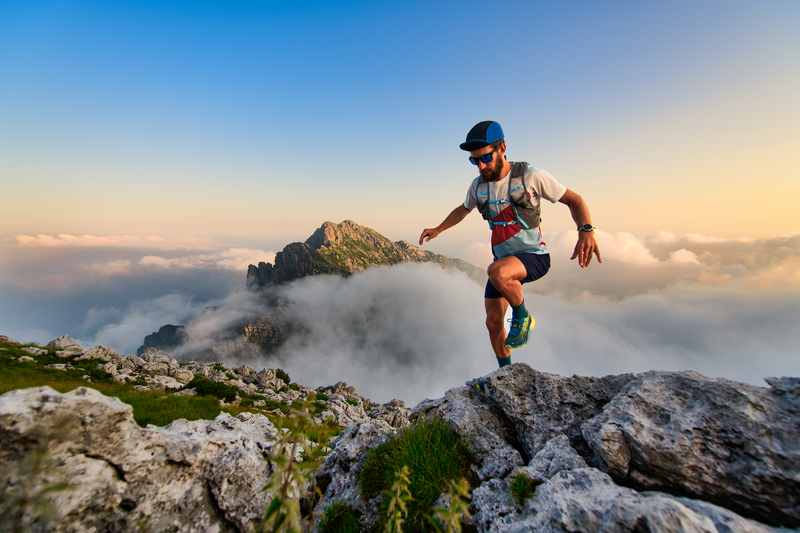
95% of researchers rate our articles as excellent or good
Learn more about the work of our research integrity team to safeguard the quality of each article we publish.
Find out more
REVIEW article
Front. Cell Dev. Biol. , 26 March 2025
Sec. Cellular Biochemistry
Volume 13 - 2025 | https://doi.org/10.3389/fcell.2025.1522821
Regular exercise positively influences bone health, enhances bone density and strength, and reduces the risk of osteoporosis. Silent information regulator of transcription 1 (SIRT1) is a deacetylase that plays a pivotal role in the regulation of various biological processes. In this review, we explore the role of SIRT1 in modulating bone metabolism in response to exercise. SIRT1 regulates crucial cellular processes, including inflammation, aging, autophagy, and oxidative stress, in bone cells such as bone marrow mesenchymal stem cells, osteoblasts, and osteoclasts, in response to exercise-induced stimuli. Notably, exercise influences bone metabolism by modulating muscle metabolism and neurotransmitters, with SIRT1 acting as a key mediator. A comprehensive understanding of SIRT1’s regulatory mechanisms will facilitate a deeper exploration of the principles underlying exercise-induced improvements in bone metabolism, ultimately providing novel insights into the treatment of bone metabolic disorders.
The skeleton is a dynamic structure that provides architectural support for posture control, and adapts to physical activity. Bones comprise a collagen matrix infused with calcium and phosphate deposits and various proteins, enabling it to store minerals and regulate nutrient and endocrine functions (Frost, 1994; Hart et al., 2020). Peak bone mass is achieved during young adulthood and declines with reduced physical activity, aging, glucocorticoids use, and metabolic diseases, increasing the risk of osteoporosis, which predisposes people to fragility fractures. This disease impairs mobility, prolongs bed rest in older patients, and even increases mortality (Tsugawa and Shiraki, 2020; Golden et al., 2014; Clynes et al., 2020).
Mechanical stimulation caused by exercise affects bone density. A lack of physical activity, such as from prolonged bed rest and microgravity, leads to insufficient mechanical stimulation and disrupts the balance between bone formation and resorption, thereby accelerating bone loss and exacerbating osteoporosis (D'Onofrio et al., 2023; Watson et al., 2018; Hu et al., 2023). In contrast, regular physical activity, such as resistance and impact exercises, improves bone remodeling and enhances bone mineralization, bone mineral density (BMD), and structural properties. This improves balance and postural stability and ultimately reducing the risk of falls, osteoporotic fractures, and related functional impairments (Kistler-Fischbacher et al., 2021; Lyu et al., 2024; Cai et al., 2023). The mechanisms through which exercise improves bone metabolism involve numerous regulatory factors.
Silent information regulator of transcription 1 (SIRT1) is a member of the sirtuin family of nicotinamide adenine dinucleotide (NAD+)-dependent class III histone deacetylases. These molecules catalyze the deacetylation of protein substrates during NAD+-mediated cleavage (Canto and Auwerx, 2012). SIRT1 regulates multiple biological processes, such as glucose and lipid metabolism, immune response, and endocrine function, in various tissues, including muscle, adipose tissue, and liver (Nogueiras et al., 2012; Huang et al., 2021; Qiang et al., 2011; Chang and Guarente, 2014). Studies have increasingly highlighted the significance of SIRT1 in bone metabolism. SIRT1 is implicated in the regulation of bone homeostasis and prevention of bone-related disorders. SIRT1 expression is reduced in the femoral neck of osteoporotic patients and is negatively correlated with sclerostin, an inhibitor of bone formation (El-Haj et al., 2016). Consistently, SIRT1 knockout mice exhibit a low bone mass phenotype. Highlighting the regulatory role of SIRT1 in bone density (Zainabadi et al., 2017). Notably, high-intensity and repeated exercise training stimulate an increase in SIRT1 expression. In response to the mechanical stress induced by exercise, SIRT1 plays a pivotal role in regulating both bone formation and resorption (Zhu et al., 2023; Kauppinen et al., 2013; Juan et al., 2023). Here, we review the mechanisms by which SIRT1 modulates bone metabolism through various direct and indirect biological processes.
Bone health is maintained through a delicate balance between bone formation, mediated by osteoblasts, and bone resorption, regulated by osteoclasts. Bone marrow mesenchymal stem cells (BMSCs), which are multipotent stem cells capable of differentiating into osteoblasts, adipocytes, and chondrocytes (Fu et al., 2019). The stable differentiation of BMSCs into osteoblasts promotes bone formation, while differentiation into adipocytes competitively inhibits it (Caplan and Bruder, 2001; Gao et al., 2023). Therefore, regulating the adipogenesis/osteogenesis ratio is essential in bone metabolism. Fortunately, some studies have shown that mechanical movement not only promotes osteogenic differentiation, but also reduces the adipogenic/osteogenic ratio and promotes bone formation (Liu et al., 2024; Shi et al., 2022).
Osteoclasts originate from the monocyte/macrophage lineage of hematopoietic stem cells. An increase in osteoclastogenesis enhances bone resorption, contributing to decreased bone density (Boyle et al., 2003). External factors such as estrogen deficiency and aging can disrupt bone homeostasis by affecting processes such as inflammation, autophagy, oxidative stress, and aging, ultimately affecting bone formation and resorption and triggering metabolic bone diseases such as osteoporosis (Curtis et al., 2015; O'Brien, 1996). Exercise reduces the levels of the receptor activator of nuclear factor-kappa B ligand (RANKL), a key membrane-bound factor involved in osteoclast differentiation, thereby reducing osteoclast activity and inhibiting bone resorption (Shi et al., 2022).
SIRT1 influences exercise-regulated osteoblast and osteoclast formation. For example, low-magnitude vibration training inhibits the SIRT1/p53/p21 pathway, alleviates osteoblast senescence, and subsequently improves bone architecture of osteoporosis, thereby reducing bone loss and fracture incidence (Wen et al., 2021; Bianchi et al., 2022). Additionally, interval running downregulates RANKL expression in bone marrow macrophages, thereby reducing osteoclast formation and bone resorption. This effectively alleviate bone loss caused by menopause and promotes high SIRT1 expression, which regulates the BMP signaling pathways to enhance osteogenic differentiation and bone formation (Kim et al., 2019). The mechanisms by which exercise regulates SIRT1 and influences bone metabolism are outlined in the following sections.
Inflammation has a profound effect on bone metabolism. During the early acute phase of inflammation, inflammatory macrophages (M1) increase the expressions of pro-inflammatory factors such as tumor necrosis factor alpha (TNF-α), IL-1, and IL-6. These factors enhance osteoclast differentiation and activity while inhibiting osteoblast differentiation (Munoz et al., 2020). In contrast, during the later chronic inflammatory phase, M1 macrophages polarize into tissue-regenerative macrophages (M2) that secrete growth factors that promote BMSC-mediated bone formation and bone remodeling (Murray and Wynn, 2011; Mosser and Edwards, 2008). However, under conditions of estrogen deficiency or aging, the failure of M1 to M2 polarization leads to the activation of RANKL and NF-κB-mediated osteoclast differentiation and bone resorption (Yao et al., 2021).
Exercise is integral in regulating the inflammatory response, preventing and reducing osteoporosis (Zhang et al., 2022). Appropriate exercise increases the secretion of anti-inflammatory cytokines, such as IL-2, IL-10, IL-12, and interferon, which support bone formation, while reducing the secretion of proinflammatory cytokines like IL-1 (Yuan et al., 2016; Zhang et al., 2022). Interestingly, high-intensity exercise can induce osteoarthritis. For example, increasing speed running (80 min/day, 5°, maximum speed 20 m/min for 60 min) promotes the level of proinflammatory factor TNF-α and reduces the levels of cartilage structural proteins COL2A and ACAN. In contrast, moderate-intensity running (40 min/day, constant speed of 8 m/min) attenuates cartilage inflammation, reduces the level of proinflammatory factor TNF-α, and results in less morphological damage to articular cartilage (as indicated by the Mankin score), along with a higher density of subchondral trabeculae (Cao et al., 2022). These findings suggest that while excessive exercise may harm bone and joints, exercise of appropriate intensity is beneficial to bone and joint health.
Exercise modulates SIRT1 expression and helps mitigate inflammation in diseases. For example, 8 weeks moderate-intensity treadmill and swimming training (1 h/day) activated SIRT1, leading to a reduction in proinflammatory factors such asIL-1β, IL-6, and TNF-α, as well as NF-κB-p65, thereby alleviating tissue damage and improving the dysfunction in type 2 diabetes mellitus mice (Lang et al., 2020; Habibi et al., 2022). Remarkably, activated SIRT1 promotes M2 macrophage formation, inhibits NF-κB and TNF-α levels, and accelerates the resolution of the inflammatory response. This, in turn, reduces osteoclast differentiation, survival and bone resorption, helping to maintain BMD (Kauppinen et al., 2013; Ye et al., 2022; Park et al., 2023).
Aging is a complex process that affects the stability of various physiological functions, including those of the skeletal and endocrine system, and leads to diseases such as osteoporosis, osteoarthritis, and sarcopenia (Safiri et al., 2020). The initial stage of aging is cellular senescence, which results from DNA damage and mitochondrial dysfunction (He et al., 2023). This process leads to cell cycle arrest, reduced activity of mesenchymal cells, and a decline in bone turnover, thereby impairing bone formation and enhancing bone resorption, ultimately contributing to bone fragility (Samsonraj et al., 2023; Falvino et al., 2024). During cellular senescence, autophagic activity, energy metabolism regulation, stress resistance, and metabolic status decline (Chen et al., 2020). Aging of BMSCs leads to functional and structural degeneration, reduced proliferative capacity, diminished osteogenic differentiation, increased adipogenic differentiation, and exacerbated bone–fat imbalance (Qadir et al., 2020; Zhang et al., 2011). Concurrently, the aging of osteoblasts further contributes to a reduction in bone formation and promotes bone aging, bone loss, and hinders skeletal healing and recovery (Lin et al., 2019).
Exercise upregulates the expression of SIRT1 in aging mice. Treadmill exercise, for example, upregulates SIRT1 expression in the bones of aging mice (Zhu et al., 2023). SIRT1 activation plays a key role in regulating BMSCs, bone collagen, and bone capillaries. The expression of SIRT1 is downregulated in aged BMSCs. Overexpression of SIRT1 has been shown to delay MSC aging without impairing their adipogenic and osteogenic potential. In contrast, knockdown of SIRT1 slows BMSCs growth and accelerates cellular aging, primarily through the activation of the aging marker P16 (Yuan et al., 2012; Ozturk et al., 2009). Furthermore, SIRT1 knockout mice exhibit a 50% reduction in cortical bone density and display significantly reduced biomechanical strength (Artsi et al., 2022). Specifically, the activation of SIRT1 leads to deacetylation and subsequent inhibition of Kremen1, a senescence-specific surface marker, which mitigates the aging of BMSCs and restores the self-repair capacity of aged bones (Wang et al., 2019; Chen et al., 2020). Additionally, SIRT1 regulates the osteogenic and adipogenic differentiation of BMSCs, promoting bone formation and suppressing fat accumulation, thereby delaying bone loss in aging mice (Song et al., 2019). Moreover, SIRT1 exerts anti-senescence effects on osteoblasts by inhibiting the degradation of bone matrix collagen, enhancing osteoblast activity, reducing DNA damage, and minimizing the accumulation of harmful substances such as cadmium (Zhang et al., 2013; Zhou et al., 2023). Notably, exercise also enhances angiogenesis, improves blood supply to the musculoskeletal system, and increases capillary density through the upregulation of SIRT1, which in turn boosts exercise endurance and performance (Das et al., 2018).
Autophagy is a metabolic process that selectively degrades and recycles damaged or aged organelles to maintain cell homeostasis and survival. Autophagy is essential for maintaining stem cell stemness and differentiation capacity (Garcia-Prat et al., 2016; Wang et al., 2020). Mitophagy promotes intracellular homeostasis by reducing intracellular reactive oxygen species (ROS) produced by damaged mitochondria and by generating ATP during degradation processes (Golden et al., 2010). In bone tissue, Autophagy determines the regenerative potential and function of BMSCs by providing the energy and metabolic precursors required for differentiation (Ceccariglia et al., 2020; Wang et al., 2023). Autophagy also regulates osteoblast differentiation and mineralization by interacting with signaling and transcription factors such as BMP and ATF4 (Wang et al., 2023; B'Chir et al., 2013; Yang et al., 2021).
Running exercise enhances autophagy levels in osteoporotic and type 2 diabetic mice, as evidenced by increased levels of the autophagy marker LC3II and improved bone density (Zhu et al., 2023; Chen et al., 2021). SIRT1-mediated autophagy improves cellular function of bone cells (Wang et al., 2021; Ye et al., 2022; Kim et al., 2022). Mechanical strain upregulate both the mRNA and protein expression of SIRT1, increasing autophagy and enhancing osteogenic differentiation of BMSCs. SIRT1 knockdown diminishes autophagy level in BMSCs by stretch stimulation, demonstrating that mechanical stimuli promote autophagy in BMSCs via SIRT1 activation, thereby regulating osteogenic differentiation (Zhu et al., 2023). Furthermore, activated SIRT1 protected osteoblast activity and differentiation from dexamethasone and fluoride damage by deacetylation of FoxO1 and its target substrates Rab7 and Bnip3, as well as the PI3K/Akt/mTOR pathway (Yang et al., 2019; Gu et al., 2016).
Oxidative stress generates ROS that regulate cell behavior, extracellular matrix comp osition, and bone tissue architecture (Cerqueni et al., 2021). Various factors, including unbalanced diet, smoking, and genetic predisposition, increase ROS levels, exacerbate oxidative stress, suppress osteoblast activity and differentiation, induce apoptosis, and compromise bone mineralization and osteogenesis (Cerqueni et al., 2021; Bai et al., 2004). H2O2-induced oxidative stress enhances adipogenic markers such as PPARγ2 and LEPTIN, and downregulates osteogenic factors such as RUNX2 and COL1A, thereby increasing the fat/bone ratio and weakening the osteogenic effect of BMSCs (Lin et al., 2018).
Exercise training restores the abnormal redox balance and downregulates the expression of hypoxia-inducible factor-1 alpha, a key regulatory factor under hypoxic conditions, thereby alleviating bone degeneration (Sierra-Ramirez et al., 2022). Swimming activates SIRT1/AMPK signaling and upregulates the antioxidant gene NRF2, leading to a reduction in pathological ROS overproduction (Zou et al., 2021). Activated SIRT1 regulates osteoclast and bone-fat imbalance. One study revealed that SIRT1 inhibits oxidative stress-related pathways in bone by deacetylating p66Shc, thereby suppressing the ROS/NF-κB signaling pathway in response to high glucose and palmitate-induced osteoclast differentiation (Qu et al., 2018). Whilst, Resveratrol-induced SIRT1 activation inhibits excessive adipogenesis, improves adipogenic/osteogenic imbalance, promotes bone formation by deacetylation of FOXO3a, the ROS-sensitive transcription factor, and increasing RUNX2 transcription (Lin et al., 2018; Tseng et al., 2011).
In addition to its direct effects on the skeleton, physical activity exerts indirect regulatory effects on bone metabolism through various systems, including the skeletal muscle, nervous system, immune system and endocrine system (Yuan and Song, 2022). For example, exercise promotes myostatin, interleukin-6, Irisin, and apelin in muscles to enter the blood, allowing them to act on bones and maintain the balance between bone absorption and bone formation (Zhao et al., 2023). Among these interactions, SIRT1 is a crucial regulatory factor in the crosstalk (Dolan et al., 2020).
A biomechanical connection exists between skeletal muscle and bone. The bone responds to the mechanical load generated by muscle contraction and adapts its mass and structure to meet the demands of movement. Skeletal muscle fibers, also known as skeletal muscle cells, are large, multinucleated cells. In individuals with sarcopenia, decreased muscle fiber volume and function lead to reduced mechanical bone loading, resulting in bone loss (Frost, 2003; Trajanoska et al., 2019). Conversely, effective muscle contraction provides specific mechanical load delivery to the bones in terms of frequency, rate, amplitude, and distribution, thereby stimulating bone growth (Hsieh and Turner, 2001; Hart et al., 2017). Moderate-to high-intensity exercise, such as progressive resistance and impact exercise training significantly improved muscle strength and BMD (O'Bryan et al., 2022; Ng et al., 2023). However, low-intensity aerobic exercise and swimming (less than 3 h/week) had no effect on BMD (Abe et al., 2022; Su et al., 2020). Exercise-induced expression of SIRT1 in muscle cells enhances muscle contraction by increasing the number of myonuclei and size of the myonuclear domain (Radak et al., 2020; Ross et al., 2018). In addition, regular exercise can promote cell proliferation by increasing SIRT1 expression in satellite cells, restoring the regenerative capacity of aged muscle and promoting the repair of muscle damage (Myers et al., 2019).
Exercise accelerates muscle energy metabolism. Increased energy expenditure during physical activity increases ATP demand, resulting in elevated levels of NAD+ (White and Schenk, 2012). Notably, decreased NAD+ levels in aging or sarcopenic populations can exacerbate physiological decline (Mills et al., 2016). However, aerobic exercise restores NAD+ levels, potentially delaying the progression of related diseases (De la Rosa et al., 2020; Hu et al., 2021; Wu et al., 2023; Mills et al., 2016). The increase in NAD+ levels provides a substrate for SIRT1, which promotes mitochondrial biogenesis through both PGC-1α-dependent and -independent pathways, thereby regulating energy metabolism, DNA damage repair, muscle cell differentiation, and functionality (White and Schenk, 2012; Koltai et al., 2010).
By regulating mitochondrial homeostasis, exercise enhances muscle function. Intense physical activity activates SIRT1, leading to increased mitochondrial biogenesis and oxidative capacity, which, in turn, improves the content and functionality of skeletal muscle mitochondria (Vargas-Ortiz et al., 2019; Williams and Gurd, 2012). Furthermore, SIRT1 activation maintains the vitality of soleus muscle mitochondria, even under the microgravity of space flight, by oxidizing palmitoylcarnitine. This activation also improves soleus muscle mass and maximum contraction, attenuates muscle atrophy, and ultimately alleviates declining femoral BMD and strength (Momken et al., 2011). Interestingly, there seems to be a gender difference in SIRT1 secretion by muscle. After 6 months of high-intensity aerobic weight loss exercise in people over 50 years old, SIRT1 expression in the vastus lateralis increased, and women were higher than men (Ryan and Li, 2023). Another study showed that after an acute session of sprint interval training, there was no gender difference in the increase of SIRT1 after exercise (Dun et al., 2024), which may be related to the type and duration of exercise. Women have a stronger ability to oxidize fat, which gives them a unique advantage in long-term endurance exercise (Maher et al., 2009).
Through biochemical stimulation, exercise accelerates muscle function. Myokines, such as myostatin and irisin, which are products of muscle secretion, also transmit signals between muscle and bone through endocrine pathways (Herrmann et al., 2020; Zhao et al., 2023). Intermittent running promotes muscle mass and growth in rats with sarcopenia by upregulating the muscle growth factor myogenin, and enhancing SIRTs (Kim et al., 2019). Irisin, a cleavage product of fibronectin type III domain-containing protein 5 (FNDC5), is a well-known muscle-secreted peptide whose expression increases during exercise and is positively correlated with bone mechanical properties (Tenorio et al., 2017; Liu et al., 2021; Zhang et al., 2020). The injection of irisin into mice induces skeletal muscle hypertrophy and enhances muscle strength. This effect is presumably mediated by alleviating skeletal muscle atrophy through muscle stem cell activation and enhanced protein synthesis (Reza et al., 2017). A study of resistance exercise in mice with myocardial infarction suggested that irisin improves myocardial fibrosis by activating the AMPK-SIRT1 pathway. Based on these findings, we infer that SIRT1 plays a vital role in the crosstalk between exercise and the muscle–bone interface.
Bone metabolism is directly controlled by the central nervous system. Various central neurohormones, neuropeptides, neurotransmitters, and their intracellular signaling pathways regulate bone metabolism (Huang et al., 2019). Brain-derived neurotrophic factor (BDNF) is widely distributed in the mammalian brain where it regulates neuronal differentiation and dopaminergic neurotransmission (Bi et al., 2024). It is an instructive mediator of central nervous system functional and structural plasticity and is essential for neuronal maintenance, support, and regeneration; it participates in memory synthesis and consolidation (Colucci-D'Amato et al., 2020; Allen et al., 2013).
BDNF regulates osteoblast differentiation, elicits new bone formation, and facilitate fracture healing in bone cells (Ida-Yonemochi et al., 2017; Kilian et al., 2014). Furthermore, BDNF facilitates the osteogenic differentiation of BMSCs by binding to the TrkB receptor and phosphorylates downstream Erk1/2 (Liu et al., 2018). Exercise promotes BDNF expression through SIRT1 activation. For example, an 8-week treadmill exercise regimen was shown to activate SIRT1 and regulates the PGC-1α/FNDC5/BDNF signaling pathway, resulting in increased BDNF concentration and decreased levels of pro-inflammatory cytokines IL-1β, IL-6, and TNF-α in the hippocampus of mice. These changes ultimately enhanced BDNF release and improved cognitive impairments (Lang et al., 2020). Similarly, 1 month of voluntary running wheel exercise induced BDNF expression through SIRT1-dependent upregulation of PGC-1α (El Hayek et al., 2019). Based on these findings, we deduce that exercise promotes bone metabolism by enhancing neural–bone–muscle regulation mediated by SIRT1.
The close connection between SIRT1 and exercise, as well as its impact on bone metabolism, suggests that SIRT1 influences bone metabolism through multiple mechanisms during exercise, including biological processes such as inflammation, aging, autophagy, and oxidative stress, and through the crosstalk between muscle–bone and nerve–bone interactions (Figure 1). We also summarize the different forms and doses of exercise that improve bone metabolism through SIRT1 (Table 1). Since there are limited studies on the direct regulation of SIRT1 by exercise to improve bone metabolism, this review is mostly indirect analysis and summary. The minor number of animal experiments is also a limitation of this article. Based on our findings, we hypothesized that SIRT1 is an emerging exercise effector with potential for regulating bone health. Further research on the specific mechanism of SIRT1-mediated exercise regulation of bone health will help to formulate more reasonable exercise prescriptions and develop effective strategies to prevent and improve bone metabolic diseases such as osteoporosis.
Figure 1. Exercise affects bone metabolism through SIRT1. SIRT1 regulates bone metabolism through inflammation, aging, autophagy and oxidative stress, and through muscle-bone and nerve-bone crosstalk. [This figure was created using BioRender (https://BioRender.com)].
LjL: Writing–original draft, Investigation. JZ: Writing–original draft, Data curation. RC: Writing–review and editing, Visualization. NW: Writing–review and editing, Investigation. YZ: Data curation, Writing–review and editing. LfL: Writing–original draft, Funding acquisition. XZ: Writing–review and editing, Methodology, Project administration, Supervision. QL: Writing–review and editing, Funding acquisition, Conceptualization.
The author(s) declare that financial support was received for the research, authorship, and/or publication of this article. This research was supported by Liaoning Science and Technology Plan (No. 2023-BS-041).
Thanks to the corresponding author for the overall control and funding of the article.
The authors declare that the research was conducted in the absence of any commercial or financial relationships that could be construed as a potential conflict of interest.
The author(s) declare that no Generative AI was used in the creation of this manuscript.
All claims expressed in this article are solely those of the authors and do not necessarily represent those of their affiliated organizations, or those of the publisher, the editors and the reviewers. Any product that may be evaluated in this article, or claim that may be made by its manufacturer, is not guaranteed or endorsed by the publisher.
Abe, K., Miyakoshi, N., Kasukawa, Y., Nozaka, K., Tsuchie, H., Sato, C., et al. (2022). Effects of teriparatide and low-intensity aerobic exercise on osteopenia in type 2 diabetes mellitus rats. J. Bone Min. Metab. 40 (2), 229–239. doi:10.1007/s00774-021-01289-0
Allen, S. J., Watson, J. J., Shoemark, D. K., Barua, N. U., and Patel, N. K. (2013). GDNF, NGF and BDNF as therapeutic options for neurodegeneration. Pharmacol. Ther. 138 (2), 155–175. doi:10.1016/j.pharmthera.2013.01.004
Artsi, H., Cohen-Kfir, E., Shahar, R., Kalish-Achrai, N., Lishinsky, N., and Dresner-Pollak, R. (2022). SIRT1 haplo-insufficiency results in reduced cortical bone thickness, increased porosity and decreased estrogen receptor alpha in bone in adult 129/Sv female mice. Front. Endocrinol. (Lausanne) 13, 1032262. doi:10.3389/fendo.2022.1032262
Bai, X. C., Lu, D., Bai, J., Zheng, H., Ke, Z. Y., Li, X. M., et al. (2004). Oxidative stress inhibits osteoblastic differentiation of bone cells by ERK and NF-kappaB. Biochem. Biophys. Res. Commun. 314 (1), 197–207. doi:10.1016/j.bbrc.2003.12.073
B'Chir, W., Maurin, A. C., Carraro, V., Averous, J., Jousse, C., Muranishi, Y., et al. (2013). The eIF2α/ATF4 pathway is essential for stress-induced autophagy gene expression. Nucleic Acids Res. 41 (16), 7683–7699. doi:10.1093/nar/gkt563
Bi, X., Fang, J., Jin, X., and Thirupathi, A. (2024). The interplay between BDNF and PGC-1 alpha in maintaining brain health: role of exercise. Front. Endocrinol. (Lausanne) 15, 1433750. doi:10.3389/fendo.2024.1433750
Bianchi, M. L., Vai, S., Baranello, G., Broggi, F., Judex, S., Hangartner, T., et al. (2022). Low-intensity vibration protects the weight-bearing skeleton and suppresses fracture incidence in boys with duchenne muscular dystrophy: a prospective, randomized, Double-Blind, Placebo-controlled clinical trial. JBMR Plus 6 (11), e10685. doi:10.1002/jbm4.10685
Boyle, W. J., Simonet, W. S., and Lacey, D. L. (2003). Osteoclast differentiation and activation. Nature 423 (6937), 337–342. doi:10.1038/nature01658
Cai, G., Lu, Y., Zhong, W., Wang, T., Li, Y., Ruan, X., et al. (2023). Piezo1-mediated M2 macrophage mechanotransduction enhances bone formation through secretion and activation of transforming growth factor-β1. Cell Prolif. 56 (9), e13440. doi:10.1111/cpr.13440
Canto, C., and Auwerx, J. (2012). Targeting sirtuin 1 to improve metabolism: all you need is NAD(+)? Pharmacol. Rev. 64 (1), 166–187. doi:10.1124/pr.110.003905
Cao, H., Zhou, X., Li, H., Wang, M., Wu, W., and Zou, J. (2022). Study on the role of MicroRNA-214 in the Rehabilitation of cartilage in mice with exercise-induced Traumatic osteoarthritis. Curr. Issues Mol. Biol. 44 (9), 4100–4117. doi:10.3390/cimb44090281
Caplan, A. I., and Bruder, S. P. (2001). Mesenchymal stem cells: building blocks for molecular medicine in the 21st century. Trends Mol. Med. 7 (6), 259–264. doi:10.1016/s1471-4914(01)02016-0
Ceccariglia, S., Cargnoni, A., Silini, A. R., and Parolini, O. (2020). Autophagy: a potential key contributor to the therapeutic action of mesenchymal stem cells. Autophagy 16 (1), 28–37. doi:10.1080/15548627.2019.1630223
Cerqueni, G., Scalzone, A., Licini, C., Gentile, P., and Mattioli-Belmonte, M. (2021). Insights into oxidative stress in bone tissue and novel challenges for biomaterials. Mater Sci. Eng. C Mater Biol. Appl. 130, 112433. doi:10.1016/j.msec.2021.112433
Chang, H. C., and Guarente, L. (2014). SIRT1 and other sirtuins in metabolism. Trends Endocrinol. Metab. 25 (3), 138–145. doi:10.1016/j.tem.2013.12.001
Chen, C., Zhou, M., Ge, Y., and Wang, X. (2020). SIRT1 and aging related signaling pathways. Mech. Ageing Dev. 187, 111215. doi:10.1016/j.mad.2020.111215
Chen, X., Yang, K., Jin, X., Meng, Z., Liu, B., Yu, H., et al. (2021). Bone autophagy: a potential Way of exercise-mediated Meg3/P62/Runx2 pathway to regulate bone formation in T2DM mice. Diabetes Metab. Syndr. Obes. 14, 2753–2764. doi:10.2147/DMSO.S299744
Clynes, M. A., Harvey, N. C., Curtis, E. M., Fuggle, N. R., Dennison, E. M., and Cooper, C. (2020). The epidemiology of osteoporosis. Br. Med. Bull. 133 (1), 105–117. doi:10.1093/bmb/ldaa005
Colucci-D'Amato, L., Speranza, L., and Volpicelli, F. (2020). Neurotrophic factor BDNF, physiological functions and therapeutic potential in Depression, neurodegeneration and brain Cancer. Int. J. Mol. Sci. 21 (20), 7777. doi:10.3390/ijms21207777
Curtis, E., Litwic, A., Cooper, C., and Dennison, E. (2015). Determinants of muscle and bone aging. J. Cell Physiol. 230 (11), 2618–2625. doi:10.1002/jcp.25001
Das, A., Huang, G. X., Bonkowski, M. S., Longchamp, A., Li, C., Schultz, M. B., et al. (2013). Impairment of an endothelial NAD(+)-H(2)S signaling network is a reversible cause of vascular aging. Cell. 173(1), 74–89. doi:10.1016/j.cell.2019.01.026
De la Rosa, A., Olaso-Gonzalez, G., Arc-Chagnaud, C., Millan, F., Salvador-Pascual, A., Garcia-Lucerga, C., et al. (2020). Physical exercise in the prevention and treatment of Alzheimer's disease. J. Sport Health Sci. 9 (5), 394–404. doi:10.1016/j.jshs.2020.01.004
Dolan, E., Varley, I., Ackerman, K. E., Pereira, R. M. R., Elliott-Sale, K. J., and Sale, C. (2020). The bone metabolic response to exercise and Nutrition. Exerc Sport Sci. Rev. 48 (2), 49–58. doi:10.1249/JES.0000000000000215
D'Onofrio, G., Kirschner, J., Prather, H., Goldman, D., and Rozanski, A. (2023). Musculoskeletal exercise: its role in promoting health and longevity. Prog. Cardiovasc Dis. 77, 25–36. doi:10.1016/j.pcad.2023.02.006
Dun, Y., Zhang, W., Du, Y., Xie, K., Liu, Y., Li, C., et al. (2024). High-intensity interval training mitigates sarcopenia and suppresses the Myoblast senescence regulator EEF1E1. J. Cachexia Sarcopenia Muscle 15 (6), 2574–2585. doi:10.1002/jcsm.13600
El-Haj, M., Gurt, I., Cohen-Kfir, E., Dixit, V., Artsi, H., Kandel, L., et al. (2016). Reduced Sirtuin1 expression at the femoral neck in women who sustained an osteoporotic hip fracture. Osteoporos. Int. 27 (7), 2373–2378. doi:10.1007/s00198-016-3536-4
El Hayek, L., Khalifeh, M., Zibara, V., Abi Assaad, R., Emmanuel, N., Karnib, N., et al. (2019). Lactate Mediates the effects of exercise on Learning and memory through SIRT1-dependent activation of hippocampal brain-derived neurotrophic factor (BDNF). J. Neurosci. 39 (13), 2369–2382. doi:10.1523/JNEUROSCI.1661-18.2019
Falvino, A., Gasperini, B., Cariati, I., Bonanni, R., Chiavoghilefu, A., Gasbarra, E., et al. (2024). Cellular senescence: the Driving Force of musculoskeletal diseases. Biomedicines 12 (9), 1948. doi:10.3390/biomedicines12091948
Frost, H. M. (1994). Wolff's Law and bone's structural adaptations to mechanical usage: an overview for clinicians. Angle Orthod. 64 (3), 175–188. doi:10.1043/0003-3219(1994)064<0175:WLABSA>2.0.CO;2
Frost, H. M. (2003). Bone's mechanostat: a 2003 update. Anat. Rec. A Discov. Mol. Cell Evol. Biol. 275 (2), 1081–1101. doi:10.1002/ar.a.10119
Fu, X., Liu, G., Halim, A., Ju, Y., Luo, Q., and Song, A. G. (2019). Mesenchymal stem cell Migration and tissue repair. Cells 8 (8), 784. doi:10.3390/cells8080784
Gao, J. W., Song, M. K., Wu, D. Z., Yan, T., Zhao, K., Huang, Y. S., et al. (2023). CircRBM23 regulates the switch between osteogenesis and adipogenesis of mesenchymal stem cells via sponging miR-338-3p. Clin. Sci. (Lond) 137 (6), 495–510. doi:10.1042/CS20220833
Garcia-Prat, L., Martinez-Vicente, M., Perdiguero, E., Ortet, L., Rodriguez-Ubreva, J., Rebollo, E., et al. (2016). Autophagy maintains stemness by preventing senescence. Nature 529 (7584), 37–42. doi:10.1038/nature16187
Golden, N. H., Abrams, S. A., and Committee on, N. (2014). Optimizing bone health in children and adolescents. Pediatrics 134 (4), e1229–e1243. doi:10.1542/peds.2014-2173
Gu, X., Han, D., Chen, W., Zhang, L., Lin, Q., Gao, J., et al. (2016). SIRT1-mediated FoxOs pathways protect against apoptosis by promoting autophagy in osteoblast-like MC3T3-E1 cells exposed to sodium fluoride. Oncotarget 7 (40), 65218–65230. doi:10.18632/oncotarget.11573
Habibi, P., Ahmadiasl, N., Nourazarian, A., and Yousefi, H. (2022). Swimming exercise improves SIRT1, NF-κB, and IL-1β protein levels and pancreatic tissue injury in ovariectomized diabetic rats. Horm. Mol. Biol. Clin. Investig. 43 (3), 345–352. doi:10.1515/hmbci-2021-0069
Hart, N. H., Newton, R. U., Tan, J., Rantalainen, T., Chivers, P., Siafarikas, A., et al. (2020). Biological basis of bone strength: anatomy, physiology and measurement. J. Musculoskelet. Neuronal Interact. 20 (3), 347–371.
Hart, N. H., Nimphius, S., Rantalainen, T., Ireland, A., Siafarikas, A., and Newton, R. U. (2017). Mechanical basis of bone strength: influence of bone material, bone structure and muscle action. J. Musculoskelet. Neuronal Interact. 17 (3), 114–139.
He, X., Hu, W., Zhang, Y., Chen, M., Ding, Y., Yang, H., et al. (2023). Cellular senescence in skeletal disease: mechanisms and treatment. Cell Mol. Biol. Lett. 28 (1), 88. doi:10.1186/s11658-023-00501-5
Herrmann, M., Engelke, K., Ebert, R., Muller-Deubert, S., Rudert, M., Ziouti, F., et al. (2020). Interactions between muscle and bone-where Physics meets Biology. Biomolecules 10 (3), 432. doi:10.3390/biom10030432
Hsieh, Y. F., and Turner, C. H. (2001). Effects of loading frequency on mechanically induced bone formation. J. Bone Min. Res. 16 (5), 918–924. doi:10.1359/jbmr.2001.16.5.918
Hu, Q., Wu, D., Walker, M., Wang, P., Tian, R., and Wang, W. (2021). Genetically encoded biosensors for evaluating NAD(+)/NADH ratio in cytosolic and mitochondrial compartments. Cell Rep. Methods 1 (7), 100116. doi:10.1016/j.crmeth.2021.100116
Hu, Y., Tian, H., Chen, W., Liu, Y., Cao, Y., Pei, H., et al. (2023). The critical role of the Piezo1/β-catenin/ATF4 Axis on the stemness of Gli1+ BMSCs during Simulated microgravity-induced bone loss. Adv. Sci. (Weinh) 10 (32), e2303375. doi:10.1002/advs.202303375
Huang, Q., Su, H., Qi, B., Wang, Y., Yan, K., Wang, X., et al. (2021). A SIRT1 activator, Ginsenoside Rc, promotes energy metabolism in Cardiomyocytes and Neurons. J. Am. Chem. Soc. 143 (3), 1416–1427. doi:10.1021/jacs.0c10836
Huang, S., Li, Z., Liu, Y., Gao, D., Zhang, X., Hao, J., et al. (2019). Neural regulation of bone remodeling: Identifying novel neural molecules and pathways between brain and bone. J. Cell Physiol. 234 (5), 5466–5477. doi:10.1002/jcp.26502
Ida-Yonemochi, H., Yamada, Y., Yoshikawa, H., and Seo, K. (2017). Locally produced BDNF promotes Sclerotic change in Alveolar bone after nerve injury. PLoS One 12 (1), e0169201. doi:10.1371/journal.pone.0169201
Juan, C. G., Matchett, K. B., and Davison, G. W. (2023). A systematic review and meta-analysis of the SIRT1 response to exercise. Sci. Rep. 13 (1), 14752. doi:10.1038/s41598-023-38843-x
Kauppinen, A., Suuronen, T., Ojala, J., Kaarniranta, K., and Salminen, A. (2013). Antagonistic crosstalk between NF-κB and SIRT1 in the regulation of inflammation and metabolic disorders. Cell Signal 25 (10), 1939–1948. doi:10.1016/j.cellsig.2013.06.007
Kilian, O., Hartmann, S., Dongowski, N., Karnati, S., Baumgart-Vogt, E., Hartel, F. V., et al. (2014). BDNF and its TrkB receptor in human fracture healing. Ann. Anat. 196 (5), 286–295. doi:10.1016/j.aanat.2014.06.001
Kim, J. M., Yang, Y. S., Xie, J., Lee, O., Kim, J., Hong, J., et al. (2022). Regulation of sclerostin by the SIRT1 stabilization pathway in osteocytes. Cell Death Differ. 29 (8), 1625–1638. doi:10.1038/s41418-022-00952-x
Kim, J. S., Jeon, J., An, J. J., and Yi, H. K. (2019). Interval running training improves age-related skeletal muscle wasting and bone loss: experiments with ovariectomized rats. Exp. Physiol. 104 (5), 691–703. doi:10.1113/EP087458
Kistler-Fischbacher, M., Weeks, B. K., and Beck, B. R. (2021). The effect of exercise intensity on bone in postmenopausal women (part 1): a systematic review. Bone 143, 115696. doi:10.1016/j.bone.2020.115696
Koltai, E., Szabo, Z., Atalay, M., Boldogh, I., Naito, H., Goto, S., et al. (2010). Exercise alters SIRT1, SIRT6, NAD and NAMPT levels in skeletal muscle of aged rats. Mech. Ageing Dev. 131 (1), 21–28. doi:10.1016/j.mad.2009.11.002
Lang, X., Zhao, N., He, Q., Li, X., Li, X., Sun, C., et al. (2020). Treadmill exercise mitigates neuroinflammation and increases BDNF via activation of SIRT1 signaling in a mouse model of T2DM. Brain Res. Bull. 165, 30–39. doi:10.1016/j.brainresbull.2020.09.015
Lin, C. H., Li, N. T., Cheng, H. S., and Yen, M. L. (2018). Oxidative stress induces imbalance of adipogenic/osteoblastic lineage commitment in mesenchymal stem cells through decreasing SIRT1 functions. J. Cell Mol. Med. 22 (2), 786–796. doi:10.1111/jcmm.13356
Lin, H., Sohn, J., Shen, H., Langhans, M. T., and Tuan, R. S. (2019). Bone marrow mesenchymal stem cells: aging and tissue engineering applications to enhance bone healing. Biomaterials 203, 96–110. doi:10.1016/j.biomaterials.2018.06.026
Liu, L., Guo, J., Chen, X., Tong, X., Xu, J., and Zou, J. (2021). The role of irisin in exercise-mediated bone health. Front. Cell Dev. Biol. 9, 668759. doi:10.3389/fcell.2021.668759
Liu, L., Guo, J., Tong, X., Zhang, M., Chen, X., Huang, M., et al. (2024). Mechanical strain regulates osteogenesis via Antxr1/LncRNA H19/Wnt/β-catenin axis. J. Cell Physiol. 239 (5), e31214. doi:10.1002/jcp.31214
Liu, Q., Lei, L., Yu, T., Jiang, T., and Kang, Y. (2018). Effect of brain-derived neurotrophic factor on the Neurogenesis and osteogenesis in bone engineering. Tissue Eng. Part A 24 (15-16), 1283–1292. doi:10.1089/ten.TEA.2017.0462
Lyu, F. F., Ramoo, V., Chui, P. L., and Ng, C. G. (2024). Efficacy of Mindfulness exercises for primary osteoporosis Pain and balance: a systematic review and Network meta-analysis. Orthop. Nurs. 43 (5), 284–299. doi:10.1097/NOR.0000000000001059
Maher, A. C., Fu, M. H., Isfort, R. J., Varbanov, A. R., Qu, X. A., and Tarnopolsky, M. A. (2009). Sex differences in global mRNA content of human skeletal muscle. PLoS One 4 (7), e6335. doi:10.1371/journal.pone.0006335
Mills, K. F., Yoshida, S., Stein, L. R., Grozio, A., Kubota, S., Sasaki, Y., et al. (2016). Long-term Administration of nicotinamide mononucleotide mitigates age-associated physiological decline in mice. Cell Metab. 24 (6), 795–806. doi:10.1016/j.cmet.2016.09.013
Momken, I., Stevens, L., Bergouignan, A., Desplanches, D., Rudwill, F., Chery, I., et al. (2011). Resveratrol prevents the wasting disorders of mechanical unloading by acting as a physical exercise mimetic in the rat. FASEB J. 25 (10), 3646–3660. doi:10.1096/fj.10-177295
Mosser, D. M., and Edwards, J. P. (2008). Exploring the full spectrum of macrophage activation. Nat. Rev. Immunol. 8 (12), 958–969. doi:10.1038/nri2448
Munoz, J., Akhavan, N. S., Mullins, A. P., and Arjmandi, B. H. (2020). Macrophage polarization and osteoporosis: a review. Nutrients 12 (10), 2999. doi:10.3390/nu12102999
Murray, P. J., and Wynn, T. A. (2011). Protective and pathogenic functions of macrophage subsets. Nat. Rev. Immunol. 11 (11), 723–737. doi:10.1038/nri3073
Myers, M. J., Shepherd, D. L., Durr, A. J., Stanton, D. S., Mohamed, J. S., Hollander, J. M., et al. (2019). The role of SIRT1 in skeletal muscle function and repair of older mice. J. Cachexia Sarcopenia Muscle 10 (4), 929–949. doi:10.1002/jcsm.12437
Ng, C. A., Gandham, A., Mesinovic, J., Owen, P. J., Ebeling, P. R., and Scott, D. (2023). Effects of moderate-to high-impact exercise training on bone structure across the Lifespan: a systematic review and meta-analysis of Randomized controlled Trials. J. Bone Min. Res. 38 (11), 1612–1634. doi:10.1002/jbmr.4899
Nogueiras, R., Habegger, K. M., Chaudhary, N., Finan, B., Banks, A. S., Dietrich, M. O., et al. (2012). Sirtuin 1 and sirtuin 3: physiological modulators of metabolism. Physiol. Rev. 92 (3), 1479–1514. doi:10.1152/physrev.00022.2011
O'Brien, M. (1996). Osteoporosis and exercise. Br. J. Sports Med. 30 (3), 191. doi:10.1136/bjsm.30.3.191
O'Bryan, S. J., Giuliano, C., Woessner, M. N., Vogrin, S., Smith, C., Duque, G., et al. (2022). Progressive resistance training for Concomitant increases in muscle strength and bone mineral density in older Adults: a systematic review and meta-analysis. Sports Med. 52 (8), 1939–1960. doi:10.1007/s40279-022-01675-2
Ozturk, M., Arslan-Ergul, A., Bagislar, S., Senturk, S., and Yuzugullu, H. (2009). Senescence and immortality in hepatocellular carcinoma. Cancer Lett. 286 (1), 103–113. doi:10.1016/j.canlet.2008.10.048
Park, J. H., Lee, J., Lee, G. R., Kwon, M., Lee, H. I., Kim, N., et al. (2023). Cholesterol sulfate inhibits osteoclast differentiation and survival by regulating the AMPK-Sirt1-NF-κB pathway. J. Cell Physiol. 238 (9), 2063–2075. doi:10.1002/jcp.31064
Qadir, A., Liang, S., Wu, Z., Chen, Z., Hu, L., and Qian, A. (2020). Senile osteoporosis: the Involvement of differentiation and senescence of bone marrow stromal cells. Int. J. Mol. Sci. 21 (1), 349. doi:10.3390/ijms21010349
Qiang, L., Lin, H. V., Kim-Muller, J. Y., Welch, C. L., Gu, W., and Accili, D. (2011). Proatherogenic abnormalities of lipid metabolism in SirT1 transgenic mice are mediated through Creb deacetylation. Cell Metab. 14 (6), 758–767. doi:10.1016/j.cmet.2011.10.007
Qu, B., Gong, K., Yang, H., Li, Y., Jiang, T., Zeng, Z., et al. (2018). SIRT1 suppresses high glucose and palmitate-induced osteoclast differentiation via deacetylating p66Shc. Mol. Cell Endocrinol. 474, 97–104. doi:10.1016/j.mce.2018.02.015
Radak, Z., Suzuki, K., Posa, A., Petrovszky, Z., Koltai, E., and Boldogh, I. (2020). The systemic role of SIRT1 in exercise mediated adaptation. Redox Biol. 35, 101467. doi:10.1016/j.redox.2020.101467
Reza, M. M., Subramaniyam, N., Sim, C. M., Ge, X., Sathiakumar, D., McFarlane, C., et al. (2017). Irisin is a pro-myogenic factor that induces skeletal muscle hypertrophy and rescues denervation-induced atrophy. Nat. Commun. 8 (1), 1104. doi:10.1038/s41467-017-01131-0
Ross, J. A., Levy, Y., Svensson, K., Philp, A., Schenk, S., and Ochala, J. (2018). SIRT1 regulates nuclear number and domain size in skeletal muscle fibers. J. Cell Physiol. 233 (9), 7157–7163. doi:10.1002/jcp.26542
Ryan, A. S., and Li, G. (2023). Sex differences in muscle SIRT1 and SIRT3 and exercise + weight loss effects on muscle sirtuins. Exp. Biol. Med. (Maywood) 248 (4), 302–308. doi:10.1177/15353702221142619
Safiri, S., Kolahi, A. A., Smith, E., Hill, C., Bettampadi, D., Mansournia, M. A., et al. (2020). Global, regional and national burden of osteoarthritis 1990-2017: a systematic analysis of the Global Burden of Disease Study 2017. Ann. Rheum. Dis. 79 (6), 819–828. doi:10.1136/annrheumdis-2019-216515
Samsonraj, R. M., Law, S. F., Chandra, A., and Pignolo, R. J. (2023). An unbiased proteomics approach to identify the senescence-associated secretory phenotype of human bone marrow-derived mesenchymal stem cells. Bone Rep. 18, 101674. doi:10.1016/j.bonr.2023.101674
Shi, Z., Wang, L., Luan, J., Yin, L., Ji, X., Zhang, W., et al. (2022). Exercise promotes bone marrow Microenvironment by inhibiting Adipsin in diet-induced Male Obese mice. Nutrients 15 (1), 19. doi:10.3390/nu15010019
Sierra-Ramirez, J. A., Saucedo-Bueno, L., Garcia-Hernandez, A. L., Martinez-Davalos, A., Rodriguez-Lopez, C., Drago-Serrano, M. E., et al. (2022). Moderate aerobic exercise on bone quality changes associated with aging and oxidative stress in BALB/c mice. J. Biomech. 135, 111035. doi:10.1016/j.jbiomech.2022.111035
Song, J., Li, J., Yang, F., Ning, G., Zhen, L., Wu, L., et al. (2019). Nicotinamide mononucleotide promotes osteogenesis and reduces adipogenesis by regulating mesenchymal stromal cells via the SIRT1 pathway in aged bone marrow. Cell Death Dis. 10 (5), 336. doi:10.1038/s41419-019-1569-2
Su, Y., Chen, Z., and Xie, W. (2020). Swimming as treatment for osteoporosis: a systematic review and meta-analysis. Biomed. Res. Int. 2020, 6210201. doi:10.1155/2020/6210201
Tenorio, B., Jimenez, T., Barrera, G., Hirsch, S., De la Maza, M. P., Troncoso, R., et al. (2017). Irisin is weakly associated with usual physical activity in young overweight women. Nutr. Hosp. 34 (3), 688–692. doi:10.20960/nh.463
Trajanoska, K., Rivadeneira, F., Kiel, D. P., and Karasik, D. (2019). Genetics of bone and muscle interactions in Humans. Curr. Osteoporos. Rep. 17 (2), 86–95. doi:10.1007/s11914-019-00505-1
Tseng, P. C., Hou, S. M., Chen, R. J., Peng, H. W., Hsieh, C. F., Kuo, M. L., et al. (2011). Resveratrol promotes osteogenesis of human mesenchymal stem cells by upregulating RUNX2 gene expression via the SIRT1/FOXO3A axis. J. Bone Min. Res. 26 (10), 2552–2563. doi:10.1002/jbmr.460
Tsugawa, N., and Shiraki, M. (2020). Vitamin K Nutrition and bone health. Nutrients 12 (7), 1909. doi:10.3390/nu12071909
Vargas-Ortiz, K., Perez-Vazquez, V., and Macias-Cervantes, M. H. (2019). Exercise and sirtuins: a Way to mitochondrial health in skeletal muscle. Int. J. Mol. Sci. 20 (11), 2717. doi:10.3390/ijms20112717
Wang, H., Hu, Z., Wu, J., Mei, Y., Zhang, Q., Zhang, H., et al. (2019). Sirt1 promotes osteogenic differentiation and increases Alveolar bone mass via Bmi1 activation in mice. J. Bone Min. Res. 34 (6), 1169–1181. doi:10.1002/jbmr.3677
Wang, J., Zhang, Y., Cao, J., Wang, Y., Anwar, N., Zhang, Z., et al. (2023). The role of autophagy in bone metabolism and clinical significance. Autophagy 19 (9), 2409–2427. doi:10.1080/15548627.2023.2186112
Wang, T., Liu, X., and He, C. (2020). Glucocorticoid-induced autophagy and apoptosis in bone. Apoptosis 25 (3-4), 157–168. doi:10.1007/s10495-020-01599-0
Wang, Y., Mei, R., Hao, S., Luo, P., Wang, P., Almatari, Y., et al. (2021). Up-regulation of SIRT1 induced by 17beta-estradiol promotes autophagy and inhibits apoptosis in osteoblasts. Aging (Albany NY) 13 (20), 23652–23671. doi:10.18632/aging.203639
Watson, S. L., Weeks, B. K., Weis, L. J., Harding, A. T., Horan, S. A., and Beck, B. R. (2018). High-intensity resistance and impact training improves bone mineral density and physical function in postmenopausal women with osteopenia and osteoporosis: the LIFTMOR Randomized controlled trial. J. Bone Min. Res. 33 (2), 211–220. doi:10.1002/jbmr.3284
Wen, J., Bao, M., Tang, M., He, X., Yao, X., and Li, L. (2021). Low magnitude vibration alleviates age-related bone loss by inhibiting cell senescence of osteogenic cells in naturally senescent rats. Aging (Albany NY) 13 (8), 12031–12045. doi:10.18632/aging.202907
White, A. T., and Schenk, S. (2012). NAD(+)/NADH and skeletal muscle mitochondrial adaptations to exercise. Am. J. Physiol. Endocrinol. Metab. 303 (3), E308–E321. doi:10.1152/ajpendo.00054.2012
Williams, C. B., and Gurd, B. J. (2012). Skeletal muscle SIRT1 and the genetics of metabolic health: therapeutic activation by pharmaceuticals and exercise. Appl. Clin. Genet. 5, 81–91. doi:10.2147/TACG.S31276
Wu, W., Yuan, S., Tang, Y., Meng, X., Peng, M., Hu, Z., et al. (2023). Effect of exercise and oral Niacinamide mononucleotide on improving mitochondrial autophagy in Alzheimer's disease. Nutrients 15 (13), 2851. doi:10.3390/nu15132851
Yang, J., Kitami, M., Pan, H., Nakamura, M. T., Zhang, H., Liu, F., et al. (2021). Augmented BMP signaling commits cranial neural crest cells to a chondrogenic fate by suppressing autophagic beta-catenin degradation. Sci. Signal 14 (665), eaaz9368. doi:10.1126/scisignal.aaz9368
Yang, X., Jiang, T., Wang, Y., and Guo, L. (2019). The role and mechanism of SIRT1 in Resveratrol-regulated osteoblast autophagy in osteoporosis rats. Sci. Rep. 9 (1), 18424. doi:10.1038/s41598-019-44766-3
Yao, Z., Getting, S. J., and Locke, I. C. (2021). Regulation of TNF-induced osteoclast differentiation. Cells 11 (1), 132. doi:10.3390/cells11010132
Ye, Q., Xu, H., Liu, S., Li, Z., Zhou, J., Ding, F., et al. (2022). Apoptotic extracellular vesicles alleviate Pg-LPS induced inflammatory responses of macrophages via AMPK/SIRT1/NF-κB pathway and inhibit osteoclast formation. J. Periodontol. 93 (11), 1738–1751. doi:10.1002/JPER.21-0657
Yuan, H. F., Zhai, C., Yan, X. L., Zhao, D. D., Wang, J. X., Zeng, Q., et al. (2012). SIRT1 is required for long-term growth of human mesenchymal stem cells. J. Mol. Med. Berl. 90 (4), 389–400. doi:10.1007/s00109-011-0825-4
Yuan, W., and Song, C. (2022). Crosstalk between bone and other organs. Med. Rev. 2 (4), 331–348. doi:10.1515/mr-2022-0018
Yuan, Y., Chen, X., Zhang, L., Wu, J., Guo, J., Zou, D., et al. (2016). The roles of exercise in bone remodeling and in prevention and treatment of osteoporosis. Prog. Biophys. Mol. Biol. 122 (2), 122–130. doi:10.1016/j.pbiomolbio.2015.11.005
Zainabadi, K., Liu, C. J., Caldwell, A. L. M., and Guarente, L. (2017). SIRT1 is a positive regulator of in vivo bone mass and a therapeutic target for osteoporosis. PLoS One 12 (9), e0185236. doi:10.1371/journal.pone.0185236
Zhang, J., Huang, X., Yu, R., Wang, Y., and Gao, C. (2020). Circulating irisin is linked to bone mineral density in geriatric Chinese men. Open Med. (Wars) 15 (1), 763–768. doi:10.1515/med-2020-0215
Zhang, J., Lazarenko, O. P., Blackburn, M. L., Shankar, K., Badger, T. M., Ronis, M. J., et al. (2011). Feeding blueberry diets in early life prevent senescence of osteoblasts and bone loss in ovariectomized adult female rats. PLoS One 6 (9), e24486. doi:10.1371/journal.pone.0024486
Zhang, L., Zheng, j., Lazarenko, O. P., Blackburn, M. L., Badger, T. M., Ronis, M. J., and Chen, J. R. (2022). Blueberry consumption prevents loss of collagen in bone matrix and inhibits senescence pathways in osteoblastic cells. Age (Dordr) 35(3), 807–20. doi:10.1007/s11357-012-9412-z
Zhang, L., Zheng, Y. L., Wang, R., Wang, X. Q., and Zhang, H. (2022). Exercise for osteoporosis: a literature review of pathology and mechanism. Front. Immunol. 13, 1005665. doi:10.3389/fimmu.2022.1005665
Zhao, Z., Yan, K., Guan, Q., Guo, Q., and Zhao, C. (2023). Mechanism and physical activities in bone-skeletal muscle crosstalk. Front. Endocrinol. (Lausanne) 14, 1287972. doi:10.3389/fendo.2023.1287972
Zhou, D., Ran, Y., Yu, R., Liu, G., Ran, D., Liu, Z., et al. (2023). SIRT1 regulates osteoblast senescence through SOD2 acetylation and mitochondrial dysfunction in the progression of Osteoporosis caused by Cadmium exposure. Chem. Biol. Interact 382, 110632. doi:10.1016/j.cbi.2023.110632
Zhu, C., Ding, H., Shi, L., Zhang, S., Tong, X., Huang, M., et al. (2023). Exercise improved bone health in aging mice: a role of SIRT1 in regulating autophagy and osteogenic differentiation of BMSCs. Front. Endocrinol. (Lausanne) 14, 1156637. doi:10.3389/fendo.2023.1156637
Keywords: SIRT1, exercise, bone health, osteoporosis, osteoblast, osteoclast
Citation: Liu L, Zhang J, Cui R, Wang N, Zhang Y, Liu L, Zhang X and Liu Q (2025) SIRT1 and exercise-induced bone metabolism: a regulatory nexus. Front. Cell Dev. Biol. 13:1522821. doi: 10.3389/fcell.2025.1522821
Received: 05 November 2024; Accepted: 04 March 2025;
Published: 26 March 2025.
Edited by:
Lidija Milkovic, Rudjer Boskovic Institute, CroatiaReviewed by:
Zhi Xia, Wenzhou University, ChinaCopyright © 2025 Liu, Zhang, Cui, Wang, Zhang, Liu, Zhang and Liu. This is an open-access article distributed under the terms of the Creative Commons Attribution License (CC BY). The use, distribution or reproduction in other forums is permitted, provided the original author(s) and the copyright owner(s) are credited and that the original publication in this journal is cited, in accordance with accepted academic practice. No use, distribution or reproduction is permitted which does not comply with these terms.
*Correspondence: Xinan Zhang, emhhbmd4YTI3MjVAMTYzLmNvbQ==; Qingfeng Liu, ZHJsaXVxZjE5NzBAMTI2LmNvbQ==
†These authors have contributed equally to this work and share first authorship
Disclaimer: All claims expressed in this article are solely those of the authors and do not necessarily represent those of their affiliated organizations, or those of the publisher, the editors and the reviewers. Any product that may be evaluated in this article or claim that may be made by its manufacturer is not guaranteed or endorsed by the publisher.
Research integrity at Frontiers
Learn more about the work of our research integrity team to safeguard the quality of each article we publish.