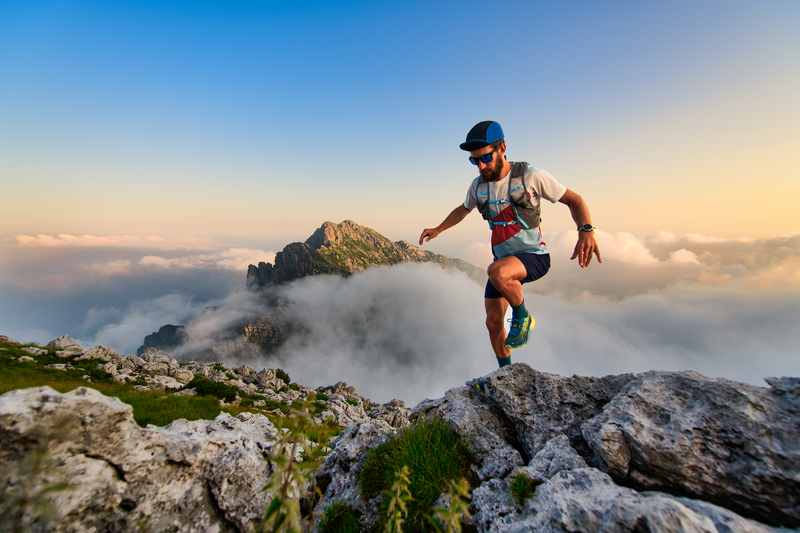
95% of researchers rate our articles as excellent or good
Learn more about the work of our research integrity team to safeguard the quality of each article we publish.
Find out more
ORIGINAL RESEARCH article
Front. Cell Dev. Biol. , 18 March 2025
Sec. Molecular and Cellular Pathology
Volume 13 - 2025 | https://doi.org/10.3389/fcell.2025.1569533
This article is part of the Research Topic Molecular Basis of Craniofacial Abnormalities View all 6 articles
Craniofacial abnormalities are among the most prevalent congenital defects, significantly affecting appearance, function, and quality of life. While the role of genetic mutations in craniofacial malformations is recognized, the underlying molecular mechanisms remain poorly understood. In this study, we investigate the role of p75 neurotrophin receptor (p75NTR) in craniofacial development by comparing wild-type (p75NTR+/+) mice against p75NTR-deficient (p75NTR−/−) knockout mice. We employed histology, micro-CT surface distance, volumetric analysis, and geometric morphometric analysis to assess craniofacial development and growth. On postnatal day 7 (P7), p75NTR−/− mice exhibited reduced skull length compared to wild-type controls. By P28, micro-CT analysis revealed significant reductions in calvarial bone volume and trabecular bone thickness in p75NTR−/− mice. Geometric morphometric analysis identified significant shape alterations in the nasal, parietal, and occipital regions, with p75NTR−/− mice showing a shortened cranium and tapered nasal bone morphology. These findings highlight the critical role of p75NTR in regulating postnatal craniofacial development. Disruption of p75NTR signaling impairs both the growth and morphological integrity of craniofacial structures, which may contribute to the pathogenesis of congenital craniofacial abnormalities. In the future, a better understanding of the molecular mechanisms through which p75NTR mediates craniofacial development may offer valuable insights for future targeted therapeutic strategies for craniofacial defects.
Craniofacial abnormalities and malformations are a group of congenital anomalies that represent a third of all birth defects and affect around 1 in every 100 newborns, significantly impacting an individual’s appearance, speech, mastication, and occlusion (Corsello and Giuffrè, 2012; Twigg and Wilkie, 2015; Ueharu and Mishina, 2023). Despite their high prevalence and substantial clinical burden, the factors driving craniofacial development and the etiology of craniofacial abnormalities remain poorly understood. These conditions frequently necessitate multidisciplinary interventions and invasive surgeries that reduce the quality of life without addressing the underlying molecular causes (Neben et al., 2016; Opriş et al., 2022; Manlove et al., 2020; Shaw, 2004).
Skeletal dysplasias are a group of genetic disorders that disrupt the development, growth, and homeostasis of bones and cartilage, constituting a significant subset of congenital disorders. With over 450 recognized conditions, these disorders vary widely in severity, from mild abnormalities to life-threatening complications (Neben et al., 2016; Warman et al., 2011). Craniofacial defects associated with skeletal dysplasia arise from complex interactions among transcription factors, growth factors, and receptors, which orchestrate the genetic patterning and morphogenesis of craniofacial structures (Neben et al., 2016; Neben and Merrill, 2015).
Craniofacial structures predominantly originate from cranial neural crest cells, which differentiate into various cell types including osteoblast, chondrocytes, adipocytes, melanocytes, neural cells, and others (Bhatt et al., 2013; Achilleos and Trainor, 2012). Multiple signaling pathways are critical regulators of cranial neural crest cells (Pereur and Dambroise, 2024; Nuckolls et al., 1999; Xu et al., 2023; Sun et al., 2024). Among these, the fibroblast growth factor (FGF) signaling pathway is a key regulator of craniofacial development and calvarial bone formation. FGF signaling orchestrates epithelial-mesenchymal interactions essential for the development of various craniofacial structures, (Prochazkova et al., 2018; Teshima et al., 2016; Klein et al., 2008). Its functions are highly tissue-specific, utilizing diverse mechanisms to regulate development.
The FGF pathway also has a well characterized role in calvarial bone, regulating osteogenesis, chondrogenic proliferation and maintaining sutures, with dysregulation of FGF receptors 1-3 (FGFR1, FGFR2, FGFR3) being known to cause calvarial abnormalities in murine models (Neben et al., 2016; Rice et al., 2000; Richbourg et al., 2024; Marcucio et al., 2019). Dysregulation of FGFR3, which reduces chondrocyte proliferation, is associated with conditions such as achondroplasia and thanatophoric dysplasia, both characterized by distinct craniofacial anomalies (Neben et al., 2016; Rousseau et al., 1994; Shiang et al., 1994; Henderson et al., 2000). Molecular studies have demonstrated that the severity of these skeletal dysplasias correlates with the degree of FGFR3 signaling activation through the MAPK or STAT1 pathways (Laederich and Horton, 2010; Krejci et al., 2008). Consequently, inhibiting these over-activated pathways with statins has shown promise as a therapeutic strategy, as statin treatment successfully corrected bone development abnormalities in mouse models (Yamashita et al., 2014). These results highlight the critical role of mouse models in driving progress toward effective therapies for craniofacial disorders linked to congenital skeletal abnormalities.
Signaling pathways are also able to crosstalk with one another to influence craniofacial development. Sonic hedgehog (SHH) signaling pathway has a characterized function regulating mitotic activity and spatial organization within midfacial growth zones (Young et al., 2010). However, when the SHH and FGF pathways interact together they gain new functions, becoming capable of regulating the migration, survival and maintenance of neural crest progenitors (Prochazka et al., 2015; da Costa et al., 2018). Another example of pathway crosstalk involves the bone morphogenetic protein (BMP) signaling pathway, which often functions in opposition to FGF signaling. BMP signaling is known to attenuate FGF signaling in calvarial development (Ueharu and Mishina, 2023; Maruyama et al., 2010). Further studies have shown that interplay between the BMP and FGF signaling pathways help regulate calvarial bone development and injury repair, emphasizing the complex nature of calvarial development (Maruyama et al., 2010; Maruyama et al., 2017).
BMP signaling is also known for its role in regulating proliferation, differentiation, apoptosis, and migration of cranial neural crest cells. Defects in BMP signaling have been linked to craniofacial skeletal deformities including craniosynostosis in murine models, emphasizing its role in calvarial bone development (Ye et al., 2022; Mimura et al., 2016; Wang et al., 2024; Ueharu and Mishina, 2023). Specifically, BMP4 plays a significant role in calvarial sutures, where it has been shown to induce expression of Msx genes, key regulators of osteogenesis essential for bone formation and development (Kim et al., 1998). Interestingly, reduced BMP4 activity has been associated with increased expression of the p75 neurotrophin receptor (p75NTR), suggesting a functional relationship between BMP4 signaling and p75NTR in the calvaria, presenting a novel area for further research (Mimura et al., 2016).
p75NTR is a membrane spanning protein in the tumor necrosis factor receptor family that can bind any neurotrophin (NGF, BDNF, NT-3, NT-4) with low-affinity (Meeker and Williams, 2015). Among these potential ligands, p75NTR is best known for its role as an NGF receptor (Liu et al., 2022). As a neurotrophin receptor, p75NTR has a well characterized role in the nervous system mediating neuronal cell survival, regulating synaptic transmission/axial elongation, and acting as a potential marker gene for various neurodegenerative diseases such as Alzheimer’s, schizophrenia, and dementia (Jourdi et al., 2024; Dechant and Barde, 2002; Liu et al., 2022; Beattie et al., 2002).
Interestingly, p75NTR (also known as CD271) is widely expressed on the surfaces of migrating neural crest cells throughout their development (Tomellini et al., 2014). It has been established as one of the most reliable surface markers to isolate neural crest cell progenitors and bone marrow stem cells (Álvarez-Viejo et al., 2015; Bühring et al., 2007; Quirici et al., 2002; Tomellini et al., 2014). In our study utilizing three distinct sets of surface markers (CD51/CD140a, CD271, and STRO-1/CD146) to isolate putative stem cell populations from primary craniofacial tissue cultures, CD271 was merged as the most effective single marker for identifying progenitor populations (Alvarez et al., 2015a).
Emerging evidence highlights the critical role of p75NTR in bone development. Our previous studies demonstrated that p75NTR positive mesenchymal stem cells (MSCs) from various craniofacial tissues exhibit the highest osteogenic potential with strong upregulation of key osteogenic markers such as DLX5, RUNX2, and BGLAP (Alvarez et al., 2015a; Alvarez et al., 2015b). Additionally, our lab has further characterized the role of p75NTR in the osteogenesis of craniofacial MSCs both in vitro and in vivo, revealing that Dlx5, a master osteogenic gene, is epigenetically activated by Lysine Demethylase 4B (KDM4B) via p75NTR-mediated NGF signaling (Liu et al., 2022). These findings were confirmed in vivo using calvarial defect regeneration mouse model. (Liu et al., 2022). Recent studies by Wang et al. also showed that p75NTR knockout mice have reduced alveolar bone mass and less osteogenic differentiation of ectomesenchymal stem cells (Wang et al., 2020).
Despite these breakthroughs, the role of p75NTR in craniofacial bone development remains uncharacterized. In our study, we aimed to elucidate the impact p75NTR has on craniofacial growth and morphogenesis by deleting p75NTR in mice and comparing wild-type (p75NTR+/+) and p75NTR-deficient (p75NTR−/−) knockout mice. Using whole-mount skeletal staining and micro-CT imaging, we assessed skull length, bone volume, bone density, and the microarchitecture of the craniofacial skeleton. Geometric morphometric analysis then allowed us to examine shape differences in the calvaria, comparing p75NTR+/+ and p75NTR−/− mice. Our findings revealed that p75NTR is critical for calvarial integrity during postnatal development, providing insight into the underlying mechanism behind craniofacial morphogenesis and opening potential pathways towards addressing craniofacial abnormalities in the future.
Beyond its well-established role in neuronal survival, p75NTR has emerged as a potential key regulator of osteogenic differentiation and bone development, prompting us to examine how p75NTR deficiencies may influence postnatal craniofacial bone development. p75NTR−/− mice were born without complications and had no statistically significant differences in size weight, or growth compared to p75NTR+/+ littermates at P0. Whole-mount skeletal staining revealed no craniofacial growth defects in neonatal p75NTR−/− mice at P0 (Figures 1A, B). However, as early as 7 days after birth (P7), whole-mount skeletal staining demonstrated a notably reduced skull length in p75NTR−/− mice compared with p75NTR+/+ mice. (Figures 1A, B). Similarly, micro-CT analysis of P28 animals revealed a significant decrease in calvarial bone volume in p75NTR−/− mice compared to p75NTR+/+ littermates (Figure 1C). Further analysis of trabecular bone microarchitecture in the region below the mandibular first molars demonstrated that the loss of p75NTR resulted in decrease in bone volume in p75NTR−/− mice compared to p75NTR+/+ mice at P28 with significantly reduced thickness of trabecular bone (Tb.Th.) and cortical bone (Cb.Th.), and increased trabecular spacing (Tb.Sp.) in p75NTR−/− mice compared to p75NTR+/+ mice (Figure 1D). Although calvarial bone mineral density (BMD) values of the frontal bone and parietal bone (Figures 1E, F) were lower in p75NTR−/− mice, the differences were not statistically significant. Collectively, these results suggest that p75NTR plays a critical role in postnatal craniofacial bone development and growth.
Figure 1. p75NTR deficiency leads to a generalized reduction in postnatal craniofacial bone formation. (A) Whole-mount skeletal staining of skulls with alcian blue and alizarin red S. (B) Comparison of skull length at P0 and P7 are plotted. n = 4 (C) total skull volume at P28 is illustrated and plotted. n = 9–10 (D) Mandibular bone volume fraction (BV/TV), trabecular bone thickness (Tb.Th.) and, cortical bone thickness (Cb.Th.), and trabecular spacing (Tb.Sp.) are illustrated and plotted. n = 5–8 (E, F) ROI for bone mineral density (BMD) analysis is indicated with a square on the frontal and parietal bones. BMD values are plotted. n = 7–12 Bar graphs show mean ± standard deviation. Statistical significances are indicated, n.s non-significant, *p ≤ 0.05, **p ≤ 0.01, ***p ≤ 0.001, ****p ≤ 0.0001.
Side-by-side visual comparison of p75NTR+/+ and p75NTR−/− P28 mouse skulls revealed a generalized reduction in calvarial skeletal dimensions in all three views in p75NTR−/− mice (Figure 2A). To further analyze the differences, we performed distance analysis using landmarks indicated in Figure 2B. These landmarks are selected to represent the overall morphology of the skull. Furthermore, these landmarks are located at transition points or boundaries between different bones so that they could be registered with high reproducibility (Kawakami and Yamamura, 2008; Liang et al., 2023). The analysis measured ten 3-dimensional (3D) surface and linear distances in the calvaria, providing detailed insights into the observed differences (Figure 2B). All ten measurements were reduced in p75NTR−/− mice compared to p75NTR+/+ mice with seven measurements showing statistically significant differences, including cranial length, skull width, middle skull height, anterior skull height, maxillary length, inter-zygomatic distance, and inter-molar maxillary distance. These dimensions were significantly smaller in p75NTR−/− mice compared to p75NTR+/+ littermates (Figure 2C). In contrast, decreases in inter-orbitary, inter-nasal, and bi-temporal surface distances were not statistically significant.
Figure 2. Length analysis shows cranium size reduction due to p75NTR deficiency. (A) Representative skull rendering for visual comparison. (B) 18 landmarks were placed on the cranium and used to access the surface and linear distances of 10 measurements. (C) Comparison of distances are illustrated. n = 7–10. Bar graphs show mean ± standard deviation. Statistical significances are indicated, *p ≤ 0.05, **p ≤ 0.01.
Next, we performed 3D segmentation and volumetric analysis of calvarial bones. This analysis revealed a significant 15%–20% decrease in the volumes of nasal, frontal, interparietal, parietal, and occipital bones (Figures 3A, B). In conclusion, these findings demonstrate a significant overall and specific volumetric reduction in p75NTR−/− mice, suggesting that p75NTR has a direct role in the developmental growth of the craniofacial bones.
Figure 3. Volumetric analysis shows cranium volumetric reduction due to p75NTR deficiency. (A) three-dimensional skull bone segmentation of nasal (blue), frontal (purple), parietal (orange), interparietal (green), and occipital (yellow). The three-dimensional coordinate boxes are in units of millimeters (mm). (B) bone segment volume and statistical significance in length difference is illustrated. n = 8–10. Bar graphs show mean ± standard deviation. Statistical significances are indicated, *p ≤ 0.05, **p ≤ 0.01, ***p ≤ 0.001, ****p ≤ 0.0001.
While size and volume offer valuable quantitative insights into craniofacial growth, morphology is critical for capturing the intricacies and complexities of craniofacial development (Hassan et al., 2020; Young et al., 2016). Notably, size reductions were not uniformly observed across all distance measurements or volume data (Figures 2, 3), necessitating us to further investigate the role of p75NTR in craniofacial morphogenesis.
Here, we utilized the geometric morphometric analysis technique to compare and quantify the calvarial shapes of p75NTR+/+ and p75NTR−/− mice in a precise approach. 41 landmarks were placed on the cranium for geometric morphometric analysis (Figure 4A; Table 1) (Shen et al., 2013; Richtsmeier et al., 2000; Hill et al., 2009; Willmore et al., 2009). Scatter plots of PC 1 and PC 2 showed distinct clusters for the p75NTR+/+ and p75NTR−/− mouse crania (Figure 4B). For cranium, we observed a clear separation of the 95%-confidence-ellipses along the PC 1 axis, accounting for 28.1% of the total variance. The Procrustes MANOVA conducted on shape data and centroid size data demonstrates significant differences between p75NTR+/+ and p75NTR−/− mouse crania (p < 0.0001).
Figure 4. GM analysis shows morphological change due to p75NTR deficiency. (A) Skeletal Landmarks used for GM analysis. (B) Scatter plots by the two first Principal Components show differences in shape between the p75NTR+/+ (light blue dots) and p75NTR−/− (orange dots) P28 mice cranium. Black arrows indicate anatomical positions with the most prominent morphological differences between the p75NTR+/+ and p75NTR−/− P28 mice cranium. Light blue and orange shaded ellipses are the 95% confidence ellipses for p75NTR+/+ (light blue ellipse) and p75NTR−/− (orange ellipse) P28 mice cranium. (C) Wireframes showing superimposition of p75NTR+/+ (blue) and p75NTR−/− (cyan) 4-week mice cranium demonstrate the shape changes that are associated with PC 1 and PC 2. Wireframes were generated from landmarks indicated in geometric morphometric analysis to provide a visual representation and demonstrate shape differences between p75NTR+/+ and p75NTR−/− groups. Based on wireframe analysis, p75NTR−/− mice exhibited reduced occipital bone size and demonstrated more angular and tapered nasal bone morphology. (D) The displacement heatmap (left) shows morphological differences distributed in the nasal, parietal, and inter-parietal bone regions. Arrows (right) indicate the direction of change, representing the difference between p75NTR+/+ and p75NTR−/− samples. n = 5–7.
Wireframe and displacement heatmap reveal morphological differences primarily distributed across the nasal, parietal, and interparietal bone regions (Figures 4C, D). In particular, PC1 indicates that the most significant shape variation is located in the caudal region of the cranium. In p75NTR−/− mice, the cranium is shorter overall, driven by shape differences associated with the shortening and narrowing of the occipital and interparietal bones. Additionally, PC2 highlights a ventral bending of the nasal-palatal portion of the cranium in p75NTR−/− mice compared to p75NTR+/+ mice, despite no apparent narrowing or shortening of the nasal bones.
In summary, geometric morphometric analysis demonstrates that p75NTR deficiency led to significant overall morphological alterations. These findings underscore the direct involvement of p75NTR in cranial morphogenesis.
Our findings highlight the critical role of p75NTR in craniofacial bone development, growth, and morphogenesis. By integrating histology, surface distance and linear length analysis, 3D segmentation, volumetric measurements, and geometric morphometric analysis, we demonstrated how p75NTR deficiency leads to significant postnatal deficits in craniofacial development. Although p75NTR-deficient mice were born without notable differences in size, weight, or craniofacial structure compared to p75NTR+/+ littermates, abnormalities became evident shortly after birth, emphasizing its essential role in postnatal growth and morphogenesis rather than embryonic development.
Craniofacial development is a uniquely complex process driven by region-specific and mosaic growth patterns requiring precise spatial and temporal coordination (Feng et al., 2009; Roth et al., 2021). Unlike the predominantly linear growth of long bones, craniofacial structures develop through intricate, shape-dependent mechanisms (Breur et al., 1991; Geetha-Loganathan et al., 2022; Kronenberg, 2003). Variations in these processes are evident in craniofacial syndromes, where growth disruptions affect multiple dimensions of the skull, underscoring the critical role of shape in both function and aesthetics (Bartzela et al., 2017; Geetha-Loganathan et al., 2022). Consequently, advanced shape analysis is essential for understanding these mechanisms and the contributions of p75NTR to craniofacial development (Matthews et al., 2022).
In this study, geometric morphometric analysis proved invaluable for quantifying craniofacial shape changes caused by p75NTR loss-of-function. Unlike traditional morphometric methods focused solely on size, geometric morphometric analysis captures subtle, localized shape variations by analyzing spatial relationships between craniofacial landmarks (Agbolade et al., 2020; Rutland et al., 2021; Hallgrimsson et al., 2015; Hassan et al., 2020; Young et al., 2016). Our findings revealed significant alterations in the nasal, interparietal, and occipital regions of p75NTR-deficient mice, highlighting the role of p75-mediated signaling in craniofacial symmetry and proportionality. Furthermore, the clustering along principal component axes demonstrated clear differences in overall craniofacial shape between p75NTR+/+ and p75NTR−/− mice. Together with volumetric and length analysis, our results suggest that p75NTR plays an essential role not only in overall bone size but also in cranial shape and structural integrity.
The C57BL/6J mouse used in this study exhibits transverse and vertical growth of its craniofacial skeleton until 4 weeks of age (Vora et al., 2015). Therefore, studying the p75NTR’s involvement in craniofacial development at 4 weeks (P28) provides insights into its function during early postnatal development. While significant craniofacial defects were observed during this period, the long-term effects of p75NTR deficiency remain unclear. Future studies should examine older mice, such as 3-month-olds and 12-month-olds, after growth completion to determine whether craniofacial defects persist or worsen over time or whether compensatory mechanisms mitigate their effects.
Another avenue for future research is using inducible tissue-specific conditional knockout mice rather than whole-body knockouts to better delineate the role of p75NTR in craniofacial development (Hall et al., 2009). This approach allows for targeted deletion of p75NTR in specific cell types, such as osteoblasts or craniofacial progenitor cells, at controlled developmental stages. This strategy would minimize systemic confounding factors by isolating tissue-specific effects and offer more precise insights into how p75NTR regulates craniofacial growth and form. Furthermore, while our study focused on genotype-dependent effects, the sex-specific differences in skeletal development may contribute to craniofacial variation in p75NTR deficient animals. Future studies should include sex-stratified analyses to further elucidate potential interactions between genetic factors and sex-specific growth patterns.
Our previous studies have demonstrated that NGF promotes osteogenic differentiation of human craniofacial MSCs via p75NTR (Liu et al., 2022). This process involves downstream activation of the JNK cascade and the identification of KDM4B as a key epigenetic regulator of NGF-p75-mediated osteogenesis (Liu et al., 2022). However, the specific ligands and signaling pathways regulating craniofacial bone development through p75NTR remain to be fully elucidated. Identifying such ligands, particularly NGF, and further characterizing their molecular interactions would provide critical insights into the mechanisms underlying craniofacial development and set the stage for therapeutic applications.
Furthermore, histological and molecular analyses are needed to elucidate the specific stages of bone formation regulated by p75NTR. Investigating gene and protein expression markers such as Runx2, Sox9, and Col2a1 could provide deeper insights into the molecular mechanisms involved. Future research combining geometric morphometric analysis, volumetric analysis, histology, and molecular profiling across different craniofacial and endochondral bone tissues will enhance our understanding of p75NTR’s role in skeletal development.
From a clinical perspective, a deeper understanding of p75NTR’s role in craniofacial development has the potential to lead to impactful therapeutic applications. BMP4, a key regulator of cranial neural crest cells and osteogenesis, appears to influence p75NTR expression (Mimura et al., 2016). BMP2, a closely related protein, is already used clinically to promote bone remodeling and is a viable alternative to bone grafts (Halloran et al., 2020). This suggests that targeting BMP4 or downstream p75NTR-mediated signaling could yield new therapeutic strategies. However, further research is necessary to realize these clinical possibilities fully.
In conclusion, p75NTR deficiency leads to significant morphological alterations and reductions in craniofacial bone size, highlighting the critical role of p75NTR in craniofacial development. The combined use of geometric morphometric and volumetric analyses proved to be a robust approach for functional genetic studies and developmental research. These methods complement each other, with geometric morphometric analysis providing insights into overall shape variation and volumetric analysis supplementing size-related information. This integrative phenotypic analysis serves as a strong foundation for future studies to elucidate p75NTR-related pathways in craniofacial dysmorphism and developmental delays, with potential applications in related research fields.
p75NTR−/− and p75NTR+/+ mice were purchased from the Jackson Laboratory (Bar Harbor, ME) and housed inside the Laboratory Animal Resource Center facilities at the University of California, San Francisco (UCSF). The Animal Research Committee (ARC) at UCSF approved and regulated all protocols used in animal experiments. The mice were bred to produce heterozygous females and males, which were then used to produce mice with different genotypes for this study. Both sexes were included for all analyses.
The skin and internal organs of P0 and P7 mice were removed for morphological analysis. After overnight fixation in 95% ethanol at room temperature, the mice were stained in Alcian blue solution (0.03% Alcian blue in 20% acetic acid/ethanol) overnight. After several hours in 70% and 95% ethanol, they were transferred to a 1% potassium hydroxide (KOH) solution for 2 h to digest and clear the tissue. After staining in Alizarin red S solution for 4 h (0.005% Alizarin red in 1% KOH), skeletons were cleared in a series of 1% KOH/25% glycerol, 0.5% KOH/50% glycerol and stored in 0.5% KOH/70% glycerol at 4°C. Images of the stained skeletons and cartilages were taken under a stereomicroscope (MVX10; Olympus, Tokyo, Japan).
After 4% paraformaldehyde fixation overnight, P28 skulls of the mice were subjected to micro-CT scanning. Microarchitectures of the cortical bone of the skulls were measured by Skyscan 1,275 μCT (Bruker, Kontich, Belgium) as previously described (Hsu et al., 2016). Bones were placed vertically in a scanning holder and scanned at the following settings: 10 μm resolution, 60 kVp energy, 166 μA intensity, 0.3-degree rotation, and an integration time of 200 ms. Two-dimensional (2D) slices from each skull were combined using NRecon and CTAn/CTVol programs (Bruker) to form a 3D reconstruction and quantify BV/TV (%), Tb.N. (mm-1), Tb.Th. (mm), and Tb.Sp. (mm) to determine the trabecular bone microarchitecture in the region below the mandibular first molar. To analyze bone mineral density (BMD), three regions of interest (ROI) on craniofacial bone tissues were used as previously described (Wei et al., 2017). All ROIs were set to 0.4 mm × 0.4 mm in size (Figures 1E,F). The first ROI was set on the frontal bone, which is located 1.5 mm anterior to the intersection point of the coronal suture and sagittal suture and 1 mm lateral to the posterior frontal suture. The second area to measure BMD of parietal bone was located 1.5 mm posterior to the intersection point of the coronal suture and sagittal suture and 1.5 mm lateral to the sagittal suture. The thickness of the ROIs was 0.4 mm to generate BMD by using 40 slices from posterior to the initial plane.
We imported Micro-CT data into 3D Slicer software (slicer.org, Massachusetts, United States) and manually segmented the cranium bone. We utilized a constant rendering threshold of 1471 HU to 9800 HU to generate isosurfaces for each anatomical region. We then placed 18 landmarks to encapsulate the cranium morphology. Using these landmarks, we accessed surface-outline distances and straight-line distances. Surface-outline spans across the skull and extrapolates the 3D size information; in comparison, the straight-line distances analyze the distance between two landmarks in a 2D manner. Seven 4-week-old p75NTR+/+ mice and five 4-week-old p75NTR−/− mice were considered for the evaluation.
We imported Micro-CT data into 3D Slicer software (slicer.org, Massachusetts, United States), and we manually segmented the nasal, interparietal, parietal, occipital, and frontal bones of the skull. We utilized a constant rendering threshold of 1471 HU to 9800 HU to generate isosurfaces for each anatomical region. Ten 4-week-old p75NTR+/+ mice and nine 4-week-old p75NTR−/− mice were considered for the evaluation. We then calculated the volume of each bone segment and evaluated the statistical difference between the p75NTR+/+ and p75NTR−/− groups.
Geometric morphometric analysis was used to capture morphological shape variation between p75NTR+/+ and p75NTR−/− mice. This method utilizes landmark-based data, allowing for more detailed analysis of shape differences that traditional linear measurements may overlook (Hallgrimsson et al., 2015; Hassan et al., 2020).
First, the Micro-CT data was imported into the 3D slicer software (slicer.org, Massachusetts, United States), and the cranium was manually segmented using the threshold parameters: 1,471 to 9800 HU. From these segmentations, isosurfaces were generated, and 41 landmarks were placed (Table 1). The landmark data was imported into the MorphoJ (ver. 2.0, Apache License, Klingenberg, C.P. 2011) program, and shape coordinate data were generated from the raw data using a Procrustes superimposition. Generalized Procrustes Analysis (GPA) was used to remove the effect of orientations, align configurations to a common centroid, and eliminate scale differences between the different samples. Principal component analysis (PCA) was used to identify shape differences and confirm the distinction between p75NTR+/+ and p75NTR−/− mice. Outlier data points with outstanding distances from the cluster centers were removed.
We analyzed Procrustes shape data using multivariate analysis of variance (MANOVA). For the bivariate data sets, we illustrated data as means with their corresponding standard deviation. We then evaluated our conventional length and volumetric data by unpaired Student’s t-test. A p-value smaller or equal to 0.05 was used to indicate statistical significance.
The original contributions presented in the study are included in the article/supplementary material, further inquiries can be directed to the corresponding author.
The animal study was approved by the UCSF Office of Research Institutional Animal Care and Use Program under the approval number AN195822. The study was conducted in accordance with the local legislation and institutional requirements.
BZ: Writing–original draft, Writing–review and editing, Data curation, Formal Analysis, Investigation, Methodology. JS: Data curation, Formal Analysis, Investigation, Writing–review and editing, Methodology. YZ: Data curation, Formal Analysis, Investigation, Methodology, Writing–review and editing, Conceptualization. EY: Writing–review and editing, Project administration, Visualization, Writing–original draft. CK-W: Project administration, Visualization, Writing–review and editing, Data curation, Formal Analysis. IC: Data curation, Investigation, Writing–review and editing. JY: Writing–review and editing, Investigation. IL-N: Writing–review and editing, Visualization. NY: Conceptualization, Methodology, Writing–review and editing. RK: Resources, Writing–review and editing. OK: Conceptualization, Resources, Writing–review and editing. CH: Conceptualization, Funding acquisition, Resources, Supervision, Writing–review and editing.
The author(s) declare that financial support was received for the research, authorship, and/or publication of this article. The authors declare that the UCSF Chancellor's Fund was received for the research, authorship, and/or publication of this article.
The authors declare that the research was conducted in the absence of any commercial or financial relationships that could be construed as a potential conflict of interest.
The author(s) declare that no Generative AI was used in the creation of this manuscript.
All claims expressed in this article are solely those of the authors and do not necessarily represent those of their affiliated organizations, or those of the publisher, the editors and the reviewers. Any product that may be evaluated in this article, or claim that may be made by its manufacturer, is not guaranteed or endorsed by the publisher.
Achilleos, A., and Trainor, P. A. (2012). Neural crest stem cells: discovery, properties and potential for therapy. Cell Res. 22, 288–304. doi:10.1038/cr.2012.11
Agbolade, O., Nazri, A., Yaakob, R., Ghani, A. A., and Cheah, Y. K. (2020). Morphometric approach to 3D soft-tissue craniofacial analysis and classification of ethnicity, sex, and age. PLoS One 15, e0228402. doi:10.1371/journal.pone.0228402
Alvarez, R., Lee, H.-L., Hong, C., and Wang, C.-Y. (2015a). Single CD271 marker isolates mesenchymal stem cells from human dental pulp. Int. J. Oral Sci. 7, 205–212. doi:10.1038/ijos.2015.29
Alvarez, R., Lee, H.-L., Wang, C.-Y., and Hong, C. (2015b). Characterization of the osteogenic potential of mesenchymal stem cells from human periodontal ligament based on cell surface markers. Int. J. Oral Sci. 7, 213–219. doi:10.1038/ijos.2015.42
Álvarez-Viejo, M., Menéndez-Menéndez, Y., and Otero-Hernández, J. (2015). CD271 as a marker to identify mesenchymal stem cells from diverse sources before culture. World J. Stem Cells 7, 470–476. doi:10.4252/wjsc.v7.i2.470
Bartzela, T. N., Carels, C., and Maltha, J. C. (2017). Update on 13 syndromes affecting craniofacial and dental structures. Front. Physiol. 8, 1038. doi:10.3389/fphys.2017.01038
Beattie, M. S., Harrington, A. W., Lee, R., Kim, J. Y., Boyce, S. L., Longo, F. M., et al. (2002). ProNGF induces p75-mediated death of oligodendrocytes following spinal cord injury. Neuron 36, 375–386. doi:10.1016/s0896-6273(02)01005-x
Bhatt, S., Diaz, R., and Trainor, P. A. (2013). Signals and switches in Mammalian neural crest cell differentiation. Cold Spring Harb. Perspect. Biol. 5, a008326. doi:10.1101/cshperspect.a008326
Breur, G. J., Vanenkevort, B. A., Farnum, C. E., and Wilsman, N. J. (1991). Linear relationship between the volume of hypertrophic chondrocytes and the rate of longitudinal bone growth in growth plates. J. Orthop. Res. 9, 348–359. doi:10.1002/jor.1100090306
Bühring, H.-J., Battula, V. L., Treml, S., Schewe, B., Kanz, L., and Vogel, W. (2007). Novel markers for the prospective isolation of human MSC. Ann. N. Y. Acad. Sci. 1106, 262–271. doi:10.1196/annals.1392.000
Corsello, G., and Giuffrè, M. (2012). Congenital malformations. J. Matern. Fetal Neonatal Med. 25 (Suppl. 1), 25–29. doi:10.3109/14767058.2012.664943
da Costa, M. C., Trentin, A. G., and Calloni, G. W. (2018). FGF8 and Shh promote the survival and maintenance of multipotent neural crest progenitors. Mech. Dev. 154, 251–258. doi:10.1016/j.mod.2018.07.012
Dechant, G., and Barde, Y.-A. (2002). The neurotrophin receptor p75(NTR): novel functions and implications for diseases of the nervous system. Nat. Neurosci. 5, 1131–1136. doi:10.1038/nn1102-1131
Feng, W., Leach, S. M., Tipney, H., Phang, T., Geraci, M., Spritz, R. A., et al. (2009). Spatial and temporal analysis of gene expression during growth and fusion of the mouse facial prominences. PLoS One 4, e8066. doi:10.1371/journal.pone.0008066
Geetha-Loganathan, P., Abramyan, J., and Buchtová, M. (2022). Editorial: cellular mechanisms during normal and abnormal craniofacial development. Front. Cell Dev. Biol. 10, 872038. doi:10.3389/fcell.2022.872038
Hall, B., Limaye, A., and Kulkarni, A. B. (2009). Overview: generation of gene knockout mice. Curr. Protoc. Cell Biol. 44 (1), 19.12.11–19.12.17. doi:10.1002/0471143030.cb1912s44
Hallgrimsson, B., Percival, C. J., Green, R., Young, N. M., Mio, W., and Marcucio, R. (2015). “Chapter twenty - morphometrics, 3D imaging, and craniofacial development,” in Current topics in developmental biology. Editor Y. Chai (New York: Academic Press), 561–597. doi:10.1016/bs.ctdb.2015.09.003
Halloran, D., Durbano, H. W., and Nohe, A. (2020). Bone morphogenetic protein-2 in development and bone homeostasis. J. Dev. Biol. 8, 19. doi:10.3390/jdb8030019
Hassan, M. G., Kaler, H., Zhang, B., Cox, T. C., Young, N., and Jheon, A. H. (2020). Effects of multi-generational soft diet consumption on mouse craniofacial morphology. Front. Physiol. 11, 783. doi:10.3389/fphys.2020.00783
Henderson, J. E., Naski, M. C., Aarts, M. M., Wang, D., Cheng, L., Goltzman, D., et al. (2000). Expression of FGFR3 with the G380R achondroplasia mutation inhibits proliferation and maturation of CFK2 chondrocytic cells. J. Bone Min. Res. 15, 155–165. doi:10.1359/jbmr.2000.15.1.155
Hill, C. A., Sussan, T. E., Reeves, R. H., and Richtsmeier, J. T. (2009). Complex contributions of Ets2 to craniofacial and thymus phenotypes of trisomic “Down syndrome” mice. Am. J. Med. Genet. Part A 149A, 2158–2165. doi:10.1002/ajmg.a.33012
Hsu, P.-Y., Tsai, M.-T., Wang, S.-P., Chen, Y.-J., Wu, J., and Hsu, J.-T. (2016). Cortical bone morphological and trabecular bone microarchitectural changes in the mandible and femoral neck of ovariectomized rats. PLoS One 11, e0154367. doi:10.1371/journal.pone.0154367
Jourdi, G., Fleury, S., Boukhatem, I., and Lordkipanidzé, M. (2024). Soluble p75 neurotrophic receptor as a reliable biomarker in neurodegenerative diseases: what is the evidence? Neural Regen. Res. 19, 536–541. doi:10.4103/1673-5374.380873
Kawakami, M., and Yamamura, K. (2008). Cranial bone morphometric study among mouse strains. BMC Evol Biol 8, 73. doi:10.1186/1471-2148-8-73
Kim, H.-J., Rice, D. P. C., Kettunen, P. J., and Thesleff, I. (1998). FGF-BMP- and Shh-mediated signalling pathways in the regulation of cranial suture morphogenesis and calvarial bone development. Development 125, 1241–1251. doi:10.1242/dev.125.7.1241
Klein, O. D., Lyons, D. B., Balooch, G., Marshall, G. W., Basson, M. A., Peterka, M., et al. (2008). An FGF signaling loop sustains the generation of differentiated progeny from stem cells in mouse incisors. Development 135, 377–385. doi:10.1242/dev.015081
Krejci, P., Salazar, L., Kashiwada, T. A., Chlebova, K., Salasova, A., Thompson, L. M., et al. (2008). Analysis of STAT1 activation by six FGFR3 mutants associated with skeletal dysplasia undermines dominant role of STAT1 in FGFR3 signaling in cartilage. PLoS One 3, e3961. doi:10.1371/journal.pone.0003961
Kronenberg, H. M. (2003). Developmental regulation of the growth plate. Nature 423, 332–336. doi:10.1038/nature01657
Laederich, M. B., and Horton, W. A. (2010). Achondroplasia: pathogenesis and implications for future treatment. Curr. Opin. Pediatr. 22, 516–523. doi:10.1097/MOP.0b013e32833b7a69
Liang, Y., Song, C., and Li, J. (2023). Morphometric analysis of the size-adjusted linear dimensions of the skull landmarks revealed craniofacial dysmorphology in Mid1-cKO mice. BMC Genomics 24, 68. doi:10.1186/s12864-023-09162-2
Liu, Z., Suh, J. S., Deng, P., Bezouglaia, O., Do, M., Mirnia, M., et al. (2022). Epigenetic regulation of NGF-mediated osteogenic differentiation in human dental mesenchymal stem cells. Stem Cells 40, 818–830. doi:10.1093/stmcls/sxac042
Manlove, A. E., Romeo, G., and Venugopalan, S. R. (2020). Craniofacial growth: current theories and influence on management. Oral Maxillofac. Surg. Clin. North Am. 32, 167–175. doi:10.1016/j.coms.2020.01.007
Marcucio, R., Hu, D., Hallgrimsson, B., and Young, N. (2019). Emergent properties of facial morphogenesis regulated by fgf signaling. FASEB J. 33, 774.18. doi:10.1096/fasebj.2019.33.1_supplement.774.18
Maruyama, T., Jiang, M., Abbott, A., Yu, H.-M. I., Huang, Q., Chrzanowska-Wodnicka, M., et al. (2017). Rap1b is an effector of Axin2 regulating crosstalk of signaling pathways during skeletal development. J. Bone Min. Res. 32, 1816–1828. doi:10.1002/jbmr.3171
Maruyama, T., Mirando, A. J., Deng, C.-X., and Hsu, W. (2010). The balance of WNT and FGF signaling influences mesenchymal stem cell fate during skeletal development. Sci. Signal 3, ra40. doi:10.1126/scisignal.2000727
Matthews, H., de Jong, G., Maal, T., and Claes, P. (2022). Static and motion facial analysis for craniofacial assessment and diagnosing diseases. Annu. Rev. Biomed. Data Sci. 5, 19–42. doi:10.1146/annurev-biodatasci-122120-111413
Meeker, R. B., and Williams, K. S. (2015). The p75 neurotrophin receptor: at the crossroad of neural repair and death. Neural Regen. Res. 10, 721–725. doi:10.4103/1673-5374.156967
Mimura, S., Suga, M., Okada, K., Kinehara, M., Nikawa, H., and Furue, M. K. (2016). Bone morphogenetic protein 4 promotes craniofacial neural crest induction from human pluripotent stem cells. Int. J. Dev. Biol. 60, 21–28. doi:10.1387/ijdb.160040mk
Neben, C. L., and Merrill, A. E. (2015). Signaling pathways in craniofacial development: insights from rare skeletal disorders. Curr. Top. Dev. Biol. 115, 493–542. doi:10.1016/bs.ctdb.2015.09.005
Neben, C. L., Roberts, R. R., Dipple, K. M., Merrill, A. E., and Klein, O. D. (2016). Modeling craniofacial and skeletal congenital birth defects to advance therapies. Hum. Mol. Genet. 25, R86–R93. doi:10.1093/hmg/ddw171
Nuckolls, G. H., Shum, L., and Slavkin, H. C. (1999). Progress toward understanding craniofacial malformations. Cleft Palate Craniofac J. 36, 12–26. doi:10.1597/1545-1569_1999_036_0012_ptucm_2.3.co_2
Opriş, D., Băciuţ, G., Bran, S., Dinu, C., Armencea, G., Opriş, H., et al. (2022). The quality of life after cleft lip and palate surgery. Med. Pharm. Rep. 95, 461–466. doi:10.15386/mpr-2472
Pereur, R., and Dambroise, E. (2024). Insights into craniofacial development and anomalies: exploring fgf signaling in zebrafish models. Curr. Osteoporos. Rep. 22, 340–352. doi:10.1007/s11914-024-00873-3
Prochazka, J., Prochazkova, M., Du, W., Spoutil, F., Tureckova, J., Hoch, R., et al. (2015). Migration of founder epithelial cells drives proper molar tooth positioning and morphogenesis. Dev. Cell 35, 713–724. doi:10.1016/j.devcel.2015.11.025
Prochazkova, M., Prochazka, J., Marangoni, P., and Klein, O. D. (2018). Bones, glands, ears and more: the multiple roles of FGF10 in craniofacial development. Front. Genet. 9, 542. doi:10.3389/fgene.2018.00542
Quirici, N., Soligo, D., Bossolasco, P., Servida, F., Lumini, C., and Deliliers, G. L. (2002). Isolation of bone marrow mesenchymal stem cells by anti-nerve growth factor receptor antibodies. Exp. Hematol. 30, 783–791. doi:10.1016/S0301-472X(02)00812-3
Rice, D. P., Aberg, T., Chan, Y., Tang, Z., Kettunen, P. J., Pakarinen, L., et al. (2000). Integration of FGF and TWIST in calvarial bone and suture development. Development 127, 1845–1855. doi:10.1242/dev.127.9.1845
Richbourg, H. A., Vidal-García, M., Brakora, K. A., Devine, J., Takenaka, R., Young, N. M., et al. (2024). Dosage-dependent effects of FGFR2 mutation on craniofacial shape and cellular dynamics of the basicranial synchondroses. Anatomical Rec. (Hoboken). doi:10.1002/ar.25398
Richtsmeier, J. T., Baxter, L. L., and Reeves, R. H. (2000). Parallels of craniofacial maldevelopment in down syndrome and Ts65Dn mice. Dev. Dyn. 217, 137–145. doi:10.1002/(SICI)1097-0177(200002)217:2<137::AID-DVDY1>3.0.CO;2-N
Roth, D. M., Bayona, F., Baddam, P., and Graf, D. (2021). Craniofacial development: neural crest in molecular embryology. Head. Neck Pathol. 15, 1–15. doi:10.1007/s12105-021-01301-z
Rousseau, J., Sikorska, H. M., Gervais, A., Bisson, G., Margaron, P., Lamoureux, G., et al. (1994). Evaluation of a 99mTc-antimyosin kit for myocardial infarct imaging. J. Nucl. Biol. Med. (1991) 38 (4 Suppl 1), 43–53.
Rutland, J. W., Bellaire, C. P., Yao, A., Arrighi-Allisan, A., Napoli, J. G., Delman, B. N., et al. (2021). The expanding role of geometric morphometrics in craniofacial surgery. J. Craniofac Surg. 32, 1104–1109. doi:10.1097/SCS.0000000000007362
Shaw, W. (2004). Global strategies to reduce the Health Care burden of craniofacial anomalies: report of WHO meetings on international collaborative research on craniofacial anomalies. Cleft Palate Craniofacial J. 41, 238–243. doi:10.1597/03-214.1
Shen, L., Ai, H., Liang, Y., Ren, X., Anthony, C. B., Goodlett, C. R., et al. (2013). Effect of prenatal alcohol exposure on bony craniofacial development: a mouse MicroCT study. Alcohol 47, 405–415. doi:10.1016/j.alcohol.2013.04.005
Shiang, R., Thompson, L. M., Zhu, Y. Z., Church, D. M., Fielder, T. J., Bocian, M., et al. (1994). Mutations in the transmembrane domain of FGFR3 cause the most common genetic form of dwarfism, achondroplasia. Cell 78, 335–342. doi:10.1016/0092-8674(94)90302-6
Sun, Q., Huang, J., Tian, J., Lv, C., Li, Y., Yu, S., et al. (2024). Key roles of Gli1 and ihh signaling in craniofacial development. Stem Cells Dev. 33, 251–261. doi:10.1089/scd.2024.0036
Teshima, T. H. N., Lourenco, S. V., and Tucker, A. S. (2016). Multiple cranial organ defects after conditionally knocking out Fgf10 in the neural crest. Front. Physiol. 7, 488. doi:10.3389/fphys.2016.00488
Tomellini, E., Lagadec, C., Polakowska, R., and Le Bourhis, X. (2014). Role of p75 neurotrophin receptor in stem cell biology: more than just a marker. Cell Mol. Life Sci. 71, 2467–2481. doi:10.1007/s00018-014-1564-9
Twigg, S. R. F., and Wilkie, A. O. M. (2015). A genetic-pathophysiological framework for craniosynostosis. Am. J. Hum. Genet. 97, 359–377. doi:10.1016/j.ajhg.2015.07.006
Ueharu, H., and Mishina, Y. (2023). BMP signaling during craniofacial development: new insights into pathological mechanisms leading to craniofacial anomalies. Front. Physiol. 14, 1170511. doi:10.3389/fphys.2023.1170511
Vora, S. R., Camci, E. D., and Cox, T. C. (2015). Postnatal ontogeny of the cranial base and craniofacial skeleton in male C57bl/6J mice: a reference standard for quantitative analysis. Front. Physiol. 6, 417. doi:10.3389/fphys.2015.00417
Wang, W., Yang, N., Wang, L., Zhu, Y., Chu, X., Xu, W., et al. (2024). The TET-Sall4-BMP regulatory axis controls craniofacial cartilage development. Cell Rep. 43, 113873. doi:10.1016/j.celrep.2024.113873
Wang, Y., Yang, K., Li, G., Liu, R., Liu, J., Li, J., et al. (2020). p75NTR-/- mice exhibit an alveolar bone loss phenotype and inhibited PI3K/Akt/β-catenin pathway. Cell Prolif. 53, e12800. doi:10.1111/cpr.12800
Warman, M. L., Cormier-Daire, V., Hall, C., Krakow, D., Lachman, R., LeMerrer, M., et al. (2011). Nosology and classification of genetic skeletal disorders: 2010 revision. Am. J. Med. Genet. Part A 155, 943–968. doi:10.1002/ajmg.a.33909
Wei, X., Thomas, N., Hatch, N. E., Hu, M., and Liu, F. (2017). Postnatal craniofacial skeletal development of female C57bl/6NCrl mice. Front. Physiol. 8, 697. doi:10.3389/fphys.2017.00697
Willmore, K. E., Roseman, C. C., Rogers, J., Cheverud, J. M., and Richtsmeier, J. T. (2009). Comparison of mandibular phenotypic and genetic integration between baboon and mouse. Evol. Biol. 36, 19–36. doi:10.1007/s11692-009-9056-9
Xu, J., Iyyanar, P. P. R., Lan, Y., and Jiang, R. (2023). Sonic hedgehog signaling in craniofacial development. Differentiation 133, 60–76. doi:10.1016/j.diff.2023.07.002
Yamashita, A., Morioka, M., Kishi, H., Kimura, T., Yahara, Y., Okada, M., et al. (2014). Statin treatment rescues FGFR3 skeletal dysplasia phenotypes. Nature 513, 507–511. doi:10.1038/nature13775
Ye, Y., Jiang, Z., Pan, Y., Yang, G., and Wang, Y. (2022). Role and mechanism of BMP4 in bone, craniofacial, and tooth development. Arch. Oral Biol. 140, 105465. doi:10.1016/j.archoralbio.2022.105465
Young, N. M., Chong, H. J., Hu, D., Hallgrímsson, B., and Marcucio, R. S. (2010). Quantitative analyses link modulation of sonic hedgehog signaling to continuous variation in facial growth and shape. Development 137, 3405–3409. doi:10.1242/dev.052340
Keywords: p75NTR, CD271, NGF, craniofacial development, craniofacial morphogenesis, calvarial development, geometric morphometric analysis
Citation: Zhao B, Suh J, Zhang Y, Yin E, Kadota-Watanabe C, Chang IW, Yaung J, Lao-Ngo I, Young NM, Kim RH, Klein OD and Hong C (2025) p75 neurotrophin receptor regulates craniofacial growth and morphology in postnatal development. Front. Cell Dev. Biol. 13:1569533. doi: 10.3389/fcell.2025.1569533
Received: 01 February 2025; Accepted: 03 March 2025;
Published: 18 March 2025.
Edited by:
Tadahiro Nagaoka, Fujita Health University, JapanReviewed by:
Lorena Maili, Stowers Institute for Medical Research, United StatesCopyright © 2025 Zhao, Suh, Zhang, Yin, Kadota-Watanabe, Chang, Yaung, Lao-Ngo, Young, Kim, Klein and Hong. This is an open-access article distributed under the terms of the Creative Commons Attribution License (CC BY). The use, distribution or reproduction in other forums is permitted, provided the original author(s) and the copyright owner(s) are credited and that the original publication in this journal is cited, in accordance with accepted academic practice. No use, distribution or reproduction is permitted which does not comply with these terms.
*Correspondence: Christine Hong, eWV1bWluLmhvbmdAdWNzZi5lZHU=
Disclaimer: All claims expressed in this article are solely those of the authors and do not necessarily represent those of their affiliated organizations, or those of the publisher, the editors and the reviewers. Any product that may be evaluated in this article or claim that may be made by its manufacturer is not guaranteed or endorsed by the publisher.
Research integrity at Frontiers
Learn more about the work of our research integrity team to safeguard the quality of each article we publish.