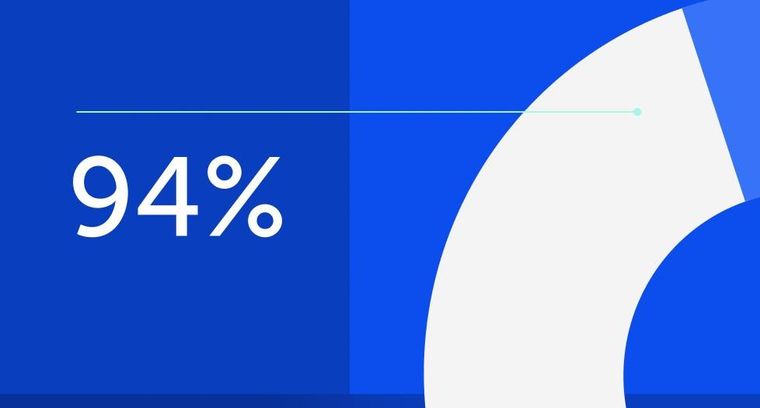
94% of researchers rate our articles as excellent or good
Learn more about the work of our research integrity team to safeguard the quality of each article we publish.
Find out more
REVIEW article
Front. Cell Dev. Biol., 11 April 2025
Sec. Cell Death and Survival
Volume 13 - 2025 | https://doi.org/10.3389/fcell.2025.1570131
This article is part of the Research TopicRole and Mechanism of Regulated Cell Death in Musculoskeletal Development, Homeostasis, and DiseasesView all 5 articles
Cuproptosis, a recently identified form of copper-dependent cell death, arises from intracellular copper dyshomeostasis. As an essential trace element, copper plays a critical role in bioenergetic metabolism, redox regulation, and synaptic transmission. However, excessive copper exerts cytotoxic effects through multiple pathways, including increased reactive oxygen species (ROS) production, apoptotic cascade activation, necrotic membrane rupture, inflammatory responses, and mitochondrial dysfunction. Distinct from other cell death mechanisms, cuproptosis is characterized by copper ion binding to acetylated mitochondrial respiratory chain proteins, leading to pathogenic protein aggregation, iron-sulfur cluster depletion, and cellular collapse. Emerging evidence underscores aberrant copper accumulation and resultant proteotoxic stress as pivotal contributors to the pathogenesis of multiple musculoskeletal pathologies, including osteoporosis, osteoarthritis, sarcopenia, osteosarcoma, intervertebral disc degeneration, spinal cord injury, and biofilm-associated orthopedic infections. Understanding the spatiotemporal regulation of cuproptosis may provide novel opportunities for advancing diagnostic and therapeutic approaches in orthopedic medicine. This review synthesizes current insights into the molecular mechanisms of cuproptosis, its pathogenic role in musculoskeletal diseases, and the potential for biomarker-driven therapeutic interventions.
Copper is an indispensable trace metal element in biological systems, extensively involved in critical physiological processes including energy metabolism, antioxidant defense, and connective tissue formation (Chen L. et al., 2022; Tian et al., 2023). As a cofactor for metalloenzymes such as cytochrome C oxidase (COX), superoxide dismutase (SOD), and lysyl oxidase (LOX), copper plays pivotal roles in maintaining mitochondrial respiratory chain function, scavenging free radicals, and promoting collagen cross-linking, which are essential for skeletal development, joint stability, and muscular contractility (Chen H. et al., 2024; Lutsenko et al., 2025). However, the strong redox activity of copper endows it with dose-dependent dual effects at the cellular level: under physiological concentrations, copper supports normal cellular functions through precise homeostatic regulation networks, whereas excessive accumulation triggers irreversible cellular damage via reactive oxygen species (ROS) generation and direct proteotoxicity (Tang et al., 2024; Tsvetkov et al., 2022; Wang J. et al., 2024; Chen J. et al., 2024). Chronic copper metabolism disorders have been well-documented in association with Wilson’s disease, neurodegenerative disorders, and various malignancies (Gromadzka et al., 2024; Gromadzka et al., 2020; Wang A. et al., 2023).
Recent advances in understanding metal-dependent apoptosis have revealed cuproptosis as a novel form of programmed cell death (Evans, 1973; Wu H. et al., 2024). In 2022, Tsvetkov et al. systematically characterized cuproptosis, revealing its core mechanism involving direct binding of excess copper ions to lipoylated proteins in the tricarboxylic acid (TCA) cycle, leading to mitochondrial protein aggregation and metabolic collapse (Tsvetkov et al., 2022). The initiation of cuproptosis strictly depends on spatiotemporal dynamics of intracellular copper concentrations (Doguer et al., 2018). Distinct from apoptosis, necroptosis, or ferroptosis, cuproptosis exhibits unique molecular signatures: caspase-independent execution, absence of lipid peroxide accumulation, coupled with mitochondrial swelling, profound dysregulation of lipoic acid metabolism pathways, and systemic failure of energy metabolism hubs (Tong et al., 2022; Tsvetkov et al., 2022) (Table 1). These features suggest cuproptosis may represent a specialized defense mechanism against metabolic imbalance, though its precise regulatory logic in tissue homeostasis and disease pathogenesis requires further elucidation.
The musculoskeletal system, as the structural foundation of locomotor function, critically relies on functional coordination among osteoblasts, osteoclasts, chondrocytes, and myocytes (Luo et al., 2024). Clinical studies reveal elevated synovial fluid copper levels in osteoarthritis patients, positively correlated with articular cartilage degradation (Yazar et al., 2005), while osteoporosis patients exhibit serum copper fluctuations closely linked to bone density dynamics (Wei et al., 2022). Additionally, muscle degenerative disease models demonstrate aberrant subcellular localization of copper transporters (Jackson, 2009). While traditional paradigms attribute these phenomena to copper-mediated oxidative damage or inflammatory cascades, emerging evidence implicates cuproptosis in disease pathogenesis (Li C. et al., 2023; Tsvetkov et al., 2022). Experimental studies demonstrate that the copper ionophore elesclomol specifically induces osteoblastic cuproptosis through mitochondrial lipoylated protein aggregation and impaired bone matrix mineralization (Muthurangan et al., 2023). Conversely, copper chelators effectively suppress synovial cell death and inflammatory cytokine release in rheumatoid arthritis models (Zhao et al., 2022). These findings collectively point to an underappreciated pathological axis: copper homeostasis imbalance may drive musculoskeletal disorders through cell type-specific cuproptosis activation.
To clarify the roles of copper homeostasis and cuproptosis in musculoskeletal disorders, this review comprehensively outlines copper metabolism and regulatory mechanisms, examines the molecular basis of cuproptosis, and systematically analyzes its research progress across these diseases. These findings aim to provide novel insights for developing precision therapeutic strategies targeting musculoskeletal pathologies.
Copper, an essential trace element in humans, plays critical roles in numerous biological processes including reactive oxygen species (ROS) detoxification, cellular energy metabolism, iron absorption, and signal transduction (Tang et al., 2024). It is obtained primarily from dietary sources such as shellfish, meat, seeds, nuts, lentils, and leafy greens (Burkhead et al., 2009). As an indispensable micronutrient for maintaining cellular homeostasis, copper is involved in cell proliferation, angiogenesis, and metastasis, necessitating strict regulatory mechanisms to prevent toxicity (Han et al., 2024; Tsvetkov et al., 2022). The recommended daily copper intake for adults ranges from 0.8 to 2.4 mg to maintain systemic copper homeostasis (Chen W. et al., 2022). Maintaining a dynamic equilibrium of copper levels is essential for normal cellular function and metabolism (Locatelli and Farina, 2025), while disruptions in copper homeostasis can lead to severe pathological conditions (An et al., 2022; Burkhead et al., 2009).
Copper deficiency impairs the activity and functionality of cuproenzymes, resulting in anemia, increased vascular and skeletal fragility, cerebral atrophy, impaired endocrine function, and neurological deficits. Additionally, it has been linked to depigmentation disorders such as vitiligo. Conversely, copper overload is cytotoxic. Chronic excess intake interferes with iron and zinc absorption, potentially leading to deficiencies in these elements. In severe cases, copper toxicity can result in hepatic injury and systemic dysfunction (Han et al., 2024).
Mammalian copper homeostasis is maintained through a tightly regulated network of proteins, including ceruloplasmin in plasma, copper transporter 1 (CTR1), the cytosolic copper chaperone ATOX1, copper efflux proteins ATP7A/B, and mitochondrial copper chaperones such as CCS, SCO1, SCO2, COX11, and COX17. Together, these components orchestrate copper absorption, transport, storage, and excretion (Tsvetkov et al., 2022). Dietary copper absorption primarily occurs in the small intestine, a process dependent on CTR1 located on the apical membrane of intestinal epithelial cells (Kardos et al., 2018). The six-transmembrane epithelial antigen of the prostate (STEAP) and duodenal cytochrome B (Dcytb) are involved in reducing divalent copper ions (Cu2+) to monovalent copper ions (Cu+) for transport via CTR1 (Teschke and Eickhoff, 2024; Wapnir, 1998), while copper efflux across the basolateral membrane requires ATP7A. As a critical copper transporter, CTR1 expression is regulated by copper levels: its expression is suppressed under high copper conditions and enhanced under low copper conditions, indicating a negative feedback mechanism governing intracellular copper absorption and utilization (Tsvetkov et al., 2022; Zhu et al., 2024).
Once absorbed, copper is released into the portal circulation via ATP7A and bound to metalloproteins such as albumin (Qiu et al., 2024). When copper is transported to the liver, hepatocytes uptake the majority of copper via CTR1 and store it intracellularly with the involvement of metallothioneins (MT1, MT2). Excess copper is secreted into bile via the ATOX1/ATP7B/ceruloplasmin pathway in vesicular form, ultimately excreted through bile secretion into the digestive tract—a major route for endogenous copper elimination (Tsvetkov et al., 2022) (Figure 1). If peripheral copper levels are insufficient to maintain homeostasis, ATP7B exports stored hepatic copper into systemic circulation, where it is bound to ceruloplasmin for transport to specific tissues or organs for utilization (An et al., 2022; Qiu et al., 2024). Additionally, low-molecular-weight copper ligands such as aspartate, histidine, and cysteine are implicated in peripheral copper absorption and utilization (Viktorinova, 2017).
Figure 1. Schematic of systemic copper metabolism. The body absorbs copper mostly through the small intestine, where it is then transported by blood to the liver for excretion into the bile. STEAP: six-transmembrane epithelial antigen of the prostate; SLC31A1(CTR1): copper transporter 1; ATP7A/B: ATPase copper transporting alpha/beta.
In peripheral tissues, copper ions are either sequestered by compounds like metallothioneins or targeted for utilization by chaperones such as ATOX1, COX17, and CCS (Liu et al., 2023). Copper homeostasis is maintained through a dynamic balance between absorption and excretion—high copper intake triggers reduced absorption and increased excretion, whereas low intake leads to enhanced absorption and reduced excretion. Furthermore, copper serves as a cofactor for key metabolic enzymes involved in critical physiological processes, including mitochondrial respiration, redox reactions, antioxidant metabolism, and the absorption and utilization of other biomolecules. These enzymes play indispensable roles in cellular respiration, endocrine function, and the development and maintenance of the central nervous system (Fukai et al., 2018; Leuci et al., 2025; Tang et al., 2024).
Cellular copper homeostasis is the foundation for macroregulatory control of copper homeostasis. Dysregulation of copper balance disrupts the intracellular environment, leading to cellular damage and death. In multicellular organisms, copper metabolism operates at both systemic and cellular levels. Copper acts as an electron acceptor or donor, participating extensively in diverse biochemical reactions. The liver, which contains the highest copper concentration in humans, serves as the primary regulatory organ for copper metabolism (Wang A. et al., 2023; Zhou et al., 2023). To prevent copper toxicity and maintain intracellular equilibrium, copper levels are tightly controlled by a sophisticated network of copper-dependent proteins, including cuproenzymes, copper chaperones, and membrane transporters.
Copper transporter 1 (CTR1) and STEAP collaboratively mediate copper uptake into cells, while copper chaperones—such as the copper chaperone for superoxide dismutase (CCS), antioxidant-1 (ATOX1), and the copper chaperone for cytochrome c oxidase (COX17)—orchestrate intracellular copper trafficking (Festa and Thiele, 2011; Gromadzka et al., 2020; Zhang et al., 2024). CCS delivers copper to superoxide dismutase 1 (SOD1), which scavenges reactive oxygen species (ROS) and maintains redox balance (Zhang et al., 2024). ATOX1 binds Cu+ and transfers it to ATP7A and ATP7B in the trans-Golgi network, facilitating cuproenzyme biosynthesis (Wu J. et al., 2023). Under conditions of copper excess, ATP7A and ATP7B export surplus copper to the plasma membrane (Feng et al., 2024). COX17 targets Cu+ to the mitochondrial intermembrane space, where it is incorporated into cytochrome c oxidase (CCO) via SCO1, supporting cellular respiration (Roy and Lutsenko, 2024).
Additionally, copper-binding molecules such as glutathione and metallothionein 1/2 (MT1/MT2) neutralize excess copper ions, ensuring cellular stability (Jia et al., 2024). When needed, Cu+ is oxidized to Cu2+by ROS. Due to its low affinity for MT, Cu2+ is released into the cytosol to participate in metabolic regulation. Intracellular Cu+ is exported via ATP7A through endosomal/Golgi pathways or directly expelled by ATP7B. During copper overload, ATP7B facilitates copper sequestration in hepatocytes, directing it toward biliary excretion to maintain systemic homeostasis. This intricate interplay among cuproenzymes, chaperones, and transporters ensures that cellular copper levels remain within physiological ranges, preventing both deficiency and toxicity (Figure 2).
Figure 2. Schematic of cellular copper metabolism. Biological processes in which copper ions are involved in cells. STEAP: six-transmembrane epithelial antigen of the prostate; CTR1:copper transporter 1; CCS: copper chaperone for superoxide dismutase; SOD1: superoxide dismutase 1; COX17: cytochrome C oxidase copper chaperone 17; COX11: cytochrome C oxidase copper chaperone 11; CCO: cytochrome C oxidase; SCO1/2: synthesis of cytochrome C oxidase 1/2; ATOX1: antioxidant 1; ATP7A/B: ATPase copper transporting alpha/beta; TGN:trans-Golgi network.
The discovery of copper-induced cell death dates back to the early 1980s (Halliwell and Gutteridge, 1984). Studies indicate that elevated copper levels promote reactive oxygen species (ROS) generation, triggering oxidative stress and DNA damage, ultimately leading to cell death (Gaetke and Chow, 2003). These findings spurred further investigation into the molecular mechanisms of copper-induced cell death and its implications for human health. Conflicting evidence suggests that, beyond ROS accumulation, excess copper may also induce cell death via apoptosis or caspase-independent pathways (Nagai et al., 2012; Tardito et al., 2011).
In March 2022, Tsvetkov et al. (2022) published groundbreaking research elucidating a novel mechanism of copper-induced cell death, termed “cuprotosis”. This distinct form of cell death is driven by excessive copper ions. The authors demonstrated that treatment with the copper ionophore elesclomol (ES) induced cell death, which could only be rescued by copper chelators, whereas inhibitors targeting apoptosis, necroptosis, oxidative stress, ROS-induced cell death, or ferroptosis showed no protective effect. These findings confirm that cuprotosis operates through unique mechanisms and signaling pathways distinct from other known cell death modalities. Crucially, cuprotosis is regulated by mitochondrial respiration. Studies reveal that cells reliant on mitochondrial respiration exhibit nearly 1,000-fold higher sensitivity to copper ionophores compared to glycolysis-dependent cells (Tsvetkov et al., 2022), underscoring the pivotal role of mitochondrial respiration in cuprotosis. This highlights a strong link between cuprotosis and the tricarboxylic acid (TCA) cycle. During cuprotosis, intracellular copper binds to lipoylated components of the TCA cycle, inducing aggregation of copper-bound lipoylated mitochondrial proteins. This disrupts the TCA cycle, impairing cellular energy production. The aggregation of these proteins, coupled with subsequent depletion of Fe-S cluster proteins—essential cofactors for electron transport and enzymatic reactions (Lill and Freibert, 2020)—triggers proteotoxic stress and culminates in cell death (Figure 2).
To elucidate how ES-induced cuprotosis targets the TCA cycle, Tsvetkov et al. conducted a genome-wide CRISPR-Cas9 loss-of-function screen, identifying ten cuprotosis-related genes. These include seven positive regulators: ferredoxin 1 (FDX1), dihydrolipoamide S-acetyltransferase (DLAT), lipoic acid synthase (LIAS), dihydrolipoamide dehydrogenase (DLD), lipoyltransferase 1 (LIPT1), pyruvate dehydrogenase E1 subunit alpha 1 (PDHA1), and pyruvate dehydrogenase E1 subunit beta (PDHB); and three negative regulators: metal regulatory transcription factor 1 (MTF1), glutaminase (GLS), and cyclin-dependent kinase inhibitor 2A (CDKN2A) (Zhou et al., 2024) (Table 2). FDX1 and protein lipoylation are central to ES-induced cuprotosis (Scheiber et al., 2014). FDX1 encodes a reductase that reduces Cu2+ to the more toxic Cu+. Protein lipoylation, a highly conserved lysine post-translational modification, regulates protein function by attaching a lipoic acid moiety to lysine residues. This modification occurs exclusively in four multi-enzyme complexes, such as the pyruvate dehydrogenase (PDH) complex (of which DLAT is a component) (Magistrato et al., 2019). Tsvetkov et al. (2022) further demonstrated that FDX1 expression correlates strongly with lipoylated proteins (Lip-DLAT) and that FDX1 knockout abolishes protein lipoylation, identifying FDX1 as an upstream regulator of this process (Wang W. et al., 2023; Zhou, et al., 2023). Subsequent experiments revealed that Cu+directly binds to Lip-DLAT, inducing its oligomerization. Concurrently, ES-treated cells exhibited reduced Fe-S cluster protein levels. The researchers also found that CuCl2 mimics ES by inducing cuprotosis, depleting glutathione (GSH), and upregulating CTR1 (SLC31A1), thereby potentiating CuCl-induced cell death (Figure 3) (Wang J. et al., 2024). In summary, cuprotosis is driven by excessive intracellular copper binding to lipoylated TCA cycle proteins, leading to oligomerization, Fe-S cluster protein loss, proteotoxic stress, and eventual cell death.
Figure 3. Schematic diagram of the molecular mechanism of cuproptosis. Cu+ is transported into the cell via SLC31A1 and out of the cell via ATP7A/B. Cu2+ is transported into the cell by copper ionophores, including ES and DSF. Cu2+ is reduced to Cu+ by FDX1. On the one hand, excessive Cu+ binds to fatty acid acylated mitochondrial proteins, causing the oligomerization of fatty acid acylated proteins, leading to proteotoxic stress, blocking TCA (tricarboxylic acid) cycle, and causing cuproptosis. On the other hand, excessive Cu+ can reduce iron-sulfur cluster proteins, resulting in abnormal electron transport chain and causing cuproptosis. FDX1: ferredoxin 1; DLAT: dihydrolipoic acid transacetylase; LIAS: Lipoic acid synthetase.
Cuprotosis involves multiple signaling pathways and interactions with critical proteins. Copper induces mitochondrial dysfunction, activating intrinsic apoptotic pathways. Key mitochondrial proteins, including cytochrome C, apoptosis-inducing factor (AIF), and Bcl-2 family members, have been implicated in copper-induced cell death (Nam et al., 2018). Copper ions also activate stress- and apoptosis-related pathways such as MAPK (mitogen-activated protein kinase), p38 MAPK, and JNK (c-Jun N-terminal kinase) (Wittung-Stafshede, 2022). Studies (Hatori and Lutsenko, 2016; Valko et al., 2005) suggest that copper enhances ROS production, which subsequently triggers cell death via NF-κB and p53 pathways. Fogh et al. (2014) further demonstrated that protein kinase C (PKC) modulates cytoskeletal dynamics and cell adhesion during copper-induced cellular alterations.
Osteoarthritis (OA), a prevalent chronic degenerative joint disease, imposes significant physical and economic burdens on aging populations. Its pathological mechanisms involve chronic inflammation predominantly affecting the synovium, articular cartilage, and subchondral bone, leading to cartilage degradation, subchondral sclerosis, and osteophyte formation (Chen et al., 2017). OA most commonly affects the knees and hips, with risk factors including age, obesity, and severe joint injury (Li F. et al., 2022). Clinically, OA management remains challenging, with joint replacement serving as the primary intervention for end-stage disease. However, increasing rates of arthroplasty, coupled with complications such as prosthesis failure, infection, and periprosthetic fractures, underscore the urgent need for early therapeutic strategies to delay disease progression (Hunter et al., 2020).
Copper metabolism exhibits a complex relationship with OA pathogenesis (Yazar et al., 2005). Under physiological conditions, copper ions act as essential cofactors for enzymes critical to cartilage homeostasis, including lysyl oxidase (LOX), superoxide dismutase (SOD), cytochrome C oxidase (Cox) (Yang J. et al., 2023). Moderate Cu2+ levels promote cartilage regeneration and bone-cartilage interface repair, mitigating tissue damage (Zhang et al., 2024). Conversely, excessive copper induces ROS generation, lipid peroxidation, and inflammatory cascades, exacerbating cartilage and bone degradation. Cuproptosis—copper-dependent cell death—is driven by intracellular copper accumulation, where Cu+ binds to lipoylated components of the tricarboxylic acid (TCA) cycle, causing protein aggregation, TCA cycle disruption, proteotoxic stress, and ultimately cell death (Zhou et al., 2021). Notably, copper accumulation has been observed in severely degraded cartilage, correlating with accelerated joint damage (Guan et al., 2023).
Current research on cuproptosis in OA focuses on copper-related genes (CRGs) as potential biomarkers and therapeutic targets (Wang W. et al., 2023). A predictive model for OA risk and progression has been established using four CRGs (DBT, DLST, FDX1, LIPT1), where FDX1 and LIPT1 are upregulated in OA, while DBT and DLST are elevated in healthy controls (Nong et al., 2023). CRGs influence OA through multiple pathways (Guan et al., 2024; He et al., 2024; Yu et al., 2024): (a). Mitochondrial Dysfunction: Impaired mitochondrial activity in joint cells (e.g., chondrocytes, synoviocytes) during OA exacerbates inflammation, apoptosis, and metabolic dysregulation. CRGs, closely linked to mitochondrial function, may amplify these pathologies when dysregulated. (b). Copper Homeostasis Disruption: CRGs modulate intracellular copper balance, impacting chondrocyte survival and mitochondrial integrity. (c). Immune Regulation: CRGs correlate with immune cell infiltration and inflammatory responses in OA synovium, suggesting roles in disease progression. (d). Protein Aggregation: CRGs regulate the expression of mitochondrial proteins (e.g., lipoylated, copper-sensitive proteins), influencing chondrocyte susceptibility to cuproptosis. (e). Metabolic Pathway Interference: CRG-associated pathways, such as the TCA cycle, are disrupted in OA, leading to metabolite accumulation and chondrocyte dysfunction.
In addition, synovitis, a hallmark of OA, drives cartilage destruction. Five CRGs (FDX1, LIPT1, PDHA1, PDHB, CDKN2A) are markedly upregulated in OA synovial tissues (Nong et al., 2023). Single-cell RNA sequencing reveals dynamic shifts in synovial cell populations during OA progression, with these CRGs emerging as candidate biomarkers or therapeutic targets for synovitis (Chang et al., 2023).
For therapeutic strategies, nanoparticle-based approaches show promise in cartilage tissue engineering. Li et al. (2024) constructed copper-based bioactive nanoparticles with Cuprorivaite as the carrier and demonstrated its potential in protecting cartilage in OA progression by inhibiting inflammation, oxidative stress, and copper-induced cell death. Zhu et al. (2022) developed a multifunctional thermoresponsive gel (HPP@Cu gel), which efficiently scavenged free radicals through copper nanodots (Cu NDs) and induced polarization of macrophages from M1 to M2 phenotypes at the inflammatory site, significantly reducing OA-induced cartilage degeneration and production of inflammatory factors. Additionally, curcumin, a natural compound, has been utilized as a copper shuttle protein to kill cancer cells intracellularly via copper transport mechanisms (Zhang et al., 2016). However, researchers have focused more on copper’s catalytic roles in various applications, with fewer studies investigating the therapeutic effects at different copper levels. Therefore, this field still requires further exploration and development.
Osteoporosis (OP) is a systemic skeletal disorder characterized by reduced bone mass and microarchitectural deterioration, leading to increased bone fragility and fracture susceptibility (Lane et al., 2000). Its pathogenesis is multifactorial, involving complex interactions of hormonal, cellular, and molecular mechanisms (Wang et al., 2009). Bone homeostasis in healthy adults is maintained by a dynamic balance between osteoblast-mediated bone formation and osteoclast-mediated bone resorption. In OP, this equilibrium is disrupted, often favoring excessive bone resorption over formation, resulting in progressive bone loss (Clynes et al., 2020). Key regulators of bone metabolism include hormones such as parathyroid hormone, estrogen, testosterone, and vitamin D, as well as cytokines like interleukins (ILs), tumor necrosis factors (TNFs), and osteoprotegerin (OPG) (Fischer and Haffner-Luntzer, 2022; Liu B. et al., 2022). Additionally, excessive reactive oxygen species (ROS) production damages bone cells via DNA lesions, protein oxidation, and cellular dysfunction (Iantomasi et al., 2023). Moreover, genetic predisposition, particularly polymorphisms affecting bone mineral density (BMD), and environmental factors such as nutritional deficiencies (e.g., calcium, vitamin D) further contribute to OP risk (Wang et al., 2009).
Emerging evidence demonstrates that the physiological hypoxic niche within bone confers cytoprotective effects against ROS-mediated oxidative damage, with the osseous tissue and medullary cavity inherently maintaining a state of natural hypoxia (Riegger et al., 2023). Both osteoblasts and osteoclasts—central orchestrators of skeletal homeostasis—possess intrinsic oxygen-sensing capacity through HIF-1ɑ signaling (Chen W. et al., 2022). This oxygen-sensitive pathway dichotomously regulates bone remodeling dynamics by coordinating anabolic/catabolic balance and modulating bone turnover rates (Knowles, 2015). However, chronic hypoxic stimulation paradoxically upregulates nuclear factor-κB ligand (RANKL) expression via HIF-1α/NF-κB transcriptional synergy, thereby disrupting homeostatic control over osteoclast differentiation (Zhang C. et al., 2023). Osteoporosis pathogenesis fundamentally represents a pathological cascade initiated by marrow microenvironmental destabilization and dysregulated intercellular crosstalk.
Cuproptosis may also alter the bone marrow microenvironment, impairing the osteogenic differentiation and promoting adipogenic differentiation of bone marrow mesenchymal stem cells (BMSCs) (Li D. et al., 2023). Mechanistically, cuproptosis-associated mitochondrial metabolic disturbances—particularly FDX1-mediated proteotoxic stress from impaired copper ion buffering—may drive disease progression through aberrant activation of apoptosis-executing pathways (Chen H. et al., 2024). Furthermore, reactive oxygen species (ROS) generated during cuproptosis intensify intracellular oxidative stress, directly damaging osteocytes while simultaneously triggering inflammatory responses that recruit immune cells to bone tissue, exacerbating bone destruction (Liu et al., 2024). This imbalance could reduce bone formation and increase fat deposition, ultimately lowering bone mineral density. Moreover, cuproptosis may further disrupt the expression of genes and proteins critical to bone metabolism, such as the osteoblast-specific transcription factors Runx2 and Osterix, as well as the RANKL/OPG system—a key molecular regulator of bone formation and resorption (Wang et al., 2024b). Additionally, cuproptosis may modify the composition of miRNAs within extracellular vesicles (EVs), which are internalized by neighboring bone cells, subsequently influencing their function (Sun et al., 2024). For instance, specific miRNAs may suppress osteoblast differentiation or enhance osteoclast activity (Li Q. C. et al., 2022). These mechanisms collectively contribute to osteoporosis pathogenesis.
Notably, certain osteoporosis therapeutics, such as bisphosphonates, may exert their effects partially through modulation of copper metabolism and cuproptosis pathways (Kanumakala et al., 2002). Building on the interplay between cuproptosis and osteoporosis, researchers are exploring novel therapeutic strategies to harness cuproptosis mechanisms for OP treatment. These include regulating copper metabolism, targeting cuproptosis-related genes and utilizing EVs to deliver miRNAs (Sun et al., 2024). The overarching goal is to fine-tune cuproptosis to preserve bone cell health and function, thereby advancing both therapeutic and preventive approaches for osteoporosis.
The development of sarcopenia, characterized by age-related loss of skeletal muscle mass and strength, has been demonstrated to result from mitochondrial dysfunction, elevated reactive oxygen species (ROS) production, and denervation (Cruz-Jentoft et al., 2019). Skeletal muscle, a highly copper-dependent and metabolically active tissue, serves as a major reservoir for copper ions (Lutsenko, 2021). Copper is indispensable not only for the initial formation of skeletal muscle—particularly myoblast proliferation and differentiation—but also for post-differentiation copper redistribution within myocytes, mitochondrial copper trafficking, and the metabolic maintenance of myofibers (Cobine et al., 2021; McCann et al., 2022). As a copper-demanding organ, skeletal muscle exhibits abundant and dynamic copper metabolism. The widespread expression of CTR1 and ATP7A/B in skeletal muscle cells indicates that intracellular copper homeostasis adheres to universal physiological principles of copper regulation, governed by the balance between copper uptake and efflux (Jin et al., 2021).
Current studies reveal that copper overload may activate multiple cell death pathways, including apoptosis, pyroptosis, ferroptosis, and cuproptosis, while promoting α-synuclein aggregation (Li et al., 2019; Yuan and Larsson, 2023). These processes lead to direct degradation of myocellular components, degeneration of the neuromuscular junction (NMJ), and subsequent skeletal muscle atrophy. These metabolic programs involve distinct regulatory mechanisms: copper overload-induced myocyte apoptosis is primarily mediated by ROS, p53, and mitochondrial dynamin-related protein dysregulation (Chen et al., 2021; Nishikawa et al., 2021). Additionally, ROS facilitate copper overload-triggered pyroptosis by upregulating tumor necrosis factor-α (TNF-α) expression and activating caspases, a process potentially requiring copper-induced inflammatory cooperation (Jin et al., 2022; Wu Z. et al., 2023).
To date, four cuproptosis-related genes—PDHA1, DLAT, PDHB, and NDUFC1—have been identified as diagnostic biomarkers for age-related skeletal muscle atrophy (Zhu et al., 2023). Further research demonstrates that PDHB and NDUFC1 act as novel molecular targets to counteract myofiber senescence. Metformin hydrochloride, a therapeutic agent for muscle atrophy, requires NDUFC1 mediation for its regulatory effects (Jiang J. et al., 2023; Zhu et al., 2023). Moreover, immunologically relevant cuproptosis-associated genes are closely linked to musculoskeletal aging progression and may serve as technical options for early diagnosis of muscle atrophy, underscoring the potential role of copper overload-induced cuproptosis in skeletal muscle senescence (Lin et al., 2022). However, the precise mechanisms by which copper overload triggers cuproptosis in aged skeletal muscle fibers remain elusive, warranting further investigation.
Intervertebral Disc Degeneration (IVDD) is a predominant contributor to low back pain, pathologically characterized by progressive structural and functional deterioration of intervertebral discs (Dowdell et al., 2017). Although surgical and pharmacological interventions have achieved partial advancements in recent years, IVDD remains highly prevalent with limited therapeutic efficacy for reversal or complete resolution (Smith et al., 2011; Wu et al., 2020). Consequently, deciphering the molecular underpinnings of disc degeneration and developing superior treatment modalities represent urgent priorities. Substantial evidence indicates that IVDD pathogenesis involves dysregulated cellular processes including aberrant cell death, oxidative stress, inflammatory/immune dysregulation, and extracellular matrix (ECM) metabolic imbalance (He et al., 2023a; Xia et al., 2024). Emerging studies now identify copper homeostasis as a critical modulator in IVDD progression (He et al., 2023b; Staszkiewicz et al., 2023). Dysfunctional copper metabolism elevates free copper ion concentrations within the disc microenvironment, which exacerbates oxidative stress via Fenton reactions, directly inflicts cellular damage, and disrupts ECM homeostasis (Chen H. et al., 2024) Furthermore, copper ions act as co-activators of inflammatory signaling pathways (NF-κB), upregulating matrix metalloproteinases (MMPs) and pro-inflammatory cytokines such as IL-1β, TNF-α, thereby establishing a self-amplifying feedback loop of “copper accumulation → oxidative damage → inflammatory amplification → ECM degradation” (Chen J. et al., 2024). Additionally, mitochondria-dependent cuproptosis accelerates IVDD by promoting nucleus pulposus cell apoptosis through the FDX1-mediated protein lipoylation axis. In a recent study, Chen X. et al. (2024) investigated cuproptosis in IDD using in vitro and in vivo models, elucidating the interplay between oxidative stress and copper sensitivity in nucleus pulposus cells (NPCs). Their findings revealed that oxidative stress activates the SP1-CTR1 axis, increasing intracellular copper influx and synergistically upregulating FDX1 expression. This drives abnormal aggregation of TCA cycle-related proteins and cuproptosis, underscoring the central role of cuproptosis in IDD progression and proposing the SP1/FDX1 pathway as a novel therapeutic target. Zhang C. et al. (2023) identified eight cuproptosis-related prognostic regulators and two molecular subtypes, constructing a nomogram model to accurately predict IDD risk, thereby offering potential biomarkers and immunotherapeutic strategies for IDD. Additionally, a recent biomarker study on intervertebral disc degeneration (IVDD) revealed significant upregulation of miR-15a-5p in degenerative discs, demonstrating its multifaceted regulatory roles in core IVDD pathomechanisms. Bioinformatic analyses identified its potential targets across multiple IVDD-associated pathways, including copper-related genes (CRGs), ferroptosis regulators, oxidative stress mediators, and immune-related factors. These findings highlight the miR-15a-5p-mRNA regulatory axis as a promising therapeutic target for precision intervention in IVDD (Li C. et al., 2023).
Osteosarcoma (OS), a malignant mesenchymal tumor predominantly affecting children and adolescents, is often diagnosed at advanced stages with distant metastases due to asymptomatic early progression (Biazzo and De Paolis, 2016). Long-term survival rates remain suboptimal (Wittig et al., 2002). Limited therapeutic advances over the past three decades, particularly for multidrug-resistant, relapsed, or metastatic patients, underscore the urgent need for prognostic biomarkers and novel therapeutic targets.
Current research on cuproptosis in OS focuses on CRGs and RNA fragments (Bian et al., 2023; Feng et al., 2023; Gao et al., 2025; Hu et al., 2023; Huang et al., 2023; Jiang et al., 2022; Jiang M. et al., 2023; Liu C. et al., 2022; Ni et al., 2023; Wang et al., 2023c; Yang et al., 2022). Dysregulated CRGs effectively predict OS diagnosis, prognosis, tumor immune microenvironment (TME), and immunotherapy response (Han et al., 2024). Yang et al. (2022) established a prognostic model using four CRGs (LIAS, LIPT1, BCL2L1, PDK1), demonstrating strong associations between CRG signatures and TME, positioning CRGs as reliable prognostic indicators for OS. Jiang J. et al. (2023) identified PDHA1 and CDKN2A as optimal diagnostic CRGs for OS. Survival analysis of 10 CRGs revealed that high CDKN2A and FDX1 expression correlates with poorer survival, while elevated LIAS, LIPT1, and PDHA1 predicts better outcomes. Hu et al. (2023) found that the high levels of FDX1 in patients with poor prognosis for OS show a strong correlation, suggesting that it may serve as a promising prognostic indicator. Similarly, another study demonstrated that elevated expression of FDX1 promotes osteosarcoma (OS) cell migration, thereby exacerbating tumor malignancy Feng et al. (2023). Consequently, targeted inhibition of FDX1 represents a promising therapeutic strategy for OS. Moreover, long non-coding RNAs (lncRNAs) as key regulators of tumor pathogenesis, invasion, and progression (Bian et al., 2023). Cuproptosis-related lncRNAs (CRLs) may serve as prognostic biomarkers, potentially modulating OS aggressiveness and outcomes via copper metabolism and cell death pathways (Gao et al., 2025; Huang et al., 2023; Jiang et al., 2022; Liu P. et al., 2022; Ni et al., 2023; Wang et al., 2023d; Xie et al., 2023). CRLs are linked to cancer-associated fibroblasts (CAFs) in OS, offering insights for survival prediction and therapy. Targeting lncRNAs involved in copper metabolism—through small molecules, siRNA, or antisense oligonucleotides (ASOs)—may control cuproptosis and tumor progression. Multi-algorithm analyses of TME and immune status across risk groups reveal higher stromal scores and CAF infiltration in low-risk patients, contrasting with upregulated immune cell subsets in high-risk cohorts, providing a framework for clinical decision-making and personalized therapies (Wang A. et al., 2023; Xie et al., 2023).
In terms of treatment strategies, copper alloys exhibit exceptional hemocompatibility, antibacterial activity, and osteogenic potential during post-resection recovery (Elborolosy et al., 2023). Cu2+enhance chemodynamic therapy (CDT) efficacy by depleting glutathione (GSH) via redox reactions (Ye et al., 2024). Copper complexes (25–100 μM) selectively inhibit OS cell viability, with significantly higher toxicity toward tumor osteoblasts than normal cells (Leon et al., 2014). Other copper-based agents similarly demonstrate OS-specific cytotoxicity with minimal osteoblast damage (Balsa et al., 2023). Chemotherapy remains indispensable in OS treatment, with cisplatin, paclitaxel, and etoposide showing distinct IC50 values between risk groups (Huang et al., 2024). Cuproptosis signatures may predict immunotherapy response and chemosensitivity. Copper chelators can also play a certain role in tumor therapy, using targeted selective action on cancer cells, inducing apoptosis of cancer cells through oxidative stress and other mechanisms (Ismail et al., 2022). At the same time, copper chelating agent can also inhibit the proliferation of vascular endothelial cells through the interaction mechanism with copper, thus playing a role in inhibiting tumor growth (Gupte and Mumper, 2009).
Targeted therapies leveraging the high intrinsic copper content of OS cells are under exploration. Copper metallocompounds may exacerbate intracellular copper overload to induce cytotoxicity, offering novel antitumor avenues. Promising agents include milciclib malate, HMN-214, GSK461364, abemaciclib, palbociclib, and PF-477736, validated as potential OS-targeted drugs (Han et al., 2024).
Rheumatoid arthritis (RA), an autoimmune disease, primarily manifests as symmetric, progressive polyarthritis affecting multiple small joints (Turk et al., 2023). Persistent synovial inflammation leads to cartilage, bone, and periarticular soft tissue damage, resulting in joint deformities and functional impairment (Radu and Bungau, 2021; Smolen et al., 2016). Elevated serum copper levels in active RA patients correlate positively with erythrocyte sedimentation rate (ESR) and morning stiffness, and inversely with hemoglobin levels, suggesting serum copper as a biomarker for RA disease activity (Xin et al., 2015). During RA progression, cuproptosis exacerbates chronic inflammation by catalyzing ROS production via the tricarboxylic acid (TCA) cycle, inducing oxidative stress that damages vasculature and connective tissues (Smolen et al., 2016). copper-related genes further regulate immune cell metabolism, driving pro-inflammatory cytokine release, autoantigen-antibody reactions, and immune complex deposition, perpetuating joint destruction (Xu et al., 2025).
Given excessive copper levels in RA, Zhao et al. (2022) demonstrated that copper induces cell death by binding to lipoylated TCA cycle components, promoting lipoylated protein aggregation and proteotoxic stress. This mechanism may affect diverse RA-associated cells, including fibroblast-like synoviocytes, effector T cells, and macrophages, contributing to inflammation, pannus formation, and bone erosion. RA patients exhibit a twofold higher incidence of osteoporosis compared to healthy populations, with studies linking copper dysregulation to osteoporotic development (Liu Y. et al., 2022). Hypoxic bone environments and glycolytic energy metabolism suppress cuproptosis, potentially enhancing survival and proliferation of osteoblasts, osteoclasts, effector T cells, and macrophages, thereby mediating osteoporosis (Tsvetkov et al., 2022). Zhou et al. (2023) integrated bioinformatics and experimental validation to conclude that cuproptosis-related genes regulate RA by modulating inflammatory factor secretion and macrophage metabolism. Ten cuproptosis-associated genes are implicated in RA processes: PDHA1 regulates glycolysis and inflammation; microRNAs (miRNAs) primarily target PDHB; GLS1 and LIPT1 modulate glutamine metabolism; DLAT governs mitochondrial function and TCA cycle activity; FDX1influences fatty acid oxidation and steroidogenesis; MTF1 and LIAS regulate copper homeostasis; HIF-1 and CDKN2A mediate cellular senescence (Zhao et al., 2022). Wang et al. found seven CRGs (ATP7A, FDX1, LIAS, LIPT1, DLD, MTF1, CDKN2A) were significantly differentially expressed in RA patients, and six of them (except MTF1) were upregulated in RA (Wang et al., 2023c). Jiang et al. also found high expression of CRGS such as DLST, DLD, and ATP7A in RA (Jiang X. et al., 2023). These genes can be used as new biomarkers of RA, and targeting the cuproptosis pathway or regulating immune infiltration may become a new strategy for RA treatment.
Additionally, copper promotes ferroptosis by inducing autophagic degradation of glutathione peroxidase 4 (GPX4) (Xue et al., 2023). Downregulation of the GSH-GPX4 axis, cystine/glutamate antiporter, and nuclear factor erythroid 2-related factor 2 (Nrf2) triggers synovial cell ferroptosis, exacerbating RA synovitis (Datta et al., 2014).
Spinal cord injury (SCI), a severe central nervous system trauma, causes permanent loss of motor, sensory, and autonomic functions below the injury level, with limited recovery (Anjum et al., 2020). Its pathology involves primary injury and progressive secondary injury cascades. Secondary injury impairs mitochondrial homeostasis, triggering calcium overload, excitotoxicity, and oxidative stress, which exacerbate neuronal damage (McDonald and Sadowsky, 2002). Mitochondrial dysfunction observed in these processes accelerates neuronal death and inhibits regeneration (Fan et al., 2018) Programmed cell death (PCD) secondary to SCI—including apoptosis, necroptosis, pyroptosis, ferroptosis, cuproptosis, and autophagy—is a critical barrier to functional recovery (Song et al., 2024). While physiological PCD may serve protective roles, excessive PCD exacerbates SCI by damaging surrounding neural tissue. Gene expression analyses reveal stage-specific PCD patterns post-SCI, suggesting therapeutic strategies to inhibit PCD pathways and induce autophagy. Interventions targeting hub genes associated with these pathways may also offer therapeutic potential (He et al., 2023a).
Dihydrolipoamide dehydrogenase (DLD), a regulator of copper toxicity, is significantly upregulated after acute SCI (ASCI) and correlates with disease severity (Li K. et al., 2023). DLD exacerbates ASCI by promoting copper toxicity, which disrupts the immune microenvironment, enhances polarization of peripheral M2 macrophages, and induces systemic immunosuppression. Post-ASCI, DLD facilitates copper binding to lipid components of the TCA cycle in peripheral blood, driving cuproptosis. This cascade disrupts immune homeostasis, alters macrophage polarization, aggravates SCI-induced immunosuppression syndrome (SCI-IDS), and worsens ASCI outcomes (Liu et al., 2022). Further analyses confirm elevated M2 macrophage infiltration in high-grade ASCI patients, correlating positively with ASIA impairment scale scores and DLD expression. Thus, DLD overexpression post-ASCI may drive adverse prognosis via macrophage polarization, positioning DLD as a therapeutic target (Li Y. et al., 2023). Mao et al. identified Mpeg1 as a hub gene related to cuproptosis, which may alleviate spinal cord tissue injury by regulating the infiltration of immune cells, such as M2 macrophages, and inhibiting inflammatory responses (Mao et al., 2025).
Mitochondrial dysfunction post-SCI amplifies injury cascades, making cuproptosis inhibition a potential therapeutic strategy. Preserving mitochondrial integrity and mitigating copper-induced damage could enhance neuronal survival and functional recovery.
Osteomyelitis, a common orthopedic infection, is typically caused by bacteria, Pseudomonas aeruginosa, or fungi, with Staphylococcus aureus (S. aureus) being the most prevalent pathogen (Lew and Waldvogel, 2004). Current diagnostic challenges include the nonspecificity of inflammatory biomarkers and the limited sensitivity of magnetic resonance imaging (MRI) in early-stage disease (Lew and Waldvogel, 1997). Thus, developing effective early diagnostic methods remains an urgent priority.
The pathogenesis and therapeutic strategies for osteomyelitis involve immune responses, with cuproptosis potentially playing a role. S. aureus biofilms evade host receptor recognition, largely mediated by Staphylococcal Protein A (SPA). SPA interacts with osteoclasts and osteoblasts, driving inflammatory cascades (Claro et al., 2011; Mendoza Bertelli et al., 2016). S. aureus also induces osteoblast death and bone destruction. Mendelsohn et al. (2023) observed mitochondrial dysfunction in chronic osteomyelitis patients, including ROS accumulation characteristic of cuproptosis. Furthermore, inhibitors targeting the programmed cell death protein 1/programmed death-ligand 1 (PD-1/PD-L1) pathway reduce mitochondrial autophagy in macrophages during S. aureus-induced osteomyelitis, thereby attenuating inflammation (Li C. et al., 2023). Inducing cuproptosis may represent a novel antibiotic-free therapeutic approach for methicillin-resistant S. aureus (MRSA)-associated osteomyelitis. Shi et al. (2024) showed that comparative analysis of CRGs and immune microenvironments between S. aureus-infected osteomyelitis patients and healthy controls identified three signature M2R-CRGs: SLC31A1, DLD, and MTF1. Among these, SLC31A1 likely modulates the immunomicroenvironment by regulating M2 macrophage polarization, MTF1 exerts anti-inflammatory effects via M2 macrophage activation, and DLD promotes inflammatory responses and accelerates osteoblast death by suppressing M2 macrophage activity (Duarte et al., 2021). A diagnostic model based on these CRGs enables early prediction of osteomyelitis risk.
Therapeutically, targeted delivery of copper ions to infection sites to trigger bacterial cuproptosis represents a promising strategy. Cu+ enhances the efficiency of iron-based Fenton reactions by facilitating Fe3+ reduction to Fe2+ (Koo et al., 2022). Concurrently, Cu+ catalyzes the generation of highly toxic hydroxyl radicals (·OH) through Fenton-like reactions (Ma et al., 2023). Li et al. (2025) developed a bone marrow mesenchymal stem cell (BMSC) membrane-engineered nanovesicle (CFE@CM) with dual bone-targeting and cuproptosis-inducing capabilities. Upon reaching osteomyelitic lesions, CFE@CM disassembles, releasing free Cu+, Fe2+, ELC, and H2O2, synergistically activating Fenton reactions and cuproptosis mechanisms. Qiu et al. have developed a nanoheterojunction catalytic reactor consisting of copper ferrite (CuFe2O4) and molybdenum disulfide (MoS2) quantum dots (CFO@MoS2) to induce the cuproptosis of bacteria using ultrasonically catalyzed binding of copper ions (Qiu et al., 2025) (Table 3).
According to the description of the association between the above diseases and cuproptosis, treatment methods include the use of nanomaterials or biomaterials to deliver copper ions to the corresponding areas for treatment, as well as to combat ROS and oxidative stress generated by cuproptosis. In addition to those, there are two other different therapeutic strategies for musculoskeletal diseases by cuproptosis, which include two aspects: the regulation of copper homeostasis by copper chelators and copper ionophoresis, and the gene and epigenetic regulation of CRGs.
Copper chelators reduce the adverse effects of copper on cells mainly by binding free copper ions in the body and promoting their excretion, while copper ionophores regulate the death of cells mainly by affecting the intake and expulsion of copper ions (Zhao et al., 2022).
The use of copper chelators may be an acceptable treatment for rheumatoid arthritis, and high copper levels in RA are an important factor in the development of the condition (Xu et al., 2025). At present, D-penicillamine and ethylenediamine tetraacetic acid have been used in the treatment of RA, and certain results have been achieved (Bamonti et al., 2011; Kumar et al., 2021), But there are still some side effects (Kumar et al., 2021). Therefore, in the future, how to better use copper chelating agent to regulate the copper level in patients with bone and joint diseases and minimize the occurrence of side effects is a possible research direction. Copper chelators can also play a certain role in tumor therapy, using targeted selective action on cancer cells, inducing apoptosis of cancer cells through oxidative stress and other mechanisms (Ismail et al., 2022). At the same time, copper chelating agent can also inhibit the proliferation of vascular endothelial cells through the interaction mechanism with copper, thus playing a role in inhibiting tumor growth (Gupte and Mumper, 2009). Conventional cancer therapies such as chemotherapy and radiotherapy suffer from limitations including poor targeting specificity and significant adverse effects, resulting in suboptimal therapeutic outcomes (Yang W. M. et al., 2023). Although immunotherapy has emerged as an advanced approach in recent years, it still faces challenges of narrow anticancer spectrum and complex treatment-related complications (Peng et al., 2022; Winer et al., 2018). Currently, therapeutic strategies targeting cuproptosis mechanisms show particular promise for osteosarcoma treatment. Cui et al. developed copper-depleting nanoparticles that induce intracellular copper depletion and subsequent apoptosis in cancer cells (Li Y. et al., 2022).
Copper ionophore can increase the intracellular copper ion level and exert anticancer activity. Ionophore exerts toxic effects on cancer cells mainly by increasing ROS production and inhibiting proteasome (Denoyer et al., 2016; Xiao et al., 2010). Wu et al. designed an ES-Cu compound capable of releasing substantial copper ions within tumor cells to trigger cuproptosis (Wu J. et al., 2024). Compared to conventional approaches, these novel therapies demonstrate multiple advantages. For instance, chemotherapeutic agents utilizing cuproptosis mechanisms exhibit enhanced chemotherapy sensitivity (Wen et al., 2023), while combination therapies with emerging modalities like phototherapy and sonotherapy achieve superior targeting precision and deeper tissue penetration (Chen et al., 2023; Ning et al., 2023).
Editing and regulating copper-death related genes is also a method with potential application value to use the copper-death mechanism to treat musculoskeletal diseases. It can enhance mitochondrial respiration and promote cuproptosis of abnormal proliferation cells by activating positive regulatory genes (FDX1, PDHA1). Inhibition of negatively regulated genes (MTF1, GLS) blocked hypoxic adaptation and glycolytic resistance to cuproptosis. For example, the use of histone deacetylase inhibitors (such as FK228) in the treatment of RA can upregulate CDKN2A and induce FLS senescence and apoptosis. Use of GLS inhibitors such as CB-839 to reduce Th17 differentiation and FLS proliferation (Zhao et al., 2022). Epigenetic regulation includes DNA methylation, histone modification (Knott and Doudna, 2018). The pharmacological management of osteoporosis primarily involves bone resorption inhibitors (e.g., bisphosphonates), bone formation promoters (e.g., parathyroid hormone), and bone metabolism regulators (e.g., calcium supplements). Current research indicates that miRNAs encapsulated in extracellular vesicles (EVs) may influence osteoblast function through modulation of cuproptosis-related genes. For instance, miR-21-5p has been demonstrated to target PDHA1, thereby suppressing mitochondrial oxidative phosphorylation (Zhuang et al., 2021) Additionally, oxidative stress has been shown to upregulate miR-183-5p levels, which subsequently inhibits mesenchymal stem cell proliferation and induces cellular senescence (Davis et al., 2017). Emerging evidence suggests that targeted delivery of specific miRNAs via EVs to rebalance bone metabolism represents a promising therapeutic strategy for osteoporosis treatment (Sun et al., 2024). Lin et al. (2025) demonstrated that by regulating CYFIP1 to bind RNMT and promoting m7G methylation of target mRNA, abnormal AURKAIP1 leads to abnormal mitochondrial translation, and upregulation of FDX1 triggers cuproptosis. It provides a new target for the treatment of osteosarcoma.
Numerous musculoskeletal disorders are closely associated with copper homeostasis and copper-induced cell death. The copper oxidation process primarily disrupts TCA cycle and may play a regulatory role in the progression of various bone and joint diseases. Cuproptosis exhibits dual attributes of being both a “core driving factor” and a “secondary outcome” in musculoskeletal diseases, depending on the disease type, pathological stage, and microenvironment context. In diseases such as osteoarthritis (OA) and osteosarcoma (OS), dysregulation of copper metabolism directly triggers cell death and inflammation via cuproptosis, serving as a key link in the pathological mechanism. In diseases like spinal cord injury (SCI) and osteomyelitis, cuproptosis is more of a manifestation of metabolic disorders following tissue damage and is regulated by other pathological processes (such as hypoxia and infection). It is necessary to further distinguish the causal timing of cuproptosis, combine multi-omics analysis and clinical intervention trials, and clarify its dominant position in specific diseases to guide the development of precision treatment strategies. Currently, many researchers are focusing on investigating the relationship between copper-induced cell death and major pathological conditions. Although certain clinical treatments for bone and joint diseases exist, most remain insufficiently effective. Targeting cuproptosis provides novel avenues for developing therapeutic strategies. Through systematic investigation of common musculoskeletal disorders and the correlation analysis between disease manifestations and specific genes involved in cuproptosis, it can be inferred that these genes likely play critical roles in the pathogenesis of musculoskeletal diseases. The discovery of cuproptosis has also deepened our understanding of these disorders and their underlying molecular mechanisms. Furthermore, cuproptosis holds potential value for screening therapeutic agents targeting these diseases.
Future research directions may emphasize strategies leveraging the strong chelating properties of copper chelators to reduce intracellular copper levels or inhibit copper transporters, thereby suppressing cuproptosis—an approach that offers innovative possibilities for disease intervention and treatment. Conversely, utilizing copper ionophores to enhance intracellular copper accumulation may exhibit therapeutic potential for osteosarcoma management. However, in-depth investigations into copper oxidases and their associated genes require extensive experimental exploration and present significant challenges. Despite these obstacles, this field remains highly promising with substantial potential for groundbreaking advancements in future research.
ZX: Writing–original draft, Investigation. HM: Writing–original draft, Data curation. HW: Methodology, Writing–review and editing. XY: Formal analysis, Writing–original draft. JR: Writing–review and editing. WZ: Writing–review and editing. AX: Supervision, Formal analysis, Writing–review and editing. LL: Funding acquisition, Supervision, Writing–review and editing.
The author(s) declare that financial support was received for the research and/or publication of this article. This work was supported by the National Science Foundation of Hubei Province (No. 2024AFB206), Key Project of Hubei Association of Higher Education (NO. 2023XA040), the Comprehensive Reform Project for Quality Construction of Undergraduate Education in Wuhan University (NO. 2024ZG223) and the Fundamental Research Funds for the Central Universities (NO. 2042023kf0027).
The authors declare that the research was conducted in the absence of any commercial or financial relationships that could be construed as a potential conflict of interest.
The authors declare that no Generative AI was used in the creation of this manuscript.
All claims expressed in this article are solely those of the authors and do not necessarily represent those of their affiliated organizations, or those of the publisher, the editors and the reviewers. Any product that may be evaluated in this article, or claim that may be made by its manufacturer, is not guaranteed or endorsed by the publisher.
An, Y., Li, S., Huang, X., Chen, X., Shan, H., and Zhang, M. (2022). The role of copper homeostasis in brain disease. Int. J. Mol. Sci. 23 (22), 13850. doi:10.3390/ijms232213850
Anjum, A., Yazid, M. D., Fauzi Daud, M., Idris, J., Ng, A. M. H., Selvi Naicker, A., et al. (2020). Spinal cord injury: Pathophysiology, Multimolecular interactions, and underlying recovery mechanisms. Int. J. Mol. Sci. 21 (20), 7533. doi:10.3390/ijms21207533
Balsa, L. M., Solerno, L. M., Rodriguez, M. R., Parajon-Costa, B. S., Gonzalez-Baro, A. C., Alonso, D. F., et al. (2023). Cu(II)-acylhydrazone complex, a potent and selective antitumor agent against human osteosarcoma: mechanism of action studies over in vitro and in vivo models. Chem. Biol. Interact. 384, 110685. doi:10.1016/j.cbi.2023.110685
Bamonti, F., Fulgenzi, A., Novembrino, C., and Ferrero, M. E. (2011). Metal chelation therapy in rheumathoid arthritis: a case report. Successful management of rheumathoid arthritis by metal chelation therapy. Biometals 24 (6), 1093–1098. doi:10.1007/s10534-011-9467-9
Bian, C., Zheng, Z., Su, J., Chang, S., Yu, H., Bao, J., et al. (2023). Copper homeostasis and cuproptosis in tumor pathogenesis and therapeutic strategies. Front. Pharmacol. 14, 1271613. doi:10.3389/fphar.2023.1271613
Biazzo, A., and De Paolis, M. (2016). Multidisciplinary approach to osteosarcoma. Acta Orthop. Belg 82 (4), 690–698.
Burkhead, J. L., Gogolin Reynolds, K. A., Abdel-Ghany, S. E., Cohu, C. M., and Pilon, M. (2009). Copper homeostasis. New Phytol. 182 (4), 799–816. doi:10.1111/j.1469-8137.2009.02846.x
Chang, B., Hu, Z., Chen, L., Jin, Z., and Yang, Y. (2023). Development and validation of cuproptosis-related genes in synovitis during osteoarthritis progress. Front. Immunol. 14, 1090596. doi:10.3389/fimmu.2023.1090596
Chen, C. H., Chou, Y. T., Yang, Y. W., and Lo, K. Y. (2021). High-dose copper activates p53-independent apoptosis through the induction of nucleolar stress in human cell lines. Apoptosis 26 (11-12), 612–627. doi:10.1007/s10495-021-01692-y
Chen, D., Shen, J., Zhao, W., Wang, T., Han, L., Hamilton, J. L., et al. (2017). Osteoarthritis: toward a comprehensive understanding of pathological mechanism. Bone Res. 5, 16044. doi:10.1038/boneres.2016.44
Chen, H., Tang, T., Xue, C., Liu, X., Xi, Z., Xie, L., et al. (2024a). Exploration and breakthrough in the mode of intervertebral disc cell death may lead to significant advances in treatments for intervertebral disc degeneration. J. Orthop. Surg. Res. 19 (1), 825. doi:10.1186/s13018-024-05280-z
Chen, J., Sun, Q., Wang, Y., and Yin, W. (2024b). Revealing the key role of cuproptosis in osteoporosis via the bioinformatic analysis and experimental validation of cuproptosis-related genes. Mamm. Genome 35 (3), 414–431. doi:10.1007/s00335-024-10049-0
Chen, K., Zhou, A., Zhou, X., Liu, Y., Xu, Y., and Ning, X. (2023). An Intelligent cell-derived Nanorobot Bridges synergistic crosstalk between Sonodynamic therapy and cuproptosis to promote cancer treatment. Nano Lett. 23 (7), 3038–3047. doi:10.1021/acs.nanolett.3c00434
Chen, L., Min, J., and Wang, F. (2022a). Copper homeostasis and cuproptosis in health and disease. Signal Transduct. Target Ther. 7 (1), 378. doi:10.1038/s41392-022-01229-y
Chen, W., Wu, P., Yu, F., Luo, G., Qing, L., and Tang, J. (2022b). HIF-1α regulates bone homeostasis and angiogenesis, participating in the occurrence of bone metabolic diseases. Cells 11 (22), 3552. doi:10.3390/cells11223552
Chen, X., Li, K., Xiao, Y., Wu, W., Lin, H., Qing, X., et al. (2024c). SP1/CTR1-mediated oxidative stress-induced cuproptosis in intervertebral disc degeneration. Biofactors 50 (5), 1009–1023. doi:10.1002/biof.2052
Chen, Z., Hu, D., Wu, D., Song, C., Sun, J., and Liu, W. (2024d). Association between serum copper levels and muscle mass: results from NHANES 2011-2016. Environ. Sci. Pollut. Res. Int. 31 (5), 6847–6856. doi:10.1007/s11356-023-31599-x
Claro, T., Widaa, A., O’Seaghdha, M., Miajlovic, H., Foster, T. J., O’Brien, F. J., et al. (2011). Staphylococcus aureus protein A binds to osteoblasts and triggers signals that weaken bone in osteomyelitis. PLoS One 6 (4), e18748. doi:10.1371/journal.pone.0018748
Clynes, M. A., Harvey, N. C., Curtis, E. M., Fuggle, N. R., Dennison, E. M., and Cooper, C. (2020). The epidemiology of osteoporosis. Br. Med. Bull. 133 (1), 105–117. doi:10.1093/bmb/ldaa005
Cobine, P. A., Moore, S. A., and Leary, S. C. (2021). Getting out what you put in: copper in mitochondria and its impacts on human disease. Biochim. Biophys. Acta Mol. Cell Res. 1868 (1), 118867. doi:10.1016/j.bbamcr.2020.118867
Cruz-Jentoft, A. J., Bahat, G., Bauer, J., Boirie, Y., Bruyere, O., Cederholm, T., et al. (2019). Sarcopenia: revised European consensus on definition and diagnosis. Age Ageing 48 (1), 16–31. doi:10.1093/ageing/afy169
Datta, S., Kundu, S., Ghosh, P., De, S., Ghosh, A., and Chatterjee, M. (2014). Correlation of oxidant status with oxidative tissue damage in patients with rheumatoid arthritis. Clin. Rheumatol. 33 (11), 1557–1564. doi:10.1007/s10067-014-2597-z
Davis, C., Dukes, A., Drewry, M., Helwa, I., Johnson, M. H., Isales, C. M., et al. (2017). MicroRNA-183-5p increases with age in bone-derived extracellular vesicles, Suppresses bone marrow stromal (stem) cell proliferation, and induces stem cell senescence. Tissue Eng. Part A 23 (21-22), 1231–1240. doi:10.1089/ten.TEA.2016.0525
Denoyer, D., Pearson, H. B., Clatworthy, S. A., Smith, Z. M., Francis, P. S., Llanos, R. M., et al. (2016). Copper as a target for prostate cancer therapeutics: copper-ionophore pharmacology and altering systemic copper distribution. Oncotarget 7 (24), 37064–37080. doi:10.18632/oncotarget.9245
Doguer, C., Ha, J. H., and Collins, J. F. (2018). Intersection of iron and copper metabolism in the mammalian intestine and liver. Compr. Physiol. 8 (4), 1433–1461. doi:10.1002/cphy.c170045
Dowdell, J., Erwin, M., Choma, T., Vaccaro, A., Iatridis, J., and Cho, S. K. (2017). Intervertebral Disk degeneration and repair. Neurosurgery 80 (3S), S46–S54. doi:10.1093/neuros/nyw078
Duarte, I. F., Caio, J., Moedas, M. F., Rodrigues, L. A., Leandro, A. P., Rivera, I. A., et al. (2021). Dihydrolipoamide dehydrogenase, pyruvate oxidation, and acetylation-dependent mechanisms intersecting drug iatrogenesis. Cell Mol. Life Sci. 78 (23), 7451–7468. doi:10.1007/s00018-021-03996-3
Elborolosy, S. A., Hussein, L. A., Mahran, H., Ammar, H. R., Sivasankaran, S., Abd El-Ghani, S. F., et al. (2023). Evaluation of the biocompatibility, antibacterial and anticancer effects of a novel nano-structured Fe-Mn-based biodegradable alloys in-vitro study. Heliyon 9 (11), e20932. doi:10.1016/j.heliyon.2023.e20932
Evans, G. W. (1973). Copper homeostasis in the mammalian system. Physiol. Rev. 53 (3), 535–570. doi:10.1152/physrev.1973.53.3.535
Fan, B., Wei, Z., Yao, X., Shi, G., Cheng, X., Zhou, X., et al. (2018). Microenvironment imbalance of spinal cord injury. Cell Transpl. 27 (6), 853–866. doi:10.1177/0963689718755778
Feng, J., Wang, J., Xu, Y., Lu, F., Zhang, J., Han, X., et al. (2023). Construction and validation of a novel cuproptosis-mitochondrion prognostic model related with tumor immunity in osteosarcoma. PLoS One 18 (7), e0288180. doi:10.1371/journal.pone.0288180
Feng, Y., Yang, Z., Wang, J., and Zhao, H. (2024). Cuproptosis: unveiling a new frontier in cancer biology and therapeutics. Cell Commun. Signal 22 (1), 249. doi:10.1186/s12964-024-01625-7
Festa, R. A., and Thiele, D. J. (2011). Copper: an essential metal in biology. Curr. Biol. 21 (21), R877–R883. doi:10.1016/j.cub.2011.09.040
Fischer, V., and Haffner-Luntzer, M. (2022). Interaction between bone and immune cells: implications for postmenopausal osteoporosis. Semin. Cell Dev. Biol. 123, 14–21. doi:10.1016/j.semcdb.2021.05.014
Fogh, B. S., Multhaupt, H. A., and Couchman, J. R. (2014). Protein kinase C, focal adhesions and the regulation of cell migration. J. Histochem Cytochem 62 (3), 172–184. doi:10.1369/0022155413517701
Fukai, T., Ushio-Fukai, M., and Kaplan, J. H. (2018). Copper transporters and copper chaperones: roles in cardiovascular physiology and disease. Am. J. Physiol. Cell Physiol. 315 (2), C186–C201. doi:10.1152/ajpcell.00132.2018
Gaetke, L. M., and Chow, C. K. (2003). Copper toxicity, oxidative stress, and antioxidant nutrients. Toxicology 189 (1-2), 147–163. doi:10.1016/s0300-483x(03)00159-8
Gao, Z., Chen, S., and Ye, W. (2025). Cuproptosis related lncRNA signature as a prognostic and therapeutic biomarker in osteosarcoma immunity. Sci. Rep. 15 (1), 221. doi:10.1038/s41598-024-84024-9
Gromadzka, G., Czerwinska, J., Krzeminska, E., Przybylkowski, A., and Litwin, T. (2024). Wilson's disease-Crossroads of genetics, inflammation and immunity/Autoimmunity: clinical and molecular Issues. Int. J. Mol. Sci. 25 (16), 9034. doi:10.3390/ijms25169034
Gromadzka, G., Tarnacka, B., Flaga, A., and Adamczyk, A. (2020). Copper dyshomeostasis in neurodegenerative diseases-therapeutic implications. Int. J. Mol. Sci. 21 (23), 9259. doi:10.3390/ijms21239259
Guan, M., Yu, Q., Zhou, G., Wang, Y., Yu, J., Yang, W., et al. (2024). Mechanisms of chondrocyte cell death in osteoarthritis: implications for disease progression and treatment. J. Orthop. Surg. Res. 19 (1), 550. doi:10.1186/s13018-024-05055-6
Guan, T., Wu, Z., Xu, C., and Su, G. (2023). The association of trace elements with arthritis in US adults: NHANES 2013-2016. J. Trace Elem. Med. Biol. 76, 127122. doi:10.1016/j.jtemb.2022.127122
Gupte, A., and Mumper, R. J. (2009). Elevated copper and oxidative stress in cancer cells as a target for cancer treatment. Cancer Treat. Rev. 35 (1), 32–46. doi:10.1016/j.ctrv.2008.07.004
Halliwell, B., and Gutteridge, J. M. (1984). Oxygen toxicity, oxygen radicals, transition metals and disease. Biochem. J. 219 (1), 1–14. doi:10.1042/bj2190001
Han, J., Luo, J., Wang, C., Kapilevich, L., and Zhang, X. A. (2024). Roles and mechanisms of copper homeostasis and cuproptosis in osteoarticular diseases. Biomed. Pharmacother. 174, 116570. doi:10.1016/j.biopha.2024.116570
Hatori, Y., and Lutsenko, S. (2016). The role of copper chaperone Atox1 in coupling redox homeostasis to intracellular copper distribution. Antioxidants (Basel) 5 (3), 25. doi:10.3390/antiox5030025
He, B., Liao, Y., Tian, M., Tang, C., Tang, Q., Ma, F., et al. (2024). Identification and verification of a novel signature that combines cuproptosis-related genes with ferroptosis-related genes in osteoarthritis using bioinformatics analysis and experimental validation. Arthritis Res. Ther. 26 (1), 100. doi:10.1186/s13075-024-03328-3
He, X., Deng, B., Ma, M., Wang, K., Li, Y., Wang, Y., et al. (2023a). Bioinformatics analysis of programmed cell death in spinal cord injury. World Neurosurg. 177, e332–e342. doi:10.1016/j.wneu.2023.06.043
He, X., Hu, W., Zhang, Y., Chen, M., Ding, Y., Yang, H., et al. (2023b). Cellular senescence in skeletal disease: mechanisms and treatment. Cell Mol. Biol. Lett. 28 (1), 88. doi:10.1186/s11658-023-00501-5
Hu, H., Yin, Y., Jiang, B., Feng, Z., Cai, T., and Wu, S. (2023). Cuproptosis signature and PLCD3 predicts immune infiltration and drug responses in osteosarcoma. Front. Oncol. 13, 1156455. doi:10.3389/fonc.2023.1156455
Huang, B., Li, G., Cao, L., Wu, S., Zhang, Y., Li, Z., et al. (2024). Nanoengineered 3D-printing scaffolds prepared by metal-coordination self-assembly for hyperthermia-catalytic osteosarcoma therapy and bone regeneration. J. Colloid Interface Sci. 672, 724–735. doi:10.1016/j.jcis.2024.06.055
Huang, L., Jin, W., Bao, Y., Zeng, X., Zhang, Y., Zhou, J., et al. (2023). Identification and validation of long noncoding RNA AC083900.1 and RP11-283C24.1 for prediction of progression of osteosarcoma. Mutat. Res. 827, 111828. doi:10.1016/j.mrfmmm.2023.111828
Hunter, D. J., March, L., and Chew, M. (2020). Osteoarthritis in 2020 and beyond: a Lancet Commission. Lancet 396 (10264), 1711–1712. doi:10.1016/S0140-6736(20)32230-3
Iantomasi, T., Romagnoli, C., Palmini, G., Donati, S., Falsetti, I., Miglietta, F., et al. (2023). Oxidative stress and inflammation in osteoporosis: molecular mechanisms involved and the relationship with microRNAs. Int. J. Mol. Sci. 24 (4), 3772. doi:10.3390/ijms24043772
Ismail, M., Yang, W., Li, Y., Wang, Y., He, W., Wang, J., et al. (2022). Biomimetic Dp44mT-nanoparticles selectively induce apoptosis in Cu-loaded glioblastoma resulting in potent growth inhibition. Biomaterials 289, 121760. doi:10.1016/j.biomaterials.2022.121760
Jackson, M. J. (2009). Skeletal muscle aging: role of reactive oxygen species. Crit. Care Med. 37 (10 Suppl. l), S368–S371. doi:10.1097/CCM.0b013e3181b6f97f
Jia, D., Liu, L., Liu, W., Li, J., Jiang, X., and Xin, Y. (2024). Copper metabolism and its role in diabetic complications: a review. Pharmacol. Res. 206, 107264. doi:10.1016/j.phrs.2024.107264
Jiang, J., Chu, D., Lai, X., Liu, L., and Tao, J. (2022). The cuproptosis-related long noncoding RNA signature predicts prognosis and Tumour immune analysis in osteosarcoma. Comput. Math. Methods Med. 2022, 6314182. doi:10.1155/2022/6314182
Jiang, J., Zhan, X., Wei, J., Fan, Q., Li, H., Li, H., et al. (2023a). Artificial intelligence reveals dysregulation of osteosarcoma and cuproptosis-related biomarkers, PDHA1, CDKN2A and neutrophils. Sci. Rep. 13 (1), 4927. doi:10.1038/s41598-023-32195-2
Jiang, M., Liu, K., Lu, S., Qiu, Y., Zou, X., Zhang, K., et al. (2023b). Verification of cuproptosis-related diagnostic model associated with immune infiltration in rheumatoid arthritis. Front. Endocrinol. (Lausanne) 14, 1204926. doi:10.3389/fendo.2023.1204926
Jiang, X., Ji, S., Yuan, F., Li, T., Cui, S., Wang, W., et al. (2023c). Pyruvate dehydrogenase B regulates myogenic differentiation via the FoxP1-Arih2 axis. J. Cachexia Sarcopenia Muscle 14 (1), 606–621. doi:10.1002/jcsm.13166
Jin, H., Xie, W., He, M., Li, H., Xiao, W., and Li, Y. (2022). Pyroptosis and sarcopenia: frontier Perspective of disease mechanism. Cells 11 (7), 1078. doi:10.3390/cells11071078
Jin, X., Liu, W., Miao, J., Tai, Z., Li, L., Guan, P., et al. (2021). Copper ions impair zebrafish skeletal myofibrillogenesis via epigenetic regulation. FASEB J. 35 (7), e21686. doi:10.1096/fj.202100183R
Kanumakala, S., Boneh, A., and Zacharin, M. (2002). Pamidronate treatment improves bone mineral density in children with Menkes disease. J. Inherit. Metab. Dis. 25 (5), 391–398. doi:10.1023/a:1020103901969
Kardos, J., Heja, L., Simon, A., Jablonkai, I., Kovacs, R., and Jemnitz, K. (2018). Copper signalling: causes and consequences. Cell Commun. Signal 16 (1), 71. doi:10.1186/s12964-018-0277-3
Knott, G. J., and Doudna, J. A. (2018). CRISPR-Cas guides the future of genetic engineering. Science 361 (6405), 866–869. doi:10.1126/science.aat5011
Knowles, H. J. (2015). Hypoxic regulation of osteoclast differentiation and bone resorption activity. Hypoxia (Auckl) 3, 73–82. doi:10.2147/HP.S95960
Koo, S., Park, O. K., Kim, J., Han, S. I., Yoo, T. Y., Lee, N., et al. (2022). Enhanced chemodynamic therapy by Cu-Fe peroxide nanoparticles: tumor microenvironment-mediated synergistic Fenton reaction. ACS Nano 16 (2), 2535–2545. doi:10.1021/acsnano.1c09171
Kumar, V., Singh, A. P., Wheeler, N., Galindo, C. L., and Kim, J. J. (2021). Safety profile of D-penicillamine: a comprehensive pharmacovigilance analysis by FDA adverse event reporting system. Expert Opin. Drug Saf. 20 (11), 1443–1450. doi:10.1080/14740338.2021.1956460
Lane, J. M., Russell, L., and Khan, S. N. (2000). Osteoporosis. Clin. Orthop. Relat. Res. 372, 139–150. doi:10.1097/00003086-200003000-00016
Leon, I. E., Porro, V., Astrada, S., Egusquiza, M. G., Cabello, C. I., Bollati-Fogolin, M., et al. (2014). Polyoxometalates as antitumor agents: Bioactivity of a new polyoxometalate with copper on a human osteosarcoma model. Chem. Biol. Interact. 222, 87–96. doi:10.1016/j.cbi.2014.10.012
Leuci, R., Brunetti, L., Tufarelli, V., Cerini, M., Paparella, M., Puvaca, N., et al. (2025). Role of copper chelating agents: between old applications and new perspectives in neuroscience. Neural Regen. Res. 20 (3), 751–762. doi:10.4103/NRR.NRR-D-24-00140
Lew, D. P., and Waldvogel, F. A. (1997). Osteomyelitis. N. Engl. J. Med. 336 (14), 999–1007. doi:10.1056/NEJM199704033361406
Lew, D. P., and Waldvogel, F. A. (2004). Osteomyelitis. Lancet 364 (9431), 369–379. doi:10.1016/S0140-6736(04)16727-5
Li, B., Jiang, T., Wang, J., Ge, H., Zhang, Y., Li, T., et al. (2024). Cuprorivaite microspheres inhibit cuproptosis and oxidative stress in osteoarthritis via Wnt/β-catenin pathway. Mater Today Bio 29, 101300. doi:10.1016/j.mtbio.2024.101300
Li, C., Wu, C., Ji, C., Xu, G., Chen, J., Zhang, J., et al. (2023a). The pathogenesis of DLD-mediated cuproptosis induced spinal cord injury and its regulation on immune microenvironment. Front. Cell Neurosci. 17, 1132015. doi:10.3389/fncel.2023.1132015
Li, C. W., Yu, K., Shyh-Chang, N., Li, G. X., Jiang, L. J., Yu, S. L., et al. (2019). Circulating factors associated with sarcopenia during ageing and after intensive lifestyle intervention. J. Cachexia Sarcopenia Muscle 10 (3), 586–600. doi:10.1002/jcsm.12417
Li, D., Gao, Z., Li, Q., Liu, X., and Liu, H. (2023b). Cuproptosis-a potential target for the treatment of osteoporosis. Front. Endocrinol. (Lausanne) 14, 1135181. doi:10.3389/fendo.2023.1135181
Li, F., Wu, X., Liu, H., Liu, M., Yue, Z., Wu, Z., et al. (2022a). Copper depletion strongly enhances ferroptosis via mitochondrial Perturbation and reduction in Antioxidative mechanisms. Antioxidants (Basel) 11 (11), 2084. doi:10.3390/antiox11112084
Li, K., Chen, Y., Lin, Y., Zhang, G., Su, J., Wu, X., et al. (2023c). PD-1/PD-L1 blockade is a potent adjuvant in treatment of Staphylococcus aureus osteomyelitis in mice. Mol. Ther. 31 (1), 174–192. doi:10.1016/j.ymthe.2022.09.006
Li, Q. C., Li, C., Zhang, W., Pi, W., and Han, N. (2022b). Potential effects of Exosomes and their MicroRNA carrier on osteoporosis. Curr. Pharm. Des. 28 (11), 899–909. doi:10.2174/1381612828666220128104206
Li, Y., Kong, C., Wang, W., Hu, F., Chen, X., Xu, B., et al. (2023d). Screening of miR-15a-5p as a potential biomarker for intervertebral disc degeneration through RNA-sequencing. Int. Immunopharmacol. 123, 110717. doi:10.1016/j.intimp.2023.110717
Li, Y., Li, J., Zhong, Y., Zhang, Q., Wu, Y., Huang, J., et al. (2025). pH-responsive and nanoenzyme-loaded artificial nanocells relieved osteomyelitis efficiently by synergistic chemodynamic and cuproptosis therapy. Biomaterials 313, 122762. doi:10.1016/j.biomaterials.2024.122762
Li, Y., Xie, W., Xiao, W., and Dou, D. (2022c). Progress in osteoarthritis research by the National natural Science foundation of China. Bone Res. 10 (1), 41. doi:10.1038/s41413-022-00207-y
Lill, R., and Freibert, S. A. (2020). Mechanisms of mitochondrial iron-sulfur protein Biogenesis. Annu. Rev. Biochem. 89, 471–499. doi:10.1146/annurev-biochem-013118-111540
Lin, S., Huang, H., Ling, M., Zhang, C., Yang, F., and Fan, Y. (2022). Development and validation of a novel diagnostic model for musculoskeletal aging (sarcopenia) based on cuproptosis-related genes associated with immunity. Am. J. Transl. Res. 14 (12), 8523–8538.
Lin, Z., Wu, Z., He, Y., Li, X., and Luo, W. (2025). CYFIP1 coordinate with RNMT to induce osteosarcoma cuproptosis via AURKAIP1 m7G modification. Mol. Med. 31 (1), 74. doi:10.1186/s10020-025-01127-3
Liu, B., Liu, Z., Feng, C., Li, C., Zhang, H., Li, Z., et al. (2022a). Identification of cuproptosis-related lncRNA prognostic signature for osteosarcoma. Front. Endocrinol. (Lausanne) 13, 987942. doi:10.3389/fendo.2022.987942
Liu, C., Liu, Y., Ma, B., Zhou, M., Zhao, X., Fu, X., et al. (2022b). Mitochondrial regulatory mechanisms in spinal cord injury: a narrative review. Med. (Baltimore) 101 (46), e31930. doi:10.1097/MD.0000000000031930
Liu, J., Gao, Z., and Liu, X. (2024). Mitochondrial dysfunction and therapeutic perspectives in osteoporosis. Front. Endocrinol. (Lausanne) 15, 1325317. doi:10.3389/fendo.2024.1325317
Liu, P., Wang, W., Li, Z., Li, Y., Yu, X., Tu, J., et al. (2022c). Ferroptosis: a new regulatory mechanism in osteoporosis. Oxid. Med. Cell Longev. 2022, 2634431. doi:10.1155/2022/2634431
Liu, T., Liu, Y., Zhang, F., and Gao, Y. (2023). Copper homeostasis dysregulation promoting cell damage and the association with liver diseases. Chin. Med. J. Engl. 136 (14), 1653–1662. doi:10.1097/CM9.0000000000002697
Liu, Y., Zhu, J., Xu, L., Wang, B., Lin, W., and Luo, Y. (2022d). Copper regulation of immune response and potential implications for treating orthopedic disorders. Front. Mol. Biosci. 9, 1065265. doi:10.3389/fmolb.2022.1065265
Locatelli, M., and Farina, C. (2025). Role of copper in central nervous system physiology and pathology. Neural Regen. Res. 20 (4), 1058–1068. doi:10.4103/NRR.NRR-D-24-00110
Luo, Y., Zheng, S., Xiao, W., Zhang, H., and Li, Y. (2024). Pannexins in the musculoskeletal system: new targets for development and disease progression. Bone Res. 12 (1), 26. doi:10.1038/s41413-024-00334-8
Lutsenko, S. (2021). Dynamic and cell-specific transport networks for intracellular copper ions. J. Cell Sci. 134 (21), jcs240523. doi:10.1242/jcs.240523
Lutsenko, S., Roy, S., and Tsvetkov, P. (2025). Mammalian copper homeostasis: physiological roles and molecular mechanisms. Physiol. Rev. 105 (1), 441–491. doi:10.1152/physrev.00011.2024
Ma, B., Zhao, Y., Liu, X., Huo, M., Wang, J., Ma, J., et al. (2023). Key modulation of ROS and HSP for effective therapy against hypoxic tumor with multifunctional Nanosystem. Int. J. Nanomedicine 18, 6829–6846. doi:10.2147/IJN.S432928
Magistrato, A., Pavlin, M., Qasem, Z., and Ruthstein, S. (2019). Copper trafficking in eukaryotic systems: current knowledge from experimental and computational efforts. Curr. Opin. Struct. Biol. 58, 26–33. doi:10.1016/j.sbi.2019.05.002
Mao, D., Chen, Q., Tong, S., Xu, Z., Yu, G., Chang, C., et al. (2025). Integrated bioinformatics analysis identified cuproptosis-related hub gene Mpeg1 as potential biomarker in spinal cord injury. Sci. Rep. 15 (1), 1993. doi:10.1038/s41598-025-86170-0
McCann, C., Quinteros, M., Adelugba, I., Morgada, M. N., Castelblanco, A. R., Davis, E. J., et al. (2022). The mitochondrial Cu+ transporter PiC2 (SLC25A3) is a target of MTF1 and contributes to the development of skeletal muscle in vitro. Front. Mol. Biosci. 9, 1037941. doi:10.3389/fmolb.2022.1037941
McDonald, J. W., and Sadowsky, C. (2002). Spinal-cord injury. Lancet 359 (9304), 417–425. doi:10.1016/S0140-6736(02)07603-1
Mendelsohn, D. H., Niedermair, T., Walter, N., Alt, V., Rupp, M., and Brochhausen, C. (2023). Ultrastructural evidence of mitochondrial dysfunction in osteomyelitis patients. Int. J. Mol. Sci. 24 (6), 5709. doi:10.3390/ijms24065709
Mendoza Bertelli, A., Delpino, M. V., Lattar, S., Giai, C., Llana, M. N., Sanjuan, N., et al. (2016). Staphylococcus aureus protein A enhances osteoclastogenesis via TNFR1 and EGFR signaling. Biochim. Biophys. Acta 1862 (10), 1975–1983. doi:10.1016/j.bbadis.2016.07.016
Muthurangan, M., Elsafadi, M., Siyal, A., Kaimkhani, Z. A., Umrani, A., AlMuraikhi, N. A., et al. (2023). Transient downregulation of NR4A1 leads to impaired osteoblast differentiation through the TGF-ß pathway, and Elesclomol (STA-4783) rescues this phenotype. Cell Biochem. Funct. 41 (5), 590–598. doi:10.1002/cbf.3814
Nagai, M., Vo, N. H., Shin Ogawa, L., Chimmanamada, D., Inoue, T., Chu, J., et al. (2012). The oncology drug elesclomol selectively transports copper to the mitochondria to induce oxidative stress in cancer cells. Free Radic. Biol. Med. 52 (10), 2142–2150. doi:10.1016/j.freeradbiomed.2012.03.017
Nam, E., Han, J., Suh, J. M., Yi, Y., and Lim, M. H. (2018). Link of impaired metal ion homeostasis to mitochondrial dysfunction in neurons. Curr. Opin. Chem. Biol. 43, 8–14. doi:10.1016/j.cbpa.2017.09.009
Ni, S., Hong, J., Li, W., Ye, M., and Li, J. (2023). Construction of a cuproptosis-related lncRNA signature for predicting prognosis and immune landscape in osteosarcoma patients. Cancer Med. 12 (4), 5009–5024. doi:10.1002/cam4.5214
Ning, S., Lyu, M., Zhu, D., Lam, J. W. Y., Huang, Q., Zhang, T., et al. (2023). Type-I AIE Photosensitizer loaded Biomimetic system Boosting cuproptosis to inhibit Breast cancer metastasis and Rechallenge. ACS Nano 17 (11), 10206–10217. doi:10.1021/acsnano.3c00326
Nishikawa, H., Fukunishi, S., Asai, A., Yokohama, K., Nishiguchi, S., and Higuchi, K. (2021). Pathophysiology and mechanisms of primary sarcopenia (Review). Int. J. Mol. Med. 48 (2), 156. doi:10.3892/ijmm.2021.4989
Nong, J., Lu, G., Huang, Y., Liu, J., Chen, L., Pan, H., et al. (2023). Identification of cuproptosis-related subtypes, characterization of immune microenvironment infiltration, and development of a prognosis model for osteoarthritis. Front. Immunol. 14, 1178794. doi:10.3389/fimmu.2023.1178794
Peng, F., Liao, M., Qin, R., Zhu, S., Peng, C., Fu, L., et al. (2022). Regulated cell death (RCD) in cancer: key pathways and targeted therapies. Signal Transduct. Target Ther. 7 (1), 286. doi:10.1038/s41392-022-01110-y
Qiu, L., Ma, S., Yang, R., Zheng, D., Huang, Y., Zhu, Z., et al. (2025). Ultrasound-activated piezoelectric heterojunction drives nanozyme catalysis to induce bacterial cuproptosis-like death and promote bone vascularization and osseointegration. Biomaterials 320, 123249. doi:10.1016/j.biomaterials.2025.123249
Qiu, Z., Liu, Q., Wang, L., Xiong, Y., Wu, J., Wang, M., et al. (2024). The copper transporter, SLC31A1, transcriptionally activated by ELF3, imbalances copper homeostasis to exacerbate cisplatin-induced acute kidney injury through mitochondrial dysfunction. Chem. Biol. Interact. 393, 110943. doi:10.1016/j.cbi.2024.110943
Radu, A. F., and Bungau, S. G. (2021). Management of rheumatoid arthritis: an Overview. Cells 10 (11), 2857. doi:10.3390/cells10112857
Riegger, J., Schoppa, A., Ruths, L., Haffner-Luntzer, M., and Ignatius, A. (2023). Oxidative stress as a key modulator of cell fate decision in osteoarthritis and osteoporosis: a narrative review. Cell Mol. Biol. Lett. 28 (1), 76. doi:10.1186/s11658-023-00489-y
Roy, S., and Lutsenko, S. (2024). Mechanism of Cu entry into the brain: many unanswered questions. Neural Regen. Res. 19 (11), 2421–2429. doi:10.4103/1673-5374.393107
Scheiber, I. F., Mercer, J. F., and Dringen, R. (2014). Metabolism and functions of copper in brain. Prog. Neurobiol. 116, 33–57. doi:10.1016/j.pneurobio.2014.01.002
Shi, X., Ni, H., Tang, L., Li, M., Wu, Y., and Xu, Y. (2024). Comprehensive gene analysis reveals cuproptosis-related gene signature associated with M2 macrophage in Staphylococcus aureus-infected osteomyelitis. J. Inflamm. Res. 17, 3057–3077. doi:10.2147/JIR.S457414
Smith, L. J., Nerurkar, N. L., Choi, K. S., Harfe, B. D., and Elliott, D. M. (2011). Degeneration and regeneration of the intervertebral disc: lessons from development. Dis. Model Mech. 4 (1), 31–41. doi:10.1242/dmm.006403
Smolen, J. S., Aletaha, D., and McInnes, I. B. (2016). Rheumatoid arthritis. Lancet 388 (10055), 2023–2038. doi:10.1016/S0140-6736(16)30173-8
Song, Q., Cui, Q., Sun, S., Wang, Y., Yuan, Y., and Zhang, L. (2024). Crosstalk between cell death and spinal cord injury: Neurology and therapy. Mol. Neurobiol. 61 (12), 10271–10287. doi:10.1007/s12035-024-04188-3
Staszkiewicz, R., Sobanski, D., Ulasavets, U., Wieczorek, J., Golec, E., Marcol, W., et al. (2023). Evaluation of the concentration of selected elements in serum patients with intervertebral disc degeneration. J. Trace Elem. Med. Biol. 77, 127145. doi:10.1016/j.jtemb.2023.127145
Sun, Y., Chen, P., and Zhao, B. (2024). Role of extracellular vesicles associated with microRNAs and their interplay with cuproptosis in osteoporosis. Noncoding RNA Res. 9 (3), 715–719. doi:10.1016/j.ncrna.2024.03.002
Tang, D., Kroemer, G., and Kang, R. (2024). Targeting cuproplasia and cuproptosis in cancer. Nat. Rev. Clin. Oncol. 21 (5), 370–388. doi:10.1038/s41571-024-00876-0
Tardito, S., Bassanetti, I., Bignardi, C., Elviri, L., Tegoni, M., Mucchino, C., et al. (2011). Copper binding agents acting as copper ionophores lead to caspase inhibition and paraptotic cell death in human cancer cells. J. Am. Chem. Soc. 133 (16), 6235–6242. doi:10.1021/ja109413c
Teschke, R., and Eickhoff, A. (2024). Wilson disease: copper-mediated cuproptosis, iron-related ferroptosis, and clinical highlights, with comprehensive and critical analysis Update. Int. J. Mol. Sci. 25 (9), 4753. doi:10.3390/ijms25094753
Tian, Z., Jiang, S., Zhou, J., and Zhang, W. (2023). Copper homeostasis and cuproptosis in mitochondria. Life Sci. 334, 122223. doi:10.1016/j.lfs.2023.122223
Tong, X., Tang, R., Xiao, M., Xu, J., Wang, W., Zhang, B., et al. (2022). Targeting cell death pathways for cancer therapy: recent developments in necroptosis, pyroptosis, ferroptosis, and cuproptosis research. J. Hematol. Oncol. 15 (1), 174. doi:10.1186/s13045-022-01392-3
Tsvetkov, P., Coy, S., Petrova, B., Dreishpoon, M., Verma, A., Abdusamad, M., et al. (2022). Copper induces cell death by targeting lipoylated TCA cycle proteins. Science 375 (6586), 1254–1261. doi:10.1126/science.abf0529
Turk, M. A., Liu, Y., and Pope, J. E. (2023). Non-pharmacological interventions in the treatment of rheumatoid arthritis: a systematic review and meta-analysis. Autoimmun. Rev. 22 (6), 103323. doi:10.1016/j.autrev.2023.103323
Valko, M., Morris, H., and Cronin, M. T. (2005). Metals, toxicity and oxidative stress. Curr. Med. Chem. 12 (10), 1161–1208. doi:10.2174/0929867053764635
Viktorinova, A. (2017). Current insights on the role of iron and copper dyshomeostasis in the pathogenesis of bilirubin neurotoxicity. Life Sci. 191, 34–45. doi:10.1016/j.lfs.2017.10.013
Wang, A., Liu, W., Jin, Y., Wei, B., Fan, Y., Guo, X., et al. (2023a). Identification of immunological characteristics and cuproptosis-related molecular clusters in Rheumatoid arthritis. Int. Immunopharmacol. 123, 110804. doi:10.1016/j.intimp.2023.110804
Wang, J., Ye, J., Yang, G., Xie, J., Miao, X., Deng, J., et al. (2024a). Fenton-like reaction Inspired. “OH catalyzed” osteogenic process for the treatment of osteoporosis. Adv. Healthc. Mater 13 (15), e2304091. doi:10.1002/adhm.202304091
Wang, W., Chen, Z., and Hua, Y. (2023b). Bioinformatics prediction and experimental validation identify a novel cuproptosis-related gene signature in human synovial inflammation during osteoarthritis progression. Biomolecules 13 (1), 127. doi:10.3390/biom13010127
Wang, X., Xie, C., and Lin, L. (2023c). Development and validation of a cuproptosis-related lncRNA model correlated to the cancer-associated fibroblasts enable the prediction prognosis of patients with osteosarcoma. J. Bone Oncol. 38, 100463. doi:10.1016/j.jbo.2022.100463
Wang, X., Zhou, M., Liu, Y., and Si, Z. (2023d). Cope with copper: from copper linked mechanisms to copper-based clinical cancer therapies. Cancer Lett. 561, 216157. doi:10.1016/j.canlet.2023.216157
Wang, Y., Chen, Y., Zhang, J., Yang, Y., Fleishman, J. S., Wang, Y., et al. (2024b). Cuproptosis: a novel therapeutic target for overcoming cancer drug resistance. Drug Resist Updat 72, 101018. doi:10.1016/j.drup.2023.101018
Wang, Y., Pei, P., Yang, K., Guo, L., and Li, Y. (2024c). Copper in colorectal cancer: from copper-related mechanisms to clinical cancer therapies. Clin. Transl. Med. 14 (6), e1724. doi:10.1002/ctm2.1724
Wang, Y., Tao, Y., Hyman, M. E., Li, J., and Chen, Y. (2009). Osteoporosis in China. Osteoporos. Int. 20 (10), 1651–1662. doi:10.1007/s00198-009-0925-y
Wapnir, R. A. (1998). Copper absorption and bioavailability. Am. J. Clin. Nutr. 67 (5 Suppl. l), 1054S–1060S. doi:10.1093/ajcn/67.5.1054S
Wei, M., Huang, Q., Dai, Y., Zhou, H., Cui, Y., Song, W., et al. (2022). Manganese, iron, copper, and selenium co-exposure and osteoporosis risk in Chinese adults. J. Trace Elem. Med. Biol. 72, 126989. doi:10.1016/j.jtemb.2022.126989
Wen, H., Qu, C., Wang, Z., Gao, H., Liu, W., Wang, H., et al. (2023). Cuproptosis enhances docetaxel chemosensitivity by inhibiting autophagy via the DLAT/mTOR pathway in prostate cancer. Faseb J. 37 (9), e23145. doi:10.1096/fj.202300980R
Winer, A., Bodor, J. N., and Borghaei, H. (2018). Identifying and managing the adverse effects of immune checkpoint blockade. J. Thorac. Dis. 10 (Suppl. 3), S480–s489. doi:10.21037/jtd.2018.01.111
Wittig, J. C., Bickels, J., Priebat, D., Jelinek, J., Kellar-Graney, K., Shmookler, B., et al. (2002). Osteosarcoma: a multidisciplinary approach to diagnosis and treatment. Am. Fam. Physician 65 (6), 1123–1132.
Wittung-Stafshede, P. (2022). Crossroads between copper ions and amyloid formation in Parkinson’s disease. Essays Biochem. 66 (7), 977–986. doi:10.1042/EBC20220043
Wu, H., Zhang, Z., Cao, Y., Hu, Y., Li, Y., Zhang, L., et al. (2024a). A self-amplifying ROS-responsive Nanoplatform for simultaneous cuproptosis and cancer immunotherapy. Adv. Sci. (Weinh) 11 (23), e2401047. doi:10.1002/advs.202401047
Wu, J., He, J., Liu, Z., Zhu, X., Li, Z., Chen, A., et al. (2024b). Cuproptosis: mechanism, role, and advances in urological malignancies. Med. Res. Rev. 44 (4), 1662–1682. doi:10.1002/med.22025
Wu, J., Lin, S., Chen, W., Lian, G., Wu, W., Chen, A., et al. (2023a). TNF-alpha contributes to sarcopenia through caspase-8/caspase-3/GSDME-mediated pyroptosis. Cell Death Discov. 9 (1), 76. doi:10.1038/s41420-023-01365-6
Wu, P. H., Kim, H. S., and Jang, I. T. (2020). Intervertebral disc diseases PART 2: a review of the current diagnostic and treatment strategies for intervertebral disc disease. Int. J. Mol. Sci. 21 (6), 2135. doi:10.3390/ijms21062135
Wu, Z., Lv, G., Xing, F., Xiang, W., Ma, Y., Feng, Q., et al. (2023b). Copper in hepatocellular carcinoma: a double-edged sword with therapeutic potentials. Cancer Lett. 571, 216348. doi:10.1016/j.canlet.2023.216348
Xia, Q., Zhao, Y., Dong, H., Mao, Q., Zhu, L., Xia, J., et al. (2024). Progress in the study of molecular mechanisms of intervertebral disc degeneration. Biomed. Pharmacother. 174, 116593. doi:10.1016/j.biopha.2024.116593
Xiao, Y., Chen, D. I., Zhang, X., Cui, Q., Fan, Y., Bi, C., et al. (2010). Molecular study on copper-mediated tumor proteasome inhibition and cell death. Int. J. Oncol. 37 (1), 81–87. doi:10.3892/ijo_00000655
Xin, L., Yang, X., Cai, G., Fan, D., Xia, Q., Liu, L., et al. (2015). Serum levels of copper and zinc in patients with rheumatoid arthritis: a meta-analysis. Biol. Trace Elem. Res. 168 (1), 1–10. doi:10.1007/s12011-015-0325-4
Xu, F., Hu, X. R., Wang, Y., and Mei, X. F. (2025). Exploring the impact of cuproptosis-related genes on immune infiltration in rheumatoid arthritis. Naunyn Schmiedeb. Arch. Pharmacol. doi:10.1007/s00210-024-03731-2
Xue, Q., Yan, D., Chen, X., Li, X., Kang, R., Klionsky, D. J., et al. (2023). Copper-dependent autophagic degradation of GPX4 drives ferroptosis. Autophagy 19 (7), 1982–1996. doi:10.1080/15548627.2023.2165323
Yang, J., Dai, D., Zhang, X., Teng, L., Ma, L., and Yang, Y. W. (2023a). Multifunctional metal-organic framework (MOF)-based nanoplatforms for cancer therapy: from single to combination therapy. Theranostics 13 (1), 295–323. doi:10.7150/thno.80687
Yang, M., Zheng, H., Xu, K., Yuan, Q., Aihaiti, Y., Cai, Y., et al. (2022). A novel signature to guide osteosarcoma prognosis and immune microenvironment: cuproptosis-related lncRNA. Front. Immunol. 13, 919231. doi:10.3389/fimmu.2022.919231
Yang, W. M., Lv, J. F., Wang, Y. Y., Xu, Y. M., Lin, J., Liu, J., et al. (2023b). The daily intake levels of copper, selenium, and zinc are associated with osteoarthritis but not with rheumatoid arthritis in a cross-sectional study. Biol. Trace Elem. Res. 201 (12), 5662–5670. doi:10.1007/s12011-023-03636-w
Yazar, M., Sarban, S., Kocyigit, A., and Isikan, U. E. (2005). Synovial fluid and plasma selenium, copper, zinc, and iron concentrations in patients with rheumatoid arthritis and osteoarthritis. Biol. Trace Elem. Res. 106 (2), 123–132. doi:10.1385/BTER:106:2:123
Ye, L., Yu, C., Xia, J., Ni, K., Zhang, Y., Ying, X., et al. (2024). Multifunctional nanomaterials via cell cuproptosis and oxidative stress for treating osteosarcoma and OS-induced bone destruction. Mater Today Bio 25, 100996. doi:10.1016/j.mtbio.2024.100996
Yu, Q., Xiao, Y., Guan, M., Zhang, X., Yu, J., Han, M., et al. (2024). Copper metabolism in osteoarthritis and its relation to oxidative stress and ferroptosis in chondrocytes. Front. Mol. Biosci. 11, 1472492. doi:10.3389/fmolb.2024.1472492
Yuan, S., and Larsson, S. C. (2023). Epidemiology of sarcopenia: Prevalence, risk factors, and consequences. Metabolism 144, 155533. doi:10.1016/j.metabol.2023.155533
Zhang, C., Li, H., Li, J., Hu, J., Yang, K., and Tao, L. (2023a). Oxidative stress: a common pathological state in a high-risk population for osteoporosis. Biomed. Pharmacother. 163, 114834. doi:10.1016/j.biopha.2023.114834
Zhang, D., Li, Y., Pan, J., Zheng, Y., and Xu, X. (2024). Copper homeostasis and cuproptosis in radiation-induced injury. Biomed. Pharmacother. 178, 117150. doi:10.1016/j.biopha.2024.117150
Zhang, P., He, J., Gan, Y., Shang, Q., Chen, H., Zhao, W., et al. (2023b). Unravelling diagnostic clusters and immune landscapes of cuproptosis patterns in intervertebral disc degeneration through dry and wet experiments. Aging (Albany NY) 15 (24), 15599–15623. doi:10.18632/aging.205449
Zhang, W., Chen, C., Shi, H., Yang, M., Liu, Y., Ji, P., et al. (2016). Curcumin is a biologically active copper chelator with antitumor activity. Phytomedicine 23 (1), 1–8. doi:10.1016/j.phymed.2015.11.005
Zhao, J., Guo, S., Schrodi, S. J., and He, D. (2022). Cuproptosis and cuproptosis-related genes in rheumatoid arthritis: implication, prospects, and perspectives. Front. Immunol. 13, 930278. doi:10.3389/fimmu.2022.930278
Zhou, J., Liu, C., Sun, Y., Francis, M., Ryu, M. S., Grider, A., et al. (2021). Genetically predicted circulating levels of copper and zinc are associated with osteoarthritis but not with rheumatoid arthritis. Osteoarthr. Cartil. 29 (7), 1029–1035. doi:10.1016/j.joca.2021.02.564
Zhou, M., Muhammad, F., Zhang, Y., Li, T., Feng, J., Zhao, J., et al. (2024). Copper depletion-induced tumor cuproptosis. Chem. Sci. 16, 4226–4236. doi:10.1039/d4sc04712e
Zhou, Y., Li, X., Ng, L., Zhao, Q., Guo, W., Hu, J., et al. (2023). Identification of copper death-associated molecular clusters and immunological profiles in rheumatoid arthritis. Front. Immunol. 14, 1103509. doi:10.3389/fimmu.2023.1103509
Zhu, C., Han, S., Zeng, X., Zhu, C., Pu, Y., and Sun, Y. (2022). Multifunctional thermo-sensitive hydrogel for modulating the microenvironment in Osteoarthritis by polarizing macrophages and scavenging RONS. J. Nanobiotechnology 20 (1), 221. doi:10.1186/s12951-022-01422-9
Zhu, Y., Chen, X., Geng, S., Li, Q., Li, Y., Yuan, H., et al. (2023). Identification of the cuproptosis-related hub genes and therapeutic agents for sarcopenia. Front. Genet. 14, 1136763. doi:10.3389/fgene.2023.1136763
Zhu, Z., Song, M., Ren, J., Liang, L., Mao, G., and Chen, M. (2024). Copper homeostasis and cuproptosis in central nervous system diseases. Cell Death Dis. 15 (11), 850. doi:10.1038/s41419-024-07206-3
Zhuang, L., Zhang, B., Liu, X., Lin, L., Wang, L., Hong, Z., et al. (2021). Exosomal miR-21-5p derived from cisplatin-resistant SKOV3 ovarian cancer cells promotes glycolysis and inhibits chemosensitivity of its progenitor SKOV3 cells by targeting PDHA1. Cell Biol. Int. 45 (10), 2140–2149. doi:10.1002/cbin.11671
Keywords: cuproptosis, osteoporosis, osteoarthritis, osteosarcoma, intervertebral disc degeneration, spinal cord injury, osteomyelitis
Citation: Xiang Z, Mei H, Wang H, Yao X, Rao J, Zhang W, Xu A and Lu L (2025) Cuproptosis and its potential role in musculoskeletal disease. Front. Cell Dev. Biol. 13:1570131. doi: 10.3389/fcell.2025.1570131
Received: 03 February 2025; Accepted: 19 March 2025;
Published: 11 April 2025.
Edited by:
Sheng Chen, Huazhong University of Science and Technology, ChinaReviewed by:
Qiuyue Ding, Guizhou Provincial People’s Hospital, ChinaCopyright © 2025 Xiang, Mei, Wang, Yao, Rao, Zhang, Xu and Lu. This is an open-access article distributed under the terms of the Creative Commons Attribution License (CC BY). The use, distribution or reproduction in other forums is permitted, provided the original author(s) and the copyright owner(s) are credited and that the original publication in this journal is cited, in accordance with accepted academic practice. No use, distribution or reproduction is permitted which does not comply with these terms.
*Correspondence: Aoshuang Xu, eGFzX21lZGljaW5lQDE2My5jb20=; Lin Lu, bGxlZHUyMDE0QDE2My5jb20=
†These authors have contributed equally to this work
Disclaimer: All claims expressed in this article are solely those of the authors and do not necessarily represent those of their affiliated organizations, or those of the publisher, the editors and the reviewers. Any product that may be evaluated in this article or claim that may be made by its manufacturer is not guaranteed or endorsed by the publisher.
Research integrity at Frontiers
Learn more about the work of our research integrity team to safeguard the quality of each article we publish.