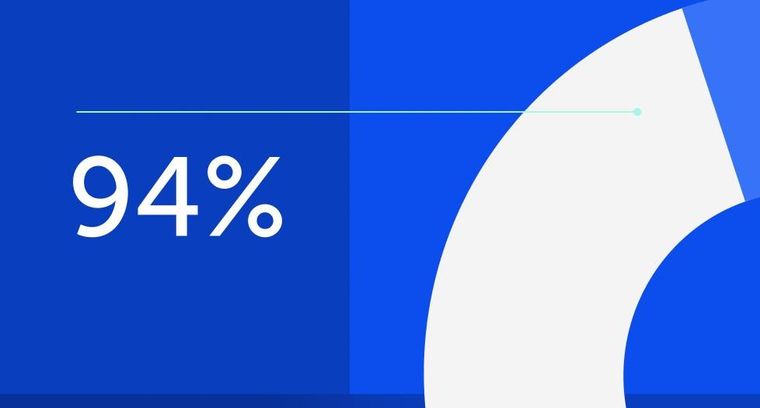
94% of researchers rate our articles as excellent or good
Learn more about the work of our research integrity team to safeguard the quality of each article we publish.
Find out more
REVIEW article
Front. Cell Dev. Biol., 27 March 2025
Sec. Stem Cell Research
Volume 13 - 2025 | https://doi.org/10.3389/fcell.2025.1549096
This article is part of the Research TopicApplication of Stem Cells in Degenerative Orthopedic DiseasesView all articles
Osteoarthritis (OA) and type 2 diabetes mellitus (T2DM) often coexist due to shared risk factors and high prevalence, but effective treatment methods are currently lacking. Mesenchymal stromal/stem cell-derived exosomes (MSC-Exos) have regenerative properties that can repair cartilage damage, lower blood sugar levels, and improve pancreatic β cell function, showing great potential in tissue repair. This review primarily explores the application of MSC-Exos in the treatment of OA and T2DM, the potential mechanisms of MSC-Exos, and the therapeutic strategies of engineered exosomes. Although MSC-Exo therapy shows promising therapeutic potential, further research is needed to validate its safety and feasibility.
GRAPHICAL ABSTRACT | The graphical abstract was created with BioRender (www.biorender.com). Exploring the feasibility of intra-articular MSC-Exo therapy for symptoms in patients with T2DM combined with OA.
OA is a chronic age-related degenerative joint disease that affects the entire synovial joint. It is characterized by structural damage to the articular hyaline cartilage, degeneration of subchondral bone, and changes in synovial tissue such as hypertrophy and increased vascularization (Loeser et al., 2012). T2DM is a metabolic disease caused by a lack of insulin or the body’s inability to use insulin effectively. T2DM is characterized by high blood sugar, and prolonged high blood sugar can lead to osmotic and oxidative stress, which can cause damage to various tissues, including bones, joints, kidneys, and the nervous system (Javeed and Matveyenko, 2018). Studies have shown that T2DM is an independent risk factor for OA (Schett et al., 2013). In T2DM, the interaction of joint degeneration and metabolic disorder can aggravate cartilage destruction and synovial inflammation, and OA and T2DM coexist to form diabetic OA (Eitner and Wildemann, 2021; Yang et al., 2024).
OA is a complex disease that affects multiple parts of the joint, including articular cartilage, subchondral bone, and synovium, and is accompanied by chronic inflammation (Courties and Sellam, 2016). Articular cartilage is composed of chondrocytes and the extracellular matrix (ECM), and its primary function is to absorb mechanical stress between bones. In OA, cartilage damage, synovial inflammation, osteophyte formation, and changes in joint morphology increase joint pressure, leading to the release of more pro-inflammatory mediators by chondrocytes, including cytokines [such as interleukin-1β (IL-1β) and tumor necrosis factor-alpha (TNF-α)], reactive oxygen species (ROS), and advanced glycation end-products (AGEs). This, in turn, triggers an increase in proteolytic enzymes [such as matrix metalloproteinases (MMPs) and aggrecanases with thrombospondin motifs (ADAMTS)], ultimately resulting in the degradation of the cartilage matrix. As shown in Figure 1, T2DM has a pathogenic effect on OA through two major pathways: (1) chronic hyperglycemia, which promotes oxidative stress, bolsters pro-inflammatory cytokines and AGEs production in joint tissues but also decreases the chondrogenic differentiation potential of MSCs, thereby further decreasing the already impaired cartilage repair in OA; and (2) insulin resistance (IR), which executes its effects both locally and also through low-grade inflammation systemically (Veronese et al., 2019). Chondrocyte damage and apoptosis might be induced due to leptin secretion from adipose tissue leading to the increased production of cytokine and MMPs (Courties and Sellam, 2016).
Figure 1. The pathological mechanisms of diabetic OA. In the coexistence of T2DM and OA, elevated blood glucose levels and insulin resistance accelerate the progression of diabetic OA through the regulation of signaling pathways. It was created with BioRender (www.biorender.com).
The main clinical treatment options for T2DM are glucose-lowering drugs and insulin therapy, but there is still a lack of a cure. Medications and other treatments can bring blood glucose levels as close to normal as possible, thereby delaying or preventing the onset of diabetes-related health problems (Su et al., 2023). While OA treatment includes surgery and medication. Surgery is aimed at repairing local cartilage damage, and medication is mainly anti-inflammatory, anti-catabolic, and symptomatic (Tejero et al., 2021; Madry, 2022). Current treatment methods can alleviate symptoms and reduce patient discomfort, but they do not provide a cure.
A study from the Osteoarthritis Initiative analyzed antidiabetic drugs and found that they may slow the progression of knee OA (Shirinsky and Shirinsky, 2017). Research indicates that the combination of metformin and cyclooxygenase-2 inhibitors might reduce the rate of joint replacement surgery in patients with OA and T2DM (Lu et al., 2018). However, Barnett et al. (2017) suggested no strong correlation between metformin treatment and OA development in diabetic patients, potentially due to confounding factors, diagnostic methods, and variations in dosage and duration of use. Additionally, studies show that long-term use of GLP-1 receptor agonists (GLP-1-RAs) can improve knee osteoarthritis (KOA) symptoms (Zhu et al., 2023), but both obese and non-obese diabetic patients using GLP-1-RAs may have an increased risk of developing KOA (Lavu et al., 2024). Given the controversy surrounding these treatments, exploring and developing new therapeutic strategies is particularly important.
Mesenchymal stromal/stem cells (MSCs) have demonstrated potential therapeutic effects in treating OA and T2DM. MSCs can promote cartilage repair and regeneration, reducing cartilage damage caused by arthritis, alleviating inflammation associated with arthritis and diabetes (Copp et al., 2023). There is growing evidence that many of the regenerative properties previously thought to be attributable to MSCs should be attributed to their secreted exosomes (Basu and Ludlow, 2016). In addition, MSC-Exos grafts have the advantages of being non-immunogenic, non-tumourigenic, and easy to store and transport compared to MSC therapy (Batrakova and Kim, 2015; Burger et al., 2015). Therefore, MSC-Exos have great potential for application in the field of tissue repair.
Therefore, this review aims to comprehensively assess the latest research progress in MSC-Exo therapy for diabetic OA, starting from relevant mechanisms and preclinical studies, summarizing current applications, analyzing feasibility and efficacy, exploring potential mechanisms, and providing directions for future research.
OA and T2DM are two common diseases that frequently coexist due to their high prevalence and shared risk factors such as age, gender, ethnicity, and metabolic disorders (e.g., obesity, hypertension, and dyslipidemia) (Alenazi et al., 2023). Although their pathological mechanisms differ, they share common pathophysiological bases, including chronic inflammation, oxidative stress, and metabolic dysregulation.
Under hyperglycemic conditions, chondrocytes from OA patients exhibit multiple dysfunctions that collectively contribute to cartilage degradation and OA progression. Firstly, hyperglycemia prevents chondrocytes from effectively downregulating the expression of glucose transporter proteins (GLUTs), leading to excessive intracellular glucose accumulation (Sun et al., 2023). This hyperglycemic environment triggers the production of ROS (Rosa et al., 2009), whose accumulation further induces the release of inflammatory mediators (such as IL-1β and NF-κB), resulting in chondrocyte degradation and apoptosis, thereby compromising cartilage tissue integrity (Anderson et al., 2023). Additionally, OA chondrocytes in a high-glucose environment express significantly higher levels of MMPs, further exacerbating cartilage matrix degradation (Seow et al., 2024). Elevated glucose levels also inhibit the differentiation of MSCs into chondrocytes, impairing the regenerative capacity of damaged cartilage in OA (Courties and Sellam, 2016).
The effects of insulin on chondrocytes are concentration-dependent. Low concentrations of insulin may exert protective effects by promoting the synthesis of proteoglycans and type II collagen, while high concentrations of insulin may inhibit autophagy and induce the release of inflammatory mediators, thereby exacerbating cartilage degradation (Ribeiro et al., 2016). This dual effect may be related to differences in insulin receptor signaling at different concentrations. For example, low concentrations of insulin may promote chondrocyte anabolism by activating the Akt signaling pathway, while high concentrations of insulin may inhibit autophagy through the protein kinase B/mechanistic target of rapamycin (Akt/mTOR) pathway, leading to cartilage matrix degradation and enhanced inflammatory responses (Zhou et al., 2023).
Significant changes in the expression of transcription factors and protein kinases involved in the life cycle of chondrocytes have been observed in OA patients with diabetes. In chondrocytes from OA and diabetic patients, the microtubule-associated protein 1 light chain 3 (LC3) expression is reduced, and phosphorylating ribosomal S6 protein kinase (p-rpS6) expression is increased, which is attributed to autophagy defects (Ribeiro et al., 2016; Zhou et al., 2018). Autophagy is a key mechanism for maintaining chondrocyte homeostasis, and the inability to clear dysfunctional organelles and macromolecules in the absence of effective autophagy suggests a poor disease prognosis (de Figueroa et al., 2015). Higher protein kinase C (PKC) phosphorylation has been observed in MSCs maintained under high glucose conditions prior to chondrogenesis (Tsai et al., 2013b). Transforming growth factor-β (TGF-β)-stimulated Wingless (Wnt)-5a overexpression activates PKC-mediated mitogen-activated protein kinases, signaling the differentiation of chondrogenic cells into functional cells (Matta and Mobasheri, 2014). Vascular endothelial growth factor (VEGF) is hypothesized to mediate cartilage catabolism and endochondral ossification in osteoarthritis (Zupan et al., 2018). Since VEGF is significantly upregulated under hyperglycemic conditions (Zhang, 2018a), it is reasonable to assume that VEGF expression is higher in diabetic osteoarthritis compared to OA alone (Tsai et al., 2013a). SRY-box transcription factor 9 (SOX9), a chondroprotective factor typically downregulated in OA, is further reduced in diabetic osteoarthritis (Haseeb et al., 2021).
MSC-Exos demonstrate significant advantages in the treatment of OA and T2DM. They enhance therapeutic effects by delivering active molecules such as microRNAs (miRNAs) and proteins to target cells (Ma et al., 2022), while also regulating inflammation, promoting tissue repair, and improving metabolism, showcasing potential through multi-target and multi-pathway mechanisms (Morente-López et al., 2022; Satyadev et al., 2023). In contrast, traditional therapies typically address only a single pathological aspect. For example, the long-term efficacy of anti-inflammatory drugs in alleviating OA symptoms has not been confirmed, and their use may lead to side effects (Rizzo et al., 2023); insulin therapy may induce hypoglycemia (Jadawji et al., 2018); and hypoglycemic agents carry risks such as weight changes and gastrointestinal discomfort, requiring frequent administration (Chawla et al., 2023).
Regarding MSC therapy, Phase I trials (Matas et al., 2024) have shown that MSCs can improve pain and joint function in OA patients, but larger-scale trials with control groups are needed for validation. Phase II studies (Lian et al., 2023) indicate that MSC treatment for T2DM is well-tolerated, though it may cause transient fever, hypoglycemia, fatigue, decreased lymphocyte levels, and increased inflammatory factors post-infusion. Despite their immunomodulatory and tissue-regenerative capabilities, MSCs still face challenges such as unstable cell sources, potential tumorigenic risks, infusion-related toxicity (Jeong et al., 2011; Fennema et al., 2017; Ma et al., 2022; Chawla et al., 2023), and negative effects on joints in metabolic mild OA (Warmink et al., 2023).
In comparison, MSC-Exos, being cell-free, avoid risks of immune rejection and abnormal cell proliferation (Lee, 2018). Due to the lower expression of surface proteins (e.g., major histocompatibility complex), MSC-Exos exhibit lower immunogenicity than their parent cells (Murphy et al., 2019). Engineered exosome strategies (Komuro et al., 2022) can further enhance their bioactivity and bioavailability. Therefore, MSC-Exos not only inherit the regenerative properties of MSCs (Basu and Ludlow, 2016) but also demonstrate superior targeting, stability, and broader mechanisms of action, offering more significant advantages over traditional therapies and MSCs.
Extracellular vesicles (EVs) are present in bodily fluids, secreted by cells, and possess a membranous structure. The particles can be categorised into four divisions based on their size: exosomes (30–150 nm), microvesicles (100–1,000 nm), apoptotic bodies (50–5,000 nm, formed after cell apoptosis), and oncosomes (1–10 μm), recently identified and detected in cancer cells (Kou et al., 2022). Abundant studies indicate that exosomes and microvesicles play a pivotal role in the functioning of EVs in various physiological and pathological processes (Jeppesen et al., 2019).
Exosomes are tiny vesicles that have been discovered to have a density ranging from 1.11 to 1.19 g/mL. When observed under an electron microscope, they exhibit a characteristic disk-like structure and a flat spherical shape (Colombo et al., 2014). Various types of cells present in different bodily fluids and cell supernatants have the ability to release exosomes in both normal and pathological circumstances (Kou et al., 2022).
Exosomes often arise from the endosomal system by processes such as internal budding and invagination of the plasma membrane. This is commonly followed by the production of multivesicular bodies (MVBs) (Kalluri and LeBleu, 2020). Endosomes are initially formed through the process of internal budding of the plasma membrane. This results in the creation of both early endosomes and late endosomes. Following a series of consecutive or dual inward folding of the plasma membrane, intraluminal vesicles (ILVs) are generated within MVBs, which serve as progenitors to exosomes. They can undergo degradation and be released into the cytoplasm through fusion with autophagosomes or lysosomes. Alternatively, they can be released into EVs through fusion with the plasma membrane, including ILVs, which leads to the production of exosomes (Piper and Katzmann, 2007) (Figure 2).
Figure 2. Biosynthesis and composition of exosomes. During the formation of exosomes, early endosomes encapsulate extracellular components and membrane proteins, forming multivesicular bodies (MVBs) that contain intraluminal vesicles (ILVs). These MVBs ultimately release exosomes through fusion with the plasma membrane or undergo degradation by fusing with autophagosomes or lysosomes. Exosomes are composed of lipids, proteins, nucleic acids, enzymes, and other components.
Exosome isolation methods each have their own advantages and disadvantages. Ultracentrifugation is the “gold standard” for exosome isolation, with approximately 80% of studies using this technique (Ludwig et al., 2018). Its advantages include no need for complex sample preparation and low cost, but it is time-consuming and offers moderate purity. Ultrafiltration is fast and high-throughput but may damage exosomes due to shear stress or cause loss due to membrane clogging (Li et al., 2017), reducing yield and prolonging processing time (Dehghani et al., 2020). Precipitation offers high yield but lower purity and is often combined with other methods (Hammerschmidt et al., 2016). Immunoaffinity capture achieves high specificity and preserves biological activity but is limited by antibody availability, small sample capacity, and long incubation times (Yang et al., 2020). Size-exclusion chromatography (SEC) is gentle and efficient, maintaining vesicle integrity (Konoshenko et al., 2018), but requires pre-processing and results in low sample concentration, often needing additional enrichment steps. Commercial kits and emerging technologies (e.g., microfluidics and tangential flow filtration) significantly improve yield and purity (Abreu et al., 2022).
After isolation, exosomes are often validated using transmission electron microscopy (TEM) (Lai et al., 2022). Exosomes are rich in bioactive molecules, including proteins (e.g., receptors, enzymes, transcription factors), nucleic acids (e.g., DNA, RNA), and lipids (Valadi et al., 2007; Simpson et al., 2009). Most exosomes carry conserved proteins, such as tetraspanins (CD81, CD63, CD9), heat shock proteins (HSP60, HSP70, HSP90), ALIX, and TSG101, which are widely used as exosome biomarkers (Zhang et al., 2019). Studies show that MSC-Exos consistently express CD9, CD63, CD81, and TSG101 but do not express calnexin and cytochrome C, making these markers commonly used for MSC-Exos characterization (Maumus et al., 2020) (Figure 3).
Figure 3. Cell source, isolation, and characterization of MSC-Exos. It was created with BioRender (www.biorender.com).
MSCs can be isolated from a variety of tissues, including adipose tissue, bone marrow, umbilical cord, synovium, or infrapatellar fat pad. The phenotype and function of MSC-Exos may vary depending on the tissue origin of the MSCs (Börger et al., 2017). Research has shown that MSC-Exos derived from different human tissues exhibit distinct biological properties and therapeutic effects in vivo (Wang et al., 2017). Therefore, differences in the tissue origin of MSC-Exos may influence their therapeutic efficacy.
The delivery route of MSC-Exos can influence therapeutic efficacy, and different routes are used depending on the disease. For example, intra-articular injection of bone marrow mesenchymal stem cell-derived exosomes (BM-MSC-Exos) is used to treat OA patients (Do et al., 2020), nebulized umbilical cord mesenchymal stem cell-derived exosomes (UC-MSC-Exos) is administered to treat severe COVID-19 patients (Chu et al., 2022), and intrathecal injection of UC-MSC-Exos is applied for patients with complete subacute spinal cord injury (Akhlaghpasand et al., 2024). However, the standardization of MSC-Exos dosing remains challenging, as different studies use varying units of measurement: some studies use micrograms by weight, others use particle counts, while some simply reference the number of MSCs used to generate the MSC-Exos (Lotfy et al., 2023). This heterogeneity in dosing metrics makes direct comparison of results across studies difficult and highlights the urgent need to establish a unified dosing standard.
Given these issues, future preclinical studies must systematically investigate critical parameters such as the selection of MSC-Exo sources, determination of the minimum effective dose, and optimization of administration routes to develop disease-specific individualized treatment protocols. These studies will provide essential theoretical foundations and practical guidance for the clinical application of MSC-Exos.
The proteins included in the MSC-Exos belong to a distinct protein subclass that governs their distinctive biological roles (Kalluri and LeBleu, 2020). Simultaneously, the enclosed mRNA and miRNA within MSC-Exos serve as the fundamental components for their functionality (Qiu et al., 2018). MSC-Exos facilitate information transfer and communication with target cells by delivering a variety of molecules, including cytokines, growth factors, signaling lipids, mRNAs, and regulatory miRNAs, thereby altering the activities and functions of the target cells (Phinney and Pittenger, 2017). Studies have shown that MSC-Exos possess the ability to protect molecules from degradation and facilitate their efficient uptake into cells through endocytosis (Bagno et al., 2018). Additionally, MSC-Exos can serve as an ideal carrier system for transiently regulating specific biological processes in target cells (Rao et al., 2022), with the advantage of enabling cell-type-specific targeted delivery through surface modifications (Yang et al., 2018). MSC-Exos which not only possess immunomodulatory and tissue regeneration capabilities but also demonstrate certain advantages in immune therapy (Wu et al., 2018). These regenerative properties make MSC-Exos a promising tool for cell-free therapy in the treatment of various diseases.
Exosomal miRNAs can be absorbed by nearby or distant cells, exerting biological effects by inhibiting target genes in recipient cells (Qin et al., 2019). These miRNAs are stable in circulation and are secreted by donor cells to act on distant recipient cells, regulating their gene expression (Broussard et al., 2016). miRNAs play a crucial role in gene expression regulation, and their functions can be divided into two categories:one is the classic negative regulatory function, where they inhibit gene expression by targeting mRNA; the other is a newly discovered function, such as acting as ligands to bind toll-like receptors (TLRs) and activate immune cells (Zhang et al., 2015). For example, exosomal miR-21 and miR-29a not only target mRNA but also trigger immune responses by binding to TLRs (Fabbri et al., 2012), a discovery that has opened new directions for miRNA functional research.
The expression levels of exosomal miRNAs can change with physiological conditions (Skog et al., 2008), and their surface proteins can also reflect their cellular or tissue origins (Mathivanan et al., 2010). Additionally, differences in the quantity and composition of exosomal miRNAs between diseased individuals and healthy individuals make them potential non-invasive biomarkers for early disease diagnosis and monitoring. Furthermore, exosomes can not only transport endogenous miRNAs but also sort and transfer exogenous miRNAs (Pegtel et al., 2010), a mechanism similar to that of endogenous miRNAs, providing new perspectives for studying intercellular communication.
In exosome functional studies, researchers use diverse technical approaches for comprehensive analysis. Proteomic analysis employs tandem mass spectrometry (labeling and label-free techniques), while miRNA profiling relies on high-throughput sequencing (Bi et al., 2022). Functional effects are explored through meta-analysis integrating miRNA and proteomics data. Bioinformatics tools like miRPathDB translate miRNA profiles into target gene information for pathway analysis (Ding et al., 2024). Validation of exosomal proteins and miRNAs is performed using Western blot and RT-qPCR. These integrated technologies provide a robust framework for exosome research.
In disease research, the genetic mechanisms of T2DM and OA are not yet fully understood, making the identification of new therapeutic targets crucial for early diagnosis and specific intervention. In recent years, computer simulation methods have shown great potential in disease research, such as predicting disease-related molecular functions (Raghav and Mann, 2024), lncRNA-miRNA interactions (Wang et al., 2022), and associations between metabolites (Sun et al., 2022). Comprehensive analysis based on transcriptomic data can identify differentially expressed genes (DEGs) and reveal their biological functions in diseases (Song and Yu, 2024). For example, the miR-29 family (including miR-29a, miR-29b, and miR-29c) plays an important role in the pathogenesis of T2DM and OA (Marttila et al., 2021; Mao et al., 2024).
Combining exosome analysis with disease research holds promise for discovering new therapeutic targets. Moreover, predicting biomarkers not only saves research time but also has potential clinical value. Research based on miRNAs provides new insights for disease diagnosis and treatment, and future studies could further explore their potential applications in precision medicine.
The phosphoinositide 3-kinase (PI3K)/Akt/mTOR signaling pathway can be activated by various molecules, including insulin, glucose, growth factors, and cytokines (Engelman et al., 2006), playing a crucial role in OA and T2DM. Intervention strategies for OA based on this pathway can be divided into two categories: (1) inhibiting the pathway to restore cartilage homeostasis, enhance autophagy, and reduce inflammation, thereby alleviating joint damage; and (2) activating the pathway to promote chondrocyte proliferation and reduce apoptosis, exerting anti-arthritic effects (Sun et al., 2020). Under normal conditions, the PI3K/Akt pathway regulates metabolism and function, but its abnormal activation (e.g., overexpression or mutation) may lead to diseases such as obesity and cancer (Huang et al., 2018). The role of the mTOR pathway is dual, as it can either counteract or promote diabetes, depending on the cell type and physiological context (Jurca et al., 2023).
Insulin regulates inflammation, metabolism, and immune responses through the PI3K/Akt/mTOR pathway. In OA, insulin activates the PI3K/Akt/mTOR pathway, inhibits autophagy, and exacerbates cartilage degradation (Qiao et al., 2020). In immune regulation, insulin inhibits Toll-like receptor 4 (TLR4) and NF-κB through PI3K/Akt, exerting anti-inflammatory effects (Zhang et al., 2016), but it may also exacerbate inflammation by promoting Th17 differentiation and suppressing Treg function (Shi et al., 2019). In T Cells, insulin enhances glucose uptake and protein synthesis through the PI3K-Akt-mTOR pathway, promoting T Cell activation (Stentz and Kitabchi, 2003). Additionally, insulin influences immune responses by regulating macrophage polarization (M1 to M2 transition) and neutrophil function (Klauder et al., 2020). In bone metabolism, insulin promotes osteoblast differentiation and bone formation through PI3K/AKT/mTOR, while also promoting osteoclastogenesis via extracellular signal-regulated kinase 1/2 (ERK1/2) (Xian et al., 2012; Oh and Lee, 2017). mTOR complex 1 (mTORC1) plays a dual role in osteoblast and osteoclast differentiation, as its overactivation may inhibit osteoclastogenesis, while its inhibition may promote osteoclast differentiation (Hiraiwa et al., 2019).
MSC-Exos regulate the PI3K/Akt/mTOR pathway by delivering miRNAs and other bioactive molecules, demonstrating significant immunomodulatory and therapeutic potential. For example, MSC-Exos promote M2 macrophage polarization by delivering miRNAs such as miR-122-5p and miR-148a-3p (Li K. et al., 2022); infrapatellar fat pad mesenchymal stem cell-derived exosomes (IPFP-MSC-Exos) inhibit the mTOR autophagy pathway via miR-100-5p, maintaining cartilage homeostasis (Wu et al., 2019); synovial mesenchymal stem cell-derived exosomes (SMSC-Exos) carrying miR-485-3p alleviate OA cartilage damage by targeting the NRP1-mediated PI3K/Akt pathway (Qiu et al., 2024). Furthermore, BM-MSC-Exos reduce IR and obesity in mice through the PI3K/AKT pathway (Shi et al., 2023). Engineering approaches can enhance exosome efficacy. For instance, Wu et al. (2024) activated the PI3K/Akt pathway in infrapatellar fat pad-derived mesenchymal stem cell (IPFP-MSCs) through TNF-α pretreatment, upregulating autophagy-related protein 16 like 1 (ATG16L1) and promoting the secretion of low-density lipoprotein receptor-related protein 1 (LRP1)-enriched exosomes, effectively preventing OA cartilage damage.
The formation of the NOD-like receptor family pyrin domain-containing 3 (NLRP3) inflammasome involves activation, assembly, and regulation. Its core mechanism involves the interaction of NLRP3 as a “receptor” and pro-Caspase-1 as an “effector.” Upon assembly, the inflammasome activates pro-Caspase-1, generating active Caspase-1, which processes pro-IL-1β and pro-IL-18 into mature IL-1β and IL-18, amplifying the inflammatory response. Simultaneously, NLRP3 inflammasome activation induces pyroptosis, further exacerbating inflammatory cell death (Broz, 2019).
In OA, pro-inflammatory factors such as IL-1β, IL-18, and TNF-α accelerate disease progression by increasing cartilage ECM degradation (Shi et al., 2019). Macrophages, the primary cells regulating OA inflammation, activate the NLRP3 inflammasome (Sanchez-Lopez et al., 2019), releasing various pro-inflammatory factors (Zhang et al., 2020) and cytokines (e.g., IL-1β and IL-18), further exacerbating OA inflammation and cartilage destruction.
In T2DM, glycolipid metabolites (e.g., glucose and free fatty acids) activate the NLRP3 inflammasome through multiple pathways (Wang et al., 2020). Chronic hyperglycemia and saturated fatty acids (e.g., palmitate) activate the NLRP3 inflammasome, promoting the secretion of IL-1β and IL-18, which interfere with insulin signaling and lead to IR (Vandanmagsar et al., 2011). Additionally, islet amyloid polypeptide (IAPP) deposits activate the NLRP3 inflammasome by disrupting lysosomes, triggering inflammatory responses (Masters et al., 2010). Studies have shown that inhibiting NLRP3 inflammasome activation (e.g., through Caspase-1 inhibitors or gene knockout) can alleviate β-cell inflammation and improve IR (Qi et al., 2019b).
Mesenchymal stem cell-derived EVs (MSC-EVs) show potential in OA treatment by slowing disease progression, promoting chondrocyte proliferation and migration, and inhibiting chondrocyte apoptosis. EVs carrying miR-1208 inhibit inflammation by targeting methyltransferase-like 3 (METTL3) to reduce NLRP3 mRNA methylation (Zhou et al., 2022). Liu et al. (2023) loaded exogenous miR-223 into MSC-EVs via electroporation and modified the MSC-EVs surface with a collagen II-targeting peptide (WYRGRL) through genetic engineering, achieving more targeted and efficient RNA delivery to cartilage. These dual-engineered EVs significantly inhibit NLRP3 inflammasome activation and chondrocyte pyroptosis, providing a novel strategy for OA treatment.
Multiple signaling pathways have been shown to play roles in OA and T2DM progression, but further research is needed to identify specific common targets (e.g., miRNAs and target genes). MSC-Exos demonstrate broad prospects in modulating inflammation and promoting tissue repair, but their precise mechanisms require further elucidation. Future research should focus on exploring the intersections of these signaling pathways to develop more precise therapeutic approaches.
The gradual degradation of cartilage matrix is the key pathology of OA, leading to structural damage of joints and subsequent injury. To stimulate the redeposition of cartilage ECM and preserve the structural integrity of cartilage, it is imperative to initiate repair mechanisms in chondrocytes and increase the expression of genes related to synthetic metabolism (Heard et al., 2015).
Several studies have shown that MSC-Exos help to maintain the equilibrium of the ECM. Zhang et al. (2018b) discovered that embryonic stem cell-derived MSC exosomes promoted the synthesis of important cartilage matrix components such as sulfated glycosaminoglycans and type II collagen, accelerating chondrocyte repair. Jammes et al. (2023) discovered that BM-MSC-Exos effectively enhanced the production of a matrix resembling transparency. This was achieved by controlling the amounts of collagen proteins, promoting the expression of proliferating cell nuclear antigen, and decreasing the synthesis of Htra1. Woo et al. (2020) showed that adipose-derived mesenchymal stem cell-derived exosomes (AD-MSC-Exos) effectively enhanced the expression of type II collagen in chondrocytes while reducing the expression of ADAMTS-5, MMP-1, MMP-3, and MMP-13, thereby alleviating cartilage matrix degradation in a monoiodoacetate-induced OA model.
Exosomes RNA has shown significant potential in promoting cartilage ECM repair. Chen et al. (2020) found that miR-136-5p from bone marrow-derived mesenchymal stem cells (BM-MSCs) upregulated the expression of type II collagen, aggrecan, and SOX9, while downregulating MMP-13 expression, thereby promoting chondrocyte migration. Xia et al. (2021) showed that BM-MSC-exosomal miR-125a-5p alleviated chondrocyte ECM degradation in post-traumatic OA by inhibiting E2F2. Zhou et al. (2022) discovered that UC-MSC-Exos suppressed the breakdown of ECM in a mouse model of OA by using miR-1208.
In the inflammatory environment and conditions of KOA, chondrocyte metabolic homeostasis is disrupted, leading to cartilage remodeling characterized by enhanced glycolytic pathway, mitochondrial dysfunction, and chondrocyte senescence (Mobasheri et al., 2017). Chen et al. (2019a) found that supplementing mitochondrial-related proteins using MSC-Exos in degenerated cartilage restored mitochondrial dysfunction and oxidative stress damage, thus rescuing energy metabolism imbalance and promoting cartilage regeneration. Wu et al. (2019) discovered that IPFP-MSC-Exos suppressed chondrocyte apoptosis by inhibiting the mTOR signaling pathway targeted at chondrocytes, thereby regulating chondrocyte metabolism and promoting ECM regeneration. Qi et al. (2019a) pointed out that BM-MSC-Exos enhance the phosphorylation of Akt while decreasing the phosphorylation of ERK and p38, which leads to the inhibition of chondrocyte apoptosis produced by mitochondria.
Cartilage’s avascular nature and limited interchange of signaling chemicals, oxygen, and nutrients pose considerable difficulties to its self-repair capacities (Carballo et al., 2017). MSC-Exos have exhibited robust capacities in selectively influencing biological processes such as the growth and death of chondrocytes (Xiang et al., 2022). Another study confirmed that AD-MSC-Exos-derived exosomal miR-338-3p transplantation can inhibit chondrocyte inflammation and degradation by targeting Runt-related transcription factor 2 (RUNX2), while promoting chondrocyte proliferation (Li C. et al., 2022a). Li et al. (2021) revealed that exosomes derived from induced pluripotent stem cell-derived MSCs and synovial fluid mesenchymal stem cell-derived exosomes (SF-MSC-Exos) significantly promoted chondrocyte proliferation and migration.
The progression of OA is directly related to the extent of inflammatory infiltration. The secretion of inflammatory cytokines triggers immune responses that contribute to the development and advancement of OA. Several studies have demonstrated that MSC-Exos can regulate inflammation by decreasing the levels of pro-inflammatory cytokines and stimulating the secretion of anti-inflammatory cytokines (Hassanzadeh et al., 2023). Furthermore, synovial macrophages are the primary immune cells in the knee joint, MSC-Exos can inhibit macrophage recruitment.
Song et al. (2023) discovered that MSC-Exos can suppress macrophage ferroptosis through the activation of the GOT1/CCR2/Nrf2/HO-1 signaling pathway. As a result, they can effectively repair cartilage degradation in OA. Ragni et al. (2020) confirmed protective and anti-inflammatory activity against macrophages and chondrocytes in computer simulations, consistent with in vitro findings that the MSC secretome and its initiation inhibited chondrocyte catabolism and inflammatory markers as well as macrophage activation. Cosenza et al. (2018) studied the immunosuppressive impacts of microvesicles and exosomes produced from MSCs on T and B lymphocytes in laboratory settings and in models of delayed-type hypersensitivit and collagen-induced arthritis.
In the pathogenesis of KOA, inflammatory responses play a crucial role. The key determinants in managing this condition seem to be the equilibrium between pro-inflammatory cytokines and anti-inflammatory cytokines, which have opposing influences. The former primarily include IL-1β, TNF-α, IL-6, IL-15, IL-17, and IL-18. Conversely, anti-inflammatory cytokines induced by TNF include IL-4, IL-10, IL-13, IL-37, among others.
Zhou et al. (2022) revealed that MSC-Exos effectively inhibit the activation of the NOD-like receptor family pyrin domain containing 3 (NLRP3) inflammasome in macrophages, resulting in reduced secretion of IL-1β and IL-18, thereby successfully alleviating OA. Wang et al. (2024) demonstrated that exosomes-shuttled lncRNA small nucleolar RNA host gene 7 (SNHG7) by BM-MSCs alleviates OA through targeting miR-485-5p/ferroptosis suppressor protein 1 (FSP1) axis-mediated chondrocytes ferroptosis and inflammation. Qiu et al. (2021) showed that SMSC-Exos containing miR-129-5p mitigate inflammation induced by IL-1β in OA by inhibiting high mobility group box 1 (HMGB1) release (Table 1).
Inflammation is a critical factor in the development of IR that is linked to T2DM associated with obesity. M1 macrophages located in adipose tissue release many substances, including TNF-α, IL-6, IL-1β, and monocyte chemotactic protein-1. These substances promote inflammation and initiate IIR in insulin-responsive cells such as adipocytes, skeletal muscle cells, and pancreatic cells (Olefsky and Glass, 2010; Tateya et al., 2013).
Su et al. (2019) reported that BM-MSC-Exos containing miR-29b regulates age-related IR by targeting sirtuin (SIRT)1 in adipocytes, muscle cells, and liver cells. Chen et al. (2021) found that UC-MSC-Exos enhance insulin sensitivity in human adipocytes by inhibiting the production of the adipokine leptin and increasing the mRNA expression of adiponectin, SIRT1, and insulin receptor substrate-1 (IRS-1). Shi et al. (2023) found that BM-MSC-Exos increased glucose uptake and improved IR in high-fat diet-fed mice and palmitic acid-treated 3T3-L1 adipocytes by activating the phosphoinositide 3-kinase (PI3K/Akt) signaling pathway and upregulating GLUT4 expression. Sun et al. (2018) demonstrated that intravenous administration of UC-MSC-Exos significantly reduced blood glucose levels and partially reversed IR in a rat model of T2DM induced by a high-fat diet and streptozotocin (STZ). The treatment with UC-MSC-Exos restored the phosphorylation of IRS-1 and Akt at tyrosine sites, thereby enhancing the expression and membrane translocation of GLUT-4 in muscle tissues. Additionally, UC-MSC-Exos promoted hepatic glycogen storage, contributing to the maintenance of glucose homeostasis. Furthermore, UC-MSC-Exos inhibited STZ-induced β cell apoptosis and restored insulin secretion function in T2DM.
One major physiological barrier for patients with T2DM is inadequate insulin secretion, leading to sustained hyperglycemia. Prolonged high blood glucose levels can deplete insulin stores and lead to compensatory insulin secretion, which damages pancreatic β cells.
Studies have shown that umbilical cord-derived mesenchymal stem cells (UC-MSCs) can induce insulin-producing cells in vitro but do not differentiate into pancreatic β cells in vivo, mainly exerting their effects through paracrine actions in T2DM (Nagaishi et al., 2016). In a study by Sharma et al. (2021), treatment with UC-MSC-Exos via intravenous injection reduced hyperglycemia in T2DM mice, increased insulin production, and improved tissue structure. Analysis of pancreatic tissue samples revealed elevated expression of genes involved in pancreatic tissue regeneration pathways (Reg2, Reg3, and Amy2b). MiRNA analysis of MSC-Exos indicated potential promotion of pancreatic regeneration pathways, possibly through modulation of the Extl3-Reg-cyclinD1 pathway. These results suggest that UC-MSC-Exos have therapeutic potential in alleviating insulin deficiency by activating pancreatic regeneration. Additionally, Xia et al. (2024) demonstrated that MSC-Exos suppressed nuclear factor erythroid 2 related factor (NRF2)-mediated ferroptosis by delivering bioactive proteins to regulate the Akt/ERK signaling pathway, thereby improving the function and quantity of β cells. They modified the β cell targeting aptamer with polyethylene glycol on the membrane surface of exosomes, and the former mediated β cell targeting was more effective in islet protection compared to unmodified MSC-Exos (Table 2).
Engineering strategies aim to overcome the limitations of natural exosomes through various approaches (Komuro et al., 2022). Currently, the application of engineered MSC-Exos in OA treatment primarily focuses on content modification, membrane property optimization, and integration with biomaterials to enhance their bioactivity and bioavailability (Figure 4).
Figure 4. Engineering strategies for MSC-Exos. To enhance the therapeutic efficacy of MSC-Exos, various engineering strategies have been developed to improve their yield, bioactivity, and bioavailability. It was created with BioRender (www.biorender.com).
Cell modification is a method to indirectly alter the contents or membrane properties of exosomes before isolation, serving as a rapid response mechanism of parental cells to environmental stimuli (Lai et al., 2014). The simplest approach for cargo loading prior to isolation involves co-incubating the target cargo with exosome-secreting cells, allowing the cargo to diffuse into the exosomes via a concentration gradient (Oskouie et al., 2018). Additionally, transfection techniques can be employed to introduce specific plasmids into cells, enabling the ectopic expression of target biomolecules within exosomes. Physical methods such as sonication, electroporation, extrusion, freeze-thaw cycles, surfactant treatment, and dialysis are also widely utilized (Luan et al., 2017). Research indicates that natural exosomes enter recipient cells through free diffusion and are subsequently internalized randomly (Lai et al., 2014).
The modification of MSC-Exos content primarily relies on the overexpression of specific non-coding RNAs to enrich therapeutic molecules. Studies have shown that miRNAs play a crucial regulatory role in exosome-mediated cartilage repair (Foo et al., 2021). Currently, researchers commonly use transfection tools such as viral vectors (Chen, 2019b), plasmids (Li F. et al., 2022), and liposomes (Liu, 2021b) to genetically engineer parent cells, thereby obtaining modified exosomes carrying specific molecules. Tao et al. (2017) found that miR-140-5p-enriched exosomes secreted by synovial mesenchymal stem cells (SMSCs) through transfection technology significantly promoted chondrocyte proliferation and migration, effectively alleviated symptoms in a rat OA model, and did not compromise ECM secretion. Morente-López et al. (2022) reported that EVs derived from UC-MSCs with lentivirus-induced miR-21 inhibition effectively reduced the levels of chemokines and cytokines in the serum of OA animals and decreased the senescence-associated secretory phenotype.
Modulating the production environment of MSC-Exos for engineering transformation has emerged as a highly promising research direction, encompassing two major aspects: biochemical factors and biophysical factors. In terms of biochemical regulation, TNF-α pretreatment significantly enhances IPFP-MSC-Exos and improves their therapeutic efficacy for OA (Wu et al., 2024). MSC-Exos derived from curcumin-induced adipose tissue exhibit enhanced antioxidant stress and anti-chondrocyte apoptosis capabilities, thereby improving their therapeutic efficacy for OA (Xu et al., 2022). Additionally, exosomes produced by strontium-substituted calcium silicate-treated bone marrow mesenchymal stem cells demonstrate a unique dual regulatory function, promoting both osteogenesis and angiogenesis (Liu, 2021a). Regarding biophysical regulation, studies have shown that hypoxia-treated MSCs not only increase exosome yield but also enhance their ability to promote cell proliferation and differentiation (Pulido-Escribano et al., 2022). Notably, MSC-EVs generated under hypoxic conditions (Hypo-EVs) exhibit superior efficacy in OA cartilage repair compared to exosomes produced under normoxic conditions (Rong et al., 2021). Furthermore, Yan and Wu (2019) demonstrated that UC-MSC-Exos cultured in a 3D hollow fiber bioreactor not only show increased yield but also significantly enhanced cartilage-protective effects.
Direct exosome engineering refers to the post-purification process of modifying exosomes through physical, chemical, or genetic methods to enhance their cargo or membrane properties, thereby improving their targeting capabilities and/or therapeutic efficacy. Some modification techniques originally applied to parent cells can also be directly utilized on exosomes (Zhu et al., 2024).
Zhao et al. (2023) discovered that cartilage-affinity peptide (CAP)-conjugated exosomes derived from subcutaneous fat MSCs could specifically deliver miR-199a-3p to target cells and deep joint tissues, significantly impacting OA progression. Zhuang et al. (2023) developed superparamagnetic iron oxide nanoparticle (SPION)-modified exosomes to load quercetin, leveraging magnetic force (MF) to enhance quercetin’s water solubility and active targeting ability, thereby improving islet protection. These findings serve as valuable references, offering new strategies to enhance the therapeutic efficiency of MSC-EVs through direct modification of isolated EVs. Additionally, Luo et al. (2019) modified exosomes with aptamers via Schiff base reactions between aldehydes and amino groups, promoting bone repair by enhancing the targeting ability of BM-MSCs. A lipid-based strategy involving the combination of antagomir-188-loaded liposomes with exosomes from cells engineered with the CXC motif chemokine receptor 4 gene was employed to prevent the shift of BM-MSCs’ osteogenic differentiation toward adipogenesis, without requiring covalent modification (Hu et al., 2021). The underlying mechanism of non-covalent methods involves electrostatic interactions between the cargo and exosomes.
The suboptimal therapeutic efficacy of OA is partly attributed to the inefficient drug delivery within the knee joint. By combining with biomaterials, MSC-Exos can achieve effective retention at pathological sites, improve drug delivery pathways, and thereby significantly enhance therapeutic outcomes (You et al., 2023).
Hydrogels, owing to their injectability and cross-linking capability under ultraviolet light, have become ideal biomaterials for tissue engineering. Their raw materials include natural polymers (such as polysaccharides like hyaluronic acid, alginate, and chitosan, as well as proteins like collagen, gelatin, and silk fibroin), synthetic polymers (such as polyglycolic acid, polycaprolactone, and polylactic acid), and composite materials (Bahraminasab et al., 2021). Research by Pang et al. (2023) demonstrated that gelatin methacryloyl hydrogel (GelMA) prolongs the release of MSC-Exos and significantly enhances their therapeutic efficacy against OA. Furthermore, advanced manufacturing methods such as electrospinning, 3D printing, and microfluidic technology have facilitated the development of nanogel/microgel porous scaffolds (Pishavar et al., 2021), further enriching their synergistic effects with exosomes. For instance, Yang et al. (Yang et al., 2023) developed an enzyme-responsive smart hydrogel using microfluidic chips by combining neovascularized bone matrix metalloproteinase-1, self-assembling hydrogels, and exosomes to synthesize injectable microgels. This hydrogel can specifically recognize neovascularization and precisely release exosomes in a spatiotemporal manner, promoting angiogenesis and osteogenesis.
Multidimensional modification strategies not only optimize the functional properties of exosomes but also open up broader prospects for their application in disease treatment. For example, Chen et al. (2024) utilized CRISPR/Cas9 technology to develop a targeted gene editing tool for fibroblast growth factor FGF18, delivered via CAP-conjugated hybrid exosomes (CAP/FGF18-hyEXO). This approach effectively activated the FGF18 gene in OA chondrocytes at the genomic level in vivo. The study confirmed that this strategy synergistically promotes cartilage regeneration, reduces inflammation, and prevents ECM degradation both in vitro and in vivo, demonstrating significant potential for clinical translation.
Exosome-based cell-free approaches have shown promising results in the treatment of OA and T2DM. However, research on using MSC-Exos to treat diabetic OA remains limited. In studies on diabetic complications, Jin et al. (2019) demonstrated that AD-MSC-Exos alleviates diabetic nephropathy by promoting podocyte autophagy flux and inhibiting apoptosis. Hu et al. (2023) showed that hypoxia-preconditioned ADSC-Exos embedded in hydrogels promote angiogenesis and accelerate diabetic wound healing. Cao et al. (2021) proved that MSC-Exos inhibit endothelial-mesenchymal transition and tube formation in diabetic retinopathy. These studies provide valuable references for exosome-based therapies targeting T2DM complicated by OA.
The biological functions of MSC-Exos vary depending on their sources (Börger et al., 2017). Exploring the characterization of MSC-Exos across different subpopulations and accurately determining their cargo content is crucial, as it may significantly alter their impact on target tissues (Forsberg et al., 2020). In both preclinical and clinical studies, the specific minimum effective dose of MSC-Exos has not yet been determined. The most commonly used route in preclinical studies is intravenous injection (Hassanzadeh et al., 2021), along with intraperitoneal and subcutaneous injections. For OA treatment, intra-articular injection is employed (Do et al., 2020). There is an urgent need to standardize the parental MSC source, therapeutic dose, and administration routes for MSC-Exos products.
The application of exosomes as biologics in clinical settings faces multiple challenges, including standardization, safety, and quality control issues, such as the lack of standardized methods for collection and isolation, the inherent heterogeneity of exosomes, and contamination from exogenous sources. To ensure the safety and efficacy of clinical-grade exosome preparations, it is essential to adhere to Good Manufacturing Practice (GMP) protocols and use serum-free media to avoid animal-derived contamination. By studying the mechanisms of action of exosomes, regulating their key active components, and employing bioengineering techniques to modify exosome phenotypes or contents, therapeutic efficacy can be enhanced while reducing adverse effects (Ferreira et al., 2022). Additionally, screening exosome biomarkers (e.g., surface receptors) and optimizing isolation and purification methods can help obtain more homogeneous and potent exosome populations (Chen et al., 2022). In the future, a deeper understanding of the mechanisms of action, along with the establishment of standardized production processes and quality control strategies, will further unlock the potential of exosomes in clinical applications.
Natural exosomes have limitations such as insufficient secretion, poor targeting, short retention time, and heterogeneity (Zhu et al., 2024), which hinder their use in large-scale clinical trials. To achieve therapeutic effects, MSC-Exos need to carry sufficient doses of bioactive factors (such as proteins or miRNAs) with functional activity to effectively trigger biological responses in target cells (Toh et al., 2018). For example, miRNA content can be enriched through methods such as endogenous or exogenous loading. Additionally, modifying exosomes using bioengineering techniques can further enhance their bioactivity and therapeutic efficacy. However, it is important to note that while the design of engineered exosomes holds unlimited potential, we must also consider issues such as their loading efficiency, differentiation from natural exosomes, biocompatibility when combined with biomaterials, and potential adverse effects that may arise from engineered exosome therapy.
MSC-Exo therapy represents a highly promising cell-free therapeutic approach. The application of bioengineering technologies has significantly enhanced the therapeutic efficacy of MSC-Exos. Further research is needed to elucidate the mechanisms underlying diabetic OA, establish standardized criteria for evaluating therapeutic effects and safety, optimize engineered exosome treatment strategies, and accelerate the clinical translation of MSC-Exos.
SX: Writing – original draft, Writing – review and editing. ML: Data curation, Writing – review and editing. YK: Methodology, Writing – review and editing. YY: Conceptualization, Writing – review and editing. RC: Formal Analysis, Writing–review and editing. YW: Investigation, Writing–review and editing. SC: Funding acquisition, Writing–review and editing. YS: Funding acquisition, Writing–review and editing, Supervision.
The author(s) declare that financial support was received for the research and/or publication of this article. The work was supported by grants from Hebei Province Key R&D Plan Project (No. 22377752D), Medical Science Research Project of Hebei Province (No. 20230486), Scientific Research Fund of the Second Hospital of Hebei Medical University (No. 2HC202210), and Hebei Provincial Medical Research Project (NO. 20210934).
The authors declare that the research was conducted in the absence of any commercial or financial relationships that could be construed as a potential conflict of interest.
The author(s) declare that no Generative AI was used in the creation of this manuscript.
All claims expressed in this article are solely those of the authors and do not necessarily represent those of their affiliated organizations, or those of the publisher, the editors and the reviewers. Any product that may be evaluated in this article, or claim that may be made by its manufacturer, is not guaranteed or endorsed by the publisher.
Abreu, C. M., Costa-Silva, B., Reis, R. L., Kundu, S. C., and Caballero, D. (2022). Microfluidic platforms for extracellular vesicle isolation, analysis and therapy in cancer. Lab a Chip 22, 1093–1125. doi:10.1039/D2LC00006G
Akhlaghpasand, M., Tavanaei, R., Hosseinpoor, M., Yazdani, K. O., Soleimani, A., Zoshk, M. Y., et al. (2024). Safety and potential effects of intrathecal injection of allogeneic human umbilical cord mesenchymal stem cell-derived exosomes in complete subacute spinal cord injury: a first-in-human, single-arm, open-label, phase I clinical trial. Stem Cell. Res. and Ther. 15, 264. doi:10.1186/s13287-024-03868-0
Alenazi, A. M., Alhowimel, A. S., Alshehri, M. M., Alqahtani, B. A., Alhwoaimel, N. A., Segal, N. A., et al. (2023). Osteoarthritis and diabetes: where are we and where should we go? Diagnostics 13, 1386. doi:10.3390/diagnostics13081386
Anderson, J. R., Johnson, E., Jenkins, R., Jacobsen, S., Green, D., Walters, M., et al. (2023). Multi-omic temporal landscape of plasma and synovial fluid-derived extracellular vesicles using an experimental model of equine osteoarthritis. Int. J. Mol. Sci. 24, 14888. doi:10.3390/ijms241914888
Bagno, L., Hatzistergos, K. E., Balkan, W., and Hare, J. M. (2018). Mesenchymal stem cell-based therapy for cardiovascular disease: progress and challenges. Mol. Ther. 26, 1610–1623. doi:10.1016/j.ymthe.2018.05.009
Bahraminasab, M., Janmohammadi, M., Arab, S., Talebi, A., Nooshabadi, V. T., Koohsarian, P., et al. (2021). Bone scaffolds: an incorporation of biomaterials, cells, and biofactors. ACS Biomaterials Sci. and Eng. 7, 5397–5431. doi:10.1021/acsbiomaterials.1c00920
Barnett, L. A., Jordan, K. P., Edwards, J. J., and van der Windt, D. A. (2017). Does metformin protect against osteoarthritis? An electronic health record cohort study. Prim. Health Care Res. and Dev. 18 (06), 623–628. doi:10.1017/s1463423617000287
Basu, J., and Ludlow, J. B. (2016). Exosomes for repair, regeneration and rejuvenation. Expert Opin. Biol. Ther. 16 (4), 489–506. doi:10.1517/14712598.2016.1131976
Batrakova, E. V., and Kim, M. S. (2015). Using exosomes, naturally-equipped nanocarriers, for drug delivery. J. Control. Release 219, 396–405. doi:10.1016/j.jconrel.2015.07.030
Bi, Y., Qiao, X., Liu, Q., Song, S., Zhu, K., Qiu, X., et al. (2022). Systemic proteomics and miRNA profile analysis of exosomes derived from human pluripotent stem cells. Stem Cell. Res. and Ther. 13, 449. doi:10.1186/s13287-022-03142-1
Börger, V., Bremer, M., Ferrer-Tur, R., Gockeln, L., Stambouli, O., Becic, A., et al. (2017). Mesenchymal stem/stromal cell-derived extracellular vesicles and their potential as novel immunomodulatory therapeutic agents. Int. J. Mol. Sci. 18, 1450. doi:10.3390/ijms18071450
Broussard, J. L., Castro, A. V. B., Iyer, M., Paszkiewicz, R. L., Bediako, I. A., Szczepaniak, L. S., et al. (2016). Insulin access to skeletal muscle is impaired during the early stages of diet-induced obesity. Obesity 24, 1922–1928. doi:10.1002/oby.21562
Broz, P. (2019). Recognition of intracellular bacteria by inflammasomes. Microbiol. Spectr. 7. doi:10.1128/microbiolspec.bai-0003-2019
Burger, D., Viñas, J. L., Akbari, S., Dehak, H., Knoll, W., Gutsol, A., et al. (2015). Human endothelial colony-forming cells protect against acute kidney injury: role of exosomes. Am. J. Pathology 185 (8), 2309–2323. doi:10.1016/j.ajpath.2015.04.010
Cao, X., Xue, L. D., Di, Y., Tian, Y. J., and Song, Y. (2021). MSC-derived exosomal lncRNA SNHG7 suppresses endothelial-mesenchymal transition and tube formation in diabetic retinopathy via miR-34a-5p/XBP1 axis. Life Sci. 272, 119232. doi:10.1016/j.lfs.2021.119232
Carballo, C. B., Nakagawa, Y., Sekiya, I., and Rodeo, S. A. (2017). Basic science of articular cartilage. Clin. Sports Med. 36 (3), 413–425. doi:10.1016/j.csm.2017.02.001
Chawla, G., Pradhan, T., and Gupta, O. (2023). An insight into the combat strategies for the treatment of type 2 diabetes mellitus. Mini Rev. Med. Chem. 24, 403–430. doi:10.2174/1389557523666230517113936
Chen, J., Li, P., Zhang, T., Xu, Z., Huang, X., Wang, R., et al. (2022). Review on strategies and technologies for exosome isolation and purification. Front. Bioeng. Biotechnol. 9, 811971. doi:10.3389/fbioe.2021.811971
Chen, M., Lu, Y., Liu, Y., Liu, Q., Deng, S., Liu, Y., et al. (2024). Injectable microgels with hybrid exosomes of chondrocyte-targeted FGF18 gene-editing and self-renewable lubrication for osteoarthritis therapy. Adv. Mater. 36, e2312559. doi:10.1002/adma.202312559
Chen, M., Zhao, Y., Zhou, L., L, M., Zhang, Q., Han, Q., et al. (2021). Exosomes derived from human umbilical cord mesenchymal stem cells enhance insulin sensitivity in insulin resistant human adipocytes. Curr. Med. Sci. 41 (1), 87–93. doi:10.1007/s11596-021-2323-4
Chen, P., Zheng, L., Wang, Y., Tao, M., Xie, Z., Xia, C., et al. (2019a). Desktop-stereolithography 3D printing of a radially oriented extracellular matrix/mesenchymal stem cell exosome bioink for osteochondral defect regeneration. Theranostics 9 (9), 2439–2459. doi:10.7150/thno.31017
Chen, S., Tang, Y., Liu, Y., Zhang, P., Lv, L., Zhang, X., et al. (2019b). Exosomes derived from miR-375-overexpressing human adipose mesenchymal stem cells promote bone regeneration. Cell. Prolif. 52, e12669. doi:10.1111/cpr.12669
Chen, X., Shi, Y., Xue, P., Ma, X., Li, J., and Zhang, J. (2020). Mesenchymal stem cell-derived exosomal microRNA-136-5p inhibits chondrocyte degeneration in traumatic osteoarthritis by targeting ELF3. Arthritis Res. and Ther. 22 (1), 256. doi:10.1186/s13075-020-02325-6
Chu, M., Wang, H., Bian, L., Huang, J., Wu, D., Zhang, R., et al. (2022). Nebulization therapy with umbilical cord mesenchymal stem cell-derived exosomes for COVID-19 pneumonia. Stem Cell. Rev. Rep. 18, 2152–2163. doi:10.1007/s12015-022-10398-w
Colombo, M., Raposo, G., and Théry, C. (2014). Biogenesis, secretion, and intercellular interactions of exosomes and other extracellular vesicles. Annu. Rev. Cell. Dev. Biol. 30, 255–289. doi:10.1146/annurev-cellbio-101512-122326
Copp, G., Robb, K. P., and Viswanathan, S. (2023). Culture-expanded mesenchymal stromal cell therapy: does it work in knee osteoarthritis? A pathway to clinical success. Cell. Mol. Immunol. 20 (6), 626–650. doi:10.1038/s41423-023-01020-1
Cosenza, S., Toupet, K., Maumus, M., Luz-Crawford, P., Blanc-Brude, O., Jorgensen, C., et al. (2018). Mesenchymal stem cells-derived exosomes are more immunosuppressive than microparticles in inflammatory arthritis. Theranostics 8 (5), 1399–1410. doi:10.7150/thno.21072
Courties, A., and Sellam, J. (2016). Osteoarthritis and type 2 diabetes mellitus: what are the links? Diabetes Res. Clin. Pract. 122, 198–206. doi:10.1016/j.diabres.2016.10.021
de Figueroa, P., Lotz, M. K., Blanco, F. J., and Caramés, B. (2015). Autophagy activation and protection from mitochondrial dysfunction in human chondrocytes. Arthritis and Rheumatology 67, 966–976. doi:10.1002/art.39025
Dehghani, M., Gulvin, S. M., Flax, J., and Gaborski, T. R. (2020). Systematic evaluation of PKH labelling on extracellular vesicle size by nanoparticle tracking analysis. Sci. Rep. 10, 9533. doi:10.1038/s41598-020-66434-7
Ding, Z., Greenberg, Z. F., Serafim, M. F., Ali, S., Jamieson, J. C., Traktuev, D. O., et al. (2024). Understanding molecular characteristics of extracellular vesicles derived from different types of mesenchymal stem cells for therapeutic translation. Extracell. Vesicle 3, 100034. doi:10.1016/j.vesic.2024.100034
Do, J. E., Dc, T. A., and Dordevic, M. (2020). IRB approved pilot safety study of an extracellular vesicle isolate product evaluating the treatment of osteoarthritis in combat-related injuries. J. Stem Cell. Res. 1, 1–10. doi:10.52793/jscr.2020.1(2)-09
Eitner, A., and Wildemann, B. (2021). Diabetes – osteoarthritis and joint pain. Bone and Jt. Res. 10 (5), 307–309. doi:10.1302/2046-3758.105.bjr-2021-0119
Engelman, J. A., Luo, J., and Cantley, L. C. (2006). The evolution of phosphatidylinositol 3-kinases as regulators of growth and metabolism. Nat. Rev. Genet. 7, 606–619. doi:10.1038/nrg1879
Fabbri, M., Paone, A., Calore, F., Galli, R., Gaudio, E., Santhanam, R., et al. (2012). MicroRNAs bind to Toll-like receptors to induce prometastatic inflammatory response, Biol. Sci., 109, E2110–E2116. doi:10.1073/pnas.1209414109
Fennema, E. M., Tchang, L. A. H., Yuan, H., van Blitterswijk, C. A., Martin, I., Scherberich, A., et al. (2017). Ectopic bone formation by aggregated mesenchymal stem cells from bone marrow and adipose tissue: a comparative study. J. Tissue Eng. Regen. Med. 12, e150–e158. doi:10.1002/term.2453
Ferreira, D., Moreira, J. N., and Rodrigues, L. R. (2022). New advances in exosome-based targeted drug delivery systems. Crit. Rev. Oncology/Hematology 172, 103628. doi:10.1016/j.critrevonc.2022.103628
Foo, J. B., Looi, Q. H., How, C. W., Lee, S. H., Al-Masawa, M. E., Chong, P. P., et al. (2021). Mesenchymal stem cell-derived exosomes and MicroRNAs in cartilage regeneration: biogenesis, efficacy, miRNA enrichment and delivery. Pharmaceuticals 14, 1093. doi:10.3390/ph14111093
Forsberg, M. H., Kink, J. A., Hematti, P., and Capitini, C. M. (2020). Mesenchymal stromal cells and exosomes: progress and challenges. Front. Cell. Dev. Biol. 8, 665. doi:10.3389/fcell.2020.00665
Hammerschmidt, N., Hobiger, S., and Jungbauer, A. (2016). Continuous polyethylene glycol precipitation of recombinant antibodies: sequential precipitation and resolubilization. Process Biochem. 51, 325–332. doi:10.1016/j.procbio.2015.11.032
Haseeb, A., Kc, R., Angelozzi, M., de Charleroy, C., Rux, D., Tower, R. J., et al. (2021). SOX9 keeps growth plates and articular cartilage healthy by inhibiting chondrocyte dedifferentiation/osteoblastic redifferentiation. Proc. Natl. Acad. Sci. 118, e2019152118. doi:10.1073/pnas.2019152118
Hassanzadeh, A., Rahman, H. S., Markov, A., Endjun, J. J., Zekiy, A. O., Chartrand, M. S., et al. (2021). Mesenchymal stem/stromal cell-derived exosomes in regenerative medicine and cancer; overview of development, challenges, and opportunities. Stem Cell. Res. and Ther. 12, 297. doi:10.1186/s13287-021-02378-7
Hassanzadeh, A., Vousooghi, N., Rahimnia, R., Razeghian, E., Rajaeian, S., Seyhoun, I., et al. (2023). Recent advances in mesenchymal stem/stromal cells (MSCs)-based approaches for osteoarthritis (OA) therapy. Cell. Biol. Int. Print. 47 (6), 1033–1048. doi:10.1002/cbin.12008
Heard, B. J., Barton, K. I., Chung, M., Achari, Y., Shrive, N. G., Frank, C. B., et al. (2015). Single intra-articular dexamethasone injection immediately post-surgery in a rabbit model mitigates early inflammatory responses and post-traumatic osteoarthritis-like alterations. J. Orthop. Res. Official Publ. Orthop. Res. Soc. 33 (12), 1826–1834. doi:10.1002/jor.22972
Hiraiwa, M., Ozaki, K., Yamada, T., Iezaki, T., Park, G., Fukasawa, K., et al. (2019). mTORC1 activation in osteoclasts prevents bone loss in a mouse model of osteoporosis. Front. Pharmacol. 10, 684. doi:10.3389/fphar.2019.00684
Hu, N., Cai, Z., Jiang, X., Wang, C., Tang, T., Xu, T., et al. (2023). Hypoxia-pretreated ADSC-derived exosome-embedded hydrogels promote angiogenesis and accelerate diabetic wound healing. Acta Biomater. 157, 175–186. doi:10.1016/j.actbio.2022.11.057
Hu, Y., Li, X., Zhang, Q., Gu, Z., Luo, Y., Guo, J., et al. (2021). Exosome-guided bone targeted delivery of Antagomir-188 as an anabolic therapy for bone loss. Bioact. Mater. 6, 2905–2913. doi:10.1016/j.bioactmat.2021.02.014
Huang, X., Liu, G., Guo, J., and Su, Z. (2018). The PI3K/AKT pathway in obesity and type 2 diabetes. Int. J. Biol. Sci. 14, 1483–1496. doi:10.7150/ijbs.27173
Jadawji, C., Crasto, W., Gillies, C., Kar, D., Davies, M. J., Khunti, K., et al. (2018). Prevalence and progression of diabetic nephropathy in South Asian, white European and African Caribbean people with type 2 diabetes: a systematic review and meta-analysis. Diabetes, Obes. Metabolism 21, 658–673. doi:10.1111/dom.13569
Jammes, M., Cassé, F., Velot, E., Bianchi, A., Audigié, F., Contentin, R., et al. (2023). Pro-inflammatory cytokine priming and purification method modulate the impact of exosomes derived from equine bone marrow mesenchymal stromal cells on equine articular chondrocytes. Int. J. Mol. Sci. 24 (18), 14169. doi:10.3390/ijms241814169
Javeed, N., and Matveyenko, A. V. (2018). Circadian etiology of type 2 diabetes mellitus. Physiology 33 (2), 138–150. doi:10.1152/physiol.00003.2018
Jeong, J.-O., Han, J. W., Kim, J. M., Cho, H. J., Park, C., Lee, N., et al. (2011). Malignant tumor formation after transplantation of short-term cultured bone marrow mesenchymal stem cells in experimental myocardial infarction and diabetic neuropathy. Circulation Res. 108, 1340–1347. doi:10.1161/CIRCRESAHA.110.239848
Jeppesen, D. K., Fenix, A. M., Franklin, J. L., Higginbotham, J. N., Zhang, Q., Zimmerman, L. J., et al. (2019). Reassessment of exosome composition. Cell. 177 (2), 428–445.e18. doi:10.1016/j.cell.2019.02.029
Jin, J., Shi, Y., Gong, J., Zhao, L., Li, Y., He, Q., et al. (2019). Exosome secreted from adipose-derived stem cells attenuates diabetic nephropathy by promoting autophagy flux and inhibiting apoptosis in podocyte. Stem Cell. Res. and Ther. 10, 95. doi:10.1186/s13287-019-1177-1
Jurca, C. M., Kozma, K., Petchesi, C. D., Zaha, D. C., Magyar, I., Munteanu, M., et al. (2023). Tuberous sclerosis, type II diabetes mellitus and the PI3K/AKT/mTOR signaling pathways—case report and literature review. Genes. 14, 433. doi:10.3390/genes14020433
Kalluri, R., and LeBleu, V. S. (2020). The biology, function, and biomedical applications of exosomes. Science 367 (6478), eaau6977. doi:10.1126/science.aau6977
Klauder, J., Henkel, J., Vahrenbrink, M., Wohlenberg, A. S., Camargo, R. G., and Püschel, G. P. (2020). Direct and indirect modulation of LPS-induced cytokine production by insulin in human macrophages. Cytokine 136, 155241. doi:10.1016/j.cyto.2020.155241
Komuro, H., Aminova, S., Lauro, K., and Harada, M. (2022). Advances of engineered extracellular vesicles-based therapeutics strategy. Sci. Technol. Adv. Mater. 23, 655–681. doi:10.1080/14686996.2022.2133342
Konoshenko, M. Y., Lekchnov, E. A., Vlassov, A. V., and Laktionov, P. V. (2018). Isolation of extracellular vesicles: general methodologies and latest trends. BioMed. Res. Internat. 1–27. doi:10.1155/2018/8545347
Kou, M., Huang, L., Yang, J., Chiang, Z., Chen, S., Liu, J., et al. (2022). Mesenchymal stem cell-derived extracellular vesicles for immunomodulation and regeneration: a next generation therapeutic tool? Cell. Death and Dis. 13 (7), 580. doi:10.1038/s41419-022-05034-x
Lai, C. P., Mardini, O., Ericsson, M., Prabhakar, S., Maguire, C., Chen, J. W., et al. (2014). Dynamic biodistribution of extracellular vesicles in vivo using a multimodal imaging reporter. ACS Nano 8, 483–494. doi:10.1021/nn404945r
Lai, J. J., Chau, Z. L., Chen, S. Y., Hill, J. J., Korpany, K. V., Liang, N. W., et al. (2022). Exosome processing and characterization approaches for research and technology development. Adv. Sci. 9, 2103222. doi:10.1002/advs.202103222
Lavu, M. S., Porto, J. R., Hecht, C. J., Kaelber, D. C., Sculco, P. K., Heckmann, N. D., et al. (2024). The five-year incidence of progression to osteoarthritis and total joint arthroplasty in patients prescribed glucagon-like peptide 1 receptor agonists. J. Arthroplasty 39, 2433–2439.e1. doi:10.1016/j.arth.2024.06.008
Lee, S. H. (2018). The advantages and limitations of mesenchymal stem cells in clinical application for treating human diseases. Osteoporos. Sarcopenia 4, 150. doi:10.1016/j.afos.2018.11.083
Li, C., Li, W., Pu, G., Wu, J., and Qin, F. (2022). Exosomes derived from miR-338-3p-modified adipose stem cells inhibited inflammation injury of chondrocytes via targeting RUNX2 in osteoarthritis. J. Orthop. Surg. Res. 17, 567. doi:10.1186/s13018-022-03437-2
Li, F., Wu, J., Li, D., Hao, L., Li, Y., Yi, D., et al. (2022). Engineering stem cells to produce exosomes with enhanced bone regeneration effects: an alternative strategy for gene therapy. J. Nanobiotechnology 20, 135. doi:10.1186/s12951-022-01347-3
Li, K., Yan, G., Huang, H., Zheng, M., Ma, K., Cui, X., et al. (2022). Anti-inflammatory and immunomodulatory effects of the extracellular vesicles derived from human umbilical cord mesenchymal stem cells on osteoarthritis via M2 macrophages. J. Nanobiotechnology 20, 38. doi:10.1186/s12951-021-01236-1
Li, P., Kaslan, M., Lee, S. H., Yao, J., and Gao, Z. (2017). Progress in exosome isolation techniques. Theranostics 7, 789–804. doi:10.7150/thno.18133
Li, S., Liu, J., Liu, S., Jiao, W., and Wang, X. (2021). Chitosan oligosaccharides packaged into rat adipose mesenchymal stem cells-derived extracellular vesicles facilitating cartilage injury repair and alleviating osteoarthritis. J. Nanobiotechnology 19 (1), 343. doi:10.1186/s12951-021-01086-x
Lian, X.-F., Lu, D. H., Liu, H. L., Liu, Y. J., Yang, Y., Lin, Y., et al. (2023). Safety evaluation of human umbilical cord-mesenchymal stem cells in type 2 diabetes mellitus treatment: a phase 2 clinical trial. World J. Clin. cases 11, 5083–5096. doi:10.12998/wjcc.v11.i21.5083
Liu, L., Yu, F., Li, L., Zhou, L., Zhou, T., Xu, Y., et al. (2021a). Bone marrow stromal cells stimulated by strontium-substituted calcium silicate ceramics: release of exosomal miR-146a regulates osteogenesis and angiogenesis. Acta Biomater. 119, 444–457. doi:10.1016/j.actbio.2020.10.038
Liu, W., Liu, A., Li, X., Sun, Z., Sun, Z., Liu, Y., et al. (2023). Dual-engineered cartilage-targeting extracellular vesicles derived from mesenchymal stem cells enhance osteoarthritis treatment via miR-223/NLRP3/pyroptosis axis: toward a precision therapy. Bioact. Mater. 30, 169–183. doi:10.1016/j.bioactmat.2023.06.012
Liu, W., Yu, M., Chen, F., Wang, L., Ye, C., Chen, Q., et al. (2021b). A novel delivery nanobiotechnology: engineered miR-181b exosomes improved osseointegration by regulating macrophage polarization. J. Nanobiotechnology 19, 269. doi:10.1186/s12951-021-01015-y
Loeser, R. F., Goldring, S. R., Scanzello, C. R., and Goldring, M. B. (2012). Osteoarthritis: a disease of the joint as an organ. Arthritis rheumatism 64 (6), 1697–1707. doi:10.1002/art.34453
Lotfy, A., AboQuella, N. M., and Wang, H. (2023). Mesenchymal stromal/stem cell (MSC)-derived exosomes in clinical trials. Stem Cell. Res. and Ther. 14, 66. doi:10.1186/s13287-023-03287-7
Lu, C.-H., Chung, C. H., Lee, C. H., Hsieh, C. H., Hung, Y. J., Lin, F. H., et al. (2018). Combination COX-2 inhibitor and metformin attenuate rate of joint replacement in osteoarthritis with diabetes: a nationwide, retrospective, matched-cohort study in Taiwan. PLOS ONE 13 (1), e0191242. doi:10.1371/journal.pone.0191242
Luan, X., Sansanaphongpricha, K., Myers, I., Chen, H., Yuan, H., and Sun, D. (2017). Engineering exosomes as refined biological nanoplatforms for drug delivery. Acta Pharmacol. Sin. 38, 754–763. doi:10.1038/aps.2017.12
Ludwig, A.-K., De Miroschedji, K., Doeppner, T. R., Börger, V., Ruesing, J., Rebmann, V., et al. (2018). Precipitation with polyethylene glycol followed by washing and pelleting by ultracentrifugation enriches extracellular vesicles from tissue culture supernatants in small and large scales. J. Extracell. Vesicles 7, 1528109. doi:10.1080/20013078.2018.1528109
Luo, Z.-W., Li, F. X. Z., Liu, Y. W., Rao, S. S., Yin, H., Huang, J., et al. (2019). Aptamer-functionalized exosomes from bone marrow stromal cells target bone to promote bone regeneration. Nanoscale 11, 20884–20892. doi:10.1039/c9nr02791b
Ma, Y., Liu, X., Long, Y., and Chen, Y. (2022). Emerging therapeutic potential of mesenchymal stem cell-derived extracellular vesicles in chronic respiratory diseases: an overview of recent progress. Front. Bioeng. Biotechnol. 10, 845042. doi:10.3389/fbioe.2022.845042
Madry, H. (2022). Surgical therapy in osteoarthritis. Osteoarthr. Cartil. 30, 1019–1034. doi:10.1016/j.joca.2022.01.012
Mao, G., Xu, W., Wan, L., Wang, H., Xu, S., Zhang, L., et al. (2024). Unveiling the bioinformatic genes and their involved regulatory mechanisms in type 2 diabetes combined with osteoarthritis. Front. Immunol. 15, 1353915. doi:10.3389/fimmu.2024.1353915
Marttila, S., Rovio, S., Mishra, P. P., Seppälä, I., Lyytikäinen, L. P., Juonala, M., et al. (2021). Adulthood blood levels of ha-miR-29b-3p associate with preterm birth and adult metabolic and cognitive health. Sci. Rep. 11, 9203. doi:10.1038/s41598-021-88465-4
Masters, S. L., Dunne, A., Subramanian, S. L., Hull, R. L., Tannahill, G. M., Sharp, F. A., et al. (2010). Activation of the NLRP3 inflammasome by islet amyloid polypeptide provides a mechanism for enhanced IL-1β in type 2 diabetes. Nat. Immunol. 11, 897–904. doi:10.1038/ni.1935
Matas, J., García, C., Poblete, D., Vernal, R., Ortloff, A., Luque-Campos, N., et al. (2024). A phase I dose-escalation clinical trial to assess the safety and efficacy of umbilical cord-derived mesenchymal stromal cells in knee osteoarthritis. Stem cells Transl. Med. 13 (3), 193–203. doi:10.1093/stcltm/szad088
Mathivanan, S., Ji, H., and Simpson, R. J. (2010). Exosomes: extracellular organelles important in intercellular communication. J. Proteomics 73, 1907–1920. doi:10.1016/j.jprot.2010.06.006
Matta, C., and Mobasheri, A. (2014). Regulation of chondrogenesis by protein kinase C: emerging new roles in calcium signalling. Cell. Signal. 26, 979–1000. doi:10.1016/j.cellsig.2014.01.011
Maumus, M., Rozier, P., Boulestreau, J., Jorgensen, C., and Noël, D. (2020). Mesenchymal stem cell-derived extracellular vesicles: opportunities and challenges for clinical translation. Front. Bioeng. Biotechnol. 8, 997. doi:10.3389/fbioe.2020.00997
Mobasheri, A., Rayman, M. P., Gualillo, O., Sellam, J., van der Kraan, P., and Fearon, U. (2017). The role of metabolism in the pathogenesis of osteoarthritis. Nat. Rev. Rheumatol. 13 (5), 302–311. doi:10.1038/nrrheum.2017.50
Morente-López, M., Mato-Basalo, R., Lucio-Gallego, S., Silva-Fernández, L., González-Rodríguez, A., De Toro, F. J., et al. (2022). Therapy free of cells vs human mesenchymal stem cells from umbilical cord stroma to treat the inflammation in OA. Cell. Mol. Life Sci. 79, 557. doi:10.1007/s00018-022-04580-z
Murphy, D. E., de Jong, O. G., Brouwer, M., Wood, M. J., Lavieu, G., Schiffelers, R. M., et al. (2019). Extracellular vesicle-based therapeutics: natural versus engineered targeting and trafficking. Exp. and Mol. Med. 51, 1–12. doi:10.1038/s12276-019-0223-5
Nagaishi, K., Mizue, Y., Chikenji, T., Otani, M., Nakano, M., Konari, N., et al. (2016). Mesenchymal stem cell therapy ameliorates diabetic nephropathy via the paracrine effect of renal trophic factors including exosomes. Sci. Rep. 6 (1), 34842. doi:10.1038/srep34842
Oh, J. H., and Lee, N. K. (2017). Up-regulation of RANK expression via ERK1/2 by insulin contributes to the enhancement of osteoclast differentiation. Mol. Cells 40, 371–377. doi:10.14348/molcells.2017.0025
Olefsky, J. M., and Glass, C. K. (2010). Macrophages, inflammation, and insulin resistance. Annu. Rev. physiology 72, 219–246. doi:10.1146/annurev-physiol-021909-135846
Oskouie, M. N., Aghili Moghaddam, N. S., Butler, A. E., Zamani, P., and Sahebkar, A. (2018). Therapeutic use of curcumin-encapsulated and curcumin-primed exosomes. J. Cell. Physiology 234, 8182–8191. doi:10.1002/jcp.27615
Pang, L., Jin, H., Lu, Z., Xie, F., Shen, H., Li, X., et al. (2023). Treatment with mesenchymal stem cell-derived nanovesicle-containing gelatin methacryloyl hydrogels alleviates osteoarthritis by modulating chondrogenesis and macrophage polarization. Adv. Healthc. Mater 12, 2300315. doi:10.1002/adhm.202300315
Pegtel, D. M., Cosmopoulos, K., Thorley-Lawson, D. A., van Eijndhoven, M. A. J., Hopmans, E. S., Lindenberg, J. L., et al. (2010). Functional delivery of viral miRNAs via exosomes. Proc. Natl. Acad. Sci. 107, 6328–6333. doi:10.1073/pnas.0914843107
Phinney, D. G., and Pittenger, M. F. (2017). Concise review: MSC-derived exosomes for cell-free therapy. Stem Cells 35 (4), 851–858. doi:10.1002/stem.2575
Piper, R. C., and Katzmann, D. J. (2007). Biogenesis and function of multivesicular bodies. Annu. Rev. Cell. Dev. Biol. 23 (1), 519–547. doi:10.1146/annurev.cellbio.23.090506.123319
Pishavar, E., Luo, H., Naserifar, M., Hashemi, M., Toosi, S., Atala, A., et al. (2021). Advanced hydrogels as exosome delivery systems for osteogenic differentiation of MSCs: application in bone regeneration. Int. J. Mol. Sci. 22, 6203. doi:10.3390/ijms22126203
Pulido-Escribano, V., Torrecillas-Baena, B., Camacho-Cardenosa, M., Dorado, G., Gálvez-Moreno, M. Á., and Casado-Díaz, A. (2022). Role of hypoxia preconditioning in therapeutic potential of mesenchymal stem-cell-derived extracellular vesicles. World J. Stem Cells 14, 453–472. doi:10.4252/wjsc.v14.i7.453
Qi, H., Liu, D. P., Xiao, D. W., Tian, D. C., Su, Y. W., and Jin, S. F. (2019a). Exosomes derived from mesenchymal stem cells inhibit mitochondrial dysfunction-induced apoptosis of chondrocytes via p38, ERK, and Akt pathways. Vitro Cell. and Dev. Biol. - Animal 55 (3), 203–210. doi:10.1007/s11626-019-00330-x
Qi, Y., Du, X., Yao, X., and Zhao, Y. (2019b). Vildagliptin inhibits high free fatty acid (FFA)-induced NLRP3 inflammasome activation in endothelial cells. Artif. Cells Nanomedicine Biotechnol. 47, 1067–1074. doi:10.1080/21691401.2019.1578783
Qiao, L., Li, Y., and Sun, S. (2020). Insulin exacerbates inflammation in fibroblast-like synoviocytes. Inflammation 43, 916–936. doi:10.1007/s10753-020-01178-0
Qin, X., Guo, H., Wang, X., Zhu, X., Yan, M., Wang, X., et al. (2019). Exosomal miR-196a derived from cancer-associated fibroblasts confers cisplatin resistance in head and neck cancer through targeting CDKN1B and ING5. Genome Biol. 20, 12. doi:10.1186/s13059-018-1604-0
Qiu, G., Zheng, G., Ge, M., Wang, J., Huang, R., Shu, Q., et al. (2018). Mesenchymal stem cell-derived extracellular vesicles affect disease outcomes via transfer of microRNAs. Stem Cell. Res. and Ther. 9 (1), 320. doi:10.1186/s13287-018-1069-9
Qiu, M., Liu, D., and Fu, Q. (2021). MiR-129-5p shuttled by human synovial mesenchymal stem cell-derived exosomes relieves IL-1β induced osteoarthritis via targeting HMGB1. Life Sci. 269, 118987. doi:10.1016/j.lfs.2020.118987
Qiu, M., Xie, Y., Tan, G., Wang, X., Huang, P., and Hong, L. (2024). Synovial mesenchymal stem cell-derived exosomal miR-485-3p relieves cartilage damage in osteoarthritis by targeting the NRP1-mediated PI3K/Akt pathway: exosomal miR-485-3p relieves cartilage damage. Heliyon 10, e24042. doi:10.1016/j.heliyon.2024.e24042
Raghav, P. K., and Mann, Z. (2024). Nano-delivery revolution: harnessing mesenchymal stem cell-derived exosomes’ potential for wound healing. Biomedicines 12, 2791. doi:10.3390/biomedicines12122791
Ragni, E., Perucca Orfei, C., De Luca, P., Mondadori, C., Viganò, M., Colombini, A., et al. (2020). Inflammatory priming enhances mesenchymal stromal cell secretome potential as a clinical product for regenerative medicine approaches through secreted factors and EV-miRNAs: the example of joint disease. Stem Cell. Res. and Ther. 11 (1), 165. doi:10.1186/s13287-020-01677-9
Rao, D., Huang, D., Sang, C., Zhong, T., Zhang, Z., and Tang, Z. (2022). Advances in mesenchymal stem cell-derived exosomes as drug delivery vehicles. Front. Bioeng. Biotechnol. 9, 797359. doi:10.3389/fbioe.2021.797359
Ribeiro, M., López de Figueroa, P., Blanco, F. J., Mendes, A. F., and Caramés, B. (2016). Insulin decreases autophagy and leads to cartilage degradation. Osteoarthr. Cartil. 24 (4), 731–739. doi:10.1016/j.joca.2015.10.017
Rizzo, M. G., Best, T. M., Huard, J., Philippon, M., Hornicek, F., Duan, Z., et al. (2023). Therapeutic perspectives for inflammation and senescence in osteoarthritis using mesenchymal stem cells, mesenchymal stem cell-derived extracellular vesicles and senolytic agents. Cells 12, 1421. doi:10.3390/cells12101421
Rong, Y., Zhang, J., Jiang, D., Ji, C., Liu, W., Wang, J., et al. (2021). Hypoxic pretreatment of small extracellular vesicles mediates cartilage repair in osteoarthritis by delivering miR-216a-5p. Acta Biomater. 122, 325–342. doi:10.1016/j.actbio.2020.12.034
Rosa, S. C., Gonçalves, J., Judas, F., Mobasheri, A., Lopes, C., and Mendes, A. F. (2009). Impaired glucose transporter-1 degradation and increased glucose transport and oxidative stress in response to high glucose in chondrocytes from osteoarthritic versus normal human cartilage. Arthritis Res. and Ther. 11 (3), R80. doi:10.1186/ar2713
Sanchez-Lopez, E., Zhong, Z., Stubelius, A., Sweeney, S. R., Booshehri, L. M., Antonucci, L., et al. (2019). Choline uptake and metabolism modulate macrophage IL-1β and IL-18 production. Cell. Metab. 29, 1350–1362.e7. doi:10.1016/j.cmet.2019.03.011
Satyadev, N., Rivera, M. I., Nikolov, N. K., and Fakoya, A. O. J. (2023). Exosomes as biomarkers and therapy in type 2 diabetes mellitus and associated complications. Front. Physiology 14, 1241096. doi:10.3389/fphys.2023.1241096
Schett, G., Kleyer, A., Perricone, C., Sahinbegovic, E., Iagnocco, A., Zwerina, J., et al. (2013). Diabetes is an independent predictor for severe osteoarthritis: results from a longitudinal cohort study. Diabetes care 36 (2), 403–409. doi:10.2337/dc12-0924
Seow, S. R., Mat, S., Ahmad Azam, A., Rajab, N. F., Safinar Ismail, I., Singh, D. K. A., et al. (2024). Impact of diabetes mellitus on osteoarthritis: a scoping review on biomarkers. Expert Rev. Mol. Med. 26, e8. doi:10.1017/erm.2024.7
Sharma, R., Kumari, M., Mishra, S., Chaudhary, D. K., Kumar, A., Avni, B., et al. (2021). Exosomes secreted by umbilical cord blood-derived mesenchymal stem cell attenuate diabetes in mice. J. Diabetes Res. 2021, 9534574. doi:10.1155/2021/9534574
Shi, H., Hao, X., Sun, Y., Zhang, H., Zhao, Y., Wang, B., et al. (2023). Bone marrow mesenchymal stem cell-derived exosomes reduce insulin resistance and obesity in mice via the PI3K/AKT signaling pathway. FEBS Open Bio 13, 1015–1026. doi:10.1002/2211-5463.13615
Shi, Y., Hu, X., Cheng, J., Zhang, X., Zhao, F., Shi, W., et al. (2019). A small molecule promotes cartilage extracellular matrix generation and inhibits osteoarthritis development. Nat. Commun. 10, 1914. doi:10.1038/s41467-019-09839-x
Shirinsky, I. V., and Shirinsky, V. S. (2017). Effects of medication-treated diabetes on incidence and progression of knee osteoarthritis: a longitudinal analysis of the Osteoarthritis Initiative data. Rheumatol. Int. 37, 983–991. doi:10.1007/s00296-017-3676-7
Simpson, R. J., Lim, J. W., Moritz, R. L., and Mathivanan, S. (2009). Exosomes: proteomic insights and diagnostic potential. Expert Rev. Proteomics 6, 267–283. doi:10.1586/epr.09.17
Skog, J., Würdinger, T., van Rijn, S., Meijer, D. H., Gainche, L., Sena-Esteves, M., et al. (2008). Glioblastoma microvesicles transport RNA and proteins that promote tumour growth and provide diagnostic biomarkers. Nat. Cell. Biol. 10, 1470–1476. doi:10.1038/ncb1800
Song, P., Sun, C., Lai, C., and Zhang, L. (2023). Exosomes derived from mesenchymal stem cells rescue cartilage injury in osteoarthritis through Ferroptosis by GOT1/CCR2 expression. Int. Immunopharmacol. 122, 110566. doi:10.1016/j.intimp.2023.110566
Song, S., and Yu, J. (2024). Identification of the shared genes in type 2 diabetes mellitus and osteoarthritis and the role of quercetin. J. Cell. Mol. Med. 28 (4), e18127. doi:10.1111/jcmm.18127
Stentz, F., and Kitabchi, A. (2003). Activated T lymphocytes in type 2 diabetes: implications from in vitro studies. Curr. Drug Targets 4, 493–503. doi:10.2174/1389450033490966
Su, J., Luo, Y., Hu, S., Tang, L., and Ouyang, S. (2023). Advances in research on type 2 diabetes mellitus targets and therapeutic agents. Int. J. Mol. Sci. 24 (17), 13381. doi:10.3390/ijms241713381
Su, T., Xiao, Y., Xiao, Y., Guo, Q., Li, C., Huang, Y., et al. (2019). Bone marrow mesenchymal stem cells-derived exosomal MiR-29b-3p regulates aging-associated insulin resistance. ACS Nano 13 (2), 2450–2462. doi:10.1021/acsnano.8b09375
Sun, F., Sun, J., and Zhao, Q. (2022). A deep learning method for predicting metabolite–disease associations via graph neural network. Briefings Bioinforma. 23, bbac266. doi:10.1093/bib/bbac266
Sun, K., Luo, J., Guo, J., Yao, X., Jing, X., and Guo, F. (2020). The PI3K/AKT/mTOR signaling pathway in osteoarthritis: a narrative review. Osteoarthr. Cartil. 28, 400–409. doi:10.1016/j.joca.2020.02.027
Sun, W., Yue, J., Xu, T., Cui, Y., Huang, D., Shi, H., et al. (2023). Xanthohumol alleviates palmitate-induced inflammation and prevents osteoarthritis progression by attenuating mitochondria dysfunction/NLRP3 inflammasome axis. Heliyon 9, e21282. doi:10.1016/j.heliyon.2023.e21282
Sun, Y., Shi, H., Yin, S., Ji, C., Zhang, X., Zhang, B., et al. (2018). Human mesenchymal stem cell derived exosomes alleviate type 2 diabetes mellitus by reversing peripheral insulin resistance and relieving β-cell destruction. ACS Nano 12 (8), 7613–7628. doi:10.1021/acsnano.7b07643
Tao, S.-C., Yuan, T., Zhang, Y. L., Yin, W. J., Guo, S. C., and Zhang, C. Q. (2017). Exosomes derived from miR-140-5p-overexpressing human synovial mesenchymal stem cells enhance cartilage tissue regeneration and prevent osteoarthritis of the knee in a rat model. Theranostics 7 (1), 180–195. doi:10.7150/thno.17133
Tateya, S., Kim, F., and Tamori, Y. (2013). Recent advances in obesity-induced inflammation and insulin resistance. Front. Endocrinol. 4, 93. doi:10.3389/fendo.2013.00093
Tejero, S., Prada-Chamorro, E., González-Martín, D., García-Guirao, A., Galhoum, A., Valderrabano, V., et al. (2021). Conservative treatment of ankle osteoarthritis. J. Clin. Med. 10, 4561. doi:10.3390/jcm10194561
Toh, W. S., Lai, R. C., Zhang, B., and Lim, S. K. (2018). MSC exosome works through a protein-based mechanism of action. Biochem. Soc. Trans. 46, 843–853. doi:10.1042/bst20180079
Tsai, C.-H., Chiang, Y. C., Chen, H. T., Huang, P. H., Hsu, H. C., and Tang, C. H. (2013a). High glucose induces vascular endothelial growth factor production in human synovial fibroblasts through reactive oxygen species generation. Biochimica Biophysica Acta 1830, 2649–2658. doi:10.1016/j.bbagen.2012.12.017
Tsai, T.-L., Manner, P. A., and Li, W.-J. (2013b). Regulation of mesenchymal stem cell chondrogenesis by glucose through protein kinase C/transforming growth factor signaling. Osteoarthr. Cartil. 21, 368–376. doi:10.1016/j.joca.2012.11.001
Valadi, H., Ekström, K., Bossios, A., Sjöstrand, M., Lee, J. J., and Lötvall, J. O. (2007). Exosome-mediated transfer of mRNAs and microRNAs is a novel mechanism of genetic exchange between cells. Nat. Cell. Biol. 9, 654–659. doi:10.1038/ncb1596
Vandanmagsar, B., Youm, Y. H., Ravussin, A., Galgani, J. E., Stadler, K., Mynatt, R. L., et al. (2011). The NLRP3 inflammasome instigates obesity-induced inflammation and insulin resistance. Nat. Med. 17, 179–188. doi:10.1038/nm.2279
Veronese, N., Cooper, C., Reginster, J. Y., Hochberg, M., Branco, J., Bruyère, O., et al. (2019). Type 2 diabetes mellitus and osteoarthritis. Seminars Arthritis Rheumatism 49 (1), 9–19. doi:10.1016/j.semarthrit.2019.01.005
Wang, J., Sun, Z., Gou, W., Adams, D. B., Cui, W., Morgan, K. A., et al. (2017). α-1 antitrypsin enhances islet engraftment by suppression of instant blood-mediated inflammatory reaction. Diabetes 66, 970–980. doi:10.2337/db16-1036
Wang, W., Zhang, L., Sun, J., Zhao, Q., and Shuai, J. (2022). Predicting the potential human lncRNA–miRNA interactions based on graph convolution network with conditional random field. Briefings Bioinforma. 23, bbac463. doi:10.1093/bib/bbac463
Wang, X., Wang, Y., Antony, V., Sun, H., and Liang, G. (2020). Metabolism-associated molecular patterns (MAMPs). Trends Endocrinol. Metabolism 31, 712–724. doi:10.1016/j.tem.2020.07.001
Wang, Y., Hu, K., Liao, C., Han, T., Jiang, F., Gao, Z., et al. (2024). Exosomes-shuttled lncRNA SNHG7 by bone marrow mesenchymal stem cells alleviates osteoarthritis through targeting miR-485-5p/FSP1 axis-mediated chondrocytes ferroptosis and inflammation. Tissue Eng. Regen. Med. 21, 1203–1216. [Preprint]. doi:10.1007/s13770-024-00668-8
Warmink, K., Rios, J. L., Varderidou-Minasian, S., Torres-Torrillas, M., van Valkengoed, D. R., Versteeg, S., et al. (2023). Mesenchymal stem/stromal cells-derived extracellular vesicles as a potentially more beneficial therapeutic strategy than MSC-based treatment in a mild metabolic osteoarthritis model. Stem Cell. Res. and Ther. 14, 137. doi:10.1186/s13287-023-03368-7
Woo, C. H., Kim, H. K., Jung, G. Y., Jung, Y. J., Lee, K. S., Yun, Y. E., et al. (2020). Small extracellular vesicles from human adipose-derived stem cells attenuate cartilage degeneration. J. Extracell. Vesicles 9 (1), 1735249. doi:10.1080/20013078.2020.1735249
Wu, J., Kuang, L., Chen, C., Yang, J., Zeng, W. N., Li, T., et al. (2019). miR-100-5p-abundant exosomes derived from infrapatellar fat pad MSCs protect articular cartilage and ameliorate gait abnormalities via inhibition of mTOR in osteoarthritis. Biomaterials 206, 87–100. doi:10.1016/j.biomaterials.2019.03.022
Wu, J., Wu, J., Xiang, W., Gong, Y., Feng, D., Fang, S., et al. (2024). Engineering exosomes derived from TNF-α preconditioned IPFP-MSCs enhance both yield and therapeutic efficacy for osteoarthritis. J. nanobiotechnology 22, 555. doi:10.1186/s12951-024-02795-9
Wu, P., Zhang, B., Shi, H., Qian, H., and Xu, W. (2018). MSC-exosome: a novel cell-free therapy for cutaneous regeneration. Cytotherapy 20 (3), 291–301. doi:10.1016/j.jcyt.2017.11.002
Xia, L., Yang, M., Zang, N., Song, J., Chen, J., Hu, H., et al. (2024). PEGylated β-cell-targeting exosomes from mesenchymal stem cells improve β cell function and quantity by suppressing NRF2-mediated ferroptosis. Int. J. Nanomedicine 19, 9575–9596. doi:10.2147/ijn.s459077
Xia, Q., Wang, Q., Lin, F., and Wang, J. (2021). miR-125a-5p-abundant exosomes derived from mesenchymal stem cells suppress chondrocyte degeneration via targeting E2F2 in traumatic osteoarthritis. Bioengineered 12 (2), 11225–11238. doi:10.1080/21655979.2021.1995580
Xian, L., Wu, X., Pang, L., Lou, M., Rosen, C. J., Qiu, T., et al. (2012). Matrix IGF-1 maintains bone mass by activation of mTOR in mesenchymal stem cells. Nat. Med. 18, 1095–1101. doi:10.1038/nm.2793
Xiang, X.-N., Zhu, S. Y., He, H. C., Yu, X., Xu, Y., and He, C. Q. (2022). Mesenchymal stromal cell-based therapy for cartilage regeneration in knee osteoarthritis. Stem Cell. Res. and Ther. 13 (1), 14. doi:10.1186/s13287-021-02689-9
Xu, C., Zhai, Z., Ying, H., Zhang, J., and Zeng, Y. (2022). Curcumin primed ADMSCs derived small extracellular vesicle exert enhanced protective effects on osteoarthritis by inhibiting oxidative stress and chondrocyte apoptosis. J. Nanobiotechnology 20, 123. doi:10.1186/s12951-022-01339-3
Yan, L., and Wu, X. (2019). Exosomes produced from 3D cultures of umbilical cord mesenchymal stem cells in a hollow-fiber bioreactor show improved osteochondral regeneration activity. Cell. Biol. Toxicol. 36, 165–178. doi:10.1007/s10565-019-09504-5
Yang, D., Zhang, W., Zhang, H., Zhang, F., Chen, L., Ma, L., et al. (2020). Progress, opportunity, and perspective on exosome isolation - efforts for efficient exosome-based theranostics. Theranostics 10, 3684–3707. doi:10.7150/thno.41580
Yang, J., Li, S., Li, Z., Yao, L., Liu, M., Tong, K. L., et al. (2024). Targeting YAP1-regulated glycolysis in fibroblast-like synoviocytes impairs macrophage infiltration to ameliorate diabetic osteoarthritis progression. Adv. Sci. (Weinheim, Baden-Wurttemberg, Ger.) 11 (5), e2304617. doi:10.1002/advs.202304617
Yang, Y., Hong, Y., Cho, E., Kim, G. B., and Kim, I. S. (2018). Extracellular vesicles as a platform for membrane-associated therapeutic protein delivery. J. Extracell. Vesicles 7, 1440131. doi:10.1080/20013078.2018.1440131
Yang, Y., Zheng, W., Tan, W., Wu, X., Dai, Z., Li, Z., et al. (2023). Injectable MMP1-sensitive microspheres with spatiotemporally controlled exosome release promote neovascularized bone healing. Acta Biomater. 157, 321–336. doi:10.1016/j.actbio.2022.11.065
You, B., Zhou, C., and Yang, Y. (2023). MSC-EVs alleviate osteoarthritis by regulating microenvironmental cells in the articular cavity and maintaining cartilage matrix homeostasis. Ageing Res. Rev. 85, 101864. doi:10.1016/j.arr.2023.101864
Zhang, H., Cai, D., and Bai, X. (2020). Macrophages regulate the progression of osteoarthritis. Osteoarthr. Cartil. 28, 555–561. doi:10.1016/j.joca.2020.01.007
Zhang, J., Li, S., Li, L., Li, M., Guo, C., Yao, J., et al. (2015). Exosome and exosomal MicroRNA: trafficking, sorting, and function. Genomics, Proteomics and Bioinforma. 13, 17–24. doi:10.1016/j.gpb.2015.02.001
Zhang, Q., Fang, W., Wang, Z. D., Yang, Y. M., and Lu, Y. Q. (2018a). VEGF levels in plasma in relation to metabolic control, inflammation, and microvascular complications in type-2 diabetes: a cohort study. Medicine 97, e0415. doi:10.1097/md.0000000000010415
Zhang, S., Chuah, S. J., Lai, R. C., Hui, J. H. P., Lim, S. K., and Toh, W. S. (2018b). MSC exosomes mediate cartilage repair by enhancing proliferation, attenuating apoptosis and modulating immune reactivity. Biomaterials 156, 16–27. doi:10.1016/j.biomaterials.2017.11.028
Zhang, Y., Liu, Y., Liu, H., and Tang, W. H. (2019). Exosomes: biogenesis, biologic function and clinical potential. Cell. and Biosci. 9, 19. doi:10.1186/-019-0282-2
Zhang, Z., Amorosa, L. F., Coyle, S. M., Macor, M. A., Birnbaum, M. J., Lee, L. Y., et al. (2016). Insulin-Dependent regulation of mTORC2-akt-FoxO suppresses TLR4 signaling in human leukocytes: relevance to type 2 diabetes. Diabetes 65, 2224–2234. doi:10.2337/db16-0027
Zhao, J., Sun, Y., Sheng, X., Xu, J., Dai, G., He, R., et al. (2023). Hypoxia-treated adipose mesenchymal stem cell-derived exosomes attenuate lumbar facet joint osteoarthritis. Mol. Med. 29, 120. doi:10.1186/s10020-023-00709-3
Zhou, H., Shen, X., Yan, C., Xiong, W., Ma, Z., Tan, Z., et al. (2022). Extracellular vesicles derived from human umbilical cord mesenchymal stem cells alleviate osteoarthritis of the knee in mice model by interacting with METTL3 to reduce m6A of NLRP3 in macrophage. Stem Cell. Res. Ther. 13 (1), 322. doi:10.1186/s13287-022-03005-9
Zhou, M., Xu, W., Wang, J., Yan, J., Shi, Y., Zhang, C., et al. (2018). Boosting mTOR-dependent autophagy via upstream TLR4-MyD88-MAPK signalling and downstream NF-κB pathway quenches intestinal inflammation and oxidative stress injury. EBioMedicine 35, 345–360. doi:10.1016/j.ebiom.2018.08.035
Zhou, W., Li, W., Wang, S., Salovska, B., Hu, Z., Tao, B., et al. (2023). An optogenetic-phosphoproteomic study reveals dynamic Akt1 signaling profiles in endothelial cells. Nat. Commun. 14, 3803. doi:10.1038/s41467-023-39514-1
Zhu, F., Wang, T., Wang, G., Yan, C., He, B., and Qiao, B. (2024). The exosome-mediated bone regeneration: an advanced horizon toward the isolation, engineering, carrying modalities, and mechanisms. Adv. Healthc. Mater. 13, e2400293. doi:10.1002/adhm.202400293
Zhu, H., Zhou, L., Wang, Q., Cai, Q., Yang, F., Jin, H., et al. (2023). Glucagon-like peptide-1 receptor agonists as a disease-modifying therapy for knee osteoarthritis mediated by weight loss: findings from the Shanghai Osteoarthritis Cohort. Ann. Rheumatic Dis. 82, 1218–1226. doi:10.1136/ard-2023-223845
Zhuang, M., Rao, L., Chen, Y., Xiao, S., Xia, H., Yang, J., et al. (2023). Controlled SPION-exosomes loaded with quercetin preserves pancreatic beta cell survival and function in type 2 diabetes mellitus. Int. J. Nanomedicine 18, 5733–5748. doi:10.2147/ijn.s422416
Zupan, J., Vrtačnik, P., Cör, A., Haring, G., Weryha, G., Visvikis-Siest, S., et al. (2018). VEGF-A is associated with early degenerative changes in cartilage and subchondral bone. Growth factors. 36, 263–273. doi:10.1080/08977194.2019.1570926
ADAMTS Aggrecanases with thrombospondin motifs
AD-MSC-Exos Adipose-derived mesenchymal stem cell-derived exosomes
AGEs Advanced glycation end-products
Akt Protein kinase B
BM-MSCs Bone marrow-derived mesenchymal stem cells
BM-MSC-Exos Bone marrow mesenchymal stem cell-derived exosomes
CAP Chondrocyte-affinity peptide
ECM Extracellular matrix
EVs Extracellular vesicles
GLP-1-RAs Glucagon-like peptide-1 receptor agonists
GLUT Glucose transporter protein
ILVs Intraluminal vesicles
IPFP-MSC-Exos Infrapatellar fat pad mesenchymal stem cell-derived exosomes
IR Insulin resistance
IRS-1 Insulin receptor substrate-1
KOA Knee osteoarthritis
miRNAs microRNAs
MMPs Matrix metalloproteinases
MSCs Mesenchymal stromal/stem cells
MSC-Exos Mesenchymal stromal/stem cell-derived exosomes
mTOR Mechanistic target of rapamycin
MVBs Multivesicular bodies
NLRP3 NOD-like receptor family pyrin domain containing 3
OA Osteoarthritis
PI3K Phosphoinositide 3-kinase
PKC Protein kinase C
ROS Reactive oxygen species
SIRT Sirtuin
SMSC-Exos Synovial mesenchymal stem cell-derived exosomes
SOX9 SRY-box transcription factor 9
STZ Streptozotocin
TNF Tumor necrosis factor
T2DM Type 2 diabetes mellitus
UC-MSCs Umbilical cord-derived mesenchymal stem cells
UC-MSC-Exos Umbilical cord mesenchymal stem cell-derived exosomes
VEGF Vascular endothelial growth factor.
Keywords: osteoarthritis, type 2 diabetes mellitus, diabetic osteoarthritis, mesenchymal stromal/stem cell-derived exosomes, microRNAs, engineered exosomes
Citation: Xie S, Liu M, Kong Y, Yang Y, Chen R, Wang Y, Cao S and Song Y (2025) Mesenchymal stromal/stem cell-derived exosomes as a potential therapeutic approach to osteoarthritis combined with type 2 diabetes mellitus. Front. Cell Dev. Biol. 13:1549096. doi: 10.3389/fcell.2025.1549096
Received: 20 December 2024; Accepted: 13 March 2025;
Published: 27 March 2025.
Edited by:
Runsang Pan, Guizhou Provincial People’s Hospital, ChinaReviewed by:
Armel Hervé Nwabo Kamdje, University of Garoua, CameroonCopyright © 2025 Xie, Liu, Kong, Yang, Chen, Wang, Cao and Song. This is an open-access article distributed under the terms of the Creative Commons Attribution License (CC BY). The use, distribution or reproduction in other forums is permitted, provided the original author(s) and the copyright owner(s) are credited and that the original publication in this journal is cited, in accordance with accepted academic practice. No use, distribution or reproduction is permitted which does not comply with these terms.
*Correspondence: Shuxing Cao, Mjg0MDI2MzdAaGVibXUuZWR1LmNu; Yongzhou Song, eW9uZ3pob3Vzb25nQGhlYm11LmVkdS5jbg==
Disclaimer: All claims expressed in this article are solely those of the authors and do not necessarily represent those of their affiliated organizations, or those of the publisher, the editors and the reviewers. Any product that may be evaluated in this article or claim that may be made by its manufacturer is not guaranteed or endorsed by the publisher.
Research integrity at Frontiers
Learn more about the work of our research integrity team to safeguard the quality of each article we publish.