- Department of Physiology and Cellular Biophysics, Columbia University Irving Medical Center, New York, NY, United States
Ferroptosis is a specific form of non-apoptotic cell death that is driven by iron-dependent phospholipid peroxidation. Research over the past decade has contributed to our understanding of how this important cell death process is regulated in mammalian systems, especially with regard to the distinct modes of induction, the role of metabolic signals, the organelles involved, implications of ferroptosis for development and aging, and how our improved understanding of the process can be exploited for therapeutic purposes. Other studies have described variants of this ancient cell death process in cyanobacteria, plants and protozoans. Emerging evidence indicates that a ferroptosis-like form of cell death exists in fruit flies (Drosophila melanogaster). Due to the extensive homology of genes in Drosophila melanogaster and Drosophila suzukii, unique aspects of ferroptosis in Drosophila melanogaster may be of particular relevance for developing targeted pesticides against Drosophila suzukii—a major invasive agricultural pest of the berry and wine industry in Southeast Asia, Europe and America. Further, aspects of ferroptosis in Drosophila melanogaster that are conserved in insects in general may provide the basis for identifying new insecticides for controlling the spread of vector-borne diseases such as malaria. In this perspective, we highlight some of the studies in Drosophila melanogaster that have sought to improve our understanding of the ferroptosis-like form of cell death that operates in this organism and conclude with a discussion of the opportunities and challenges for studying this phenomenon in fruit flies.
Introduction
Ferroptosis is a nonapoptotic form of cell death that is triggered by iron-dependent lipid peroxidation (Stockwell et al., 2017). It has been implicated in ischemic organ injury and neurodegeneration. Drug-resistant tumors are very vulnerable to ferroptosis. Consequently, pharmacological suppression or induction of ferroptosis holds great therapeutic promise for the treatment of many diseases.
The execution of ferroptosis depends on the transition metal ion, ferrous iron (Fe2+), reactive oxygen species (ROS), and phospholipids containing polyunsaturated fatty acid (PUFA) side chains (Figure 1) (Yang and Stockwell, 2016; Stockwell et al., 2017; Yan et al., 2021a). A major regulator of ferroptosis is the micronutrient selenium, required for the biosynthesis of ROS-scavenging selenoproteins such as glutathione peroxidase 4 (GPX4). GPX4 specifically reduces phospholipid hydroperoxides and oxidized lipoproteins to their native state; and several studies in mammalian systems have shown that disruption of GPX4 triggers lipid peroxidation and ferroptosis (reviewed in (Li et al., 2020)). The function of GPX4 is connected to an antiporter called system xc−. System xc− is a cystine/glutamate antiporter as it transports cystine (the oxidized form of cysteine) into cells while concurrently extruding glutamate. Cystine, through a series of biochemical reactions, ultimately suppresses ferroptosis by contributing to GPX4 activity. RSL3 and erastin are chemical inhibitors of GPX4 and the system xc− antiporter, respectively, and are frequently used to induce ferroptosis. In this perspective, we trace some of the studies that have informed our understanding and proposal of a ferroptosis-like form of cell death in Drosophila melanogaster (Dm).
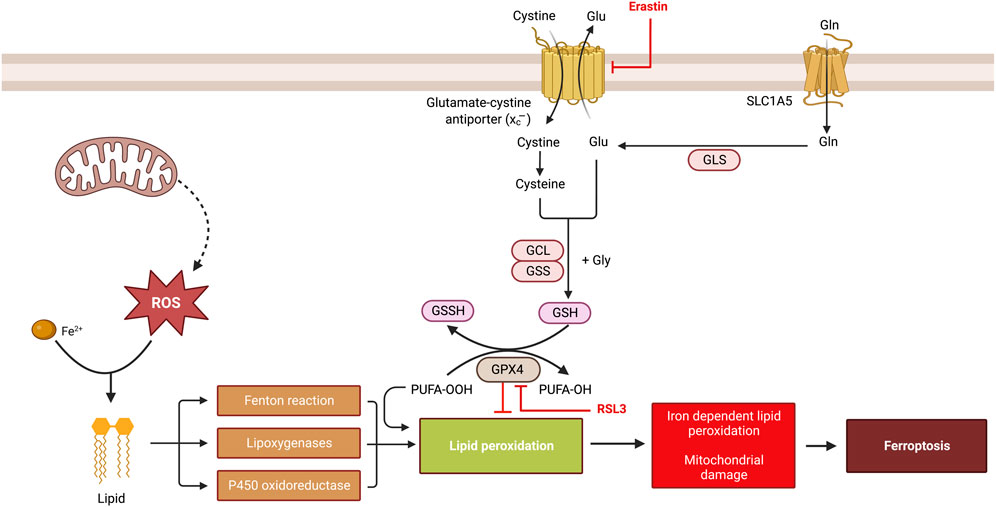
FIGURE 1. An overview of ferroptosis. Ferroptosis is a form of regulated cell death that is characterized by shrunken mitochondria with preserved nuclei and is triggered by iron-dependent lipid peroxidation. A central regulator of ferroptosis is glutathione peroxidase 4 (GPX4), which uses the cofactor, glutathione, to degrade lipid peroxides. During glutathione synthesis, cystine (the oxidized form of cysteine) can be imported into cells via the system xc–cystine/glutamate antiporter—a transmembrane protein complex containing subunits SLC7A11 and SLC3A2 that can be inhibited by erastin. Following conversion to cysteine, glycine and glutamate can combine with cysteine to generate the reduced form of glutathione. Thus, a disruption of GPX4 or any other component in the glutathione biosynthesis pathway increases lipid peroxidation. Lipid peroxidation can also result from the activity of various lipoxygenases, cytochrome P450 oxidoreductases and the Fenton reaction as shown. RSL3 is an inhibitor of GPX4.
A rudimentary form of ferroptosis has been observed in multiple organisms
Although most studies on ferroptosis have been performed in mammalian systems, an ancestral form of ferroptotic cell death has been observed in organisms ranging from cyanobacteria and plants to Saccharomyces pombe and trypanosomes (Bogacz and Krauth-Siegel, 2018; Aguilera et al., 2022; Liu et al., 2022). In Saccharomyces pombe, a ferroptosis-like form of cell death has been observed in response to deletion of a pentatricopeptide repeat (PPR) gene, ppr2, which encodes a general mitochondrial translation factor (Liu et al., 2022). In the African trypanosome, Trypanosoma brucei, mutations in tryparedoxin peroxidase, an ortholog of glutathione peroxidase 4, results in several ultrastructural changes in mitochondria that are reminiscent of ferroptosis. Importantly, the lethal phenotype associated with knocking out ppr2 or tryparedoxin peroxidase is suppressed by established ferroptosis inhibitors such as deferoxamine and ferrostatin-1 (Schaffroth et al., 2016; Bogacz and Krauth-Siegel, 2018; Liu et al., 2022). Similarly, a form of ferroptosis induced in response to thermal stress and dubbed c-ferroptosis has been described in the cyanobacterium, Synechocystis sp. PCC 6803, as it shows many of the hallmarks observed in mammalian ferroptosis (Aguilera et al., 2022). In Arabidopsis thaliana, a ferroptosis-like form of cell death is induced in response to thermal stress at 55°C but not 77°C and also bears many biochemical similarities to mammalian ferroptosis (Distefano et al., 2017). For instance, glutathione depletion and other markers of oxidative stress are also observed in the ferroptosis-like form of cell death in Arabidopsis thaliana, which could be rescued by deuterated polyunsaturated fatty acids; but in contrast to mammalian systems, it can be suppressed by calcium chelators as well (Distefano et al., 2017). Taken together, these observations provide strong evidence that at least a rudimentary form of ferroptosis can be induced in many organisms.
Consequently, a number of research groups have performed studies that point to the existence of a ferroptosis-like form of cell death in Dm. Here, we summarize some of the findings in fruit flies that have informed our understanding of ferroptosis, some open questions on ferroptosis that can be addressed in Dm, and a discussion of the challenges and opportunities for studying this phenomenon in this organism.
Iron homeostasis in Drosophila melanogaster
In mammalian systems, ferroptosis is linked to the regulation of iron abundance (Stockwell, 2022). Accordingly, studies of iron regulation in fruit flies should provide clues on how the ferroptosis-like form of cell death in fruit flies is regulated. In this regard, it has been shown that homozygous mutants of the iron-chelating proteins, ferritin 1 heavy chain homolog (Fer1HCH) and ferritin 2 light chain homolog (Fer2LCH)—both of which have significant homology to the human iron-chelating proteins, FTH1, FTHL17, FTMT, and FTL—are larval lethal (Missirlis et al., 2007; Li, 2010); but homozygous mutants of Mitoferrin, a mitochondrial iron transporter, survive to adulthood (Metzendorf and Lind, 2010). Homozygous mutants of ZIP13, a zinc and iron permease family member that normally supplies iron to ferritin, are also larval lethal (Zhao and Zhou, 2020). However, a ferroptosis-like form of cell death has not yet been explored in any of these whole-organism mutants (Missirlis et al., 2007; Li, 2010; Metzendorf and Lind, 2010; Zhao and Zhou, 2020), although it has been reported that mutant Fer2LCH wing disc clones have mitochondrial defects that are reminiscent of ferroptosis (Mumbauer et al., 2019). Another study showed that overexpression of ZIP13 in the midgut is sufficient to rescue the lethality of whole-organism ZIP13 mutants (Zhao and Zhou, 2020). Moreover, RNAi-mediated knockdown and overexpression of ferritin in the whole midgut leads to an accumulation of iron in the intestine and a reduction in total body iron, respectively (Tang and Zhou, 2013). These results indicate that the midgut is an essential organ for iron regulation and may be an important organ for investigating a ferroptosis-like form of cell death in fruit flies. Indeed, investigating a putative Dm form of ferroptosis in the gut would have the added advantage of being in an organ that should be readily accessible to various inhibitors and activators of ferroptosis added to various dietary regimens.
Studies in mammalian systems have revealed that one avenue by which iron enters the circulatory system from the gut involves uptake of ferrous iron by ferroportin in the basolateral membrane. After oxidation to ferric iron, it is then taken up by transferrin with the assistance of hephaestin—a multi-copper oxidase (MCO). At least 4 paralogs of MCO have been described in Dm (MCO1-4) (Dittmer and Kanost, 2010). Among these paralogs, MCO3 mutants may be particularly relevant for studying ferroptosis, as they display elevated iron stores in the whole body (Wang et al., 2018).
Iron homeostasis can also be regulated by the iron-responsive element/iron-regulatory protein (IRE/IRP) regulatory network. Although there are two IRPs in Dm – IRP1A and IRP1B – only IRP1A has been shown to bind to IREs (Lind et al., 2006). When iron levels fall significantly, IRP1A binds to IREs in the 3’ UTRs of Fer1HCH and the gene encoding succinate dehydrogenase B (sdhb) to inhibit their translation (Nichol et al., 2002; Dunkov and Georgieva, 2006). In contrast, when iron levels rise, IRP1A is involved in Fe–S cluster biogenesis or translocates to the nucleus to limit the expression of genes involved in steroid hormone biosynthesis and other biosynthetic processes that use iron as a co-factor (Huynh et al., 2019; Hernandez-Gallardo and Missirlis, 2020). It would be interesting to investigate how disruption of IRP1A when iron levels rise impinges on the ferroptosis-like form of cell death induced in fruit flies, in view of the fact that in mammalian systems knockdown of IRP2 promotes erastin-induced cell death (Reed and Pellecchia, 2012).
The mitochondrion is an important organelle for regulating iron homeostasis. Excess iron can be buffered by mitochondrial ferritin—encoded by Fer3HCH. It has been reported that ubiquitous overexpression of mitochondrial ferritin in Dm improves resistance to oxidative stress and confers tolerance to erastin-induced lethality; however, how this impinges on the biochemical and molecular markers of ferroptosis has not been explored (Missirlis et al., 2006; Wang et al., 2016). The link between mitochondrial dysfunction and ferroptosis is an attractive topic to study as the enhanced superoxide production that typically occurs in response to mitochondrial dysfunction may react with ferrous iron to trigger Fenton reactions and subsequent lipid peroxidation. With the availability of many mitochondrial mutants, transgenic RNAi and CRISPR constructs that target mitochondrial proteins, this is perhaps the area of investigation where fruit fly genetics may contribute the most to our understanding of ferroptosis.
A ferroptosis-like form of cell death is induced in response to disruption of isocitrate dehydrogenase 2 in flight muscles
To begin to uncover a possible link between mitochondrial dysfunction and ferroptosis in Dm, we explored the effect of disrupting mitochondrial NADPH production in Dm thoraces as a result of RNAi-mediated knockdown of isocitrate dehydrogenase 2 (IDH2) (Murari et al., 2022). Blue native polyacrylamide gel electrophoresis revealed that knockdown of IDH2 impairs the assembly of the oxidative phosphorylation (OXPHOS) system. The extent of OXPHOS disintegration depends on the extent of disruption of IDH2, with only complex I assembly impaired when IDH2 disruption is mild (using a weaker transgenic RNAi construct), but with multiple OXPHOS complexes impaired when knockdown of IDH2 is severe. Additionally, several pro-ferroptotic markers, such as ROS, lipid peroxidation and labile iron were all increased in mitochondria isolated from the IDH2 mutant flies. Notably, the ferroptosis hallmarks of preserved nuclear integrity and shrunken mitochondria were observed in transmission electron micrographs of flight muscles from IDH2 mutant flies; and raising these flies on two ferroptosis inhibitors—ferrostatin-1 and liproxstatin-1—potently rescued their ferroptosis ultrastructural phenotypes and early lethality. Importantly, although the longevity of fruit flies with severe IDH2 knockdown in flight muscles was severely reduced to about 10 days at 25°C, there was no evidence of elevated caspase activity, which rules out apoptosis as a major cell death mechanism in these samples. Overall, these data indicate that a ferroptosis-like form of cell death is induced in flight muscles as a result of severely disrupting IDH2 function.
However, several questions remain unanswered about this phenotype. For instance, numerous studies in mammalian systems have shown that the predominant membrane-associated lipids that undergo peroxidation during ferroptosis are phospholipids with PUFA side chains such as arachidonic acid (20:4) and adrenic acid (22:4) (Kagan et al., 2017). Nevertheless, it has been consistently shown that fruit flies do not appear to have the capacity to synthesize C20 and C22 PUFAs, even when fed a diet containing the PUFA precursors linoleic acid and α-linolenic acid (Yoshioka et al., 1985; Shen et al., 2010). This observation is further buttressed by the fact that fruit flies lack the genes for Δ5 and Δ6 fatty acid desaturases—essential enzymes in the pathways for the biosynthesis of PUFAs like arachidonic, eicosapentaenoic and docosahexaenoic acids. Thus, in contrast to mammalian systems, peroxidation of arachidonic acid may not be a prerequisite for the execution of a ferroptosis-like form of cell death in Dm. This would have major ramifications for how a ferroptosis-like form of cell death is regulated in Dm, as it will indicate that at least some of the enzymes driving lipid peroxidation in fruit flies are likely to be different from those required in mammalian systems. For instance, in mammalian systems, iron-dependent enzymes such as arachidonate lipoxygenases (ALOX) appear to have a role in the formation of lipid hydroperoxides that precipitate ferroptosis (Kuhn et al., 2015; Yang et al., 2016; Wenzel et al., 2017); although other studies have questioned this result (Shah et al., 2018). With their inability to synthesize C20 and C22 PUFAs and lack of readily identifiable ALOX orthologs, it appears other enzymes may take up this role in Dm.
Interestingly, cytochrome P450 oxidoreductase (POR) also promotes phospholipid peroxidation during ferroptosis, most likely in conjunction with cytochrome b5 reductase (CYB5R1) (Zou et al., 2020; Yan et al., 2021b). Genetic ablation of POR and CYB5R1 decreases ROS production, prevents lipid peroxidation and inhibits ferroptosis (Ghosh et al., 1997; Zou et al., 2020; Yan et al., 2021b). As several cytochrome reductases exist in Dm and cytochrome reductases have been implicated in xenobiotic detoxification in insects (Nauen et al., 2022), phospholipid peroxidation by POR may be an aspect of mammalian ferroptosis that is conserved in fruit flies.
Challenges and opportunities for exploring how the ferroptosis-like form of cell death in Drosophila melanogaster is regulated
Unlike other cellular degradative processes such as autophagy and apoptosis where bona fide markers exist, definitive markers of ferroptosis remain to be found. This has been particularly problematic for Drosophila geneticists as only a handful of studies have sought to examine ferroptosis in Dm (Table 1). However, most investigators of ferroptosis in mammalian systems use suppression of the cell death process by established ferroptosis inhibitors as evidence of ferroptosis (Ide et al., 2021; Ide and Souma, 2022; Mishima et al., 2022; Hirata et al., 2023; Ryan et al., 2023). A previous elegant review by Rui Kang and colleagues described a range of morphologic, genetic, biochemical, and protein hallmarks of ferroptosis, while also pointing out the ambiguity of using any of these markers and the importance of suppressing any putative ferroptosis phenotype with established ferroptosis inhibitors (Chen et al., 2021). With persistent debate in the field about the need for a uniform definition of markers for ferroptosis, recently Brent Stockwell proposed that in mammalian systems at least three of the following four indices must be present for the cell death process to be regarded as ferroptosis: 1) Shrunken and dense mitochondria in transmission electron micrographs; 2) Lipid peroxidation; 3) Transcriptional induction of CHAC1, PTGS2, SLC7A11 and ACSL4, and transcriptional downregulation of RGS4; and 4) Increased abundance and plasma localization of the transferrin receptor, TfR1 (Stockwell, 2022). Consequently, perhaps the first challenge that would have to be resolved quickly involves identifying suitable ferroptosis markers in Dm. Of the four ferroptosis markers proposed for mammalian systems, the latter two are difficult to test in Dm. In this regard, it is unclear which of the many transferin receptor paralogs in fruit flies would be mobilized to the plasma membrane when ferroptosis is induced, and for which an antibody needs to be generated. It is also ambiguous as to whether the five genes proposed as transcriptional markers of ferroptosis in mammalian systems behave similarly in fruit flies.
IDH2 is a component of a complex NADPH-dependent ROS-detoxifying system that operates in both the cytosol and mitochondrion (Figure 2). Hydrogen peroxide generated in mitochondria can be detoxified by an NADPH-thioredoxin-peroxiredoxin branch of the system (NADPH-TXN-PRDX system) or by an alternate branch, the NADPH-glutathione-glutathione peroxidase system (NADPH-GSH-GPX system). An analogous dual NADPH-dependent ROS detoxifying system operates in the cytosol as well (Figure 2). It would be interesting to explore how other components of this NADPH-linked ROS detoxifying network regulate the mode of ferroptosis that occurs in Dm.
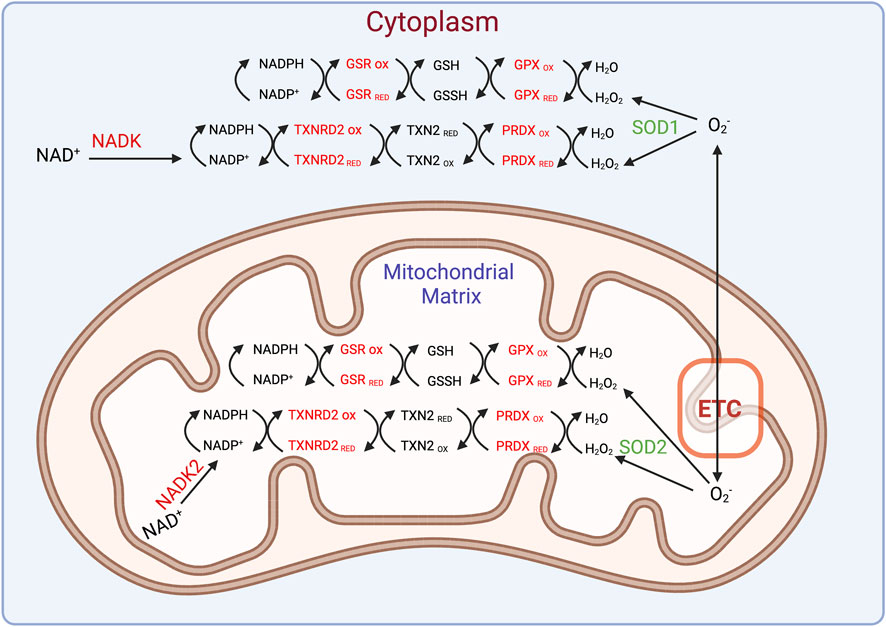
FIGURE 2. An intricate ROS scavenging network operates in the mitochondrion and cytosol. The superoxide radical can be generated in the mitochondrion or elsewhere and converted into hydrogen peroxide by superoxide dismutases 1 (cytosolic) or 2 (mitochondrial). The ensuing hydrogen peroxide can be detoxified through a series of redox reactions involving several enzymes such as GPXs, glutathione reductase (GSR), peroxiredoxin (PRDX), and thioredoxin reductase 2 (TXNRD2) as shown.
Acyl-CoA synthetase long chain family member 4 (ACSL4) and lysophosphatidylcholine acyltransferase 3 (LPCAT3) are two regulators of lipid metabolism that have been implicated in ferroptosis in mammalian cells (reviewed in (Stockwell, 2022)). ACSL4 has the ability to ligate long chain PUFAs such as arachidonic acid with coenzyme A, which can subsequently be esterified into phospholipids by various LPCATs. Although orthologs of ACSL4 and LPCAT3 exist in Dm, it is unclear if they regulate ferroptosis in this organism in view of their apparent lack of arachidonic acid. Orthologs of components of the system xc− antiporter, GPX4 and several other regulators of ferroptosis in mammalin systems exist in fruit flies; but whether and how they contribute to the ferroptosis-like form of cell death is unknown. Interestingly, in Dm, GST-S1 performs a function analogous to GPX4 in mammals as it is required for the conjugation of lipid peroxidation end products (Singh et al., 2001). Therefore, it is essential to identify the role of all the basal regulators of ferroptosis in Dm, and subsequently identify and functionally characterize their interactomes, to elucidate conserved and Dm-specific aspects of ferroptosis induction.
RNA-sequencing analyses uncovered several genes implicated in lipid metabolism that are induced in response to IDH2 disruption in flight muscles that could have implications for the regulation of the ferroptosis-like form of cell death in Dm (Murari et al., 2022). Among them are acyl-CoA oxidase 3, acyl-CoA binding protein 6, regulators of triglyceride homeostasis in the endoplasmic reticulum and several lipases. In mammalian systems, at least one lipase—a phospholipase referred to as PLA2G6—has been implicated in ferroptosis via its ability to eliminate oxidised PUFA tails from phospholipids. A macroscopic examination of the flies with impaired IDH2 expression in thoraces revealed emaciated abdomens that were potently rescued by treatment with ferrostatin-1 and liproxstatin-1, perhaps a reflection of the enhanced lipolysis of fat stores in the abdomen. Accordingly, it would be interesting to examine how the multiple regulators of lipid metabolism induced in response to IDH2 disruption contribute to the ferroptosis-like form of cell death in Dm.
Other genes induced in IDH2-knockdown thoraces are regulators of Fe-S cluster biosynthesis, general regulators of oxidative stress and apoptosis, DNA repair genes, and regulators of protein homeostasis (Murari et al., 2022). The upregulation of regulators of the mitochondrial unfolded protein response (UPRmt) provides an opportunity to study how oxidised lipids impact the UPRmt. Also of note was the upregulation of several long non-coding RNAs, which can be explored for possible regulatory links to ferroptosis induction. We note, however, that none of the transcriptional ferroptosis markers in mammalian systems were readily apparent as robustly altered suitable markers in the RNA-sequencing dataset. However, the RNA sequencing experiment was performed four days after the flies eclosed as adults; so we cannot exclude the possibility that quantitative RT-PCR over a time series—perhaps between one and seven days after the flies eclose—would confirm the authenticity of these markers. Finally, although knockdown of IDH2 was restricted to the flight muscles, there appears to be a cell non-autonomous component, as several molecules implicated in signaling such as rasp, Ilp8, Fst, TotM, Diedel, Ag5r2 and CG16995 were induced alongside a number of metabolite transporters. Therefore, it would also be worth exploring how these genes, and possibly metabolites, impinge on interorgan communication triggered by inducing a ferroptotic-like form of cell death in Dm flight muscles.
As is apparent for other modes of mitochondrial dysfunction, IDH2 knockdown activates a compensatory signaling cascade involving multiple stress-responsive kinases (Murari et al., 2022). Interestingly, in mammalian systems, activation of AMPK during ferroptosis leads to the phosphorylation of acetyl-CoA carboxylase to limit PUFA biosynthesis (Lee et al., 2020). It would be interesting to dissect what the phosphorylation targets are for all kinases activated during the ferroptosis-like form of cell death in Dm, and how their phosphorylation contributes to ferroptosis.
Finally, other muscle degenerative pathways that operate in fruit flies are the age-dependent accumulation of misfolded protein aggregates (polyubiquitin aggregates) and muscle degeneration induced as part of systemic organ wasting in gut cancer models (Demontis and Perrimon, 2010; Kwon et al., 2015). Once a working understanding of the fundamentals of ferroptosis has been established in fruit flies, it will be necessary to examine how ferroptosis intersects with these other muscle degenerative processes.
Concluding remarks
The stage is set for pursuing studies in Dm aimed at uncovering how a ferroptotic-like form of cell death is regulated in this organism. Different tissues in Dm may be suitable for addressing different aspects of ferroptosis. For instance, the high lipid content of the fat body may be more appropriate for providing answers about how lipid metabolism regulates ferroptosis than an organ such as the flight muscles, which may be more suitable for dissecting the importance of mitochondria in the process. Just as differences exist between how other cell degradative processes such as apoptosis and autophagy are regulated in fruit flies and mammalian systems, we anticipate that there are likely to be Dm-specific mechanisms of ferroptosis induction. For instance, chaperone-mediated autophagy (CMA) has not been observed in Dm, and the importance of cytochrome c release during apoptosis in fruit flies is still debated (Dorstyn et al., 2004; Clavier et al., 2016; Hung et al., 2021). The absence of CMA would likely have implications for ferroptosis regulation in Dm as in mammalian systems, CMA regulates degradation of GPX4 (Wu et al., 2019). Nevertheless, there are likely to be areas of synergy between fruit fly and mammalian research on ferroptosis that would improve our understanding of ferroptosis. Due to its close relatedness to Dm, unique aspects of ferroptosis in Dm may be of particular relevance for developing targeted pesticides against spotted-wing Drosophila (Drosophila suzukii), a major invasive agricultural pest of the berry and wine industry in Southeast Asia, Europe and America that causes annual losses of more than $500 million (Tait et al., 2021). Finally, aspects of ferroptosis in Dm that are conserved in insects in general may provide the basis for identifying new insecticides for controlling the spread of vector-borne diseases such as malaria.
Author contributions
EO-A conceived and wrote the review, with some feedback from SS. Both authors made the figure. All authors contributed to the article and approved the submitted version.
Funding
Funding for this perspective was provided by a Schaefer Research Scholars Award, NIH grant GM147902-01A1 (R01) and start-up funds from the Department of Physiology and Cellular Biophysics of the Columbia University Irving Medical Center to EO-A. SS was supported by the aforementioned funds to EO-A.
Acknowledgments
We thank members of the Owusu-Ansah lab for general discussions.
Conflict of interest
The authors declare that the research was conducted in the absence of any commercial or financial relationships that could be construed as a potential conflict of interest.
The author SS declared that they were an editorial board member of Frontiers, at the time of submission. This had no impact on the peer review process and the final decision.
Publisher’s note
All claims expressed in this article are solely those of the authors and do not necessarily represent those of their affiliated organizations, or those of the publisher, the editors and the reviewers. Any product that may be evaluated in this article, or claim that may be made by its manufacturer, is not guaranteed or endorsed by the publisher.
References
Aguilera, A., Berdun, F., Bartoli, C., Steelheart, C., Alegre, M., Bayir, H., et al. (2022). C-ferroptosis is an iron-dependent form of regulated cell death in cyanobacteria. J. Cell. Biol. 221 (2), e201911005. doi:10.1083/jcb.201911005
Bogacz, M., and Krauth-Siegel, R. L. (2018). Tryparedoxin peroxidase-deficiency commits trypanosomes to ferroptosis-type cell death. Elife 7, e37503. doi:10.7554/eLife.37503
Chen, X., Comish, P. B., Tang, D., and Kang, R. (2021). Characteristics and biomarkers of ferroptosis. Front. Cell. Dev. Biol. 9, 637162. doi:10.3389/fcell.2021.637162
Clavier, A., Rincheval-Arnold, A., Colin, J., Mignotte, B., and Guénal, I. (2016). Apoptosis in Drosophila: Which role for mitochondria? Apoptosis 21 (3), 239–251. doi:10.1007/s10495-015-1209-y
Demontis, F., and Perrimon, N. (2010). FOXO/4E-BP signaling in Drosophila muscles regulates organism-wide proteostasis during aging. Cell. 143 (5), 813–825. doi:10.1016/j.cell.2010.10.007
Distefano, A. M., Martin, M. V., Córdoba, J. P., Bellido, A. M., D'Ippólito, S., Colman, S. L., et al. (2017). Heat stress induces ferroptosis-like cell death in plants. J. Cell. Biol. 216 (2), 463–476. doi:10.1083/jcb.201605110
Dittmer, N. T., and Kanost, M. R. (2010). Insect multicopper oxidases: Diversity, properties, and physiological roles. Insect Biochem. Mol. Biol. 40 (3), 179–188. doi:10.1016/j.ibmb.2010.02.006
Dorstyn, L., Mills, K., Lazebnik, Y., and Kumar, S. (2004). The two cytochrome c species, DC3 and DC4, are not required for caspase activation and apoptosis in Drosophila cells. J. Cell. Biol. 167 (3), 405–410. doi:10.1083/jcb.200408054
Dunkov, B., and Georgieva, T. (2006). Insect iron binding proteins: Insights from the genomes. Insect Biochem. Mol. Biol. 36 (4), 300–309. doi:10.1016/j.ibmb.2006.01.007
Ghosh, M. K., Mukhopadhyay, M., and Chatterjee, I. B. (1997). NADPH-Initiated cytochrome P450-dependent free iron-independent microsomal lipid peroxidation: Specific prevention by ascorbic acid. Mol. Cell. Biochem. 166 (1-2), 35–44. doi:10.1023/a:1006841228483
Hernandez-Gallardo, A. K., and Missirlis, F. (2020). Cellular iron sensing and regulation: Nuclear IRP1 extends a classic paradigm. Biochim. Biophys. Acta Mol. Cell. Res. 1867 (7), 118705. doi:10.1016/j.bbamcr.2020.118705
Hirata, Y., Cai, R., Volchuk, A., Steinberg, B. E., Saito, Y., Matsuzawa, A., et al. (2023). Lipid peroxidation increases membrane tension, Piezo1 gating, and cation permeability to execute ferroptosis. Curr. Biol. 33 (7), 1282–1294 e5. doi:10.1016/j.cub.2023.02.060
Hung, Y. C., Huang, K. L., Chen, P. L., Li, J. L., Lu, S. H. A., Chang, J. C., et al. (2021). UQCRC1 engages cytochrome c for neuronal apoptotic cell death. Cell. Rep. 36 (12), 109729. doi:10.1016/j.celrep.2021.109729
Huynh, N., Ou, Q., Cox, P., Lill, R., and King-Jones, K. (2019). Glycogen branching enzyme controls cellular iron homeostasis via Iron Regulatory Protein 1 and mitoNEET. Nat. Commun. 10 (1), 5463. doi:10.1038/s41467-019-13237-8
Ide, K., and Souma, T. (2022). In vivo assessment of ferroptosis and ferroptotic stress in mice. Curr. Protoc. 2 (4), e413. doi:10.1002/cpz1.413
Ide, S., Kobayashi, Y., Ide, K., Strausser, S. A., Abe, K., Herbek, S., et al. (2021). Ferroptotic stress promotes the accumulation of pro-inflammatory proximal tubular cells in maladaptive renal repair. Elife 10, e68603. doi:10.7554/eLife.68603
Kagan, V. E., Mao, G., Qu, F., Angeli, J. P. F., Doll, S., Croix, C. S., et al. (2017). Oxidized arachidonic and adrenic PEs navigate cells to ferroptosis. Nat. Chem. Biol. 13 (1), 81–90. doi:10.1038/nchembio.2238
Kuhn, H., Banthiya, S., and van Leyen, K. (2015). Mammalian lipoxygenases and their biological relevance. Biochim. Biophys. Acta 1851 (4), 308–330. doi:10.1016/j.bbalip.2014.10.002
Kwon, Y., Song, W., Droujinine, I. A., Hu, Y., Asara, J. M., and Perrimon, N. (2015). Systemic organ wasting induced by localized expression of the secreted insulin/IGF antagonist ImpL2. Dev. Cell. 33 (1), 36–46. doi:10.1016/j.devcel.2015.02.012
Lee, H., Zandkarimi, F., Zhang, Y., Meena, J. K., Kim, J., Zhuang, L., et al. (2020). Energy-stress-mediated AMPK activation inhibits ferroptosis. Nat. Cell. Biol. 22 (2), 225–234. doi:10.1038/s41556-020-0461-8
Li, J., Cao, F., Yin, H. L., Huang, Z. J., Lin, Z. T., Mao, N., et al. (2020). Ferroptosis: Past, present and future. Cell. Death Dis. 11 (2), 88. doi:10.1038/s41419-020-2298-2
Li, S. (2010). Identification of iron-loaded ferritin as an essential mitogen for cell proliferation and postembryonic development in Drosophila. Cell. Res. 20 (10), 1148–1157. doi:10.1038/cr.2010.102
Lind, M. I., Missirlis, F., Melefors, O., Uhrigshardt, H., Kirby, K., Phillips, J. P., et al. (2006). Of two cytosolic aconitases expressed in Drosophila, only one functions as an iron-regulatory protein. J. Biol. Chem. 281 (27), 18707–18714. doi:10.1074/jbc.M603354200
Liu, Z., Ebrahim, A., Wu, X., Li, M., and Huang, Y. (2022). Loss of PPR protein Ppr2 induces ferroptosis-like cell death in Schizosaccharomyces pombe. Arch. Microbiol. 204 (7), 360. doi:10.1007/s00203-022-02970-2
Metzendorf, C., and Lind, M. I. (2010). Drosophila mitoferrin is essential for male fertility: Evidence for a role of mitochondrial iron metabolism during spermatogenesis. BMC Dev. Biol. 10, 68. doi:10.1186/1471-213X-10-68
Mishima, E., Ito, J., Wu, Z., Nakamura, T., Wahida, A., Doll, S., et al. (2022). A non-canonical vitamin K cycle is a potent ferroptosis suppressor. Nature 608 (7924), 778–783. doi:10.1038/s41586-022-05022-3
Missirlis, F., Holmberg, S., Georgieva, T., Dunkov, B. C., Rouault, T. A., and Law, J. H. (2006). Characterization of mitochondrial ferritin in Drosophila. Proc. Natl. Acad. Sci. U. S. A. 103 (15), 5893–5898. doi:10.1073/pnas.0601471103
Missirlis, F., Kosmidis, S., Brody, T., Mavrakis, M., Holmberg, S., Odenwald, W. F., et al. (2007). Homeostatic mechanisms for iron storage revealed by genetic manipulations and live imaging of Drosophila ferritin. Genetics 177 (1), 89–100. doi:10.1534/genetics.107.075150
Mumbauer, S., Pascual, J., Kolotuev, I., and Hamaratoglu, F. (2019). Ferritin heavy chain protects the developing wing from reactive oxygen species and ferroptosis. PLoS Genet. 15 (9), e1008396. doi:10.1371/journal.pgen.1008396
Murari, A., Goparaju, N. S. V., Rhooms, S. K., Hossain, K. F. B., Liang, F. G., Garcia, C. J., et al. (2022). IDH2-mediated regulation of the biogenesis of the oxidative phosphorylation system. Sci. Adv. 8 (19), eabl8716. doi:10.1126/sciadv.abl8716
Nauen, R., Bass, C., Feyereisen, R., and Vontas, J. (2022). The role of cytochrome P450s in insect toxicology and resistance. Annu. Rev. Entomol. 67, 105–124. doi:10.1146/annurev-ento-070621-061328
Nichol, H., Law, J. H., and Winzerling, J. J. (2002). Iron metabolism in insects. Annu. Rev. Entomol. 47, 535–559. doi:10.1146/annurev.ento.47.091201.145237
Reed, J. C., and Pellecchia, M. (2012). Ironing out cell death mechanisms. Cell. 149 (5), 963–965. doi:10.1016/j.cell.2012.05.009
Ryan, S. K., Zelic, M., Han, Y., Teeple, E., Chen, L., Sadeghi, M., et al. (2023). Microglia ferroptosis is regulated by SEC24B and contributes to neurodegeneration. Nat. Neurosci. 26 (1), 12–26. doi:10.1038/s41593-022-01221-3
Schaffroth, C., Bogacz, M., Dirdjaja, N., Nißen, A., and Krauth-Siegel, R. L. (2016). The cytosolic or the mitochondrial glutathione peroxidase-type tryparedoxin peroxidase is sufficient to protect procyclic Trypanosoma brucei from iron-mediated mitochondrial damage and lysis. Mol. Microbiol. 99 (1), 172–187. doi:10.1111/mmi.13223
Shah, R., Shchepinov, M. S., and Pratt, D. A. (2018). Resolving the role of lipoxygenases in the initiation and execution of ferroptosis. ACS Cent. Sci. 4 (3), 387–396. doi:10.1021/acscentsci.7b00589
Shen, L. R., Lai, C. Q., Feng, X., Parnell, L. D., Wan, J. B., Wang, J. D., et al. (2010). Drosophila lacks C20 and C22 PUFAs. J. Lipid Res. 51 (10), 2985–2992. doi:10.1194/jlr.M008524
Singh, S. P., Coronella, J. A., Benes, H., Cochrane, B. J., and Zimniak, P. (2001). Catalytic function of Drosophila melanogaster glutathione S-transferase DmGSTS1-1 (GST-2) in conjugation of lipid peroxidation end products. Eur. J. Biochem. 268 (10), 2912–2923. doi:10.1046/j.1432-1327.2001.02179.x
Stockwell, B. R. (2022). Ferroptosis turns 10: Emerging mechanisms, physiological functions, and therapeutic applications. Cell. 185 (14), 2401–2421. doi:10.1016/j.cell.2022.06.003
Stockwell, B. R., Friedmann Angeli, J. P., Bayir, H., Bush, A. I., Conrad, M., Dixon, S. J., et al. (2017). Ferroptosis: A regulated cell death nexus linking metabolism, redox biology, and disease. Cell. 171 (2), 273–285. doi:10.1016/j.cell.2017.09.021
Tait, G., Mermer, S., Stockton, D., Lee, J., Avosani, S., Abrieux, A., et al. (2021). Drosophila suzukii (Diptera: Drosophilidae): A decade of research towards a sustainable integrated pest management program. J. Econ. Entomol. 114 (5), 1950–1974. doi:10.1093/jee/toab158
Tang, X., and Zhou, B. (2013). Ferritin is the key to dietary iron absorption and tissue iron detoxification in Drosophila melanogaster. FASEB J. 27 (1), 288–298. doi:10.1096/fj.12-213595
Wang, X., Yin, S., Yang, Z., and Zhou, B. (2018). Drosophila multicopper oxidase 3 is a potential ferroxidase involved in iron homeostasis. Biochim. Biophys. Acta Gen. Subj. 1862 (8), 1826–1834. doi:10.1016/j.bbagen.2018.04.017
Wang, Y. Q., Chang, S. Y., Wu, Q., Gou, Y. J., Jia, L., Cui, Y. M., et al. (2016). The protective role of mitochondrial ferritin on erastin-induced ferroptosis. Front. Aging Neurosci. 8, 308. doi:10.3389/fnagi.2016.00308
Wenzel, S. E., Tyurina, Y. Y., Zhao, J., St Croix, C. M., Dar, H. H., Mao, G., et al. (2017). PEBP1 wardens ferroptosis by enabling lipoxygenase generation of lipid death signals. Cell. 171 (3), 628–641. doi:10.1016/j.cell.2017.09.044
Wu, Z., Geng, Y., Lu, X., Shi, Y., Wu, G., Zhang, M., et al. (2019). Chaperone-mediated autophagy is involved in the execution of ferroptosis. Proc. Natl. Acad. Sci. U. S. A. 116 (8), 2996–3005. doi:10.1073/pnas.1819728116
Yan, B., Ai, Y., Sun, Q., Ma, Y., Cao, Y., Wang, J., et al. (2021b). Membrane damage during ferroptosis is caused by oxidation of phospholipids catalyzed by the oxidoreductases POR and CYB5R1. Mol. Cell. 81 (2), 355–369 e10. doi:10.1016/j.molcel.2020.11.024
Yan, H. F., Zou, T., Tuo, Q. Z., Xu, S., Li, H., Belaidi, A. A., et al. (2021a). Ferroptosis: Mechanisms and links with diseases. Signal Transduct. Target Ther. 6 (1), 49. doi:10.1038/s41392-020-00428-9
Yang, W. S., Kim, K. J., Gaschler, M. M., Patel, M., Shchepinov, M. S., and Stockwell, B. R. (2016). Peroxidation of polyunsaturated fatty acids by lipoxygenases drives ferroptosis. Proc. Natl. Acad. Sci. U. S. A. 113 (34), E4966–E4975. doi:10.1073/pnas.1603244113
Yang, W. S., and Stockwell, B. R. (2016). Ferroptosis: Death by lipid peroxidation. Trends Cell. Biol. 26 (3), 165–176. doi:10.1016/j.tcb.2015.10.014
Yoshioka, T., Inoue, H., Kasama, T., Seyama, Y., Nakashima, S., Nozawa, Y., et al. (1985). Evidence that arachidonic acid is deficient in phosphatidylinositol of Drosophila heads. J. Biochem. 98 (3), 657–662. doi:10.1093/oxfordjournals.jbchem.a135322
Zhao, M., and Zhou, B. (2020). A distinctive sequence motif in the fourth transmembrane domain confers ZIP13 iron function in Drosophila melanogaster. Biochim. Biophys. Acta Mol. Cell. Res. 1867 (2), 118607. doi:10.1016/j.bbamcr.2019.118607
Keywords: Drosophila, ferroptosis, GPX4, iron, lipid peroxidation, ROS
Citation: Saini S and Owusu-Ansah E (2023) Dissecting the mechanism of regulation of a ferroptosis-like form of cell death in Drosophila melanogaster. Front. Cell. Death 2:1209641. doi: 10.3389/fceld.2023.1209641
Received: 21 April 2023; Accepted: 12 May 2023;
Published: 20 June 2023.
Edited by:
Kenta Moriwaki, Toho University, JapanReviewed by:
Natsuki Shinoda, The University of Tokyo, JapanTomokazu Souma, Duke University, United States
Copyright © 2023 Saini and Owusu-Ansah. This is an open-access article distributed under the terms of the Creative Commons Attribution License (CC BY). The use, distribution or reproduction in other forums is permitted, provided the original author(s) and the copyright owner(s) are credited and that the original publication in this journal is cited, in accordance with accepted academic practice. No use, distribution or reproduction is permitted which does not comply with these terms.
*Correspondence: Edward Owusu-Ansah, ZW8yMzY0QGN1bWMuY29sdW1iaWEuZWR1