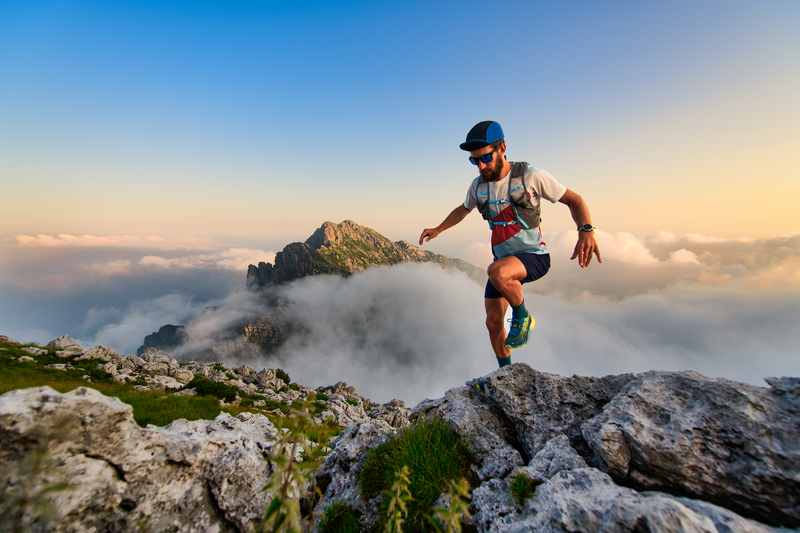
94% of researchers rate our articles as excellent or good
Learn more about the work of our research integrity team to safeguard the quality of each article we publish.
Find out more
MINI REVIEW article
Front. Cell Death , 27 February 2023
Sec. Apoptosis
Volume 2 - 2023 | https://doi.org/10.3389/fceld.2023.1147605
This article is part of the Research Topic Reviews in Cell Death Research View all 6 articles
Recent studies have furthered our understanding of how dying and living cells interact in different physiological contexts, however the signaling that initiates and mediates apoptosis and apoptosis-induced proliferation are more complex than previously thought. One increasingly important area of study is the biophysical control of apoptosis. In addition to biochemical regulation, biophysical signals (including redox chemistry, bioelectric gradients, acoustic and magnetic stimuli) are also known yet understudied regulators of both cell death and apoptosis-induced proliferation. Mounting evidence suggests biophysical signals may be key targets for therapeutic interventions. This review highlights what is known about the role of biophysical signals in controlling cell death mechanisms during development, regeneration, and carcinogenesis. Since biophysical signals can be controlled spatiotemporally, bypassing the need for genetic manipulation, further investigation may lead to fine-tuned modulation of apoptotic pathways to direct desired therapeutic outcomes.
A fundamental property of cells is the ability to physiologically respond to environmental stimuli, where outcomes are cell type and state dependent. Thus, a central biological concept is understanding intracellular responses to different environmental cues. While progress has been made in uncovering the traditional cellular signaling (i.e., protein, lipid, hormone, carbohydrate, RNA, and other small molecule signals) that controls cell fate decisions, it is underappreciated that biophysical forces such as mechanical, magnetic, acoustic, redox, and bioelectrical forces shape biology at the individual cell and whole tissue level [reviewed in (Costa et al., 2012; Sun et al., 2022)]. Biophysical signals are often discussed in terms of effects on stem cells in development and regeneration, yet mounting evidence indicates these forces also regulate cell death decisions (Morbidelli et al., 2005; Shuryak et al., 2007; Nanayakkara et al., 2019). Since dying cells actively participate in physiological processes including tissue morphogenesis, stem cell proliferation, and immune responses (Gregory and Pound, 2011; Elliott and Ravichandran, 2016; Medina et al., 2020; Guerin et al., 2021; Riley and Bock, 2022), the use of biophysical signaling to modulate cell death may be a complementary therapeutic approach for a variety of diseases.
Apoptosis is one of the most well-studied forms of regulated cell death; cell destruction involves DNA fragmentation, cell shrinkage, and membrane blebbing. Apoptosis proceeds through well-defined signaling mechanisms (both intrinsic and extrinsic), where caspase proteins are the main executioners of the death responses. Generally, death stimuli result in activation of initiator caspases (Caspases -2, -8, -9, and -10), where exposure of the catalytic domain allows for activation of executioner pro-caspases -3, -6, or -7 [reviewed in-depth in (Galluzzi et al., 2018; Green, 2022)]. Downstream signaling leads to the formation of apoptotic bodies and extracellular vesicles [reviewed in (Kakarla et al., 2020)], which are quickly engulfed by macrophages or taken up by surrounding cells (Budai et al., 2019).
There are many different types of stimuli that initiate and regulate apoptotic pathways, and we are only beginning to uncover all the mechanisms involved. Perhaps the most well studied are the biochemical regulators such as the BCL-2 family, which includes both anti-apoptotic and proapoptotic genes (Warren et al., 2019). Increasingly, biophysical regulators have been shown to play an equally important role in controlling apoptosis and apoptosis-induced proliferation (AiP). This dance between regulated cell death and proliferation is delicate; misregulation of either process at any developmental stage can result in debilitating diseases including neurodegenerative disorders (Ghavami et al., 2014), autoimmune conditions (Lleo et al., 2008), and carcinogenesis (Mohamed et al., 2017). Thus, modulation of apoptosis by broad spectrum manipulation has been proposed as a treatment strategy for these ailments [reviewed in (Liu et al., 2019; Carneiro and El-Deiry, 2020)]. To effectively use apoptotic modulation as a means to treat disease, the field will benefit in acquiring a better understanding of both biophysical and biochemical cell death initiation mechanisms and how these two interact. Genetic modulation of apoptosis has been well characterized, but our understanding of biophysical modulation of cell death is limited. In this review, we focus on key biophysical controls of apoptosis such as: redox, quantum (sub-atomic). Ionizing radiation, and bioelectric, and highlight how signaling thresholds guide physiological outcomes.
Reactive oxygen species (ROS), including the hydroxyl radical (•OH), superoxide anion (O2−), and hydrogen peroxide (H2O2), are essential regulators of many cell processes. Intracellular and extracellular ROS are generated following cellular stress and during homeostasis, where mitochondria, membrane-associated NADPH oxidases, and dual oxidases are major sources (Geiszt et al., 2003; Bedard and Krause, 2007; Murphy, 2009). While low-intermediate ROS levels (<100 nM intracellularly) are required for proliferation signaling (Tan and Suda, 2018), higher ROS levels (>100 nM intracellularly) often trigger cell death (Sies and Jones, 2020). ROS can initiate apoptosis directly through DNA damage or regulation of caspase cysteine catalytic sites (Katoh et al., 2004; Barbouti et al., 2007). Cell death occurs indirectly when ROS act as intracellular messengers (Circu and Aw, 2010) and through oxidation of regulatory pro-apoptotic proteins (Redza-Dutordoir and Averill-Bates, 2016). For example, ROS-activated p53 can downregulate pro-survival genes (like BCL-2 and IAPs) or facilitate apoptosis through transcriptional activation of Apaf-1 and death ligands (Yoshida and Miki, 2010).
A dependency for ROS and ROS-mediated signaling has also arisen as a common theme in models of AiP in regeneration and disease [reviewed in (Diwanji and Bergmann, 2018)]. For example, ROS accumulate at the injury site following zebrafish fin amputation (Gauron et al., 2013) and after Xenopus tadpole tail amputation (Love et al., 2013) where a wave of apoptosis follows (Tseng et al., 2007). In these models, proliferation of neighboring stem cells is likely initiated by ROS-induced apoptosis. This phenomenon is most well characterized in Drosophila, where ROS, JNK signaling, and apoptosis are required for eye and wing imaginal disc regeneration (Fan et al., 2014; Fogarty et al., 2016). Similarly, ROS generated in dying cells stimulates production of signaling molecules. Apoptotic hepatocytes in the regenerating mammalian liver generate ROS, which facilitates the expression of interleukin-11 (IL-11). JAK/STAT activation by IL-11 is then followed by liver regrowth (Nishina et al., 2012).
ROS-dependent AiP appears to be conserved across several models, highlighting potential therapeutic benefits of modulating relative ROS concentrations, particularly in regenerative medicine. Although high ROS levels are associated with cancer cell death in successful treatment [reviewed in (Moloney and Cotter, 2018; Wang et al., 2021)], ROS-dependent AiP may also drive carcinogenesis. For example, hepatocytes treated with the carcinogen diethylnitrosamine undergo cell death following ROS accumulation, where a JNK signaling cascade results in proliferation and survival of remaining hepatocytes. This ROS-dependent AiP promotes formation of hepatocellular carcinoma (Maeda et al., 2005). The clear mechanistic links between ROS, cell death, proliferation, and carcinogenesis underscore the need to further investigate the role of oxidative stress-induced cell death in both growth inducing and prevention scenarios, and to determine how differing ROS levels effect cellular outcomes in different physiological contexts.
A cell’s resting potential, or membrane voltage, is governed by the endogenous flow of ions through membrane channels and gap junctions. Changes in membrane electrical potential, driven by fluctuations in ion flow, can dynamically regulate cell activities such as migration, proliferation, differentiation, and cell death. Bioelectric signaling has been shown to alter gene expression during embryogenesis, regeneration, homeostasis, and disease [reviewed in (Levin, 2021)], making electrochemical control of cell fate a prime area for therapeutic development. For a comprehensive review of bioelectric signaling mechanisms that regulate cell fate (including cell death) in various contexts, we direct readers to (Mclaughlin and Levin, 2018; George and Bates, 2022). Cell death is tightly linked to ion flux, where cell shrinkage observed during apoptosis results from a net efflux of intracellular K+ and Cl− and an influx of Na+ (Bortner and Cidlowski, 1998; Arrebola et al., 2005). Changes in ion flux (in particular, Ca2+) are activators of apoptotic programs [reviewed in (Kondratskyi et al., 2015)]. Increased cytosolic Ca2+ concentrations can promote apoptosis through ER-stress (mitochondria-independent) (Nakagawa et al., 2000; Morishima et al., 2002), changes in mitochondrial outer-membrane permeability (Rapizzi et al., 2002; De Stefani et al., 2012), Fas-induced apoptosis mediated by transient receptor potential (TRP) channels (Xu et al., 2013), and through inhibition of voltage-gated calcium channels that activates pro-apoptotic p53 and p38-MAPK signaling (Dziegielewska et al., 2014). Thus, it is clear that a cell’s decision to die is sensitive to changes in intracellular ion concentrations, where bioelectric signaling must be tightly regulated to elicit desired cellular outcomes.
Studies in models of development and regeneration highlight that bioelectric signaling can act cooperatively with both apoptosis and proliferation to promote new growth. For example, apoptosis and proliferation during Xenopus CNS development is controlled by bioelectric signaling (Pai et al., 2015). Perturbation of voltage-gated potassium channels by injection of Kv1.5 mRNA in developing embryos resulted in increased apoptotic levels and reduced proliferation in the neural tube, while interrupting signaling in the tadpole brain resulted in the opposite effect of reduced apoptosis and increased proliferation (Pai et al., 2015). Both bioelectrical signaling and apoptosis have been shown to be required for regenerative growth, for example during Xenopus eye and limb regeneration (Tseng et al., 2007; Kha et al., 2018; Kha et al., 2023). During planarian head regeneration, the H+K+-ATPase has been shown to be required for regenerative apoptosis to occur (Beane et al., 2011; Beane et al., 2013). During Xenopus limb regeneration, bioelectric signaling has been implicated in inducing apoptosis in uninjured control limbs following amputation of contralateral limbs (Busse et al., 2018), supporting a role for modulation of membrane voltage and apoptosis in regulating the regenerative response in a whole organism.
The current data indicate that bioelectrical signaling is upstream of apoptosis, but this relationship is likely context-dependent and may not be conserved in all regenerative contexts. For instance, while both apoptosis and chloride channel activation occur during axolotl regeneration, pharmacological inhibition of chloride channels did not affect cell death levels as measured by TUNEL (Franklin et al., 2017). Regardless, this is still an important area of focus considering the body of literature linking misregulation of intracellular ion concentrations and induction of apoptosis, as well as genome-wide analyses revealing that upregulation of cell death genes is a conserved response to depolarization in embryogenesis, spinal cord regeneration, and human stem cells (Pai et al., 2016). Based on literature findings, it is possible that, like other biophysical mechanisms, bioelectric signaling may dictate cellular outcomes in a threshold dependent manner. Given that bioelectric signals can potentiate injury information to distant tissues, are critical regulators of apoptosis, and are required for regeneration in most models, investigations into the effects of bioelectricity on cell death and apoptotic machinery in governing new growth may be a ripe new area of study.
Ionizing radiation (IR), (including particle radiation, ultraviolet light, X-rays, and gamma rays) are known to damage biological macromolecules like DNA, RNA, lipids, and proteins, where cellular insults often result in the induction of apoptosis through both intrinsic and extrinsic mechanisms, and ER stress pathways (Maier et al., 2016; Sia et al., 2020). While cell death due to IR exposure is generally viewed as a negative consequence, low doses of IR are used as a successful treatment to induce cancer cell apoptosis. Radiotherapy with IR leads to shrinkage of primary tumors in a number of cases (Surucu et al., 2016), yet disease re-emergence following treatment is a widespread issue (Jiang et al., 2020). Somewhat counterintuitively, high levels of tumor apoptosis are associated with poor prognoses and patient outcomes for a variety of cancers (Ichim and Tait, 2016), highlighting apoptotic signaling as a potential mechanism for tumor re-emergence after radiotherapy.
Two possible explanations for this seeming paradox are potential AiP of remaining tumor cells or sublethal activation of apoptotic machinery (mainly caspases) that leads to cancer cell survival and genomic instability [reviewed in (Eskandari and Eaves, 2022)]. In support of the former, many studies have implicated a role for AiP in driving tumor repopulation following either radiotherapy or chemotherapy (Huang et al., 2011; Donato et al., 2014; Cheng et al., 2019). For instance, radiation treatment in a murine xenograft breast cancer model led to production of the cancer cell mitogen prostaglandin E2 (PGE2) (Huang et al., 2011). Stimulation of this “phoenix-rising” pathway was later associated with wound healing and inducing mammalian liver regeneration (Li et al., 2010). In support of the latter explanation, apoptotic machinery activation (such as caspase activation) can mediate tumor cell survival and contribute to increased genomic instability in remaining cells. For example, caspase-3 knockout in lung cancer cells treated with X-rays in vitro had lower DNA damage responses, which reduced the growth capacity of dying tumor cells (Zhao et al., 2020). Similarly, non-lethal activation of caspase-3 caused persistent DNA damage following irradiation, where damaged cells acquired oncogenic properties (Liu et al., 2015). These examples demonstrate that IR-induced apoptosis can contribute to tumor repopulation, supporting the need to investigate the effects of both dying and undead cells on the surrounding tumor microenvironment to improve therapeutic outcomes. Studies on the effects of IR dosage may also shed light on potential tumor repopulation mechanisms governed by dying cells.
The use of weak static magnetic fields (MFs), radiofrequency MFs, and electromagnetic fields have a long therapeutic history, where recently they have been proposed as treatments for arthritic pain (Ross et al., 2019) and potential modulators of cellular inflammatory responses that contribute to disease (Ross and Harrison, 2013; Kubat et al., 2015). However, the use of MFs is also gaining traction as a non-invasive method to control cellular activities (proliferation, differentiation, and apoptosis) and to modulate tissue growth (Zadeh-Haghighi and Simon, 2022). Weak MFs (<1 mT) have been shown to alter apoptosis and cell activities, such as in several tumor cell lines (lung cancer, gastric cancer, pancreatic cancer, and nephroblastoma) where exposure to MFs resulted in increased levels of ROS, DNA damage, and apoptosis, with reductions in cell proliferation (Yuan et al., 2020). Similarly, exposure to 400 µT weak MFs increased ROS levels in mouse embryonic stem cells (Bekhite et al., 2010). While changes in ROS levels due to MF exposure appear to be a driver of apoptosis, MFs can also induce apoptosis through non-redox mechanisms [reviewed in (Xu et al., 2021)], mainly by modulating pathways like p53 and MAPK.
MF effects are context dependent, which is not surprising as all cell signaling is context dependent. For example, hypomagnetic fields (<5 µT) increased ROS levels in fibrosarcoma cells, but reduced cell survival of mouse skeletal muscle (Martino and Castello, 2011; Fu et al., 2016). It is important to recognize that different MF strengths can have different (and even opposite) effects on ROS levels, even in the same context; 200 µT inhibits while 500 µT increases superoxide levels and regenerative proliferation during planarian regeneration (Kinsey et al., 2023). These effects are consistent with the theoretical model for how MFs exert control at the quantum level to produce cellular changes in intracellular and extracellular ROS generation [mechanisms reviewed in (Zadeh-Haghighi and Simon, 2022)].
These data demonstrate that many diverse cell types are responsive to MFs in physiologically meaningful ways. Thus, sub-atomic control of apoptosis and apoptosis-induced proliferation through quantum effects has been proposed as an exciting new avenue of therapeutic research (Hack et al., 2021). Likely the strongest benefit of this approach is the potential to tune applications of MFs to use some field strengths to remove unwanted cells and different field strengths to increase proliferation in regenerative or growth promoting settings.
How biophysical signals modulate apoptotic responses is a fertile research area that deserves more attention. Filling in this knowledge gap will not only help to fine-tune current therapeutic strategies but has the potential to lead to novel approaches. Manipulating biophysical control of apoptosis in vivo may confer select advantages over genetic manipulation, in part due to the possibilities for non-invasive approaches. For example, high intensity focused ultrasound has been used to thermally ablate soft tissue tumors in the clinic for decades [reviewed in (Zhou, 2011)]. While largely successful, these treatments can lead to fibrosis (Peek et al., 2015), therefore understanding how dying tumor cells communicate with neighboring tissue following thermal ablation may help to improve patient outcomes. Vibroacoustic stimuli offer other promising non-invasive methods to modulate cell death and proliferation, such as the use of infrasound to inhibit neurogenesis in the rat dentate gyrus (Liu et al., 2010) or induce apoptosis of cardiomyocytes (Pei et al., 2011). Additionally, while biophysical therapies have been studied widely in the context of cancer and regeneration, the known roles of apoptosis in neurodegenerative disorders and autoimmune conditions [reviewed in (Singh et al., 2019)] provide abundant areas for further investigation. Given that redox and bioelectric signaling are known modulators of neurogenesis and neurodegeneration, investigation into biophysical modulation of cell death mediated effects in the nervous system would be an interesting research avenue.
One essential question in need of answering is how biophysical signals are translated into apoptotic responses. One potential mediator could be non-coding RNAs (ncRNAs). Biophysical signals can modulate expression of ncRNAs (Aryankalayil et al., 2018; Zuo et al., 2022), which have emerged as modulators of apoptosis in cancer (Lima et al., 2011; Zhou et al., 2018; Zhang et al., 2020). These raise the possibility that biophysical signaling could be used to control expression of ncRNAs in dying cells. If true, ncRNA could represent a general mediator of biophysical signals, linking biophysical signaling with canonical cell signaling pathways.
An interesting aspect to the biophysical control of apoptosis and downstream cellular activities is that the different biophysical mechanisms are often integrated with each other, leading to a stronger response. This is supported by evidence that biophysical mechanisms work via a threshold model. For example, bioelectrical signals have been shown to regulate morphogen gradients, such as during Xenopus regeneration (Tseng et al., 2010). Furthermore, ROS signaling is a classic threshold example, with lower levels required for homeostasis and high levels driving cell death (Sies and Jones, 2020). MFs can increase or decrease ROS levels through quantum effects, such as during regeneration where exposure to variable weak MF strengths has been shown to increase or decrease ROS-mediated tissue growth (Van Huizen, 2019).
The use of MFs to control cell activities has exciting clinical implications. For instance, non-invasive application of MFs in combination with radiotherapy was able to reduce ROS levels and enhance tumor cell specific apoptosis in vivo (Iwamoto et al., 2021). Similarly, ex vivo application of high-frequency electric fields to modulate ion flow has the potential to induce cancer cell death with reduced off-target effects (Fukushiro-Lopes et al., 2018; Berkelmann et al., 2019), as malignant cell types are often enriched in ion channels and have different membrane voltages than surrounding tissues (Ko et al., 2013; Williams et al., 2013). Lastly, we note apoptosis is a complex process with points of regulation at a variety of stages (onset of death signaling, cell destruction, phagocytosis of cell debris, etc.) and that biophysical stimuli have the potential to mediate apoptosis in different ways at each of the different stages. While most studies focus on signaling upstream of cell destruction, we posit that a comprehensive characterization of the biophysical mechanisms governing cell death at all stages will be fundamental to establishing and refining therapeutic strategies utilizing manipulation of apoptosis.
SH, WB, and KA-ST contributed to the conception and revision of the manuscript. SH wrote the first draft. All authors read and approved the submitted version.
Funding was provided by grants to KA-ST from NASA (80NSSC20M0043) and the NIH (1R16GM146672) and to WB from NSF (2105474).
We regret any omissions due to word limitations. We thank the members of the Beane and Tseng labs for helpful discussions and comments.
The authors declare that the research was conducted in the absence of any commercial or financial relationships that could be construed as a potential conflict of interest.
All claims expressed in this article are solely those of the authors and do not necessarily represent those of their affiliated organizations, or those of the publisher, the editors and the reviewers. Any product that may be evaluated in this article, or claim that may be made by its manufacturer, is not guaranteed or endorsed by the publisher.
Arrebola, F., Zabiti, S., Cañizares, F. J., Cubero, M. A., Crespo, P. V., and Fernández-Segura, E. (2005). Changes in intracellular sodium, chlorine, and potassium concentrations in staurosporine-induced apoptosis. J. Cell. Physiol. 204, 500–507. doi:10.1002/jcp.20306
Aryankalayil, M. J., Chopra, S., Levin, J., Eke, I., Makinde, A., Das, S., et al. (2018). Radiation-induced long noncoding RNAs in a mouse model after whole-body irradiation. Radiat. Res. 189, 251–263. doi:10.1667/RR14891.1
Barbouti, A., Amorgianiotis, C., Kolettas, E., Kanavaros, P., and Galaris, D. (2007). Hydrogen peroxide inhibits caspase-dependent apoptosis by inactivating procaspase-9 in an iron-dependent manner. Free Radic. Biol. Med. 43, 1377–1387. doi:10.1016/j.freeradbiomed.2007.06.020
Beane, W. S., Morokuma, J., Adams, D. S., and Levin, M. (2011). A chemical genetics approach reveals H,K-ATPase-mediated membrane voltage is required for planarian head regeneration. Chem. Biol. 18, 77–89. doi:10.1016/j.chembiol.2010.11.012
Beane, W. S., Morokuma, J., Lemire, J. M., and Levin, M. (2013). Bioelectric signaling regulates head and organ size during planarian regeneration. Development 140, 313–322. doi:10.1242/dev.086900
Bedard, K., and Krause, K. H. (2007). The NOX family of ROS-generating NADPH oxidases: Physiology and pathophysiology. Physiol. Rev. 87, 245–313. doi:10.1152/physrev.00044.2005
Bekhite, M. M., Finkensieper, A., Abou-Zaid, F. A., El-Shourbagy, I. K., Omar, K. M., Figulla, H. R., et al. (2010). Static electromagnetic fields induce vasculogenesis and chondro-osteogenesis of mouse embryonic stem cells by reactive oxygen species-mediated up-regulation of vascular endothelial growth factor. Stem Cells Dev. 19, 731–743. doi:10.1089/scd.2008.0266
Berkelmann, L., Bader, A., Meshksar, S., Dierks, A., Hatipoglu Majernik, G., Krauss, J. K., et al. (2019). Tumour-treating fields (TTFields): Investigations on the mechanism of action by electromagnetic exposure of cells in telophase/cytokinesis. Sci. Rep. 9, 7362. doi:10.1038/s41598-019-43621-9
Bortner, C. D., and Cidlowski, J. A. (1998). A necessary role for cell shrinkage in apoptosis. Biochem. Pharmacol. 56, 1549–1559. doi:10.1016/s0006-2952(98)00225-1
Budai, Z., Ujlaky-Nagy, L., Kis, G. N., Antal, M., Banko, C., Bacso, Z., et al. (2019). Macrophages engulf apoptotic and primary necrotic thymocytes through similar phosphatidylserine-dependent mechanisms. FEBS Open Bio 9, 446–456. doi:10.1002/2211-5463.12584
Busse, S. M., Mcmillen, P. T., and Levin, M. (2018). Cross-limb communication during Xenopus hindlimb regenerative response: Non-local bioelectric injury signals. Development 145 (19), dev164210. doi:10.1242/dev.164210
Carneiro, B. A., and El-Deiry, W. S. (2020). Targeting apoptosis in cancer therapy. Nat. Rev. Clin. Oncol. 17, 395–417. doi:10.1038/s41571-020-0341-y
Cheng, J., He, S., Wang, M., Zhou, L., Zhang, Z., Feng, X., et al. (2019). The caspase-3/pkcδ/akt/VEGF-A signaling pathway mediates tumor repopulation during radiotherapy. Clin. Cancer Res. 25, 3732–3743. doi:10.1158/1078-0432.CCR-18-3001
Circu, M. L., and Aw, T. Y. (2010). Reactive oxygen species, cellular redox systems, and apoptosis. Free Radic. Biol. Med. 48, 749–762. doi:10.1016/j.freeradbiomed.2009.12.022
Costa, P., Almeida, F. V., and Connelly, J. T. (2012). Biophysical signals controlling cell fate decisions: How do stem cells really feel? Int. J. Biochem. Cell. Biol. 44, 2233–2237. doi:10.1016/j.biocel.2012.09.003
De Stefani, D., Bononi, A., Romagnoli, A., Messina, A., De Pinto, V., Pinton, P., et al. (2012). VDAC1 selectively transfers apoptotic Ca2+ signals to mitochondria. Cell. Death Differ. 19, 267–273. doi:10.1038/cdd.2011.92
Diwanji, N., and Bergmann, A. (2018). An unexpected friend - ROS in apoptosis-induced compensatory proliferation: Implications for regeneration and cancer. Semin. Cell. Dev. Biol. 80, 74–82. doi:10.1016/j.semcdb.2017.07.004
Donato, A. L., Huang, Q., Liu, X., Li, F., Zimmerman, M. A., and Li, C. Y. (2014). Caspase 3 promotes surviving melanoma tumor cell growth after cytotoxic therapy. J. Invest. Dermatol 134, 1686–1692. doi:10.1038/jid.2014.18
Dziegielewska, B., Brautigan, D. L., Larner, J. M., and Dziegielewski, J. (2014). T-type Ca2+ channel inhibition induces p53-dependent cell growth arrest and apoptosis through activation of p38-MAPK in colon cancer cells. Mol. Cancer Res. 12, 348–358. doi:10.1158/1541-7786.MCR-13-0485
Elliott, M. R., and Ravichandran, K. S. (2016). The dynamics of apoptotic cell clearance. Dev. Cell. 38, 147–160. doi:10.1016/j.devcel.2016.06.029
Eskandari, E., and Eaves, C. J. (2022). Paradoxical roles of caspase-3 in regulating cell survival, proliferation, and tumorigenesis. J. Cell. Biol. 221, e202201159. doi:10.1083/jcb.202201159
Fan, Y., Wang, S., Hernandez, J., Yenigun, V. B., Hertlein, G., Fogarty, C. E., et al. (2014). Genetic models of apoptosis-induced proliferation decipher activation of JNK and identify a requirement of EGFR signaling for tissue regenerative responses in Drosophila. PLoS Genet. 10, e1004131. doi:10.1371/journal.pgen.1004131
Fogarty, C. E., Diwanji, N., Lindblad, J. L., Tare, M., Amcheslavsky, A., Makhijani, K., et al. (2016). Extracellular reactive oxygen species drive apoptosis-induced proliferation via Drosophila macrophages. Curr. Biol. 26, 575–584. doi:10.1016/j.cub.2015.12.064
Franklin, B. M., Voss, S. R., and Osborn, J. L. (2017). Ion channel signaling influences cellular proliferation and phagocyte activity during axolotl tail regeneration. Mech. Dev. 146, 42–54. doi:10.1016/j.mod.2017.06.001
Fu, J. P., Mo, W. C., Liu, Y., and He, R. Q. (2016). Decline of cell viability and mitochondrial activity in mouse skeletal muscle cell in a hypomagnetic field. Bioelectromagnetics 37, 212–222. doi:10.1002/bem.21968
Fukushiro-Lopes, D. F., Hegel, A. D., Rao, V., Wyatt, D., Baker, A., Breuer, E. K., et al. (2018). Preclinical study of a Kv11.1 potassium channel activator as antineoplastic approach for breast cancer. Oncotarget 9, 3321–3337. doi:10.18632/oncotarget.22925
Galluzzi, L., Vitale, I., Aaronson, S. A., Abrams, J. M., Adam, D., Agostinis, P., et al. (2018). Molecular mechanisms of cell death: Recommendations of the nomenclature committee on cell death 2018. Cell. Death Differ. 25, 486–541. doi:10.1038/s41418-017-0012-4
Gauron, C., Rampon, C., Bouzaffour, M., Ipendey, E., Teillon, J., Volovitch, M., et al. (2013). Sustained production of ROS triggers compensatory proliferation and is required for regeneration to proceed. Sci. Rep. 3, 2084. doi:10.1038/srep02084
Geiszt, M., Witta, J., Baffi, J., Lekstrom, K., and Leto, T. L. (2003). Dual oxidases represent novel hydrogen peroxide sources supporting mucosal surface host defense. Faseb J. 17, 1502–1504. doi:10.1096/fj.02-1104fje
George, L. F., and Bates, E. A. (2022). Mechanisms underlying influence of bioelectricity in development. Front. Cell. Dev. Biol. 10, 772230.
Ghavami, S., Shojaei, S., Yeganeh, B., Ande, S. R., Jangamreddy, J. R., Mehrpour, M., et al. (2014). Autophagy and apoptosis dysfunction in neurodegenerative disorders. Prog. Neurobiol. 112, 24–49. doi:10.1016/j.pneurobio.2013.10.004
Green, D. R. (2022). Caspase activation and inhibition. Cold Spring Harb. Perspect. Biol. 14, a041020. doi:10.1101/cshperspect.a041020
Gregory, C. D., and Pound, J. D. (2011). Cell death in the neighbourhood: Direct microenvironmental effects of apoptosis in normal and neoplastic tissues. J. Pathol. 223, 177–194. doi:10.1002/path.2792
Guerin, D. J., Kha, C. X., and Tseng, K. A.-S. (2021). From cell death to regeneration: Rebuilding after injury. Front. Cell. Dev. Biol. 9, 655048. doi:10.3389/fcell.2021.655048
Hack, S. J., Kinsey, L. J., and Beane, W. S. (2021). An open question: Is non-ionizing radiation a tool for controlling apoptosis-induced proliferation? Int. J. Mol. Sci. 22, 11159. doi:10.3390/ijms222011159
Huang, Q., Li, F., Liu, X., Li, W., Shi, W., Liu, F. F., et al. (2011). Caspase 3-mediated stimulation of tumor cell repopulation during cancer radiotherapy. Nat. Med. 17, 860–866. doi:10.1038/nm.2385
Ichim, G., and Tait, S. W. (2016). A fate worse than death: Apoptosis as an oncogenic process. Nat. Rev. Cancer 16, 539–548. doi:10.1038/nrc.2016.58
Iwamoto, K. S., Sandstrom, R. E., Bryan, M., Liu, Y., Elgart, S. R., Sheng, K., et al. (2021). Weak magnetic fields enhance the efficacy of radiation therapy. Adv. Radiat. Oncol. 6, 100645. doi:10.1016/j.adro.2021.100645
Jiang, M.-J., Gu, D. N., Dai, J. J., Huang, Q., and Tian, L. (2020). Dark side of cytotoxic therapy: Chemoradiation-induced cell death and tumor repopulation. Trends Cancer. 6, 419–431.
Kakarla, R., Hur, J., Kim, Y. J., Kim, J., and Chwae, Y. J. (2020). Apoptotic cell-derived exosomes: Messages from dying cells. Exp. Mol. Med. 52, 1–6. doi:10.1038/s12276-019-0362-8
Katoh, I., Tomimori, Y., Ikawa, Y., and Kurata, S. (2004). Dimerization and processing of procaspase-9 by redox stress in mitochondria. J. Biol. Chem. 279, 15515–15523. doi:10.1074/jbc.M311819200
Kha, C. X., Nava, I., and Tseng, K. A.-S. (2023). V-atpase regulates retinal progenitor cell proliferation during eye regrowth in Xenopus. J. Ocular Pharmacol. Ther [Epub ahead of print].
Kha, C. X., Son, P. H., Lauper, J., and Tseng, K. A. (2018). A model for investigating developmental eye repair in Xenopus laevis. Exp. Eye Res. 169, 38–47. doi:10.1016/j.exer.2018.01.007
Kinsey, L., van Huizena, A., and Beane, W. (2023). Weak magnetic fields modulate superoxide to control planarian regeneration. Front. Phys. 10. doi:10.3389/fphy.2022.1086809
Ko, J. H., Ko, E. A., Gu, W., Lim, I., Bang, H., and Zhou, T. (2013). Expression profiling of ion channel genes predicts clinical outcome in breast cancer. Mol. Cancer 12, 106. doi:10.1186/1476-4598-12-106
Kondratskyi, A., Kondratska, K., Skryma, R., and Prevarskaya, N. (2015). Ion channels in the regulation of apoptosis. Biochim. Biophys. Acta 1848, 2532–2546. doi:10.1016/j.bbamem.2014.10.030
Kubat, N. J., Moffett, J., and Fray, L. M. (2015). Effect of pulsed electromagnetic field treatment on programmed resolution of inflammation pathway markers in human cells in culture. J. Inflamm. Res. 8, 59–69. doi:10.2147/JIR.S78631
Levin, M. (2021). Bioelectric signaling: Reprogrammable circuits underlying embryogenesis, regeneration, and cancer. Cell. 184, 1971–1989. doi:10.1016/j.cell.2021.02.034
Li, F., Huang, Q., Chen, J., Peng, Y., Roop, D. R., Bedford, J. S., et al. (2010). Apoptotic cells activate the "phoenix rising" pathway to promote wound healing and tissue regeneration. Sci. Signal 3, ra13. doi:10.1126/scisignal.2000634
Lima, R. T., Busacca, S., Almeida, G. M., Gaudino, G., Fennell, D. A., and Vasconcelos, M. H. (2011). MicroRNA regulation of core apoptosis pathways in cancer. Eur. J. Cancer 47, 163–174. doi:10.1016/j.ejca.2010.11.005
Liu, J., Liu, W., and Yang, H. (2019). Balancing apoptosis and autophagy for Parkinson's disease therapy: Targeting BCL-2. ACS Chem. Neurosci. 10, 792–802. doi:10.1021/acschemneuro.8b00356
Liu, X., He, Y., Li, F., Huang, Q., Kato, T. A., Hall, R. P., et al. (2015). Caspase-3 promotes genetic instability and carcinogenesis. Mol. Cell. 58, 284–296. doi:10.1016/j.molcel.2015.03.003
Lleo, A., Selmi, C., Invernizzi, P., Podda, M., and Gershwin, M. E. (2008). The consequences of apoptosis in autoimmunity. J. Autoimmun. 31, 257–262. doi:10.1016/j.jaut.2008.04.009
Love, N. R., Chen, Y., Ishibashi, S., Kritsiligkou, P., Lea, R., Koh, Y., et al. (2013). Amputation-induced reactive oxygen species are required for successful Xenopus tadpole tail regeneration. Nat. Cell. Biol. 15, 222–228. doi:10.1038/ncb2659
Maeda, S., Kamata, H., Luo, J. L., Leffert, H., and Karin, M. (2005). IKKbeta couples hepatocyte death to cytokine-driven compensatory proliferation that promotes chemical hepatocarcinogenesis. Cell. 121, 977–990. doi:10.1016/j.cell.2005.04.014
Maier, P., Hartmann, L., Wenz, F., and Herskind, C. (2016). Cellular pathways in response to ionizing radiation and their targetability for tumor radiosensitization. Int. J. Mol. Sci. 17, 102. doi:10.3390/ijms17010102
Martino, C. F., and Castello, P. R. (2011). Modulation of hydrogen peroxide production in cellular systems by low level magnetic fields. PLoS One 6, e22753. doi:10.1371/journal.pone.0022753
Mclaughlin, K. A., and Levin, M. (2018). Bioelectric signaling in regeneration: Mechanisms of ionic controls of growth and form. Dev. Biol. 433, 177–189.
Medina, C. B., Mehrotra, P., Arandjelovic, S., Perry, J. S. A., Guo, Y., Morioka, S., et al. (2020). Metabolites released from apoptotic cells act as tissue messengers. Nature 580, 130–135. doi:10.1038/s41586-020-2121-3
Mohamed, M. S., Bishr, M. K., Almutairi, F. M., and Ali, A. G. (2017). Inhibitors of apoptosis: Clinical implications in cancer. Apoptosis 22, 1487–1509. doi:10.1007/s10495-017-1429-4
Moloney, J. N., and Cotter, T. G. (2018). ROS signalling in the biology of cancer. Semin. Cell. Dev. Biol. 80, 50–64. doi:10.1016/j.semcdb.2017.05.023
Morbidelli, L., Monici, M., Marziliano, N., Cogoli, A., Fusi, F., Waltenberger, J., et al. (2005). Simulated hypogravity impairs the angiogenic response of endothelium by up-regulating apoptotic signals. Biochem. Biophys. Res. Commun. 334, 491–499. doi:10.1016/j.bbrc.2005.06.124
Morishima, N., Nakanishi, K., Takenouchi, H., Shibata, T., and Yasuhiko, Y. (2002). An endoplasmic reticulum stress-specific caspase cascade in apoptosis. Cytochrome c-independent activation of caspase-9 by caspase-12. J. Biol. Chem. 277, 34287–34294. doi:10.1074/jbc.M204973200
Murphy, M. P. (2009). How mitochondria produce reactive oxygen species. Biochem. J. 417, 1–13. doi:10.1042/BJ20081386
Nakagawa, T., Zhu, H., Morishima, N., Li, E., Xu, J., Yankner, B. A., et al. (2000). Caspase-12 mediates endoplasmic-reticulum-specific apoptosis and cytotoxicity by amyloid-beta. Nature 403, 98–103. doi:10.1038/47513
Nanayakkara, G. K., Wang, H., and Yang, X. (2019). Proton leak regulates mitochondrial reactive oxygen species generation in endothelial cell activation and inflammation - a novel concept. Arch. Biochem. Biophys. 662, 68–74. doi:10.1016/j.abb.2018.12.002
Nishina, T., Komazawa-Sakon, S., Yanaka, S., Piao, X., Zheng, D. M., Piao, J. H., et al. (2012). Interleukin-11 links oxidative stress and compensatory proliferation. Sci. Signal 5, ra5. doi:10.1126/scisignal.2002056
Pai, V. P., Lemire, J. M., Chen, Y., Lin, G., and Levin, M. (2015). Local and long-range endogenous resting potential gradients antagonistically regulate apoptosis and proliferation in the embryonic CNS. Int. J. Dev. Biol. 59, 327–340. doi:10.1387/ijdb.150197ml
Pai, V. P., Martyniuk, C. J., Echeverri, K., Sundelacruz, S., Kaplan, D. L., and Levin, M. (2016). Genome-wide analysis reveals conserved transcriptional responses downstream of resting potential change in Xenopus embryos, axolotl regeneration, and human mesenchymal cell differentiation. Regen. (Oxf) 3, 3–25. doi:10.1002/reg2.48
Peek, M. C., Ahmed, M., Napoli, A., Ten Haken, B., Mcwilliams, S., Usiskin, S. I., et al. (2015). Systematic review of high-intensity focused ultrasound ablation in the treatment of breast cancer. Br. J. Surg. 102, 873–882. doi:10.1002/bjs.9793
Pei, Z. H., Chen, B. Y., Tie, R., Zhang, H. F., Zhao, G., Qu, P., et al. (2011). Infrasound exposure induces apoptosis of rat cardiac myocytes by regulating the expression of apoptosis-related proteins. Cardiovasc. Toxicol. 11, 341–346.
Rapizzi, E., Pinton, P., Szabadkai, G., Wieckowski, M. R., Vandecasteele, G., Baird, G., et al. (2002). Recombinant expression of the voltage-dependent anion channel enhances the transfer of Ca2+ microdomains to mitochondria. J. Cell. Biol. 159, 613–624. doi:10.1083/jcb.200205091
Redza-Dutordoir, M., and Averill-Bates, D. A. (2016). Activation of apoptosis signalling pathways by reactive oxygen species. Biochim. Biophys. Acta 1863, 2977–2992. doi:10.1016/j.bbamcr.2016.09.012
Riley, J. S., and Bock, F. J. (2022). Voices from beyond the grave: The impact of apoptosis on the microenvironment. Biochim. Biophys. Acta Mol. Cell. Res. 1869, 119341. doi:10.1016/j.bbamcr.2022.119341
Ross, C. L., Ang, D. C., and Almeida-Porada, G. (2019). Targeting mesenchymal stromal cells/pericytes (MSCs) with pulsed electromagnetic field (PEMF) has the potential to treat rheumatoid arthritis. Front. Immunol. 10, 266. doi:10.3389/fimmu.2019.00266
Ross, C. L., and Harrison, B. S. (2013). Effect of pulsed electromagnetic field on inflammatory pathway markers in RAW 264.7 murine macrophages. J. Inflamm. Res. 6, 45–51. doi:10.2147/JIR.S40269
Shuryak, I., Sachs, R. K., and Brenner, D. J. (2007). Biophysical models of radiation bystander effects: 1. Spatial effects in three-dimensional tissues. Radiat. Res. 168, 741–749. doi:10.1667/RR1117.1
Sia, J., Szmyd, R., Hau, E., and Gee, H. E. (2020). Molecular mechanisms of radiation-induced cancer cell death: A primer. Front. Cell. Dev. Biol. 8, 41. doi:10.3389/fcell.2020.00041
Sies, H., and Jones, D. P. (2020). Reactive oxygen species (ROS) as pleiotropic physiological signalling agents. Nat. Rev. Mol. Cell. Biol. 21, 363–383. doi:10.1038/s41580-020-0230-3
Singh, R., Letai, A., and Sarosiek, K. (2019). Regulation of apoptosis in health and disease: The balancing act of BCL-2 family proteins. Nat. Rev. Mol. Cell. Biol. 20, 175–193. doi:10.1038/s41580-018-0089-8
Sun, G., Li, J., Zhou, W., Hoyle, R. G., and Zhao, Y. (2022). Electromagnetic interactions in regulations of cell behaviors and morphogenesis. Front. Cell. Dev. Biol. 10, 1014030. doi:10.3389/fcell.2022.1014030
Surucu, M., Shah, K. K., Mescioglu, I., Roeske, J. C., Small, W., Choi, M., et al. (2016). Decision trees predicting tumor shrinkage for head and neck cancer: Implications for adaptive radiotherapy. Technol. Cancer Res. Treat. 15, 139–145. doi:10.1177/1533034615572638
Tan, D. Q., and Suda, T. (2018). Reactive oxygen species and mitochondrial homeostasis as regulators of stem cell fate and function. Antioxid. Redox Signal 29, 149–168. doi:10.1089/ars.2017.7273
Tseng, A. S., Adams, D. S., Qiu, D., Koustubhan, P., and Levin, M. (2007). Apoptosis is required during early stages of tail regeneration in Xenopus laevis. Dev. Biol. 301, 62–69. doi:10.1016/j.ydbio.2006.10.048
Tseng, A. S., Beane, W. S., Lemire, J. M., Masi, A., and Levin, M. (2010). Induction of vertebrate regeneration by a transient sodium current. J. Neurosci. 30, 13192–13200. doi:10.1523/JNEUROSCI.3315-10.2010
van Huizen, A. V., Morton, J. M., Kinsey, L. J., von Kannon, D. G., Saad, M. A., Birkholz, T. R., et al. (2019). Weak magnetic fields alter stem cell–mediated growth. Sci. Adv. 5, eaau7201. doi:10.1126/sciadv.aau7201
Wang, Y., Qi, H., Liu, Y., Duan, C., Liu, X., Xia, T., et al. (2021). The double-edged roles of ROS in cancer prevention and therapy. Theranostics 11, 4839–4857. doi:10.7150/thno.56747
Warren, C. F. A., Wong-Brown, M. W., and Bowden, N. A. (2019). BCL-2 family isoforms in apoptosis and cancer. Cell. Death Dis. 10, 177. doi:10.1038/s41419-019-1407-6
Williams, S., Bateman, A., and O'Kelly, I. (2013). Altered expression of two-pore domain potassium (K2P) channels in cancer. PLoS One 8, e74589. doi:10.1371/journal.pone.0074589
Xu, A., Wang, Q., Lv, X., and Lin, T. (2021). Progressive study on the non-thermal effects of magnetic field therapy in oncology. Front. Oncol. 11, 638146. doi:10.3389/fonc.2021.638146
Xu, M., Li, X., Walsh, S. W., Zhang, Y., Abais, J. M., Boini, K. M., et al. (2013). Intracellular two-phase Ca2+ release and apoptosis controlled by TRP-ML1 channel activity in coronary arterial myocytes. Am. J. Physiol. Cell. Physiol. 304, C458–C466. doi:10.1152/ajpcell.00342.2012
Yoshida, K., and Miki, Y. (2010). The cell death machinery governed by the p53 tumor suppressor in response to DNA damage. Cancer Sci. 101, 831–835. doi:10.1111/j.1349-7006.2010.01488.x
Yuan, L. Q., Wang, C., Lu, D. F., Zhao, X. D., Tan, L. H., and Chen, X. (2020). Induction of apoptosis and ferroptosis by a tumor suppressing magnetic field through ROS-mediated DNA damage. Aging (Albany NY) 12, 3662–3681. doi:10.18632/aging.102836
Zadeh-Haghighi, H., and Simon, C. (2022). Magnetic field effects in biology from the perspective of the radical pair mechanism. J. R. Soc. Interface 19, 20220325. doi:10.1098/rsif.2022.0325
Zhang, L., Li, C., and Su, X. (2020). Emerging impact of the long noncoding RNA MIR22HG on proliferation and apoptosis in multiple human cancers. J. Exp. Clin. Cancer Res. 39, 271. doi:10.1186/s13046-020-01784-8
Zhao, M., Wang, Y., Zhao, Y., He, S., Zhao, R., Song, Y., et al. (2020). Caspase-3 knockout attenuates radiation-induced tumor repopulation via impairing the ATM/p53/Cox-2/PGE(2) pathway in non-small cell lung cancer. Aging (Albany NY) 12, 21758–21776. doi:10.18632/aging.103984
Zhou, R., Wu, Y., Wang, W., Su, W., Liu, Y., Wang, Y., et al. (2018). Circular RNAs (circRNAs) in cancer. Cancer Lett. 425, 134–142. doi:10.1016/j.canlet.2018.03.035
Zhou, Y. F. (2011). High intensity focused ultrasound in clinical tumor ablation. World J. Clin. Oncol. 2, 8–27. doi:10.5306/wjco.v2.i1.8
Keywords: apoptosis, cell death, ROS, reactive oxygen species, bioelectric signaling, cancer, regeneration, quantum biology
Citation: Hack SJ, Beane WS and Tseng KA-S (2023) Biophysics at the edge of life and death: Radical control of apoptotic mechanisms. Front. Cell. Death 2:1147605. doi: 10.3389/fceld.2023.1147605
Received: 18 January 2023; Accepted: 13 February 2023;
Published: 27 February 2023.
Edited by:
Olivier Micheau, Université de Bourgogne, FranceReviewed by:
Victor J. Yuste, Autonomous University of Barcelona, SpainCopyright © 2023 Hack, Beane and Tseng. This is an open-access article distributed under the terms of the Creative Commons Attribution License (CC BY). The use, distribution or reproduction in other forums is permitted, provided the original author(s) and the copyright owner(s) are credited and that the original publication in this journal is cited, in accordance with accepted academic practice. No use, distribution or reproduction is permitted which does not comply with these terms.
*Correspondence: Kelly Ai-Sun Tseng, a2VsbHkudHNlbmdAdW5sdi5lZHU=
Disclaimer: All claims expressed in this article are solely those of the authors and do not necessarily represent those of their affiliated organizations, or those of the publisher, the editors and the reviewers. Any product that may be evaluated in this article or claim that may be made by its manufacturer is not guaranteed or endorsed by the publisher.
Research integrity at Frontiers
Learn more about the work of our research integrity team to safeguard the quality of each article we publish.