- 1Department of Neurology, West China Hospital, Sichuan University, Chengdu, China
- 2West China Center of Excellence for Pancreatitis, Institute of Integrated Traditional Chinese and Western Medicine, West China Hospital, Sichuan University, Chengdu, China
- 3Regenerative Medicine Research Center, Sichuan University West China Hospital, Chengdu, Sichuan, China
Macroautophagy/autophagy is an intracellular degradation pathway that has an important effect on both healthy and diseased pancreases. It protects the structure and function of the pancreas by maintaining organelle homeostasis and removing damaged organelles. A variety of pancreas-related diseases, such as diabetes, pancreatitis, and pancreatic cancer, are closely associated with autophagy. Genetic studies that address autophagy confirm this view. Loss of autophagy homeostasis (lack or overactivation) can lead to a series of adverse reactions, such as oxidative accumulation, increased inflammation, and cell death. There is growing evidence that stimulating or inhibiting autophagy is a potential therapeutic strategy for various pancreatic diseases. In this review, we discuss the multiple roles of autophagy in physiological and pathological conditions of the pancreas, including its role as a protective or pathogenic factor.
1 Introduction
Intracellular components are not constant but rather are in a state of dynamic equilibrium. Organelles and proteins are constantly produced, while dysfunctional or redundant components are removed. At least two intracellular cycling pathways are known: the ubiquitin‒proteasome system and the autophagy system (Shrestha et al., 2020). The former degrades ubiquitin-labelled proteins; target proteins are degraded by the proteasome in combination with many enzymes (Zhang et al., 2020). The latter, autophagy, involves the digestion of cytoplasmic components and organelles through lysosomes (Lee A. et al., 2022). In addition, autophagy helps to remove misfolded and aggregated proteins and plays an important role in tissues with high protein synthesis rates, such as the pancreas (Hinzman et al., 2022; Ramachandran et al., 2021; She et al., 2021).
Even without any external stimulation, autophagy, which is called basic autophagy, still occurs in pancreatic cells (Antonucci et al., 2015). Basic autophagy occurs at a low level and is rapidly activated in response to cellular stress, such as hunger (Chediack et al., 2012), oxidation (Pérez et al., 2015), endoplasmic reticulum (ER) stress (Yazıcı et al., 2023; Lugea et al., 2011), or destructive stimulation (Ito et al., 2009; Telbisz et al., 2002). This response is usually beneficial, helping pancreatic cells cope with environmental stress and avoid death. However, in the absence of autophagy, this protective mechanism is not activated. For example, blocking pancreatic autophagy has been shown to increase the sensitivity of mice to bacterial lipopolysaccharide, and more severe vacuolization and inflammation of pancreatic cells have been observed (Xia et al., 2020). This finding suggests that the activation of autophagy limits pancreatic injury. However, autophagy is not always beneficial, and recent studies have shown that excessive autophagy can aggravate pancreatic damage. There is evidence that, compared with that in WT mice, excessive microtubule-associated protein 1 light chain 3 (LC3) in acinar cells in transgenic GFP-LC3 mice destabilizes the autophagy homeostatic state and exacerbates pancreatitis damages (Mareninova et al., 2020).
This article reviews the important role of autophagy in mediating pancreatic homeostasis; discusses the relationship between autophagy and pancreas-related diseases, including pancreatitis, diabetes and pancreatic cancer; and discusses genetic studies that have addressed autophagy.
2 Brief introduction to autophagy function and classification
Autophagy is the biological process by which lysosomes or vacuoles degrade organelles, proteins and other cellular components. The whole process is complex and orderly and is strictly controlled by the synergistic action of at least 30 autophagy-related genes (ATGs) and their products (Zhang et al., 2019). Small-molecule degradation products, such as amino acids and fatty acids, can be recycled (Tang et al., 2022). There are three known types of autophagy: microautophagy, chaperone-mediated autophagy, and macroautophagy (Figure 1). The latter is the most classic type of autophagy. The bilayer membrane structure (also known as the separation membrane or phagocytic mass) appears near the ER. The plasma membrane, ER, Golgi apparatus, recycling endosomes and mitochondria are possible sources of the autophagy membrane (Puri et al., 2014). With the elongation, bending and closure of the membrane structure, autophagosomes are formed. Autophagosomes fuse with lysosomes to form autolysosomes, where the contents are degraded. The second most common type of autophagy is microautophagy, in which lysosomes sag inwards and directly engulf and absorb target cargo (Schuck, 2020). The third type of autophagy, chaperone-mediated autophagy, does not involve membrane recombination; substrate protein containing a specific amino acid sequence (KFERQ) enters the lysosomal membrane via a process mediated by a molecular chaperone (Hsc70) (Diceglie et al., 2021). The most classic and most intensively studied of the above types is macroautophagy, which is referred to as autophagy.
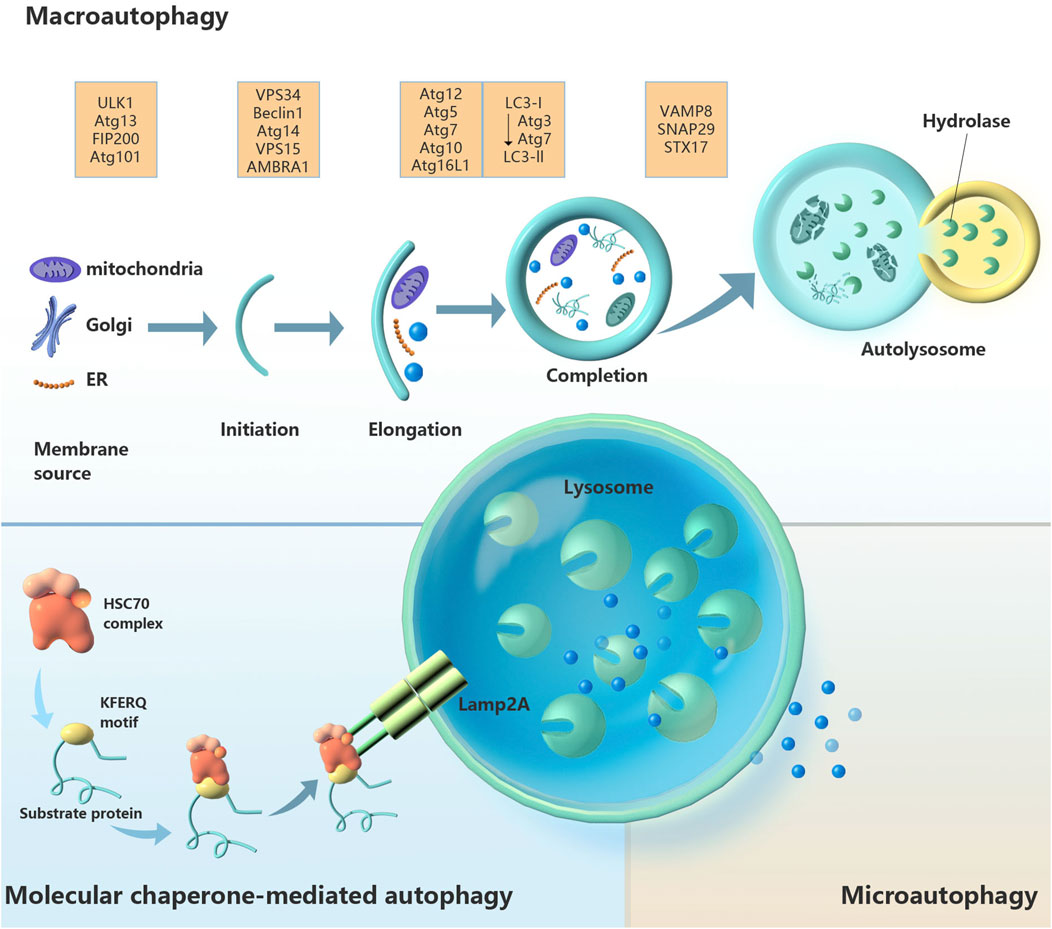
Figure 1. Schematic diagram of the three types of autophagy regulation: macroautophagy, chaperone-mediated autophagy and microautophagy. Abbreviations: AMBRA1, autophagy and beclin 1 regulator 1; Atg, autophagy-related gene; FIP200, focal adhesion kinase family-interacting protein of 200 kD; Hsc70, heat-shock cognate 70; Lamp2A, lysosomal-associated membrane protein 2a; LC3, microtubule-associated protein 1 light chain 3; SNAP29, synaptosome-associated protein 29; Stx17, syntaxin 17; ULK1, unc-51-like autophagy-activating kinase 1; VAMP8, vesicle-associated membrane protein 8; Vps, vacuolar protein sorting.
In fact, autophagy has long been considered nonselective. Later studies revealed that autophagy can selectively degrade proteins and organelles (Yang et al., 2019). Damaged organelles rely on autophagy clearance, and different autophagy subtypes can be defined by their degradation products, such as ER-phagy (removal of ER), mitophagy (removal of mitochondria), ribophagy (removal of ribosome), pexophagy (removal of peroxisome), ribophagy (removal of ribosomes) and lipophagy (removal of lipid droplets). Through the above processes, autophagy plays a crucial role in maintaining cell homeostasis and supporting various biological functions in cells.
3 Measurement of autophagy activity
The methods used to measure autophagy include static and dynamic methods. The former is still accepted as an index of autophagy activity. Static measurements included Western blot, immunohistochemistry and immunofluorescence analyses of autophagy-related proteins (such as p62 and LC3-II) (Wang Y. et al., 2020; Broggi et al., 2021), transmission electron microscopy (He et al., 2022), assessments of TOR and ATG1 kinase activity (Klionsky et al., 2008), and fluorescence microscopy (Santiago-O’Farrill et al., 2020). LC3-II is generated through lipidation of LC3-I and is subsequently recruited to the autophagosome membrane. Upon fusion of the autophagosome with the lysosome, the autolysosome is formed and LC3-II is delipidated back to LC3-I. Hence, LC3-II is commonly utilized as a marker for autophagy. Additionally, P62 is another autophagy marker, similar to LC3, as it interacts directly with LC3 and undergoes selective degradation within autolysosomes. The expression of autophagy-associated proteins and the state of autophagosomes reflect the transient level of autophagy. A higher LC3-II content, lower p62 content and higher autophagosome content are indicative of higher autophagy levels. However, autophagy is a complex and dynamic process, and sometimes static analysis alone is not enough to truly judge the level of autophagic flux in cells (Liu X. Y. et al., 2022). When the fusion of autophagosomes with lysosomes is inhibited, an accumulation of autophagosomes is noted; nevertheless, autophagic flux is diminished (Lerner et al., 2016). Similarly, rapamycin can increase the expression level of the p62 gene and decrease the level of the p62 protein (Cristofani et al., 2018). In contrast, dynamic measurements tend to analyse autophagic flux, which can more accurately reflect the whole process of autophagy (Chen and Gibson, 2022). For researchers to demonstrate that autophagy is activated, autophagy must be blocked. However, instead, autophagy should be activated to prove that autophagy is blocked. There is no absolute standard for defining the state of autophagy. Therefore, multiangle and dynamic measurements of autophagic flux are helpful for evaluating the autophagy status of cells, tissues or organisms.
4 “Zymophagy” - a new selective autophagy pathway
Zymophagy is a cell rescue mechanism that occurs in acinar cells in response to zymogenic activation (Figure 2) (Wang Q. et al., 2022; Ropolo et al., 2019). Activated forms of zymogens are identified, isolated, and targeted for elimination, thereby decreasing pancreatic injury (Wang et al., 2019; Vaccaro et al., 2022). This protective pathophysiological process may involve complex regulatory mechanisms, but many questions remain unanswered. For example, how does zymophagy occur? How are the activated zymogen granules labeled for zymophagy? Which organelles are involved?
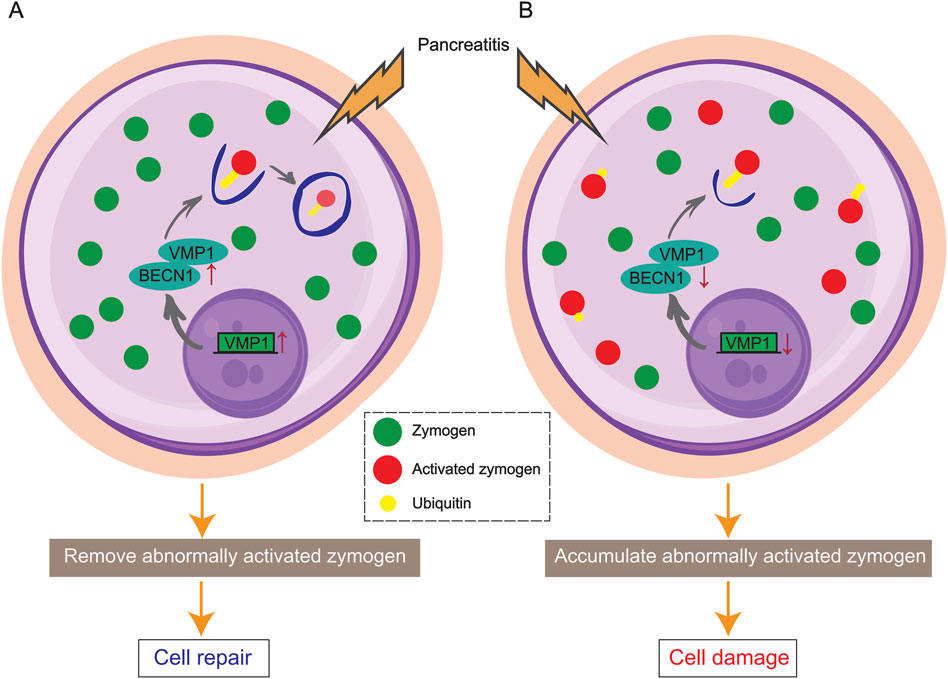
Figure 2. Schematic diagram showing VMP1-mediated zymophagy. (A) VMP1 activates and binds to BECN1, triggering zymophagy to remove activated zymogens and repair the pancreas. (B) In the absence of VMP1, activated zymogens accumulate in acinar cells, leading to pancreatic damage and pancreatitis.
In acinar cells, zymogen ubiquitination is involved in the early zymophagy process. TRIM33 (an E3 ligase) reportedly participates in the ubiquitin modification of trypsin and activates zymophagy (Wang Q. et al., 2022). Following ubiquitination, trypsin binds to vacuole membrane protein 1 (VMP1), a key player in the initiation of autophagy, and is subsequently sequestered to prevent enzymatic damage. VMP1 functions in conjunction with BECN1/Beclin1 to orchestrate this cellular process (Vaccaro et al., 2008; Grasso et al., 2009; Wang S. et al., 2022).
VMP1 was first identified in rats with experimental acute pancreatitis (AP) and has since been the subject of extensive research as a crucial player in zymophagy (Dusetti et al., 2002). In a healthy state, the expression of VMP1 triggers autophagy, but not zymophagy and not pancreatitis. Studies have shown that knocking down VMP1 inhibits the formation of autophagosomes triggered by rapamycin and starvation (Vaccaro et al., 2008). Deletion of VMP1 in mouse acinar cells led to tissue inflammation within 8 days, while knockout of Atg5 and Atg7 took longer to induce inflammation (Wang S. et al., 2022). In an acute pancreatitis model induced by stimulation with the cholecystokinin receptor, a change in the localization of VMP1 was observed, as VMP1 translocated from the base of acinar cells to a region rich in zymogen granules (Grasso et al., 2011). Compared with wild-type mice, ElaI-Vmp1 mice (pancreatic acinar-specific transgenic mice) exhibited significantly reduced cellular inflammation and necrosis after pancreatitis was induced with caerulein (Grasso et al., 2011). In contrast, in the absence of VMP1, acinar cells fail to respond appropriately to spontaneously and prematurely activated zymogen in the pancreas, potentially leading to acinar cell damage and pancreatitis (Wang S. et al., 2022). The reports highlight the positive impact of the VMP1-mediated autophagy pathway and zymophagy, shedding light on the pancreas' self-protection mechanisms in both normal and diseased states.
5 Autophagy and pancreatic homeostasis
Here, we explore pancreatic autophagy in the context of energy deficiency and the direct relationship between autophagy and organelle homeostasis to analyse the role of autophagy in different physiological and pathological conditions in the pancreas.
5.1 Energy deficiency and autophagy
Autophagy was originally discovered during starvation and is used by cells as a survival strategy when energy is scarce (Wang F. et al., 2020). In a long-term fasting study in birds, autophagy was induced in a variety of digestive organs, and the pancreatic mass decreased by more than 20% (Chediack et al., 2012). In another study, fasting increased the expression of the pancreatic 9cRA protein, which in turn supported the role of FoxO1 in pancreatic β cells, including reducing oxidative stress, promoting autophagy and reducing DNA damage, partly by inducing Atg7 mRNA (Yoo et al., 2023). Hunger during pregnancy affects not only the pregnant woman but also the foetus. Studies have shown that the consumption of a low-protein diet by mothers enhances neonatal pancreatic autophagy and induces ER stress in β cells (Yang et al., 2020). Although these results fill some gaps, the following questions remain unanswered: What role does β-cell ER stress play in this process? How much crosstalk occurs between ER stress and autophagy, and how does the level of pancreatic autophagy change when the foetus returns to a normal diet after birth? Future research may help in fully understanding the mechanisms underlying the nutritional limitations of autophagy in foetuses.
Generally, hypoxia has been considered one of the means of inducing autophagy (Liu et al., 2019). However, a study using islet cells showed that this is not the case. Hypoxia increases ROS and downregulates the expression of autophagy-related proteins in pancreatic β-cells, and the antioxidant NAC reverses this trend (Wu et al., 2022). Autophagy in pancreatic β cells may play a protective role in hypoxia. Similarly, in pancreatic stellate cells, autophagic flux does not increase due to hypoxia, possibly because cells meet their energy needs in other ways; autophagy is not necessary (Estaras et al., 2022). In fact, it is worth exploring whether more severe oxygen deprivation activates autophagy in pancreatic stellate cells.
5.2 ER homeostasis and autophagy
Acinar cells have a very high biosynthesis rate; many proteins are produced in the ER and Golgi apparatus. ER strictly controls the quality of proteins to ensure their correct folding and modification. Under pathological conditions of impaired ER function, the excessive accumulation of unfolded or aggregated proteins leads to ER stress (Zhu et al., 2019). ER stress triggers a series of signalling pathways that relieve ER stress; for example, the unfolded protein response (UPR) is activated, which downregulates general translation and upregulates the transcription of genes that mediate ER stress (Huiting et al., 2018). ER stress and the UPR activate ER-phagy continuously. One study revealed that cell cycle progression gene 1 (CCPG1) is a receptor that mediates ER autophagy (Smith and Wilkinson, 2018a). It binds to ATG8 family proteins and RB1CC1/FIP200 independently and separately, which promotes ER autophagy. CCPG1 drives ER degradation, prevents the excessive accumulation of ER lumen proteins in pancreatic acinar cells, alleviates further UPR production, and ultimately protects the pancreas (Smith and Wilkinson, 2018b; Smith et al., 2018). Accordingly, when these steady-state pathways cannot resolve ER stress, acinar cells tend to undergo apoptosis (Jia et al., 2019).
5.3 Mitochondrial homeostasis and autophagy
Healthy mitochondria are essential for the synthesis, storage and secretion of trypsin in pancreatic cells. In mammals, there are two types of mitochondrial autophagy: PTEN-induced kinase 1 (PINK1)-dependent and -independent autophagy (Zhao et al., 2020; Xu et al., 2021). PINK1 is a serine/threonine kinase expressed in mitochondria that plays an important role in initiating mitochondrial autophagy (Li et al., 2019). PINK1-independent autophagy can directly induce mitochondrial degradation, which is mediated by BNIP3/BNIP3L/FUNC1 (Wang Y. et al., 2022).
PINK1 and the E3 ubiquitin ligase Parkin are involved in mitochondrial autophagy. Under basic conditions, PINK1 is transferred to the mitochondrial inner membrane, where it is then rapidly cleaved and degraded by the protease presenilin-related rhomboid-like (PARL) (Kim et al., 2022; Sonn et al., 2022). PINK1 in dysfunctional mitochondria accumulates in the outer membrane and is activated by autophosphorylation at Ser228 to recruit and activate Parkin (Rasool et al., 2018). After Parkin activation, many protein substrates are ubiquitinated, and autophagy receptors (such as OPTN and NDP52) are recruited (Li et al., 2022). Two ubiquitin positive feedback circuits regulate PINK1/Parkin-mediated mitochondrial autophagy: phosphorylated ubiquitin or the ubiquitin-like Atg8 protein family (Padman et al., 2019). Then, autophagy signal activation initiates mitochondrial autophagy and clears damaged mitochondria.
Although PINK1 has been widely studied as a major regulator of mitochondrial autophagy, recent studies have shown that PINK1 does not play an important role in basic mitochondrial autophagy. In Pink1-KO mice, only pancreatic islet tissue exhibited changes in basal mitophagy due to the loss of Pink1; other tissues were not affected (McWilliams et al., 2018). The possible reason is that the regulation of mitochondrial autophagy is complex and environment dependent and that there is a PINK1-independent pathway involved in basic mitochondrial autophagy. There is a compensatory increase in PINK1-independent mitochondrial autophagy in response to PINK1 deletion. Notably, the increased level of basic mitochondrial autophagy in islets may be due to the activation of compensatory autophagy signals and help to relieve metabolic pressure (McWilliams et al., 2018). However, the following questions remain: What is the precise function of PINK1 in mammalian mitochondria? How does the compensatory mechanism of PINK1-independent mitochondrial autophagy work?
5.4 Lysosomal homeostasis and autophagy
The central role of lysosomes in autophagy has long been known. Autophagy depends on the effective fusion of lysosomes and autophagosomes to remove damaged or ageing proteins (Gao et al., 2020). Once the lysosome itself is damaged, the damage can be sensed by galactose lectin, which is subsequently recruited to the lysosome to repair or remove the damaged lysosomal membrane (Hu et al., 2022). Galactose lectins include galactose lectin-3 (Gal-3) and galactose lectin-9 (Gal-9). At the molecular level, Gal-9 and lysosome-associated membrane protein 2 (LAMP-2) have been shown to play important roles in maintaining lysosomal homeostasis and pancreatic autophagy, as well as in preventing pancreatic disease (Sudhakar et al., 2020). Gal-3 has been shown to be associated with pancreatic cancer autophagy. For example, Gal-3 deficiency leads to a decrease in LC3 levels in pancreatic cancer cells (da Silva Filho et al., 2020). LAMP-2 is a major membrane protein component and is involved in the occurrence and maintenance of lysosomes (Mareninova et al., 2015). Pathological changes in lysosomal membrane proteins can lead to lysosomal dysfunction. In addition, LAMP-2 is a key protein that mediates autophagy-related lysosome formation, and its depletion impairs autophagic flux (Li et al., 2018).
6 Autophagy and pancreatic physiology
The importance of autophagy in maintaining pancreatic homeostasis in the physiological environment has been elucidated in experimental animals via genetic changes in autophagy (Table 1). These experimental models involving deletion of autophagy-related genes may provide insights into the mechanistic role of autophagy in pancreatic health and disease.
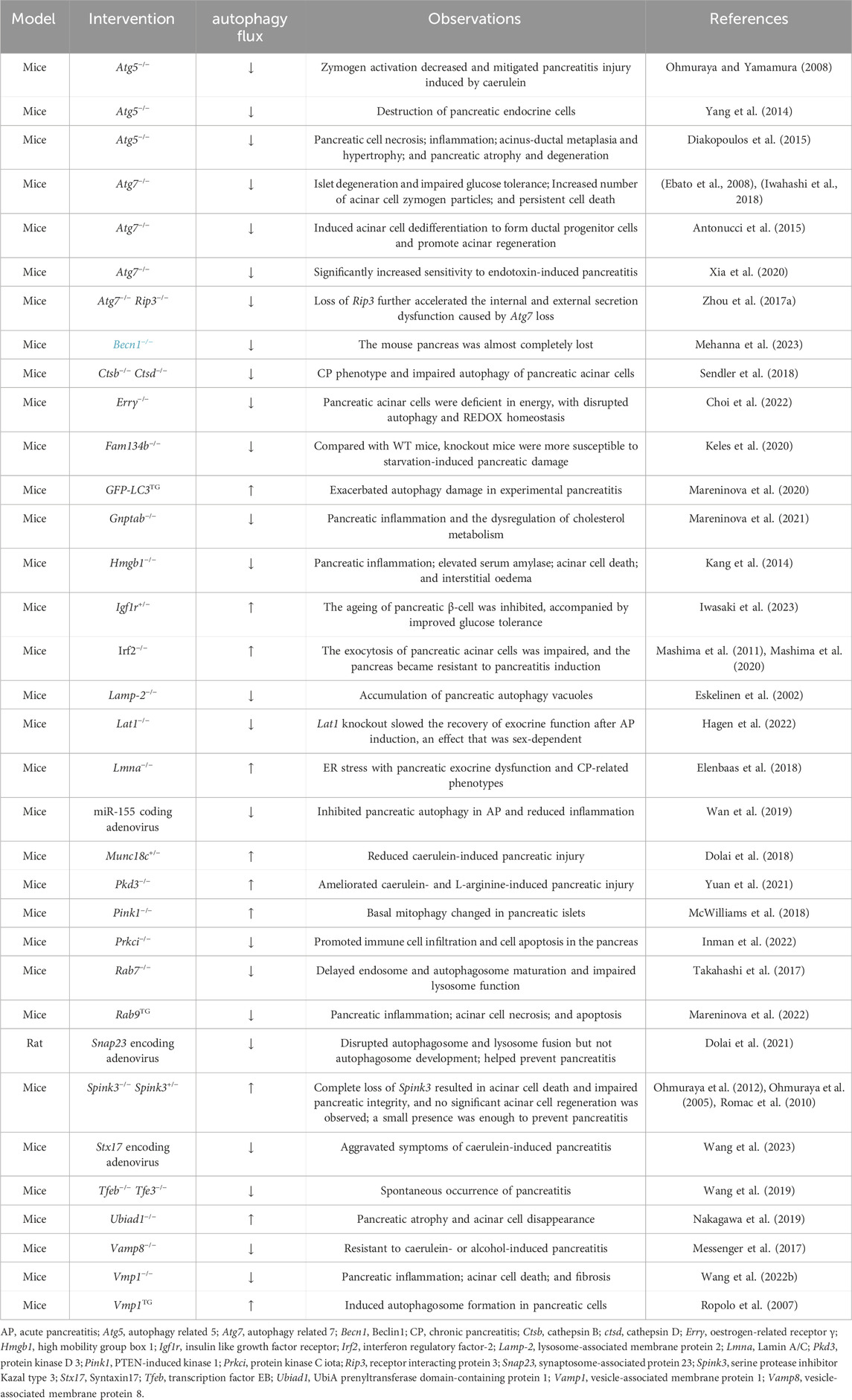
Table 1. Genetic studies investigating the relationship between autophagy and pancreatic physiology in vivo.
The premature activation of trypsinogen by lysosomal hydrolase is a characteristic event and key step in the occurrence of pancreatitis, and one of the important influencing factors is the inhibition of digestive enzyme secretion. In acinar cells, the secretion of zymogen granules is regulated by vesicle-associated membrane proteins (VAMPs), including VAMP2 and VAMP8 (which mediate early secretion and mid-late secretion, respectively). A sharp decrease in VAMP8 leads to the accumulation of intracellular trypsin and the loss of endosomes, which are potential mechanisms of pancreatitis (Pilliod et al., 2022). Vamp8−/− mice were protected from CCK-8-induced zymogen accumulation and acinar damage, suggesting that CCK-8-induced pancreatitis inhibits VAMP8-related zymogen secretion rather than VAMP2-related zymogen secretion (Messenger et al., 2017). It is reasonable to believe that maintaining VAMP8-dependent pro-enzyme secretion helps to reduce the accumulation and activation of proenzymes during pancreatitis, which is one of the potential strategies for reducing pancreatitis injury. Furthermore, the intravenous injection of a miR-155-encoded adenovirus has been shown to alleviate pancreatic injury by reducing the accumulation of autophagosomes in AP mouse cells (Wan et al., 2019). Similarly, in Atg5-deficient mice, zymogen activation was reduced in the pancreatic acini due to a lack of autophagy, thereby alleviating caerulein-induced pancreatitis injury (Ohmuraya and Yamamura, 2008). Furthermore, another study has shown that pancreatic damage induced by caerulein or alcohol is limited in mice intravenously injected with encoding adenovirus for synaptosome-associated protein of 23 (SNAP23) (Dolai et al., 2021).
A trypsin inhibitor, serine protease inhibitor Kazal type 3 (SPINK3) is essential for the integrity of the pancreas, and its absence leads to excessive autophagy in acinar cells (Ohmuraya et al., 2012). Spink3−/− mice die of pancreatic autophagy within a few days after birth, surviving less than 15 days (Ohmuraya et al., 2005). Although SPINK3 plays an important role in maintaining acinar autophagy and cell homeostasis, a low level of this protein seems to be sufficient to prevent pancreatitis. A small amount of SPINK3 expression in Spink3+/− mice did not affect the increased sensitivity to experimental pancreatitis (Romac et al., 2010). The pancreas of adult mice undergo significant changes when the UbiA prenyltransferase domain-containing protein 1 (Ubiad1) is systemically knocked out. These changes include pancreatic atrophy and loss of acinar cells, and are accompanied by enhanced autophagy (Nakagawa et al., 2019). Laminin is associated with pancreatitis because mice without lamin A/C (LMNA) in the pancreas exhibit ER stress, pancreatic exocrine dysfunction and a series of CP-related phenotypes (Elenbaas et al., 2018).
Although the above results suggest that autophagy contributes to the occurrence of pancreatic injury, a large amount of evidence shows that autophagy plays an indispensable role in maintaining pancreatic homeostasis. For example, endocrine cell destruction and a range of similar tissue manifestations, such as inflammation, necrosis, acinar-ductal metaplasia and hypertrophy, and pancreatic atrophy and degeneration, were observed in mice with a deletion of pancreas-specific Atg5 (Yang et al., 2014; Diakopoulos et al., 2015). Consistent with these findings, Atg7-deficient mice also exhibited impaired endocrine systems and reduced insulin secretion (Ebato et al., 2008). In addition, protective and anti-inflammatory effects of autophagy in the pancreas have been confirmed. An increase in the number of acinar cell zymogen granules and persistent cell death were observed in Atg7-deficient mice with pancreatic autophagy deficiency, and these mice exhibited significantly increased sensitivity to endotoxin-induced pancreatitis (Iwahashi et al., 2018; Chen et al., 2022). It has been reported that the deletion of receptor interacting protein 3 (Rip3, a necroptotic signalling factor) exacerbates the acinar loss caused by Atg7 deficiency and is related to immune cell infiltration (Zhou et al., 2017a). The pancreas-specific knockdown of syntaxin17 (Stx17) exacerbates the symptoms of caerulein-induced pancreatitis, which is associated with the disruption of protective autophagy (Wang et al., 2023). In addition, Farnesoid X receptor (FXR) plays a protective role in pancreatitis by restoring pancreatic autophagy through the enhancement of Oxidative stress-induced growth inhibitor 1 (OSGIN1, a tumor suppressor) (Zheng et al., 2022). Pancreatic loss of Fxr increases the sensitivity of mice to acute and chronic pancreatitis induced by caerulein, but GW4064 (an agonist of FXR) limits pancreatic damage (Zheng et al., 2022). The Overexpression of interleukin-22 (IL-22, an inflammation-related factor) significantly alleviates pancreatic necrosis, apoptosis and tissue inflammation induced by caerulein (Feng et al., 2012). Notably, Atg7 deletion also triggers a regeneration mechanism that induces acinar cells to dedifferentiate into ductal progenitor cells, which contributes to the recovery of acinar tissue function (Antonucci et al., 2015). However, LAT1 promotes the regeneration of pancreatic cells after AP in a sex-dependent manner (Hagen et al., 2022).
Many zymogen granules were observed in the cytoplasm of Irf2−/− mouse acinar cells, indicating that IRF2 is a key factor in mediating zymogen-related exocytosis, at least in acinar cells (Mashima et al., 2011). In addition, Irf2−/− acinar cells can partially resist the induction of pancreatitis, an effect that is related to the significant upregulation of the calcium-binding proteins S100 g and Anxa10 (Mashima et al., 2020). Another study also revealed that Munc18c+/− mice lacked basolateral exocytosis of zymogen granules and exhibited mild pancreatitis under caerulein overstimulation (Dolai et al., 2018). The above results suggest that zymogen granule exocytosis may play a more important role in the occurrence of pancreatitis than the increase in the number of autolysosomes. Further exploration of the exocytosis of these zymogen granules will help in obtaining a more comprehensive understanding of pancreatitis.
The absence of protein kinase D3 (Pkd3) in the pancreas promotes autophagy and limits injury in experimental pancreatitis (Yuan et al., 2021). The inhibition of typical (LC3-mediated) autophagy and activation of atypical (RAB9-mediated) autophagy were observed in mice overexpressing RAB9, which resulted in pancreatitis-like damage (Mareninova et al., 2022). RAB9-mediated atypical autophagy cannot completely replace LC3-mediated classical autophagy, at least in pancreatic cells. In fact, VMP1 also affects the homeostasis of pancreatic acinar cells. VMP1 was conditionally overexpressed in mouse pancreatic acinar cells, which induced the production of many vacuoles in acinar cells (Ropolo et al., 2007). However, mice with Vmp1 deletion in pancreatic acinar cells rapidly develop pathological changes similar to those observed in human chronic pancreatitis (Wang S. et al., 2022).
Caerulein-induced autophagy deficiency in acinar cells is due to a decrease in the number of lysosomes, which may be related to the fact that caerulein induces mTOR and promotes the degradation of the transcription factor EB (TFEB) (Wang et al., 2019). A decrease in nuclear TFEB in acinar cells was observed in a mouse model of pancreatitis, an effect consistent with what has been observed in human pancreatitis. Tfeb−/− mice exhibit spontaneous severe pancreatitis and pancreatic fibrosis, and worsen caerulein-induced experimental pancreatitis (Wang et al., 2019). In contrast, mice overexpressing TFEB demonstrate a protective effect against alcohol-induced pancreatic tissue damage (Wang S. et al., 2020). Because lysosomal biogenesis depends on TFEB, activating TFEB to enhance lysosomal activity is likely a possible strategy for the prevention and treatment of pancreatitis.
The role of LAMP-2 in autophagy has been studied in Lamp-2-deficient mice (Eskelinen et al., 2002). LAMP-2 deficiency directly or indirectly leads to the accumulation of autophagic vacuoles in multiple tissues, including the pancreas. Similarly, mice lacking protein kinase C iota (PRKCI, a serine/threonine protein kinase) in the pancreas exhibit autophagic destruction of acinar cells, which promotes pancreatic immune cell infiltration and apoptosis (Inman et al., 2022). Similarly, pancreatic-specific Rab7 deletion hinders the progression of autophagy to autophagic lysosomes and affects endosome maturation and endocytosis, which leads to more severe tissue inflammation (Takahashi et al., 2017). The relationship between cathepsin and autophagy has also been reported recently. The results showed that neither cathepsin B (CTSB) nor cathepsin D (CTSD) alone could cause autophagy damage (Sendler et al., 2018). However, mice with simultaneous deletions of Ctsb and Ctsd exhibited impaired autophagy, indicating that both co-regulate pancreatic autophagy (Sendler et al., 2018). Similarly, trypsin activity increases during AP in Ctsb- and cathepsin L (Ctsl)-knockout mice (Chen et al., 2022).
Bmp4 is a protein that regulates insulin synthesis, processing, and transport. The results from transgenic mice showed that autophagy is also involved in the response of the pancreas to hunger (Yasunaga et al., 2011). Interestingly, starvation for 36 h induces pancreatic damage in Fam134b−/− mice but not in WT mice (Keles et al., 2020). Senescence is related to the functional degradation of tissues and cells. A recent study reported that the deletion of Igf1r in the pancreas of mice inhibits pancreatic β-cell senescence, accompanied by improved glucose tolerance (Iwasaki et al., 2023). In addition, Gnptab−/− mice spontaneously develop pancreatitis and cholesterol metabolism disorders (Mareninova et al., 2021). Consistent with these findings, energy deficiency in pancreatic acinar cells and loss of autophagy and redox homeostasis were observed in oestrogen-related receptor γ (Errγ) conditional knockout mice (Choi et al., 2022).
7 Autophagy and pancreatic pathology
In numerous animal models of pancreatic disease, the modulation of autophagy, whether through pharmacological or genetic interventions, is linked to alterations in disease severity or progression (Table 2).
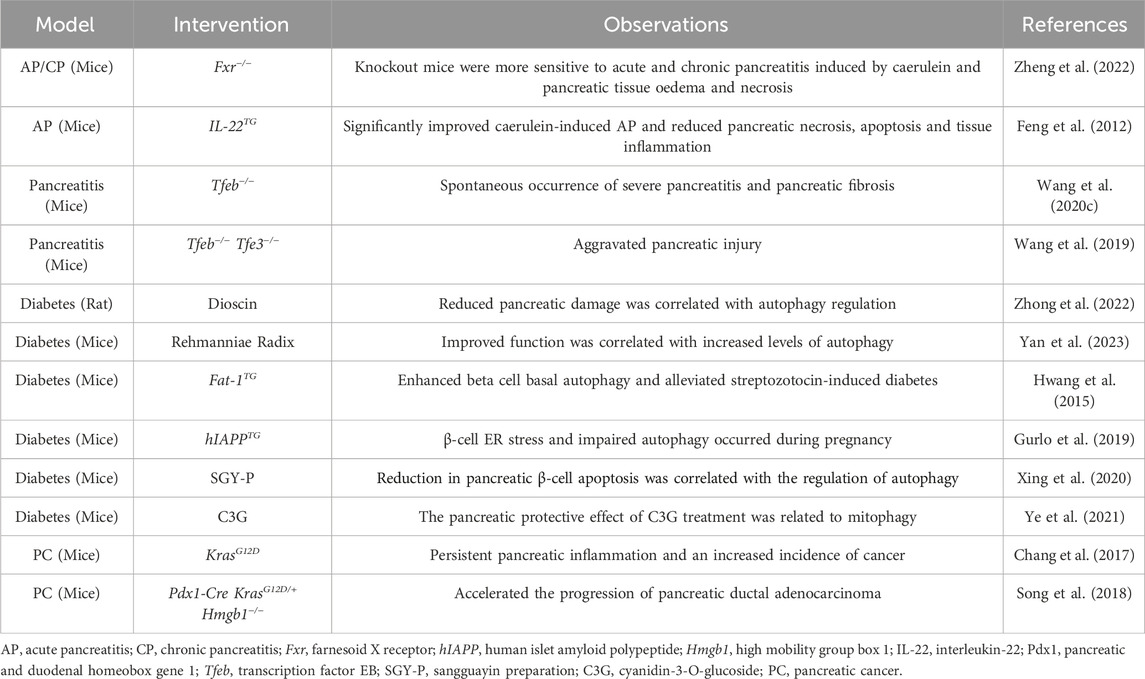
Table 2. Pharmacological and genetic studies examining the relationship between autophagy and pancreatic pathology in vivo.
7.1 Diabetes
The pancreas is a key organ for regulating carbohydrate metabolism, especially glucose metabolism. The pancreas secretes two hormones that are essential for the regulation of blood sugar: insulin and glucagon. Glucagon is the main hormone that increases blood sugar, while insulin is the only hormone with a hypoglycaemic effect in animals (Gong et al., 2019). Islet β-cells are the only source of insulin in the blood, and their dysfunction or loss can lead to diabetes (Le et al., 2022). Studies have shown that diabetes can in turn induce pancreatic tissue cell senescence (Xue et al., 2022). The pathogenesis of diabetes is complex, causing damage to the pancreas itself, and is accompanied by changes in multiple systems throughout the body, including nutritional and metabolic disorders, tissue ageing, hormone level changes (Ruze et al., 2023).
Islet amyloid polypeptide (IAPP) is secreted by islet β cells, and its misfolding or aggregation is related to β cell loss and stress. Human IAPP (hIAPP) transgenic mice exhibited pregnancy-induced ER stress and autophagy damage (Gurlo et al., 2019). This study revealed the pathogenesis of gestational diabetes mellitus associated with autophagy. Db/db mice, characterized by a lack of leptin receptors, spontaneously develop type 2 diabetes and oxidative stress damage in the islets (Xing et al., 2020). Cyanidin-3-O-glucoside (C3G, a plant extract) restores pancreatic function in diabetic mice by activating partial autophagy of mitochondria (Ye et al., 2021). Compared with those of control mice, mice overexpressing Fat-1 exhibited greater levels of basic autophagy in β cells and reduced diabetic damage induced by streptozotocin (Hwang et al., 2015).
The role and mechanism of islet β-cell apoptosis in diabetes have been widely studied. However, the role and mechanism of islet β-cell autophagy in diabetes are still unclear. (Grasso et al., 2009) used streptozotocin (STZ), which is a drug that selectively targets and destroys islet β cells, to establish an experimental diabetes model. According to their results, autophagy is triggered in the early cellular events of diabetes in STZ-induced rats, VMP1 activation occurs in islet cells and the interaction of VMP1-BECN1 stimulates this process (Grasso et al., 2009). Notably, STZ may stimulate islet cells to produce ROS through autophagy, because ROS regulate the activity of ATG4, a key factor in autophagy (Grasso et al., 2009; Lee A. R. et al., 2022). These results suggest that autophagy may contribute to STZ-induced β-cell damage; however, the role of autophagy in diabetes appears to be contradictory. Autophagy may also play a protective role during diabetes, limiting rather than exacerbating cell death. Autophagy plays an active role by removing damaged organelles and ageing cells. For example, autophagy helps clear diabetes-induced damaged mitochondria (Wang et al., 2017). When autophagy is disrupted, cell homeostasis and stress responses become uncontrolled (Muralidharan et al., 2021).
Controlling autophagy is a very promising strategy for the treatment of diabetes. Increasing the level of autophagy can effectively reduce oxidative stress and apoptosis in experimental diabetic animals (Zhu X. et al., 2021; Wang et al., 2018; Liu C. et al., 2022). Oxidative stress is considered the key factor in diabetic injury, and autophagy protects cells from oxidative stress by degrading oxidative stress products. Therefore, drugs like dioscin (Zhong et al., 2022) and Rehmanniae Radix (Yan et al., 2023) can strongly reduce diabetic damage by activating autophagy. In addition, the activation of autophagy can relieve pressure on the ER and improve mitochondrial function (Zhong et al., 2022). Moreover, the protection of cells from apoptosis reduces the inflammatory response and dysfunction.
7.2 Pancreatitis
7.2.1 Increase in autophagosomes but inhibition of autophagy flux
Since Chiari proposed the scientific hypothesis of pancreatitis, it has been generally accepted that pancreatitis is caused by the abnormal activation of zymogens within the pancreas (Dolai et al., 2021; Huang et al., 2020). The pathogenesis of pancreatitis is complex. Early cellular events include dysfunctional autophagy, the pathological exocytosis of zymogen granules, and the activation of trypsin and IKKβ (Saluja et al., 2019). A recent study reported a common component involved in these three events, namely, soluble N-ethylmaleimide-sensitive factor attachment receptor (SNARE) proteins, which include Munc18c and STX17 (Dolai et al., 2021). These proteins are located in the plasma membrane under physiological conditions and are then transferred to autophagosomes during pathological conditions, where they mediate pathological basolateral exocytosis and IKKβ-mediated autolysis (Dolai et al., 2018; Dolai et al., 2021).
Experimental pancreatitis models constructed with cholecystokinin, caerulein, alcohol and Coxsackie virus have been used to simulate human pancreatitis and explore the aetiology of the disease (Han et al., 2022; Kim et al., 2019). In these models, vacuoles accumulate in acinar cells, mainly autophagosomes or autolysosomes, usually with large volumes (Mareninova et al., 2021). The accumulation of large vacuoles in acinar cells observed via histology or transmission electron microscopy provides strong evidence for the diagnosis of pancreatitis. The number and size of autophagic vacuoles and the levels of the autophagy-associated proteins p62 and LC3-II are increased in WT mice (Mareninova et al., 2020). These results reflect the impaired autophagic flux in pancreatitis, findings that are consistent with what has been observed in human disease (Biczo et al., 2018). Several studies have further revealed the relationship between autophagy and pancreatitis; for example, in GFP-LC3 transgenic mice with induced pancreatitis, more intense autophagosome accumulation was observed, which was associated with more severe tissue damage (higher serum amylase levels) (Biczo et al., 2018). Researchers have also shown that bone morphogenetic protein in the pancreas is associated with the production of vacuoles in acinar cells and elevated LC3-II levels (Cao et al., 2013).
7.2.2 Regulating autophagy: a good method for treating pancreatitis
Based on the above analysis, autophagy dysregulation is a key event in pancreatitis. Therefore, one reasonable strategy is to restore blocked or disrupted autophagy to an efficient and unobstructed state. The formation and degradation of autolysosomes are critical for autophagosome clearance, and lysosomal abnormalities have been found in both experimental pancreatitis models and human pancreatitis patients (Mareninova et al., 2021; Şentürk et al., 2019; Chen et al., 2020). Therefore, increasing the number and function of lysosomes, promoting the fusion of autophagosome and lysosome, and enhancing the degradation of autolysosome may contribute to alleviating pancreatic injury. The rationality of this view is supported by several studies (Wang et al., 2023; Zheng et al., 2022; Li et al., 2020). Notably, moderate levels of autophagy, rather than blindly activating autophagy, are beneficial. In fact, the inhibition of overactivated autophagy also helps to combat pancreatitis (Feng et al., 2012). Overall, although the mechanism of autophagy in pancreatitis is unclear, regulating autophagy is one of the most promising strategies for the treatment of this disease.
7.3 Pancreatic cancer
Pancreatic cancer is a highly lethal tumour characterized by strong proliferation, high invasion and multiple drug resistance (Boukrout et al., 2021). Autophagy plays a dual role in pancreatic cancer and is highly involved in its occurrence and development (Wei et al., 2019). On the one hand, autophagy, as a protective mechanism, is used to maintain cell homeostasis and genomic stability and prevent normal cells from transforming into malignant cells (Rahman et al., 2022). Without autophagy, toxic and harmful components (such as damaged organelles and damaged proteins) cannot be removed from pancreatic cells, leading to oxidative stress and subsequent DNA damage (Zhou et al., 2017b). On the other hand, autophagy is involved in the occurrence of pancreatic cancer and serves as a survival strategy for tumour cells to help them respond to environmental stress (Iovanna, 2017; Molejon et al., 2018).
The occurrence of pancreatic cancer is closely related to the presence of the Kras oncogene. Kras mutations are associated with the epithelial–mesenchymal transition (EMT) process and mediate cell carcinogenesis and cancer metastasis. An increasing number of studies have shown that autophagy plays a pivotal role in the malignant transformation of pancreatic cells mediated by Kras mutation. It has been reported that the overexpression of VMP1 exacerbates the tumour-promoting effect of Kras, an effect that can be reversed by chloroquine (an autophagy inhibitor) (Iovanna, 2017). Increased expression of the PRKCI in KrasG12D transgenic mice (often used as an experimental animal model of pancreatic carcinogenesis), a finding that is consistent with what has been observed in human disease (Scotti et al., 2012). The deletion of pancreatic PRKCI blocks the autophagy of acinar cells and the transition from pancreatic intraepithelial neoplasia to pancreatic cancer (Inman et al., 2022). Notably, despite the presence of carcinogenic Kras expression, the transformation of pancreatic cells to eventual pancreatic cancer is limited (Todoric et al., 2017). These findings show that additional regulatory pathways are involved in the development of pancreatic cancer. This complex process includes a series of specific factors, such as p53 mutation (Yang et al., 2014), p62 accumulation (Todoric et al., 2017), lncRNA regulation (Shafabakhsh et al., 2021), the antioxidant stress response (Gong et al., 2016) and the activation of inflammatory signalling pathways (Iovanna, 2016).
KCH (Pdx1-Cre;KrasG12D/+;Hmgb1−/−) mice were developed as a model of accelerated pancreatic ductal adenocarcinoma progression (Song et al., 2018). Oral JTC801 (a compound with strong anticancer activity) effectively limits tumour growth and changes the expression of autophagy-related markers (Song et al., 2018). High-fat, high-calorie diet (HFCD) feeding significantly increased the incidence of cancer in KrasG12D-expressing mice, an effect that was associated with persistent pancreatic inflammation and autophagy disorders (Chang et al., 2017).
In both animal and human specimens, cancer tissue has greater autophagy activity than does surrounding tissue (Hirayama et al., 2009). When pancreatic cell lines are exposed to hypoxia, autophagy helps to increase cell survival (Owada et al., 2017). Similarly, pancreatic cancer also depends on autophagy for survival in the absence of nutrients (Yuan et al., 2022; Zhou et al., 2022). In other words, pancreatic cancer cells can use autophagy to cope with nutritional limitations caused by strong cell proliferation. In addition, autophagy is also a means by which pancreatic cancer cells escape immunosuppression (Zhu X. G. et al., 2021) and the action of drugs (Liu et al., 2014). However, further studies are needed to clarify whether these tumours promote autophagy-related processes via unique or shared potential mechanisms.
It is thought that the role of autophagy in different stages of tumorigenesis is dynamic. In pancreatic cells, autophagy disorders can cause genomic disorders and PanIN lesions (Yang et al., 2014). However, more precancerous lesions do not transform into more pancreatic cancer cells but rather into fewer cells. Blocking autophagy in pancreatic cancer not only suppresses the survival of cancer cells but also helps to disrupt autophagy in pancreatic cancer stem cells, thus enhancing the therapeutic efficacy of anticancer drugs (Leng et al., 2021). Therefore, autophagy is an important target for the treatment and prevention of pancreatic cancer. However, little is known about the specific mechanisms by which autophagy acts as both a tumour suppressor and a tumour enhancer in pancreatic cancer, and elucidating these mechanisms will be helpful for improving treatment strategies for individuals with pancreatic cancer.
8 Conclusion and future prospects
Autophagy plays an important role in the physiology and pathology of the pancreas and plays different roles in different stages (Figure 3). Basic autophagy and moderate autophagy in response to environmental stress are considered protective and protect pancreatic cells from dysfunction and apoptosis. Extreme levels of autophagy (overactivation or autophagy disorder) can become destructive and may mediate more severe cell death and tissue damage. Numerous questions remain unresolved, particularly regarding the impact of other forms of autophagy, such as microautophagy and chaperone-mediated autophagy, on pancreatic function. Equally important, additional types of selective autophagy need to be considered, including fat autophagy, ribosomal autophagy and peroxidase autophagy. For example, ferritin autophagy has been shown to help promote the survival of pancreatic cancer and help it acquire therapeutic resistance (Jain and Amaravadi, 2022).
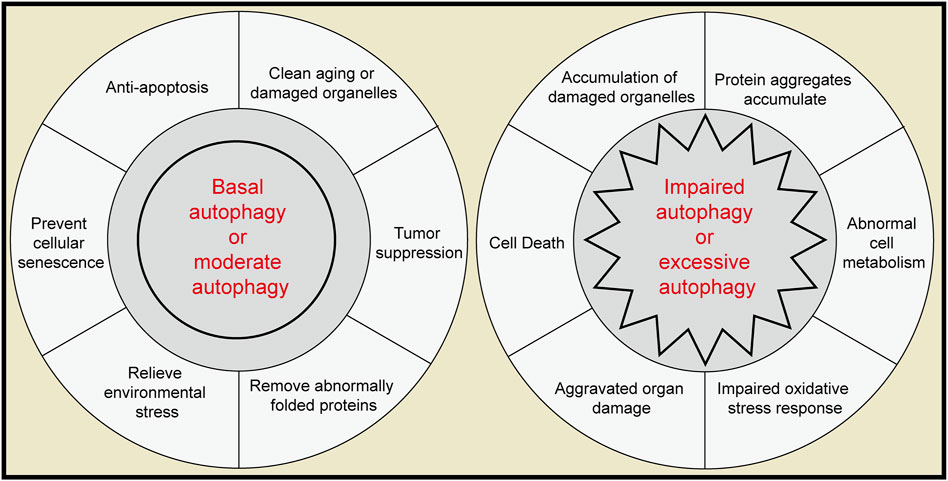
Figure 3. The role of autophagy in the pancreas. Left, moderate autophagy acts as a protective factor in the pancreas. Right, extreme autophagy can act as a pathogenic agent in the pancreas.
In fact, autophagy in the pancreas is pluripotent, and its mechanism may be closely and complexly related to the life processes of other cells. Future research will help in better understanding pancreatic autophagy and adjusting treatment strategies.
Author contributions
ZZ: Conceptualization, Investigation, Methodology, Validation, Visualization, Writing–original draft. PZ: Conceptualization, Formal Analysis, Methodology, Resources, Software, Writing–original draft. JL: Methodology, Project administration, Writing–original draft. JY: Conceptualization, Methodology, Supervision, Validation, Writing–original draft. YJ: Software, Validation, Writing–original draft. MW: Supervision, Writing–review and editing. WT: Funding acquisition, Supervision, Writing–review and editing. LL: Conceptualization, Supervision, Writing–review and editing.
Funding
The author(s) declare that financial support was received for the research, authorship, and/or publication of this article. This work was supported by the National Natural Science Foundation of China [Grant numbers 82174264, 82004227]; and the Natural Science Foundation of Sichuan [Grant numbers 23NSFSC2951].
Acknowledgments
The authors are grateful to American Journal Experts (AJE) for their assistance with language editing.
Conflict of interest
The authors declare that the research was conducted in the absence of any commercial or financial relationships that could be construed as a potential conflict of interest.
Publisher’s note
All claims expressed in this article are solely those of the authors and do not necessarily represent those of their affiliated organizations, or those of the publisher, the editors and the reviewers. Any product that may be evaluated in this article, or claim that may be made by its manufacturer, is not guaranteed or endorsed by the publisher.
References
Antonucci, L., Fagman, J. B., Kim, J. Y., Todoric, J., Gukovsky, I., Mackey, M., et al. (2015). Basal autophagy maintains pancreatic acinar cell homeostasis and protein synthesis and prevents ER stress. Proc. Natl. Acad. Sci. U. S. A. 112, E6166–E6174. doi:10.1073/pnas.1519384112
Biczo, G., Vegh, E. T., Shalbueva, N., Mareninova, O. A., Elperin, J., Lotshaw, E., et al. (2018). Mitochondrial dysfunction, through impaired autophagy, leads to endoplasmic reticulum stress, deregulated lipid metabolism, and pancreatitis in animal models. Gastroenterology 154, 689–703. doi:10.1053/j.gastro.2017.10.012
Boukrout, N., Souidi, M., Lahdaoui, F., Duchêne, B., Neve, B., Coppin, L., et al. (2021). Antagonistic roles of the tumor suppressor miR-210-3p and oncomucin MUC4 forming a negative feedback loop in pancreatic adenocarcinoma. Cancers 13, 6197. doi:10.3390/cancers13246197
Broggi, G., Salvatorelli, L., Barbagallo, D., Certo, F., Altieri, R., Tirrò, E., et al. (2021). Diagnostic utility of the immunohistochemical expression of serine and arginine rich splicing factor 1 (SRSF1) in the differential diagnosis of adult gliomas. Cancers 13, 2086. doi:10.3390/cancers13092086
Cao, Y., Yang, W., Tyler, M. A., Gao, X., Duan, C., Kim, S. O., et al. (2013). Noggin attenuates cerulein-induced acute pancreatitis and impaired autophagy. Pancreas 42, 301–307. doi:10.1097/MPA.0b013e31825b9f2c
Chang, H. H., Moro, A., Takakura, K., Su, H. Y., Mo, A., Nakanishi, M., et al. (2017). Incidence of pancreatic cancer is dramatically increased by a high fat, high calorie diet in KrasG12D mice. PloS one 12, e0184455. doi:10.1371/journal.pone.0184455
Chediack, J. G., Funes, S. C., Cid, F. D., Filippa, V., and Caviedes-Vidal, E. (2012). Effect of fasting on the structure and function of the gastrointestinal tract of house sparrows (Passer domesticus). Comp. Biochem. physiology. Part A, Mol. and Integr. physiology 163, 103–110. doi:10.1016/j.cbpa.2012.05.189
Chen, Q., Li, J., Ma, J., Yang, X., Ni, M., Zhang, Y., et al. (2020). Fibroblast growth factor 21 alleviates acute pancreatitis via activation of the Sirt1-autophagy signalling pathway. J. Cell. Mol. Med. 24, 5341–5351. doi:10.1111/jcmm.15190
Chen, W., Imasaka, M., Iwama, H., Nishiura, H., and Ohmuraya, M. (2022). Double deficiency of cathepsin B and L in the mouse pancreas alters trypsin activity without affecting acute pancreatitis severity. Pancreatol. official J. Int. Assoc. Pancreatol. (IAP) 22, 880–886. doi:10.1016/j.pan.2022.08.011
Chen, Y., and Gibson, S. B. (2022). Tumor suppressing subtransferable candidate 4 expression prevents autophagy-induced cell death following temozolomide treatment in glioblastoma cells. Front. cell Dev. Biol. 10, 823251. doi:10.3389/fcell.2022.823251
Choi, J., Oh, T. G., Jung, H. W., Park, K. Y., Shin, H., Jo, T., et al. (2022). Estrogen-related receptor γ maintains pancreatic acinar cell function and identity by regulating cellular metabolism. Gastroenterology 163, 239–256. doi:10.1053/j.gastro.2022.04.013
Cristofani, R., Montagnani Marelli, M., Cicardi, M. E., Fontana, F., Marzagalli, M., Limonta, P., et al. (2018). Dual role of autophagy on docetaxel-sensitivity in prostate cancer cells. Cell Death Dis. 9, 889. doi:10.1038/s41419-018-0866-5
da Silva Filho, A. F., de Sousa, L. M., Consonni, S. R., da Rocha Pitta, M. G., Carvalho, H. F., and de Melo Rêgo, M. J. B. (2020). Galectin-3 expression in pancreatic cell lines under distinct autophagy-inducing stimulus. Microsc. Microanal. official J. Microsc. Soc. Am. Microbeam Analysis Soc. Microsc. Soc. Can. 26, 1187–1197. doi:10.1017/s1431927620024526
Diakopoulos, K. N., Lesina, M., Wörmann, S., Song, L., Aichler, M., Schild, L., et al. (2015). Impaired autophagy induces chronic atrophic pancreatitis in mice via sex- and nutrition-dependent processes. Gastroenterology 148, 626–638. doi:10.1053/j.gastro.2014.12.003
Diceglie, C., Anelli, G. M., Martelli, C., Serati, A., Lo Dico, A., Lisso, F., et al. (2021). Placental antioxidant defenses and autophagy-related genes in maternal obesity and gestational diabetes mellitus. Nutrients 13, 1303. doi:10.3390/nu13041303
Dolai, S., Liang, T., Orabi, A. I., Xie, L., Holmyard, D., Javed, T. A., et al. (2018). Depletion of the membrane-fusion regulator Munc18c attenuates caerulein hyperstimulation-induced pancreatitis. J. Biol. Chem. 293, 2510–2522. doi:10.1074/jbc.RA117.000792
Dolai, S., Takahashi, T., Qin, T., Liang, T., Xie, L., Kang, F., et al. (2021). Pancreas-specific SNAP23 depletion prevents pancreatitis by attenuating pathological basolateral exocytosis and formation of trypsin-activating autolysosomes. Autophagy 17, 1–14. doi:10.1080/15548627.2020.1852725
Dusetti, N. J., Jiang, Y., Vaccaro, M. I., Tomasini, R., Azizi Samir, A., Calvo, E. L., et al. (2002). Cloning and expression of the rat vacuole membrane protein 1 (VMP1), a new gene activated in pancreas with acute pancreatitis, which promotes vacuole formation. Biochem. biophysical Res. Commun. 290, 641–649. doi:10.1006/bbrc.2001.6244
Ebato, C., Uchida, T., Arakawa, M., Komatsu, M., Ueno, T., Komiya, K., et al. (2008). Autophagy is important in islet homeostasis and compensatory increase of beta cell mass in response to high-fat diet. Cell metab. 8, 325–332. doi:10.1016/j.cmet.2008.08.009
Elenbaas, J. S., Bragazzi Cunha, J., Azuero-Dajud, R., Nelson, B., Oral, E. A., Williams, J. A., et al. (2018). Lamin A/C maintains exocrine pancreas homeostasis by regulating stability of RB and activity of E2F. Gastroenterology 154, 1625–1629. doi:10.1053/j.gastro.2018.01.024
Eskelinen, E. L., Illert, A. L., Tanaka, Y., Schwarzmann, G., Blanz, J., Von Figura, K., et al. (2002). Role of LAMP-2 in lysosome biogenesis and autophagy. Mol. Biol. cell 13, 3355–3368. doi:10.1091/mbc.e02-02-0114
Estaras, M., Martinez, R., Garcia, A., Ortiz-Placin, C., Iovanna, J. L., Santofimia-Castaño, P., et al. (2022). Melatonin modulates metabolic adaptation of pancreatic stellate cells subjected to hypoxia. Biochem. Pharmacol. 202, 115118. doi:10.1016/j.bcp.2022.115118
Feng, D., Park, O., Radaeva, S., Wang, H., Yin, S., Kong, X., et al. (2012). Interleukin-22 ameliorates cerulein-induced pancreatitis in mice by inhibiting the autophagic pathway. Int. J. Biol. Sci. 8, 249–257. doi:10.7150/ijbs.3967
Gao, J., Wei, B., de Assuncao, T. M., Liu, Z., Hu, X., Ibrahim, S., et al. (2020). Hepatic stellate cell autophagy inhibits extracellular vesicle release to attenuate liver fibrosis. J. Hepatology 73, 1144–1154. doi:10.1016/j.jhep.2020.04.044
Gong, J., Belinsky, G., Sagheer, U., Zhang, X., Grippo, P. J., and Chung, C. (2016). Pigment epithelium-derived factor (PEDF) blocks Wnt3a protein-induced autophagy in pancreatic intraepithelial neoplasms. J. Biol. Chem. 291, 22074–22085. doi:10.1074/jbc.M116.729962
Gong, Y., Yang, J., Liu, Q., Cai, J., Zheng, Y., Zhang, Y., et al. (2019). IGF1 knockdown hinders myocardial development through energy metabolism dysfunction caused by ROS-dependent FOXO activation in the chicken heart. Oxid. Med. Cell Longev. 2019, 7838754. doi:10.1155/2019/7838754
Grasso, D., Ropolo, A., Lo Ré, A., Boggio, V., Molejón, M. I., Iovanna, J. L., et al. (2011). Zymophagy, a novel selective autophagy pathway mediated by VMP1-USP9x-p62, prevents pancreatic cell death. J. Biol. Chem. 286, 8308–8324. doi:10.1074/jbc.M110.197301
Grasso, D., Sacchetti, M. L., Bruno, L., Lo Ré, A., Iovanna, J. L., Gonzalez, C. D., et al. (2009). Autophagy and VMP1 expression are early cellular events in experimental diabetes. Pancreatology 9, 81–88. doi:10.1159/000178878
Gurlo, T., Kim, S., Butler, A. E., Liu, C., Pei, L., Rosenberger, M., et al. (2019). Pregnancy in human IAPP transgenic mice recapitulates beta cell stress in type 2 diabetes. Diabetologia 62, 1000–1010. doi:10.1007/s00125-019-4843-z
Hagen, C. M., Roth, E., Graf, T. R., Verrey, F., Graf, R., Gupta, A., et al. (2022). Loss of LAT1 sex-dependently delays recovery after caerulein-induced acute pancreatitis. World J. Gastroenterol. 28, 1024–1054. doi:10.3748/wjg.v28.i10.1024
Han, X., Li, B., Bao, J., Wu, Z., Chen, C., Ni, J., et al. (2022). Endoplasmic reticulum stress promoted acinar cell necroptosis in acute pancreatitis through cathepsinB-mediated AP-1 activation. Front. Immunol. 13, 968639. doi:10.3389/fimmu.2022.968639
He, Y., Dong, X. H., Zhu, Q., Xu, Y. L., Chen, M. L., and Liu, Z. (2022). Ultrasound-triggered microbubble destruction enhances the radiosensitivity of glioblastoma by inhibiting PGRMC1-mediated autophagy in vitro and in vivo. Mil. Med. Res. 9, 9. doi:10.1186/s40779-022-00369-0
Hinzman, C. P., Singh, B., Bansal, S., Li, Y., Iliuk, A., Girgis, M., et al. (2022). A multi-omics approach identifies pancreatic cancer cell extracellular vesicles as mediators of the unfolded protein response in normal pancreatic epithelial cells. J. Extracell. vesicles 11, e12232. doi:10.1002/jev2.12232
Hirayama, A., Kami, K., Sugimoto, M., Sugawara, M., Toki, N., Onozuka, H., et al. (2009). Quantitative metabolome profiling of colon and stomach cancer microenvironment by capillary electrophoresis time-of-flight mass spectrometry. Cancer Res. 69, 4918–4925. doi:10.1158/0008-5472.can-08-4806
Hu, H., Li, P., Qiu, J., Zhao, M., Kuang, M., Zhang, Z., et al. (2022). Optical visualization of red-GQDs' organelles distribution and localization in living cells. Front. Pharmacol. 13, 932807. doi:10.3389/fphar.2022.932807
Huang, H., Swidnicka-Siergiejko, A. K., Daniluk, J., Gaiser, S., Yao, Y., Peng, L., et al. (2020). Transgenic expression of PRSS1(r122H) sensitizes mice to pancreatitis. Gastroenterology 158, 1072–1082. doi:10.1053/j.gastro.2019.08.016
Huiting, L. N., Samaha, Y., Zhang, G. L., Roderick, J. E., Li, B., Anderson, N. M., et al. (2018). UFD1 contributes to MYC-mediated leukemia aggressiveness through suppression of the proapoptotic unfolded protein response. Leukemia 32, 2339–2351. doi:10.1038/s41375-018-0141-x
Hwang, W. M., Bak, D. H., Kim, D. H., Hong, J. Y., Han, S. Y., Park, K. Y., et al. (2015). Omega-3 polyunsaturated fatty acids may attenuate streptozotocin-induced pancreatic β-cell death via autophagy activation in Fat1 transgenic mice. Endocrinol. metabolism (Seoul, Korea) 30, 569–575. doi:10.3803/EnM.2015.30.4.569
Inman, K. S., Liu, Y., Scotti Buzhardt, M. L., Leitges, M., Krishna, M., Crawford, H. C., et al. (2022). Prkci regulates autophagy and pancreatic tumorigenesis in mice. Cancers 14, 796. doi:10.3390/cancers14030796
Iovanna, J. L. (2016). Autophagy induced during pancreatitis promotes KRAS-dependent transformation in the pancreas. Front. Oncol. 6, 226. doi:10.3389/fonc.2016.00226
Iovanna, J. L. (2017). Autophagy contributes to the initiation of pancreatic cancer. Med. Sci. M/S 33, 335–339. doi:10.1051/medsci/20173303022
Ito, M., Nakagawa, H., Okada, T., Miyazaki, S., and Matsuo, S. (2009). ER-stress caused by accumulated intracistanal granules activates autophagy through a different signal pathway from unfolded protein response in exocrine pancreas cells of rats exposed to fluoride. Archives Toxicol. 83, 151–159. doi:10.1007/s00204-008-0341-7
Iwahashi, K., Hikita, H., Makino, Y., Shigekawa, M., Ikezawa, K., Yoshioka, T., et al. (2018). Autophagy impairment in pancreatic acinar cells causes zymogen granule accumulation and pancreatitis. Biochem. biophysical Res. Commun. 503, 2576–2582. doi:10.1016/j.bbrc.2018.07.018
Iwasaki, K., Lalani, B., Kahng, J., Carapeto, P., Sanjines, S., Hela, F., et al. (2023). Decreased IGF1R attenuates senescence and improves function in pancreatic β-cells. Front. Endocrinol. (Lausanne) 14, 1203534. doi:10.3389/fendo.2023.1203534
Jain, V., and Amaravadi, R. K. (2022). Pumping iron: ferritinophagy promotes survival and therapy resistance in pancreatic cancer. Cancer Discov. 12, 2023–2025. doi:10.1158/2159-8290.Cd-22-0734
Jia, S., Xu, X., Zhou, S., Chen, Y., Ding, G., and Cao, L. (2019). Fisetin induces autophagy in pancreatic cancer cells via endoplasmic reticulum stress- and mitochondrial stress-dependent pathways. Cell Death Dis. 10, 142. doi:10.1038/s41419-019-1366-y
Kang, R., Zhang, Q., Hou, W., Yan, Z., Chen, R., Bonaroti, J., et al. (2014). Intracellular Hmgb1 inhibits inflammatory nucleosome release and limits acute pancreatitis in mice. Gastroenterology 146, 1097–1107. doi:10.1053/j.gastro.2013.12.015
Keles, U., Iscan, E., Yilmaz, H. E., Karakülah, G., Suner, A., Bal, E., et al. (2020). Differential expression of full-length and NH(2) terminally truncated FAM134B isoforms in normal physiology and cancer. Am. J. Physiol. Gastrointest. Liver Physiol. 319, G733–g747. doi:10.1152/ajpgi.00094.2020
Kim, C. W., Yoo, H. J., Park, J. H., Oh, J. E., and Lee, H. K. (2019). Exogenous interleukin-33 contributes to protective immunity via cytotoxic T-cell priming against mucosal influenza viral infection. Viruses 11, 840. doi:10.3390/v11090840
Kim, M., Nikouee, A., Sun, Y., Zhang, Q. J., Liu, Z. P., and Zang, Q. S. (2022). Evaluation of parkin in the regulation of myocardial mitochondria-associated membranes and cardiomyopathy during endotoxemia. Front. cell Dev. Biol. 10, 796061. doi:10.3389/fcell.2022.796061
Klionsky, D. J., Abeliovich, H., Agostinis, P., Agrawal, D. K., Aliev, G., Askew, D. S., et al. (2008). Guidelines for the use and interpretation of assays for monitoring autophagy in higher eukaryotes. Autophagy 4, 151–175. doi:10.4161/auto.5338
Le, T. H., Kim, J. H., and Park, S. J. (2022). A Co-doped carbon dot/silver nanoparticle nanocomposite-based fluorescence sensor for metformin hydrochloride detection. Nanomater. Basel, Switz. 12, 1297. doi:10.3390/nano12081297
Lee, A., Kondapalli, C., Virga, D. M., Lewis, T. L., Koo, S. Y., Ashok, A., et al. (2022a). Aβ42 oligomers trigger synaptic loss through CAMKK2-AMPK-dependent effectors coordinating mitochondrial fission and mitophagy. Nat. Commun. 13, 4444. doi:10.1038/s41467-022-32130-5
Lee, A. R., Woo, J. S., Lee, S. Y., Na, H. S., Cho, K. H., Lee, Y. S., et al. (2022b). Mitochondrial transplantation ameliorates the development and progression of osteoarthritis. Immune Netw. 22, e14. doi:10.4110/in.2022.22.e14
Leng, S., Huang, W., Chen, Y., Yang, Y., Feng, D., Liu, W., et al. (2021). SIRT1 coordinates with the CRL4B complex to regulate pancreatic cancer stem cells to promote tumorigenesis. Cell death Differ. 28, 3329–3343. doi:10.1038/s41418-021-00821-z
Lerner, T. R., de Souza Carvalho-Wodarz, C., Repnik, U., Russell, M. R. G., Borel, S., Diedrich, C. R., et al. (2016). Lymphatic endothelial cells are a replicative niche for Mycobacterium tuberculosis. J. Clin. investigation 126, 1093–1108. doi:10.1172/jci83379
Li, B., Wu, J., Bao, J., Han, X., Shen, S., Ye, X., et al. (2020). Activation of α7nACh receptor protects against acute pancreatitis through enhancing TFEB-regulated autophagy. Biochimica biophysica acta. Mol. basis Dis. 1866, 165971. doi:10.1016/j.bbadis.2020.165971
Li, J., Ma, C., Long, F., Yang, D., Liu, X., Hu, Y., et al. (2019). Parkin impairs antiviral immunity by suppressing the mitochondrial reactive oxygen species-nlrp3 Axis and antiviral inflammation. iScience 16, 468–484. doi:10.1016/j.isci.2019.06.008
Li, X., Hou, P., Ma, W., Wang, X., Wang, H., Yu, Z., et al. (2022). SARS-CoV-2 ORF10 suppresses the antiviral innate immune response by degrading MAVS through mitophagy. Cell. and Mol. Immunol. 19, 67–78. doi:10.1038/s41423-021-00807-4
Li, Z., Zhu, S., Huang, L., Shang, M., Yu, C., Zhu, H., et al. (2018). Exendin-4 impairs the autophagic flux to induce apoptosis in pancreatic acinar AR42J cells by down-regulating LAMP-2. Biochem. biophysical Res. Commun. 496, 294–301. doi:10.1016/j.bbrc.2018.01.037
Liu, C., Sun, W., Zhu, T., Shi, S., Zhang, J., Wang, J., et al. (2022b). Glia maturation factor-β induces ferroptosis by impairing chaperone-mediated autophagic degradation of ACSL4 in early diabetic retinopathy. Redox Biol. 52, 102292. doi:10.1016/j.redox.2022.102292
Liu, H., Zhang, Z., Xiong, W., Zhang, L., Du, Y., Liu, Y., et al. (2019). Long non-coding RNA MALAT1 mediates hypoxia-induced pro-survival autophagy of endometrial stromal cells in endometriosis. J. Cell. Mol. Med. 23, 439–452. doi:10.1111/jcmm.13947
Liu, K., Huang, J., Xie, M., Yu, Y., Zhu, S., Kang, R., et al. (2014). MIR34A regulates autophagy and apoptosis by targeting HMGB1 in the retinoblastoma cell. Autophagy 10, 442–452. doi:10.4161/auto.27418
Liu, X. Y., Peng, J., He, F., Tursun, X., Li, S. P., Xin, X. L., et al. (2022a). Shabyar ameliorates high glucose induced retinal pigment epithelium injury through suppressing aldose reductase and AMPK/mTOR/ULK1 autophagy pathway. Front. Pharmacol. 13, 852945. doi:10.3389/fphar.2022.852945
Lugea, A., Waldron, R. T., French, S. W., and Pandol, S. J. (2011). Drinking and driving pancreatitis: links between endoplasmic reticulum stress and autophagy. Autophagy 7, 783–785. doi:10.4161/auto.7.7.15594
Mareninova, O. A., Dillon, D. L., Wightman, C. J. M., Yakubov, I., Takahashi, T., Gaisano, H. Y., et al. (2022). Rab9 mediates pancreatic autophagy switch from canonical to noncanonical, aggravating experimental pancreatitis. Cell. Mol. gastroenterology hepatology 13, 599–622. doi:10.1016/j.jcmgh.2021.09.017
Mareninova, O. A., Jia, W., Gretler, S. R., Holthaus, C. L., Thomas, D. D. H., Pimienta, M., et al. (2020). Transgenic expression of GFP-LC3 perturbs autophagy in exocrine pancreas and acute pancreatitis responses in mice. Autophagy 16, 2084–2097. doi:10.1080/15548627.2020.1715047
Mareninova, O. A., Sendler, M., Malla, S. R., Yakubov, I., French, S. W., Tokhtaeva, E., et al. (2015). Lysosome associated membrane proteins maintain pancreatic acinar cell homeostasis: LAMP-2 deficient mice develop pancreatitis. Cell. Mol. gastroenterology hepatology 1, 678–694. doi:10.1016/j.jcmgh.2015.07.006
Mareninova, O. A., Vegh, E. T., Shalbueva, N., Wightman, C. J., Dillon, D. L., Malla, S., et al. (2021). Dysregulation of mannose-6-phosphate-dependent cholesterol homeostasis in acinar cells mediates pancreatitis. J. Clin. investigation 131, e146870. doi:10.1172/jci146870
Mashima, H., Sato, T., Horie, Y., Nakagawa, Y., Kojima, I., Ohteki, T., et al. (2011). Interferon regulatory factor-2 regulates exocytosis mechanisms mediated by SNAREs in pancreatic acinar cells. Gastroenterology 141, 1102–1113. e1101-1108. doi:10.1053/j.gastro.2011.05.051
Mashima, H., Takahashi, K., Sekine, M., Matsumoto, S., Asano, T., Uehara, T., et al. (2020). The role of calcium-binding protein S100g (CalbindinD-9K) and annexin A10 in acute pancreatitis. Biochem. biophysical Res. Commun. 526, 692–698. doi:10.1016/j.bbrc.2020.03.155
McWilliams, T. G., Prescott, A. R., Montava-Garriga, L., Ball, G., Singh, F., Barini, E., et al. (2018). Basal mitophagy occurs independently of PINK1 in mouse tissues of high metabolic demand. Cell metab. 27, 439–449. doi:10.1016/j.cmet.2017.12.008
Mehanna, S., Arakawa, S., Imasaka, M., Chen, W., Nakanishi, Y., Nishiura, H., et al. (2023). Beclin1 is essential for the pancreas development. Dev. Biol. 504, 113–119. doi:10.1016/j.ydbio.2023.09.008
Messenger, S. W., Jones, E. K., Holthaus, C. L., Thomas, D. D. H., Cooley, M. M., Byrne, J. A., et al. (2017). Acute acinar pancreatitis blocks vesicle-associated membrane protein 8 (VAMP8)-dependent secretion, resulting in intracellular trypsin accumulation. J. Biol. Chem. 292, 7828–7839. doi:10.1074/jbc.M117.781815
Molejon, M. I., Swayden, M., Fanale, D., Bintz, J., Gayet, O., Soubeyran, P., et al. (2018). Chloroquine plays a cell-dependent role in the response to treatment of pancreatic adenocarcinoma. Oncotarget 9, 30837–30846. doi:10.18632/oncotarget.25745
Muralidharan, C., Conteh, A. M., Marasco, M. R., Crowder, J. J., Kuipers, J., de Boer, P., et al. (2021). Pancreatic beta cell autophagy is impaired in type 1 diabetes. Diabetologia 64, 865–877. doi:10.1007/s00125-021-05387-6
Nakagawa, K., Fujiwara, K., Nishimura, A., Murakami, C., Kawamoto, K., Ichinose, C., et al. (2019). UBIAD1 plays an essential role in the survival of pancreatic acinar cells. Int. J. Mol. Sci. 20, 1971. doi:10.3390/ijms20081971
Ohmuraya, M., Hirota, M., Araki, M., Mizushima, N., Matsui, M., Mizumoto, T., et al. (2005). Autophagic cell death of pancreatic acinar cells in serine protease inhibitor Kazal type 3-deficient mice. Gastroenterology 129, 696–705. doi:10.1016/j.gastro.2005.05.057
Ohmuraya, M., Sugano, A., Hirota, M., Takaoka, Y., and Yamamura, K. (2012). Role of intrapancreatic SPINK1/spink3 expression in the development of pancreatitis. Front. physiology 3, 126. doi:10.3389/fphys.2012.00126
Ohmuraya, M., and Yamamura, K. (2008). Autophagy and acute pancreatitis: a novel autophagy theory for trypsinogen activation. Autophagy 4, 1060–1062. doi:10.4161/auto.6825
Owada, S., Ito, K., Endo, H., Shida, Y., Okada, C., Nezu, T., et al. (2017). An adaptation system to avoid apoptosis via autophagy under hypoxic conditions in pancreatic cancer cells. Anticancer Res. 37, 4927–4934. doi:10.21873/anticanres.11902
Padman, B. S., Nguyen, T. N., Uoselis, L., Skulsuppaisarn, M., Nguyen, L. K., and Lazarou, M. (2019). LC3/GABARAPs drive ubiquitin-independent recruitment of Optineurin and NDP52 to amplify mitophagy. Nat. Commun. 10, 408. doi:10.1038/s41467-019-08335-6
Pérez, S., Pereda, J., Sabater, L., and Sastre, J. (2015). Redox signaling in acute pancreatitis. Redox Biol. 5, 1–14. doi:10.1016/j.redox.2015.01.014
Pilliod, J., Gélinas-Faucher, M., and Leclerc, N. (2022). Unconventional secretion of tau by VAMP8 impacts its intra- and extracellular cleavage. Front. cell Dev. Biol. 10, 912118. doi:10.3389/fcell.2022.912118
Puri, C., Renna, M., Bento, C. F., Moreau, K., and Rubinsztein, D. C. (2014). ATG16L1 meets ATG9 in recycling endosomes: additional roles for the plasma membrane and endocytosis in autophagosome biogenesis. Autophagy 10, 182–184. doi:10.4161/auto.27174
Rahman, M. A., Ahmed, K. R., Parvez, M. A. K., Lee, I. S., and Kim, B. (2022). Therapeutic aspects and molecular targets of autophagy to control pancreatic cancer management. Biomedicines 10, 1459. doi:10.3390/biomedicines10061459
Ramachandran, S., Kaushik, I. S., and Srivastava, S. K. (2021). Pimavanserin: a novel autophagy modulator for pancreatic cancer treatment. Cancers 13, 5661. doi:10.3390/cancers13225661
Rasool, S., Soya, N., Truong, L., Croteau, N., Lukacs, G. L., and Trempe, J. F. (2018). PINK1 autophosphorylation is required for ubiquitin recognition, EMBO Rep. 19, e44981. doi:10.15252/embr.201744981
Romac, J. M., Ohmuraya, M., Bittner, C., Majeed, M. F., Vigna, S. R., Que, J., et al. (2010). Transgenic expression of pancreatic secretory trypsin inhibitor-1 rescues SPINK3-deficient mice and restores a normal pancreatic phenotype. Am. J. Physiol. Gastrointest. Liver Physiol. 298, G518–G524. doi:10.1152/ajpgi.00431.2009
Ropolo, A., Grasso, D., Pardo, R., Sacchetti, M. L., Archange, C., Lo Re, A., et al. (2007). The pancreatitis-induced vacuole membrane protein 1 triggers autophagy in mammalian cells. J. Biol. Chem. 282, 37124–37133. doi:10.1074/jbc.M706956200
Ropolo, A., Grasso, D., and Vaccaro, M. I. (2019). Measuring autophagy in pancreatitis. Methods Mol. Biol. Clift. N.J. 1880, 541–554. doi:10.1007/978-1-4939-8873-0_35
Ruze, R., Song, J., Yin, X., Chen, Y., Xu, R., Wang, C., et al. (2023). Mechanisms of obesity- and diabetes mellitus-related pancreatic carcinogenesis: a comprehensive and systematic review. Signal Transduct. Target. Ther. 8, 139. doi:10.1038/s41392-023-01376-w
Saluja, A., Dudeja, V., Dawra, R., and Sah, R. P. (2019). Early intra-acinar events in pathogenesis of pancreatitis. Gastroenterology 156, 1979–1993. doi:10.1053/j.gastro.2019.01.268
Santiago-O’Farrill, J. M., Weroha, S J., Hou, X., Oberg, A. L., Heinzen, E. P., Maurer, M. J., et al. (2020). Poly(adenosine diphosphate ribose) polymerase inhibitors induce autophagy-mediated drug resistance in ovarian cancer cells, xenografts, and patient-derived xenograft models. 126, 894–907. doi:10.1002/cncr.32600
Schuck, S. (2020). Microautophagy - distinct molecular mechanisms handle cargoes of many sizes. J. cell Sci. 133, jcs246322. doi:10.1242/jcs.246322
Scotti, M. L., Smith, K. E., Butler, A. M., Calcagno, S. R., Crawford, H. C., Leitges, M., et al. (2012). Protein kinase C iota regulates pancreatic acinar-to-ductal metaplasia. PloS one 7, e30509. doi:10.1371/journal.pone.0030509
Sendler, M., Weiss, F. U., Golchert, J., Homuth, G., van den Brandt, C., Mahajan, U. M., et al. (2018). Cathepsin B-mediated activation of trypsinogen in endocytosing macrophages increases severity of pancreatitis in mice. Gastroenterology 154, 704–718. doi:10.1053/j.gastro.2017.10.018
Şentürk, M., Lin, G., Zuo, Z., Mao, D., Watson, E., Mikos, A. G., et al. (2019). Ubiquilins regulate autophagic flux through mTOR signalling and lysosomal acidification. Nat. cell Biol. 21, 384–396. doi:10.1038/s41556-019-0281-x
Shafabakhsh, R., Arianfar, F., Vosough, M., Mirzaei, H. R., Mahjoubin-Tehran, M., Khanbabaei, H., et al. (2021). Autophagy and gastrointestinal cancers: the behind the scenes role of long non-coding RNAs in initiation, progression, and treatment resistance. Cancer gene Ther. 28, 1229–1255. doi:10.1038/s41417-020-00272-7
She, J., Feng, N., Zheng, W., Zheng, H., Cai, P., Zou, H., et al. (2021). Zearalenone exposure disrupts blood-testis barrier integrity through excessive Ca(2+)-mediated autophagy. Toxins 13, 875. doi:10.3390/toxins13120875
Shrestha, N., Liu, T., Ji, Y., Reinert, R. B., Torres, M., Li, X., et al. (2020). Sel1L-Hrd1 ER-associated degradation maintains β cell identity via TGF-β signaling. J. Clin. investigation 130, 3499–3510. doi:10.1172/jci134874
Smith, M. D., Harley, M. E., Kemp, A. J., Wills, J., Lee, M., Arends, M., et al. (2018). CCPG1 is a non-canonical autophagy cargo receptor essential for ER-phagy and pancreatic ER proteostasis. Dev. cell 44, 217–232. doi:10.1016/j.devcel.2017.11.024
Smith, M. D., and Wilkinson, S. (2018a). CCPG1, a cargo receptor required for reticulophagy and endoplasmic reticulum proteostasis. Autophagy 14, 1090–1091. doi:10.1080/15548627.2018.1441473
Smith, M. D., and Wilkinson, S. (2018b). “CCPG1, an unconventional cargo receptor for ER-phagy, maintains pancreatic acinar cell health,” in Molecular and cellular oncology 5. doi:10.1080/23723556.2018.1441631e1441631
Song, X., Zhu, S., Xie, Y., Liu, J., Sun, L., Zeng, D., et al. (2018). JTC801 induces pH-dependent death specifically in cancer cells and slows growth of tumors in mice. Gastroenterology 154, 1480–1493. doi:10.1053/j.gastro.2017.12.004
Sonn, S. K., Song, E. J., Seo, S., Kim, Y. Y., Um, J. H., Yeo, F. J., et al. (2022). Peroxiredoxin 3 deficiency induces cardiac hypertrophy and dysfunction by impaired mitochondrial quality control. Redox Biol. 51, 102275. doi:10.1016/j.redox.2022.102275
Sudhakar, J. N., Lu, H. H., Chiang, H. Y., Suen, C. S., Hwang, M. J., Wu, S. Y., et al. (2020). Lumenal Galectin-9-Lamp2 interaction regulates lysosome and autophagy to prevent pathogenesis in the intestine and pancreas. Nat. Commun. 11, 4286. doi:10.1038/s41467-020-18102-7
Takahashi, K., Mashima, H., Miura, K., Maeda, D., Goto, A., Goto, T., et al. (2017). Disruption of small GTPase Rab7 exacerbates the severity of acute pancreatitis in experimental mouse models. Sci. Rep. 7, 2817. doi:10.1038/s41598-017-02988-3
Tang, H., Zheng, Z., Wang, H., Wang, L., Zhao, G., and Wang, P. (2022). Vitamin K2 modulates mitochondrial dysfunction induced by 6-hydroxydopamine in SH-SY5Y cells via mitochondrial quality-control loop. Nutrients 14, 1504. doi:10.3390/nu14071504
Telbisz, A., Kovács, A. L., and Somosy, Z. (2002). Influence of X-ray on the autophagic-lysosomal system in rat pancreatic acini. Micron. 33, 143–151. doi:10.1016/s0968-4328(01)00005-1
Todoric, J., Antonucci, L., Di Caro, G., Li, N., Wu, X., Lytle, N. K., et al. (2017). Stress-activated NRF2-MDM2 cascade controls neoplastic progression in pancreas. Cancer cell 32, 824–839. doi:10.1016/j.ccell.2017.10.011
Vaccaro, M. I., Mitchell, F., Rivera, F., and Gonzalez, C. D. (2022). Protein expression in exocrine pancreatic diseases. Focus on VMP1 mediated autophagy. Adv. protein Chem. Struct. Biol. 132, 175–197. doi:10.1016/bs.apcsb.2022.07.001
Vaccaro, M. I., Ropolo, A., Grasso, D., and Iovanna, J. L. (2008). A novel mammalian trans-membrane protein reveals an alternative initiation pathway for autophagy. Autophagy 4, 388–390. doi:10.4161/auto.5656
Wan, J., Yang, X., Ren, Y., Li, X., Zhu, Y., Haddock, A. N., et al. (2019). Inhibition of miR-155 reduces impaired autophagy and improves prognosis in an experimental pancreatitis mouse model. Cell Death Dis. 10, 303. doi:10.1038/s41419-019-1545-x
Wang, F., Xu, C., Reece, E. A., Li, X., Wu, Y., Harman, C., et al. (2017). Protein kinase C-alpha suppresses autophagy and induces neural tube defects via miR-129-2 in diabetic pregnancy. Nat. Commun. 8, 15182. doi:10.1038/ncomms15182
Wang, F., Zhang, R., Feng, W., Tsuchiya, D., Ballew, O., Li, J., et al. (2020b). Autophagy of an amyloid-like translational repressor regulates meiotic exit. Dev. cell 52, 141–151. doi:10.1016/j.devcel.2019.12.017
Wang, Q., Yu, J., Gao, W., Sun, Y., Liu, X., Lv, Z., et al. (2022a). The lncRNA TCONS_00021785/miR-21-5p/Trim33 axis regulates VMP1-mediated zymophagy, reduces the activation of trypsinogen, and promotes acinar cell recovery. Cell death Discov. 8, 65. doi:10.1038/s41420-022-00862-4
Wang, S., Chao, X., Jiang, X., Wang, T., Rodriguez, Y., Yang, L., et al. (2022b). Loss of acinar cell VMP1 triggers spontaneous pancreatitis in mice. Autophagy 18, 1572–1582. doi:10.1080/15548627.2021.1990672
Wang, S., Ni, H. M., Chao, X., Ma, X., Kolodecik, T., De Lisle, R., et al. (2020c). Critical role of TFEB-mediated lysosomal biogenesis in alcohol-induced pancreatitis in mice and humans. Cell. Mol. gastroenterology hepatology 10, 59–81. doi:10.1016/j.jcmgh.2020.01.008
Wang, S., Ni, H. M., Chao, X., Wang, H., Bridges, B., Kumer, S., et al. (2019). Impaired TFEB-mediated lysosomal biogenesis promotes the development of pancreatitis in mice and is associated with human pancreatitis. Autophagy 15, 1954–1969. doi:10.1080/15548627.2019.1596486
Wang, T. T., Zhang, L. C., Qin, Z., Chen, S. J., Zeng, J. M., Li, J. Y., et al. (2023). Decreased syntaxin17 expression contributes to the pathogenesis of acute pancreatitis in murine models by impairing autophagic degradation. Acta Pharmacol. Sin. 44, 2445–2454. doi:10.1038/s41401-023-01139-x
Wang, Y., Peng, B., Ning, C., He, S., Yang, H., Mao, Y., et al. (2022c). Characterization of immune features and immunotherapy response in subtypes of hepatocellular carcinoma based on mitophagy. Front. Immunol. 13, 966167. doi:10.3389/fimmu.2022.966167
Wang, Y., Song, X., Li, Z., Liu, N., Yan, Y., Li, T., et al. (2020a). MicroRNA-103 protects coronary artery endothelial cells against H(2)O(2)-induced oxidative stress via BNIP3-mediated end-stage autophagy and antipyroptosis pathways. Oxid. Med. Cell Longev. 2020, 8351342. doi:10.1155/2020/8351342
Wang, Y., Zheng, Z. J., Jia, Y. J., Yang, Y. L., and Xue, Y. M. (2018). Role of p53/miR-155-5p/sirt1 loop in renal tubular injury of diabetic kidney disease. J. Transl. Med. 16, 146. doi:10.1186/s12967-018-1486-7
Wei, D. M., Jiang, M. T., Lin, P., Yang, H., Dang, Y. W., Yu, Q., et al. (2019). Potential ceRNA networks involved in autophagy suppression of pancreatic cancer caused by chloroquine diphosphate: a study based on differentially-expressed circRNAs, lncRNAs, miRNAs and mRNAs. Int. J. Oncol. 54, 600–626. doi:10.3892/ijo.2018.4660
Wu, J., Gu, X., Zhang, J., Mi, Z., He, Z., Dong, Y., et al. (2022). 4-OI protects MIN6 cells from oxidative stress injury by reducing LDHA-mediated ROS generation. Biomolecules 12, 1236. doi:10.3390/biom12091236
Xia, L., Xu, Z., Zhou, X., Bergmann, F., Grabe, N., Büchler, M. W., et al. (2020). Impaired autophagy increases susceptibility to endotoxin-induced chronic pancreatitis. Cell Death Dis. 11, 889. doi:10.1038/s41419-020-03050-3
Xing, Q. C., Liu, X., Li, W., Chen, Y. Z., and Chen, J. (2020). Sangguayin preparation prevents palmitate-induced apoptosis by suppressing endoplasmic reticulum stress and autophagy in db/db mice and MIN6 pancreatic β-cells. Chin. J. Nat. Med. 18, 472–480. doi:10.1016/s1875-5364(20)30054-6
Xu, J., Wang, L., Zhang, L., Zheng, F., Wang, F., Leng, J., et al. (2021). Mono-2-ethylhexyl phthalate drives progression of PINK1-parkin-mediated mitophagy via increasing mitochondrial ROS to exacerbate cytotoxicity. Redox Biol. 38, 101776. doi:10.1016/j.redox.2020.101776
Xue, W. J., He, C. F., Zhou, R. Y., Xu, X. D., Xiang, L. X., Wang, J. T., et al. (2022). High glucose and palmitic acid induces neuronal senescence by NRSF/REST elevation and the subsequent mTOR-related autophagy suppression. Mol. brain 15, 61. doi:10.1186/s13041-022-00947-2
Yan, J. N., Liu, X. Q., Meng, X. L., Ren, K. l., Wu, X. M., Zhang, H., et al. (2023). Effects of Rehmanniae Radix and Rehmanniae Radix Praeparata on proteomics and autophagy in mice with type 2 diabetes mellitus induced by high-fat diet coupled with streptozotocin. Zhongguo Zhong yao za zhi = Zhongguo zhongyao zazhi = China J. Chin. materia medica 48, 1535–1545. doi:10.19540/j.cnki.cjcmm.20220901.301
Yang, A., Rajeshkumar, N. V., Wang, X., Yabuuchi, S., Alexander, B. M., Chu, G. C., et al. (2014). Autophagy is critical for pancreatic tumor growth and progression in tumors with p53 alterations. Cancer Discov. 4, 905–913. doi:10.1158/2159-8290.cd-14-0362
Yang, M., Chen, P., Liu, J., Zhu, S., Kroemer, G., Klionsky, D. J., et al. (2019). Clockophagy is a novel selective autophagy process favoring ferroptosis. Sci. Adv. 5, eaaw2238. doi:10.1126/sciadv.aaw2238
Yang, M., Zhang, D., Li, Y., and Xin, Y. (2020). Maternal protein restriction increases autophagy in the pancreas of newborn rats. J. Nutr. Sci. vitaminology 66, 168–175. doi:10.3177/jnsv.66.168
Yasunaga, M., Oumi, N., Osaki, M., Kazuki, Y., Nakanishi, T., Oshimura, M., et al. (2011). Establishment and characterization of a transgenic mouse model for in vivo imaging of Bmp4 expression in the pancreas. PloS one 6, e24956. doi:10.1371/journal.pone.0024956
Yazıcı, Ö., Kara, M., Boran, T., and Ozhan, G. (2023). The role of endoplasmic reticulum stress in cell injury induced by methimazole on pancreatic cells. Adv. Pharm. Bull. 13, 196–201. doi:10.34172/apb.2023.042
Ye, X., Chen, W., Tu, P., Jia, R., Liu, Y., Li, Y., et al. (2021). Food-derived cyanidin-3-O-glucoside alleviates oxidative stress: evidence from the islet cell line and diabetic db/db mice. Food and Funct. 12, 11599–11610. doi:10.1039/d1fo02385c
Yoo, H. S., Moss, K. O., Cockrum, M. A., Woo, W., and Napoli, J. L. (2023). Energy status regulates levels of the RAR/RXR ligand 9-cis-retinoic acid in mammalian tissues: glucose reduces its synthesis in β-cells. J. Biol. Chem. 299, 105255. doi:10.1016/j.jbc.2023.105255
Yuan, J., Chheda, C., Piplani, H., Geng, M., Tan, G., Thakur, R., et al. (2021). Pancreas-specific deletion of protein kinase D attenuates inflammation, necrosis, and severity of acute pancreatitis. Biochimica biophysica acta. Mol. basis Dis. 1867, 165987. doi:10.1016/j.bbadis.2020.165987
Yuan, M., Tu, B., Li, H., Pang, H., Zhang, N., Fan, M., et al. (2022). Cancer-associated fibroblasts employ NUFIP1-dependent autophagy to secrete nucleosides and support pancreatic tumor growth. Nat. cancer 3, 945–960. doi:10.1038/s43018-022-00426-6
Zhang, F., Xu, R., Chai, R., Xu, Q., Liu, M., Chen, X., et al. (2020). Deubiquitinase inhibitor b-AP15 attenuated LPS-induced inflammation via inhibiting ERK1/2, JNK, and NF-kappa B. Front. Mol. Biosci. 7, 49. doi:10.3389/fmolb.2020.00049
Zhang, X., Jin, L., Tian, Z., Wang, J., Yang, Y., Liu, J., et al. (2019). Nitric oxide inhibits autophagy and promotes apoptosis in hepatocellular carcinoma. Cancer Sci. 110, 1054–1063. doi:10.1111/cas.13945
Zhao, Y., Feng, X., Li, B., Sha, J., Wang, C., Yang, T., et al. (2020). Dexmedetomidine protects against lipopolysaccharide-induced acute kidney injury by enhancing autophagy through inhibition of the PI3K/AKT/mTOR pathway. Front. Pharmacol. 11, 128. doi:10.3389/fphar.2020.00128
Zheng, Y., Sun, W., Wang, Z., Liu, J., Shan, C., He, C., et al. (2022). Activation of pancreatic acinar FXR protects against pancreatitis via osgin1-mediated restoration of efficient autophagy. Washington, D.C. doi:10.34133/2022/97840819784081
Zhong, Y., Liu, J., Sun, D., Guo, T., Yao, Y., Xia, X., et al. (2022). Dioscin relieves diabetic nephropathy via suppressing oxidative stress and apoptosis, and improving mitochondrial quality and quantity control. Food and Funct. 13, 3660–3673. doi:10.1039/d1fo02733f
Zhou, R., Kusaka, E., Wang, Y., Zhang, J., Webb, A., and Carrico-Moniz, D. (2022). Isoprenylated coumarin exhibits anti-proliferative effects in pancreatic cancer cells under nutrient starvation by inhibiting autophagy. Anticancer Res. 42, 2835–2845. doi:10.21873/anticanres.15765
Zhou, X., Xie, L., Bergmann, F., Endris, V., Strobel, O., Büchler, M. W., et al. (2017b). The bile acid receptor FXR attenuates acinar cell autophagy in chronic pancreatitis. Cell death Discov. 3, 17027. doi:10.1038/cddiscovery.2017.27
Zhou, X., Xie, L., Xia, L., Bergmann, F., Büchler, M. W., Kroemer, G., et al. (2017a). RIP3 attenuates the pancreatic damage induced by deletion of ATG7. Cell Death Dis. 8, e2918. doi:10.1038/cddis.2017.313
Zhu, H., Bhatt, B., Sivaprakasam, S., Cai, Y., Liu, S., Kodeboyina, S. K., et al. (2019). Ufbp1 promotes plasma cell development and ER expansion by modulating distinct branches of UPR. Nat. Commun. 10, 1084. doi:10.1038/s41467-019-08908-5
Zhu, X., Hu, X., Lou, J., Li, J., Xu, Y., Yu, G., et al. (2021a). Liraglutide, a TFEB-mediated autophagy agonist, promotes the viability of random-pattern skin flaps. Oxid. Med. Cell Longev. 2021, 6610603. doi:10.1155/2021/6610603
Zhu, X. G., Chudnovskiy, A., Baudrier, L., Prizer, B., Liu, Y., Ostendorf, B. N., et al. (2021b). Functional genomics in vivo reveal metabolic dependencies of pancreatic cancer cells. Cell metab. 33, 211–221.e216. doi:10.1016/j.cmet.2020.10.017
Glossary
Keywords: autophagy, pancreas, zymophagy, organelle homeostasis, genetic studies
Citation: Zhou Z, Zhang P, Li J, Yao J, Jiang Y, Wan M, Tang W and Liu L (2024) Autophagy and the pancreas: Healthy and disease states. Front. Cell Dev. Biol. 12:1460616. doi: 10.3389/fcell.2024.1460616
Received: 06 July 2024; Accepted: 12 September 2024;
Published: 24 September 2024.
Edited by:
Elaine Maria Souza-Fagundes, Federal University of Minas Gerais, BrazilReviewed by:
Cristina Moraes Junta, Faculdade Santa Casa BH, BrazilSung Min Son, University of Cambridge, United Kingdom
Copyright © 2024 Zhou, Zhang, Li, Yao, Jiang, Wan, Tang and Liu. This is an open-access article distributed under the terms of the Creative Commons Attribution License (CC BY). The use, distribution or reproduction in other forums is permitted, provided the original author(s) and the copyright owner(s) are credited and that the original publication in this journal is cited, in accordance with accepted academic practice. No use, distribution or reproduction is permitted which does not comply with these terms.
*Correspondence: Ling Liu, bmV1cm9sb2d5bGl1bGluZ0AxNjMuY29t; Wenfu Tang, dGFuZ3dmQHNjdS5lZHUuY24=
†These authors have contributed equally to this work and share first authorship.