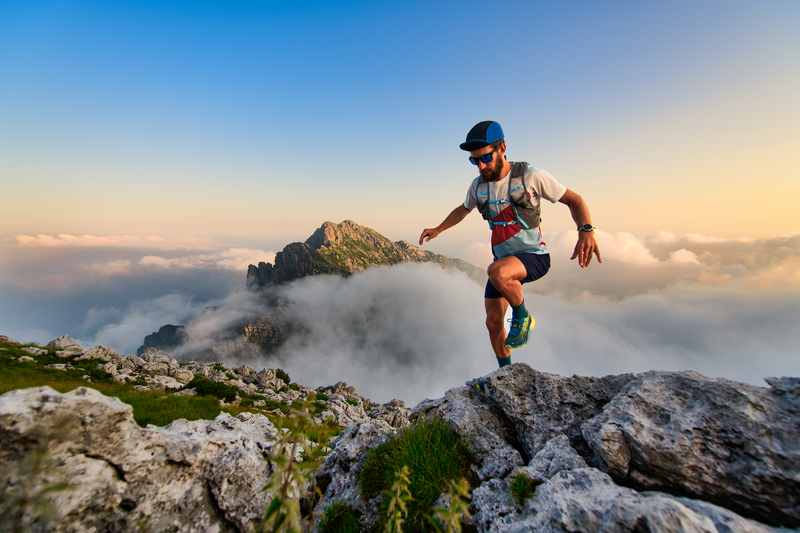
95% of researchers rate our articles as excellent or good
Learn more about the work of our research integrity team to safeguard the quality of each article we publish.
Find out more
REVIEW article
Front. Cell Death , 19 March 2025
Sec. Cellular Stress and Survival: Crosslinks, Intersections, and Pathways
Volume 4 - 2025 | https://doi.org/10.3389/fceld.2025.1470093
When yeast cells are transferred to water only, they remain viable for several days. However, when transferred to water with glucose, there is a rapid loss of viability. This phenomenon is termed Sugar-Induced Cell Death (SICD). In addition to glucose, SICD can be induced by an array of different sugars and is thought to be triggered by increased levels of intracellular reactive oxygen species (ROS) generated upon transfer to sugar-only solutions. Although not termed SICD, a similar response is observed in mammalian cells, whereby high glucose induces cell death, especially in cases of hyperglycemia and diabetes. In contrast, cancer cells thrive under conditions of high glucose. In this review, we summarize the current understanding of SICD in yeast and highlight studies showing the presence of a similar phenomenon in mammalian cells, High Glucose-Induced Cell Death (HGICD). We end with a discussion on mechanisms by which cancer cells evade HGICD. Unlike other types of cell death in yeast, SICD has not yet been thoroughly reviewed. Therefore, this review represents the first comprehensive review of SICD in yeast with a comparison to HGICD in other eukaryotes.
Like other eukaryotic cells, yeast can undergo several types of cell death. According to the guidelines and recommendations on yeast cell death nomenclature (Carmona-Gutierrez et al., 2018), death is classified into two broad groups, which is: accidental cell death (ACD) or regulated cell death (RCD). ACD is rapid upon exposure to extreme conditions and is unavoidable even through genetic or pharmacological interventions. On the other hand, RCD occurs in response to mild stress and is dependent on defined molecular machinery. RCD can also be modulated by genetics and pharmacological interventions. There are distinct types of RCD in yeast, including death with features similar to apoptosis of higher eukaryotes, though specific mechanisms differ. For the scope of this review, we maintain the term apoptosis. Apoptosis is usually associated with externalization of phosphatidylserine (PS) to the outer leaflet of the cell membrane, chromatin condensation, DNA fragmentation, cell shrinkage, mitochondrial outer membrane permeabilization (MOMP), irreversible loss of mitochondrial membrane potential, and dependence on de novo protein synthesis (Carmona-Gutierrez et al., 2018) (Table 1). Apoptosis can be dependent or independent of the yeast metacaspase, YCA1 (Carmona-Gutierrez et al., 2018; Büttner et al., 2011; Falcone and Mazzoni, 2016; Carmona-Gutierrez et al., 2010). Currently, YCA1 is the only identified yeast metacaspase (Madeo et al., 2002), however, other proteases may have related functions (Hauptmann and Lehle, 2008).
In yeast, cell death can also occur through necrosis, either primary necrosis or secondary. Primary necrosis is characterized by the absence of apoptotic markers, while secondary necrosis usually follows apoptosis (Carmona-Gutierrez et al., 2018). Primary necrosis is further categorized into accidental necrosis, which usually occurs due to severe stress and is uncontrollable, or regulated necrosis, which occurs through a precise genetically controlled mechanism. Unlike accidental necrosis, regulated necrosis can be inhibited by genetic and pharmacological interventions (Carmona-Gutierrez et al., 2018; Eisenberg et al., 2010). Necrosis in yeast is usually associated with the absence of apoptotic markers along with an increase in cell volume, membrane permeabilization, nucleo-cytosolic translocation of Nhp6A, disintegration of subcellular structures (Table 1), MOMP, and loss of mitochondrial membrane potential (Carmona-Gutierrez et al., 2018; Carmona-Gutierrez et al., 2010; Madeo et al., 2009; Ruckenstuhl et al., 2010).
Yeast can undergo RCD and ACD upon exposure to extrinsic factors such as H2O2, acetic acid, heat, or pheromones (Guaragnella et al., 2007; Severin and Hyman, 2002; Zhang et al., 2006; Alby et al., 2010; Stolp et al., 2022). Typically, lower concentrations of these stimuli result in regulated cell death while higher concentrations result in rapid necrosis (Severin and Hyman, 2002; Ahn et al., 2005; Chaves et al., 2021). Since the discovery of yeast metacaspase, YCA1 (Madeo et al., 2002), a huge effort has been made to elucidate the cell death pathways in yeast and to determine similarities to other eukaryotic cells (Falcone and Mazzoni, 2016; Carmona-Gutierrez et al., 2010). Cell death has been studied in response to by-products of metabolism such as acetic acid, ethanol, and H2O2, amongst others. H2O2-induced cell death is well documented in mammalian cells, since it is a common ROS cells must mitigate for survival (Guaragnella et al., 2007; Chidawanyika and Supattapone, 2021). On the other hand, acetic acid is a common stressor and its accumulation is a major issue in the fermentation industry due to its impact on the yield and quality of the fermentation products (Chaves et al., 2021; Giannattasio et al., 2013). Although glucose is a universal energy source and poses a serious impact on mammalian cell survival, glucose- and sugar-induced cell death has been relatively understudied in yeast, perhaps because sugars are most often associated with positive effects on growth and survival of yeast. In this review, we explore sugars as triggers for cell death. We then discuss the occurrence of SICD in mammalian cells and follow with the evasion of SICD by cancer cells. Resolving the molecular mechanism and genetic regulation of SICD in yeast may allow this system to become a valuable tool in studying hyperglycemia-induced cell death and cancer metabolism. Additionally, this system can be utilized for screening and for the discovery of novel therapeutics and targets.
Sugar-induced cell death (SICD) is a phenomenon described in Saccharomyces cerevisiae where cells rapidly lose viability in the presence of glucose and the absence of other nutrients to support their growth (Granot and Snyder, 1991). This phenomenon was first described in 1991 by David Granot while studying the nutrients required for cell-cycle induction in S. cerevisiae. Granot discovered that cells transferred to water-only or a nitrogen-containing solution remained viable for several days, however, cells transferred to a 2% glucose solution rapidly lost viability (Granot and Snyder, 1991). This loss of viability was seen in exponential phase and stationary phase cells, as well as spores. Cells incubated in glucose-only solutions showed morphological and physiological changes synonymous with growth. The characteristic large vacuole of stationary phase cells disperses in glucose-only solutions and in synthetic media or rich media with glucose. Additionally, adenine biosynthesis and earlier bud emergence were shown under these conditions. Interestingly, these growth events were not observed in water-only or synthetic media lacking glucose, suggesting that glucose is solely sufficient to initiate growth (Granot and Snyder, 1991). These morphological and metabolic changes were also observed in cells incubated in other carbon sources, both fermentable and non-fermentable, in the absence of other nutrients. This supports the hypothesis that glucose, and sugars in general, induce growth, however, in the absence of other nutrients to support growth, the cells die (Granot and Snyder, 1993).
SICD has been widely studied in S. cerevisiae, however, other species and strains are currently being explored for the occurrence of this phenotype. SICD is screened for by culturing the organism to be tested in either rich or minimal media, under its optimal growth temperature, to either stationary or exponential phase, followed by washing the cells twice in water and then transferring them to either water-only or a glucose-only solution, which is typically a 2% concentration. In all reported strains, cells exposed to solutions of glucose-only or other sugars exhibited decreased viability compared to cells incubated in water-only for the same time. SICD was reported in several strains of S. cerevisiae, including both yeast mating types. The strains studied were: W303-1A (MATa) (Hoeberichts et al., 2010), BY4741(MATa) (Lee et al., 2011a; Dušková et al., 2021; Avtukh et al., 2023; Lee et al., 2011b; Du et al., 2015), BY4742 (MATα) (Avtukh et al., 2023), A364A (MATα), S288c (MATα) (Granot and Snyder, 1991; Du et al., 2015), Y426 (MATa) (Granot and Snyder, 1993; Granot et al., 2003), Y426/Y427 (MATa/MATα) (Granot and Snyder, 1991; Granot and Snyder, 1993), DFY632 (MATa) (Granot and Dai, 1997), DBY7286 (MATα) (Yoshimoto et al., 2009), SEY6210 (MATα) (Bidiuk et al., 2021; Valiakhmetov et al., 2019). SICD was also demonstrated in Saccharomyces pastorianus, the allopolyploid hybrid of S. cerevisiae and S. bayanus. This study tested the viability of cells in a 10% glucose solution instead of the conventional 2% concentration (Yoshimoto et al., 2009). Both S. pastorianus and S. cerevisiae survived in YPD with 10% glucose and in water-only at 20οC, however, in a solution of 10% glucose-only, there was a 99% reduction in the viability of both strains (Yoshimoto et al., 2009). It is not clear why 10% glucose was used in this study as standard YPD media with 2% glucose is sufficient for cultivation in the lab, however, S. pastorianus is a lager yeast and higher concentration of sugar is often used for fermentation (Miller et al., 2012). Nevertheless, it is unknown whether 2% glucose would induce SICD in S. pastorianus.
Lactose was shown to induce cell death in the yeast K. lactis, which is known for its ability to use lactose as a sole carbon source. Lactose enters these cells via lactose permease and is then hydrolyzed to glucose and galactose by β-galactosidase. However, in mutants lacking LAC4, the gene that encodes β-galactosidase, lactose becomes toxic to cells, and cell death is induced (Lodi and Donnini, 2005). Interestingly, when the permease is disrupted in Δlac4, the cells were able to grow on lactose and cell death was prevented, suggesting that the uptake of lactose led to cell death (Lodi and Donnini, 2005). This death was described as substrate-accelerated death and is said to be because of high intracellular osmotic pressure due to excessive uptake of lactose. Plating cells on media with KCl to determine survival by colony forming unit (CFU) assays resulted in enhanced recovery of cells (Lodi and Donnini, 2005).
It was previously reported that Candida albicans does not undergo SICD (Du et al., 2015), however, cell death does occur in response to N-acetylglucosamine (Glc-NAC) (Du et al., 2015). Glc-NAC is an important carbon source and signaling molecule for C. albicans in the human gut. When C. albicans was transferred to water-only and incubated at 30°C, cells remained viable for several days. A similar response was observed in 2% glucose and 2% sorbitol solutions. However, in the presence of Glc-NAC, the cells show hallmarks of both apoptosis and necrosis. There was a rapid loss of viability accompanied by reactive oxygen species (ROS) accumulation, DNA fragmentation, and externalization of phosphatidylserine, followed by propidium iodide (PI) internalization. This loss of viability in the presence of Glc-NAC was termed GICD. GICD was also observed at 25°C and 37°C, and in YNB media with Glc-NAC as the sole carbon source (Du et al., 2015).
Several studies have shown that glucose entry and phosphorylation are critical for SICD. L-glucose, a glucose analog that cannot enter S. cerevisiae, and 6-deoxy-glucose, a glucose analog that can enter S. cerevisiae but cannot be phosphorylated, failed to induce SICD (Figure 1), suggesting that glucose entry and its phosphorylation is critical for SICD (Granot and Snyder, 1991; Granot and Dai, 1997).
Figure 1. An overview of the current understanding of SICD in S. cerevisiae. The early events of SICD are displayed in the left half of the figure, while the cell death response is depicted in the right half. Left: Glucose enters the cell and is phosphorylated. Glucose uptake also activates plasma membrane H+-ATPases, and H+ ions are pumped out of the cells resulting in acidification of the extracellular environment. The Crabtree effect is disrupted; therefore, fermentation is blocked, and glucose is metabolized by respiration and oxidative phosphorylation, which is evidenced by increased oxygen consumption upon transfer to a glucose-only solution. ATP levels remain low perhaps due to suboptimal phosphate levels and ROS production increase. Right: Accumulation of intracellular ROS triggers either necrosis or apoptosis when cells are transferred to a glucose-only solution during exponential phase or stationary phase, respectively. This is followed by externalization of PS on the outer leaflet of the plasma membrane, degradation of the nuclear membrane, fragmentation of DNA, depolarization and permeabilization of the plasma membrane, increased K+ efflux, nucleotide and small molecule release from the cell, and the extracellular environment remains acidified, in the early stages of SICD. Created in BioRender. Parbhudayal, R. (2025) https://BioRender.com/p47w704.
The requirement of glucose phosphorylation for SICD was further confirmed by genetic studies using mutants unable to phosphorylate specific sugars. In S. cerevisiae, hexokinase 1 (HXK1) and hexokinase II (HXK11) function to phosphorylate both glucose and fructose. A double mutant, Δhxk1hxk2, cannot phosphorylate fructose and therefore these cells cannot grow on fructose as a sole carbon source. Fructose induces SICD in WT cells, however, it was found that the Δhxk1hxk2 retained viability in a fructose-only solution (Granot and Snyder, 1993).
Another study confirmed the importance of phosphorylation for SICD with the use of a S. cerevisiae triple mutant, DFY632, which is deficient in HXKI, HXKII, and GLK1. GLK1 encodes glucokinase 1, which only phosphorylates glucose (Granot and Dai, 1997; Herrero et al., 1999). Therefore, the DFY632 strain cannot grow in media with glucose or fructose as a sole carbon source. As a result, this mutant also remains viable in glucose- and fructose-only solutions. Additionally, when DFY632 was transformed with a plasmid containing a hexokinase from Arabidopsis thaliana which allows for growth on glucose and fructose, the cells regained their ability to grow with glucose or fructose as sole carbon sources. When DFY632 transformed cells were transferred to glucose-only solutions, the cells exhibited SICD (Granot and Dai, 1997). This model system also allowed for examination of the rate of phosphorylation on SICD, because the A. thaliana hexokinase phosphorylates glucose and fructose with differential affinity, with a Km of 4.4 µM for glucose and 17 mM for fructose. When DFY632 transformed cells are grown in concentrations of glucose that allow for glucose phosphorylation, the rate of SICD in glucose is faster than that in fructose. Similarly, when grown in higher concentrations of the sugars to allow for phosphorylation of both, the rate of SICD was similar. Therefore, the rate of SICD is directly related to the rate of sugar phosphorylation (Granot and Dai, 1997). Since the rate of glucose phosphorylation is also directly proportional to the growth rate (Rolland et al., 2002), the increased growth induction by glucose is likely to trigger cell death. This also suggests that glucose 6-phosphate or intermediates downstream of glucose metabolism are directly or indirectly triggering cell death.
It remains unclear whether glycolysis remains active after cells are transferred to glucose-only solutions. While glycolytic intermediates were detected intracellularly, a baseline (in water -only) was not reported to determine if glucose incubation changes the levels of these intermediates. However, it was shown that exogenous supplementation of phosphate and succinate, which protect against SICD, increased the levels of glucose-6 phosphate and fructose-1-6 bisphosphate, indicating that glycolysis may be active, however, the levels were not reported in the absence of glucose (Lee et al., 2011a).
Several studies have shown that SICD in S. cerevisiae is associated with an increase in intracellular ROS levels, similar to other types of cell death (Granot and Snyder, 1991; Lee et al., 2011a; Lee et al., 2011b; Granot et al., 2003; Valiakhmetov et al., 2019). Accordingly, exogenous supplementation of antioxidants protected cells from SICD (Granot et al., 2003; Valiakhmetov et al., 2019). Using the 2,7-dihydrochloro-fluorescein dye, it was shown that stationary phase cells incubated in 2% glucose solutions have significantly higher levels of ROS than cells in water-only. Addition of 10 mM of ascorbic acid to 2% glucose solutions showed significant protection from SICD, however, there was not 100% protection, suggesting that ROS may not be the sole cause of SICD (Granot et al., 2003). Additionally, although intracellular ROS levels increase immediately upon transfer to glucose-only solutions, the cells survive if they are transferred back to water (Granot and Snyder, 1993).
Triclabendazole, an anti-helminthic drug, can partially protect stationary phase cells of S. cerevisiae from SICD by reducing the levels of intracellular ROS. This drug was also shown to partially protect against H2O2-induced cell death (Lee et al., 2011b; Lee et al., 2013). Similarly, exponential phase cells can also be protected from SICD by ascorbic acid or glutathione (GSH) (Valiakhmetov et al., 2019). The source of ROS for SICD is yet to be determined, however, since SICD also occurs in petite colonies, it is unlikely that mitochondria are the source of ROS for SICD (Granot et al., 2003; Valiakhmetov et al., 2019).
Even though mitochondria are believed to be a major producer of ROS, peroxisomes and the endoplasmic reticulum (ER) are also known to produce ROS (Yoboue et al., 2018). Deletion of mitochondrial DNA in S. cerevisiae also results in ROS accumulation (Leadsham et al., 2013; Yi et al., 2018), which is exacerbated by loss of cytochrome c oxidase (COX). Interestingly, Yno1p (NADPH oxidase), localized on the ER, was shown to be a significant contributor of ROS in strains with impaired mitochondrial function and reduced COX activity (Leadsham et al., 2013; Yi et al., 2018; Rinnerthaler et al., 2012). Single mutation of YNO1 or RAS2 in mitochondrial deficient cells resulted in reduced ROS accumulation, however, double mutants did not produce synergistic phenotype, suggesting YNO1 and RAS2 function is dependent on each other. Additionally, overexpression of Yno1p triggers YCA1-dependent cell death through increased Yno1p-generated ROS accumulation (Leadsham et al., 2013; Yi et al., 2018; Rinnerthaler et al., 2012). A model was proposed in which dysfunctional mitochondria function through RAS to promote an environment conducive to ROS accumulation (Leadsham et al., 2013; Yi et al., 2018; Rinnerthaler et al., 2012), therefore, it would be interesting to explore the involvement of such extramitochondrial sources of ROS in SICD.
S. cerevisiae preferentially utilizes glucose through aerobic fermentation and switches off respiration until glucose is depleted. This process is commonly described as overflow metabolism or the Crabtree effect in yeast (Malina et al., 2021). Inhibition of respiration with Antimycin A or EtBr (ethidium bromide), in the presence of glucose, protected cells from SICD with a corresponding reduction in ROS levels. Disrupting the TCA cycle by mutation of SDH1, SDH2, and FUM1, or the glyoxylate cycle by mutation of the key gene, ICL1, also protected against SICD. While these mutations resulted in decreased ROS levels in glucose-only solutions, the ROS levels and cell death in water-only were much greater than in the WT (Lee et al., 2011a). Therefore, it is difficult to conclude whether these mutations protected against SICD or if glucose enhanced survival of these mutants. The author proposed that SICD is due to disruption of the Crabtree effect, therefore, downregulation of respiration and oxidative phosphorylation will protect from SICD. This is also supported by evidence of increased oxygen consumption in cells transferred to glucose-only solutions (Lee et al., 2011a). Studies that have reported intracellular ATP levels found that incubation in glucose solutions results in depletion of ATP, which is not seen in cells incubated in water-only, leading to the conclusion that ATP exhaustion may also contribute to cell death in the presence of sugars (Lee et al., 2011a; Dušková et al., 2021).
Furthermore, WT cells can be protected from SICD by exogenous supplementation of succinate, and to a lesser extent, acetate, in the presence of glucose. However, both succinate and acetate induce cell death in the absence of glucose (Lee et al., 2011a). Succinate enters mitochondria through Dic1 transporter in exchange for phosphate, depleting the phosphate in the mitochondrial matrix, thereby inhibiting ATP synthesis (Lee et al., 2011a). On the other hand, exogenous supplementation of phosphate in the presence of glucose also prevents SICD through induction of 1-6 bisphosphate synthesis, which is known to inhibit respiration (Lee et al., 2011a; Díaz-Ruiz et al., 2008). It was proposed that in glucose-only solutions, intracellular phosphate levels are low and since phosphate activates phosphofructokinase (PFK) to synthesize fructose 1,6-bisphosphate, fructose 1,6-bisphosphate synthesis remains low. However, exogenous supplementation of phosphate restores the intracellular phosphate deficit and increases fructose 1,6-bisphosphate synthesis, thereby upregulating ATP production. ATP then allosterically inhibits PFK to downregulate respiration, and protect against SICD (Lee et al., 2011a).
When yeast cells are transferred to glucose-only solutions, there is a rapid decline in extracellular pH from pH 7.0 to pH 3.7 after 5 min, whereupon the pH remains constant. In contrast, when cells are transferred to water only, the pH declines from 7.0 to 6.0 over an hour (Bidiuk et al., 2021). It was shown that neutralizing the extracellular pH can prevent SICD (Bidiuk et al., 2021) (Figure 2). The rapid decline in pH observed in the presence of glucose likely cause a significant difference in pH on the plasma membrane. Dissipation of the H+ ions by ionophores protects against cell death and causes a lower degree of acidification of the extracellular environment (Bidiuk et al., 2021). This study showed that Rim101/PacC, which senses alkaline stress, and cAMP protein kinase pathway (PKA), the activation of which can lead to sensitivity to alkaline stress, are not involved in protection against SICD in neutral conditions, as these mutants remained protected from SICD in neutral conditions (Bidiuk et al., 2021).
Figure 2. Mechanisms that prevent SICD. SICD in S. cerevisiae can be prevented through various mechanisms, mainly resulting in a reduction in intracellular ROS levels. This can occur by blocking glucose uptake and/or phosphorylation, through the addition of antioxidants such as ascorbic acid and glutathione to reduce ROS levels, by supplementation with nitrogen to support amino acid synthesis and restoration of carbon to nitrogen balance, through the addition of KCl to decrease the potential of the plasma membrane, through neutralization of extracellular pH, and by inhibition of respiration and oxidative phosphorylation by agents such as Antimycin A and EtBr. All mechanisms known to prevent SICD result in a decline in intracellular ROS. Created in BioRender. Parbhudayal, R. (2025) https://BioRender.com/i39g802.
Acidification of the media after glucose incubation can also be due to the release of Krebs cycle acids (Kotyk et al., 1999), or acetic acid, which is known to induce RCD in S. cerevisiae (Chaves et al., 2021). Interestingly, analysis by mass spectrometry, by both liquid chromatography (LC-MS) and gas chromatography (GC-MS), did not reveal any compounds of molecular weight corresponding to Krebs cycle acids or acetic acid in supernatants collected from S. cerevisiae BY4741 incubated in a 100 mM glucose-only solution for an hour at 30°C (Avtukh et al., 2023). Octanoic acid was detected at an estimated concentration of 0.7µM, which is too low to induce cell death in S. cerevisiae (Avtukh et al., 2023).
Antioxidants such as ascorbic acid and GSH used in SICD studies were neutralized and buffered to pH 7 to prevent toxicity to cells due to the low pH of their solutions. Considering the effect of pH on SICD, it becomes unclear if the observed protective effect against SICD was due to antioxidant activity or due to the impact pH has on SICD (Granot et al., 2003; Valiakhmetov et al., 2019).
When exponential phase cells are transferred to glucose-only solutions, there is a rapid increase in the membrane potential of the cells (Hoeberichts et al., 2010; Bidiuk et al., 2021). This can be prevented by either buffering the extracellular environment to pH 7 (Bidiuk et al., 2021) or by the addition of KCl to the glucose solutions (Hoeberichts et al., 2010; Bidiuk et al., 2021). Since KCl prevents SICD even in an acidified environment, the authors proposed that membrane potential may play a more important role in SICD than pH (Bidiuk et al., 2021).
The role of K+ in SICD was further examined through mutational studies. Single or double deletion of TRK1 and/or TRK2 K+ uniporters increased sensitivity to SICD (Hoeberichts et al., 2010; Dušková et al., 2021). Similarly, double deletion of HAL4 and HAL5, which activates Trk-mediated K+ uptake, also increased sensitivity to SICD (Hoeberichts et al., 2010). Accordingly, reducing K+ efflux using a triple mutant lacking three major K+ efflux transporters in S. cerevisiae, ENA1, NHA1, and TOK1, protects against H2O2-mediated cell death and SICD, suggesting a universal role of K+ in the regulation of cell death (Hoeberichts et al., 2010). This K+ efflux resulted in vacuolar lysis, perhaps due to loss of intracellular osmolarity—the first step in autolysis (Hoeberichts et al., 2010).
Glucose is known to activate plasma membrane ATPases to signal growth and nutrient uptake (Serrano, 1983). It was shown that mutants with reduced plasma membrane H+-ATPase activity are protected from SICD, suggesting that indeed H+-ATPase activity is activated under SICD conditions and is critical for SICD to occur (Hoeberichts et al., 2010). Together, this led to a proposed mechanism whereby glucose falsely activates H+-ATPases, in the absence of external K+, which leads to the dielectric breakdown of the plasma membrane, causing small molecules, including K+ ions and nucleotides, to leak out of the cell. Therefore, SICD can be prevented by reducing the plasma membrane H+-ATPase activity, increasing K+ influx, and decreasing K+ efflux (Hoeberichts et al., 2010; Dušková et al., 2021) (Figures 1, 2). It is important to note that protection from SICD seen with modulation of K+ transport and H+-ATPase activity also led to a decline in intracellular ROS (Hoeberichts et al., 2010).
The relationship between K+ transport and glucose uptake appears to be quite complex as suppressor mutations in glucose transport genes, HXT1 and HXT3 were shown to lead to increased K+ uptake and survival on K+-limiting media of Δtrk1Δtrk2 strains of S. cerevisiae without affecting glucose transport (Liang et al., 1998). It is yet to be determined how such mutations would affect SICD and if this could be a potential target for prevention of SICD as well. An overview of SICD in S. cerevisiae is summarized in Figure 1.
During SICD, exponential and stationary phase yeast cells undergo different cell death phenotypes. The markers of cell death are discussed below and summarized in Table 1.
Interestingly, it was shown that cells in the S-phase of the heterogenous exponential phase population were more susceptible to SICD (Valiakhmetov et al., 2019). SICD in exponential phase cells resembles that of primary necrosis. When exponential phase cells were exposed to glucose-only solutions, PI internalization peaked within an hour of incubation. This was accompanied by a corresponding increase in intracellular ROS levels which can be reduced by the addition of antioxidants such as ascorbic acid and GSH (Valiakhmetov et al., 2019). The nucleus appeared enlarged, and chromatins were released into the cytosol, as demonstrated by electron microscopy, perhaps due to disruption of the nuclear membrane. There was no externalization of PS on the plasma membrane, and cell death was not dependent on the yeast metacaspase, YCA1 or on de novo protein synthesis (Valiakhmetov et al., 2019) (Table 1).
SICD in stationary phase cells shows characteristics of mammalian apoptosis (Granot et al., 2003). Unlike exponential phase cells where SICD is detected within an hour (Valiakhmetov et al., 2019), SICD in stationary phase cells develops over several hours to days (Carmona-Gutierrez et al., 2018; Granot et al., 2003; Madeo et al., 1999). This is perhaps owing to the upregulation of ROS defense mechanisms to protect from oxidative stress due to respiration and the diauxic shift (Leadsham et al., 2013; Galdieri et al., 2010) or due to induction of autophagy by depleted glucose in stationary phase (Adachi et al., 2017; Iwama and Ohsumi, 2019). Stationary phase cells undergoing SICD appear smaller in size (Granot and Snyder, 1991; Granot et al., 2003) with fragmentation of the nucleus, DNA and RNA, in addition to the presence of a ruptured cell membrane and vesicle formation (Granot et al., 2003). These features were not observed when cells were incubated in water-only or sorbitol solutions (Granot and Snyder, 1991; Granot et al., 2003). There was also an increase in intracellular ROS which can be mitigated by antioxidants supplementation, such as ascorbic acid and GSH (Granot et al., 2003). Glucose induction of apoptosis in stationary phase cells is more often seen in dividing cells than non-dividing cells, suggesting that high glucose is reducing efficiency of G0/G1 arrest in the stationary phase leading to rapid induction of apoptosis in this population (Weinberger et al., 2010) (Table 1).
SICD in stationary phase cells was shown to be independent of YCA1 and calcineurin (Hoeberichts et al., 2010). Pheromone-induced cell death in S. cerevisiae is independent of YCA1 but was shown to be modulated by calcineurin which is a Ca2+-calmodulin-activated protein phosphatase that promotes survival. Mutants deficient in CNB1, which encodes a major regulatory unit of calcineurin, showed increased sensitivity to pheromone-induced cell death, however, the extent of SICD was like that of the WT, suggesting that SICD is independent of this pathway (Severin and Hyman, 2002; Zhang et al., 2006; Hoeberichts et al., 2010). Although SICD in stationary phase cells is independent of YCA1, caspase-independent pathways are yet to be explored for their involvement in SICD.
SICD of stationary phase cells was also shown to be accompanied by vacuole collapse and the release of K+ and nucleotides by the cell as discussed above (Hoeberichts et al., 2010). On the other hand, mutants deficient in STE20 were shown to be resistant to SICD. STE20 is the yeast homolog of mammalian Mst1, which is involved in programmed cell death. During H2O2-induced cell death, Ste20p is translocated to the nucleus where it phosphorylates histone H2B, which leads to DNA fragmentation. Δste20 are not only resistant to H2O2 -induced cell death, but also to SICD suggesting similarities in the regulatory mechanism of SICD and other types of cell death (Ahn et al., 2005; Du and Liang, 2006).
SICD in S. cerevisiae has been studied at 20οC (Yoshimoto et al., 2009), 25οC (Granot and Snyder, 1991) 30οC (Granot and Snyder, 1991; Granot and Snyder, 1993), and 37οC (Granot and Snyder, 1991; Granot and Dai, 1997), but is said to be enhanced at 37οC (Granot and Dai, 1997). More studies are needed to understand the role of temperature in SICD to determine if there is a viable response with varied temperature.
CFU assays done to determine the survival of S. cerevisiae after exposure to glucose revealed the formation of petite colonies. Petite colonies are known to be deficient in mitochondrial respiration and carry mutations or exhibit a loss of mitochondrial DNA. These colonies were unable to grow on non-fermentable carbon sources, thereby confirming these were petite colonies deficient in respiration. However, it was found that petite colonies were even more sensitive to SICD than the parental cells, suggesting that respiration or mitochondrial DNA is not required for SICD (Lee et al., 2011a; Granot et al., 2003). Additionally, respiratory-deficient petite colonies of S. pastorianus obtained by EtBr treatment also resulted in SICD (Yoshimoto et al., 2009). SICD was demonstrated in both aerobic and anaerobic conditions in S. pastorianus when transferred to a 10% glucose solution. Interestingly, SICD in aerobic and anaerobic conditions resulted in the same extent of death, where a 99% decrease in viability was found (Yoshimoto et al., 2009). This further supports the idea that SICD is not dependent on the presence of oxygen or respiration.
Several fermentable (glucose, fructose, and sucrose) and non-fermentable (maltose, galactose, acetate, ethanol, and glycerol) carbon sources were evaluated for their ability to induce SICD in S. cerevisiae. All tested carbon sources induced SICD, however, fermentable carbon sources resulted in more cell death than non-fermentable (Granot and Snyder, 1993; Dušková et al., 2021). Interestingly, maltose, a sugar that is not metabolizable by S. cerevisiae Y426/Y427, did not affect cell viability when transferred to a maltose-only solution, viability resembled that of cells in water-only (Granot and Snyder, 1993), however, maltose was shown to induce SICD in S. cerevisiae BY4741, which suggests that there may be strain specific responses (Dušková et al., 2021). The rate of SICD induced by each respective sugar seems to be correlated to the rate of uptake and usage of the sugar by the strain tested (Granot and Snyder, 1993). What is even more interesting is that these carbon sources also induce the early growth events as seen with glucose, that is, vacuole dispersal, earlier bud emergence, and induction of adenine biosynthesis (Granot and Snyder, 1993), suggesting that these sugars are affecting a common secondary messenger, directly or indirectly, leading to induction of these growth events which is followed by cell death (Granot and Snyder, 1993).
Disruption of the adenylate synthase pathway, which plays an integral role in glucose metabolism in S. cerevisiae (Tamaki, 2007), did not prevent any of the growth-related events nor cell death. (Granot and Snyder, 1991). Temperature-sensitive mutations in CDC25 or CDC35, which result in decreased cAMP levels, did not prevent cell death (Granot and Snyder, 1991). Additionally, activation of cAMP/PKA through deletion of IRA2, which results in elevated levels of cAMP in the presence of glucose, did not affect cell death (Bidiuk et al., 2021; Park et al., 2005). Together, these studies indicate that the adenylate synthase signaling pathway is not involved in SICD. Therefore, cAMP is not the secondary messenger involved in signaling SICD.
SICD can be induced by glucose concentrations as low as 0.1% (5.55 mM) (Granot and Snyder, 1993). The high-affinity glucose permease, SNF3, was found to be important for SICD in low concentrations of glucose but not high concentrations of glucose. Mutants deficient in SNF3 grow poorly on 0.1% glucose, but grow well in 2% glucose, and do not undergo SICD in 0.1% glucose, but do undergo SICD in 2% glucose. On the contrary, 0.1% glucose was sufficient to induce SICD in the WT cells, although at a comparatively slower rate than 2% glucose (Granot and Snyder, 1993). This suggests that glucose uptake is essential for SICD.
Nitrogen availability is important for cell cycle progression and survival of yeast as it is required for biogenesis of several macromolecules owing to its incorporation into nucleotides and amino acids. In the absence of nitrogen, non-essential nitrogenous compounds, including excess purines, pyrimidines, and proteins, may be degraded to synthesize essential compounds. In the presence of ammonia, intermediates of glycolysis and the TCA cycle serve as a backbone for amino acid synthesis. Glycolysis intermediates participate in the synthesis of histidine, serine, alanine, cysteine, glycine, tryptophan, phenylalanine, tyrosine, valine, leucine, and isoleucine. The TCA intermediate, oxaloacetate, can be utilized for the synthesis of asparagine, methionine, and threonine (Nishimura, 2024; Magasanik and Kaiser, 2002; Rødkær and Færgeman, 2014) (Figure 3).
Figure 3. Carbon and nitrogen are important for the survival of S. cerevisiae. When yeast cells are exposed to glucose in the absence of nitrogen, cell death occurs. Likewise, cell death occurs when yeast cells are exposed to nitrogen in the absence of glucose. However, in the presence of both, glucose and nitrogen, in a balanced ratio, the cells survive. Nitrogen is important for amino acid and nucleotide synthesis. NH4 combines with intermediates of glycolysis and the TCA cycle to produce amino acids. On the other hand, NH4 can be assimilated from the degradation of excess purines, pyrimidines, and amino acids. Created in BioRender. Parbhudayal, R. (2025) https://BioRender.com/u63n824.
Glutamate, a preferred nitrogen source and nitrogen donor, is particularly important for longevity of S. cerevisiae (Wu et al., 2013). Glutamate is readily made from α-ketoglutarate (AKG) and ammonia, while it is also degraded back to AKG and ammonia for the biosynthesis of other amino acids, including proline and arginine or combine with ammonia to produce glutamine (Figure 3) (Magasanik and Kaiser, 2002; Rødkær and Færgeman, 2014). It was shown that ammonium and glutamate can rescue S. pombe from mitotic catastrophe that would result in cell death (Zemlianski et al., 2024). Glutamate is also a precursor for GSH, through which it was shown to extend the life span of S. cerevisiae through reduction of ROS accumulation (Magasanik and Kaiser, 2002; Rødkær and Færgeman, 2014; Wu et al., 2013), thereby highlighting a versatile role of glutamate in protection against cell death.
In addition to nitrogen availability, studies suggest that nitrogen and carbon balance play a role in cell survival and in the prevention of SICD (Figure 3). It is important to note that some of these studies were performed in synthetic media, whereas in SICD studies, cells are in water supplemented with the respective sugars. The importance of a balance in nitrogen and carbon availability in preventing SICD was demonstrated in S. pastorianus where it was shown that supplementation of nitrogen to 10% glucose prevented SICD, however, when the nitrogen concentration was low, cell death was even more dramatic than cells in glucose-only (Yoshimoto et al., 2009).
Furthermore, it was shown that both carbon and nitrogen, provided independently, induce cell death. Cell death induced by ammonium in yeast has not been well studied and was thought to be overlooked because conventional growth media contain high enough levels of potassium, which was shown to protect against ammonium toxicity (Hess et al., 2006). It was demonstrated that in minimal medium, nitrogen, in the form of (NH4)2SO4, results in decreased growth under low potassium concentrations in synthetic media. To detoxify excess (NH4)2SO4, the cells excrete amino acids. Interestingly, as potassium concentration increased, ammonium toxicity and amino acid excretion decreased. This phenotype was observed in S. cerevisiae S288c, CEN.PK and Σ (Hess et al., 2006).
These findings are further supported by another study showing that balance in nitrogen and carbon is important for the longevity of S. cerevisiae PYCC 4072. In the presence of 0.5% glucose, 0.05%–0.5% (NH4)2SO4 can maintain viability of PYCC 4072, however, in 2% glucose, 0.1%–1% (NH4)2SO4 led to progressive loss of viability. Interestingly, increasing nitrogen concentrations beyond 134 mg/L significantly shortened chronological life span (CLS) due to replicative stress. The same response was observed when glutamine was used as a nitrogen source, suggesting that nitrogen is regulating this response. On the other hand, the poorly metabolized nitrogen source, urea, did not shorten or extend CLS (Santos et al., 2016).
Additionally, it was shown in synthetic media that as the concentration of nitrogen increased, glucose consumption also increased (Hess et al., 2006). As opposed to cells undergoing SICD where cells in glucose-only solutions show disruption of the Crabtree effect, cells in yeast nitrogen base (YNB) media undergo fermentation followed by respiration (Santos et al., 2016).
Transferring stationary phase cells of S. cerevisiae BY4742 to (NH4)2SO4 in water reduced cell survival by inducing apoptosis followed by secondary necrosis. In the first 2 days, evidence of apoptosis was observed—that is, ROS accumulation, DNA fragmentation, chromatin condensation, nuclear fragmentation, and externalization of phosphatidylserine in the absence of membrane permeabilization (Santos et al., 2012). Interestingly, after 2 days of incubation in water and (NH4)2SO4, cells stained positive for Annexin V, PI and Nhp6Ap. Nhp6Ap which is the yeast homolog of the human chromatin-bound non-histone protein HMGB1 (high mobility group box 1), which is known to release from the nucleus during necrosis, was translocated to the cytosol— suggesting a switch from apoptosis to secondary necrosis occurred (Santos et al., 2012). Deletion of AIF1 and YCA1, which encode the apoptosis-inducing factor and the only identified yeast metacaspase, respectively, did not prevent cell death (Santos et al., 2012). A later study also reported that a decrease in CFU was observed by 48 h when stationary phase cells of S. cerevisiae BY4741 were transferred to 0.5% of (NH4)2SO4 in water without any added glucose (Dušková et al., 2021). Together, these studies highlight the importance of a balance in nitrogen and carbon to ensure survival and growth of S. cerevisiae, as the absence or insufficiency of either can halt growth and trigger cell death (Figure 3).
In another study it was shown that intracellular ROS levels of S. cerevisiae in synthetic media supplemented with 2% glucose are much higher than that of cells in YPD media with 2% glucose. It was also shown that 10% glucose induced more rapid cell death in synthetic medium than in YPD medium suggesting that an imbalance between carbon and nitrogen can lead to cell death, as this imbalance will differ between synthetic medium and rich YPD medium (Weinberger et al., 2010). This conclusion is further supported by another study demonstrating a reduction in survival of S. cerevisiae cultured in both synthetic media and YPD, supplemented with 10% glucose in comparison to the standard 2% concentration. The loss in viability was shown to be associated with increased intracellular ROS and DNA damage, and interestingly, cell death was more rapid in synthetic medium than YPD medium (Weinberger et al., 2010). Therefore, even in a nutrient-rich environment, an imbalance of either nitrogen or carbon can trigger cell death.
A pathway of interest in unraveling the mechanism of SICD may be the target of rapamycin (TOR) signaling pathway, particularly TOR complex 1 (TORC1) kinase. TORC1 kinase is involved in sensing nutrients, stimulating growth, and regulating aging. TORCI is upregulated by nitrogen, amino acids, and glucose and is downregulated under nutrient-deprived conditions or treatment with rapamycin (Adachi et al., 2017; Iwama and Ohsumi, 2019; Foltman and Sanchez-Diaz, 2023). Glucose was shown to activate TORC1 at multiple stages in glycolysis, starting at phosphorylation to produce glucose 6-phosphate, formation of fructose 1,6-bisphosphate and completion of glycolysis (Alfatah et al., 2023; Deprez et al., 2018). Therefore, while nitrogen starvation under SICD conditions may downregulate TORC1 signaling, glucose phosphorylation may be acting in reverse to upregulate TORC1 kinase.
Inhibition of TORC1 by rapamycin and its downregulation by nutrient deprivation was shown to extend CLS, through the activation of autophagy. Autophagy is important for survival, as it not only removes toxic byproducts from the cell but also recycles nutrients under conditions of deprivation. Autophagy is known to extend CLS and promote survival, whereby an aberrant regulation of autophagy is associated with cell death and a diseased state (Adachi et al., 2017; Moruno et al., 2012; Farré and Subramani, 2016). Additionally, TORC1 upregulation inhibits autophagy through phosphorylation of Atg13, thereby preventing interaction with other Atg proteins, ultimately preventing the formation of the autophagosome, whereas downregulation induces autophagy as it allows for the hypophosphorylated state of Atg13 (Adachi et al., 2017; Moruno et al., 2012; Farré and Subramani, 2016). It is likely that glucose phosphorylation during SICD is upregulating TORC1 signaling which inhibits autophagy and consequently, cell death is activated. However, this is yet to be investigated under SICD conditions.
Although not denoted as SICD, a similar phenotype is seen in bacterial cells, described as substrate-accelerated death. This occurs when the substrate that limits growth of a population accelerates their death when they are starved and then transferred to the limiting substrate (Postgate and Hunter, 1963). This was described in several bacterial species including E. coli, Aerobacter aerogenes, and Serratia marcescens, whereby glucose, mannitol, and glycerol would induce death of cells starved from the respective substrate. Interestingly, cells starved from mannitol would also die when transferred to glucose (Postgate and Hunter, 1963), which one can argue is perhaps SICD.
Like yeast, mammalian cells also undergo cell death in response to intrinsic and extrinsic factors. Likewise, SICD may not be restricted to unicellular organisms (Figure 4), as similar cell death responses are seen in various mammalian cell types in response to high glucose (Table 2). This will be denoted as high glucose-induced cell death (HGICD). Glucose has been shown to induce cell death in several mammalian cell types, especially under conditions of diabetes and hyperglycemia. In this section, we discuss studies highlighting the presence of HGICD in normal cell lines and discuss events leading up to cell death after exposure to high glucose concentrations.
Figure 4. Response of different cell types to high glucose. Bacteria, yeast and normal mammalian cells undergo cell death in response to high glucose. However, cancer cells acquired the ability to bypass SICD in high glucose conditions and instead respond with increased proliferation. Lower concentrations of glucose favor proliferation in yeast and bacteria (given that nitrogen is present) and in normal mammalian cells. Lower glucose concentrations may also prevent cancer cell proliferation. Created in BioRender. Parbhudayal, R. (2025) https://BioRender.com/a51e042.
Table 2. Similarities and differences between high glucose-induced responses in Saccharomyces cerevisiae and mammalian cells.
Several in-vitro studies using human umbilical vein endothelial cells (HUVECs) showed that under conditions of uncontrolled diabetes, glucose induces the death of endothelial cells and decreases their growth by lowering the mitotic index (Lorenzi et al., 1985; Curcio and Ceriello, 1992; Ceriello et al., 1996; Detaille et al., 2005; McCaig et al., 2018). High glucose levels (25 mM) were shown to increase oxidative stress in endothelial cells which then upregulates the expression of antioxidant genes such as catalase, superoxide dismutase (SOD), and glutathione peroxide. However, this increased expression is not sufficient to prevent delayed cell replication. Endothelial cells transfected to express high levels of glutathione peroxide are not affected by elevated glucose levels, further confirming that the glucose-induced changes are associated with the production of ROS (Ceriello et al., 1996). Additionally, supplementation with antioxidants such as SOD, GSH, and catalase not only prevents delayed growth but can restore growth to levels seen in lower glucose concentrations (Curcio and Ceriello, 1992).
In a separate study, high ambient glucose (30 mM) was shown to induce apoptosis in HUVECs accompanied by DNA fragmentation (Baumgartner-Parzer et al., 1995). Apoptosis in HUVECs is associated with increased ROS generation and caspase-3 activation (Ho et al., 2000). H2O2 levels increased after the glucose concentration increased from 19 mM to 33 mM. Ascorbic acid (100 µM), an antioxidant, lowered ROS levels, and, prevented caspase-3 activation and apoptosis (Ho et al., 2000). High glucose was shown to induce apoptosis through c-Jun-NH2-terminal kinases (JNKs)/stress-activated protein kinases (SAPK), which can be activated by ROS and are implicated in several disease states due their function in regulating apoptosis. It was suggested that high glucose-induced ROS may activate SAPK/JNK, which then triggers caspase-3 activation leading to cell death (Ho et al., 2000).
Another study examined the effects of high glucose (30 mM) on phosphoinositol-3 kinase/protein kinase B PI3/Akt signaling pathway, an instrumental pathway regulating growth and proliferation, in HUVECs. It was shown that high glucose (30 mM) induced PI3/Akt-dependent production of ROS and upregulation of inducible cyclooxygenase-2 (COX-2) protein expression. This led to a corresponding increase in the release of Prostaglandin E2 (PGE2), increased caspase-3 activity, and apoptosis in HUVECs (Sheu et al., 2005). Inhibition of PI3K/Atk activity using inhibitors or by transfection, suppressed COX-2 expression, PGE2 generation, caspase-3 activation and cell death (Sheu et al., 2005).
Additionally, HGICD is not limited to HUVECs, high glucose (25 mM) also induces oxidative stress in mesangial cells by intracellularly decreasing GSH levels and reducing gene expression and protein levels of genes involved in GSH biosynthesis. This high glucose effect can be abolished by the addition of antioxidants such as Trolox and lipoic acid (Catherwood et al., 2002). High glucose (50 mM) also increased cell death by TNFα induction of apoptosis in U937 monocytes. This cell death was shown to be independent of caspases and interestingly, a shift from apoptosis to inflammatory RIP1 necroptosis was observed in U937 monocyte and Jurket T cells (McCaig et al., 2018). Interestingly, in the absence of RIP1, cell death resembles that of apoptosis and is dependent on caspases (McCaig et al., 2018).
Studies on retinal endothelial cells exposed to high glucose (30 mM) also revealed signs of cell damage and cell death. Lactate dehydrogenase (LDH) leakage was observed by 12 h of exposure to high glucose with a corresponding induction of apoptosis. TEM imaging revealed chromatin condensation, degranulation in the endoplasmic reticulum (ER), and vacuolization in the mitochondria of the retinal endothelial cells. There was also an increase in cleaved-caspase-3 and bax (pro-apoptotic) coupled with a decrease in bcl-2 (anti-apoptotic) protein levels, further confirming that cells underwent apoptosis. This was shown to be mediated via p53 activation as p53 protein levels were increased under high glucose exposure and p53 inhibition abolished the apoptotic phenotype seen under high glucose exposure (Li et al., 2018).
Interestingly, metformin prevented cell death and cytochrome c release induced by 30 mM glucose in HUVACs, human microvascular endothelial cells (HMECs), and bovine aortic endothelial cells (BAECs) (Detaille et al., 2005). It is worth noting that this glucose-induced phenotype is specific to D-glucose and cannot be induced by the non-metabolizable L-glucose or mannitol (Detaille et al., 2005; McCaig et al., 2018; Catherwood et al., 2002; Li et al., 2018).
Like yeast, high glucose/hyperglycemia results in inhibition of autophagy which favors cellular damage and a diseased state in mammalian cells. High glucose levels were shown to reduce autophagy in renal podocytes, leading to development of diabetic nephropathy while dietary restrictions protected against diabetic nephropathy through regulation of autophagy (Kitada et al., 2011; Zhao et al., 2023). Aberrant regulation of autophagy is also associated with diabetic retinopathy, through disruption of mTOR-dependent (mammalian TOR) autophagy, with diabetic cardiomyopathy, through the accumulation of defective organelles in the cardiomyocytes which contributes to the increased risk of cardiovascular diseases in people with diabetes, and with diabetic hepatopathy, due to an increase in triglyceride and lipid accumulation in hepatocytes (Zhao et al., 2023). Upregulation of autophagy is currently being studied as a potential target for therapeutics to treat metabolic diseases such as diabetes due to its cytoprotective effect (Gonzalez et al., 2011).
To summarize, glucose-induced cell death is a well-characterized phenomenon in mammalian cells due to its implications in diabetes, and in hyperglycemia associated pathophysiology. This phenomenon appears to be universal in mammalian cells as it was observed in several cell lines and shares similarities to that of yeast SICD. Like S. cerevisiae, HGICD in mammalian cells can also occur through multiple cell death pathways and is signaled by intracellular accumulation of ROS. The similarities between high glucose induced cell death in S. cerevisiae and mammalian cells (Table 2) enables S. cerevisiae to be used a model system to understand the seemingly conserved high glucose response in eukaryotic cells. Additionally, S. cerevisiae may be used as a model system for screening and testing new therapeutics against ROS accumulation, TORC1 signaling, autophagy and cell death, amongst other potential targets.
Cancer cells are known for their ability to increase glucose metabolism by increasing the rate of glycolysis (Hamanaka and Chandel, 2012). Interestingly, these cells can bypass this seemingly universal method of HGICD (Figure 4). Restoring HGICD in tumor cells may be an attractive mechanism to control proliferation of these cells. A phenomenon similar to the Crabtree effect, the Warburg effect, is a hallmark of cancer cells. The Warburg effect describes the ability of cancer cells to aerobically ferment glucose to lactate (aerobic glycolysis) rather than metabolizing glucose through respiration (Otto, 2016). Furthermore, as a eukaryote, S. cerevisiae shares many similarities with mammalian cells, hence why it is an excellent model system for cancer studies (Ferreira et al., 2019). In this section, we touch on the mechanism through which cancer cells may evade HGICD and the potential of HGICD being used in the design of new therapeutics.
The impact of hyperglycemia on cancer progression is particularly intriguing. Hyperglycemia was reported in several studies to increase tumor proliferation, invasion, migration and epidermal-to-mesenchymal transition (EMT) through multiple mechanisms (Li et al., 2019). For instance, pancreatic cancer cell lines increase proliferation with increased glucose concentrations of up to 50 mM. High glucose led to increased expression of epidermal growth factor (EGF), which is known to increase proliferation when expressed in cancer cells, and increased transactivation of the EGF receptor, (EGFR) (Han et al., 2011). High glucose also induces EGFR activation in breast cancer cells and may also inhibit EGFR degradation, resulting in increased cell proliferation (Hou et al., 2017). This is further supported by another study showing that high glucose in combination with insulin increases cell proliferation, migration, invasiveness, and increased levels of EMT markers through the increase in ROS in breast cancer cell lines (Flores-López et al., 2016). Interestingly, treatment with antioxidants can reduce the levels of ROS and its above listed consequences (Flores-López et al., 2016).
Glucose can also induce expression of chemoresistance markers and decrease expression of proteins being targeted by anti-cancer drugs in a gastric cancer cell line and breast cancer (Al Qahtani et al., 2017; Rozpȩdowska et al., 2011). More importantly, this response was also seen in patients with gastric cancer, hyperglycemia, and diabetes. Such patients are more likely to poorly respond to chemotherapy and experience decreased survival compared to patients with gastric cancer only (Zhao et al., 2015). In breast cancer, glucose at 25 mM led to increased expression of insulin-like growth factor binding protein 2 (IGFBP-2), whose expression is positively associated with tumor progression. IGBP-2 expression was also associated with chemoresistance of breast cancer cells in 25 mM glucose while hypoxia negate chemoresistance induced by high glucose and also the high glucose-induced IGBFP-2 expression (Al Qahtani et al., 2017).
In tumor cells, increased glycolysis was shown to be important, not so much for ATP production, but for biogenesis of cofactors, nucleic acids, and lipids required for proliferation (Hamanaka and Chandel, 2012; Reitzer et al., 1979). Tumor cells evade high glucose levels through the upregulation of several key genes in glycolysis and the evasion of feedback mechanisms. As previously reviewed (Hamanaka and Chandel, 2012; Lin et al., 2020; Sharma et al., 2019; Yamamoto et al., 1990; Wissing et al, 2004), cancer cells upregulate the expression of the high affinity glucose transporter, GLUT1, for enhanced glucose uptake (Yamamoto et al., 1990) and phosphofructokinase 2 (PFK-2) which converts fructose 6-phosphate to fructose 2,6-bisphosphate, an activator of PFK-1. PFK-1 converts fructose 6-phosphate to fructose 1,6-bisphosphate which allosterically inhibits PFK-1. PFK-1 can also be inhibited by high ATP levels, therefore the upregulation of PFK-2 allows for the maintenance of high glycolytic flux (Hamanaka and Chandel, 2012). Additionally, pyruvate kinase (PK) is differentially regulated to maintain higher expression of the slower isoform, PK-M2, allowing for phospho-enol pyruvate (PEP) to be converted to pyruvate in a non-ATP generating pathway. Pyruvate is then converted to lactate and NAD+ is generated (Hamanaka and Chandel, 2012). The activity of pyruvate dehydrogenase (PD) which converts pyruvate to acetyl-CoA is inhibited by upregulation of pyruvate dehydrogenase kinase (PDK) to favor lactate formation. Tumor cells also overexpress lactate dehydrogenase A (LDHA), which converts pyruvate into lactate and NADH to NAD+. LDHA overexpression allows for sufficient production of NAD+ for continued glycolytic flux. Metabolism is also modified to upregulate the pentose phosphate pathway (PPP) which generates NADPH for redox balance and ribose-5-phosphate for nucleic acid generation which was shown to be important for tumor progression, invasion, and metastasis (Lin et al., 2020; Sharma et al., 2019).
Cancer cells also display several mechanisms to evade cell death by upregulating pathways associated with protection against oxidative stress to combat the increased ROS levels (Sharma et al., 2019), including increased NADPH production (Lin et al., 2020; Sharma et al., 2019). Additionally, cancer cells upregulate the transcription factor erythroid 2-related factor 2 (NRF2) which is stabilized under conditions of oxidative stress. NRF2 drives the expression of key enzymes involved in GSH synthesis and regulates the levels of intracellular cysteine, which is required for GSH synthesis. Additionally, NRF2 regulates the utilization of GSH by controlling the expression of other ROS-detoxifying enzymes including glutathione peroxidase 2 (GPX2) and several glutathione S-transferases (McGrath-Morrow et al., 2009; Hu et al., 2006) (Figure 5).
Figure 5. Mechanism of cancer cell evasion of HGICD death through modulation of gene expression. In cancer cells, glucose uptake is increased through GLUT1 overexpression. Glucose is channeled into PPP for nucleotide synthesis and NADPH production, which aid in redox balance, which in turn aids in antioxidant defense. Aerobic glycolysis is upregulated through the inhibition of PDH by PDK, thereby blocking Acetyl Co-A synthesis, and through the upregulation of LDHA, which produces lactate. PFK-2 and PK-M2 are upregulated to increase glycolytic flux and there is downregulation of the TCA cycle. Glutamine uptake is also increased through upregulation of the ASCT2 transporter and glutaminolysis is upregulated to produce nitrogen and anaplerotic metabolites of the TCA cycle. GSH synthesis and utilization is regulated by NRF2. NRF2 regulates expression of GSTs and GPX2 to prevent ROS accumulation and cell death. Targets to reinstate SICD in cancer cells are presented in green. Created in BioRender. Parbhudayal, R. (2025) https://BioRender.com/x76i233.
Another important hallmark of cancer cells is glutaminolysis—that is the lysis of glutamine to produce glutamate in the mitochondria (Yang et al., 2017). In cancer cells, glutamine enters through the ACST2 transporter, which is overexpressed on the plasma membrane to fulfill the high glutamine demand of the cells (Yang et al., 2017; Lyu et al., 2023). Glutamine is transported to the mitochondria where glutaminase (GA) catalyzes the conversion of glutamine to glutamate which is then converted to AKG by glutamate dehydrogenase (GLUD) (Yang et al., 2017). As discussed above, glutamate is a precursor for GSH synthesis, which is important for coping with oxidative stress (Sharma et al., 2019; McGrath-Morrow et al., 2009; Hu et al., 2006; Yang et al., 2017). Moreover, glutamine is an important nutrient source for cancer cells as it was shown to be an excellent substrate for oxidative metabolism in tumor cells. It serves as a carbon backbone for TCA intermediates and other amino acids, and as a nitrogen donor for the synthesis of other amino acids and nucleotides (Reitzer et al., 1979; Yang et al., 2017) (Figure 5).
Taken together, the studies discussed in this section highlight the importance of glucose homeostasis to prevent tumor progression. The ability of cancer cells to bypass HGICD through various mechanisms highlights the robustness of these cell types and the importance of homeostasis. Increased ROS resistance and glutaminolysis may be two important pathways contributing to the evasion of HGICD by cancer cells. Glutaminolysis may be of particular importance in evading HGICD as it not only supplies anaplerotic metabolites but also supplies the cell with nitrogen.
It was hypothesized that SICD in S. cerevisiae occurs because of a disruption in the Crabtree effect (Lee et al., 2011a), therefore, if the Crabtree effect is maintained, then SICD might be prevented. In the case of cancer cells, glucose is metabolized through aerobic glycolysis, emphasizing the importance of the Warburg effect to sustain proliferation in cancer cells. Therefore, disruption of the Warburg effect may lead to the reestablishment of HGICD in cancer cells. It is possible that some therapeutics may inadvertently function to reinstate HGICD in cancer cells. Inhibitors targeting LDH activity have shown remarkable results, since reduction in LDH activity may favor mitochondrial pyruvate carriers to transport pyruvate to the mitochondria for oxidative phosphorylation as opposed to lactate generation. Reduction of LDHA activity was shown to decrease glycolytic flux, reduce NAD+ levels, increase ROS accumulation, and trigger cell death (Rai et al., 2020; Rai et al., 2017; Stine et al., 2022; Le et al., 2010; Gillies et al., 1981). LDH inhibitors gained attention from biotech companies for potential therapeutics, however, poor drug kinetics halted their development (Stine et al., 2022). Recently, it was discovered that pyrazole-based LDH inhibitors have improved pharmacokinetic properties and possess the ability to reduce glycolytic flux in cancer cells, leading to reduced NAD+ production and reduced cell proliferation in A673 sarcoma cells and pancreatic cancer cells, making them an attractive candidate for therapeutic development (Rai et al., 2020; Rai et al., 2017; Stine et al., 2022) through perhaps reinstating HGICD in cancer cells. Similarly, glutaminolysis inhibitors targeting ASCT2 transport, blocking uptake of glutamine, may devoid the cell of nitrogen and anaplerotic metabolites required for NADH production and trigger cell death. ASCT2 inhibition has been explored and proven to be a remarkable target for cancer therapeutic. Inhibition of ASCT2 in cancer cells resulted in decreased glutamine uptake, repression of mTORC1 (mammalian TORC1) signaling, and cell death (van Geldermalsen et al., 2016). Recently, C118P, a drug currently in clinical trials, was found to inhibit ASCT2 and demonstrated reduced glutamine uptake, thus reducing growth and proliferation of breast cancer cells with a corresponding increase in apoptosis (Lyu et al., 2023). While ASCT2 inhibition is an attractive target, inhibitors may have multiple targets, thereby diminishing their efficacy (Bröer et al., 2018; Jiang et al., 2020).
This paper is the first comprehensive review on SICD in yeast. We summarize the current understanding of SICD in yeast and its relation to mammalian cells in an attempt to understand the universal nature of SICD in eukaryotes. While the field of cell death in yeast is currently blooming, most studies are concentrated on acetic acid and H2O2-induced cell death. The presence of SICD in bacteria, yeast, and mammalian cells (HGICD) suggests that SICD may be a fundamental mechanism that is likely to be conserved across kingdoms. Significant work remains to be completed in order to determine the molecular mechanisms and genetic pathways involved in SICD in yeast.
To develop a comprehensive understanding of SICD in yeast, the method to induce SICD must be standardized. As discussed above, SICD studies are performed under a range of different temperatures, although David Granot initially reported that SICD is enhanced at 37οC. We propose to standardize temperature for SICD studies at 37οC, not only because it produces a more rapid cell death response, but to also maintain physiological relevance to mammalian cells. Additionally, transcriptomic analysis of S. cerevisiae exposed to glucose prior to induction of cell death may offer significant insights into the molecular mechanisms of SICD by uncovering genes and regulators involved in this process. While it is expected that glucose may impact numerous signaling pathways, it is important to understand at what stage of glucose metabolism cell death is triggered. Transcriptomics can be coupled with proteomic and metabolomic studies to further understand differences in metabolism under conditions of survival in comparison to SICD, in addition to assessing the possibility of secreted metabolites inducing cell death. Comparative transcriptomic and metabolomic analysis of SICD in stationary phase and exponential phase cells may unravel essential pathways that produce a delayed cell death response in stationary phase cells, adding invaluable insights towards potential mechanisms that prevent SICD.
Since mammalian cells undergo a similar cell death phenotype in response to high glucose, prevention of HGICD may be of paramount benefit to those with diabetes. Given the similarities between SICD in yeast and HGICD in mammalian cells, SICD pathways in S. cerevisiae may serve as a model for studies on high glucose-induced cell death in mammalian cell lines, allowing for rapid progression in the field. Unraveling the mechanism of SICD in S. cerevisiae by investigating how glycolysis, TCA cycle, oxidative phosphorylation, and the Crabtree effect is regulated followed by investigation of the events leading to cell death such as TORC1, PKA signaling, and autophagy, may be beneficial in establishing yeast as a model system to study hyperglycemia and diabetes.
The similarities between SICD in yeast and mammalian cells suggest pathways involved in these processes may be conserved in eukaryotes. Further investigation is needed to confirm conservation in bacterial cells, which may add to the novelty of this pathway as a target for therapeutics for metabolic diseases. Insights on the molecular mechanism of SICD in yeast and similarities shared with mammalian cells may facilitate the design of therapeutics targeting the prevention of HGICD in mammalian cells seen in cases of hyperglycemia and diabetes.
The ability of cancer cells to escape HGICD through metabolic reprogramming may provide us with essential information on nature’s way of evading HGICD in mammalian cells and SICD in yeast. Upregulation of glutamine uptake in cancer cell reprogramming highlights the importance of balance in carbon and nitrogen for cell survival and longevity. The indication of SICD being a fundamental and evolutionary conserved mechanism suggests that reinstatement of this pathway in cancer cells may be beneficial towards treating and preventing cancer progression. Together, the mechanism of SICD/HGICD may offer useful insights when designing therapeutics for cancer, hyperglycemia, and diabetes. Additionally, SICD in S. cerevisiae may be a valuable model to screen and discover new therapeutic targets and therapeutics, and also study mechanisms of action.
RP: Writing–review and editing, Writing–original draft. H-PC: Writing–review and editing, Conceptualization, Supervision.
The author(s) declare financial support was received for the research, authorship, and/or publication of this article. This work is supported in part by a grant (GM081147) to H-P.C. from the National Institutes of Health.
We thank Cheng lab members, especially Ertan Kastrat, for reading and editing this manuscript.
The authors declare that the research was conducted in the absence of any commercial or financial relationships that could be construed as a potential conflict of interest.
All claims expressed in this article are solely those of the authors and do not necessarily represent those of their affiliated organizations, or those of the publisher, the editors and the reviewers. Any product that may be evaluated in this article, or claim that may be made by its manufacturer, is not guaranteed or endorsed by the publisher.
Adachi, A., Koizumi, M., and Ohsumi, Y. (2017). Autophagy induction under carbon starvation conditions is negatively regulated by carbon catabolite repression. J. Biol. Chem. 292 (48), 19905–19918. doi:10.1074/jbc.M117.817510
Ahn, S. H., Cheung, W. L., Hsu, J. Y., Diaz, R. L., Smith, M. M., and Allis, C. D. (2005). Sterile 20 kinase phosphorylates histone H2B at serine 10 during hydrogen peroxide-induced apoptosis in S. cerevisiae. Cell. 120 (1), 25–36. doi:10.1016/j.cell.2004.11.016
Alby, K., Schaefer, D., Sherwood, R. K., Jones, S. K., and Bennett, R. J. (2010). Identification of a cell death pathway in Candida albicans during the response to pheromone. Eukaryot. Cell. 9 (11), 1690–1701. doi:10.1128/EC.00155-10
Alfatah, M., Cui, L., Goh, C. J. H., Cheng, T. Y. N., Zhang, Y., Naaz, A., et al. (2023). Metabolism of glucose activates TORC1 through multiple mechanisms in Saccharomyces cerevisiae. Cell. Rep. 42 (10), 113205. doi:10.1016/j.celrep.2023.113205
Al Qahtani, A., Holly, J., and Perks, C. (2017). Hypoxia negates hyperglycaemia-induced chemo-resistance in breast cancer cells: the role of insulin-like growth factor binding protein 2. Oncotarget 8 (43), 74635–74648. doi:10.18632/oncotarget.20287
Avtukh, A., Baskunov, B., Keshelava, V., and Valiakhmetov, A. (2023). Sugar-induced cell death in the yeast S. cerevisiae is accompanied by the release of octanoic acid, which does not originate from the fatty acid synthesis type II mitochondrial system. Appl. Microbiol. 3 (3), 722–734. doi:10.3390/applmicrobiol3030050
Baumgartner-Parzer, S. M., Wagner, L., Pettermann, M., Grillari, J., Gessl, A., and Waldhäusl, W. (1995). High-glucose--triggered apoptosis in cultured endothelial cells. Diabetes 44 (11), 1323–1327. doi:10.2337/diab.44.11.1323
Bidiuk, V. A., Alexandrov, A. I., and Valiakhmetov, A. Y. (2021). Extracellular pH and high concentration of potassium regulate the primary necrosis in the yeast Saccharomyces cerevisiae. Arch. Microbiol. 204 (1), 35. doi:10.1007/s00203-021-02708-6
Bröer, A., Fairweather, S., and Bröer, S. (2018). Disruption of amino acid homeostasis by novel ASCT2 inhibitors involves multiple targets. Front. Pharmacol. 9, 785. doi:10.3389/fphar.2018.00785
Büttner, S., Ruli, D., Vögtle, F. N., Galluzzi, L., Moitzi, B., Eisenberg, T., et al. (2011). A yeast BH3-only protein mediates the mitochondrial pathway of apoptosis. Embo J. 30 (14), 2779–2792. doi:10.1038/emboj.2011.197
Carmona-Gutierrez, D., Bauer, M. A., Zimmermann, A., Aguilera, A., Austriaco, N., Ayscough, K., et al. (2018). Guidelines and recommendations on yeast cell death nomenclature. Microb. Cell. 5 (1), 4–31. doi:10.15698/mic2018.01.607
Carmona-Gutierrez, D., Eisenberg, T., Büttner, S., Meisinger, C., Kroemer, G., and Madeo, F. (2010). Apoptosis in yeast: triggers, pathways, subroutines. Cell. Death Differ. 17 (5), 763–773. doi:10.1038/cdd.2009.219
Catherwood, M. A., Powell, L. A., Anderson, P., McMaster, D., Sharpe, P. C., and Trimble, E. R. (2002). Glucose-induced oxidative stress in mesangial cells. Kidney Int. 61 (2), 599–608. doi:10.1046/j.1523-1755.2002.00168.x
Ceriello, A., dello Russo, P., Amstad, P., and Cerutti, P. (1996). High glucose induces antioxidant enzymes in human endothelial cells in culture. Evidence linking hyperglycemia and oxidative stress. Diabetes 45 (4), 471–477. doi:10.2337/diab.45.4.471
Chaves, S. R., Rego, A., Martins, V. M., Santos-Pereira, C., Sousa, M. J., and Côrte-Real, M. (2021). Regulation of cell death induced by acetic acid in yeasts. Front. Cell. Dev. Biol. 9, 642375. doi:10.3389/fcell.2021.642375
Chidawanyika, T., and Supattapone, S. (2021). Hydrogen peroxide-induced cell death in mammalian cells. J. Cell. Signal 2 (3), 206–211. doi:10.33696/signaling.2.052
Curcio, F., and Ceriello, A. (1992). Decreased cultured endothelial cell proliferation in high glucose medium is reversed by antioxidants: new insights on the pathophysiological mechanisms of diabetic vascular complications. Vitro Cell. Dev. Biol. 28a (11-12), 787–790. doi:10.1007/BF02631069
Deprez, M.-A., Eskes, E., Winderickx, J., and Wilms, T. (2018). The TORC1-Sch9 pathway as a crucial mediator of chronological lifespan in the yeast Saccharomyces cerevisiae. FEMS Yeast Res. 18 (5). doi:10.1093/femsyr/foy048
Detaille, D., Guigas, B., Chauvin, C., Batandier, C., Fontaine, E., Wiernsperger, N., et al. (2005). Metformin prevents high-glucose-induced endothelial cell death through a mitochondrial permeability transition-dependent process. Diabetes 54 (7), 2179–2187. doi:10.2337/diabetes.54.7.2179
Díaz-Ruiz, R., Avéret, N., Araiza, D., Pinson, B., Uribe-Carvajal, S., Devin, A., et al. (2008). Mitochondrial oxidative phosphorylation is regulated by fructose 1,6-bisphosphate: a possible role in crabtree effect induction? J. Biol. Chem. 283 (40), 26948–26955. doi:10.1074/jbc.M800408200
Du, H., Guan, G., Li, X., Gulati, M., Tao, L., Cao, C., et al. (2015). N-Acetylglucosamine-Induced cell death in Candida albicans and its implications for adaptive mechanisms of nutrient sensing in yeasts. mBio 6 (5), 013766–e1415. doi:10.1128/mBio.01376-15
Du, H., and Liang, Y. (2006). Saccharomyces cerevisiae ste20 mutant showing resistance to glucose-induced cell death. Yi Chuan Xue Bao 33 (7), 664–668. doi:10.1016/S0379-4172(06)60097-8
Dušková, M., Cmunt, D., Papoušková, K., Masaryk, J., and Sychrová, H. (2021). Minority potassium-uptake system Trk2 has a crucial role in yeast survival of glucose-induced cell death. Microbiology 167 (6). doi:10.1099/mic.0.001065
Eisenberg, T., Carmona-Gutierrez, D., Büttner, S., Tavernarakis, N., and Madeo, F. (2010). Necrosis in yeast. Apoptosis 15 (3), 257–268. doi:10.1007/s10495-009-0453-4
Falcone, C., and Mazzoni, C. (2016). External and internal triggers of cell death in yeast. Cell. Mol. Life Sci. 73 (11-12), 2237–2250. doi:10.1007/s00018-016-2197-y
Farré, J.-C., and Subramani, S. (2016). Mechanistic insights into selective autophagy pathways: lessons from yeast. Nat. Rev. Mol. Cell. Biol. 17 (9), 537–552. doi:10.1038/nrm.2016.74
Ferreira, R., Limeta, A., and Nielsen, J. (2019). Tackling cancer with yeast-based technologies. Trends Biotechnol. 37 (6), 592–603. doi:10.1016/j.tibtech.2018.11.013
Flores-López, L. A., Martínez-Hernández, M. G., Viedma-Rodríguez, R., Díaz-Flores, M., and Baiza-Gutman, L. A. (2016). High glucose and insulin enhance uPA expression, ROS formation and invasiveness in breast cancer-derived cells. Cell. Oncol. 39 (4), 365–378. doi:10.1007/s13402-016-0282-8
Foltman, M., and Sanchez-Diaz, A. (2023). TOR complex 1: orchestrating nutrient signaling and cell cycle progression. Int. J. Mol. Sci. 24 (21), 15745. doi:10.3390/ijms242115745
Galdieri, L., Mehrotra, S., Yu, S., and Vancura, A. (2010). Transcriptional regulation in yeast during diauxic shift and stationary phase. Omics 14 (6), 629–638. doi:10.1089/omi.2010.0069
Giannattasio, S., Guaragnella, N., Zdralević, M., and Marra, E. (2013). Molecular mechanisms of Saccharomyces cerevisiae stress adaptation and programmed cell death in response to acetic acid. Front. Microbiol. 4, 33. doi:10.3389/fmicb.2013.00033
Gillies, R. J., Ugurbil, K., den Hollander, J. A., and Shulman, R. G. (1981). 31P NMR studies of intracellular pH and phosphate metabolism during cell division cycle of Saccharomyces cerevisiae. Proc. Natl. Acad. Sci. U. S. A. 78 (4), 2125–2129. doi:10.1073/pnas.78.4.2125
Gonzalez, C. D., Lee, M. S., Marchetti, P., Pietropaolo, M., Towns, R., Vaccaro, M. I., et al. (2011). The emerging role of autophagy in the pathophysiology of diabetes mellitus. Autophagy 7 (1), 2–11. doi:10.4161/auto.7.1.13044
Granot, D., and Dai, N. (1997). Sugar induced cell death in yeast is dependent on the rate of sugar phosphorylation as determined by Arabidopsis thaliana hexokinase. Cell. Death Differ. 4 (7), 555–559. doi:10.1038/sj.cdd.4400280
Granot, D., Levine, A., and Dor-Hefetz, E. (2003). Sugar-induced apoptosis in yeast cells. FEMS Yeast Res. 4 (1), 7–13. doi:10.1016/S1567-1356(03)00154-5
Granot, D., and Snyder, M. (1991). Glucose induces cAMP-independent growth-related changes in stationary-phase cells of Saccharomyces cerevisiae. Proc. Natl. Acad. Sci. U. S. A. 88 (13), 5724–5728. doi:10.1073/pnas.88.13.5724
Granot, D., and Snyder, M. (1993). Carbon source induces growth of stationary phase yeast cells, independent of carbon source metabolism. Yeast 9 (5), 465–479. doi:10.1002/yea.320090503
Guaragnella, N., Antonacci, L., Passarella, S., Marra, E., and Giannattasio, S. (2007). Hydrogen peroxide and superoxide anion production during acetic acid-induced yeast programmed cell death. Folia Microbiol. (Praha) 52 (3), 237–240. doi:10.1007/BF02931304
Hamanaka, R. B., and Chandel, N. S. (2012). Targeting glucose metabolism for cancer therapy. J. Exp. Med. 209 (2), 211–215. doi:10.1084/jem.20120162
Han, L., Ma, Q., Li, J., Liu, H., Li, W., Ma, G., et al. (2011). High glucose promotes pancreatic cancer cell proliferation via the induction of EGF expression and transactivation of EGFR. PLOS ONE 6 (11), e27074. doi:10.1371/journal.pone.0027074
Hauptmann, P., and Lehle, L. (2008). Kex1 protease is involved in yeast cell death induced by defective N-glycosylation, acetic acid, and chronological aging. J. Biol. Chem. 283 (27), 19151–19163. doi:10.1074/jbc.M801303200
Herrero, P., Flores, L., de la Cera, T., and Moreno, F. (1999). Functional characterization of transcriptional regulatory elements in the upstream region of the yeast GLK1 gene. Biochem. J., 343 (2): p. 319–325. doi:10.1042/bj3430319
Hess, D. C., Lu, W., Rabinowitz, J. D., and Botstein, D. (2006). Ammonium toxicity and potassium limitation in yeast. PLoS Biol. 4 (11), e351. doi:10.1371/journal.pbio.0040351
Ho, F. M., Liu, S. H., Liau, C. S., Huang, P. J., and Lin-Shiau, S. Y. (2000). High glucose-induced apoptosis in human endothelial cells is mediated by sequential activations of c-Jun NH(2)-terminal kinase and caspase-32</sub> -Terminal Kinase and Caspase-3. Circulation, 101(22): p. 2618–2624. doi:10.1161/01.cir.101.22.2618
Hoeberichts, F. A., Perez-Valle, J., Montesinos, C., Mulet, J. M., Planes, M. D., Hueso, G., et al. (2010). The role of K+ and H+ transport systems during glucose- and H2O2-induced cell death in Saccharomyces cerevisiae. Yeast 27 (9), 713–725. doi:10.1002/yea.1767
Hou, Y., Zhou, M., Xie, J., Chao, P., Feng, Q., and Wu, J. (2017). High glucose levels promote the proliferation of breast cancer cells through GTPases. Breast Cancer (Dove Med. Press) 9, 429–436. doi:10.2147/BCTT.S135665
Hu, R., Xu, C., Shen, G., Jain, M. R., Khor, T. O., Gopalkrishnan, A., et al. (2006). Identification of Nrf2-regulated genes induced by chemopreventive isothiocyanate PEITC by oligonucleotide microarray. Life Sci. 79 (20), 1944–1955. doi:10.1016/j.lfs.2006.06.019
Iwama, R., and Ohsumi, Y. (2019). Analysis of autophagy activated during changes in carbon source availability in yeast cells. J. Biol. Chem. 294 (14), 5590–5603. doi:10.1074/jbc.RA118.005698
Jiang, H., Zhang, N., Tang, T., Feng, F., Sun, H., and Qu, W. (2020). Target the human alanine/serine/cysteine transporter 2(ASCT2): achievement and future for novel cancer therapy. Pharmacol. Res. 158, 104844. doi:10.1016/j.phrs.2020.104844
Kitada, M., Takeda, A., Nagai, T., Ito, H., Kanasaki, K., and Koya, D. (2011). Dietary restriction ameliorates diabetic nephropathy through anti-inflammatory effects and regulation of the autophagy via restoration of Sirt1 in diabetic wistar fatty (fa/fa) rats: a model of type 2 diabetes. Exp. Diabetes Res. 2011, 908185–908211. doi:10.1155/2011/908185
Kotyk, A., Lapathitis, G., and Krenkova, S. (1999). Glucose- and K+-induced acidification in different yeast species. Folia Microbiol. 44 (3), 295–298. doi:10.1007/BF02818550
Le, A., Cooper, C. R., Gouw, A. M., Dinavahi, R., Maitra, A., Deck, L. M., et al. (2010). Inhibition of lactate dehydrogenase A induces oxidative stress and inhibits tumor progression. Proc. Natl. Acad. Sci. U. S. A. 107 (5), 2037–2042. doi:10.1073/pnas.0914433107
Leadsham, J. E., Sanders, G., Giannaki, S., Bastow, E. L., Hutton, R., Naeimi, W. R., et al. (2013). Loss of cytochrome c oxidase promotes RAS-dependent ROS production from the ER resident NADPH oxidase, Yno1p, in yeast. Cell. Metab. 18 (2), 279–286. doi:10.1016/j.cmet.2013.07.005
Lee, Y. J., Burlet, E., Galiano, F., Circu, M. L., Aw, T. Y., Williams, B. J., et al. (2011a). Phosphate and succinate use different mechanisms to inhibit sugar-induced cell death in yeast: insight into the Crabtree effect. J. Biol. Chem. 286 (23), 20267–20274. doi:10.1074/jbc.M110.209379
Lee, Y. J., Burlet, E., Wang, S., Xu, B., Huang, S., Galiano, F. J., et al. (2011b). Triclabendazole protects yeast and mammalian cells from oxidative stress: identification of a potential neuroprotective compound. Biochem. Biophys. Res. Commun. 414 (1), 205–208. doi:10.1016/j.bbrc.2011.09.057
Lee, Y. J., Shi, R., and Witt, S. N. (2013). The small molecule triclabendazole decreases the intracellular level of cyclic AMP and increases resistance to stress in Saccharomyces cerevisiae. PLoS ONE 8 (5), e64337. doi:10.1371/journal.pone.0064337
Li, Q., Pang, L., Shi, H., Yang, W., Liu, X., Su, G., et al. (2018). High glucose concentration induces retinal endothelial cell apoptosis by activating p53 signaling pathway. Int. J. Clin. Exp. Pathol. 11 (5), 2401–2407.
Li, W., Zhang, X., Sang, H., Zhou, Y., Shang, C., Wang, Y., et al. (2019). Effects of hyperglycemia on the progression of tumor diseases. J. Exp. and Clin. Cancer Res. 38 (1), 327. doi:10.1186/s13046-019-1309-6
Liang, H., Ko, C. H., Herman, T., and Gaber, R. F. (1998). Trinucleotide insertions, deletions, and point mutations in glucose transporters confer K+ uptake in Saccharomyces cerevisiae. Mol. Cell. Biol. 18 (2), 926–935. doi:10.1128/mcb.18.2.926
Lin, X., Xiao, Z., Chen, T., Liang, S. H., and Guo, H. (2020). Glucose metabolism on tumor plasticity, diagnosis, and treatment. Front. Oncol. 10, 317. doi:10.3389/fonc.2020.00317
Lodi, T., and Donnini, C. (2005). Lactose-induced cell death of beta-galactosidase mutants in Kluyveromyces lactis. FEMS Yeast Res. 5 (8), 727–734. doi:10.1016/j.femsyr.2005.01.005
Lorenzi, M., Cagliero, E., and Toledo, S. (1985). Glucose toxicity for human endothelial cells in culture. Delayed replication, disturbed cell cycle, and accelerated death. Diabetes 34 (7), 621–627. doi:10.2337/diab.34.7.621
Lyu, X. D., Liu, Y., Wang, J., Wei, Y. C., Han, Y., Li, X., et al. (2023). A novel ASCT2 inhibitor, C118P, blocks glutamine transport and exhibits antitumour efficacy in breast cancer. Cancers (Basel) 15 (20), 5082. doi:10.3390/cancers15205082
Madeo, F., Carmona-Gutierrez, D., Ring, J., Büttner, S., Eisenberg, T., and Kroemer, G. (2009). Caspase-dependent and caspase-independent cell death pathways in yeast. Biochem. Biophys. Res. Commun. 382 (2), 227–231. doi:10.1016/j.bbrc.2009.02.117
Madeo, F., Fröhlich, E., Ligr, M., Grey, M., Sigrist, S. J., Wolf, D. H., et al. (1999). Oxygen stress: a regulator of apoptosis in yeast. J. Cell. Biol. 145 (4), 757–767. doi:10.1083/jcb.145.4.757
Madeo, F., Herker, E., Maldener, C., Wissing, S., Lächelt, S., Herlan, M., et al. (2002). A caspase-related protease regulates apoptosis in yeast. Mol. Cell. 9 (4), 911–917. doi:10.1016/s1097-2765(02)00501-4
Magasanik, B., and Kaiser, C. A. (2002). Nitrogen regulation in Saccharomyces cerevisiae. Gene 290 (1-2), 1–18. doi:10.1016/s0378-1119(02)00558-9
Malina, C., Yu, R., Björkeroth, J., Kerkhoven, E. J., and Nielsen, J. (2021). Adaptations in metabolism and protein translation give rise to the Crabtree effect in yeast. Proc. Natl. Acad. Sci. 118 (51), e2112836118. doi:10.1073/pnas.2112836118
McCaig, W. D., Patel, P. S., Sosunov, S. A., Shakerley, N. L., Smiraglia, T. A., Craft, M. M., et al. (2018). Hyperglycemia potentiates a shift from apoptosis to RIP1-dependent necroptosis. Cell. Death Discov. 4, 55. doi:10.1038/s41420-018-0058-1
McGrath-Morrow, S., Lauer, T., Yee, M., Neptune, E., Podowski, M., Thimmulappa, R. K., et al. (2009). Nrf2 increases survival and attenuates alveolar growth inhibition in neonatal mice exposed to hyperoxia. Am. J. Physiol. Lung Cell. Mol. Physiol. 296 (4), L565–L573. doi:10.1152/ajplung.90487.2008
Miller, K. P., Gowtham, Y. K., Henson, J. M., and Harcum, S. W. (2012). Xylose isomerase improves growth and ethanol production rates from biomass sugars for both Saccharomyces pastorianus and Saccharomyces cerevisiae. Biotechnol. Prog. 28 (3), 669–680. doi:10.1002/btpr.1535
Moruno, F., Pérez-Jiménez, E., and Knecht, E. (2012). Regulation of autophagy by glucose in Mammalian cells. Cells 1 (3), 372–395. doi:10.3390/cells1030372
Nishimura, A. (2024). Regulations and functions of proline utilization in yeast Saccharomyces cerevisiae. Biosci. Biotechnol. Biochem. 88 (2), 131–137. doi:10.1093/bbb/zbad165
Otto, A. M. (2016). Warburg effect(s)—a biographical sketch of Otto Warburg and his impacts on tumor metabolism. Cancer and Metabolism 4 (1), 5. doi:10.1186/s40170-016-0145-9
Park, J. I., Grant, C. M., and Dawes, I. W. (2005). The high-affinity cAMP phosphodiesterase of Saccharomyces cerevisiae is the major determinant of cAMP levels in stationary phase: involvement of different branches of the Ras-cyclic AMP pathway in stress responses. Biochem. Biophys. Res. Commun. 327 (1), 311–319. doi:10.1016/j.bbrc.2004.12.019
Postgate, J. R., and Hunter, J. R. (1963). Acceleration of bacterial death by grown substrates. Nature 198 (4877), 273. doi:10.1038/198273a0
Rai, G., Brimacombe, K. R., Mott, B. T., Urban, D. J., Hu, X., Yang, S. M., et al. (2017). Discovery and optimization of potent, cell-active pyrazole-based inhibitors of lactate dehydrogenase (LDH). J. Med. Chem. 60 (22), 9184–9204. doi:10.1021/acs.jmedchem.7b00941
Rai, G., Urban, D. J., Mott, B. T., Hu, X., Yang, S. M., Benavides, G. A., et al. (2020). Pyrazole-based lactate dehydrogenase inhibitors with optimized cell activity and pharmacokinetic properties. J. Med. Chem. 63 (19), 10984–11011. doi:10.1021/acs.jmedchem.0c00916
Reitzer, L. J., Wice, B. M., and Kennell, D. (1979). Evidence that glutamine, not sugar, is the major energy source for cultured HeLa cells. J. Biol. Chem. 254 (8), 2669–2676. doi:10.1016/s0021-9258(17)30124-2
Rinnerthaler, M., Büttner, S., Laun, P., Heeren, G., Felder, T. K., Klinger, H., et al. (2012). Yno1p/Aim14p, a NADPH-oxidase ortholog, controls extramitochondrial reactive oxygen species generation, apoptosis, and actin cable formation in yeast. Proc. Natl. Acad. Sci. U. S. A. 109 (22), 8658–8663. doi:10.1073/pnas.1201629109
Rødkær, S. V., and Færgeman, N. J. (2014). Glucose- and nitrogen sensing and regulatory mechanisms in Saccharomyces cerevisiae. FEMS Yeast Res. 14 (5), 683–696. doi:10.1111/1567-1364.12157
Rolland, F., Winderickx, J., and Thevelein, J. M. (2002). Glucose-sensing and -signalling mechanisms in yeast. FEMS Yeast Res. 2 (2), 183–201. doi:10.1111/j.1567-1364.2002.tb00084.x
Rozpȩdowska, E., Galafassi, S., Johansson, L., Hagman, A., Piškur, J., and Compagno, C. (2011). Candida albicans-a pre-whole genome duplication yeast - is predominantly aerobic and a poor ethanol producer. FEMS Yeast Res. 11 (3), 285–291. doi:10.1111/j.1567-1364.2010.00715.x
Ruckenstuhl, C., Carmona-Gutierrez, D., and Madeo, F. (2010). The sweet taste of death: glucose triggers apoptosis during yeast chronological aging. Aging (Albany NY) 2 (10), 643–649. doi:10.18632/aging.100223
Santos, J., Leitão-Correia, F., Sousa, M. J., and Leão, C. (2016). Nitrogen and carbon source balance determines longevity, independently of fermentative or respiratory metabolism in the yeast Saccharomyces cerevisiae. Oncotarget 7 (17), 23033–23042. doi:10.18632/oncotarget.8656
Santos, J., Sousa, M. J., and Leão, C. (2012). Ammonium is toxic for aging yeast cells, inducing death and shortening of the chronological lifespan. PLoS ONE 7 (5), e37090. doi:10.1371/journal.pone.0037090
Serrano, R. (1983). In vivo glucose activation of the yeast plasma membrane ATPase. FEBS Lett. 156 (1), 11–14. doi:10.1016/0014-5793(83)80237-3
Severin, F. F., and Hyman, A. A. (2002). Pheromone induces programmed cell death in S. cerevisiae. Curr. Biol. 12 (7), R233–R235. doi:10.1016/s0960-9822(02)00776-5
Sharma, A., Boise, L. H., and Shanmugam, M. (2019). Cancer metabolism and the evasion of apoptotic cell death. Cancers (Basel) 11 (8), 1144. doi:10.3390/cancers11081144
Sheu, M. L., Ho, F. M., Yang, R. S., Chao, K. F., Lin, W. W., Lin-Shiau, S. Y., et al. (2005). High glucose induces human endothelial cell apoptosis through a phosphoinositide 3-kinase–regulated cyclooxygenase-2 pathway. Arteriosclerosis, Thrombosis, Vasc. Biol. 25 (3), 539–545. doi:10.1161/01.ATV.0000155462.24263.e4
Stine, Z. E., Schug, Z. T., Salvino, J. M., and Dang, C. V. (2022). Targeting cancer metabolism in the era of precision oncology. Nat. Rev. Drug Discov. 21 (2), 141–162. doi:10.1038/s41573-021-00339-6
Stolp, Z. D., Kulkarni, M., Liu, Y., Zhu, C., Jalisi, A., Lin, S., et al. (2022). Yeast cell death pathway requiring AP-3 vesicle trafficking leads to vacuole/lysosome membrane permeabilization. Cell. Rep. 39 (2), 110647. doi:10.1016/j.celrep.2022.110647
Tamaki, H. (2007). Glucose-stimulated cAMP-protein kinase A pathway in yeast Saccharomyces cerevisiae. J. Biosci. Bioeng. 104 (4), 245–250. doi:10.1263/jbb.104.245
Valiakhmetov, A. Y., Kuchin, A. V., Suzina, N. E., Zvonarev, A. N., and Shepelyakovskaya, A. O. (2019). Glucose causes primary necrosis in exponentially grown yeast Saccharomyces cerevisiae. FEMS Yeast Res. 19 (3), foz019. doi:10.1093/femsyr/foz019
van Geldermalsen, M., Wang, Q., Nagarajah, R., Marshall, A. D., Thoeng, A., Gao, D., et al. (2016). ASCT2/SLC1A5 controls glutamine uptake and tumour growth in triple-negative basal-like breast cancer. Oncogene 35 (24), 3201–3208. doi:10.1038/onc.2015.381
Weinberger, M., Mesquita, A., Caroll, T., Marks, L., Yang, H., Zhang, Z., et al. (2010). Growth signaling promotes chronological aging in budding yeast by inducing superoxide anions that inhibit quiescence. Aging (Albany NY) 2 (10), 709–726. doi:10.18632/aging.100215
Wissing, S., Ludovico, P., Herker, E., Büttner, S., Engelhardt, S. M., Decker, T., et al. (2004). An AIF orthologue regulates apoptosis in yeast. J. Cell. Biol. 166 (7), 969–974. doi:10.1083/jcb.200404138
Wu, Z., Song, L., Liu, S. Q., and Huang, D. (2013). Independent and additive effects of glutamic acid and methionine on yeast longevity. PLoS One 8 (11), e79319. doi:10.1371/journal.pone.0079319
Yamamoto, T., Seino, Y., Fukumoto, H., Koh, G., Yano, H., Inagaki, N., et al. (1990). Over-expression of facilitative glucose transporter genes in human cancer. Biochem. biophysical Res. Commun. 170 (1), 223–230. doi:10.1016/0006-291x(90)91263-r
Yang, L., Venneti, S., and Nagrath, D. (2017). Glutaminolysis: a hallmark of cancer metabolism. Annu. Rev. Biomed. Eng. 19, 163–194. doi:10.1146/annurev-bioeng-071516-044546
Yi, D.-G., Hong, S., and Huh, W.-K. (2018). Mitochondrial dysfunction reduces yeast replicative lifespan by elevating RAS-dependent ROS production by the ER-localized NADPH oxidase Yno1. PLOS ONE 13 (6), e0198619. doi:10.1371/journal.pone.0198619
Yoboue, E. D., Sitia, R., and Simmen, T. (2018). Redox crosstalk at endoplasmic reticulum (ER) membrane contact sites (MCS) uses toxic waste to deliver messages. Cell. Death and Dis. 9 (3), 331. doi:10.1038/s41419-017-0033-4
Yoshimoto, H., Ohuchi, R., Ikado, K., Yoshida, S., Minato, T., Ishiguro, T., et al. (2009). Sugar induces death of the bottom fermenting yeast Saccharomyces pastorianus. J. Biosci. Bioeng. 108 (1), 60–62. doi:10.1016/j.jbiosc.2008.12.022
Zemlianski, V., Marešová, A., Princová, J., Holič, R., Häsler, R., Ramos Del Río, M. J., et al. (2024). Nitrogen availability is important for preventing catastrophic mitosis in fission yeast. J. Cell. Sci. 137 (12), jcs262196. doi:10.1242/jcs.262196
Zhang, N.-N., Dudgeon, D. D., Paliwal, S., Levchenko, A., Grote, E., and Cunningham, K. W. (2006). Multiple signaling pathways regulate yeast cell death during the response to mating pheromones. Mol. Biol. Cell. 17 (8), 3409–3422. doi:10.1091/mbc.e06-03-0177
Zhao, W., Chen, R., Zhao, M., Li, L., Fan, L., and Che, X. M. (2015). High glucose promotes gastric cancer chemoresistance in vivo and in vitro. Mol. Med. Rep. 12 (1), 843–850. doi:10.3892/mmr.2015.3522
Keywords: sugar induced death, hyperglycemia, glucose metabolism, regulated cell death, yeast programmed cell death, necrosis, diabetes, cancer cell glucose uptake and metabolism
Citation: Parbhudayal R and Cheng H-P (2025) Exploring sugar-induced cell death in yeast: implications for diabetes and cancer research. Front. Cell Death 4:1470093. doi: 10.3389/fceld.2025.1470093
Received: 25 July 2024; Accepted: 27 February 2025;
Published: 19 March 2025.
Edited by:
Susana Chaves, University of Minho, PortugalReviewed by:
Jia Li, University of North Carolina at Charlotte, United StatesCopyright © 2025 Parbhudayal and Cheng. This is an open-access article distributed under the terms of the Creative Commons Attribution License (CC BY). The use, distribution or reproduction in other forums is permitted, provided the original author(s) and the copyright owner(s) are credited and that the original publication in this journal is cited, in accordance with accepted academic practice. No use, distribution or reproduction is permitted which does not comply with these terms.
*Correspondence: Hai-Ping Cheng, aGFpcGluZy5jaGVuZ0BsZWhtYW4uY3VueS5lZHU=
Disclaimer: All claims expressed in this article are solely those of the authors and do not necessarily represent those of their affiliated organizations, or those of the publisher, the editors and the reviewers. Any product that may be evaluated in this article or claim that may be made by its manufacturer is not guaranteed or endorsed by the publisher.
Research integrity at Frontiers
Learn more about the work of our research integrity team to safeguard the quality of each article we publish.