- 1Department of Orthopedics, The Second Hospital of Hebei Medical University, Shijiazhuang, Hebei, China
- 2Hebei Medical University-National University of Ireland Galway Stem Cell Research Center, Hebei Medical University, Shijiazhuang, Hebei, China
- 3Hebei Research Center for Stem Cell Medical Translational Engineering, Hebei Medical University, Shijiazhuang, Hebei, China
- 4Department of Oral Medicine, The Second Hospital of Hebei Medical University, Shijiazhuang, Hebei, China
- 5Hebei Key Laboratory of Rare Disease, Shijiazhuang, Hebei, China
Bone diseases such as osteoporosis and osteoarthritis have become important human health problems, requiring a deeper understanding of the pathogenesis of related diseases and the development of more effective treatments. Bone organoids are three-dimensional tissue masses that are useful for drug screening, regenerative medicine, and disease modeling because they may mimic the structure and physiological activities of organs. Here, we describe various potential methods for culturing bone-related organoids from different stem cells, detailing the construction processes and highlighting the main applications of these bone organoid models. The application of bone organoids in different skeletal diseases is highlighted, and current and promising bone organoids for drug screening and regenerative medicine as well as the latest technological advancements in bone organoids are discussed, while the future development of bone organoids is discussed. Looking forward, it will provide a reference for constructing bone organoids with more complete structures and functions and applying them to biomedical research.
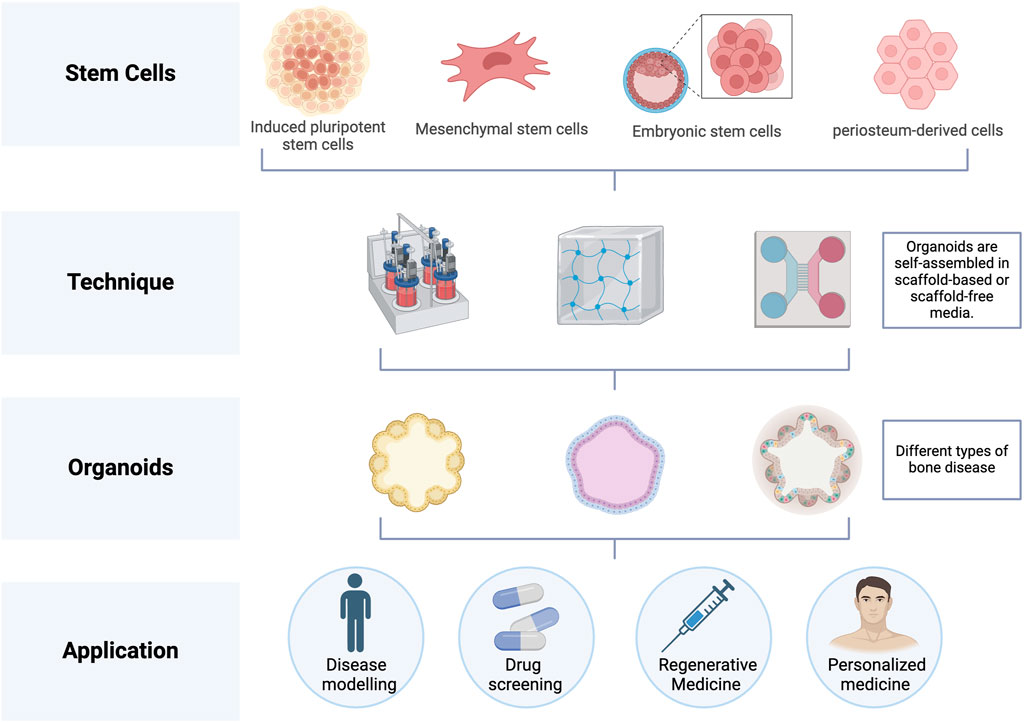
GRAPHICAL ABSTRACT | The graphical abstract was created with BioRender (www.biorender.com).
1 Introduction
Bone diseases have been affecting tens of millions of individuals around the world, especially diseases with long recovery periods such as osteoporosis, osteoarthritis (OA) and bone-related cancers, which have brought a huge economic burden (Xu et al., 2018). As the elderly population grows, the incidence of these diseases increases year by year, which has a serious impact on patients’ quality of life and physical and mental health (Bouvard et al., 2021; Hunter et al., 2020). On the other hand, the pathophysiology of bone disorders is still not fully understood. Preclinical bone research currently relies heavily on animal models and two-dimensional mammalian cell cultures. However, traditional two-dimensional (2D) cell culture models have many limitations, such as the lack of interactions between cells and cells and matrix in vivo (Duval et al., 2017), and the inability to accurately simulate the physiological microenvironment in vivo (Lin et al., 2019). Although animal models more closely mimic the natural biological environment, their establishment is complex, time-consuming, and labor-intensive. Additionally, physiological differences between animals and humans pose challenges to clinical translation (Samvelyan et al., 2020). In addition, bone defects are also a clinical problem. Bone defects caused by trauma, infection, tumors, etc. require bone transplantation or artificial bone materials to repair (Dimitriou et al., 2011). However, these methods face challenges such as limited availability of sources, risk of infection, immune rejection, and inadequate healing. Therefore, to overcome the limitations of traditional research methods, people began to explore more advanced research technologies, such as in vitro three-dimensional cell culture systems. This in vitro three-dimensional (3D) system can more accurately simulate the interaction between cells and matrix in the body, provide a research platform that is closer to the real biological in vivo environment, and is expected to become an effective alternative to bridge the gap between traditional two-dimensional cell culture and animal models (Kamm et al., 2018).
Bone organoids are 3D self-renewing and self-organizing micro-bone tissues grown in vitro from progenitor cells (such as osteoblast progenitors) or stem cells (including bone stem cells, embryonic stem cells, etc.), and can also incorporate differentiated cells like osteoclasts to mimic bone remodeling (Fatehullah et al., 2016). They can simulate the complexity of bone tissue and its remodeling process in a high-fidelity and controllable way and are designed to simulate the effects of microgravity and degeneration on trabecular bone (Park et al., 2021; Iordachescu et al., 2021). Various biocompatible materials, including Matrigel and synthetic alternative hydrogels, are utilized in the production of bone organoids to support their self-organization. Unlike traditional bone tissue engineering, the construction of bone organoids relies on the differentiation and self-organizing capabilities of stem cells. It uses a series of methods such as bioactive materials and bioreactors to guide cells to gradually differentiate and self-organize under the influence of microenvironmental signals, assembling into bone microtissues in vitro rather than inducing stem cell differentiation and achieving bone regeneration through a combination of seed cells, scaffold materials, and growth factors (Yin et al., 2016). By mimicking the in vivo development of bone tissue, bone organoids not only offer novel strategies for bone defect repair but also more accurately replicate the structure and function of bone. This provides a valuable research platform for studying bone diseases, testing drugs, and advancing basic orthopedic research. Latest research shows that researchers have successfully developed self-assembled human skeletal organoids that can be used for disease modeling and drug testing and display spontaneous polarization of cartilage and bone components (Abraham et al., 2021). From a materials biology perspective, hydrogels have been studied as materials for building bone organ tissue (Wu et al., 2023). Additionally, dental pulp stem cells have been investigated as a potential vascular source that could improve the survival of bone marrow stem cells within bone organoids (Li et al., 2023). The complexity of bone organoids and the heterogeneity of osteogenic stem/progenitor cells pose challenges for constructing organisms that model the skeletal system (Qing et al., 2023). To guide future research in this field, current efforts focus on evaluating skeletal stem cells, environmental variables, and potential organoid culture media candidates (Qing et al., 2023). Bone has inherent regenerative ability, and bone organoids provide a platform for studying bone regeneration technology (Zhao et al., 2023). The construction and application of bone organoids show broad prospects in organ development, drug screening and mechanism research (Chen et al., 2022a). This review focuses on recent advances in bone organoids, focusing on their applications in different skeletal diseases, and then describes the importance of bone organoids in the field of regenerative medicine. Finally, some perspectives and perspectives in bone organoid development are discussed.
2 Generation of bone organoids
Bone organoids are 3D, self-renewing, and self-organizing miniaturized bone tissues derived from stem cells or progenitor cells, exhibiting biomimetic spatial properties. They are developed using bioactive materials (Fatehullah et al., 2016). A range of biocompatible materials, including hydrogels, is used to support the self-organization of bone tissue, facilitating the creation of biomimetic structures. Establishing bone organoids involves several key steps (Figure 1). First, the appropriate cell source must be selected. Next, biomaterials are introduced as a supportive matrix to facilitate the growth and differentiation of the cells into 3D organoids. Finally, specific construction techniques are applied to shape the organoids into 3D spherical structures.
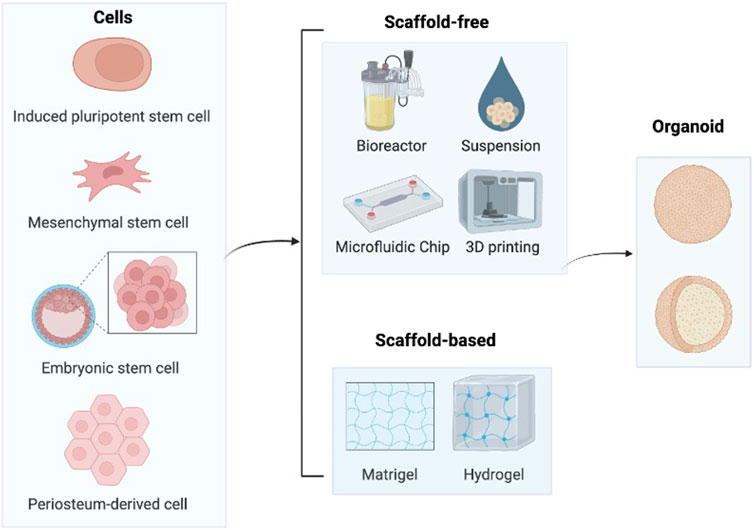
Figure 1. Construction process of bone organoids. In order to cultivate cartilage organoids, stem cells such as iPSCs, MSCs, ESCs, and hPDCs are primarily utilized. After that, these cells self-assemble to create organoids in medium with or without scaffolds. Image created with BioRender (www.biorender.com).
2.1 Stem cell model
Stem cell models are essential tools in biomedical research and regenerative medicine, providing critical insights into human development and disease mechanisms. Organoids, three-dimensional structures derived from various stem cell types, serve as valuable models for these studies. They can be generated from human induced pluripotent stem cells (hiPSCs), which are adult cells reprogrammed to a pluripotent state capable of differentiating into any cell type; human mesenchymal stem cells (hMSCs), which can differentiate into bone, cartilage, and fat cells; human embryonic stem cells (hESCs), pluripotent cells derived from early-stage embryos; and human periosteum-derived cells (hPDCs), which are obtained from the periosteum and are notable for their potential in bone and cartilage regeneration. These diverse stem cell origins enable the modeling of complex tissues and the exploration of cellular behaviors in a controlled laboratory setting.
2.2 Induced pluripotent stem cell
iPSCs are populations reprogrammed from fibroblasts with the potential to differentiate into a wide variety of cell types. In 2018, new discoveries were made in bone tissue engineering, and iPSC-derived organ tissues have great potential to treat fractures and bone defects (Perez et al., 2018). Researchers have utilized iPSCs as building blocks for bone implants aiming to promote bone repair by inducing osteoblast differentiation (Perez et al., 2018), emphasizing the importance of iPSC-based approaches in addressing delayed fracture healing and segmental bone defects. Connor et al. (2020) created osteochondral organoids using mouse induced pluripotent stem cells to study cartilage-bone interactions, providing insights for modeling joint diseases such as osteoarthritis (Connor et al., 2020). The development of iPSCs-derived hematopoietic organoids and hiPSCs-derived bone organoids in 2022 has shown promise in creating complex bone organoids for disease modeling and discovery (Frenz-Wiessner et al., 2023; Frenz et al., 2022). In 2022, The ability of iPSCs as cartilage organoid starting cells was validated by the researchers’ effective induction and characterization of iPSCs to develop into cartilage organoids in vitro (Zhong et al., 2022). Additionally, in 2024, researchers discovered that one way to generate bone marrow-like organoids (BMOs) is to use iPSCs to create a complex bone marrow niche to sustain lifelong hematopoiesis (Frenz-Wiessner et al., 2024). This approach has the potential to be a breakthrough in generating sufficient numbers of osteoblasts for a deeper understanding of bone and joint diseases (Huang et al., 2023). IPSCs are now a potent cell source for creating cartilage organoids as a result.
2.3 Mesenchymal stem cell
hMSCs were initially extensively studied in bone tissue engineering and have since been found to express and secrete a range of bioactive factors that play anti-inflammatory and immunoregulatory roles (Fu et al., 2017). Recent research has focused on integrating MSCs into organoid systems for applications in various fields. For example, the use of bone morphogenetic protein-2 loaded scaffolds to construct bone organoids in vivo has been shown to encourage the development of therapeutically beneficial cells, including MSCs (Dai et al., 2023). In 2023, some researchers have provided a protocol for isolating functional MSCs from bone organisms in vivo, providing an alternative to autologous MSCs (Deng et al., 2023). Co-culture of bone marrow MSCs with hematopoietic cells has been shown to support the growth of hematopoietic cells in bone marrow organ tissues, although current methods still have generation limitations (Khan et al., 2023). The latest research in 2024 shows that basement membrane extract enhances the endochondral ossification phenotype of MSCs cartilage tissue (Hinako et al., 2024). Taken together, these findings indicate that MSCs-derived bone organ tissue has great potential for regenerative medicine, disease modeling, and therapeutic applications.
2.4 Embryonic stem cell
ESCs are pluripotent cells capable of indefinite proliferation and differentiation into various lineages in vitro. They are derived from primitive gonads or early mammalian embryos. The study of Xu et al. (2002) confirmed that bone morphogenetic protein 4 (BMP4) can induce the differentiation of human ES cells into trophoblast cells, indicating that the use of specific growth factors can drive stem cells to differentiate into desired cell lines (such as bone cells). Research by Karp et al. (2006) pointed out that when hESCs are cultured in vitro, osteogenesis can be promoted if the embryoid body stage is removed. This finding suggests that by regulating the culture conditions of hESCs, they can be directed to differentiate into specific cell types, such as osteoblasts, which are critical for the development of hESC-derived bone organ tissue. In addition, Demirci et al. (2020) discussed the generation of hematopoietic stem/progenitor cells from human embryonic stem cells and beyond through organoid-induced differentiation. Lauren et al. (2021) successfully constructed craniofacial cartilage organoids by differentiating ESCs became neural crest stem cells, then proceeded with more differentiation and self-organization (Foltz et al., 2024). The strong expression of cartilage marker proteins in cartilage organoids, as revealed by mass spectrometry analysis, provides new insights into the development of craniofacial cartilage and cell signaling. This indicates that by adjusting the culture conditions of human embryonic stem cells, their potential to differentiate into specific cell lineages, such as bone cells, can be enhanced.
2.5 Human periosteum-derived cell
Bone tissues and organs derived from hPDCs have garnered significant attention in regenerative medicine and tissue engineering. Periosteum-derived cells, especially hPDCs, have been shown to have the potential for osteogenic differentiation and bone formation (Park et al., 2021a). These cells are an important source of tissue regeneration that can be extracted from people having surgery for issues relating to their bones (Bolander et al., 2020). In 2021, the development of organoid-based cartilage implants using hPDCs has also achieved promising results in tissue regeneration (Gabriella et al., 2021). These implants exhibit region-specific characteristics like native cartilage. Furthermore, the use of acellular cartilage ECM culture coating in 2022 has been shown to drive rapid and efficient bone organogenesis using hPDCs (Vas et al., 2022). The latest research in 2023 have shown that hPDCs have osteogenic differentiation capacity when exposed to factors such as BMP-9 (Park et al., 2023). This highlights the potential of utilizing hPDCs in bone tissue engineering applications. These studies highlight the great potential of utilizing hPDCs to create functional tissue constructs.
We compared various bone tissue organoid protocols in Table 1.
This table provides a clear overview of the current methodologies in bone organoid research and their respective strengths and weaknesses, offering valuable insights for future developments in the field. To address the future perspectives in the field of bone tissue organoids, it is crucial to focus on several key areas. First, advancing the scalability and reproducibility of organoid protocols will be essential for translating these models from research to clinical applications. This involves refining the techniques for generating organoids from diverse cell sources and optimizing scaffold materials to better mimic the complex microenvironment of human bone tissue. Additionally, enhancing the integration of bone organoids with vascular and immune components will improve their physiological relevance and functionality. Future research should also explore personalized approaches, utilizing patient-specific cells to develop tailored treatments for bone diseases. Moreover, the development of high-throughput screening methods and advanced imaging technologies will facilitate the assessment of drug efficacy and toxicity in bone organoids. By addressing these challenges, bone tissue organoids have the potential to revolutionize bone regeneration and repair, offering innovative solutions for treating bone diseases and injuries more effectively.
3 Tissue explant culture and biomaterials
The use of tissue explant cultures and biomaterials in bone organ research has been a crucial tool for a wide range of studies. In 2020, a new method has been proposed utilizing human trabecular bone explants filled with chitosan/gel/hydroxyapatite biomaterials to determine the osseointegration process in vitro and in vivo (Przekora et al., 2021). This study highlights the potential of tissue explant cultures to assess bone implant biocompatibility while avoiding the need for animal testing. Meanwhile, a proof-of-concept study of trauma-induced in vitro tissue survival was conducted using a muscle biomaterial-based osteogenic organoid system (He et al., 2020). To advance bone tissue engineering applications, this study examined tissue behavior and cell survival using a novel in vitro biomaterial organoid bioreactor system based on skeletal muscle tissue. In 2023, the regenerative potential of injectable hydrogels in inhibiting catabolic protein expression and promoting human nucleus pulposus cell matrix expression was studied in a tissue explant culture model (Cherif et al., 2023). This study focuses on the regeneration of naturally degenerated human intervertebral discs using a loaded organ culture model. Additionally, Osteoarthritis Models: From Animals to Tissue Engineering (2023) discusses the differentiation of bone organ tissue from stem or progenitor cells through appropriate induction, emphasizing the importance of biomaterial-based 3D microstructures in tissue engineering (Dou et al., 2023). In bioprinting for bone tissue engineering, the utilization of different types of biomaterials, cells, and growth factors has also been explored (Yazdanpanah et al., 2022). In summary, the combination of tissue explant culture and biomaterials holds great promise for advancing bone organ development and regenerative medicine research (Figure 2).
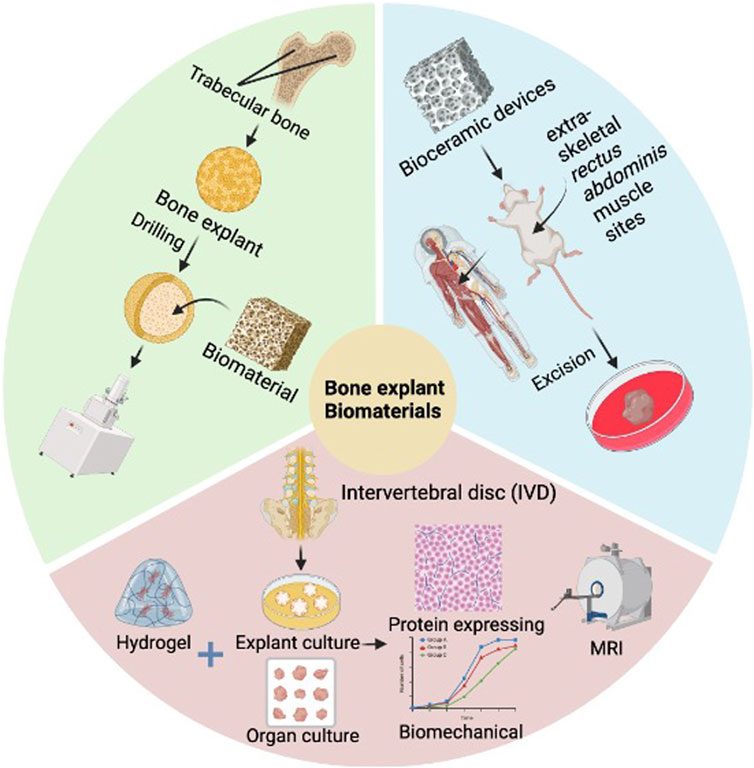
Figure 2. Application of tissue explant culture and biomaterials in bone diseases. This figure shows trabecular bone explants filled with biomaterials, osteogenic organoids utilizing muscle biomaterials, and hydrogel injection in an IVD tissue explant culture model. The combination of tissue explant culture and biomaterials provides an important experimental platform and technical means for studying the developmental mechanisms of bone organs, construction of disease models, drug screening and regenerative medicine treatment. Image created with BioRender (www.biorender.com).
Table 2 compares the main advantages and disadvantages of stem cell models and tissue explant culture. Stem cell models have high differentiation potential and scalability and are suitable for disease modeling and drug screening. They can be genetically modified but may lack complex tissue microenvironments. Tissue explant culture maintains the original tissue structure and is closer to in vivo conditions but has a limited lifespan and is not easy to expand. These two comparisons are of great reference value for choosing the most suitable research method.
4 Bone organoid types
Recent advances in the field of organoid research have led to the development of various types of bone organoids (Table 3). Cartilaginous organoids are primarily used to support and protect body structures such as the pinnae and nose. In 2020, researchers successfully used mouse induced pluripotent stem cells to create osteochondral organoids to simulate cartilage-bone interactions (Perez et al., 2018). Myeloid organoids are responsible for hematopoietic and immune functions. Olijnik et al., 2024. proposed a protocol to generate human myeloid organoids from induced pluripotent stem cells that combines multiple hematopoietic and stromal elements for disease modeling and drug discovery (Aude-Anais et al., 2024). Similarly, researchers from Radboudumc and Eindhoven University of Technology successfully intertwined various bone cells to create an organoid capable of independently generating new woven bone (Akiva et al., 2021; Radboudumc, 2021). In addition to woven bone organoids, trabecular bone organoids have also been developed. Through the guidance of osteoblasts to generate mineralized bone tissue and develop a phenotype resembling that of bone lining cells, trabecular bone organoids mimic trabecular bone plants, providing a model for studying bone structure and function (Chen et al., 2022a). The natural fracture healing process involves the formation of a cartilage intermediate (callus), which provides mechanical stability, enhances vascular regeneration, and recruits osteoprogenitor cells to induce bone formation. Researchers designed callus organoids using bone marrow stem cell-loaded hydrogel microspheres for rapid bone regeneration, achieving efficient bone regeneration within 4 weeks and highly outlining the involvement of callus in the endochondral ossification process of diverse cellular compositions and behaviors (Xie et al., 2022). Callus organoids are engineered to replicate the callus that forms during the natural healing process. Researchers demonstrate the development of engineered callus organoid bioassemblies that exhibit predictive long bone healing in vivo (Nilsson Hall et al., 2020).
5 Application of organoids in bone diseases
Bone organoids more accurately replicate physiological and pathological conditions. To date, various ideas and methodologies have demonstrated tremendous potential for creating disease organoid models, and some bone disease organoids have been produced (Figure 3).
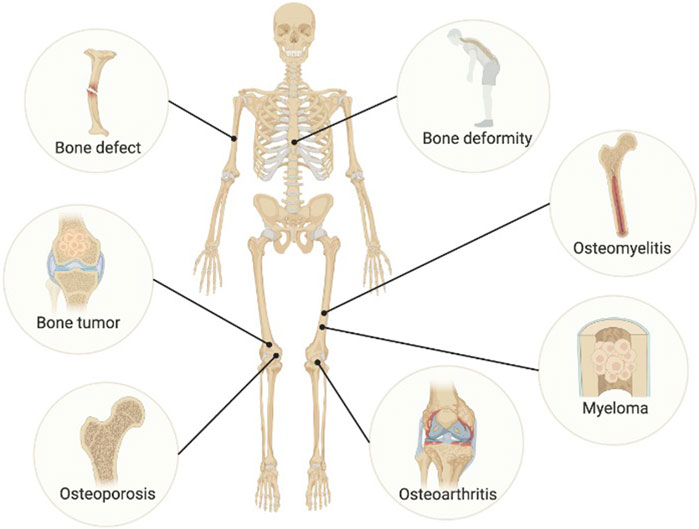
Figure 3. Disease modeling of bone organoids. Image created with BioRender (www.biorender.com).
5.1 Osteoarthritis model
OA is a common degenerative joint disease characterized by pathological changes in both cartilage and bone. Connor et al. (2020) demonstrated the use of mouse induced pluripotent stem cells to create osteochondral organoids to model cartilage-bone interactions, an innovative approach that is promising for studying pathological changes in cartilage and bone associated with OA. Despite the paucity of studies on organoids in the skeletal system, especially cartilage tissue, the 2022 findings on the inhibition of FOX1 and FOXO3 in osteoarthritis progression highlights the potential of cartilaginous organoids as disease models (Yu et al., 2022). Furthermore, trabecular bone organoids have been proposed as models to study the regulation of bone resorption in diseases such as osteoarthritis (Park et al., 2021). Organoid models provide new possibilities for osteoarthritis research.
5.2 Osteoporosis model
Osteoporosis is a prevalent metabolic bone disease marked by bone loss, microstructural degradation, and increased fragility. In vitro osteoporosis models can be more easily and conveniently constructed by enhancing osteoclast activity and proportion in bone organoids, and by simulating reduced mechanical stimulation using microgravity bioreactors and similar techniques. In vitro culture of osteoporotic organoids has been considered a cost-effective and time-saving method for studying bone and joint diseases (Frenz-Wiessner et al., 2023). Additionally, trabecular bone organoids have been developed in 2021 to study the regulation of bone remodeling activities and address issues such as osteoporosis and reduced bone density (Iordachescu et al., 2021). The development of a 2022 “three-in-one” bone repair strategy for osteoporotic fractures involves the use of bone organoids to promote defect repair and address bone loss (Chen et al., 2022b).
5.3 Bone tumor model
Primary or secondary cancers of the human skeletal system and its associated tissues are referred to as bone tumors (Zhou et al., 2021). Research on bone tumors frequently depends on tumor cell lines and animal models. However, culturing tumor cell lines in vitro can lead to the loss of the tumor’s genetic heterogeneity, as they are removed from their original microenvironment. Establishing animal models requires an expensive and lengthy process. In contrast, tumor organoids better maintain the phenotypic and genetic features of the original tumor and can be expanded and cultured in vitro. In addition, tumor organoids can also demonstrate the occurrence and development of tumors. Recent research in 2023 shows that next-generation sequencing and organoid models have been used to study bone cancers, including osteosarcoma, using 3D culture models (Rausch et al., 2023).
5.4 Bone defect model
A bone defect is characterized by a partial or complete loss of bone tissue, typically resulting from trauma, infection, tumor excision, or other bone diseases. In 2019, Tan et al. developed a supramolecular hydrogel containing SDF-1 and BMP-2 that significantly promoted periodontal bone regeneration in rats (Tan et al., 2019). This approach resulted in high bone regeneration rates, demonstrating the potential of organoids in bone defect healing. In 2022, researchers successfully designed callus organoids that can rapidly regenerate bone in a short time, demonstrating the efficiency of this method in large bone defects (Xie et al., 2022). Furthermore, in 2024 Cardier et al. (2024) developed an osteogenic organoid to induce bone formation in patients with congenital tibial pseudarthrosis, demonstrating the potential of organoids to promote osteogenesis in challenging cases. These studies demonstrate the potential of organoids to enhance bone healing through various mechanisms, including growth factor release, cell differentiation, and tissue engineering strategies.
5.5 Bone marrow disease models (myeloma, osteomyelitis)
Osteomyelitis is a severe inflammation of the bones and bone marrow, often caused by infections such as Staphylococcus aureus (Hofstee et al., 2020). In the treatment of osteomyelitis, bone organoids can be used as a bioactive material to repair and regenerate damaged bone tissue. Bone organoids can also provide a suitable microenvironment that helps attract and direct the body’s own stem cells or other repair cells to promote the development and maintenance of bone tissue. Recent research has focused on developing advanced models to study the pathological mechanisms of osteomyelitis. One of the latest models is the 3D osteomyelitis model, which involves culturing bone or bone mimics with host cells to simulate the disease process (Hofstee et al., 2020).
Myeloma is a blood tumor that originates from plasma cells in the bone marrow and manifests as bone destruction, anemia, hypercalcemia, and kidney damage. Recent research has focused on developing 3D bone organoids to study myeloma bone disease (MBD) and potential treatment options. Visconti et al. (2021) aimed to create a well-characterized 3D bone organoid model for this purpose. Multiple myeloma is a hematologic malignancy primarily found in the bone marrow microenvironment. Human myeloid organoids have been used to model cancer-induced perturbations, demonstrating their utility in understanding disease (Azar et al., 2021). Patient-derived 3D models of multiple myeloma have also been developed for personalized medicine in 2022, using bone marrow organoids to culture patients’ malignant cells (Lourenço et al., 2022). Overall, the combination of advanced bone marrow disease models and bone organoids furthers our understanding of bone infection and may provide more effective treatment options for patients.
5.6 Bone deformity model
Bone deformity refers to the abnormal development of bones in form or structure, potentially resulting in irregular shapes or functional impairments. Bone organoids can be employed to repair bone defects and deformities caused by trauma, congenital abnormalities, or other factors. By implanting bone organoids into the affected area, the growth and repair of new bone tissue can be promoted, thereby improving the form and function of bone deformities. It can provide patients with personalized and effective treatment plans and provide new ways for research and understanding of diseases.
6 Drug screening
Most medications undergo extensive research, including both in vivo and in vitro testing, before gaining clinical approval. This process also applies to drugs used in the treatment of bone diseases, such as those with angiogenic, anti-inflammatory, antiresorptive, and bone-growth-promoting properties. However, in the traditional drug development methods, there are major shortcomings such as long organ toxicity assessment period and high cost (Horvath et al., 2016). The use of organoids for drug testing can, on the one hand, avoid errors caused by the huge biological differences between experimental animals and humans; on the other hand, organoids can enable high-throughput screening of drugs due to their availability and scalability. One of the challenges of utilizing organoids for drug screening is the need to create large numbers of homogeneous organoids suitable for high-throughput screening. Technologies like immersion bioprinting have been developed to overcome this limitation, allowing for the generation of tissue organoids in multiwell plates and thereby increasing the throughput of screening processes (Maloney et al., 2020). In addition, advances in patient-derived organoid culture platforms have optimized conditions for drug screening, allowing organoids to reach the optimal stage of drug testing in a short period of time (Nie et al., 2021). Self-assembled human skeletal organoids derived from bone and cartilage tissue have shown spontaneous polarization of their components, providing valuable models for disease modeling and drug testing (Abraham et al., 2021). In conclusion, bone organoids have important application potential in drug screening. By simulating the structure and function of bone tissue in vivo, bone organoids can give a more accurate in vitro research platform for evaluating the therapeutic efficacy and toxicity of drug candidates for bone-related diseases.
7 Regenerative medicine
Clinically, large-area bone defects that cannot heal on their own are typically addressed through autologous bone grafting, allogeneic bone grafting, or the use of artificial bone materials. However, autologous bone transplantation has limitations, including a restricted supply of material and the need for additional surgeries. Allogeneic bone transplantation carries risks such as disease transmission and immune rejection. Meanwhile, artificial bone materials like bioceramics and bone cement often lack osteoinductive properties and have issues with degradation rates. Problems such as mismatch with bone regeneration (Crispim and Ito, 2021). Bone organoids have become a potential solution for bone regeneration and repair, providing prospects for construction and application in various research fields such as organ development, drug screening, and mechanism research (Chen et al., 2022a). Research has demonstrated that callus organoids can be successfully engineered to promote quick and efficient bone repair, demonstrating the potential of bone organoids in tissue engineering regenerative medicine research (Xie et al., 2022). In addition, the application of organoids in skeletal disease research has shown promising results, highlighting the status and prospects of using organoids to understand and treat skeletal diseases (Wu et al., 2023a). Strategies targeting the microenvironment have also been proposed to guide advanced bone regeneration, emphasizing the importance of considering the surrounding environment in promoting effective bone repair (Hao et al., 2023). The aforementioned research indicates that bone organoids hold significant promise for use in OA in vitro modeling and regenerative medicine.
8 Latest technological advancements
Recent technological advances in bone organoids encompass biomaterial-based 3D printing, advanced imaging technologies, and innovations in bone organoid chips. 3D printing technology enables precise control over the shape and structure of bone organoids, facilitating personalized customization and enhancing the efficiency and success rate of bone regeneration. In 2021, Researchers have explored the application of 3D printed bone tissue engineering scaffolds in the field of stem cells (Su et al., 2021). They discussed the advantages of 3D printing technology over traditional methods and identified some limitations and future research directions in the field. In the study of bone organoids, the use of advanced imaging techniques is critical to understanding their structure, function, and growth processes. In recent years, various advanced imaging technologies such as MRI (magnetic resonance imaging), CT (computed tomography), micro-CT (micro-computed tomography), PET (positron emission tomography), optical imaging technologies (such as fluorescence microscopy, co-focusing microscope) and so on are widely used in the study of bone organoids. Bone organoid chip is a miniaturized device used to simulate the microenvironment and function of human bone tissue. On a chip, bone organoids can introduce blood perfusion and joint synovial fluid stimulation through microfluidic technology, and accurately simulate the biomechanical properties of bone tissue. In addition, integrating different organoids on a chip can also establish musculoskeletal systems or even more complex systems for studying multi-system diseases or testing drug toxicity (Skardal et al., 2017). By using bone organoid chips, researchers can simulate the physiological and pathological states of bone tissue in the body, such as bone cell proliferation, differentiation, bone matrix deposition, and interaction with the immune system (Figure 4). This technology helps to gain a deeper understanding of bone biological processes, study the mechanisms of bone-related diseases, evaluate the efficacy and toxicity of drugs, and develop personalized treatments. Advances in these technologies will promote the application of bone organoids in bone disease treatment and regenerative medicine and provide more effective solutions for clinical treatment.
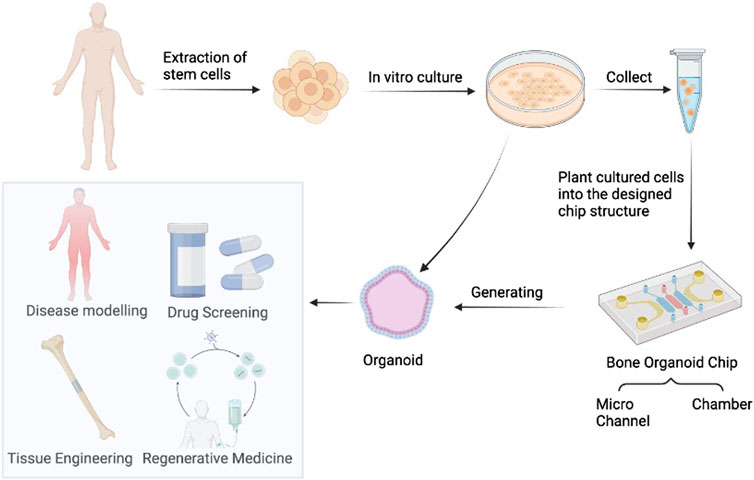
Figure 4. Bone Organoids and organoids-on-chips. These generated organoids have potential for drug screening, disease modeling, regenerative medicine, and tissue engineering. Image created with BioRender (www.biorender.com).
While these technological advancements have significantly improved the development and application of bone organoids, several critical areas require further exploration to move beyond the current state of the art:
Integration of Multimodal Approaches: The integration of 3D printing, advanced imaging, and organoid chip technology into a single, cohesive platform is still in its infancy. Future research should focus on combining these technologies to create more comprehensive and realistic models of bone tissue that can better simulate in vivo conditions.
Real-Time Monitoring and Dynamic Modelling: Current imaging techniques are often limited to static snapshots, which do not fully capture the dynamic processes occurring within bone organoids. Developing real-time monitoring systems and dynamic modeling approaches will be crucial for advancing our understanding of bone biology and disease mechanisms.
Scalability and Clinical Translation: While 3D printing and organoid chips offer promising avenues for personalized medicine, scaling these technologies for widespread clinical use remains a challenge. Addressing issues related to scalability, reproducibility, and cost-effectiveness will be essential for translating these innovations from the lab to the clinic.
Multiscale and Multifactorial Models: Existing organoid models often lack the complexity needed to study multi-system interactions, such as the interplay between bone tissue and immune responses. Developing multiscale and multifactorial models that can incorporate these interactions will enhance the relevance of bone organoids for studying systemic diseases and evaluating therapeutic interventions.
By addressing these challenges, future research can push the boundaries of current bone organoid technology, leading to more effective and clinically relevant solutions for bone regeneration and disease treatment.
9 Challenges and limitations
As a potential 3D in vitro culture model, bone organoids offer advantages that traditional 2D cell cultures and animal models lack. They hold significant promise in disease modeling, drug screening, regenerative medicine, and basic research. However, despite their potential, bone organoids still face some limitations. First, the cell types used in cultivating bone organoids are relatively simple, with most being limited to replicating specific bone functions, such as osteogenesis or bone marrow hematopoiesis. Bone tissue has a highly complex milieu that includes the circulatory system, extracellular matrix, and many different cell types. The complexity of this environment is challenging for existing organoid models to fully replicate, which has certain limits when it comes to replicating real bone tissue. To fully replicate the complexity of bone tissue, further research and advancements in cell co-culture techniques, 3D scaffolds materials, and bioreactor design are essential. Scaffold-free methods used to generate bone micro-organoids can be considered an extension of 3D printing technology, offering significant potential for the application of multifunctional bone tissue. Vascularization of bone organoids is a major challenge. Due to the lack of a vascular network, bone organoids cannot obtain sufficient nutrients through diffusion of blood and tissue fluid for cells beyond 200 μm from the capillaries (He et al., 2022). Due to the lack of a vascular network, the nutrient and waste exchange of organoids mainly relies on diffusion, which is inefficient in larger organoids and may lead to hypoxia and death of internal cells. Researchers are exploring a variety of technologies including microfluidic systems, genetically engineered vascularization, and bioprinting. In addition, co-culturing of endothelial cells or stimulating angiogenesis using VEGF, FGF, etc. may be expected to solve this problem.
Secondly, the construction of bone organoids lacks standardization. The process of producing organoids is complex and involves numerous variables, such as the source of cells, the components of the culture medium, and the materials used to create the 3D scaffold. The homogeneity and repeatability of organoids across laboratories are hampered by the difficulties in controlling these characteristics. Improving the repeatability and dependability of organoid models requires the establishment of consistent policies and norms. Organoids have demonstrated significant potential in basic research, but their translation into clinical therapies is still hindered by several challenges. This covers the immunological reaction and biocompatibility discrepancies between organoids and human tissues as well as methods for guaranteeing the uniformity and caliber of organoids produced on a wide scale. Innovative thinking and interdisciplinary cooperation are needed to solve these issues.
In addition to the difficulties faced in the formation of bone organoids, effective treatments need to be explored and identified to address bone-related diseases, especially bone-related cancers. Combined with CRISPR-Cas9 gene editing technology, disease-related genes can be screened, knocked out, or knocked in bone organoids, which can help to reproduce bone diseases, explore pathogenesis, and conduct gene-targeted therapy (Hendriks et al., 2020). Establishing a patient-derived bone tumor organoid biobank may also promote research on the effectiveness and safety of anti-tumor drugs (Jacob et al., 2020). As organoid technology continues to mature, bone organoids are expected to play a greater role in related fields.
Although bone organoids still encounter numerous challenges and limitations in their development and application, they exhibit significant potential in the treatment of bone diseases and regenerative medicine. With ongoing research and the advancement of new biomaterials like hydrogels, bone organoids are anticipated to surpass traditional matrix gels, enabling the more effective generation and maintenance of complex, multi-cellular bone organoids. Through continuous technological innovation and multidisciplinary cooperation, it is expected that these obstacles will be overcome in the future, and the research and application level of bone organoids will be further improved, providing more reliable and efficient tools for the diagnosis and treatment of bone diseases.
10 Conclusion and prospective
The field of bone organoids is rapidly evolving, with researchers exploring a variety of methods to generate these complex structures. The development and application of bone organoids hold great promise in advancing the field of bone regeneration and repair, offering innovative solutions for bone diseases and injuries. Further research in this area is critical, particularly to address existing limitations and to advance the clinical applications of bone organoids for enhancing bone repair and regeneration. It is essential to focus on improving the scalability of organoid technology and ensuring that the models accurately mimic human bone tissue. Additionally, exploring how these organoids can be effectively utilized for treating bone diseases is necessary.
In conclusion, bone organoids not only hold significant promise for advancing basic research but are also expected to bring revolutionary changes to future clinical applications. Our review highlights the latest advancements and identifies key areas for future research, emphasizing the transformative potential of bone organoids in addressing bone diseases and advancing the field of bone regeneration and repair. Continued innovation and interdisciplinary collaboration will be crucial in realizing the full potential of this technology.
Author contributions
YK: Writing–original draft. YY: Writing–review and editing, Data curation. YH: Writing–review and editing, Methodology. YW: Writing–review and editing, Investigation. WL: Writing–review and editing, Investigation. YS: Writing–review and editing, Supervision, Funding acquisition.
Funding
The author(s) declare that financial support was received for the research, authorship, and/or publication of this article. The work was supported by grants from Hebei Province Key R&D Plan Project (No. 22377752D), Medical Science Research Project of Hebei Province (No. 20230486), Hebei Provincial Government-funded Clinical Talent Training Project in 2024 (No. ZF2024052), and Scientific Research Fund of the Second Hospital of Hebei Medical University (No. 2HC202210).
Conflict of interest
The authors declare that the research was conducted in the absence of any commercial or financial relationships that could be construed as a potential conflict of interest.
Publisher’s note
All claims expressed in this article are solely those of the authors and do not necessarily represent those of their affiliated organizations, or those of the publisher, the editors and the reviewers. Any product that may be evaluated in this article, or claim that may be made by its manufacturer, is not guaranteed or endorsed by the publisher.
Abbreviations
2D, Two-dimensional; 3D, Three-dimensional; hiPSCs, Human induced pluripotent stem cells; hMSCs, Human mesenchymal stem cells; hESCs, Human embryonic stem cells; hPDCs, Human periosteum-derived cells; BMOs, Bone myeloid organoids; BMP4, Bone morphogenetic protein 4; IVD, Intervertebral disc; OA, Osteoarthritis; MBD, Myeloma bone disease; MRI, Magnetic resonance imaging; CT, Computed tomography; Micro-CT, micro-computed tomography; PET, Positron emission tomography.
References
Abraham, D. M., Herman, C., Witek, L., Cronstein, B. N., Flores, R. L., and Coelho, P. G. (2021). Self-assembling human skeletal organoids for disease modeling and drug testing. J. Biomed. Mater. Res. Part B Appl. Biomaterials 110, 871–884. doi:10.1002/jbm.b.34968
Akiva, A., Melke, J., Ansari, S., Liv, N., Meijden, R., Erp, M., et al. (2021). An organoid for woven bone. Adv. Funct. Mater. 31, 2010524. doi:10.1002/adfm.202010524
Aude-Anais, O., Rodriguez-Romera, A., Wong, Z. C., Shen, Y., Reyat, J. S., Jooss, N. J., et al. (2024). Generating human bone marrow organoids for disease modeling and drug discovery. Nat. Protoc. 19, 2117–2146. doi:10.1038/s41596-024-00971-7
Azar, J., Bahmad, H. F., Daher, D., Moubarak, M. M., Hadadeh, O., Monzer, A., et al. (2021). The use of stem cell-derived organoids in disease modeling: an update. Int. J. Mol. Sci. 22, 7667. doi:10.3390/ijms22147667
Bolander, J., Herpelinck, T., and Luyten, F. P. (2020). Periosteum derived cells in skeletal tissue regeneration. Springer eBooks, 101–137. doi:10.1007/978-3-319-08831-0_6
Bouvard, B., Annweiler, C., and Legrand, E. (2021). Osteoporosis in older adults. Jt. Bone Spine 88, 105135. doi:10.1016/j.jbspin.2021.105135
Cardier, J. E., Dylana, D.-S., Wittig, O., Sierra, G., Pulido, J., Moreno, R., et al. (2024). Osteogenic organoid for bone regeneration: healing of bone defect in congenital pseudoarthrosis of the tibia. Int J. Artif. Organs 47, 107–114. doi:10.1177/03913988231220844
Chen, S., Chen, X., Geng, Z., and Su, J. (2022a). The horizon of bone organoid: a perspective on construction and application. Bioact. Mater. 18, 15–25. doi:10.1016/j.bioactmat.2022.01.048
Chen, X., Hu, Y., and Geng, Z. (2022b). Osteoporotic fracture “Three-in-one” bone-repair strategy. Front Endocrinol (Lausanne) Lausanne, Switzerland: Frontiers Media SA 13, 910602. doi:10.26904/rf-142-2800316543
Cherif, H., Li, L., Snuggs, J., Li, X., Sammon, C., Li, J., et al. (2023). Injectable hydrogel induces regeneration of naturally degenerate human intervertebral discs in a loaded organ culture model. Acta biomater. 176, 201–220. doi:10.1016/j.actbio.2023.12.041
Connor, S. K., Katz, D. B., Oswald, S. J., Groneck, L., and Guilak, F. (2020). Formation of osteochondral organoids from murine induced pluripotent stem cells. Tissue Eng. Part A 27, 1099–1109. doi:10.1089/ten.tea.2020.0273
Crispim, J. F., and Ito, K. (2021). De novo neo-hyaline-cartilage from bovine organoids in viscoelastic hydrogels. Acta Biomater. 128, 236–249. doi:10.1016/j.actbio.2021.04.008
Dai, K., Zhang, Q., Deng, S., Yu, Y., Zhu, F., Zhang, S., et al. (2023). A BMP-2–triggered in vivo osteo-organoid for cell therapy. Sci. Adv. 9, eadd1541. doi:10.1126/sciadv.add1541
Demirci, S., Haro-Mora, J. J., Leonard, A., Drysdale, C., Malide, D., Keyvanfar, K., et al. (2020). Definitive hematopoietic stem/progenitor cells from human embryonic stem cells through serum/feeder-free organoid-induced differentiation. Stem Cell Res. Ther. 11, 493. doi:10.1186/s13287-020-02019-5
Deng, S., Zhu, F., Dai, K., Wang, J., and Liu, C. (2023). Harvest of functional mesenchymal stem cells derived from in vivo osteo-organoids. Biomater. Transl. 4, 270–279. doi:10.12336/biomatertransl.2023.04.006
Dimitriou, R., Jones, E., McGonagle, D., and Giannoudis, P. V. (2011). Bone regeneration: current concepts and future directions. BMC Med. 9, 66. doi:10.1186/1741-7015-9-66
Dou, H., Wang, S., Hu, J., Song, J., Zhang, C., Wang, J., et al. (2023). Osteoarthritis models: from animals to tissue engineering. J. Tissue Eng. 14, 20417314231172584. doi:10.1177/20417314231172584
Duval, K., Grover, H., Han, L.-H., Mou, Y., Pegoraro, A. F., Fredberg, J., et al. (2017). Modeling physiological events in 2D vs. 3D cell culture. Physiology 32, 266–277. doi:10.1152/physiol.00036.2016
Fatehullah, A., Tan, S. H., and Barker, N. (2016). Organoids as an in vitro model of human development and disease. Nat. Cell Biol. 18, 246–254. doi:10.1038/ncb3312
Foltz, L., Nagashree, A., Jean-Marc, L., Levy, T., Possemato, A., Ariss, M., et al. (2024). Craniofacial chondrogenesis in organoids from human stem cell-derived neural crest cells. iScience 27, 109585. doi:10.1016/j.isci.2024.109585
Frenz, S., Goek, I., Buser, M., Salewskij, K., Fairley, S., Conca, R., et al. (2022). Generation of human induced pluripotent stem cell-derived bone marrow organoids. Blood 140, 1682–1683. doi:10.1182/blood-2022-166653
Frenz-Wiessner, S., Fairley, S., Buser, M., Goek, I., Kirill, S., Jonsson, G., et al. (2023). Human induced pluripotent stem cell-derived bone marrow organoids to model hematopoietic development. Blood 142, 2708. doi:10.1182/blood-2023-184523
Frenz-Wiessner, S., Fairley, S. D., Buser, M., Goek, I., Salewskij, K., Jonsson, G., et al. (2024). Generation of complex bone marrow organoids from human induced pluripotent stem cells. Nat. Methods 21, 868–881. doi:10.1038/s41592-024-02172-2
Fu, Y., Karbaat, L., Wu, L., Leijten, J., Both, S. K., and Karperien, M. (2017). Trophic effects of mesenchymal stem cells in tissue regeneration. Tissue Eng. Part B Rev. 23, 515–528. doi:10.1089/ten.teb.2016.0365
Gabriella, N. H., Wai, L. T., Andrikopoulos, K. S., Leire, C.-F., Voyiatzis, G. A., Geris, L., et al. (2021). Patterned, organoid-based cartilaginous implants exhibit zone specific functionality forming osteochondral-like tissues in vivo. Biomaterials 273, 120820. doi:10.1016/j.biomaterials.2021.120820
Hao, S., Wang, M., Yin, Z., Jing, Y., Bai, L., and Su, J. (2023). Microenvironment-targeted strategy steers advanced bone regeneration. Mater. Today Bio 22, 100741. doi:10.1016/j.mtbio.2023.100741
He, T., Jörg, H., Chevalier, Y., and Klar, R. M. (2020). Trauma induced tissue survival in vitro with a muscle-biomaterial based osteogenic organoid system: a proof of concept study. BMC Biotechnol. 20, 8. doi:10.1186/s12896-020-0602-y
He, Y., Li, H., Yu, Z., Li, L., Chen, X., Yang, A., et al. (2022). Exosomal let-7f-5p derived from mineralized osteoblasts promotes the angiogenesis of endothelial cells via the DUSP1/Erk1/2 signaling pathway. J. tissue Eng. Regen. Med. 16, 1184–1195. doi:10.1002/term.3358
Hendriks, D., Clevers, H., and Artegiani, B. (2020). CRISPR-cas tools and their application in genetic engineering of human stem cells and organoids. Cell Stem Cell 27, 705–731. doi:10.1016/j.stem.2020.10.014
Hinako, N., Yamasaki, S., Suzuki, N., Suzuki, A., Okamoto, S., Kanematsu, T., et al. (2024). Basement membrane extract potentiates the endochondral ossification phenotype of bone marrow-derived mesenchymal stem cell-based cartilage organoids. Biochem. biophysical Res. Commun. 701, 149583. doi:10.1016/j.bbrc.2024.149583
Hofstee, M. I., Muthukrishnan, G., Atkins, G. J., Riool, M., Thompson, K., Morgenstern, M., et al. (2020). Current concepts of osteomyelitis: from pathologic mechanisms to advanced research methods. Am. J. Pathology 190, 1151–1163. doi:10.1016/j.ajpath.2020.02.007
Horvath, P., Aulner, N., Bickle, M., Davies, A. M., Nery, E. D., Ebner, D., et al. (2016). Screening out irrelevant cell-based models of disease. Nat. Rev. Drug Discov. 15, 751–769. doi:10.1038/nrd.2016.175
Huang, J., Zhang, L., Lu, A., and Liang, C. (2023). Organoids as innovative models for bone and joint diseases. Cells 12, 1590. doi:10.3390/cells12121590
Hunter, D. J., March, L., and Chew, M. (2020). Osteoarthritis in 2020 and beyond: a lancet commission. Lancet 396, 1711–1712. doi:10.1016/S0140-6736(20)32230-3
Iordachescu, A., Hughes, E. A. B., Joseph, S., Hill, E. J., Grover, L. M., and Metcalfe, A. D. (2021). Trabecular bone organoids: a micron-scale “humanised” prototype designed to study the effects of microgravity and degeneration. Microgravity 7, 17. doi:10.1038/s41526-021-00146-8
Jacob, F., Salinas, R. D., Zhang, D. Y., Nguyen, P. T. T., Schnoll, J. G., Wong, S. Z. H., et al. (2020). A patient-derived glioblastoma organoid model and biobank recapitulates inter- and intra-tumoral heterogeneity. Cell 180, 188–204. doi:10.1016/j.cell.2019.11.036
Kamm, R. D., Bashir, R., Arora, N., Dar, R. D., Gillette, M. U., Griffith, L. G., et al. (2018). Perspective: the promise of multi-cellular engineered living systems. Apl. Bioeng. 2, 040901. doi:10.1063/1.5038337
Karp, J. M., Ferreira, L. S., Khademhosseini, A., Kwon, A. H., Yeh, J., and Langer, R. S. (2006). Cultivation of human embryonic stem cells without the embryoid body step enhances osteogenesis in vitro. STEM CELLS 24, 835–843. doi:10.1634/stemcells.2005-0383
Khan, A. O., Rodriguez-Romera, A., Reyat, J. S., Olijnik, A.-A., Colombo, M., Wang, G., et al. (2023). Human bone marrow organoids for disease modeling, discovery, and validation of therapeutic targets in hematologic malignancies. Cancer Discov. 13, 364–385. doi:10.1158/2159-8290.cd-22-0199
Li, A., Sasaki, J.-I., Abe, G. L., Katata, C., Sakai, H., and Imazato, S. (2023). Vascularization of a bone organoid using dental pulp stem cells. Stem cells Int. 2023, 5367887–5367889. doi:10.1155/2023/5367887
Lin, A., Sved Skottvoll, F., Rayner, S., Pedersen-Bjergaard, S., Sullivan, G., Krauss, S., et al. (2019). 3D cell culture models and organ on a chip: meet separation science and mass spectrometry. Electrophoresis 41, 56–64. doi:10.1002/elps.201900170
Lauren, F., Tyler, L., Anthony, P., and Mark, G. (2021). Craniofacial cartilage organoids from human embryonic stem cells via a neural crest cell intermediate. doi:10.1101/2021.05.31.446459
Lourenço, D., Lopes, R., Pestana, C., Queirós, A. C., João, C., and Carneiro, E. A. (2022). Patient-derived multiple myeloma 3D models for personalized medicine—are we there yet? Int. J. Mol. Sci. 23, 12888. doi:10.3390/ijms232112888
Maloney, E., Clark, C., Sivakumar, H., Yoo, K., Aleman, J., Rajan, S. A. P., et al. (2020). Immersion bioprinting of tumor organoids in multi-well plates for increasing chemotherapy screening throughput. Micromachines 11, 208. doi:10.3390/mi11020208
Nie, L., Liu, Y., Che, Y., Li, Y., Wang, W., Jin, J., et al. (2021). Establishment of patient-derived organoid culture platform of mantle cell lymphoma. Blood 138, 3508. doi:10.1182/blood-2021-152994
Nilsson Hall, G., Mendes, L. F., Gklava, C., Geris, L., Luyten, F. P., and Papantoniou, I. (2020). Developmentally engineered callus organoid bioassemblies exhibit predictive in vivo long bone healing. Adv. Sci. Weinh 7, 1902295. doi:10.1002/advs.201902295
Olijnik, A. A., Rodriguez-Romera, A., Wong, Z. C., Shen, Y., Reyat, J. S., Jooss, N. J., et al. (2024). Generating human bone marrow organoids for disease modeling and drug discovery. Nature protocols 19 (7), 2117–2146. doi:10.1038/s41596-024-00971-7
Park, J.-H., Koh, E.-B., Seo, Y.-J., Oh, H.-S., and Byun, J.-H. (2023). BMP-9 improves the osteogenic differentiation ability over BMP-2 through p53 signaling in vitro in human periosteum-derived cells. Int. J. Mol. Sci. 24, 15252. doi:10.3390/ijms242015252
Park, J.-H., Park, S. A., Kang, Y.-H., So, M. H., Koh, E.-B., Hwang, S.-C., et al. (2021a). Zinc sulfate stimulates osteogenic phenotypes in periosteum-derived cells and Co-cultures of periosteum-derived cells and THP-1 cells. Life 11, 410. doi:10.3390/life11050410
Park, Y., Cheong, E., Kwak, J.-G., Carpenter, R., Shim, J.-H., and Lee, J. (2021). Trabecular bone organoid model for studying the regulation of localized bone remodeling. Sci. Adv. 7, eabd6495. doi:10.1126/sciadv.abd6495
Perez, J. R., Kouroupis, D., Li, D. J., Best, T. M., Kaplan, L., and Correa, D. (2018). Tissue engineering and cell-based therapies for fractures and bone defects. Front. Bioeng. Biotechnol. 6, 105. doi:10.3389/fbioe.2018.00105
Przekora, A., Kazimierczak, P., and Wójcik, M. (2021). Ex vivo determination of chitosan/curdlan/hydroxyapatite biomaterial osseointegration with the use of human trabecular bone explant: new method for biocompatibility testing of bone implants reducing animal tests. Mater. Sci. Eng. C 119, 111612. doi:10.1016/j.msec.2020.111612
Qing, J., Guo, Q., Longwei, Lv, Zhang, X., Liu, Y., Heng, B. C., et al. (2023). Organoid culture development for skeletal systems. Tissue Eng. Part B, Rev 29, 545–557. doi:10.1089/ten.teb.2023.0022
Rausch, M., Iqbal, N., Pathak, S., Owston, H. E., and Ganguly, P. (2023). Organoid models and next-generation sequencing for bone marrow and related disorders. Organoids 2, 123–139. doi:10.3390/organoids2030010
Radboudumc (2021). Researchers grow most lifelike bone organoid to date - greatly increasing our understanding of bone formation and bone diseases. Available at: https://www.radboudumc.nl/en/news/2021/researchers-grow-most-lifelike-woven-bone-yet-starting-from-cells. (Accessed August 14, 2024)
Samvelyan, H. J., Hughes, D., Stevens, C., and Staines, K. A. (2020). Models of osteoarthritis: relevance and new insights. Calcif. Tissue Int. 109, 243–256. doi:10.1007/s00223-020-00670-x
Skardal, A., Murphy, S. V., Devarasetty, M., Mead, I., Kang, H.-W., Seol, Y.-J., et al. (2017). Multi-tissue interactions in an integrated three-tissue organ-on-a-chip platform. Sci. Rep. 7, 8837. doi:10.1038/s41598-017-08879-x
Su, X., Wang, T., and Guo, S. (2021). Applications of 3D printed bone tissue engineering scaffolds in the stem cell field. Regen. Ther. 16, 63–72. doi:10.1016/j.reth.2021.01.007
Tan, J., Zhang, M., Hai, Z., Wu, C., Lin, J., Kuang, W., et al. (2019). Sustained release of two bioactive factors from supramolecular hydrogel promotes periodontal bone regeneration. ACS nano 13, 5616–5622. doi:10.1021/acsnano.9b00788
Vas, W. J., Shah, M., Roberts, H. C., and Roberts, S. J. (2022). Decellularised cartilage ECM culture coatings drive rapid and robust chondrogenic differentiation of human periosteal cells. Bioengineering 9, 203. doi:10.3390/bioengineering9050203
Visconti, R. J., Kolaja, K., and Cottrell, J. A. (2021). A functional three-dimensional microphysiological human model of myeloma bone disease. J. Bone Mineral Res. 36, 1914–1930. doi:10.1002/jbmr.4404
Wu, J., Yang, Z., Yang, B., and Huang, H. (2023a). The application of organoids in the research of skeletal diseases: current status and prospects. Stud. Health Technol. Inf. 308, 597–604. doi:10.3233/SHTI230890
Wu, S., Wu, X., Wang, X., and Su, J. (2023). Hydrogels for bone organoid construction: from a materiobiological perspective. J. Mater. Sci. and Technol. 136, 21–31. doi:10.1016/j.jmst.2022.07.008
Xie, C., Liang, R., Ye, J., Peng, Z., Sun, H., Zhu, Q., et al. (2022). High-efficient engineering of osteo-callus organoids for rapid bone regeneration within one month. Biomaterials 288, 121741. doi:10.1016/j.biomaterials.2022.121741
Xu, H., Jiao, Y., Qin, S., Zhao, W., Chu, Q., and Wu, K. (2018). Organoid technology in disease modelling, drug development, personalized treatment and regeneration medicine. Exp. Hematol. and Oncol. 7, 30. doi:10.1186/s40164-018-0122-9
Xu, R.-H., Chen, X., Li, D. S., Li, R., Addicks, G. C., Glennon, C., et al. (2002). BMP4 initiates human embryonic stem cell differentiation to trophoblast. Nat. Biotechnol. 20, 1261–1264. doi:10.1038/nbt761
Yazdanpanah, Z., Johnston, J. D., Cooper, D. M. L., and Chen, X. (2022). 3D bioprinted scaffolds for bone tissue engineering: state-of-the-art and emerging technologies. Front. Bioeng. Biotechnol. 10, 824156. doi:10.3389/fbioe.2022.824156
Yin, X., Mead, B. E., Safaee, H., Langer, R., Karp, J. M., and Levy, O. (2016). Engineering stem cell organoids. Cell Stem Cell 18, 25–38. doi:10.1016/j.stem.2015.12.005
Yu, Y., Wang, J., Li, Y., Chen, Y., and Cui, W. (2022). Cartilaginous organoids: advances, applications, and perspectives. Adv. nanoBiomed Res. 3. doi:10.1002/anbr.202200114
Zhao, D., Qimanguli, S., Li, Y., Tang, Y., and Cui, W. (2023). Bone organoids: recent advances and future challenges. Adv. Healthc. Mater. 13. doi:10.1002/adhm.202302088
Zhong, A.Li, Shang, J., Xiang, S., Li, E. N., Yagi, H., Riewruja, K., et al. (2022). Articular tissue-mimicking organoids derived from mesenchymal stem cells and induced pluripotent stem cells. Organoids 1, 135–148. doi:10.3390/organoids1020011
Keywords: bone diseases, bone organoids, stem cells, regenerative medicine, biomaterials
Citation: Kong Y, Yang Y, Hou Y, Wang Y, Li W and Song Y (2024) Advance in the application of organoids in bone diseases. Front. Cell Dev. Biol. 12:1459891. doi: 10.3389/fcell.2024.1459891
Received: 05 July 2024; Accepted: 20 August 2024;
Published: 03 September 2024.
Edited by:
Yahima Frión-Herrera, University of Padua, ItalyReviewed by:
Md. Shaifur Rahman, Atomic Energy Research Establishment, BangladeshChing-Fang Chang, Cincinnati Children’s Hospital Medical Center, United States
Copyright © 2024 Kong, Yang, Hou, Wang, Li and Song. This is an open-access article distributed under the terms of the Creative Commons Attribution License (CC BY). The use, distribution or reproduction in other forums is permitted, provided the original author(s) and the copyright owner(s) are credited and that the original publication in this journal is cited, in accordance with accepted academic practice. No use, distribution or reproduction is permitted which does not comply with these terms.
*Correspondence: Yongzhou Song, eGlleW9uZ2ZlbmdAaGVibXUuZWR1LmNu