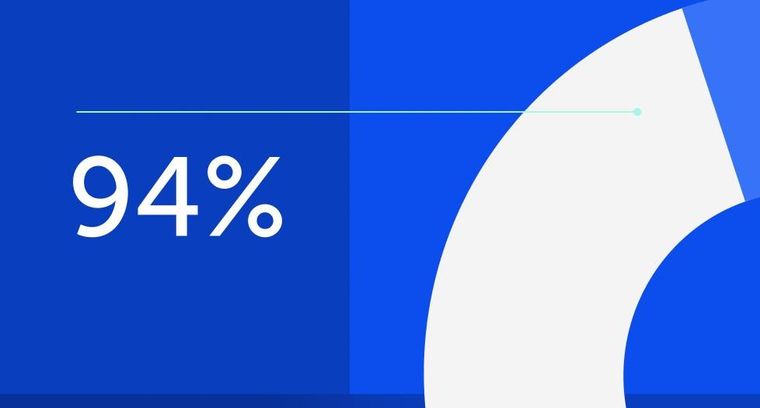
94% of researchers rate our articles as excellent or good
Learn more about the work of our research integrity team to safeguard the quality of each article we publish.
Find out more
SPECIALTY GRAND CHALLENGE article
Front. Cell Death, 05 December 2022
Sec. Model Systems in Cell Death
Volume 1 - 2022 | https://doi.org/10.3389/fceld.2022.1087903
This article is part of the Research TopicGrand Challenges in Cell Death ResearchView all articles
In 1929, the Nobel Prize winning physiologist August Krough observed that “For a large number of problems there will be some animal of choice, or a few such animals, on which it can be most conveniently studied.” (Krogh, 1929). Known as the Krough Principal, this appreciation of a “model systems” approach has been foundational for many aspects of basic biology, from the use of the squid giant axon to define the ionic basis of the action potential to the use of the fruit fly to unlock the molecular basis of biological clocks. While the ultimate goal for many researchers may be to gain a better understanding of human development and/or pathogenesis, the complexity of mammalian systems often makes direct analyses challenging. Invertebrates and other “simpler” model systems often display adaptations that exaggerate normal cellular processes that make them attractive vehicles for the analysis of specific traits.
This approach has also proven to be foundational for the study of cell death. The term “programmed cell death” (PCD) (now commonly referred to as “regulated cell death” to distinguish it from “accidental cell death” (Galluzzi et al., 2018)) was coined by Lockshin and Williams in 1965 to describe the precisely timed loss of the intersegmental muscles of Lepidoptera at the end of metamorphosis (Lockshin and Williams, 1965). These giant cells (each of which is ∼5 mm long and up to 1 mm in diameter depending on the species) initiate PCD coincident with the emergence of the adult moth from the overlying pupal cuticle. Few other naturally occurring examples of PCD are so exquisitely timed or offer such prodigious amounts of clean cellular material for molecular and biochemical analyses (e.g., Tsuji et al., 2020). However, it was another invertebrate model, the nematode Caenorhabditis elegans, that propelled the field of cell death from a small cottage industry with a few dozen investigators in the 1970s and 1980s into a massive research enterprise that has produced more than 560,000 publications during the past 30 years. One of the unique features of C. elegans that make it such an attractive model is that it displays “cell consistency”, meaning that every individual has the same number of somatic cells. By performing detailed lineage analyses, the identity and fate of every single cell was described by Sulston and Horvitz (Sulston and Horvitz, 1977). For about 20% of the cells, their fate is to die, primarily via apoptosis. At the time this work was conducted it was not well understood if PCD during development reflected the simple wasting away of surplus/unnecessary cells, active murder by neighboring cells, or cell-autonomous suicide. Using a clever genetic trick that prevented dying cells from being phagocytosed and thus rapidly removed, the Horvitz lab demonstrated that the ability of cells to die required the activity of specific genes that acted in a cell autonomous manner, and thus represented cellular suicide (Ellis and Horvitz, 1986; Yuan and Horvitz, 1990). This model allowed them to identify both Ced-3 (caspase) and Ced-4 (Apaf1) as essential components of the killing machinery (Yuan and Horvitz, 1992; Yuan et al., 1993). Subsequent studies demonstrated that Ced-9 encodes a potent and essential survival protein that protects most cells from death during development (Hengartner et al., 1992). Apoptosis is such an ancient and evolutionarily conserved program that the human anti-apoptosis protein Bcl-2 can functionally substitute for Ced-9 in transgenic worms, despite a having last shared a common ancestor some 600 million years ago (Vaux et al., 1992).
While C. elegans has been invaluable for defining the core machinery of apoptosis, it has more limited utility for some other lines of investigation. For example, early in the history of the field, three primary mechanisms of cell death were recognized-apoptosis (type I degeneration), necrosis, and type II degeneration (autophagy dependent cell death (ADCD) (Schweichel and Merker, 1973; Clarke, 1990). Even though C. elegans has been foundational for the study of apoptosis, this organism does not appear to significantly employ ADCD to mediate cell loss during development, meaning that other organisms have proven to be more useful for exploring this mechanism (Takacs-Vellai et al., 2005). (This highlights the reality that while a given model system may represent an exceptional choice for one line of investigation, it may be more limited utility for another).
The primary mechanism of autophagy, macro-autophagy, involves the sequestration of damaged organelles and bulk cytoplasm into double membrane vesicles that ultimately fuse with lysosomes to form autophagosomes, where the contents are degraded and recycled (Gómez-Virgilio et al., 2022). In many cases this process allows cells to survive starvation and other insults and can even serve to suppress apoptosis in some situations (Bata and Cosford, 2021). Consequently, it was unclear if autophagy during cell death reflects an aborted effort for survival or an authentic cell killing mechanism (Schwartz, 2021). In fact, it was suggested that the term “autophagic cell death” may be a misnomer and that “cell death with autophagy” might be a more accurate descriptor of the process (Kroemer and Levine, 2008). In this regard, the fruit fly Drosophila has proven to be perhaps the best model for addressing this question. (While lacking the cell consistency of C. elegans, Drosophila does support an exceptional array of genetic tools that allow researchers to analyze and/or manipulate essentially any gene, at any stage of development, in almost any cell (e.g., Heigwer et al., 2018; Zirin et al., 2022). The ability to perform genome-wide RNAi and CRISPR screens has proven to be an invaluable approach for identifying key components of genetic pathways and modifiers that regulate them.
Genetic studies targeting the autophagy pathway in Drosophila have demonstrated unambiguously that autophagy is required for the loss of specific cells during metamorphosis (reviewed in Zhang and Baehrecke, 2015; Yalonetskaya et al., 2018). During metamorphosis, larval-specific tissues are degraded or remodeled while adult-specific structures form to meet the animal’s new lifestyle. Among the tissues that experience extensive cell death are the salivary gland and portions of the gut (Restifo and White, 1992). The Baehrecke lab employed gene inactivation or knockdown to target components of the apoptosis and autophagy pathways in order to investigate the molecular mechanisms that mediate the loss of these cells. They found that salivary gland death requires both apoptosis and autophagy, while the demise of the midgut depended exclusively on autophagy (Martin and Baehrecke, 2004; Berry and Baehrecke 2007; Denton et al., 2009, 2012). These studies were foundational for demonstrating unambiguously that autophagy can mediate the death of some cells.
Independent studies with the slime mold Dictyostelium further supported a functional role for autophagy in cell death during development. These social amoebae live most of their lives as solitary cells consuming bacteria and other organic substrates. However, when faced with starvation, individual cells aggregate and form a multicellular fruiting body with ∼100,000 members. The stalk cells die as a normal component of development, which facilitates the dissemination of the spores. Genetic and pharmacological studies suggest that the loss of these cells is dependent on the autophagy pathway although some questions remain (Mesquita et al., 2017).
These examples highlight some of ways in which model systems have proven to be invaluable tools for examining foundational aspects of cell death. Obviously, many other key insights have not been included in this brief summery. That said, there are still major areas of the field where our understanding is far from complete. Some examples of where model systems could provide invaluable insights to advance the field are described below.
For many researchers, the terms “programmed cell death” and “apoptosis” are used interchangeably. This is clearly an over simplification that ignores the fact that more than a dozen distinct cell death programs have been described, each with its own underlying biochemical mechanisms and roles in development, homeostasis, and/or pathogenesis. In addition to the classic programs of necrosis, apoptosis, and ADCD described above, other distinct pathways include: ferroptosis, parthanatos, entosis, necroptosis, pyroptosis, NETotic cell death, mitochondrial permeability transition, linker cell death, and mitotic catastrophe (Abraham et al., 2007; Galluzzi et al., 2018; Tang et al., 2019; Nirmala and Lopus, 2020). This year alone, copper-dependent cell death, cuproptosis, and erebosis, a cell death process that involves the loss of the cytoskeleton and the accumulation of Angiotensin-Converting Enzyme, were described for the first time as novel cell death pathways (Ciesielski et al., 2022; Tsvetkov et al., 2022). It should be noted that erebosis was identified as a potentially distinct mode of cell death that occurs during gut cell turnover during fruit fly metamorphosis (Ciesielski et al., 2022).
This list includes several forms of programmed necrosis, including: ferroptosis, necroptosis, pyroptosis, and NETosis (Galluzzi et al., 2018). These deaths result in cellular swelling and ultimately lysis, leading to the release of highly inflammatory cellular constituents termed DAMPs (damage-associated molecular patterns) (Nakano et al., 2022). While these pathways were initially described in mammals, they appear to represent ancient programs. For example, ferroptosis has been observed in such diverse organisms as insects (Mumbauer et al., 2019) and plants (Distéfano et al., 2021). Pyroptosis, a process whose best described role is cytokine activation, has been observed in corals (Jiang et al., 2020). While largely presumed to function as a defense against pathogens, necrotic cell deaths may serve other roles as well. For instance in flies, a p53-dependent necrotic program is activated during spermatogenesis, unrelated to infection (Napoletano et al., 2017). How did these ancient necrotic programs evolve and how are they controlled?
Non-mammalian models, with their diverse developmental strategies and powerful genetic tools, represent promising vehicles for discovering yet new cell death programs. In addition, employing a phylogenetic approach should provide fundamental insights into the breadth and evolution of cell death mechanisms.
Not surprisingly, the regulation of cell death is a complex and carefully orchestrated set of regulatory processes and there are numerous examples of interplay between different cell death programs. The activation of autophagy following nutrient deprivation can serve to enhance survival and prevent the activation of apoptosis (reviewed in Ploumi et al., 2022). While there are many molecular mechanisms for this cross-talk, perhaps the best characterized example is Beclin-1, which sits at the intersection of autophagy and apoptosis (He and Levine, 2010). Beclin-1 binds to class III phosphatidylinositol-3 kinase, a key mediator of autophagosome formation (Kihara et al., 2001). As a BH3-only protein, it can also bind the anti-apoptotic protein Bcl-2, which functions to inhibit apoptosis. Other BH3-only proteins can displace the Bcl-2 from Beclin-1, leading to the initiation of apoptosis (Elgendy et al., 2011). Beclin-1 is also a caspase-3 substrate, and its cleavage produces a C-terminal fragment that can directly trigger mitochondrial permeability and the subsequent release of pro-apoptotic factors (Wirawan et al., 2010; Zhu et al., 2010). Thus, there are numerous cross-regulatory interactions between the proteins that mediate autophagy and apoptosis.
The pro-inflammatory cytokine tumor necrosis factor-α (TNF-α) can drive both apoptosis and necroptosis by binding to membrane-associated TNF Receptors (TNFR). This leads to the formation of the death-inducing signaling complex (DISC) and a cascade of events that results in the autocatalytic activation of pro-caspase-8. This in turn drives the extrinsic apoptosis pathway via caspase-3 activation, and the intrinsic pathway by converting BID into the mitochondrially targeted tBid (Li et al., 1998). Separately, TNFR activation can also recruit RIPK1 and other proteins to form the necrosome, a signal transduction cascade that triggers necroptosis (Li et al., 2021). There is significant crosstalk between these two cell death pathways. For example, caspase-8 can cleave RIPK1, and thus simultaneously drive apoptosis and inhibit necroptosis. Paradoxically, in addition to its well-defined role in necroptosis, RIPK1 can also trigger pro-caspase activation and apoptosis (Zhang et al., 2019). Thus, like autophagy and apoptosis, there is a complex interplay between necroptosis and apoptosis.
As the old proverb states, “the devil is in the details”. If all of these different cell death programs arose independently during evolution, when and how did they develop the capacity to regulate one another? Are they all regulated in the same manner within the same tissue or within the same organism? Did the capacity to cross regulate different cell death programs provide organisms with fundamentally new developmental or homeostatic capabilities? Defining these signal transduction pathways will be essential to understanding fundamental aspects of development, immune responses, and pathogenesis.
Caspases are best known for driving apoptosis. Initiator caspases, like caspases-8 and -9, activate executioner pro-caspase zymogens (eg. caspases-3, -6, -7, and/or -10), which in turn cleave a myriad of cellular substrates resulting in the well-orchestrated process of apoptosis (Green, 2022). Caspase-1 (also known as interleukin-1 beta-converting enzyme) was the first caspase described and it plays essential roles in inflammation by cleaving pro-inflammatory cytokines like interleukins-1β and -18 (Kostura et al., 1989). These cytokines then drive a range of potent inflammatory responses including pyroptosis, a form of programmed necrosis that facilitates the clearance of pathogens (reviewed in Kesavardhana et al., 2020).
While a prevailing assumption in the field is that caspase activation represents an irrevocable “go/no go” event in apoptosis, focal caspase activation has been shown to serve important developmental roles in several model systems. Indeed, fruit flies expressing the fluorescent caspase reporter CasExpress reveal that caspases are active in a range of tissues during development without the subsequent demise of the cell (Ding et al., 2016). A number of studies have demonstrated that transient caspase activity is required for normal development and homeostasis in animals (reviewed in Nakajima and Kuranaga, 2017). For example, activation of the initiator caspase Dronc in fruit flies (a caspase-9 ortholog) has been shown to mediate elasticity in the salivary gland by cleaving actin without triggering concurrent cell death (Kang et al., 2017). Focal caspase activation can also drive mitosis by releasing mitogens in an apoptosis-induced regeneration process in the wing disc (Huh et al., 2004; Amcheslavsky et al., 2018; Sun et al., 2020). As well, during neurodevelopment, focal caspase activation prunes dendrites in the nervous system, thus facilitating the removal of surplus or inappropriate synaptic connections without overt damage to the cell (Williams et al., 2006; Ertürk et al., 2014).
Is the transient activation of caspases a more generalized process during development? If so, what regulatory processes restrict caspase activity so that it doesn’t snowball into apoptosis? Some mechanisms that focally restrain caspase activity include restricting caspases to specific subcellular locations and/or the judicious expression of inhibitor of apoptosis proteins (Vasudevan and Ryoo, 2015). Are there others? Understanding how caspases can be exploited to help refine developmental processes is both a fundamental and largely unexplored topic. It is likely that the diversity of cellular and developmental specializations displayed by different invertebrates may represent useful models for exploring these questions.
Like animals, plants employ PCD at multiple stages of development (reviewed in Sueldo and van der Hoorn, 2017). During the formation of the xylem, a structure that facilitates the transport of water and nutrients from the roots to the rest of the plant, tracheary cells undergo an autolytic cell death in order to form the hollow vessel. The death of tapetum cells in the anthers of flowering plants is required for proper pollen formation. During seed development the suspensor cells transport nutrients from parental tissues to the early embryo and then undergo cell death as embryogenesis progresses.
Plants can also reactively trigger the hypersensitive response (HR), a rapid onset focal cell death process that restricts the spread of pathogens (Balint-Kurti, 2019). In most cases, HR death is mediated by a family of nucleotide-binding site and leucine-rich repeat domains (NLR) resistance genes (Chakraborty et al., 2018). HR-mediated death displays some aspects of apoptosis (cellular shrinkage, genomic DNA degradation, TUNEL staining, mitochondrial depolarization) and certain features of autophagy (vesicles with cellular constituents) but does not cleanly fall into any of the cell death programs described for animal cells (Mur et al., 2008). Indeed, it has been assumed that apoptosis does not exist in plants (Locato and De Gara, 2018). This raises several questions about the evolution of cell death programs in plants and how they may have evolved from a common eukaryotic ancestor. For example, while plants do not encode caspases, they do utilize cysteine metacaspases in some cell death processes (Minina et al., 2017). Unlike canonical caspases that cleave after aspartates, the metacaspases cleave after lysine and arginine residues (Fagundes et al., 2015). Another possible mechanism for HR-mediated death is the NLR protein ZAR1. It has been shown to associate with other binding partners to form a membrane associated pentaramic channel known as the resistosome (Wang et al., 2019). It is possible that this triggers a necrotic type of cell death that nevertheless displays some apoptotic features.
Far less is known about the mechanisms of cell death in plants relative to animals. This is disappointing since plants make up the majority of the biomass on the planet and they serve essential ecological roles in terms of creating diverse habitats, food, CO2 sequestration, and even atmospheric oxygen. Consequently, a deeper analysis of plant cell death would not only enhance our understanding of important basic biological processes, it may also empower us to better help plants survive in a rapidly changing environment.
PCD serves a myriad of roles in eukaryotes, including: sculpting the body (e.g., loss of interdigital cells during limb formation), removing deleterious cells (e.g., self-reactive thymocytes during negative selection), matching populations of interacting cells (e.g., motor neurons and muscles), etc. (reviewed in Milligan and Schwartz, 1997; Fuchs and Steller, 2011). However, at first glance, it is counter intuitive that PCD might serve some beneficial role for prokaryotes, unicellular organisms that lack tissues. However, there are many circumstances where bacteria can aggregate to form multicellular communities (Lyons and Kolter, 2015). Within this context, the ability of some cells to undergo an altruistic death can confer significant benefits to the larger community, and thus ultimately benefit the clonally-related condemned cell. These deaths can aid in sporulation, biofilm maintenance, mycelium formation, the uptake of extracellular DNA via transformation, antibiotic production, etc. (reviewed in Allocati et al., 2015). For example, biofilms, which can contribute to pathogenesis in several human disorders including cystic fibrosis, urinary tract infections and endocarditis, display cell death at specific stages of development. These autolytic deaths provide nutrients for the colony and thus facilitate survival.
Three primary cell death pathways have been described in bacteria: apoptosis-like death (ALD), thymineless death (TLD), and toxin-antitoxin (TA) mediated death (Lee and Lee, 2019). ALD displays many features of apoptosis, including DNA fragmentation and phosphatidylserine externalization. TLD-mediated death occurs following thymine restriction and results in substantial single-stranded DNA breakage and chromosome segregation failure during replication. Separately, some bacteria produce toxins that can induce the death of the bacterial cell but are held in check by the co-expression of an anti-toxin protein. During times of stress, the destruction of the antitoxin functionally de-represses toxin activity, resulting in the demise of the cell.
The range of cell death mechanisms available to prokaryotes has likely not been fully described. In addition, it is not clear if and how these more ancient prokaryotic cell death programs may have contributed to the evolution of eukaryotic cell death mechanisms like apoptosis. Indeed, the death of prokaryotes has been referred to as “proapoptosis” (Hochman, 1997). There is compelling evidence that mitochondria represent ancient bacterial symbionts (Margulis, 1975). Did these prokaryotic cells bring their own cell death mechanisms to eukaryotes (Koonin and Aravind, 2002)? Mitochondria play essential roles in driving the intrinsic apoptosis pathway and are central to other cell death programs as well, like autophagy (Abate et al., 2020). While caspases are not present in the bacterial genome, some bacteria like Helicobacter pylori, display DNA fragmentation and electron dense DNA when they die (Cellini et al., 2001). Is this process driven by the larger metacaspase family of proteases or some other enzymatic system yet to be characterized (Basmaciyan and Casanova 2019)?
Understanding prokaryotic cell death will not only enhance our fundamental understanding of the origin and diversification of cell death programs, they may also provide tools for the prevention or remediation of some of the more devastating plant and animal bacterial pathogens. Early work on the use of PCD as a target in antibiotic treatment (Tanouchi et al., 2013) provides just one example of some of the potential uses of prokaryotic PCD mechanisms to improve both agricultural productivity and human and animal health.
While there has been amazing progress in the field of cell death research over the past 30 years, many aspects remain to be discovered. The PCD community has moved away from the rather simplisitic view that all cell deaths can be binned into one of three programs (apoptosis, necrosis and ADCD) and now recognize that there are perhaps a dozen or more distinct pathways. How many still await description? How did these programs evolve and how are they used to help engineer embryogenesis and maintain homeostasis? How does misregulation of cell death contribute to pathogenesis and can we use these insights to develop better therapeutics? The use of non-mammalian model systems can help advance these goals. For example, a variety of genetic engineering approaches can be used to create humanized animal models in worms, flies and fish can be exploited for high throughput drug screens to identify new therapeutics (Rajan et al., 2020; Savitsky et al., 2020; Zhu et al., 2020). One of the great promises of the field is that we will be able to activate or inhibit cell death in a lineage-specific manner to block disease or enhance regeneration. For us to achieve that potential will require substantially more basic research to define the molecular mechanisms and their interactions that drive cell death. Model systems in cell death (MSCD) looks forward to publishing papers that help meet these challenges.
The author confirms being the sole contributor of this work and has approved it for publication.
This work was supported by the Eugene M. and Ronnie Isenberg Endowment.
I would like to thank Drs. Zhenggang Liu (NIH), Margaret Riley (UMass), and Madelaine Bartlett (UMass) for a critical reading of the manuscript and helpful suggestions.
The author declares that the research was conducted in the absence of any commercial or financial relationships that could be construed as a potential conflict of interest.
All claims expressed in this article are solely those of the authors and do not necessarily represent those of their affiliated organizations, or those of the publisher, the editors and the reviewers. Any product that may be evaluated in this article, or claim that may be made by its manufacturer, is not guaranteed or endorsed by the publisher.
Abate, M., Festa, A., Falco, M., Lombardi, A., Luce, A., Grimaldi, A., et al. (2020). Mitochondria as playmakers of apoptosis, autophagy and senescence. Semin. Cell Dev. Biol. 98, 139–153. doi:10.1016/j.semcdb.2019.05.022
Abraham, M. C., Lu, Y., and Shaham, S. (2007). A morphologically conserved nonapoptotic program promotes linker cell death in Caenorhabditis elegans. Dev. Cell 12, 73–86. doi:10.1016/j.devcel.2006.11.012
Allocati, N., Masulli, M., Di Ilio, C., and De Laurenzi, V. (2015). Die for the community: An overview of programmed cell death in bacteria. Cell Death Dis. 6 (1), e1609. doi:10.1038/cddis.2014.570
Amcheslavsky, A., Wang, S., Fogarty, C. E., Lindblad, J. L., Fan, Y., and Bergmann, A. (2018). Plasma membrane localization of apoptotic caspases for non-apoptotic functions. Dev. Cell 45, 450–464. doi:10.1016/j.devcel.2018.04.020
Balint-Kurti, P. (2019). The plant hypersensitive response: Concepts, control and consequences. Mol. Plant Pathol. 20, 1163–1178. doi:10.1111/mpp.12821
Basmaciyan, L., and Casanova, M. (2019). Cell death in leishmania. Parasite 26, 71. doi:10.1051/parasite/2019071
Bata, N., and Cosford, N. D. P. (2021). Cell survival and cell death at the intersection of autophagy and apoptosis: Implications for current and future cancer therapeutics. ACS Pharmacol. Transl. Sci. 4, 1728–1746. doi:10.1021/acsptsci.1c00130
Berry, D. L., and Baehrecke, E. H. (2007). Growth arrest and autophagy are required for salivary gland cell degradation in Drosophila. Cell 131, 1137–1148. doi:10.1016/j.cell.2007.10.048
Cellini, L., Robuffo, I., Maraldi, N. M., and Donelli, G. (2001). Searching the point of no return in Helicobacter pylori life: Necrosis and/or programmed death? J. Appl. Microbiol. 90, 727–732. doi:10.1046/j.1365-2672.2001.01300.x
Chakraborty, J., Ghosh, P., and Das, S. (2018). Autoimmunity in plants. Planta 248, 751–767. doi:10.1007/s00425-018-2956-0
Ciesielski, H. M., Nishida, H., Takano, T., Fukuhara, A., Otani, T., Ikegawa, Y., et al. (2022). Erebosis, a new cell death mechanism during homeostatic turnover of gut enterocytes. PLoS Biol. 20, e3001586. doi:10.1371/journal.pbio.3001586
Clarke, P. G. H. (1990). Developmental cell death: Morphological diversity and multiple mechanisms. Anat. Embryol. 181, 195–213. doi:10.1007/BF00174615
Denton, D., Chang, T. K., Nicolson, S., Shravage, B., Simin, R., Baehrecke, E. H., et al. (2012). Relationship between growth arrest and autophagy in midgut programmed cell death in Drosophila. Cell Death Differ. 19, 1299–1307. doi:10.1038/cdd.2012.43
Denton, D., Shravage, B., Simin, R., Mills, K., Berry, D. L., Baehrecke, E. H., et al. (2009). Autophagy, not apoptosis, is essential for midgut cell death in Drosophila. Curr. Biol. 19, 1741–1746. doi:10.1016/j.cub.2009.08.042
Ding, A. X., Sun, G., Argaw, Y. G., Wong, J. O., Easwaran, S., and Montell, D. J. (2016). CasExpress reveals widespread and diverse patterns of cell survival of caspase-3 activation during development in vivo. Elife 5, e10936. doi:10.7554/eLife.10936
Distéfano, A. M., López, G. A., Setzes, N., Marchetti, F., Cainzos, M., Cascallares, M., et al. (2021). Ferroptosis in plants: Triggers, proposed mechanisms, and the role of iron in modulating cell death. J. Exp. Bot. 72, 2125–2135. doi:10.1093/jxb/eraa425
Elgendy, M., Sheridan, C., Brumatti, G., and Martin, S. J. (2011). Oncogenic Ras-induced expression of Noxa and Beclin-1 promotes autophagic cell death and limits clonogenic survival. Mol. Cell 42, 23–35. doi:10.1016/j.molcel.2011.02.009
Ellis, H. M., and Horvitz, H. R. (1986). Genetic control of programmed cell death in the nematode C. elegans. Cell 44, 817–829. doi:10.1016/0092-8674(86)90004-8
Ertürk, A., Wang, Y., and Sheng, M. (2014). Local pruning of dendrites and spines by caspase-3-dependent and proteasome-limited mechanisms. J. Neurosci. 34, 1672–1688. doi:10.1523/JNEUROSCI.3121-13.2014
Fagundes, D., Bohn, B., Cabreira, C., Leipelt, F., Dias, N., Bodanese-Zanettini, M. H., et al. (2015). Caspases in plants: Metacaspase gene family in plant stress responses. Funct. Integr. Genomics 15, 639–649. doi:10.1007/s10142-015-0459-7
Fuchs, Y., and Steller, H. (2011). Programmed cell death in animal development and disease. Cell 147, 742–758. doi:10.1016/j.cell.2011.10.033
Galluzzi, L., Vitale, I., Aaronson, S. A., Abrams, J. M., Adam, D., Agostinis, P., et al. (2018). Molecular mechanisms of cell death: Recommendations of the nomenclature committee on cell death 2018. Cell Death Differ. 25, 486–541. doi:10.1038/s41418-017-0012-4
Gómez-Virgilio, L., Silva-Lucero, M. D., Flores-Morelos, D. S., Gallardo-Nieto, J., Lopez-Toledo, G., Abarca-Fernandez, A. M., et al. (2022). Autophagy: A key regulator of homeostasis and disease: An overview of molecular mechanisms and modulators. Cells 11, 2262. doi:10.3390/cells11152262
Green, D. R. (2022). Caspases and their substrates. Cold Spring Harb. Perspect. Biol. 14, a041012. doi:10.1101/cshperspect.a041012
He, C., and Levine, B. (2010). The Beclin 1 interactome. Curr. Opin. Cell Biol. 22, 140–149. doi:10.1016/j.ceb.2010.01.001
Heigwer, F., Port, F., and Boutros, M. (2018). RNA interference (RNAi) screening in Drosophila. Screen. Drosophila Genet. 208, 853–874. doi:10.1534/genetics.117.300077
Hengartner, M. O., Ellis, R. E., and Horvitz, H. R. (1992). Caenorhabditis elegans gene ced-9 protects cells from programmed cell death. Nature 356, 494–499. doi:10.1038/356494a0
Hochman, A. (1997). Programmed cell death in prokaryotes. Crit. Rev. Microbiol. 23, 207–214. doi:10.3109/10408419709115136
Huh, J. R., Guo, M., and Hay, B. A. (2004). Compensatory proliferation induced by cell death in the Drosophila wing disc requires activity of the apical cell death caspase Dronc in a nonapoptotic role. Curr. Biol. 14, 1262–1266. doi:10.1016/j.cub.2004.06.015
Jiang, S., Zhou, Z., Sun, Y., Zhang, T., and Sun, L. (2020). Coral gasdermin triggers pyroptosis. Sci. Immunol. 5, eabd2591. doi:10.1126/sciimmunol.abd2591
Kang, Y., Neuman, S. D., and Bashirullah, A. (2017). Tango7 regulates cortical activity of caspases during reaper-triggered changes in tissue elasticity. Nat. Commun. 8, 603. doi:10.1038/s41467-017-00693-3
Kesavardhana, S., Malireddi, R. K. S., and Kanneganti, T. D. (2020). Caspases in cell death, inflammation, and pyroptosis. Annu. Rev. Immunol. 38, 567–595. doi:10.1146/annurev-immunol-073119-095439
Kihara, A., Kabeya, Y., Ohsumi, Y., and Yoshimori, T. (2001). Beclin-phosphatidylinositol 3-kinase complex functions at the trans-Golgi network. EMBO Rep. 2, 330–5.
Koonin, E. V., and Aravind, L. (2002). Origin and evolution of eukaryotic apoptosis: The bacterial connection. Cell Death Differ. 9, 394–404. doi:10.1038/sj.cdd.4400991
Kostura, M. J., Tocci, M. J., Limjuco, G., Chin, J., Cameron, P., Hillman, A. G., et al. (1989). Identification of a monocyte specific pre-interleukin 1 beta convertase activity. Proc. Natl. Acad. Sci. U. S. A. 86, 5227–5231. doi:10.1073/pnas.86.14.5227
Kroemer, G., and Levine, B. (2008). Autophagic cell death: The story of a misnomer. Nat. Rev. Mol. Cell Biol. 9, 1004–1010. doi:10.1038/nrm2529
Lee, H., and Lee, D. G. (2019). Programmed cell death in bacterial community: Mechanisms of action, causes and consequences. J. Microbiol. Biotechnol. 29, 1014–1021. doi:10.4014/jmb.1904.04017
Li, H., Zhu, H., Xu, C. J., and Yuan, J. (1998). Cleavage of BID by caspase 8 mediates the mitochondrial damage in the Fas pathway of apoptosis. Cell 94, 491–501. doi:10.1016/s0092-8674(00)81590-1
Li, X., Zhong, C. Q., Wu, R., Xu, X., Yang, Z. H., Cai, S., et al. (2021). RIP1-dependent linear and nonlinear recruitments of caspase-8 and RIP3 respectively to necrosome specify distinct cell death outcomes. Protein Cell 12, 858–876. doi:10.1007/s13238-020-00810-x
Locato, V., and De Gara, L. (2018). Programmed cell death in plants: An overview. Methods Mol. Biol. 1743, 1–8.
Lockshin, R. A., and Williams, C. M. (1965). Programmed cell death--I. Cytology of degeneration in the intersegmental muscles of the pernyi silkmoth. J. Insect Physiol. 11, 123–133. doi:10.1016/0022-1910(65)90099-5
Lyons, N. A., and Kolter, R. (2015). On the evolution of bacterial multicellularity. Curr. Opin. Microbiol. 24, 21–28. doi:10.1016/j.mib.2014.12.007
Margulis, L. (1975). Symbiotic theory of the origin of eukaryotic organelles; criteria for proof. Symp. Soc. Exp. Biol. 29, 21–38.
Martin, D. N., and Baehrecke, E. H. (2004). Caspases function in autophagic programmed cell death in Drosophila. Development 131, 275–284. doi:10.1242/dev.00933
Mesquita, A., Cardenal-Muñoz, E., Dominguez, E., Muñoz-Braceras, S., Nuñez-Corcuera, B., Phillips, B. A., et al. (2017). Autophagy in Dictyostelium: Mechanisms, regulation and disease in a simple biomedical model. Autophagy 13, 24–40. doi:10.1080/15548627.2016.1226737
Milligan, C. E., and Schwartz, L. M. (1997). Programmed cell death during animal development. Br. Med. Bull. 53, 570–590. doi:10.1093/oxfordjournals.bmb.a011631
Minina, E. A., Coll, N. S., Tuominen, H., and Bozhkov, P. V. (2017). Metacaspases versus caspases in development and cell fate regulation. Cell Death Differ. 24, 1314–1325. doi:10.1038/cdd.2017.18
Mumbauer, S., Pascual, J., Kolotuev, I., and Hamaratoglu, F. (2019). Ferritin heavy chain protects the developing wing from reactive oxygen species and ferroptosis. PLoS Genet. 15, e1008396. doi:10.1371/journal.pgen.1008396
Mur, L. A., Kenton, P., Lloyd, A. J., Ougham, H., and Prats, E. (2008). The hypersensitive response; the centenary is upon us but how much do we know? J. Exp. Bot. 59, 501–520. doi:10.1093/jxb/erm239
Nakajima, Y. I., and Kuranaga, E. (2017). Caspase-dependent non-apoptotic processes in development. Cell Death Differ. 24, 1422–1430. doi:10.1038/cdd.2017.36
Nakano, H., Murai, S., and Moriwaki, K. (2022). Regulation of the release of damage-associated molecular patterns from necroptotic cells. Biochem. J. 479, 677–685. doi:10.1042/BCJ20210604
Napoletano, F., Gibert, B., Yacobi-Sharon, K., Vincent, S., Favrot, C., Mehlen, P., et al. (2017). p53-dependent programmed necrosis controls germ cell homeostasis during spermatogenesis. PLoS Genet. 13, e1007024. doi:10.1371/journal.pgen.1007024
Nirmala, J. G., and Lopus, M. (2020). Cell death mechanisms in eukaryotes. Cell Biol. Toxicol. 36, 145–164. doi:10.1007/s10565-019-09496-2
Ploumi, C., Papandreou, M. E., and Tavernarakis, N. (2022). The complex interplay between autophagy and cell death pathways. Biochem. J. 479, 75–90. doi:10.1042/BCJ20210450
Rajan, V., Melong, N., Hing Wong, W., King, B., Tong, S. R., Mahajan, N., et al. (2020). Humanized zebrafish enhance human hematopoietic stem cell survival and promote acute myeloid leukemia clonal diversity. Haematologica 105, 2391–2399. doi:10.3324/haematol.2019.223040
Restifo, L. L., and White, K. (1992). Mutations in a steroid hormone-regulated gene disrupt the metamorphosis of internal tissues in Drosophila: Salivary glands, muscle, and gut. Rouxs Arch. Dev. Biol. 201, 221–234. doi:10.1007/BF00188753
Savitsky, M., Solis, G. P., Kryuchkov, M., and Katanaev, V. L. (2020). Humanization of Drosophila gαo to model GNAO1 paediatric encephalopathies. Biomedicines 8, 395. doi:10.3390/biomedicines8100395
Schwartz, L. M. (2021). Autophagic cell death during development - ancient and mysterious. Front. Cell Dev. Biol. 9, 656370. doi:10.3389/fcell.2021.656370
Schweichel, J. U., and Merker, H. J. (1973). The morphology of various types of cell death in prenatal tissues. Teratology 7, 253–266. doi:10.1002/tera.1420070306
Sueldo, D. J., and van der Hoorn, R. A. L. (2017). Plant life needs cell death, but does plant cell death need Cys proteases? FEBS J. 284, 1577–1585. doi:10.1111/febs.14034
Sulston, J. E., and Horvitz, H. R. (1977). Post-embryonic cell lineages of the nematode Caenorhabditis elegans. Dev. Biol. 82, 110–156. doi:10.1016/0012-1606(77)90158-0
Sun, G., Ding, X. A., Argaw, Y., Guo, X., and Montell, D. J. (2020). Akt1 and dCIZ1 promote cell survival from apoptotic caspase activation during regeneration and oncogenic overgrowth. Nat. Commun. 11, 5726. doi:10.1038/s41467-020-19068-2
Takacs-Vellai, K., Vellai, T., Puoti, A., Passannante, M., Wicky, C., Streit, A., et al. (2005). Inactivation of the autophagy gene bec-1 triggers apoptotic cell death in C. elegans. Curr. Biol. 15, 1513–1517. doi:10.1016/j.cub.2005.07.035
Tang, D., Kang, R., Berghe, T. V., Vandenabeele, P., and Kroemer, G. (2019). The molecular machinery of regulated cell death. Cell Res. 29, 347–364. doi:10.1038/s41422-019-0164-5
Tanouchi, Y., Lee, A. J., Meredith, H., and You, L. (2013). Programmed cell death in bacteria and implications for antibiotic therapy. Trends Microbiol. 21, 265–270. doi:10.1016/j.tim.2013.04.001
Tsuji, J., Thomson, T., Chan, E., Brown, C. K., Oppenheimer, J., Bigelow, C., et al. (2020). High-resolution analysis of differential gene expression during skeletal muscle atrophy and programmed cell death. Physiol. Genomics 52 (10), 492–511. doi:10.1152/physiolgenomics.00047.2020
Tsvetkov, P., Coy, S., Petrova, B., Dreishpoon, M., Verma, A., Abdusamad, M., et al. (2022). Copper induces cell death by targeting lipoylated TCA cycle proteins. Science 375, 1254–1261. doi:10.1126/science.abf0529
Vasudevan, D., and Ryoo, H. D. (2015). Regulation of cell death by IAPs and their antagonists. Curr. Top. Dev. Biol. 114, 185–208. doi:10.1016/bs.ctdb.2015.07.026
Vaux, D. L., Weissman, I. L., and Kim, S. K. (1992). Prevention of programmed cell death in Caenorhabditis elegans by human bcl-2. Science 258, 1955–1957. doi:10.1126/science.1470921
Wang, J., Hu, M., Wang, J., Qi, J., Han, Z., Wang, G., et al. (2019). Reconstitution and structure of a plant NLR resistosome conferring immunity. Science 364 (6435), eaav5870. doi:10.1126/science.aav5870
Williams, D. W., Kondo, S., Krzyzanowska, A., Hiromi, Y., and Truman, J. W. (2006). Local caspase activity directs engulfment of dendrites during pruning. Nat. Neurosci. 9, 1234–1236. doi:10.1038/nn1774
Wirawan, E., Vande Walle, L., Kersse, K., CorneliS, S., Claerhout, S., Vanoverberghe, I., et al. (2010). Caspase-mediated cleavage of Beclin-1 inactivates Beclin-1-induced autophagy and enhances apoptosis by promoting the release of proapoptotic factors from mitochondria. Cell Death Dis. 1, e18. doi:10.1038/cddis.2009.16
Yalonetskaya, A., Mondragon, A. A., Elguero, J., and McCall, K. (2018). I spy in the developing fly a multitude of ways to die. J. Dev. Biol. 22, 26. doi:10.3390/jdb6040026
Yuan, J., and Horvitz, H. R. (1992). The Caenorhabditis elegans cell death gene ced-4 encodes a novel protein and is expressed during the period of extensive programmed cell death. Development 116, 309–320. doi:10.1242/dev.116.2.309
Yuan, J., Shaham, S., Ledoux, S., Ellis, H. M., and Horvitz, H. R. (1993). The C. elegans cell death gene ced-3 encodes a protein similar to mammalian interleukin-1 beta-converting enzyme. Cell 75, 641–652. doi:10.1016/0092-8674(93)90485-9
Yuan, J. Y., and Horvitz, H. R. (1990). The Caenorhabditis elegans genes ced-3 and ced-4 act cell autonomously to cause programmed cell death. Dev. Biol. 138, 33–41. doi:10.1016/0012-1606(90)90174-h
Zhang, H., and Baehrecke, E. H. (2015). Eaten alive: Novel insights into autophagy from multicellular model systems. Trends Cell Biol. 25, 376–387. doi:10.1016/j.tcb.2015.03.001
Zhang, X., Dowling, J. P., and Zhang, J. (2019). RIPK1 can mediate apoptosis in addition to necroptosis during embryonic development. Cell Death Dis. 10, 245. doi:10.1038/s41419-019-1490-8
Zhu, B., Mak, J. C. H., Morris, A. P., Marson, A. G., Barclay, J. W., Sills, G. J., et al. (2020). Functional analysis of epilepsy-associated variants in STXBP1/Munc18-1 using humanized Caenorhabditis elegans. Epilepsia 61, 810–821. doi:10.1111/epi.16464
Zhu, Y., Zhao, L., Liu, L., Gao, P., Tian, W., Wang, X., et al. (2010). Beclin 1 cleavage by caspase-3 inactivates autophagy and promotes apoptosis. Protein Cell 1, 468–477. doi:10.1007/s13238-010-0048-4
Keywords: programmed cell death, apoptosis, invertebrate, autophagy, plant, bacteria, C. elegans
Citation: Schwartz LM (2022) Model systems in cell death-grand challenge. Front. Cell Death 1:1087903. doi: 10.3389/fceld.2022.1087903
Received: 02 November 2022; Accepted: 17 November 2022;
Published: 05 December 2022.
Edited by:
Zhenggang Liu, National Institutes of Health (NIH), United StatesCopyright © 2022 Schwartz. This is an open-access article distributed under the terms of the Creative Commons Attribution License (CC BY). The use, distribution or reproduction in other forums is permitted, provided the original author(s) and the copyright owner(s) are credited and that the original publication in this journal is cited, in accordance with accepted academic practice. No use, distribution or reproduction is permitted which does not comply with these terms.
*Correspondence: Lawrence M. Schwartz, TE1TQHVtYXNzLmVkdQ==
Disclaimer: All claims expressed in this article are solely those of the authors and do not necessarily represent those of their affiliated organizations, or those of the publisher, the editors and the reviewers. Any product that may be evaluated in this article or claim that may be made by its manufacturer is not guaranteed or endorsed by the publisher.
Research integrity at Frontiers
Learn more about the work of our research integrity team to safeguard the quality of each article we publish.