- 1Department of Spine Surgery and Innovative Laboratory of Orthopedics, Shenzhen Nanshan People’s Hospital, Shenzhen, Guangdong, China
- 2Orthopedic Laboratory, Orthopedic Department and Hubei Sports Medicine Center, Wuhan Fourth Hospital, Wuhan, China
Intervertebral disc degeneration (IVDD) is the leading cause of low back pain, where degeneration and death of nucleus pulposus cells within the intervertebral disc (IVD) can be obviously revealed. This degeneration can result in an imbalance in the extracellular matrix due to the loss of proteoglycans and water content, which can further lead to catabolic and anabolic dysfunction of the IVD. Recently, the dysfunction of cartilage endplate (CEP) during aging has drawn large attention due to its essential functions in contributing nutrient exchange and maintaining IVD homeostasis. Furthermore, the inflammation and disturbed homeostasis of CEP not only accelerate the degradation of nucleus pulposus extracellular matrix, but also exacerbate IVDD by causing nucleus pulposus cell death through other pathological factors. Here in this review, we summarized the possible pathological factors and the underlying mechanisms of the CEP inflammation-induced IVDD, including exosomes degeneration, CEP calcification, ferroptosis, mechanical changes, and cell senescence. Besides, changes of miRNAs, pain-related neural reflex arc and pathways associated with CEP inflammation-induced IVDD are also reviewed. In addition, new strategies specifically designed for CEP inflammation-induced IVDD are also discussed in the last section. We hope this paper can not only offer some new insights for advancing novel strategies for treating IVDD, but also serve as a valuable reference for researchers in this field.
1 Introduction
Intervertebral disc (IVD) is a kind of fibrocartilaginous joint that is composed by a central nucleus pulposus (NP) in the center and a peripheral annulus fibrosus (AF) around the NP, while the cartilage endplate (CEP) is located between the intervertebral disc and the vertebral body (Dowdell et al., 2017). Functionally, the IVD plays important roles in bearing weight, absorbing shock, buffering cushion, and maintaining stability of the spine. However, intervertebral disc degeneration (IVDD) is recognized as the culprit of low back pain, which greatly reduces the quality of human life (Maher et al., 2017). The development of IVDD is a complex pathological process involving multiple factors, including extracellular matrix (ECM) depletion, fibration and dehydration of nucleus pulposus, extensive CEP injury, and subchondral sclerosis, which jointly decrease the height of intervertebral disc (Che et al., 2020; Lu et al., 2020; Tang et al., 2019). Besides, elevated inflammatory cytokines such as IL-1 and TNF-α in the IVD are revealed to be closely associated with disc degeneration, where IL-1 is reported to directly mediate various pro-inflammatory mediators and matrix metalloproteinases (MMPs), resulting in a disturbed ECM metabolism of the intervertebral disc (Gao et al., 2024). While the activation of TNF-α can lead to the expansion of inflammation cascades, and apoptosis of nucleus pulposus cells (NPCs) can be induced through its corresponding receptors of TNFR in such inflammatory circumstance, which will further result in spine dysfunction if left untreated (Alkhatib et al., 2014; Chen et al., 2017; Kang et al., 2015; Sutovsky et al., 2017).
Cartilage endplate (CEP) is a kind of thin hyaline-like cartilage layer covering the cranial and caudal ends of NP, with its thickness estimated to be 0.1–1.6 mm. As we know, blood vessels only enter the outer space of the annulus fibrous, and the CEP thus acts as the main supply of nutrients and oxygen for inner cells of the disc by permeation (Figure 1) (Huang et al., 2014). However, persistent chronic inflammation of CEP elicited by pathological factors can lead to CEP damages, which can further result in chondrocyte aging, loss of cell phenotype, and reduced differentiation capacity by the released inflammatory cytokines due to the degradation of disc ECM (Dudli et al., 2016; Moore, 2006). In 1988, Modic et al. detected and defined the different manifestations of CEP inflammation on MRI as Modic changes, and further divided them into three types: Modic type I (inflammatory phase - T1 low signal, T2 high signal), Modic type II (fat phase - T1 high signal, T2 high signal) and Modic type III (sclerotic phase - T1 low signal, T2 low signal) (MODIC et al., 1988).
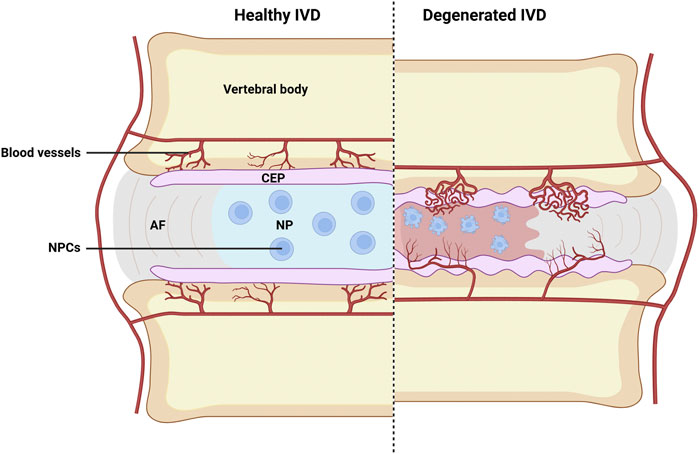
Figure 1. Schematic illustration of healthy and degenerated IVD. Where CEP destruction, abnormal infiltration of blood vessels, inflammation of nucleus pulposus, and evident NP cells apoptosis can all be observed in degenerated IVD. AF, fibrous rings; NP, nucleus pulposus; CEP, cartilage endplate; NPCs, nucleus pulposus cells. Image created with BioRender (www.biorender.com).
As the knowledge of IVDD advances, researchers find that CEP inflammation is closely related to the development of IVDD (Li et al., 2010; Wang et al., 2017; Wong et al., 2019). The disturbance of CEP will cause pathological damage to the development of IVVD, because the main supply of nutrition and oxygen for the IVD was restricted and damaged during CEP inflammation. In addition, IVDD caused by injury, inflammation, and infection will lead to CEP inflammation as well (Crockett et al., 2017; Ganko et al., 2015; Weiler et al., 2005). However, the causal relationship and underlying mechanisms between CEP inflammation and IVDD are rarely reported. To advance our knowledge on the development of IVDD, this study mainly summarizes on how CEP inflammation is interconnected to the development of IVDD.
2 Current knowledge on intervertebral disc degeneration
The volume of NP cells (NPCs) occupied in IVD only account for 1% of the IVD, but their roles are indispensable for the physiology and biomechanics of IVD (Silwal et al., 2023). NPCs are important for regulating the metabolism of NP ECM, where collagen type II (Col II) and proteoglycan are the key contents (Zhang G. Z. et al, 2021). The main function of those structures is to cushion and decentralize the pressure suffered by the spine during loading (Chang et al., 2022). Therefore, the physiological function of NP ECM is crucial for maintaining the structure and function of the IVD, as well as the development and progression of IVDD (Xing et al., 2021), because the disturbance of ECM metabolism will lead to the loss of proteoglycan and water content of the IVD (Roh et al., 2021). Many studies have shown that inflammation, oxidative stress, abnormal mechanical load, and other pathological factors are involved in the development of IVDD (Huang et al., 2023; Kang et al., 2023; Wu et al., 2022). For example, inflammatory mediators such as IL-1β can disrupt the metabolic homeostasis of the ECM (Wang et al., 2020). Mitochondrial dysfunction can also contribute to the development of IVDD by producing reactive oxygen species (ROS), where excessive ROS activates the expression of various oxidative stress biomarkers, including phospholipase and NO, which can further result in DNA damage, lipid metabolism, and protein synthesis disorders in IVD (Chen et al., 2024). Of note, abnormal mechanical stress can accelerate ECM degradation and IVDD development as well (Zhou et al., 2024).
As we know, CEP acts as a key component of the spine. The normal physiological function of CEP is important in maintaining spinal cord function and providing nutritional support for IVD (Maatta et al., 2016). The inflammation and degeneration of CEP can downregulate its physiological function, and can further lead to the development of IVDD (Ma et al., 2024). However, pathological factors that can result in inflammation and degeneration of the CEP are not clearly presented. Scholars reported that abnormal exosome synthesis, cell calcification, iron overload, abnormal mechanical load, cell senescence, and other factors are accountably involved in this pathological process, which jointly or separately destroy the metabolic balance and accelerate the development of IVDD (Figure 2). Therefore, it is particularly important to explore the pathological factors that lead to the degeneration of NPCs.
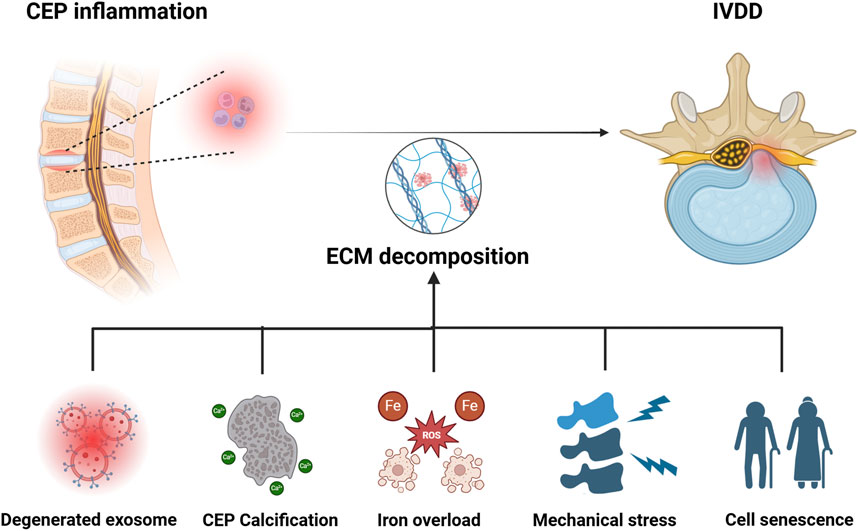
Figure 2. Pathological mechanisms of IVDD caused by CEP inflammation. Degenerated exosome, calcification of CEP, iron overload, mechanical stress, and senescence of NP cells are all involved in the development of CEP inflammation induced IVDD. Image created with BioRender (www.biorender.com).
3 Potential mechanisms underlying IVDD induced by CEP inflammation
3.1 Exosomes degeneration
Progenitor cells in human CEP can differentiate into osteoblasts, adipocytes, and chondrocytes (He et al., 2018), which are named as cartilage endplate stem cells (CESCs) by researchers. As reported, CESCs can maintain the integration of structure and function of CEP by paracrine activation of the SDF-1/CXCR4 and ERK1/2 signal pathways, which pathways are conducive for accelerating the proliferation and regeneration of NPCs. Moreover, CESCs can release a type of extracellular vesicle called CESCs-derived exosomes (Phinney and Pittenger, 2017). As demonstrated, those exosomes play important roles in intercellular communication by transferring certain proteins and RNAs, to control inflammation and prevent tissue degeneration by inhibiting cell apoptosis (Fan et al., 2020; Xu et al., 2019). What’s more, such exosomes can not only induce the CESCs to differentiate into NPCs, but also can promote the proliferation of NPCs, which altogether delay the development and progression of IVDD (Luo et al., 2021a). However, CEP inflammation significantly weakens the function of exosomes released from CESCs, and accountably aggravates the progression of IVDD (Luo et al., 2021b).
Accordingly, persistent inflammation can result in the CESCs to degenerate, which changes the contents of those secreted exosomes, where such exosomes are named as degenerated CESC-derived exosomes (D-exos) by researchers. Compared to normal CESC-derived exosomes (N-exos), the efficacy of D-exos on reversing IVDD is greatly weakened, and the mechanisms are as follows: firstly, N-exos can activate autophagy associated PI3K/Akt signaling pathways to inhibit the apoptosis of NPCs; secondly, N-exos can significantly reduce apoptotic proteins such as cleaved caspase3 and Bax in NPCs, while anti-apoptotic protein Bcl-2 is increased in such process (Luo et al., 2021b). Meanwhile, N-exos can also activate HIF-1α/Wnt signaling pathways to promote the transformation of CESCs into NPCs by an autocrine mechanism (Luo et al., 2021a). Compared to N-exos, D-exos carried fewer anti-apoptotic proteins, their ability to activate PI3K/AKT signaling pathways in NPCs and HIF-1α/Wnt signaling pathways in CESCs is also decreased. Those changes in D-exos downregulate NPCs autophagy and cause the NPCs to develop apoptosis, resulting in reduction of NPCs (Figure 3) (Luo et al., 2021b).
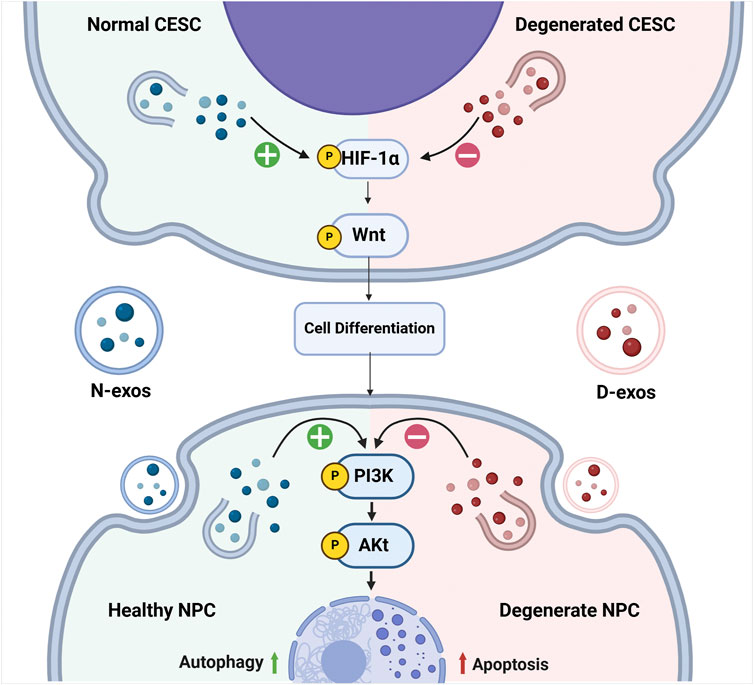
Figure 3. The changes of associated pathways elicited by CEP inflammation during the development of IVDD. Normal CESC-derived exosomes (N-exos) can effectively activate the PI3K/AKT signaling pathway in NPCs and HIF-1α/Wnt signaling pathways in CESCs, which further enhances autophagy, alleviates NPC cell apoptosis, and improves NPC differentiation. While the degenerated CESC-derived exosomes (D-exos) do the opposite, resulting in decreased number of the NPCs. Image created with BioRender (www.biorender.com).
Studies also find that N-exos can affect the progression of IVDD by their carried miRNAs. For example, miR-532-5p released by N-exos can downregulate the expression of caspase-3, caspase-9, caspase-8, and MMP-1 in NPCs, while upregulate the expression of collagen type I (Col I), collagen type II (Col II) and proteoglycan. Besides, miR-532-5p can inhibit the release of inflammatory factors such as IL-6 and IL-1β by targeting RASSF5 (Zhu et al., 2020). MiR-125-5p secreted by N-exos can inhibit the apoptosis of nucleus pulposus tissue by downregulating the gene expressions of SUV39H1, Bax, MMP13, and p62, while upregulate the gene expressions of Bcl2, ACAN, LC3-II/I. However, the expression levels of miR-532-5p and miR-125-5p are downregulated in D-exos (Chen and Jiang, 2022).
3.2 CEP calcification
Calcification of the CEP can also lead to the development of IVDD. As we mentioned previously, the degeneration of CEP can result in the release of proinflammatory cytokines, such as tumor necrosis factor and interleukins (Brisby, 2006). Moreover, those inflammatory factors can increase the risk of the CEP to calcification and chondroid tissue formation as well (Bessueille and Magne, 2015; Joshi et al., 2016). Researchers find that the TNF-α can predispose vascular smooth muscle cells (VSMCs) to form calcium deposits (Guerrero et al., 2012; Lencel et al., 2011; Masuda et al., 2013), where the release of bone morphogenetic protein 2 (BMP-2), a potent osteosynthesis factor, is revealed to accelerate the calcification of involved tissues (Nakagawa et al., 2010). Furthermore, TNF-α can also reduce the levels of extracellular inorganic pyrophosphate (PPi), which is reported to be a potent endogenous inhibitor of calcification (Lencel et al., 2011; Zhao et al., 2012). In addition, some researchers have confirmed that the inflammation of CEP is closely associated with the calcification of IVD (Joshi et al., 2016).
Besides, the calcified CEP can affect the nutrition and oxygen exchange of the IVD as well. As we described previously, the intervertebral disc is an avascular tissue structure, where capillaries originated from the vertebral body terminate at the periphery of intervertebral disc. Therefore, nutrients of the NP cells needed, such as glucose and oxygen, can only be transported by diffusion through the CEP (Sakai and Grad, 2015). Due to this anatomy of the disc, the permeability of the CEP can be significantly decreased when the CEP becomes calcified, those changes will further decrease the glucose and oxygen delivered to the IVD, and further result in intervertebral disc degeneration over time. Extracellular Ca2+ content is found to elevate after CEP calcification in the microenvironment of IVD as well. As reported, the elevated Ca2+ can activate the extracellular calcium-sensing receptor (CASR), a C-g protein-coupled receptor (GPCR), to regulate the synthesis and secretion of parathyroid hormone (Brown, 2013). While the activation of CASR is responsible for the aggravation of denatured NP cells (Grant et al., 2016), as decreased secretion and accumulation of beneficial matrix molecules, such as Col II and proteoglycans, can be observed in the NP cells during this process (Lakstins et al., 2021; Wong et al., 2019; Zhao et al., 2007). Besides, ECM synthesis-catabolism imbalance will be developed within the NP cells during CEP calcification, where more ECM is degraded than produced (DeLucca et al., 2016; Kim et al., 2009). Over time, the water content within the IVD is significantly decreased, and intervertebral disc subsidence will then occur (Figure 4) (van Uden et al., 2017).
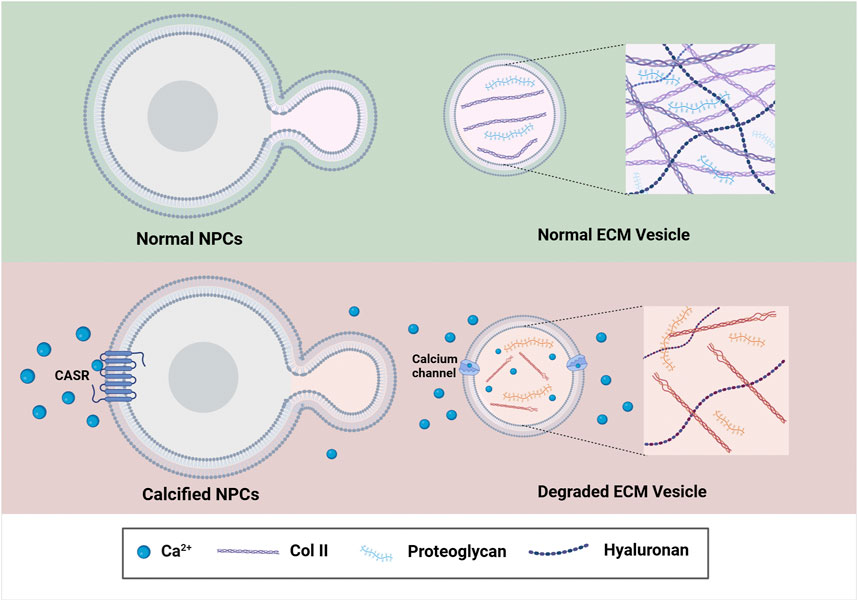
Figure 4. The underlying mechanism of CEP Calcification associated with the development of IVDD. Increased Ca2+ in the IVD microenvironment enhance the expression of CASR, which further reduces ECM synthesis by downstream signaling and finally contributes to the calcification of the IVD. Image created with BioRender (www.biorender.com).
Moreover, calcified IVDs are more likely to rupture and herniate than non-calcified IVDs and normal IVDs, because the loading stress of the spine is abnormally distributed when CEP gets calcification, which greatly increases the risk of developing vertebral fractures and fissures (Fearing et al., 2018). In addition, calcified intervertebral disc can result in caseous degeneration due to calcified deposits, which can even be spontaneously liquefied in the late stage. Calcification can also cause damage to the annulus fibrosus or surrounding soft tissue, and inflammation can further accelerate this process, which altogether lead to the development of IVD herniation (Court et al., 2018; Yu et al., 2020; Yue et al., 2016). Besides, Calcified tissue in CEP can destroy the microstructure of bone trabeculae, which is another factor that leads to endplate fracture (Crockett et al., 2017). Moreover, the calcified tissue in CEP can result in anisotropic cracks of CEP as well. Those cracks in CEP can further accelerate the loss of water content in the IVD, while simultaneously increase the flow of inflammatory cytokines and cells (Crockett et al., 2017).
3.3 Iron overload
Iron is considered as the most abundant trace element in the human body. Nowadays, various studies have proved that iron’s abnormal deposition is correlated to the development of IVDD and CEP inflammation (Jing et al., 2021a; Jing et al., 2021b; Nieuwenhuizen et al., 2013). Unfortunately, our human body lacks the ability to get rid of excess iron, which causes the irons to be gradually accumulated as we become aged (Tian et al., 2022). Recent studies report that chondrocyte senescence and degeneration are largely involved in iron toxicity caused by oxidative stress and iron overload (Li et al., 2023; Yao et al., 2021). Accordingly, the balance of iron metabolism within cells is regulated by iron transporters DMT1 and FPN1, where DMT1 serves as the transporter for taking up iron and FPN1 is responsible for facilitating the outflow of iron (Galy et al., 2023), while ferritin is responsible for storing iron (Zeidan et al., 2021). Some studies have shown that when CEP is inflamed, the inflammatory cytokines IL-6 and TNF-α can upregulate the expression of DMT1 while decreasing the expression of FPN1 in cells (Li et al., 2021; Urrutia et al., 2013), which changes significantly elevate iron depositions in the body. Meanwhile, IL-6 and TNF-α also increase the expression of hepcidin (Frazier et al., 2011), an another factor that destroys iron homeostasis (Stephenson et al., 2014).
Iron overload can produce large amounts of reactive oxygen species (ROS) by electron transfer in the mitochondrial oxidative respiratory chain (Mishima et al., 2022), where excess ROS is closely associated with mitochondrial dysfunction and DNA damage (Chen et al., 2020). Scholars have proved that iron overload can aggravate oxidative stress and mitochondrial function in a dose-dependent manner (He Q. et al, 2023), which further induces iron-dependent cell death (Ferroptosis) of the CEP and NP cells (Dixon et al., 2012). Ferroptosis is a recently discovered mode of cell death, a process characterized by lipid peroxidation catalyzed by iron ions (Tang et al., 2020). Nowadays, many reports have pointed out that ferroptosis is closely related to IVDD (Zhang P. et al, 2023; Zhu et al., 2023). In a TBHP-induced oxidative stress model, the increased changes of ferroptosis-associated marker and lipid peroxidation are positively correlated with IVD cell degeneration (Fan et al., 2023).
Moreover, excess iron in NP cells can activate transcription factor 3 (ATF3), a positive regulator of ferroptosis (Fu et al., 2021; Wang et al., 2019), while inhibit glutathione peroxidase 4 (GPX4) and cystine/glutamate antiporter SLC7A11 (xCT) (Wang W. et al, 2022; Zhang et al., 2020), which changes significantly induce ferroptotic cell death in the NP cells. Another major cause of ferroptosis is lipid peroxidation (Yang et al., 2020). In the human body, fatty acids are the major components used for the synthesis of phospholipid bilayers of cell membranes, and those components are also major substrates for energy metabolism (Bersuker et al., 2019). When Fe2+ are largely accumulated in the cytoplasm, toxic lipid ROS are produced (Doll et al., 2016), where the polyunsaturated fatty acids (PUFA) of phospholipids in the cell membrane are more likely to be bound by ROS due to its highly expressed active hydroxyl radicals (Kayagaki et al., 2021; Yan et al., 2021). Lipid peroxidation driven by free radicals produces lipid oxygen peroxides (LOOH), a enzyme that can damage the continuity of the lipid bilayer by disrupting the integration of cell membrane, thereby inducing ferroptosis of the NP cells (Fan et al., 2023).
Iron overload is also correlated with aging and degeneration of the chondrocytes (Hou et al., 2016; Jing et al., 2021a). Although abnormal iron ions have played some beneficial roles in increasing the expression of Col10 and Runx2 to promote the formation and mineralization of CEP (Wang W. et al, 2022; Yao et al., 2019). However, excessive iron deposition upregulates matrix metalloproteinases, such as Mmp3 and Mmp13 in CEP cells, which evidently reduces the expression of Sox9 and Col II (Wang W. et al, 2022), thus facilitating the breakdown of cartilage matrix and expediting the deterioration of CEP, and finally contributes to the development of IVDD (Yuan et al., 2019).
3.4 Mechanical changes
Non-physiological mechanical load is one of the important factors known to affect IVDD as well (Belavy et al., 2016; Vergari et al., 2016; Wuertz et al., 2009). While the relationship between mechanics and biology is complex, it has been established that CEP inflammation can lead to microfracture of the cartilage endplate and abnormal mechanical loading (Feng C. et al, 2018; Xiao et al., 2018; Zheng et al., 2019). As reported, The presence of inflammatory mediators such as TNF-α increase the sensitivity of the CEP to mechanical loading, thereby aggravate mechanical stresses applied to the CEP, which contribute to the development of microfractures (Din et al., 2021). Besides, the persistent production of proinflammatory substances can expand the broken endplate, or even extend to the whole NP and AF (Crockett et al., 2017; Kameda et al., 2017). In addition, inflammation increases vascular permeability of the damaged CEP, which can allow low-virulence bacteria to infiltrate into the IVD along the newly formed capillaries, and this is another factor that is associated with the development of IVDD (Hernandez et al., 2020). Moreover, the existence of inflammatory mediators like TNF-α can aggravate the outcomes of mechanical stress exerted on the IVD (Liu et al., 2023; Snuggs et al., 2021; Zhang A. et al, 2023). Under the stimulation of inflammatory factors, abnormally changed osmotic stress elicited by mechanical loading can more fiercely disrupt the F-actin structure and cell volume of the NP cells (Kletsas et al., 2014), even a complete absence of intracellular F-actin in the NP cells can be observed (Kletsas et al., 2014). By in vitro experiments, researchers reveal that those morphological and biophysical characteristics of the NP cells suffered are irreversible, even those cells are cultured in normal conditions after the discontinuation of inflammatory stimulus (Jacobsen et al., 2021; Kletsas et al., 2014).
Interestingly, recent relevant studies reveal that cells isolated from IVD tissue with inflammation show different responses to mechanical stress compared to cells isolated from normal IVD tissue (Mainardi et al., 2022; Tavakoli et al., 2020). It has been reported that normal AF cells can maintain proteoglycan production when mechanical stretch strains of low intensity (1%) and physiological frequency (1 Hz) are applied (Pratsinis et al., 2016). Besides, NP cells can observe an anabolism response under low-to-moderate intensity stretch, and only higher intensities promote a catabolism response (Fearing et al., 2018). However, Inflammatory factors such as TNF-α would alter the sensitivity of IVD to normal mechanical stress, thereby nullifying this beneficial effect under low-to-moderate mechanical stretch (Torre et al., 2018). Increased evidences have shown that stretch and inflammatory signaling are interacted in the degeneration process of AF cells (Linder et al., 2021; Ramanathan et al., 2022; Wang A. G. et al, 2022), because scholars discover that inflammatory signalings can alter the cytoskeletal mechanical transduction of the IVDs in recent studies. In AF cells, the expression of F-actin stress fiber of α-tubulin is found to be enhanced following TNF-α treatment, which may predispose the AF cells susceptible to stress of mechanical strain (Gonçalves et al., 2022). Moreover, mechanical stretches suffered by AF cells can activate inflammatory receptors such as TLR as well (Mohd Isa et al., 2022), while the changes of those receptors are demonstrated to be correlated with cytoskeletal remodeling and biomechanical alterations of the NP cells.
3.5 Cell senescence
Senescence is also another factor that leads to the development of IVDD. Currently, multiple studies have showed close relationships between senescent NP Cells and IVDD (Zhang K. et al, 2021; Zhang et al., 2024). Short-term exposure to senescence is harmless due to the clearance of the immunological effect, but long-term exposure will lead to uncontrolled chronic inflammation owing to the disrupted homeostasis of the immune system in this condition (Calcinotto et al., 2019; Herranz and Gil, 2018). As researchers reported, chronic inflammation of CEP is recognized as a characteristic feature of senescence (Baechle et al., 2023; Salvioli et al., 2023). In such pathological status, overproduced inflammatory factors such as IL-1β and TNF-α are observed and can subsequently lead to senescence of the NP cells (Capoor et al., 2021; Zhu et al., 2022). Studies have shown that endplate chondrocytes are involved in the regulation of cell proliferation, differentiation, and senescence through the Hippo-Yes-associated (YAP) pathways (Pan et al., 2021; Sladitschek-Martens et al., 2022), and the decrease of YAP1 can further stimulates the senescence of IVD cells during inflammation in the CEP (Kong et al., 2023).
Besides, increased inflammation of the cartilage endplate can result in elevated expression of SA-β-Gal, an age-related protein (Purmessur et al., 2013). TNF-α generated during inflammation also induces downregulated expressions of proteoglycan, Col I, and Col II in the NP cells as well. For instance, Kang et al. found the proportion of aging markers (e.g., P16 and p53) in TNF-α-induced NP cells were increased in an IVDD inflammation model (Li et al., 2019). In addition, senescence can also impact the cell cycle of the NP cells, where senescence induced by TNF-α is more likely to make the NP cells to stay in G0/G1 phase. While S phase is decreased during this process, indicating an occurrence of cell growth arrest. Besides, the proliferation of NP cells is also inhibited after senescence induced by TNF-α (Li et al., 2019). In addition, other pro-inflammatory mediators, such as IL-2, IL-4, IL-8, IFN-γ, and prostaglandin 2, are also responsible for aggravating the aging of the NP cells, which combinatorially lead to the development of IVDD (Huang et al., 2018; Miyagi et al., 2022; Risbud and Shapiro, 2013).
As cells becoming aged, a variety of pro-inflammatory cytokines, chemokines, growth factors, and MMPs can be secreted, which are then named as senescence-associated secretory phenotype (SASP) by scholars (Patil et al., 2019). Accordingly, increased production of MMPs can lead to hydrolysis of Col II and proteoglycan around the NP cells (Bedore et al., 2014; Qin et al., 2022), which disrupts the metabolic balance of the NP ECM, predisposing the human body easier to develop IVDD (Chen et al., 2022; Cherif et al., 2020). SASP can induce senescence of the neighboring cells by autocrine and paracrine mechanisms as well (He X. et al, 2023; Herranz and Gil, 2018). Moreover, the cell division cycle can be prolonged by SASP through an autocrine pathway, which makes the NP cells to more likely be stayed in a static phase (G0 phase) (Ji et al., 2023), resulting in decreased S and M phases (Figure 5) (Georgilis et al., 2018; Hubackova et al., 2015). Noteworthily, the cessation of cell cycle induced by SASP is often irreversible (Acosta et al., 2013). Besides, other cytokines such as IL-6, monocyte chemotactic protein-1, and IGF binding proteins can also make some contributions to the development of NP senescence by paracrine mechanisms (Freund et al., 2010; Jeon et al., 2017).
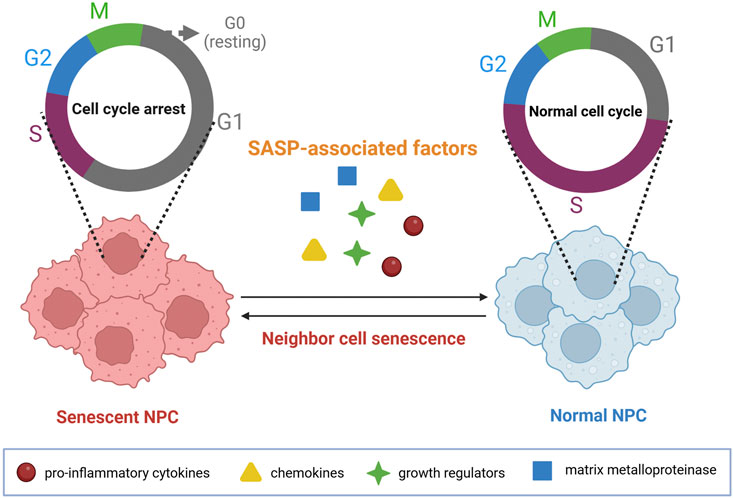
Figure 5. SASP induces cell cycle arrest within NP cells. SASP-associated factors, such as proinflammatory cytokines, chemokines, growth regulators, and matrix metalloproteinase, can significantly lead to the senescence of neighboring cells by the paracrine pathways. Image created with BioRender (www.biorender.com).
3.6 Others
As reported, miRNAs are also discovered to involve in the development of IVDD induced by CEP inflammation (Chandan et al., 2020). The expressions of miRNAs such as miR-640 and miR-194 are upregulated during IVDD, which changes evidently reduce the chondrogenic differentiation of CESCs and increase the osteogenic differentiation of CESCs (Cazzanelli and Wuertz-Kozak, 2020; Ma et al., 2024). For instance, Dong et al. found that upregulated expression of miR-640 is observed in the inflamed IVD cells, and stimulation of IVD cells with TNF-α and IL-1β can reciprocally upregulate miR-640 expression as well. In addition, miR-640 is found to be involved in NP cell apoptosis, where upregulation of MMP-3 and MMP-9 and downregulation of proteoglycan and Col II of the NP cells can be observed (Dong et al., 2019). Moreover, upregulation of miR-194 of the NP cells induced by IL-6 and TNF-α is revealed to upregulate the expression of CUL4A and CUL4B genes, which are then demonstrated to be positively correlated with the severity of IVDD (Chen et al., 2019). Except for the aforementioned miRNAs, miR-194 and miR-515 are reported to involve in the development of IVDD by degrading chondroitin sulfate synthase CHSY-1/2/3 of the NP cells (Hu et al., 2017).
In recent years, abnormal changes in pain-related neural pathways have aroused great interest in scholars, and those abnormal changes are discovered to be intimately correlated with the development of IVDD (Kim et al., 2020). As we know, substance P (SP) and calcitonin gene-related peptide (CGRP) are important neurotransmitters to regulate pain perception and transmission in the nervous system. Interestingly, positive sensory and sympathetic markers of the nervous systems are detected in degenerated IVD tissues (Sainoh et al., 2014; Song et al., 2020), where increased CGRP expression is detected in painful and degenerative discs (Ahmed et al., 2019). Blocking SP or CGRP production in dorsal root ganglion (DRG) neurons of the IVD can relieve pain symptoms significantly (He et al., 2020). Of note, TNF-α and IL-1β are considered to be the main inflammatory mediators to produce new nerve fibers that lead to IVD pain (Risbud and Shapiro, 2013). When CEP is inflamed, TNF-α and IL-1β infiltrate the annulus fibrosus through the damaged cartilage endplate, which promotes local nerve endings to infiltrate into the nucleus pulposus and induce pain by stimulating nociceptor responses (Sun et al., 2022). Then, those evoked pain sensations transmit to the DRG via generated electronic potential by nociceptors, which will then result in increased secretion and transport of neurotransmitters, such as SP and CGRP, into the corresponding levels of IVD via their corresponding receptors (Seidel et al., 2013; Wise et al., 2020). Those neurotransmitters can affect the neurons in the central nervous system as well, including the ventromedial hypothalamic nucleus (VMH) and paraventricular nucleus (PVN) of the hypothalamus, where excited VMH and PVN can reciprocally increase the activities of sympathetic nervous system and DRG, by secreting corresponding neurotransmitters (Sun et al., 2022) (Figure 6).
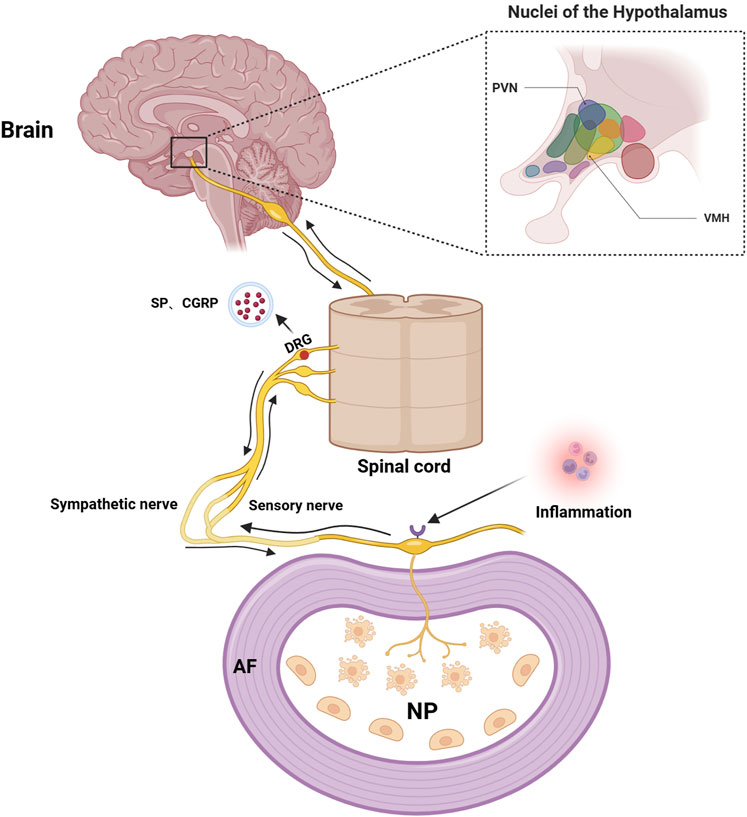
Figure 6. Schematic illustration of the IVD-DRG/sympathetic nerve-VMH/PVN axis. TNF-α and IL-1β evoke IVDD pain by reacting with their nociceptors of the local nerve endings when CEP is inflamed, which transmits pain sensations to the DRG and further increases secretion and transport of neurotransmitters into the corresponding levels of IVD via their receptors. Those neurotransmitters can then affect the neurons in the central nervous system, such as the ventromedial hypothalamic nucleus (VMH) and paraventricular nucleus (PVN) of the hypothalamus, to reciprocally regulate the IVDD pain. Image created with BioRender (www.biorender.com).
4 Perspectives and future directions
IVDD is a kind of degenerative disease of the spine, and severe social and economic burdens can be caused by this disease if left untreated. In healthy IVD, the balance between anabolism and catabolism of the ECM is important for the stability of the spine. However, pro-inflammatory factors produced by CEP inflammation significantly disrupt this balance, and ultimately result in the development of IVDD due to the loss of ECM (Crump et al., 2023; Zou et al., 2023). To what we have described previously, changes of secreted exosomes, cell calcification, iron overload, mechanical stress, and cellular senescence, are all contributing factors for the development of CEP inflammation-induced IVDD. However, strategies dedicated to treating this disease are remained to advance. Nowadays, exosomes are becoming more and more welcomed for treating degenerated diseases (Wei et al., 2019; Zhu et al., 2018), and exosomes can also be engineered to render them with special functions, which include virus transfection, ultrasound-assisted drug loading, and cointubation with certain proteins (Luo et al., 2021b). Therefore, engineered exosomes can be a promising direction for treating IVDD.
Except for exosomes, MSC transplantation has also detected beneficial roles in treating IVDD. By injecting nanofiber sponge-like microbeads with loaded MSCs, the cell phenotype of nucleus pulposus can be maintained by anti-microRNA-199a released by MSCs, where calcification can also be inhibited simultaneously (Feng et al., 2020; Hu et al., 2024). Furthermore, iron chelators (DFO), antioxidants (NAC), and ferroptosis inhibitors (Fe-1) are also good alternatives for preventing the degeneration of endplate chondrocytes that are caused by iron overload (Wang W. et al, 2022). As demonstrated, DFO prevents the downregulation of GPX4 and SLC7A11 by reducing iron load, while NAC and Fe-1 inhibit oxidative stress and ferroptosis (Jeney, 2017; Martacic et al., 2018). Infliximab is discovered to be powerful in inhibiting the production of pro-inflammatory cytokines by binding and isolating TNF-α, and thus can be another alternative for treating IVDD (Syversen et al., 2021). In addition, some Chinese medicine extracts, such as Resveratrol, can be helpful in reversing the harmful effects elicited by inflammatory cytokines (e.g., IL-1β and TNF-α) on the NP cells as well, where ROS elimination and G0/1 cell cycle promotion effects are observed (Li et al., 2019).
In addition, miRNA-based therapies for IVDD have also been developed. For example, an injectable MMP-degradable hydrogels containing miR-29a was developed for inhibiting IVD fibrosis and reversing IVDD (Feng G. et al, 2018). Interestingly, chondroitin sulfate transplantation can ameliorate the decreased chondroitin sulfate synthesis caused by miR-194 and miR-515, which further reverses the development of IVDD (Luo et al., 2023). Besides, Enhancing the function of neurotransmitters in focal environment of the IVD, such as exogenous administration of NPY or VIP, or inhibiting harmful neurotransmitters by local antagonisms of CGRP or SP, are potential approaches for future treatment of IVDD (Sun et al., 2024). Nevertheless, more treatment strategies specifically targeted to the IVDD are still needed to explore in the future.
5 Conclusion
CEP plays an important and indispensable role in maintaining the function of the intervertebral disc, as this structure not only cushions and distributes the mechanical load of the spine, but also is the key supply for providing nutrition and oxygen for the discs. However, CEP becomes inflamed when suffered from uncontrolled factors, where inflammatory factors such as IL-1β and TNF-α disrupt the metabolic balance of ECM synthesis and further lead to the degradation of intervertebral discs. Moreover, CEP inflammation can influence exosome function of CESCs, promote IVD calcification, induce iron overload of NP cells, increase IVD sensitivity to mechanical stress, and induce cellular senescence as well, which factors all accountably contribute to the development of IVDD. Meanwhile, this paper has also reviewed relevant miRNAs and pain-related neural pathways involved in this process, such as miR-640 and CGRP. At last, potential treatment strategies are also reviewed in the perspective section. We hope this review can not only provide new ideas and references for treating IVDD, but also inspire researchers in this field to develop more advanced strategies in the future.
Author contributions
HY: Writing–original draft. XC: Writing–original draft. JC: Writing–review and editing. YD: Writing–review and editing. YH: Writing–review and editing. LQ: Funding acquisition, Supervision, Writing–review and editing. JT: Funding acquisition, Supervision, Writing–review and editing. WY: Writing–review and editing.
Funding
The author(s) declare that financial support was received for the research, authorship, and/or publication of this article. The work was supported by National Natural Science Foundation of China Youth Fund Project (Grant No. 82402772 and 82302748), Guangdong Basic and Applied Basic Research Foundation of Guangdong Province-Zhongwei Biological Research Transformation Center Joint Program (Grant No. 2022A1515220036), and Major Project of Nanshan Health Committee (Grant No. NSZD2023036, NSZD2023009, NSZD2024019, and NS2024011).
Conflict of interest
The authors declare that the research was conducted in the absence of any commercial or financial relationships that could be construed as a potential conflict of interest.
Publisher’s note
All claims expressed in this article are solely those of the authors and do not necessarily represent those of their affiliated organizations, or those of the publisher, the editors and the reviewers. Any product that may be evaluated in this article, or claim that may be made by its manufacturer, is not guaranteed or endorsed by the publisher.
References
Acosta, J. C., Banito, A., Wuestefeld, T., Georgilis, A., Janich, P., Morton, J. P., et al. (2013). A complex secretory program orchestrated by the inflammasome controls paracrine senescence. Nat. Cell Biol. 15 (8), 978–990. doi:10.1038/ncb2784
Ahmed, A. S., Berg, S., Alkass, K., Druid, H., Hart, D. A., Svensson, C. I., et al. (2019). NF-κB-Associated pain-related neuropeptide expression in patients with degenerative disc disease. Int. J. Mol. Sci. 20 (3), 658–672. doi:10.3390/ijms20030658
Alkhatib, B., Rosenzweig, D. H., Krock, E., Roughley, P. J., Beckman, L., Steffen, T., et al. (2014). Acute mechanical injury of the human intervertebral disc: link to degeneration and pain. Eur. Cell Mater 28, 98–111. ; discussion 110-1. doi:10.22203/ecm.v028a08
Baechle, J. J., Chen, N., Makhijani, P., Winer, S., Furman, D., and Winer, D. A. (2023). Chronic inflammation and the hallmarks of aging. Mol. Metab. 74, 101755–101781. doi:10.1016/j.molmet.2023.101755
Bedore, J., Leask, A., and Séguin, C. A. (2014). Targeting the extracellular matrix: matricellular proteins regulate cell–extracellular matrix communication within distinct niches of the intervertebral disc. Matrix Biol. 37, 124–130. doi:10.1016/j.matbio.2014.05.005
Belavy, D. L., Albracht, K., Bruggemann, G. P., Vergroesen, P. P., and van Dieen, J. H. (2016). Can exercise positively influence the intervertebral disc? Sports Med. 46 (4), 473–485. doi:10.1007/s40279-015-0444-2
Bersuker, K., Hendricks, J. M., Li, Z., Magtanong, L., Ford, B., Tang, P. H., et al. (2019). The CoQ oxidoreductase FSP1 acts parallel to GPX4 to inhibit ferroptosis. Nature 575 (7784), 688–692. doi:10.1038/s41586-019-1705-2
Bessueille, L., and Magne, D. (2015). Inflammation: a culprit for vascular calcification in atherosclerosis and diabetes. Cell Mol. Life Sci. 72 (13), 2475–2489. doi:10.1007/s00018-015-1876-4
Brisby, H. (2006). Pathology and possible mechanisms of nervous system response to disc degeneration. Bone and Jt. Surg. 88, 68–71. doi:10.2106/JBJS.E.01282
Brown, E. M. (2013). Role of the calcium-sensing receptor in extracellular calcium homeostasis. Best. Pract. Res. Clin. Endocrinol. Metab. 27 (3), 333–343. doi:10.1016/j.beem.2013.02.006
Calcinotto, A., Kohli, J., Zagato, E., Pellegrini, L., Demaria, M., and Alimonti, A. (2019). Cellular senescence: aging, cancer, and injury. Physiol. Rev. 99 (2), 1047–1078. doi:10.1152/physrev.00020.2018
Capoor, M. N., Konieczna, A., McDowell, A., Ruzicka, F., Smrcka, M., Jancalek, R., et al. (2021). Pro-inflammatory and neurotrophic factor responses of cells derived from degenerative human intervertebral discs to the opportunistic pathogen cutibacterium acnes. Int. J. Mol. Sci. 22 (5), 2347–2363. doi:10.3390/ijms22052347
Cazzanelli, P., and Wuertz-Kozak, K. (2020). MicroRNAs in intervertebral disc degeneration, apoptosis, inflammation, and mechanobiology. Int. J. Mol. Sci. 21 (10), 3601–3616. doi:10.3390/ijms21103601
Chandan, K., Gupta, M., and Sarwat, M. (2020). Role of host and pathogen-derived MicroRNAs in immune regulation during infectious and inflammatory diseases. Front. Immunol. 10, 3081–3095. doi:10.3389/fimmu.2019.03081
Chang, H., Cai, F., Zhang, Y., Jiang, M., Yang, X., Qi, J., et al. (2022). Silencing gene-engineered injectable hydrogel microsphere for regulation of extracellular matrix metabolism balance. Small Methods 6 (4), 2101201–2101210. doi:10.1002/smtd.202101201
Che, H., Li, J., Li, Y., Ma, C., Liu, H., Qin, J., et al. (2020). p16 deficiency attenuates intervertebral disc degeneration by adjusting oxidative stress and nucleus pulposus cell cycle. Elife 9, e52570. doi:10.7554/eLife.52570
Chen, B., Zhu, R., Hu, H., Zhan, M., Wang, T., Huang, F., et al. (2022). Elimination of senescent cells by senolytics facilitates bony endplate microvessel formation and mitigates disc degeneration in aged mice. Front. Cell Dev. Biol. 10, 853688–853701. doi:10.3389/fcell.2022.853688
Chen, D., and Jiang, X. (2022). Exosomes-derived miR-125-5p from cartilage endplate stem cells regulates autophagy and ECM metabolism in nucleus pulposus by targeting SUV38H1. Exp. Cell Res. 414 (1), 113066–113076. doi:10.1016/j.yexcr.2022.113066
Chen, J., Xuan, J., Gu, Y. T., Shi, K. S., Xie, J. J., Chen, J. X., et al. (2017). Celastrol reduces IL-1β induced matrix catabolism, oxidative stress and inflammation in human nucleus pulposus cells and attenuates rat intervertebral disc degeneration in vivo. Biomed. Pharmacother. 91, 208–219. doi:10.1016/j.biopha.2017.04.093
Chen, X., Li, J., Kang, R., Klionsky, D. J., and Tang, D. (2020). Ferroptosis: machinery and regulation. Autophagy 17 (9), 2054–2081. doi:10.1080/15548627.2020.1810918
Chen, X., Zhang, A., Zhao, K., Gao, H., Shi, P., Chen, Y., et al. (2024). The role of oxidative stress in intervertebral disc degeneration: mechanisms and therapeutic implications. Ageing Res. Rev. 98, 102323. doi:10.1016/j.arr.2024.102323
Chen, Z., Han, Y., Deng, C., Chen, W., Jin, L., Chen, H., et al. (2019). Inflammation-dependent downregulation of miR-194-5p contributes to human intervertebral disc degeneration by targeting CUL4A and CUL4B. J. Cell. Physiology 234 (11), 19977–19989. doi:10.1002/jcp.28595
Cherif, H., Bisson, D. G., Mannarino, M., Rabau, O., Ouellet, J. A., and Haglund, L. (2020). Senotherapeutic drugs for human intervertebral disc degeneration and low back pain. eLife 9, 54693–54718. doi:10.7554/eLife.54693
Court, C., Mansour, E., and Bouthors, C. (2018). Thoracic disc herniation: surgical treatment. Orthop. Traumatol. Surg. Res. 104 (1S), S31–S40. doi:10.1016/j.otsr.2017.04.022
Crockett, M. T., Kelly, B. S., van Baarsel, S., and Kavanagh, E. C. (2017). Modic type 1 vertebral endplate changes: injury, inflammation, or infection? AJR Am. J. Roentgenol. 209 (1), 167–170. doi:10.2214/AJR.16.17403
Crump, K. B., Alminnawi, A., Bermudez-Lekerika, P., Compte, R., Gualdi, F., McSweeney, T., et al. (2023). Cartilaginous endplates: a comprehensive review on a neglected structure in intervertebral disc research. Jor Spine 6 (4), 1294–1316. doi:10.1002/jsp2.1294
DeLucca, J. F., Cortes, D. H., Jacobs, N. T., Vresilovic, E. J., Duncan, R. L., and Elliott, D. M. (2016). Human cartilage endplate permeability varies with degeneration and intervertebral disc site. J. Biomech. 49 (4), 550–557. doi:10.1016/j.jbiomech.2016.01.007
Din, R. U., Cheng, X., and Yang, H. (2021). Diagnostic role of magnetic resonance imaging in low back pain caused by vertebral endplate degeneration. J. Magnetic Reson. Imaging 55 (3), 755–771. doi:10.1002/jmri.27858
Dixon, S. J., Lemberg, K. M., Lamprecht, M. R., Skouta, R., Zaitsev, E. M., Gleason, C. E., et al. (2012). Ferroptosis: an iron-dependent form of nonapoptotic cell death. Cell 149 (5), 1060–1072. doi:10.1016/j.cell.2012.03.042
Doll, S., Proneth, B., Tyurina, Y. Y., Panzilius, E., Kobayashi, S., Ingold, I., et al. (2016). ACSL4 dictates ferroptosis sensitivity by shaping cellular lipid composition. Nat. Chem. Biol. 13 (1), 91–98. doi:10.1038/nchembio.2239
Dong, W., Liu, J., Lv, Y., Wang, F., Liu, T., Sun, S., et al. (2019). miR-640 aggravates intervertebral disc degeneration via NF-κB and WNT signalling pathway. Cell Prolif. 52 (5), 12664–12677. doi:10.1111/cpr.12664
Dowdell, J., Erwin, M., Choma, T., Vaccaro, A., Iatridis, J., and Cho, S. K. (2017). Intervertebral disk degeneration and repair. Neurosurgery 80 (3S), S46–S54. doi:10.1093/neuros/nyw078
Dudli, S., Fields, A. J., Samartzis, D., Karppinen, J., and Lotz, J. C. (2016). Pathobiology of modic changes. Eur. Spine J. 25 (11), 3723–3734. doi:10.1007/s00586-016-4459-7
Fan, C., Chu, G., Yu, Z., Ji, Z., Kong, F., Yao, L., et al. (2023). The role of ferroptosis in intervertebral disc degeneration. Front. Cell Dev. Biol. 11, 1219840. doi:10.3389/fcell.2023.1219840
Fan, C., Zhang, E., Joshi, J., Yang, J., Zhang, J., and Zhu, W. (2020). Utilization of human induced pluripotent stem cells for cardiac repair. Front. Cell Dev. Biol. 8, 36. doi:10.3389/fcell.2020.00036
Fearing, B. V., Hernandez, P. A., Setton, L. A., and Chahine, N. O. (2018). Mechanotransduction and cell biomechanics of the intervertebral disc. JOR Spine 1 (3), 1026–1045. doi:10.1002/jsp2.1026
Feng, C., Liu, M., Fan, X., Yang, M., Liu, H., and Zhou, Y. (2018). Intermittent cyclic mechanical tension altered the microRNA expression profile of human cartilage endplate chondrocytes. Mol. Med. Rep. 17 (4), 5238–5246. doi:10.3892/mmr.2018.8517
Feng, G., Zha, Z., Huang, Y., Li, J., Wang, Y., Ke, W., et al. (2018). Sustained and bioresponsive two-stage delivery of therapeutic miRNA via polyplex micelle-loaded injectable hydrogels for inhibition of intervertebral disc fibrosis. Adv. Healthc. Mater 7 (21), e1800623. doi:10.1002/adhm.201800623
Feng, G., Zhang, Z., Dang, M., Rambhia, K. J., and Ma, P. X. (2020). Nanofibrous spongy microspheres to deliver rabbit mesenchymal stem cells and anti-miR-199a to regenerate nucleus pulposus and prevent calcification. Biomaterials 256, 120213–120228. doi:10.1016/j.biomaterials.2020.120213
Frazier, M. D., Mamo, L. B., Ghio, A. J., and Turi, J. L. (2011). Hepcidin expression in human airway epithelial cells is regulated by interferon-γ. Respir. Res. 12, 100. doi:10.1186/1465-9921-12-100
Freund, A., Orjalo, A. V., Desprez, P.-Y., and Campisi, J. (2010). Inflammatory networks during cellular senescence: causes and consequences. Trends Mol. Med. 16 (5), 238–246. doi:10.1016/j.molmed.2010.03.003
Fu, D., Wang, C., Yu, L., and Yu, R. (2021). Induction of ferroptosis by ATF3 elevation alleviates cisplatin resistance in gastric cancer by restraining Nrf2/Keap1/xCT signaling. Cell. and Mol. Biol. Lett. 26 (1), 26. doi:10.1186/s11658-021-00271-y
Galy, B., Conrad, M., and Muckenthaler, M. (2023). Mechanisms controlling cellular and systemic iron homeostasis. Nat. Rev. Mol. Cell Biol. 25 (2), 133–155. doi:10.1038/s41580-023-00648-1
Ganko, R., Rao, P. J., Phan, K., and Mobbs, R. J. (2015). Can bacterial infection by low virulent organisms be a plausible cause for symptomatic disc degeneration? A systematic review. Spine (Phila Pa 1976) 40 (10), E587–E592. doi:10.1097/BRS.0000000000000832
Gao, S., Wang, C., Qi, L., Liang, S., Qu, X., Liu, W., et al. (2024). Bushen huoxue formula inhibits IL-1β-induced apoptosis and extracellular matrix degradation in the nucleus pulposus cells and improves intervertebral disc degeneration in rats. J. Inflamm. Res. 17, 121–136. doi:10.2147/JIR.S431609
Georgilis, A., Klotz, S., Hanley, C. J., Herranz, N., Weirich, B., Morancho, B., et al. (2018). PTBP1-Mediated alternative splicing regulates the inflammatory secretome and the pro-tumorigenic effects of senescent cells. Cancer Cell 34 (1), 85–102.e9. doi:10.1016/j.ccell.2018.06.007
Gonçalves, R. M., Saggese, T., Yong, Z., Ferreira, J. R., Ignatius, A., Wilke, H.-J., et al. (2022). Interleukin-1β more than mechanical loading induces a degenerative phenotype in human annulus fibrosus cells, partially impaired by anti-proteolytic activity of mesenchymal stem cell secretome. Front. Bioeng. Biotechnol. 9, 802789–802801. doi:10.3389/fbioe.2021.802789
Grant, M. P., Epure, L. M., Bokhari, R., Roughley, P., Antoniou, J., and Mwale, F. (2016). Human cartilaginous endplate degeneration is induced by calcium and the extracellular calcium-sensing receptor in the intervertebral disc. Eur. Cell Mater 32, 137–151. doi:10.22203/ecm.v032a09
Guerrero, F., Montes de Oca, A., Aguilera-Tejero, E., Zafra, R., Rodriguez, M., and Lopez, I. (2012). The effect of vitamin D derivatives on vascular calcification associated with inflammation. Nephrol. Dial. Transpl. 27 (6), 2206–2212. doi:10.1093/ndt/gfr555
He, M., Pang, J., Sun, H., Zheng, G., Lin, Y., and Ge, W. (2020). Overexpression of TIMP3 inhibits discogenic pain by suppressing angiogenesis and the expression of substance P in nucleus pulposus. Mol. Med. Rep. 21, 1163–1171. doi:10.3892/mmr.2020.10922
He, Q., Yang, J., Pan, Z., Zhang, G., Chen, B., Li, S., et al. (2023). Biochanin A protects against iron overload associated knee osteoarthritis via regulating iron levels and NRF2/System xc-/GPX4 axis. Biomed. and Pharmacother. 157, 113915. doi:10.1016/j.biopha.2022.113915
He, X., Hu, W., Zhang, Y., Chen, M., Ding, Y., Yang, H., et al. (2023). Cellular senescence in skeletal disease: mechanisms and treatment. Cell. and Mol. Biol. Lett. 28 (1), 88–108. doi:10.1186/s11658-023-00501-5
He, Z., Jia, M., Yu, Y., Yuan, C., and Wang, J. (2018). Roles of SDF-1/CXCR4 axis in cartilage endplate stem cells mediated promotion of nucleus pulposus cells proliferation. Biochem. Biophys. Res. Commun. 506 (1), 94–101. doi:10.1016/j.bbrc.2018.10.069
Hernandez, P. A., Jacobsen, T. D., and Chahine, N. O. (2020). Actomyosin contractility confers mechanoprotection against TNFα-induced disruption of the intervertebral disc. Sci. Adv. 6 (34), eaba2368–14. doi:10.1126/sciadv.aba2368
Herranz, N., and Gil, J. (2018). Mechanisms and functions of cellular senescence. J. Clin. Investigation 128 (4), 1238–1246. doi:10.1172/JCI95148
Hou, W., Xie, Y., Song, X., Sun, X., Lotze, M. T., Zeh, H. J., et al. (2016). Autophagy promotes ferroptosis by degradation of ferritin. Autophagy 12 (8), 1425–1428. doi:10.1080/15548627.2016.1187366
Hu, B., Zhou, C. X., Tian, Y., Cao, P., Chen, H., Shi, C., et al. (2017). Inflammatory microRNA-194 and -515 attenuate the biosynthesis of chondroitin sulfate during human intervertebral disc degeneration. Oncotarget 8 (30), 49303–49317. doi:10.18632/oncotarget.17571
Hu, S., Zhu, M., Xing, H., Xue, Y., Li, J., Wang, Z., et al. (2024). Thread-structural microneedles loaded with engineered exosomes for annulus fibrosus repair by regulating mitophagy recovery and extracellular matrix homeostasis. Bioact. Mater. 37, 1–13. doi:10.1016/j.bioactmat.2024.03.006
Huang, S.-J., Yan, J.-Q., Luo, H., Zhou, L.-Y., and Luo, J.-G. (2018). IL-33/ST2 signaling contributes to radicular pain by modulating MAPK and NF-κB activation and inflammatory mediator expression in the spinal cord in rat models of noncompressive lumber disk herniation. J. Neuroinflammation 15 (1), 12–24. doi:10.1186/s12974-017-1021-4
Huang, Y. C., Urban, J. P., and Luk, K. D. (2014). Intervertebral disc regeneration: do nutrients lead the way? Nat. Rev. Rheumatol. 10 (9), 561–566. doi:10.1038/nrrheum.2014.91
Huang, Z. N., Wang, Z. Y., Cheng, X. F., Huang, Z. Z., Han, Y. L., Cui, Y. Z., et al. (2023). Melatonin alleviates oxidative stress-induced injury to nucleus pulposus-derived mesenchymal stem cells through activating PI3K/Akt pathway. J. Orthop. Transl. 43, 66–84. doi:10.1016/j.jot.2023.10.002
Hubackova, S., Kucerova, A., Michlits, G., Kyjacova, L., Reinis, M., Korolov, O., et al. (2015). IFNγ induces oxidative stress, DNA damage and tumor cell senescence via TGFβ/SMAD signaling-dependent induction of Nox4 and suppression of ANT2. Oncogene 35 (10), 1236–1249. doi:10.1038/onc.2015.162
Jacobsen, T. D., Hernandez, P. A., and Chahine, N. O. (2021). Inhibition of toll-like receptor 4 protects against inflammation-induced mechanobiological alterations to intervertebral disc cells. Eur. Cells Mater. 41, 576–591. doi:10.22203/eCM.v041a37
Jeney, V. (2017). Clinical impact and cellular mechanisms of iron overload-associated bone loss. Front. Pharmacol. 8, 77–88. doi:10.3389/fphar.2017.00077
Jeon, O. H., Kim, C., Laberge, R.-M., Demaria, M., Rathod, S., Vasserot, A. P., et al. (2017). Local clearance of senescent cells attenuates the development of post-traumatic osteoarthritis and creates a pro-regenerative environment. Nat. Med. 23 (6), 775–781. doi:10.1038/nm.4324
Ji, S., Xiong, M., Chen, H., Liu, Y., Zhou, L., Hong, Y., et al. (2023). Cellular rejuvenation: molecular mechanisms and potential therapeutic interventions for diseases. Signal Transduct. Target. Ther. 8 (1), 116–155. doi:10.1038/s41392-023-01343-5
Jing, X., Du, T., Li, T., Yang, X., Wang, G., Liu, X., et al. (2021a). The detrimental effect of iron on OA chondrocytes: importance of pro-inflammatory cytokines induced iron influx and oxidative stress. J. Cell. Mol. Med. 25 (12), 5671–5680. doi:10.1111/jcmm.16581
Jing, X., Lin, J., Du, T., Jiang, Z., Li, T., Wang, G., et al. (2021b). Iron overload is associated with accelerated progression of osteoarthritis: the role of DMT1 mediated iron homeostasis. Front. Cell Dev. Biol. 8, 594509. doi:10.3389/fcell.2020.594509
Joshi, F. R., Rajani, N. K., Abt, M., Woodward, M., Bucerius, J., Mani, V., et al. (2016). Does vascular calcification accelerate inflammation? a substudy of the dal-PLAQUE trial. J. Am. Coll. Cardiol. 67 (1), 69–78. doi:10.1016/j.jacc.2015.10.050
Kameda, T., Sekiguchi, M., Kaneuchi, Y., and Konno, S.-i. (2017). Investigation of the effect of diabetes on radiculopathy induced by nucleus pulposus application to the DRG in a spontaneously diabetic rat model. Spine 42 (23), 1749–1756. doi:10.1097/BRS.0000000000002299
Kang, L., Zhang, H., Jia, C., Zhang, R., and Shen, C. (2023). Epigenetic modifications of inflammation in intervertebral disc degeneration. Ageing Res. Rev. 87, 101902. doi:10.1016/j.arr.2023.101902
Kang, R., Li, H., Rickers, K., Ringgaard, S., Xie, L., and Bunger, C. (2015). Intervertebral disc degenerative changes after intradiscal injection of TNF-α in a porcine model. Eur. Spine J. 24 (9), 2010–2016. doi:10.1007/s00586-015-3926-x
Kayagaki, N., Kornfeld, O. S., Lee, B. L., Stowe, I. B., O’Rourke, K., Li, Q., et al. (2021). NINJ1 mediates plasma membrane rupture during lytic cell death. Nature 591 (7848), 131–136. doi:10.1038/s41586-021-03218-7
Kim, H. S., Wu, P. H., and Jang, I.-T. (2020). Lumbar degenerative disease Part 1: anatomy and pathophysiology of intervertebral discogenic pain and radiofrequency ablation of basivertebral and sinuvertebral nerve treatment for chronic discogenic back pain: a prospective case series and review of literature. Int. J. Mol. Sci. 21 (4), 1483–1510. doi:10.3390/ijms21041483
Kim, K. W., Chung, H. N., Ha, K. Y., Lee, J. S., and Kim, Y. Y. (2009). Senescence mechanisms of nucleus pulposus chondrocytes in human intervertebral discs. Spine J. 9 (8), 658–666. doi:10.1016/j.spinee.2009.04.018
Kletsas, D., Maidhof, R., Jacobsen, T., Papatheodorou, A., and Chahine, N. O. (2014). Inflammation induces irreversible biophysical changes in isolated nucleus pulposus cells. PLoS ONE 9 (6), e99621. doi:10.1371/journal.pone.0099621
Kong, L., Xie, Y. S., Ma, X. D., Huang, Y., and Shang, X. F. (2023). Mechanism of YAP1 in the senescence and degeneration of endplate chondrocytes induced by intermittent cyclic mechanical tension. J. Orthop. Surg. Res. 18 (1), 229. doi:10.1186/s13018-023-03704-w
Lakstins, K., Arnold, L., Gunsch, G., Flanigan, D., Khan, S., Gadde, N., et al. (2021). Characterization of the human intervertebral disc cartilage endplate at the molecular, cell, and tissue levels. J. Orthop. Res. 39 (9), 1898–1907. doi:10.1002/jor.24854
Lencel, P., Delplace, S., Pilet, P., Leterme, D., Miellot, F., Sourice, S., et al. (2011). Cell-specific effects of TNF-α and IL-1β on alkaline phosphatase: implication for syndesmophyte formation and vascular calcification. Lab. Invest. 91 (10), 1434–1442. doi:10.1038/labinvest.2011.83
Li, F. C., Zhang, N., Chen, W. S., and Chen, Q. X. (2010). Endplate degeneration may be the origination of the vacuum phenomenon in intervertebral discs. Med. Hypotheses 75 (2), 169–171. doi:10.1016/j.mehy.2010.02.012
Li, L., Acioglu, C., Heary, R. F., and Elkabes, S. (2021). Role of astroglial toll-like receptors (TLRs) in central nervous system infections, injury and neurodegenerative diseases. Brain, Behav. Immun. 91, 740–755. doi:10.1016/j.bbi.2020.10.007
Li, S., He, Q., Chen, B., Zeng, J., Dou, X., Pan, Z., et al. (2023). Cardamonin protects against iron overload induced arthritis by attenuating ROS production and NLRP3 inflammasome activation via the SIRT1/p38MAPK signaling pathway. Sci. Rep. 13 (1), 13744. doi:10.1038/s41598-023-40930-y
Li, X., Lin, F., Wu, Y., Liu, N., Wang, J., Chen, R., et al. (2019). Resveratrol attenuates inflammation environment-induced nucleus pulposus cell senescence in vitro. Biosci. Rep. 39 (5), BSR20190126–36. doi:10.1042/BSR20190126
Linder, T., Papaplioura, E., Ogurlu, D., Geyrhofer, S., Hummelbrunner, S., Schachner, D., et al. (2021). Investigation of leoligin derivatives as NF-κΒ inhibitory agents. Biomedicines 10 (1), 62–77. doi:10.3390/biomedicines10010062
Liu, C., Gao, X., Lou, J., Li, H., Chen, Y., Chen, M., et al. (2023). Aberrant mechanical loading induces annulus fibrosus cells apoptosis in intervertebral disc degeneration via mechanosensitive ion channel Piezo1. Arthritis Res. and Ther. 25 (1), 117. doi:10.1186/s13075-023-03093-9
Lu, Y., Zhou, L., He, S., Ren, H. L., Zhou, N., and Hu, Z. M. (2020). Lycopene alleviates disc degeneration under oxidative stress through the Nrf2 signaling pathway. Mol. Cell Probes 51, 101559. doi:10.1016/j.mcp.2020.101559
Luo, H., Wang, Z., He, Z., Ling, Z., Wang, H., Zhu, J., et al. (2023). Injectable chondroitin sulfate-grafted self-antioxidant hydrogels ameliorate nucleus pulposus degeneration against overactive inflammation. Biomater. Sci. 11 (10), 3629–3644. doi:10.1039/d3bm00359k
Luo, L., Gong, J., Zhang, H., Qin, J., Li, C., Zhang, J., et al. (2021a). Cartilage endplate stem cells transdifferentiate into nucleus pulposus cells via autocrine exosomes. Front. Cell Dev. Biol. 9, 648201–648215. doi:10.3389/fcell.2021.648201
Luo, L., Jian, X., Sun, H., Qin, J., Wang, Y., Zhang, J., et al. (2021b). Cartilage endplate stem cells inhibit intervertebral disc degeneration by releasing exosomes to nucleus pulposus cells to activate Akt/autophagy. Stem Cells 39 (4), 467–481. doi:10.1002/stem.3322
Ma, Z., Liu, X., Zhang, M., Wu, Z., Zhang, X., Li, S., et al. (2024). Research progress on the role of cartilage endplate in intervertebral disc degeneration. Cell Biochem. Funct. 42 (7), e4118. doi:10.1002/cbf.4118
Maatta, J. H., MacGregor, A., Karppinen, J., and Williams, F. M. (2016). The relationship between Modic changes and intervertebral disc degeneration. BMC Musculoskelet. Disord. 17 (1), 371. doi:10.1186/s12891-016-1198-1
Maher, C., Underwood, M., and Buchbinder, R. (2017). Non-specific low back pain. Lancet 389 (10070), 736–747. doi:10.1016/S0140-6736(16)30970-9
Mainardi, A., Cambria, E., Occhetta, P., Martin, I., Barbero, A., Schären, S., et al. (2022). Intervertebral disc-on-a-chip as advanced in vitro model for mechanobiology research and drug testing: a review and perspective. Front. Bioeng. Biotechnol. 9, 826867–826885. doi:10.3389/fbioe.2021.826867
Martacic, J., Filipovic, M. K., Borozan, S., Cvetkovic, Z., Popovic, T., Arsic, A., et al. (2018). N-acetyl-l-cysteine protects dental tissue stem cells against oxidative stress in vitro. Clin. Oral Investig. 22 (8), 2897–2903. doi:10.1007/s00784-018-2377-2
Masuda, M., Miyazaki-Anzai, S., Levi, M., Ting, T. C., and Miyazaki, M. (2013). PERK-eIF2α-ATF4-CHOP signaling contributes to TNFα-induced vascular calcification. J. Am. Heart Assoc. 2 (5), e000238. doi:10.1161/JAHA.113.000238
Mishima, E., Ito, J., Wu, Z., Nakamura, T., Wahida, A., Doll, S., et al. (2022). A non-canonical vitamin K cycle is a potent ferroptosis suppressor. Nature 608 (7924), 778–783. doi:10.1038/s41586-022-05022-3
Miyagi, M., Uchida, K., Inoue, S., Takano, S., Nakawaki, M., Kawakubo, A., et al. (2022). A high body mass index and the vacuum phenomenon upregulate pain-related molecules in human degenerated intervertebral discs. Int. J. Mol. Sci. 23 (6), 2973–2984. doi:10.3390/ijms23062973
Modic, M. T., Steinberg, P. M., Ross, J. S., Masaryk, T. J., and Carter, J. R. (1988). Degenerative disk disease: assessment of changes in vertebral body marrow with MR imaging. Radiology 166, 193–199. doi:10.1148/radiology.166.1.3336678
Mohd Isa, I. L., Teoh, S. L., Mohd Nor, N. H., and Mokhtar, S. A. (2022). Discogenic low back pain: anatomy, pathophysiology and treatments of intervertebral disc degeneration. Int. J. Mol. Sci. 24 (1), 208–224. doi:10.3390/ijms24010208
Moore, R. J. (2006). The vertebral endplate: disc degeneration, disc regeneration. Eur. Spine J. 15 (Suppl. 3), S333–S337. doi:10.1007/s00586-006-0170-4
Nakagawa, Y., Ikeda, K., Akakabe, Y., Koide, M., Uraoka, M., Yutaka, K. T., et al. (2010). Paracrine osteogenic signals via bone morphogenetic protein-2 accelerate the atherosclerotic intimal calcification in vivo. Arterioscler. Thromb. Vasc. Biol. 30 (10), 1908–1915. doi:10.1161/ATVBAHA.110.206185
Nieuwenhuizen, L., Schutgens, R. E. G., van Asbeck, B. S., Wenting, M. J., van Veghel, K., Roosendaal, G., et al. (2013). Identification and expression of iron regulators in human synovium: evidence for upregulation in haemophilic arthropathy compared to rheumatoid arthritis, osteoarthritis, and healthy controls. Haemophilia 19 (4), e218–e227. doi:10.1111/hae.12208
Pan, X., Wu, B., Fan, X., Xu, G., Ou, C., and Chen, M. (2021). YAP accelerates vascular senescence via blocking autophagic flux and activating mTOR. J. Cell Mol. Med. 25 (1), 170–183. doi:10.1111/jcmm.15902
Patil, P., Falabella, M., Saeed, A., Lee, D., Kaufman, B., Shiva, S., et al. (2019). Oxidative stress-induced senescence markedly increases disc cell bioenergetics. Mech. Ageing Dev. 180, 97–106. doi:10.1016/j.mad.2019.04.006
Phinney, D. G., and Pittenger, M. F. (2017). Concise review: MSC-derived exosomes for cell-free therapy. Stem Cells 35 (4), 851–858. doi:10.1002/stem.2575
Pratsinis, H., Papadopoulou, A., Neidlinger-Wilke, C., Brayda-Bruno, M., Wilke, H. J., and Kletsas, D. (2016). Cyclic tensile stress of human annulus fibrosus cells induces MAPK activation: involvement in proinflammatory gene expression. Osteoarthr. Cartil. 24 (4), 679–687. doi:10.1016/j.joca.2015.11.022
Purmessur, D., Walter, B. A., Roughley, P. J., Laudier, D. M., Hecht, A. C., and Iatridis, J. (2013). A role for TNFα in intervertebral disc degeneration: a non-recoverable catabolic shift. Biochem. Biophysical Res. Commun. 433 (1), 151–156. doi:10.1016/j.bbrc.2013.02.034
Qin, S., Wang, Y., Li, L., Liu, J., Xiao, C., Duan, D., et al. (2022). Early-life vitamin B12 orchestrates lipid peroxidation to ensure reproductive success via SBP-1/SREBP1 in Caenorhabditis elegans. Cell Rep. 40 (12), 111381–111397. doi:10.1016/j.celrep.2022.111381
Ramanathan, R., Firdous, A., Dong, Q., Wang, D., Lee, J., Vo, N., et al. (2022). Investigation into the anti-inflammatory properties of metformin in intervertebral disc cells. Jor Spine 5 (2), 1197–1204. doi:10.1002/jsp2.1197
Risbud, M. V., and Shapiro, I. M. (2013). Role of cytokines in intervertebral disc degeneration: pain and disc content. Nat. Rev. Rheumatol. 10 (1), 44–56. doi:10.1038/nrrheum.2013.160
Roh, E., Darai, A., Kyung, J., Choi, H., Kwon, S., Bhujel, B., et al. (2021). Genetic therapy for intervertebral disc degeneration. Int. J. Mol. Sci. 22 (4), 1579–1592. doi:10.3390/ijms22041579
Sainoh, T., Sakuma, Y., Miyagi, M., Orita, S., Yamauchi, K., Inoue, G., et al. (2014). Efficacy of anti–nerve growth factor therapy for discogenic neck pain in rats. Spine 39 (13), E757–E762. doi:10.1097/BRS.0000000000000340
Sakai, D., and Grad, S. (2015). Advancing the cellular and molecular therapy for intervertebral disc disease. Adv. Drug Deliv. Rev. 84, 159–171. doi:10.1016/j.addr.2014.06.009
Salvioli, S., Basile, M. S., Bencivenga, L., Carrino, S., Conte, M., Damanti, S., et al. (2023). Biomarkers of aging in frailty and age-associated disorders: state of the art and future perspective. Ageing Res. Rev. 91, 102044–102067. doi:10.1016/j.arr.2023.102044
Seidel, M. F., Wise, B. L., and Lane, N. E. (2013). Nerve growth factor: an update on the science and therapy. Osteoarthr. Cartil. 21 (9), 1223–1228. doi:10.1016/j.joca.2013.06.004
Silwal, P., Nguyen-Thai, A. M., Mohammad, H. A., Wang, Y., Robbins, P. D., Lee, J. Y., et al. (2023). Cellular senescence in intervertebral disc aging and degeneration: molecular mechanisms and potential therapeutic opportunities. Biomolecules 13 (4), 686–715. doi:10.3390/biom13040686
Sladitschek-Martens, H. L., Guarnieri, A., Brumana, G., Zanconato, F., Battilana, G., Xiccato, R. L., et al. (2022). YAP/TAZ activity in stromal cells prevents ageing by controlling cGAS-STING. Nature 607 (7920), 790–798. doi:10.1038/s41586-022-04924-6
Snuggs, J. W., Bunning, R. A. D., and Le Maitre, C. L. (2021). Osmotic adaptation of nucleus pulposus cells: the role of aquaporin 1, aquaporin 4 and transient receptor potential vanilloid 4. Eur. Cells Mater. 41, 121–141. doi:10.22203/eCM.v041a09
Song, X.-X., Jin, L.-Y., Li, X.-F., Luo, Y., and Yu, B.-W. (2020). Substance P mediates estrogen modulation proinflammatory cytokines release in intervertebral disc. Inflammation 44 (2), 506–517. doi:10.1007/s10753-020-01347-1
Stephenson, E., Nathoo, N., Mahjoub, Y., Dunn, J. F., and Yong, V. W. (2014). Iron in multiple sclerosis: roles in neurodegeneration and repair. Nat. Rev. Neurol. 10 (8), 459–468. doi:10.1038/nrneurol.2014.118
Sun, K., Jiang, J., Wang, Y., Sun, X., Zhu, J., Xu, X., et al. (2022). The role of nerve fibers and their neurotransmitters in regulating intervertebral disc degeneration. Ageing Res. Rev. 81, 101733–101751. doi:10.1016/j.arr.2022.101733
Sun, K., Sun, J., Yan, C., Sun, J., Xu, X., and Shi, J. (2024). Sympathetic neurotransmitter, VIP, delays intervertebral disc degeneration via FGF18/FGFR2-mediated activation of akt signaling pathway. Adv. Biol. (Weinh) 8 (3), e2300250. doi:10.1002/adbi.202300250
Sutovsky, J., Benco, M., Sutovska, M., Kocmalova, M., Pappova, L., Miklusica, J., et al. (2017). Cytokine and chemokine profile changes in patients with lower segment lumbar degenerative spondylolisthesis. Int. J. Surg. 43, 163–170. doi:10.1016/j.ijsu.2017.06.024
Syversen, S. W., Jørgensen, K. K., Goll, G. L., Brun, M. K., Sandanger, Ø., Bjørlykke, K. H., et al. (2021). Effect of therapeutic drug monitoring vs standard therapy during maintenance infliximab therapy on disease control in patients with immune-mediated inflammatory diseases: a randomized clinical trial. JAMA 326 (23), 2375–2384. doi:10.1001/jama.2021.21316
Tang, D., Chen, X., Kang, R., and Kroemer, G. (2020). Ferroptosis: molecular mechanisms and health implications. Cell Res. 31 (2), 107–125. doi:10.1038/s41422-020-00441-1
Tang, Z., Hu, B., Zang, F., Wang, J., Zhang, X., and Chen, H. (2019). Nrf2 drives oxidative stress-induced autophagy in nucleus pulposus cells via a Keap1/Nrf2/p62 feedback loop to protect intervertebral disc from degeneration. Cell Death Dis. 10 (7), 510. doi:10.1038/s41419-019-1701-3
Tavakoli, J., Diwan, A. D., and Tipper, J. L. (2020). Advanced strategies for the regeneration of lumbar disc annulus fibrosus. Int. J. Mol. Sci. 21 (14), 4889–4908. doi:10.3390/ijms21144889
Tian, Y., Tian, Y., Yuan, Z., Zeng, Y., Wang, S., Fan, X., et al. (2022). Iron metabolism in aging and age-related diseases. Int. J. Mol. Sci. 23 (7), 3612. doi:10.3390/ijms23073612
Torre, O. M., Mroz, V., Bartelstein, M. K., Huang, A. H., and Iatridis, J. C. (2018). Annulus fibrosus cell phenotypes in homeostasis and injury: implications for regenerative strategies. Ann. N. Y. Acad. Sci. 1442 (1), 61–78. doi:10.1111/nyas.13964
Urrutia, P., Aguirre, P., Esparza, A., Tapia, V., Mena, N. P., Arredondo, M., et al. (2013). Inflammation alters the expression of DMT1, FPN1 and hepcidin, and it causes iron accumulation in central nervous system cells. J. Neurochem. 126 (4), 541–549. doi:10.1111/jnc.12244
van Uden, S., Silva-Correia, J., Oliveira, J. M., and Reis, R. L. (2017). Current strategies for treatment of intervertebral disc degeneration: substitution and regeneration possibilities. Biomater. Res. 21, 22. doi:10.1186/s40824-017-0106-6
Vergari, C., Mansfield, J., Meakin, J. R., and Winlove, P. C. (2016). Lamellar and fibre bundle mechanics of the annulus fibrosus in bovine intervertebral disc. Acta Biomater. 37, 14–20. doi:10.1016/j.actbio.2016.04.002
Wang, A. G., Son, M., Kenna, E., Thom, N., and Tay, S. (2022). NF-κB memory coordinates transcriptional responses to dynamic inflammatory stimuli. Cell Rep. 40 (7), 111159–111174. doi:10.1016/j.celrep.2022.111159
Wang, F., Gao, Z.-X., Cai, F., Sinkemani, A., Xie, Z.-Y., Shi, R., et al. (2017). Formation, function, and exhaustion of notochordal cytoplasmic vacuoles within intervertebral disc: current understanding and speculation. Oncotarget 8, 57800–57812. doi:10.18632/oncotarget.18101
Wang, L., Gu, Y., Zhao, H., Chen, R., Chen, W., Qi, H., et al. (2020). Dioscin attenuates interleukin 1β (IL-1β)-Induced catabolism and apoptosis via modulating the toll-like receptor 4 (TLR4)/Nuclear factor kappa B (NF-κB) signaling in human nucleus pulposus cells. Med. Sci. Monit. 26, e923386. doi:10.12659/MSM.923386
Wang, L., Liu, Y., Du, T., Yang, H., Lei, L., Guo, M., et al. (2019). ATF3 promotes erastin-induced ferroptosis by suppressing system Xc<sup/>. Cell Death and Differ. 27 (2), 662–675. doi:10.1038/s41418-019-0380-z
Wang, W., Jing, X., Du, T., Ren, J., Liu, X., Chen, F., et al. (2022). Iron overload promotes intervertebral disc degeneration via inducing oxidative stress and ferroptosis in endplate chondrocytes. Free Radic. Biol. Med. 190, 234–246. doi:10.1016/j.freeradbiomed.2022.08.018
Wei, F., Li, M., Crawford, R., Zhou, Y., and Xiao, Y. (2019). Exosome-integrated titanium oxide nanotubes for targeted bone regeneration. Acta Biomater. 86, 480–492. doi:10.1016/j.actbio.2019.01.006
Weiler, C., Nerlich, A. G., Bachmeier, B. E., and Boos, N. (2005). Expression and distribution of tumor necrosis factor alpha in human lumbar intervertebral discs: a study in surgical specimen and autopsy controls. Spine 30 (1), 44–54. doi:10.1097/01.brs.0000149186.63457.20
Wise, B. L., Seidel, M. F., and Lane, N. E. (2020). The evolution of nerve growth factor inhibition in clinical medicine. Nat. Rev. Rheumatol. 17 (1), 34–46. doi:10.1038/s41584-020-00528-4
Wong, J., Sampson, S. L., Bell-Briones, H., Ouyang, A., Lazar, A. A., Lotz, J. C., et al. (2019). Nutrient supply and nucleus pulposus cell function: effects of the transport properties of the cartilage endplate and potential implications for intradiscal biologic therapy. Osteoarthr. Cartil. 27 (6), 956–964. doi:10.1016/j.joca.2019.01.013
Wu, J., Chen, Y., Liao, Z., Liu, H., Zhang, S., Zhong, D., et al. (2022). Self-amplifying loop of NF-κB and periostin initiated by PIEZO1 accelerates mechano-induced senescence of nucleus pulposus cells and intervertebral disc degeneration. Mol. Ther. 30 (10), 3241–3256. doi:10.1016/j.ymthe.2022.05.021
Wuertz, K., Godburn, K., MacLean, J. J., Barbir, A., Donnelly, J. S., Roughley, P. J., et al. (2009). In vivo remodeling of intervertebral discs in response to short- and long-term dynamic compression. J. Orthop. Res. 27 (9), 1235–1242. doi:10.1002/jor.20867
Xiao, L., Xu, S., Xu, Y., Liu, C., Yang, B., Wang, J., et al. (2018). TGF-β/SMAD signaling inhibits intermittent cyclic mechanical tension-induced degeneration of endplate chondrocytes by regulating the miR-455-5p/RUNX2 axis. J. Cell Biochem. 119 (12), 10415–10425. doi:10.1002/jcb.27391
Xing, H., Zhang, Z., Mao, Q., Wang, C., Zhou, Y., Zhou, X., et al. (2021). Injectable exosome-functionalized extracellular matrix hydrogel for metabolism balance and pyroptosis regulation in intervertebral disc degeneration. J. Nanobiotechnology 19 (1), 264–278. doi:10.1186/s12951-021-00991-5
Xu, G., Zhang, B., Ye, J., Cao, S., Shi, J., Zhao, Y., et al. (2019). Exosomal miRNA-139 in cancer-associated fibroblasts inhibits gastric cancer progression by repressing MMP11 expression. Int. J. Biol. Sci. 15 (11), 2320–2329. doi:10.7150/ijbs.33750
Yan, H.-F., Zou, T., Tuo, Q.-z., Xu, S., Li, H., Belaidi, A. A., et al. (2021). Ferroptosis: mechanisms and links with diseases. Signal Transduct. Target. Ther. 6 (1), 49. doi:10.1038/s41392-020-00428-9
Yang, R. Z., Xu, W. N., Zheng, H. L., Zheng, X. F., Li, B., Jiang, L. S., et al. (2020). Involvement of oxidative stress-induced annulus fibrosus cell and nucleus pulposus cell ferroptosis in intervertebral disc degeneration pathogenesis. J. Cell. Physiology 236 (4), 2725–2739. doi:10.1002/jcp.30039
Yao, X., Jing, X., Guo, J., Sun, K., Deng, Y., Zhang, Y., et al. (2019). Icariin protects bone marrow mesenchymal stem cells against iron overload induced dysfunction through mitochondrial fusion and fission, PI3K/AKT/mTOR and MAPK pathways. Front. Pharmacol. 10, 163. doi:10.3389/fphar.2019.00163
Yao, X., Sun, K., Yu, S., Luo, J., Guo, J., Lin, J., et al. (2021). Chondrocyte ferroptosis contribute to the progression of osteoarthritis. J. Orthop. Transl. 27, 33–43. doi:10.1016/j.jot.2020.09.006
Yu, L., Wen, J. K., Wang, S., Wang, W. H., Yu, J. M., and Ye, X. J. (2020). Removal of calcified lumbar disc herniation with endoscopic-matched ultrasonic osteotome - our preliminary experience. Br. J. Neurosurg. 34 (1), 80–85. doi:10.1080/02688697.2019.1687850
Yuan, F. L., Xu, R. S., Ye, J. X., Zhao, M. D., Ren, L. J., and Li, X. (2019). Apoptotic bodies from endplate chondrocytes enhance the oxidative stress-induced mineralization by regulating PPi metabolism. J. Cell. Mol. Med. 23 (5), 3665–3675. doi:10.1111/jcmm.14268
Yue, B., Chen, B., Zou, Y. W., Xi, Y. M., Ren, X. F., Xiang, H. F., et al. (2016). Thoracic intervertebral disc calcification and herniation in adults: a report of two cases. Eur. Spine J. 25 (Suppl. 1), 118–123. doi:10.1007/s00586-015-4214-5
Zeidan, R. S., Han, S. M., Leeuwenburgh, C., and Xiao, R. (2021). Iron homeostasis and organismal aging. Ageing Res. Rev. 72, 101510. doi:10.1016/j.arr.2021.101510
Zhang, A., Cheng, Z., Chen, Y., Shi, P., Gan, W., and Zhang, Y. (2023). Emerging tissue engineering strategies for annulus fibrosus therapy. Acta Biomater. 167, 1–15. doi:10.1016/j.actbio.2023.06.012
Zhang, G. Z., Liu, M. Q., Chen, H. W., Wu, Z. L., Gao, Y. C., Ma, Z. J., et al. (2021). NF-κB signalling pathways in nucleus pulposus cell function and intervertebral disc degeneration. Cell Prolif. 54 (7), 13057–13071. doi:10.1111/cpr.13057
Zhang, K., Zhang, Y., Zhang, C., and Zhu, L. (2021). Upregulation of P53 promotes nucleus pulposus cell apoptosis in intervertebral disc degeneration through upregulating NDRG2. Cell Biol. Int. 45 (9), 1966–1975. doi:10.1002/cbin.11650
Zhang, P., Rong, K., Guo, J., Cui, L., Kong, K., Zhao, C., et al. (2023). Cynarin alleviates intervertebral disc degeneration via protecting nucleus pulposus cells from ferroptosis. Biomed. and Pharmacother. 165, 115252. doi:10.1016/j.biopha.2023.115252
Zhang, X., Huang, Z., Xie, Z., Chen, Y., Zheng, Z., Wei, X. a., et al. (2020). Homocysteine induces oxidative stress and ferroptosis of nucleus pulposus via enhancing methylation of GPX4. Free Radic. Biol. Med. 160, 552–565. doi:10.1016/j.freeradbiomed.2020.08.029
Zhang, X., Zhang, Z., Zou, X., Wang, Y., Qi, J., Han, S., et al. (2024). Unraveling the mechanisms of intervertebral disc degeneration: an exploration of the p38 MAPK signaling pathway. Front. Cell Dev. Biol. 11, 1324561–1324578. doi:10.3389/fcell.2023.1324561
Zhao, C. Q., Wang, L. M., Jiang, L. S., and Dai, L. Y. (2007). The cell biology of intervertebral disc aging and degeneration. Ageing Res. Rev. 6 (3), 247–261. doi:10.1016/j.arr.2007.08.001
Zhao, G., Xu, M. J., Zhao, M. M., Dai, X. Y., Kong, W., Wilson, G. M., et al. (2012). Activation of nuclear factor-kappa B accelerates vascular calcification by inhibiting ankylosis protein homolog expression. Kidney Int. 82 (1), 34–44. doi:10.1038/ki.2012.40
Zheng, Q., Li, X. X., Xiao, L., Shao, S., Jiang, H., Zhang, X. L., et al. (2019). MicroRNA-365 functions as a mechanosensitive microRNA to inhibit end plate chondrocyte degeneration by targeting histone deacetylase 4. Bone 128, 115052. doi:10.1016/j.bone.2019.115052
Zhou, Q., Pu, X., Qian, Z., Chen, H., Wang, N., Wang, S., et al. (2024). Nuclear receptor Rev-erbα alleviates intervertebral disc degeneration by recruiting NCoR–HDAC3 co-repressor and inhibiting NLRP3 inflammasome. Cell Prolif., 13720–13734. doi:10.1111/cpr.13720
Zhu, F., Duan, W., Zhong, C., Ji, B., and Liu, X. (2022). The protective effects of dezocine on interleukin-1β-induced inflammation, oxidative stress and apoptosis of human nucleus pulposus cells and the possible mechanisms. Bioengineered 13 (1), 1399–1410. doi:10.1080/21655979.2021.2017700
Zhu, G., Yang, X., Peng, C., Yu, L., and Hao, Y. (2020). Exosomal miR-532-5p from bone marrow mesenchymal stem cells reduce intervertebral disc degeneration by targeting RASSF5. Exp. Cell Res. 393 (2), 112109. doi:10.1016/j.yexcr.2020.112109
Zhu, J., Sun, R., Sun, K., Yan, C., Jiang, J., Kong, F., et al. (2023). The deubiquitinase USP11 ameliorates intervertebral disc degeneration by regulating oxidative stress-induced ferroptosis via deubiquitinating and stabilizing Sirt3. Redox Biol. 62, 102707. doi:10.1016/j.redox.2023.102707
Zhu, L.-P., Tian, T., Wang, J.-Y., He, J.-N., Chen, T., Pan, M., et al. (2018). Hypoxia-elicited mesenchymal stem cell-derived exosomes facilitates cardiac repair through miR-125b-mediated prevention of cell death in myocardial infarction. Theranostics 8 (22), 6163–6177. doi:10.7150/thno.28021
Keywords: intervertebral disc degeneration, cartilage endplate, inflammation, exosomes, calcification, ferroptosis, senescence
Citation: Yang H, Chen X, Chen J, Dong Y, Huang Y, Qin L, Tan J and Yi W (2024) The pathogenesis and targeted therapies of intervertebral disc degeneration induced by cartilage endplate inflammation. Front. Cell Dev. Biol. 12:1492870. doi: 10.3389/fcell.2024.1492870
Received: 08 September 2024; Accepted: 21 November 2024;
Published: 02 December 2024.
Edited by:
Sheng Chen, Huazhong University of Science and Technology, ChinaReviewed by:
Caihong Zhu, Soochow University Medical College, ChinaChencheng Feng, Third Military Medical University, China
Copyright © 2024 Yang, Chen, Chen, Dong, Huang, Qin, Tan and Yi. This is an open-access article distributed under the terms of the Creative Commons Attribution License (CC BY). The use, distribution or reproduction in other forums is permitted, provided the original author(s) and the copyright owner(s) are credited and that the original publication in this journal is cited, in accordance with accepted academic practice. No use, distribution or reproduction is permitted which does not comply with these terms.
*Correspondence: Jie Tan, dGFuamllQGFsdS5zY3UuZWR1LmNu; Lei Qin, ZHJfcWlubGVpQDE2My5jb20=
†These authors have contributed equally to this work and share first authorship