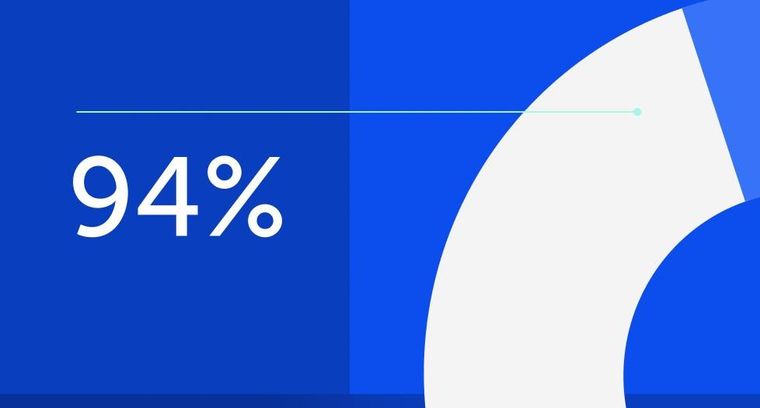
94% of researchers rate our articles as excellent or good
Learn more about the work of our research integrity team to safeguard the quality of each article we publish.
Find out more
REVIEW article
Front. Cell Dev. Biol., 26 March 2025
Sec. Embryonic Development
Volume 13 - 2025 | https://doi.org/10.3389/fcell.2025.1552988
This article is part of the Research TopicZebrafish: Bona Fide Pathophysiological Models of Human DiseasesView all 8 articles
Congenital cataracts are a leading cause of vision loss in children and can be an isolated finding or associated with systemic abnormalities. Isolated congenital cataracts are most commonly associated with pathogenic variants in one of the Crystallin genes. The α-Crystallins are small heat shock proteins that act as chaperones in the lens and other organs throughout the body to prevent protein aggregation and maintain tissue function. In contrast, the ß- and γ-Crystallins are structural proteins that are predominantly expressed in the mature lens and regulate its refractive index. However, the role of the Crystallins during lens development such that pathogenic variants result in inherited cataracts is less well-defined. As zebrafish allow real-time visualization of lens development, genetic manipulation of both the endogenous Crystallin genes as well as the use of transgenic overexpression of identified pathogenic variants yields important insight into the pathogenesis of congenital cataracts. Herein, we review the similarities and differences between human and zebrafish Crystallin genes. Further, we discuss the use of zebrafish as a model for congenital cataracts and explore the mechanisms that underlie the role of Crystallins in lens development. A better understanding of the genetic causes of congenital cataracts will lead to breakthroughs in preventing blindness from congenital cataracts and associated complications.
Congenital lens disorders and cataracts are estimated to affect 20,000 to 40,000 children worldwide (Rahi et al., 2001; Sheeladevi et al., 2016; Bell et al., 2020). A challenge with congenital lens disorders is that there are multiple etiologies (e.g., genetic, infectious, toxic), and the eye findings can be found in isolation (∼70%), associated with other ocular anomalies (∼15%), or as part of wider systemic syndromes (∼15%) (Bell et al., 2020; Yi et al., 2011; Patel et al., 2019). Isolated unilateral ocular findings, especially without family history of similar eye findings, are typically attributed to somatic mutations, however it is important to still consider genetic work-up in these patients as inherited eye diseases can often present asymmetrically due to incomplete penetrance, mosaicism, epigenetic or environmental factors. Patients with bilateral findings should be referred for genetic evaluation in order to determine if there is an identifiable genetic etiology and potential systemic involvement (Bell et al., 2020; Gillespie et al., 2016).
Variants in the crystallin genes (CRY) are predicted to account for approximately half of isolated non-syndromic inherited cataracts, however, their role in lens development is not well-defined (Shiels and Hejtmancik, 2015; Shiels and Hejtmancik, 2017; Berry et al., 2020). The crystallins comprise ∼90% of the proteins within the mature lens and are divided into α, ß, and γ subtypes, all of which have been associated with autosomal dominant and/or autosomal recessive congenital cataracts (Bell et al., 2020; Berry et al., 2020; Wistow, 2012). The most commonly reported congenital cataract-causing variants involve the CRYAA, CRYGD, CRYBB1, CRYBA1 genes (Berry et al., 2020; Wistow, 2012).
In humans there are 2 α-Crystallin subunits, the αA and the αB, which are encoded by the CRYAA and the CRYAB genes (Berry et al., 2020; Wistow and Piatigorsky, 1988; Horwitz, 2003). The α-Crystallins belong to the small heat shock protein superfamily. They serve as molecular chaperones preventing other proteins from aggregating, thereby maintaining lens clarity (Wistow, 2012; Wistow and Piatigorsky, 1988). Further, these proteins exist in oligomers that are often greater than 20 subunits and have a highly conserved crystallin domain, hydrophobic N-terminal and flexible C-terminal extension (Wistow, 2012). The crystallin domain responds to environmental changes (e.g., pH and metal ions) and together with the N-terminal domain bind destabilized proteins. These oligomers are in a constant state of flux as the exchange of smaller multimers is necessary for chaperone function (Wistow, 2012). Further, CRYAB evolved as a stress-induced heat shock protein and is modified by phosphorylation resulting in changes in oligomer size and rate of protein binding (Wistow, 2012; Clark et al., 2012; Thornell and Aquilina, 2015; Muranova et al., 2018). While CRYAA is predominantly expressed within the lens, CRYAB is also found in other tissues including cardiac and skeletal muscles. Thus CRYAB variants in addition to congenital cataracts is associated with cardiomyopathies and skeletal myopathies (Berry et al., 2020; Reis and Semina, 2019).
The ß- and γ-Crystallins are a separate family of proteins which are predominantly expressed in the lens and function to maintain its refractive index (Wistow, 2012; Slingsby and Clout, 1999). In humans, the ß-Crystallins consist of six subunits encoded by the CRYBA1, CRYBA2, CRYBA4, CRYBB1, CRYBB2, and CRYBB3 genes, with the first three being acidic forms and the last three basic (Berry et al., 2020; Wistow, 2012). The 5 γ-Crystallins are encoded by the CRYGA, CRYGB, CRYGC, CRYGD, and CRYGS genes and are clustered on Chromosome 2q.9 (Wistow, 2012; Shiloh et al., 1986), Other mammals have three additional γ-Crystallin genes (CRYGE, CRYGF, and CRYGN), however, these have become pseudogenes in humans with no evidence of expression (Wistow, 2012; Riazuddin et al., 2005; Wistow et al., 2005). The ß- and γ-Crystallins have a different structure than the α-Crystallins, consisting of two domains each composed of Greek key motifs (Berry et al., 2020; Wistow, 2012). ß-Crystallins also can have N- or C-terminal extensions which allows formation of small oligomers, while γ-Crystallins are found as simple monomers (Wistow, 2012; Wistow and Piatigorsky, 1988).
Zebrafish are important in modeling and investigating congenital diseases due to the genetic and anatomic similarities with the human eye and the accessibility of real-time imaging (Gross and Perkins, 2008). The live imaging is particularly useful for visualizing subtle changes in the lens due to genetic variants that could otherwise be missed by in vitro studies (Greiling and Clark, 2009; Mochizuki et al., 2017). In the past, numerous advances in lens embryology were made using chick lenses. However, the zebrafish is likely a better model as its embryology more closely resembles the human lens. The advent of techniques, such as CRISPR-Cas9 gene manipulation and Cre-Lox tissue and time specific knockouts, give important insight into eye development and the evaluation of genetic ocular anomalies including inherited cataracts (Zhao et al., 2021; Peng et al., 2024).
Lens development in zebrafish is similar to that in humans and is closely linked to overall eye development which involves interactions between neural epithelium, surface epithelium and neural crest cells. Evagination of the neural epithelial-derived optic vesicles from the diencephalon of the developing brain occurs at the six-somite stage (12 h post-fertilization (hpf)) in zebrafish and around day 25 in humans (Chow and Lang, 2001; Zagozewski et al., 2014; Abitbol, 2015). The optic vesicles then interact with the overlying surface epithelium to stimulate invagination of the bilayered optic cup and to induce the lens placode within the surface ectoderm in zebrafish by 16 hpf and in humans around day 28 (Canto-Soler and Adler, 2006; Fuhrmann, 2010). As the optic cup differentiates into the outer retinal pigment epithelium and the inner neural retina, the lens vesicle separates from the overlying surface epithelium which will ultimately become the corneal epithelium (Fuhrmann, 2010; Cardozo et al., 2023). While in humans a hollow lens vesicle forms, in zebrafish the lens cells delaminate en masse from the surface ectoderm (Dahm et al., 2007). This difference may be due to the close apposition of the lens and the cornea in the zebrafish eye that creates a singular optical refracting surface in an aqueous habitat versus the dual refracting surfaces of the cornea/tear film and the lens in the human eye exposed to air. Nevertheless, this embryological difference does not appear to affect the transparency of the lens or refractive power, and is not likely to be influenced by crystallin function or their variants. Concurrently, the mesodermal- and neural crest-derived periocular mesenchyme migrates into the anterior segment both between the edge of the optic cup and the surface ectoderm as well as through the optic fissure along the inferonasal aspect of the cup (Williams et al., 2017; Williams and Bohnsack, 2020). Within the anterior segment, the neural crest cells then migrate and contribute to the corneal stroma and endothelium, iris stroma, ciliary body stroma, and sclera (McMenamin, 1989). The optic fissure also transmits the hyaloid vasculature that is the main vascular network inside the vitreous cavity and connects with the tunica vasculosa which encases the lens and supports the developing anterior chamber (Lingam et al., 2021). Both the hyaloid vasculature and the tunica vasculosa resorb following anterior segment development, and failure to do so can result in mild abnormalities such as Mittendorf dots, Bergmeisters papillae, anterior polar cataract, and pupillary membranes or severe visually significant anomalies such as anterior and/or posterior persistent fetal vasculature (Shastry, 2009; Lutty and McLeod, 2018).
Within the lens, the posterior lens epithelial cells differentiate into primary lens fiber cells (Cvekl and Ashery-Padan, 2014; Kumar and Reilly, 2020). These cells proliferate to fill the primordial lenticular space and remain within the center of the lens to form the fetal lens nucleus. Daughter cells continue to proliferate and give rise to lens epithelial cells and lens fiber cells, which are evident in the zebrafish by 36 hpf and in human embryos by 60 days (Cvekl and Ashery-Padan, 2014). Lens epithelial cells are a cuboidal monolayer and secrete a basement membrane which clinically is referred to as the lens capsule. The lens capsule protects the lens fibers and facilitates through the zonules the attachment of the lens to the circumferential ciliary processes (Cvekl and Ashery-Padan, 2014; Kumar and Reilly, 2020).
The majority of cells become secondary lens fiber cells in the meridional zone which can be seen in the 72hpf zebrafish larvae. These cells exit the cell cycle and elongate to form concentric shells throughout life that give rise to the lens nucleus and surrounding cortex. Differentiation into lens fiber cells involves downregulation of epithelial markers, increase in the synthesis and orderly packing of crystallin proteins, increase in expression of water channels such as aquaporins, and degradation of cytoplasmic organelles in order to provide the refractive power of the lens and maintain its transparency (Bassnett, 2009; Bassnett et al., 2011). Various transcription factors, c-maf, l-maf, prox1, and pax6 have been shown in animal models to be essential in regulating the differentiation of lens epithelial cells into fiber cells and in chick and mice specifically the expression of crystallin gene expression (Cvekl and Ashery-Padan, 2014; Yang et al., 2006). Further, since the lens fiber cells lack organelles, protein turnover is regulated by the chaperone activity of the α-Crystallin proteins (Sharma and Santhoshkumar, 2009). On the other hand, the ß- and γ-Crystallins regulate the refractive index of the lens as the fibers continue to deposit post-natally (Wistow, 2012; Sharma and Santhoshkumar, 2009). Thus, the lens is a dynamic tissue and understanding its early development and further growth is important in appreciating disease pathogenesis.
Cataract formation occurs in humans as well as zebrafish with age, due to aggregation of proteins resulting in loss of lens clarity (Khatiwada et al., 2024). Conversely, congenital cataracts are a result of disruption in lens development and have been attributed to numerous genes including those that encode transcription factors that regulate signaling pathways, cytoskeletal proteins that serve as intracellular scaffolds, and membrane-bound proteins that regulate cell-cell communication within the avascular lens (Bell et al., 2020; Berry et al., 2020). Interestingly, although variants in crystallin genes are the most frequently reported gene family associated with congenital cataracts, their role in lens development is less well-defined, as they are most commonly thought of as maintaining tissue clarity in the mature lens.
While crystallin proteins are important in maintaining lens clarity and refractive index post-natally, the CRY genes also play a role during lens development. Animal studies are just starting to give insight into the signaling networks that are regulated by the different cry genes. Zebrafish underwent ancestral teleost genome duplication during evolution resulting in multiple genes (sometimes two to four separate genes) for many mammalian genes (Taylor et al., 2003). As a result, while humans have 2 CRYA, 6 CRYB and 5 CRYG genes, zebrafish have 3 crya, 13 cryb, and 40 cryg genes. In addition, there are four crybg genes, which are predominantly non-lens paralogs (Farnsworth et al., 2021). There is a high degree of homology between zebrafish and human α-Crystallins, but more divergence with the ß and γ proteins (Farnsworth et al., 2021; Posner, 2003). Other than gene expression data, there is limited functional data available on either the ß-and γ-Crystallins (Wistow et al., 2005; Farnsworth et al., 2021). Further, zebrafish lack the human equivalents of CRYGA, CRYGB, CRYGC, and CRYGD genes as the majority of cryg genes in fish belong to the aquatic specific γM group. The γM group are methionine rich, affording higher density proteins that are hypothesized to facilitate light refraction in aqueous rather than atmospheric environments (Mahler et al., 2013). Within the adult zebrafish lens, the γ-Crystallins are most prevalent (47%) with the ß-Crystallins second at 36% of the total protein. Only a small portion of proteins are α-Crystallins (8%) in the mature lens (Posner et al., 2008). Nevertheless, the smaller prevalence of the α-Crystallins in the post-natal lens does not preclude their importance in lens development.
The zebrafish Cryaa protein shares 72% identity in amino acid sequence with the human CRYAA protein (Table 1) (Runkle et al., 2002) Functional analysis shows similar chaperone activity between the zebrafish Cryaa and human CRYAA proteins. However, the zebrafish form is less stable at high temperatures, and functions at lower temperatures compared to the human, which likely reflects species-specific environmental differences (Dahlman et al., 2005). However, there are two cryab genes, cryaba and cryabb, due to ancestral teleost genome duplication (Smith et al., 2006; Mishra et al., 2018). The Cryaba and Cryabb proteins share 61% and 58% homology, respectively to the human CRYAB protein (Table 2) (Smith et al., 2006) The zebrafish αB-crystallins overall show less baseline chaperone activity than the αA-Crystallins, which may correlate with its function as a stress-induced heat shock protein that requires post-translational modifications such as phosphorylation to become fully active (Smith et al., 2006). Single cell transcriptome analysis recently demonstrated that cryaa is exclusively expressed within the lens fiber cells and is the earliest expressed Crystallin gene, starting by 48 hpf and increasing in expression over the next 3 days. In contrast cryaba and cryabb genes are predominantly expressed in non-ocular tissues during the zebrafish embryonic and (0–72 hpf) early larval (72–120 hpf) phases (Farnsworth et al., 2021).
Attention on the α-Crystallins as causes of congenital cataracts in zebrafish was first brought into focus due to the spontaneous cloche mutant, which in addition to hematopoietic and vascular abnormalities was also found to have cataracts by 72 hpf (Goishi et al., 2006). Initial studies reported decreased cryaa mRNA and Cryaa protein expression, however later studies with more sophisticated molecular capacity demonstrated that the cataracts were not due to lack of cryaa, but rather from general disruption of lens cell homeostasis (Goishi et al., 2006; Posner et al., 2019). Nevertheless, Posner et al. showed that cryaa mutant zebrafish created by CRISPR-Cas9 gene editing had lens abnormalities by 72 hpf (Posner et al., 2023). Approximately 60% of cryaa −/− mutant embryos obtained from cryaa −/− x cryaa −/− crosses showed lens changes that had varying levels of severity ranging from subtle areas of “roughness” in the primary fiber cells or along the peripheral fiber cell boundaries to more severe findings of central pitting and abnormal interfaces between fiber cells (Posner et al., 2023). Further, there was a mild delay in lens fiber cell denucleation from 72 to 96 hpf, however, ultimately loss of cryaa did not prevent loss of cell nuclei within the fiber cells. Zou et al. also reported a cryaa −/− mutant zebrafish that was created by TALEN nuclease gene editing and showed similar phenotype and penetrance (∼50%) amongst progeny derived from heterozygous cryaa ± x cryaa ± crosses (Zou et al., 2015). Interestingly, they found that embryos derived from homozygous cryaa −/− x cryaa −/− crosses showed higher phenotypic penetrance of greater than 90% and attributed this difference to cryaa transcripts received through maternal transmission in the heterozygous crosses. As the mutant analysis performed by Posner et al. were also derived from homozygous cryaa −/− crosses, the difference in phenotypic penetrance is not clear. A possible explanation could be the influence of the wildtype background that was utilized. Interestingly, the AB strain (which was used in Zou et al.) shows higher frequency (16%) of cataracts at 96 hpf compared to the TL strain (9%) (Khatiwada et al., 2024). The studies by Posner et al. utilized the ZDR strain, which to date has no published data as to the frequency of early onset cataracts, but 27% of this wildtype strain develop cataracts by 18 months of age (Posner et al., 2024; Posner et al., 2023). Due to differences in the propensity for baseline cataracts in different strains, the type of strain used in a study should be taken into consideration.
Additional animal studies on the function of the cryaa gene have utilized pathogenic variants identified in patients with congenital cataracts. The R49C variant was first identified in a 4-generation family with inherited cataracts and alters the N-terminal domain of the CRYAA protein (Mackay et al., 2003). This variant is predicted to have increased affinity for undamaged proteins thereby saturating the binding sites, leading to chaperone/protein aggregation and ultimately cataract formation (Koteiche and McHaourab, 2006; McHaourab et al., 2009). Zebrafish which were knock-ins of the R49C variant, created by injection of the transgene under a lens-specific promotor showed a similar phenotype to the cryaa −/− mutants with lens changes detectable by 72 hpf in many, but not all affected fish (Mishra et al., 2018; Wu et al., 2018). The frequency of lens changes increased when the R49C cryaa transgene was paired with the cryaa −/− null background. Interestingly, the R49C variant caused aggregation of destabilized crystallin proteins resulting in more frequent lens abnormalities in zebrafish larvae (Wu et al., 2018). Knock-in of the R49C variant in mice showed cataracts by 2 days post-natally, and further studies demonstrated various alterations within the lens including altered biosynthesis and metabolism pathways. This led to increased creatine kinase activity and lactic acid and changes in histone expression, specifically increased H2 and decreased H3 (Andley et al., 2018; Frankfater et al., 2020). Additional studies in mice have shown that disruption of various H2 and H3 genes also results in lens abnormalities through changes in DNA methylation and ultimately downstream gene expression (Vetrivel et al., 2019; Andley et al., 2020; Hamilton et al., 2020). Similar molecular studies on the effects of the R49C variant as well as downstream targets of cryaa during lens development have yet to be performed in zebrafish. In summary, cryaa plays a role in zebrafish lens development such that alterations in expression increase risk of congenital lens abnormalities, but additional studies are required to understand the downstream targets of cryaa in the zebrafish lens.
Although cryaa variants are more commonly associated with congenital cataracts, cryab variants have also been identified in patients with inherited cataracts. In zebrafish, there are differing reports regarding cryaba −/− and cryabb −/− null mutants. Posner et al. reported that cryaba −/− and the cryaba −/− larvae showed no significant developmental lens abnormalities at 72 and 96 hpf (Posner et al., 2023). However, this same group later showed that close to half of cryaba −/− mutant zebrafish developed age-related cataracts by 2 years of age, compared to approximately 25% in wildtype and cryaa −/− mutant zebrafish (Posner et al., 2024). In contrast, Mishra et al. found cataracts in 72–96 hpf in cryaba ± and −/− as well as cryabb ± and −/− zebrafish, with higher frequencies seen in homozygous mutants compared to heterozygous fish. Further, the double cryaba −/−; cryabb −/− mutants and the double cryaa −/−; cryaba −/− mutants showed even higher frequency of lens abnormalities, ranging between 75% and 95% of fish (Mishra et al., 2018). The cryaba −/− and cryabb −/− mutant fish in Mishra et al. did have a more mild phenotype compared to the morpholino oligonucleotide knockdown of Cryaba and Cryabb expression through inhibition of protein translation (Zou et al., 2015). However, this may be due to systemic toxic effects and inhibition of overall growth and development from the morpholinos. This difference in phenotypes between the Posner and Mishra studies may be due to the pathogenicity of the knockout, although both studies verified absence of expression of the corresponding mRNA. Further, lenses from adult cryabb −/− mutants in Posner et al. were found to express a truncated version of the Cryabb protein which is predicted to contain the anti-aggregation chaperone site. The presence of this truncated form of Cryabb was not verified in embryonic lenses, as well as this does not account for the difference in phenotypes between the two cryaba −/− mutants presented by Posner et al. and Mishra et al (Posner et al., 2023) Similar to as discussed above, the differences in phenotypes may also be due to the strain background, but additional studies are required to further understand this discrepancy.
Like the CRYAA gene, certain pathogenic variants have been identified in CRYAB in association with inherited cataracts, and this information has been utilized to study the effects of these variants on protein structure and function and the subsequent downstream effects within tissues (Vicart et al., 1998). A R120G variant of CRYAB, was found in affected members of a family to be associated with cardiomyopathy, skeletal myopathy, and “discrete lens opacities” of unknown age at onset (Vicart et al., 1998). The R120G variant in CRYAB is located within the α-crystalline domain and results in protein destabilization with oligomer disruption and subsequent aggregation (Bova et al., 1999; Darvazi et al., 2024). The effects of the R120G variant on the CRYAB protein do not seem to have the same extent of effects as the R49C variant has on the CRYAA protein (Wu et al., 2018), and their phenotypes in humans appear to vary, as well (Berry et al., 2020; Mackay et al., 2003). In zebrafish, knock-in of a R120G cryab transgene under a lens-specific promotor resulted in minor lens defects, which were magnified in penetrance and severity when crossed into the cryaba −/−; cryabb −/− double mutants (Wu et al., 2018). Mouse studies also found that knocking in the R120G Cryab variant caused cataracts, but with a slightly delayed onset (at 2 weeks) compared to the R49C Cryaa variant (Andley et al., 2018).
In mice, the R120G Cryab variant had less effect on histone expression, but did alter metabolic pathways involved in nucleic acid synthesis and degradation and endoplasmic reticulum protein processing (Andley et al., 2018; Frankfater et al., 2020). Studies assessing downstream effects of the R120G cryab variant in zebrafish are lacking. Nevertheless, these studies give important insight into the role of cryaba and cryabb in lens development and the effects of pathogenic variants that result in early cataract formation.
Additional studies link together the αB-crystallins with regulating lysosomal activities, which are necessary for organelle degradation within differentiating lens fiber cells (Costello et al., 2013; Morishita et al., 2013; Chauss et al., 2014; Cui et al., 2020). Pathogenic variants in the HSF4 gene, which encodes the heat shock transcription factor, HSF4, are associated with cataracts in humans and similarly knockout of Hsf4 in mice or zebrafish results in early onset cataracts (Bu et al., 2002; Min et al., 2004; Ke et al., 2006; Gao et al., 2017). Further studies demonstrated decreased expression of cryaba and aberrantly increased lysosomal pH in the lenses of hsf4 −/− mutant fish (Cui et al., 2020). This in vivo zebrafish data paired with in vitro studies from mouse lens epithelial cells suggests that Hsf4 regulates expression of αB-crystallin, which binds to the ATP6V1A-mTORC1 complex. As αB-crystallin stabilizes the complex and prevents degradation, the ATP6V1A as part of the proton pump maintains the acidic environment within the lysosome while mTORC1 is activated on the surface of the lysosome (Cui et al., 2020). Within zebrafish, knockout of hsf4 is hypothesized to lead to decreased cryaba expression thereby impairing lysosomal function by increasing its pH and inhibiting enucleation and organelle degradation within the lens fiber cells (Cui et al., 2020; Gao et al., 2017).
Further studies have shown that αB-crystallins are also involved in cholesterol biosynthesis within the lens (Frankfater et al., 2020; Park et al., 2023). Cholesterols are synthesized within the lens and are necessary at high concentrations to maintain the physical properties of the lens fiber cell membranes and reduce oxygen transport to decrease oxidative damage (Zelenka, 1984; Widomska and Subczynski, 2019). Defects in cholesterol synthesis are associated with cataracts (Cenedella, 1996). In zebrafish, cataract formation in cryaba −/− null mutants were reversed by increasing cholesterol biosynthesis (Park et al., 2023). In this study by Park et al., the cryaba −/− was crossed with a nrf2 −/− mutant, which surprisingly rescued the lens abnormalities. Transcriptome analysis further delineated that the double mutants (cryaba −/−, nrf2 −/−) showed upregulation of enzymes involved in cholesterol biosynthesis including cyp51, dhcr24, ebp, las, msmol, sqlea, hmgcra, and hmgcs1.80 Nrf2 encodes a transcription factor that mitigates oxidative stress, and so it is not clear as to the exact mechanism by which this resulted in increased cholesterol biosynthesis. Further, this study did not show specific downregulation of these cholesterol enzymes in the cryaba −/− mutants, however, mouse studies with the Cryab R120G knock-in did show that the mutant protein decreased the content of some minor sterols within the lens (Frankfater et al., 2020). Thus, additional studies are needed for better understanding the relationship between the αB-crystallins and cholesterol synthesis within the lens.
While the α-crystallins are typically associated with congenital cataracts, variants in CRYAB are also associated with skeletal myopathies and cardiomyopathies, including myofibrillar, restrictive, and hypertrophic subtypes (Dimauro et al., 2018; Thorkelsson and Chin, 2024). Within the heart, CRYAB prevents protein misfolding and protects against proteotoxicity (Mitra et al., 2013; McLendon and Robbins, 2015). Variants in CRYAB impair chaperone function thereby preventing translocation from the cytosol to the cytoskeleton, hindering oligomer exchange and decreasing binding of substrates. This ultimately leads to abnormal muscle striations and tissue dysfunction, causing many cardiovascular diseases (Thorkelsson and Chin, 2024). In zebrafish embryos, while cryaa is predominantly expressed in the developing lens, cryaba and cryabb are expressed in other cells including muscle progenitor cells and the primordial heart by 48 hpf (Farnsworth et al., 2021). In Mishra et al., both the cryaba −/− and the cryabb −/− mutants had normal hearts, but showed pericardial edema and dilated cardiomyopathy in response to environmental stress or exposure to external glucocorticoids (Mishra et al., 2018). The G154S variant in CRYAB, associated with myofibrillar myopathy, was evaluated in zebrafish and resulted in myofibrillar aggregates and disruption of muscle fiber structure (Reilich et al., 2010; Vattemi et al., 2011; Cannone et al., 2023). In addition the double cryaba −/−; nrf2 −/− zebrafish mutant showed worse cardiac findings especially with treatment of glucocorticoids than either of the single mutants, suggesting a link between oxidative stress and αB-crystallins within the heart (Park et al., 2023). However, the cryabb gene was increased in nrf2 −/− mutant heart tissues as well as by exogenous treatment with dexamethasone, suggesting a differential response in gene regulation as well as oxidative stress of the two cryab genes in zebrafish. Thus, additional studies are needed to determine the different roles of cryaba and cryabb in the zebrafish heart, as well as how this information could be translated to mammalian systems with only 1 Cryab gene.
There is greater divergence of the ß- and γ-Crystallin genes between fish, mice, and humans, and thus less information is available as to their function in the lens during development as well as post-natally. Single cell transcriptome analysis recently showed that the four cryba1 (cryba1l1, cryba1l2, cryba1a, cryba1b), two cryba2 (cryba2a, cryba2b), one cryba4, four crybb1 (crybb1, crybb1l1, crybb1l2, crybb1l3) and one crybb2 genes were predominantly expressed in the lens fiber and/or lens epithelial cells in zebrafish embryos. The crybb3 gene is unique in that it is not expressed within the lens during zebrafish development and shares overlapping expression with the crybg genes (crybg1a, crybg1b) in the pharyngeal and otic regions. The remaining crybg genes, crybg2 and crybgx have indeterminate expression and lens fiber expression, respectively (Farnsworth et al., 2021). To date there are no models for any of the endogenous zebrafish ß- or γ-Crystallin genes. However, zebrafish have been used to analyze the human CRYBB1 promoter, showing that lens-specific expression was driven by a150 bp region around the start site that contained a binding site for the MAF transcription factor (Hou et al., 2006).
The majority of the γ-Crystallins in zebrafish are in the M-group, which evolved for an aqueous environment. Single cell transcriptome analysis demonstrated the majority of crygm genes are expressed within lens fiber cells, although there are some expressed in skeletal muscle, gastrointestinal epithelial cells, kidney and liver (Farnsworth et al., 2021). As knockout analysis of the endogenous γ-Crystallins would likely yield minimal information regarding human disease, these models have not been reported. Nevertheless, the I4F and V76D CRYGD variants predicted to destabilize the crystallin protein have been transgenically expressed in zebrafish under the cryaa promotor (Mishra et al., 2012; Wu et al., 2016). While 30%–60% of zebrafish carrying either of the variants showed mild lens abnormalities (crystal-like droplets), fish that had the double I4F/V76D transgene had the greatest penetrance (∼80%) of lens defects with more than half having significant abnormalities (irregular protuberances in the center of the lens). These lens defects showed similarity to the cryaa−/− null mutant published by the same group as well as the R49C CRYAA and R120G CRYAB transgenic zebrafish (Mishra et al., 2018; Wu et al., 2018). Thus, although the γD-Crystallin does not have a zebrafish equivalent, these studies showed that knock-in of variants in CRYGD shares a phenotype with the zebrafish and human α-Crystallin genes.
Variants in the Crystallin genes account for approximately 1/3 of inherited cases of childhood cataracts. Although the α-Crystallins are well-characterized small heat shock proteins that act as chaperones to prevent protein aggregation, the ß- and γ-Crystallins are a separate superfamily with less defined functions. Genetic testing of individuals with congenital cataracts has yielded a plethora of pathogenic variants in all of the Crystallin genes, but their specific functions during lens development are not well understood (Bell et al., 2020; Berry et al., 2020). Zebrafish studies have given important insight into the role of the α-Crystallin genes in lens development and determining downstream targets and signaling pathways. Although there is greater species divergence of the ß- and γ-Crystallins than α-Crystallins, the use of zebrafish to study the effects of pathogenic crystallin variants remains an important tool for better understanding the pathogenesis of congenital cataracts.
Ongoing studies are curating the CRY gene variants associated with cataracts in humans. As demonstrated by previous studies, zebrafish can be used to study the pathogenic effects of these variants by using lens-specific Cre transgenic lines as well as CRISPR-Cas9 gene manipulation. Improving our understanding of congenital cataract pathogenesis can ultimately decrease childhood vision loss by deploying molecular targeted treatments to prenatally preventing or postnatally curing the molecular abnormalities.
JR: Conceptualization, Writing–original draft, Writing–review and editing. AW: Conceptualization, Writing–original draft, Writing–review and editing. BB: Conceptualization, Data curation, Writing–original draft, Writing–review and editing.
The author(s) declare that financial support was received for the research and/or publication of this article. This study was supported by an unrestricted grant from the Research to Prevent Blindness.
The authors declare that the research was conducted in the absence of any commercial or financial relationships that could be construed as a potential conflict of interest.
The author(s) declare that no Generative AI was used in the creation of this manuscript.
All claims expressed in this article are solely those of the authors and do not necessarily represent those of their affiliated organizations, or those of the publisher, the editors and the reviewers. Any product that may be evaluated in this article, or claim that may be made by its manufacturer, is not guaranteed or endorsed by the publisher.
Abitbol, M. M. (2015). Networks of genes governing the development of optic and otic vesicles: implications for eye and ear development. Invest Ophthalmol. Vis. Sci. 56, 892. doi:10.1167/iovs.15-16420
Andley, U. P., Naumann, B. N., Hamilton, P. D., and Bozeman, S. L. (2020). Changes in relative histone abundance and heterochromatin in αa-crystallin and αb-crystallin knock-in mutant mouse lenses. BMC Res. Notes 13, 315. doi:10.1186/s13104-020-05154-7
Andley, U. P., Tycksen, E., McGlasson-Naumann, B. N., and Hamilton, P. D. (2018). Probing the changes in gene expression due to α-crystallin mutations in mouse models of hereditary human cataract. PLoS One 13, e0190817. doi:10.1371/journal.pone.0190817
Bassnett, S. (2009). On the mechanism of organelle degradation in the vertebrate lens. Exp. Eye Res. 88, 133–139. doi:10.1016/j.exer.2008.08.017
Bassnett, S., Shi, Y., and Vrensen, G. F. (2011). Biological glass: structural determinants of eye lens transparency. Philos. Trans. R. Soc. Lond B Biol. Sci. 366, 1250–1264. doi:10.1098/rstb.2010.0302
Bell, S. J., Oluonye, N., Harding, P., and Moosajee, M. (2020). Congenital cataract: a guide to genetic and clinical management. Ther. Adv. Rare Dis. 1, 2633004020938061. doi:10.1177/2633004020938061
Berry, V., Ionides, A., Pontikos, N., Georgiou, M., Yu, J., Ocaka, L. A., et al. (2020). The genetic landscape of crystallins in congenital cataract. Orphanet J. Rare Dis. 15, 333. doi:10.1186/s13023-020-01613-3
Bova, M. P., Yaron, O., Huang, Q., Ding, L., Haley, D. A., Stewart, P. L., et al. (1999). Mutation r120g in alphab-crystallin, which is linked to a desmin-related myopathy, results in an irregular structure and defective chaperone-like function. Proc. Natl. Acad. Sci. U. S. A. 96, 6137–6142. doi:10.1073/pnas.96.11.6137
Bu, L., Jin, Y., Shi, Y., Chu, R., Ban, A., Eiberg, H., et al. (2002). Mutant DNA-binding domain of hsf4 is associated with autosomal dominant lamellar and marner cataract. Nat. Genet. 31, 276–278. doi:10.1038/ng921
Cannone, E., Guglielmi, V., Marchetto, G., Tobia, C., Gnutti, B., Cisterna, B., et al. (2023). Human mutated myot and cryab genes cause a myopathic phenotype in zebrafish. Int. J. Mol. Sci. 24, 11483. doi:10.3390/ijms241411483
Canto-Soler, M. V., and Adler, R. (2006). Optic cup and lens development requires pax6 expression in the early optic vesicle during a narrow time window. Dev. Biol. 294, 119–132. doi:10.1016/j.ydbio.2006.02.033
Cardozo, M. J., Sánchez-Bustamante, E., and Bovolenta, P. (2023). Optic cup morphogenesis across species and related inborn human eye defects. Development 150, dev200399. doi:10.1242/dev.200399
Cenedella, R. J. (1996). Cholesterol and cataracts. Surv. Ophthalmol. 40, 320–337. doi:10.1016/s0039-6257(96)82007-8
Chauss, D., Basu, S., Rajakaruna, S., Ma, Z., Gau, V., Anastas, S., et al. (2014). Differentiation state-specific mitochondrial dynamic regulatory networks are revealed by global transcriptional analysis of the developing chicken lens. G3 (Bethesda) 4, 1515–1527. doi:10.1534/g3.114.012120
Chow, R. L., and Lang, R. A. (2001). Early eye development in vertebrates. Annu. Rev. Cell Dev. Biol. 17, 255–296. doi:10.1146/annurev.cellbio.17.1.255
Clark, A. R., Lubsen, N. H., and Slingsby, C. (2012). Shsp in the eye lens: crystallin mutations, cataract and proteostasis. Int. J. Biochem. Cell Biol. 44, 1687–1697. doi:10.1016/j.biocel.2012.02.015
Costello, M. J., Brennan, L. A., Basu, S., Chauss, D., Mohamed, A., Gilliland, K. O., et al. (2013). Autophagy and mitophagy participate in ocular lens organelle degradation. Exp. Eye Res. 116, 141–150. doi:10.1016/j.exer.2013.08.017
Cui, X., Feng, R., Wang, J., Du, C., Pi, X., Chen, D., et al. (2020). Heat shock factor 4 regulates lysosome activity by modulating the αb-crystallin-atp6v1a-mtor complex in ocular lens. Biochim. Biophys. Acta Gen. Subj. 1864, 129496. doi:10.1016/j.bbagen.2019.129496
Cvekl, A., and Ashery-Padan, R. (2014). The cellular and molecular mechanisms of vertebrate lens development. Development 141, 4432–4447. doi:10.1242/dev.107953
Dahlman, J. M., Margot, K. L., Ding, L., Horwitz, J., and Posner, M. (2005). Zebrafish alpha-crystallins: protein structure and chaperone-like activity compared to their mammalian orthologs. Mol. Vis. 11, 88–96.
Dahm, R., Schonthaler, H. B., Soehn, A. S., van Marle, J., and Vrensen, G. F. J. M. (2007). Development and adult morphology of the eye lens in the zebrafish. Exp. Eye Res. 85, 74–89. doi:10.1016/j.exer.2007.02.015
Darvazi, M., Ghorbani, M., Ramazi, S., Allahverdi, A., and Abdolmaleki, P. (2024). A computational study of the r120g mutation in human αb-crystallin: implications for structural stability and functionality. J. Biomol. Struct. Dyn. 42, 5788–5798. doi:10.1080/07391102.2023.2229434
Dimauro, I., Antonioni, A., Mercatelli, N., and Caporossi, D. (2018). The role of αb-crystallin in skeletal and cardiac muscle tissues. Cell Stress Chaperones 23, 491–505. doi:10.1007/s12192-017-0866-x
Farnsworth, D. R., Posner, M., and Miller, A. C. (2021). Single cell transcriptomics of the developing zebrafish lens and identification of putative controllers of lens development. Exp. Eye Res. 206, 108535. doi:10.1016/j.exer.2021.108535
Frankfater, C., Bozeman, S. L., Hsu, F. F., and Andley, U. P. (2020). Alpha-crystallin mutations alter lens metabolites in mouse models of human cataracts. PLoS One 15, e0238081. doi:10.1371/journal.pone.0238081
Fuhrmann, S. (2010). Eye morphogenesis and patterning of the optic vesicle. Curr. Top. Dev. Biol. 93, 61–84. doi:10.1016/B978-0-12-385044-7.00003-5
Gao, M., Huang, Y., Wang, L., Liu, F., Liao, S., et al. (2017). Hsf4 regulates lens fiber cell differentiation by activating p53 and its downstream regulators. Cell Death Dis. 8, e3082. doi:10.1038/cddis.2017.478
Gillespie, R. L., Urquhart, J., Anderson, B., Williams, S., Waller, S., Ashworth, J., et al. (2016). Next-generation sequencing in the diagnosis of metabolic disease marked by pediatric cataract. Ophthalmology 123, 217–220. doi:10.1016/j.ophtha.2015.06.035
Goishi, K., Shimizu, A., Najarro, G., Watanabe, S., Rogers, R., Zon, L. I., et al. (2006). Alphaa-crystallin expression prevents gamma-crystallin insolubility and cataract formation in the zebrafish cloche mutant lens. Development 133, 2585–2593. doi:10.1242/dev.02424
Greiling, T. M., and Clark, J. I. (2009). Early lens development in the zebrafish: a three-dimensional time-lapse analysis. Dev. Dyn. 238, 2254–2265. doi:10.1002/dvdy.21997
Gross, J. M., and Perkins, B. D. (2008). Zebrafish mutants as models for congenital ocular disorders in humans. Mol. Reprod. Dev. 75, 547–555. doi:10.1002/mrd.20831
Hamilton, P. D., Bozeman, S. L., and Andley, U. P. (2020). Creatine kinase/α-crystallin interaction functions in cataract development. Biochem. Biophys. Rep. 22, 100748. doi:10.1016/j.bbrep.2020.100748
Hou, H. H., Kuo, M. Y., Luo, Y. W., and Chang, B. E. (2006). Recapitulation of human betab1-crystallin promoter activity in transgenic zebrafish. Dev. Dyn. 235, 435–443. doi:10.1002/dvdy.20652
Ke, T., Wang, Q. K., Ji, B., Wang, X., Liu, P., Zhang, X., et al. (2006). Novel hsf4 mutation causes congenital total white cataract in a Chinese family. Am. J. Ophthalmol. 142, 298–303. doi:10.1016/j.ajo.2006.03.056
Khatiwada, B., Jones, J. L., Zhao, D., Gasperini, R. J., McComish, B. J., and Burdon, K. P. (2024). Comparison of baseline cataract rates in ab and tl wildtype zebrafish strains. Exp. Eye Res. 243, 109908. doi:10.1016/j.exer.2024.109908
Koteiche, H. A., and McHaourab, H. S. (2006). Mechanism of a hereditary cataract phenotype. Mutations in alphaa-crystallin activate substrate binding. J. Biol. Chem. 281, 14273–14279. doi:10.1074/jbc.M512938200
Kumar, B., and Reilly, M. A. (2020). The development, growth, and regeneration of the crystalline lens: a review. Curr. Eye Res. 45, 313–326. doi:10.1080/02713683.2019.1681003
Lingam, G., Sen, A. C., Lingam, V., Bhende, M., Padhi, T. R., and Xinyi, S. (2021). Ocular coloboma-a comprehensive review for the clinician. Eye (Lond) 35, 2086–2109. doi:10.1038/s41433-021-01501-5
Lutty, G. A., and McLeod, D. S. (2018). Development of the hyaloid, choroidal and retinal vasculatures in the fetal human eye. Prog. Retin Eye Res. 62, 58–76. doi:10.1016/j.preteyeres.2017.10.001
Mackay, D. S., Andley, U. P., and Shiels, A. (2003). Cell death triggered by a novel mutation in the alphaa-crystallin gene underlies autosomal dominant cataract linked to chromosome 21q. Eur. J. Hum. Genet. 11, 784–793. doi:10.1038/sj.ejhg.5201046
Mahler, B., Chen, Y., Ford, J., Thiel, C., Wistow, G., and Wu, Z. (2013). Structure and dynamics of the fish eye lens protein, γm7-crystallin. Biochemistry 52, 3579–3587. doi:10.1021/bi400151c
McHaourab, H. S., Godar, J. A., and Stewart, P. L. (2009). Structure and mechanism of protein stability sensors: chaperone activity of small heat shock proteins. Biochemistry 48, 3828–3837. doi:10.1021/bi900212j
McLendon, P. M., and Robbins, J. (2015). Proteotoxicity and cardiac dysfunction. Circ. Res. 116, 1863–1882. doi:10.1161/CIRCRESAHA.116.305372
McMenamin, P. G. (1989). A morphological study of the inner surface of the anterior chamber angle in pre and postnatal human eyes. Curr. Eye Res. 8, 727–739. doi:10.3109/02713688909025808
Min, J. N., Zhang, Y., Moskophidis, D., and Mivechi, N. F. (2004). Unique contribution of heat shock transcription factor 4 in ocular lens development and fiber cell differentiation. Genesis 40, 205–217. doi:10.1002/gene.20087
Mishra, S., Stein, R. A., and McHaourab, H. S. (2012). Cataract-linked γd-crystallin mutants have weak affinity to lens chaperones α-crystallins. FEBS Lett. 586, 330–336. doi:10.1016/j.febslet.2012.01.019
Mishra, S., Wu, S. Y., Fuller, A. W., Wang, Z., Rose, K. L., Schey, K. L., et al. (2018). Loss of αb-crystallin function in zebrafish reveals critical roles in the development of the lens and stress resistance of the heart. J. Biol. Chem. 293, 740–753. doi:10.1074/jbc.M117.808634
Mitra, A., Basak, T., Datta, K., Naskar, S., Sengupta, S., and Sarkar, S. (2013). Role of α-crystallin b as a regulatory switch in modulating cardiomyocyte apoptosis by mitochondria or endoplasmic reticulum during cardiac hypertrophy and myocardial infarction. Cell Death Dis. 4, e582. doi:10.1038/cddis.2013.114
Mochizuki, T., Luo, Y. J., Tsai, H. F., Hagiwara, A., and Masai, I. (2017). Cell division and cadherin-mediated adhesion regulate lens epithelial cell movement in zebrafish. Development 144, 708–719. doi:10.1242/dev.138909
Morishita, H., Eguchi, S., Kimura, H., Sasaki, J., Sakamaki, Y., Robinson, M. L., et al. (2013). Deletion of autophagy-related 5 (atg5) and pik3c3 genes in the lens causes cataract independent of programmed organelle degradation. J. Biol. Chem. 288, 11436–11447. doi:10.1074/jbc.M112.437103
Muranova, L. K., Sudnitsyna, M. V., and Gusev, N. B. (2018). αb-crystallin phosphorylation: advances and problems. Biochem. (Mosc) 83, 1196–1206. doi:10.1134/S000629791810005X
Park, J., MacGavin, S., Niederbrach, L., and Mchaourab, H. S. (2023). Interplay between nrf2 and αb-crystallin in the lens and heart of zebrafish under proteostatic stress. Front. Mol. Biosci. 10, 1185704. doi:10.3389/fmolb.2023.1185704
Patel, A., Hayward, J. D., Tailor, V., Nyanhete, R., Ahlfors, H., Gabriel, C., et al. (2019). The oculome panel test: next-generation sequencing to diagnose a diverse range of genetic developmental eye disorders. Ophthalmology 126, 888–907. doi:10.1016/j.ophtha.2018.12.050
Peng, X., Jia, X., Shang, G., Xue, M., Jiang, M., Chen, D., et al. (2024). The generation and characterization of a transgenic zebrafish line with lens-specific cre expression. Mol. Vis. 30, 123–136.
Posner, M. (2003). A comparative view of alpha crystallins: the contribution of comparative studies to understanding function. Integr. Comp. Biol. 43, 481–491. doi:10.1093/icb/43.4.481
Posner, M., Garver, T., Kaye, T., Brdicka, S., Suttle, M., Patterson, B., et al. (2024). Loss of αba-crystallin, but not αa-crystallin, increases age-related cataract in the zebrafish lens. Exp. Eye Res. 244, 109918. doi:10.1016/j.exer.2024.109918
Posner, M., Hawke, M., Lacava, C., Prince, C. J., Bellanco, N. R., and Corbin, R. W. (2008). A proteome map of the zebrafish (danio rerio) lens reveals similarities between zebrafish and mammalian crystallin expression. Mol. Vis. 14, 806–814.
Posner, M., McDonald, M. S., Murray, K. L., and Kiss, A. J. (2019). Why does the zebrafish cloche mutant develop lens cataract? PLoS One 14, e0211399. doi:10.1371/journal.pone.0211399
Posner, M., Murray, K. L., Andrew, B., Brdicka, S., Roberts, A., Franklin, K., et al. (2023). Impact of α-crystallin protein loss on zebrafish lens development. Exp. Eye Res. 227, 109358. doi:10.1016/j.exer.2022.109358
Rahi, J. S., and Dezateux, C.British Congenital Cataract Interest Group (2001). Measuring and interpreting the incidence of congenital ocular anomalies: lessons from a national study of congenital cataract in the UK. Invest Ophthalmol. Vis. Sci. 42, 1444–1448.
Reilich, P., Schoser, B., Schramm, N., Krause, S., Schessl, J., Kress, W., et al. (2010). The p.G154s mutation of the alpha-b crystallin gene (cryab) causes late-onset distal myopathy. Neuromuscul. Disord. 20, 255–259. doi:10.1016/j.nmd.2010.01.012
Reis, L. M., and Semina, E. V. (2019). Genetic landscape of isolated pediatric cataracts: extreme heterogeneity and variable inheritance patterns within genes. Hum. Genet. 138, 847–863. doi:10.1007/s00439-018-1932-x
Riazuddin, S. A., Yasmeen, A., Yao, W., Sergeev, Y. V., Zhang, Q., Zulfiqar, F., et al. (2005). Mutations in betab3-crystallin associated with autosomal recessive cataract in two pakistani families. Invest Ophthalmol. Vis. Sci. 46, 2100–2106. doi:10.1167/iovs.04-1481
Runkle, S., Hill, J., Kantorow, M., Horwitz, J., and Posner, M. (2002). Sequence and spatial expression of zebrafish (danio rerio) alphaa-crystallin. Mol. Vis. 8, 45–50.
Sharma, K. K., and Santhoshkumar, P. (2009). Lens aging: effects of crystallins. Biochim. Biophys. Acta 1790, 1095–1108. doi:10.1016/j.bbagen.2009.05.008
Shastry, B. S. (2009). Persistent hyperplastic primary vitreous: congenital malformation of the eye. Clin. Exp. Ophthalmol. 37, 884–890. doi:10.1111/j.1442-9071.2009.02150.x
Sheeladevi, S., Lawrenson, J. G., Fielder, A. R., and Suttle, C. M. (2016). Global prevalence of childhood cataract: a systematic review. Eye (Lond) 30, 1160–1169. doi:10.1038/eye.2016.156
Shiels, A., and Hejtmancik, J. F. (2015). Molecular genetics of cataract. Prog. Mol. Biol. Transl. Sci. 134, 203–218. doi:10.1016/bs.pmbts.2015.05.004
Shiels, A., and Hejtmancik, J. F. (2017). Mutations and mechanisms in congenital and age-related cataracts. Exp. Eye Res. 156, 95–102. doi:10.1016/j.exer.2016.06.011
Shiloh, Y., Donlon, T., Bruns, G., Breitman, M. L., and Tsui, L. C. (1986). Assignment of the human gamma-crystallin gene cluster (cryg) to the long arm of chromosome 2, region q33-36. Hum. Genet. 73, 17–19. doi:10.1007/BF00292656
Slingsby, C., and Clout, N. J. (1999). Structure of the crystallins. Eye (Lond) 13 (Pt 3b), 395–402. doi:10.1038/eye.1999.113
Smith, A. A., Wyatt, K., Vacha, J., Vihtelic, T. S., Zigler, J. S., Wistow, G. J., et al. (2006). Gene duplication and separation of functions in alphab-crystallin from zebrafish (danio rerio). Febs J. 273, 481–490. doi:10.1111/j.1742-4658.2005.05080.x
Taylor, J. S., Braasch, I., Frickey, T., Meyer, A., and Van de Peer, Y. (2003). Genome duplication, a trait shared by 22000 species of ray-finned fish. Genome Res. 13, 382–390. doi:10.1101/gr.640303
Thorkelsson, A., and Chin, M. T. (2024). Role of the alpha-b-crystallin protein in cardiomyopathic disease. Int. J. Mol. Sci. 25, 2826. doi:10.3390/ijms25052826
Thornell, E., and Aquilina, A. (2015). Regulation of αa- and αb-crystallins via phosphorylation in cellular homeostasis. Cell Mol. Life Sci. 72, 4127–4137. doi:10.1007/s00018-015-1996-x
Vattemi, G., Neri, M., Piffer, S., Vicart, P., Gualandi, F., Marini, M., et al. (2011). Clinical, morphological and genetic studies in a cohort of 21 patients with myofibrillar myopathy. Acta Myol. 30, 121–126.
Vetrivel, S., Tiso, N., Kügler, A., Irmler, M., Horsch, M., Beckers, J., et al. (2019). Mutation in the mouse histone gene hist2h3c1 leads to degeneration of the lens vesicle and severe microphthalmia. Exp. Eye Res. 188, 107632. doi:10.1016/j.exer.2019.03.024
Vicart, P., Caron, A., Guicheney, P., Li, Z., Prévost, M. C., Faure, A., et al. (1998). A missense mutation in the alphab-crystallin chaperone gene causes a desmin-related myopathy. Nat. Genet. 20, 92–95. doi:10.1038/1765
Widomska, J., and Subczynski, W. K. (2019). Why is very high cholesterol content beneficial for the eye lens but negative for other organs? Nutrients 11, 1083. doi:10.3390/nu11051083
Williams, A. L., and Bohnsack, B. L. (2020). The ocular neural crest: specification, migration, and then what? Front. Cell Dev. Biol. 8, 595896. doi:10.3389/fcell.2020.595896
Williams, A. L., Eason, J., Chawla, B., and Bohnsack, B. L. (2017). Cyp1b1 regulates ocular fissure closure through a retinoic acid-independent pathway. Invest Ophthalmol. Vis. Sci. 48, 1084–1097. doi:10.1167/iovs.16-20235
Wistow, G. (2012). The human crystallin gene families. Hum. Genomics 6, 26. doi:10.1186/1479-7364-6-26
Wistow, G., Wyatt, K., David, L., Gao, C., Bateman, O., Bernstein, S., et al. (2005). Gamman-crystallin and the evolution of the betagamma-crystallin superfamily in vertebrates. Febs J. 272, 2276–2291. doi:10.1111/j.1742-4658.2005.04655.x
Wistow, G. J., and Piatigorsky, J. (1988). Lens crystallins: the evolution and expression of proteins for a highly specialized tissue. Annu. Rev. Biochem. 57, 479–504. doi:10.1146/annurev.bi.57.070188.002403
Wu, S. Y., Zou, P., Fuller, A. W., Mishra, S., Wang, Z., Schey, K. L., et al. (2016). Expression of cataract-linked γ-crystallin variants in zebrafish reveals a proteostasis network that senses protein stability. J. Biol. Chem. 291, 25387–25397. doi:10.1074/jbc.M116.749606
Wu, S. Y., Zou, P., Mishra, S., and Mchaourab, H. S. (2018). Transgenic zebrafish models reveal distinct molecular mechanisms for cataract-linked αa-crystallin mutants. PLoS One 13, e0207540. doi:10.1371/journal.pone.0207540
Yang, Y., Stopka, T., Golestaneh, N., Wang, Y., Wu, K., et al. (2006). Regulation of alphaa-crystallin via pax6, c-maf, creb and a broad domain of lens-specific chromatin. Embo J. 25, 2107–2118. doi:10.1038/sj.emboj.7601114
Yi, J., Yun, J., Li, Z. K., Xu, C. T., and Pan, B. R. (2011). Epidemiology and molecular genetics of congenital cataracts. Int. J. Ophthalmol. 4, 422–432. doi:10.3980/j.issn.2222-3959.2011.04.20
Zagozewski, J. L., Zhang, Q., and Eisenstat, D. D. (2014). Genetic regulation of vertebrate eye development. Clin. Genet. 86, 453–460. doi:10.1111/cge.12493
Zhao, D., Jones, J. L., Gasperini, R. J., Charlesworth, J. C., Liu, G. S., and Burdon, K. P. (2021). Rapid and efficient cataract gene evaluation in f0 zebrafish using crispr-cas9 ribonucleoprotein complexes. Methods 194, 37–47. doi:10.1016/j.ymeth.2020.12.004
Keywords: congenital cataracts, zebrafish, crystallins, cryaa, cryab
Citation: Rossen JL, Williams AL and Bohnsack BL (2025) Zebrafish as a model for crystallin-associated congenital cataracts in humans. Front. Cell Dev. Biol. 13:1552988. doi: 10.3389/fcell.2025.1552988
Received: 29 December 2024; Accepted: 18 March 2025;
Published: 26 March 2025.
Edited by:
James Alan Marrs, Purdue University Indianapolis, United StatesReviewed by:
Jian Sun, University of Delaware, United StatesCopyright © 2025 Rossen, Williams and Bohnsack. This is an open-access article distributed under the terms of the Creative Commons Attribution License (CC BY). The use, distribution or reproduction in other forums is permitted, provided the original author(s) and the copyright owner(s) are credited and that the original publication in this journal is cited, in accordance with accepted academic practice. No use, distribution or reproduction is permitted which does not comply with these terms.
*Correspondence: Brenda L. Bohnsack, YmJvaG5zYWNrQGx1cmllY2hpbGRyZW5zLm9yZw==
Disclaimer: All claims expressed in this article are solely those of the authors and do not necessarily represent those of their affiliated organizations, or those of the publisher, the editors and the reviewers. Any product that may be evaluated in this article or claim that may be made by its manufacturer is not guaranteed or endorsed by the publisher.
Research integrity at Frontiers
Learn more about the work of our research integrity team to safeguard the quality of each article we publish.