- Department of Cellular Neurobiology, Georg-August-University, Göttingen, Germany
Elevated expression of acetylcholinesterase (AChE) is a common characteristic of apoptotic cells in both invertebrate and vertebrate species. While increased levels of acetylcholinesterase sensitize cells to apoptogenic stimuli, its absence or pharmacological inactivation interferes with apoptotic cell death. acetylcholinesterase may exert its pro-apoptotic function directly as an integral component of the apoptotic molecular machinery or indirectly by limiting the availability of receptor ligands and structural binding partners that promote cell survival under non-apoptogenic conditions. acetylcholinesterase promotes formation of the apoptosome and degrades DNA after nuclear accumulation. Its esterase activity limits the availability of acetylcholine as ligand for cell membrane-located nicotinic and muscarinic ACh-receptors and mitochondrial nicotinic ACh-receptors that normally support vital physiological states. Studies on insects suggest, that cytokine-activated cell-protective pathways may suppress acetylcholinesterase overexpression under apoptogenic conditions to prevent apoptotic cell death. We provide an overview of studies on various organisms and cell types that summarizes the contribution of acetylcholinesterase to the progress of apoptosis via multiple mechanisms.
Introduction
The term Apoptosis describes a regulated series of intracellular events that leads to self destruction of individual cells. This form of programmed cell death is associated with particular morphological changes that include, membrane enlargement, disintegration of chromatin structure causing nuclear condensation and destruction of organelles and macromolecules within membrane-surrounded compartments that form through blebbing (Hengartner, 2000; Lawen, 2003). Subsequently, neighbouring cells and/or macrophages detect these apoptotic bodies, engulf the membrane-enclosed debris and remove it from the respective tissues. Since the release of degradation products into the extracellular space is prevented, apoptotic cell death is not followed by inflammatory responses, in contrast to necrotic and other types of cell death (Renehan et al., 2001).
Apoptosis represents a tightly regulated set of mechanisms to remove excess or no longer required cells during development and eliminate damaged, malfunctioning or infected cells to maintain functionality of tissues (Raff, 1998; Hengartner, 2000). The evolutionary origin of the eukaryotic apoptotic system is tightly connected to the endosymbiontic origin of mitochondria, subsequent horizontal gene transfer and recruitment of transducers and effectors into eukaryotic apoptotic pathways (Koonin and Aravind, 2002; Degterev and Yuan, 2008).
In mammalian cells, apoptosis can be initiated by external signals (extrinsic pathway) including death receptor activation (by TNF-α, FasL and apolipoproteins), hormones, X-ray and UV-light amongst others, or internal conditions (intrinsic pathway) including accumulation of reactive oxygen species, oxygen and/or nutrient shortage, toxins, mechanical damage and malfunction of proteins and signaling pathways (Hengartner, 2000; Saelens et al., 2004). Both pathways ultimately activate series of cysteine proteases, both activator caspases that activate other caspases and executor caspases that proteolytically degrade cellular components and activate DNases (Kumar, 2007; McLuskey and Mottram, 2015; Bell and Megeny, 2017).
In the intrinsic pathway, caspase activation is initiated by the apoptosome, a cytosol-located multimolecular adaptor protein complex consisting of several Apaf-1 (protease-activating factor-1), procaspase-9, acetylcholinesterase and cytochrome c, which is released into the cytosol through regulated multimeric pore complexes from mitochondrial intermembraneous compartments (Acehan et al., 2002; Yuan and Akey, 2013). Conformational changes resulting from formation of the apoptosome complex lead to the conversion of procaspase-9 to active caspase-9 which subsequently may activate executioner caspase-3 and other downstream factors that promote or execute the disintegration of cellular components (Earnshaw et al., 1999; Kumar, 2007). Acetylcholinesterase (AChE) hydrolyzes the neurotransmitter acetylcholine and hence terminates synaptic signal transmission at vertebrate neuromuscular junctions and parasympathetic terminals as well as at various central and peripheral cholinergic synapses of vertebrates and invertebrates (Zhang et al., 2002). However, ACh is a phylogenetically ancient universal signaling molecule (already present in prokaryotes) that is produced by the majority of cell types in all organisms and directly or indirectly affects the physiology of any cell via various types of receptors (Wessler and Kirkpatrick, 2020). Consequently, AChE is expressed in various tissues with and without cholinergic innervation and is involved in multiple processes including cellular adhesion, cell growth, cell differentiation, amyloid fiber assembly and apoptosis (Small et al., 1996; Karczmar, 2010; Zhang and Greenberg, 2012; Rotundo, 2017). In addition, catalytically inactive domains with high sequence similarity to AChE, that probably share a common evolutionary origin (Swillens et al., 1986), serve as homo- or heteromeric binding regions for adhesion molecules [neuroligins, neurotactin, gliotactin, glutactin (Grisaru et al., 1999; Cao and Tabuchi, 2017)] and assembly of protein complexes [homodimerization of thyroglobulin (Park and Arvan, 2004)]. Acetylcholinesterase is coded by a single gene in vertebrates but invertebrates may contain multiple AChE genes (nematode Caenorhabditis elegans: 4; most insects: 2). As a result of multiple genes and/or alternative splicing, different AChE molecules with different functions, different affinities to join multimeric complexes and different (sub-)cellular localisation are present in all animals. The vertebrate ACHE gene is spliced into three alternative transcripts that contain identical esterase domains but differ in their carboxy terminal regions which determine localization and interaction with other molecules (Grisaru et al., 1999; Hicks et al., 2011; Rotundo, 2017).
Major splice variants include AChE-S (synaptic), the principal type in brain and muscle, AChE-R (readthrough) expressed in embryonic tissues, tumor cells and in the brain following psychosocial, chemical or physical stress, and AChE-E (erythrocytic) expressed on erythrocyte membranes of some mammals and in muscle fibers of some vertebrates (reviewed in: Grisaru et al., 1999; Zhang and Greenberg, 2012). As studied in mice and humans, the variety of the major splice variants is further increased by alternative promoter usage leading to the selection of different first exons and N-terminal extension of translated AChE protein (Meshorer et al., 2004).
AChE promotes apoptosis
Altered presence and functions of AChE are associated with various degenerative diseases including Alzheimer’s disease, Parkinson’s disease and cancer in various tissues (Small et al., 1996; Perry et al., 2002; Toiber et al., 2008; Zhang et al., 2013).
A growing number of studies indicate that AChE plays a major role as regulator and executor of apoptosis. Apoptogenic stimuli increase AChE levels in vertebrate and invertebrate species. This has been demonstrated in various mammalian tissues (including brain, retina, kidney, colon, endothelial cells, myoblast, bone), cell lines (including PC12, neuroblastoma and HeLa cells) (reviewed in Campoy et al., 2016; Zhang and Greenberg, 2012) and cells from invertebrates including nematodes and insects (Kang et al., 2011; Kim et al., 2014; Knorr et al., 2021). Elevated AChE levels may also result from reduced degradation, as has been demonstrated in amyloid beta-stimulated murine neuroblastoma cells (Hu et al., 2003). While presence of AChE per se does not initiate apoptosis, elevated levels sensitize the induction of apoptosis under pathogenic or physiologically challenging conditions (Jin et al., 2004; Knorr et al., 2022). Some studies indicated that only particular splice variants of mammalian AChE induce apoptosis in the investigated cell types, such as N-AChE-S in cholinergic cortex neurons and glioblastoma cells (Toiber et al., 2008). In contrast, absence or catalytic inactivation of AChE have been correlated with reduced sensitivity to apoptogenic stimuli and reduced apoptotic cell death in various cell types including PC12, HEK293, HeLa, neuroblastoma, substantia nigra dopaminergic neurons, primary cortical neurons, renal cells, and insect neurons (Carlson et al., 1998; Zhang et al., 2002; Jin et al., 2004; Park et al., 2004; Toiber et al., 2008; Ye et al., 2010; Zhang et al., 2013; Zhang et al., 2013; Yao et al., 2016; Knorr et al., 2020). AChE expression and activity is dysregulated in various tumors while the direction (elevated or reduced) depends on tumor type (Ruiz-Espejo et al., 2002; Syed et al., 2008; Lu et al., 2013) and low levels of AChE activity in lung cancer cells has been associated with reduced sensitivity against the chemotherapeutic cisplatin (Lu et al., 2013).
Direct and indirect involvement of AChE in apoptosis
AChE may promote apoptosis by various direct or indirect mechanisms involving 1) apoptosome formation, 2) degradation of nuclear DNA, 3) ACh-hydrolization leading to reduced cytomembrane-located ACh-receptor activation, 4) modulating the activation of mitochondria-located nicotinic receptors and 5) non-catalytic mechanisms through association with other molecules in trophic cell-to-cell contacts. We will summarize the current knowledge on the role of AChE in some particular apoptotic mechanisms.
AChE promotes apoptosome formation
The mammalian apoptosome is a cytosolic multi-protein complex of up to ∼1.4 MDa that assembles upon release of cytochrome c from mitochondrial intermembrane space and mediates activation of the initiator caspase-9 (Zou et al., 1999; Yuan et al., 2013; Chai and Shi, 2014). Its major scaffolding factor is Apaf-1 (apoptotic protease-activating factor-1), an adaptor protein harboring three distinct domains for molecular interactions with other apoptosomal proteins. In non-apoptotic cells, Apaf-1 assumes an autoinhibitory conformation that prevents interaction with procaspase-9. Following the release of cytochrome c during initial phases of mitochondria-dependent apoptosis Apaf-1/cytochrome C complexes are established and each seven of those assemble per apoptosome (cofactor dATP/ATP is required). This enables the recruitment of procaspase-9 zymogen and its subsequent transformation into activated caspase-9 (Chai and Shi, 2014). Various additional factors that either promote or inhibit the formation of the apoptosome have been identified (Hu et al., 1998; Cain et al., 2002; Chandra et al., 2006; Bao and Shi, 2007; Bratton and Salvesen, 2010). One factor that seems to be essential for this process, at least in some cell types, is AChE. Downregulation of AChE has been demonstrated to prevent the interaction of Apaf-1 with cytochrome C (and subsequent Apaf-1 oligomerization and caspase-9 activation) in various cell types subjected to different apoptogenic stimuli (Park et al., 2004; Park et al., 2008). Detailed analysis of various etoposide-treated cell types revealed direct interaction of AChE with cytochrome C as an absolute requirement for subsequent association of Apaf-1 and other apoptosomal proteins (Park et al., 2008). Co-immunoprecipitation studies on a human colorectal adenocarcinoma cell line (HT-29) and other cell lines revealed that AChE first associates with caveolin-1 before it can bind cytochrome C (Park et al., 2008). It was demonstrated that this initial complex subsequently associates with Apaf-1, though without identification of the direct interacting molecular component. Caveolin-1 is a structural membrane protein that forms small invaginations (“caveolae”) of the cytoplasmic membrane that concentrate a large variety of signaling molecules and regulate multiple cellular processes including vesicular transport, cell cycle progression, cell migration, survival and apoptosis (Williams and Lisanti, 2004; Boscher and Nabi, 2012). Both pro-apoptotic and pro-survival functions of caveolin-1 have been described, hence its role in apoptosis seems to depend on cell type and cellular context. Similar to AChE, caveolin-1 can sensitize cells to apoptogenic stimuli (Liu et al., 2001; Torres et al., 2007) and dysregulated functions have been detected in certain tumor types (Wang et al., 2008; Zhao et al., 2009) (reviewed by Quest et al., 2013). Whether AChE (with or without caveolin-1) is a general requirement for apoptosome formation in mitochondria-dependent apoptosis of mammalian cell types remains to be demonstrated by future studies. Moreover, all mammalian AChE possess a N-terminal secretory signal peptide causing translation into the endoplasmatic reticulum and targeting to secretory pathways. Interaction with cytochrome C requires the presence of AChE in the cytoplasm but mechanisms that target functional AChE to the cytoplasm have so far not been identified (Campoy et al., 2016; refer to Hegde and Bernstein, 2006 for a general discussion of potential mechanisms).
AChE degrades nuclear DNA
Degradation of nuclear DNA is an important process in apoptosis. Various types of DNases sequentially cleave chromatin first into large segments, then into internucleosomal fragments and finally into small nucleotides (reviewed by Aleksandrushkina and Vanyushin, 2012). Decomposition of DNA into oligonucleotides and nucleotides may occur after engulfment of apoptotic bodies by phagocytes. Among various types of DNases, especially caspase-activated DNase (CAD) and endonuclease G (EndoG) have been implicated in apoptosis-related DNA degradation. Ubiquitously expressed CAD is released from inhibition by caspase-3-mediated cleavage of its inhibitor ICAD (inhibitor of CAD). CAD cleaves the DNA in spacer regions between nucleosomes to generate multimers of ∼180 bp that can be detected as “DNA ladder” by gel electrophoresis (Enari et al., 1998; Samejima and Earnshaw, 2005). Under apoptogenic conditions EndoG is released from mitochondrial intermembrane space into the cytoplasm and subsequently translocates to the nucleus. Activation of EndoG-mediated DNase function is independent of caspases (Irvine et al., 2005; David et al., 2006). Both, CAD and EndoG produce DNA cleavage ends that can be detected by TUNEL labeling. However, apoptosis is typically not dependent on a particular type of DNase since experimental deactivation of single DNases does not prevent DNA destruction suggesting that sequential and parallel contribution of various types of DNases has evolved to assure efficient degradation of DNA. In line with this, DNases recruited for apoptosis seem to depend on cell type, developmental status and the nature and intensity of the apoptogenic stimulus (Aleksandrushkina and Vanyushin, 2012). Several studies reported nuclear localization and DNA-hydrolyzing activity of AChE. Apoptogenic stimulus-induced nuclear accumulation of AChE has been reported in various cell lines including HeLA, PC12, COS1, HUVEC, MMEC-1 and normal rat kidney cells (Perry et al., 2002; Jin et al., 2004; Xie et al., 2012; Du et al., 2015), in human neuroblastoma cells (Yang et al., 2002) and in mouse dopaminergic and hippocampal neurons (Zhang et al., 2013; Zhang et al., 2013; Du et al., 2015). These studies provided some evidence that AChE may first accumulate in the cytoplasm and translocates to the nucleus during apoptosis execution, where it can be detected throughout the middle and late phases of apoptosis (Yang et al., 2002; Jin et al., 2004; Xie et al., 2011). Since AChE is a designated export protein and normally translated into the lumen of the rough endoplasmic reticulum, mechanisms leading to its nuclear entry (directly from ER into nucleus or via transient appearance in the cytoplasm or other pathway) are not clear (Campoy et al., 2016). Targeted translocation of larger proteins (>40 kDa) to the nucleus is typically associated with nuclear localization signals that are recognized by transport proteins to initiate active transport through pore complexes in the nuclear envelope (reviewed by Cautain et al., 2015). Apoptosis includes the nucleocytoplasmic exchange of signals and execution factors. Apoptotic mechanisms may alter the molecular transport across nuclear membranes by masking/demasking of nuclear localization signals on cargo molecules, modulating the activity of transport molecules (importins and exportins) or alteration of the nuclear pore complexes (increased pore diameter and caspase-mediated modifications) (reviewed by Ferrando-May 2005). Studies on hydrogen peroxide-exposed PC12 cells and ischemia-reperfusion-treated mouse kidneys revealed the caspase-mediated production of 55 kDa cleavage product from 68 kDa full length AChE-S (Xie et al., 2011). Only after activation of caspases and generation of the cleavage product, both the 55 kDa and the original 68 kDa AChE-S molecules translocated from the cytoplasm into the nucleus. Thus, in addition to the appearance of the N-terminally truncated version of AChE-S other apoptosis-initiated processes enabled the nuclear entry of full-length AChE. Santos and coworkers reported the presence of a 55 kDa AChE isoform that, together with a 70 kDa AChE was already present in nuclei of intact non-apoptotic cells of various types (Santos et al., 2007). Additional studies should clarify whether the 55 kDA AChE isoforms were identical and why the conditions for their nuclear localization differed in both studies (Santos et al., 2007; Xie et al., 2011). At least 67 C-terminal amino acids of AChE (fused to GFP) are required for translocation to nuclei of apoptotic COS 1 and PC12 cells (Perry et al., 2002). Du and coworkers demonstrated that heterologously generated purified mouse (aa 32–579) and human (aa 32–578) AChE polypeptides associate and cleave naked plasmid and genomic DNA as well as chromosomal DNA in fixed cellular nuclei and apoptotic HeLa cells (Du et al., 2015). DNA cleavage by AChE was independent of its catalytic center for ACh hydrolysis and persisted in a truncated polypeptide consisting of aa 32–138 of human AChE-S. This suggested that AChE harbors two independent domains with esterase and DNase functions. Whether the reported DNase activity is a general characteristic of AChE and whether/under which conditions nuclear AChE contributes significantly to apoptotic DNA degradation (in addition to dedicated nucleases) has to be determined by future studies.
AChE reduces the activation of cell membrane ACh receptors
AChE hydrolyzes extracellular ACh and limits the activation of cytoplasmic membrane-located cholinergic receptors (Figure 1). These receptors include various subtypes of ionotropic and other nicotinic ACh-receptors and metabotropic muscarinic ACh-receptors that activate G proteins and intracellular signaling cascades. The vast majority of mammalian cells, many cell types in other species and various cancer cells express muscarinic and/or nicotinic ACh-receptors and receive ACh signals of various origins implicated in the regulation of multiple cellular mechanisms (Spindel, 2012; Wessler and Kirkpatrick, 2020). ACh stimulation presumably affects all intracellular signaling pathways (Wessler et al., 2003) and membrane-located ACh receptors have been shown to initiate trophic mechanisms by activating intracellular pathways that lead to the expression of pro-survival genes. Hence survival-promoting activation of cell membrane located ACh-receptors is reduced by AChE-mediated hydrolysis of extracellular ACh. Involvement of cell membrane nicotinic ACh-receptors: Many studies apply nicotine for selective activation of nicotinic ACh-receptors. Since nicotine is membrane permeable the observed effects may result from activation of receptors in cell surface membranes and/or receptors in mitochondrial membranes (see below). Distinction between effects mediated by these differently located receptors requires additional controls. Anti-apoptotic effects mediated by cell membrane located nicotinic ACh-receptors have been described in mouse spinal cord and brain neurons (Carlson et al., 1998; Garrido et al., 2001), rat brain neurons (Kaneko et al., 1997; Kihara et al., 1998) and different tumor cell lines (Wright et al., 1993). Cell-protective mechanisms are often initiated by α7 subunit-containing nicotinic receptors that display high Ca2+ permeability and may involve PI3K/Akt signaling (Liu and Zhao, 2004; Yu et al., 2011) eventually stimulating the expression of anti-apoptotic Bcl-2 (Kihara et al., 2001). In addition to ionotropic effects, nicotinic ACh–receptors can directly stimulate intracellular kinases and protein tyrosine phosphatases (Swope and Huganir, 1994; Kihara et al., 2001) or physically associate with receptors for growth factors (epidermal growth factor EGF, vascular endothelial growth factor VEGF, Chernyavsky et al., 2015) to modulate their effects on cellular physiology. Involvement of cell membrane muscarinic ACh-receptors: Muscarinic ACh-receptors signal through different types of G proteins and especially M1-, M3-and M5-receptors that activate Gq proteins have been implicated with anti-apoptotic effects. An intracellularly located C-terminal region of these receptors appears to be responsible for anti-apoptotic effects, that occur independently of typical cell-protective pathways involving PI3K, MAPK, PKB/Akt or phospholipase C (Budd et al., 2003; 2004). Muscarinic agonists inhibit apoptosis (initiated different stimuli) in tumor cell lines (Lindenboim et al., 1995; Leloup et al., 2000; De Sarno et al., 2003; Budd et al., 2004), transfected hamster ovary cells (Budd et al., 2004) and neurons (Koh et al., 1991; Yan et al., 1995). Anti-apoptotic mechanisms can rely on regulation of transcription and/or stabilization of pro-survival Bcl-2 protein (Budd et al., 2004). Other mechanisms induced by extracellular AChE accumulation: Experimentally increased levels of extracellular AChE induced death neuronal and glia-like cells that was associated with the induction of TUNEL-positive nuclear labeling (Carlson et al., 1998). Promotion of cell death persisted in the presence of inhibitors of AChE’s esterase activity. Though blocking of AChE’s cytotoxic effect by low temperature suggested enzyme-driven processes, the mechanism how extracellular AChE activates apoptosis (endocytosis? Intracellular signaling pathway?) remains to be identified.
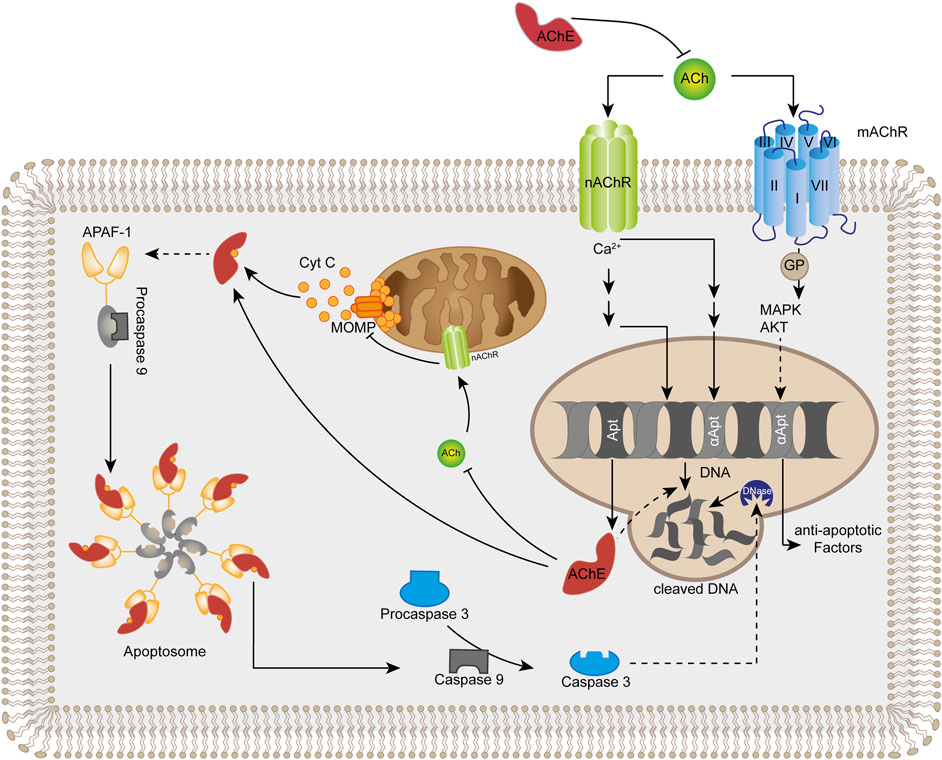
FIGURE 1. Summary of acetylcholinesterase (AChE) -mediated direct and indirect mechanisms that promote apoptosis. Apoptogenic conditions typically induce elevated levels of AChE. Extracellular AChE limits the activation of membrane-located nicotinic (nAChR) and muscarinic (mAChR) ACh-receptors that initiate pro-survival intracellular processes. Intracellular accumulation of AChE limits the activation of mitochondrial nicotinic ACh-receptors leading to opening of mitochondrial outer membrane permeability channels complexes (MOMP) and release of cytochrome C (Cyt C). Cytochrome C associates directly with cytosolic AChE leading to formation of the apoptosome that activates the initiator caspase 9 from its inactive proenzyme. Caspase 9 converts procaspase 3 into active executor caspase 3 which degrades macromolecules and activates nuclear DNases. Additionally, AChE may accumulate in the nucleus to directly degrade DNA. ACh acetylcholine, APAF-1 apoptotic protease-activating factor-1, GP G protein, MAPK mitogen-activated protein kinase, AKT protein kinase B.
AChE limits ACh-binding to mitochondrial nicotinic ACh receptors
Mitochondrial outer membranes contain various molecular subtypes of nicotinic ACh-receptors in a tissue-specific manner (Lykhmus et al., 2014; Skok and Lykhmus, 2016; Skok, 2022). Nicotinic receptors have been evolved long before the appearance of neurons as multifunctional receptors that mediate physiological responses to various kinds of signals (Hampl et al., 2008). Various studies indicated that elevated presence of mitochondrial nicotinic ACh-receptors mediates resistance against apoptogenic signals and increases cell viability of normal and malignant cells under unfavorable physiological conditions (Grando, 2014; Uspenska et al., 2018). Moreover, different types of nicotinic ACh-receptors promoted survival during exposure to different types of proapoptotic stress factors (Lykhmus et al., 2014). In contrast to their function as ligand-gated cation channels in excitable cells, mitochondrial nicotinic ACh-receptors (like some types of plasma membrane located types) mediate their effects through ion-independent direct interaction with other proteins including intramitochondrial kinases and components of the mitochondrial permeability transition pore (MPTP) (Chernyavsky et al., 2009; 2010; Gergalova et al., 2012; Skok and Lykhmus, 2016). Especially nicotinic receptors that contain the subunits α7 and β2 have been implicated with regulating the release of mitochondria-derived pro-apoptotic molecules (Lykhmus et al., 2014). In addition to ACh, which can be generated by cytosolic and mitochondrial cholineacetyltransferase (Wessler et al., 2012), nicotine and choline can activate mitochondrial receptors (Gergalova et al., 2012). Since permanent binding to mitochondrial nicotinic receptors is required to prevent release of cytochrome C and reactive oxygen species through mitochondrial outer membrane pores (Gergalova et al., 2012), cytosol-localized AChE activity that limits the availability of the respective ligands can promote the release of these proapototic factors from mitochondria (Baldessarini and Karobath, 1973). Hence, elevated levels of cytosolic AChE promote the release of cytochrome c from mitochondrial intermembrane space which subsequently enables apoptosome formation and caspase activation leading to apoptosis.
AChE as structural component
AChE associates with various intracellular molecules involved in apoptotic pathways such as cytochrome c and caveolin-1 (Park et al., 2008; see above). AChE also binds extracellularly exposed proteins such as laminin in order to modulate their receptor-binding properties (Layer et al., 2005). Various cell adhesion molecules (including neuroligins, gliotactin, glutactin) contain catalytically inactive AChE-homologous domains that bind to other molecules (Grisaru et al., 1999; Karczmar, 2010). Interaction with their designated binding partners (e.g., neurexins that associate with AChE-like domains of neuroligins) on other cells initiates intracellular events in both cells that regulate neurite outgrowth, cell-to-cell contacts and synaptogenesis (Grisaru et al., 1999). Elevated levels of AChE induced by apoptogenic stimuli may interfere with regular protein-protein interactions by saturating binding sites for AChE-like domains, weakening cell adhesion and altering cellular physiology.
AChE in invertebrate apoptosis
Current knowledge suggests that complex regulation of apoptosis including mitochondrial cytochrome c release and apoptosome-mediated activation of caspases evolved in early metazoans (Zmasek and Godzik, 2013; Chai and Shi, 2014; Green and Fitzgerald, 2016). Elevated levels of AChE expression induced by apoptogenic stressors and apoptosis inhibition by experimental reduction of AChE presence and/or catalytical activity have also been detected in insects (Knorr et al., 2020; Knorr et al., 2022) paralleling previous reports from studies with vertebrate cells. The molecular mechanisms underlying AChE’s pro-apoptotic functions have not been revealed in any invertebrate species. Pro-apoptotic elevation of AChE expression in locust and beetle neurons is suppressed by activation of CRLF3 (cytokine receptor-like factor 3) stimulation with various cytokine ligands (Knorr et al., 2022). CRLF3 represents a phylogenetically (from cnidarian to mammals) conserved cytokine receptor that initiates anti-apoptotic processes via activation of intracellular JAK/STAT signaling (Hahn et al. 2017; Hahn et al. 2019). Since this anti-apoptotic pathway is stimulated by various cell-protective cytokine receptors, the prevention of AChE accumulation under apoptogenic conditions, might represent a general cytoprotective mechanism in insect, invertebrate and vertebrate cells. CRLF3 belongs to group 1 of the prototypic class I cytokine receptors that also includes the vertebrate-specific erythropoietin receptor EpoR (Boulay et al., 2003; Lionge and Ward, 2007). A connection between EpoR activation and elevated expression of AChE-E via GATA1 transcription factors promoting survival and maturation was reported from mammalian erythrocyte progenitor cells (Xu et al., 2018).
Summary
Increased expression and/or activity of AChE sensitizes cells for apoptogenic stimuli and promotes various apoptotic cellular processes. AChE can either directly contribute to apoptotic events by promoting apoptosome formation or degrading nuclear DNA or indirectly by limiting the availability of ACh as a ligand for ACh-receptors on cytomembranes and mitochondrial membranes. However, the pathways from sensing the apoptogenic stimulus to AChE accumulation and targeting to particular cellular compartments as well as the mode of AChE action have only been incompletely identified and likely vary between cell types, their physiological state and the type of apoptogenic insult. While elevated presence and activity of AChE seems to contribute to cell loss in degenerative diseases (including Alzheimer’s and Parkinson’s Disease) reduced AChE may impair apoptosis in cancer cells and therefore interfere with chemotherapy. Increasing knowledge about the induction and functions of AChE in apoptosis may identify points of intervention to prevent the disease-related dysregulations of AChE.
Author contributions
DK, DD and RH wrote manuscript.
Funding
DK and RH were supported by project grants from the Deutsche Forschungsgemeinschaft (DFG; project numbers 398214842 and 499371712). DD is a student at Bahçeşehir University (Istanbul, Turkey) who contributed to this manuscript during a research stay in the Department of Cellular Neurobiology (Göttingen, Germany) in 2022.
Acknowledgments
The authors are thankful for the support of the Open Access Publication Funds of the Georg-August-University Goettingen.
Conflict of interest
The authors declare that the research was conducted in the absence of any commercial or financial relationships that could be construed as a potential conflict of interest.
Publisher’s note
All claims expressed in this article are solely those of the authors and do not necessarily represent those of their affiliated organizations, or those of the publisher, the editors and the reviewers. Any product that may be evaluated in this article, or claim that may be made by its manufacturer, is not guaranteed or endorsed by the publisher.
References
Acehan, D., Jiang, X., Morgan, D. G., Heuser, J. E., Wang, X., and Akey, C. W. (2002). Three-dimensional structure of the apoptosome: Implications for assembly, procaspase-9 binding, and activation. Mol. Cell. 9, 423–432. doi:10.1016/S1097-2765(02)00442-2
Aleksandrushkina, N. I., and Vanyushin, B. F. (2012). Endonucleases and apoptosis in animals. Biochem. Mosc. 77, 1436–1451. doi:10.1134/S0006297912130032
Baldessarini, R. J., and Karobath, M. (1973). Biochemical Physiology of central synapses. Ann. Rev. Physiol. 35, 273–304. doi:10.1146/annurev.ph.35.030173.001421
Bao, O., and Shi, Y. (2007). Apoptosome: A platform for the activation of initiator caspases. Cell. Death Differ. 14, 56–65. doi:10.1038/sj.cdd.4402028
Bell, R. A. V., and Megeney, L. A. (2017). Evolution of caspase-mediated cell death and differentiation: Twins separated at birth. Cell. Death Differ. 24, 1359–1368. doi:10.1038/cdd.2017.37
Boscher, C., and Nabi, I. R. (2012). Caveolin-1: Role in cell signaling. Adv. Exp. Med. Biol. 729, 29–50. doi:10.1007/978-1-4614-1222-9_3
Boulay, J. L., O’Shea, J. J., and Paul, W. E. (2003). Molecular phylogeny within type I cytokines and their cognate receptors. Immunity 19, 159–163. doi:10.1016/s1074-7613(03)00211-5
Bratton, S. B., and Salvesen, G. S. (2010). Regulation of the apaf-1-caspase-9-apoptosome. J. Cell. Sci. 123, 3209–3214. doi:10.1242/jcs.073643
Budd, D. C., McDonald, J., Emsley, N., Cain, K., and Tobin, A. B. (2003). The C-terminal tail of the M3-muscarinic receptor possesses anti-apoptotic properties. J. Biol. Chem. 278 (21), 19565–19573. doi:10.1074/jbc.M211670200
Budd, D. C., Spragg, E. J., Ridd, K., and Tobin, A. B. (2004). Signalling of the M3-muscarinic receptor to the anti-apoptotic pathway. Biochem. J. 381, 43–49. doi:10.1042/BJ20031705
Cain, K., Bratton, S. B., and Cohen, G. M. (2002). The apaf-1 apoptosome: A large caspase-activating complex. Biochimie 84, 203–214. doi:10.1016/s0300-9084(02)01376-7
Campoy, F. J., Vidal, C. J., Muñoz-Delgado, E., Montenegro, M. F., Cabezas-Herrera, J., and Nieto-Cerón, S. (2016). Cholinergic system and cell proliferation. Chem. Biol. Interact. 259, 257–265. doi:10.1016/j.cbi.2016.04.014
Cao, X., and Tabuchi, K. (2017). Functions of synapse adhesion molecules neurexin/neuroligins and neurodevelopmental disorders. Neurosci. Res. 116, 3–9. doi:10.1016/j.neures.2016.09.005
Carlson, N. G., Bacchi, A., Rogers, S. W., and Gahring, L. C. (1998). Nicotine blocks TNF-alpha-mediated neuroprotection to NMDA by an alpha-bungarotoxin-sensitive pathway. J. Neurobiol. 35, 29–36. doi:10.1002/(sici)1097-4695(199804)35:1<29:aid-neu3>3.0.co;2-d
Cautain, B., Hill, R., de Pedro, N., and Link, W. (2015). Components and regulation of nuclear transport processes. FEBS J. 282 (3), 445–462. doi:10.1111/febs.13163
Chai, J., and Shi, Y. (2014). Apoptosome and inflammasome: Conserved machineries for caspase activation. Natl. Sci. Rev. 1, 101–118. doi:10.1093/nsr/nwt025
Chandra, D., Bratton, S. B., Person, M. D., Tian, Y., Martin, A. G., Ayres, M., et al. (2006). Intracellular nucleotides act as critical prosurvival factors by binding to cytochrome C and inhibiting apoptosome. Cell. 125 (7), 1333–1346. doi:10.1016/j.cell.2006.05.026
Chernyavsky, A. I., Arredondo, J., Galitovskiy, V., Qian, J., and Grando, S. A. (2010). Upregulation of nuclear factor-kappaB expression by SLURP-1 is mediated by alpha7-nicotinic acetylcholine receptor and involves both ionic events and activation of protein kinases. Am. J. Physiol. Cell. Physiol. 299 (5), C903–C911. doi:10.1152/ajpcell.00216.2010
Chernyavsky, A. I., Arredondo, J., Qian, J., Galitovskiy, V., and Grando, S. A. (2009). Coupling of ionic events to protein kinase signaling cascades upon activation of alpha7 nicotinic receptor: Cooperative regulation of alpha2-integrin expression and rho kinase activity. J. Biol. Chem. 284 (33), 22140–22148. doi:10.1074/jbc.M109.011395
Chernyavsky, A. I., Shchepotin, I. B., and Grando, S. A. (2015). Mechanisms of growth-promoting and tumor-protecting effects of epithelial nicotinic acetylcholine receptors. Int. Immunopharmacol. 29 (1), 36–44. doi:10.1016/j.intimp.2015.05.033
David, K. K., Sasaki, M., Yu, S. W., Dawson, T. M., and Dawson, V. L. (2006). EndoG is dispensable in embryogenesis and apoptosis. Cell. Death Differ. 13 (7), 1147–1155. doi:10.1038/sj.cdd.4401787
De Sarno, P., Shestopal, S. A., King, T. D., Zmijewska, A., Song, L., and Jope, R. S. (2003). Muscarinic receptor activation protects cells from apoptotic effects of DNA damage, oxidative stress, and mitochondrial inhibition. J. Biol. Chem. 278 (13), 11086–11093. doi:10.1074/jbc.M212157200
Degterev, A., and Yuan, J. (2008). Expansion and evolution of cell death programmes. Nat. Rev. Mol. Cell. Biol. 9, 378–390. doi:10.1038/nrm2393
Du, A., Xie, J., Guo, K., Yang, L., Wan, Y., OuYang, Q., et al. (2015). A novel role for synaptic acetylcholinesterase as an apoptotic deoxyribonuclease. Cell. Discov. 1, 15002. doi:10.1038/celldisc.2015.2
Earnshaw, W. C., Martins, L. M., and Kaufmann, S. H. (1999). Mammalian caspases: Structure, activation, substrates, and functions during apoptosis. Annu. Rev. Biochem. 68, 383–424. doi:10.1146/annurev.biochem.68.1.383
Enari, M., Sakahira, H., Yokoyama, H., Okawa, K., Iwamatsu, A., and Nagata, S. (1998). A caspase-activated DNase that degrades DNA during apoptosis, and its inhibitor ICAD. Nature 391 (6662), 43–50. doi:10.1038/34112
Ferrando-May, E. (2005). Nucleocytoplasmic transport in apoptosis. Cell. Death Differ. 12 (10), 1263–1276. doi:10.1038/sj.cdd.4401626
Garrido, R., Mattson, M. P., Hennig, B., and Toborek, M. (2001). Nicotine protects against arachidonic-acid-induced caspase activation, cytochrome c release and apoptosis of cultured spinal cord neurons. J. Neurochem. 76 (5), 1395–1403. doi:10.1046/j.1471-4159.2001.00135.x
Gergalova, G., Lykhmus, O., Kalashnyk, O., Koval, L., Chernyshov, V., Kryukova, E., et al. (2012). Mitochondria express α7 nicotinic acetylcholine receptors to regulate Ca2+ accumulation and cytochrome c release: Study on isolated mitochondria. PLoS One 7 (2), e31361. doi:10.1371/journal.pone.0031361
Grando, S. A. (2014). Connections of nicotine to cancer. Nat. Rev. Cancer 14 (6), 419–429. doi:10.1038/nrc3725
Green, D. R., and Fitzgerald, P. (2016). Just so stories about the evolution of apoptosis. Curr. Biol. 26, R620–R627. doi:10.1016/j.cub.2016.05.023
Grisaru, D., Sternfeld, M., Eldor, A., Glick, D., and Soreq, H. (1999). Structural roles of acetylcholinesterase variants in biology and pathology. Eur. J. Biochem. 264, 672–686. doi:10.1046/j.1432-1327.1999.00693.x
Hahn, N., Büschgens, L., Schwedhelm-Domeyer, N., Bank, S., Geurten, B. R. H., Neugebauer, P., et al. (2019). The orphan cytokine receptor CRLF3 emerged with the origin of the nervous system and is a neuroprotective erythropoietin receptor in locusts. Front. Mol. Neurosci. 12, 251. doi:10.3389/fnmol.2019.00251
Hahn, N., Knorr, D. Y., Liebig, J., Wüstefeld, L., Peters, K., Büscher, M., et al. (2017). The insect ortholog of the human orphan cytokine receptor CRLF3 is a neuroprotective erythropoietin receptor. Front. Mol. Neurosci. 10, 22–311. doi:10.3389/fnmol.2017.00223
Hampl, V., Silberman, J. D., Stechmann, A., Diaz-Triviño, S., Johnson, P. J., and Roger, A. J. (2008). Genetic evidence for a mitochondriate ancestry in the 'amitochondriate' flagellate Trimastix pyriformis. PLoS One 3 (1), e1383. doi:10.1371/journal.pone.0001383
Hegde, R. S., and Bernstein, H. D. (2006). The surprising complexity of signal sequences. Trends Biochem. Sci. 31, 563–571. doi:10.1016/j.tibs.2006.08.004
Hicks, D., John, D., Makova, N. Z., Henderson, Z., Nalivaeva, N. N., and Turner, A. J. (2011). Membrane targeting, shedding and protein interactions of brain acetylcholinesterase. J. Neurochem. 116, 742–746. doi:10.1111/j.1471-4159.2010.07032.x
Hu, W., Gray, N. W., and Brimijoin, S. (2003). Amyloid-beta increases acetylcholinesterase expression in neuroblastoma cells by reducing enzyme degradation. J. Neurochem. 86, 470–478. doi:10.1046/j.1471-4159.2003.01855.x
Hu, Y., Ding, L., Spencer, D. M., and Nunez, G. (1998). WD-40 repeat region regulates Apaf-1 self-association and procaspase-9 activation. J. Biol. Chem. 273 (50), 33489–33494. doi:10.1074/jbc.273.50.33489
Irvine, R. A., Adachi, N., Shibata, D. K., Cassell, G. D., Yu, K., Karanjawala, Z. E., et al. (2005). Generation and characterization of endonuclease G null mice. Mol. Cell. Biol. 25 (1), 294–302. doi:10.1128/MCB.25.1.294-302.2005
Jin, Q. H., He, H. Y., Shi, Y. F., Lu, H., and Zhang, X. J. (2004). Overexpression of acetylcholinesterase inhibited cell proliferation and promoted apoptosis in NRK cells. Acta Pharmacol. Sin. 25, 1013–1021.
Kaneko, S., Maeda, T., Kume, T., Kochiyama, H., Akaike, A., Shimohama, S., et al. (1997). Nicotine protects cultured cortical neurons against glutamate-induced cytotoxicity via alpha7-neuronal receptors and neuronal CNS receptors. Brain Res. 765 (1), 135–140. doi:10.1016/s0006-8993(97)00556-8
Kang, J. S., Lee, D.-W., Koh, Y. H., and Lee, S. H. (2011). A soluble acetylcholinesterase provides chemical defense against xenobiotics in the pinewood nematode. PLoS ONE 6 (4), e19063. doi:10.1371/journal.pone.0019063
Karczmar, A. G. (2010). Cholinesterases (ChEs) and the cholinergic system in ontogenesis and phylogenesis, and non-classical roles of cholinesterases-A review. Chem. Biol. Interact. 187, 34–43. doi:10.1016/j.cbi.2010.03.009
Kihara, T., Shimohama, S., Sawada, H., Honda, K., Nakamizo, T., Shibasaki, H., et al. (2001). Alpha 7 nicotinic receptor transduces signals to phosphatidylinositol 3-kinase to block A beta-amyloid-induced neurotoxicity. J. Biol. Chem. 276 (17), 13541–13546. doi:10.1074/jbc.M008035200
Kihara, T., Shimohama, S., Urushitani, M., Sawada, H., Kimura, J., Kume, T., et al. (1998). Stimulation of alpha4beta2 nicotinic acetylcholine receptors inhibits beta-amyloid toxicity. Brain Res. 792 (2), 331–334. doi:10.1016/s0006-8993(98)00138-3
Kim, Y. H., Kwon, D. H., Ahn, H. M., Koh, Y. H., and Lee, S. H. (2014). Induction of soluble AChE expression via alternative splicing by chemical stress in Drosophila melanogaster. Insect Biochem. Mol. Biol. 48, 75–82. doi:10.1016/j.ibmb.2014.03.001
Knorr, D. Y., Georges, N. S., Pauls, S., and Heinrich, R. (2020). Acetylcholinesterase promotes apoptosis in insect neurons. Apoptosis 25, 730–746. doi:10.1007/s10495-020-01630-4
Knorr, D. Y., Hartung, D., Schneider, K., Hintz, L., Pies, H. S., and Heinrich, R. (2021). Locust hemolymph conveys erythropoietin-like cytoprotection via activation of the cytokine receptor CRLF3. Front. Physiol. 12, 648245. doi:10.3389/fphys.2021.648245
Knorr, D. Y., Schneider, K., Büschgens, L., Förster, J., Georges, N. S., Geurten, B. R. H., et al. (2022). Protection of insect neurons by erythropoietin/CRLF3-mediated regulation of pro-apoptotic acetylcholinesterase. Sci. Rep. 12 (1), 18565. doi:10.1038/s41598-022-22035-0
Koh, J. Y., Palmer, E., and Cotman, C. W. (1991). Activation of the metabotropic glutamate receptor attenuates N-methyl-D-aspartate neurotoxicity in cortical cultures. Proc. Natl. Acad. Sci. U. S. A. 88 (21), 9431–9435. doi:10.1073/pnas.88.21.9431
Koonin, E. V., and Aravind, L. (2002). Origin and evolution of eukaryotic apoptosis: The bacterial connection. Cell. Death Differ. 9, 394–404. doi:10.1038/sj.cdd.4400991
Kumar, S. (2007). Caspase function in programmed cell death. Cell. Death Differ. 14, 32–43. doi:10.1038/sj.cdd.4402060
Layer, P. G., Allebrandt, K., Andermann, P., Bodur, E., Boopathy, R., Bytyqi, A. H., et al. (2005). On the multifunctionality of cholinesterases. Chem. Biol. Interact. 157-158, 37–41. doi:10.1016/j.cbi.2005.10.006
Leloup, C., Michaelson, D. M., Fisher, A., Hartmann, T., Beyreuther, K., and Stein, R. (2000). M1 muscarinic receptors block caspase activation by phosphoinositide 3-kinase- and MAPK/ERK-independent pathways. Cell. Death Differ. 7 (9), 825–833. doi:10.1038/sj.cdd.4400713
Lindenboim, L., Pinkas-Kramarski, R., Sokolovsky, M., and Stein, R. (1995). Activation of muscarinic receptors inhibits apoptosis in PC12M1 cells. J. Neurochem. 64 (6), 2491–2499. doi:10.1046/j.1471-4159.1995.64062491.x
Lionge, C., and Ward, A. C. (2007). Evolution of class I cytokine receptors. BMC Evol. Biol. 7, 120. doi:10.1186/1471-2148-7-120
Liu, J., Lee, P., Galbiati, F., Kitsis, R. N., and Lisanti, M. P. (2001). Caveolin-1 expression sensitizes fibroblastic and epithelial cells to apoptotic stimulation. Am. J. Physiol. Cell. Physiol. 280 (4), C823–C835. doi:10.1152/ajpcell.2001.280.4.C823
Liu, Q., and Zhao, B. (2004). Nicotine attenuates beta-amyloid peptide-induced neurotoxicity, free radical and calcium accumulation in hippocampal neuronal cultures. Br. J. Pharmacol. 141 (4), 746–754. doi:10.1038/sj.bjp.0705653
Lu, L., Zhang, X., Zhang, B., Wu, J., and Zhang, X. (2013). Synaptic acetylcholinesterase targeted by microRNA-212 functions as a tumor suppressor in non-small cell lung cancer. Int. J. Biochem. Cell. Biol. 45, 2530–2540. doi:10.1016/j.biocel.2013.08.007
Lykhmus, O., Gergalova, G., Koval, L., Zhmak, M., Komisarenko, S., and Skok, M. (2014). Mitochondria express several nicotinic acetylcholine receptor subtypes to control various pathways of apoptosis induction. Int. J. Biochem. Cell. Biol. 53, 246–252. doi:10.1016/j.biocel.2014.05.030
McLuskey, K., and Mottram, J. C. (2015). Comparative structural analysis of the caspase family with other clan CD cysteine peptidases. Biochem. J. 466, 219–232. doi:10.1042/BJ20141324
Meshorer, E., Toiber, D., Zurel, D., Sahly, I., Dori, A., Cagnano, E., et al. (2004). Combinatorial complexity of 5′ alternative acetylcholinesterase transcripts and protein products. J. Biol. Chem. 279, 29740–29751. doi:10.1074/jbc.M402752200
Park, S. E., Jeong, S. H., Yee, S., Kim, H., Soung, Y. H., Ha, N. C., et al. (2008). Interactions of acetylcholinesterase with caveolin-1 and subsequently with cytochrome c are required for apoptosome formation. Carcinogenesis 29, 729–737. doi:10.1093/carcin/bgn036
Park, S. E., Kim, N. D., and Yoo, Y. H. (2004). Acetylcholinesterase plays a pivotal role in apoptosome formation. Cancer Res. 64, 2652–2655. doi:10.1158/0008-5472.can-04-0649
Park, Y. N., and Arvan, P. (2004). The acetylcholinesterase homology region is essential for normal conformational maturation and secretion of thyroglobulin. J. Biol. Chem. 279, 17085–17089. doi:10.1074/jbc.M314042200
Perry, C., Sklan, E. H., Birikh, K., Shapira, M., Trejo, L., Eldor, A., et al. (2002). Complex regulation of acetylcholinesterase gene expression in human brain tumors. Oncogene 21, 8428–8441. doi:10.1038/sj.onc.1205945
Quest, A. F., Lobos-González, L., Nuñez, S., Sanhueza, C., Fernández, J. G., Aguirre, A., et al. (2013). The caveolin-1 connection to cell death and survival. Curr. Mol. Med. 13 (2), 266–281. doi:10.2174/156652413804810745
Renehan, A. G., Booth, C., and Potten, C. S. (2001). What is apoptosis and why is it important? BMJ 322, 1536–1538. doi:10.1136/bmj.322.7301.1536
Rotundo, R. L. (2017). Biogenesis, assembly and trafficking of acetylcholinesterase. J. Neurochem. 142, 52–58. doi:10.1111/jnc.13982
Ruiz-Espejo, F., Cabezas-Herrera, J., Illana, J., Campoy, F. J., and Vidal, C. J. (2002). Cholinesterase activity and acetylcholinesterase glycosylation are altered in human breast cancer. Breast cancer Res. Treat. 72, 11–22. doi:10.1023/a:1014904701723
Saelens, X., Festjens, N., Vande Walle, L., Van Gurp, M., Van Loo, G., and Vandenabeele, P. (2004). Toxic proteins released from mitochondria in cell death. Oncogene 23, 2861–2874. doi:10.1038/sj.onc.1207523
Samejima, K., and Earnshaw, W. (2005). Trashing the genome: The role of nucleases during apoptosis. Nat. Rev. Mol. Cell. Biol. 6 (9), 677–688. doi:10.1038/nrm1715
Santos, S. C. R., Vala, I., Miguel, C., Barata, J. T., Garção, P., Agostinho, P., et al. (2007). Expression and subcellular localization of a novel nuclear acetylcholinesterase protein. J. Biol.Chem. 282, 25597–25603. doi:10.1074/jbc.M700569200
Skok, M. (2022). Mitochondrial nicotinic acetylcholine receptors: Mechanisms of functioning and biological significance. Int. J. Biochem. Cell. Biol. 143, 106138. doi:10.1016/j.biocel.2021.106138
Skok, M., and Lykhmus, O. (2016). The role of α7 nicotinic acetylcholine receptors and α7-specific antibodies in neuroinflammation related to alzheimer disease. Curr. Pharm. Des. 22 (14), 2035–2049. doi:10.2174/1381612822666160127112914
Small, D. H., Michaelson, S., and Sberna, G. (1996). non-classical actions of cholinesterases: Role in cellular differentiation, tumorigenesis and Alzheimer’s Disease. Neurochem. Int. 28, 453–483. doi:10.1016/0197-0186(95)00099-2
Spindel, E. R. (2012). Muscarinic receptor agonists and antagonists: Effects on cancer. Handb. Exp. Pharmacol. 208, 451–468. doi:10.1007/978-3-642-23274-9_19
Swillens, S., Ludgate, M., Mercken, L., Dumont, J. E., and Vassart, G. (1986). Analysis of sequence and structure homologies between thyroglobulin and acetylcholinesterase: Possible functional and clinical significance. Biochem. Biophys. Res. Commun. 137, 142–148. doi:10.1016/0006-291x(86)91187-3
Swope, S. L., and Huganir, R. L. (1994). Binding of the nicotinic acetylcholine receptor to SH2 domains of Fyn and Fyk protein tyrosine kinases. J. Biol. Chem. 269 (47), 29817–29824. doi:10.1016/s0021-9258(18)43954-3
Syed, M., Fenoglio-Preiser, C., Skau, K. A., and Weber, G. F. (2008). Acetylcholinesterase supports anchorage independence in colon cancer. Clin. Exp. Metastasis 25, 787–798. doi:10.1007/s10585-008-9192-0
Toiber, D., Berson, A., Greenberg, D., Melamed-Book, N., Diamant, S., and Soreq, H. (2008). N-acetylcholinesterase-induced apoptosis in alzheimer’s disease. PLoS One 3, e3108. doi:10.1371/journal.pone.0003108
Torres, V. A., Tapia, J. C., Rodriguez, D. A., Lladser, A., Arredondo, C., Leyton, L., et al. (2007). E-cadherin is required for caveolin-1-mediated down-regulation of the inhibitor of apoptosis protein survivin via reduced beta-catenin-Tcf/Lef-dependent transcription. Mol. Cell. Biol. 27 (21), 7703–7717. doi:10.1128/MCB.01991-06
Uspenska, K., Lykhmus, O., Obolenskaya, M., Pons, S., Maskos, U., Komisarenko, S., et al. (2018). Mitochondrial nicotinic acetylcholine receptors support liver cells viability after partial hepatectomy. Front. Pharmacol. 9, 626. doi:10.3389/fphar.2018.00626
Wang, Y., Yu, J., and Zhan, Q. (2008). BRCA1 regulates caveolin-1 expression and inhibits cell invasiveness. Biochem. Biophys. Res. Commun. 370 (2), 201–206. doi:10.1016/j.bbrc.2008.03.031
Wessler, I., Kilbinger, H., Bittinger, F., Unger, R., and Kirkpatrick, C. J. (2003). The non-neuronal cholinergic system in humans: Expression, function and pathophysiology. Life Sci. 72 (18-19), 2055–2061. doi:10.1016/s0024-3205(03)00083-3
Wessler, I., and Kirkpatrick, C. J. (2020). Cholinergic signaling controls immune functions and promotes homeostasis. Int. Immunopharmacol. 83, 106345. doi:10.1016/j.intimp.2020.106345
Wessler, I., Michel-Schmidt, R., Brochhausen, C., and Kirkpatrick, C. J. (2012). Subcellular distribution of choline acetyltransferase by immunogold electron microscopy in non-neuronal cells: Placenta, airways and murine embryonic stem cells. Life Sci. 91 (21-22), 977–980. doi:10.1016/j.lfs.2012.05.012
Williams, T. M., and Lisanti, M. P. (2004). The caveolin proteins. Genome Biol. 5 (3), 214. doi:10.1186/gb-2004-5-3-214
Wright, S. C., Zhong, J., Zheng, H., and Larrick, J. W. (1993). Nicotine inhibition of apoptosis suggests a role in tumor promotion. FASEB J. 7 (11), 1045–1051. doi:10.1096/fasebj.7.11.8370474
Xie, J., Jiang, H., Wan, Y. H., Du, A. Y., Guo, K. J., Liu, T., et al. (2011). Induction of a 55 kDa acetylcholinesterase protein during apoptosis and its negative regulation by the Akt pathway. J. Mol. Cell. Biol. 3, 250–259. doi:10.1093/jmcb/mjq047
Xie, J., Xiang, D. B., Wang, H., Zhao, C., Chen, J., Xiong, F., et al. (2012). Inhibition of Tcf-4 induces apoptosis and enhances chemosensitivity of colon cancer cells. PLoS One 7 (9), e45617. doi:10.1371/journal.pone.0045617
Xu, M. L., Luk, W. K. W., Bi, C. W. C., Liu, E. Y. L., Wu, K. Q. Y., Yao, P., et al. (2018). Erythropoietin regulates the expression of dimeric form of acetylcholinesterase during differentiation of erythroblast. J. Neurochem. 146, 390–402. doi:10.1111/jnc.14448
Yan, G. M., Lin, S. Z., Irwin, R. P., and Paul, S. M. (1995). Activation of muscarinic cholinergic receptors blocks apoptosis of cultured cerebellar granule neurons. Mol. Pharmacol. 47 (2), 248–257.
Yang, L., He, H. Y., and Zhang, X. J. (2002). Increased expression of intranuclear AChE involved in apoptosis of SK-N-SH cells. Neurosci. Res. 42, 261–268. doi:10.1016/S0168-0102(02)00005-6
Yao, D., Wang, J., Wang, G., Jiang, Y., Shang, L., Zhao, Y., et al. (2016). Design, synthesis and biological evaluation of coumarin derivatives as novel acetylcholinesterase inhibitors that attenuate H2O2-induced apoptosis in SH-SY5Y cells. Bioorg Chem. 68, 112–123. doi:10.1016/j.bioorg.2016.07.013
Ye, W., Gong, X., Xie, J., Wu, J., Zhang, X., Ouyang, Q., et al. (2010). AChE deficiency or inhibition decreases apoptosis and p53 expression and protects renal function after ischemia/reperfusion. Apoptosis 15, 474–487. doi:10.1007/s10495-009-0438-3
Yu, W., Mechawar, N., Krantic, S., and Quirion, R. (2011). α7 Nicotinic receptor activation reduces β-amyloid-induced apoptosis by inhibiting caspase-independent death through phosphatidylinositol 3-kinase signaling. J. Neurochem. 119 (4), 848–858. doi:10.1111/j.1471-4159.2011.07466.x
Yuan, S., and Akey, C. W. (2013). Apoptosome structure, assembly, and procaspase activation. Structure 21, 501–515. doi:10.1016/j.str.2013.02.024
Yuan, Y., Jiang, C. Y., Xu, H., Sun, Y., Hu, F. F., Bian, J. C., et al. (2013). Cadmium-induced apoptosis in primary rat cerebral cortical neurons culture is mediated by a calcium signaling pathway. PLoS One 8 (5), e64330. doi:10.1371/journal.pone.0064330
Zhang, J., Li, D., Ge, P., Yang, M., Guo, Y., Zhu, K. Y., et al. (2013b). RNA interference revealed the roles of two carboxylesterase genes in insecticide detoxification in Locusta migratoria. Chemosphere 93, 1207–1215. doi:10.1016/j.chemosphere.2013.06.081
Zhang, X., Lu, L., Liu, S., Ye, W., Wu, J., and Zhang, X. (2013a). Acetylcholinesterase deficiency decreases apoptosis in dopaminergic neurons in the neurotoxin model of Parkinson’s disease. Int J Biochem. Cell. Biol 45, 265–272. doi:10.1016/j.biocel.2012.11.015
Zhang, X-J., and Greenberg, D. S. (2012). Acetylcholinesterase involvement in apoptosis. Front. Mol. Neurosci. 5, 40. doi:10.3389/fnmol.2012.00040
Zhang, X. J., Yang, L., Zhao, Q., Caen, J. P., He, H. Y., Jin, Q. H., et al. (2002). Induction of acetylcholinesterase expression during apoptosis in various cell types. Cell. Death Differ. 9, 790–800. doi:10.1038/sj.cdd.4401034
Zhao, X., Liu, Y., Ma, Q., Wang, X., Jin, H., Mehrpour, M., et al. (2009). Caveolin-1 negatively regulates TRAIL-induced apoptosis in human hepatocarcinoma cells. Biophys. Res. Commun. 378 (1), 21–26. doi:10.1016/j.bbrc.2008.10.123
Zmasek, C. M., and Godzik, A. (2013). Evolution of the animal apoptosis network. Cold Spring Harb. Perspect. Biol. 5, a008649. doi:10.1101/cshperspect.a008649
Keywords: acetylcholinesterase, pro-apoptotic, apoptosome, DNase, acetylcholine receptor, nicotinic, muscarinic, mitochondria
Citation: Knorr DY, Demirbas D and Heinrich R (2023) Multifaceted promotion of apoptosis by acetylcholinesterase. Front. Cell. Death 2:1169966. doi: 10.3389/fceld.2023.1169966
Received: 20 February 2023; Accepted: 04 April 2023;
Published: 17 April 2023.
Edited by:
Olivier Micheau, Université de Bourgogne, FranceReviewed by:
Victor J. Yuste, Autonomous University of Barcelona, SpainPatrick Legembre, University of Limoges, France
Copyright © 2023 Knorr, Demirbas and Heinrich. This is an open-access article distributed under the terms of the Creative Commons Attribution License (CC BY). The use, distribution or reproduction in other forums is permitted, provided the original author(s) and the copyright owner(s) are credited and that the original publication in this journal is cited, in accordance with accepted academic practice. No use, distribution or reproduction is permitted which does not comply with these terms.
*Correspondence: Ralf Heinrich, cmhlaW5yaTFAZ3dkZy5kZQ==