- Department of Biosciences, Biotechnologies and Environment - University of Bari “A. Moro”, Bari, Italy
Mitochondria are multifaceted organelles acting as energy, metabolic and signaling hubs in the cells. They play a central role in biological processes aimed at maintaining cell homeostasis and regulating cell fate upon changing environments. Alterations in mitochondrial functions can affect cell stress response through different mechanisms, leading to adaptation or death. In this perspective, we focus on mitochondrial communication and its relevance for cytoprotective strategies aimed at controlling synthesis, degradation and recycling processes. The advantage of using yeast as a model organism for improving our understanding of the molecular mechanisms behind cell stress responses to mitochondrial dysfunction is described. New challenges for studying the interplay between mitochondrial retrograde signaling and autophagy/mitophagy pathways are highlighted.
1 Introduction
Mitochondria play a multifaceted role in a variety of cellular processes controlling homeostasis and cell fate upon physiological and non-physiological conditions. They firstly act as bioenergetics powerhouses through the electron transport chain (ETC) and the oxidative phosphorylation (OXPHOS) by providing the largest source of cellular ATP upon aerobic conditions. Their bioenergetic role is strictly connected to cell metabolism and mitochondria behave as anabolic centres for the synthesis of Tricarboxylic acid (TCA) cycle intermediates, amino acids, fatty acids, nucleotides, cholesterol, glucose and heme. At this regard, mitochondrial carriers (MCs), belonging to the superfamily of transporters named the MCF, or in mammals SLC25 (solute carrier family 25), shuttle a variety of metabolites across the inner mitochondrial membrane (IMM) to ensure proper trafficking for inter-organelle communication and the mainteinance of REDOX homeostasis (Passarella et al., 2021; Palmieri et al., 2020; Gorgoglione et al., 2019; Todisco et al., 2016). Mitochondria are also essential for the clearance of metabolic by-products, such as reactive oxygen species (ROS) or ammonia, which need to be re-purposed (Spinelli and Haigis, 2018). Alterations in these crucial functions of mitochondria compromise cell homeostasis and underlie the basis for the occurrence of pathological processes. At this regard, the role of mitochondria as signaling hubs at cellular and organismal level is emerging as a relevant aspect in biology and biomedical sciences (Stoolman et al., 2022). The mitochondrial communication with other cellular compartments offers a new perspective for the comprehension of mechanistic clues guiding cellular stress response upon aging, cell transformation and progression, metabolic disease, innate immunity, inflammation and bacterial/viral infections (Jin et al., 2019; 52 (1): 13–23; Ippolito et al., 2020; Nakhle et al., 2020; Fontana and Limonta, 2021; Newman and Shadel, 2023; Kim et al., 2024; Son and Lee, 2019). Therefore, mitochondria have been recognized as the processor of the cell and, together with the other compartments, constitute the mitochondrial information processing system (MIPS). MIPS is able to sense and integrate endogenous and environmental inputs and transduce the signals into adaptive responses (Picard and Shirihai, 2022).
1.1 Mitochondrial communication
In the cellular communication framework, mitochondria sequentially act as sensors, integrators and transducers of the molecular and non-molecular inputs transformed into an output response aligned with the energetic and metabolic states of the whole cellular system. The interaction between mitochondria and other cellular compartments plays a major role in the process of signal integration and can occur at different levels, including mito-nuclear communication, intra-organellar and inter-organellar-communication. The mito-nuclear communication include the anterograde pathway, from the nucleus to the mitochondria, through which nucleus controls the proteins and information transmitted to the mitochondria and the retrograde pathway, from the mitochondria to the nucleus, commonly activated by mitochondrial dysfunctions, such as loss of mitochondrial DNA or, ETC impairment (Butow and Avadhani, 2004; Lu et al., 2009). These pathways coordinate the communication between mitochondria and the nucleus during homeostasis and mitochondrial stress by activating a transcriptional response which modulates the expression of specific genes (Quirós et al., 2016).
The mitochondrial interactions with other cell compartments are also affected by fusion and fission events of the IMM and outer mitochondrial membrane (OMM) that play a crucial role in maintaining mitochondrial architecture, accomplishing the metabolic needs and ensuring intracellular and extracellular signaling. Mitofusins play a major role in controlling these functions and alterations of mitochondrial architecture are implicated in metabolic disorders, cancer and aging (Schrepfer and Scorrano, 2016).
There is little information about what determines sites of mitochondrial fission within the mitochondrial network, but it is known that mitochondrial division occurs at membrane contact sites between endoplasmic reticulum (ER) and mitochondria (Friedman et al., 2011). Thus, ER tubules may play an active role in defining the position of mitochondrial division sites and in inter-organellar cross-talk (Friedman et al., 2011). Recently, inter-mitochondrial signaling by either nanotubes or direct interaction via inter-mitochondrial junctions has been intensively studied and membrane contact sites between mitochondria and other organelles, such as peroxisomes or lysosomes, beyond ER, have emerged as important mediators in the regulation of fundamental cellular processes, including cell death and proliferation, proteostasis and mitophagy (Genovese et al., 2022 and references therein). The peroxisome-mitochondria connection includes the physical and functional interaction between these organelles (Schrader et al., 2015). The best known example is the metabolic cooperation in the beta-oxidation of fatty acids, which is typical of mammals and fungi. In addition, peroxisomes share a redox relationship with mitochondria contributing to cellular ROS homeostasis and affecting cell fate. Very little is known about how peroxisomes and mitochondria exchange co-factors, metabolites, and signaling molecules and how these organelle’s dysfunction reciprocally influence health and disease processes (Fransen et al., 2017).
Among the inter-organelle interactions and communication mechanisms, ER–mitochondria and mitochondria–lysosomes are the most studied and characterized. ER and lysosomes represent the hub of protein synthesis and degradation processes, respectively, and are connected by the process of protein homeostasis (proteostasis). Deregulation of proteostasis due to accumulation of misfolded proteins or protein aggregates is considered an hallmark of neurodegenerative and aging-related diseases (Moehle et al., 2019). In the presence of dysfunctional mitochondria, an adaptive transcriptional response known as mitochondrial Unfolded Protein Response (mtUPR) is activated to preserve function in healthy organelles and recover activity in damaged compartments. This pathway was initially discovered in mammalian cells, and further characterized in C. elegans (Pellegrino et al., 2013).
1.2 Mitochondrial quality control
The mitochondrial functionality itself is related to the control of mitochondrial quality (MQC). This is referred as mitochondrial homeostasis and is a tightly regulated process at both molecular and organelle level. A number of pathways and mechanisms cooperate and control mitochondrial proteome and/or mitochondrial content especially during stress times and adequate regulation of these mitochondrial quality control pathways are crucial for cellular homeostasis (Roca-Portoles and Tait, 2021; Wui Ng, MY et al., 2021). Cell response will vary depending on stress type and intensity and on a range of factors able to sense and transmit the nature of the encountered perturbation. For example, at organelle level, upon external stimuli, mitochondria can change their morphology by activating fission or fusion events: upon a severe or prolonged stress, mitochondria undergo fission, meanwhile, upon a mild or low stress, mitochondria favor fusion (Van Der Bliek, 2009). Also starvation and low glucose or, ETC inhibition can induce fusion and fission processes, respectively. To face persistent damage, another cellular homeostatic mechanism is to remove damaged organelles through proteolytic pathways that can be non-selective for autophagy and selective for mitophagy. Autophagy and mitophagy are evolutionary conserved from yeast to mammals: Autophagy Related Genes (ATG genes), specific mitophagy mediators and mechanisms have been identified and characterized for their role during different steps of the process (Kumar R. and Reichert A.S., 2021). In case of mitochondrial defects, the removal of damaged mitochondria is a cytoprotective strategy to prevent the accumulation of dysfunctional organelles with consequent deleterious effects (Zimmermann and Reichert, 2017). That mitochondrial fission can act upstream of mitophagy upon mitochondrial stress has been envisaged (Valera-Alberni and Canto, 2018).
Growing evidence indicates that the cytosolic ubiquitin–proteasome system (UPS), a multi-component system responsible for the removal of proteins from different cellular compartments, represents an important component of MQC. As support of this, several proteomic screens of mitochondria in different organisms (mouse, yeast, plants) identified ubiquitinated proteins (Sickmann et al., 2003; Bohovych et al., 2015). First of all, UPS has been shown to participate in the removal of several mitochondria-targeted proteins before or during their import into the mitochondria. Therefore, mistargeted or misfolded mitochondria-destined proteins are identified as aberrant and recognized by the UPS for removal: finding such poly-ubiquitinated proteins in the cytosol may reflect a role of the UPS in controlling levels and/or quality of proteins targeted to mitochondria (den Braven et al., 2021). Furthermore, UPS can access the OMM sub-proteome and mediate retro-translocation and degradation of OMM resident proteins. Recently Basch et al. described a mitochondrial quality control mechanism based on the cooperation of Msp1, an OMM anchored ATPase, with the proteasome. The authors found that yeast Msp1 cooperates with the proteasome to extract and degrade proteins arrested at the mitochondrial TOM complex. The proteasome seems to be actively involved in the extraction of trapped proteins from the OMM and proteasome inhibition effectively blocks this process (Basch et al., 2020). Moreover, it has been demonstrated that the majority of known OMM associated substrates of the UPS system are proteins central for the regulation of either apoptosis or mitochondrial membrane dynamics (Yonashiro et al., 2006; Karbowski et al., 2007). For example, the degradation of OMM-associated anti-apoptotic proteins, such as Bcl-2 and Mcl1, require their poly-ubiquitination and the activity of the 26S proteasome (Stewart et al., 2010). In yeast it has been shown that the mitochondrial fusion protein Fzo1 is ubiquitylated while still associated with the membrane and that the proteasome inhibitor MG132, as well as proteasome mutations, can suppress its degradation (Neutzner et al., 2007). These results indicate that poly-Ub-dependent proteasomal degradation is involved in Fzo1 turnover.
Recent attention has also been posed to control strategies at molecular level coupled to mitochondrial import mechanisms (Moehle et al., 2019). Impairment of mitochondrial protein import can result in the accumulation of precursors in the cytosol and other organelles activating a proteotoxic stress response, which results in the transmission of a signal to the nucleus to increase the expression of proteases and chaperones to address the abnormal mitochondrial protein load.
The integrity of mitochondrial membrane potential, affecting protein movement across the IMM, is an important aspect of mitochondrial import machinery. Therefore, the status of mitochondrial import can be considered as a faithful readout for mitochondrial dysfunction. At this regard, the mtUPR characterized in C. elegans is an example of retrograde signaling in which a defect in mitochondrial protein import activates an ATFS1-mediated transcriptional response to counteract proteotoxicity (Nargund et al., 2012). The mtUPR plays a dual role, from one side it senses proteotoxic stress within mitochondria and induces a recovery gene expression program, from the other side activates a metabolic shift from OXPHOS to glycolysis (Nargund et al., 2015). It is also known that the mtUPR and mitophagy are complementary processes, where the mtUPR acts in the first line of defense to counteract mitochondrial proteotoxic stress, and mitophagy in the removal of most severely damaged mitochondria (Pellegrino and Haynes, 2015). In Figure 1 the described mechanisms of main MQC processes, such as biogenesis, dynamics, proteostasis and mitophagy upon stress, are depicted. Although they can occur distinctly, it is of note that all of them can be connected one with the other in the cellular context.
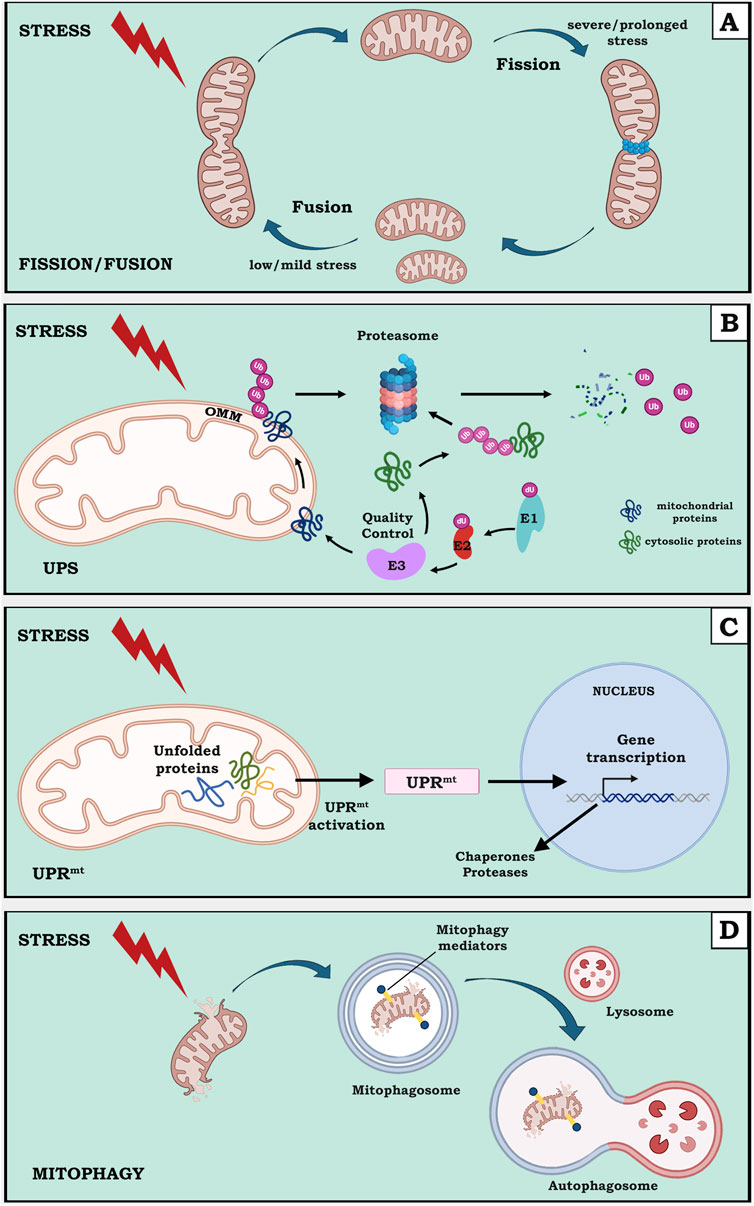
Figure 1. A schematic view of mitochondrial quality control (MQC) mechanisms upon cell stress. Stress type and intensity can activate different mechanisms of MQC processes, including biogenesis, dynamics, proteostasis and mitophagy. (A) upon a severe or prolonged stress, mitochondria undergo fission, meanwhile, upon a low or mild stress, mitochondria favor fusion (B) cytosolic or aberrant proteins associated to OMM are poly-ubiquinated and degraded through UPS, involving the concerted action of E1, E2 and E3 enzymes for ubiquitination (C) misfolded mitochondrial proteins activate a UPRmt leading to a mitochondria-to nucleus transcriptional reprogramming increasing the expression of chaperones and proteases for stress adaptation (D) chronic mitochondrial damage leads to selective degradation of mitochondria through mitophagy, involving specific mediators and the formation of an autophagosome deriving from the fusion between a double membrane mitophagosome and a lysosome.
Another related strategy which has been emerging recently is the production and budding of mitochondria-derived membranous structures, such as mitochondria-derived vesicles (MDVs) in human cells and mitochondria-derived compartments (MDCs) in yeast (Soubannier et al., 2012). In both cases, they represent an intermediate step in mitochondrial protein degradation where dysfunctional mitochondrial subdomains, including specific mitochondrial proteins (TOM or OXPHOS from the, ETC), are targeted for lysosomal/vacuolar degradation. The molecular mechanisms behind the biogenesis and the cargo selectivity of MDVs and MDCs are still poorly understood and are a promising line of research in the field of mitochondrial homeostasis. In addition, understanding the mechanisms behind the integration of quality control processes is essential for selective interventions in disease models. At this regard, the role of MDVs in balancing fission and fusion processes and mediating either immunomodulation mechanisms or the interconnectivity with other organelles has been well established (Hazan et al., 2024; Picca et al., 2023).
1.3 Yeast Saccharomyces cerevisiae as a model to study the impact of mitochondrial dysfunction on cell stress response
It is clear that mitochondria are not isolated entities acting as energy providers to the rest of the cell. Instead, a constant communication of these organelles with other cell compartments is essential to control homeostasis and physiological processes. Nucleus, ER, peroxisomes and lysosomes functionally cooperate and physically interact with mitochondria under different conditions, however the mode and mechanism of organelle crosstalk is still a research challenge (Zhang Y et al., 2023). On the other hand, dysregulation of organelle cross talk may lead to severe mitochondrial dysfunction (Figure 2). Cytoprotective strategies can be activated by the cells to sense and resolve mitochondrial stress. Therefore, the communication of mitochondria with other organelles is strictly related to stress response (Kim K.H. & Lee C.B. 2024 and references therein). For all these reasons, it is important to have cellular/organismal models to investigate the organelle communication network at different layers of complexity.
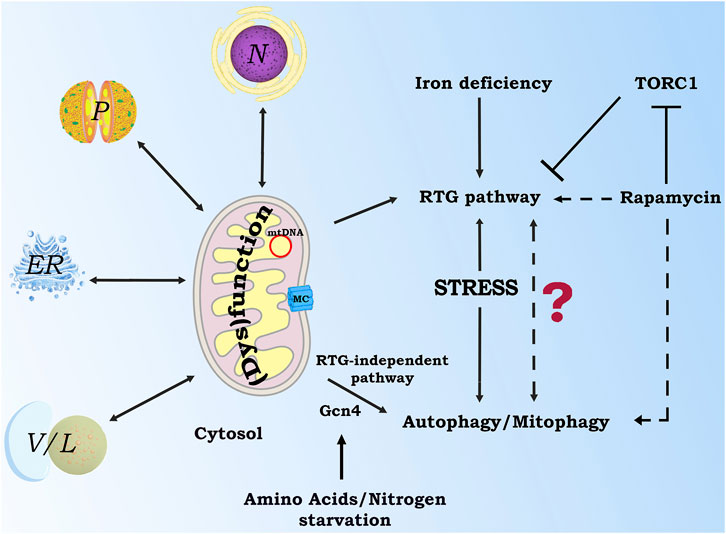
Figure 2. Possible interplay between mitochondrial retrograde signaling and autophagy/mitophagy processes in response to cell stress in a yeast model. Alterations in mitochondrial functions or stress conditions can activate cytoprotective strategies, including retrograde pathways, dependent or independent on RTG genes, or autophagy/mitophagy processes. In this scenario, inter-organellar cross talk is fundamental to sustain metabolic signaling and reprogramming. Whether and how the cells choose between these cellular adaptive strategies is an open question. Nucleus (N), peroxisomes (P), endoplasmic reticulum (ER), vacuoles/lysosomes (V/L), mitochondrial carrier (MC). Dashed lines indicate future research challenges which could be explored.
The budding yeast Saccharomyces cerevisiae is an ideal model organism to study the impact of mitochondrial functionality on cell stress response and inter-organellar cross talk. In fact, yeast is a facultative anaerobe able to grow and survive in the presence of different mitochondrial dysfunctions, from defects in the OXPHOS and ETC to partial or complete lack of mitochondrial DNA (mtDNA). Many adaptive strategies to mitochondrial dysfunction have been described before and are conserved from yeast to mammals, although with some differences. The mitochondria-cytosol-nucleus cross talk activated by mitochondrial dysfunction has been well characterized in terms of key pathways and molecular components in yeast cells (Guaragnella et al., 2018). Retrograde Genes (RTG), including RTG1, RTG2 and RTG3, are involved in the metabolic reprogramming to adapt the cells to mitochondrial dysfunctions (Z. Liu and Butow, 2006). Meanwhile, an RTG-independent retrograde signaling related to amino acid metabolism has been described (Guaragnella and Butow, 2003). Mitochondrial dysfunction can also affect the cytosolic proteome causing mitochondrial precursors overaccumulation (mPOS) and a transcriptional response activated by the stress due to unimported mitochondrial precursor proteins (Wang and Chen, 2015; Wrobel et al., 2015; Coyne et al., 2018). The mechanisms behind are poorly understood although the proteotoxic stress should originate from protein misfolding in the IMM (Y. Liu et al., 2015). A drastic accumulation and aggregation of mitochondrial precursor proteins in the cytosol can ultimately kill the cells. Nevertheless, mitochondria can actively improve cytosolic proteostasis under different conditions: this suggests a puzzling framework for the mitochondria–cytosol proteostatic cross talk (Guaragnella et al., 2018 and references therein).
Altered mitochondrial functionality, mainly affecting mitochondrial potential, has been correlated to selective mitophagy processes, pointing a role to a cellular cytoprotective strategy for controlling organelle quality and nutrient availability and sustaining survival (Bhatia-Kissova and Camougrand, 2021 and references therein). At this regard, a molecular axis involving the mitochondrial protein phosphatase Aup1 and the transcription factor Rtg3 has been proposed for the induction of mitophagy (Journo et al., 2009). Aup1 is required for efficient mitophagy in stationary phase yeast cells. Concomitantly, Aup1 is required for the correct translocation of Rtg3 protein from the cytosol to the nucleus and the RTG-target genes are induced in an Aup1-dependent fashion upon mitophagic conditions. These evidences highlight the molecular mechanisms behind the pro-survival and physiological role of mitophagy in aging cultures.
1.4 Interplay between mitochondrial retrograde signaling and autophagy/mitophagy in stress response
Several evidences suggest an interplay between retrograde response and mitochondrial quality control in the form of autophagy and mitophagy. In particular, TORC1 (target of rapamycin complex 1), an evolutionarily conserved nutrient-responsive protein kinase complex, seems to be an active player in the signaling mechanisms. It is well established that rapamycin inhibits TORC1 causing activation of autophagy/mitophagy and eliciting the induction of retrograde pathway. The metabolic basis behind TORC1 pathway activation are still far to be elucidated and include environmental signals ranging from carbon, nitrogen, phosphate and metal ion limitations.
So, if it is clear that nutrient-dependent mechanisms guide the response, how distinct cytoprotective strategies are coordinated based on specific environmental cues is still a matter of investigation. Snf1, the conserved sensor and regulator of metabolism, is activated by glucose deprivation and involved in the RTG-dependent mitochondrial response to ER stress concomitantly with TORC1 inhibition (Hijazi et al., 2020). TORC1 inhibition is known to induce the expression of Gcn4, the transcriptional activator of the general amino acid control response pathway, which is also a positive regulator of selected ATG genes during amino acid and nitrogen starvation in yeast (Metur and Klionsky, 2024 and references therein). It is of note that Gcn4 is required for the activation of an RTG-independent retrograde signaling in ρ0 petite cells lacking mitochondrial DNA (Guaragnella and Butow, 2003). Interestingly, certain mitochondrial transporters mediating the exchange of nutrients and metabolites across the IMM, might be directly or indirectly involved in the challenge for cytoprotection through the MDC pathway to prevent a deregulated or unsustainable influx of nutrients into the mitochondria. (Bhatia-Kissova and Camougrand, 2021).
Yeast requires different micronutrients to support their growth and metabolic processes, therefore, the bioavailability of manganese, phosphate, zinc, and iron is critical for efficient proteome function and stress tolerance. A recent work describes how the level of cytoplasmic manganese can affect autophagy, mitophagy and retrograde signaling presumably through TORC1 activation (Nicastro et al., 2022). Autophagy is robustly activated in response to zinc starvation, which is reversed upon adding zinc to the media (Kawamata et al., 2017). However, how free cellular zinc is sensed and transduced to initiate autophagy induction remains to be investigated. Adaptation to iron deficiency requires the global reorganization of cellular metabolism in order to optimize iron utilization. It is of note that upon iron limitations, beyond the increase of components of the iron deficiency response, also the mitochondrial retrograde pathway is activated (Romero et al., 2019). In parallel, TORC1 is inhibited during the progress of iron deficiency and autophagy is required during metabolic transition when iron is limiting (Horie et al., 2017).
Autophagy is also involved in the maintenance of mitochondrial respiratory function, as autophagy-defective mutants accumulated high level of ROS, leading to respiratory deficiency and resulting in the loss of mitochondria DNA (mtDNA) during nitrogen starvation (Suzuki et al., 2011). A link between autophagy induced by nitrogen deprivation and cellular response to osmostress has also been observed (Prick et al., 2006; Bothammal et al., 2022). The response to hyperosmotic stress of yeast cells involves the activation of Hog1 with consecutive induction of glycerol-synthesizing genes, resulting in an increased production of glycerol (Hohmann, 2002) and a loss of Hog1 causes osmosensitivity of the autophagy (Prick et al., 2006). Also, the activity of the transcriptional complex Rtg1/3 has been shown to be modulated by Hog1: HOG1 and the RTG pathway may interact sequentially in the stress signaling cascade and the RTG pathway may play a role in the metabolic communication between peroxisomes and mitochondria for osmoadaptation. Mitochondria are key determinants for osmostress response and different adaptive strategies might be activated depending on the genetic and metabolic context (Pastor et al., 2009; Guaragnella et al., 2021; Di Noia et al., 2023).
Among other types of stress conditions, oxidative stress plays an evolutionary conserved regulatory role on autophagy/mitophagy. At this regard, specific upregulation of the catalase, CTT1, has been shown to be dependent on RTG2 providing cytoprotection against acetic-acid induced regulated cell death in yeast (Guaragnella et al., 2019). Accordingly, impaired function of mitochondrial matrix redox enzymes activates an adaptive response specifically involving the catalase Ctt1 (Calabrese et al., 2019). In this case, the retrograde signals allowing the nuclear transcriptional response have not been identified.
A connection between heat shock and autophagy has also been reported in yeast, where Hsp31 mini-family members can potentially serve as negative regulators of autophagy (Miller-Fleming et al., 2014). Given some similarities related to the diauxic shift and stationary phase transition between HSP31 knock out strains and RTG mutants, it cannot be excluded an interplay between heat shock proteins and mitochondrial retrograde signaling to preserve cell survival.
2 Discussion
The accumulation of dysfunctional mitochondria is a physiological process during the cell’s lifetime and cells can either succumb to mitochondrial dysfunction, by starting death processes, or activate adaptive mechanisms to counteract the damage. Thus, mitochondrial dysfunction can be observed from a double perspective in the context of cell homeostasis. Here we highlighted the relevance of mitochondrial signaling for the stimulation of cytoprotective strategies in the presence of mitochondrial dysfunction. In particular, we focused on the retrograde response pathways and autophagic/mitophagic processes. From one side, the retrograde response facilitates the metabolic remodelling to compensate for the loss of mitochondrial function; from the other side, autophagy/mitophagy can either provide building blocks for amino acids and protein synthesis or remove damaged and malfunctioning organelles.
Although the functional connection between retrograde signaling and autophagy/mitophagy processes has been reported, their coordination is still puzzling and it will need further investigations. In this regard, yeast S. cerevisiae provides a suitable model to gain insight into the molecular aspects behind anabolic and catabolic stress responses. In particular, yeast cells exposed to osmostress could help to reveal new understandings in this direction. Evidences obtained in osmo-stressed yeast show that an RTG-dependent retrograde signaling is activated upon treatment with sodium chloride and affects the kinetics of osmoadaptation (Ruiz-Roig et al., 2012; Guaragnella et al., 2021). It is interesting to point out that a faster response is observed when respiratory capacity is abolished and RTG-independent signaling are involved in the concomitant absence of mitochondrial DNA and a specific mitochondrial carrier, named Rim2p (Di Noia et al., 2023; Di Noia et al., 2024).
These data, together with the evidences showing autophagic traits due to NaCl stress (Bothammal et al., 2022), pose the attention to this model for revealing new clues on the role of mitochondria in the synthesis, degradation and recycling processes during stress conditions. The intracellular concentration of different metabolites’ pools may be one of the key points to modulate cytoprotective strategies, such as specific signaling pathways or induction of autophagic processes. In this context, the involvement of mitochondrial carrier (MCs) that are widespread in all eukaryotes represents a fascinating aspect that deserves further investigation. Figure 2 describes signaling pathways, proteins and processes activated by mitochondrial dysfunction and/or cellular stress in a yeast model. The involvement of inter-organellar cross talk in stress response is also highlighted. Future research challenges, including the possible interplay between mitochondrial retrograde signaling and autophagy/mitophagy processes, are proposed.
Data availability statement
The original contributions presented in the study are included in the article/supplementary material, further inquiries can be directed to the corresponding author.
Author contributions
NG: Conceptualization, Writing–original draft, Writing–review and editing. MADN: Conceptualization, Writing–original draft, Writing–review and editing. AP: Formal Analysis, Visualization, Software, Writing–review and editing.
Funding
The author(s) declare that financial support was received for the research, authorship, and/or publication of this article. AP is recipient of a PhD fellowship funded by PNRR D.M.117/2023 and by Acquedotto Pugliese S.p.A. The funder was not involved in the study design, collection, analysis, interpretation of data, the writing of this article, or the decision to submit it for publication.
Conflict of interest
The authors declare that the research was conducted in the absence of any commercial or financial relationships that could be construed as a potential conflict of interest.
Publisher’s note
All claims expressed in this article are solely those of the authors and do not necessarily represent those of their affiliated organizations, or those of the publisher, the editors and the reviewers. Any product that may be evaluated in this article, or claim that may be made by its manufacturer, is not guaranteed or endorsed by the publisher.
References
Basch, M., Wagner, M., Rolland, S., Carbonell, A., Zeng, R., Khosravi, S., et al. (2020). Msp1 cooperates with the proteasome for extraction of arrested mitochondrial import intermediates. Mol. Biol. Cell. 31 (8), 753–767. doi:10.1091/MBC.E19-06-0329
Bhatia-Kissova, I., and Camougrand, N. (2021). Mitophagy in yeast: decades of research. Cells 10 (Issue 12), 3541. doi:10.3390/cells10123541
Bohovych, I., Chan, S. S. L., and Khalimonchuk, O. (2015). Mitochondrial protein quality control: the mechanisms guarding mitochondrial health. Antioxidants Redox Signal. 22 (12), 977–994. doi:10.1089/ars.2014.6199
Bothammal, P., Prasad, M., Muralitharan, G., and Natarajaseenivasan, K. (2022). Leptospiral lipopolysaccharide mediated Hog1 phosphorylation in Saccharomyces cerevisiae directs activation of autophagy. Microb. Pathog. 173, 105840. doi:10.1016/j.micpath.2022.105840
Butow, R. A., and Avadhani, N. G. (2004). Mitochondrial signaling: the retrograde response. Mol. Cell. 14, 1–15. doi:10.1016/s1097-2765(04)00179-0
Calabrese, G., Peker, E., Amponsah, P. S., Hoehne, M. N., Riemer, T., Mai, M., et al. (2019). Hyperoxidation of mitochondrial peroxiredoxin limits H 2 O 2 -induced cell death in yeast. EMBO J. 38 (18), e101552. doi:10.15252/embj.2019101552
Coyne, L. P., and Chen, X. J. (2018). mPOS is a novel mitochondrial trigger of cell death – implications for neurodegeneration. FEBS Lett. 592 (5), 759–775. doi:10.1002/1873-3468.12894
den Braven, F., Gupta, A., and Becker, T. (2021). Protein quality control at the mitochondrial surface. Front. Cell. Dev. Biol. 9, 795685. doi:10.3389/fcell.2021.795685
Di Noia, M. A., Ocheja, O. B., Scarcia, P., Pisano, I., Messina, E., Agrimi, G., et al. (2024). Lack of mitochondrial DNA provides metabolic advantage in yeast osmoadaptation. Biomolecules 14 (6), 704. doi:10.3390/biom14060704
Di Noia, M. A., Scarcia, P., Agrimi, G., Ocheja, O. B., Wahid, E., Pisano, I., et al. (2023). Inactivation of HAP4 accelerates RTG-dependent osmoadaptation in Saccharomyces cerevisiae. Int. J. Mol. Sci. 24 (6), 5320. doi:10.3390/ijms24065320
Fontana, F., and Limonta, P. (2021). The multifaceted roles of mitochondria at the crossroads of cell life and death in cancer. Free Radic. Biol. Med. 176, 203–221. doi:10.1016/j.freeradbiomed.2021.09.024
Fransen, M., Lismont, C., and Walton, P. (2017). The peroxisome-mitochondria connection: how and why? Int. J. Mol. Sci. 18 (Issue 6), 1126. doi:10.3390/ijms18061126
Friedman, J. R., Lackner, L. L., West, M., DiBenedetto, J. R., Nunnari, J., and Voeltz, G. K. (2011). ER tubules mark sites of mitochondrial division. Science 334 (6054), 358–362. doi:10.1126/science.1207385
Genovese, I., Fornetti, E., and Ruocco, G. (2022). Mitochondria inter-organelle relationships in cancer protein aggregation. Front. Cell. Dev. Biol. 10, 1062993. doi:10.3389/fcell.2022.1062993
Gorgoglione, R., Porcelli, V., Santoro, A., Daddabbo, L., Vozza, A., Monnè, M., et al. (2019). The human uncoupling proteins 5 and 6 (UCP5/SLC25A14 and UCP6/SLC25A30) transport sulfur oxyanions, phosphate and dicarboxylates. Biochim. Biophys. Acta Bioenerg. 1860 (9), 724–733. doi:10.1016/j.bbabio.2019.07.010
Guaragnella, N., Agrimi, G., Scarcia, P., Suriano, C., Pisano, I., Bobba, A., et al. (2021). Rtg signaling sustains mitochondrial respiratory capacity in hog1-dependent osmoadaptation. Microorganisms 9 (9), 1894. doi:10.3390/microorganisms9091894
Guaragnella, N., and Butow, R. A. (2003). ATO3 encoding a putative outward ammonium transporter is an RTG-independent retrograde responsive gene regulated by GCN4 and the ssy1-ptr3-ssy5 amino acid sensor system. J. Biol. Chem. 278 (46), 45882–45887. doi:10.1074/jbc.M309301200
Guaragnella, N., Coyne, L. P., Chen, X. J., and Giannattasio, S. (2018). Mitochondria-cytosol-nucleus crosstalk: learning from Saccharomyces cerevisiae. FEMS Yeast Res. 18 (Issue 8), foy088. doi:10.1093/femsyr/foy088
Guaragnella, N., Stirpe, M., Marzulli, D., Mazzoni, C., and Giannattasio, S. (2019). Acid stress triggers resistance to acetic acid-induced regulated cell death through Hog1 activation which requires RTG2 in yeast. Oxidative Med. Cell. Longev. 2019, 4651062. doi:10.1155/2019/4651062
Hazan, R., Pines, O., and Saada, A. (2024). “Mitochondrial derived vesicles- quo vadis?,” in FEBS journal. doi:10.1111/febs.17103
Hijazi, I., Knupp, J., and Chang, A. (2020). Retrograde signaling mediates an adaptive survival response to endoplasmic reticulum stress in Saccharomyces cerevisiae. J. Cell. Sci. 133 (6), jcs241539. doi:10.1242/JCS.241539
Hohmann, S. (2002). Osmotic stress signaling and osmoadaptation in yeasts. Microbiol. Mol. Biol. Rev. 66 (2), 300–372. doi:10.1128/mmbr.66.2.300-372.2002
Horie, T., Kawamata, T., Matsunami, M., and Ohsumi, Y. (2017). Recycling of iron via autophagy is critical for the transition from glycolytic to respiratory growth. J. Biol. Chem. 292 (20), 8533–8543. doi:10.1074/jbc.M116.762963
Ippolito, L., Giannoni, E., Chiarugi, P., and Parri, M. (2020). Mitochondrial redox hubs as promising targets for anticancer therapy. Front. Oncol. 10, 256. doi:10.3389/fonc.2020.00256
Jin, Y., Huynh, D. T. N., Kang, K. W., Myung, C. S., and Heo, K. S. (2019). Inhibition of p90RSK activation sensitizes triple-negative breast cancer cells to cisplatin by inhibiting proliferation, migration and EMT. BMB Rep. 52 (12), 706–711. doi:10.5483/BMBRep.2019.52.12.234
Journo, D., Mor, A., and Abeliovich, H. (2009). Aup1-mediated regulation of Rtg3 during mitophagy. J. Biol. Chem. 284 (51), 35885–35895. doi:10.1074/jbc.M109.048140
Karbowski, M., Neutzner, A., and Youle, R. J. (2007). The mitochondrial E3 ubiquitin ligase MARCH5 is required for Drp1 dependent mitochondrial division. J. Cell. Biol. 178 (1), 71–84. doi:10.1083/jcb.200611064
Kawamata, T., Horie, T., Matsunami, M., Sasaki, M., and Ohsumi, Y. (2017). Zinc starvation induces autophagy in yeast. J. Biol. Chem. 292 (20), 8520–8530. doi:10.1074/jbc.M116.762948
Kim, K. H., and Lee, C. B. (2024). Socialized mitochondria: mononuclear crosstalk in stress. Exp. Mol. Med. 56 (5), 1033–1042. doi:10.1038/s12276-024-01211-4
Kim, S., Ramalho, T. R., and Haynes, C. M. (2024). Regulation of proteostasis and innate immunity via mitochondria-nuclear communication. J. Cell. Biol. 223 (3), e202310005. doi:10.1083/jcb.202310005
Kumar, R., and Reichert, A. S. (2021). Common principles and specific mechanisms of mitophagy from yeast to humans. Int. J. Mol. Sci. 22 (9), 4363. doi:10.3390/ijms22094363
Liu, Y., Wang, X., and Chen, X. J. (2015). Misfolding of mutant adenine nucleotide translocase in yeast supports a novel mechanism of Ant1-induced muscle diseases. Mol. Biol. Cell. 26 (11), 1985–1994. doi:10.1091/mbc.E15-01-0030
Liu, Z., and Butow, R. A. (2006). Mitochondrial retrograde signaling. Annu. Rev. Genet. 40, 159–185. doi:10.1146/annurev.genet.40.110405.090613
Lu, J., Sharma, L. K., and Bai, Y. (2009). Implications of mitochondrial DNA mutations and mitochondrial dysfunction in tumorigenesis. Cell. Res. 19 (7), 802–815. doi:10.1038/cr.2009.69
Metur, S. P., and Klionsky, D. J. (2024). Nutrient-dependent signaling pathways that control autophagy in yeast. FEBS Lett. 598 (1), 32–47. doi:10.1002/1873-3468.14741
Miller-Fleming, L., Antas, P., Pais, T. F., Smalley, J. L., Giorgini, F., and Outeiro, T. F. (2014). Yeast DJ-1 superfamily members are required for diauxic-shift reprogramming and cell survival in stationary phase. Proc. Natl. Acad. Sci. U. S. A. 111 (19), 7012–7017. doi:10.1073/pnas.1319221111
Moehle, E. A., Shen, K., and Dillin, A. (2019). Mitochondrial proteostasis in the context of cellular and organismal health and aging. J. Biol. Chem. 294 (14), 5396–5407. doi:10.1074/jbc.TM117.000893
Nakhle, J., Rodriguez, A. M., and Vignais, M. L. (2020). Multifaceted roles of mitochondrial components and metabolites in metabolic diseases and cancer. Int. J. Mol. Sci. 21 (12), 4405–4433. doi:10.3390/ijms21124405
Nargund, A. M., Fiorese, C. J., Pellegrino, M. W., Deng, P., and Haynes, C. M. (2015). Mitochondrial and nuclear accumulation of the transcription factor ATFS-1 promotes OXPHOS recovery during the UPRmt. Mol. Cell. 58 (1), 123–133. doi:10.1016/j.molcel.2015.02.008
Nargund, A. M., Pellegrino, M. W., Fiorese, C. J., Baker, B. M., and Haynes, C. M. (2012). Mitochondrial import efficiency of ATFS-1 regulates mitochondrial UPR activation. Science 337 (6094), 587–590. doi:10.1126/science.1223560
Neutzner, A., Youle, R. J., and Karbowski, M. (2007). Outer mitochondrial membrane protein degradation by the proteasome. Novartis Found. Symp. 287, 14.
Newman, L. E., and Shadel, G. S. (2023). Annual review of biochemistry mitochondrial DNA release in innate immune signaling. doi:10.1146/annurev-biochem-032620
Nicastro, R., Gaillard, H., Zarzuela, L., Péli-Gulli, M. P., Fernández-García, E., Tomé, M., et al. (2022). Manganese is a physiologically relevant TORC1 activator in yeast and mammals. ELife 11, e80497. doi:10.7554/ELIFE.80497
Palmieri, F., Scarcia, P., and Monné, M. (2020). Diseases caused by mutations in mitochondrial carrier genes SLC25: a review. MDPI Ag. 10 (Issue 4), 655. doi:10.3390/biom10040655
Passarella, S., Schurr, A., and Portincasa, P. (2021). Mitochondrial transport in glycolysis and gluconeogenesis: achievements and perspectives. Int. J. Mol. Sci. 22 (23), 12620. doi:10.3390/ijms222312620
Pastor, M. M., Proft, M., and Pascual-Ahuir, A. (2009). Mitochondrial function is an inducible determinant of osmotic stress adaptation in yeast. J. Biol. Chem. 284 (44), 30307–30317. doi:10.1074/jbc.M109.050682
Pellegrino, M. W., and Haynes, C. M. (2015). Mitophagy and the mitochondrial unfolded protein response in neurodegeneration and bacterial infection. BMC Biol. 13 (Issue 1), 22. doi:10.1186/s12915-015-0129-1
Pellegrino, M. W., Nargund, A. M., and Haynes, C. M. (2013). Signaling the mitochondrial unfolded protein response. Biochimica Biophysica Acta - Mol. Cell. Res. 1833 (2), 410–416. doi:10.1016/j.bbamcr.2012.02.019
Picard, M., and Shirihai, O. S. (2022). Mitochondrial signal transduction. Cell. Metab. 34 (11), 1620–1653. doi:10.1016/j.cmet.2022.10.008
Picca, A., Guerra, F., Calvani, R., Coelho-Júnior, H. J., Landi, F., Bucci, C., et al. (2023). Mitochondrial-derived vesicles: the good, the bad, and the ugly. Int. J. Mol. Sci. 24 (Issue 18), 13835. doi:10.3390/ijms241813835
Prick, T., Thumm, M., Köhrer, K., Häussinger, D., and Vom Dahl, S. (2006). In yeast, loss of Hog1 leads to osmosensitivity of autophagy. Biochem. J. 394 (1), 153–161. doi:10.1042/BJ20051243
Quirós, P. M., Mottis, A., and Auwerx, J. (2016). Mitonuclear communication in homeostasis and stress. Nat. Rev. Mol. Cell. Biol. 17 (4), 213–226. doi:10.1038/nrm.2016.23
Roca-Portoles, A., and Tait, S. W. G. (2021). Mitochondrial quality control: from molecule to organelle. Cell. Mol. Life Sci. 78 (8), 3853–3866. doi:10.1007/s00018-021-03775-0
Romero, A. M., Ramos-Alonso, L., Montellá-Manuel, S., García-Martínez, J., de la Torre-Ruiz, M. Á., Pérez-Ortín, J. E., et al. (2019). A genome-wide transcriptional study reveals that iron deficiency inhibits the yeast TORC1 pathway. Biochimica Biophysica Acta - Gene Regul. Mech. 1862 (9), 194414. doi:10.1016/j.bbagrm.2019.194414
Ruiz-Roig, C., Noriega, N., Duch, A., Posas, F., and De Nadal, E. (2012). The Hog1 SAPK controls the Rtg1/Rtg3 transcriptional complex activity by multiple regulatory mechanisms. Mol. Biol. Cell. 23 (21), 4286–4296. doi:10.1091/mbc.E12-04-0289
Schrader, M., Costello, J., Godinho, L. F., and Islinger, M. (2015). Peroxisome-mitochondria interplay and disease. J. Inherit. Metabolic Dis. 38 (4), 681–702. doi:10.1007/s10545-015-9819-7
Schrepfer, E., and Scorrano, L. (2016). Mitofusins, from mitochondria to metabolism. Mol. Cell. 61 (5), 683–694. doi:10.1016/j.molcel.2016.02.022
Sickmann, A., Rg Reinders, J., Wagner, Y., Joppich, C., Zahedi, R., Meyer, H. E., et al. (2003). The proteome of Saccharomyces cerevisiae mitochondria. Proc. Natl. Acad. Sci. U. S. A. 100, 13207–13212. doi:10.1073/pnas.2135385100
Son, J. M., and Lee, C. (2019). Mitochondria: multifaceted regulators of aging. BMB Rep. 52 (1), 13–23. doi:10.5483/BMBRep.2019.52.1.300
Soubannier, V., McLelland, G. L., Zunino, R., Braschi, E., Rippstein, P., Fon, E. A., et al. (2012). A vesicular transport pathway shuttles cargo from mitochondria to lysosomes. Curr. Biol. 22 (2), 135–141. doi:10.1016/j.cub.2011.11.057
Spinelli, J. B., and Haigis, M. C. (2018). The multifaceted contributions of mitochondria to cellular metabolism. Nat. Cell. Biol. 20 (7), 745–754. doi:10.1038/s41556-018-0124-1
Stewart, D. P., Koss, B., Bathina, M., Perciavalle, R. M., Bisanz, K., and Opferman, J. T. (2010). Ubiquitin-independent degradation of antiapoptotic MCL-1. Mol. Cell. Biol. 30 (12), 3099–3110. doi:10.1128/mcb.01266-09
Stoolman, J. S., Porcelli, A. M., and Martínez-Reyes, I. (2022). Editorial: mitochondria as a hub in cellular signaling. Front. Cell. Dev. Biol. 10, 981464. doi:10.3389/fcell.2022.981464
Suzuki, S. W., Onodera, J., and Ohsumi, Y. (2011). Starvation induced cell death in autophagy-defective yeast mutants is caused by mitochondria dysfunction. PLoS ONE 6 (2), e17412. doi:10.1371/journal.pone.0017412
Todisco, S., Di Noia, M. A., Onofrio, A., Parisi, G., Punzi, G., Redavid, G., et al. (2016). Identification of new highly selective inhibitors of the human ADP/ATP carriers by molecular docking and in vitro transport assays. Biochem. Pharmacol. 15 (100), 112–132. doi:10.1016/j.bcp.2015.11.019
Valera-Alberni, M., and Canto, C. (2018). Mitochondrial stress management: a dynamic journey. Cell. Stress 2 (No. 10), 253–274. doi:10.15698/cst2018.10.158
Van Der Bliek, A. M. (2009). Fussy mitochondria fuse in response to stress. EMBO J. 28 (11), 1533–1534. doi:10.1038/emboj.2009.130
Wang, X., and Chen, X. J. (2015). A cytosolic network suppressing mitochondria-mediated proteostatic stress and cell death. Nature 524 (7566), 481–484. doi:10.1038/nature14859
Wrobel, L., Topf, U., Bragoszewski, P., Wiese, S., Sztolsztener, M. E., Oeljeklaus, S., et al. (2015). Mistargeted mitochondrial proteins activate a proteostatic response in the cytosol. Nature 524 (7566), 485–488. doi:10.1038/nature14951
Wui Ng, M. Y., Wai, T., and Simonsen, A. (2021). Quality control of the mitochondrion. Dev. Cell. 56 (7), 881–905. doi:10.1016/j.devcel.2021.02.009
Yonashiro, R., Ishido, S., Kyo, S., Fukuda, T., Goto, E., Matsuki, Y., et al. (2006). A novel mitochondrial ubiquitin ligase plays a critical role in mitochondrial dynamics. EMBO J. 25 (15), 3618–3626. doi:10.1038/sj.emboj.7601249
Zhang, Y., Wu, Y., Zhang, M., Li, Z., Liu, B., Liu, H., et al. (2023). Synergistic mechanism between the endoplasmic reticulum and mitochondria and their crosstalk with other organelles. Cell. Death Discov. 9 (1), 51. doi:10.1038/s41420-023-01353-w
Keywords: mitochondria, cell stress response, inter-organelle crosstalk, yeast, retrograde signaling pathway, autophagy, mitophagy
Citation: Guaragnella N, Di Noia MA and Primavera A (2024) Mitochondrial (dys) function: a double edge sword in cell stress response. Front. Cell. Death 3:1467272. doi: 10.3389/fceld.2024.1467272
Received: 19 July 2024; Accepted: 04 December 2024;
Published: 18 December 2024.
Edited by:
Rosa Bonaventura, National Research Council (CNR), ItalyReviewed by:
Shirisha Nagotu, Indian Institute of Technology Guwahati, IndiaCopyright © 2024 Guaragnella, Di Noia and Primavera. This is an open-access article distributed under the terms of the Creative Commons Attribution License (CC BY). The use, distribution or reproduction in other forums is permitted, provided the original author(s) and the copyright owner(s) are credited and that the original publication in this journal is cited, in accordance with accepted academic practice. No use, distribution or reproduction is permitted which does not comply with these terms.
*Correspondence: Nicoletta Guaragnella, bmljb2xldHRhLmd1YXJhZ25lbGxhQHVuaWJhLml0