., 2004). Adding to the appeal of carbohydrate antigens is the ongoing, systematic characterization of parasite glycobiology regarding their structure, function and biosynthesis (Nyame et al., 2004; Rodrigues et al., 2015). In this review, we will discuss the advantages and disadvantages of modern, carbohydrate antigen-based subunit vaccines, and reflect on the latest developments of carbohydrate vaccines for major protozoan parasites.
Carbohydrate Vaccine Anatomy
Carbohydrates Antigens
Carbohydrates are abundant on the surfaces of all cells and exist as poly- and oligosaccharides attached to proteins and lipids (Horlacher and Seeberger, 2008). They are involved in key biological processes such as cell adhesion, modulatory processes, and structural functions (Varki and Lowe, 2009). For pathogenic microbes, carbohydrate interactions are utilized for attachment (Kline et al., 2009) and invasion (de Groot et al., 2013). In turn, pathogenic microbial carbohydrates can be recognized by host immune systems to induce the production of carbohydrate-specific antibodies that can serve protective functions (Astronomo and Burton, 2010). Carbohydrates have been exploited for protective vaccination for decades given their crucial roles in development, growth, and disease (Varki and Lowe, 2009).
Carbohydrate biosynthesis is not directly template-driven as is the case for nucleic acid and proteins (Rodrigues et al., 2015). Instead, their biosynthesis is a complex, multi-enzymatic process (Delorenzi et al., 2002) forming linear and branched molecules with varied linkages. The result is a class of biopolymers of great complexity and diversity. Their heterogeneity means that access to pure, defined carbohydrates remains a challenge (Liu et al., 2006; Adibekian et al., 2011; Geissner and Seeberger, 2016). Microbial cell culture can be a readily available biological source of carbohydrate antigens, however carbohydrate isolation can be complex and even tedious (Geissner and Seeberger, 2016). Furthermore, isolation is not so straightforward for carbohydrates of low abundance or when microbial culture is not possible. This is especially true for parasites, which generally require more complex culturing conditions compared to bacteria. Fortunately, carbohydrate synthesis technology continues to advance and is increasingly becoming an alternative to biological isolation to source carbohydrates (Anish et al., 2014). Moreover, carbohydrate synthesis allows for pure, completely defined carbohydrate antigens as a basis for synthetic carbohydrate vaccines.
Carrier Proteins
Virtually all vaccines rely on antibody production and subsequent immunological memory against the target antigen for their protective effect (Zinkernagel, 2003). Accordingly, the immune response against the antigen is a key consideration when it comes to vaccine design where strong, long lasting immune responses are desired. In this regard, proteins and carbohydrates are generally regarded as thymus dependent or thymus independent antigens, respectively, which characterizes the type of antibody immune response they elicit.
Thymus Dependent and Thymus Independent Antigens
Thymus dependent (TD) antigens, such as proteins, are taken up by antigen presenting cells (APCs). The endocytosed antigen is processed through a series of catalytic steps which liberate small peptide fragments. These peptide fragments form complexes with MHCII molecules and thereby are able to be displayed on the MHCII molecules of the APC. T cells specific to the displayed MHCII-peptide complex are co-stimulated by the APC (Avci and Kasper, 2010). The activated T cells go on to “help” the antigen-specific B cells, promoting their proliferation, affinity maturation, antibody isotype switching and long lasting immunological memory (Pulendran and Ahmed, 2011).
Carbohydrate antigens are classed as thymus independent (TI) antigens and are poorly immunogenic compared to TD antigens (Weintraub, 2003). Carbohydrate antigens are recognized by APCs through pathogen recognition receptors (Blander and Sander, 2012), endocytosed, and processed into oligosaccharide epitopes. These oligosaccharides are not presented on MHCII molecules, but instead are presented directly on the APC cell surface to activate carbohydrate antigen-specific B cells. Due to the lack of T cell activation, B cells are activated in the absence of affinity maturation and isotype switching (Mond et al., 1995) which leads to the predominant production of low affinity IgM antibodies, and only low levels of IgG (Mond and Kokai-Kun, 2008; Astronomo and Burton, 2010; Berti and Adamo, 2013). The IgM antibodies bind in the micromolar range, compared to TD antigen-derived IgG antibodies that bind in the nanomolar range (Broecker et al., 2016; Geissner et al., 2016). B and T cell memory is often not achieved and the immune response is short lived (Adams et al., 2008; Mond and Kokai-Kun, 2008; Hütter and Lepenies, 2015). Moreover, children less than 2 years of age fail to mount an antibody immune response to TI antigens (Weintraub, 2003; Landers et al., 2005).
Glycoconjugates
In the 1920s and 1930s, covalent conjugation of carbohydrate antigens to protein scaffolds was first explored to investigate the interaction between the TD antigen properties of proteins with TI carbohydrate antigens (Avery, 1931). This early work formed the basis of the first glycoconjugate carbohydrate vaccines produced in the 1980s (Schneerson et al., 1980; Beuvery et al., 1982; Wessels et al., 1993), where bacterial capsular polysaccharides (CPS) were covalently linked to so-called carrier proteins. Immunization with these CPS-protein glycoconjugates enabled T cell-mediated B cell activation against the target carbohydrate antigen, and induced long term immune memory even in infants (Stein, 1992).
The mechanism whereby TI carbohydrate antigens can activate the immune system like TD protein antigens via glycoconjugation remains an open area of research. A long-standing mechanistic explanation argues that the carbohydrate-protein conjugate is recognized by carbohydrate-specific B cells. The protein component of the conjugate is subsequently endocytosed, processed and presented on MHCII molecules of the carbohydrate specific B cell. Through the MHCII-peptide complex a peptide-specific T cell can activate the carbohydrate-specific B cell, resulting in TD immune responses against the desired carbohydrate antigen (Figure 1; Lucas et al., 2005). A more recently proposed second mechanism argues that after uptake by carbohydrate-specific B cells the glycoconjugate is processed into glycopeptide fragments. Thus, the peptide portion of the fragment can form an MHCII-peptide complex, enabling the simultaneous presentation of the hydrophilic carbohydrate portion to carbohydrate-specific T cells. The T cell interaction activates the presenting B cell accordingly (Avci et al., 2011).
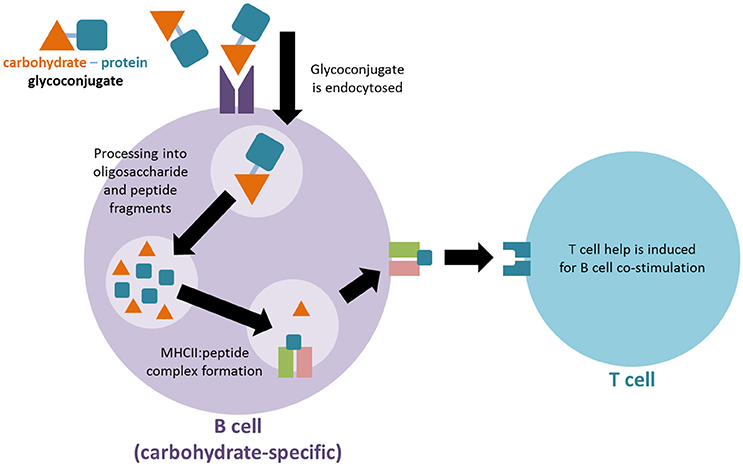
Figure 1. Proposed mechanism for immune activation of T cells by glycoconjugate vaccines. The carbohydrate-protein conjugate is recognized by carbohydrate-specific B cells. The protein component of the conjugate is subsequently endocytosed, processed and presented on MHCII molecules of the carbohydrate specific B cell in order to activate a T cell for co-stimulation.
Five TD carrier proteins are currently used in licensed carbohydrate vaccines (Pichichero, 2013). Of these licensed carrier proteins, a non-toxic mutant of diphtheria toxin (CRM197) is often used in vaccine development research for parasitic diseases. Another carrier protein often used in parasite vaccine development is keyhole limpet haemocyanin (KLH). Unlike many other carrier proteins KLH is itself highly glycosylated and is a good promotor of TD and TI responses (Nyame et al., 2004), however it is not licensed for human use.
The linker conjugating the carrier protein to the carbohydrate antigen is another key consideration of carbohydrate vaccines. Synthetic carbohydrates with defined carrier protein binding sites are advantageous for generating well-defined glycoconjugates with predictable conjugation sites. To minimize the immunogenicity of the linker (Buskas et al., 2004; Gotze et al., 2015) short linkers with no functional groups are best used to ensure that immune responses against the desired antigen epitope are not detrimentally affected. The processing of the linker after uptake by APCs is also an important consideration, to allow for the release of vaccine oligosaccharide and peptide moieties for antigenic display.
Adjuvants
Adjuvants are substances that modulate or bolster an effective immune response against the antigens in the vaccine. Formulations are typically emulsions and vesicles that can serve as a delivery vehicle for antigen vaccine components and allow for the slow release of vaccine antigen components over time. Adjuvants can assist antigen immunogenicity by increasing local inflammation and antigen uptake by APCs, and aid in their migration to lymph nodes (Petrovsky and Aguilar, 2004; Di Pasquale et al., 2015). Ideally, adjuvants reduce the amount of antigen or number of immunizations needed for vaccination (Petrovsky and Aguilar, 2004).
Aluminum-based mineral (Alum) salts served as the only adjuvants for human vaccines for decades (Zimmermann and Lepenies, 2015) and are used for a wide range of vaccines that aim to predominantly induce antibody-mediated immune responses (Bhowmick et al., 2014). Despite its worldwide use, research on its mechanism of action is ongoing (De Gregorio et al., 2008). Today, alum, virosomes, lipid A derived adjuvants, and squalene adjuvants are all in use for human licensed vaccines (Astronomo and Burton, 2010; Di Pasquale et al., 2015; Zimmermann and Lepenies, 2015) and are commonly used in vaccine research. Crude Freund's adjuvant (CFA) is a powerful immunogen often used as an adjuvant for candidate parasite vaccines, but it is not approved for licensed human vaccines.
Modern vaccines that use better defined, or even synthetic antigens, are generally less immunogenic than crude, whole organism vaccines. Research is ongoing in advancing the efficacy of adjuvant systems for vaccines (Petrovsky and Aguilar, 2004). Many of these new adjuvant systems that are currently being tested in bacterial vaccines are of great interest for adaption to candidate vaccines of parasite diseases. For example, an adjuvant used in animal models, α-GalCer, was covalently attached to S. pneumoniae CPS to act as both carrier and adjuvant for the carbohydrate antigen (Cavallari et al., 2014). Endosomal processing by carbohydrate-specific B cells displays α-GalCer antigens via CD1d, stimulating iNKT cells (a class of T cells) to promote B cell hypermutation, class-switching and immunological memory (Cavallari and De Libero, 2017). Zwitterionic polysaccharides (ZPSs) are another emerging class of potentially self-adjuvanting carrier isolated from commensal anaerobic bacteria. ZPSs have the special property of containing one positive and one negative charge on adjacent monosaccharides. When they are processed by APCs this crucially enables their presentation on MHCII complexes, leading to T cell activation. Thus, they are able to activate adaptive immune responses for conjugated carbohydrate antigens in the absence of carrier protein (Berti and Adamo, 2013), leading to the possibility of fully-carbohydrate vaccines (Nishat and Andreana, 2016).
The Success of Carbohydrate Vaccines
The concept of carbohydrate antigen vaccines started in the 1920s with the first published evidence that “residue antigens” of Streptococcus pneumoniae were the CPS of the bacterium (Heidelberger and Avery, 1923, 1924), later demonstrated to be important for virulence and serotype specificity (Hütter and Lepenies, 2015). The CPS of S. pneumoniae was shown to produce CPS-specific antibodies (Tillett and Francis, 1929) that protected against the disease symptoms of S. pneumoniae (Hütter and Lepenies, 2015), leading to the first CPS antigen vaccine against S. pneumoniae in 1947 (Grabenstein and Klugman, 2012). The advent of antibiotics at around the same time somewhat stalled vaccine research, as antibiotics became the preferred method for bacterial disease prevention (Grabenstein and Klugman, 2012; Hütter and Lepenies, 2015). The rise of antibiotic resistance in the following decades (Davies and Davies, 2010) led to a resurgence in carbohydrate vaccine research (Vliegenthart, 2006).
In the 1970s and 80s, CPS-based vaccines for S. pneumoniae were licensed and approved in the USA and Europe. Increasing numbers of strain-specific CPS were added to further itinerations of the vaccine to increase its efficacy, culminating into a 23-valent CPS antigen vaccine first licensed in 1983, protecting vaccinated adults against 87% of S. pneumoniae disease in the USA (Grabenstein and Klugman, 2012; Cavallari and De Libero, 2017). Glyconjugate carbohydrate vaccines were later introduced from the 1990s onward to allow for vaccination of broader demographics, especially infants. Glycoconjugate carbohydrate vaccines against disease caused by H. influenzae type b infection lead to its virtual elimination within countries with widespread coverage (Lindberg, 1999). Similarly, the use of glycoconjugate vaccines against Neisseria meningitides has also met with effective results (Girard et al., 2006). Vaccines for S. pneumoniae are also now glycoconjugates. Several other glycoconjugate vaccines are currently under development, such as a carbohydrate vaccine for group B streptococcus (De Gregorio and Rappuoli, 2014; Lepenies, 2015). Today, glycoconjugate vaccines have substituted pure carbohydrate vaccines where possible, with the most common carrier proteins being CRM197 and tetanus toxin (Cavallari and De Libero, 2017).
Exploiting Carbohydrate Antigens for Protozoan Parasite Vaccines
The key components of CPS carbohydrate vaccines—antigen, carrier protein, linker, and adjuvant—have remained largely consistent since their inception. Current parasite vaccine research follows the same component model in this regard. However, as illustrated in the following sections, a recurring theme for carbohydrate parasite vaccines is the need to steer away from traditional prophylactic vaccine development strategies that were successfully applied to bacterial infections.
In addition to carbohydrate vaccines that can induce sterile protection against the parasite itself, vaccinating against deleterious immune responses in host-parasite interactions is another strategy. Much of the pathology of parasitic disease is due to the host's own immune responses against the parasite. For example, the deadly manifestation of severe malaria is a result of the host's own immune response against parasite-derived molecules, causing the toxic, hyperinflammatory response associated with severe malaria (Boutlis et al., 2002; Krishnegowda et al., 2005; Patel et al., 2007). The Plasmodium falciparum-specific glycoform of glycosylphosphatidylinositol (GPI) was identified as the putative toxin implicated in disease (Schofield and Hackett, 1993). GPI molecules are present on the surface of virtually all eukaryotic cells and serve as surface protein anchors, but parasite-specific GPIs occur at relatively high levels in parasitic protozoa (Gowda, 2002). Vaccination strategies aimed at neutralizing the effect of this malaria toxin are being pursued (Schofield, 2007). Similar carbohydrate vaccine strategies for other protozoan parasites are also employed (Buxbaum, 2013). Anti-toxin vaccines have proven successful for other microbial infections, such as the diphtheria toxoid vaccine (Playfair et al., 1990; Schofield, 2007).
We will explore the latest developments for both traditional and non-traditional carbohydrate vaccine approaches for three of the world's major protozoan parasitic diseases—malaria, toxoplasmosis, and leishmaniasis.
Plasmodium
Malaria is an intra-erythrocytic parasitic disease caused by Plasmodium protozoan species. It is among the most devastating infectious diseases of human history and over 3.3 billion people across 97 countries are at risk of infection today (WHO, 2013b, 2014). An estimated 198 million new clinical cases of malaria occur globally with over 80% of new clinical cases occur in sub-saharan Africa alone (WHO, 2013b). Severe malaria disease develops in 5% of P. falciparum infections and accounts for 98% of all malaria-related deaths. There are an estimated 584,000 deaths per year attributed to severe disease, mostly young children under the age of 5 (WHO, 2013b). Estimates of severe disease-related deaths are as high as 1.2 million (Murray et al., 2012). Without a vaccine, at least €2.4 billion are spent annually on malaria control programs using bed nets, insecticides and drug treatments (WHO, 2013b).
A malaria vaccine should be possible considering that naturally acquired immunity to disease symptoms develops over time. Vaccines against malaria aim to reduce morbidity and mortality, and should be effective in protecting against severe malaria. In the long term, the vaccine should also protect against all clinical disease (WHO, 2013a). To this end, many stages of the parasite lifecycle are targeted by vaccines that decrease parasite load. Examples of some prophylactic vaccines include the whole P. falciparum sporozoite vaccine currently in field trials (Seder et al., 2013), and the protein antigen based RTS,S vaccine which is the most advanced example of malaria vaccine currently under development in Phase III (John et al., 2008; Tinto et al., 2015; Gosling and von Seidlein, 2016) and Phase IV trials (WHO, 2016b). At present, the RTS,S vaccine has not been licensed for use as a malaria vaccine (WHO, 2016a).
Anti-Toxin Vaccine
Severe malaria pathology is largely considered to arise from toxic effects of P. falciparum GPI (PfGPI), which exists either as protein free glycolipids, or as the major carbohydrate modifications for proteins essential for erythrocyte invasion (Gowda et al., 1997; Schofield and Grau, 2005). PfGPI-specific antibodies are found in adults of malaria-endemic areas, and may be inhibiting the ability of PfGPI to induce the hyper-inflammatory response associated with severe malaria. This is still up for debate, since studies that find an association between PfGPI-specific antibody titer and protection from severe malaria (Brasseur et al., 1990; Naik et al., 2000; Gowda, 2002; Keenihan et al., 2003; Perraut et al., 2005) are balanced by studies that find no such association (de Souza et al., 2002; Boutlis et al., 2005; Cissoko et al., 2006; Gomes et al., 2013; Mbengue et al., 2016). However, PfGPI-specific antibodies are reported to show relevant action in modulating immune responses by protecting immune cells against severe P. falciparum-induced inflammatory responses in-vitro (Schofield et al., 1993; de Souza et al., 2010).
To evaluate the effect of using PfGPI in an anti-toxin vaccine, a PfGPI hexasaccharide was synthesized, conjugated to KLH carrier protein and emulsified in CFA (Figure 2). C57BL/6 mice were immunized with the glycoconjugate, and then challenged with P. berghei ANKA in a mouse model of malaria. Immunization resulted in significant protection against severe malaria, with clearly reduced death rates at 75% survival. The immunized mice were also protected from acidosis, pulmonary oedema, cerebral syndrome and fatality characteristic of the disease model. Apparently, the induction of protective, PfGPI-specific antibodies ameliorated a hyper-inflammatory response against the PfGPI toxin, specifically shown to neutralize the parasite-induced production of TNF-α by macrophages in vitro. Furthermore, vaccination did not change parasitaemia levels, demonstrating that the effect of the carbohydrate vaccine candidate was through the neutralization of GPI's toxic effects, rather than interfering with parasite replication (Schofield et al., 2002).
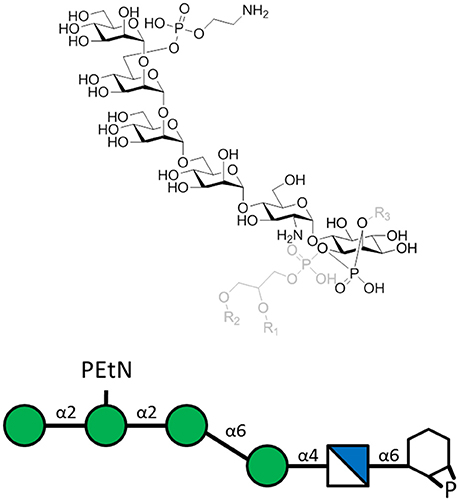
Figure 2. The P. falciparum GPI hexasaccharide of the sequence α-Man-(1-2)-α-[PEtN-6]Man-(1-2)-α-Man(1-6)-α-Man-(1-4)-α-GlcN-(1-6)-myo-Ino-1,2-cyclic-phosphate that was chemically synthesized, conjugated to KLH, and used for immunization. The carrier protein was attached to the top PEtN moiety, where PEtN is an abbreviation for phosphoethanolamine. The full PfGPI (in gray) is shown for context, where R groups denote lipid moieties.
Following this work, synthetic PfGPI were utilized as biomarkers to improve our understanding of the PfGPI-specific antibody response (Kamena et al., 2008; Tamborrini et al., 2010). The synthesis of PfGPI molecules continues to be explored (Gurale et al., 2016).
Sterile Immunity Vaccine
In healthy adults, up to 1–5% of circulating IgG and IgM are specific to the carbohydrate known as α-Gal (Figure 3; Macher and Galili, 2008). In contrast to other mammals, humans do not express α-Gal (Galili and Swanson, 1991) allowing for immune reactivity to this carbohydrate (Galili et al., 1984). Exposure to microbiota expressing this glycan drives production of α-Gal-specific antibodies (Macher and Galili, 2008) which are believed to contribute to immune resistance against α-Gal-expressing pathogenic microbes (Bishop and Gagneux, 2007; Cywes-Bentley et al., 2013). P. falciparum and other animal model Plasmodium spp. express the α-Gal carbohydrate (Galili et al., 1998) possibly bound to GPI-anchored surface proteins (Yilmaz et al., 2014). Early studies already uncovered an association between α-Gal-specific IgM and protection from P. falciparum infection in humans. Thus, the effect of immunization on the production of α-Gal-specific antibodies was investigated (Yilmaz et al., 2014).
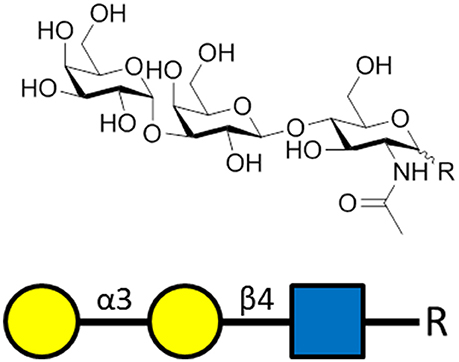
Figure 3. The α-gal carbohydrate epitope of the sequence α-Gal-(1-3)-β-Gal-(1-4)-GlcNAc-R that was used as the antigen for generating α-gal-specific antibodies.
Genetically modified mice unable to express α-Gal (Yang et al., 1998) were immunized for the production of α-Gal IgG and IgM. The immunizations were either inoculation with α-Gal expressing E. coli bacteria, α-Gal rich rabbit RBCs, or synthetic α-Gal conjugated to BSA. Adjuvants used were CFA and toll-like receptor 9 agonists that enhanced the immunogenicity. The mice were then challenged with P. berghei ANKA infection by Anopheles mosquito bites. It was found that α-Gal immunization reduced the risk of parasite transmission, thereby providing sterile immunity. This sterile immunity appears to be due to the cytotoxic action of α-Gal specific IgM and subclasses of IgG against the inoculating parasites. Moreover, the antibodies were shown to inhibit hepatocyte transmigration of the parasite (Yilmaz et al., 2014).
Sterile immunity against malaria infection through the induction of α-Gal-specific antibodies is not similarly present for people living in malaria endemic regions, possibly due to low levels of naturally acquired, protective α-gal-specific antibodies. Promoting a T cell-dependent immune response against the α-Gal carbohydrate was shown to enhance the protective effect of these antibodies in the mouse model (Yilmaz et al., 2014). Moreover, naturally acquired α-gal-specific antibodies may enhance the immunogenicity of antigens enriched with α-gal epitopes by augmenting the T cell response following immunization. This suggests that coupling the α-Gal carbohydrate to existing malaria vaccine candidates could enhance their immunogenicity (Benatuil et al., 2005; Yilmaz et al., 2014). The effect of α-Gal carbohydrate vaccination against other protozoan parasites expressing α-Gal, such as Trypanosoma spp. and Leishmania spp., may also be considered (Yilmaz et al., 2014).
Toxoplasma
Toxoplasma gondii is found worldwide, capable of infecting nucleated cells of many warm-blooded animals (McLeod et al., 2009; Debierre-Grockiego and Schwarz, 2010) and is estimated to infect half of the world's population (Liu et al., 2012). The disease burden for humans has been well-documented (McLeod et al., 2009). Transmission to humans is either through consumption of food contaminated with tissue cysts and meat products from infected animals or by ingestion of oocysts released in the feces of infected cats (Kijlstra and Jongert, 2008).
Immunocompetant individuals tolerate T. gondii infection which is either asymptomatic or manifest with mild flu-like symptoms. However, the formation of parasite-containing tissue cysts prevents the clearance of the parasite after infection (Montoya and Liesenfeld, 2004). When latent carriers of T. gondii are later immunocompromized they are at risk of severe inflammatory reactions in the brain and central nervous system after liberation of the parasites from these cysts (Luft and Remington, 1992). Pregnant women encountering their first infection can also transmit the parasite to the unborn child leading to retardation or abortion (Remington et al., 2004).
Early vaccination strategies using live parasites (Cutchins and Warren, 1956) and fixed parasites (Krahenbuhl et al., 1972) protected from subsequent challenge. Today, vaccines against toxoplasmosis aim to limit acute parasitemia, protect against congenital toxoplasmosis, reduce the number of tissue cysts, or lessen parasite transmission (Kur et al., 2009). To date, sterile immunity against T. gondii has not been achieved (Jongert et al., 2009). A live vaccine for veterinary toxoplasmosis exists to give limited protection during pregnancy (Buxton and Innes, 1995) but is not approved for human use and does not fully eliminate the parasite (Liu et al., 2012).
GPI Vaccine
Glycolipid GPI anchors of T. gondii (TgGPI) have been considered as possible vaccine antigens due to their effect in modulating inflammatory TNF-α responses against the parasite (Debierre-Grockiego, 2010). In 2015, TgGPI were explored as possible vaccine candidates for the first time.
GPI anchors are highly abundant on T. gondii (~106 copies per cell) (Tsai et al., 2012) and exist as a protein-attached or protein-free glycoform (Figure 4). Both glycoforms induce inflammatory reactions like macrophage TNF-α production (Debierre-Grockiego et al., 2003) through TLR-2 and TLR-4 signaling (Debierre-Grockiego et al., 2007), which exacerbates toxoplasmosis in mice (Hunter et al., 1996). A vaccine that induces TgGPI-specific antibodies could ameliorate TgGPI-mediated inflammatory effects and lower disease burden (Debierre-Grockiego, 2010).
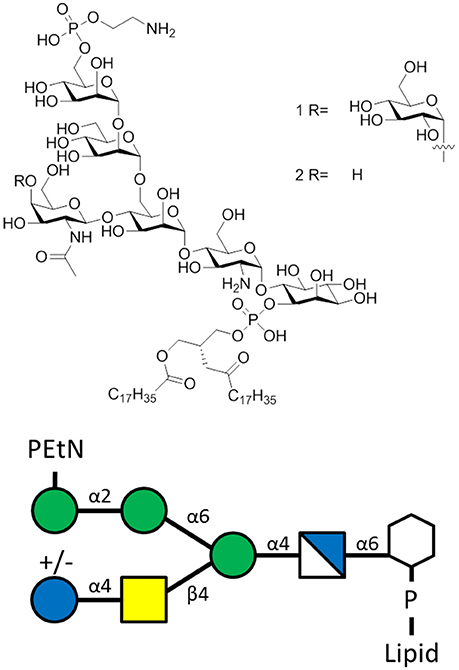
Figure 4. The T. gondii GPI of the sequence α-Man-(1-2)-α-Man-(1-6)-α-Man-(1-4)-α-GlcN-(1-6)-myo-Ino with lipid moiety. Glycoform 1R bears a α-Glc(1-4)-β-GalNAc side chain, while glycoform 2R lacks the glucose moiety.
The two major TgGPI glycoforms were synthethized and covalently conjugated to CRM197. BALB/c mice were then immunized with either one of the glycoconjugates before challenge with virulent T. gondii RH strain. Immunization did not provide protection for mice in the lethal challenge model. Furthermore, the induced antibodies failed to exhibit any significant effect on the inflammatory response for either group (Gotze et al., 2015). Analysis of the immune response indicates that antibody induction was directed away from the desired carbohydrate side branch of the TgGPI, and more toward the linker used to attach the carbohydrate to the carrier protein. In a potential next step, the formulation has to be adjusted (Gotze et al., 2015).
Ongoing research utilizing synthetic TgGPI molecules has helped to identify biomarkers of acute and latent infection. During acute infection, high levels of TgGPI-specific IgM and IgG are present, while latent infection shows a reduced IgM response (Gotze et al., 2014).
Leishmania
There are two million new cases of leishmaniasis every year as the disease is increasingly becoming a worldwide health burden (Desjeux, 2004). The vector borne, facultative intracellular parasite (Chappuis et al., 2007) enters mononuclear, phagocytotic cells such as macrophages. Cutaneous leishmaniasis is the most common form of disease, notable for skin ulcers that result in disability and scarring for the infected patient (Seeberger, 2007; WHO, 2017). Leishmania donovani causes visceral leishmaniasis and is the most severe form of disease characterized by fever, substantial weight loss, anemia, swelling of liver and spleen, and possible death. It is believed to be second only to malaria in terms of fatal infection (Seeberger, 2007; Aebischer, 2014). Patients are treated with antimony drugs which are costly, toxic and increasingly ineffective against resistant parasite strains. A vaccine against leishmaniasis is a desirable, economic strategy to combat this disease (Lee et al., 2012; Singh et al., 2016).
A cocktail of heat-killed Leishmania parasites is a clinically tested vaccine. However, the efficacy of the vaccine to prevent disease is not confirmed (Armijos et al., 2004; Velez et al., 2005). Other attempts involving killed or attenuated parasites for leishmanial vaccine development have not resulted in a licensed vaccine (Topuzogullari et al., 2013).
Vaccine Efforts
Leishmania parasites express lipophosphoglycans (LPG) on their cell surface. This molecule is composed of a GPI anchor, a repeating phosphorylated disaccharide fragment, and variable cap oligosaccharides (Seeberger, 2007). The LPGs are important for survival and virulence of the parasite (Spath et al., 2000) and vaccination preparations with purified LPG is protective against cutaneous leishmaniasis (McConville et al., 1987; Russell and Alexander, 1988; Moll et al., 1989). Regarding the variable capping oligosaccharide, a unique capping tetrasaccharide (Figure 5) was identified as vital for parasite invasion of macrophages (Descoteaux and Turco, 2002) and was the key component of a synthetic carbohydrate vaccine for leishmaniasis (Liu et al., 2006).
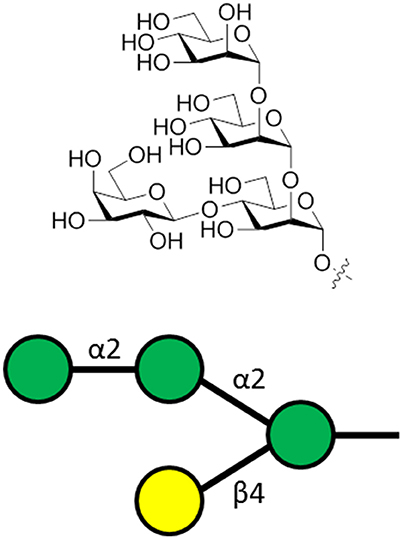
Figure 5. The general structure of the capping tetrasaccharide α-Man-(1-2)-α-Man-(1-2)-[β-Gal-(1-4)]-α-Man of Leishmania LPG. This oligosaccharide is attached to phosphoglycan repeating units, followed by a GPI anchor.
The capping oligosaccharide moiety of LPG was synthesized in initial immunological studies exploiting this antigen for vaccination. The carbohydrate was loaded onto virosomes that served as an integrated carrier and adjuvant, before being used to immunize BALB/c mice. Oligosaccharide-specific IgM and IgG1 responses were produced, indicating that the immune response to the carbohydrate antigen was T cell-dependent. Furthermore, the antibodies against the synthetic carbohydrate were cross-reactive with natural carbohydrate antigens of Leishmania parasites indicating its possible utility as a vaccine antigen (Liu et al., 2006). A further study immunized BALB/c mice with synthetic LPG capping oligosaccharides conjugated to CRM197 and emulsified in CFA. This vaccine candidate produced IgG antibodies specific for the parasite. The oligosaccharides were used to evaluate immune responses of infected humans and dogs as the basis for a diagnostic test (Anish et al., 2013). Given the carbohydrate vaccines currently in production, animal model challenge studies to test the protective effect of these synthetic molecules is the next logical step.
LPG vaccination to protect against cutaneous leishmaniasis employed animal challenge studies that evaluated the LPG component of whole L. amazonensis antigen (LaAg). It was known that intramuscular, systemic immunization of LaAg results in deleterious disease outcomes after challenge, however LPG depletion rendered LaAg protective against Leishmania infection with respect to lesion growth and parasite load compared to non-depleted LaAg. This indicated that LPG was the component responsible for deleterious effects of LaAg inoculation. During further investigations, mice were intranasally vaccinated with LPG alone to determine whether intranasal vaccination of the disease-promoting component could promote protection against cutaneous leishmaniasis (Pinheiro et al., 2007). Intranasal vaccination with LPG was protective, and provided further evidence for the potential of utilizing LPG in a carbohydrate antigen vaccine that would protect against cutaneous leishmaniasis.
Recently, the role of L. mexicana protein-free GPI molecules (GIPL) in disease has been elucidated, shedding more light on immunologic pathways affecting glycolipid-specific antibody responses (Figure 6). After it was determined that L. mexicana infection induces GIPL-specific IgG1 responses, a monoclonal antibody against GIPL was shown to bind to the surface of parasites, and promote IL-10 production in macrophages co-cultured with parasite. Production of IL-10 is deleterious as it blocks an effective immune response that is needed to kill parasites and resolve skin lesions in cutaneous leishmaniasis. In humans, GIPL-specific antibodies are produced in response to infection with cutaneous leishmaniasis. Opsonization of parasites with these antibodies was shown to promote deleterious IL-10 production in macrophages. The role of GIPL-specific antibodies in both mouse and humans opens the possibility of generating a carbohydrate vaccine that can induce competing, non-pathogenic antibody isotypes that can protect against L. mexicana infection (Buxbaum, 2013).
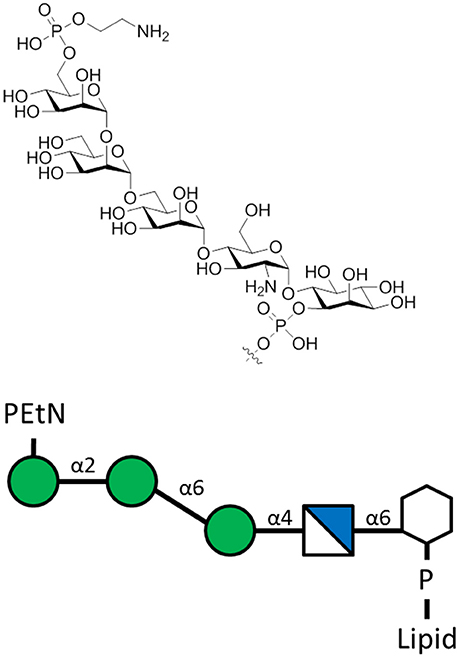
Figure 6. The L. mexicana GIPLs contain the sequence PEtN-6-α-Man-(1-2)-α-Man-(1-6)-α-Man-(1-4)-α-GlcN-(1-6)-myo-Ino-2-phosphate attached to a lipid base that anchors into the membrane (McConville et al., 1993). PEtN is an abbreviation for phosphoethanolamine. Additions to this base sequence are found for many of the GIPLs. Note the structure of the carbohydrate chain is the same as the sequence of protein-free P. falciparum GPI anchors (Assis et al., 2012).
Concluding Remarks
Carbohydrate vaccines represent a promising application of glycobiology to human health. The immense successes of bacterial CPS vaccines should, in theory, be similarly achievable with parasite carbohydrate vaccines. With continued advances in parasite culture handling, and carbohydrate synthesis technologies, it is a great time to apply the development strategies employed for bacterial CPS vaccine research to parasite carbohydrate vaccine research.
Author Contributions
JJ contributed to the drafting of the article. PS and JJ contributed to all revisions of the article.
Funding
The authors thank the Max-Planck Society for generous financial support. JJ is funded by DFG Research Training Group Parasite Infections (GRK 2046).
Conflict of Interest Statement
The authors declare that the research was conducted in the absence of any commercial or financial relationships that could be construed as a potential conflict of interest.
Acknowledgments
The authors gratefully acknowledge Paulina Kaplonek for critically editing the manuscript.
References
Adams, E. W., Ratner, D. M., Seeberger, P. H., and Hacohen, N. (2008). Carbohydrate-mediated targeting of antigen to dendritic cells leads to enhanced presentation of antigen to T cells. Chembiochem 9, 294–303. doi: 10.1002/cbic.200700310
Adibekian, A., Stallforth, P., Hecht, M. L., Werz, D. B., Gagneux, P., and Seeberger, P. H. (2011). Comparative bioinformatics analysis of the mammalian and bacterial glycomes. Chem. Sci. 2, 337–344. doi: 10.1039/C0SC00322K
Aebischer, T. (2014). Leishmania spp. proteome data sets: a comprehensive resource for vaccine development to target visceral leishmaniasis. Front. Immun. 5:260. doi: 10.3389/fimmu.2014.00260
Anish, C., Martin, C. E., Wahlbrink, A., Bogdan, C., Ntais, P., Antoniou, M., et al. (2013). Immunogenicity and diagnostic potential of synthetic antigenic cell surface glycans of leishmania. Acs Chem. Biol. 8, 2412–2422. doi: 10.1021/cb400602k
Anish, C., Schumann, B., Pereira, C. L., and Seeberger, P. H. (2014). Chemical biology approaches to designing defined carbohydrate vaccines. Chem. Biol. 21, 38–50. doi: 10.1016/j.chembiol.2014.01.002
Armijos, R. X., Weigel, M. M., Calvopina, M., Hidalgo, A., Cevallos, W., and Correa, J. (2004). Safety, immunogenecity, and efficacy of an autoclaved Leishmania amazonensis vaccine plus BCG adjuvant against new world cutaneous leishmaniasis. Vaccine 22, 1320–1326. doi: 10.1016/j.vaccine.2003.06.002
Assis, R. R., Ibraim, I. C., Noronha, F. S., Turco, S. J., and Soares, R. P. (2012). Glycoinositolphospholipids from Leishmania braziliensis and L. infantum: modulation of innate immune system and variations in carbohydrate structure. PLoS Negl. Trop. Dis. 6:e1543. doi: 10.1371/journal.pntd.0001543
Astronomo, R. D., and Burton, D. R. (2010). Carbohydrate vaccines: developing sweet solutions to sticky situations? Nat Rev. Drug Discov 9, 308–324. doi: 10.1038/nrd3012
Avci, F. Y., and Kasper, D. L. (2010). How bacterial carbohydrates influence the adaptive immune system. Annu. Rev. Immun. 28, 107–130. doi: 10.1146/annurev-immunol-030409-101159
Avci, F. Y., Li, X. M., Tsuji, M., and Kasper, D. L. (2011). A mechanism for glycoconjugate vaccine activation of the adaptive immune system and its implications for vaccine design. Nat. Med. 17, 1602–1609. doi: 10.1038/nm.2535
Avery, O. T. (1931). Chemo-immunological studies on conjugated carbohydrate-proteins: V. the immunological specifity of an antigen prepared by combining the capsular polysaccharide of type III pneumococcus with foreign protein. J. Exp. Med. 54, 437–447.
Benatuil, L., Kaye, J., Rich, R. F., Fishman, J. A., Green, W. R., and Iacomini, J. (2005). The influence of natural antibody specificity on antigen immunogenicity. Eur. J. Immun. 35, 2638–2647. doi: 10.1002/eji.200526146
Berti, F., and Adamo, R. (2013). Recent mechanistic insights on glycoconjugate vaccines and future perspectives. ACS Chem. Biol. 8, 1653–1663. doi: 10.1021/cb400423g
Beuvery, E. C., Vanrossum, F., and Nagel, J. (1982). Comparison of the induction of immunoglobulin-M and immunoglobulin-G antibodies in mice with purified pneumococcal type-3 and meningococcal group-C polysaccharides and their protein conjugates. Infect. Immun. 37, 15–22.
Bhowmick, S., Ravindran, R., and Ali, N. (2014). IL-4 contributes to failure, and colludes with IL-10 to exacerbate Leishmania donovani infection following administration of a subcutaneous leishmanial antigen vaccine. BMC Microbiol. 14:8 doi: 10.1186/1471-2180-14-8
Bishop, J. R., and Gagneux, P. (2007). Evolution of carbohydrate antigens — microbial forces shaping host glycomes? Glycobiology 17, 23R–34R. doi: 10.1093/glycob/cwm005
Blander, J. M., and Sander, L. E. (2012). Beyond pattern recognition: five immune checkpoints for scaling the microbial threat. Nat. Rev. Immun. 12, 215–225. doi: 10.1038/nri3167
Boutlis, C. S., Gowda, D. C., Naik, R. S., Maguire, G. P., Mgone, C. S., Bockarie, M. J., et al. (2002). Antibodies to Plasmodium falciparum glycosylphosphatidylinositols: inverse association with tolerance of parasitemia in Papua New Guinean children and adults. Infect. Immun. 70, 5052–5057. doi: 10.1128/IAI.70.9.5052-5057.2002
Boutlis, C. S., Riley, E. M., Anstey, N. M., and de Souza, J. B. (2005). Glycosylphosphatidylinositols in malaria pathogenesis and immunity: potential for therapeutic inhibition and vaccination. Curr. Top Microbiol. Immunol. 297, 145–185. doi: 10.1007/3-540-29967-x_5
Brasseur, P., Ballet, J. J., and Druilhe, P. (1990). Impairment of Plasmodium-falciparum-specific antibody-response in severe malaria. J. Clin. Microbiol. 28, 265–268.
Broecker, F., Hanske, J., Martin, C. E., Baek, J. Y., Wahlbrink, A., Wojcik, F., et al. (2016). Multivalent display of minimal Clostridium difficile glycan epitopes mimics antigenic properties of larger glycans. Nat. Commun. 7:11224. doi: 10.1038/ncomms11224
Buskas, T., Li, Y. H., and Boons, G. J. (2004). The immunogenicity of the tumor-associated antigen Lewis(y) may be suppressed by a bifunctional cross-linker required for coupling to a carrier protein. Chem. Eur. J. 10, 3517–3524. doi: 10.1002/chem.200400074
Buxbaum, L. U. (2013). Leishmania mexicana infection induces igg to parasite surface glycoinositol phospholipids that can induce il-10 in mice and humans. PLoS Negl. Trop. Dis. 7:e2224. doi: 10.1371/journal.pntd.0002224
Buxton, D., and Innes, E. A. (1995). A commercial vaccine for ovine toxoplasmosis. Parasitology 110, S11–S16. doi: 10.1017/S003118200000144X
Cavallari, M., and De Libero, G. (2017). From immunologically archaic to neoteric glycovaccines. Vaccines 5:4. doi: 10.3390/vaccines5010004
Cavallari, M., Stallforth, P., Kalinichenko, A., Rathwell, D. C. K., Gronewold, T. M. A., Adibekian, A., et al. (2014). A semisynthetic carbohydrate-lipid vaccine that protects against S-pneumoniae in mice. Nat. Chem. Biol. 10, 950–956. doi: 10.1038/nchembio.1650
Chappuis, F., Sundar, S., Hailu, A., Ghalib, H., Rijal, S., Peeling, R. W., et al. (2007). Visceral leishmaniasis: what are the needs for diagnosis, treatment and control? Nat. Rev. Microbiol.5, 873–882. doi: 10.1038/nrmicro1748
Cissoko, Y., Daou, M., Lyke, K. E., Dicko, A., Diarra, I., Kone, A., et al. (2006). Serum antibody levels to glycosylphosphatidylinositols in specimens derived from matched Malian children with severe or uncomplicated Plasmodium falciparum malaria and healthy controls. Am. J. Trop. Med. Hyg. 75, 199–204.
Cutchins, E. C., and Warren, J. (1956). Immunity patterns in the Guinea Pig following Toxoplasma infection and vaccination with killed Toxoplasma. Am. J. Trop. Med. Hyg. 5, 197–209. doi: 10.4269/ajtmh.1956.5.197
Cywes-Bentley, C., Skurnik, D., Zaidi, T., Roux, D., DeOliveira, R. B., Garrett, W. S., et al. (2013). Antibody to a conserved antigenic target is protective against diverse prokaryotic and eukaryotic pathogens. Proc. Nat. Acad. Sci. U.S.A. 110, E2209–E2218. doi: 10.1073/pnas.1303573110
Davies, J., and Davies, D. (2010). Origins and evolution of antibiotic resistance. Microbiol. Mol. Biol. Rev. 74, 417–433. doi: 10.1128/MMBR.00016-10
Debierre-Grockiego, F. (2010). Glycolipids are potential targets for protozoan parasite diseases. Trends Parasitol. 26, 404–411. doi: 10.1016/j.pt.2010.04.006
Debierre-Grockiego, F., Azzouz, N., Schmidt, J., Dubremetz, J. F., Geyer, H., Geyer, R., et al. (2003). Roles of glycosylphosphatidylinositols of Toxoplasma gondii - induction of tumor necrosis factor-alpha production in macrophages. J. Biol. Chem. 278, 32987–32993. doi: 10.1074/jbc.M304791200
Debierre-Grockiego, F., Campos, M. A., Azzouz, N., Schmidt, J., Bieker, U., Resende, A. G., et al. (2007). Activation of TLR2 and TLR4 by glycosylphosphatidylinositols derived from Toxoplasma gondii. J. Immunol. 179, 1129–1137. doi: 10.4049/jimmunol.179.2.1129
Debierre-Grockiego, F., and Schwarz, R. T. (2010). Immunological reactions in response to apicomplexan glycosylphosphatidylinositols. Glycobiology 20, 801–811. doi: 10.1093/glycob/cwq038
De Gregorio, E., and Rappuoli, R. (2014). From empiricism to rational design: a personal perspective of the evolution of vaccine development. Nat. Rev. Immunol. 14, 505–514. doi: 10.1038/nri3694
De Gregorio, E., Tritto, E., and Rappuoli, R. (2008). Alum adjuvanticity: unraveling a century old mystery. Eur. J. Immunol. 38, 2068–2071. doi: 10.1002/eji.200838648
de Groot, P. W. J., Bader, O., de Boer, A. D., Weig, M., and Chauhan, N. (2013). Adhesins in human fungal pathogens: glue with plenty of stick. Eukaryotic Cell 12, 470–481. doi: 10.1128/EC.00364-12
Delorenzi, M., Sexton, A., Shams-Eldin, H., Schwarz, R. T., Speed, T., and Schofield, L. (2002). Genes for glycosylphosphatidylinositol toxin biosynthesis in Plasmodium falciparum. Infect. Immun. 70, 4510–4522. doi: 10.1128/IAI.70.8.4510-4522.2002
Descoteaux, A., and Turco, S. J. (2002). Functional aspects of the Leishmania donovani lipophosphoglycan during macrophage infection. Microb. Infect. 4, 975–981. doi: 10.1016/S1286-4579(02)01624-6
Desjeux, P. (2004). Leishmaniasis: current situation and new perspectives. Comp. Immunol. Microbiol. Infect. Dis. 27, 305–318. doi: 10.1016/j.cimid.2004.03.004
de Souza, J. B., Runglall, M., Corran, P. H., Okell, L. C., Kumar, S., Gowda, D. C., et al. (2010). Neutralization of malaria glycosylphosphatidylinositol in vitro by serum IgG from malaria-exposed individuals. Infect. Immun. 78, 3920–3929. doi: 10.1128/IAI.00359-10
de Souza, J. B., Todd, J., Krishegowda, G., Gowda, D. C., Kwiatkowski, D., and Riley, E. M. (2002). Prevalence and boosting of antibodies to Plasmodium falciparum glycosylphosphatidylinositols and evaluation of their association with protection from mild and severe clinical malaria. Infect. Immun. 70, 5045–5051. doi: 10.1128/IAI.70.9.5045-5051.2002
Di Pasquale, A., Preiss, S., Da Silva, F. T., and Garcon, N. (2015). Vaccine adjuvants: from 1920 to 2015 and beyond. Vaccines 3, 320–343. doi: 10.3390/vaccines3020320
Galili, U., LaTemple, D. C., and Radic, M. Z. (1998). A sensitive assay for measuring alpha-gal epitope expression on cells by a monoclonal anti-Gal antibody. Transplantation 65, 1129–1132. doi: 10.1097/00007890-199804270-00020
Galili, U., Rachmilewitz, E. A., Peleg, A., and Flechner, I. (1984). A unique natural human-igg antibody with anti-alpha-galactosyl specificity. J. Exp. Med. 160, 1519–1531. doi: 10.1084/jem.160.5.1519
Galili, U., and Swanson, K. (1991). Gene-sequences suggest inactivation of alpha-1,3-galactosyltransferase in catarrhines after the divergence of apes from monkeys. Proc. Natl. Acad. Sci. U.S.A. 88, 7401–7404. doi: 10.1073/pnas.88.16.7401
Geissner, A., Pereira, C. L., Leddermann, M., Anish, C., and Seeberger, P. H. (2016). Deciphering antigenic determinants of Streptococcus pneumoniae serotype 4 capsular polysaccharide using synthetic oligosaccharides. ACS Chem. Biol. 11, 335–344. doi: 10.1021/acschembio.5b00768
Geissner, A., and Seeberger, P. H. (2016). Glycan arrays: from basic biochemical research to bioanalytical and biomedical applications. Annu. Rev. Anal. Chem. 9, 223–247. doi: 10.1146/annurev-anchem-071015-041641
Girard, M. P., Preziosi, M. P., Aguado, M. T., and Kieny, M. P. (2006). A review of vaccine research and development: meningococcal disease. Vaccine 24, 4692–4700. doi: 10.1016/j.vaccine.2006.03.034
Gomes, L. R., Totino, P. R., Sanchez, M. C., Daniel, E. P., Macedo, C. S., Fortes, F., et al. (2013). Asymptomatic infection in individuals from the municipality of Barcelos, (Brazilian Amazon) is not associated with the anti-Plasmodium falciparum glycosylphosphatidylinositol antibody response. Mem. Inst. Oswaldo Cruz 108, 796–800. doi: 10.1590/0074-0276108062013018
Gosling, R., and von Seidlein, L. (2016). The future of the RTS, S/AS01 malaria vaccine: an alternative development plan. PLoS Med. 13:e1001994. doi: 10.1371/journal.pmed.1001994
Gotze, S., Azzouz, N., Tsai, Y. H., Gross, U., Reinhardt, A., Anish, C., et al. (2014). Diagnosis of toxoplasmosis using a synthetic glycosylphosphatidylinositol glycan. Angew. Chem. Int. Ed Engl. 53, 13701–13705. doi: 10.1002/anie.201406706
Gotze, S., Reinhardt, A., Geissner, A., Azzouz, N., Tsai, Y. H., Kurucz, R., et al. (2015). Investigation of the protective properties of glycosylphosphatidylinositol-based vaccine candidates in a Toxoplasma gondii mouse challenge model. Glycobiology 25, 984–991. doi: 10.1093/glycob/cwv040
Gowda, D. C. (2002). Structure and activity of glycosylphosphatidylinositol anchors of Plasmodium falciparum. Microb. Infect. 4, 983–990. doi: 10.1016/S1286-4579(02)01619-2
Gowda, D. C., Gupta, P., and Davidson, E. A. (1997). Glycosylphosphatidylinositol anchors represent the major carbohydrate modification in proteins of intraerythrocytic stage Plasmodium falciparum. J. Biol. Chem. 272, 6428–6439. doi: 10.1074/jbc.272.10.6428
Grabenstein, J. D., and Klugman, K. P. (2012). A century of pneumococcal vaccination research in humans. Clin. Microbiol. Infect. 18, 15–24. doi: 10.1111/j.1469-0691.2012.03943.x
Gurale, B. P., He, Y., Cui, X. K., Dinh, H., Dhawane, A. N., Lucchi, N. W., et al. (2016). Toward the development of the next generation of a rapid diagnostic test: synthesis of glycophosphatidylinositol, (GPI) analogues of plasmodium falciparum and immunological characterization. Bioconjug. Chem. 27, 2886–2899. doi: 10.1021/acs.bioconjchem.6b00542
Heidelberger, M., and Avery, O. T. (1923). The soluble specific substance of pneumococcus. J. Exp. Med. 38, 0073–0079. doi: 10.1084/jem.38.1.73
Heidelberger, M., and Avery, O. T. (1924). The soluble specific substance of pneumococcus second paper. J. Exp. Med. 40, 301–316. doi: 10.1084/jem.40.3.301
Hoffman, S. L., Vekemans, J., Richie, T. L., and Duffy, P. E. (2015). The march toward malaria vaccines. Vaccine 33, D13–D23. doi: 10.1016/j.vaccine.2015.07.091
Horlacher, T., and Seeberger, P. H. (2008). Carbohydrate arrays as tools for research and diagnostics. Chem. Soc. Rev. 37, 1414–1422. doi: 10.1039/b708016f
Hotez, P. J., Alvarado, M., Basanez, M. G., Bolliger, I., Bourne, R., Boussinesq, M., et al. (2014). The global burden of disease study 2010: interpretation and implications for the neglected tropical diseases. PLoS Neglected Trop. Dis. 8:e2865. doi: 10.1371/journal.pntd.0002865
Hunter, C. A., Suzuki, Y., Subauste, C. S., and Remington, J. S. (1996). Cells and cytokines in resistance to Toxoplasma gondii. Toxoplasma Gondii 219, 113–125. doi: 10.1007/978-3-642-51014-4_11
Hütter, J., and Lepenies, B. (2015). Carbohydrate-based vaccines: an overview. Methods Mol. Biol. 1331, 1–10. doi: 10.1007/978-1-4939-2874-3_1
John, C. C., Tande, A. J., Moormann, A. M., Sumba, P. O., Lanar, D. E., Min, X. M., et al. (2008). Antibodies to pre-erythrocytic Plasmodium falciparum antigens and risk of clinical malaria in Kenyan children. J. Infect. Dis. 197, 519–526. doi: 10.1086/526787
Jongert, E., Roberts, C. W., Gargano, N., Forster-Wald, E., and Petersen, E. (2009). Vaccines against Toxoplasma gondii: challenges and opportunities. Mem. Inst. Oswaldo Cruz 104, 252–266. doi: 10.1590/S0074-02762009000200019
Kamena, F., Tamborrini, M., Liu, X., Kwon, Y. U., Thompson, F., Pluschke, G., et al. (2008). Synthetic GPI array to study antitoxic malaria response. Nat. Chem. Biol. 4, 238–240. doi: 10.1038/nchembio.75
Keenihan, S. N. H., Ratiwayanto, S., Soebianto, S., Krisin, Marwoto, H., Krishnegowda, G., et al. (2003). Age-dependent impairment of IgG responses to glycosylphosphatidylinositol with equal exposure to Plasmodium falciparum among Javanese migrants to Papua, Indonesia. Am. J. Trop. Med. Hygiene 69, 36–41.
Kijlstra, A., and Jongert, E. (2008). Control of the risk of human toxoplasmosis transmitted by meat. Int. J. Parasitol. 38, 1359–1370. doi: 10.1016/j.ijpara.2008.06.002
Kline, K. A., Falker, S., Dahlberg, S., Normark, S., and Henriques-Normark, B. (2009). Bacterial adhesins in host-microbe interactions. Cell Host Microb. 5, 580–592. doi: 10.1016/j.chom.2009.05.011
Krahenbuhl, J. L., Remington, J. S., and Ruskin, J. (1972). Use of killed vaccines in immunization against an intracellular parasite - Toxoplasma-gondii. J. Immunol. 108, 425–431.
Krishnegowda, G., Hajjar, A. M., Zhu, J., Douglass, E. J., Uematsu, S., Akira, S., et al. (2005). Induction of proinflammatory responses in macrophages by the glycosylphosphatidylinositols of Plasmodium falciparum: cell signaling receptors, glycosylphosphatidylinositol, (GPI) structural requirement, and regulation of GPI activity. J. Biol. Chem. 280, 8606–8616. doi: 10.1074/jbc.M413541200
Kur, J., Holec-Gasior, L., and Hiszczynska-Sawicka, E. (2009). Current status of toxoplasmosis vaccine development. Expert Rev. Vaccines 8, 791–808. doi: 10.1586/erv.09.27
Landers, C. D., Chelvarajan, R. L., and Bondada, S. (2005). The role of B cells and accessory cells in the neonatal response to TI-2 antigens. Immunol. Res. 31, 25–36. doi: 10.1385/IR:31:1:25
Lee, B. Y., Bacon, K. M., Shah, M., Kitchen, S. B., Connor, D. L., and Slayton, R. B. (2012). The Economic value of a visceral leishmaniasis vaccine in Bihar State, India. Am. J. Trop. Med. Hygiene 86, 417–425. doi: 10.4269/ajtmh.2012.10-0415
Lepenies, B. (2015). Carbohydrate-based vaccines preface. Methods Mol. Biol. 1331, v–vi. doi: 10.1007/978-1-4939-2874-3
Lindberg, A. A. (1999). Glycoprotein conjugate vaccines. Vaccine 17, S28–S36. doi: 10.1016/s0264-410x(99)00232-7
Liu, Q., Das Singla, L., and Zhou, H. Y. (2012). Vaccines against Toxoplasma gondii Status, challenges and future directions. Hum. Vaccin. Immunother. 8, 1305–1308. doi: 10.4161/hv.21006
Liu, X. Y., Siegrist, S., Amacker, M., Zurbriggen, R., Pluschke, G., and Seeberger, P. H. (2006). Enhancement of the immunogenicity of synthetic carbohydrates by conjugation to virosomes: a leishmaniasis vaccine candidate. ACS Chem. Biol. 1, 161–164. doi: 10.1021/cb600086b
Lucas, A. H., Apicella, M. A., and Taylor, C. E. (2005). Carbohydrate moieties as vaccine candidates. Clin. Infect. Dis. 41, 705–712. doi: 10.1086/432582
Luft, B. J., and Remington, J. S. (1992). Toxoplasmic encephalitis in aids. Clin. Infect. Dis. 15, 211–222. doi: 10.1093/clinids/15.2.211
Macher, B. A., and Galili, U. (2008). The Gal alpha 1,3Gal beta 1,4GlcNAc-R, (alpha-Gal) epitope: a carbohydrate of unique evolution and clinical relevance. Biochim. Biophys. Acta 1780, 75–88. doi: 10.1016/j.bbagen.2007.11.003
Mbengue, B., Niang, B., Niang, M. S., Varela, M. L., Fall, B., Fall, M. M., et al. (2016). Inflammatory cytokine and humoral responses toPlasmodium falciparumglycosylphosphatidylinositols correlates with malaria immunity and pathogenesis. Immun. Inflam. Dis. 4, 24–34. doi: 10.1002/iid3.89
McConville, M. J., Bacic, A., Mitchell, G. F., and Handman, E. (1987). Lipophosphoglycan of leishmania-major that vaccinates against cutaneous leishmaniasis contains an alkylglycerophosphoinositol lipid anchor. Proc. Natl. Acad. Sci. U.S.A. 84, 8941–8945. doi: 10.1073/pnas.84.24.8941
McConville, M. J., Collidge, T. A. C., Ferguson, M. A. J., and Schneider, P. (1993). The glycoinositol phospholipids of Leishmania-Mexicana promastigotes. Evidence for the presence of 3 distinct pathways of glycolipid biosynthesis. J. Biol. Chem. 268, 15595–15604.
McLeod, R., Kieffer, F., Sautter, M., Hosten, T., and Pelloux, H. (2009). Why prevent, diagnose and treat congenital toxoplasmosis? Mem. Inst. Oswaldo Cruz 104, 320–344.
Moll, H., Mitchell, G. F., McConville, M. J., and Handman, E. (1989). Evidence for T-Cell Recognition in Mice of a Purified Lipophosphoglycan from Leishmania-Major. Infect. Immun. 57, 3349–3356.
Mond, J. J., and Kokai-Kun, J. F. (2008). The multifunctional role of antibodies in the protective response to bacterial T cell-independent antigens. Spec. Complement. Hum. Immun Respon. Infect. 319, 17–40. doi: 10.1007/978-3-540-73900-5_2
Mond, J. J., Lees, A., and Snapper, C. M. (1995). T-cell-independent antigens type-2. Annu. Rev. Immunol. 13, 655–692. doi: 10.1146/annurev.iy.13.040195.003255
Montoya, J. G., and Liesenfeld, O. (2004). Toxoplasmosis. Lancet 363, 1965–1976. doi: 10.1016/S0140-6736(04)16412-X
Murray, C. J. L., Rosenfeld, L. C., Lim, S. S., Andrews, K. G., Foreman, K. J., Haring, D., et al. (2012). Global malaria mortality between 1980 and 2010: a systematic analysis. Lancet 379, 413–431.
Naik, R. S., Branch, O. H., Woods, A. S., Vijaykumar, M., Perkins, D. J., Nahlen, B. L., et al. (2000). Glycosylphosphatidylinositol anchors of Plasmodium falciparum: molecular characterization and naturally elicited antibody response that may provide immunity to malaria pathogenesis. J. Exp. Med. 192, 1563–1576. doi: 10.1084/jem.192.11.1563
Nishat, S., and Andreana, P. (2016). Entirely carbohydrate-based vaccines: an emerging field for specific and selective immune responses. Vaccines 4:19. doi: 10.3390/vaccines4020019
Nyame, A. K., Kawar, Z. S., and Cummings, R. D. (2004). Antigenic glycans in parasitic infections: implications for vaccines and diagnostics. Arch. Biochem. Biophys. 426, 182–200. doi: 10.1016/j.abb.2004.04.004
Patel, S. N., Lu, Z., Ayi, K., Serghides, L., Gowda, D. C., and Kain, K. C. (2007). Disruption of CD36 impairs cytokine response to plasmodium falciparum glycosylphosphatidylinositol and confers susceptibility to severe and fatal malaria in vivo. J. Immunol. 178, 3954–3961. doi: 10.4049/jimmunol.178.6.3954
Perraut, R., Diatta, B., Marrama, L., Garraud, O., Jambou, R., Longacre, S., et al. (2005). Differential antibody responses to Plasmodium falciparum glycosylphosphatidylinositol anchors in patients with cerebral and mild malaria. Microb. Infect. 7, 682–687. doi: 10.1016/j.micinf.2005.01.002
Petrovsky, N., and Aguilar, J. C. (2004). Vaccine adjuvants: current state and future trends. Immunol. Cell Biol. 82, 488–496. doi: 10.1111/j.0818-9641.2004.01272.x
Pichichero, M. E. (2013). Protein carriers of conjugate vaccines characteristics, development, and clinical trials. Hum. Vaccin. Immunother. 9, 2505–2523. doi: 10.4161/hv.26109
Pinheiro, R. O., Pinto, E. F., Guedes, H. L. D., Agrellos, O. A., de Mattos, K. A., Saraiva, E. M., et al. (2007). Protection against cutaneous leishmaniasis by intranasal vaccination with lipophosphoglycan. Vaccine 25, 2716–2722. doi: 10.1016/j.vaccine.2006.05.093
Playfair, J. H. L., Taverne, J., Bate, C. A. W., and Brian de Souza, J. (1990). The malaria vaccine: anti-parasite or anti-disease? Immunol. Today 11, 25–27.
Pulendran, B., and Ahmed, R. (2011). Immunological mechanisms of vaccination. Nat. Immunol. 12, 509–517. doi: 10.1038/ni.2039
Remington, J. S., Thulliez, P., and Montoya, J. G. (2004). Recent developments for diagnosis of toxoplasmosis. J. Clin. Microbiol. 42, 941–945. doi: 10.1128/JCM.42.3.941-945.2004
Rodrigues, J. A., Acosta-Serrano, A., Aebi, M., Ferguson, M. A. J., Routier, F. H., Schiller, I., et al. (2015). Parasite glycobiology: a bittersweet symphony. PLoS Pathog. 11:e1005169. doi: 10.1371/journal.ppat.1005169
Russell, D. G., and Alexander, J. (1988). Effective immunization against cutaneous leishmaniasis with defined membrane-antigens reconstituted into liposomes. J. Immunol. 140, 1274–1279.
Schneerson, R., Barrera, O., Sutton, A., and Robbins, J. B. (1980). Preparation, characterization, and immunogenicity of hemophilus-influenzae type-B polysaccharide-protein conjugates. J. Exp. Med. 152, 361–376. doi: 10.1084/jem.152.2.361
Schofield, L. (2007). Rational approaches to developing an anti-disease vaccine against malaria. Microb. Infect. 9, 784–791. doi: 10.1016/j.micinf.2007.02.010
Schofield, L., and Grau, G. E. (2005). Immunological processes in malaria pathogenesis. Nat. Rev. Immunol. 5, 722–735. doi: 10.1038/nri1686
Schofield, L., and Hackett, F. (1993). Signal transduction in host cells by a glycosylphosphatidylinositol toxin of malaria parasites. J. Exp. Med. 177, 145–153. doi: 10.1084/jem.177.1.145
Schofield, L., Hewitt, M. C., Evans, K., Siomos, M.-A., and Seeberger, P. H. (2002). Synthetic GPI as a candidate anti-toxic vaccine in a model of malaria. Nature 418, 785–789. doi: 10.1038/nature00937
Schofield, L., Vivas, L., Hackett, F., Gerold, P., Schwarz, R. T., and Tachado, S. (1993). Neutralizing monoclonal antibodies to glycosylphosphatidylinositol, the dominant TNF-alpha-inducing toxin of Plasmodium falciparum: prospects for the immunotherapy of severe malaria. Ann. Trop. Med. Parasitol. 87, 617–626. doi: 10.1080/00034983.1993.11812820
Seder, R. A., Chang, L. J., Enama, M. E., Zephir, K. L., Sarwar, U. N., Gordon, I. J., et al. (2013). Protection against malaria by intravenous immunization with a nonreplicating sporozoite vaccine. Science 341, 1359–1365. doi: 10.1126/science.1241800
Seeberger, P. H. (2007). Chemical Glycomics – From Carbohydrate Arrays to a Malaria Vaccine. (Bozen).
Singh, O. P., Singh, B., Chakravarty, J., and Sundar, S. (2016). Current challenges in treatment options for visceral leishmaniasis in India: a public health perspective. Infect. Dis. Poverty 5:19. doi: 10.1186/s40249-016-0112-2
Spath, G. F., Epstein, L., Leader, B., Singer, S. M., Avila, H. A., Turco, S. J., et al. (2000). Lipophosphoglycan is a virulence factor distinct from related glycoconjugates in the protozoan parasite leishmania major. Proc. Natl. Acad. Sci. U.S.A. 97, 9258–9263. doi: 10.1073/pnas.160257897
Stein, K. E. (1992). Thymus-independent and thymus-dependent responses to polysaccharide antigens. J. Infect. Dis. 165, S49–S52. doi: 10.1093/infdis/165-Supplement_1-S49
Tamborrini, M., Liu, X., Mugasa, J. P., Kwon, Y. U., Kamena, F., Seeberger, P. H., et al. (2010). Synthetic glycosylphosphatidylinositol microarray reveals differential antibody levels and fine specificities in children with mild and severe malaria. Bioorg. Med. Chem. 18, 3747–3752. doi: 10.1016/j.bmc.2010.04.059
Tillett, W. S., and Francis, T. (1929). Cutaneous reactions to the polysaccharides and proteins of pneumococcus in lobar pneumonia. J. Exp. Med. 50, 687–U152. doi: 10.1084/jem.50.5.687
Tinto, H., D'Alessandro, U., Sorgho, H., Valea, I., Tahita, M. C., Kabore, W., et al. (2015). Efficacy and safety of RTS,S/AS01 malaria vaccine with or without a booster dose in infants and children in Africa: final results of a phase 3, individually randomised, controlled trial. Lancet 386, 31–45. doi: 10.1016/S0140-6736(15)60721-8
Topuzogullari, M., Koc, R. C., Isoglu, S. D., Bagirova, M., Akdeste, Z., Elcicek, S., et al. (2013). Conjugation, characterization and toxicity of lipophosphoglycan-polyacrylic acid conjugate for vaccination against leishmaniasis. J. Biomed. Sci. 20:35. doi: 10.1186/1423-0127-20-35
Tsai, Y. H., Liu, X. Y., and Seeberger, P. H. (2012). Chemical biology of glycosylphosphatidylinositol anchors. Angew. Chem. Int. Ed. 51, 11438–11456. doi: 10.1002/anie.201203912
Varki, A., and Lowe, J. B. (2009). Biological Roles of Glycans. Essentials of Glycobiology, eds A. Varki, R. D. Cummings, J. D. Esko et al. Harbor, NY: Cold Spring Harbor.
Velez, I. D., Gilchrist, K., Arbelaez, M. P., Rojas, C. A., Puerta, J. A., Antunes, C. M. F., et al. (2005). Failure of a killed Leishmania amazonensis vaccine against American cutaneous leishmaniasis in Colombia. Trans. R. Soc. Trop. Med. Hyg. 99, 593–598. doi: 10.1016/j.trstmh.2005.04.002
Vliegenthart, J. F. G. (2006). Carbohydrate based vaccines. FEBS Lett. 580, 2945–2950. doi: 10.1016/j.febslet.2006.03.053
Weintraub, A. (2003). Immunology of bacterial polysaccharide antigens. Carbohydr. Res. 338, 2539–2547. doi: 10.1016/j.carres.2003.07.008
Wessels, M. R., Paoletti, L. C., Rodewald, A. K., Michon, F., Difabio, J., Jennings, H. J., et al. (1993). Stimulation of protective antibodies against type-Ia and type-Ib group-B streptococci by a type-Ia polysaccharide-tetanus toxoid conjugate vaccine. Infect. Immun. 61, 4760–4766.
WHO (2016a). Available online at: http://www.who.int/immunization/research/development/malaria_vaccine_qa/en/
WHO (2017). Leishmaniasis - The Disease and its Epidemiology. Retrieved from: http://www.who.int/leishmaniasis/disease_epidemiology/en
Yang, Y. G., deGoma, E., Ohdan, H., Bracy, J. L., Xu, Y. X., Iacomini, J., et al. (1998). Tolerization of anti-Gal alpha 1-3Gal natural antibody-forming B cells by induction of mixed chimerism. J. Exp. Med. 187, 1335–1342. doi: 10.1084/jem.187.8.1335
Yilmaz, B., Portugal, S., Tuan Tran, M., Gozzelino, R., Ramos, S., Gomes, J., et al. (2014). Gut microbiota elicits a protective immune response against malaria transmission. Cell 159, 1277–1289. doi: 10.1016/j.cell.2014.10.053
Zimmermann, S., and Lepenies, B. (2015). Glycans as vaccine antigens and adjuvants: immunological Considerations 1331, 11–26. doi: 10.1007/978-1-4939-2874-3_2
Keywords: carbohydrate, vaccine, malaria, toxoplasmosis, leishmaniasis
Citation: Jaurigue JA and Seeberger PH (2017) Parasite Carbohydrate Vaccines. Front. Cell. Infect. Microbiol. 7:248. doi: 10.3389/fcimb.2017.00248
Received: 21 February 2017; Accepted: 26 May 2017;
Published: 12 June 2017.
Edited by:
Brice Rotureau, Institut Pasteur, FranceReviewed by:
Susu M. Zughaier, Emory University, United StatesCharles Kelly, King's College London, United Kingdom
Rashika El Ridi, Cairo University, Egypt
Copyright © 2017 Jaurigue and Seeberger. This is an open-access article distributed under the terms of the Creative Commons Attribution License (CC BY). The use, distribution or reproduction in other forums is permitted, provided the original author(s) or licensor are credited and that the original publication in this journal is cited, in accordance with accepted academic practice. No use, distribution or reproduction is permitted which does not comply with these terms.
*Correspondence: Peter H. Seeberger, cGV0ZXIuc2VlYmVyZ2VyQG1waWtnLm1wZy5kZQ==