- 1Department of Integrative Immunobiology, Duke University School of Medicine, Durham, United States
- 2Department of Molecular Biology and Biochemistry, Sue and Bill Gross Stem Cell Research Center, University of California, Irvine, United States
Cancer is a complex disease characterized by specific “mission-critical” events that drive the uncontrolled growth and spread of tumor cells and their offspring. These events are essential for the advancement of the disease. One of the main contributors to these events is dysregulation of cell death pathways—such as apoptosis, necroptosis, ferroptosis, autophagy, pyroptosis, cuproptosis, parthanatos and—allows cancer cells to avoid programmed cell death and continue proliferating unabated. The different cell death pathways in cancers provide useful targets for cancer treatment. This review examines recent progresses in the preclinical and clinical development of targeting dysregulated cell death pathways for cancer treatment. To develop effective cancer therapies, it is essential to identify and target these mission-critical events that prevent tumor cells from timely death. By precisely targeting these crucial events, researchers can develop therapies with maximum impact and minimal side effects. A comprehensive understanding of the molecular and cellular mechanisms underlying these regulated cell death pathways will further the development of highly effective and personalized cancer treatments.
1 Introduction
Cancer, an intricate disease characterized by uncontrolled cell proliferation and evasion of regulated cell death mechanisms, is a significant global health concern (Brown et al., 2023; Bhat et al., 2024). Among the several cellular mechanisms disrupted in cancer, the regulation of cell death pathways is crucial (Peng et al., 2022; Tong et al., 2022; Gong et al., 2023; Hadian and Stockwell, 2023). Programmed cell death (PCD), also known as regulated cell death (RCD), is a genetically controlled process in which cells die in an orderly manner (Koren and Fuchs, 2021; Gong et al., 2023). RCD encompasses several mechanisms, including apoptosis, necroptosis, autophagy and the newly identified pathways of pyroptosis, ferroptosis, cuproptosis, and parthanatos (Galluzzi et al., 2018) (Figure 1). Each of these mechanisms is crucial for maintaining cellular balance and responding to cellular stress (Tang et al., 2019; Lamichhane and Samir, 2023). When mammalian cells experience irreversible disruptions in their internal or external milieu, they can initiate several signal transduction cascades that ultimately result in cell death (Kayagaki et al., 2024; Newton et al., 2024). In cancer, the disruption of these pathways not only enables the initiation and progression of tumors but also significantly affects treatment resistance and patient outcomes (Table 1) (Gong et al., 2023). Each of these RCD patterns is triggered and propagated through molecular pathways that exhibit significant connectivity (Tang et al., 2019) (Figure 2). Each variant of RCD exhibits a diverse array of morphological characteristics, ranging from complete to partial programmed cell death, which elicit unique immunomodulatory properties, including anti-inflammatory effects, promotion of immune tolerance, enhancement of inflammation, and immunogenicity. Apoptosis, marked by regulated cell shrinkage and membrane blebbing, typically leads to anti-inflammatory outcomes since apoptotic cells are phagocytosed without provoking immune activation (Elmore, 2007). Autophagy is a process of cellular degradation that generally promotes cell survival; however, under prolonged stress, it can result in cell death. Autophagy can either suppress or promote inflammation based on the context, as it regulates the immune response by degrading immune modulators or releasing signals that activate immune cells (Liu et al., 2023). Necroptosis, characterized by membrane rupture and the release of cellular contents, triggers inflammation by activating immune cells via damage-associated molecular patterns (DAMPs) (Kaczmarek et al., 2013). In a similar manner, pyroptosis, characterized by pore formation and cell lysis, enhances inflammation through the release of pro-inflammatory cytokines such as IL-1β (Liu Y. et al., 2024). Cuproptosis, a form of cell death that relies on copper, inflicts damage on the mitochondria and has the potential to trigger immune responses, although its specific immunomodulatory characteristics are still under investigation (Springer et al., 2024). Ferroptosis, initiated by iron-dependent lipid peroxidation, has the potential to promote inflammation via the release of DAMPs, which in turn can affect immune responses (Qi and Peng, 2023). Parthanatos, resulting from excessive PARP activation that leads to significant DNA damage, can trigger inflammation while potentially fostering immune tolerance in chronic conditions (Huang et al., 2022). In summary, these RCD pathways influence immune dynamics by either inhibiting or facilitating immune activation, thereby affecting cancer progression and treatment results. (Galluzzi et al., 2018; Liao M. et al., 2022).
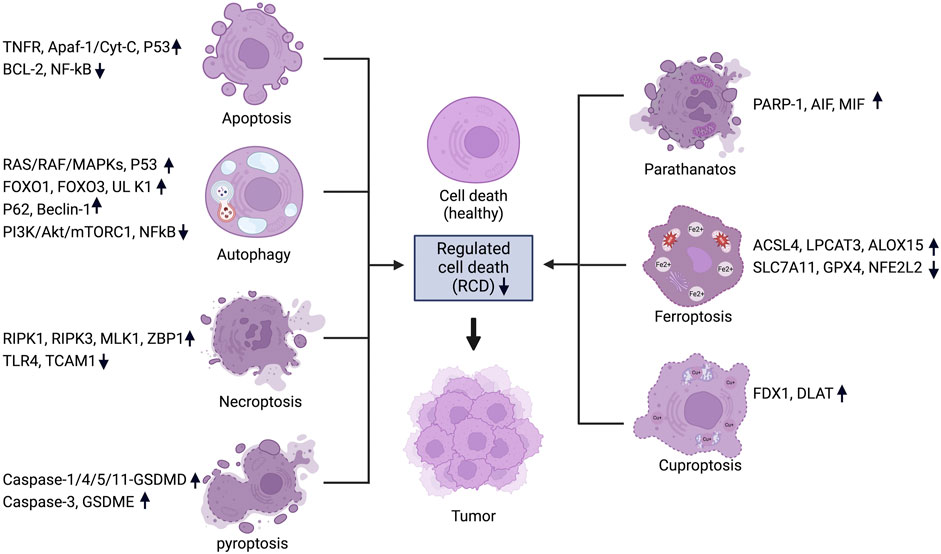
Figure 1. Regulated cell death pathways in cancer and their associated genes. For each RCD pathway, a set of key regulators are listed and the change of expression levels are indicated. Figure created using Biorender.
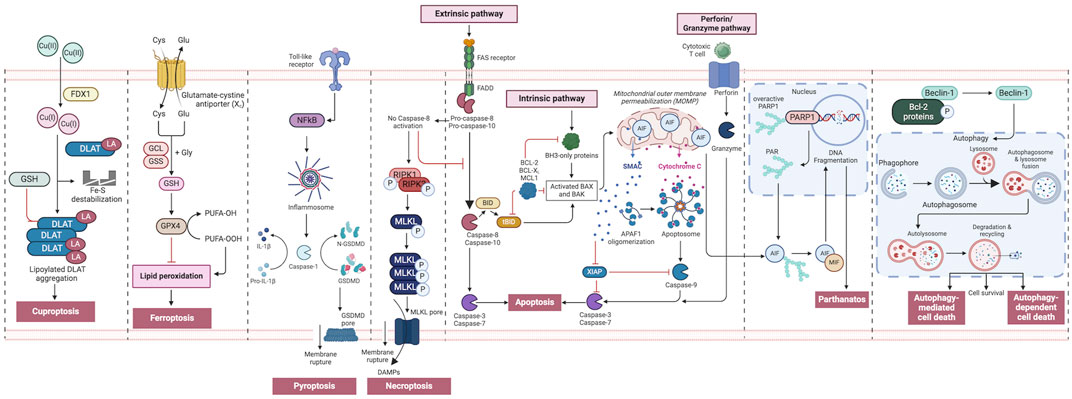
Figure 2. An overview of regulated cell death processes. A summary of the RCD pathways involved in cancer pathogenesis. Intrinsic apoptosis: Following an inherent fatal signal, BH3-only proteins activate BAX and BAK either directly or indirectly by binding to and blocking BCL-2 proteins. The mitochondrial outer membrane is then permeabilized (MOMP), releasing cytochrome C (Cyt C) and SMAC, the latter of which can suppress apoptosis. The apoptosome is subsequently produced, which activates caspase-9, followed by caspases 3 and 7, and initiates apoptosis. Extrinsic apoptosis: When death receptors (TNFR1, FAS, or TRAIL-R) receive an extrinsic fatal signal, they join with pro-caspase-8 and -10 to create complex I. Complex IIa is then generated, resulting in caspase-8 and -10 activation. Apoptosis is then initiated either directly by cleaving caspases-3 and -7, or indirectly by cleaving BID into tBID and activating BAX and BAK. Granzyme pathway: Cytotoxic T-cells are the main controllers for the granzyme pathway, which results in caspase-10 activation that in turn activates caspase-3. Granzyme B can activate caspases in the targeted cell. Necroptosis occurs when an extrinsic fatal signal is received but caspase-8 is not activated. Complex IIb (also known as the necrosome) is generated. This causes RIPK 1 and 3 to phosphorylate and activate mixed lineage kinase domain-like pseudokinase (MLKL). MLKL then forms a complex, causing the release of cytokines, chemokines, and damage-associated molecular patterns (DAMPS). Finally, this causes inflammation and necroptosis of the cell. Pyroptosis occurs when toll-like receptors (e.g., TLR4) detect an external fatal signal. Nuclear factor kappa B (NF-KB) signaling is initiated. This causes inflammasome development and subsequent caspase-1 activation. Then, pro-IL-1b is converted to active IL-1b, and gasdermin D (GSDMD) is broken down into N-GSDMD fragments resulting in inflammation and pyroptosis of the cell. Parthanatos occurs when an inherent fatal signal arises (for example, high reactive oxygen species accumulation), poly [ADP-ribose] polymerase 1 (PARP-1) is activated. Overactivation of PARP-1 can result in the accumulation of PAR polymer and the translocation of apoptosis inhibitory factor (AIF) from the mitochondria. AIF forms a compound with macrophage migration inhibitory factor (MIF) and re-enters the nucleus. Ultimately, this leads to cell parthanatos and DNA fragmentation. Autophagic Cell Death: Beclin-1 generally forms a complex with Bcl-2 proteins. After they have been phosphorylated and inactivated, free Beclin-1 can start autophagy. Ferroptosis occurs exclusively when there is an imbalance in the regulatory system, leading to the accumulation of lipid peroxide to a lethal threshold. Transferrin (TF) binds to extracellular Fe3+ and facilitates its transport into cells via transferrin receptor 1 (TfR1), where it is then converted to Fe2+. Later, intracellular divalent metal transporter 1 (DMT1) and zinc transporter 8/14 (ZIP8/14) store the Fe2+ in the intracellular labile iron pool (LIP). Fe2+ transfers electrons through the Fenton reaction with peroxide, resulting in the production of oxidizing free radicals. Following an excessive accumulation of iron within cells, numerous free radicals interact with polyunsaturated fatty acids (PUFA) found in the phospholipids of cell membranes resulting in the formation of lipid peroxides, which ultimately lead to cell death. The intracellular antioxidant stress system depends on GPX4 to eliminate surplus lipid peroxides. The Cystine/glutamate antiport (system xc−) facilitates the movement of glutamate from within cells to the outside, while simultaneously transporting cystine from the outside into cells. Cuproptosis: FDX1 plays a crucial role as a copper ion carrier in the induction of cell death and is involved in the regulation of protein lipoylation. Elevated copper levels foster the accumulation and functional impairment of lipoylated proteins, leading to instability of iron–sulfur cluster proteins, protein toxicity stress, and ultimately cell death. In addition, excessive copper binds to lipoylated DLAT, triggering abnormal oligomerization of DLAT and the formation of DLAT foci. This process contributes to cellular protein toxicity stress, further exacerbating cell death. Figure created using Biorender.
Targeting various RCD pathways to treat cancer has been under intensive investigation for several decades (Peng et al., 2022). Research in the last decade has revealed novel RCD pathways and with these discoveries, progress has been made in clinical application to target these newly identified pathways for cancer treatment (Man et al., 2017; Seehawer et al., 2018; Zhou et al., 2021; Wang Y. et al., 2022; Zhang C. et al., 2022). Furthermore, therapeutic approaches that target these RCD pathways have been used in combination with immunotherapeutic agents to further enhance their efficacies (Tong et al., 2022). Such combined approaches have the potential to significantly improve patient outcomes. Despite notable advancements, major challenges such as treatment resistance exist. This review summarizes recent advancement in preclinical and clinical development to target RCD pathways in cancer from a therapeutic standpoint, exploring how alterations in these mechanisms contribute to cancer development and impact the efficacy of current treatment methods.
1.1 Dysregulated apoptosis in cancer and targeting strategies for therapy
Apoptosis is a vital intracellular mechanism that maintains tissue homeostasis in an organism by regulating cell populations (Elmore, 2007; Akhtar and Bokhari, 2024) (Figure 1). However, in cancer, cells lose their capacity to undergo apoptosis-induced death, which results in unchecked cell proliferation (Morana et al., 2022). Therefore, targeting the regulation of the apoptosis signaling pathway can be one of the crucial methods to improve cancer treatment (Pfeffer and Singh, 2018). Apoptosis is characterized by cell shrinkage, chromatin condensation, membrane blebbing, DNA breakage, and apoptotic body formation (Elmore, 2007). It involves two primary pathways: the extrinsic pathway, triggered by death receptors, and the intrinsic pathway, regulated by mitochondria (Zhang et al., 2005; Jan and Chaudhry, 2019) (Figure 2). The extrinsic pathways are controlled by transmembrane death receptors belonging to the CD95 (Apo-1 or Fas)/TRAIL/tumor-necrosis factor (TNF) receptor 1 family. When death ligands such as TNFα (tumor necrosis factor-alpha), Fas ligand (FasL), or TRAIL bind to their corresponding cell surface receptors—TNFR1, Fas, and death receptors 4 and 5 (DR4/5)—it triggers a signaling cascade. This ligand-receptor interaction leads to the recruitment and activation of caspase-8, an initiator caspase, which in turn activates downstream effector caspases (Annibaldi and Walczak, 2020). The mitochondrion is involved in the other primary route that is responsible for death signaling. It performs the function of an integrating sensor of numerous death insults by releasing cytochrome c into the cytosol, where it then activates caspase. It is believed that the mitochondrial route is the primary target of survival signaling pathways (Elmore, 2007). The Bcl-2 family controls the mitochondrial (intrinsic) pathway, which is triggered by damage of the mitochondria and the subsequent release of cytochrome c. This route is initiated by cytotoxic agents and UV radiation. Cytochrome c, Apaf-1, d-ATP/ATP, and procaspase-9 interact to form an apoptosome, which then triggers the caspase cascade (Wang and Youle, 2009). Additionally, a third pathway related to endoplasmic reticulum (ER) stress has also been described (Iurlaro and Munoz-Pinedo, 2016). Stress causes mutant proteins to accumulate in the endoplasmic reticulum, disrupting the balance between protein folding and protein requirement. This event triggers the unfolded protein response (UPR), which identifies and modulates ER stress (Schroder and Kaufman, 2005; Gardner et al., 2013). Key sensors in the UPR—ATF6 (activating transcription factor 6), IRE1α (inositol-requiring enzyme 1 alpha), and PERK (protein kinase R-like ER kinase)—are activated when misfolded protein concentrations exceed a certain threshold. If the stress is too severe or prolonged, the UPR can shift from a protective role to triggering apoptosis, in order to eliminate the affected cell and prevent damage (Spencer and Finnie, 2020). Despite having distinct mechanisms of initiation, these intrinsic, extrinsic and stress-induced pathways all lead to activation of a series of proteolytic enzymes that are members of the caspase family (Elmore, 2007; McIlwain et al., 2015) (Figure 2). The caspases, which are cascades of cysteine aspartyl proteases are produced as dormant zymogens, which are then activated by proteolytic cleavage. This is normally accomplished by the action of upstream apical caspases (McIlwain et al., 2015). Apart from these intrinsic, extrinsic and stress-induced processes, there exists an additional pathway that entails T cell mediated cytotoxicity and perforin/granzyme-dependent cell death. The cell death inducing enzymes in this pathway are granzyme B and granzyme A proteases (Trapani and Smyth, 2002).
Cancer cells often overexpress proteins that prevent the apoptotic cascade from being activated, including Bcl-2 and related anti-apoptotic proteins such as Bcl-xL, Mcl-1, A1/Bf1 and Bcl-w (Table 1) (Lowe and Lin, 2000). Targeting these proteins has become a strategy to inhibit cancer proliferation and promote cell death (Frenzel et al., 2009; Carneiro and El-Deiry, 2020). Developing cancer drugs targeting the apoptosis pathway represents the first phase of clinical development in the field (Jan and Chaudhry, 2019). A comprehensive list of compounds targeting apoptotic pathways and demonstrating anti-cancer properties is presented in Table 2. ABT-737 was the initial chemical inhibitor targeting Bcl-2, Bcl-xL and Bcl-w (Del Gaizo Moore et al., 2007). It binds to the hydrophobic pocket of Bcl-2 family members and has shown efficacy against lung cancer, especially when combined with chemotherapy and radiation therapy. Navitoclax (ABT-263) demonstrates anti-cancer properties, particularly when used with MEK or tyrosine kinase inhibitors against solid tumors (Tse et al., 2008; Walensky et al., 2004) (ABT-199), a potent Bcl-2 inhibitor, has shown promising outcomes for treating acute myeloid leukemia (AML), chronic lymphocytic leukemia (CLL) and non-Hodgkin lymphoma (NHL) (Souers et al., 2013). Selective Bcl-xL inhibitors include a vaccine for prostate cancer and ABBV-155, an antibody-drug conjugate being studied as monotherapy or for use in combination with taxanes for solid tumors (Walensky et al., 2004).
BH3 mimetics have been effectively created using stapled peptides that specifically bind through protein-protein interactions and have an improved ability to enter the cell (Ali et al., 2019). SAHBA (Stabilized Alpha-Helix of BCL-2 Domains) mimics the α-helical BH3 section of proapoptotic BID, efficiently enters leukemia cells, binds to Bcl-xL, and promotes apoptosis (Chang et al., 2013). Targeting Bax using small molecules like SMBA1-3, which bind directly to Bax and inhibit the phosphorylation of S184, promotes cytochrome c release and apoptosis (Li et al., 2017).
The Bcl-2 family member Mcl-1 can prevent apoptosis induced by multiple apoptotic triggers such as radiation and chemotherapy (Wertz et al., 2011; Widden and Placzek, 2021). AM-8621 attaches to the Mcl-1 binding pocket, displaces BIM and induces apoptosis in a myeloma cell line (Wei et al., 2020). Derivatives AMG 176 and AZD5991 have shown notable outcomes in combination with venetoclax and chemotherapy (Caenepeel et al., 2018; Tron et al., 2018). Mcl-1 inhibitors VU661013 and S63845 show promise in treating blood cancers and overcoming resistance to venetoclax when used in combination with other therapies (Carneiro and El-Deiry, 2020; Satta and Grant, 2020). In addition, IAP inhibitors have been used to target apoptosis in cancer (Fulda and Vucic, 2012; Monian and Jiang, 2012). Antagonists like LCL161 and birinapant (TL32711) show promising anti-tumor effects, particularly in combination with chemotherapy, radiation and the immune checkpoint inhibitor (ICI) anti-PD1 pembrolizumab (Amaravadi et al., 2015; Yang et al., 2019).
Agonist antibodies were also created targeting DR4 and DR5 due to their favorable half-life and notable preclinical efficacy (Hymowitz et al., 1999; LeBlanc and Ashkenazi, 2003). The only clinically tested anti-DR4 monoclonal antibody is mapatumumab, a completely human DR4-agonistic antibody with selective and strong binding to DR4 and high cytotoxicity (Pukac et al., 2005). Mapatumumab was tested in phase I and II clinical trials for HCC, NSCLC, colorectal cancer, and refractory non-Hodgkin’s lymphoma (Greco et al., 2008; Hotte et al., 2008; Trarbach et al., 2010; Younes et al., 2010; von Pawel et al., 2014; Ciuleanu et al., 2016), but none of the assays met the initial objectives, ending clinical development. Unlike DR4, several DR5 agonist antibodies have been developed and tested in clinic including Conatumumab, Drozitumab, Lexatumumab, LBY135, Tigatuzumab, and DS-8273a (Belyanskaya et al., 2007; Herbst et al., 2010; Kang et al., 2011; Forero-Torres et al., 2013; Burvenich et al., 2016; Dominguez et al., 2017; Forero et al., 2017). Conatumumab and Drozitumab demonstrated efficacy in advanced solid tumors, while Lexatumumab was tested in prostate and bladder cancer cells (Shimada et al., 2007; Herbst et al., 2010; Rocha Lima et al., 2012). DS-8273a is the newest clinically tested anti-DR5 antibody. The initial study showed that DS-8273a might be used to eliminate myeloid-derived suppressor cells in advanced cancer patients, but no objective response was seen (Dominguez et al., 2017). It is tested in three more clinical trials to assess its safety in advanced solid tumors and lymphomas or its efficacy in combination with Nivolumab in advanced colorectal cancer and unresectable stage II and IV melanoma (Dubuisson and Micheau, 2017). In addition, chimeric mouse–human antibodies LBY135 and Tigatuzumab were developed. Solid advanced cancers tolerated LBY135 well, and Tigatuzumab was investigated for relapsed lymphoma or solid malignancies (Forero-Torres et al., 2010; Sharma et al., 2014). Conatumumab and Drozitumumab reached phase II clinical trials, but Lexatumumab, LBY-135, and Tigatuzumab did not (Dubuisson and Micheau, 2017).
The p53 protein, a critical tumor suppressor, is often altered or deactivated in various malignancies, making it an ideal target for therapeutic treatments. Several p53-targeted medicines have been developed to help restore or improve p53 function. MDM2 inhibitors, including Nutlin-3, APG-115, RG7388, DS-3032, and MK-8242, suppress the p53-MDM2 interaction, stabilizing p53 and inducing apoptosis in malignancies such as gastric cancer and leukemia (Ding et al., 2013; Levine, 2022). Other MDM2 antagonists include AMG-232, HDM201, BI 907828, and ALRN-6924 (Carneiro and El-Deiry, 2020; Peng et al., 2022). MDMX inhibitors, such as XI-011 and DIMP53-1, restore p53 stability by inducing apoptosis and reducing migration in cervical and colon malignancies (Soares et al., 2017; Zhang J. et al., 2022). Small compounds such as PRIMA-1 (APR-017), APR-246 (Eprenetapopt), and COTI-2 restore mutant p53 to a functional state, reactivating its tumor-suppressive capabilities, with potential therapeutic uses in a variety of malignancies (Berke et al., 2022). The p53 agonist HO-3867 restores transcriptional repression in mutant p53, especially in ovarian cancer, resulting in cell death (Devor et al., 2021).
Cyclophilin A (CypA) inhibitors, such as HL001, impede MDM2-mediated p53 degradation, resulting in cell cycle arrest and death in NSCLC (Lu et al., 2017). Natural compounds such as Renieramycin T (RT) (Petsri et al., 2019) and Protopine (Son et al., 2019) stabilize p53, inducing apoptosis in lung and colon tumors, respectively, whereas Andrographolide (ANDRO) degrades mutant p53 (Sato et al., 2018). Actinomycin V and TCCP also increase p53 expression, which causes apoptosis in many cancer cells (Lin S. Q. et al., 2019; Rashmi et al., 2019). Heat-shock protein inhibitors, such as Mortaparib (Plus), reactivate p53 by disrupting its association with mortalin, causing apoptosis in colorectal and breast malignancies (Sari et al., 2021). Furtherrmore, Protoporphyrin IX (PpIX) targets both p53 and its homolog p73, which promotes apoptosis in CLL (Son et al., 2019).
Novel therapeutics include gold complexes like MC3, which upregulate p53 via the ROS formation and have shown effectiveness in colorectal cancer (Dabiri et al., 2019), as well as platinum-based compounds like bromocoumarinplatin 1 and diplatin, which activate p53 to overcome cisplatin or carboplatin resistance respectively in lung cancer (Lin X. et al., 2019; Ma et al., 2020). Other small compounds, such as DJ34, kill leukemia stem cells by inhibiting c-Myc and activating p53 (Tadele et al., 2021), whereas AQ-101 inhibits MDM2 to activate p53 and increase apoptosis in leukemia (Gu et al., 2018).
Research is exploring inhibitors of uncontrolled oncogenic effectors such as PI3K, AKT, β-catenin, Myc, CDKs, mTOR, and VEGF (Sever and Brugge, 2015). CDK4/6 inhibitors like palbociclib enhance cell death and induce cell cycle arrest in various cancers (Tao et al., 2016). Epigenetic strategies focused on inducing apoptosis in cancer cells involve histone deacetylase (HDAC) inhibitors and Bromodomain and Extra-Terminal motif (BET) inhibitors (Bolden et al., 2006; Kim et al., 2018). HDAC inhibitors, such as panobinostat, enhance Noxa expression, reduce Mcl-1 levels, and increase sensitivity to Bcl-2 inhibitors (Liu et al., 2018). They also enhance the effectiveness of MEK inhibitors and venetoclax in treating multiple myeloma (DiNardo et al., 2019). BET inhibitors like ABBV-075, when combined with venetoclax, demonstrate promising outcomes in patients with cutaneous T cell lymphoma (CTCL) (Kim et al., 2018). The hypomethylating agent azacytidine, when combined with venetoclax and ABT-737, has shown promising results (Mishra et al., 2023).
1.2 Targeting autophagy for cancer therapy
Autophagy, a critical mechanism for maintaining cellular balance by removing damaged organelles and protein aggregates, can also facilitate cell death (Liu et al., 2023). Cells can undergo autophagy-related cell death in two primary ways: autophagy-dependent cell death (ADCD) which transpires independently of other programmed death mechanisms and autophagy-mediated cell death (AMCD) that occurs when autophagy-related molecules directly engage with those implicated in forms of cell death. (Zhou et al., 2022) (Figure 1). Moreover, autophagy is linked to other cell death mechanisms, such as apoptosis, necrosis, and ferroptosis, through a variety of processes (Dunkle and He, 2011; Gordy and He, 2012; Chen et al., 2018; Liu J. et al., 2020; Peng et al., 2022) (Figure 2).
Three distinct forms of autophagy have been identified: macroautophagy, microautophagy, and chaperone-mediated autophagy (CMA) (Parzych and Klionsky, 2014). Microautophagy is a form of autophagy in which lytic organelles autonomously engulf and degrade cytoplasmic components. It is essential for regulating biosynthesis, transport, metabolic adaptability, organelle remodeling, and the maintenance of cellular component quality (Saha et al., 2018). Macroautophagic autophagosomes convey cellular constituents for destruction to endosomes or lysosomes (Feng et al., 2014). Autophagy starts with an isolating membrane known as the phagophore, which encases a portion of the cytoplasm. The Atg9 protein promotes growth by supplying crucial lipid constituents. The Atg1 and Atg9 proteins, together with a phosphatidylinositol 3-kinase complex, govern this activity. In the subsequent phase, two conjugation steps transpire. The first activation entails Atg12 and the Atg7 protein. The Atg12 protein is conveyed to the Atg10 protein, leading to a covalent connection with Atg5. The Atg12-Atg5 complexes subsequently associate with the Atg16L protein. The ATG12-ATG5-ATG16L1 complex is essential for the production of autophagosomes. The second step of conjugation involves the proteins Atg3, Atg4, Atg7, and LC3. The Atg4 protease cleaves proLC3, resulting in the formation of LC3-I. Subsequently, the Atg7, Atg3, and Atg12-Atg5-Atg16L proteins are conjugated. The LC3-I protein interacts with the lipophilic phosphatidyle-thanolamine (PE) to generate the LC3-II form. These stages generate the autophagosome, which encapsulates a segment of the cytoplasm and proteins. The outer membrane of the autophagosome fuses with the lysosome to form an autophagolysosome. Lysosomal enzymes facilitate the digestion of the autophagolysosome’s inner membrane and its contents (Gomez-Virgilio et al., 2022; Liu et al., 2023). Chaperone-mediated autophagy (CMA) removes damaged proteins during fasting or oxidative stress. The chaperone complex links the protein’s target motif to facilitate lysosome trafficking. In the lysosome, the complex interacts with LAMP-2A’s cytoplasmic tail and is destroyed (Bejarano and Cuervo, 2010).
Autophagy plays a complex role in cancer, acting as both an inhibitor and promoter of tumor growth (Chavez-Dominguez et al., 2020; Nawrocki et al., 2020; Debnath et al., 2023). It can help cancer cells avoid damage induced by chemotherapeutics and promote chemoresistance (Table 1) (Nawrocki et al., 2020; Debnath et al., 2023). Preclinical research using chemotherapeutics like cyclophosphamide, imatinib, and vorinostat has shown that autophagy reduces the effectiveness of these drugs and contributes to acquired resistance (Mele et al., 2020). Furthermore, autophagy aids cancer cells in adapting to chemotherapy (Ahmadi-Dehlaghi et al., 2023).
Autophagy inhibitors have shown promise in combination with chemotherapeutic and targeted immunotherapeutic drugs (Table 3). While many prospective autophagy inhibitors are being developed, chloroquine (CQ) and its derivative hydroxychloroquine (HCQ) are the only approved drugs (Wang et al., 2011). HCQ, like CQ, suppresses autophagy by blocking lysosomal acidification and autophagosome degradation but has lower toxicity (Sui et al., 2013; Cook et al., 2014; Pellegrini et al., 2014; Lee et al., 2015). A Phase II trial for muscle-invasive bladder cancer is investigating the combination of HCQ with gemcitabine and cisplatin for systemic chemotherapy (Ojha et al., 2016). Similarly, in breast cancer, HCQ combined with tamoxifen was found more effective in suppressing autophagy in estrogen-positive (ER+) cell lines (Cook et al., 2014). In renal cell carcinoma, HCQ combined with temsirolimus led to increased apoptosis by inhibiting autophagy (Lee et al., 2015). Early phase I/II trials of HCQ have focused on adult solid tumors, including pancreatic adenocarcinoma, melanoma, colorectal carcinoma, myeloma, lymphoma and renal cell carcinoma, using chemotherapy drugs such as temsirolimus, bortezomib, temozolomide, vorinostat, and doxorubicin (Llovet et al., 2008; Mahalingam et al., 2014; Rangwala et al., 2014a; Rangwala et al., 2014b; Rosenfeld et al., 2014; Vogl et al., 2014; Wolpin et al., 2014). HCQ doses ranged from 400 mg to 600 mg twice daily, showing tolerability with partial responses and stable disease in some patients (Carew and Nawrocki, 2017). For advanced solid tumors and melanoma, HCQ combined with 150 mg/m2 of temozolomide showed 27% stable disease and 14% partial response in wildtype melanoma (Cheng et al., 2009; Rangwala et al., 2014b). The combination of HCQ and rapamycin, an inhibitor of mTORC1 activity, was well-tolerated in advanced solid tumor (Rangwala et al., 2014a). In myeloma, HCQ combined with bortezomib improved the efficiency of proteasome inhibitors by causing the accumulation of misfolded proteins, with 45% of patients showing stable disease. The most common adverse effects were gastrointestinal issues and cytopenias (Vogl et al., 2014).
Autophagy activation by drugs like sorafenib, a multi-tyrosine kinase mTOR inhibitor used for hepatocellular cancer, is being explored as a potential cause of drug resistance (Llovet et al., 2008). Clinical trials have investigated HCQ in hepatocellular cancer (Cheng et al., 2009) and targeted immunotherapeutic treatments, such as checkpoint inhibitors, have limited efficacy and high costs. Combining autophagy modulators like HCQ with immune checkpoint inhibitors (ICI) has the potential to improve efficacy and reduce treatment costs. Several inhibitors targeting different stages of autophagy are under investigation including inhibitors of upstream signaling molecules: SBI-0206965 (ULK1 inhibitor) (Tang et al., 2017), Spautin-1 (Beclin1 inhibitor), SAR405 (Vps18 kinase inhibitor), and gambogic acid (induces caspase-mediated cleavage of autophagy proteins) (Ishaq et al., 2014; Pasquier, 2015). Autophagy initiation inhibitors include ATG4 inhibitors NSC185058 and NSC377071, and Verteporfin (inhibits early-stage autophagosome formation) (Donohue et al., 2011; Akin et al., 2014). Lysosomal inhibitors include ROC325 (Nawrocki et al., 2019), Lys05 (Amaravadi and Winkler, 2012), DQ661 (Rebecca et al., 2017), and DC661 (Rebecca et al., 2019).
Preclinical trials have also explored autophagy’s potential to enhance radiation therapy (Kim et al., 2008; Kuwahara et al., 2011). For example, in a lung cancer mouse model, combining Z-DEVD (caspase-3 inhibitor), RAD001 (mTOR inhibitor), and irradiation induced the highest levels of autophagy and associated radiation damage. This suggests that inhibiting both apoptosis and mTOR during radiotherapy could improve outcomes in non-small cell lung cancer patients (Kim et al., 2008). Similarly, in glioma cells, autophagy induction by silver nanoparticles (AgNPs) and/or radiation was confirmed by applying 3-methyladenine (3-MA), highlighting selective autophagy as a promising therapeutic avenue for effective cancer treatment (Wu et al., 2015).
1.3 Necroptosis in cancer development and treatment
Programmed inflammatory cell death, known as necroptosis, was first identified as an alternative to apoptosis following the activation of death domain receptors (Degterev et al., 2005; Dhuriya and Sharma, 2018) (Figure 1). Necroptosis is a regulated type of necrosis that is dependent on receptor interacting kinase-1 (RIPK1) and RIPK3 phosphorylating mixed-lineage kinase-like (MLKL) (Vandenabeele et al., 2010; Sun et al., 2012; Newton et al., 2014) (Figure 2). The necroptotic process begins when RNA- and DNA-sensing molecules and cell surface death receptors including FasRs, TNFR1, IFN receptors, and TLRs are activated (Kaiser et al., 2013). There are two ways that cell death signaling continues (Pasparakis and Vandenabeele, 2015). Complex I, a survival complex that communicates via NF-kB, can be created by TNF-α. RIPK1 deubiquitination transforms the complex into apoptotic complex IIa. When caspase-8 is absent and RIPK3 is elevated, the complex forms IIb (the necrosome). The death domain-related proteins RPK1, RPK3, and Fas on this necrosome directly phosphorylate the kinase domain-like protein (MLKL) to induce necroptosis. MLKL phosphorylation forms an oligomer that punctures the plasma membrane, killing the cell. Calmodulin-dependent protein kinase and mitochondrial serine/threonine protein phosphatase II are other RIPK3 downstream effects (He et al., 2009; Cai et al., 2014; Wang et al., 2014; Murphy, 2020). Necroptotic cell death is characterized by cell membrane perforation, elevated intracellular osmotic pressure, cell rounding and swelling, organelle swelling, impaired mitochondrial activity, mitochondrial membrane potential loss, nuclear chromatin loss, and plasma membrane rupture (Dhuriya and Sharma, 2018). Plasma membrane rupture causes potassium efflux, cytokines, and chemokines, which cause inflammation and immunological responses (Dhuriya and Sharma, 2018).
Necroptosis is involved in various aspects of tumor biology, including tumor development, necrosis, metastasis and the immune response within tumors (Najafov et al., 2017; Gong et al., 2019; Yan et al., 2022; Meier et al., 2024). This cell death pathway exhibits both pro- and anti-tumorigenic effects (Ye et al., 2023). Major regulators of necroptosis are often downregulated in cancer cells, correlating with unfavorable outcomes (Table1) (Yan et al., 2022). Necroptosis has emerged as a novel target for anticancer therapy due to its significant role in tumor biology (Gong et al., 2019).
Several natural compounds and small molecule inhibitors are known to induce necroptosis in cancer cells (listed in Table 4) (Wu et al., 2020). Chloroquine increases the expression of endogenous RIPK3 in colorectal cancer cell lines, with necroptosis being the mechanism (Meng et al., 2016). Shikonin, derived from a Chinese medicinal herb, induces necroptosis in nasopharyngeal carcinoma cells by enhancing reactive oxygen species (ROS) production and increasing RIPK1, RIPK3 and MLKL expression (Liu et al., 2019). Emodin triggers necroptosis in glioma cell lines by activating the TNF/RIPK1/RIPK3 pathway (Zhou et al., 2020). Neoalbaconol (NA), a compound derived from the fungus Albatrellus confluens, has been found to trigger necroptosis by facilitating the autocrine release of TNFα through the modulation of the RIPK/NF-κB signaling pathway and RIPK3-dependent reactive oxygen species (ROS) generation (Yu et al., 2015). The steroid glycoside Ophiopogonin D induces necroptosis in prostate cancer cells by activating RIPK1 (Lu et al., 2020). Resibufogenin inhibits colorectal cancer cell line growth by increasing RIPK3 expression (Han et al., 2018). The initiation of necroptosis can also be influenced by adjusting upstream signaling pathways, such as using the sphingosine analog FTY720 (fingolimod), which triggers necroptosis in human lung cancer cells by interacting with the I2PP2A/SET oncoprotein and activating the PP2A/RIPK1 pathway (Saddoughi et al., 2013).
Nanoparticles to induce necroptosis in cancer cells is another emerging field (Mohammadinejad et al., 2019). Although the antifungal agent Shikonin shows potential, its clinical use is limited due to poor tumor specificity, low water solubility, short bloodstream half-life, and high risk of side effects on healthy tissues (Boulos et al., 2019). To address these issues, Feng et al. developed an Fe(III)-shikonin supramolecular nanomedicine (FSSN) using metal-polyphenol coordination of Fe(III) and shikonin, demonstrating improved water solubility and reduced cytotoxicity in normal cells and induced both ferroptosis and necroptosis (Feng et al., 2022). In CT26 colon cancer cells, graphene oxide nanoparticles triggered necroptosis by enhancing RIPK1, RIPK3, and HMGB1 activity (Chen et al., 2015). Similarly, selenium nanoparticles induced necroptosis in prostate adenocarcinoma cells by increasing ROS production and TNF and interferon regulatory factor 1 expression (Sonkusre, 2019). Folate-sodium alginate-cholesterol nanoparticles delivering doxorubicin and metformin achieved targeted accumulation and induced various forms of programmed cell death, including necroptosis, apoptosis, and pyroptosis in xenograft melanoma tumors (Song et al., 2021). Myricetin-loaded solid lipid nanoparticles (MYC-SLNs) enhanced necroptosis in A549 cells by increasing RIPK3 and MLKL expression without affecting apoptosis and without apparent effects on the growth and health of MRC5 cells (Alidadi et al., 2022). Ma et al. developed star-PCL-azo-PEG micelles (sPCPEG-azo) to deliver dimethyl fumarate (DMF) specifically to the colon, inducing necroptosis by eliminating GSH, increasing ROS levels and activating MAPKs (Ma Z. G. et al., 2016).
In a study conducted by Liu et al., MLKL inhibitor necrosulfonamide (NSA) was shown to significantly delay tumor growth, thus offering compelling evidence of the role necroptosis plays in promoting tumor development (Liu et al., 2016). In mice, the use of necrostatin-1, another necroptosis inhibitor, has been found to be effective in reducing colitis-associated tumorigenesis (Liu et al., 2015). There is ongoing testing of the RIPK1 inhibitor, GSK2982772, in phase 2a clinical studies for individuals with inflammatory disease. Furthermore, in a clinical trial (NCT04739618), researchers explored the potential benefits of nonablative cryosurgical freezing-induced necroptosis followed by immunotherapeutic drug injection in metastatic solid tumors. The immunotherapy included pembrolizumab (anti-PD1), ipilimumab (anti-CTLA-4), and GM-CSF. The aim was to assess the overall response rate of radiographic changes (Tong et al., 2022). In addition, induction of apoptosis has also been shown to reverse drug resistance. Xu Zhao et al., effectively employed trichothecin to trigger necroptosis in cancers that are resistant to chemotherapy. Mechanistically, the natural secondary metabolite trichothecin significantly increased the expression of RIPK3. Subsequently, RIPK3 enhanced the phosphorylation of MLKL and activated mitochondrial energy metabolism and ROS production. This novel approach sensitizes cancer cells to cisplatin therapy (Zhao X. et al., 2021).
In addition, it has been shown that necroptosis-inducing drugs could impact the effectiveness of ICIs in individuals with cancer (Tang et al., 2020). Using a viral vaccination strategy, Hoecke et al. were able to effectively deliver the necroptosis mediator MLKL to tumor cells, resulting in the promotion of necroptotic death and the enhancement of antitumor immunity. Increased immunity directly against neo-epitopes was responsible for the potent antitumor immunity (Van Hoecke et al., 2020). In addition, the RNA editing enzyme ADAR1 has been widely recognized for its role in suppressing Z-type dsRNA, a substrate for ZBP1. This suppression mechanism leads to resistance and limited responsiveness to ICIs (Zhang T. et al., 2022). However, the small-molecule drug CBL0137 has the ability to directly induce the formation of Z-type dsDNA in cells. This in turn activates ZBP1-dependent necroptosis and effectively reverses the insensitivity to ICIs in mouse melanoma models (Zhang T. et al., 2022). In addition, cIAPs have the ability to hinder the RIPK1-dependent necroptosis process. However, this inhibition can be counteracted by Smac mimetics which then trigger the activation of the necroptotic death pathway in Burkitt’s lymphoma cell lines (Koch et al., 2021). In melanoma, the response to ICIs can be enhanced by using Smac mimetics which have a direct impact on immune cells such as B cells, MDSCs, DCs, and cytotoxic T cells (Michie et al., 2020). Based on the evidence, it appears that necroptosis could potentially be employed to enhance the readiness of the tumor microenvironment for immunotherapy.
Even with progress in necroptosis research, various obstacles impede its application in cancer treatment. The practicality of necroptosis, having potential as an alternative therapy for tumors resistant to apoptosis, continues to be debated. Hitomi et al. (2008) identified a cellular signaling network that regulated necroptosis and implicated two suppressor genes, CYLD and EDD1, and four Ras-related proteins, suggesting a role in tumorigenesis. CYLD gene mutations in tumorigenic epidermal cells promote carcinoma aggressiveness by increasing angiogenic factor production, which is crucial to epidermal cancer malignancy (Alameda et al., 2010). RIPK3 and CYLD were downregulated in CLL cells, and LEF1 represses CYLD. Together, necroptosis may be crucial to carcinogenesis (Reed, 2006). Tumor heterogeneity presents a significant challenge, as numerous cancers are deficient or have mutated for essential necroptosis regulators such as RIPK3 or MLKL, which restricts the effectiveness of necroptosis inducers. RIPK3-r, a truncated splice variation of RIPK3, was dramatically elevated in colon and lung tumors compared to matched normal tissues, suggesting that it may be a primary splice form involved in carcinogenesis, according to Yang et al. (2005). The RIPK3 gene lies on chromosome 14q11.2, which is mutated in several malignancies, including nasopharyngeal carcinoma and T cell leukemia/lymphoma (Kasof et al., 2000). In non-Hodgkin lymphoma, RIPK3 gene polymorphisms increases tumor risk (Wu et al., 2012). Furthermore, existing inducers exhibit a lack of selectivity, resulting in uncontrolled inflammation and the possibility of harming healthy tissues, which raises concerns regarding off-target effects and systemic toxicity. Necroptosis may enhance anti-tumor immunity due to its pro-inflammatory characteristics, yet it also has the potential to facilitate tumor progression by creating a pro-tumor environment. Necroptosis of tumor cells can affect the TME in a way that can contribute to tumor growth because the inflammation associated with necroptosis can stimulate cell division, genetic instability, angiogenesis and metastasis (Negroni et al., 2020). Furthermore, the restricted clinical evidence, primarily based on preclinical models, along with the unpredictable nature of necroptosis outcomes, adds to the complexity of its application. Tumors can develop resistance to necroptosis, similar to how they respond to therapies that induce apoptosis. Consequently, additional research is essential to enhance targeting specificity, reduce inflammatory risks, and confirm the efficacy of necroptosis-based treatments in clinical environments.
1.4 The roles of pyroptosis in cancer cell survival and treatment strategies
Pyroptosis is a form of RCD associated with inflammatory responses. It is triggered by human caspase-1, -3, -4, -5 (mouse caspase-11), −6, −8, and −9 and NLRP3 and has significant therapeutic implications for several malignancies due to its profound effects on the invasion, proliferation, and metastasis of tumor cells (Table 1) (Shi J. et al., 2014; Fang et al., 2020; Zheng and Li, 2020; Rao et al., 2022; Wei et al., 2022) (Figure 1). Gasdermin (GSDM) superfamily members, GSDMA-GSDME, which are essential to pyroptosis, are triggered by caspases and perforate the plasma membrane (Kayagaki et al., 2015; Shi et al., 2015; Rogers et al., 2019) (Figure 2). The characteristic features of pyroptosis in cancer involve gasdermins family protein cleavage and polymerization, both N-terminal and C-terminal junction domain cleavage, and activated N-terminal regions. The N-terminal generates a cell membrane pore by binding to membrane lipids, phosphatidylinositol, and cardiolipin, causing cell osmotic swelling, plasma membrane rupture, and death (Ding et al., 2016; Feng et al., 2018). Gasdermins create 10–20 nm holes in cell membranes, releasing cell contents slowly and potentially causing inflammatory responses. The cells become flattened eventually and create 1–5 μm apoptotic body-like protrusions. Nuclear concentration and chromatin DNA breaking occur as cells enlarge to rupture plasma membrane (Zhang et al., 2018). Pyroptotic pathway can occur either through classical or non-classical pathway in cancer. The classical pathway is activated by pathogen-associated molecular patterns (PAMPs) or damage-associated molecular patterns (DAMPs) (Chen and Nunez, 2010; Franchi et al., 2012). Cytoplasmic pattern recognition receptors (PRRs) identify them. Based on particular inputs, nod-like receptors (NLRs) or melanoma deficiency factor 2-like receptors (ALRs) produce inflammatory bodies and activate caspase-1. After Caspase-1 cleaves GSDMD, its N-terminal aggregates into cell membrane holes (Amarante-Mendes et al., 2018; Zheng D. et al., 2020) (Figure 2). Additionally, caspase-1 cleaves pro-IL-1β and pro-IL-18 into mature IL-1β and IL-18, which are then released via the membrane hole. The nonc-lassical pyrolytic pathway requires caspase-4/-5/-11 activation. After lipopolysaccharide (LPS) stimulates the cytoplasm, caspase-4/caspase-5/caspase-11 (the human equivalent of mouse caspase-11 caspase-4/caspase-5) can directly bind to the conserved structure of LPS, lipoprotein A, causing oligomerization, activation, and the N-terminal of GSDMD to be cleaved and localized to the cell membrane to form membrane pores (Kayagaki et al., 2015). Pyroptosis is quicker and more violent than apoptosis, releasing several pro-inflammatory molecules. Cell scorch is caused by inflammatory corpuscles and GSDM family proteins.
Several chemotherapeutic drugs, including cisplatin, paclitaxel, 5-FU, lobaplatin and others have been found to trigger pyroptosis in tumor cells (Table 5) (Zhang C. C. et al., 2019; Jia et al., 2023). Chemotherapy-induced pyroptosis is frequently the result of GSDME pathway activation. Chemotherapy drug Lobaplatin triggers pyroptosis in cervical cancer and colorectal cancer by activating GSDME (Chen et al., 2022). This effect is achieved by activating caspase-3/9 through the ROS/JNK/BAX mitochondrial apoptosis pathway (Yu et al., 2019). 5-FU triggers pyroptosis in gastric cancer cells via GSDME instead of GSDMD (Wang Y. et al., 2018). When exposed to cisplatin or 5-FU, GSDME+/+ mice experience significant intestinal damage and infiltration of immune cells. On the other hand, GSDME−/− mice show less injury, indicating that triggering pyroptosis in cancer cells might offer a potential alternative approach for cancer treatment (Yu and He, 2017).
Clinical trials have demonstrated that the combination of PD-L1 inhibitors with chemotherapy or radiation can effectively eliminate tumor cells through pyroptosis induction (Reck et al., 2019). This approach has shown promising results in terms of improved patient survival rates, surpassing those observed in patients solely treated with PD-L1 inhibitors. In breast cancer cells, the presence of Trimethylamine N-oxide (TMAO) can trigger GSDME-mediated pyroptosis (Wang H. et al., 2022), and when TMAO is combined with PD-1, it has the potential to enhance the antitumor effects of anti-PD-1 (Jia et al., 2023).
CAR-T cells have been successfully utilized to effectively treat hematological malignancies, yielding favorable outcomes (Gill and Brudno, 2021). However, cytokine release syndrome (CRS) is a significant side effect of this technology. When CAR-T cells release granzyme B, it can trigger pyroptosis by activating the caspase-3/GSDME pathway (Liu Y. et al., 2020). Interestingly, the elimination of GSDME through knockout has been found to effectively prevent CRS. Furthermore, the presence of perforin/granzyme B in CAR-T cells, as opposed to in CD8+ T cells, triggers GSDME-mediated pyroptosis in target cells (Liu Y. et al., 2020). These findings underscore the clinical importance of pyroptosis in immunotherapy. The release of IL-1β and IL-18 by pyroptotic cells, along with other DAMPs, can attract immune cells like dendritic cells (DCs) and macrophages (MFs) to engulf the pyroptotic cells (Wang Q. et al., 2018; Karki and Kanneganti, 2021). Mature DCs display tumor-specific antigens to activate cytotoxic T lymphocytes, which then eliminate tumors (Wang et al., 2013).
Targeted drugs have also been discovered that selectively trigger pyroptosis in tumor cells (Table 5). Val-boroPro triggers pyroptosis in primary acute myeloid leukemia (AML) cells by activating the inflammasome sensor protein CARD8 which then activates procaspase-1 (Johnson et al., 2018). In a melanoma study, it was found that the combination of BRAFi and MEKi could potentially have an antitumor effect by inducing pyroptosis through GSDME (Erkes et al., 2020). Additionally, the combination of DDP and BI2536 (a PLK1 kinase inhibitor) was observed to induce pyroptosis in esophageal cancer cells (Wu M. et al., 2019). In a study conducted by Dobrin et al., triple-negative breast cancer cells were exposed to ivermectin, resulting in the activation of the pannexin-1 pathway. This activation led to the overexpression of P2X4/P2X7 receptors, the release of ATP and ultimately the induction of pyroptosis (Draganov et al., 2015). Several drugs, including metformin, anthocyanin, and DHA have been found to trigger GSDMD mediated pyroptosis in different types of cancers (Pizato et al., 2018; Wang L. et al., 2019; Yue et al., 2019).
1.5 Clinical development targeting ferroptosis for cancer treatment
Ferroptosis is a recently discovered RCD pathway distinguished by oxidative and non-apoptotic mechanisms. It is characterized by iron-dependent lipid peroxide damage in mitochondria and a lack of glutathione peroxidase 4 (GPX4) and is distinct from apoptosis, autophagy, and necrosis (Dixon et al., 2012) (Figures 1, 2). From a morphological perspective, the cell membrane stays intact while developing blisters. The mitochondria decrease in size, and their membrane density increases. The mitochondrial cristae may either reduce in number or vanish entirely. The nucleus retains its typical size, while the chromatin remains uncondensed. Ferroptosis takes place when there is a disruption in the regulatory system, resulting in the buildup of lipid peroxide to a critical level. Transferrin (TF) attaches to extracellular Fe3+ and aids in its transport into cells through transferrin receptor 1 (TfR1), where it is subsequently transformed into Fe2+ (Masaldan et al., 2018). Subsequently, intracellular divalent metal transporter 1 (DMT1) and zinc transporter 8/14 (ZIP8/14) facilitate the storage of Fe2+ in the intracellular labile iron pool (LIP) (Sterling et al., 2017). Fe2+ can transfer electrons via the Fenton reaction with peroxide, leading to the generation of oxidizing free radicals. After an excessive buildup of iron within cells, many free radicals engage with polyunsaturated fatty acids (PUFA) present in the phospholipids of cell membranes, leading to the creation of lipid peroxides, which ultimately result in cell death (Doll et al., 2017). The intracellular antioxidant stress system relies on GPX4 to remove excess lipid peroxides. The Cystine/glutamate antiport (system xc−) enables the transfer of glutamate from inside cells to the exterior, while concurrently bringing cystine from outside into the cells. Inhibiting cysteine with system xc− blockers like erastin reduces the necessary cysteine levels for GSH production and disrupts GSH synthesis. GPX4 facilitates the hydrolysis of lipid peroxide through the action of GSH. Enhancing ferroptosis requires the inhibition of system Xc−, depletion of GSH, and deactivation of GPX4 (Yang et al., 2014).
Cancer cells’ higher iron (Fe) accumulation makes them more susceptible to ferroptotic cell death, thereby impacting tumor development, proliferation and metastasis (Table 1) (Maru et al., 2022; Lei et al., 2024; Zhou Q. et al., 2024). Several ferroptosis inducers have been developed, and their effectiveness varies in different cancer types (listed in Table 6) (Luo et al., 2024; Zhou Q. et al., 2024). Sorafenib, an FDA-approved chemotherapeutic for hepatocellular carcinoma (HCC), renal cell carcinoma (RCC), and thyroid cancer stimulates ferroptosis by inhibiting system XC− and glutathione (GSH) formation (Dixon et al., 2014; Sun et al., 2017). Combining sorafenib with sulfasalazine can further inhibit sulfur-based amino acid metabolism, triggering ferroptosis in HCC cells both in vitro and in vivo (Wang K. et al., 2021). In NSCLC and colon cancer, cisplatin induces ferroptosis by depleting GSH and inactivating GPX4 (Guo et al., 2018). Etoposide, a phenolic anticancer drug, depletes GSH in myeloperoxidase-rich myelogenous leukemia cells, reducing GPX4 and triggering ferroptosis (Kagan et al., 2017). Further, Ma et al. demonstrated that the combination of the lysosome disruptor siramesine with the tyrosine kinase inhibitor lapatinib resulted in the ferroptotic death of breast cancer cells. This was achieved by blocking iron transport and inducing lipid peroxidation (Ma S. et al., 2016). The combination of DHA with cisplatin triggered cell death in pancreatic ductal adenocarcinoma (PDAC) by promoting the degradation of GPX4, creation of ROS and the degradation of ferritin, leading to induction of ferroptosis (Du J. et al., 2021).
Nanotechnology enhances RCD induction by delivering inducers directly to tumors (Mohammadinejad et al., 2019). FePt@MoS2 nanoparticles, for example, release Fe(II) in the tumor microenvironment, accelerating the Fenton reaction and triggering ferroptosis in various cancer cell lines (Zhang D. et al., 2019). Similarly, additional research showed that zero-valent iron nanoparticles transformed Fe(II) to enhance the Fenton reaction, resulting in mitochondrial lipid peroxidation in oral cancer cells (Huang K. J. et al., 2019). In addition, FSSN based on the metal-polyphenol coordination of Fe(III) and shikonin, led to necroptosis and a reduced GSH level induced ferroptosis in mouse breast cancer cell lines (Feng et al., 2022).
Radiation therapy induces ferroptotic cell death by generating reactive oxygen species (ROS), leading to lipid peroxidation (Lang et al., 2019; Lei et al., 2020; Ye et al., 2020). ROS extract electrons from polyunsaturated fatty acids (PUFAs), forming lipid peroxyl radicals and hydroperoxides. Radiation also upregulates ACSL4 to facilitate PUFA-phospholipid production and also reduces GSH levels, impairing GPX4 and promoting ferroptosis (Lei et al., 2020; Ye et al., 2020; Zhang C. et al., 2022). Moreover, studies have demonstrated that disulfiram induces lysosomal membrane permeabilization through a process dependent on reactive oxygen species (ROS), leading to ferroptosis induction and enhancing the vulnerability of cells to radiation (Ye L. et al., 2021).
ICIs have advanced cancer therapy, but their efficacy is limited without tumor-associated antigens (Ding et al., 2022; Kou et al., 2023). CD8+ T lymphocytes can suppress tumors by triggering necroptosis, pyroptosis, and ferroptosis (Tang et al., 2020; Chen L. et al., 2021; Liao P. et al., 2022; Wang Z. et al., 2022). Unique RCDs in the TME stimulate proinflammatory cytokines and cytotoxic T cell infiltration, enhancing ICI responsiveness (Workenhe et al., 2020). Lipid peroxides generated during ferroptosis signal DCs to present tumor antigens to CD8+ T cells, improving immunotherapy (Zhao et al., 2022). Combining ferroptosis inducers with ICIs may enhance cancer cell susceptibility to immunotherapy. Wang and colleagues have demonstrated that the concurrent administration of a GPX4 inhibitor, cyst(e)inase, and PD-L1 inhibition enhances T cell-mediated antitumor immune responses and synergistically promotes ferroptotic death of cancer cells (Wang W. et al., 2019). On the other hand, ferroptosis inhibition therapy yielded greater antitumor efficacy when used in combination with anti-PD-1 antibodies [346].
However, ferroptosis can sometimes promote tumor initiation and progression (Dang et al., 2022). Ferroptosis-induced inflammation may drive necroinflammation-associated malignancies, and immune cell susceptibility to ferroptosis can undermine tumor suppression or promote tumor development (Bell et al., 2024). Ferroptotic cancer cells may also have immunosuppressive effects that enhance tumor growth (Chen X. et al., 2021; Qi and Peng, 2023). In addition, the ferroptosis of non-tumor cells is linked to a diminished ability to combat tumors due to a decrease in the generation of cytotoxic cytokines. Utilizing the ferroptosis inhibitor ferrostatin-1 effectively prevents CD8+ T cell ferroptosis by inhibiting lipid peroxidation (Wang W. et al., 2019). As a result, the production of pro-inflammatory cytokines is enhanced, leading to the elimination of tumors. Ferroptosis inhibition yields enhanced antitumor effectiveness when combined with anti-PD-1 antibodies (Ma et al., 2021). Further, Inhibiting ferroptosis could mitigate adverse effects from therapies promoting it, suggesting its suppression might be a viable cancer treatment strategy in certain contexts.
Currently, a phase II clinical study is assessing the ferroptosis inhibitor MIT-001 for preventing oral mucositis in lymphoma or multiple myeloma patients undergoing conditioning chemotherapy with autologous hematopoietic stem cell transplantation (NCT05493800).
1.6 New roles for cuproptosis in cancer cell death and targeting strategies
In 2019, Tsvetkov et al. discovered Cu-dependent death while investigating the anticancer mechanism of the Cu ionophore elesclomol (Tsvetkov et al., 2019). It was discovered that administering elesclomol to a mouse model of multiple myeloma decreased the cancer cells’ resistance to the damage caused by proteasome inhibitors. Mechanistically, reduced Cu(I) is produced when elesclomol-bound Cu(II) interacts with the mitochondrial enzyme ferredoxin 1 (FDX1), raising ROS levels (Nagai et al., 2012; Tsvetkov et al., 2019). Lipid peroxidation was once thought to be the cause of elesclomol’s lethality (Gao et al., 2021). Later, in 2022, they reported that intracellular copper build-up causes mitochondrial lipoylated protein oligomerization and destabilizes Fe–S cluster proteins, resulting in cuproptosis, an independent mode of cell death that is different from other RCD pathways (Ge et al., 2022). Research in the domains of cancer pathology and cell physiology has long focused on the role of copper in tumor progression, with studies emphasizing the critical connection between cuproptosis and cancer. Tumor angiogenesis and metastasis are activated by copper, a proangiogenic factor (Xu et al., 2022). Dysfunctional copper metabolism is the cause of both radioresistance and chemoresistance (Liu et al., 2022; Yang et al., 2022). Increased serum copper levels have been linked in a number of studies to disease invasion and tumor stage in patients with breast, lung, and colorectal cancer (Baszuk et al., 2021; Cui et al., 2021; Tsang et al., 2022). On the other hand, cuproptosis causes endothelial cell dysfunction, oxidative stress, and mitochondrial damage in malignant cells by interfering with lipid metabolism (Halliwell and Chirico, 1993; Ruiz et al., 2021).
Further, elevated Cu has been strongly associated with the increased expression level of hypoxia-inducible factor 1α (Feng et al., 2009; Wu Z. et al., 2019), inducing angiogenesis, and neovascularization leading to increased production of vascular endothelial growth factor (Zimna and Kurpisz, 2015). Elevated expression of intracellular Cu-dependent protein MEMO1, an oncogenic protein, has been associated with migration and invasion of breast and lung cancer cells (MacDonald et al., 2014). Zhang et al. have demonstrated that MEMO1 preferentially binds to Cu(I) and not Cu(II) and thus protects cells from redox activity (Zhang et al., 2022d). Consequently, releasing Cu ions and preventing the spread of tumor cells may be achieved by devising a suitable strategy to disrupt the Cu(I) binding site on the MEMO1 protein.
Cuproptosis may prevent the spread of cancer cells and reduce their proliferation (Li J. et al., 2022; Feng et al., 2024). Cuproptotic tumors exhibit reduced angiogenesis and respond well to therapy with sunitinib and sorafenib (Li K. et al., 2022). Cancer cells have developed mechanisms to defend against Cu-induced apoptosis (Table 1). For example, individuals with hepatocellular carcinoma (HCC) had significantly reduced levels of the critical cuproptosis regulator FDX1, making HCC cells resistant to cuproptosis (Zhang Z. et al., 2022). More advanced tumor-node-metastasis stages are closely linked to reduced FDX1 expression. Additionally, shorter survival rates have been associated with decreased FDX1 expression across various cancer types (Wang T. et al., 2022).
Copper ionophores, or cuproptosis-related drugs which trigger cuproptosis, may hold promise for future tumor treatments (Table 7) (Springer et al., 2024; Wang Y. et al., 2024). Elesclomol (ES) and Disulfiram (DFS) induce apoptosis by transporting copper ions into cells and mitochondria, resulting in the oligomerization of dihydrolipoamide s-acetyltransferase, decreased stability of Fe-S clusters and interaction with Npl4 (Reeder et al., 2011). Copper complexes with bis(thiosemicarbazone) ligands raise copper ion levels in both cancer cells and in Chlamydia-infected host cells (Cater et al., 2013; Marsh et al., 2017). Furthermore, derivatives of quinolines also function as copper ionophores (Oliveri et al., 2017; Oliveri, 2022). Derivatives from simple compounds such as 3-Hydroxyflavone (Dai et al., 2017), as well as more intricate copper ionophores like Hydrophilic Temperature-Sensitive Liposomes (Gaal et al., 2020) and a copper ionophore designed using salicylaldehyde isonicotinoyl hydrazone (Ji et al., 2018), also increase copper levels inside cells.
Among these agents, Elesclomol (ES) and Disulfiram (DFS) are currently undergoing evaluation in clinical trials (Xie J. et al., 2023). Recent trials investigating ES (O'Day et al., 2013) and DSF (Kelley et al., 2021) have demonstrated excellent safety profiles. Current research in this area is focused on nanomedicines that combine copper ions with copper ionophores (Lee et al., 2023; Zhou et al., 2023). Combining other cancer treatments with cuproptosis-related therapy may yield improved outcomes. Overall, copper ionophores may have greater efficacy in tumors with elevated mitochondrial metabolism. In the phase III clinical trial of ES, the impact of ES varied among individuals with low serum LDH levels (O'Day et al., 2013). Thus, serum LDH levels may serve as a prognostic indicator in the future clinical use of cuproptosis-related medications, helping to assess the potential effectiveness of these drugs. To summarize, copper ionophores can be combined with targeted therapeutic agents like TKI and PI. This combination is most effective in tumors with high mitochondrial metabolic status. Additionally, LDH can be used as a predictor to guide treatment before drug administration and as a prognostic indicator afterward. Further research is necessary to ascertain the feasibility of cuproptosis-inducing therapies in select patients with distinct types of cancer.
1.7 Parthanatos as target in cancer treatment
Parthanatos is a cell death mechanism controlled by PARP-1 and is distinct from apoptosis and necroptosis (Harraz et al., 2008) (Figures 1, 2). In parthanatos, abnormal PARP-1 activation causes excessive PAR production (Dawson and Dawson, 2004), mitochondrial membrane depolarization decreases ATP and NADPH levels, and triggers AIF translocation from mitochondria to the nucleus. Additionally, AIF binds to MIF nuclease, activating it (Wang Y. et al., 2019). After translocating to the nucleus, AIF and MIF cause nuclear shrinkage, chromatin agglutination, and big DNA fragments (15–50 KB) that cause parthanatos (Zhou et al., 2021). The lack of caspase is its main characteristic.
There is a strong correlation between parthanatos and tumor formation and progression (Zhou et al., 2021). The expression level of PARP-1 in breast cancer, ovarian cancer, endometrial cancer, lung cancer, skin cancer and non-Hodgkin’s lymphoma is elevated compared to normal tissues, thus establishing a strong association between parthanatos and these cancers (Harraz et al., 2008; Fong et al., 2009; Galia et al., 2012; Dorsam et al., 2018; Pazzaglia and Pioli, 2019). PARP-1 knockout mice showed a considerable decrease in susceptibility to epithelial malignancies. Downregulating PARP-1 protein hinders the action of NF-κB and the expression of tumor-promoting proteins controlled by NF-κB, thereby preventing the induction of parthanatos (Pazzaglia and Pioli, 2019). Additionally, the absence of PARP-1 in mice resulted in a notable decrease in the occurrence of colorectal cancer caused by oxymethane (AOM) and dextran sulfate sodium (DSS). Reducing PARP-1 protein levels may effectively prevent induced colorectal cancer by suppressing the expression of cyclin D and STAT3 (Dorsam et al., 2018).
The impact of parthanatos on carcinogenesis and tumor development manifests in two key dimensions (Zhou et al., 2021). During rapid cellular proliferation, DNA is highly susceptible to radiotherapy or chemotherapy, leading to tumor cell death. PARP-1 plays a crucial role in DNA repair and is essential for tumor cell survival. Hence, inducing apoptosis in tumor cells can be achieved by suppressing PARP-1 activity. Conversely, the occurrence of parthanatos primarily arises from the abnormal activation of PARP-1. Promoting parthanatos in tumor cells by augmenting PARP-1 activity can impede tumor cell proliferation. Given PARP-1’s involvement in several DNA repair pathways and its role in maintaining genomic stability (Yang et al., 2020), modulating PARP-1 activity may be therapeutic for treating associated malignancies (Table 8).
In clinical trials, PARP inhibitors are mostly administered to cancer patients with homologous recombination repair deficiencies including those with breast and ovarian cancers carrying BRCA1 and BRCA2 mutations (gBRCA1/2m) and castration-resistant prostate cancer. Currently, Olaparib (Clarke et al., 2024; Fenton and Hussain, 2024; Kawamoto et al., 2024; Lee et al., 2024; Shah et al., 2024), niraparib (Wu X. et al., 2024), rucaparib (Monk et al., 2022; Sayyid et al., 2024), veliparib (Mizuno et al., 2023; Rodler et al., 2023; Zhao et al., 2023; Dieras et al., 2024; Kashbour et al., 2024; Sun and Li, 2024) and talazoparib (Fizazi et al., 2024; Heiss et al., 2024; Narang et al., 2024; Piha-Paul et al., 2024; Telli et al., 2024) hinder the cancer-fighting effects of parthanatos by suppressing the catalytic function of PARP-1 and PARP-2 (Fong et al., 2009; Sandhu et al., 2013; Mateo et al., 2016; de Bono et al., 2017; Nishikawa et al., 2017; Wu X. et al., 2024).
β-Lapachone, a naturally occurring compound derived from the bark of the lapacho tree, triggers parthanatos by activating the NQO1-dependent ROS-mediated RIPK1-PARP1-AIF pathway, leading to the death of hepatocellular carcinoma cells (Zhao W. et al., 2021). This process was prevented by the inclusion of a PARP-1-specific inhibitor (Park et al., 2014). Deoxypodophyllotoxin (DPT), a naturally occurring chemical derived from Anthriscus sylvestris, effectively suppressed glioma growth by promoting the generation of excessive reactive oxygen species (ROS), enhancing PARP-1 expression and facilitating AIF translocation to the cell nucleus. This has been shown in both xenograft glioma models and in glioma cells cultured in vitro (Ma D. et al., 2016).
2 Conclusion and future perspective
The evolution of cancer therapy always involves trial and error, but discovery of novel mechanisms to target the mission-critical events shared by all tumors offers a glimpse of previously unthinkable therapeutic possibilities (Debela et al., 2021; Levantini, 2023). Understanding carcinogenesis, especially through the identification of altered cellular processes that maintain cancer cells and the development of diagnostic and prognostic biomarkers, has been made possible by studying these altered cell death pathways (Koren and Fuchs, 2021; Peng et al., 2022; Zhou Y. et al., 2024). Since RCD pathways are fundamental to the genesis of all tumors, they present clear targets for therapeutic intervention in all cancer types (Koren and Fuchs, 2021; Peng et al., 2022; Gong et al., 2023). Moreover, detecting abnormalities in these signaling pathways can aid in identifying the DNA, mRNA and protein mutations present in cancer cells, and may play a significant role in determining the efficacy of specific targeted therapies (Waarts et al., 2022; Chitluri and Emerson, 2024; Liu B. et al., 2024). While a tumor’s mutational profile may impact a therapy’s effectiveness, identifying altered RCD pathways may yield identification of novel targets.
The complexity of the cellular signaling that occurs in tumor cells presents the biggest obstacle to addressing the dysregulated pathways in distinct cancers (Bou Antoun and Chioni, 2023; Swanton et al., 2024). Crosstalk and inhibitory feedback mechanisms are just two examples of the many elements that obstruct targeted signaling pathways. Additionally, the risk of resistance selection exists with all tumor therapies and this risk may be exacerbated by the genetic plasticity present in most malignancies (Emran et al., 2022; Khan et al., 2024).
The primary therapeutic challenge in targeting RCD pathways for cancer treatment lies in the emergence of resistance mechanisms (D'Amico and De Amicis, 2024). Cancer cells often experience genetic and epigenetic changes that enable them to evade or inhibit cell death signals, even in the presence of targeted therapies designed to activate these pathways (Ozyerli-Goknar and Bagci-Onder, 2021; Tufail et al., 2024). For instance, the overexpression of anti-apoptotic proteins like BCL-2 and BCL-XL or mutations in tumor suppressors such as TP53 can inhibit apoptosis, allowing cancer cells to escape death induced by chemotherapy (Mohammad et al., 2015). In a similar vein, autophagy—a mechanism that enables cells to survive under stress—can be exploited by cancer cells to endure therapeutic damage, resulting in certain cancers, such as pancreatic and lung cancer, becoming resistant to drugs aimed at metabolic pathways (Li et al., 2019; Mele et al., 2020).
Ferroptosis, serves as another significant instance where resistance develops (Nie et al., 2022). The overexpression of GPX4, a lipid peroxidase enzyme, diminishes oxidative stress and inhibits ferroptosis-mediated cell death (Xie Y. et al., 2023), enabling cells to escape therapies aimed at triggering this type of RCD, particularly in liver and pancreatic cancers. Resistance to necroptosis, arises from the inactivation of essential regulators such as RIPK1 and RIPK3, resulting in treatment resistance in cancers including glioblastoma (Xie Y. et al., 2023) and colorectal cancer (Feng et al., 2015). In cuproptosis, cancer cells evade copper-induced cell death by disrupting copper ion homeostasis, with changes in proteins such as FDX1 and DLAT contributing to resistance in lung and melanoma cancers (Abdullah et al., 2024).
Moreover, pyroptosis, can be inhibited by the dysregulation of inflammasome components such as NLRP3 and caspase-1 (Zheng M. et al., 2020). This enables cancer cells to evade the inflammatory response typically associated with pyroptosis. This evasion mechanism has been noted in cancers including colorectal, gastric, and breast cancer. Ultimately, parthanatos, associated with the overactivation of PARP1 due to DNA damage, is often evaded in breast and ovarian cancers by the overexpression of PARP1 or mutations in related pathways, which diminishes the effectiveness of PARP inhibitors in these instances (Pazzaglia and Pioli, 2019).
These examples illustrate how cancer cells’ capacity to manipulate and resist RCD pathways complicates therapeutic strategies. The flexibility and redundancy in cell death mechanisms necessitate the creation of combination therapies or innovative strategies to re-sensitize cancer cells, highlighting the importance of addressing these resistance mechanisms across different cancers. Furthermore, recent high-throughput sequencing data demonstrate the significance of these dysregulated signaling pathways in sustaining supportive TMEs that facilitate the growth and metastasis of numerous solid tumors (Wang et al., 2023). Understanding the composition and function of the TME is thus crucial for deciphering the impact of genetic and epigenetic changes that occur in tumors and the cells that surround them. By studying various tumor types, researchers may identify common pathways that contribute to tumor development.
While the caveats associated with targeting RCD pathways for cancer therapies described above are challenging, the most effective approach to address these issues likely requires use of more advanced combination therapies that target multiple lesions unique to tumors simultaneously. Building a pathway interaction network to determine the functional dependencies between different signaling pathways may offer new perspectives on disease causes and lead to development of more effective drug formulations. Future research should place a stronger emphasis on the utilization of combination therapies for studies employing patient-derived xenografts, organoids/tumoroids and genetically modified mouse models to target oncogenic signaling pathways, RCD and the TME.
Author contributions
RS: Writing–original draft, Writing–review and editing. CW: Writing–original draft, Writing–review and editing. Y-WH: Conceptualization, Writing–original draft, Writing–review and editing.
Funding
The author(s) declare that no financial support was received for the research, authorship, and/or publication of this article.
Conflict of interest
The authors declare that the research was conducted in the absence of any commercial or financial relationships that could be construed as a potential conflict of interest.
The author(s) declared that they were an editorial board member of Frontiers, at the time of submission. This had no impact on the peer review process and the final decision.
Publisher’s note
All claims expressed in this article are solely those of the authors and do not necessarily represent those of their affiliated organizations, or those of the publisher, the editors and the reviewers. Any product that may be evaluated in this article, or claim that may be made by its manufacturer, is not guaranteed or endorsed by the publisher.
References
Abdollahzadeh, H., Pazhang, Y., Zamani, A., and Sharafi, Y. (2024). Green synthesis of copper oxide nanoparticles using walnut shell and their size dependent anticancer effects on breast and colorectal cancer cell lines. Sci. Rep. 14 (1), 20323. doi:10.1038/s41598-024-71234-4
Abdullah, K. M., Kaushal, J. B., Takkar, S., Sharma, G., Alsafwani, Z. W., Pothuraju, R., et al. (2024). Copper metabolism and cuproptosis in human malignancies: unraveling the complex interplay for therapeutic insights. Heliyon 10 (5), e27496. doi:10.1016/j.heliyon.2024.e27496
Agalakova, N. I. (2024). Chloroquine and chemotherapeutic compounds in experimental cancer treatment. Int. J. Mol. Sci. 25 (2), 945. doi:10.3390/ijms25020945
Ahmadi-Dehlaghi, F., Mohammadi, P., Valipour, E., Pournaghi, P., Kiani, S., and Mansouri, K. (2023). Autophagy: a challengeable paradox in cancer treatment. Cancer Med. 12 (10), 11542–11569. doi:10.1002/cam4.5577
Akin, D., Wang, S. K., Habibzadegah-Tari, P., Law, B., Ostrov, D., Li, M., et al. (2014). A novel ATG4B antagonist inhibits autophagy and has a negative impact on osteosarcoma tumors. Autophagy 10 (11), 2021–2035. doi:10.4161/auto.32229
Alam, M., Alam, S., Shamsi, A., Adnan, M., Elasbali, A. M., Al-Soud, W. A., et al. (2022). Bax/Bcl-2 cascade is regulated by the EGFR pathway: therapeutic targeting of non-small cell lung cancer. Front. Oncol. 12, 869672. doi:10.3389/fonc.2022.869672
Alameda, J. P., Moreno-Maldonado, R., Navarro, M., Bravo, A., Ramirez, A., Page, A., et al. (2010). An inactivating CYLD mutation promotes skin tumor progression by conferring enhanced proliferative, survival and angiogenic properties to epidermal cancer cells. Oncogene 29 (50), 6522–6532. doi:10.1038/onc.2010.378
Aleksandrova, K. V., and Suvorova, I. I. (2023). Evaluation of the effectiveness of various autophagy inhibitors in A549 cancer stem cells. Acta Naturae 15 (1), 19–25. doi:10.32607/actanaturae.11891
Ali, A. M., Atmaj, J., Van Oosterwijk, N., Groves, M. R., and Domling, A. (2019). Stapled peptides inhibitors: a new window for target drug discovery. Comput. Struct. Biotechnol. J. 17, 263–281. doi:10.1016/j.csbj.2019.01.012
Alidadi, H., Ashtari, A., Samimi, A., Karami, M. A., and Khorsandi, L. (2022). Myricetin loaded in solid lipid nanoparticles induces apoptosis in the HT-29 colorectal cancer cells via mitochondrial dysfunction. Mol. Biol. Rep. 49 (9), 8537–8545. doi:10.1007/s11033-022-07683-9
Amarante-Mendes, G. P., Adjemian, S., Branco, L. M., Zanetti, L. C., Weinlich, R., and Bortoluci, K. R. (2018). Pattern recognition receptors and the host cell death molecular machinery. Front. Immunol. 9, 2379. doi:10.3389/fimmu.2018.02379
Amaravadi, R. K., Schilder, R. J., Martin, L. P., Levin, M., Graham, M. A., Weng, D. E., et al. (2015). A phase I study of the SMAC-mimetic birinapant in adults with refractory solid tumors or lymphoma. Mol. Cancer Ther. 14 (11), 2569–2575. doi:10.1158/1535-7163.MCT-15-0475
Amaravadi, R. K., and Winkler, J. D. (2012). Lys05: a new lysosomal autophagy inhibitor. Autophagy 8 (9), 1383–1384. doi:10.4161/auto.20958
Andreeff, M., Kelly, K. R., Yee, K., Assouline, S., Strair, R., Popplewell, L., et al. (2016). Results of the phase I trial of RG7112, a small-molecule MDM2 antagonist in leukemia. Clin. Cancer Res. 22 (4), 868–876. doi:10.1158/1078-0432.CCR-15-0481
Annibaldi, A., and Walczak, H. (2020). Death receptors and their ligands in inflammatory disease and cancer. Cold Spring Harb. Perspect. Biol. 12 (9), a036384. doi:10.1101/cshperspect.a036384
Bakar-Ates, F., and Ozkan, E. (2024). Synergistic ferroptosis in triple-negative breast cancer cells: paclitaxel in combination with Erastin induced oxidative stress and Ferroportin-1 modulation in MDA-MB-231 cells. Naunyn Schmiedeb. Arch. Pharmacol. doi:10.1007/s00210-024-03523-8
Basuli, D., Tesfay, L., Deng, Z., Paul, B., Yamamoto, Y., Ning, G., et al. (2017). Iron addiction: a novel therapeutic target in ovarian cancer. Oncogene 36 (29), 4089–4099. doi:10.1038/onc.2017.11
Baszuk, P., Marciniak, W., Derkacz, R., Jakubowska, A., Cybulski, C., Gronwald, J., et al. (2021). Blood copper levels and the occurrence of colorectal cancer in Poland. Biomedicines 9 (11), 1628. doi:10.3390/biomedicines9111628
Bejarano, E., and Cuervo, A. M. (2010). Chaperone-mediated autophagy. Proc. Am. Thorac. Soc. 7 (1), 29–39. doi:10.1513/pats.200909-102JS
Bell, H. N., Stockwell, B. R., and Zou, W. (2024). Ironing out the role of ferroptosis in immunity. Immunity 57 (5), 941–956. doi:10.1016/j.immuni.2024.03.019
Belyanskaya, L. L., Marti, T. M., Hopkins-Donaldson, S., Kurtz, S., Felley-Bosco, E., and Stahel, R. A. (2007). Human agonistic TRAIL receptor antibodies Mapatumumab and Lexatumumab induce apoptosis in malignant mesothelioma and act synergistically with cisplatin. Mol. Cancer 6, 66. doi:10.1186/1476-4598-6-66
Benguigui, M., Weitz, I. S., Timaner, M., Kan, T., Shechter, D., Perlman, O., et al. (2019). Copper oxide nanoparticles inhibit pancreatic tumor growth primarily by targeting tumor initiating cells. Sci. Rep. 9 (1), 12613. doi:10.1038/s41598-019-48959-8
Berke, T. P., Slight, S. H., and Hyder, S. M. (2022). Role of reactivating mutant p53 protein in suppressing growth and metastasis of triple-negative breast cancer. Onco Targets Ther. 15, 23–30. doi:10.2147/OTT.S342292
Bhat, G. R., Sethi, I., Sadida, H. Q., Rah, B., Mir, R., Algehainy, N., et al. (2024). Cancer cell plasticity: from cellular, molecular, and genetic mechanisms to tumor heterogeneity and drug resistance. Cancer Metastasis Rev. 43 (1), 197–228. doi:10.1007/s10555-024-10172-z
Blagosklonny, M. V. (2023). Cancer prevention with rapamycin. Oncotarget 14, 342–350. doi:10.18632/oncotarget.28410
Blockhuys, S., Celauro, E., Hildesjo, C., Feizi, A., Stal, O., Fierro-Gonzalez, J. C., et al. (2017). Defining the human copper proteome and analysis of its expression variation in cancers. Metallomics 9 (2), 112–123. doi:10.1039/c6mt00202a
Blockhuys, S., and Wittung-Stafshede, P. (2017). Roles of copper-binding proteins in breast cancer. Int. J. Mol. Sci. 18 (4), 871. doi:10.3390/ijms18040871
Bolden, J. E., Peart, M. J., and Johnstone, R. W. (2006). Anticancer activities of histone deacetylase inhibitors. Nat. Rev. Drug Discov. 5 (9), 769–784. doi:10.1038/nrd2133
Boschi, A., Martini, P., Janevik-Ivanovska, E., and Duatti, A. (2018). The emerging role of copper-64 radiopharmaceuticals as cancer theranostics. Drug Discov. Today 23 (8), 1489–1501. doi:10.1016/j.drudis.2018.04.002
Bou Antoun, N., and Chioni, A. M. (2023). Dysregulated signalling pathways driving anticancer drug resistance. Int. J. Mol. Sci. 24 (15), 12222. doi:10.3390/ijms241512222
Boulos, J. C., Rahama, M., Hegazy, M. F., and Efferth, T. (2019). Shikonin derivatives for cancer prevention and therapy. Cancer Lett. 459, 248–267. doi:10.1016/j.canlet.2019.04.033
Brewer, G. J., Dick, R. D., Grover, D. K., LeClaire, V., Tseng, M., Wicha, M., et al. (2000). Treatment of metastatic cancer with tetrathiomolybdate, an anticopper, antiangiogenic agent: phase I study. Clin. Cancer Res. 6 (1), 1–10.
Brown, J. S., Amend, S. R., Austin, R. H., Gatenby, R. A., Hammarlund, E. U., and Pienta, K. J. (2023). Updating the definition of cancer. Mol. Cancer Res. 21 (11), 1142–1147. doi:10.1158/1541-7786.MCR-23-0411
Burvenich, I. J., Lee, F. T., Guo, N., Gan, H. K., Rigopoulos, A., Parslow, A. C., et al. (2016). In vitro and in vivo evaluation of (89)Zr-DS-8273a as a theranostic for anti-death receptor 5 therapy. Theranostics 6 (12), 2225–2234. doi:10.7150/thno.16260
Caenepeel, S., Brown, S. P., Belmontes, B., Moody, G., Keegan, K. S., Chui, D., et al. (2018). AMG 176, a selective MCL1 inhibitor, is effective in hematologic cancer models alone and in combination with established therapies. Cancer Discov. 8 (12), 1582–1597. doi:10.1158/2159-8290.CD-18-0387
Cai, Z., Jitkaew, S., Zhao, J., Chiang, H. C., Choksi, S., Liu, J., et al. (2014). Plasma membrane translocation of trimerized MLKL protein is required for TNF-induced necroptosis. Nat. Cell Biol. 16 (1), 55–65. doi:10.1038/ncb2883
Cao, L., and Mu, W. (2021). Necrostatin-1 and necroptosis inhibition: pathophysiology and therapeutic implications. Pharmacol. Res. 163, 105297. doi:10.1016/j.phrs.2020.105297
Carew, J. S., and Nawrocki, S. T. (2017). Drain the lysosome: development of the novel orally available autophagy inhibitor ROC-325. Autophagy 13 (4), 765–766. doi:10.1080/15548627.2017.1280222
Carneiro, B. A., and El-Deiry, W. S. (2020). Targeting apoptosis in cancer therapy. Nat. Rev. Clin. Oncol. 17 (7), 395–417. doi:10.1038/s41571-020-0341-y
Cater, M. A., Pearson, H. B., Wolyniec, K., Klaver, P., Bilandzic, M., Paterson, B. M., et al. (2013). Increasing intracellular bioavailable copper selectively targets prostate cancer cells. ACS Chem. Biol. 8 (7), 1621–1631. doi:10.1021/cb400198p
Chan, N., Willis, A., Kornhauser, N., Ward, M. M., Lee, S. B., Nackos, E., et al. (2017). Influencing the tumor microenvironment: a phase II study of copper depletion using tetrathiomolybdate in patients with breast cancer at high risk for recurrence and in preclinical models of lung metastases. Clin. Cancer Res. 23 (3), 666–676. doi:10.1158/1078-0432.CCR-16-1326
Chang, Y. S., Graves, B., Guerlavais, V., Tovar, C., Packman, K., To, K. H., et al. (2013). Stapled α-helical peptide drug development: a potent dual inhibitor of MDM2 and MDMX for p53-dependent cancer therapy. Proc. Natl. Acad. Sci. U. S. A. 110 (36), E3445–E3454. doi:10.1073/pnas.1303002110
Chatran, M., Pilehvar-Soltanahmadi, Y., Dadashpour, M., Faramarzi, L., Rasouli, S., Jafari-Gharabaghlou, D., et al. (2018). Synergistic anti-proliferative effects of metformin and silibinin combination on T47D breast cancer cells via hTERT and cyclin D1 inhibition. Drug Res. (Stuttg) 68 (12), 710–716. doi:10.1055/a-0631-8046
Chavez-Dominguez, R., Perez-Medina, M., Lopez-Gonzalez, J. S., Galicia-Velasco, M., and Aguilar-Cazares, D. (2020). The double-edge sword of autophagy in cancer: from tumor suppression to pro-tumor activity. Front. Oncol. 10, 578418. doi:10.3389/fonc.2020.578418
Chen, G., Ding, X. F., Bouamar, H., Pressley, K., and Sun, L. Z. (2019). Everolimus induces G(1) cell cycle arrest through autophagy-mediated protein degradation of cyclin D1 in breast cancer cells. Am. J. Physiol. Cell Physiol. 317 (2), C244–C252. doi:10.1152/ajpcell.00390.2018
Chen, G. Y., Meng, C. L., Lin, K. C., Tuan, H. Y., Yang, H. J., Chen, C. L., et al. (2015). Graphene oxide as a chemosensitizer: diverted autophagic flux, enhanced nuclear import, elevated necrosis and improved antitumor effects. Biomaterials 40, 12–22. doi:10.1016/j.biomaterials.2014.11.034
Chen, G. Y., and Nunez, G. (2010). Sterile inflammation: sensing and reacting to damage. Nat. Rev. Immunol. 10 (12), 826–837. doi:10.1038/nri2873
Chen, J., Ge, L., Shi, X., Liu, J., Ruan, H., Heng, D., et al. (2022). Lobaplatin induces pyroptosis in cervical cancer cells via the caspase-3/GSDME pathway. Anticancer Agents Med. Chem. 22 (11), 2091–2097. doi:10.2174/1871520621666211018100532
Chen, L., Niu, X., Qiao, X., Liu, S., Ma, H., Shi, X., et al. (2021a). Characterization of interplay between autophagy and ferroptosis and their synergistical roles on manipulating immunological tumor microenvironment in squamous cell carcinomas. Front. Immunol. 12, 739039. doi:10.3389/fimmu.2021.739039
Chen, Q., Kang, J., and Fu, C. (2018). The independence of and associations among apoptosis, autophagy, and necrosis. Signal Transduct. Target Ther. 3, 18. doi:10.1038/s41392-018-0018-5
Chen, X., Kang, R., Kroemer, G., and Tang, D. (2021b). Ferroptosis in infection, inflammation, and immunity. J. Exp. Med. 218 (6), e20210518. doi:10.1084/jem.20210518
Cheng, A. L., Kang, Y. K., Chen, Z., Tsao, C. J., Qin, S., Kim, J. S., et al. (2009). Efficacy and safety of sorafenib in patients in the Asia-Pacific region with advanced hepatocellular carcinoma: a phase III randomised, double-blind, placebo-controlled trial. Lancet Oncol. 10 (1), 25–34. doi:10.1016/S1470-2045(08)70285-7
Chisholm, C. L., Wang, H., Wong, A. H., Vazquez-Ortiz, G., Chen, W., Xu, X., et al. (2016). Ammonium tetrathiomolybdate treatment targets the copper transporter ATP7A and enhances sensitivity of breast cancer to cisplatin. Oncotarget 7 (51), 84439–84452. doi:10.18632/oncotarget.12992
Chitluri, K. K., and Emerson, I. A. (2024). The importance of protein domain mutations in cancer therapy. Heliyon 10 (6), e27655. doi:10.1016/j.heliyon.2024.e27655
Choi, Y. K., Kang, J. I., Han, S., Kim, Y. R., Jo, J., Kang, Y. W., et al. (2020). L-ascorbic acid inhibits breast cancer growth by inducing IRE/JNK/CHOP-related endoplasmic reticulum stress-mediated p62/SQSTM1 accumulation in the nucleus. Nutrients 12 (5), 1351. doi:10.3390/nu12051351
Ciuleanu, T., Bazin, I., Lungulescu, D., Miron, L., Bondarenko, I., Deptala, A., et al. (2016). A randomized, double-blind, placebo-controlled phase II study to assess the efficacy and safety of mapatumumab with sorafenib in patients with advanced hepatocellular carcinoma. Ann. Oncol. 27 (4), 680–687. doi:10.1093/annonc/mdw004
Clarke, N. W., Armstrong, A. J., Oya, M., Shore, N., Procopio, G., Daniel Guedes, J., et al. (2024). Efficacy and safety of olaparib plus abiraterone versus placebo plus abiraterone in the first-line treatment of patients with asymptomatic/mildly symptomatic and symptomatic metastatic castration-resistant prostate cancer: analyses from the phase 3 PROpel trial. Eur. Urol. Oncol. doi:10.1016/j.euo.2024.09.013
Cluzeau, T., Sebert, M., Rahme, R., Cuzzubbo, S., Lehmann-Che, J., Madelaine, I., et al. (2021). Eprenetapopt Plus Azacitidine in TP53-Mutated Myelodysplastic Syndromes and Acute Myeloid Leukemia: A Phase II Study by the Groupe Francophone des Myelodysplasies (GFM). J. Clin. Oncol. 39 (14), 1575–1583. doi:10.1200/JCO.20.02342
Cook, K. L., Warri, A., Soto-Pantoja, D. R., Clarke, P. A., Cruz, M. I., Zwart, A., et al. (2014). Hydroxychloroquine inhibits autophagy to potentiate antiestrogen responsiveness in ER+ breast cancer. Clin. Cancer Res. 20 (12), 3222–3232. doi:10.1158/1078-0432.CCR-13-3227
Cui, L., Gouw, A. M., LaGory, E. L., Guo, S., Attarwala, N., Tang, Y., et al. (2021). Mitochondrial copper depletion suppresses triple-negative breast cancer in mice. Nat. Biotechnol. 39 (3), 357–367. doi:10.1038/s41587-020-0707-9
Dabiri, Y., Abu El Maaty, M. A., Chan, H. Y., Wolker, J., Ott, I., Wolfl, S., et al. (2019). p53-Dependent anti-proliferative and pro-apoptotic effects of a gold(I) N-heterocyclic carbene (NHC) complex in colorectal cancer cells. Front. Oncol. 9, 438. doi:10.3389/fonc.2019.00438
Dai, F., Yan, W. J., Du, Y. T., Bao, X. Z., Li, X. Z., and Zhou, B. (2017). Structural basis, chemical driving forces and biological implications of flavones as Cu(II) ionophores. Free Radic. Biol. Med. 108, 554–563. doi:10.1016/j.freeradbiomed.2017.04.023
D'Amico, M., and De Amicis, F. (2024). Challenges of regulated cell death: implications for therapy resistance in cancer. Cells 13 (13), 1083. doi:10.3390/cells13131083
Dang, Q., Sun, Z., Wang, Y., Wang, L., Liu, Z., and Han, X. (2022). Ferroptosis: a double-edged sword mediating immune tolerance of cancer. Cell Death Dis. 13 (11), 925. doi:10.1038/s41419-022-05384-6
Dawson, V. L., and Dawson, T. M. (2004). Deadly conversations: nuclear-mitochondrial cross-talk. J. Bioenerg. Biomembr. 36 (4), 287–294. doi:10.1023/B:JOBB.0000041755.22613.8d
Debela, D. T., Muzazu, S. G., Heraro, K. D., Ndalama, M. T., Mesele, B. W., Haile, D. C., et al. (2021). New approaches and procedures for cancer treatment: current perspectives. SAGE Open Med. 9, 20503121211034366. doi:10.1177/20503121211034366
Debnath, J., Gammoh, N., and Ryan, K. M. (2023). Autophagy and autophagy-related pathways in cancer. Nat. Rev. Mol. Cell Biol. 24 (8), 560–575. doi:10.1038/s41580-023-00585-z
de Bono, J., Ramanathan, R. K., Mina, L., Chugh, R., Glaspy, J., Rafii, S., et al. (2017). Phase I, dose-escalation, two-Part Trial of the PARP inhibitor talazoparib in patients with advanced germline BRCA1/2 mutations and selected sporadic cancers. Cancer Discov. 7 (6), 620–629. doi:10.1158/2159-8290.CD-16-1250
Degterev, A., Huang, Z., Boyce, M., Li, Y., Jagtap, P., Mizushima, N., et al. (2005). Chemical inhibitor of nonapoptotic cell death with therapeutic potential for ischemic brain injury. Nat. Chem. Biol. 1 (2), 112–119. doi:10.1038/nchembio711
Del Gaizo Moore, V., Brown, J. R., Certo, M., Love, T. M., Novina, C. D., and Letai, A. (2007). Chronic lymphocytic leukemia requires BCL2 to sequester prodeath BIM, explaining sensitivity to BCL2 antagonist ABT-737. J. Clin. Invest. 117 (1), 112–121. doi:10.1172/JCI28281
Deng, W., Xiong, X., Lu, M., Huang, S., Luo, Y., Wang, Y., et al. (2024). Curcumin suppresses colorectal tumorigenesis through restoring the gut microbiota and metabolites. BMC Cancer 24 (1), 1141. doi:10.1186/s12885-024-12898-z
Devor, E. J., Schickling, B. M., Lapierre, J. R., Bender, D. P., Gonzalez-Bosquet, J., and Leslie, K. K. (2021). The synthetic curcumin analog HO-3867 rescues suppression of PLAC1 expression in ovarian cancer cells. Pharm. (Basel) 14 (9), 942. doi:10.3390/ph14090942
Dhuriya, Y. K., and Sharma, D. (2018). Necroptosis: a regulated inflammatory mode of cell death. J. Neuroinflammation 15 (1), 199. doi:10.1186/s12974-018-1235-0
Di Cosimo, S., Perez-Garcia, J. M., Bellet, M., Dalenc, F., Gil Gil, M. J., Ruiz Borrego, M., et al. (2023). Palbociclib with fulvestrant or letrozole in endocrine-sensitive patients with HR-positive/HER2-negative advanced breast cancer: a detailed safety analysis of the randomized parsifal trial. Oncologist 28 (1), 23–32. doi:10.1093/oncolo/oyac205
Dieras, V., Han, H. S., Wildiers, H., Friedlander, M., Ayoub, J. P., Puhalla, S. L., et al. (2024). Veliparib with carboplatin and paclitaxel in BRCA-mutated advanced breast cancer (BROCADE3): final overall survival results from a randomized phase 3 trial. Eur. J. Cancer 200, 113580. doi:10.1016/j.ejca.2024.113580
Dilley, R. L., Poh, W., Gladstone, D. E., Herman, J. G., Showel, M. M., Karp, J. E., et al. (2014). Poly(ADP-ribose) polymerase inhibitor CEP-8983 synergizes with bendamustine in chronic lymphocytic leukemia cells in vitro. Leuk. Res. 38 (3), 411–417. doi:10.1016/j.leukres.2013.12.019
DiNardo, C. D., Pratz, K., Pullarkat, V., Jonas, B. A., Arellano, M., Becker, P. S., et al. (2019). Venetoclax combined with decitabine or azacitidine in treatment-naive, elderly patients with acute myeloid leukemia. Blood 133 (1), 7–17. doi:10.1182/blood-2018-08-868752
Ding, J., Wang, K., Liu, W., She, Y., Sun, Q., Shi, J., et al. (2016). Pore-forming activity and structural autoinhibition of the gasdermin family. Nature 535 (7610), 111–116. doi:10.1038/nature18590
Ding, P., Wen, L., Tong, F., Zhang, R., Huang, Y., and Dong, X. (2022). Mechanism underlying the immune checkpoint inhibitor-induced hyper-progressive state of cancer. Cancer Drug Resist 5 (1), 147–164. doi:10.20517/cdr.2021.104
Ding, Q., Zhang, Z., Liu, J. J., Jiang, N., Zhang, J., Ross, T. M., et al. (2013). Discovery of RG7388, a potent and selective p53-MDM2 inhibitor in clinical development. J. Med. Chem. 56 (14), 5979–5983. doi:10.1021/jm400487c
Dixon, S. J., Lemberg, K. M., Lamprecht, M. R., Skouta, R., Zaitsev, E. M., Gleason, C. E., et al. (2012). Ferroptosis: an iron-dependent form of nonapoptotic cell death. Cell 149 (5), 1060–1072. doi:10.1016/j.cell.2012.03.042
Dixon, S. J., Patel, D. N., Welsch, M., Skouta, R., Lee, E. D., Hayano, M., et al. (2014). Pharmacological inhibition of cystine-glutamate exchange induces endoplasmic reticulum stress and ferroptosis. Elife 3, e02523. doi:10.7554/eLife.02523
Dohner, H., Pratz, K. W., DiNardo, C. D., Wei, A. H., Jonas, B. A., Pullarkat, V., et al. (2024). Genetic risk stratification and outcomes among treatment-naive patients with AML treated with venetoclax and azacitidine. Blood, 2024024944. doi:10.1182/blood.2024024944
Doll, S., Proneth, B., Tyurina, Y. Y., Panzilius, E., Kobayashi, S., Ingold, I., et al. (2017). ACSL4 dictates ferroptosis sensitivity by shaping cellular lipid composition. Nat. Chem. Biol. 13 (1), 91–98. doi:10.1038/nchembio.2239
Dominguez, G. A., Condamine, T., Mony, S., Hashimoto, A., Wang, F., Liu, Q., et al. (2017). Selective targeting of myeloid-derived suppressor cells in cancer patients using DS-8273a, an agonistic TRAIL-R2 antibody. Clin. Cancer Res. 23 (12), 2942–2950. doi:10.1158/1078-0432.CCR-16-1784
Donohue, E., Tovey, A., Vogl, A. W., Arns, S., Sternberg, E., Young, R. N., et al. (2011). Inhibition of autophagosome formation by the benzoporphyrin derivative verteporfin. J. Biol. Chem. 286 (9), 7290–7300. doi:10.1074/jbc.M110.139915
Dorsam, B., Seiwert, N., Foersch, S., Stroh, S., Nagel, G., Begaliew, D., et al. (2018). PARP-1 protects against colorectal tumor induction, but promotes inflammation-driven colorectal tumor progression. Proc. Natl. Acad. Sci. U. S. A. 115 (17), E4061–E4070. doi:10.1073/pnas.1712345115
Draganov, D., Gopalakrishna-Pillai, S., Chen, Y. R., Zuckerman, N., Moeller, S., Wang, C., et al. (2015). Modulation of P2X4/P2X7/Pannexin-1 sensitivity to extracellular ATP via Ivermectin induces a non-apoptotic and inflammatory form of cancer cell death. Sci. Rep. 5, 16222. doi:10.1038/srep16222
Du, J., Wang, X., Li, Y., Ren, X., Zhou, Y., Hu, W., et al. (2021a). DHA exhibits synergistic therapeutic efficacy with cisplatin to induce ferroptosis in pancreatic ductal adenocarcinoma via modulation of iron metabolism. Cell Death Dis. 12 (7), 705. doi:10.1038/s41419-021-03996-y
Du, Y., Zhao, H. C., Zhu, H. C., Jin, Y., and Wang, L. (2021b). Ferroptosis is involved in the anti-tumor effect of lycorine in renal cell carcinoma cells. Oncol. Lett. 22 (5), 781. doi:10.3892/ol.2021.13042
Dubuisson, A., and Micheau, O. (2017). Antibodies and derivatives targeting DR4 and DR5 for cancer therapy. Antibodies (Basel) 6 (4), 16. doi:10.3390/antib6040016
Duffy, M. J., Murray, A., Synnott, N. C., O'Donovan, N., and Crown, J. (2017). Vitamin D analogues: potential use in cancer treatment. Crit. Rev. Oncol. Hematol. 112, 190–197. doi:10.1016/j.critrevonc.2017.02.015
Dunkle, A., and He, Y. W. (2011). Apoptosis and autophagy in the regulation of T lymphocyte function. Immunol. Res. 49 (1-3), 70–86. doi:10.1007/s12026-010-8195-5
Eling, N., Reuter, L., Hazin, J., Hamacher-Brady, A., and Brady, N. R. (2015). Identification of artesunate as a specific activator of ferroptosis in pancreatic cancer cells. Oncoscience 2 (5), 517–532. doi:10.18632/oncoscience.160
Elmore, S. (2007). Apoptosis: a review of programmed cell death. Toxicol. Pathol. 35 (4), 495–516. doi:10.1080/01926230701320337
Emran, T. B., Shahriar, A., Mahmud, A. R., Rahman, T., Abir, M. H., Siddiquee, M. F., et al. (2022). Multidrug resistance in cancer: understanding molecular mechanisms, immunoprevention and therapeutic approaches. Front. Oncol. 12, 891652. doi:10.3389/fonc.2022.891652
Erkes, D. A., Cai, W., Sanchez, I. M., Purwin, T. J., Rogers, C., Field, C. O., et al. (2020). Mutant BRAF and MEK inhibitors regulate the tumor immune microenvironment via pyroptosis. Cancer Discov. 10 (2), 254–269. doi:10.1158/2159-8290.CD-19-0672
Fang, Y., Tian, S., Pan, Y., Li, W., Wang, Q., Tang, Y., et al. (2020). Pyroptosis: a new frontier in cancer. Biomed. Pharmacother. 121, 109595. doi:10.1016/j.biopha.2019.109595
Fantone, S., Piani, F., Olivieri, F., Rippo, M. R., Sirico, A., Di Simone, N., et al. (2024). Role of slc7a11/xCT in ovarian cancer. Int. J. Mol. Sci. 25 (1), 587. doi:10.3390/ijms25010587
Feldmann, F., Schenk, B., Martens, S., Vandenabeele, P., and Fulda, S. (2017). Sorafenib inhibits therapeutic induction of necroptosis in acute leukemia cells. Oncotarget 8 (40), 68208–68220. doi:10.18632/oncotarget.19919
Feng, S., Fox, D., and Man, S. M. (2018). Mechanisms of gasdermin family members in inflammasome signaling and cell death. J. Mol. Biol. 430 (18 Pt B), 3068–3080. doi:10.1016/j.jmb.2018.07.002
Feng, W., Shi, W., Liu, S., Liu, H., Liu, Y., Ge, P., et al. (2022). Fe(III)-Shikonin supramolecular nanomedicine for combined therapy of tumor via ferroptosis and necroptosis. Adv. Healthc. Mater 11 (2), e2101926. doi:10.1002/adhm.202101926
Feng, W., Ye, F., Xue, W., Zhou, Z., and Kang, Y. J. (2009). Copper regulation of hypoxia-inducible factor-1 activity. Mol. Pharmacol. 75 (1), 174–182. doi:10.1124/mol.108.051516
Feng, X., Song, Q., Yu, A., Tang, H., Peng, Z., and Wang, X. (2015). Receptor-interacting protein kinase 3 is a predictor of survival and plays a tumor suppressive role in colorectal cancer. Neoplasma 62 (4), 592–601. doi:10.4149/neo_2015_071
Feng, Y., He, D., Yao, Z., and Klionsky, D. J. (2014). The machinery of macroautophagy. Cell Res. 24 (1), 24–41. doi:10.1038/cr.2013.168
Feng, Y., Yang, Z., Wang, J., and Zhao, H. (2024). Cuproptosis: unveiling a new frontier in cancer biology and therapeutics. Cell Commun. Signal 22 (1), 249. doi:10.1186/s12964-024-01625-7
Fenton, S. E., and Hussain, M. (2024). Olaparib monotherapy or in combination with abiraterone for treating mutated metastatic castration-resistant prostate cancer: alone or stronger together? Expert Opin. Investig. Drugs 33 (10), 993–999. doi:10.1080/13543784.2024.2391828
Fizazi, K., Azad, A. A., Matsubara, N., Carles, J., Fay, A. P., De Giorgi, U., et al. (2024). First-line talazoparib with enzalutamide in HRR-deficient metastatic castration-resistant prostate cancer: the phase 3 TALAPRO-2 trial. Nat. Med. 30 (1), 257–264. doi:10.1038/s41591-023-02704-x
Fong, P. C., Boss, D. S., Yap, T. A., Tutt, A., Wu, P., Mergui-Roelvink, M., et al. (2009). Inhibition of poly(ADP-ribose) polymerase in tumors from BRCA mutation carriers. N. Engl. J. Med. 361 (2), 123–134. doi:10.1056/NEJMoa0900212
Forero, A., Bendell, J. C., Kumar, P., Janisch, L., Rosen, M., Wang, Q., et al. (2017). First-in-human study of the antibody DR5 agonist DS-8273a in patients with advanced solid tumors. Invest. New Drugs 35 (3), 298–306. doi:10.1007/s10637-016-0420-1
Forero-Torres, A., Infante, J. R., Waterhouse, D., Wong, L., Vickers, S., Arrowsmith, E., et al. (2013). Phase 2, multicenter, open-label study of tigatuzumab (CS-1008), a humanized monoclonal antibody targeting death receptor 5, in combination with gemcitabine in chemotherapy-naive patients with unresectable or metastatic pancreatic cancer. Cancer Med. 2 (6), 925–932. doi:10.1002/cam4.137
Forero-Torres, A., Shah, J., Wood, T., Posey, J., Carlisle, R., Copigneaux, C., et al. (2010). Phase I trial of weekly tigatuzumab, an agonistic humanized monoclonal antibody targeting death receptor 5 (DR5). Cancer Biother Radiopharm. 25 (1), 13–19. doi:10.1089/cbr.2009.0673
Franchi, L., Munoz-Planillo, R., and Nunez, G. (2012). Sensing and reacting to microbes through the inflammasomes. Nat. Immunol. 13 (4), 325–332. doi:10.1038/ni.2231
Frenzel, A., Grespi, F., Chmelewskij, W., and Villunger, A. (2009). Bcl2 family proteins in carcinogenesis and the treatment of cancer. Apoptosis 14 (4), 584–596. doi:10.1007/s10495-008-0300-z
Fulda, S., and Vucic, D. (2012). Targeting IAP proteins for therapeutic intervention in cancer. Nat. Rev. Drug Discov. 11 (2), 109–124. doi:10.1038/nrd3627
Gaal, A., Garay, T. M., Horvath, I., Mathe, D., Szollosi, D., Veres, D. S., et al. (2020). Development and in vivo application of a water-soluble anticancer copper ionophore system using a temperature-sensitive liposome formulation. Pharmaceutics 12 (5), 466. doi:10.3390/pharmaceutics12050466
Galia, A., Calogero, A. E., Condorelli, R., Fraggetta, F., La Corte, A., Ridolfo, F., et al. (2012). PARP-1 protein expression in glioblastoma multiforme. Eur. J. Histochem 56 (1), e9. doi:10.4081/ejh.2012.e9
Galluzzi, L., Vitale, I., Aaronson, S. A., Abrams, J. M., Adam, D., Agostinis, P., et al. (2018). Molecular mechanisms of cell death: recommendations of the nomenclature committee on cell death 2018. Cell Death Differ. 25 (3), 486–541. doi:10.1038/s41418-017-0012-4
Gan, L., Wang, J., Xu, H., and Yang, X. (2011). Resistance to docetaxel-induced apoptosis in prostate cancer cells by p38/p53/p21 signaling. Prostate 71 (11), 1158–1166. doi:10.1002/pros.21331
Gandhi, L., Camidge, D. R., Ribeiro de Oliveira, M., Bonomi, P., Gandara, D., Khaira, D., et al. (2011). Phase I study of Navitoclax (ABT-263), a novel Bcl-2 family inhibitor, in patients with small-cell lung cancer and other solid tumors. J. Clin. Oncol. 29 (7), 909–916. doi:10.1200/JCO.2010.31.6208
Gao, W., Huang, Z., Duan, J., Nice, E. C., Lin, J., and Huang, C. (2021). Elesclomol induces copper-dependent ferroptosis in colorectal cancer cells via degradation of ATP7A. Mol. Oncol. 15 (12), 3527–3544. doi:10.1002/1878-0261.13079
Gardner, B. M., Pincus, D., Gotthardt, K., Gallagher, C. M., and Walter, P. (2013). Endoplasmic reticulum stress sensing in the unfolded protein response. Cold Spring Harb. Perspect. Biol. 5 (3), a013169. doi:10.1101/cshperspect.a013169
Gaschler, M. M., Andia, A. A., Liu, H., Csuka, J. M., Hurlocker, B., Vaiana, C. A., et al. (2018). FINO(2) initiates ferroptosis through GPX4 inactivation and iron oxidation. Nat. Chem. Biol. 14 (5), 507–515. doi:10.1038/s41589-018-0031-6
Ge, E. J., Bush, A. I., Casini, A., Cobine, P. A., Cross, J. R., DeNicola, G. M., et al. (2022). Connecting copper and cancer: from transition metal signalling to metalloplasia. Nat. Rev. Cancer 22 (2), 102–113. doi:10.1038/s41568-021-00417-2
Ghasemi, P., Shafiee, G., Ziamajidi, N., and Abbasalipourkabir, R. (2023). Copper nanoparticles induce apoptosis and oxidative stress in SW480 human colon cancer cell line. Biol. Trace Elem. Res. 201 (8), 3746–3754. doi:10.1007/s12011-022-03458-2
Gill, H. (2024). Chemotherapy-free approaches to newly-diagnosed acute promyelocytic leukaemia: is oral-arsenic trioxide/all-trans retinoic acid/ascorbic acid the answer? Expert Rev. Hematol. 17 (10), 661–667. doi:10.1080/17474086.2024.2391098
Gill, S., and Brudno, J. N. (2021). CAR T-cell therapy in hematologic malignancies: clinical role, toxicity, and unanswered questions. Am. Soc. Clin. Oncol. Educ. Book 41, 1–20. doi:10.1200/EDBK_320085
Giordano, F., D'Amico, M., Montalto, F. I., Malivindi, R., Chimento, A., Conforti, F. L., et al. (2023). Cdk4 regulates glioblastoma cell invasion and stemness and is target of a notch inhibitor plus resveratrol combined treatment. Int. J. Mol. Sci. 24 (12), 10094. doi:10.3390/ijms241210094
Gomez-Virgilio, L., Silva-Lucero, M. D., Flores-Morelos, D. S., Gallardo-Nieto, J., Lopez-Toledo, G., Abarca-Fernandez, A. M., et al. (2022). Autophagy: a key regulator of homeostasis and disease: an overview of molecular mechanisms and modulators. Cells 11 (15), 2262. doi:10.3390/cells11152262
Gong, L., Huang, D., Shi, Y., Liang, Z., and Bu, H. (2023). Regulated cell death in cancer: from pathogenesis to treatment. Chin. Med. J. Engl. 136 (6), 653–665. doi:10.1097/CM9.0000000000002239
Gong, Y., Fan, Z., Luo, G., Yang, C., Huang, Q., Fan, K., et al. (2019). The role of necroptosis in cancer biology and therapy. Mol. Cancer 18 (1), 100. doi:10.1186/s12943-019-1029-8
Gordy, C., and He, Y. W. (2012). The crosstalk between autophagy and apoptosis: where does this lead? Protein Cell 3 (1), 17–27. doi:10.1007/s13238-011-1127-x
Goy, A., Hernandez-Ilzaliturri, F. J., Kahl, B., Ford, P., Protomastro, E., and Berger, M. (2014). A phase I/II study of the pan Bcl-2 inhibitor obatoclax mesylate plus bortezomib for relapsed or refractory mantle cell lymphoma. Leuk. Lymphoma 55 (12), 2761–2768. doi:10.3109/10428194.2014.907891
Greco, F. A., Bonomi, P., Crawford, J., Kelly, K., Oh, Y., Halpern, W., et al. (2008). Phase 2 study of mapatumumab, a fully human agonistic monoclonal antibody which targets and activates the TRAIL receptor-1, in patients with advanced non-small cell lung cancer. Lung Cancer 61 (1), 82–90. doi:10.1016/j.lungcan.2007.12.011
Gu, L., Zhang, H., Liu, T., Draganov, A., Yi, S., Wang, B., et al. (2018). Inhibition of MDM2 by a rhein-derived compound AQ-101 suppresses cancer development in SCID mice. Mol. Cancer Ther. 17 (2), 497–507. doi:10.1158/1535-7163.MCT-17-0566
Guo, J., Xu, B., Han, Q., Zhou, H., Xia, Y., Gong, C., et al. (2018). Ferroptosis: a novel anti-tumor action for cisplatin. Cancer Res. Treat. 50 (2), 445–460. doi:10.4143/crt.2016.572
Hadian, K., and Stockwell, B. R. (2023). The therapeutic potential of targeting regulated non-apoptotic cell death. Nat. Rev. Drug Discov. 22 (9), 723–742. doi:10.1038/s41573-023-00749-8
Halliwell, B., and Chirico, S. (1993). Lipid peroxidation: its mechanism, measurement, and significance. Am. J. Clin. Nutr. 57 (5 Suppl. l), 715S–725S. doi:10.1093/ajcn/57.5.715S
Han, Q., Ma, Y., Wang, H., Dai, Y., Chen, C., Liu, Y., et al. (2018). Resibufogenin suppresses colorectal cancer growth and metastasis through RIP3-mediated necroptosis. J. Transl. Med. 16 (1), 201. doi:10.1186/s12967-018-1580-x
Han, W., Li, L., Qiu, S., Lu, Q., Pan, Q., Gu, Y., et al. (2007). Shikonin circumvents cancer drug resistance by induction of a necroptotic death. Mol. Cancer Ther. 6 (5), 1641–1649. doi:10.1158/1535-7163.MCT-06-0511
Hangauer, M. J., Viswanathan, V. S., Ryan, M. J., Bole, D., Eaton, J. K., Matov, A., et al. (2017). Drug-tolerant persister cancer cells are vulnerable to GPX4 inhibition. Nature 551 (7679), 247–250. doi:10.1038/nature24297
Harraz, M. M., Dawson, T. M., and Dawson, V. L. (2008). Advances in neuronal cell death 2007. Stroke 39 (2), 286–288. doi:10.1161/STROKEAHA.107.511857
Harshman, L. C., Kroeger, N., Rha, S. Y., Donskov, F., Wood, L., Tantravahi, S. K., et al. (2014). First-line Mammalian target of rapamycin inhibition in metastatic renal cell carcinoma: an analysis of practice patterns from the International Metastatic Renal Cell Carcinoma Database Consortium. Clin. Genitourin. Cancer 12 (5), 335–340. doi:10.1016/j.clgc.2014.03.003
He, S., Wang, L., Miao, L., Wang, T., Du, F., Zhao, L., et al. (2009). Receptor interacting protein kinase-3 determines cellular necrotic response to TNF-alpha. Cell 137 (6), 1100–1111. doi:10.1016/j.cell.2009.05.021
Heiss, B. L., Chang, E., Gao, X., Truong, T., Brave, M. H., Bloomquist, E., et al. (2024). US food and drug administration approval summary: talazoparib in combination with enzalutamide for treatment of patients with homologous recombination repair gene-mutated metastatic castration-resistant prostate cancer. J. Clin. Oncol. 42 (15), 1851–1860. doi:10.1200/JCO.23.02182
Herbst, R. S., Kurzrock, R., Hong, D. S., Valdivieso, M., Hsu, C. P., Goyal, L., et al. (2010). A first-in-human study of conatumumab in adult patients with advanced solid tumors. Clin. Cancer Res. 16 (23), 5883–5891. doi:10.1158/1078-0432.CCR-10-0631
Hergueta-Redondo, M., Sarrio, D., Molina-Crespo, A., Vicario, R., Bernado-Morales, C., Martinez, L., et al. (2016). Gasdermin B expression predicts poor clinical outcome in HER2-positive breast cancer. Oncotarget 7 (35), 56295–56308. doi:10.18632/oncotarget.10787
Hitomi, J., Christofferson, D. E., Ng, A., Yao, J., Degterev, A., Xavier, R. J., et al. (2008). Identification of a molecular signaling network that regulates a cellular necrotic cell death pathway. Cell 135 (7), 1311–1323. doi:10.1016/j.cell.2008.10.044
Hotte, S. J., Hirte, H. W., Chen, E. X., Siu, L. L., Le, L. H., Corey, A., et al. (2008). A phase 1 study of mapatumumab (fully human monoclonal antibody to TRAIL-R1) in patients with advanced solid malignancies. Clin. Cancer Res. 14 (11), 3450–3455. doi:10.1158/1078-0432.CCR-07-1416
Hu, Z., Cai, M., Zhang, Y., Tao, L., and Guo, R. (2020). miR-29c-3p inhibits autophagy and cisplatin resistance in ovarian cancer by regulating FOXP1/ATG14 pathway. Cell Cycle 19 (2), 193–206. doi:10.1080/15384101.2019.1704537
Hua, L., Zhu, G., and Wei, J. (2018). MicroRNA-1 overexpression increases chemosensitivity of non-small cell lung cancer cells by inhibiting autophagy related 3-mediated autophagy. Cell Biol. Int. 42 (9), 1240–1249. doi:10.1002/cbin.10995
Hua, Y., Zheng, Y., Yao, Y., Jia, R., Ge, S., and Zhuang, A. (2023). Metformin and cancer hallmarks: shedding new lights on therapeutic repurposing. J. Transl. Med. 21 (1), 403. doi:10.1186/s12967-023-04263-8
Huang, C. Y., and Yu, L. C. (2015). Pathophysiological mechanisms of death resistance in colorectal carcinoma. World J. Gastroenterol. 21 (41), 11777–11792. doi:10.3748/wjg.v21.i41.11777
Huang, K. J., Wei, Y. H., Chiu, Y. C., Wu, S. R., and Shieh, D. B. (2019a). Assessment of zero-valent iron-based nanotherapeutics for ferroptosis induction and resensitization strategy in cancer cells. Biomater. Sci. 7 (4), 1311–1322. doi:10.1039/c8bm01525b
Huang, P., Chen, G., Jin, W., Mao, K., Wan, H., and He, Y. (2022). Molecular mechanisms of parthanatos and its role in diverse diseases. Int. J. Mol. Sci. 23 (13), 7292. doi:10.3390/ijms23137292
Huang, Y. F., Kuo, M. T., Liu, Y. S., Cheng, Y. M., Wu, P. Y., and Chou, C. Y. (2019b). A dose escalation study of trientine plus carboplatin and pegylated liposomal doxorubicin in women with a first relapse of epithelial ovarian, tubal, and peritoneal cancer within 12 Months after platinum-based chemotherapy. Front. Oncol. 9, 437. doi:10.3389/fonc.2019.00437
Hymowitz, S. G., Christinger, H. W., Fuh, G., Ultsch, M., O'Connell, M., Kelley, R. F., et al. (1999). Triggering cell death: the crystal structure of Apo2L/TRAIL in a complex with death receptor 5. Mol. Cell 4 (4), 563–571. doi:10.1016/s1097-2765(00)80207-5
Ishaq, M., Khan, M. A., Sharma, K., Sharma, G., Dutta, R. K., and Majumdar, S. (2014). Gambogic acid induced oxidative stress dependent caspase activation regulates both apoptosis and autophagy by targeting various key molecules (NF-κB, Beclin-1, p62 and NBR1) in human bladder cancer cells. Biochim. Biophys. Acta 1840 (12), 3374–3384. doi:10.1016/j.bbagen.2014.08.019
Iurlaro, R., and Munoz-Pinedo, C. (2016). Cell death induced by endoplasmic reticulum stress. FEBS J. 283 (14), 2640–2652. doi:10.1111/febs.13598
Jan, R., and Chaudhry, G. E. (2019). Understanding apoptosis and apoptotic pathways targeted cancer therapeutics. Adv. Pharm. Bull. 9 (2), 205–218. doi:10.15171/apb.2019.024
Jeon, S. M., and Shin, E. A. (2018). Exploring vitamin D metabolism and function in cancer. Exp. Mol. Med. 50 (4), 20–14. doi:10.1038/s12276-018-0038-9
Ji, Y., Dai, F., and Zhou, B. (2018). Designing salicylaldehyde isonicotinoyl hydrazones as Cu(II) ionophores with tunable chelation and release of copper for hitting redox Achilles heel of cancer cells. Free Radic. Biol. Med. 129, 215–226. doi:10.1016/j.freeradbiomed.2018.09.017
Jia, Y., Wang, X., Deng, Y., Li, S., Xu, X., Qin, Y., et al. (2023). Pyroptosis provides new strategies for the treatment of cancer. J. Cancer 14 (1), 140–151. doi:10.7150/jca.77965
Jiang, Y., Shen, X., Zhi, F., Wen, Z., Gao, Y., Xu, J., et al. (2023). An overview of arsenic trioxide-involved combined treatment algorithms for leukemia: basic concepts and clinical implications. Cell Death Discov. 9 (1), 266. doi:10.1038/s41420-023-01558-z
Johnson, D. C., Taabazuing, C. Y., Okondo, M. C., Chui, A. J., Rao, S. D., Brown, F. C., et al. (2018). DPP8/DPP9 inhibitor-induced pyroptosis for treatment of acute myeloid leukemia. Nat. Med. 24 (8), 1151–1156. doi:10.1038/s41591-018-0082-y
Joly, F., Fabbro, M., Follana, P., Lequesne, J., Medioni, J., Lesoin, A., et al. (2022). A phase II study of Navitoclax (ABT-263) as single agent in women heavily pretreated for recurrent epithelial ovarian cancer: the MONAVI - GINECO study. Gynecol. Oncol. 165 (1), 30–39. doi:10.1016/j.ygyno.2022.01.021
Kaczmarek, A., Vandenabeele, P., and Krysko, D. V. (2013). Necroptosis: the release of damage-associated molecular patterns and its physiological relevance. Immunity 38 (2), 209–223. doi:10.1016/j.immuni.2013.02.003
Kagan, V. E., Mao, G., Qu, F., Angeli, J. P., Doll, S., Croix, C. S., et al. (2017). Oxidized arachidonic and adrenic PEs navigate cells to ferroptosis. Nat. Chem. Biol. 13 (1), 81–90. doi:10.1038/nchembio.2238
Kaiser, W. J., Sridharan, H., Huang, C., Mandal, P., Upton, J. W., Gough, P. J., et al. (2013). Toll-like receptor 3-mediated necrosis via TRIF, RIP3, and MLKL. J. Biol. Chem. 288 (43), 31268–31279. doi:10.1074/jbc.M113.462341
Kamgar-Dayhoff, P., and Brelidze, T. I. (2021). Multifaceted effect of chlorpromazine in cancer: implications for cancer treatment. Oncotarget 12 (14), 1406–1426. doi:10.18632/oncotarget.28010
Kang, Z., Chen, J. J., Yu, Y., Li, B., Sun, S. Y., Zhang, B., et al. (2011). Drozitumab, a human antibody to death receptor 5, has potent antitumor activity against rhabdomyosarcoma with the expression of caspase-8 predictive of response. Clin. Cancer Res. 17 (10), 3181–3192. doi:10.1158/1078-0432.CCR-10-2874
Karki, R., and Kanneganti, T. D. (2021). The 'cytokine storm': molecular mechanisms and therapeutic prospects. Trends Immunol. 42 (8), 681–705. doi:10.1016/j.it.2021.06.001
Kashbour, M., Alhadeethi, A., Awwad, S., Yassin, M., Amin, A., Abed, M., et al. (2024). The efficacy of Veliparib in combination with chemotherapy in the treatment of lung cancer: systematic review and meta-analysis. Expert Rev. Anticancer Ther., 1–11. doi:10.1080/14737140.2024.2417770
Kasof, G. M., Prosser, J. C., Liu, D., Lorenzi, M. V., and Gomes, B. C. (2000). The RIP-like kinase, RIP3, induces apoptosis and NF-kappaB nuclear translocation and localizes to mitochondria. FEBS Lett. 473 (3), 285–291. doi:10.1016/s0014-5793(00)01473-3
Kasznicki, J., Sliwinska, A., and Drzewoski, J. (2014). Metformin in cancer prevention and therapy. Ann. Transl. Med. 2 (6), 57. doi:10.3978/j.issn.2305-5839.2014.06.01
Kawamoto, Y., Yamai, T., Ikezawa, K., Seiki, Y., Watsuji, K., Hirao, T., et al. (2024). Clinical significance of germline breast cancer susceptibility gene (gBRCA) testing and olaparib as maintenance therapy for patients with pancreatic cancer. BMC Cancer 24 (1), 1000. doi:10.1186/s12885-024-12722-8
Kayagaki, N., Stowe, I. B., Lee, B. L., O'Rourke, K., Anderson, K., Warming, S., et al. (2015). Caspase-11 cleaves gasdermin D for non-canonical inflammasome signalling. Nature 526 (7575), 666–671. doi:10.1038/nature15541
Kayagaki, N., Webster, J. D., and Newton, K. (2024). Control of cell death in health and disease. Annu. Rev. Pathol. 19, 157–180. doi:10.1146/annurev-pathmechdis-051022-014433
Keldsen, N., Havsteen, H., Vergote, I., Bertelsen, K., and Jakobsen, A. (2003). Altretamine (hexamethylmelamine) in the treatment of platinum-resistant ovarian cancer: a phase II study. Gynecol. Oncol. 88 (2), 118–122. doi:10.1016/s0090-8258(02)00103-8
Kelley, K. C., Grossman, K. F., Brittain-Blankenship, M., Thorne, K. M., Akerley, W. L., Terrazas, M. C., et al. (2021). A Phase 1 dose-escalation study of disulfiram and copper gluconate in patients with advanced solid tumors involving the liver using S-glutathionylation as a biomarker. BMC Cancer 21 (1), 510. doi:10.1186/s12885-021-08242-4
Khan, S. U., Fatima, K., Aisha, S., and Malik, F. (2024). Unveiling the mechanisms and challenges of cancer drug resistance. Cell Commun. Signal 22 (1), 109. doi:10.1186/s12964-023-01302-1
Kim, K. W., Hwang, M., Moretti, L., Jaboin, J. J., Cha, Y. I., and Lu, B. (2008). Autophagy upregulation by inhibitors of caspase-3 and mTOR enhances radiotherapy in a mouse model of lung cancer. Autophagy 4 (5), 659–668. doi:10.4161/auto.6058
Kim, S. R., Lewis, J. M., Cyrenne, B. M., Monico, P. F., Mirza, F. N., Carlson, K. R., et al. (2018). BET inhibition in advanced cutaneous T cell lymphoma is synergistically potentiated by BCL2 inhibition or HDAC inhibition. Oncotarget 9 (49), 29193–29207. doi:10.18632/oncotarget.25670
Kindler, H. L., Richards, D. A., Garbo, L. E., Garon, E. B., Stephenson, J. J., Rocha-Lima, C. M., et al. (2012). A randomized, placebo-controlled phase 2 study of ganitumab (AMG 479) or conatumumab (AMG 655) in combination with gemcitabine in patients with metastatic pancreatic cancer. Ann. Oncol. 23 (11), 2834–2842. doi:10.1093/annonc/mds142
Koch, A., Jeiler, B., Roedig, J., van Wijk, S. J. L., Dolgikh, N., and Fulda, S. (2021). Smac mimetics and TRAIL cooperate to induce MLKL-dependent necroptosis in Burkitt's lymphoma cell lines. Neoplasia 23 (5), 539–550. doi:10.1016/j.neo.2021.03.003
Kona, S. V., and Kalivendi, S. V. (2024). The USP10/13 inhibitor, spautin-1, attenuates the progression of glioblastoma by independently regulating RAF-ERK mediated glycolysis and SKP2. Biochim. Biophys. Acta Mol. Basis Dis. 1870 (7), 167291. doi:10.1016/j.bbadis.2024.167291
Koo, G. B., Morgan, M. J., Lee, D. G., Kim, W. J., Yoon, J. H., Koo, J. S., et al. (2015). Methylation-dependent loss of RIP3 expression in cancer represses programmed necrosis in response to chemotherapeutics. Cell Res. 25 (6), 707–725. doi:10.1038/cr.2015.56
Koren, E., and Fuchs, Y. (2021). Modes of regulated cell death in cancer. Cancer Discov. 11 (2), 245–265. doi:10.1158/2159-8290.CD-20-0789
Kou, L., Xie, X., Chen, X., Li, B., Li, J., and Li, Y. (2023). The progress of research on immune checkpoint inhibitor resistance and reversal strategies for hepatocellular carcinoma. Cancer Immunol. Immunother. 72 (12), 3953–3969. doi:10.1007/s00262-023-03568-3
Krutilina, R. I., Hartman, K. L., Oluwalana, D., Playa, H. C., Parke, D. N., Chen, H., et al. (2022). Sabizabulin, a potent orally bioavailable colchicine binding site agent, suppresses HER2+ breast cancer and metastasis. Cancers (Basel) 14 (21), 5336. doi:10.3390/cancers14215336
Kuwahara, Y., Oikawa, T., Ochiai, Y., Roudkenar, M. H., Fukumoto, M., Shimura, T., et al. (2011). Enhancement of autophagy is a potential modality for tumors refractory to radiotherapy. Cell Death Dis. 2 (6), e177. doi:10.1038/cddis.2011.56
Lage, H., Helmbach, H., Grottke, C., Dietel, M., and Schadendorf, D. (2001). DFNA5 (ICERE-1) contributes to acquired etoposide resistance in melanoma cells. FEBS Lett. 494 (1-2), 54–59. doi:10.1016/s0014-5793(01)02304-3
Lamichhane, P. P., and Samir, P. (2023). Cellular stress: modulator of regulated cell death. Biol. (Basel) 12 (9), 1172. doi:10.3390/biology12091172
Lang, X., Green, M. D., Wang, W., Yu, J., Choi, J. E., Jiang, L., et al. (2019). Radiotherapy and immunotherapy promote tumoral lipid oxidation and ferroptosis via synergistic repression of SLC7A11. Cancer Discov. 9 (12), 1673–1685. doi:10.1158/2159-8290.CD-19-0338
Lara, P. N., Villanueva, L., Ibanez, C., Erman, M., Lee, J. L., Heinrich, D., et al. (2024). A randomized, open-label, phase 3 trial of pembrolizumab plus epacadostat versus sunitinib or pazopanib as first-line treatment for metastatic renal cell carcinoma (KEYNOTE-679/ECHO-302). BMC Cancer 23 (Suppl. 1), 1253. doi:10.1186/s12885-023-10971-7
LeBlanc, H. N., and Ashkenazi, A. (2003). Apo2L/TRAIL and its death and decoy receptors. Cell Death Differ. 10 (1), 66–75. doi:10.1038/sj.cdd.4401187
Lee, H. O., Mustafa, A., Hudes, G. R., and Kruger, W. D. (2015). Hydroxychloroquine destabilizes phospho-S6 in human renal carcinoma cells. PLoS One 10 (7), e0131464. doi:10.1371/journal.pone.0131464
Lee, J. M., Brady, M. F., Miller, A., Moore, R. G., MacKay, H., McNally, L., et al. (2024). Cediranib and olaparib combination compared with cediranib or olaparib alone, or chemotherapy in platinum-resistant or primary platinum-refractory ovarian cancer: NRG-GY005. J. Clin. Oncol., JCO2400683. doi:10.1200/JCO.24.00683
Lee, J. M., Kim, H. S., Kim, A., Chang, Y. S., Lee, J. G., Cho, J., et al. (2022). ABT-737, a BH3 mimetic, enhances the therapeutic effects of ionizing radiation in K-ras mutant non-small cell lung cancer preclinical model. Yonsei Med. J. 63 (1), 16–25. doi:10.3349/ymj.2022.63.1.16
Lee, S. Y., Seo, J. H., Kim, S., Hwang, C., Jeong, D. I., Park, J., et al. (2023). Cuproptosis-inducible chemotherapeutic/cascade catalytic reactor system for combating with breast cancer. Small 19 (35), e2301402. doi:10.1002/smll.202301402
Lehmann, S., Bykov, V. J., Ali, D., Andren, O., Cherif, H., Tidefelt, U., et al. (2012). Targeting p53 in vivo: a first-in-human study with p53-targeting compound APR-246 in refractory hematologic malignancies and prostate cancer. J. Clin. Oncol. 30 (29), 3633–3639. doi:10.1200/JCO.2011.40.7783
Lei, G., Zhang, Y., Koppula, P., Liu, X., Zhang, J., Lin, S. H., et al. (2020). The role of ferroptosis in ionizing radiation-induced cell death and tumor suppression. Cell Res. 30 (2), 146–162. doi:10.1038/s41422-019-0263-3
Lei, G., Zhuang, L., and Gan, B. (2024). The roles of ferroptosis in cancer: tumor suppression, tumor microenvironment, and therapeutic interventions. Cancer Cell 42 (4), 513–534. doi:10.1016/j.ccell.2024.03.011
Levantini, E. (2023). Novel therapeutic targets in cancers. Int. J. Mol. Sci. 24 (19), 14660. doi:10.3390/ijms241914660
Levine, A. J. (2022). Targeting the P53 protein for cancer therapies: the translational impact of P53 research. Cancer Res. 82 (3), 362–364. doi:10.1158/0008-5472.CAN-21-2709
Li, C., Zhang, J., Pan, P., Zhang, J., Hou, X., Wang, Y., et al. (2024a). Humanistic health management and cancer: associations of psychology, nutrition, and exercise with cancer progression and pathogenesis. Adv. Sci. (Weinh) 11 (22), e2400665. doi:10.1002/advs.202400665
Li, H., Liu, W., Zhang, X., Wu, F., Sun, D., and Wang, Z. (2021). Ketamine suppresses proliferation and induces ferroptosis and apoptosis of breast cancer cells by targeting KAT5/GPX4 axis. Biochem. Biophys. Res. Commun. 585, 111–116. doi:10.1016/j.bbrc.2021.11.029
Li, J., Chen, S., Liao, Y., Wang, H., Zhou, D., and Zhang, B. (2022a). Arecoline is associated with inhibition of cuproptosis and proliferation of cancer-associated fibroblasts in oral squamous cell carcinoma: a potential mechanism for tumor metastasis. Front. Oncol. 12, 925743. doi:10.3389/fonc.2022.925743
Li, K., Tan, L., Li, Y., Lyu, Y., Zheng, X., Jiang, H., et al. (2022b). Cuproptosis identifies respiratory subtype of renal cancer that confers favorable prognosis. Apoptosis 27 (11-12), 1004–1014. doi:10.1007/s10495-022-01769-2
Li, L. G., Peng, X. C., Yang, Z. Y., Han, N., Gou, C. L., Shi, J., et al. (2024b). Dihydroartemisinin-driven selective anti-lung cancer proliferation by binding to EGFR and inhibition of NRAS signaling pathway-induced DNA damage. Sci. Rep. 14 (1), 11704. doi:10.1038/s41598-024-62126-8
Li, Q., Lv, D., Sun, X., Wang, M., Cai, L., Liu, F., et al. (2024c). Inetetamab combined with sirolimus and chemotherapy for the treatment of HER2-positive metastatic breast cancer patients with abnormal activation of the PI3K/Akt/mTOR pathway after trastuzumab treatment. Cancer Innov. 3 (5), e145. doi:10.1002/cai2.145
Li, R., Ding, C., Zhang, J., Xie, M., Park, D., Ding, Y., et al. (2017). Modulation of Bax and mTOR for cancer therapeutics. Cancer Res. 77 (11), 3001–3012. doi:10.1158/0008-5472.CAN-16-2356
Li, X., Zhou, Y., Li, Y., Yang, L., Ma, Y., Peng, X., et al. (2019). Autophagy: a novel mechanism of chemoresistance in cancers. Biomed. Pharmacother. 119, 109415. doi:10.1016/j.biopha.2019.109415
Liao, M., Qin, R., Huang, W., Zhu, H. P., Peng, F., Han, B., et al. (2022a). Targeting regulated cell death (RCD) with small-molecule compounds in triple-negative breast cancer: a revisited perspective from molecular mechanisms to targeted therapies. J. Hematol. Oncol. 15 (1), 44. doi:10.1186/s13045-022-01260-0
Liao, P., Wang, W., Wang, W., Kryczek, I., Li, X., Bian, Y., et al. (2022b). CD8(+) T cells and fatty acids orchestrate tumor ferroptosis and immunity via ACSL4. Cancer Cell 40 (4), 365–378.e6. doi:10.1016/j.ccell.2022.02.003
Lin, S. Q., Jia, F. J., Zhang, C. Y., Liu, F. Y., Ma, J. H., Han, Z., et al. (2019a). Actinomycin V suppresses human non-small-cell lung carcinoma A549 cells by inducing G2/M phase arrest and apoptosis via the p53-dependent pathway. Mar. Drugs 17 (10), 572. doi:10.3390/md17100572
Lin, X., Jia, Y., Dong, X., Shen, J., Jin, Y., Li, Y., et al. (2019b). Diplatin, a novel and low-toxicity anti-lung cancer platinum complex, activation of cell death in tumors via a ROS/JNK/p53-Dependent pathway, and a low rate of acquired treatment resistance. Front. Pharmacol. 10, 982. doi:10.3389/fphar.2019.00982
Lindemann, A., Patel, A. A., Silver, N. L., Tang, L., Liu, Z., Wang, L., et al. (2019). COTI-2, A novel thiosemicarbazone derivative, exhibits antitumor activity in HNSCC through p53-dependent and -independent mechanisms. Clin. Cancer Res. 25 (18), 5650–5662. doi:10.1158/1078-0432.CCR-19-0096
Liu, B., Zhou, H., Tan, L., Siu, K. T. H., and Guan, X. Y. (2024a). Exploring treatment options in cancer: tumor treatment strategies. Signal Transduct. Target Ther. 9 (1), 175. doi:10.1038/s41392-024-01856-7
Liu, J., Kuang, F., Kroemer, G., Klionsky, D. J., Kang, R., and Tang, D. (2020a). Autophagy-dependent ferroptosis: machinery and regulation. Cell Chem. Biol. 27 (4), 420–435. doi:10.1016/j.chembiol.2020.02.005
Liu, J., Yuan, Y., Cheng, Y., Fu, D., Chen, Z., Wang, Y., et al. (2022). Copper-based metal-organic framework overcomes cancer chemoresistance through systemically disrupting dynamically balanced cellular redox homeostasis. J. Am. Chem. Soc. 144 (11), 4799–4809. doi:10.1021/jacs.1c11856
Liu, S., Yao, S., Yang, H., Liu, S., and Wang, Y. (2023). Autophagy: regulator of cell death. Cell Death Dis. 14 (10), 648. doi:10.1038/s41419-023-06154-8
Liu, T., Sun, X., and Cao, Z. (2019). Shikonin-induced necroptosis in nasopharyngeal carcinoma cells via ROS overproduction and upregulation of RIPK1/RIPK3/MLKL expression. Onco Targets Ther. 12, 2605–2614. doi:10.2147/OTT.S200740
Liu, X., Zhou, M., Mei, L., Ruan, J., Hu, Q., Peng, J., et al. (2016). Key roles of necroptotic factors in promoting tumor growth. Oncotarget 7 (16), 22219–22233. doi:10.18632/oncotarget.7924
Liu, Y., Fang, Y., Chen, X., Wang, Z., Liang, X., Zhang, T., et al. (2020b). Gasdermin E-mediated target cell pyroptosis by CAR T cells triggers cytokine release syndrome. Sci. Immunol. 5 (43), eaax7969. doi:10.1126/sciimmunol.aax7969
Liu, Y., Mondello, P., Erazo, T., Tannan, N. B., Asgari, Z., de Stanchina, E., et al. (2018). NOXA genetic amplification or pharmacologic induction primes lymphoma cells to BCL2 inhibitor-induced cell death. Proc. Natl. Acad. Sci. U. S. A. 115 (47), 12034–12039. doi:10.1073/pnas.1806928115
Liu, Y., Pan, R., Ouyang, Y., Gu, W., Xiao, T., Yang, H., et al. (2024b). Pyroptosis in health and disease: mechanisms, regulation and clinical perspective. Signal Transduct. Target Ther. 9 (1), 245. doi:10.1038/s41392-024-01958-2
Liu, Z. Y., Wu, B., Guo, Y. S., Zhou, Y. H., Fu, Z. G., Xu, B. Q., et al. (2015). Necrostatin-1 reduces intestinal inflammation and colitis-associated tumorigenesis in mice. Am. J. Cancer Res. 5 (10), 3174–3185.
Llovet, J. M., Ricci, S., Mazzaferro, V., Hilgard, P., Gane, E., Blanc, J. F., et al. (2008). Sorafenib in advanced hepatocellular carcinoma. N. Engl. J. Med. 359 (4), 378–390. doi:10.1056/NEJMoa0708857
Loi, S., Karapetis, C. S., McCarthy, N., Oakman, C., Redfern, A., White, M., et al. (2022). Palbociclib plus letrozole as treatment for postmenopausal women with hormone receptor-positive/human epidermal growth factor receptor 2-negative advanced breast cancer for whom letrozole therapy is deemed appropriate: an expanded access study in Australia and India. Asia Pac J. Clin. Oncol. 18 (6), 560–569. doi:10.1111/ajco.13653
Lowe, S. W., and Lin, A. W. (2000). Apoptosis in cancer. Carcinogenesis 21 (3), 485–495. doi:10.1093/carcin/21.3.485
Lu, W., Cheng, F., Yan, W., Li, X., Yao, X., Song, W., et al. (2017). Selective targeting p53(WT) lung cancer cells harboring homozygous p53 Arg72 by an inhibitor of CypA. Oncogene 36 (33), 4719–4731. doi:10.1038/onc.2017.41
Lu, X., Chen, L., Chen, Y., Shao, Q., and Qin, W. (2015). Bafilomycin A1 inhibits the growth and metastatic potential of the BEL-7402 liver cancer and HO-8910 ovarian cancer cell lines and induces alterations in their microRNA expression. Exp. Ther. Med. 10 (5), 1829–1834. doi:10.3892/etm.2015.2758
Lu, Z., Wu, C., Zhu, M., Song, W., Wang, H., Wang, J., et al. (2020). Ophiopogonin D' induces RIPK1-dependent necroptosis in androgen-dependent LNCaP prostate cancer cells. Int. J. Oncol. 56 (2), 439–447. doi:10.3892/ijo.2019.4945
Luo, Y., Bai, X. Y., Zhang, L., Hu, Q. Q., Zhang, N., Cheng, J. Z., et al. (2024). Ferroptosis in cancer therapy: mechanisms, small molecule inducers, and novel approaches. Drug Des. Devel Ther. 18, 2485–2529. doi:10.2147/DDDT.S472178
Ma, D., Lu, B., Feng, C., Wang, C., Wang, Y., Luo, T., et al. (2016a). Deoxypodophyllotoxin triggers parthanatos in glioma cells via induction of excessive ROS. Cancer Lett. 371 (2), 194–204. doi:10.1016/j.canlet.2015.11.044
Ma, J., Li, L., Yue, K., Li, Y., Liu, H., Wang, P. G., et al. (2020). Bromocoumarinplatin, targeting simultaneously mitochondria and nuclei with p53 apoptosis pathway to overcome cisplatin resistance. Bioorg Chem. 99, 103768. doi:10.1016/j.bioorg.2020.103768
Ma, S., Henson, E. S., Chen, Y., and Gibson, S. B. (2016b). Ferroptosis is induced following siramesine and lapatinib treatment of breast cancer cells. Cell Death Dis. 7 (7), e2307. doi:10.1038/cddis.2016.208
Ma, S., Zhu, J., Wang, M., Zhu, J., Wang, W., Xiong, Y., et al. (2022). A cuproptosis-related long non-coding RNA signature to predict the prognosis and immune microenvironment characterization for lung adenocarcinoma. Transl. Lung Cancer Res. 11 (10), 2079–2093. doi:10.21037/tlcr-22-660
Ma, X., Xiao, L., Liu, L., Ye, L., Su, P., Bi, E., et al. (2021). CD36-mediated ferroptosis dampens intratumoral CD8(+) T cell effector function and impairs their antitumor ability. Cell Metab. 33 (5), 1001–1012.e5. doi:10.1016/j.cmet.2021.02.015
Ma, Z. G., Ma, R., Xiao, X. L., Zhang, Y. H., Zhang, X. Z., Hu, N., et al. (2016c). Azo polymeric micelles designed for colon-targeted dimethyl fumarate delivery for colon cancer therapy. Acta Biomater. 44, 323–331. doi:10.1016/j.actbio.2016.08.021
MacDonald, G., Nalvarte, I., Smirnova, T., Vecchi, M., Aceto, N., Dolemeyer, A., et al. (2014). Memo is a copper-dependent redox protein with an essential role in migration and metastasis. Sci. Signal 7 (329), ra56. doi:10.1126/scisignal.2004870
Mahalingam, D., Mita, M., Sarantopoulos, J., Wood, L., Amaravadi, R. K., Davis, L. E., et al. (2014). Combined autophagy and HDAC inhibition: a phase I safety, tolerability, pharmacokinetic, and pharmacodynamic analysis of hydroxychloroquine in combination with the HDAC inhibitor vorinostat in patients with advanced solid tumors. Autophagy 10 (8), 1403–1414. doi:10.4161/auto.29231
Maleki Vareki, S., Salim, K. Y., Danter, W. R., and Koropatnick, J. (2018). Novel anti-cancer drug COTI-2 synergizes with therapeutic agents and does not induce resistance or exhibit cross-resistance in human cancer cell lines. PLoS One 13 (1), e0191766. doi:10.1371/journal.pone.0191766
Man, S. M., Karki, R., and Kanneganti, T. D. (2017). Molecular mechanisms and functions of pyroptosis, inflammatory caspases and inflammasomes in infectious diseases. Immunol. Rev. 277 (1), 61–75. doi:10.1111/imr.12534
Mann, B. S., Johnson, J. R., Cohen, M. H., Justice, R., and Pazdur, R. (2007). FDA approval summary: vorinostat for treatment of advanced primary cutaneous T-cell lymphoma. Oncologist 12 (10), 1247–1252. doi:10.1634/theoncologist.12-10-1247
Markowski, M. C., Tutrone, R., Pieczonka, C., Barnette, K. G., Getzenberg, R. H., Rodriguez, D., et al. (2022). A phase ib/II study of sabizabulin, a novel oral cytoskeleton disruptor, in men with metastatic castration-resistant prostate cancer with progression on an androgen receptor-targeting agent. Clin. Cancer Res. 28 (13), 2789–2795. doi:10.1158/1078-0432.CCR-22-0162
Marsh, J. W., Djoko, K. Y., McEwan, A. G., and Huston, W. M. (2017). Copper(II)-bis(thiosemicarbazonato) complexes as anti-chlamydial agents. Pathog. Dis. 75 (7). doi:10.1093/femspd/ftx084
Maru, D., Hothi, A., Bagariya, C., and Kumar, A. (2022). Targeting ferroptosis pathways: a novel strategy for cancer therapy. Curr. Cancer Drug Targets 22 (3), 234–244. doi:10.2174/1568009622666220211122745
Masaldan, S., Clatworthy, S. A. S., Gamell, C., Meggyesy, P. M., Rigopoulos, A. T., Haupt, S., et al. (2018). Iron accumulation in senescent cells is coupled with impaired ferritinophagy and inhibition of ferroptosis. Redox Biol. 14, 100–115. doi:10.1016/j.redox.2017.08.015
Mason, K. A., Valdecanas, D., Hunter, N. R., and Milas, L. (2008). INO-1001, a novel inhibitor of poly(ADP-ribose) polymerase, enhances tumor response to doxorubicin. Invest. New Drugs 26 (1), 1–5. doi:10.1007/s10637-007-9072-5
Mateo, J., Moreno, V., Gupta, A., Kaye, S. B., Dean, E., Middleton, M. R., et al. (2016). An adaptive study to determine the optimal dose of the tablet formulation of the PARP inhibitor olaparib. Target Oncol. 11 (3), 401–415. doi:10.1007/s11523-016-0435-8
Matteoni, S., Matarrese, P., Ascione, B., Buccarelli, M., Ricci-Vitiani, L., Pallini, R., et al. (2021). Anticancer properties of the antipsychotic drug chlorpromazine and its synergism with temozolomide in restraining human glioblastoma proliferation in vitro. Front. Oncol. 11, 635472. doi:10.3389/fonc.2021.635472
McIlwain, D. R., Berger, T., and Mak, T. W. (2015). Caspase functions in cell death and disease. Cold Spring Harb. Perspect. Biol. 7 (4), a026716. doi:10.1101/cshperspect.a026716
Meier, P., Legrand, A. J., Adam, D., and Silke, J. (2024). Immunogenic cell death in cancer: targeting necroptosis to induce antitumour immunity. Nat. Rev. Cancer 24 (5), 299–315. doi:10.1038/s41568-024-00674-x
Mele, L., Del Vecchio, V., Liccardo, D., Prisco, C., Schwerdtfeger, M., Robinson, N., et al. (2020). The role of autophagy in resistance to targeted therapies. Cancer Treat. Rev. 88, 102043. doi:10.1016/j.ctrv.2020.102043
Meng, M. B., Wang, H. H., Cui, Y. L., Wu, Z. Q., Shi, Y. Y., Zaorsky, N. G., et al. (2016). Necroptosis in tumorigenesis, activation of anti-tumor immunity, and cancer therapy. Oncotarget 7 (35), 57391–57413. doi:10.18632/oncotarget.10548
Michie, J., Kearney, C. J., Hawkins, E. D., Silke, J., and Oliaro, J. (2020). The immuno-modulatory effects of inhibitor of apoptosis protein antagonists in cancer immunotherapy. Cells 9 (1), 207. doi:10.3390/cells9010207
Mishra, R., Zokaei Nikoo, M., Veeraballi, S., and Singh, A. (2023). Venetoclax and hypomethylating agent combination in myeloid malignancies: mechanisms of synergy and challenges of resistance. Int. J. Mol. Sci. 25 (1), 484. doi:10.3390/ijms25010484
Mizuno, M., Ito, K., Nakai, H., Kato, H., Kamiura, S., Ushijima, K., et al. (2023). Veliparib with frontline chemotherapy and as maintenance in Japanese women with ovarian cancer: a subanalysis of efficacy, safety, and antiemetic use in the phase 3 VELIA trial. Int. J. Clin. Oncol. 28 (1), 163–174. doi:10.1007/s10147-022-02258-x
Mohammad, R. M., Muqbil, I., Lowe, L., Yedjou, C., Hsu, H. Y., Lin, L. T., et al. (2015). Broad targeting of resistance to apoptosis in cancer. Semin. Cancer Biol. 35, S78–S103. doi:10.1016/j.semcancer.2015.03.001
Mohammadinejad, R., Moosavi, M. A., Tavakol, S., Vardar, D. O., Hosseini, A., Rahmati, M., et al. (2019). Necrotic, apoptotic and autophagic cell fates triggered by nanoparticles. Autophagy 15 (1), 4–33. doi:10.1080/15548627.2018.1509171
Molina-Crespo, A., Cadete, A., Sarrio, D., Gamez-Chiachio, M., Martinez, L., Chao, K., et al. (2019). Intracellular delivery of an antibody targeting gasdermin-B reduces HER2 breast cancer aggressiveness. Clin. Cancer Res. 25 (15), 4846–4858. doi:10.1158/1078-0432.CCR-18-2381
Monian, P., and Jiang, X. (2012). Clearing the final hurdles to mitochondrial apoptosis: regulation post cytochrome C release. Exp. Oncol. 34 (3), 185–191.
Monk, B. J., Parkinson, C., Lim, M. C., O'Malley, D. M., Oaknin, A., Wilson, M. K., et al. (2022). A randomized, phase III trial to evaluate rucaparib monotherapy as maintenance treatment in patients with newly diagnosed ovarian cancer (ATHENA-MONO/GOG-3020/ENGOT-ov45). J. Clin. Oncol. 40 (34), 3952–3964. doi:10.1200/JCO.22.01003
Morana, O., Wood, W., and Gregory, C. D. (2022). The apoptosis paradox in cancer. Int. J. Mol. Sci. 23 (3), 1328. doi:10.3390/ijms23031328
Morgan, M. J., and Kim, Y. S. (2022). Roles of RIPK3 in necroptosis, cell signaling, and disease. Exp. Mol. Med. 54 (10), 1695–1704. doi:10.1038/s12276-022-00868-z
Motzer, R. J., Escudier, B., Oudard, S., Hutson, T. E., Porta, C., Bracarda, S., et al. (2008). Efficacy of everolimus in advanced renal cell carcinoma: a double-blind, randomised, placebo-controlled phase III trial. Lancet 372 (9637), 449–456. doi:10.1016/S0140-6736(08)61039-9
Murphy, J. M. (2020). The killer pseudokinase mixed lineage kinase domain-like protein (MLKL). Cold Spring Harb. Perspect. Biol. 12 (8), a036376. doi:10.1101/cshperspect.a036376
Nagai, M., Vo, N. H., Shin Ogawa, L., Chimmanamada, D., Inoue, T., Chu, J., et al. (2012). The oncology drug elesclomol selectively transports copper to the mitochondria to induce oxidative stress in cancer cells. Free Radic. Biol. Med. 52 (10), 2142–2150. doi:10.1016/j.freeradbiomed.2012.03.017
Nagourney, A. J., Gipoor, J. B., Evans, S. S., D'Amora, P., Duesberg, M. S., Bernard, P. J., et al. (2023). Therapeutic targeting of P53: a comparative analysis of APR-246 and COTI-2 in human tumor primary culture 3-D explants. Genes (Basel) 14 (3), 747. doi:10.3390/genes14030747
Najafov, A., Chen, H., and Yuan, J. (2017). Necroptosis and cancer. Trends Cancer 3 (4), 294–301. doi:10.1016/j.trecan.2017.03.002
Narang, A., Hage Chehade, C., Ozay, Z. I., Nordblad, B., Swami, U., and Agarwal, N. (2024). Talazoparib for the treatment of prostate cancer. Expert Opin. Pharmacother. 25 (13), 1717–1727. doi:10.1080/14656566.2024.2397002
Nawrocki, S. T., Han, Y., Visconte, V., Przychodzen, B., Espitia, C. M., Phillips, J., et al. (2019). The novel autophagy inhibitor ROC-325 augments the antileukemic activity of azacitidine. Leukemia 33 (12), 2971–2974. doi:10.1038/s41375-019-0529-2
Nawrocki, S. T., Wang, W., and Carew, J. S. (2020). Autophagy: new insights into its roles in cancer progression and drug resistance. Cancers (Basel) 12 (10), 3005. doi:10.3390/cancers12103005
Negroni, A., Colantoni, E., Cucchiara, S., and Stronati, L. (2020). Necroptosis in intestinal inflammation and cancer: new concepts and therapeutic perspectives. Biomolecules 10 (10), 1431. doi:10.3390/biom10101431
Newton, K., Dugger, D. L., Wickliffe, K. E., Kapoor, N., de Almagro, M. C., Vucic, D., et al. (2014). Activity of protein kinase RIPK3 determines whether cells die by necroptosis or apoptosis. Science 343 (6177), 1357–1360. doi:10.1126/science.1249361
Newton, K., Strasser, A., Kayagaki, N., and Dixit, V. M. (2024). Cell death. Cell 187 (2), 235–256. doi:10.1016/j.cell.2023.11.044
Nie, Z., Chen, M., Gao, Y., Huang, D., Cao, H., Peng, Y., et al. (2022). Ferroptosis and tumor drug resistance: current status and major challenges. Front. Pharmacol. 13, 879317. doi:10.3389/fphar.2022.879317
Nishikawa, T., Matsumoto, K., Tamura, K., Yoshida, H., Imai, Y., Miyasaka, A., et al. (2017). Phase 1 dose-escalation study of single-agent veliparib in Japanese patients with advanced solid tumors. Cancer Sci. 108 (9), 1834–1842. doi:10.1111/cas.13307
Nor Hisam, N. S., Ugusman, A., Rajab, N. F., Ahmad, M. F., Fenech, M., Liew, S. L., et al. (2021). Combination therapy of navitoclax with chemotherapeutic agents in solid tumors and blood cancer: a review of current evidence. Pharmaceutics 13 (9), 1353. doi:10.3390/pharmaceutics13091353
O'Day, S. J., Eggermont, A. M., Chiarion-Sileni, V., Kefford, R., Grob, J. J., Mortier, L., et al. (2013). Final results of phase III SYMMETRY study: randomized, double-blind trial of elesclomol plus paclitaxel versus paclitaxel alone as treatment for chemotherapy-naive patients with advanced melanoma. J. Clin. Oncol. 31 (9), 1211–1218. doi:10.1200/JCO.2012.44.5585
Ojha, R., Jha, V., and Singh, S. K. (2016). Gemcitabine and mitomycin induced autophagy regulates cancer stem cell pool in urothelial carcinoma cells. Biochim. Biophys. Acta 1863 (2), 347–359. doi:10.1016/j.bbamcr.2015.12.002
Oliveri, V. (2022). Selective targeting of cancer cells by copper ionophores: an overview. Front. Mol. Biosci. 9, 841814. doi:10.3389/fmolb.2022.841814
Oliveri, V., Lanza, V., Milardi, D., Viale, M., Maric, I., Sgarlata, C., et al. (2017). Amino- and chloro-8-hydroxyquinolines and their copper complexes as proteasome inhibitors and antiproliferative agents. Metallomics 9 (10), 1439–1446. doi:10.1039/c7mt00156h
Ozyerli-Goknar, E., and Bagci-Onder, T. (2021). Epigenetic deregulation of apoptosis in cancers. Cancers (Basel) 13 (13), 3210. doi:10.3390/cancers13133210
Paik, P. K., Rudin, C. M., Pietanza, M. C., Brown, A., Rizvi, N. A., Takebe, N., et al. (2011). A phase II study of obatoclax mesylate, a Bcl-2 antagonist, plus topotecan in relapsed small cell lung cancer. Lung Cancer 74 (3), 481–485. doi:10.1016/j.lungcan.2011.05.005
Parikh, S. A., Kantarjian, H., Schimmer, A., Walsh, W., Asatiani, E., El-Shami, K., et al. (2010). Phase II study of obatoclax mesylate (GX15-070), a small-molecule BCL-2 family antagonist, for patients with myelofibrosis. Clin. Lymphoma Myeloma Leuk. 10 (4), 285–289. doi:10.3816/CLML.2010.n.059
Park, E. J., Min, K. J., Lee, T. J., Yoo, Y. H., Kim, Y. S., and Kwon, T. K. (2014). β-Lapachone induces programmed necrosis through the RIP1-PARP-AIF-dependent pathway in human hepatocellular carcinoma SK-Hep1 cells. Cell Death Dis. 5 (5), e1230. doi:10.1038/cddis.2014.202
Park, J. M., Huang, S., Wu, T. T., Foster, N. R., and Sinicrope, F. A. (2013). Prognostic impact of Beclin 1, p62/sequestosome 1 and LC3 protein expression in colon carcinomas from patients receiving 5-fluorouracil as adjuvant chemotherapy. Cancer Biol. Ther. 14 (2), 100–107. doi:10.4161/cbt.22954
Parzych, K. R., and Klionsky, D. J. (2014). An overview of autophagy: morphology, mechanism, and regulation. Antioxid. Redox Signal 20 (3), 460–473. doi:10.1089/ars.2013.5371
Pasparakis, M., and Vandenabeele, P. (2015). Necroptosis and its role in inflammation. Nature 517 (7534), 311–320. doi:10.1038/nature14191
Pasquier, B. (2015). SAR405, a PIK3C3/Vps34 inhibitor that prevents autophagy and synergizes with MTOR inhibition in tumor cells. Autophagy 11 (4), 725–726. doi:10.1080/15548627.2015.1033601
Pazzaglia, S., and Pioli, C. (2019). Multifaceted role of PARP-1 in DNA repair and inflammation: pathological and therapeutic implications in cancer and non-cancer diseases. Cells 9 (1), 41. doi:10.3390/cells9010041
Pellegrini, P., Strambi, A., Zipoli, C., Hagg-Olofsson, M., Buoncervello, M., Linder, S., et al. (2014). Acidic extracellular pH neutralizes the autophagy-inhibiting activity of chloroquine: implications for cancer therapies. Autophagy 10 (4), 562–571. doi:10.4161/auto.27901
Peng, F., Liao, M., Qin, R., Zhu, S., Peng, C., Fu, L., et al. (2022). Regulated cell death (RCD) in cancer: key pathways and targeted therapies. Signal Transduct. Target Ther. 7 (1), 286. doi:10.1038/s41392-022-01110-y
Petsri, K., Chamni, S., Suwanborirux, K., Saito, N., and Chanvorachote, P. (2019). Renieramycin T induces lung cancer cell apoptosis by targeting mcl-1 degradation: a new insight in the mechanism of action. Mar. Drugs 17 (5), 301. doi:10.3390/md17050301
Pfeffer, C. M., and Singh, A. T. K. (2018). Apoptosis: a target for anticancer therapy. Int. J. Mol. Sci. 19 (2), 448. doi:10.3390/ijms19020448
Piccolo, M., Ferraro, M. G., Iazzetti, F., Santamaria, R., and Irace, C. (2024). Insight into iron, oxidative stress and ferroptosis: therapy targets for approaching anticancer strategies. Cancers (Basel) 16 (6), 1220. doi:10.3390/cancers16061220
Piha-Paul, S. A., Tseng, C., Leung, C. H., Yuan, Y., Karp, D. D., Subbiah, V., et al. (2024). Phase II study of talazoparib in advanced cancers with BRCA1/2, DNA repair, and PTEN alterations. NPJ Precis. Oncol. 8 (1), 166. doi:10.1038/s41698-024-00634-6
Pizato, N., Luzete, B. C., Kiffer, L., Correa, L. H., de Oliveira Santos, I., Assumpcao, J. A. F., et al. (2018). Omega-3 docosahexaenoic acid induces pyroptosis cell death in triple-negative breast cancer cells. Sci. Rep. 8 (1), 1952. doi:10.1038/s41598-018-20422-0
Pu, F., Chen, F., Zhang, Z., Shi, D., Zhong, B., Lv, X., et al. (2022). Ferroptosis as a novel form of regulated cell death: implications in the pathogenesis, oncometabolism and treatment of human cancer. Genes Dis. 9 (2), 347–357. doi:10.1016/j.gendis.2020.11.019
Pukac, L., Kanakaraj, P., Humphreys, R., Alderson, R., Bloom, M., Sung, C., et al. (2005). HGS-ETR1, a fully human TRAIL-receptor 1 monoclonal antibody, induces cell death in multiple tumour types in vitro and in vivo. Br. J. Cancer 92 (8), 1430–1441. doi:10.1038/sj.bjc.6602487
Qi, D., and Peng, M. (2023). Ferroptosis-mediated immune responses in cancer. Front. Immunol. 14, 1188365. doi:10.3389/fimmu.2023.1188365
Qin, K., Zhang, F., Wang, H., Wang, N., Qiu, H., Jia, X., et al. (2023). circRNA circSnx12 confers Cisplatin chemoresistance to ovarian cancer by inhibiting ferroptosis through a miR-194-5p/SLC7A11 axis. BMB Rep. 56 (2), 184–189. doi:10.5483/BMBRep.2022-0175
Rangwala, R., Chang, Y. C., Hu, J., Algazy, K. M., Evans, T. L., Fecher, L. A., et al. (2014a). Combined MTOR and autophagy inhibition: phase I trial of hydroxychloroquine and temsirolimus in patients with advanced solid tumors and melanoma. Autophagy 10 (8), 1391–1402. doi:10.4161/auto.29119
Rangwala, R., Leone, R., Chang, Y. C., Fecher, L. A., Schuchter, L. M., Kramer, A., et al. (2014b). Phase I trial of hydroxychloroquine with dose-intense temozolomide in patients with advanced solid tumors and melanoma. Autophagy 10 (8), 1369–1379. doi:10.4161/auto.29118
Rao, Z., Zhu, Y., Yang, P., Chen, Z., Xia, Y., Qiao, C., et al. (2022). Pyroptosis in inflammatory diseases and cancer. Theranostics 12 (9), 4310–4329. doi:10.7150/thno.71086
Rashmi, K. C., Harsha Raj, M., Paul, M., Girish, K. S., Salimath, B. P., and Aparna, H. S. (2019). A new pyrrole based small molecule from Tinospora cordifolia induces apoptosis in MDA-MB-231 breast cancer cells via ROS mediated mitochondrial damage and restoration of p53 activity. Chem. Biol. Interact. 299, 120–130. doi:10.1016/j.cbi.2018.12.005
Rebecca, V. W., Nicastri, M. C., Fennelly, C., Chude, C. I., Barber-Rotenberg, J. S., Ronghe, A., et al. (2019). PPT1 promotes tumor growth and is the molecular target of chloroquine derivatives in cancer. Cancer Discov. 9 (2), 220–229. doi:10.1158/2159-8290.CD-18-0706
Rebecca, V. W., Nicastri, M. C., McLaughlin, N., Fennelly, C., McAfee, Q., Ronghe, A., et al. (2017). A unified approach to targeting the lysosome's degradative and growth signaling roles. Cancer Discov. 7 (11), 1266–1283. doi:10.1158/2159-8290.CD-17-0741
Reck, M., Schenker, M., Lee, K. H., Provencio, M., Nishio, M., Lesniewski-Kmak, K., et al. (2019). Nivolumab plus ipilimumab versus chemotherapy as first-line treatment in advanced non-small-cell lung cancer with high tumour mutational burden: patient-reported outcomes results from the randomised, open-label, phase III CheckMate 227 trial. Eur. J. Cancer 116, 137–147. doi:10.1016/j.ejca.2019.05.008
Redman, B. G., Esper, P., Pan, Q., Dunn, R. L., Hussain, H. K., Chenevert, T., et al. (2003). Phase II trial of tetrathiomolybdate in patients with advanced kidney cancer. Clin. Cancer Res. 9 (5), 1666–1672.
Reed, J. C. (2006). Drug insight: cancer therapy strategies based on restoration of endogenous cell death mechanisms. Nat. Clin. Pract. Oncol. 3 (7), 388–398. doi:10.1038/ncponc0538
Reeder, N. L., Kaplan, J., Xu, J., Youngquist, R. S., Wallace, J., Hu, P., et al. (2011). Zinc pyrithione inhibits yeast growth through copper influx and inactivation of iron-sulfur proteins. Antimicrob. Agents Chemother. 55 (12), 5753–5760. doi:10.1128/AAC.00724-11
Rocha Lima, C. M., Bayraktar, S., Flores, A. M., MacIntyre, J., Montero, A., Baranda, J. C., et al. (2012). Phase Ib study of drozitumab combined with first-line mFOLFOX6 plus bevacizumab in patients with metastatic colorectal cancer. Cancer Invest. 30 (10), 727–731. doi:10.3109/07357907.2012.732163
Rodler, E., Sharma, P., Barlow, W. E., Gralow, J. R., Puhalla, S. L., Anders, C. K., et al. (2023). Cisplatin with veliparib or placebo in metastatic triple-negative breast cancer and BRCA mutation-associated breast cancer (S1416): a randomised, double-blind, placebo-controlled, phase 2 trial. Lancet Oncol. 24 (2), 162–174. doi:10.1016/S1470-2045(22)00739-2
Rogers, C., Erkes, D. A., Nardone, A., Aplin, A. E., Fernandes-Alnemri, T., and Alnemri, E. S. (2019). Gasdermin pores permeabilize mitochondria to augment caspase-3 activation during apoptosis and inflammasome activation. Nat. Commun. 10 (1), 1689. doi:10.1038/s41467-019-09397-2
Roh, J. L., Kim, E. H., Jang, H. J., Park, J. Y., and Shin, D. (2016). Induction of ferroptotic cell death for overcoming cisplatin resistance of head and neck cancer. Cancer Lett. 381 (1), 96–103. doi:10.1016/j.canlet.2016.07.035
Rosenfeld, M. R., Ye, X., Supko, J. G., Desideri, S., Grossman, S. A., Brem, S., et al. (2014). A phase I/II trial of hydroxychloroquine in conjunction with radiation therapy and concurrent and adjuvant temozolomide in patients with newly diagnosed glioblastoma multiforme. Autophagy 10 (8), 1359–1368. doi:10.4161/auto.28984
Rudin, C. M., Hann, C. L., Garon, E. B., Ribeiro de Oliveira, M., Bonomi, P. D., Camidge, D. R., et al. (2012). Phase II study of single-agent navitoclax (ABT-263) and biomarker correlates in patients with relapsed small cell lung cancer. Clin. Cancer Res. 18 (11), 3163–3169. doi:10.1158/1078-0432.CCR-11-3090
Ruiz, L. M., Libedinsky, A., and Elorza, A. A. (2021). Role of copper on mitochondrial function and metabolism. Front. Mol. Biosci. 8, 711227. doi:10.3389/fmolb.2021.711227
Russo, A. L., Kwon, H. C., Burgan, W. E., Carter, D., Beam, K., Weizheng, X., et al. (2009). In vitro and in vivo radiosensitization of glioblastoma cells by the poly (ADP-ribose) polymerase inhibitor E7016. Clin. Cancer Res. 15 (2), 607–612. doi:10.1158/1078-0432.CCR-08-2079
Saddoughi, S. A., Gencer, S., Peterson, Y. K., Ward, K. E., Mukhopadhyay, A., Oaks, J., et al. (2013). Sphingosine analogue drug FTY720 targets I2PP2A/SET and mediates lung tumour suppression via activation of PP2A-RIPK1-dependent necroptosis. EMBO Mol. Med. 5 (1), 105–121. doi:10.1002/emmm.201201283
Safi, R., Nelson, E. R., Chitneni, S. K., Franz, K. J., George, D. J., Zalutsky, M. R., et al. (2014). Copper signaling axis as a target for prostate cancer therapeutics. Cancer Res. 74 (20), 5819–5831. doi:10.1158/0008-5472.CAN-13-3527
Saghatelyan, T., Tananyan, A., Janoyan, N., Tadevosyan, A., Petrosyan, H., Hovhannisyan, A., et al. (2020). Efficacy and safety of curcumin in combination with paclitaxel in patients with advanced, metastatic breast cancer: a comparative, randomized, double-blind, placebo-controlled clinical trial. Phytomedicine 70, 153218. doi:10.1016/j.phymed.2020.153218
Saha, S., Panigrahi, D. P., Patil, S., and Bhutia, S. K. (2018). Autophagy in health and disease: a comprehensive review. Biomed. Pharmacother. 104, 485–495. doi:10.1016/j.biopha.2018.05.007
Salazar, R., Garcia-Carbonero, R., Libutti, S. K., Hendifar, A. E., Custodio, A., Guimbaud, R., et al. (2018). Phase II study of BEZ235 versus everolimus in patients with mammalian target of rapamycin inhibitor-naive advanced pancreatic neuroendocrine tumors. Oncologist 23 (7), 766–e90. doi:10.1634/theoncologist.2017-0144
Sandhu, S. K., Schelman, W. R., Wilding, G., Moreno, V., Baird, R. D., Miranda, S., et al. (2013). The poly(ADP-ribose) polymerase inhibitor niraparib (MK4827) in BRCA mutation carriers and patients with sporadic cancer: a phase 1 dose-escalation trial. Lancet Oncol. 14 (9), 882–892. doi:10.1016/S1470-2045(13)70240-7
Sari, A. N., Elwakeel, A., Dhanjal, J. K., Kumar, V., Sundar, D., Kaul, S. C., et al. (2021). Identification and characterization of mortaparib(plus)-A novel triazole derivative that targets mortalin-p53 interaction and inhibits cancer-cell proliferation by wild-type p53-dependent and -independent mechanisms. Cancers (Basel) 13 (4), 835. doi:10.3390/cancers13040835
Sato, H., Hiraki, M., Namba, T., Egawa, N., Baba, K., Tanaka, T., et al. (2018). Andrographolide induces degradation of mutant p53 via activation of Hsp70. Int. J. Oncol. 53 (2), 761–770. doi:10.3892/ijo.2018.4416
Satta, T., and Grant, S. (2020). Enhancing venetoclax activity in hematological malignancies. Expert Opin. Investig. Drugs 29 (7), 697–708. doi:10.1080/13543784.2020.1789588
Sayyid, R. K., Bernardino, R., Chavarriaga, J., Gleave, A., Kumar, R., and Fleshner, N. E. (2024). Rucaparib monotherapy in the heavily pre-treated metastatic castrate-resistant prostate cancer setting: practical considerations and alternate treatment approaches. Transl. Androl. Urol. 13 (5), 884–888. doi:10.21037/tau-23-671
Schroder, M., and Kaufman, R. J. (2005). ER stress and the unfolded protein response. Mutat. Res. 569 (1-2), 29–63. doi:10.1016/j.mrfmmm.2004.06.056
Sciegienka, S. J., Solst, S. R., Falls, K. C., Schoenfeld, J. D., Klinger, A. R., Ross, N. L., et al. (2017). D-penicillamine combined with inhibitors of hydroperoxide metabolism enhances lung and breast cancer cell responses to radiation and carboplatin via H(2)O(2)-mediated oxidative stress. Free Radic. Biol. Med. 108, 354–361. doi:10.1016/j.freeradbiomed.2017.04.001
Secchiero, P., Bosco, R., Celeghini, C., and Zauli, G. (2011). Recent advances in the therapeutic perspectives of Nutlin-3. Curr. Pharm. Des. 17 (6), 569–577. doi:10.2174/138161211795222586
Seehawer, M., Heinzmann, F., D'Artista, L., Harbig, J., Roux, P. F., Hoenicke, L., et al. (2018). Necroptosis microenvironment directs lineage commitment in liver cancer. Nature 562 (7725), 69–75. doi:10.1038/s41586-018-0519-y
Sever, R., and Brugge, J. S. (2015). Signal transduction in cancer. Cold Spring Harb. Perspect. Med. 5 (4), a006098. doi:10.1101/cshperspect.a006098
Shah, M., Green, J., Hudacko, R., and Cohen, A. J. (2024). Clinical response to olaparib in a patient with leptomeningeal carcinomatosis in newly diagnosed breast cancer with germline BRCA2 mutation. JCO Precis. Oncol. 8, e2400063. doi:10.1200/PO.24.00063
Sharifi-Rad, J., Herrera-Bravo, J., Kamiloglu, S., Petroni, K., Mishra, A. P., Monserrat-Mesquida, M., et al. (2022). Recent advances in the therapeutic potential of emodin for human health. Biomed. Pharmacother. 154, 113555. doi:10.1016/j.biopha.2022.113555
Sharma, S., de Vries, E. G., Infante, J. R., Oldenhuis, C. N., Gietema, J. A., Yang, L., et al. (2014). Safety, pharmacokinetics, and pharmacodynamics of the DR5 antibody LBY135 alone and in combination with capecitabine in patients with advanced solid tumors. Invest. New Drugs 32 (1), 135–144. doi:10.1007/s10637-013-9952-9
Shi, J., Zhao, Y., Wang, K., Shi, X., Wang, Y., Huang, H., et al. (2015). Cleavage of GSDMD by inflammatory caspases determines pyroptotic cell death. Nature 526 (7575), 660–665. doi:10.1038/nature15514
Shi, J., Zhao, Y., Wang, Y., Gao, W., Ding, J., Li, P., et al. (2014a). Inflammatory caspases are innate immune receptors for intracellular LPS. Nature 514 (7521), 187–192. doi:10.1038/nature13683
Shi, Y., Zhou, F., Jiang, F., Lu, H., Wang, J., and Cheng, C. (2014b). PARP inhibitor reduces proliferation and increases apoptosis in breast cancer cells. Chin. J. Cancer Res. 26 (2), 142–147. doi:10.3978/j.issn.1000-9604.2014.02.13
Shimada, K., Skouta, R., Kaplan, A., Yang, W. S., Hayano, M., Dixon, S. J., et al. (2016). Global survey of cell death mechanisms reveals metabolic regulation of ferroptosis. Nat. Chem. Biol. 12 (7), 497–503. doi:10.1038/nchembio.2079
Shimada, O., Wu, X., Jin, X., Nouh, M. A., Fiscella, M., Albert, V., et al. (2007). Human agonistic antibody to tumor necrosis factor-related apoptosis-inducing ligand receptor 2 induces cytotoxicity and apoptosis in prostate cancer and bladder cancer cells. Urology 69 (2), 395–401. doi:10.1016/j.urology.2006.12.007
Shimony, S., Stone, R. M., and Stahl, M. (2022). Venetoclax combination therapy in acute myeloid leukemia and myelodysplastic syndromes. Curr. Opin. Hematol. 29 (2), 63–73. doi:10.1097/MOH.0000000000000698
Singh, J., Novik, Y., Stein, S., Volm, M., Meyers, M., Smith, J., et al. (2014). Phase 2 trial of everolimus and carboplatin combination in patients with triple negative metastatic breast cancer. Breast Cancer Res. 16 (2), R32. doi:10.1186/bcr3634
Sinha, B. K., Murphy, C., Brown, S. M., Silver, B. B., Tokar, E. J., and Bortner, C. D. (2024). Mechanisms of cell death induced by erastin in human ovarian tumor cells. Int. J. Mol. Sci. 25 (16), 8666. doi:10.3390/ijms25168666
Slamon, D. J., Dieras, V., Rugo, H. S., Harbeck, N., Im, S. A., Gelmon, K. A., et al. (2024). Overall survival with palbociclib plus letrozole in advanced breast cancer. J. Clin. Oncol. 42 (9), 994–1000. doi:10.1200/JCO.23.00137
Soares, J., Espadinha, M., Raimundo, L., Ramos, H., Gomes, A. S., Gomes, S., et al. (2017). DIMP53-1: a novel small-molecule dual inhibitor of p53-MDM2/X interactions with multifunctional p53-dependent anticancer properties. Mol. Oncol. 11 (6), 612–627. doi:10.1002/1878-0261.12051
Son, Y., An, Y., Jung, J., Shin, S., Park, I., Gwak, J., et al. (2019). Protopine isolated from Nandina domestica induces apoptosis and autophagy in colon cancer cells by stabilizing p53. Phytother. Res. 33 (6), 1689–1696. doi:10.1002/ptr.6357
Song, B., Wang, W., Tang, X., Goh, R. M. W., Thuya, W. L., Ho, P. C. L., et al. (2023). Inhibitory potential of resveratrol in cancer metastasis: from biology to therapy. Cancers (Basel) 15 (10), 2758. doi:10.3390/cancers15102758
Song, M., Xia, W., Tao, Z., Zhu, B., Zhang, W., Liu, C., et al. (2021). Self-assembled polymeric nanocarrier-mediated co-delivery of metformin and doxorubicin for melanoma therapy. Drug Deliv. 28 (1), 594–606. doi:10.1080/10717544.2021.1898703
Sonkusre, P. (2019). Specificity of biogenic selenium nanoparticles for prostate cancer therapy with reduced risk of toxicity: an in vitro and in vivo study. Front. Oncol. 9, 1541. doi:10.3389/fonc.2019.01541
Souers, A. J., Leverson, J. D., Boghaert, E. R., Ackler, S. L., Catron, N. D., Chen, J., et al. (2013). ABT-199, a potent and selective BCL-2 inhibitor, achieves antitumor activity while sparing platelets. Nat. Med. 19 (2), 202–208. doi:10.1038/nm.3048
Spencer, B. G., and Finnie, J. W. (2020). The role of endoplasmic reticulum stress in cell survival and death. J. Comp. Pathol. 181, 86–91. doi:10.1016/j.jcpa.2020.10.006
Springer, C., Humayun, D., and Skouta, R. (2024). Cuproptosis: unraveling the mechanisms of copper-induced cell death and its implication in cancer therapy. Cancers (Basel) 16 (3), 647. doi:10.3390/cancers16030647
Sterling, J., Guttha, S., Song, Y., Song, D., Hadziahmetovic, M., and Dunaief, J. L. (2017). Iron importers Zip8 and Zip14 are expressed in retina and regulated by retinal iron levels. Exp. Eye Res. 155, 15–23. doi:10.1016/j.exer.2016.12.008
Sui, X., Chen, R., Wang, Z., Huang, Z., Kong, N., Zhang, M., et al. (2013). Autophagy and chemotherapy resistance: a promising therapeutic target for cancer treatment. Cell Death Dis. 4 (10), e838. doi:10.1038/cddis.2013.350
Sun, J., Wei, Q., Zhou, Y., Wang, J., Liu, Q., and Xu, H. (2017). A systematic analysis of FDA-approved anticancer drugs. BMC Syst. Biol. 11 (Suppl. 5), 87. doi:10.1186/s12918-017-0464-7
Sun, L., Wang, H., Wang, Z., He, S., Chen, S., Liao, D., et al. (2012). Mixed lineage kinase domain-like protein mediates necrosis signaling downstream of RIP3 kinase. Cell 148 (1-2), 213–227. doi:10.1016/j.cell.2011.11.031
Sun, W., and Li, J. (2024). Efficacy and safety of veliparib in the treatment of advanced/metastatic breast cancer: a meta-analysis of phase II and III randomized controlled trials. J. Chemother. 36 (6), 441–448. doi:10.1080/1120009X.2023.2281760
Sussman, R. T., Ricci, M. S., Hart, L. S., Sun, S. Y., and El-Deiry, W. S. (2007). Chemotherapy-resistant side-population of colon cancer cells has a higher sensitivity to TRAIL than the non-SP, a higher expression of c-Myc and TRAIL-receptor DR4. Cancer Biol. Ther. 6 (9), 1490–1495. doi:10.4161/cbt.6.9.4905
Swanton, C., Bernard, E., Abbosh, C., Andre, F., Auwerx, J., Balmain, A., et al. (2024). Embracing cancer complexity: hallmarks of systemic disease. Cell 187 (7), 1589–1616. doi:10.1016/j.cell.2024.02.009
Tadele, D. S., Robertson, J., Crispin, R., Herrera, M. C., Chlubnova, M., Piechaczyk, L., et al. (2021). A cell competition-based small molecule screen identifies a novel compound that induces dual c-Myc depletion and p53 activation. J. Biol. Chem. 296, 100179. doi:10.1074/jbc.RA120.015285
Tamm, I., Kornblau, S. M., Segall, H., Krajewski, S., Welsh, K., Kitada, S., et al. (2000). Expression and prognostic significance of IAP-family genes in human cancers and myeloid leukemias. Clin. Cancer Res. 6 (5), 1796–1803.
Tan, T., Li, J., Luo, R., Wang, R., Yin, L., Liu, M., et al. (2021). Recent advances in understanding the mechanisms of elemene in reversing drug resistance in tumor cells: a review. Molecules 26 (19), 5792. doi:10.3390/molecules26195792
Tang, D., Kang, R., Berghe, T. V., Vandenabeele, P., and Kroemer, G. (2019). The molecular machinery of regulated cell death. Cell Res. 29 (5), 347–364. doi:10.1038/s41422-019-0164-5
Tang, F., Hu, P., Yang, Z., Xue, C., Gong, J., Sun, S., et al. (2017). SBI0206965, a novel inhibitor of Ulk1, suppresses non-small cell lung cancer cell growth by modulating both autophagy and apoptosis pathways. Oncol. Rep. 37 (6), 3449–3458. doi:10.3892/or.2017.5635
Tang, M., Crown, J., and Duffy, M. J. (2023). Degradation of MYC by the mutant p53 reactivator drug, COTI-2 in breast cancer cells. Invest. New Drugs 41 (4), 541–550. doi:10.1007/s10637-023-01368-1
Tang, R., Xu, J., Zhang, B., Liu, J., Liang, C., Hua, J., et al. (2020). Ferroptosis, necroptosis, and pyroptosis in anticancer immunity. J. Hematol. Oncol. 13 (1), 110. doi:10.1186/s13045-020-00946-7
Taniguchi, K., Yamachika, S., He, F., and Karin, M. (2016). p62/SQSTM1-Dr. Jekyll and Mr. Hyde that prevents oxidative stress but promotes liver cancer. FEBS Lett. 590 (15), 2375–2397. doi:10.1002/1873-3468.12301
Tao, Z., Le Blanc, J. M., Wang, C., Zhan, T., Zhuang, H., Wang, P., et al. (2016). Coadministration of trametinib and palbociclib radiosensitizes KRAS-mutant non-small cell lung cancers in vitro and in vivo. Clin. Cancer Res. 22 (1), 122–133. doi:10.1158/1078-0432.CCR-15-0589
Telli, M. L., Litton, J. K., Beck, J. T., Jones, J. M., Andersen, J., Mina, L. A., et al. (2024). Neoadjuvant talazoparib in patients with germline BRCA1/2 mutation-positive, early-stage triple-negative breast cancer: exploration of tumor BRCA mutational status. Breast Cancer 31 (5), 886–897. doi:10.1007/s12282-024-01603-4
Tong, X., Tang, R., Xiao, M., Xu, J., Wang, W., Zhang, B., et al. (2022). Targeting cell death pathways for cancer therapy: recent developments in necroptosis, pyroptosis, ferroptosis, and cuproptosis research. J. Hematol. Oncol. 15 (1), 174. doi:10.1186/s13045-022-01392-3
Trapani, J. A., and Smyth, M. J. (2002). Functional significance of the perforin/granzyme cell death pathway. Nat. Rev. Immunol. 2 (10), 735–747. doi:10.1038/nri911
Trarbach, T., Moehler, M., Heinemann, V., Kohne, C. H., Przyborek, M., Schulz, C., et al. (2010). Phase II trial of mapatumumab, a fully human agonistic monoclonal antibody that targets and activates the tumour necrosis factor apoptosis-inducing ligand receptor-1 (TRAIL-R1), in patients with refractory colorectal cancer. Br. J. Cancer 102 (3), 506–512. doi:10.1038/sj.bjc.6605507
Tron, A. E., Belmonte, M. A., Adam, A., Aquila, B. M., Boise, L. H., Chiarparin, E., et al. (2018). Discovery of Mcl-1-specific inhibitor AZD5991 and preclinical activity in multiple myeloma and acute myeloid leukemia. Nat. Commun. 9 (1), 5341. doi:10.1038/s41467-018-07551-w
Tsang, T., Gu, X., Davis, C. I., Posimo, J. M., Miller, Z. A., and Brady, D. C. (2022). BRAFV600E-Driven lung adenocarcinoma requires copper to sustain autophagic signaling and processing. Mol. Cancer Res. 20 (7), 1096–1107. doi:10.1158/1541-7786.MCR-21-0250
Tse, C., Shoemaker, A. R., Adickes, J., Anderson, M. G., Chen, J., Jin, S., et al. (2008). ABT-263: a potent and orally bioavailable Bcl-2 family inhibitor. Cancer Res. 68 (9), 3421–3428. doi:10.1158/0008-5472.CAN-07-5836
Tsvetkov, P., Coy, S., Petrova, B., Dreishpoon, M., Verma, A., Abdusamad, M., et al. (2022). Copper induces cell death by targeting lipoylated TCA cycle proteins. Science 375 (6586), 1254–1261. doi:10.1126/science.abf0529
Tsvetkov, P., Detappe, A., Cai, K., Keys, H. R., Brune, Z., Ying, W., et al. (2019). Mitochondrial metabolism promotes adaptation to proteotoxic stress. Nat. Chem. Biol. 15 (7), 681–689. doi:10.1038/s41589-019-0291-9
Tucci, M., Stucci, S., Savonarola, A., Resta, L., Cives, M., Rossi, R., et al. (2014). An imbalance between Beclin-1 and p62 expression promotes the proliferation of myeloma cells through autophagy regulation. Exp. Hematol. 42 (10), 897–908. doi:10.1016/j.exphem.2014.06.005
Tufail, M., Hu, J. J., Liang, J., He, C. Y., Wan, W. D., Huang, Y. Q., et al. (2024). Hallmarks of cancer resistance. iScience 27 (6), 109979. doi:10.1016/j.isci.2024.109979
Tzifi, F., Economopoulou, C., Gourgiotis, D., Ardavanis, A., Papageorgiou, S., and Scorilas, A. (2012). The role of BCL2 family of apoptosis regulator proteins in acute and chronic leukemias. Adv. Hematol. 2012, 524308. doi:10.1155/2012/524308
Vandenabeele, P., Galluzzi, L., Vanden Berghe, T., and Kroemer, G. (2010). Molecular mechanisms of necroptosis: an ordered cellular explosion. Nat. Rev. Mol. Cell Biol. 11 (10), 700–714. doi:10.1038/nrm2970
Van Hoecke, L., Riederer, S., Saelens, X., Sutter, G., and Rojas, J. J. (2020). Recombinant viruses delivering the necroptosis mediator MLKL induce a potent antitumor immunity in mice. Oncoimmunology 9 (1), 1802968. doi:10.1080/2162402X.2020.1802968
Varisli, L., Cen, O., and Vlahopoulos, S. (2020). Dissecting pharmacological effects of chloroquine in cancer treatment: interference with inflammatory signaling pathways. Immunology 159 (3), 257–278. doi:10.1111/imm.13160
Viswanathan, V. S., Ryan, M. J., Dhruv, H. D., Gill, S., Eichhoff, O. M., Seashore-Ludlow, B., et al. (2017). Dependency of a therapy-resistant state of cancer cells on a lipid peroxidase pathway. Nature 547 (7664), 453–457. doi:10.1038/nature23007
Vogl, D. T., Stadtmauer, E. A., Tan, K. S., Heitjan, D. F., Davis, L. E., Pontiggia, L., et al. (2014). Combined autophagy and proteasome inhibition: a phase 1 trial of hydroxychloroquine and bortezomib in patients with relapsed/refractory myeloma. Autophagy 10 (8), 1380–1390. doi:10.4161/auto.29264
von Pawel, J., Harvey, J. H., Spigel, D. R., Dediu, M., Reck, M., Cebotaru, C. L., et al. (2014). Phase II trial of mapatumumab, a fully human agonist monoclonal antibody to tumor necrosis factor-related apoptosis-inducing ligand receptor 1 (TRAIL-R1), in combination with paclitaxel and carboplatin in patients with advanced non-small-cell lung cancer. Clin. Lung Cancer 15 (3), 188–196. doi:10.1016/j.cllc.2013.12.005
Vuorinen, R. L., Paunu, N., Turpeenniemi-Hujanen, T., Reunamo, T., Jekunen, A., Kataja, V., et al. (2019). Sunitinib first-line treatment in metastatic renal cell carcinoma: costs and effects. Anticancer Res. 39 (10), 5559–5564. doi:10.21873/anticanres.13749
Waarts, M. R., Stonestrom, A. J., Park, Y. C., and Levine, R. L. (2022). Targeting mutations in cancer. J. Clin. Invest. 132 (8), e154943. doi:10.1172/JCI154943
Walensky, L. D., Kung, A. L., Escher, I., Malia, T. J., Barbuto, S., Wright, R. D., et al. (2004). Activation of apoptosis in vivo by a hydrocarbon-stapled BH3 helix. Science 305 (5689), 1466–1470. doi:10.1126/science.1099191
Wang, C., and Youle, R. J. (2009). The role of mitochondria in apoptosis. Annu. Rev. Genet. 43, 95–118. doi:10.1146/annurev-genet-102108-134850
Wang, F., Gouttia, O. G., Wang, L., and Peng, A. (2021a). PARP1 upregulation in recurrent oral cancer and treatment resistance. Front. Cell Dev. Biol. 9, 804962. doi:10.3389/fcell.2021.804962
Wang, H., Rong, X., Zhao, G., Zhou, Y., Xiao, Y., Ma, D., et al. (2022a). The microbial metabolite trimethylamine N-oxide promotes antitumor immunity in triple-negative breast cancer. Cell Metab. 34 (4), 581–594.e8. doi:10.1016/j.cmet.2022.02.010
Wang, H., Sun, L., Su, L., Rizo, J., Liu, L., Wang, L. F., et al. (2014). Mixed lineage kinase domain-like protein MLKL causes necrotic membrane disruption upon phosphorylation by RIP3. Mol. Cell 54 (1), 133–146. doi:10.1016/j.molcel.2014.03.003
Wang, K., Zhang, Z., Tsai, H. I., Liu, Y., Gao, J., Wang, M., et al. (2021b). Branched-chain amino acid aminotransferase 2 regulates ferroptotic cell death in cancer cells. Cell Death Differ. 28 (4), 1222–1236. doi:10.1038/s41418-020-00644-4
Wang, L., Li, K., Lin, X., Yao, Z., Wang, S., Xiong, X., et al. (2019a). Metformin induces human esophageal carcinoma cell pyroptosis by targeting the miR-497/PELP1 axis. Cancer Lett. 450, 22–31. doi:10.1016/j.canlet.2019.02.014
Wang, Q., Imamura, R., Motani, K., Kushiyama, H., Nagata, S., and Suda, T. (2013). Pyroptotic cells externalize eat-me and release find-me signals and are efficiently engulfed by macrophages. Int. Immunol. 25 (6), 363–372. doi:10.1093/intimm/dxs161
Wang, Q., Ren, M., Feng, F., Chen, K., and Ju, X. (2018a). Treatment of colon cancer with liver X receptor agonists induces immunogenic cell death. Mol. Carcinog. 57 (7), 903–910. doi:10.1002/mc.22811
Wang, Q., Shao, X., Zhang, Y., Zhu, M., Wang, F. X. C., Mu, J., et al. (2023). Role of tumor microenvironment in cancer progression and therapeutic strategy. Cancer Med. 12 (10), 11149–11165. doi:10.1002/cam4.5698
Wang, Q., Wang, P., Zhang, L., Tessema, M., Bai, L., Xu, X., et al. (2020). Epigenetic regulation of RIP3 suppresses necroptosis and increases resistance to chemotherapy in NonSmall cell lung cancer. Transl. Oncol. 13 (2), 372–382. doi:10.1016/j.tranon.2019.11.011
Wang, Q., Zhou, J., Cheng, A., Liu, Y., Guo, J., Li, X., et al. (2024a). Artesunate-binding FABP5 promotes apoptosis in lung cancer cells via the PPARγ-SCD pathway. Int. Immunopharmacol. 143 (Pt 1), 113381. doi:10.1016/j.intimp.2024.113381
Wang, T., Liu, Y., Li, Q., Luo, Y., Liu, D., and Li, B. (2022b). Cuproptosis-related gene FDX1 expression correlates with the prognosis and tumor immune microenvironment in clear cell renal cell carcinoma. Front. Immunol. 13, 999823. doi:10.3389/fimmu.2022.999823
Wang, W., Green, M., Choi, J. E., Gijon, M., Kennedy, P. D., Johnson, J. K., et al. (2019b). CD8(+) T cells regulate tumour ferroptosis during cancer immunotherapy. Nature 569 (7755), 270–274. doi:10.1038/s41586-019-1170-y
Wang, W., Lu, Z., Wang, M., Liu, Z., Wu, B., Yang, C., et al. (2022c). The cuproptosis-related signature associated with the tumor environment and prognosis of patients with glioma. Front. Immunol. 13, 998236. doi:10.3389/fimmu.2022.998236
Wang, W., Zhang, L., and Sun, Z. (2022d). Eliciting pyroptosis to fuel cancer immunotherapy: mechanisms and strategies. Cancer Biol. Med. 19 (7), 948–964. doi:10.20892/j.issn.2095-3941.2022.0049
Wang, X., Chen, Y., Wang, X., Tian, H., Wang, Y., Jin, J., et al. (2021c). Stem cell factor SOX2 confers ferroptosis resistance in lung cancer via upregulation of SLC7A11. Cancer Res. 81 (20), 5217–5229. doi:10.1158/0008-5472.CAN-21-0567
Wang, X., Xu, S., Zhang, L., Cheng, X., Yu, H., Bao, J., et al. (2021d). Vitamin C induces ferroptosis in anaplastic thyroid cancer cells by ferritinophagy activation. Biochem. Biophys. Res. Commun. 551, 46–53. doi:10.1016/j.bbrc.2021.02.126
Wang, Y., Chen, Y., Zhang, J., Yang, Y., Fleishman, J. S., Wang, Y., et al. (2024b). Cuproptosis: a novel therapeutic target for overcoming cancer drug resistance. Drug Resist Updat 72, 101018. doi:10.1016/j.drup.2023.101018
Wang, Y., Luo, W., and Wang, Y. (2019c). PARP-1 and its associated nucleases in DNA damage response. DNA Repair (Amst) 81, 102651. doi:10.1016/j.dnarep.2019.102651
Wang, Y., Peng, R. Q., Li, D. D., Ding, Y., Wu, X. Q., Zeng, Y. X., et al. (2011). Chloroquine enhances the cytotoxicity of topotecan by inhibiting autophagy in lung cancer cells. Chin. J. Cancer 30 (10), 690–700. doi:10.5732/cjc.011.10056
Wang, Y., Yin, B., Li, D., Wang, G., Han, X., and Sun, X. (2018b). GSDME mediates caspase-3-dependent pyroptosis in gastric cancer. Biochem. Biophys. Res. Commun. 495 (1), 1418–1425. doi:10.1016/j.bbrc.2017.11.156
Wang, Y., Zhang, L., and Zhou, F. (2022e). Cuproptosis: a new form of programmed cell death. Cell Mol. Immunol. 19 (8), 867–868. doi:10.1038/s41423-022-00866-1
Wang, Y. H., and Scadden, D. T. (2015). Harnessing the apoptotic programs in cancer stem-like cells. EMBO Rep. 16 (9), 1084–1098. doi:10.15252/embr.201439675
Wang, Z., Yao, J., Dong, T., and Niu, X. (2022f). Definition of a novel cuproptosis-relevant lncRNA signature for uncovering distinct survival, genomic alterations, and treatment implications in lung adenocarcinoma. J. Immunol. Res. 2022, 2756611. doi:10.1155/2022/2756611
Wei, A. H., Roberts, A. W., Spencer, A., Rosenberg, A. S., Siegel, D., Walter, R. B., et al. (2020). Targeting MCL-1 in hematologic malignancies: rationale and progress. Blood Rev. 44, 100672. doi:10.1016/j.blre.2020.100672
Wei, X., Xie, F., Zhou, X., Wu, Y., Yan, H., Liu, T., et al. (2022). Role of pyroptosis in inflammation and cancer. Cell Mol. Immunol. 19 (9), 971–992. doi:10.1038/s41423-022-00905-x
Wertz, I. E., Kusam, S., Lam, C., Okamoto, T., Sandoval, W., Anderson, D. J., et al. (2011). Sensitivity to antitubulin chemotherapeutics is regulated by MCL1 and FBW7. Nature 471 (7336), 110–114. doi:10.1038/nature09779
Widden, H., and Placzek, W. J. (2021). The multiple mechanisms of MCL1 in the regulation of cell fate. Commun. Biol. 4 (1), 1029. doi:10.1038/s42003-021-02564-6
Wise-Draper, T. M., Moorthy, G., Salkeni, M. A., Karim, N. A., Thomas, H. E., Mercer, C. A., et al. (2017). A phase ib study of the dual PI3K/mTOR inhibitor dactolisib (BEZ235) combined with everolimus in patients with advanced solid malignancies. Target Oncol. 12 (3), 323–332. doi:10.1007/s11523-017-0482-9
Wolpin, B. M., Rubinson, D. A., Wang, X., Chan, J. A., Cleary, J. M., Enzinger, P. C., et al. (2014). Phase II and pharmacodynamic study of autophagy inhibition using hydroxychloroquine in patients with metastatic pancreatic adenocarcinoma. Oncologist 19 (6), 637–638. doi:10.1634/theoncologist.2014-0086
Workenhe, S. T., Nguyen, A., Bakhshinyan, D., Wei, J., Hare, D. N., MacNeill, K. L., et al. (2020). De novo necroptosis creates an inflammatory environment mediating tumor susceptibility to immune checkpoint inhibitors. Commun. Biol. 3 (1), 645. doi:10.1038/s42003-020-01362-w
Wu, F., Huang, F., Jiang, N., Su, J., Yao, S., Liang, B., et al. (2024a). Identification of ferroptosis related genes and pathways in prostate cancer cells under erastin exposure. BMC Urol. 24 (1), 78. doi:10.1186/s12894-024-01472-1
Wu, H., Lin, J., Liu, P., Huang, Z., Zhao, P., Jin, H., et al. (2015). Is the autophagy a friend or foe in the silver nanoparticles associated radiotherapy for glioma? Biomaterials 62, 47–57. doi:10.1016/j.biomaterials.2015.05.033
Wu, M., Wang, Y., Yang, D., Gong, Y., Rao, F., Liu, R., et al. (2019a). A PLK1 kinase inhibitor enhances the chemosensitivity of cisplatin by inducing pyroptosis in oesophageal squamous cell carcinoma. EBioMedicine 41, 244–255. doi:10.1016/j.ebiom.2019.02.012
Wu, W., Liu, P., and Li, J. (2012). Necroptosis: an emerging form of programmed cell death. Crit. Rev. Oncol. Hematol. 82 (3), 249–258. doi:10.1016/j.critrevonc.2011.08.004
Wu, X., Zhu, J., Yin, R., Yang, J., Liu, J., Wang, J., et al. (2024b). Niraparib maintenance therapy using an individualised starting dose in patients with platinum-sensitive recurrent ovarian cancer (NORA): final overall survival analysis of a phase 3 randomised, placebo-controlled trial. EClinicalMedicine 72, 102629. doi:10.1016/j.eclinm.2024.102629
Wu, Y., Dong, G., and Sheng, C. (2020). Targeting necroptosis in anticancer therapy: mechanisms and modulators. Acta Pharm. Sin. B 10 (9), 1601–1618. doi:10.1016/j.apsb.2020.01.007
Wu, Z., Zhang, W., and Kang, Y. J. (2019b). Copper affects the binding of HIF-1α to the critical motifs of its target genes. Metallomics 11 (2), 429–438. doi:10.1039/c8mt00280k
Xie, J., Yang, Y., Gao, Y., and He, J. (2023a). Cuproptosis: mechanisms and links with cancers. Mol. Cancer 22 (1), 46. doi:10.1186/s12943-023-01732-y
Xie, Y., Kang, R., Klionsky, D. J., and Tang, D. (2023b). GPX4 in cell death, autophagy, and disease. Autophagy 19 (10), 2621–2638. doi:10.1080/15548627.2023.2218764
Xie, Y., Zhu, S., Song, X., Sun, X., Fan, Y., Liu, J., et al. (2017). The tumor suppressor p53 limits ferroptosis by blocking DPP4 activity. Cell Rep. 20 (7), 1692–1704. doi:10.1016/j.celrep.2017.07.055
Xu, Y., Liu, S. Y., Zeng, L., Ma, H., Zhang, Y., Yang, H., et al. (2022). An enzyme-engineered nonporous copper(I) coordination polymer nanoplatform for cuproptosis-based synergistic cancer therapy. Adv. Mater 34 (43), e2204733. doi:10.1002/adma.202204733
Xuzhang, W., Lu, T., Jin, W., Yu, Y., Li, Z., Shen, L., et al. (2024). Cisplatin-induced pyroptosis enhances the efficacy of PD-L1 inhibitor in small-cell lung cancer via GSDME/IL12/CD4Tem Axis. Int. J. Biol. Sci. 20 (2), 537–553. doi:10.7150/ijbs.89080
Yan, H., Luo, B., Wu, X., Guan, F., Yu, X., Zhao, L., et al. (2021). Cisplatin induces pyroptosis via activation of MEG3/NLRP3/caspase-1/GSDMD pathway in triple-negative breast cancer. Int. J. Biol. Sci. 17 (10), 2606–2621. doi:10.7150/ijbs.60292
Yan, J., Wan, P., Choksi, S., and Liu, Z. G. (2022). Necroptosis and tumor progression. Trends Cancer 8 (1), 21–27. doi:10.1016/j.trecan.2021.09.003
Yang, B., Jiang, J., Wu, H., and Lu, Q. (2024). Topical BCl-2 inhibitor (ABT-737) attenuates skin photoaging in mice. Exp. Dermatol 33 (3), e15051. doi:10.1111/exd.15051
Yang, D., Shu, T., Zhao, H., Sun, Y., Xu, W., and Tu, G. (2020). Knockdown of macrophage migration inhibitory factor (MIF), a novel target to protect neurons from parthanatos induced by simulated post-spinal cord injury oxidative stress. Biochem. Biophys. Res. Commun. 523 (3), 719–725. doi:10.1016/j.bbrc.2019.12.115
Yang, L., Kumar, B., Shen, C., Zhao, S., Blakaj, D., Li, T., et al. (2019). LCL161, a SMAC-mimetic, preferentially radiosensitizes human papillomavirus-negative head and neck squamous cell carcinoma. Mol. Cancer Ther. 18 (6), 1025–1035. doi:10.1158/1535-7163.MCT-18-1157
Yang, M., Wu, X., Hu, J., Wang, Y., Wang, Y., Zhang, L., et al. (2022). COMMD10 inhibits HIF1α/CP loop to enhance ferroptosis and radiosensitivity by disrupting Cu-Fe balance in hepatocellular carcinoma. J. Hepatol. 76 (5), 1138–1150. doi:10.1016/j.jhep.2022.01.009
Yang, W. S., SriRamaratnam, R., Welsch, M. E., Shimada, K., Skouta, R., Viswanathan, V. S., et al. (2014). Regulation of ferroptotic cancer cell death by GPX4. Cell 156 (1-2), 317–331. doi:10.1016/j.cell.2013.12.010
Yang, Y., Hu, W., Feng, S., Ma, J., and Wu, M. (2005). RIP3 beta and RIP3 gamma, two novel splice variants of receptor-interacting protein 3 (RIP3), downregulate RIP3-induced apoptosis. Biochem. Biophys. Res. Commun. 332 (1), 181–187. doi:10.1016/j.bbrc.2005.04.114
Yao, X., Xie, R., Cao, Y., Tang, J., Men, Y., Peng, H., et al. (2021). Simvastatin induced ferroptosis for triple-negative breast cancer therapy. J. Nanobiotechnology 19 (1), 311. doi:10.1186/s12951-021-01058-1
Ye, K., Chen, Z., and Xu, Y. (2023). The double-edged functions of necroptosis. Cell Death Dis. 14 (2), 163. doi:10.1038/s41419-023-05691-6
Ye, L., Jin, F., Kumar, S. K., and Dai, Y. (2021a). The mechanisms and therapeutic targets of ferroptosis in cancer. Expert Opin. Ther. Targets 25 (11), 965–986. doi:10.1080/14728222.2021.2011206
Ye, L. F., Chaudhary, K. R., Zandkarimi, F., Harken, A. D., Kinslow, C. J., Upadhyayula, P. S., et al. (2020). Radiation-induced lipid peroxidation triggers ferroptosis and synergizes with ferroptosis inducers. ACS Chem. Biol. 15 (2), 469–484. doi:10.1021/acschembio.9b00939
Ye, Z. Q., Chen, H. B., Zhang, T. Y., Chen, Z., Tian, L., and Gu, D. N. (2021b). MicroRNA-7 modulates cellular senescence to relieve gemcitabine resistance by targeting PARP1/NF-κB signaling in pancreatic cancer cells. Oncol. Lett. 21 (2), 139. doi:10.3892/ol.2020.12400
Yoshii, J., Yoshiji, H., Kuriyama, S., Ikenaka, Y., Noguchi, R., Okuda, H., et al. (2001). The copper-chelating agent, trientine, suppresses tumor development and angiogenesis in the murine hepatocellular carcinoma cells. Int. J. Cancer 94 (6), 768–773. doi:10.1002/ijc.1537
Younes, A., Vose, J. M., Zelenetz, A. D., Smith, M. R., Burris, H. A., Ansell, S. M., et al. (2010). A Phase 1b/2 trial of mapatumumab in patients with relapsed/refractory non-Hodgkin's lymphoma. Br. J. Cancer 103 (12), 1783–1787. doi:10.1038/sj.bjc.6605987
Yu, J., Li, S., Qi, J., Chen, Z., Wu, Y., Guo, J., et al. (2019). Cleavage of GSDME by caspase-3 determines lobaplatin-induced pyroptosis in colon cancer cells. Cell Death Dis. 10 (3), 193. doi:10.1038/s41419-019-1441-4
Yu, X., Deng, Q., Li, W., Xiao, L., Luo, X., Liu, X., et al. (2015). Neoalbaconol induces cell death through necroptosis by regulating RIPK-dependent autocrine TNFα and ROS production. Oncotarget 6 (4), 1995–2008. doi:10.18632/oncotarget.3038
Yu, X., and He, S. (2017). GSDME as an executioner of chemotherapy-induced cell death. Sci. China Life Sci. 60 (11), 1291–1294. doi:10.1007/s11427-017-9142-2
Yuan, B., Liao, F., Shi, Z. Z., Ren, Y., Deng, X. L., Yang, T. T., et al. (2020). Dihydroartemisinin inhibits the proliferation, colony formation and induces ferroptosis of lung cancer cells by inhibiting PRIM2/slc7a11 Axis. Onco Targets Ther. 13, 10829–10840. doi:10.2147/OTT.S248492
Yuan, J., Song, J., Chen, C., Lv, X., Bai, J., Yang, J., et al. (2022). Combination of ruxolitinib with ABT-737 exhibits synergistic effects in cells carrying concurrent JAK2(V617F) and ASXL1 mutations. Invest. New Drugs 40 (6), 1194–1205. doi:10.1007/s10637-022-01297-5
Yue, E., Tuguzbaeva, G., Chen, X., Qin, Y., Li, A., Sun, X., et al. (2019). Anthocyanin is involved in the activation of pyroptosis in oral squamous cell carcinoma. Phytomedicine 56, 286–294. doi:10.1016/j.phymed.2018.09.223
Yun, J., Mullarky, E., Lu, C., Bosch, K. N., Kavalier, A., Rivera, K., et al. (2015). Vitamin C selectively kills KRAS and BRAF mutant colorectal cancer cells by targeting GAPDH. Science 350 (6266), 1391–1396. doi:10.1126/science.aaa5004
Zanardi, E., Verzoni, E., Grassi, P., Necchi, A., Giannatempo, P., Raggi, D., et al. (2015). Clinical experience with temsirolimus in the treatment of advanced renal cell carcinoma. Ther. Adv. Urol. 7 (3), 152–161. doi:10.1177/1756287215574457
Zhang, C., Liu, X., Jin, S., Chen, Y., and Guo, R. (2022a). Ferroptosis in cancer therapy: a novel approach to reversing drug resistance. Mol. Cancer 21 (1), 47. doi:10.1186/s12943-022-01530-y
Zhang, C. C., Li, C. G., Wang, Y. F., Xu, L. H., He, X. H., Zeng, Q. Z., et al. (2019a). Chemotherapeutic paclitaxel and cisplatin differentially induce pyroptosis in A549 lung cancer cells via caspase-3/GSDME activation. Apoptosis 24 (3-4), 312–325. doi:10.1007/s10495-019-01515-1
Zhang, D., Cui, P., Dai, Z., Yang, B., Yao, X., Liu, Q., et al. (2019b). Tumor microenvironment responsive FePt/MoS(2) nanocomposites with chemotherapy and photothermal therapy for enhancing cancer immunotherapy. Nanoscale 11 (42), 19912–19922. doi:10.1039/c9nr05684j
Zhang, J., Yu, G., Yang, Y., Wang, Y., Guo, M., Yin, Q., et al. (2022b). A small-molecule inhibitor of MDMX suppresses cervical cancer cells via the inhibition of E6-E6AP-p53 axis. Pharmacol. Res. 177, 106128. doi:10.1016/j.phrs.2022.106128
Zhang, N., Hartig, H., Dzhagalov, I., Draper, D., and He, Y. W. (2005). The role of apoptosis in the development and function of T lymphocytes. Cell Res. 15 (10), 749–769. doi:10.1038/sj.cr.7290345
Zhang, T., Yin, C., Fedorov, A., Qiao, L., Bao, H., Beknazarov, N., et al. (2022c). ADAR1 masks the cancer immunotherapeutic promise of ZBP1-driven necroptosis. Nature 606 (7914), 594–602. doi:10.1038/s41586-022-04753-7
Zhang, X., Walke, G. R., Horvath, I., Kumar, R., Blockhuys, S., Holgersson, S., et al. (2022d). Memo1 binds reduced copper ions, interacts with copper chaperone Atox1, and protects against copper-mediated redox activity in vitro. Proc. Natl. Acad. Sci. U. S. A. 119 (37), e2206905119. doi:10.1073/pnas.2206905119
Zhang, X., Wang, H., Yu, M., Ma, K., and Ning, L. (2022e). Inhibition of autophagy by 3-methyladenine promotes migration and invasion of colon cancer cells through epithelial mesenchymal transformation. Transl. Cancer Res. 11 (8), 2834–2842. doi:10.21037/tcr-22-1736
Zhang, Y., Chen, X., Gueydan, C., and Han, J. (2018). Plasma membrane changes during programmed cell deaths. Cell Res. 28 (1), 9–21. doi:10.1038/cr.2017.133
Zhang, Y., Tan, H., Daniels, J. D., Zandkarimi, F., Liu, H., Brown, L. M., et al. (2019c). Imidazole ketone erastin induces ferroptosis and slows tumor growth in a mouse lymphoma model. Cell Chem. Biol. 26 (5), 623–633. doi:10.1016/j.chembiol.2019.01.008
Zhang, Z., Lin, J., Yang, L., and Li, Y. (2023). Osimertinib inhibits brain metastases and improves long-term survival in a patient with advanced squamous cell lung cancer: a case report and literature review. Front. Oncol. 13, 1188772. doi:10.3389/fonc.2023.1188772
Zhang, Z., Zeng, X., Wu, Y., Liu, Y., Zhang, X., and Song, Z. (2022f). Cuproptosis-related risk score predicts prognosis and characterizes the tumor microenvironment in hepatocellular carcinoma. Front. Immunol. 13, 925618. doi:10.3389/fimmu.2022.925618
Zhao, G., Feng, E., and Liu, Y. (2023). Efficacy and safety of veliparib combined with traditional chemotherapy for treating patients with lung cancer: a comprehensive review and meta-analysis. PeerJ 11, e16402. doi:10.7717/peerj.16402
Zhao, G., Han, X., Zheng, S., Li, Z., Sha, Y., Ni, J., et al. (2016). Curcumin induces autophagy, inhibits proliferation and invasion by downregulating AKT/mTOR signaling pathway in human melanoma cells. Oncol. Rep. 35 (2), 1065–1074. doi:10.3892/or.2015.4413
Zhao, L., Zhou, X., Xie, F., Zhang, L., Yan, H., Huang, J., et al. (2022). Ferroptosis in cancer and cancer immunotherapy. Cancer Commun. (Lond) 42 (2), 88–116. doi:10.1002/cac2.12250
Zhao, W., Jiang, L., Fang, T., Fang, F., Liu, Y., Zhao, Y., et al. (2021a). β-Lapachone selectively kills hepatocellular carcinoma cells by targeting NQO1 to induce extensive DNA damage and PARP1 hyperactivation. Front. Oncol. 11, 747282. doi:10.3389/fonc.2021.747282
Zhao, X., Quan, J., Tan, Y., Liu, Y., Liao, C., Li, Z., et al. (2021b). RIP3 mediates TCN-induced necroptosis through activating mitochondrial metabolism and ROS production in chemotherapy-resistant cancers. Am. J. Cancer Res. 11 (3), 729–745.
Zheng, D., Liwinski, T., and Elinav, E. (2020a). Inflammasome activation and regulation: toward a better understanding of complex mechanisms. Cell Discov. 6, 36. doi:10.1038/s41421-020-0167-x
Zheng, M., Williams, E. P., Malireddi, R. K. S., Karki, R., Banoth, B., Burton, A., et al. (2020b). Impaired NLRP3 inflammasome activation/pyroptosis leads to robust inflammatory cell death via caspase-8/RIPK3 during coronavirus infection. J. Biol. Chem. 295 (41), 14040–14052. doi:10.1074/jbc.RA120.015036
Zheng, Z., Bian, Y., Zhang, Y., Ren, G., and Li, G. (2020c). Metformin activates AMPK/SIRT1/NF-κB pathway and induces mitochondrial dysfunction to drive caspase3/GSDME-mediated cancer cell pyroptosis. Cell Cycle 19 (10), 1089–1104. doi:10.1080/15384101.2020.1743911
Zheng, Z., and Li, G. (2020). Mechanisms and therapeutic regulation of pyroptosis in inflammatory diseases and cancer. Int. J. Mol. Sci. 21 (4), 1456. doi:10.3390/ijms21041456
Zhou, J., Li, G., Han, G., Feng, S., Liu, Y., Chen, J., et al. (2020). Emodin induced necroptosis in the glioma cell line U251 via the TNF-α/RIP1/RIP3 pathway. Invest. New Drugs 38 (1), 50–59. doi:10.1007/s10637-019-00764-w
Zhou, J., Yu, Q., Song, J., Li, S., Li, X. L., Kang, B. K., et al. (2023). Photothermally triggered copper payload release for cuproptosis-promoted cancer synergistic therapy. Angew. Chem. Int. Ed. Engl. 62 (12), e202213922. doi:10.1002/anie.202213922
Zhou, Q., Meng, Y., Li, D., Yao, L., Le, J., Liu, Y., et al. (2024a). Ferroptosis in cancer: from molecular mechanisms to therapeutic strategies. Signal Transduct. Target Ther. 9 (1), 55. doi:10.1038/s41392-024-01769-5
Zhou, Y., Li, J., Xu, X., Zhao, M., Zhang, B., Deng, S., et al. (2019). (64)Cu-based radiopharmaceuticals in molecular imaging. Technol. Cancer Res. Treat. 18, 1533033819830758. doi:10.1177/1533033819830758
Zhou, Y., Liu, L., Tao, S., Yao, Y., Wang, Y., Wei, Q., et al. (2021). Parthanatos and its associated components: promising therapeutic targets for cancer. Pharmacol. Res. 163, 105299. doi:10.1016/j.phrs.2020.105299
Zhou, Y., Manghwar, H., Hu, W., and Liu, F. (2022). Degradation mechanism of autophagy-related proteins and research progress. Int. J. Mol. Sci. 23 (13), 7301. doi:10.3390/ijms23137301
Zhou, Y., Tao, L., Qiu, J., Xu, J., Yang, X., Zhang, Y., et al. (2024b). Tumor biomarkers for diagnosis, prognosis and targeted therapy. Signal Transduct. Target Ther. 9 (1), 132. doi:10.1038/s41392-024-01823-2
Keywords: regulated cell death pathway, cancer therapy, apoptosis, autophagy, necroptosis, ferroptosis, pyroptosis, cuproptosis
Citation: Saxena R, Welsh CM and He Y-W (2024) Targeting regulated cell death pathways in cancers for effective treatment: a comprehensive review. Front. Cell Dev. Biol. 12:1462339. doi: 10.3389/fcell.2024.1462339
Received: 11 July 2024; Accepted: 05 November 2024;
Published: 15 November 2024.
Edited by:
Inna N. Lavrik, University Hospital Magdeburg, GermanyReviewed by:
Shawn B. Bratton, University of Texas MD Anderson Cancer Center, United StatesSuchandrima Saha, Stony Brook Medicine, United States
Copyright © 2024 Saxena, Welsh and He. This is an open-access article distributed under the terms of the Creative Commons Attribution License (CC BY). The use, distribution or reproduction in other forums is permitted, provided the original author(s) and the copyright owner(s) are credited and that the original publication in this journal is cited, in accordance with accepted academic practice. No use, distribution or reproduction is permitted which does not comply with these terms.
*Correspondence: You-Wen He, eW91d2VuLmhlQGR1a2UuZWR1; Ruchi Saxena, c2F4ZW5hLnJ1Y2hpQGhvdG1haWwuY29t