- 1Emergency Department, The Second Affiliated Hospital of Jiaxing University, Jiaxing, Zhejiang, China
- 2Department of Surgery, The Second Affiliated Hospital of Jiaxing University, Jiaxing, Zhejiang, China
- 3Department of general practice, Taozhuang Branch of the First People’s Hospital of Jiashan, Jiaxing, Zhejiang, China
Pancreatic cancer (PC) remains one of the most lethal malignancies, primarily due to its intrinsic resistance to conventional therapies. MicroRNAs (miRNAs), key regulators of gene expression, have been identified as crucial modulators of drug resistance mechanisms in this cancer type. This review synthesizes recent advancements in our understanding of how miRNAs influence treatment efficacy in PC. We have thoroughly summarized and discussed the complex role of miRNA in mediating drug resistance in PC treatment. By highlighting specific miRNAs that are implicated in drug resistance pathways, we provide insights into their functional mechanisms and interactions with key molecular targets. We also explore the potential of miRNA-based strategies as novel therapeutic approaches and diagnostic tools to overcome resistance and improve patient outcomes. Despite promising developments, challenges such as specificity, stability, and effective delivery of miRNA-based therapeutics remain. This review aims to offer a critical perspective on current research and propose future directions for leveraging miRNA-based interventions in the fight against PC.
1 Introduction
PC, the most common type being pancreatic adenocarcinoma (PDAC), remains one of the deadliest malignancies, characterized by its aggressive nature, late-stage diagnosis, and resistance to conventional therapies (Cai et al., 2021; Farhangnia et al., 2024; Furukawa, 2009; Neoptolemos et al., 2018; Park et al., 2021; Qin et al., 2020). Despite advances in surgical techniques, radiation, and systemic treatments, including chemotherapy and targeted therapies, the overall survival (OS) rate for PDAC patients remains dismally low, with a 5-year survival rate of less than 10% (Chen K. et al., 2021; Shaib et al., 2006). For the small proportion of patients (approximately 15%–20%) diagnosed at a resectable stage, surgical resection remains the primary treatment modality, offering the best chance for long-term survival (Siegel et al., 2021). However, even after surgery, recurrence rates are high, necessitating the use of adjuvant therapies such as gemcitabinewith with nab-paclitaxel or FOLFIRINOX to improve survival outcomes (Evans et al., 2024). For patients with borderline resectable or locally advanced disease, neoadjuvant chemoradiotherapy is often employed to shrink tumors and potentially enable resection (Eshmuminov et al., 2023; Ghaneh et al., 2023). In contrast, the majority of PDAC cases are diagnosed at an unresectable or metastatic stage, where systemic chemotherapy becomes the mainstay of treatment (Park et al., 2021). Gemcitabine with nab-paclitaxel or FOLFIRINOX are commonly used regimens in the treatment of PDAC. The latter is a combination chemotherapy regimen that includes four drugs: 5-fluorouracil (5-FU), leucovorin, oxaliplatin, and irinotecan. These drugs have different mechanisms of action and work synergistically to enhance treatment efficacy. For example, 5-FU is a pyrimidine analog that interferes with DNA synthesis by inhibiting thymidylate synthase, leading to cell death. However, the overall effectiveness of these therapies is often hampered by significant toxicity and the rapid development of resistance (Shirakawa et al., 2023). Radiotherapy is occasionally employed for local control, but its utility is similarly limited by resistance (Ben-Josef and Lawrence, 2011). The high prevalence of chemoresistance and radioresistance in PDAC underscores the urgent need for novel therapeutic strategies to improve patient outcomes and overcome these barriers (Bear et al., 2020; Tiriac et al., 2018).
Recent research has highlighted the pivotal role of miRNAs, small non-coding RNAs that regulate gene expression at the post-transcriptional level, in mediating drug resistance. miRNAs have emerged as key regulators of various cellular processes, including proliferation, apoptosis, and metastasis, all of which are crucial in the context of cancer therapy (Saliminejad et al., 2019; Sun et al., 2010; Wilczynska and Bushell, 2015). In addition, miRNAs play a crucial role in the regulation of gene expression by acting as post-transcriptional modulators (Lou et al., 2018). In PDAC, miRNA expression is often dysregulated, with some miRNAs being upregulated and others downregulated, contributing to tumorigenesis and disease progression (Madadjim et al., 2024). Dysregulation can result from genetic and epigenetic alterations, such as mutations, DNA methylation, or histone modifications, as well as abnormal transcription factor activity (Baradaran et al., 2019; Pan G. et al., 2021; Singh et al., 2012; Wang et al., 2021). For example, oncogenic miRNAs (oncomiRs) are frequently upregulated in PDAC, promoting tumor growth and chemoresistance by targeting tumor suppressor genes (Fathi et al., 2021). Conversely, tumor-suppressive miRNAs are often downregulated, leading to unchecked activation of oncogenic pathways (Fathi et al., 2021). In PC, abnormal miRNA expression patterns are also related to the regulation of drug resistance mechanisms, affecting the efficacy of chemotherapy drugs and radiotherapy. Previous studies, including a review by Radu et al., have highlighted the potential of circulating miRNAs as non-invasive biomarkers in digestive tract tumors, including PDAC (Albulescu et al., 2011). These miRANs, which are unregulated during tumorigenesis and progression, can reflect the molecular state of tumors and provide insights into drug resistance mechanisms. Targeting specific miRNAs, either to restore tumor suppressor miRNAs or to inhibit carcinogenic miRNAs, can serve as novel therapeutic strategies to overcome PDAC resistance and improve therapeutic outcomes.
Understanding the interplay between miRNAs and drug resistance pathways is essential for developing novel therapeutic strategies and improving treatment outcomes. miRNAs can affect drug resistance through multiple mechanisms, such as the regulation of drug metabolism, modulation of drug targets, and alteration of cellular stress responses (Abo-Al-Ela and Faggio, 2021; Du et al., 2019; Gupta et al., 2021; Khan et al., 2023; Lu and Rothenberg, 2018; Zhang et al., 2019). Moreover, their involvement in the epithelial-to-mesenchymal transition (EMT) (Pan et al., 2021b; Uhan and Hauptman, 2021), a process linked to increased drug resistance, further underscores their relevance in PDAC therapy.
In this review, we aim to provide a comprehensive analysis of the current knowledge regarding the role of miRNAs in PDAC drug resistance. We will explore the mechanistic insights into how specific miRNAs contribute to resistance against various therapeutic modalities, including chemotherapy, targeted therapies, and immunotherapy. Additionally, we will discuss the potential of miRNAs as biomarkers for predicting treatment response and as therapeutic targets to overcome drug resistance. By synthesizing recent findings, this review seeks to enhance our understanding of miRNA-mediated drug resistance and to propose future research directions that could lead to more effective treatment strategies for PDAC.
2 The role of miRNAs in PDAC
miRNAs are small non-coding RNAs approximately 22 nucleotides in length that regulate gene expression post-transcriptionally by binding to the 3′untranslated regions (3′UTR) of target mRNAs (Ambros, 2004; Kim and Nam, 2006). In the canonical pathway, the biogenesis of miRNAs involves the transcription of primary miRNA, which is then processed by the Drosha enzyme into precursor miRNA, followed by further cleavage into mature miRNAs by the Dicer enzyme in the cytoplasm (Alarcon et al., 2015; Fellmann et al., 2013; Ha and Kim, 2014; Meister, 2023; Son et al., 2023; Zapletal et al., 2022). Mature miRNAs associate with the RNA-induced silencing complex to bind and regulate target mRNA expression, influencing various cellular processes such as proliferation, apoptosis, and stress responses (Gregory et al., 2005; Iwakawa and Tomari, 2022; Jiang et al., 2019; Lynam-Lennon et al., 2009; Marsit et al., 2006). In the non-canonical pathway, the processing of pri-miRNA is not entirely dependent on Drosha. Different enzymes may be involved in the processing, such as Dicer directly processing incompletely cleaved pri-miRNA or generating pre-miRNA through other enzyme systems, including Argonaute proteins (Ha and Kim, 2014). Figure 1 illustrates the biogenesis of miRNAs.
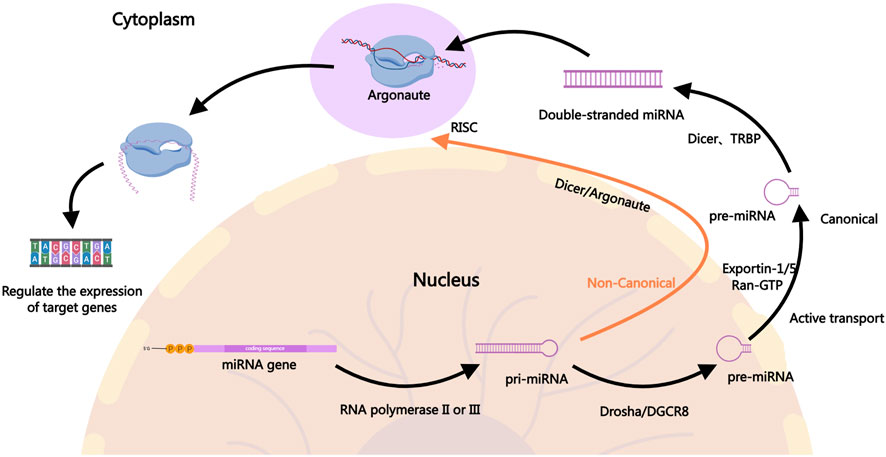
Figure 1. The Biogenesis of miRNAs. In the canonical pathway, miRNA biogenesis begins in the nucleus where pri-miRNAs are transcribed by RNA polymerase II. The pri-miRNAs are then cleaved by the Drosha-DGCR8 complex to form precursor pre-miRNAs, which are exported to the cytoplasm via Exportin-1, Exportin-5, and others. In the cytoplasm, the pre-miRNAs are further processed by Dicer into mature miRNA duplexes. One strand of the duplex, the guide strand, is incorporated into the RISC, where it guides the complex to target mRNAs for silencing or degradation, ultimately regulating gene expression. In the non-canonical pathway, the generation of pri-miRNA is similar to the canonical pathway, but the processing of pri-miRNA into pre-miRNA does not fully depend on Drosha. In the non-canonical pathway, Dicer may directly process incompletely cleaved pri-miRNA or generate pre-miRNA through other enzyme systems, such as Argonaute proteins. In some cases, miRNAs from the non-canonical pathway may be directly cleaved and processed by mediators like Ago2 (a form of Argonaute protein). The mature miRNAs generated through the non-canonical pathway typically enter the RISC in the cytoplasm and participate in gene expression regulation. They may inhibit the translation of target genes or promote their degradation by binding to the 3′UTR of target mRNA.
In PDAC, the expression patterns of miRNAs are significantly altered. These aberrant miRNA expressions are closely linked to key tumor characteristics, including tumor proliferation, metastasis, and drug resistance (Fang et al., 2018; Gu et al., 2022; Liu et al., 2021). For instance, miR-21, miR-155, and miR-210 are highly expressed in PDAC cells, where they promote tumor growth and metastasis by regulating cell cycle-related proteins and anti-apoptotic factors (Abue et al., 2015; Ho et al., 2010; Pang et al., 2015; Sicard et al., 2013). Conversely, the downregulation of miR-34a and miR-143 is associated with increased tumor aggressiveness and poor prognosis in PDAC (Xie et al., 2019; Xin et al., 2019).
miRNAs also play a critical role in the development of drug resistance in PDAC. Drug resistance is a major cause of treatment failure in PDAC and involves complex molecular mechanisms. miRNAs influence drug efficacy through mechanisms such as regulating drug metabolism, modulating drug target expression, and altering cellular stress responses (Pan et al., 2021c). For example, miR-21 enhances resistance to chemotherapeutic agents by inhibiting tumor suppressor genes such as PTEN and RECK (Giovannetti et al., 2010; Park et al., 2009). Additionally, miR-155 and miR-200 are involved in drug resistance by regulating EMT processes and cell cycle-related proteins, affecting drug sensitivity (Li et al., 2009; Patel et al., 2017).
Given the pivotal role of miRNAs in PDAC, researchers have begun to explore their potential as therapeutic targets and biomarkers. Specific modulation of miRNA expression may help overcome drug resistance and improve therapeutic outcomes. Furthermore, miRNA expression profiles could serve as diagnostic and prognostic biomarkers for PDAC, aiding in the development of personalized treatment strategies (Daoud et al., 2019; Ge et al., 2022). In summary, miRNAs play multifaceted roles in the pathogenesis, progression, and drug resistance of PDAC. A deeper understanding of miRNA functions provides crucial insights into the biological characteristics of PDAC and offers important clues for developing novel therapeutic strategies.
3 miRNAs in PDAC resistance
miRNAs have emerged as key regulators in the development of resistance to both chemotherapy and radiotherapy in PDAC. Due to their ability to post-transcriptionally regulate multiple genes involved in cell survival, apoptosis, and DNA repair, miRNAs significantly impact the effectiveness of these conventional treatments (Figure 2). Chemotherapy resistance, which limits the success of agents like gemcitabine (GEM), and radiotherapy resistance, which reduces the efficacy of radiation-induced cancer cell death, are both influenced by miRNA-mediated pathways. Understanding these mechanisms is essential for addressing treatment challenges and developing more effective therapeutic strategies to overcome resistance in PDAC.
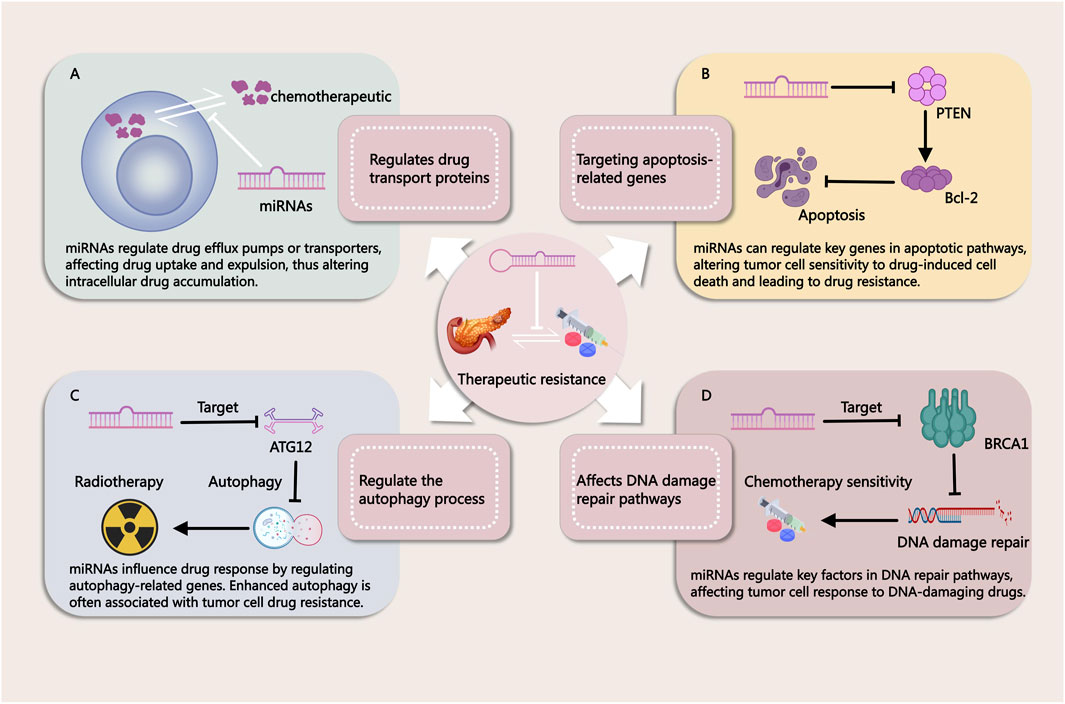
Figure 2. Mechanisms by Which miRNAs Influence Tumor Treatment Resistance. (A) miRNAs regulate drug efflux pumps or transport proteins, affecting drug uptake and efflux in cancer cells. This modulation alters intracellular drug accumulation, contributing to treatment resistance. (B) miRNAs modulate key genes in apoptotic pathways, thereby influencing the sensitivity of tumor cells to drug-induced cell death. Changes in apoptosis regulation can lead to resistance against chemotherapy. (C) miRNAs regulate autophagy-related genes, impacting cellular responses to therapy. Enhanced autophagy is often associated with drug resistance in tumor cells. (D) miRNAs influence DNA repair pathways by targeting critical factors involved in DNA damage response. This modulation alters the effectiveness of DNA-damaging agents, contributing to therapy resistance.
3.1 miRNAs in chemotherapy resistance
Chemotherapy for PDAC primarily relies on drugs such as GEM, 5-FU, and cisplatin, with the aim of shrinking tumors and controlling the disease (Okusaka and Furuse, 2020; Rehman et al., 2022). However, chemoresistance remains a major challenge in treatment, often arising from drug efflux mechanisms, enhanced DNA repair capabilities, suppression of apoptotic pathways, and the complexity of the tumor microenvironment (TME) (Perkhofer et al., 2021; Qian et al., 2019).
Several studies have evaluated the relationship between miR-21 expression and OS in patients with PDAC undergoing GEM treatment. High miR-21 expression has been significantly associated with shorter OS and linked to increased aggressiveness and GEM resistance in PDAC. In both cell and tissue samples, overexpression of miR-21 reduced the antiproliferative effects of GEM and induced apoptosis, while upregulating matrix metalloproteinase (MMP)-2/MMP-9 and vascular endothelial growth factor (VEGF) expression. Inhibition of phosphorylated Akt by phosphoinositide 3-kinase (PI3K) inhibitors and rapamycin blocked pre-miR-21-induced GEM resistance. These findings suggest that miR-21 mediates GEM resistance by regulating apoptosis, Akt phosphorylation, and genes related to invasive behavior, providing mechanistic insights for developing new targeted therapies (Giovannetti et al., 2010). Additionally, other studies have shown that miR-21 confers resistance to 5-FU in PDAC cells by suppressing tumor suppressor genes PTEN and PDCD4. In resistant PDAC cells, miR-21 expression is significantly upregulated, and its inhibition reduces 5-FU resistance. Conversely, overexpression of miR-21 promotes PDAC cell proliferation, migration, and invasion. Restoration of PTEN and PDCD4 expression reverses miR-21-induced resistance and cellular migration, suggesting that miR-21 is a key regulator of 5-FU resistance in PDAC (Wei et al., 2016). Furthermore, miR-21 overexpression has been significantly correlated with shorter disease-free survival (DFS) in patients undergoing GEM adjuvant therapy following radical surgery (Morinaga et al., 2016). Therefore, miR-21 may serve as an important biomarker for predicting GEM resistance in PDAC patients.
In another study, miR-210 was found to be significantly downregulated in GEM-resistant PDAC cells, while its overexpression enhanced GEM sensitivity and exhibited cytotoxic effects on resistant cells. Mechanistically, miR-210 induces caspase-3-mediated apoptosis and inhibits cell proliferation and metastasis by suppressing the expression of ABCC5. A negative correlation between miR-210 and ABCC5 expression was observed in PDAC cell lines and patient tissues, indicating that miR-210 plays a key role in GEM resistance by regulating ABCC5, making it a potential therapeutic target in PDAC. In vivo experiments, microRNA-210 transfection almost completely inhibited the growth, proliferation and metastasis of tumor xenografts, and had no obvious side effects on mice (Amponsah et al., 2017). However, another study demonstrated that exosomes derived from GEM-resistant BxR-CSCs promote resistance in GEM-sensitive BxS and PANC-1 PDAC cells by inhibiting GEM-induced cell cycle arrest and apoptosis, and by enhancing cell migration and tubule formation. miR-210 was significantly upregulated in exosomes from BxR-CSCs, and its expression in GEM-treated BxS and PANC-1 cells increased in a dose-dependent manner. Transfection with miR-210 mimics led to biological changes similar to those induced by exosomes, including activation of the mTOR signaling pathway. These findings suggest that exosomes from GEM-resistant PDAC stem cells mediate resistance through miR-210, affecting GEM-sensitive cells’ resistance properties (Yang et al., 2020). Moreover, research has shown that DLEU2L acts as a competing endogenous RNA (ceRNA) for miR-210-3p in GEM resistance in PDAC. DLEU2L is downregulated in PDAC tissues and binds to miR-210-3p. Overexpression of DLEU2L and silencing of miR-210-3p suppress PDAC cell proliferation, migration, and invasion, while promoting apoptosis. These effects are achieved by inhibiting the Warburg effect (aerobic glycolysis) and the AKT/mTOR signaling pathway. Additionally, BRCA2 was identified as a target gene of miR-210-3p, and DLEU2L upregulated BRCA2 through the ceRNA mechanism. Overexpression of DLEU2L and miR-210-3p interference effectively inhibited pancreatic tumor progression, indicating that DLEU2L plays a crucial role in miR-210-3p-mediated GEM resistance. In xenograft models, circ_0013587 overexpression sensitized erlotinib-resistant AsPC-1 cells to erlotinib (Xu F. et al., 2021). Additionally, miRNAs such as let-7b and miR-374b may play significant roles in cisplatin resistance. For example, overexpression of miR-374b can restore cisplatin sensitivity in the cisplatin-resistant BxPC3-R cells (Schreiber et al., 2016; Shao et al., 2015).
Dicer, a crucial enzyme in miRNA biogenesis, plays a vital role in miRNA maturation. Studies have shown that compared to parental PANC-1 cells, Dicer expression is significantly upregulated in GEM-resistant PANC-1/GEM cells, and elevated Dicer levels are associated with an increased risk of PDAC. Inhibition of Dicer significantly reduces GEM resistance in PANC-1/GEM cells, whereas its overexpression enhances resistance. Further research has revealed that the transcription factor Sp1 targets the promoter region of Dicer, and the ERK/Sp1 signaling pathway regulates Dicer expression in PANC-1/GEM cells, correlating with PDAC progression. PANC-1/GEM cells (GEM/shDicer) with stable Dicer knockdown were injected subcutaneously into NOD/SCID mice treated with gemcitabine 3 weeks later. The PANC-1/GEM mice had larger tumors and were more resistant to gemcitabine than the PANC-1 mice. Knocking down Dicer in PANC-1/GEM cells can shorten the cell cycle and inhibit the growth of pancreatic cancer. This suggests that targeting the ERK/Sp1/Dicer pathway could provide a novel therapeutic strategy to overcome GEM resistance in PDAC (Su et al., 2021).
Further studies on miRNA-mediated chemotherapy resistance in PDAC are summarized in Table 1 for a more comprehensive overview.
3.2 miRNAs in radiotherapy resistance
miRNAs play a crucial role in regulating PDAC’s sensitivity to radiation therapy. They can modulate various cellular processes, including cell cycle progression, DNA repair, and apoptosis, which are essential for determining the effectiveness of radiation treatment. Specific miRNAs have been identified as key regulators of the radiation response in PDAC cells. Understanding the roles of these miRNAs can provide valuable insights into improving the efficacy of radiation therapy and overcoming resistance in PDAC treatment.
Research has shown that miR-26a is transiently upregulated in PDAC cells following radiation therapy, before returning to normal levels. Although miR-26a is generally classified as a tumor-suppressive miRNA, its transient upregulation significantly promotes radiation resistance, while stable overexpression inhibits it. The transient upregulation of miR-26a enhances radiation resistance by promoting cell cycle arrest and DNA damage repair. miR-26a directly targets the oncogene HMGA2, increasing radiosensitivity, and may also affect PTGS2 by delaying PGE2 synthesis, thereby promoting tumor regeneration (Jiang et al., 2024). Additionally, study has found that miR-23b plays a critical role in pancreatic cancer radiotherapy by regulating the autophagic process. Studies have shown that overexpression of miR-23b inhibits autophagy, enhancing radiosensitivity, whereas miR-23b inhibition promotes autophagy and increases radioresistance. miR-23b exerts its effect by suppressing the expression of the autophagy-related gene ATG12, thereby reducing autophagic flux and influencing the cancer cells’ response to radiotherapy. Therefore, evaluating miR-23b expression levels could serve as a predictive biomarker for radiosensitivity. Autophagy inhibitors, such as chloroquine, can be used in combination to enhance radiosensitivity. Furthermore, delivering miR-23b mimics or inhibitors to modulate autophagy may provide a novel therapeutic approach to improve the efficacy of radiotherapy (Wang et al., 2013a). Ionizing radiation can activate mTOR in PDAC cells by reducing miR-99b expression. miR-99b negatively regulates mTOR expression. The use of the mTOR inhibitor AZD8055 (10 nM, 100 nM, 500 nM) significantly enhances the inhibitory and apoptotic effects of radiation on PDAC cells. In a human PDAC xenograft model, fractionated radiation combined with AZD8055 treatment significantly improved antitumor effects compared to radiation or AZD8055 treatment alone, resulting in a notable reduction in tumor volume. These results suggest that combining AZD8055 with radiation can effectively overcome radiation resistance in PDAC (Wei et al., 2013). Moreover, miR-153 enhances radiation sensitivity by directly targeting the jagged-1 (JAG1) Notch ligand. Adding recombinant JAG1 protein can reverse the therapeutic effects of miR-153 (Zhao et al., 2021).
Above evidence demonstrates that miRNAs play a pivotal role in regulating radiation therapy resistance in PDAC. They influence critical processes such as cell cycle progression, DNA repair, and apoptosis, highlighting their potential as targets to enhance treatment outcomes and address resistance.
3.3 The role of the TME in therapeutic resistance in PDAC and the involvement of miRNAs
The TME plays a pivotal role in modulating the therapeutic response in PDAC (Sherman and Beatty, 2023). The TME consists of a complex network of stromal cells, immune cells, extracellular matrix components, and signaling molecules that interact with tumor cells, thereby influencing tumor progression and resistance to treatment (Werba et al., 2023). In PDAC, the TME is often characterized by a dense stroma, consisting of cancer-associated fibroblasts (CAFs), myofibroblasts, and extracellular matrix proteins, which contribute to tumor progression and treatment resistance (Werba et al., 2023). Furthermore, the TME is rich in inflammatory cytokines, growth factors, and hypoxic regions, all of which create a hostile environment that protects the tumor from conventional therapies such as chemotherapy, radiotherapy, and immunotherapy (Ho et al., 2020).
CAFs, which are a major component of the PDAC stroma, promote tumor progression by secreting cytokines, growth factors, and extracellular matrix components that not only facilitate tumor cell survival but also contribute to drug resistance (Yamashita and Kumamoto, 2024). They create a physical barrier that limits drug delivery and reduce the efficacy of chemotherapies (Ferrara et al., 2021). Additionally, CAFs secrete exosomes that carry signaling molecules, including miRNAs, which can influence the behavior of neighboring cells within the TME, such as immune cells and tumor cells (Qi et al., 2023).
Qi et al. have shown that CAFs-derived exosomes in PDAC do not inherently promote GEM resistance under normal conditions. However, after exposure to GEM, CAFs may undergo changes that enhance their ability to contribute to GEM resistance. After GEM treatment, CAFs promote chemotherapy resistance of PDAC cells by secreting exosomes and maintaining signal communication with cancer cells. Mechanistically, miR-3173-5p from CAF exosomes sponges ACSL4 and inhibits ferroptosis after uptake by cancer cells (Qi et al., 2023). In addition, other study has shown that GEM can upregulate the expression of Snail and miR-146a in PDAC CAFs exosomes, thereby increasing the proliferation and survival of epithelial cells, and Snail and miR-146a can be delivered to cells through CAF exosomes (Richards et al., 2017).CAF-derived exosomes can also regulate the levels and functions of miR-125b-5p, PTEN-targeting miRNAs (including miR-21, miR-181a, miR-221, miR-222, and miR-92a), and miR-224-3p, thereby promoting the progression and drug resistance of PDAC (Guo et al., 2023a; Richards et al., 2022; Zhang et al., 2024). However, there is no evidence that these CAF-controlled miRNAs can regulate the activity of immune cells in PDAC.
The immune cells within the TME, including tumor-associated macrophages (TAMs), also play significant roles in mediating therapeutic resistance (Khalaf et al., 2021). TAMs, which are often polarized into the pro-tumorigenic M2 phenotype, promote tumor growth, angiogenesis, and immune suppression (Pan et al., 2020). miRNAs have been shown to regulate TAM polarization and activity, thereby influencing the immune landscape within the tumor. For instance, a study has shown that TAMs contribute to gemcitabine resistance in PDAC through the secretion of exosomes containing miR-365. These exosomes transfer miR-365 to cancer cells, leading to gemcitabine inactivation by upregulating the triphospho-nucleotide pool and inducing cytidine deaminase. Blocking miR-365 or inhibiting exosome secretion enhances gemcitabine sensitivity, highlighting TAM-derived miR-365 as a key regulator of drug resistance and a potential therapeutic target (Binenbaum et al., 2018).
Moreover, hypoxia, a common feature of the PDAC microenvironment, has been linked to the upregulation of specific miRNAs that contribute to tumor progression and drug resistance (Chen et al., 2024). Under hypoxic conditions, HIF-1α influences the expression of MKLN1-AS by directly binding to anoxic response elements in the MKLN1-AS promoter region. By binding to miR-185-5p as a competitive endogenous RNA, MKLN1-AS regulates the expression of TEAD1 and promotes cell proliferation, migration and tumor growth. TEAD1 then facilitated the development of PDAC (Chen et al., 2024).
In addition to immune cells and stromal cells, extracellular matrix components, such as fibronectin and collagen, interact with integrins on tumor cells, facilitating survival and drug resistance (Jiang et al., 2022). miRNAs can regulate integrin expression, further influencing the ability of PDAC tumors to resist therapy. For example, it has been shown that MiR-760 enhances the sensitivity of pancreatic cancer cells to gemcitabine by regulating integrin β1 (Yang et al., 2019).
Above evidence demonstrates that the TME in PDAC plays a crucial role in shaping the response to therapy by promoting resistance through stromal cell signaling, immune modulation, and extracellular matrix remodeling. miRNAs are key players in regulating these processes, influencing the interactions between tumor cells and their microenvironment.
4 Targeting miRNAs to combat treatment resistance in PDAC
Current studies have shown that miRNAs have become key regulatory factors in the development of drug resistance in PDAC, with multiple pathways affecting tumor progression. Identifying specific miRNA targets provides a promising approach to overcoming drug resistance in PDAC. By targeting these miRNAs, it is possible to modulate key signaling pathways, enhance therapeutic sensitivity, and improve the overall effectiveness of current therapies.
Studies have shown that DNA methyltransferase DNMT1 suppresses miR-34a expression by hypermethylating the miR-34a promoter, thereby enhancing the activation of the Notch signaling pathway. In PDAC patients, DNMT1 expression is negatively correlated with miR-34a levels. Knockdown of DNMT1 reduces miR-34a promoter methylation, increases miR-34a expression, and inhibits Notch pathway activation. By modulating the DNMT1/miR-34a axis to downregulate the Notch pathway, PDAC cells exhibit significantly enhanced sensitivity to molecular targeted therapies. Hence, indirectly enhancing miR-34a expression by targeting DNMT may be a potential therapeutic target for pancreatic cancer (Ma et al., 2020).
Additionally, it has been discovered that PDAC cells contain a side population (SP) with stem cell-like properties, capable of inducing rapid and aggressive tumor formation in nude mice. The expression of miR-21 and miR-221 differs significantly between SP and non-side population (NSP) cells. Intervening in SP cells with antisense oligonucleotides (ASOs) against miR-21 and miR-221 significantly reduces the proportion of SP cells, impairs their differentiation and downstream gene regulation, and increases their sensitivity to chemotherapy agents GEM and 5-FU. Combined treatment with miR-21 and miR-221 ASOs is more effective than individual treatments, markedly inhibiting primary tumor growth and metastasis, especially in the L3.6 pl (Gres)-SP pancreatic tumor model. This suggests that targeting miR-21 and miR-221 may be a promising strategy for addressing stem cell-like subpopulations in PDAC (Zhao et al., 2015).
Moreover, miR-23B has been identified as an effective autophagy inhibitor. By targeting the 3′UTR of autophagy-related gene ATG12, it reduces autophagy activity, thereby enhancing radiation-induced cell death in PDAC cells. The use of miR-23B mimics or ATG12 inhibitors significantly enhanced the radiotherapy sensitivity of PDAC. Given that PDAC cells exhibit high basal autophagy levels, which may contribute to treatment resistance, miR-23B’s ability to inhibit autophagy suggests that it could improve the therapeutic response in PDAC, providing a potential avenue for optimizing treatment strategies (Wang et al., 2013b).
Furthermore, research has shown that the tumor suppressor p53 regulates the expression of GPR55 through miR-34b-3p. Inhibition of GPR55 decreases anchorage-dependent and -independent growth, cell cycle progression, MAPK pathway activation, and ribonucleotide reductase levels in PDAC cells. GPR55 knockdown also reduces tumor cell proliferation and MAPK pathway activity in KPC mice. In vivo, GPR55 gene deletion significantly extends the survival of KPC mouse models. Notably, combining the GPR55 antagonist cannabidiol (CBD) with the chemotherapeutic agent GEM extends the survival of KPC mice nearly threefold compared to GEM alone. These findings suggest that the combination of CBD and GEM may offer a novel therapeutic approach to improve the prognosis of PDAC patients (Ferro et al., 2018).
miR-30a-5p is downregulated in PDAC and is associated with poor prognosis. Upregulation of miR-30a-5p inhibits tumor cell proliferation, increases apoptosis, and significantly enhances sensitivity to GEM chemotherapy. In GEM-resistant PDAC cells, miR-30a-5p expression is markedly reduced. Further investigation identified FOXD1 as a direct target of miR-30a-5p, suggesting that the miR-30a-5p/FOXD1/ERK axis plays a crucial role in the development of GEM resistance in PDAC. Upregulation of its expression with miR-30a-5p mimics can enhance the sensitivity of PDAC to GEM, making it a potential therapeutic target to overcome GEM resistance (Zhou et al., 2019).
We summarize additional relevant targets in Table 2 for a more intuitive presentation. To clinically validate the therapeutic potential of miRNA targeting in pancreatic cancer, a promising approach could involve the use of in situ hybridization (ISH) analysis to detect specific miRNAs in patient tissues (Chu et al., 2019; Volpi et al., 2018). ISH offers a powerful method for visualizing miRNA expression patterns directly in the context of the tumor microenvironment, providing insights into how miRNAs are distributed and potentially linked to therapeutic resistance mechanisms (Chu et al., 2019; Volpi et al., 2018). Given the heterogeneity of pancreatic ductal adenocarcinoma (PDAC), ISH could be particularly valuable in identifying miRNA signatures that correlate with treatment responses or resistance in clinical samples. Moreover, it could help pinpoint miRNAs involved in tumor progression, metastasis, and the modulation of the tumor immune microenvironment, all of which are key factors in drug resistance. For example, previous studies have shown that locked nucleic acid-ISH analysis of miR-21 may be an important predictor of gemcitabine resistance in pancreatic cancer patients receiving adjuvant gemcitabine therapy after therapeutic resection (Morinaga et al., 2016).
5 Summary and future directions
In summary, miRNAs have emerged as pivotal regulators in the context of drug resistance in PDAC, a malignancy notorious for its poor prognosis and limited treatment options. Our review has comprehensively analyzed the role of miRNAs in modulating the effectiveness of conventional therapies, including GEM, 5-FU, oxaliplatin and radiotherapy, by influencing key mechanisms such as drug metabolism, cellular apoptosis, and DNA repair pathways. We have elucidated how specific miRNAs contribute to resistance by targeting critical signaling pathways and have discussed the potential of leveraging these molecules for therapeutic and diagnostic purposes.
The potential of miRNA-based strategies as innovative tools for overcoming drug resistance is promising, with advances in miRNA profiling and targeted delivery systems offering new avenues for intervention. However, several challenges must be addressed to translate these findings into clinical practice. Key issues include ensuring the specificity of miRNA-targeted therapies, improving the stability and bioavailability of miRNA-based drugs, and developing efficient delivery systems to enhance therapeutic efficacy.
Recent studies have highlighted the critical role of miRNAs in mediating the response of PDAC to radiotherapy. Dysregulation of miRNAs can contribute to the development of radiotherapy resistance by modulating various cellular processes, including autophagy, apoptosis, and DNA damage repair. For example, miR-23b has been shown to inhibit autophagy by targeting the autophagy-related gene ATG12, which in turn enhances radiosensitivity in PDAC cells. Conversely, miR-23b inhibition promotes autophagy and confers radioresistance (Wang et al., 2013b). The interplay between miRNAs and autophagy, as well as their regulation of key cellular pathways involved in the DNA damage response, represents a promising area of research to better understand the mechanisms underlying radiotherapy resistance. Given their ability to modulate multiple molecular targets, miRNAs offer potential as both biomarkers for predicting treatment response and as therapeutic targets to overcome radiotherapy resistance in PDAC. Further investigation into the miRNA-mediated regulation of radioresistance in PDAC will provide critical insights into developing more effective and personalized treatment strategies.
Future research should focus on the following areas: identifying and validating novel miRNAs and their targets involved in drug resistance, developing advanced delivery systems that can precisely target PDAC cells while minimizing off-target effects and exploring combinatorial approaches that integrate miRNA-based therapies with existing treatments to enhance overall efficacy. Additionally, further investigation into the role of miRNAs in resistance mechanisms across different stages of PDAC and their interactions with the TME could provide deeper insights and facilitate the development of more effective therapeutic strategies.
Coupling ISH with tissue microarrays or multiplex staining could enhance its diagnostic utility, allowing for the simultaneous analysis of miRNA expression alongside other biomarkers that contribute to therapy resistance (Eckstein et al., 2019; Rotman et al., 2020). The integration of these techniques could potentially inform the development of personalized miRNA-based therapies, guiding treatment decisions based on individual miRNA profiles and their role in resistance pathways. Furthermore, advancements in RNA sequencing technologies, such as single-cell RNA sequencing, could complement ISH by providing a more comprehensive analysis of miRNA expression at the single-cell level, thereby offering deeper insights into the functional role of specific miRNAs in PDAC (Chen X. et al., 2021; Ligorio et al., 2019). While these approaches are still in the early stages of exploration, their clinical application could pave the way for miRNA-based diagnostic tools and therapies, which could ultimately improve the treatment outcomes of PDAC patients.
It is worth mentioning that, currently, there is limited research on the role of miRNAs in immune therapy resistance specifically in PDAC. However, substantial evidence exists from studies in other cancer types (Romano and Kwong, 2018; Yang et al., 2023). In various malignancies, miRNAs have been found to significantly influence immune therapy outcomes by modulating immune evasion mechanisms, immune cell infiltration, and the expression of immune checkpoint proteins (Kousar et al., 2022; Tang et al., 2022). For instance, miRNAs can impact the effectiveness of immune checkpoint inhibitors by regulating the expression of PD-L1 or affecting T cell activation (Hong et al., 2020; Zhang et al., 2020; Zheng et al., 2023). These insights highlight the potential of exploring miRNA-based strategies to overcome immune therapy resistance in PDAC, offering new avenues for enhancing treatment efficacy.
By addressing these challenges and exploring these future directions, we can advance the field of miRNA-based therapies and potentially improve the clinical management of PDAC, ultimately enhancing patient outcomes and survival rates.
Author contributions
FD: Conceptualization, Project administration, Visualization, Writing–original draft, Writing–review and editing. JZ: Visualization, Writing–original draft, Writing–review and editing. YW: Project administration, Visualization, Writing–review and editing. ZG: Visualization, Writing–review and editing. WL: Project administration, Visualization, Writing–original draft, Writing–review and editing. ZS: Conceptualization, Funding acquisition, Visualization, Writing–original draft, Writing–review and editing.
Funding
The author(s) declare that financial support was received for the research, authorship, and/or publication of this article. This research was supported by the Medical Health Science and Technology Project of Zhejiang Provincial Health Commission (2022KY1246), and the Science and Technology Bureau of Jiaxing City (2023AZ31002 and 2022AZ10009).
Acknowledgments
We sincerely appreciate the potential editors and reviewers for their succinct comments on improving this manuscript.
Conflict of interest
The authors declare that the research was conducted in the absence of any commercial or financial relationships that could be construed as a potential conflict of interest.
Publisher’s note
All claims expressed in this article are solely those of the authors and do not necessarily represent those of their affiliated organizations, or those of the publisher, the editors and the reviewers. Any product that may be evaluated in this article, or claim that may be made by its manufacturer, is not guaranteed or endorsed by the publisher.
Abbreviations
MicroRNAs, miRNAs; Pancreatic cancer, PC; Epithelial-to-mesenchymal transition, EMT; 3′untranslated regions, 3′UTR; Overall survival, OS; Pancreatic ductal adenocarcinoma, PDAC; Metalloproteinase, MMP; Vascular endothelial growth factor, VEGF; Phosphoinositide 3-kinase, PI3K; 5-fluorouracil, 5-FU; Tumor microenvironment, TME; Cancer-associated fibroblasts, CAFs; Tumor-associated macrophages, TAMs; Disease-free survival, DFS; Competing endogenous RNA, ceRNA; Gemcitabine, GEM; Cancer stem cell, CSC; Connexin 43, Cx43; Glucocorticoids, GCs; Jagged-1, JAG1; Side population, SP; Non-side population, NSP; Antisense oligonucleotides, ASOs; Cannabidiol, CBD.
References
Abo-Al-Ela, H. G., and Faggio, C. (2021). MicroRNA-mediated stress response in bivalve species. Ecotoxicol. Environ. Saf. 208, 111442. doi:10.1016/j.ecoenv.2020.111442
Abue, M., Yokoyama, M., Shibuya, R., Tamai, K., Yamaguchi, K., Sato, I., et al. (2015). Circulating miR-483-3p and miR-21 is highly expressed in plasma of pancreatic cancer. Int. J. Oncol. 46 (2), 539–547. doi:10.3892/ijo.2014.2743
Abukiwan, A., Nwaeburu, C. C., Bauer, N., Zhao, Z., Liu, L., Gladkich, J., et al. (2019). Dexamethasone-induced inhibition of miR-132 via methylation promotes TGF-β-driven progression of pancreatic cancer. Int. J. Oncol. 54 (1), 53–64. doi:10.3892/ijo.2018.4616
Alarcon, C. R., Lee, H., Goodarzi, H., Halberg, N., and Tavazoie, S. F. (2015). N6-methyladenosine marks primary microRNAs for processing. Nature 519 (7544), 482–485. doi:10.1038/nature14281
Albulescu, R., Neagu, M., Albulescu, L., and Tanase, C. (2011). Tissular and soluble miRNAs for diagnostic and therapy improvement in digestive tract cancers. Expert Rev. Mol. Diagn 11 (1), 101–120. doi:10.1586/erm.10.106
Al-Sisan, S. M., Zihlif, M. A., and Hammad, H. M. (2023). Differential miRNA expression of hypoxic MCF7 and PANC-1 cells. Front. Endocrinol. (Lausanne) 14, 1110743. doi:10.3389/fendo.2023.1110743
Ambros, V. (2004). The functions of animal microRNAs. Nature 431 (7006), 350–355. doi:10.1038/nature02871
Amponsah, P. S., Fan, P., Bauer, N., Zhao, Z., Gladkich, J., Fellenberg, J., et al. (2017). microRNA-210 overexpression inhibits tumor growth and potentially reverses gemcitabine resistance in pancreatic cancer. Cancer Lett. 388, 107–117. doi:10.1016/j.canlet.2016.11.035
Baradaran, B., Shahbazi, R., and Khordadmehr, M. (2019). Dysregulation of key microRNAs in pancreatic cancer development. Biomed. Pharmacother. 109, 1008–1015. doi:10.1016/j.biopha.2018.10.177
Bear, A. S., Vonderheide, R. H., and O'Hara, M. H. (2020). Challenges and opportunities for pancreatic cancer immunotherapy. Cancer Cell 38 (6), 788–802. doi:10.1016/j.ccell.2020.08.004
Ben-Josef, E., and Lawrence, T. S. (2011). Radiotherapy: the importance of local control in pancreatic cancer. Nat. Rev. Clin. Oncol. 9 (1), 9–10. doi:10.1038/nrclinonc.2011.182
Binenbaum, Y., Fridman, E., Yaari, Z., Milman, N., Schroeder, A., Ben David, G., et al. (2018). Transfer of miRNA in macrophage-derived exosomes induces drug resistance in pancreatic adenocarcinoma. Cancer Res. 78 (18), 5287–5299. doi:10.1158/0008-5472.CAN-18-0124
Cai, J., Chen, H., Lu, M., Zhang, Y., Lu, B., You, L., et al. (2021). Advances in the epidemiology of pancreatic cancer: trends, risk factors, screening, and prognosis. Cancer Lett. 520, 1–11. doi:10.1016/j.canlet.2021.06.027
Chaudhary, A. K., Mondal, G., Kumar, V., Kattel, K., and Mahato, R. I. (2017). Chemosensitization and inhibition of pancreatic cancer stem cell proliferation by overexpression of microRNA-205. Cancer Lett. 402, 1–8. doi:10.1016/j.canlet.2017.05.007
Chen, J., Li, L., Feng, Y., Zhao, Y., Sun, F., Zhou, X., et al. (2024). MKLN1-AS promotes pancreatic cancer progression as a crucial downstream mediator of HIF-1α through miR-185-5p/TEAD1 pathway. Cell Biol. Toxicol. 40 (1), 30. doi:10.1007/s10565-024-09863-8
Chen, K., Wang, Q., Li, M., Guo, H., Liu, W., Wang, F., et al. (2021a). Single-cell RNA-seq reveals dynamic change in tumor microenvironment during pancreatic ductal adenocarcinoma malignant progression. EBioMedicine 66, 103315. doi:10.1016/j.ebiom.2021.103315
Chen, M., Liu, X., Lu, J., Teng, H., Yu, C., Liu, Y., et al. (2023). Dysregulation of the circ_0087502/miR-1179/TGFBR2 pathway supports gemcitabine resistance in pancreatic cancer. Cancer Biol. Ther. 24 (1), 2258566. doi:10.1080/15384047.2023.2258566
Chen, X., Kang, R., Kroemer, G., and Tang, D. (2021b). Targeting ferroptosis in pancreatic cancer: a double-edged sword. Trends Cancer 7 (10), 891–901. doi:10.1016/j.trecan.2021.04.005
Chhatriya, B., Mukherjee, M., Ray, S., Sarkar, P., Chatterjee, S., Nath, D., et al. (2019). Comparison of tumour and serum specific microRNA changes dissecting their role in pancreatic ductal adenocarcinoma: a meta-analysis. BMC Cancer 19 (1), 1175. doi:10.1186/s12885-019-6380-z
Chu, Y. H., Hardin, H., Zhang, R., Guo, Z., and Lloyd, R. V. (2019). In situ hybridization: introduction to techniques, applications and pitfalls in the performance and interpretation of assays. Semin. Diagn Pathol. 36 (5), 336–341. doi:10.1053/j.semdp.2019.06.004
Cioffi, M., Trabulo, S. M., Sanchez-Ripoll, Y., Miranda-Lorenzo, I., Lonardo, E., Dorado, J., et al. (2015). The miR-17-92 cluster counteracts quiescence and chemoresistance in a distinct subpopulation of pancreatic cancer stem cells. Gut 64 (12), 1936–1948. doi:10.1136/gutjnl-2014-308470
Daoud, A. Z., Mulholland, E. J., Cole, G., and McCarthy, H. O. (2019). MicroRNAs in Pancreatic Cancer: biomarkers, prognostic, and therapeutic modulators. BMC Cancer 19 (1), 1130. doi:10.1186/s12885-019-6284-y
Du, J., Li, M., Huang, Q., Liu, W., Li, W. Q., Li, Y. J., et al. (2019). The critical role of microRNAs in stress response: therapeutic prospect and limitation. Pharmacol. Res. 142, 294–302. doi:10.1016/j.phrs.2018.12.007
Eckstein, M., Sailer, V., Nielsen, B. S., Wittenberg, T., Wiesmann, V., Lieb, V., et al. (2019). Co-staining of microRNAs and their target proteins by miRNA in situ hybridization and immunohistofluorescence on prostate cancer tissue microarrays. Lab. Invest 99 (10), 1527–1534. doi:10.1038/s41374-019-0251-8
Eshmuminov, D., Aminjonov, B., Palm, R. F., Malleo, G., Schmocker, R. K., Abdallah, R., et al. (2023). FOLFIRINOX or gemcitabine-based chemotherapy for borderline resectable and locally advanced pancreatic cancer: a multi-institutional, patient-level, meta-analysis and systematic review. Ann. Surg. Oncol. 30 (7), 4417–4428. doi:10.1245/s10434-023-13353-2
Evans, D., Ghassemi, N., Hajibandeh, S., Hajibandeh, S., Romman, S., Laing, R. W., et al. (2024). Meta-analysis of adjuvant chemotherapy versus no adjuvant chemotherapy for resected stage I pancreatic cancer. Surgery 175 (6), 1470–1479. doi:10.1016/j.surg.2023.11.027
Fan, P., Liu, L., Yin, Y., Zhao, Z., Zhang, Y., Amponsah, P. S., et al. (2016). MicroRNA-101-3p reverses gemcitabine resistance by inhibition of ribonucleotide reductase M1 in pancreatic cancer. Cancer Lett. 373 (1), 130–137. doi:10.1016/j.canlet.2016.01.038
Fang, C., Dai, C. Y., Mei, Z., Jiang, M. J., Gu, D. N., Huang, Q., et al. (2018). microRNA-193a stimulates pancreatic cancer cell repopulation and metastasis through modulating TGF-β2/TGF-βRIII signalings. J. Exp. Clin. Cancer Res. 37 (1), 25. doi:10.1186/s13046-018-0697-3
Farhangnia, P., Khorramdelazad, H., Nickho, H., and Delbandi, A. A. (2024). Current and future immunotherapeutic approaches in pancreatic cancer treatment. J. Hematol. Oncol. 17 (1), 40. doi:10.1186/s13045-024-01561-6
Fathi, M., Ghafouri-Fard, S., Abak, A., and Taheri, M. (2021). Emerging roles of miRNAs in the development of pancreatic cancer. Biomed. Pharmacother. 141, 111914. doi:10.1016/j.biopha.2021.111914
Fellmann, C., Hoffmann, T., Sridhar, V., Hopfgartner, B., Muhar, M., Roth, M., et al. (2013). An optimized microRNA backbone for effective single-copy RNAi. Cell Rep. 5 (6), 1704–1713. doi:10.1016/j.celrep.2013.11.020
Ferino, A., Miglietta, G., Picco, R., Vogel, S., Wengel, J., and Xodo, L. E. (2018). MicroRNA therapeutics: design of single-stranded miR-216b mimics to target KRAS in pancreatic cancer cells. RNA Biol. 15 (10), 1273–1285. doi:10.1080/15476286.2018.1526536
Ferrara, B., Pignatelli, C., Cossutta, M., Citro, A., Courty, J., and Piemonti, L. (2021). The extracellular matrix in pancreatic cancer: description of a complex network and promising therapeutic options. Cancers (Basel) 13 (17), 4442. doi:10.3390/cancers13174442
Ferro, R., Adamska, A., Lattanzio, R., Mavrommati, I., Edling, C. E., Arifin, S. A., et al. (2018). GPR55 signalling promotes proliferation of pancreatic cancer cells and tumour growth in mice, and its inhibition increases effects of gemcitabine. Oncogene 37 (49), 6368–6382. doi:10.1038/s41388-018-0390-1
Funamizu, N., Lacy, C. R., Kamada, M., Yanaga, K., and Manome, Y. (2019). MicroRNA-200b and -301 are associated with gemcitabine response as biomarkers in pancreatic carcinoma cells. Int. J. Oncol. 54 (3), 991–1000. doi:10.3892/ijo.2019.4676
Furukawa, T. (2009). Molecular pathology of pancreatic cancer: implications for molecular targeting therapy. Clin. Gastroenterol. Hepatol. 7 (11 Suppl. l), S35–S39. doi:10.1016/j.cgh.2009.07.035
Ge, W., Goga, A., He, Y., Silva, P. N., Hirt, C. K., Herrmanns, K., et al. (2022). miR-802 suppresses acinar-to-ductal reprogramming during early pancreatitis and pancreatic carcinogenesis. Gastroenterology 162 (1), 269–284. doi:10.1053/j.gastro.2021.09.029
Georgikou, C., Yin, L., Gladkich, J., Xiao, X., Sticht, C., Torre, C., et al. (2020). Inhibition of miR30a-3p by sulforaphane enhances gap junction intercellular communication in pancreatic cancer. Cancer Lett. 469, 238–245. doi:10.1016/j.canlet.2019.10.042
Ghaneh, P., Palmer, D., Cicconi, S., Jackson, R., Halloran, C. M., Rawcliffe, C., et al. (2023). Immediate surgery compared with short-course neoadjuvant gemcitabine plus capecitabine, FOLFIRINOX, or chemoradiotherapy in patients with borderline resectable pancreatic cancer (ESPAC5): a four-arm, multicentre, randomised, phase 2 trial. Lancet Gastroenterol. Hepatol. 8 (2), 157–168. doi:10.1016/S2468-1253(22)00348-X
Giovannetti, E., Funel, N., Peters, G. J., Del Chiaro, M., Erozenci, L. A., Vasile, E., et al. (2010). MicroRNA-21 in pancreatic cancer: correlation with clinical outcome and pharmacologic aspects underlying its role in the modulation of gemcitabine activity. Cancer Res. 70 (11), 4528–4538. doi:10.1158/0008-5472.CAN-09-4467
Gregory, R. I., Chendrimada, T. P., Cooch, N., and Shiekhattar, R. (2005). Human RISC couples microRNA biogenesis and posttranscriptional gene silencing. Cell 123 (4), 631–640. doi:10.1016/j.cell.2005.10.022
Gu, J., Huang, W., Wang, X., Zhang, J., Tao, T., Zheng, Y., et al. (2022). Hsa-miR-3178/RhoB/PI3K/Akt, a novel signaling pathway regulates ABC transporters to reverse gemcitabine resistance in pancreatic cancer. Mol. Cancer 21 (1), 112. doi:10.1186/s12943-022-01587-9
Guo, Y., Li, H., and Sun, C. (2023a). Exosomal miR-125b-5p derived from cancer-associated fibroblasts promotes the growth, migration, and invasion of pancreatic cancer cells by decreasing adenomatous polyposis coli (APC) expression. J. Gastrointest. Oncol. 14 (2), 1064–1076. doi:10.21037/jgo-23-198
Guo, Y., Wu, H., Xiong, J., Gou, S., Cui, J., and Peng, T. (2023b). miR-222-3p-containing macrophage-derived extracellular vesicles confer gemcitabine resistance via TSC1-mediated mTOR/AKT/PI3K pathway in pancreatic cancer. Cell Biol. Toxicol. 39 (4), 1203–1214. doi:10.1007/s10565-022-09736-y
Gupta, A., Andresen, J. L., Manan, R. S., and Langer, R. (2021). Nucleic acid delivery for therapeutic applications. Adv. Drug Deliv. Rev. 178, 113834. doi:10.1016/j.addr.2021.113834
Ha, M., and Kim, V. N. (2014). Regulation of microRNA biogenesis. Nat. Rev. Mol. Cell Biol. 15 (8), 509–524. doi:10.1038/nrm3838
Hasegawa, S., Eguchi, H., Nagano, H., Konno, M., Tomimaru, Y., Wada, H., et al. (2014). MicroRNA-1246 expression associated with CCNG2-mediated chemoresistance and stemness in pancreatic cancer. Br. J. Cancer 111 (8), 1572–1580. doi:10.1038/bjc.2014.454
Ho, A. S., Huang, X., Cao, H., Christman-Skieller, C., Bennewith, K., Le, Q. T., et al. (2010). Circulating miR-210 as a novel hypoxia marker in pancreatic cancer. Transl. Oncol. 3 (2), 109–113. doi:10.1593/tlo.09256
Ho, W. J., Jaffee, E. M., and Zheng, L. (2020). The tumour microenvironment in pancreatic cancer - clinical challenges and opportunities. Nat. Rev. Clin. Oncol. 17 (9), 527–540. doi:10.1038/s41571-020-0363-5
Hong, W., Xue, M., Jiang, J., Zhang, Y., and Gao, X. (2020). Circular RNA circ-CPA4/let-7 miRNA/PD-L1 axis regulates cell growth, stemness, drug resistance and immune evasion in non-small cell lung cancer (NSCLC). J. Exp. Clin. Cancer Res. 39 (1), 149. doi:10.1186/s13046-020-01648-1
Hua, Y. Q., Zhu, Y. D., Xie, G. Q., Zhang, K., Sheng, J., Zhu, Z. F., et al. (2019). Long non-coding SBF2-AS1 acting as a competing endogenous RNA to sponge microRNA-142-3p to participate in gemcitabine resistance in pancreatic cancer via upregulating TWF1. Aging (Albany NY) 11 (20), 8860–8878. doi:10.18632/aging.102307
Huang, B., Wang, J., Chen, Q., Qu, C., Zhang, J., Chen, E., et al. (2019). Gemcitabine enhances OSI-027 cytotoxicity by upregulation of miR-663a in pancreatic ductal adenocarcinoma cells. Am. J. Transl. Res. 11 (1), 473–485. Available at: https://www.ncbi.nlm.nih.gov/pubmed/30788003.
Huang, H., Xiong, G., Shen, P., Cao, Z., Zheng, L., Zhang, T., et al. (2017). MicroRNA-1285 inhibits malignant biological behaviors of human pancreatic cancer cells by negative regulation of YAP1. Neoplasma 64 (3), 358–366. doi:10.4149/neo_2017_306
Huang, J. S., Egger, M. E., Grizzle, W. E., and McNally, L. R. (2013). MicroRNA-100 regulates IGF1-receptor expression in metastatic pancreatic cancer cells. Biotech. Histochem 88 (7), 397–402. doi:10.3109/10520295.2012.762460
Iwakawa, H. O., and Tomari, Y. (2022). Life of RISC: formation, action, and degradation of RNA-induced silencing complex. Mol. Cell 82 (1), 30–43. doi:10.1016/j.molcel.2021.11.026
Jiang, A., Dong, C., Li, B., Zhang, Z., Chen, Y., Ning, C., et al. (2019). MicroRNA-206 regulates cell proliferation by targeting G6PD in skeletal muscle. FASEB J. 33 (12), 14083–14094. doi:10.1096/fj.201900502RRRR
Jiang, M. J., Lin, C. J., Liu, F. R., Mei, Z., Gu, D. N., and Tian, L. (2024). Pancreatic cancer cells hijack tumor suppressive microRNA-26a to promote radioresistance and potentiate tumor repopulation. Heliyon 10 (10), e31346. doi:10.1016/j.heliyon.2024.e31346
Jiang, Y., Zhang, H., Wang, J., Liu, Y., Luo, T., and Hua, H. (2022). Targeting extracellular matrix stiffness and mechanotransducers to improve cancer therapy. J. Hematol. Oncol. 15 (1), 34. doi:10.1186/s13045-022-01252-0
Khalaf, K., Hana, D., Chou, J. T., Singh, C., Mackiewicz, A., and Kaczmarek, M. (2021). Aspects of the tumor microenvironment involved in immune resistance and drug resistance. Front. Immunol. 12, 656364. doi:10.3389/fimmu.2021.656364
Khan, P., Siddiqui, J. A., Kshirsagar, P. G., Venkata, R. C., Maurya, S. K., Mirzapoiazova, T., et al. (2023). MicroRNA-1 attenuates the growth and metastasis of small cell lung cancer through CXCR4/FOXM1/RRM2 axis. Mol. Cancer 22 (1), 1. doi:10.1186/s12943-022-01695-6
Kim, V. N., and Nam, J. W. (2006). Genomics of microRNA. Trends Genet. 22 (3), 165–173. doi:10.1016/j.tig.2006.01.003
Kousar, K., Ahmad, T., Abduh, M. S., Kanwal, B., Shah, S. S., Naseer, F., et al. (2022). miRNAs in regulation of tumor microenvironment, chemotherapy resistance, immunotherapy modulation and miRNA therapeutics in cancer. Int. J. Mol. Sci. 23 (22), 13822. doi:10.3390/ijms232213822
Li, L., He, Z., Zhu, C., Chen, S., Yang, Z., Xu, J., et al. (2020). MiR-137 promotes anoikis through modulating the AKT signaling pathways in Pancreatic Cancer. J. Cancer 11 (21), 6277–6285. doi:10.7150/jca.44037
Li, Y., VandenBoom, T. G., Kong, D., Wang, Z., Ali, S., Philip, P. A., et al. (2009). Up-regulation of miR-200 and let-7 by natural agents leads to the reversal of epithelial-to-mesenchymal transition in gemcitabine-resistant pancreatic cancer cells. Cancer Res. 69 (16), 6704–6712. doi:10.1158/0008-5472.CAN-09-1298
Ligorio, M., Sil, S., Malagon-Lopez, J., Nieman, L. T., Misale, S., Di Pilato, M., et al. (2019). Stromal microenvironment shapes the intratumoral architecture of pancreatic cancer. Cell 178 (1), 160–175. doi:10.1016/j.cell.2019.05.012
Liu, F., Liu, B., Qian, J., Wu, G., Li, J., and Ma, Z. (2017). miR-153 enhances the therapeutic effect of gemcitabine by targeting Snail in pancreatic cancer. Acta Biochim. Biophys. Sin. (Shanghai) 49 (6), 520–529. doi:10.1093/abbs/gmx039
Liu, L., Han, S., Xiao, X., An, X., Gladkich, J., Hinz, U., et al. (2022). Glucocorticoid-induced microRNA-378 signaling mediates the progression of pancreatic cancer by enhancing autophagy. Cell Death Dis. 13 (12), 1052. doi:10.1038/s41419-022-05503-3
Liu, M., Zhang, Y., Yang, J., Zhan, H., Zhou, Z., Jiang, Y., et al. (2021). Zinc-dependent regulation of ZEB1 and YAP1 coactivation promotes epithelial-mesenchymal transition plasticity and metastasis in pancreatic cancer. Gastroenterology 160 (5), 1771–1783.e1. doi:10.1053/j.gastro.2020.12.077
Lou, W., Liu, J., Gao, Y., Zhong, G., Ding, B., Xu, L., et al. (2018). MicroRNA regulation of liver cancer stem cells. Am. J. Cancer Res. 8 (7), 1126–1141. Available at: https://www.ncbi.nlm.nih.gov/pubmed/30094089.
Lu, H., Lu, S., Yang, D., Zhang, L., Ye, J., Li, M., et al. (2019). MiR-20a-5p regulates gemcitabine chemosensitivity by targeting RRM2 in pancreatic cancer cells and serves as a predictor for gemcitabine-based chemotherapy. Biosci. Rep. 39 (5). doi:10.1042/BSR20181374
Lu, T. X., and Rothenberg, M. E. (2018). MicroRNA. J. Allergy Clin. Immunol. 141 (4), 1202–1207. doi:10.1016/j.jaci.2017.08.034
Lynam-Lennon, N., Maher, S. G., and Reynolds, J. V. (2009). The roles of microRNA in cancer and apoptosis. Biol. Rev. Camb Philos. Soc. 84 (1), 55–71. doi:10.1111/j.1469-185X.2008.00061.x
Ma, Y., Chai, N., Jiang, Q., Chang, Z., Chai, Y., Li, X., et al. (2020). DNA methyltransferase mediates the hypermethylation of the microRNA 34a promoter and enhances the resistance of patient-derived pancreatic cancer cells to molecular targeting agents. Pharmacol. Res. 160, 105071. doi:10.1016/j.phrs.2020.105071
Madadjim, R., An, T., and Cui, J. (2024). MicroRNAs in pancreatic cancer: advances in biomarker discovery and therapeutic implications. Int. J. Mol. Sci. 25 (7), 3914. doi:10.3390/ijms25073914
Marin-Muller, C., Li, D., Lu, J. M., Liang, Z., Vega-Martinez, O., Crawford, S. E., et al. (2023). Nanoparticle-mediated therapy with miR-198 sensitizes pancreatic cancer to gemcitabine treatment through downregulation of VCP-mediated autophagy. Pharmaceutics 15 (8), 2038. doi:10.3390/pharmaceutics15082038
Marsit, C. J., Eddy, K., and Kelsey, K. T. (2006). MicroRNA responses to cellular stress. Cancer Res. 66 (22), 10843–10848. doi:10.1158/0008-5472.CAN-06-1894
Meister, G. (2023). MicroRNA uses a gym to get fit for cuts by Dicer enzyme. Nature 615 (7951), 218–219. doi:10.1038/d41586-023-00478-3
Meng, Q., Liang, C., Hua, J., Zhang, B., Liu, J., Zhang, Y., et al. (2020). A miR-146a-5p/TRAF6/NF-kB p65 axis regulates pancreatic cancer chemoresistance: functional validation and clinical significance. Theranostics 10 (9), 3967–3979. doi:10.7150/thno.40566
Morinaga, S., Nakamura, Y., Atsumi, Y., Murakawa, M., Yamaoku, K., Aoyama, T., et al. (2016). Locked nucleic acid in situ hybridization analysis of MicroRNA-21 predicts clinical outcome in patients after resection for pancreatic cancer treated with adjuvant gemcitabine monotherapy. Anticancer Res. 36 (3), 1083–1088.
Nagano, H., Tomimaru, Y., Eguchi, H., Hama, N., Wada, H., Kawamoto, K., et al. (2013). MicroRNA-29a induces resistance to gemcitabine through the Wnt/β-catenin signaling pathway in pancreatic cancer cells. Int. J. Oncol. 43 (4), 1066–1072. doi:10.3892/ijo.2013.2037
Neoptolemos, J. P., Kleeff, J., Michl, P., Costello, E., Greenhalf, W., and Palmer, D. H. (2018). Therapeutic developments in pancreatic cancer: current and future perspectives. Nat. Rev. Gastroenterol. Hepatol. 15 (6), 333–348. doi:10.1038/s41575-018-0005-x
Okazaki, J., Tanahashi, T., Sato, Y., Miyoshi, J., Nakagawa, T., Kimura, T., et al. (2020). MicroRNA-296-5p promotes cell invasion and drug resistance by targeting bcl2-related ovarian killer, leading to a poor prognosis in pancreatic cancer. Digestion 101 (6), 794–806. doi:10.1159/000503225
Okusaka, T., and Furuse, J. (2020). Recent advances in chemotherapy for pancreatic cancer: evidence from Japan and recommendations in guidelines. J. Gastroenterol. 55 (4), 369–382. doi:10.1007/s00535-020-01666-y
Pan, G., Liu, Y., Shang, L., Zhou, F., and Yang, S. (2021a). EMT-associated microRNAs and their roles in cancer stemness and drug resistance. Cancer Commun. (Lond) 41 (3), 199–217. doi:10.1002/cac2.12138
Pan, Y., Li, K., Tao, X., Li, N., Huang, J., Liu, J., et al. (2021b). MicroRNAs in pancreatic cancer and chemoresistance. Pancreas 50 (10), 1334–1342. doi:10.1097/MPA.0000000000001934
Pan, Y., Li, K., Tao, X., Zhao, Y., Chen, Q., Li, N., et al. (2021c). MicroRNA-34a alleviates gemcitabine resistance in pancreatic cancer by repression of cancer stem cell renewal. Pancreas 50 (9), 1260–1266. doi:10.1097/MPA.0000000000001920
Pan, Y., Yu, Y., Wang, X., and Zhang, T. (2020). Tumor-associated macrophages in tumor immunity. Front. Immunol. 11, 583084. doi:10.3389/fimmu.2020.583084
Pang, W., Su, J., Wang, Y., Feng, H., Dai, X., Yuan, Y., et al. (2015). Pancreatic cancer-secreted miR-155 implicates in the conversion from normal fibroblasts to cancer-associated fibroblasts. Cancer Sci. 106 (10), 1362–1369. doi:10.1111/cas.12747
Parasramka, M. A., Ali, S., Banerjee, S., Deryavoush, T., Sarkar, F. H., and Gupta, S. (2013). Garcinol sensitizes human pancreatic adenocarcinoma cells to gemcitabine in association with microRNA signatures. Mol. Nutr. Food Res. 57 (2), 235–248. doi:10.1002/mnfr.201200297
Park, J. K., Lee, E. J., Esau, C., and Schmittgen, T. D. (2009). Antisense inhibition of microRNA-21 or -221 arrests cell cycle, induces apoptosis, and sensitizes the effects of gemcitabine in pancreatic adenocarcinoma. Pancreas 38 (7), e190–e199. doi:10.1097/MPA.0b013e3181ba82e1
Park, W., Chawla, A., and O'Reilly, E. M. (2021). Pancreatic cancer: a review. JAMA 326 (9), 851–862. doi:10.1001/jama.2021.13027
Patel, G. K., Khan, M. A., Bhardwaj, A., Srivastava, S. K., Zubair, H., Patton, M. C., et al. (2017). Exosomes confer chemoresistance to pancreatic cancer cells by promoting ROS detoxification and miR-155-mediated suppression of key gemcitabine-metabolising enzyme, DCK. Br. J. Cancer 116 (5), 609–619. doi:10.1038/bjc.2017.18
Perkhofer, L., Gout, J., Roger, E., Kude de Almeida, F., Baptista Simoes, C., Wiesmuller, L., et al. (2021). DNA damage repair as a target in pancreatic cancer: state-of-the-art and future perspectives. Gut 70 (3), 606–617. doi:10.1136/gutjnl-2019-319984
Qi, R., Bai, Y., Li, K., Liu, N., Xu, Y., Dal, E., et al. (2023). Cancer-associated fibroblasts suppress ferroptosis and induce gemcitabine resistance in pancreatic cancer cells by secreting exosome-derived ACSL4-targeting miRNAs. Drug Resist Updat 68, 100960. doi:10.1016/j.drup.2023.100960
Qian, Y., Xiong, Y., Feng, D., Wu, Y., Zhang, X., Chen, L., et al. (2019). Coix seed extract enhances the anti-pancreatic cancer efficacy of gemcitabine through regulating ABCB1- and ABCG2-mediated drug efflux: a bioluminescent pharmacokinetic and pharmacodynamic study. Int. J. Mol. Sci. 20 (21), 5250. doi:10.3390/ijms20215250
Qin, C., Yang, G., Yang, J., Ren, B., Wang, H., Chen, G., et al. (2020). Metabolism of pancreatic cancer: paving the way to better anticancer strategies. Mol. Cancer 19 (1), 50. doi:10.1186/s12943-020-01169-7
Rajabpour, A., Afgar, A., Mahmoodzadeh, H., Radfar, J. E., Rajaei, F., and Teimoori-Toolabi, L. (2017). MiR-608 regulating the expression of ribonucleotide reductase M1 and cytidine deaminase is repressed through induced gemcitabine chemoresistance in pancreatic cancer cells. Cancer Chemother. Pharmacol. 80 (4), 765–775. doi:10.1007/s00280-017-3418-2
Rehman, M., Khaled, A., and Noel, M. (2022). Cytotoxic chemotherapy in advanced pancreatic cancer. Hematol. Oncol. Clin. North Am. 36 (5), 1011–1018. doi:10.1016/j.hoc.2022.07.006
Ren, Z. G., Dong, S. X., Han, P., and Qi, J. (2016). miR-203 promotes proliferation, migration and invasion by degrading SIK1 in pancreatic cancer. Oncol. Rep. 35 (3), 1365–1374. doi:10.3892/or.2015.4534
Richards, K. E., Xiao, W., and Hill, R.On Behalf Of The Usc Pancreas Research, T (2022). Cancer-associated fibroblasts confer gemcitabine resistance to pancreatic cancer cells through PTEN-targeting miRNAs in exosomes. Cancers (Basel) 14 (11), 2812. doi:10.3390/cancers14112812
Richards, K. E., Zeleniak, A. E., Fishel, M. L., Wu, J., Littlepage, L. E., and Hill, R. (2017). Cancer-associated fibroblast exosomes regulate survival and proliferation of pancreatic cancer cells. Oncogene 36 (13), 1770–1778. doi:10.1038/onc.2016.353
Romano, G., and Kwong, L. N. (2018). Diagnostic and therapeutic applications of miRNA-based strategies to cancer immunotherapy. Cancer Metastasis Rev. 37 (1), 45–53. doi:10.1007/s10555-017-9716-7
Rotman, J., den Otter, L. A. S., Bleeker, M. C. G., Samuels, S. S., Heeren, A. M., Roemer, M. G. M., et al. (2020). PD-L1 and PD-L2 expression in cervical cancer: regulation and biomarker potential. Front. Immunol. 11, 596825. doi:10.3389/fimmu.2020.596825
Saliminejad, K., Khorram Khorshid, H. R., Soleymani Fard, S., and Ghaffari, S. H. (2019). An overview of microRNAs: biology, functions, therapeutics, and analysis methods. J. Cell Physiol. 234 (5), 5451–5465. doi:10.1002/jcp.27486
Schreiber, R., Mezencev, R., Matyunina, L. V., and McDonald, J. F. (2016). Evidence for the role of microRNA 374b in acquired cisplatin resistance in pancreatic cancer cells. Cancer Gene Ther. 23 (8), 241–245. doi:10.1038/cgt.2016.23
Shaib, Y. H., Davila, J. A., and El-Serag, H. B. (2006). The epidemiology of pancreatic cancer in the United States: changes below the surface. Aliment. Pharmacol. Ther. 24 (1), 87–94. doi:10.1111/j.1365-2036.2006.02961.x
Shao, Y., Zhang, L., Cui, L., Lou, W., Wang, D., Lu, W., et al. (2015). LIN28B suppresses microRNA let-7b expression to promote CD44+/LIN28B+ human pancreatic cancer stem cell proliferation and invasion. Am. J. Cancer Res. 5 (9), 2643–2659. Available at: https://www.ncbi.nlm.nih.gov/pubmed/26609473.
Sherman, M. H., and Beatty, G. L. (2023). Tumor microenvironment in pancreatic cancer pathogenesis and therapeutic resistance. Annu. Rev. Pathol. 18, 123–148. doi:10.1146/annurev-pathmechdis-031621-024600
Shirakawa, T., Makiyama, A., Shimokawa, M., Otsuka, T., Shinohara, Y., Koga, F., et al. (2023). C-reactive protein/albumin ratio is the most significant inflammatory marker in unresectable pancreatic cancer treated with FOLFIRINOX or gemcitabine plus nab-paclitaxel. Sci. Rep. 13 (1), 8815. doi:10.1038/s41598-023-34962-7
Sicard, F., Gayral, M., Lulka, H., Buscail, L., and Cordelier, P. (2013). Targeting miR-21 for the therapy of pancreatic cancer. Mol. Ther. 21 (5), 986–994. doi:10.1038/mt.2013.35
Siegel, R. L., Miller, K. D., Fuchs, H. E., and Jemal, A. (2021). Cancer statistics, 2021. CA Cancer J. Clin. 71 (1), 7–33. doi:10.3322/caac.21654
Singh, P. K., Brand, R. E., and Mehla, K. (2012). MicroRNAs in pancreatic cancer metabolism. Nat. Rev. Gastroenterol. Hepatol. 9 (6), 334–344. doi:10.1038/nrgastro.2012.63
Son, S., Kim, B., Yang, J., and Kim, V. N. (2023). Role of the proline-rich disordered domain of DROSHA in intronic microRNA processing. Genes Dev. 37 (9-10), 383–397. doi:10.1101/gad.350275.122
Srivastava, S. K., Bhardwaj, A., Arora, S., Tyagi, N., Singh, S., Andrews, J., et al. (2015). MicroRNA-345 induces apoptosis in pancreatic cancer cells through potentiation of caspase-dependent and -independent pathways. Br. J. Cancer 113 (4), 660–668. doi:10.1038/bjc.2015.252
Su, Y. H., Hsu, T. W., Chen, H. A., Su, C. M., Huang, M. T., Chuang, T. H., et al. (2021). ERK-mediated transcriptional activation of Dicer is involved in gemcitabine resistance of pancreatic cancer. J. Cell Physiol. 236 (6), 4420–4434. doi:10.1002/jcp.30159
Sun, W., Julie Li, Y. S., Huang, H. D., Shyy, J. Y., and Chien, S. (2010). microRNA: a master regulator of cellular processes for bioengineering systems. Annu. Rev. Biomed. Eng. 12, 1–27. doi:10.1146/annurev-bioeng-070909-105314
Takiuchi, D., Eguchi, H., Nagano, H., Iwagami, Y., Tomimaru, Y., Wada, H., et al. (2013). Involvement of microRNA-181b in the gemcitabine resistance of pancreatic cancer cells. Pancreatology 13 (5), 517–523. doi:10.1016/j.pan.2013.06.007
Tang, W. W., Bauer, K. M., Barba, C., Ekiz, H. A., and O'Connell, R. M. (2022). miR-aculous new avenues for cancer immunotherapy. Front. Immunol. 13, 929677. doi:10.3389/fimmu.2022.929677
Tian, X., Shivapurkar, N., Wu, Z., Hwang, J. J., Pishvaian, M. J., Weiner, L. M., et al. (2016). Circulating microRNA profile predicts disease progression in patients receiving second-line treatment of lapatinib and capecitabine for metastatic pancreatic cancer. Oncol. Lett. 11 (3), 1645–1650. doi:10.3892/ol.2016.4101
Tiriac, H., Belleau, P., Engle, D. D., Plenker, D., Deschenes, A., Somerville, T. D. D., et al. (2018). Organoid profiling identifies common responders to chemotherapy in pancreatic cancer. Cancer Discov. 8 (9), 1112–1129. doi:10.1158/2159-8290.CD-18-0349
Uhan, S., and Hauptman, N. (2021). Metastatic EMT phenotype is governed by MicroRNA-200-mediated competing endogenous RNA networks. Cells 11 (1), 73. doi:10.3390/cells11010073
Volpi, C. C., Gualeni, A. V., Pietrantonio, F., Vaccher, E., Carbone, A., and Gloghini, A. (2018). Bright-field in situ hybridization detects gene alterations and viral infections useful for personalized management of cancer patients. Expert Rev. Mol. Diagn 18 (3), 259–277. doi:10.1080/14737159.2018.1440210
Wang, L., Mu, N., and Qu, N. (2021). Methylation of the miR-29b-3p promoter contributes to angiogenesis, invasion, and migration in pancreatic cancer. Oncol. Rep. 45 (1), 65–72. doi:10.3892/or.2020.7832
Wang, P., Zhang, J., Zhang, L., Zhu, Z., Fan, J., Chen, L., et al. (2013a). MicroRNA 23b regulates autophagy associated with radioresistance of pancreatic cancer cells. Gastroenterology 145 (5), 1133–1143. doi:10.1053/j.gastro.2013.07.048
Wang, P., Zhang, L., Chen, Z., and Meng, Z. (2013b). MicroRNA targets autophagy in pancreatic cancer cells during cancer therapy. Autophagy 9 (12), 2171–2172. doi:10.4161/auto.26463
Wang, W., Zhao, L., Wei, X., Wang, L., Liu, S., Yang, Y., et al. (2016). MicroRNA-320a promotes 5-FU resistance in human pancreatic cancer cells. Sci. Rep. 6, 27641. doi:10.1038/srep27641
Wang, Z. C., Huang, F. Z., Xu, H. B., Sun, J. C., and Wang, C. F. (2019). MicroRNA-137 inhibits autophagy and chemosensitizes pancreatic cancer cells by targeting ATG5. Int. J. Biochem. Cell Biol. 111, 63–71. doi:10.1016/j.biocel.2019.01.020
Wei, F., Liu, Y., Guo, Y., Xiang, A., Wang, G., Xue, X., et al. (2013). miR-99b-targeted mTOR induction contributes to irradiation resistance in pancreatic cancer. Mol. Cancer 12, 81. doi:10.1186/1476-4598-12-81
Wei, X., Wang, W., Wang, L., Zhang, Y., Zhang, X., Chen, M., et al. (2016). MicroRNA-21 induces 5-fluorouracil resistance in human pancreatic cancer cells by regulating PTEN and PDCD4. Cancer Med. 5 (4), 693–702. doi:10.1002/cam4.626
Werba, G., Weissinger, D., Kawaler, E. A., Zhao, E., Kalfakakou, D., Dhara, S., et al. (2023). Single-cell RNA sequencing reveals the effects of chemotherapy on human pancreatic adenocarcinoma and its tumor microenvironment. Nat. Commun. 14 (1), 797. doi:10.1038/s41467-023-36296-4
Wilczynska, A., and Bushell, M. (2015). The complexity of miRNA-mediated repression. Cell Death Differ. 22 (1), 22–33. doi:10.1038/cdd.2014.112
Wu, X., Huang, J., Yang, Z., Zhu, Y., Zhang, Y., Wang, J., et al. (2020). MicroRNA-221-3p is related to survival and promotes tumour progression in pancreatic cancer: a comprehensive study on functions and clinicopathological value. Cancer Cell Int. 20, 443. doi:10.1186/s12935-020-01529-9
Xie, F., Li, C., Zhang, X., Peng, W., and Wen, T. (2019). MiR-143-3p suppresses tumorigenesis in pancreatic ductal adenocarcinoma by targeting KRAS. Biomed. Pharmacother. 119, 109424. doi:10.1016/j.biopha.2019.109424
Xin, X., Lin, F., Wang, Q., Yin, L., and Mahato, R. I. (2019). ROS-responsive polymeric micelles for triggered simultaneous delivery of PLK1 inhibitor/miR-34a and effective synergistic therapy in pancreatic cancer. ACS Appl. Mater Interfaces 11 (16), 14647–14659. doi:10.1021/acsami.9b02756
Xu, F., Wu, H., Xiong, J., and Peng, T. (2021a). Long non-coding RNA DLEU2L targets miR-210-3p to suppress gemcitabine resistance in pancreatic cancer cells via BRCA2 regulation. Front. Mol. Biosci. 8, 645365. doi:10.3389/fmolb.2021.645365
Xu, H., Chen, R., Shen, Q., Yang, D., Peng, H., Tong, J., et al. (2021b). Overexpression of circular RNA circ_0013587 reverses erlotinib resistance in pancreatic cancer cells through regulating the miR-1227/E-cadherin pathway. Front. Oncol. 11, 754146. doi:10.3389/fonc.2021.754146
Yamashita, K., and Kumamoto, Y. (2024). CAFs-associated genes (CAFGs) in pancreatic ductal adenocarcinoma (PDAC) and novel therapeutic strategy. Int. J. Mol. Sci. 25 (11), 6003. doi:10.3390/ijms25116003
Yang, D., Hu, Z., Xu, J., Tang, Y., Wang, Y., Cai, Q., et al. (2019). MiR-760 enhances sensitivity of pancreatic cancer cells to gemcitabine through modulating Integrin β1. Biosci. Rep. 39 (11). doi:10.1042/BSR20192358
Yang, H., Liu, Y., Chen, L., Zhao, J., Guo, M., Zhao, X., et al. (2023). miRNA-based therapies for lung cancer: opportunities and challenges? Biomolecules 13 (6), 877. doi:10.3390/biom13060877
Yang, Z., Zhao, N., Cui, J., Wu, H., Xiong, J., and Peng, T. (2020). Exosomes derived from cancer stem cells of gemcitabine-resistant pancreatic cancer cells enhance drug resistance by delivering miR-210. Cell Oncol. (Dordr) 43 (1), 123–136. doi:10.1007/s13402-019-00476-6
Ye, Z. Q., Chen, H. B., Zhang, T. Y., Chen, Z., Tian, L., and Gu, D. N. (2021). MicroRNA-7 modulates cellular senescence to relieve gemcitabine resistance by targeting PARP1/NF-κB signaling in pancreatic cancer cells. Oncol. Lett. 21 (2), 139. doi:10.3892/ol.2020.12400
Yu, G., Jia, B., Cheng, Y., Zhou, L., Qian, B., Liu, Z., et al. (2017). MicroRNA-429 sensitizes pancreatic cancer cells to gemcitabine through regulation of PDCD4. Am. J. Transl. Res. 9 (11), 5048–5055. Available at: https://www.ncbi.nlm.nih.gov/pubmed/29218103.
Yu, Q., Xiu, Z., Jian, Y., Zhou, J., Chen, X., Chen, X., et al. (2022). microRNA-497 prevents pancreatic cancer stem cell gemcitabine resistance, migration, and invasion by directly targeting nuclear factor kappa B 1. Aging (Albany NY) 14 (14), 5908–5924. doi:10.18632/aging.204193
Yu, S., Wang, M., Zhang, H., Guo, X., and Qin, R. (2024). Resistance to gemcitabine is mediated by the circ_0036627/miR-145/S100A16 axis in pancreatic cancer. J. Cell Mol. Med. 28 (12), e18444. doi:10.1111/jcmm.18444
Zapletal, D., Taborska, E., Pasulka, J., Malik, R., Kubicek, K., Zanova, M., et al. (2022). Structural and functional basis of mammalian microRNA biogenesis by Dicer. Mol. Cell 82 (21), 4064–4079.e13. doi:10.1016/j.molcel.2022.10.010
Zhan, T., Chen, X., Tian, X., Han, Z., Liu, M., Zou, Y., et al. (2020). MiR-331-3p links to drug resistance of pancreatic cancer cells by activating WNT/β-Catenin signal via ST7L. Technol. Cancer Res. Treat. 19, 1533033820945801. doi:10.1177/1533033820945801
Zhan, T., Huang, X., Tian, X., Chen, X., Ding, Y., Luo, H., et al. (2018). Downregulation of MicroRNA-455-3p links to proliferation and drug resistance of pancreatic cancer cells via targeting TAZ. Mol. Ther. Nucleic Acids 10, 215–226. doi:10.1016/j.omtn.2017.12.002
Zhang, H., Zhu, C., He, Z., Chen, S., Li, L., and Sun, C. (2020). LncRNA PSMB8-AS1 contributes to pancreatic cancer progression via modulating miR-382-3p/STAT1/PD-L1 axis. J. Exp. Clin. Cancer Res. 39 (1), 179. doi:10.1186/s13046-020-01687-8
Zhang, L., Chen, Y., Dai, Y., Mou, W., Deng, P., Jin, Y., et al. (2024). Cancer-associated fibroblast-derived exosome Leptin promotes malignant biological lineage in pancreatic ductal adenocarcinoma by regulating ABL2 via miR-224-3p. Mol. Biol. Rep. 51 (1), 995. doi:10.1007/s11033-024-09928-1
Zhang, L., Liao, Y., and Tang, L. (2019). MicroRNA-34 family: a potential tumor suppressor and therapeutic candidate in cancer. J. Exp. Clin. Cancer Res. 38 (1), 53. doi:10.1186/s13046-019-1059-5
Zhao, Y., Zhao, L., Ischenko, I., Bao, Q., Schwarz, B., Niess, H., et al. (2015). Antisense inhibition of microRNA-21 and microRNA-221 in tumor-initiating stem-like cells modulates tumorigenesis, metastasis, and chemotherapy resistance in pancreatic cancer. Target Oncol. 10 (4), 535–548. doi:10.1007/s11523-015-0360-2
Zhao, Z., Shen, X., Zhang, D., Xiao, H., Kong, H., Yang, B., et al. (2021). miR-153 enhances the therapeutic effect of radiotherapy by targeting JAG1 in pancreatic cancer cells. Oncol. Lett. 21 (4), 300. doi:10.3892/ol.2021.12561
Zheng, R., Gao, F., Mao, Z., Xiao, Y., Yuan, L., Huang, Z., et al. (2023). LncRNA BCCE4 genetically enhances the PD-L1/PD-1 interaction in smoking-related bladder cancer by modulating miR-328-3p-USP18 signaling. Adv. Sci. (Weinh) 10 (30), e2303473. doi:10.1002/advs.202303473
Keywords: pancreatic cancer, microRNA, drug resistance, therapeutic targeting, chemoresistance mechanisms
Citation: Dong F, Zhou J, Wu Y, Gao Z, Li W and Song Z (2025) MicroRNAs in pancreatic cancer drug resistance: mechanisms and therapeutic potential. Front. Cell Dev. Biol. 12:1499111. doi: 10.3389/fcell.2024.1499111
Received: 20 September 2024; Accepted: 30 December 2024;
Published: 15 January 2025.
Edited by:
Andrew Liss, Harvard Medical School, United StatesReviewed by:
Cristiana Tanase, Victor Babes National Institute of Pathology (INCDVB), RomaniaCelia Cintas, The University of Manchester, United Kingdom
Copyright © 2025 Dong, Zhou, Wu, Gao, Li and Song. This is an open-access article distributed under the terms of the Creative Commons Attribution License (CC BY). The use, distribution or reproduction in other forums is permitted, provided the original author(s) and the copyright owner(s) are credited and that the original publication in this journal is cited, in accordance with accepted academic practice. No use, distribution or reproduction is permitted which does not comply with these terms.
*Correspondence: Weiwei Li, ZG9jdG9ybGkwODEyQDE2My5jb20=; Zhengwei Song, ZG9jdG9yc29uZ3p3QHpqeHUuZWR1LmNu