- Department of Neurosurgery, Department of Cardiovascular Surgery, West China Hospital, Sichuan University, Chengdu, China
The brain has traditionally been considered an “immune-privileged” organ lacking a lymphatic system. However, recent studies have challenged this view by identifying the presence of the glymphatic system and meningeal lymphatic vessels (MLVs). These discoveries offer new opportunities for waste clearance and treatment of central nervous system (CNS) diseases. Various strategies have been developed based on these pathways, including modulation of glymphatic system function, enhancement of meningeal lymphatic drainage, and utilization of these routes for drug delivery. Consequently, this review explores the developmental features and physiological roles of the cerebral lymphatic system as well as its significance in various CNS disorders. Notably, strategies for ameliorating CNS diseases have been discussed with a focus on enhancing glymphatic system and MLVs functionality through modulation of physiological factors along with implementing pharmacological and physical treatments. Additionally, emphasis is placed on the potential use of the CNS lymphatic system in drug delivery while envisioning future directions in terms of mechanisms, applications, and translational research.
1 Introduction
The lymphatic system plays a vital role in transporting interstitial fluid (ISF) from various tissues back to circulation, facilitating immune surveillance and response, as well as maintaining fluid balance (Oliver et al., 2020). Recent studies have provided evidence for its multifunctional role and positive influence on organ-specific physiological functions and disease processes (Petrova and Koh, 2020). The brain, previously believed to lack traditional lymphatic vessels, is considered an organ with unique immune privilege (Louveau et al., 2015a). Consequently, the mechanisms employed by the brain to eliminate the metabolic waste generated by cerebral tissues remain unclear, and this clearance process significantly contributes to the pathogenesis, progression, and prognostic assessment of central nervous system (CNS) diseases. Interestingly, over two centuries ago, Paolo Mascagni described the existence of meningeal lymphatic vessels (MLVs), but his findings were overlooked due to contradicting mainstream beliefs at that time (Sandrone et al., 2019). In 2012, Iliff et al. (2012) discovered an internal cerebrospinal exchange and clearance pathway that relies on the Aquaporin-4 (AQP4) protein located at the astrocytic endfeet and penetrating vessel perivascular space. Through this route, similar to that of peripheral lymphatic system function, large molecules along with pathological proteins and interstitial solutes exit the brain parenchyma into cerebrospinal fluid (CSF) (Iliff et al., 2012). Given its similarity in mechanism with the peripheral lymphatic system, it was appropriately named the glymphatic system by the scientific community (Louveau et al., 2015a) (Figures 1, 2).
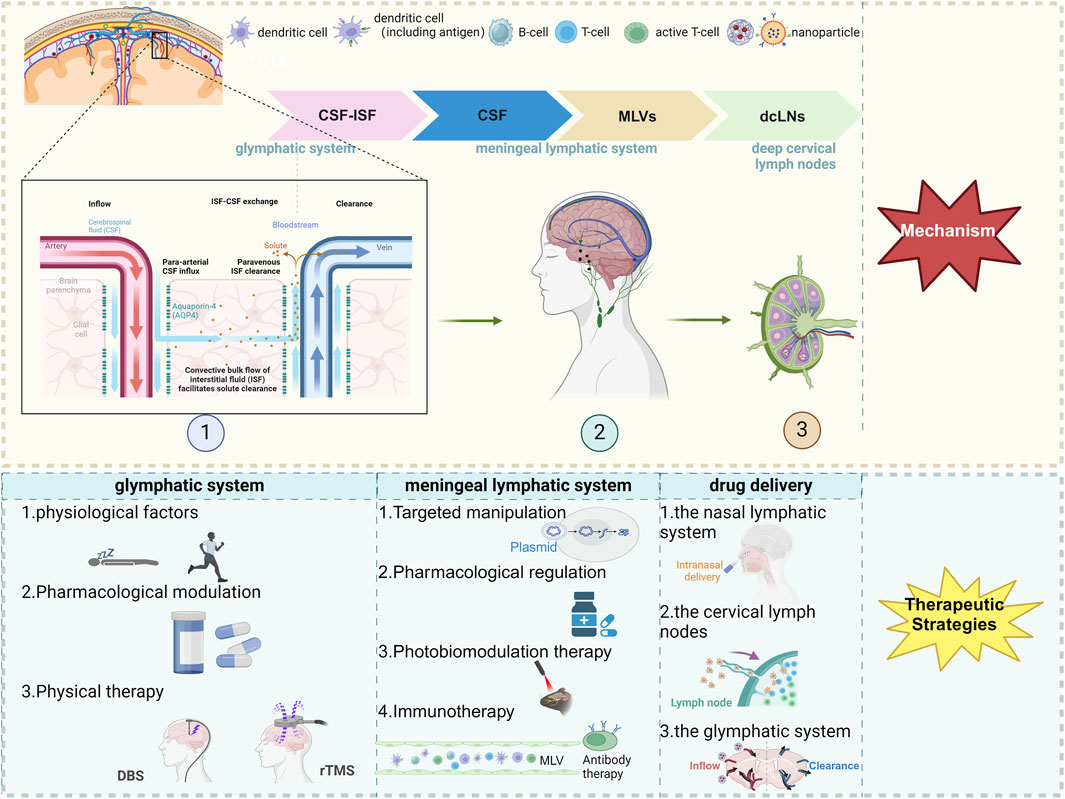
Figure 1. The scheme of therapeutic approaches to CNS diseases via the meningeal lymphatic and glymphatic systems. Created with Biorender.com. Abbreviations: CSF, cerebrospinal fluid; ISF, interstitial fluid; MLVs, meningeal lymphatic vessels; dcLNs, deep cervical lymph nodes; AQP4, Aquaporin-4; DBS, deep brain stimulation; rTMS, repetitive Transcranial Magnetic Stimulation.
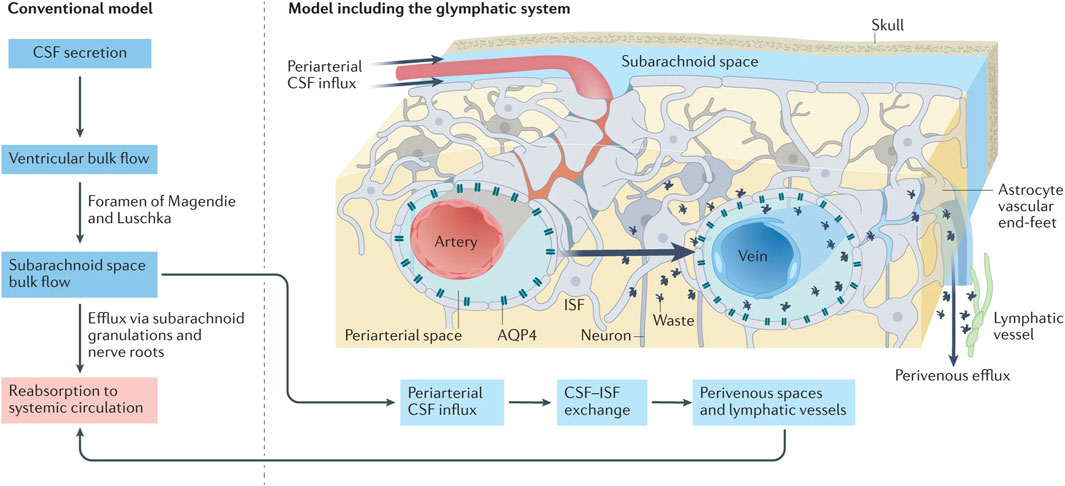
Figure 2. Under traditional CSF drainage patterns (left), the CSF, producing in the ventricular arachnoid mater, circulates within the subarachnoid space before ultimately being reabsorbed back into the blood circulation. In the emerging CSF drainage model under the participation of the glymphatic system (right), the CSF can access the brain parenchyma via perivascular spaces surrounding the arteries, subsequently exchange with the ISF, which infiltrates into the subarachnoid space containing CSF via perivascular spaces surrounding the veins (reproduced from Lohela et al., 2022, with permission from Springer Nature). Abbreviations: CSF, cerebrospinal fluid; ISF, interstitial fluid.
In 2015, research conducted by Aspelund et al. (2015); Louveau et al. (2015b) revealed the existence of MLVs in the dorsal and basal regions of the brain, which are lined with fully differentiated lymphatic endothelial cells (LECs) resembling classic lymphatic vessels found in peripheral tissues. These MLVs express lymphatic markers such as Vascular Endothelial Growth Factor Receptor 3 (VEGFR3) and Prospero-Related Homeobox Protein 1 (PROX1), challenging the conventional notion of cerebral immune “privilege.” Subsequently, scientists have further substantiated the presence of MLVs at the skull base within the meninges, consistent with Aspelund et al. (2015) findings, and revealed that these basal MLVs possess lymphatic valves (Ahn et al., 2019). Furthermore, MLVs were identified as conduits for CSF drainage into deep cervical lymph nodes (dcLNs) (Louveau et al., 2015b). High-resolution magnetic resonance imaging (MRI) has been employed to demonstrate the presence of MLVs in both humans and non-human primates (Absinta et al., 2017). More interestingly, researchers utilized light-sheet fluorescence microscopy and MRI to achieve comprehensive whole-head imaging of intact MLVs in mice and humans alike (Figure 3) (Da Mesquita, 2022; Jacob et al., 2022). Collectively, these findings provide compelling evidence for the existence of a functional CNS lymphatic system.
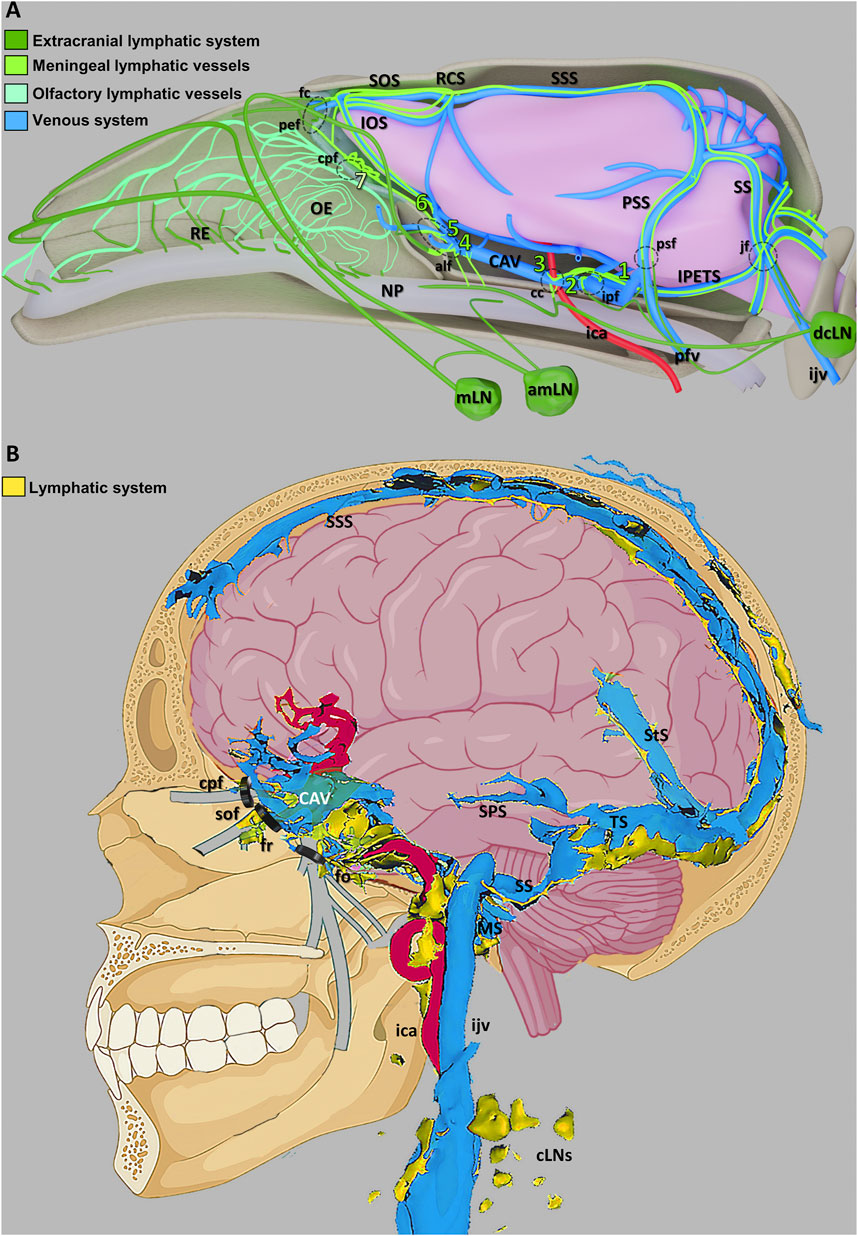
Figure 3. Schematic illustration of the CNS venous and lymphatic systems in mice and humans (A) A summary of the mouse head’s lymphatic CSF draining circuitry. (B) Diagram showing the human dural venolymphatic complex with lymphatic discharges (reproduced from Jacob et al., 2022, licensed under CC BY 4.0). Abbreviations: RE, respiratory epithelium; OE, olfactory epithelium; NP, nasopharynx; mLN, mandibular lymph node; amLN, accessory mandibular LN; IOS, inferior olfactory sinus; SOS, superior olfactory sinus; RCS, rostral confluence of sinuses; CAV, cavernous sinus; SSS, superior sagittal sinus; PSS, petrosquamous sinus; SS, sigmoid sinus; IPETS, inferior petrosal sinus; dcLN, deep cervical LN; sfc, foramen caecum; pef, posterior ethmoid foramen; cpf, cribriform plate foramina; alf, anterior lacerated foramen; cc, carotid canal; ipf, interpterygoid foramen; ica, internal carotid artery; pfv, posterior facial vein; psf, petrosquamous fissure; jf, jugular foramen; ijv, internal jugular vein; SPS, superior petrosal sinus; StS, straight sinus; TS, transverse sinus; SS, sigmoid sinuses; MS, marginal sinus; cLNs, cervical LNs; sof, superior orbital fissure; fr, foramen rotundum; fo, foramen ovale.
Additionally, the recently discovered CNS lymphatic system exhibits crucial physiological functions in eliminating metabolic waste from the brain and regulating immunity (Rasmussen et al., 2022). Dysfunction of this system have been observed in various CNS diseases (Rasmussen et al., 2022). This review outlines both developmental features and physiological functions of the CNS lymphatic system while also discussing its implications in different diseases. Furthermore, it emphasizes strategies for intervening in brain diseases by targeting the CNS lymphatic system, specifically focusing on modulating the glymphatic system, manipulating MLVs, and utilizing drug delivery mediated by the CNS lymphatic system to achieve therapeutic objectives for CNS diseases. Finally, this article concludes with a summary of strategies involving the CNS lymphatic system for treating CNS diseases and provides insights into potential directions for future research (Figure 1).
2 Developmental features, physiological functions, and disease associations of the CNS lymphatic system
2.1 Developmental features of the CNS lymphatic system
The glymphatic system is not a lymphatic structure comprising fully matured LECs (Iliff et al., 2012). However, owing to its similar functionality as peripheral lymphatic vessels in promoting the clearance and drainage of metabolic waste from the ISF of the brain, it is referred to as the glymphatic system. Its primary constituents include astrocytes and their end-feet protein, AQP4 (Iliff et al., 2012), along with perivascular spaces known as Virchow-Robin spaces (VRS) (Rennels et al., 1985). Astrocytes mainly play a supportive role by adhering to the penetrating vessels of the brain. Meanwhile, AQP4 is expressed on their surface, enabling the delivery of nutrient-rich CSF into the brain parenchyma for nourishing neurons, glial cells, and other neurocytes (Plog and Nedergaard, 2018). The perivascular spaces encompass both arteriolar and venular perivascular spaces that serve as conduits for the inflow of CSF and outflow of ISF (Rasmussen et al., 2018). Penetrating intracerebral arteries traverse these spaces to reach deep into blind-ended regions of brain parenchyma. Facilitated by the action of AQP4, CSF can follow these spaces and infiltrate extensively within the brain parenchyma. This pathway also provides an opportunity for intrathecally injected drugs in CSF to access deep regions of brain parenchyma (Lohela et al., 2022).
The development of MLVs is characterized by multiple facets. After birth, MLVs develop and extend in synchrony with the growth direction of nerves and blood vessels (Antila et al., 2017). By utilizing MRI techniques, non-invasive visualization and quantification of MLVs, along with studying the flow dynamics within the human MLVs, have been successfully accomplished (Kim et al., 2023). Preliminary results indicate that the flow velocity in dorsal MLVs ranges from 2.2 to 2.7 mm per second in humans. Moreover, a gender discrepancy in MLV volumes is apparent between males and females, with male MLV volumes significantly exceeding those of females (Jacob et al., 2022). In both mice and humans, the drainage capacity of MLVs diminishes with age (Da Mesquita et al., 2018; Albayram et al., 2022). Remarkably, the MLVs exhibited plasticity and regenerative capacity. An increase in Vascular Endothelial Growth Factor C (VEGF-C), a positive factor promoting lymphatic vessel development, induces the sprouting, development, and maintenance of MLVs. Conversely, silencing or blocking VEGF-C signaling leads to noticeable degradation of MLVs, whereas the knockout of Vascular Endothelial Growth Factor D (VEGF-D) does not significantly impact them (Antila et al., 2017). Basal MLVs, adjacent to the subarachnoid space (SAS), possess lymphatic valves akin to peripheral lymphatic vessels and serve as “hotspot” sites for clearing large molecules within CSF (Ahn et al., 2019).
2.2 Physiological functions of the CNS lymphatic system
While there is no direct anatomical connection between the glymphatic system and the meningeal lymphatic system, they functionally interact to collectively form a unique network responsible for waste clearance and immune regulation in the brain (Louveau et al., 2015b). In terms of waste clearance, these systems collaboratively establish a CSF drainage pathway: CSF-ISF-CSF-MLV-dcLNs (Li G. et al., 2022). Iliff et al. (2012) demonstrated that the majority of the CSF in the SAS infiltrates into the brain parenchyma. As shown in Figure 2, CSF flows into the brain parenchyma through perivascular spaces surrounding pia mater arteries and penetrating arterioles, where it undergoes mixing as well as nutrients and metabolic waste exchange with ISF . ISF, loaded with interstitial solutes, is expelled from the brain parenchyma via venous perivascular routes (intracerebral and caudal rhinal veins) and eliminated into the CSF (Iliff et al., 2012).
The dural lymphatic vessels then uptake absorb ISF and CSF from the SAS, subsequently transporting them to the dcLNs (Aspelund et al., 2015; Louveau et al., 2015b) (Figure 3). The principal site of absorption is identified as the MLVs located at the base of the skull (Ahn et al., 2019). This discovery offers a novel perspective on the clearance of large molecular solutes in neurodegenerative disorders. Furthermore, CSF is drained through multiple pathways. Traditionally, it is posited that arachnoid granulations facilitate the absorption of CSF from the SAS via a valve-like mechanism, allowing CSF to enter and ultimately be absorbed by the venous sinuses (Welch and Friedman, 1960; Wolpow and Schaumburg, 1972) (Figure 2). Additionally, the arachnoid cuff exit points, formed by discontinuities as the bridging veins traverse the arachnoid barrier, create openings that permit the exchange of CSF and molecules between the SAS and the dura mater, facilitating CSF drainage (Smyth et al., 2024). Recently, the nasopharyngeal lymphatic plexus (NPLP) has been demonstrated to serve as a major hub for the outflow of CSF to the dcLNs (Yoon et al., 2024). Research indicates that the NPLP is connected with upstream lymphatic vessels, including those near the pituitary gland and the cavernous sinus, as well as through the cribriform plate, where CSF is drained to the dcLNs via the deep cervical lymphatic vessels. These CSF drainage structures collaborate with the glymphatic system and the meningeal lymphatic system to jointly sustain the balance of cerebral fluids and the clearance of metabolic waste.
On the other hand, both the glymphatic system and the meningeal lymphatic system play crucial roles in immune cell drainage and immunosurveillance, functioning synergistically (Li G. et al., 2022). Prior to the discovery of the brain lymphatic system, research on neuroimmunity primarily focused on microglia. However, with the identification of this system, it has revitalized studies in neuroimmunology (Sun et al., 2018). Research indicates that antigens generated within the CNS can travel through the glymphatic pathway to reach the SAS before draining into MLVs or passing through the cribriform plate into nasal lymphatics and eventually reaching cervical lymph nodes (cLNs). This process stimulates adaptive immune responses (Louveau et al., 2015a; Louveau et al., 2015b). Mechanistically, neuroinflammation can induce the formation of MLVs adjacent to the cribriform plate. These newly formed lymphatic vessels containing CD4+ T cells and CD11c+ cells capable of capturing CNS antigens and presenting them to T cells (Hsu et al., 2022). Additionally, a substantial number of immune cells are located around the meningeal sinuses which serve as an ecological niche known as a “regional immune center.” These cells collaborate with MLVs to perform neuroimmunosurveillance (Rustenhoven et al., 2021; Smyth et al., 2024). Studies have demonstrated that CNS antigens present in the CSF reside around dural sinuses, where they are presented by antigen presenting cells associated with dura mater for patrolling T cells from venous sinuses. These activated T cells migrate through interstitial spaces of MLV endothelial cells into MLVs and are subsequently drained towards cLNs, thereby inducing the generation of T cell effector functions and promoting tissue retention (Rustenhoven et al., 2021). Therefore, MLVs provide a feasible physical conduit for the interaction between CNS antigens and peripheral T cells.
2.3 CNS diseases associations of the CNS lymphatic system
Several studies have highlighted dysfunction in CNS lymphatic drainage across a range of brain diseases (Table 1). For instance, in models of subarachnoid hemorrhage (SAH), the glymphatic system is obstructed by fibrin clot blockages in perivascular spaces, thereby impeding CSF inflow into the brain parenchyma (Pu et al., 2019). In cytotoxic brain edema, hypoxia-induced energy depletion results in glymphatic system dysfunction, promoting CSF inflow into the brain parenchyma while inhibiting ISF outflow (Thrane et al., 2014). In the meningeal lymphatic system of idiopathic Parkinson’s disease (PD) patients, a reduction in MLV flow was observed in the superior sagittal sinus and sigmoid sinus regions, along with delayed CSF drainage in deep lymph nodes (Ding et al., 2021), and impaired clearance of α-synuclein (α-syn) by CNS lymphatics (Zou et al., 2019). These findings provide evidence of MLV dysfunction in PD patients. Moreover, both the meningeal lymphatic drainage capability and MLV coverage were compromised in an Alzheimer’s disease (AD) mouse model, accompanied by cognitive impairment (Da Mesquita et al., 2018). Dysfunctions in both the glymphatic system and MLVs frequently co-occur in CNS diseases. Glymphatic system dysfunction often results in inadequate removal of metabolic waste from CSF through downstream MLVs, while disruption of MLVs hinders glymphatic clearance of ISF, leading to concurrent dysfunction between these systems (Da Mesquita et al., 2018; Li W. et al., 2022). For instance, traumatic brain injury (TBI) can impair AQP4 polarity and reduce glymphatic function by approximately 60% (Iliff et al., 2014). Concurrently, TBI can cause persistent MLV dysfunction for up to 1 month post-injury, indicating an interconnected pathology (Bolte et al., 2020). Besides, evidence for a simultaneous dysfunction of both glymphatic system and meningeal lymphatics in the context of chronic migraine conditions has been consolidated through non-invasive MRI imaging techniques (Wu et al., 2024). Analyses highlight an attenuated diffusion of water molecules surrounding the vasculature in individuals grappling with chronic migraines, in stark contrast to a control population manifesting healthy parameters. Investigations further reveal a decelerated fluid flow rate within the MLVs, culminating in a compromised effectiveness in CSF clearance, thereby convincingly illustrating a simultaneous dysfunction of both lymphatic systems (Table 1).
Furthermore, in the presence of preexisting CNS diseases, impaired CNS lymphatic drainage often acts as an exacerbating factor. For instance, in an AD mouse model, inhibition of glymphatic system function via AQP4 knockout led to decelerated CSF inflow into the brain parenchyma, resulting in heightened accumulation of Amyloid-β (Aβ) and more pronounced cognitive deficits (Iliff et al., 2012). In a PD mouse model, dysfunction of glymphatic system drainage caused by ligation of the MLVs exacerbated α-syn accumulation, increased neuroinflammation, and worsened motor function impairment (Zou et al., 2019). In the context of stroke, mice with impaired MLVs often present with a larger infarct volume compared to wild-type mice (Yanev et al., 2020) (Table 1). When considering brain tumors, such as glioma and metastatic melanoma, obstruction of dorsal skull MLVs in mouse models diminishes the therapeutic effects of anti-Programmed Cell Death Protein 1/Cytotoxic T-Lymphocyte-Associated Protein 4 checkpoint combined therapy on these tumors (Hu et al., 2020). In an AD mouse model, ablation of MLVs intensifies AD pathology and cognitive performance decline by promoting Aβ accumulation, activating microglia proliferation, causing behavioral deficits and deterioration, while also reducing the effectiveness of anti-Aβ immunotherapy (Da Mesquita et al., 2018; Wang et al., 2019). Conversely, the latest research suggests that the blockade of MLVs function did not lead to an increase in the accumulation of pathological proteins in AD mouse models, nor did it exacerbate cognitive impairments (Antila et al., 2024). Such discrepancies may be attributed to variations in animal models, methodologies, and compensatory mechanisms of CSF dynamics. The complexity of CNS disease pathophysiology involves numerous factors and pathways, with various studies potentially revealing different aspects of this network. Therefore, further investigation of MLVs in the CNS diseases to clarify their role in disease pathology is needed.
The evidence presented indicates that dysfunction in the glymphatic system and meningeal lymphatic system is observed across a spectrum of neurological diseases, where compromised CNS lymphatic drainage frequently exacerbates disease severity, thus establishing a detrimental cycle. Therefore, the regulation of CNS lymphatic functions assumes paramount importance for the effective treatment of CNS diseases.
3 Glymphatic system-mediated therapeutic strategies for CNS disease
The glymphatic system, by means of its exchange between CSF and ISF, is integrally involved in the clearance of metabolic waste and the provision of nutrients within the brain (Figure 2). This crucial function is essential for maintaining cerebral health and preventing neurodegenerative diseases (Ding et al., 2023). Nonetheless, impairments in glymphatic functioning often coincide with various conditions, resulting in the obstruction of pathological macromolecules and hindered distribution of metabolic waste. As a result, intraneural accumulation occurs, subsequently contributing to the development of neurodegenerative diseases (Kress et al., 2014). Therefore, interventions targeting glymphatic system function through physiological factors, pharmacological modulation, and physical therapy hold substantial therapeutic potential.
3.1 Modulating physiological factors to intervene in the glymphatic system
3.1.1 Sleep regulation
The role of sleep in eliminating metabolic waste from the brain is crucial. In fruit fly models of AD, sleep deprivation exacerbates the accumulation of Aβ, which shows an inverse correlation with the reported duration of nocturnal sleep (Shokri-Kojori et al., 2018). It has been demonstrated that during sleep, there is approximately a 60% increase in intercellular space, promoting enhanced convective flux of ISF within brain tissue and significantly improving CSF-ISF exchange (Xie et al., 2013). Furthermore, the glymphatic system in mice exhibits a pronounced diurnal rhythm where maintaining such rhythms relies on the polarization of AQP4 in the vascular endfeet of astrocytes as a fundamental factor (Hablitz et al., 2020). Moreover, during non-rapid eye movement sleep, the slow-wave neural activity can induce fluctuations in cerebral blood flow. These blood flow oscillations are coupled with the macroscopic oscillations of CSF, proving that neural activity can regulate the outflow of CSF (Fultz et al., 2019). This, in turn, indirectly affects the efficiency of the exchange between CSF and ISF within the glymphatic system, accelerating the clearance of metabolic waste. Thus, by modulating the sleep-wake cycle, it may be possible to harness the clearance functionality of the glymphatic system for improving neurodegenerative diseases.
3.1.2 Alteration of body position
Different body positions during sleep also impact the function of the glymphatic system. Studies have discovered that the lateral position, or side-lying posture, is most effective in facilitating cerebral glymphatic transport and waste clearance, while the prone position demonstrates lower efficiency in waste removal (Lee et al., 2015). Furthermore, research has validated the influence of body position on glymphatic transport in humans. By utilizing upright MRI technology, images were acquired from 30 healthy volunteers in both standing and supine postures. It was observed that the CSF pulse volume during the cardiac cycle increased by approximately 57.6% in the supine position compared to standing (Muccio et al., 2021). This finding substantiates the notion that there is an enhanced CSF when one is a supine position, thus facilitating convection within the glymphatic system. This new perspective opens up an avenues for further exploration of the interplay between body position, brain-waste clearance, aging, and a series of neurodegenerative diseases. Additionally, it offers crucial insight for researchers: the influence of body position should not be overlooked when conducting imaging studies on glymphatic system functionality.
3.1.3 Thermoregulation
Body temperature is an influential factor in the functioning of the glymphatic system and cannot be overlooked. Research indicates that early administration of hypothermic treatment can inhibit the velocity of CSF, thereby enhancing the immune response within the glymphatic system in a murine model of cerebral trauma (Li et al., 2024). This internal fluid process has been confirmed by high-temporal and spatial-resolution near-infrared two-region (NIR-II) imaging. Furthermore, hypothermic treatment upregulates the expression of genes associated with cerebral tissue repair (Li et al., 2024). Further investigation is required to determine whether this approach can improve pathological outcomes following cerebral injury (Ding et al., 2023).
3.1.4 Physical exercise
Physical exercise plays a substantial role in promoting the clearance of metabolites through the glymphatic system, reducing neuroinflammation, and enhancing cognitive function. Studies have shown that voluntary exercise in aged mice improves spatial memory capabilities in water maze tests. This activity stimulates the expression of AQP4 protein and expedites solute macromolecule clearance within the glymphatic system, thereby reducing the deposition of Aβ (Maliszewska-Cyna et al., 2016; He et al., 2017). Furthermore, it has been further evidenced that exercise can mitigate the activation of microglial cells and astrocytes, highlighting its potential to alleviate neuroinflammation (He et al., 2017). Consequently, incorporating moderate exercise into treatment plans for patients with neurodegenerative diseases could serve as a strategic mechanism to mitigate pathological accumulation in AD while potentially fostering cognitive improvements.
Research has emphasized the role of cerebral artery pulse activity as a crucial driving force for the movement of CSF within and throughout the brain tissue. A reduction in arterial pulsation is associated with a slower pace of CSF-ISF exchange, thus slowing down the clearance of macromolecules, including Aβ and tau (Iliff et al., 2013). With aging, there is a decline in the efficiency of the glymphatic system, responsible for clearing large solute molecules, which is linked to reduced pulsation in cerebral artery walls (Iliff et al., 2012; Kress et al., 2014). Modulating these physiological factors that influence glymphatic system function may partly involve changes in cerebral artery pulsation penetrability. Furthermore, identifying novel physiological factors that regulate the penetrability of cerebral artery pulsations and promote CSF flow into brain tissue holds significant implications for clearing metabolic waste.
3.2 Pharmacological modulation of glymphatic system functionality
3.2.1 Targeted pharmacological modulation
AQP4 is expressed on the foot processes of astrocytes, which form the outer walls of the perivascular space. This allows CSF to permeate the brain parenchyma, playing a crucial role in maintaining fluid balance within the brain’s barriers dynamically (Peng et al., 2023). Genetic deletion of AQP4 in murine models leads to a conspicuous reduction in CSF influx into the brain parenchyma (Mestre et al., 2018). In an AD mouse model characterized by tau pathology accumulation, both the CSF-ISF exchange of the glymphatic system and the polarization of AQP4 were impaired, with further exacerbation caused by TGN-02-induced pharmacological inhibition of AQP4. These findings support the notion that targeting AQP4 could be essential for eliminating waste from CNS diseases (Harrison et al., 2020). Therefore, rectifying alterations in AQP4 polarity through genetic or pharmacological means holds significant therapeutic potential for improving various CNS diseases.
Research has indicated that following an ischemic stroke, the decline in Dystrophin 71 (DP71) expression can lead to impaired AQP4 polarity, resulting in compromised function of the glymphatic system and hindering the clearance of vasogenic edema from ischemic sites (Yang et al., 2024). It has been discovered that a nucleic acid-based drug overexpressing DP71 can effectively improve brain edema by promoting co-localization and interaction between AQP4 and DP71, consequently restoring AQP4 polarity (Yang et al., 2024). Similarly, in a mouse model of PD, reduced CSF influx and efflux result in decreased efficiency of CSF-ISF exchange and compromised AQP4 polarity (Zou et al., 2019). Mechanistically, PD causes abnormal activation of Matrix Metalloproteinase-9 (MMP9), which triggers cleavage of β-dystroglycan, impacting AQP4 polarity and subsequently damaging the function of the glymphatic system (Fang et al., 2021; Si et al., 2024). Pharmacological inhibition of MMP9 using the inhibitor GM6001 not only restores the polarity of AQP4 and contacts between the basement membrane and astrocyte end-feet but also exhibits neuroprotective effects and promotes metabolic homeostasis in PD (Si et al., 2024). These findings suggest that inhibiting MMP-9 to restore AQP4 polarity could be a potent strategy for enhancing glymphatic system function in PD.
3.2.2 Traditional pharmacological modulation
The modulation of glymphatic system function is acknowledged as a significant role played by traditional hormonal therapeutics and their antagonists (Yang et al., 2024). In the context of a TBI model, cerebral edema occurring alongside a norepinephrine storm presents challenges to glymphatic system functionality. The utilization of norepinephrine receptor antagonists (prazosin, propranolol, and atipamezole) has been observed to enhance CSF influx into brain tissue and effectively clear cellular debris therein. This mitigates tau accumulation, neuroinflammation, and alleviates cerebral edema (Hussain et al., 2023). Moreover, research has revealed that glucocorticoid dexamethasone (Sun et al., 2024) along with selective α2-adrenergic receptor agonist dexmedetomidine (Persson et al., 2022) fosters CSF influx into brain tissue and improves glymphatic system functionality. However, further investigation is required to determine the specific underlying mechanisms involved. It should be noted that invasive procedures, such as intracranial injections, can significantly disrupt glymphatic system function; thus they should be avoided when exploring its functionality (Mestre et al., 2018).
3.3 Physical therapy
Emerging studies suggest that gamma multisensory stimulation exerts regulatory effects on the glymphatic system and ameliorates the pathological manifestations of AD. Researchers have induced corresponding neural activities in certain brain areas through multisensory gamma stimulation, which enhances clearance functionalities within the glymphatic system, leading to a reduction in amyloid protein accumulation in AD mouse models (Murdock et al., 2024). This outcome is attributed to gamma stimulation, which promotes polarization of the water channel protein AQP4 in astroglial cells and expands MLVs. Moreover, it promotes glymphatic clearance by mediating pulsations in cerebral arteries through Vasointestinal Peptide (VIP) neuron-dependent activity (Nedergaard and Goldman, 2020). Repetitive transcranial magnetic stimulation (rTMS) has exhibited similar effects. Notably, early high-frequency rTMS treatment in AD mouse models effectively reduces long-term memory loss and mitigates AD-related pathological development, including Aβ deposition and glial cell activation, by enhancing the excretory efficiency of the glymphatic system (Lin et al., 2021). Consequently, gamma multisensory stimulation and rTMS, along with other physical therapies alike, represent promising therapeutic strategies for improving glymphatic system function, thereby ameliorating the prognosis of neurodegenerative diseases.
In summary, modulation of glymphatic system functionality holds significant value for the treatment of CNS diseases. The enhancement of this system’s capabilities through physiological regulation, pharmacological intervention, and physical therapy can facilitate more efficient clearance of detrimental substances within the brain parenchyma. This process may mitigate or prevent the onset and progression of neurodegenerative disorders.
4 Therapeutic strategies for modulating the meningeal lymphatic system to address CNS diseases
Abundant research indicates that dysfunction in the drainage of MLVs is observed in neurological disorders, and adverse MLV conditions may exacerbate a wide range of diseases, including neurodegenerative diseases such as AD and PD (Da Mesquita et al., 2018). Therefore, augmenting the functionality of MLVs is an important approach for delaying or preventing neurological diseases. Current methodologies encompass genetic techniques, pharmacological interventions, photobiomodulation therapy (PMT), and immunological therapies.
4.1 Targeted manipulation techniques for modulating MLVs
4.1.1 VEGF-C
The potential role of VEGF-C in the enhancement of various neurological diseases has been extensively documented due to its reported ability to promote the development, formation, and maintenance of MLVs (Antila et al., 2017). Initially, direct administration of VEGF-C was employed for treating these conditions. In neurodegenerative diseases, impaired MLV function can hinder the exchange between CSF and ISF, leading to reduced clearance of pathological macromolecules and metabolic waste in the brain as well as cognitive impairment (Da Mesquita et al., 2018). Treatment with VEGF-C in elderly murine models has demonstrated an enhanced exclusionary effect on large molecules by MLVs within CSF, thereby strengthening the renewal and perfusion processes within intracranial CSF while subsequently improving learning and memory performance (Da Mesquita et al., 2018). In neurovascular disorders like stroke, studies have shown that the overexpression of VEGF-C enhances the formation of MLVs and meningeal lymphatic drainage, while reducing microglial activation and neuroinflammation. This mitigates motor function impairment, ameliorates the prognosis of ischemic stroke (Boisserand et al., 2024). Within the context of neuroinfectious diseases, researchers infected mouse models with Japanese encephalitis virus (JEV) and observed that post-CNS viral infection promotes neogenesis and expansion of MLVs but can also induce their dysfunction (Li X. et al., 2022). Photocoagulating MLVs or ligating draining lymph nodes in virus-infected mice exacerbates host’s neural tissue damage and increases mortality rate. However, gel-encapsulated VEGF-C recombinant peptide treatment appears to restore MLV function after application, reducing CNS damage caused by the virus, and enhancing survival rate (Li X. et al., 2022).
Additionally, indirect methods of increasing VEGF-C levels can be used for the treatment of brain diseases. Recent research has demonstrated that transplantation of cranial precursor cells in a murine model with premature cranial closure fosters the growth and development of MLVs, leading to the restoration of intracranial pressure (ICP), improved cerebral perfusion, and enhanced cognitive function. These beneficial effects are primarily attributed to the ability of cranial precursor cells to secrete VEGF-C (Ma et al., 2023). In conclusion, VEGF-C emerges as a potent therapeutic agent for safeguarding MLVs and holds promise for managing diverse neurological conditions. However, further investigations are warranted to ascertain their long-term therapeutic efficacy and overall safety.
4.1.2 PIEZO1
The Piezo-Type Mechanosensitive Ion Channel Component 1 (PIEZO1) protein is an ion channel present on the cellular membrane, which transduces mechanical signals into electrochemical signals in response to mechanical stimuli, thereby facilitating intracellular signal transduction (Hill et al., 2022). It has been corroborated to be involved in endothelial cell genesis, proliferation, and repair processes (Zhang et al., 2022). In mouse models of premature cranial closure, increased ICP has been observed to affect CSF flow, consequently restricting the sprouting and expansion of MLVs (Aspelund and Alitalo, 2024). The employment of Yoda1 as a PIEZO1 agonist improves CSF flow dynamics, reduces ICP levels, promotes the growth and maintenance of MLVs, and enhances cerebral waste clearance as well as immune functionality (Matrongolo et al., 2023). The significant role of PIEZO1 has also been confirmed in mouse models of Down syndrome and hydrocephalus (Choi et al., 2024), where Yoda1 markedly alleviates symptoms related to excessive CSF accumulation and ventricular expansion. Therefore, considering its ability to regulate MLV growth and development effectively, the PIEZO1 agonist Yoda1 holds promising potential for future applications in modulating MLVs. However, further investigation is required to elucidate the specific mechanisms by which it regulates ICP levels and promotes MLV development while assessing its long-term therapeutic effects.
4.1.3 DSCR1
The protein Down Syndrome Critical Region 1 (DSCR1) is characterized by its elevated expression in the cerebral tissues of individuals with Down syndrome on chromosome 21 (Baek et al., 2009). It is known to regulate angiogenesis and endothelial cell activation (Minami et al., 2009). Recent findings have also demonstrated its role as a regulatory factor in the development of MLVs (Choi et al., 2021). Investigations have revealed that overexpression of DSCR1 in AD mouse models promotes the development of dorsal MLVs, enhances the clearance of Aβ, and facilitates memory function. Mechanistically, DSCR1 exerts its effects through its downstream factor, Nuclear Factor of Activated T (NFAT) cells, which regulates the Wingless-Related Integration Site (Wnt) signaling pathway, thereby improving MLV function (Choi et al., 2021). Nevertheless, further exploration is required to elucidate the precise underlying mechanisms.
4.1.4 CGRP
Calcitonin Gene-Related Peptide (CGRP), derived from unique RNA splicing forms of the calcitonin gene, plays a significant role in disease pathogenesis by inducing vascular dilation and regulating inflammatory responses (Russo and Hay, 2023). In the context of chronic migraine, CGRP signaling impacts disease severity through modulation of immunocyte interactions and CSF outflow in MLVs (Nelson-Maney et al., 2024). Researchers have identified Calcitonin-Receptor-Like Receptor (CALCRL) and Receptor Activity-Modifying Protein 1 (RAMP1) as specific receptors for CGRP, which mediate biological functionality through receptor-ligand-initiated signal transduction (Zhang et al., 2016; Bowen et al., 2023). Anti-CGRP therapy involving the knock-out of Calcrl and Ramp1 genes has demonstrated efficacy in alleviating symptoms of chronic migraines by restoring CSF drainage function of MLVs (Nelson-Maney et al., 2024). However, further verification through clinical trials is needed to assess the effects of such anti-CGRP treatments on prolonged CSF outflow and related conditions.
4.1.5 THBS1
Thrombospondin-1 (THBS1) is an extracellular matrix protein and a member of the thrombospondin family, playing crucial roles in angiogenesis regulation, cell-signal transduction facilitation, and immune regulation (Kale et al., 2021). In the context of SAH, evidence reveals that red blood cells (RBCs) drain from MLVs to cLNs. When MLVs are obstructed, they exhibit exacerbated neuroinflammation, subsequently worsening neural consciousness (Chen et al., 2020). Maintaining clear MLV drainage, thus, holds substantial significance for the prognosis of SAH. Researchers have employed single-cell RNA sequencing and spatial transcriptomics technology to identify a strong correlation between calgranulin (S100A6) and THBS1 with SAH prognosis. THBS1 binds with CD47, causing apoptosis in LECs through Signal Transducer and Activator of Transcription 3 (STAT3)/B-Cell Lymphoma 2 (Bcl-2) signaling pathway as a regulatory mechanism (Wang et al., 2023). Hence, disrupting THBS1 and CD47 complexes via genetic or pharmacological methods such as gene editing, RNA interference, protein degradation, or competitive drug inhibition presents a promising strategy to restore MLV functionality, leading to improved SAH prognosis.
4.1.6 NET
Neutrophil extracellular traps (NETs) encompass web-shaped DNA structures released by activated neutrophils, which cannot be classified as intracellular proteins (Abaricia et al., 2021). Research has emphasized the significance of targeting NETs to regulate MLV functionality (Wang et al., 2021). NETs have been detected in brain tissue and cerebral microthrombi of patients with cerebrovascular diseases, showing an inverse correlation with patient prognosis (Kang et al., 2020; Denorme et al., 2022). In conditions of bacterial meningitis induced by pneumococcal infection, a high presence of NETs within brain tissue obstructs the exchange of CSF and ISF, as well as the inflow of CSF into cLNs. Treatment with DNase I to degrade NETs has shown improvements in the circulatory pathway between CSF-ISF-CSF-MLVs-dcLNs and amelioration of cerebral edema symptoms (Pavan et al., 2021). Complementary to this, another research team has identified similar complications related to NET-induced CSF circulation diseases in SAH and explored potential treatment options involving the administration of DNase I and anti-Ly6G antibodies. These treatments have led to enhanced CSF circulation, reduced formation of cerebral microthrombi, and favorable outcomes for mice prognosis (Hao et al., 2023). This team further investigated the mechanisms underlying the exacerbation of hydrocephalus following intraventricular hemorrhage caused by NETs dysfunctioning MLVs, impeding the flow of CSF and macromolecules into cLNs via MLVs. Mechanistically, the activation of lymphatic endothelial membrane protein Fractalkine Receptor (CX3CR1) by NETs triggers damage to lymphatic endothelia and the formation of thrombi within the MLVs (Zhang et al., 2024). However, further extensive exploration is warranted to elucidate the specific mechanisms underlying the interaction between NETs and CX3CR1. Specifically, researchers have found that genetically knocking out CX3CR1 and manipulating NET degradation with DNase I can effectively enhance lymphatic brain drainage and alleviate symptoms associated with hydrocephalus (Zhang et al., 2024). Therefore, targeting NETs through enzymatic degradation and genetic manipulation emerges as a promising therapeutic strategy for cerebral diseases.
4.2 Pharmacological regulation of MLVs
4.2.1 Atorvastatin
Atorvastatin, a well-established lipid-lowering medication, has been demonstrated to foster the resorption of subdural hematoma while mitigating the inflammation induced by the hematoma (Li et al., 2014; Quan et al., 2019). Recent investigations have revealed that following subdural hemorrhage in rats, endothelial cells in MLVs exhibit injuries characterized by dilated luminal spaces and disrupted interconnections. The underlying mechanism involves induced dephosphorylation of Extracellular Signal-Regulated Kinases 1 and 2 (ERK1/2) in meningeal LECs. The efficacy of Atorvastatin in reversing dephosphorylation in LECs has been ascertained, ameliorating basal MLV drainage and facilitating the clearance of subdural hematomas (Yuan et al., 2024).
4.2.2 Borneol
Borneol, a naturally occurring bicyclic monoterpene with high lipophilicity capable of crossing the blood-brain barrier (BBB), has recently been investigated (Li et al., 2012; Zhang et al., 2017). Upon oral administration of borneol microemulsion to mice, an increased clearance rate of Aβ from MLVs was observed, consequently facilitating the entry of large molecules into dcLNs and ameliorating the behavior and cognitive abilities in AD mice (Wu et al., 2023). Mechanistically, the application of borneol microemulsion was found to elevate the expression levels of Lymphatic Vessel Endothelial Hyaluronan Receptor 1 (LYVE-1), Forkhead Box C2 (FOXC2), and VEGF-C in the murine meninges, promoting the formation of MLVs while enhancing their diameter and permeability. Furthermore, borneol demonstrated its ability to decrease the levels of nitric oxide in the meninges, stimulating lymphatic vessel contraction and accelerating the clearance of Aβ (Wu et al., 2023). Hence, borneol emerges as a potential pharmacological agent capable of restoring MLV function and improving the prognosis for neurodegenerative diseases.
4.2.3 Vitamin D
Vitamin D is recognized for its endothelial-protective properties (Schroder-Heurich et al., 2019). Liu et al. (2020) observed that subdural hematoma clearance involves drainage into dcLNs via MLVs. Subsequent to subdural hematoma formation, the development and functionality of MLVs are compromised, resulting in a reduced drainage rate and downregulation of lymphangiogenesis-associated molecular markers such as VEGF-C, LYVE1 and FOXC2. In a study by Chen et al. (2024), the administration of Vitamin D to mice with subdural hematomas significantly decreased hematoma volume while facilitating hematoma drainage to dcLNs via MLVs. After treatment with Vitamin D, an upregulation of PROX1, LYVE1, and other markers related to lymphatic vessel functionality was observed alongside a decrease in the expression of inflammatory factors Interleukin-6 (IL-6), IL-8, and Tumor Necrosis Factor α (TNF-α). Therefore, it can be concluded that Vitamin D promotes absorption of hematomas and exerts anti-inflammatory effects by preserving MLV integrity.
4.3 Photobiomodulation therapy
With the advancement of precision medicine, there is an increasing demand for non-invasive and non-traumatic treatment modalities (Salehpour et al., 2022). PMT, an emerging approach for neuroprotection, employs visible and near-infrared light to stimulate the biochemical activities of mitochondrial components, thereby modulating cellular processes (Wong-Riley et al., 2005). Consequently, researchers have utilized non-invasive near-infrared light treatments to mediate cerebral diseases by regulating the lymphatic system (Salehpour et al., 2022). For instance, by directing near-infrared irradiation upon MLVs based on their superficial distribution characteristics, investigators observed improved cognitive function and reduced Aβ deposition in AD mice. This was attributed mechanistically to PMT’s regulation of mitochondrial metabolism and cell conjunction in meningeal LECs (Zinchenko et al., 2019; Wang M. et al., 2024). Additionally, near-infrared light irradiation of MLVs at doses of 5 and 10 J/cm2 demonstrated increased permeability of the lymphatic endothelium, promoting relaxation of lymphatic vessels and enhancing clearance and drainage of macromolecules within the brain (Semyachkina-Glushkovskaya et al., 2020). Furthermore, accelerating the elimination of RBCs is of utmost importance in alleviating complications arising from intraventricular hemorrhage in preterm infants. Following low-level infrared light stimulation of MLVs, it was discovered that the removal RBCs within the ventricles was accelerated, leading to their drainage to dcLNs through MLVs. This process effectively reduces the toxic side effects associated with intraventricular RBC retention and enhances neural function recovery after hemorrhage (Li et al., 2023). While PMT targeting MLVs holds significant implications for the treatment of brain diseases, further exploration is warranted due to current limitations such as cranial scattering effects, laser wavelength optimization, irradiation duration adjustment, and potential side reactions.
4.4 Immunotherapy for brain diseases mediated by MLVs
In the exploration of immunotherapeutic strategies for brain diseases, a growing body of research has substantiated the integral role played by MLVs in this field (Schiller et al., 2021). Subsequent sections will elucidate the immunomodulatory functions of MLVs in various cerebral diseases and how these pathways can be targeted to develop innovative therapeutic strategies.
Initially focusing on MLV-mediated immunotherapy in cerebral neoplasms, accumulating evidence suggests that VEGF-C signaling plays a crucial role in enhancing immune surveillance of brain tumors (Song et al., 2020). By delivering VEGF-C mRNA through an adenovirus vector, researchers have successfully enhanced specific expression of VEGF-C within the brain, thereby eliciting adaptive immune responses in deep lymph nodes. This cascade facilitates the migration of CD8+ T cells towards the microenvironment of glioblastomas via MLVs, ultimately reshaping the immune microenvironment with the ultimate goal being tumor destruction (Song et al., 2020). Moreover, the efficacy of inhibiting glioblastoma growth can be significantly boosted by combining immune checkpoint inhibitors with VEGF-C mRNA treatment (Song et al., 2020), offering new strategic avenues for GBM therapy. Further investigations have indicated that the conjunction of VEGF-C mRNA therapy with radiotherapy - by expanding MLVs - promotes dendritic cell migration and activates CD8+ T cells, thereby amplifying radiotherapy-induced antitumor immune responses (Zhou et al., 2022). However, whether MLVs can enhance the release of tumor-specific antigens induced by radiotherapy necessitates additional scrutiny.
In contrast to malignancies, treatment strategies for autoimmune disorders need the prevention of immune cell activation and brain invasion (Hsu et al., 2019). Take experimental autoimmune encephalomyelitis (EAE), a mouse model of multiple sclerosis, for instance; disease pathogenesis is accompanied by the development of lymph vessels at the cribriform plate. These vessels facilitate the transportation of inflammatory signals from the CNS to cLNs, triggering adaptive immune responses (Hsu et al., 2019). By utilizing MAZ51, a VEGFR3 tyrosine kinase inhibitor that hinders VEGFR3-dependent lymphangiogenesis and eliminates MLVs, researchers have observed a reduction in the severity of EAE and suppression of inflammatory reactions by brain-reactive T cells (Hsu et al., 2019; Li G. et al., 2022). Nevertheless, the long-term implications of MLV blockade on overall health continue to prompt further investigations. In addition, researchers have initiated a clinical trial (NCT05414487) utilizing Ofatumumab as an immunomodulatory drug for treating multiple sclerosis patients to explore its impact on MLVs and dynamic changes in immune cells. Consequently, scientists have dedicated significant efforts towards elucidating strategies for autoimmune disease treatment involving MLVs, yet additional research is still required.
In neurodegenerative diseases, such as AD, the immunological role mediated by MLVs remains crucial. Elderly mice often demonstrate functional impairment of MLVs, leading to the accumulation of misfolded proteins and metabolic waste within the brain parenchyma. This accumulation triggers neurodegenerative diseases (Da Mesquita et al., 2018). Rectifying this age-related dysfunction in older mice represents a promising therapeutic target for managing neurodegenerative diseases. Through single-cell RNA sequencing, researchers have identified prominent alterations in meningeal immunity among elderly mice. These alterations are characterized by a notable increase in Interferon γ (IFN-γ) expression primarily attributed to activated CD4+ T cells and CD8+ cytotoxic T cells, which ultimately affects CSF drainage through MLVs (Rustenhoven et al., 2023). Therefore, it has been demonstrated that the drainage function of MLVs can be significantly improved by peripherally administering anti-IFNγ neutralizing antibodies, verifying the reversibility of age-related MLV impairment (Rustenhoven et al., 2023).
In conclusion, MLVs are observed to play a multifaceted and intricate role in the therapeutic management of cerebral diseases. By fostering an in-depth comprehension of the functionality and regulatory mechanisms of MLVs under varying disease states, there lies potential for developing more precise and efficacious strategies to improve the quality of life for individuals diagnosed with brain diseases. Notably, VEGF-C has been recognized for its significant potential clinical translation among these therapeutic approaches. Copious research reports (Salvador et al., 2024), suggest that VEGF-C, as a well-established lymphangiogenic factor, stimulates growth and development of the lymphatic system and promotes MLVs’ drainage, consequently improving CNS disease pathology and clinical manifestations. However, transitioning these findings to clinical practice may likely necessitate additional scrutiny to ascertain the optimal therapeutic strategies, dosage, and safety.
5 Therapeutic drug delivery mediated by the CNS lymphatic system
5.1 Drug delivery mediated by MLVs
The existence of the BBB serves as a significant hindrance to the treatment of CNS diseases (Terstappen et al., 2021). MLVs, serving as conduits connecting the peripheral lymphatic system with the CNS (Aspelund et al., 2015), offer a potential pathway for delivering drugs to the intracranial space. Drug delivery through this route may hold the prospect of precision therapy for CNS diseases. In the treatment of glioblastoma, the injection of Poly(lactic-co-glycolic acid) (PLGA) nanoparticles loaded with Indocyanine Green (ICG) near the cLNs, it was found that the nanoparticles entered the MLVs, which then resulted in a 44-fold higher uptake of the nanoparticle drugs in the brain than conventional intravenous injection. The excitation of ICG by near-infrared light for photodynamic treatment of tumors demonstrated that the residual nanoparticles significantly inhibited glioblastoma growth (Zhao et al., 2020). Additionally, immune cell membranes have also been utilized to encapsulate drugs for delivery via MLVs to treat CNS diseases. For instance, curcumin encapsulated within natural killer cell membranes has been utilized in PD, and it was discovered that the encapsulated drug colocalized with MLVs marked by Lyve-1, with a marked enrichment observed within the brain (Liu et al., 2023). Additionally, research has demonstrated that Fibroblast Growth Factor-21 (FGF21), a neuroprotective factor (Chen et al., 2019), when encapsulated in BV2 cell membranes, similarly exhibits the ability to traverse the MLVs and target areas affected by AD. This approach has been shown to reduce the accumulation of tau protein and ameliorate neuroinflammation (Wang H. C. et al., 2024). Therefore, drug delivery mediated by the MLVs offers a novel approach for the treatment of CNS diseases; however, its feasibility and the underlying mechanisms necessitate further experimental validation.
5.2 Drug delivery mediated by the glymphatic system
In addition to transporting drugs from peripheral to intracranial locations, the conveyance of drugs from the CSF to the deep brain parenchyma also has significant implications. Studies have shown that systemic injections of hypertonic saline can enhance the binding of anti-Aβ antibodies to Aβ in AD mouse models, owing to a reduction in brain tissue volume caused by elevated plasma osmotic pressure. Interestingly, this effect enlarges the perivascular space and enhances glymphatic system function while leaving brain arterial volume unaffected (Plog et al., 2018). Similar effects of hypertonic saline have been substantiated in spinal drug treatments as well (Blomqvist et al., 2022). Furthermore, researchers have initiated investigations aiming to utilize the glymphatic system for directing nanoparticles from CSF into deep brain parenchymal regions. It has been established that following systemic injection of hypertonic saline and intrathecal administration of radioactively labeled gold nanoparticles, both single-photon emission computed tomography and MRI detected extensive distribution of nanoparticles within deep brain tissue regions, with rapid renal metabolic clearance occurring within 24 h (Lilius et al., 2023). Leveraging the perivascular space in the glymphatic system as a pathway for delivering immunopharmacological agents or nanodrugs into deep brain parenchymal regions presents a promising approach. These insights lay a solid foundation for new strategies involving intrathecal drug delivery assisted by the glymphatic system.
6 Discussion
This review proffers an overview of the therapeutic potential and challenges associated with applying the CNS lymphatic system in CNS diseases. Initially, a historical perspective on the discovery of the CNS lymphatic system is revisited, along with an examination of the anatomical features of the glymphatic system and MLVs, highlighting their crucial roles in waste clearance and immunological regulation. Dysfunctions within these systems are intrinsically linked to an array of CNS disorders, including AD and PD, among others. Subsequently, strategies aimed at modulating brain diseases are explored by enhancing the functionality of the CNS lymphatic system through adjustments in physiological factors, pharmaceutical interventions, and physical therapy methods. Lastly, the unique utility of the CNS lymphatic system in drug delivery provides novel strategies for circumventing BBB. Nevertheless, further research in this field is still in its early stages, necessitating additional experimental and clinical studies to confirm the clinical applicability of these findings.
The CNS lymphatic system holds significant therapeutic potential for intervening in CNS diseases. Despite extensive research efforts dedicated to investigating its physiological function, pathological mechanisms, and strategies for disease treatment, certain limitations persist that demand solutions. In terms of anatomical structure, it remains unclear whether MLVs exist in the pia mater and if their structure can be revealed using current electron microscopy technology. From a mechanistic standpoint, further investigation is required to elucidate the precise functional mechanisms of the CNS lymphatic system in CSF circulation, metabolic waste clearance, and immune regulation though non-invasive high-resolution imaging techniques. Addressing these inquiries would establish a theoretical foundation for implementing treatment strategies. Moreover, identifying novel therapeutic targets within the CNS lymphatic system is of significant importance for the development of new treatment approaches. Examination of applications suggests that further development of novel therapeutic strategies for CNS diseases, such as integrating nanotechnology and chemical synthesis technology in MLV-mediated nano-drug delivery, is warranted. Whereas, it is important to note the potential variability in therapeutic efficacy due to inter-individual differences in the CNS lymphatic system. Urgent questions remain regarding the differential role of the CNS lymphatic system under various disease conditions and how to personalize treatment plans according to individual variances.
Apart from mechanistic aspects of the CNS lymphatic system that beckon further exploration, there are inherent challenges within its clinical applications that must be overcome. Primarily, interventions in the CNS lymphatic system require rigorous examination through increased pre-clinical and clinical research to thoroughly evaluate the long-term safety and efficacy of these treatments. Furthermore, identifying optimal dosage for these therapies may present significant challenges, given that medication doses derived from animal models do not directly apply to humans. Patients may exhibit varied responses to treatments, differentiated drug absorption, and varied metabolic rates, necessitating the deployment of stringent, multi-centered, large-scale clinical trials to evaluate the medicinal dosage against corresponding efficacy. Notably, these therapeutic modalities frequently entail drug delivery challenges, given the unique characteristics of CNS disease sites, including the impediments posed by the BBB and the blood-cerebrospinal fluid barrier. The methodologies employed in animal models, such as craniotomy for the administration of therapeutics, are often not feasible for translation to human applications. Consequently, there is an imperative need to develop drug delivery systems that are tailored for human use, particularly for the delivery of labile genetic and nucleic acid therapeutic agents, which are susceptible to degradation. Research at present largely remains confined to the animal level, evidencing a glaring dearth of direct human study, notably in the relation between meningeal lymphatic function and human cognitive function.
In conclusion, the study of the CNS lymphatic system has not only augmented our comprehension of CNS diseases but also engendered potential for innovative treatment strategies. Future research agendas necessitate overcoming existing limitations, delving deeper into the roles and mechanisms of the CNS lymphatic system, as well as developing therapeutic strategies for CNS diseases. It is highly anticipated that continuous research and innovation will lead to more efficacious treatment modalities emerging for patients burdened with CNS afflictions.
Author contributions
RZ: Writing–original draft, Methodology, Investigation, Conceptualization. JL: Writing–original draft, Validation, Investigation. XL: Writing–original draft, Investigation. SZ: Writing–review and editing, Supervision.
Funding
The author(s) declare that no financial support was received for the research, authorship, and/or publication of this article.
Acknowledgments
I would like to thank Prof. D. F. of the editorial office–publishing development of Frontiers for reviewing my proposal for this review article and providing positive suggestions.
Conflict of interest
The authors declare that the research was conducted in the absence of any commercial or financial relationships that could be construed as a potential conflict of interest.
Publisher’s note
All claims expressed in this article are solely those of the authors and do not necessarily represent those of their affiliated organizations, or those of the publisher, the editors and the reviewers. Any product that may be evaluated in this article, or claim that may be made by its manufacturer, is not guaranteed or endorsed by the publisher.
References
Abaricia, J. O., Shah, A. H., and Olivares-Navarrete, R. (2021). Substrate stiffness induces neutrophil extracellular trap (NET) formation through focal adhesion kinase activation. Biomaterials 271, 120715. doi:10.1016/j.biomaterials.2021.120715
Absinta, M., Ha, S. K., Nair, G., Sati, P., Luciano, N. J., Palisoc, M., et al. (2017). Human and nonhuman primate meninges harbor lymphatic vessels that can be visualized noninvasively by MRI. Elife 6, e29738. doi:10.7554/eLife.29738
Ahn, J. H., Cho, H., Kim, J. H., Kim, S. H., Ham, J. S., Park, I., et al. (2019). Meningeal lymphatic vessels at the skull base drain cerebrospinal fluid. Nature 572 (7767), 62–66. doi:10.1038/s41586-019-1419-5
Albayram, M. S., Smith, G., Tufan, F., Tuna, I. S., Bostanciklioglu, M., Zile, M., et al. (2022). Non-invasive MR imaging of human brain lymphatic networks with connections to cervical lymph nodes. Nat. Commun. 13 (1), 203. doi:10.1038/s41467-021-27887-0
Antila, S., Chilov, D., Nurmi, H., Li, Z., Näsi, A., Gotkiewicz, M., et al. (2024). Sustained meningeal lymphatic vessel atrophy or expansion does not alter Alzheimer's disease-related amyloid pathology. Nat. Cardiovasc. Res. 3, 474–491. doi:10.1038/s44161-024-00445-9
Antila, S., Karaman, S., Nurmi, H., Airavaara, M., Voutilainen, M. H., Mathivet, T., et al. (2017). Development and plasticity of meningeal lymphatic vessels. J. Exp. Med. 214 (12), 3645–3667. doi:10.1084/jem.20170391
Aspelund, A., and Alitalo, K. (2024). Yoda1 opens the lymphatic path for craniosynostosis therapy. J. Clin. Invest. 134 (4), e176858. doi:10.1172/jci176858
Aspelund, A., Antila, S., Proulx, S. T., Karlsen, T. V., Karaman, S., Detmar, M., et al. (2015). A dural lymphatic vascular system that drains brain interstitial fluid and macromolecules. J. Exp. Med. 212 (7), 991–999. doi:10.1084/jem.20142290
Baek, K. H., Zaslavsky, A., Lynch, R. C., Britt, C., Okada, Y., Siarey, R. J., et al. (2009). Down's syndrome suppression of tumour growth and the role of the calcineurin inhibitor DSCR1. Nature 459 (7250), 1126–1130. doi:10.1038/nature08062
Blomqvist, K. J., Skogster, M. O. B., Kurkela, M. J., Rosenholm, M. P., Ahlstrom, F. H. G., Airavaara, M. T., et al. (2022). Systemic hypertonic saline enhances glymphatic spinal cord delivery of lumbar intrathecal morphine. J. Control Release 344, 214–224. doi:10.1016/j.jconrel.2022.03.022
Boisserand, L. S. B., Geraldo, L. H., Bouchart, J., El Kamouh, M. R., Lee, S., Sanganahalli, B. G., et al. (2024). VEGF-C prophylaxis favors lymphatic drainage and modulates neuroinflammation in a stroke model. J. Exp. Med. 221 (4), e20221983. doi:10.1084/jem.20221983
Bolte, A. C., Dutta, A. B., Hurt, M. E., Smirnov, I., Kovacs, M. A., McKee, C. A., et al. (2020). Meningeal lymphatic dysfunction exacerbates traumatic brain injury pathogenesis. Nat. Commun. 11 (1), 4524. doi:10.1038/s41467-020-18113-4
Bowen, A. J., Huang, Y. W., Chen, J. Y., Pauli, J. L., Campos, C. A., and Palmiter, R. D. (2023). Topographic representation of current and future threats in the mouse nociceptive amygdala. Nat. Commun. 14 (1), 196. doi:10.1038/s41467-023-35826-4
Chen, J., Wang, L., Xu, H., Xing, L., Zhuang, Z., Zheng, Y., et al. (2020). Meningeal lymphatics clear erythrocytes that arise from subarachnoid hemorrhage. Nat. Commun. 11 (1), 3159. doi:10.1038/s41467-020-16851-z
Chen, S., Chen, S. T., Sun, Y., Xu, Z., Wang, Y., Yao, S. Y., et al. (2019). Fibroblast growth factor 21 ameliorates neurodegeneration in rat and cellular models of Alzheimer's disease. Redox Biol. 22, 101133. doi:10.1016/j.redox.2019.101133
Chen, Y., Liu, X., Yuan, J., Dong, S., Nie, M., Jiang, W., et al. (2024). Vitamin D accelerates the subdural hematoma clearance through improving the meningeal lymphatic vessel function. Mol. Cell Biochem. doi:10.1007/s11010-023-04918-6
Choi, C., Park, J., Kim, H., Chang, K. T., Park, J., and Min, K. T. (2021). DSCR1 upregulation enhances dural meningeal lymphatic drainage to attenuate amyloid pathology of Alzheimer's disease. J. Pathol. 255 (3), 296–310. doi:10.1002/path.5767
Choi, D., Park, E., Choi, J., Lu, R., Yu, J. S., Kim, C., et al. (2024). Piezo1 regulates meningeal lymphatic vessel drainage and alleviates excessive CSF accumulation. Nat. Neurosci. 27 (5), 913–926. doi:10.1038/s41593-024-01604-8
Da Mesquita, S. (2022). Charting the meningeal lymphatic network. J. Exp. Med. 219 (8), e20220891. doi:10.1084/jem.20220891
Da Mesquita, S., Louveau, A., Vaccari, A., Smirnov, I., Cornelison, R. C., Kingsmore, K. M., et al. (2018). Functional aspects of meningeal lymphatics in ageing and Alzheimer's disease. Nature 560 (7717), 185–191. doi:10.1038/s41586-018-0368-8
Denorme, F., Portier, I., Rustad, J. L., Cody, M. J., de Araujo, C. V., Hoki, C., et al. (2022). Neutrophil extracellular traps regulate ischemic stroke brain injury. J. Clin. Invest. 132 (10), e154225. doi:10.1172/jci154225
Ding, X. B., Wang, X. X., Xia, D. H., Liu, H., Tian, H. Y., Fu, Y., et al. (2021). Impaired meningeal lymphatic drainage in patients with idiopathic Parkinson's disease. Nat. Med. 27 (3), 411–418. doi:10.1038/s41591-020-01198-1
Ding, Z., Fan, X., Zhang, Y., Yao, M., Wang, G., Dong, Y., et al. (2023). The glymphatic system: a new perspective on brain diseases. Front. Aging Neurosci. 15, 1179988. doi:10.3389/fnagi.2023.1179988
Fang, Y., Dai, S., Jin, C., Si, X., Gu, L., Song, Z., et al. (2021). Aquaporin-4 polymorphisms are associated with cognitive performance in Parkinson's disease. Front. Aging Neurosci. 13, 740491. doi:10.3389/fnagi.2021.740491
Fultz, N. E., Bonmassar, G., Setsompop, K., Stickgold, R. A., Rosen, B. R., Polimeni, J. R., et al. (2019). Coupled electrophysiological, hemodynamic, and cerebrospinal fluid oscillations in human sleep. Science 366 (6465), 628–631. doi:10.1126/science.aax5440
Hablitz, L. M., Pla, V., Giannetto, M., Vinitsky, H. S., Staeger, F. F., Metcalfe, T., et al. (2020). Circadian control of brain glymphatic and lymphatic fluid flow. Nat. Commun. 11 (1), 4411. doi:10.1038/s41467-020-18115-2
Hao, X., Zeng, Z., Liang, L., Feng, Z., Li, W., Xiong, B., et al. (2023). The role of neutrophil extracellular traps in early microthrombosis and brain injury after subarachnoid hemorrhage in mice. Transl. Stroke Res. 14 (5), 752–765. doi:10.1007/s12975-022-01074-9
Harrison, I. F., Ismail, O., Machhada, A., Colgan, N., Ohene, Y., Nahavandi, P., et al. (2020). Impaired glymphatic function and clearance of tau in an Alzheimer's disease model. Brain 143 (8), 2576–2593. doi:10.1093/brain/awaa179
He, X. F., Liu, D. X., Zhang, Q., Liang, F. Y., Dai, G. Y., Zeng, J. S., et al. (2017). Voluntary exercise promotes glymphatic clearance of amyloid beta and reduces the activation of astrocytes and microglia in aged mice. Front. Mol. Neurosci. 10, 144. doi:10.3389/fnmol.2017.00144
Hill, R. Z., Loud, M. C., Dubin, A. E., Peet, B., and Patapoutian, A. (2022). PIEZO1 transduces mechanical itch in mice. Nature 607 (7917), 104–110. doi:10.1038/s41586-022-04860-5
Hsu, M., Laaker, C., Madrid, A., Herbath, M., Choi, Y. H., Sandor, M., et al. (2022). Neuroinflammation creates an immune regulatory niche at the meningeal lymphatic vasculature near the cribriform plate. Nat. Immunol. 23 (4), 581–593. doi:10.1038/s41590-022-01158-6
Hsu, M., Rayasam, A., Kijak, J. A., Choi, Y. H., Harding, J. S., Marcus, S. A., et al. (2019). Neuroinflammation-induced lymphangiogenesis near the cribriform plate contributes to drainage of CNS-derived antigens and immune cells. Nat. Commun. 10 (1), 229. doi:10.1038/s41467-018-08163-0
Hu, X., Deng, Q., Ma, L., Li, Q., Chen, Y., Liao, Y., et al. (2020). Meningeal lymphatic vessels regulate brain tumor drainage and immunity. Cell Res. 30 (3), 229–243. doi:10.1038/s41422-020-0287-8
Hussain, R., Tithof, J., Wang, W., Cheetham-West, A., Song, W., Peng, W., et al. (2023). Potentiating glymphatic drainage minimizes post-traumatic cerebral oedema. Nature 623 (7989), 992–1000. doi:10.1038/s41586-023-06737-7
Iliff, J. J., Chen, M. J., Plog, B. A., Zeppenfeld, D. M., Soltero, M., Yang, L., et al. (2014). Impairment of glymphatic pathway function promotes tau pathology after traumatic brain injury. J. Neurosci. 34 (49), 16180–16193. doi:10.1523/jneurosci.3020-14.2014
Iliff, J. J., Wang, M., Liao, Y., Plogg, B. A., Peng, W., Gundersen, G. A., et al. (2012). A paravascular pathway facilitates CSF flow through the brain parenchyma and the clearance of interstitial solutes, including amyloid β. Sci. Transl. Med. 4 (147), 147ra111. doi:10.1126/scitranslmed.3003748
Iliff, J. J., Wang, M., Zeppenfeld, D. M., Venkataraman, A., Plog, B. A., Liao, Y., et al. (2013). Cerebral arterial pulsation drives paravascular CSF-interstitial fluid exchange in the murine brain. J. Neurosci. 33 (46), 18190–18199. doi:10.1523/jneurosci.1592-13.2013
Jacob, L., de Brito Neto, J., Lenck, S., Corcy, C., Benbelkacem, F., Geraldo, L. H., et al. (2022). Conserved meningeal lymphatic drainage circuits in mice and humans. J. Exp. Med. 219 (8), e20220035. doi:10.1084/jem.20220035
Kale, A., Rogers, N. M., and Ghimire, K. (2021). Thrombospondin-1 CD47 signalling: from mechanisms to medicine. Int. J. Mol. Sci. 22 (8), 4062. doi:10.3390/ijms22084062
Kang, L., Yu, H., Yang, X., Zhu, Y., Bai, X., Wang, R., et al. (2020). Neutrophil extracellular traps released by neutrophils impair revascularization and vascular remodeling after stroke. Nat. Commun. 11 (1), 2488. doi:10.1038/s41467-020-16191-y
Kim, J. H., Yoo, R. E., Choi, S. H., and Park, S. H. (2023). Non-invasive flow mapping of parasagittal meningeal lymphatics using 2D interslice flow saturation MRI. Fluids Barriers CNS 20 (1), 37. doi:10.1186/s12987-023-00446-z
Kress, B. T., Iliff, J. J., Xia, M., Wang, M., Wei, H. S., Zeppenfeld, D., et al. (2014). Impairment of paravascular clearance pathways in the aging brain. Ann. Neurol. 76 (6), 845–861. doi:10.1002/ana.24271
Lee, H., Xie, L., Yu, M., Kang, H., Feng, T., Deane, R., et al. (2015). The effect of body posture on brain glymphatic transport. J. Neurosci. 35 (31), 11034–11044. doi:10.1523/jneurosci.1625-15.2015
Li, D., Liu, S., Yu, T., Liu, Z., Sun, S., Bragin, D., et al. (2023). Photostimulation of brain lymphatics in male newborn and adult rodents for therapy of intraventricular hemorrhage. Nat. Commun. 14 (1), 6104. doi:10.1038/s41467-023-41710-y
Li, G., Cao, Y., Tang, X., Huang, J., Cai, L., and Zhou, L. (2022a). The meningeal lymphatic vessels and the glymphatic system: potential therapeutic targets in neurological disorders. J. Cereb. Blood Flow. Metab. 42 (8), 1364–1382. doi:10.1177/0271678x221098145
Li, T., Wang, D., Tian, Y., Yu, H., Wang, Y., Quan, W., et al. (2014). Effects of atorvastatin on the inflammation regulation and elimination of subdural hematoma in rats. J. Neurol. Sci. 341 (1-2), 88–96. doi:10.1016/j.jns.2014.04.009
Li, W., Chen, D., Liu, N., Luan, Y., Zhu, S., and Wang, H. (2022b). Modulation of lymphatic transport in the central nervous system. Theranostics 12 (3), 1117–1131. doi:10.7150/thno.66026
Li, W., Sun, B., Zhang, X., Liu, T., Zhu, W., Liu, X., et al. (2024). Near-infrared-II imaging revealed hypothermia regulates neuroinflammation following brain injury by increasing the glymphatic influx. ACS Nano 18 (21), 13836–13848. doi:10.1021/acsnano.4c02652
Li, W. R., Chen, R. Y., Yang, L., Huang, T. L., Xu, Q. W., Mi, S. Q., et al. (2012). Pharmacokinetics of natural borneol after oral administration in mice brain and its effect on excitation ratio. Eur. J. Drug Metab. Pharmacokinet. 37 (1), 39–44. doi:10.1007/s13318-011-0058-5
Li, X., Qi, L., Yang, D., Hao, S., Zhang, F., Zhu, X., et al. (2022c). Meningeal lymphatic vessels mediate neurotropic viral drainage from the central nervous system. Nat. Neurosci. 25 (5), 577–587. doi:10.1038/s41593-022-01063-z
Lilius, T. O., Mortensen, K. N., Deville, C., Lohela, T. J., Staeger, F. F., Sigurdsson, B., et al. (2023). Glymphatic-assisted perivascular brain delivery of intrathecal small gold nanoparticles. J. Control Release 355, 135–148. doi:10.1016/j.jconrel.2023.01.054
Lin, Y., Jin, J., Lv, R., Luo, Y., Dai, W., Li, W., et al. (2021). Repetitive transcranial magnetic stimulation increases the brain's drainage efficiency in a mouse model of Alzheimer's disease. Acta Neuropathol. Commun. 9 (1), 102. doi:10.1186/s40478-021-01198-3
Liu, J., Gao, D., Hu, D., Lan, S., Liu, Y., Zheng, H., et al. (2023). Delivery of biomimetic liposomes via meningeal lymphatic vessels route for targeted therapy of Parkinson’s disease. Res. (Wash D C) 6, 0030. doi:10.34133/research.0030
Liu, S., Sun, X., Ren, Q., Chen, Y., Dai, T., Yang, Y., et al. (2024). Glymphatic dysfunction in patients with early-stage amyotrophic lateral sclerosis. Brain 147 (1), 100–108. doi:10.1093/brain/awad274
Liu, X., Gao, C., Yuan, J., Xiang, T., Gong, Z., Luo, H., et al. (2020). Subdural haematomas drain into the extracranial lymphatic system through the meningeal lymphatic vessels. Acta Neuropathol. Commun. 8 (1), 16. doi:10.1186/s40478-020-0888-y
Lohela, T. J., Lilius, T. O., and Nedergaard, M. (2022). The glymphatic system: implications for drugs for central nervous system diseases. Nat. Rev. Drug Discov. 21 (10), 763–779. doi:10.1038/s41573-022-00500-9
Louveau, A., Harris, T. H., and Kipnis, J. (2015a). Revisiting the mechanisms of CNS immune privilege. Trends Immunol. 36 (10), 569–577. doi:10.1016/j.it.2015.08.006
Louveau, A., Smirnov, I., Keyes, T. J., Eccles, J. D., Rouhani, S. J., Peske, J. D., et al. (2015b). Structural and functional features of central nervous system lymphatic vessels. Nature 523 (7560), 337–341. doi:10.1038/nature14432
Ma, L., Chang, Q., Pei, F., Liu, M., Zhang, W., Hong, Y. K., et al. (2023). Skull progenitor cell-driven meningeal lymphatic restoration improves neurocognitive functions in craniosynostosis. Cell Stem Cell 30 (11), 1472–1485.e7. doi:10.1016/j.stem.2023.09.012
Maliszewska-Cyna, E., Xhima, K., and Aubert, I. (2016). A comparative study evaluating the impact of physical exercise on disease progression in a mouse model of alzheimer's disease. J. Alzheimers Dis. 53 (1), 243–257. doi:10.3233/jad-150660
Matrongolo, M. J., Ang, P. S., Wu, J., Jain, A., Thackray, J. K., Reddy, A., et al. (2023). Piezo1 agonist restores meningeal lymphatic vessels, drainage, and brain- CSF perfusion in craniosynostosis and aged mice. J. Clin. Invest. 134 (4), e171468. doi:10.1172/jci171468
Mestre, H., Hablitz, L. M., Xavier, A. L., Feng, W., Zou, W., Pu, T., et al. (2018). Aquaporin-4-dependent glymphatic solute transport in the rodent brain. Elife 7, e40070. doi:10.7554/eLife.40070
Minami, T., Yano, K., Miura, M., Kobayashi, M., Suehiro, J., Reid, P. C., et al. (2009). The Down syndrome critical region gene 1 short variant promoters direct vascular bed-specific gene expression during inflammation in mice. J. Clin. Invest. 119 (8), 2257–2270. doi:10.1172/jci35738
Muccio, M., Chu, D., Minkoff, L., Kulkarni, N., Damadian, B., Damadian, R. V., et al. (2021). Upright versus supine MRI: effects of body position on craniocervical CSF flow. Fluids Barriers CNS 18 (1), 61. doi:10.1186/s12987-021-00296-7
Murdock, M. H., Yang, C. Y., Sun, N., Pao, P. C., Blanco-Duque, C., Kahn, M. C., et al. (2024). Multisensory gamma stimulation promotes glymphatic clearance of amyloid. Nature 627 (8002), 149–156. doi:10.1038/s41586-024-07132-6
Nedergaard, M., and Goldman, S. A. (2020). Glymphatic failure as a final common pathway to dementia. Science 370 (6512), 50–56. doi:10.1126/science.abb8739
Nelson-Maney, N. P., Balint, L., Beeson, A. L., Serafin, D. S., Kistner, B. M., Douglas, E. S., et al. (2024). Meningeal lymphatic CGRP signaling governs pain via cerebrospinal fluid efflux and neuroinflammation in migraine models. J. Clin. Invest. 134, e175616. doi:10.1172/jci175616
Oliver, G., Kipnis, J., Randolph, G. J., and Harvey, N. L. (2020). The lymphatic vasculature in the 21(st) century: novel functional roles in homeostasis and disease. Cell 182 (2), 270–296. doi:10.1016/j.cell.2020.06.039
Pavan, C., Xavier, A. L. R., Ramos, M., Fisher, J., Kritsilis, M., Linder, A., et al. (2021). DNase treatment prevents cerebrospinal fluid block in early experimental pneumococcal meningitis. Ann. Neurol. 90 (4), 653–669. doi:10.1002/ana.26186
Peng, S., Liu, J., Liang, C., Yang, L., and Wang, G. (2023). Aquaporin-4 in glymphatic system, and its implication for central nervous system disorders. Neurobiol. Dis. 179, 106035. doi:10.1016/j.nbd.2023.106035
Persson, N. D. A., Uusalo, P., Nedergaard, M., Lohela, T. J., and Lilius, T. O. (2022). Could dexmedetomidine be repurposed as a glymphatic enhancer? Trends Pharmacol. Sci. 43 (12), 1030–1040. doi:10.1016/j.tips.2022.09.007
Petrova, T. V., and Koh, G. Y. (2020). Biological functions of lymphatic vessels. Science 369 (6500), eaax4063. doi:10.1126/science.aax4063
Plog, B. A., Mestre, H., Olveda, G. E., Sweeney, A. M., Kenney, H. M., Cove, A., et al. (2018). Transcranial optical imaging reveals a pathway for optimizing the delivery of immunotherapeutics to the brain. JCI Insight 3 (20), e120922. doi:10.1172/jci.insight.120922
Plog, B. A., and Nedergaard, M. (2018). The glymphatic system in central nervous system health and disease: past, present, and future. Annu. Rev. Pathol. 13, 379–394. doi:10.1146/annurev-pathol-051217-111018
Pu, T., Zou, W., Feng, W., Zhang, Y., Wang, L., Wang, H., et al. (2019). Persistent malfunction of glymphatic and meningeal lymphatic drainage in a mouse model of subarachnoid hemorrhage. Exp. Neurobiol. 28 (1), 104–118. doi:10.5607/en.2019.28.1.104
Quan, W., Zhang, Z., Li, P., Tian, Q., Huang, J., Qian, Y., et al. (2019). Role of regulatory T cells in atorvastatin induced absorption of chronic subdural hematoma in rats. Aging Dis. 10 (5), 992–1002. doi:10.14336/ad.2018.0926
Rasmussen, M. K., Mestre, H., and Nedergaard, M. (2018). The glymphatic pathway in neurological disorders. Lancet Neurol. 17 (11), 1016–1024. doi:10.1016/s1474-4422(18)30318-1
Rasmussen, M. K., Mestre, H., and Nedergaard, M. (2022). Fluid transport in the brain. Physiol. Rev. 102 (2), 1025–1151. doi:10.1152/physrev.00031.2020
Rennels, M. L., Gregory, T. F., Blaumanis, O. R., Fujimoto, K., and Grady, P. A. (1985). Evidence for a 'paravascular' fluid circulation in the mammalian central nervous system, provided by the rapid distribution of tracer protein throughout the brain from the subarachnoid space. Brain Res. 326 (1), 47–63. doi:10.1016/0006-8993(85)91383-6
Russo, A. F., and Hay, D. L. (2023). CGRP physiology, pharmacology, and therapeutic targets: migraine and beyond. Physiol. Rev. 103 (2), 1565–1644. doi:10.1152/physrev.00059.2021
Rustenhoven, J., Drieu, A., Mamuladze, T., de Lima, K. A., Dykstra, T., Wall, M., et al. (2021). Functional characterization of the dural sinuses as a neuroimmune interface. Cell 184 (4), 1000–1016.e27. doi:10.1016/j.cell.2020.12.040
Rustenhoven, J., Pavlou, G., Storck, S. E., Dykstra, T., Du, S., Wan, Z., et al. (2023). Age-related alterations in meningeal immunity drive impaired CNS lymphatic drainage. J. Exp. Med. 220 (7), e20221929. doi:10.1084/jem.20221929
Salehpour, F., Khademi, M., Bragin, D. E., and DiDuro, J. O. (2022). Photobiomodulation therapy and the glymphatic system: promising applications for augmenting the brain lymphatic drainage system. Int. J. Mol. Sci. 23 (6), 2975. doi:10.3390/ijms23062975
Salvador, A. F. M., Abduljawad, N., and Kipnis, J. (2024). Meningeal lymphatics in central nervous system diseases. Annu. Rev. Neurosci. 47, 323–344. doi:10.1146/annurev-neuro-113023-103045
Sandrone, S., Moreno-Zambrano, D., Kipnis, J., and van Gijn, J. (2019). A (delayed) history of the brain lymphatic system. Nat. Med. 25 (4), 538–540. doi:10.1038/s41591-019-0417-3
Schiller, M., Ben-Shaanan, T. L., and Rolls, A. (2021). Neuronal regulation of immunity: why, how and where? Nat. Rev. Immunol. 21 (1), 20–36. doi:10.1038/s41577-020-0387-1
Schroder-Heurich, B., von Hardenberg, S., Brodowski, L., Kipke, B., Meyer, N., Borns, K., et al. (2019). Vitamin D improves endothelial barrier integrity and counteracts inflammatory effects on endothelial progenitor cells. Faseb J. 33 (8), 9142–9153. doi:10.1096/fj.201802750RR
Semyachkina-Glushkovskaya, O., Abdurashitov, A., Dubrovsky, A., Klimova, M., Agranovich, I., Terskov, A., et al. (2020). Photobiomodulation of lymphatic drainage and clearance: perspective strategy for augmentation of meningeal lymphatic functions. Biomed. Opt. Express 11 (2), 725–734. doi:10.1364/boe.383390
Shokri-Kojori, E., Wang, G. J., Wiers, C. E., Demiral, S. B., Guo, M., Kim, S. W., et al. (2018). β-Amyloid accumulation in the human brain after one night of sleep deprivation. Proc. Natl. Acad. Sci. U. S. A. 115 (17), 4483–4488. doi:10.1073/pnas.1721694115
Si, X., Dai, S., Fang, Y., Tang, J., Wang, Z., Li, Y., et al. (2024). Matrix metalloproteinase-9 inhibition prevents aquaporin-4 depolarization-mediated glymphatic dysfunction in Parkinson's disease. J. Adv. Res. 56, 125–136. doi:10.1016/j.jare.2023.03.004
Smyth, L. C. D., Xu, D., Okar, S. V., Dykstra, T., Rustenhoven, J., Papadopoulos, Z., et al. (2024). Identification of direct connections between the dura and the brain. Nature 627 (8002), 165–173. doi:10.1038/s41586-023-06993-7
Song, E., Mao, T., Dong, H., Boisserand, L. S. B., Antila, S., Bosenberg, M., et al. (2020). VEGF-C-driven lymphatic drainage enables immunosurveillance of brain tumours. Nature 577 (7792), 689–694. doi:10.1038/s41586-019-1912-x
Sun, B., Fang, D., Li, W., Li, M., and Zhu, S. (2024). NIR-II nanoprobes for investigating the glymphatic system function under anesthesia and stroke injury. J. Nanobiotechnology 22 (1), 200. doi:10.1186/s12951-024-02481-w
Sun, B. L., Wang, L. H., Yang, T., Sun, J. Y., Mao, L. L., Yang, M. F., et al. (2018). Lymphatic drainage system of the brain: a novel target for intervention of neurological diseases. Prog. Neurobiol. 163-164, 118–143. doi:10.1016/j.pneurobio.2017.08.007
Terstappen, G. C., Meyer, A. H., Bell, R. D., and Zhang, W. (2021). Strategies for delivering therapeutics across the blood-brain barrier. Nat. Rev. Drug Discov. 20 (5), 362–383. doi:10.1038/s41573-021-00139-y
Thrane, A. S., Rangroo Thrane, V., and Nedergaard, M. (2014). Drowning stars: reassessing the role of astrocytes in brain edema. Trends Neurosci. 37 (11), 620–628. doi:10.1016/j.tins.2014.08.010
Wang, H. C., Yang, W., Xu, L., Han, Y. H., Lin, Y., Lu, C. T., et al. (2024a). BV2 membrane-coated PEGylated-liposomes delivered hFGF21 to cortical and hippocampal microglia for alzheimer's disease therapy. Adv. Healthc. Mater. 13 (19), e2400125. doi:10.1002/adhm.202400125
Wang, L., Zhang, Y., Zhao, Y., Marshall, C., Wu, T., and Xiao, M. (2019). Deep cervical lymph node ligation aggravates AD-like pathology of APP/PS1 mice. Brain Pathol. 29 (2), 176–192. doi:10.1111/bpa.12656
Wang, M., Yan, C., Li, X., Yang, T., Wu, S., Liu, Q., et al. (2024b). Non-invasive modulation of meningeal lymphatics ameliorates ageing and Alzheimer's disease-associated pathology and cognition in mice. Nat. Commun. 15 (1), 1453. doi:10.1038/s41467-024-45656-7
Wang, R., Zhu, Y., Liu, Z., Chang, L., Bai, X., Kang, L., et al. (2021). Neutrophil extracellular traps promote tPA-induced brain hemorrhage via cGAS in mice with stroke. Blood 138 (1), 91–103. doi:10.1182/blood.2020008913
Wang, X., Zhang, A., Yu, Q., Wang, Z., Wang, J., Xu, P., et al. (2023). Single-cell RNA sequencing and spatial transcriptomics reveal pathogenesis of meningeal lymphatic dysfunction after experimental subarachnoid hemorrhage. Adv. Sci. (Weinh) 10 (21), e2301428. doi:10.1002/advs.202301428
Welch, K., and Friedman, V. (1960). The cerebrospinal fluid valves. Brain 83, 454–469. doi:10.1093/brain/83.3.454
Wolpow, E. R., and Schaumburg, H. H. (1972). Structure of the human arachnoid granulation. J. Neurosurg. 37 (6), 724–727. doi:10.3171/jns.1972.37.6.0724
Wong-Riley, M. T., Liang, H. L., Eells, J. T., Chance, B., Henry, M. M., Buchmann, E., et al. (2005). Photobiomodulation directly benefits primary neurons functionally inactivated by toxins: role of cytochrome c oxidase. J. Biol. Chem. 280 (6), 4761–4771. doi:10.1074/jbc.M409650200
Wu, C. H., Chang, F. C., Wang, Y. F., Lirng, J. F., Wu, H. M., Pan, L. H., et al. (2024). Impaired glymphatic and meningeal lymphatic functions in patients with chronic migraine. Ann. Neurol. 95 (3), 583–595. doi:10.1002/ana.26842
Wu, Y., Zhang, T., Li, X., Wei, Y., Li, X., Wang, S., et al. (2023). Borneol-driven meningeal lymphatic drainage clears amyloid-β peptide to attenuate Alzheimer-like phenotype in mice. Theranostics 13 (1), 106–124. doi:10.7150/thno.76133
Xie, L., Kang, H., Xu, Q., Chen, M. J., Liao, Y., Thiyagarajan, M., et al. (2013). Sleep drives metabolite clearance from the adult brain. Science 342 (6156), 373–377. doi:10.1126/science.1241224
Yanev, P., Poinsatte, K., Hominick, D., Khurana, N., Zuurbier, K. R., Berndt, M., et al. (2020). Impaired meningeal lymphatic vessel development worsens stroke outcome. J. Cereb. Blood Flow. Metab. 40 (2), 263–275. doi:10.1177/0271678x18822921
Yang, J., Cao, C., Liu, J., Liu, Y., Lu, J., Yu, H., et al. (2024). Dystrophin 71 deficiency causes impaired aquaporin-4 polarization contributing to glymphatic dysfunction and brain edema in cerebral ischemia. Neurobiol. Dis. 199, 106586. doi:10.1016/j.nbd.2024.106586
Yoon, J. H., Jin, H., Kim, H. J., Hong, S. P., Yang, M. J., Ahn, J. H., et al. (2024). Nasopharyngeal lymphatic plexus is a hub for cerebrospinal fluid drainage. Nature 625 (7996), 768–777. doi:10.1038/s41586-023-06899-4
Yuan, J., Liu, X., Nie, M., Chen, Y., Liu, M., Huang, J., et al. (2024). Inactivation of ERK1/2 signaling mediates dysfunction of basal meningeal lymphatic vessels in experimental subdural hematoma. Theranostics 14 (1), 304–323. doi:10.7150/thno.87633
Zhang, Q., Chen, Y., Li, Y., Feng, Z., Liang, L., Hao, X., et al. (2024). Neutrophil extracellular trap-mediated impairment of meningeal lymphatic drainage exacerbates secondary hydrocephalus after intraventricular hemorrhage. Theranostics 14 (5), 1909–1938. doi:10.7150/thno.91653
Zhang, X., Hou, L., Li, F., Zhang, W., Wu, C., Xiang, L., et al. (2022). Piezo1-mediated mechanosensation in bone marrow macrophages promotes vascular niche regeneration after irradiation injury. Theranostics 12 (4), 1621–1638. doi:10.7150/thno.64963
Zhang, X. G., Shan, C., Zhu, J. Z., Bao, X. Y., Tong, Q., Wu, X. F., et al. (2017). Additive neuroprotective effect of borneol with mesenchymal stem cells on ischemic stroke in mice. Front. Physiol. 8, 1133. doi:10.3389/fphys.2017.01133
Zhang, Y., Xu, J., Ruan, Y. C., Yu, M. K., O'Laughlin, M., Wise, H., et al. (2016). Implant-derived magnesium induces local neuronal production of CGRP to improve bone-fracture healing in rats. Nat. Med. 22 (10), 1160–1169. doi:10.1038/nm.4162
Zhao, P., Le, Z., Liu, L., and Chen, Y. (2020). Therapeutic delivery to the brain via the lymphatic vasculature. Nano Lett. 20 (7), 5415–5420. doi:10.1021/acs.nanolett.0c01806
Zhou, C., Ma, L., Xu, H., Huo, Y., and Luo, J. (2022). Meningeal lymphatics regulate radiotherapy efficacy through modulating anti-tumor immunity. Cell Res. 32 (6), 543–554. doi:10.1038/s41422-022-00639-5
Zinchenko, E., Navolokin, N., Shirokov, A., Khlebtsov, B., Dubrovsky, A., Saranceva, E., et al. (2019). Pilot study of transcranial photobiomodulation of lymphatic clearance of beta-amyloid from the mouse brain: breakthrough strategies for non-pharmacologic therapy of Alzheimer's disease. Biomed. Opt. Express 10 (8), 4003–4017. doi:10.1364/boe.10.004003
Keywords: meningeal lymphatic vessels, glymphatic system, CNS diseases, drug delivery, neurodegenerative diseases
Citation: Zhang R, Li J, Li X and Zhang S (2024) Therapeutic approaches to CNS diseases via the meningeal lymphatic and glymphatic system: prospects and challenges. Front. Cell Dev. Biol. 12:1467085. doi: 10.3389/fcell.2024.1467085
Received: 19 July 2024; Accepted: 28 August 2024;
Published: 06 September 2024.
Edited by:
Zoltán Jakus, Semmelweis University, HungaryReviewed by:
Laszlo Balint, University of North Carolina at Chapel Hill, United StatesMiriam Echevarria Irusta, Instituto de Biomedicina de Sevilla (IBIS), Spain
Copyright © 2024 Zhang, Li, Li and Zhang. This is an open-access article distributed under the terms of the Creative Commons Attribution License (CC BY). The use, distribution or reproduction in other forums is permitted, provided the original author(s) and the copyright owner(s) are credited and that the original publication in this journal is cited, in accordance with accepted academic practice. No use, distribution or reproduction is permitted which does not comply with these terms.
*Correspondence: Si Zhang, emhhbmdzaUB3Y2hzY3UuY24=
†These authors have contributed equally to this work