- 1Department of Cardiovascular Physiology, Graduate School of Medicine, Dentistry and Pharmaceutical Sciences, Okayama University, Okayama, Japan
- 2Human Information Systems Laboratories, Kanazawa Institute of Technology, Hakusan, Japan
- 3Department of Applied Bioscience, Kanazawa Institute of Technology, Hakusan, Japan
The contractile apparatus, stress fiber (SF), is connected to the cell adhesion machinery, focal adhesion (FA), at the termini of SF. The SF-FA complex is essential for various mechanical activities of cells, including cell adhesion to the extracellular matrix (ECM), ECM rigidity sensing, and cell migration. This mini-review highlights the importance of SF mechanics in these cellular activities. Actin-crosslinking proteins solidify SFs by attenuating myosin-driven flows of actin and myosin filaments within the SF. In the solidified SFs, viscous slippage between actin filaments in SFs and between the filaments and the surrounding cytosol is reduced, leading to efficient transmission of myosin-generated contractile force along the SFs. Hence, SF solidification via actin crosslinking ensures exertion of a large force to FAs, enabling FA maturation, ECM rigidity sensing and cell migration. We further discuss intracellular mechanisms for tuning crosslinker-modulated SF mechanics and the potential relationship between the aberrance of SF mechanics and pathology including cancer.
Introduction
The interaction between the stress fiber (SF) and the focal adhesion (FA) is crucial for numerous cellular processes, ranging from cellular structural maintenance and adhesion to the extracellular matrix (ECM) to ECM rigidity sensing, cell motility, and differentiation (Totsukawa et al., 1999; Kobayashi and Sokabe, 2010; Plotnikov et al., 2012; Tojkander et al., 2012; Maharam et al., 2015; Gupta et al., 2016; Lee and Kumar, 2020). Structurally, SFs are composed of consecutive, sarcomere-like contractile units (CUs). In each CU, 10 to 30 actin filaments are bundled by actin crosslinking proteins including myosin II, α-actinin, and filamin (Cramer et al., 1997; Pellegrin and Mellor, 2007; Kemp and Brieher, 2018). Myosin II converts the energy of ATP hydrolysis into contractile force (Vale and Milligan, 2000; Kasza and Zallen, 2011). This force is dynamically transmitted along the continuum of SF to its endpoints (Hayakawa et al., 2008). At the termini of SFs, FAs connect SFs with the extracellular matrix (ECM). The FA comprises approximately 500 kinds of proteins including integrin, talin, and vinculin that serve as primary proteins mediating the connection between the ECM and SF (Sastry and Burridge, 2000; Kuo et al., 2011; Hirata et al., 2014; Burridge and Guilluy, 2016). FAs attach cells to the ECM and act as a hub to transduce extracellular mechanical cues such as rigidity and topography of ECM to intracellular signals (Humphries et al., 2009; Kanchanawong et al., 2010).
Recent studies highlight cellular responsiveness to ECM substrate stiffness. A well-known cellular response to substrate rigidity is “durotaxis,” where cells seeded on ECMs with varying stiffness exhibit a preference for migrating toward more rigid regions (Lo et al., 2000; Isenberg et al., 2009). Moreover, the stiffness of the ECM also influences cell differentiation. For instance, on softer substrates (<1 kPa), mesenchymal stem cells manifest neuron-like characteristics. In contrast, on stiffer substrates (>30 kPa), they differentiate into osteoblast-like cells (Discher et al., 2005; Engler et al., 2006). Exertion of myosin-generated contractile force to FAs underpins the rigidity-sensing mechanism. Recent studies emphasize the significance of SF mechanical properties in modulating cellular architecture and mechanosensation by altering the efficacy of the contractile force transmission along SFs to FAs (Ehrlicher et al., 2015; Doss et al., 2020; Katsuta et al., 2023). This mini-review aims to illuminate the mechanisms of myosin-generated force transmission along SF and its effects on cell functions such as FA-mediated cell adhesion, ECM rigidity sensing, and cell migration.
Actin crosslinkers modulate mechanical properties of SFs and the efficiency of contractile force transmission along SFs
In adherent cells, SFs and FAs serve as the primary machinery for anchoring cells to the ECM (Figure 1A). The SF is composed of actin filaments, non-muscle myosin II (NMMII) and actin-crosslinking proteins. SFs play a crucial role in generating myosin-based contractile force and in transmitting generated force to FAs. From the mechanical point of view, the SF acts not just as a passive elastic string but also as an active viscoelastic matter (Kumar et al., 2006; Tanner et al., 2010; Oakes et al., 2017). Subcellular laser ablation has been employed to understand the mechanical properties of SFs in situ, where each SF is severed and its viscoelasticity is analyzed from the retraction kinetics of the severed SF. When an SF is incised, it physically retracts throughout its length. The displacement rate of the severed edge was high immediately after severing but slowed down exponentially and eventually became zero at the steady state (Kumar et al., 2006; Wu et al., 2012). This phenomenon was congruent with the Kelvin-Voigt model, characterized as a viscoelastic entity represented by an elastic spring and a viscous dashpot in parallel (Cañadas et al., 2002; Kumar et al., 2006; Tanner et al., 2010).
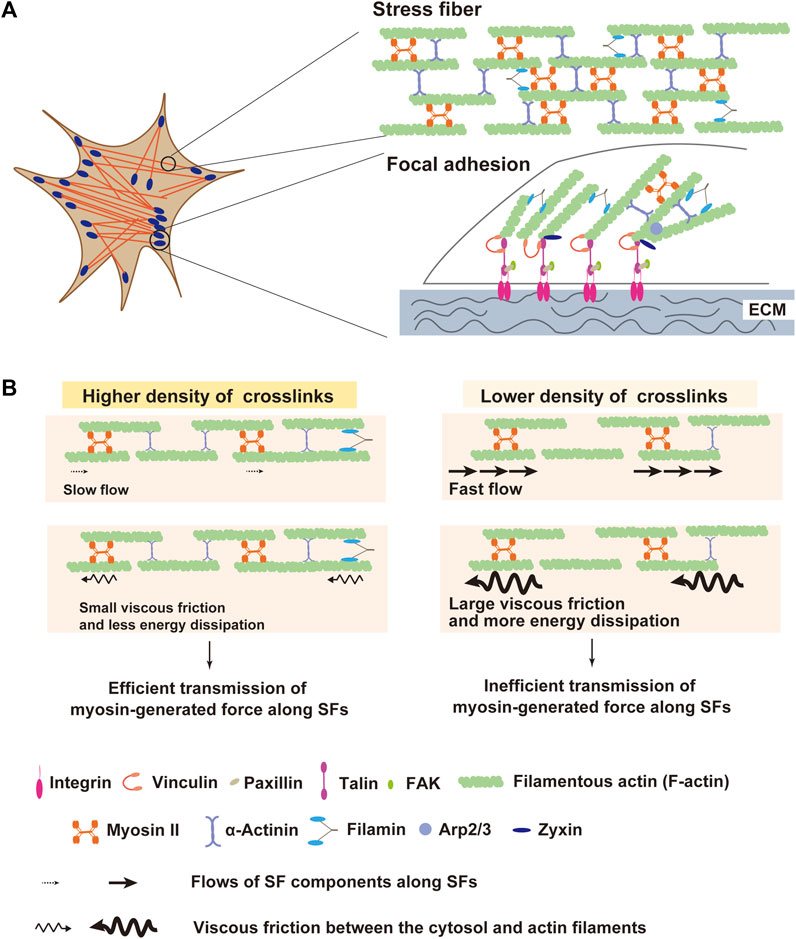
Figure 1. Structures of stress fiber (SF) and focal adhesion (SF) as a machinery of force transmission to extracellular matrix (ECM). (A) Schematic diagram of SF and FA. SFs are composed of filamentous actin (F-actin), myosin II, and crosslinking proteins including α-actinin and filamin. At the termini of SFs, FAs connect them with extracellular matrix (ECM). FAs are comprised of ∼500 proteins including integrin, vinculin, talin, and zyxin. Contractile force transmits through SFs to FAs, which enables adherent cells to pull ECM. (B) Schematic illustration of the influence of the actin crosslinker density on SF mechanics. Actin crosslinking proteins solidify SFs by reducing the mobility of SF components along SFs. When crosslinkers are sparsely distributed, SFs become fluidized by the increased mobility of SF components. In this situation, myosin-generated energy experiences a large dissipation due to elevated viscous friction between SF and surrounding cytosol, leading to inefficient force transmission along SFs.
Actin crosslinking proteins are vital factors that affect mechanical properties of SFs, similar to the case of crosslinkers in synthetic polymer networks (Gardel et al., 2004; Lieleg et al., 2009; Schmoller et al., 2009; Norstrom and Gardel, 2011). For instance, an increase in the affinity of α-actinin for actin has been shown to increase the elasticity of actin filament gels in vitro (Wachsstock et al., 1994). Recent studies from our and other groups have shown that several SF-crosslinking proteins including α-actinin, filamin, and non-muscle myosin IIB (NMMIIB) contribute to the elasticity of SFs in living melanoma, osteosarcoma and myoblast cells (Kasza et al., 2009; Weißenbruch et al., 2021; Katsuta et al., 2023). Depletion of all α-actinin isoforms significantly accelerates flows of myosin II and F-actin along SFs, which is driven by the myosin II activity (Katsuta et al., 2023). Thus, α-actinin-mediated crosslinks solidify SFs by reducing the mobility of SF components along SFs. Consistently, atomic force microscopy (AFM) measurements showed that α-actinin depletion drastically decreased the elasticity of SFs (Katsuta et al., 2023). Solidification of the actin cytoskeleton by α-actinin-mediated crosslinks has also been shown in vitro using the particle-tracking microrheology method (Ehrlicher et al., 2015), which measures local mechanical properties of the environment by tracking the motions of individual microparticles embedded in the environment (Tseng et al., 2002; Wirtz, 2009).
An increase in the flow speed of the SF components upon α-actinin depletion would elevate viscous resistance between the SF and the surrounding cytosol and, thereby, increase the escape of myosin-generated force from the SF into the cytosol. Thus, it is predicted that SF fluidization in α-actinin-depleted cells may lower the efficiency of transmission of contractile force along the SF (Figure 1B). Consistent with this prediction, α-actinin depletion causes a decrease in traction stress exerted on ECM, even though it does not reduce the myosin activity in SFs (Katsuta et al., 2023). Traction stress exerted on ECM depends on myosin activity (Jorrisch et al., 2013; Chang and Kumar, 2015), and forced activation of myosin with the non-specific phosphatase inhibitor calyculin A increases the traction stress (Jerrell and Parekh, 2014; Katsuta et al., 2023). However, in α-actinin-depleted cells, calyculin A treatment did not increase traction stress (Katsuta et al., 2023), suggesting that elevated myosin II force failed to be transmitted efficiently. Since calyculin A treatment of α-actinin-depleted cells increased the flow speed of SF components, elevated viscous friction between the flowing SF components and the cytosol in calyculin-A-treated cells might impair the calyculin-A-induced increase in traction stress. To our knowledge, no study directly measured viscous resistance in living cells. In general, viscous resistance is proportional to the mobility of the interface in a Newtonian fluid. Given that the cytosol is a Newtonian fluid, when the relationship between the distance from SFs and the displacement rate of the microparticles embedded in the cytosol is measured, it is predicted that the rate gets smaller in a distance-dependent manner. Here, the gradient of the rate to the distance is proportional to the viscous resistance between the SF and the cytosol. This hypothesis awaits future studies.
α-Actinin is accumulated not only along SFs but also at FAs, wherein it binds to both actin and integrin (Roca-Cusachs et al., 2013). This raises another possibility that lowered traction stress in α-actinin-depleted cells might be caused by less efficient force transmission at SF-FA junctions. However, depletion of filamin, another actin crosslinking protein, also lowers traction stress exertion to ECM at FAs without affecting non-muscle myosin II activity (Esue et al., 2009; Kasza et al., 2009; Thomas et al., 2015), suggesting that reduction in expression of actin crosslinkers decreases traction stress by causing dissipation of transmitting force along SFs rather than by disconnecting the SF-FA linkage. This notion is also supported by the fact that the α-actinin 4 mutant with higher binding affinity for F-actin leads to an increase in traction stress (Ehrlicher et al., 2015). This elevation of traction stress may be derived from the attenuation of the flow of actin filaments along SFs in the α-actinin 4 mutant-expressing cells.
Contrary to the results described above, some studies have shown that depletion of α-actinin 4 increases traction stress in fibroblasts (Roca-Cusachs et al., 2013; Doss et al., 2020). One possible basis for the inconsistency is that α-actinin 4 depletion may increase the density of myosin II binding to actin filaments perhaps due to an increase of myosin binding sites along each filament in fibroblasts (Shao et al., 2010a), which possibly elevates contractile force generation. As another possibility, a large difference in the actin binding affinity of α-actinin isoforms may be involved in the inconsistency. α-Actinin 1 and 4 form a heterodimer which is an abundant form of α-actinin dimers in many human cell lines (Foley and Young, 2013). Therefore, depletion of α-actinin 4 is likely to increase the amount of α-actinin 1 homodimers. Since the affinity of α-actinin 1 for actin is about 100 times higher than that of α-actinin 4 (Goldmann and Isenberg, 1993; Weins et al., 2007; Ferrer et al., 2008; Thomas and Robinson, 2017), the shift from α-actinin 1/α-actinin 4 heterodimers to α-actinin 1 homodimers would elevate the stability of actin crosslinks, thereby solidifying SFs and increasing the efficiency of force transmission along SFs. Nevertheless, depletion of α-actinin 1 in α-actinin-4-KD cells, which would result in a decrease of stable actin crosslinks, reduces traction stress (Doss et al., 2020), again suggesting the importance of α-actinin crosslinks in the myosin-generated contractile force transmission. Taken together, it is suggested that actin crosslinkers fine-tune force transmission along SFs by averting fluidization of SFs. Alterations in force transmission would modulate the balance between myosin-based force generation and the magnitude of traction stress exerted on ECM.
Cellular tensegrity is a concept for the intracellular cytoskeletal integrity that the cytoskeleton in a living cell is stabilized by tensile prestress and maintained through a complementary force balance between contractile actomyosin fibers and compression-bearing elements (e.g., nucleus and microtubule) (Ingber, 2003; Ingber et al., 2014). Thus, modulation of SF mechanics by actin crosslinker proteins potentially affects the shape, distribution, and dynamics of the nucleus and microtubules in living cells. Transmission of tensile force to the nucleus is mainly through a class of SFs called perinuclear actin cap that are associated with the apical surface of the nucleus (Khatau et al., 2009). α-Actinin is enriched in the perinuclear actin cap (Shiu et al., 2018), and depletion of α-actinin may reduce force transmission along the perinuclear actin cap to the nucleus. Indeed, α-actinin depletion causes a change in the shape and the position of the nucleus in a cell and disrupts the symmetry of the actomyosin network (Shao et al., 2010a; Maninová and Vomastek, 2016; Senger et al., 2019). Recently, it has been revealed that nuclear deformation by SFs causes opening of nuclear pores, which induces nuclear localization of Yes-associated protein (YAP) (Elosegui-Artola et al., 2017; Panciera et al., 2017; Shiu et al., 2018; Lee et al., 2019; Koushki et al., 2023), a nucleo-cytoplasm-shuttling transcriptional coactivator that promotes gene expression involved in cell proliferation and differentiation (Zhao et al., 2010; Dupont et al., 2011; Pavel et al., 2018). Therefore, actin crosslinker-modulated mechanics of SFs may play a crucial role in the mechanical regulation of YAP-mediated cell proliferation and differentiation, which should be further tested in future studies.
FA maturation is altered by mechanical properties of SFs
FAs are composed of over 500 kinds of proteins that include adhesion molecules, scaffold proteins, and assorted enzymes (Kuo et al., 2011), and connect SFs to ECM (Figure 1A). FA maturation by protein accumulation depends largely on intracellular and extracellular mechanical conditions, such as myosin contractile force and ECM rigidity (Bremer et al., 1991; Galbraith et al., 2002; Pasapera et al., 2010; Walcott et al., 2011; Rens and Merks, 2020). Force application by optical tweezers to fibronectin-coated beads that attach to the fibroblast surface has shown that fibroblasts respond to the restraining force on beads through local strengthening of the linkage between the beads and the cytoskeleton, allowing stronger force to be exerted on the integrins (Galbraith et al., 2002; Arbore et al., 2019). Conversely, when contractile force generation by myosin II is inhibited with blebbistatin in fibroblasts, compositional maturation of FA is perturbed (Hirata et al., 2008; Pasapera et al., 2010; Oakes et al., 2012). These results suggest that force applied to the FA is essential for FA maturation. As discussed in the above section, the mechanical property of SFs has a large impact on the contractile force transmission along SFs and force exertion to FAs and ECM. Hence, accumulation of FA components might also be modulated by the mechanical properties of SFs. Indeed, studies have shown that depletion of α-actinin, which lowers the efficiency of force transmission along SFs by fluidizing SFs, inhibits FA elongation and maturation in fibroblasts, although nascent FA formation is not affected (Choi et al., 2008; Roca-Cusachs et al., 2013). Moreover, filamin-mediated crosslinks of actin filaments, which make actin filament network more solid-like (Tseng et al., 2004), also increase traction force exertion and contribute to the accumulation of FA proteins in melanoma cells and fibroblasts (Kasza et al., 2009; Lynch et al., 2011).
The FA protein zyxin localizes to matured FAs, but not to nascent adhesions, dependently on tension at FAs, and promotes actin polymerization at FAs (Lele et al., 2006; Hirata et al., 2008; Nguyen et al., 2010; Legerstee et al., 2019). Upon depletion of α-actinin, zyxin is delocalized from FAs in osteosarcoma cells and myoblasts (Feng et al., 2013; Katsuta et al., 2023). Although zyxin could directly bind to α-actinin, a mutant form of zyxin with a deletion of the α-actinin-binding site still localized to FAs (Nix et al., 2001), suggesting that zyxin localization at FAs was not dependent on its direct binding to α-actinin. Interestingly, localization of all the proteins at matured FAs is not affected by the depletion of actin crosslinkers. Vinculin, one of common FA proteins, binds to another FA protein talin in a “force-dependent” manner through the force-induced exposure of vinculin binding sites in a talin molecule (Papagrigoriou et al., 2004; Del Rio et al., 2009; Hirata et al., 2014; Yao et al., 2014). However, multiple studies have shown that localization of vinculin and talin to FAs is independent of the expression level of α-actinin in osteosarcoma cells, myoblasts and keratinocytes (Feng et al., 2013; Hamill et al., 2015; Katsuta et al., 2023). As a potential basis underlying differential effects of α-actinin expression on FA localization of zyxin and vinculin, the force level required for FA localization may be different between zyxin and vinculin. Accumulation of vinculin to FAs was observed at the force larger than 2–3 nN per FA, whilst 10–30 nN per FA was required for zyxin accumulation at FAs (Balaban et al., 2001; Uemura et al., 2011). Although traction force at single FAs is 5–50 nN, which largely differs between FAs, cell types, and rigidity and topography of the extracellular substrate (Trichet et al., 2012; Zhou et al., 2017; Eckert et al., 2021), depletion of α-actinin-mediated crosslinks lowers traction stress by 30%–60% (Katsuta et al., 2023). Thus, α-actinin depletion may lower traction force at FAs to the extent that inhibits zyxin accumulation but is still larger than that required for vinculin accumulation at FAs. Taken together, the actin crosslinker-regulated mechanical property of SFs may be one of the key factors that affect FA maturation by modulating the transmission of contractile force along SFs to FAs.
Interestingly, when α-actinin is depleted, zyxin is not only delocalized from FAs but also accumulated along the entire length of SFs in osteosarcoma cells and myoblasts (Feng et al., 2013; Katsuta et al., 2023). Previous studies have shown that LIM domain proteins including zyxin directly bind to actin filaments in fibroblasts when filaments are tensed by myosin II activity (Smith et al., 2010;Smith et al., 2014). One possible mechanism of zyxin recruitment to SFs with sparse actin crosslinks is as follows; when actin filaments are sparsely crosslinked in an SF, only a small population of actin filaments would be involved in transmitting myosin force along the SF at each time point and sustain a larger tensile force than those in SFs with a high crosslink density. These highly tensed actin filaments could provide binding sites of zyxin in α-actinin-depleted SFs. Consistently, stretching of SFs with a normal α-actinin level also causes zyxin accumulation along SFs in fibroblasts (Yoshigi et al., 2005). Zyxin accumulated along SFs promotes actin polymerization with the aid of Ena/VASP proteins and, thereby, structurally reinforces SFs that are subjected to mechanical challenges such as excess extension or depletion of actin crosslinkers (Yoshigi et al., 2005; Smith et al., 2010; Katsuta et al., 2023).
Possible roles of SF mechanics in ECM rigidity sensing and cell migration
One of the essential roles of the SF-FA system is to sense the rigidity of ECM. At FAs, cells sense the rigidity of ECM by pulling it with myosin-generated contractile force (Lohner et al., 2019; Doss et al., 2020). As SF mechanics mediated by actin crosslinking proteins largely affect traction force exertion through modulation of force transmission along SFs, actin crosslinking proteins may be involved in cellular sensing of the substrate rigidity. Myosin II is more activated in fibroblasts on stiffer substrates, which leads to exerting larger traction stress on the substrates (Schäfer and Radmacher, 2005; Frey et al., 2006; Fouchard et al., 2011). However, melanoma and myoblast cells deficient in α-actinin or filamin A exhibit a failure in the substrate rigidity-dependent increase in traction stress (Byfield et al., 2009; Katsuta et al., 2023) (Figure 2A). This suggests a possibility that a decrease in actin crosslinks hampers rigidity sensing at FAs due to lowered efficiency of force transmission to FAs. At the leading edge of a fibroblast, a single-sarcomere-like complex called contractile unit (CU) has been reported as a minimal machinery for rigidity sensing (Ghassemi et al., 2012; Wolfenson et al., 2015; Meacci et al., 2016). Similar to SFs, CUs are composed of actin and myosin II filaments, tropomyosin, and α-actinin (Ghassemi et al., 2012; Wolfenson et al., 2015; Meacci et al., 2016). CUs link the adjacent integrin clusters separated by 1–2 μm, and pull on these integrin clusters to locally deform ECM (Ghassemi et al., 2012). Recruitment of α-actinin to CUs has been reported to be an essential step for CUs to exert a certain level of force required for sensing ECM rigidity (Wolfenson et al., 2015; Meacci et al., 2016). It is conceivable that actin crosslinking proteins avert slippage of actin and myosin II filaments in both SFs of multiple sarcomere units in series and CUs of single-sarcomere units.
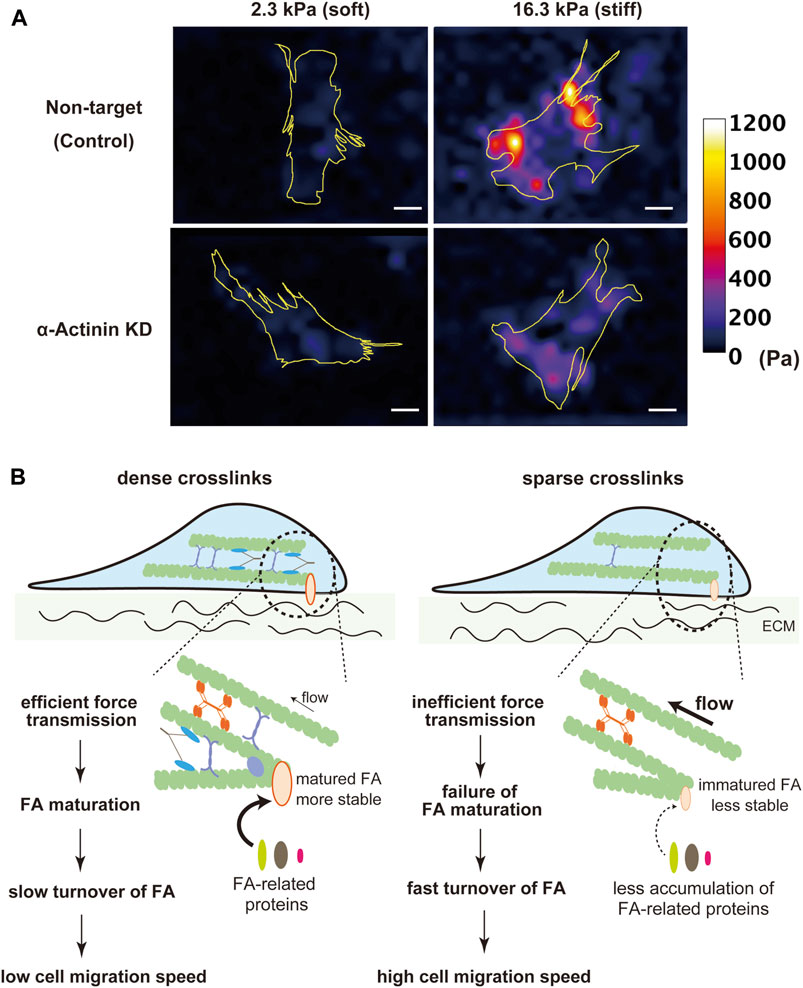
Figure 2. Contribution of actin crosslinking proteins to ECM rigidity sensing. (A) Traction stress images of α-actinin KD and non-target (control) C2C12 myoblast cells on fibronectin-coated 2.3 kPa and 16.3 kPa polyacrylamide gel substrates. Non-target cells on stiffer substrates exerted larger traction stress than on softer substrates. The rigidity-dependent increase in traction stress was attenuated in α-actinin KD cells. Yellow lines indicate outlines of cells. The heatmap scale of traction stress is common between non-target and α-actinin KD cells. Scale bars, 20 μm. (B) Schematic diagram of the possible roles of actin crosslinkers in cell migration. Cells with a high density of actin crosslinking proteins transmit contractile force more efficiently to FAs, which stabilizes FAs, attenuates FA turnover, and, thereby, lowers the cell migration speed.
Cell migration is another cellular activity that is regulated by intracellular and extracellular mechanical conditions. During cell migration, traction force exerted from FAs to ECM drives forward translocation of the cell body (Fournier et al., 2010; Yamaguchi and Knaut, 2022). On the other hand, even though assembly/disassembly dynamics of FAs are required for continuous cell migration, the tensile force acting on FAs stabilizes them and hampers their disassembly (Trichet et al., 2012; Zhou et al., 2017). Therefore, the velocity of cell migration is determined by a balance between these opposing effects of myosin-based force exerted on FAs. Since actin crosslinkers largely affect force transmission along SFs to FAs, the cell migration velocity is potentially regulated by the expression level of actin crosslinkers. Indeed, previous studies have shown an inverse relationship between the expression levels of actin crosslinking proteins, such as α-actinin, myosin IIB, or filamin, and the velocity of fibroblast migration (Glück and Ben-Ze’ev, 1994; Lo et al., 2003; Ketebo et al., 2021). With a low density of actin crosslinking proteins, smaller force exerting on FAs makes FAs less stable, leading to an increase in the speed of cell migration with a higher FA turnover rate (Figure 2B).
ECM stiffness also affects cell migration, mainly through alteration of FA stability (Lange and Fabry, 2013; Hadden et al., 2017). One outstanding example is durotaxis, in which cells migrate from softer to stiffer substrates (Lo et al., 2000; Isenberg et al., 2009). A proposed mechanism for durotaxis is that cellular protrusions on stiffer regions exert larger force to FAs, which makes FAs more stable, leading to a movement of the cell body toward stiffer regions (Plotnikov et al., 2012; Shellard and Mayor, 2021). Since actin crosslinkers can modulate rigidity sensing and cell migration, it is possible that actin crosslinking proteins affect durotaxis. Indeed, a small reduction of MYHIIB expression significantly diminished durotaxis (Raab et al., 2012), which supports the possibility that actin crosslinkers contribute to rigidity-dependent migration.
It has been well established that cancer cells plated on stiff substrates show more aggressive phenotypes than those on soft substrates (Paszek et al., 2005; McKenzie et al., 2018; DuChez et al., 2019; Espina et al., 2022). Actin crosslinking proteins, α-actinin, myosin II, and fascin, are highly upregulated in multiple human cancers, where they promote invasive cell behaviors and correlate with poor patient prognosis (Vignjevic et al., 2007; Jayo and Parsons, 2010; Jansen et al., 2011; Ouderkirk and Krendel, 2014; Schoumacher et al., 2014; Jayo et al., 2016; Chen et al., 2021). While the detailed mechanism of how the upregulated expression of actin crosslinkers alters cancer cell behaviors remains to be elucidated, it is possible that by increasing efficiency of force transmission to FAs, actin crosslinkers may enable exertion of large traction stress even on soft substrates to promote maturation of FAs. Force-induced maturation of FAs would lead to activation of FA-mediated signaling including the focal adhesion kinase (FAK) pathway that is essential for enhancing proliferation and invasion of cancer cells (Chan et al., 2009; Shibue and Weinberg, 2009; Pasapera et al., 2010).
Whilst discussion above is mainly based on results obtained using cells cultured on 2D substrates, recent studies have shown that cell migration modes are different in between 2D and 3D environments. Fibroblasts, smooth muscle cells and some cancer cells in 3D ECM exhibit mesenchymal migration which is characterized by an elongated cell shape with long membrane protrusions, strong adhesion to surrounding ECM, and proteolytic degradation of the ECM (Friedl and Weigelin, 2008). This type of cell migration depends on actomyosin contractility-induced stabilization of cell adhesion to ECM (Doyle et al., 2015). Filamin A is required for mesenchymal migration of macrophages through the formation of cell adhesions to 3D ECM (Guiet et al., 2012), which is consistent with our notion in 2D that sparse crosslinks of actin filaments destabilize cell adhesion due to impaired contractile force transmission to adhesion sites. Impairments in FA maturation and cell migration in 3D environments are also observed upon filamin B depletion in A549 lung carcinoma cells and HT1080 fibrosarcoma cells (Iguchi et al., 2015). However, compared with the case of cell migration on the 2D surface, knowledge about the role of actin crosslinking proteins in cell migration in 3D environments is limited and needs to be revealed in future studies.
Actin-crosslinking proteins modulate cellular responses to externally applied mechanical stimuli
In living tissues, cells are exposed to various kinds of mechanical stresses including stretch and shear stress, and these external mechanical stimuli alter cell behaviors (Janmey and Weitz, 2004). For instance, wound healing is accelerated by uniaxial cyclic stretch (Skutek et al., 2001; Huang et al., 2013; Kawai et al., 2023), and laminar shear stress applied to vascular endothelial cells relaxes vascular smooth muscle by promoting nitric oxide production (Harrison et al., 2006; Ando and Yamamoto, 2010). External forces act on or are transmitted to the SF-FA complex, which causes cellular responses (Paul et al., 2008; Colombelli et al., 2009).
Sustained stretch of the extracellular substrate induces rapid FA growth and tyrosine phosphorylation of FAK at FAs (Wang et al., 2001; Chen et al., 2013). Actin crosslinking by α-actinin or filamin causes stiffening of actin networks in response to the stretch (Xu et al., 2000; Schmoller et al., 2010), which would enhance stretch-induced development of tension at FAs that connect between the actin cytoskeleton and the extracellular substrate (Kumar et al., 2019). Thus, actin crosslinkers may facilitate mechanotransduction at FAs in response to stretching of the extracellular substrate.
When cells are subjected to uniaxial cyclic stretching, SFs oriented in parallel to stretch axis are disassembled (Dartsch and Betz, 1989; Roshanzadeh et al., 2020). This process relies on severing of unloaded actin filaments by cofilin (Hayakawa et al., 2011). If an SF is a pure elastic object, tensile stress is repeatedly increased in the cyclically stretched SF in phase with the stretch cycle. By contrast, when a totally viscous object is subjected to cyclic stretching, tensile and compressive stresses are developed alternately in the object (Stamenović, 2008; Hirata and Sokabe, 2022). Since actin crosslinkers modulate the viscoelastic property of SFs, the difference in the crosslinker density in SFs should have a large impact on mechanical stress development in SFs in response to cyclic stretching. Especially, in less elastic SFs with a low density of actin crosslinkers, cyclic stretching would cause development of a significant compressive stress in SFs, which is likely to decrease myosin-based pretension in actin filaments within the SFs, thereby promoting cofilin-dependent severing of the actin filaments and disassembly of the SFs. Such enhanced disassembly of less-crosslinked SFs oriented in parallel to the axis of uniaxial cyclic stretching may facilitate the reorientation of SFs and cells in response to the stretching; both SFs and cells become aligned perpendicular to the stretch axis (Zielinski et al., 2018; Kumar et al., 2019).
Although interactions between filamentous actin and actin crosslinking proteins can withstand external strains during deformation of actin cytoskeleton through unfolding and conformational transitions of crosslinking proteins (Rivero et al., 1996; D’Addario et al., 2001; Ferrer et al., 2008; Jackson et al., 2008; Hoffman et al., 2012; Razinia et al., 2012; Le et al., 2017), excessive stiffening of SFs with a high density of actin crosslinkers may conversely make cells fragile against mechanical deformation. Podocytes expressing α-actinin 4 mutants with increased binding affinity to F-actin, which induce familial focal segmental glomerular sclerosis (Kaplan et al., 2000), show breakages in their actin cytoskeleton upon periodic 10% uniaxial stretch (Feng et al., 2018; Feng et al., 2020). Such breakage might be caused by reduced plasticity of the actin cytoskeleton with tight crosslinks of actin filaments. Although further studies are required to elucidate how actin crosslinking proteins alter cytoskeleton remodeling in living cells under various mechanical stimuli, it is possible that they largely regulate mechanical response of SFs and FAs. Furthermore, it is notable that conformational changes of actin crosslinking proteins in response to deformation of the actin network may also contribute to cellular mechanotransduction. For example, mechanical strain of the filamin A-crosslinked actin network causes conformational changes of filamin A, altering its interaction with its binding partners including β1 integrin and FilGAP (Ehrlicher et al., 2011).
Concluding remarks
The complex interplay between SFs, FAs, and ECM is fundamental to diverse cellular functions including migration, proliferation and differentiation. In this review, we have focused on the effects of actin crosslinking proteins, which stabilize SFs and play pivotal roles in FA maturation, substrate rigidity sensing, cell migration, and mechanotransduction. Mutations in these crosslinkers have been revealed to be associated with a diverse spectrum of pathologies. For instance, mutations in α-actinin isoforms that upregulate or downregulate stability of actin crosslinks have been linked to several human diseases including autosomal-dominant congenital macrothrombocytopenia (Kanhai et al., 2018; O’Sullivan et al., 2021), dilated or hypertrophic cardiomyopathy (Lindholm et al., 2021), familial form of FSGS (Kaplan et al., 2000; Yang and Glass, 2008), and immunological diseases (Kikonomou et al., 2011). Mutations in filamin A also induce a wide spectrum of diseases including skeletal dysplasia, neuronal migration abnormality, cardiovascular malformation, intellectual disability, and intestinal obstruction (Robertson et al., 2003; Robertson, 2005; Eltahir et al., 2016; Sasaki et al., 2019). An in-vivo study has shown that depletion of filamin A in vascular smooth muscle cells induces lower blood pressure due to aortic dilation and increases in atrial compliance (Retailleau et al., 2016). Furthermore, multiple proteomic studies have implicated fascin contribution to several neurological diseases, such as seizure and Alzheimer disease (Spencer et al., 2011; Lamb and Tootle, 2020). While detailed mechanisms of how these protein mutations cause disease pathogenesis are still under active investigation, alteration of the mechanical properties of SFs can be involved in disease manifestation.
The actin binding affinity of actin crosslinkers including α-actinin, filamin, and plastin can be modulated by intracellular Ca2+ levels (Burridge and Feramisco, 1981; Glenney et al., 1981; Nakamura et al., 2005; Kasza et al., 2009; Foley and Young, 2014; Drmota Prebil et al., 2016; Lehne and Bogdan, 2023). Ca2+ binding to EF-hands of these actin-binding proteins decreases their affinity for actin binding and elasticity of the actin network in vitro (Pinotsis et al., 2020). Consistent with this, a decrease in the intracellular Ca2+ concentration ([Ca2+]i) causes an increase in cytoskeletal stiffness, suggesting that intracellular Ca2+ modulates actin crosslinker-mediated mechanical properties of the actin cytoskeleton (Brundage et al., 1991; Wei et al., 2012). Accordingly, the transmission of myosin-generated force in the actin cytoskeleton to FAs might be increased under the low [Ca2+]i condition, which was supported by the finding that FAs were stabilized and cell motility was hampered under such condition (Wei et al., 2012). [Ca2+]i is upregulated by various extracellular stimuli. For example, a range of ligands for G-protein-coupled receptors (GPCRs) induces IP3-mediated Ca2+ release from intracellular Ca2+ stores such as endoplasmic reticulum (Lock et al., 2019; Wang et al., 2019; Woll and Van Petegem, 2021), and mechanical stresses (e.g., cyclic stretch and shear stress) cause Ca2+ influxes via activation of mechanosensitive channels (Clapham, 2007; Murata et al., 2014; Sato et al., 2015). Substrate stiffness also regulates the magnitude and the frequency of [Ca2+]i increases in mesenchymal stem cells (Kim et al., 2009). Binding of phosphatidylinositol 4,5-bisphosphate (PIP2) to actin crosslinker proteins also modulates their affinity for actin (Greenwood et al., 2000; Izaguirre et al., 2001; Fraley et al., 2003; Shao et al., 2010b; Foley and Young, 2014). The intracellular level of PIP2 is regulated by various ligands for GPCRs or receptor tyrosine kinases (e.g., PDGF receptor) through activation of phospholipase C (PLC), phosphoinositide 3-kinase (PI3K), or phosphatase and tensin homologue deleted on chromosome 10 (PTEN) (Balla, 2013; Cocco et al., 2015; Liu et al., 2018; Harraz et al., 2020; He et al., 2021). Taken together, it is conceivable that actin crosslinking and SF mechanics are potentially modulated by multiple physical and chemical factors in the extracellular environments.
Despite substantial attempts to reveal SF-FA mechanics and its role in cell behaviors, much remains elusive. First, from both molecular and biophysical perspectives, we need more observations to quantitatively understand how actin crosslinking proteins alter mechanical properties of SFs, FA maturation and rigidity sensing. Second, although the affinity of actin crosslinker proteins for actin is affected by Ca2+ and PIP2, when and where cells utilize these regulation systems remain to be solved. Furthermore, it is an open question how malignant cells alter the rigidity sensing systems to facilitate their invasiveness and proliferation. Further studies focusing on these points will significantly enhance our understanding of how actin crosslinker-dependent modulation of the SF-FA machinery contributes to regulations of cellular functions.
Author contributions
HK: Writing–original draft, Funding acquisition, Conceptualization. MS: Writing–review and editing. HH: Writing–original draft, Supervision, Funding acquisition, Conceptualization, Writing–review and editing.
Funding
The authors declare that financial support was received for the research, authorship, and/or publication of this article. This study was supported by JSPS KAKENHI (21H05127 to HH and 24K18084 to HK).
Conflict of interest
The authors declare that the research was conducted in the absence of any commercial or financial relationships that could be construed as a potential conflict of interest.
The authors declared that they were an editorial board member of Frontiers, at the time of submission. This had no impact on the peer review process and the final decision.
Publisher’s note
All claims expressed in this article are solely those of the authors and do not necessarily represent those of their affiliated organizations, or those of the publisher, the editors and the reviewers. Any product that may be evaluated in this article, or claim that may be made by its manufacturer, is not guaranteed or endorsed by the publisher.
References
Ando, J., and Yamamoto, K. (2010). Effects of shear stress and stretch on endothelial function. Antioxid. Redox Signal. 15, 1389–1403. doi:10.1089/ars.2010.3361
Arbore, C., Perego, L., Sergides, M., and Capitanio, M. (2019). Probing force in living cells with optical tweezers: from single-molecule mechanics to cell mechanotransduction. Biophys. Rev. 11, 765–782. doi:10.1007/s12551-019-00599-y
Balaban, N. Q., Schwarz, U. S., Riveline, D., Goichberg, P., Tzur, G., Sabanay, I., et al. (2001). Force and focal adhesion assembly: a close relationship studied using elastic micropatterned substrates. Nat. Cell Biol. 35 (3), 466–472. doi:10.1038/35074532
Balla, T. (2013). Phosphoinositides: tiny lipids with giant impact on cell regulation. Physiol. Rev. 93, 1019–1137. doi:10.1152/physrev.00028.2012
Bremer, A., Millonig, R. C., Sutterlin, R., Engel, A., Pollard, T. D., and Aebi, U. (1991). The structural basis for the intrinsic disorder of the actin filament: the lateral slipping model. J. Cell Biol. 115, 689–703. doi:10.1083/JCB.115.3.689
Brundage, R. A., Fogarty, K. E., Tuft, R. A., and Fay, F. S. (1991). Calcium gradients underlying polarization and chemotaxis of eosinophils. Science 254, 703–706. doi:10.1126/science.1948048
Burridge, K., and Feramisco, J. R. (1981). Non-muscle alpha actinins are calcium-sensitive actin-binding proteins. Nature 294, 565–567. doi:10.1038/294565a0
Burridge, K., and Guilluy, C. (2016). Focal adhesions, stress fibers and mechanical tension. Exp. Cell Res. 343, 14–20. doi:10.1016/J.YEXCR.2015.10.029
Byfield, F. J., Wen, Q., Levental, I., Nordstrom, K., Arratia, P. E., Miller, R. T., et al. (2009). Absence of filamin A prevents cells from responding to stiffness gradients on gels coated with collagen but not fibronectin. Biophys. J. 96, 5095–5102. doi:10.1016/j.bpj.2009.03.046
Cañadas, P., Laurent, V. M., Oddou, C., Isabey, D., and Wendling, S. (2002). A cellular tensegrity model to analyse the structural viscoelasticity of the cytoskeleton. J. Theor. Biol. 218, 155–173. doi:10.1006/jtbi.2002.3064
Chan, K. T., Cortesio, C. L., and Huttenlocher, A. (2009). FAK alters invadopodia and focal adhesion composition and dynamics to regulate breast cancer invasion. J. Cell Biol. 185, 357–370. doi:10.1083/jcb.200809110
Chang, C.-W., and Kumar, S. (2015). Differential contributions of nonmuscle myosin II isoforms and functional domains to stress fiber mechanics. Sci. Rep. 5, 13736. doi:10.1038/srep13736
Chen, M., Sun, L.-X., Yu, L., Liu, J., Sun, L.-C., Yang, Z.-H., et al. (2021). MYH9 is crucial for stem cell-like properties in non-small cell lung cancer by activating mTOR signaling. Cell Death Discov. 7, 282. doi:10.1038/s41420-021-00681-z
Chen, Y., Pasapera, A. M., Koretsky, A. P., and Waterman, C. M. (2013). Orientation-specific responses to sustained uniaxial stretching in focal adhesion growth and turnover. Proc. Natl. Acad. Sci. U. S. A. 110, E2352–E2361. doi:10.1073/pnas.1221637110
Choi, C. K., Vicente-Manzanares, M., Zareno, J., Whitmore, L. A., Mogilner, A., and Horwitz, A. R. (2008). Actin and alpha-actinin orchestrate the assembly and maturation of nascent adhesions in a myosin II motor-independent manner. Nat. Cell Biol. 10, 1039–1050. doi:10.1038/ncb1763
Cocco, L., Follo, M. Y., Manzoli, L., and Suh, P.-G. (2015). Phosphoinositide-specific phospholipase C in health and disease. J. Lipid Res. 56, 1853–1860. doi:10.1194/jlr.R057984
Colombelli, J., Besser, A., Kress, H., Reynaud, E. G., Girard, P., Caussinus, E., et al. (2009). Mechanosensing in actin stress fibers revealed by a close correlation between force and protein localization. J. Cell Sci. 122, 1665–1679. doi:10.1242/jcs.042986
Cramer, L. P., Siebert, M., and Mitchison, T. J. (1997). Identification of novel graded polarity actin filament bundles in locomoting heart fibroblasts: implications for the generation of motile force. J. Cell Biol. 136, 1287–1305. doi:10.1083/JCB.136.6.1287
D’Addario, M., Arora, P. D., Fan, J., Ganss, B., Ellen, R. P., and McCulloch, C. A. (2001). Cytoprotection against mechanical forces delivered through beta 1 integrins requires induction of filamin A. J. Biol. Chem. 276, 31969–31977. doi:10.1074/jbc.M102715200
Dartsch, P. C., and Betz, E. (1989). Response of cultured endothelial cells to mechanical stimulation. Basic Res. Cardiol. 84, 268–281. doi:10.1007/BF01907974
Del Rio, A., Perez-Jimenez, R., Liu, R., Roca-Cusachs, P., Fernandez, J. M., and Sheetz, M. P. (2009). Stretching single talin rod molecules activates vinculin binding. Science 323, 638–641. doi:10.1126/SCIENCE.1162912
Discher, D. E., Janmey, P., and Wang, Y. L. (2005). Tissue cells feel and respond to the stiffness of their substrate. Science 310, 1139–1143. doi:10.1126/SCIENCE.1116995
Doss, B. L., Pan, M., Gupta, M., Grenci, G., Mège, R. M., Lim, C. T., et al. (2020). Cell response to substrate rigidity is regulated by active and passive cytoskeletal stress. Proc. Natl. Acad. Sci. U. S. A. 117, 12817–12825. doi:10.1073/PNAS.1917555117
Doyle, A. D., Carvajal, N., Jin, A., Matsumoto, K., and Yamada, K. M. (2015). Local 3D matrix microenvironment regulates cell migration through spatiotemporal dynamics of contractility-dependent adhesions. Nat. Commun. 6, 8720. doi:10.1038/ncomms9720
Drmota Prebil, S., Slapšak, U., Pavšič, M., Ilc, G., Puž, V., de Almeida Ribeiro, E., et al. (2016). Structure and calcium-binding studies of calmodulin-like domain of human non-muscle α-actinin-1. Sci. Rep. 6, 27383. doi:10.1038/srep27383
DuChez, B. J., Doyle, A. D., Dimitriadis, E. K., and Yamada, K. M. (2019). Durotaxis by human cancer cells. Biophys. J. 116, 670–683. doi:10.1016/j.bpj.2019.01.009
Dupont, S., Morsut, L., Aragona, M., Enzo, E., Giulitti, S., Cordenonsi, M., et al. (2011). Role of YAP/TAZ in mechanotransduction. Nature 474, 179–183. doi:10.1038/nature10137
Eckert, J., Abouleila, Y., Schmidt, T., and Mashaghi, A. (2021). Single cell micro-pillar-based characterization of endothelial and fibroblast cell mechanics. Micro 1, 242–249. doi:10.3390/MICRO1020018
Ehrlicher, A. J., Krishnan, R., Guo, M., Bidan, C. M., Weitz, D. A., and Pollak, M. R. (2015). Alpha-actinin binding kinetics modulate cellular dynamics and force generation. Proc. Natl. Acad. Sci. U. S. A. 112, 6619–6624. doi:10.1073/PNAS.1505652112
Ehrlicher, A. J., Nakamura, F., Hartwig, J. H., Weitz, D. A., and Stossel, T. P. (2011). Mechanical strain in actin networks regulates FilGAP and integrin binding to filamin A. Nature 478, 260–263. doi:10.1038/nature10430
Elosegui-Artola, A., Andreu, I., Beedle, A. E. M., Lezamiz, A., Uroz, M., Kosmalska, A. J., et al. (2017). Force triggers YAP nuclear entry by regulating transport across nuclear pores. Cell 171, 1397–1410.e14. doi:10.1016/j.cell.2017.10.008
Eltahir, S., Ahmad, K. S., Al-Balawi, M. M., Bukhamsien, H., Al-Mobaireek, K., Alotaibi, W., et al. (2016). Lung disease associated with filamin A gene mutation: a case report. J. Med. Case Rep. 10, 97. doi:10.1186/s13256-016-0871-1
Engler, A. J., Sen, S., Sweeney, H. L., and Discher, D. E. (2006). Matrix elasticity directs stem cell lineage specification. Cell 126, 677–689. doi:10.1016/j.cell.2006.06.044
Espina, J. A., Marchant, C. L., and Barriga, E. H. (2022). Durotaxis: the mechanical control of directed cell migration. FEBS J. 289, 2736–2754. doi:10.1111/febs.15862
Esue, O., Tseng, Y., and Wirtz, D. (2009). Alpha-actinin and filamin cooperatively enhance the stiffness of actin filament networks. PLoS One 4, e4411. doi:10.1371/JOURNAL.PONE.0004411
Feng, D., Kumar, M., Muntel, J., Gurley, S. B., Birrane, G., Stillman, I. E., et al. (2020). Phosphorylation of ACTN4 leads to podocyte vulnerability and proteinuric glomerulosclerosis. J. Am. Soc. Nephrol. 31, 1479–1495. doi:10.1681/ASN.2019101032
Feng, D., Notbohm, J., Benjamin, A., He, S., Wang, M., Ang, L.-H., et al. (2018). Disease-causing mutation in α-actinin-4 promotes podocyte detachment through maladaptation to periodic stretch. Proc. Natl. Acad. Sci. U. S. A. 115, 1517–1522. doi:10.1073/pnas.1717870115
Feng, Y., Ngu, H., Alford, S. K., Ward, M., Yin, F., and Longmore, G. D. (2013). α-Actinin1 and 4 tyrosine phosphorylation is critical for stress fiber establishment, maintenance and focal adhesion maturation. Exp. Cell Res. 319, 1124–1135. doi:10.1016/J.YEXCR.2013.02.009
Ferrer, J. M., Lee, H., Chen, J., Pelz, B., Nakamura, F., Kamm, R. D., et al. (2008). Measuring molecular rupture forces between single actin filaments and actin-binding proteins. Proc. Natl. Acad. Sci. U. S. A. 105, 9221–9226. doi:10.1073/pnas.0706124105
Foley, K. S., and Young, P. W. (2013). An analysis of splicing, actin-binding properties, heterodimerization and molecular interactions of the non-muscle α-actinins. Biochem. J. 452, 477–488. doi:10.1042/BJ20121824
Foley, K. S., and Young, P. W. (2014). The non-muscle functions of actinins: an update. Biochem. J. 459, 1–13. doi:10.1042/BJ20131511
Fouchard, J., Mitrossilis, D., and Asnacios, A. (2011). Acto-myosin based response to stiffness and rigidity sensing. Cell adh. Migr. 5, 16–19. doi:10.4161/cam.5.1.13281
Fournier, M. F., Sauser, R., Ambrosi, D., Meister, J. J., and Verkhovsky, A. B. (2010). Force transmission in migrating cells. J. Cell Biol. 188, 287–297. doi:10.1083/JCB.200906139
Fraley, T. S., Tran, T. C., Corgan, A. M., Nash, C. A., Hao, J., Critchley, D. R., et al. (2003). Phosphoinositide binding inhibits alpha-actinin bundling activity. J. Biol. Chem. 278, 24039–24045. doi:10.1074/jbc.M213288200
Frey, M. T., Tsai, I. Y., Russell, T. P., Hanks, S. K., and Wang, Y. L. (2006). Cellular responses to substrate topography: role of myosin II and focal adhesion kinase. Biophys. J. 90, 3774–3782. doi:10.1529/biophysj.105.074526
Friedl, P., and Weigelin, B. (2008). Interstitial leukocyte migration and immune function. Nat. Immunol. 9, 960–969. doi:10.1038/ni.f.212
Galbraith, C. G., Yamada, K. M., and Sheetz, M. P. (2002). The relationship between force and focal complex development. J. Cell Biol. 159, 695–705. doi:10.1083/jcb.200204153
Gardel, M. L., Shin, J. H., MacKintosh, F. C., Mahadevan, L., Matsudaira, P., and Weitz, D. A. (2004). Elastic behavior of cross-linked and bundled actin networks. Science 304, 1301–1305. doi:10.1126/science.1095087
Ghassemi, S., Meacci, G., Liu, S., Gondarenko, A. A., Mathur, A., Roca-Cusachs, P., et al. (2012). Cells test substrate rigidity by local contractions on submicrometer pillars. Proc. Natl. Acad. Sci. U. S. A. 109, 5328–5333. doi:10.1073/PNAS.1119886109
Glenney, J. R., Kaulfus, P., Matsudaira, P., and Weber, K. (1981). F-actin binding and bundling properties of fimbrin, a major cytoskeletal protein of microvillus core filaments. J. Biol. Chem. 256, 9283–9288. doi:10.1016/S0021-9258(19)52543-1
Glück, U., and Ben-Ze’ev, A. (1994). Modulation of alpha-actinin levels affects cell motility and confers tumorigenicity on 3T3 cells. J. Cell Sci. 107, 1773–1782. doi:10.1242/jcs.107.7.1773
Goldmann, W. H., and Isenberg, G. (1993). Analysis of filamin and alpha-actinin binding to actin by the stopped flow method. FEBS Lett. 336, 408–410. doi:10.1016/0014-5793(93)80847-n
Greenwood, J. A., Theibert, A. B., Prestwich, G. D., and Murphy-Ullrich, J. E. (2000). Restructuring of focal adhesion plaques by PI 3-kinase. Regulation by PtdIns (3,4,5)-p(3) binding to alpha-actinin. J. Cell Biol. 150, 627–642. doi:10.1083/jcb.150.3.627
Guiet, R., Vérollet, C., Lamsoul, I., Cougoule, C., Poincloux, R., Labrousse, A., et al. (2012). Macrophage mesenchymal migration requires podosome stabilization by filamin A. J. Biol. Chem. 287, 13051–13062. doi:10.1074/jbc.M111.307124
Gupta, M., Doss, B., Lim, C. T., Voituriez, R., and Ladoux, B. (2016). Single cell rigidity sensing: a complex relationship between focal adhesion dynamics and large-scale actin cytoskeleton remodeling. Cell Adh. Migr. 10, 554–567. doi:10.1080/19336918.2016.1173800
Hadden, W. J., Young, J. L., Holle, A. W., McFetridge, M. L., Kim, D. Y., Wijesinghe, P., et al. (2017). Stem cell migration and mechanotransduction on linear stiffness gradient hydrogels. Proc. Natl. Acad. Sci. U. S. A. 114, 5647–5652. doi:10.1073/pnas.1618239114
Hamill, K. J., Hiroyasu, S., Colburn, Z. T., Ventrella, R. V., Hopkinson, S. B., Skalli, O., et al. (2015). Alpha actinin-1 regulates cell-matrix adhesion organization in keratinocytes: consequences for skin cell motility. J. Invest. Dermatol. 135, 1043–1052. doi:10.1038/JID.2014.505
Harraz, O. F., Hill-Eubanks, D., and Nelson, M. T. (2020). PIP2: a critical regulator of vascular ion channels hiding in plain sight. Proc. Natl. Acad. Sci. 117, 20378–20389. doi:10.1073/pnas.2006737117
Harrison, D. G., Widder, J., Grumbach, I., Chen, W., Weber, M., and Searles, C. (2006). Endothelial mechanotransduction, nitric oxide and vascular inflammation. J. Intern. Med. 259, 351–363. doi:10.1111/j.1365-2796.2006.01621.x
Hayakawa, K., Tatsumi, H., and Sokabe, M. (2008). Actin stress fibers transmit and focus force to activate mechanosensitive channels. J. Cell Sci. 121, 496–503. doi:10.1242/jcs.022053
Hayakawa, K., Tatsumi, H., and Sokabe, M. (2011). Actin filaments function as a tension sensor by tension-dependent binding of cofilin to the filament. J. Cell Biol. 195, 721–727. doi:10.1083/jcb.201102039
He, Y., Sun, M. M., Zhang, G. G., Yang, J., Chen, K. S., Xu, W. W., et al. (2021). Targeting PI3K/Akt signal transduction for cancer therapy. Signal Transduct. Target. Ther. 6, 425. doi:10.1038/s41392-021-00828-5
Hirata, H., and Sokabe, M. (2022). “Measurement and manipulation of cellular forces using silicone elastomers,” Material-based mechanobiology. Editors J. Nakanishi, and K. Uto (London: The Royal Society of Chemistry), 0, 64–84. doi:10.1039/9781839165375-00064
Hirata, H., Tatsumi, H., Lim, C. T., and Sokabe, M. (2014). Force-dependent vinculin binding to talin in live cells: a crucial step in anchoring the actin cytoskeleton to focal adhesions. Am J Physiol Cell Physiol. 306, C607–C620. doi:10.1152/ajpcell.00122.2013
Hirata, H., Tatsumi, H., and Sokabe, M. (2008). Mechanical forces facilitate actin polymerization at focal adhesions in a zyxin-dependent manner. J. Cell Sci. 121, 2795–2804. doi:10.1242/JCS.030320
Hoffman, L. M., Jensen, C. C., Chaturvedi, A., Yoshigi, M., and Beckerle, M. C. (2012). Stretch-induced actin remodeling requires targeting of zyxin to stress fibers and recruitment of actin regulators. Mol. Biol. Cell 23, 1846–1859. doi:10.1091/mbc.E11-12-1057
Huang, C., Miyazaki, K., Akaishi, S., Watanabe, A., Hyakusoku, H., and Ogawa, R. (2013). Biological effects of cellular stretch on human dermal fibroblasts. J. Plast. Reconstr. Aesthetic Surg. 66, e351–e361. doi:10.1016/j.bjps.2013.08.002
Humphries, J. D., Byron, A., Bass, M. D., Craig, S. E., Pinney, J. W., Knight, D., et al. (2009). Proteomic analysis of integrin-associated complexes identifies RCC2 as a dual regulator of Rac1 and Arf6. Sci. Signal. 2, ra51. doi:10.1126/SCISIGNAL.2000396
Iguchi, Y., Ishihara, S., Uchida, Y., Tajima, K., Mizutani, T., Kawabata, K., et al. (2015). Filamin B enhances the invasiveness of cancer cells into 3D collagen matrices. Cell Struct. Funct. 40, 61–67. doi:10.1247/csf.15001
Ingber, D. E. (2003). Tensegrity I. Cell structure and hierarchical systems biology. J. Cell Sci. 116, 1157–1173. doi:10.1242/jcs.00359
Ingber, D. E., Wang, N., and Stamenovic, D. (2014). Tensegrity, cellular biophysics, and the mechanics of living systems. Rep. Prog. Phys. 77, 046603. doi:10.1088/0034-4885/77/4/046603
Isenberg, B. C., DiMilla, P. A., Walker, M., Kim, S., and Wong, J. Y. (2009). Vascular smooth muscle cell durotaxis depends on substrate stiffness gradient strength. Biophys. J. 97, 1313–1322. doi:10.1016/J.BPJ.2009.06.021
Izaguirre, G., Aguirre, L., Hu, Y. P., Lee, H. Y., Schlaepfer, D. D., Aneskievich, B. J., et al. (2001). The cytoskeletal/non-muscle isoform of alpha-actinin is phosphorylated on its actin-binding domain by the focal adhesion kinase. J. Biol. Chem. 276, 28676–28685. doi:10.1074/JBC.M101678200
Jackson, W. M., Jaasma, M. J., Tang, R. Y., and Keaveny, T. M. (2008). Mechanical loading by fluid shear is sufficient to alter the cytoskeletal composition of osteoblastic cells. Am. J. Physiol. Physiol. 295, C1007–C1015. doi:10.1152/ajpcell.00509.2007
Janmey, P. A., and Weitz, D. A. (2004). Dealing with mechanics: mechanisms of force transduction in cells. Trends biochem. Sci. 29, 364–370. doi:10.1016/j.tibs.2004.05.003
Jansen, S., Collins, A., Yang, C., Rebowski, G., Svitkina, T., and Dominguez, R. (2011). Mechanism of actin filament bundling by fascin. J. Biol. Chem. 286, 30087–30096. doi:10.1074/jbc.M111.251439
Jayo, A., Malboubi, M., Antoku, S., Chang, W., Ortiz-Zapater, E., Groen, C., et al. (2016). Fascin regulates nuclear movement and deformation in migrating cells. Dev. Cell 38, 371–383. doi:10.1016/j.devcel.2016.07.021
Jayo, A., and Parsons, M. (2010). Fascin: a key regulator of cytoskeletal dynamics. Int. J. Biochem. Cell Biol. 42, 1614–1617. doi:10.1016/j.biocel.2010.06.019
Jerrell, R. J., and Parekh, A. (2014). Cellular traction stresses mediate extracellular matrix degradation by invadopodia. Acta Biomater. 10, 1886–1896. doi:10.1016/j.actbio.2013.12.058
Jorrisch, M. H., Shih, W., and Yamada, S. (2013). Myosin IIA deficient cells migrate efficiently despite reduced traction forces at cell periphery. Biol. Open 2, 368–372. doi:10.1242/bio.20133707
Kanchanawong, P., Shtengel, G., Pasapera, A. M., Ramko, E. B., Davidson, M. W., Hess, H. F., et al. (2010). Nanoscale architecture of integrin-based cell adhesions. Nature 468, 580–584. doi:10.1038/nature09621
Kanhai, D., Mulder, R., Ploos van Amstel, H. K., Schutgens, R., Lukens, M., and Tamminga, R. Y. J. (2018). Familial macrothrombocytopenia due to a double mutation in cis in the alpha-actinin 1 gene (ACTN1), previously considered to be chronic immune thrombocytopenic purpura. Pediatr. Blood Cancer 65, e27418. doi:10.1002/pbc.27418
Kaplan, J. M., Kim, S. H., North, K. N., Rennke, H., Correia, L. A., Tong, H. Q., et al. (2000). Mutations in ACTN4, encoding alpha-actinin-4, cause familial focal segmental glomerulosclerosis. Nat. Genet. 24, 251–256. doi:10.1038/73456
Kasza, K. E., Nakamura, F., Hu, S., Kollmannsberger, P., Bonakdar, N., Fabry, B., et al. (2009). Filamin A is essential for active cell stiffening but not passive stiffening under external force. Biophys. J. 96, 4326–4335. doi:10.1016/j.bpj.2009.02.035
Kasza, K. E., and Zallen, J. A. (2011). Dynamics and regulation of contractile actin-myosin networks in morphogenesis. Curr. Opin. Cell Biol. 23, 30–38. doi:10.1016/j.ceb.2010.10.014
Katsuta, H., Okuda, S., Nagayama, K., Machiyama, H., Kidoaki, S., Kato, M., et al. (2023). Actin crosslinking by α-actinin averts viscous dissipation of myosin force transmission in stress fibers. iScience 26, 106090. doi:10.1016/J.ISCI.2023.106090
Kawai, K., Ishise, H., Kubo, T., Larson, B., Fujiwara, T., Nishimoto, S., et al. (2023). Stretching promotes wound contraction through enhanced expression of endothelin receptor B and TRPC3 in fibroblasts. Plast. Reconstr. Surg. Glob. open 11, e4954. doi:10.1097/GOX.0000000000004954
Kemp, J. P., and Brieher, W. M. (2018). The actin filament bundling protein α-actinin-4 actually suppresses actin stress fibers by permitting actin turnover. J. Biol. Chem. 293, 14520–14533. doi:10.1074/JBC.RA118.004345
Ketebo, A. A., Park, C., Kim, J., Jun, M., and Park, S. (2021). Probing mechanobiological role of filamin A in migration and invasion of human U87 glioblastoma cells using submicron soft pillars. Nano Converg. 8, 19. doi:10.1186/s40580-021-00267-6
Khatau, S. B., Hale, C. M., Stewart-Hutchinson, P. J., Patel, M. S., Stewart, C. L., Searson, P. C., et al. (2009). A perinuclear actin cap regulates nuclear shape. Proc. Natl. Acad. Sci. U. S. A. 106, 19017–19022. doi:10.1073/pnas.0908686106
Kikonomou, K. G., Zachou, K., and Dalekos, G. N. (2011). Alpha-actinin: a multidisciplinary protein with important role in B-cell driven autoimmunity. Autoimmun. Rev. 10, 389–396. doi:10.1016/J.AUTREV.2010.12.009
Kim, T.-J., Seong, J., Ouyang, M., Sun, J., Lu, S., Hong, J. P., et al. (2009). Substrate rigidity regulates Ca2+ oscillation via RhoA pathway in stem cells. J. Cell. Physiol. 218, 285–293. doi:10.1002/jcp.21598
Kobayashi, T., and Sokabe, M. (2010). Sensing substrate rigidity by mechanosensitive ion channels with stress fibers and focal adhesions. Curr. Opin. Cell Biol. 22, 669–676. doi:10.1016/J.CEB.2010.08.023
Koushki, N., Ghagre, A., Srivastava, L. K., Molter, C., and Ehrlicher, A. J. (2023). Nuclear compression regulates YAP spatiotemporal fluctuations in living cells. Proc. Natl. Acad. Sci. 120, e2301285120. doi:10.1073/pnas.2301285120
Kumar, A., Shutova, M. S., Tanaka, K., Iwamoto, D. V., Calderwood, D. A., Svitkina, T. M., et al. (2019). Filamin A mediates isotropic distribution of applied force across the actin network. J. Cell Biol. 218, 2481–2491. doi:10.1083/jcb.201901086
Kumar, S., Maxwell, I. Z., Heisterkamp, A., Polte, T. R., Lele, T. P., Salanga, M., et al. (2006). Viscoelastic retraction of single living stress fibers and its impact on cell shape, cytoskeletal organization, and extracellular matrix mechanics. Biophys. J. 90, 3762–3773. doi:10.1529/biophysj.105.071506
Kuo, J.-C., Han, X., Hsiao, C.-T., Yates III, J. R., and Waterman, C. M. (2011). Analysis of the myosin-II-responsive focal adhesion proteome reveals a role for β-Pix in negative regulation of focal adhesion maturation. Nat. Cell Biol. 13, 383–393. doi:10.1038/ncb2216
Lamb, M. C., and Tootle, T. L. (2020). Fascin in cell migration: more than an actin bundling protein. Biol. (Basel). 9, 403. doi:10.3390/biology9110403
Lange, J. R., and Fabry, B. (2013). Cell and tissue mechanics in cell migration. Exp. Cell Res. 319, 2418–2423. doi:10.1016/J.YEXCR.2013.04.023
Le, S., Hu, X., Yao, M., Margadant, F. M., Sheetz, M. P., Yan, J., et al. (2017). Mechanotransmission and mechanosensing of human alpha-actinin 1. Cell Rep. 21, 2714–2723. doi:10.1016/j.celrep.2017.11.040
Lee, J. Y., Dominguez, A. A., Nam, S., Stowers, R. S., Qi, L. S., and Chaudhuri, O. (2019). Identification of cell context-dependent YAP-associated proteins reveals β1 and β4 integrin mediate YAP translocation independently of cell spreading. Sci. Rep. 9, 17188. doi:10.1038/s41598-019-53659-4
Lee, S., and Kumar, S. (2020). Cofilin is required for polarization of tension in stress fiber networks during migration. J. Cell Sci. 133, jcs243873. doi:10.1242/jcs.243873
Legerstee, K., Geverts, B., Slotman, J. A., and Houtsmuller, A. B. (2019). Dynamics and distribution of paxillin, vinculin, zyxin and VASP depend on focal adhesion location and orientation. Sci. Rep. 9, 10460. doi:10.1038/s41598-019-46905-2
Lehne, F., and Bogdan, S. (2023). Getting cells into shape by calcium-dependent actin cross-linking proteins. Front. Cell Dev. Biol. 11, 1171930. doi:10.3389/fcell.2023.1171930
Lele, T. P., Pendse, J., Kumar, S., Salanga, M., Karavitis, J., and Ingber, D. E. (2006). Mechanical forces alter zyxin unbinding kinetics within focal adhesions of living cells. J. Cell. Physiol. 207, 187–194. doi:10.1002/jcp.20550
Lieleg, O., Schmoller, K. M., Claessens, M. M. A. E., and Bausch, A. R. (2009). Cytoskeletal polymer networks: viscoelastic properties are determined by the microscopic interaction potential of cross-links. Biophys. J. 96, 4725–4732. doi:10.1016/J.BPJ.2009.03.038
Lindholm, M. E., Jimenez-Morales, D., Zhu, H., Seo, K., Amar, D., Zhao, C., et al. (2021). Mono- and biallelic protein-truncating variants in alpha-actinin 2 cause cardiomyopathy through distinct mechanisms. Circ. Genomic Precis. Med. 14, e003419. doi:10.1161/CIRCGEN.121.003419
Liu, C., Deb, S., Ferreira, V. S., Xu, E., and Baumgart, T. (2018). Kinetics of PTEN-mediated PI(3,4,5)P3 hydrolysis on solid supported membranes. PLoS One 13, e0192667. doi:10.1371/journal.pone.0192667
Lo, C.-M., Buxton, D. B., Chua, G. C. H., Dembo, M., Adelstein, R. S., and Wang, Y.-L. (2003). Nonmuscle myosin IIB is involved in the guidance of fibroblast migration. Mol. Biol. Cell 15, 982–989. doi:10.1091/mbc.e03-06-0359
Lo, C. M., Wang, H. B., Dembo, M., and Wang, Y. L. (2000). Cell movement is guided by the rigidity of the substrate. Biophys. J. 79, 144–152. doi:10.1016/S0006-3495(00)76279-5
Lock, J. T., Smith, I. F., and Parker, I. (2019). Spatial-temporal patterning of Ca2+ signals by the subcellular distribution of IP3 and IP3 receptors. Semin. Cell Dev. Biol. 94, 3–10. doi:10.1016/j.semcdb.2019.01.012
Lohner, J., Rupprecht, J. F., Hu, J., Mandriota, N., Saxena, M., de Araujo, D. P., et al. (2019). Large and reversible myosin-dependent forces in rigidity sensing. Nat. Phys. 15, 689–695. doi:10.1038/s41567-019-0477-9
Lynch, C. D., Gauthier, N. C., Biais, N., Lazar, A. M., Roca-Cusachs, P., Yu, C.-H., et al. (2011). Filamin depletion blocks endoplasmic spreading and destabilizes force-bearing adhesions. Mol. Biol. Cell 22, 1263–1273. doi:10.1091/mbc.e10-08-0661
Maharam, E., Yaport, M., Villanueva, N. L., Akinyibi, T., Laudier, D., He, Z., et al. (2015). Rho/Rock signal transduction pathway is required for MSC tenogenic differentiation. Bone Res. 3, 15015. doi:10.1038/boneres.2015.15
Maninová, M., and Vomastek, T. (2016). Dorsal stress fibers, transverse actin arcs, and perinuclear actin fibers form an interconnected network that induces nuclear movement in polarizing fibroblasts. FEBS J. 283, 3676–3693. doi:10.1111/febs.13836
McKenzie, A. J., Hicks, S. R., Svec, K. V., Naughton, H., Edmunds, Z. L., and Howe, A. K. (2018). The mechanical microenvironment regulates ovarian cancer cell morphology, migration, and spheroid disaggregation. Sci. Rep. 8, 7228. doi:10.1038/S41598-018-25589-0
Meacci, G., Wolfenson, H., Liu, S., Stachowiak, M. R., Iskratsch, T., Mathur, A., et al. (2016). α-Actinin links extracellular matrix rigidity-sensing contractile units with periodic cell-edge retractions. Mol. Biol. Cell 27, 3471–3479. doi:10.1091/MBC.E16-02-0107
Murata, N., Ito, S., Furuya, K., Takahara, N., Naruse, K., Aso, H., et al. (2014). Ca2+ influx and ATP release mediated by mechanical stretch in human lung fibroblasts. Biochem. Biophys. Res. Commun. 453, 101–105. doi:10.1016/j.bbrc.2014.09.063
Nakamura, F., Hartwig, J. H., Stossel, T. P., and Szymanski, P. T. (2005). Ca2+ and calmodulin regulate the binding of filamin A to actin filaments. J. Biol. Chem. 280, 32426–32433. doi:10.1074/jbc.M502203200
Nguyen, T. N., Uemura, A., Shih, W., and Yamada, S. (2010). Zyxin-mediated actin assembly is required for efficient wound closure. J. Biol. Chem. 285, 35439–35445. doi:10.1074/JBC.M110.119487
Nix, D. A., Fradelizi, J., Bockholt, S., Menichi, B., Louvard, D., Friederich, E., et al. (2001). Targeting of zyxin to sites of actin membrane interaction and to the nucleus. J. Biol. Chem. 276, 34759–34767. doi:10.1074/JBC.M102820200
Norstrom, M., and Gardel, M. L. (2011). Shear thickening of F-actin networks crosslinked with non-muscle myosin IIB. Soft Matter 7, 3228–3233. doi:10.1039/C0SM01157F
Oakes, P. W., Beckham, Y., Stricker, J., and Gardel, M. L. (2012). Tension is required but not sufficient for focal adhesion maturation without a stress fiber template. J. Cell Biol. 196, 363–374. doi:10.1083/jcb.201107042
Oakes, P. W., Wagner, E., Brand, C. A., Probst, D., Linke, M., Schwarz, U. S., et al. (2017). Optogenetic control of RhoA reveals zyxin-mediated elasticity of stress fibres. Nat. Commun. 81 (8), 15817. doi:10.1038/ncomms15817
O’Sullivan, L. R., Cahill, M. R., and Young, P. W. (2021). The importance of alpha-actinin proteins in platelet formation and function, and their causative role in congenital macrothrombocytopenia. Int. J. Mol. Sci. 22, 9363. doi:10.3390/ijms22179363
Ouderkirk, J. L., and Krendel, M. (2014). Non-muscle myosins in tumor progression, cancer cell invasion, and metastasis. Cytoskelet. Hob. 71, 447–463. doi:10.1002/cm.21187
Panciera, T., Azzolin, L., Cordenonsi, M., and Piccolo, S. (2017). Mechanobiology of YAP and TAZ in physiology and disease. Nat. Rev. Mol. Cell Biol. 18, 758–770. doi:10.1038/NRM.2017.87
Papagrigoriou, E., Gingras, A. R., Barsukov, I. L., Bate, N., Fillingham, I. J., Patel, B., et al. (2004). Activation of a vinculin-binding site in the talin rod involves rearrangement of a five-helix bundle. EMBO J. 23, 2942–2951. doi:10.1038/SJ.EMBOJ.7600285
Pasapera, A. M., Schneider, I. C., Rericha, E., Schlaepfer, D. D., and Waterman, C. M. (2010). Myosin II activity regulates vinculin recruitment to focal adhesions through FAK-mediated paxillin phosphorylation. J. Cell Biol. 188, 877–890. doi:10.1083/jcb.200906012
Paszek, M. J., Zahir, N., Johnson, K. R., Lakins, J. N., Rozenberg, G. I., Gefen, A., et al. (2005). Tensional homeostasis and the malignant phenotype. Cancer Cell 8, 241–254. doi:10.1016/j.ccr.2005.08.010
Paul, R., Heil, P., Spatz, J. P., and Schwarz, U. S. (2008). Propagation of mechanical stress through the actin cytoskeleton toward focal adhesions: model and experiment. Biophys. J. 94, 1470–1482. doi:10.1529/BIOPHYSJ.107.108688
Pavel, M., Renna, M., Park, S. J., Menzies, F. M., Ricketts, T., Füllgrabe, J., et al. (2018). Contact inhibition controls cell survival and proliferation via YAP/TAZ-autophagy axis. Nat. Commun. 9, 2961. doi:10.1038/s41467-018-05388-x
Pellegrin, S., and Mellor, H. (2007). Actin stress fibres. J. Cell Sci. 120, 3491–3499. doi:10.1242/JCS.018473
Pinotsis, N., Zielinska, K., Babuta, M., Arolas, J. L., Kostan, J., Khan, M. B., et al. (2020). Calcium modulates the domain flexibility and function of an α-actinin similar to the ancestral α-actinin. Proc. Natl. Acad. Sci. 117, 22101–22112. doi:10.1073/pnas.1917269117
Plotnikov, S. V., Pasapera, A. M., Sabass, B., and Waterman, C. M. (2012). Force fluctuations within focal adhesions mediate ECM-rigidity sensing to guide directed cell migration. Cell 151, 1513–1527. doi:10.1016/j.cell.2012.11.034
Raab, M., Swift, J., Dingal, P. C. D. P., Shah, P., Shin, J.-W., and Discher, D. E. (2012). Crawling from soft to stiff matrix polarizes the cytoskeleton and phosphoregulates myosin-II heavy chain. J. Cell Biol. 199, 669–683. doi:10.1083/jcb.201205056
Razinia, Z., Mäkelä, T., Ylänne, J., and Calderwood, D. A. (2012). Filamins in mechanosensing and signaling. Annu. Rev. Biophys. 41, 227–246. doi:10.1146/annurev-biophys-050511-102252
Rens, E. G., and Merks, R. M. H. (2020). Cell shape and durotaxis explained from cell-extracellular matrix forces and focal adhesion dynamics. iScience 23, 101488. doi:10.1016/j.isci.2020.101488
Retailleau, K., Arhatte, M., Demolombe, S., Jodar, M., Baudrie, V., Offermanns, S., et al. (2016). Smooth muscle filamin A is a major determinant of conduit artery structure and function at the adult stage. Pflügers Arch. - Eur. J. Physiol. 468, 1151–1160. doi:10.1007/s00424-016-1813-x
Rivero, F., Köppel, B., Peracino, B., Bozzaro, S., Siegert, F., Weijer, C. J., et al. (1996). The role of the cortical cytoskeleton: F-actin crosslinking proteins protect against osmotic stress, ensure cell size, cell shape and motility, and contribute to phagocytosis and development. J. Cell Sci. 109, 2679–2691. doi:10.1242/jcs.109.11.2679
Robertson, S. P. (2005). Filamin A: phenotypic diversity. Curr. Opin. Genet. Dev. 15, 301–307. doi:10.1016/j.gde.2005.04.001
Robertson, S. P., Twigg, S. R. F., Sutherland-Smith, A. J., Biancalana, V., Gorlin, R. J., Horn, D., et al. (2003). Localized mutations in the gene encoding the cytoskeletal protein filamin A cause diverse malformations in humans. Nat. Genet. 33, 487–491. doi:10.1038/ng1119
Roca-Cusachs, P., Rio, A. del, Puklin-Faucher, E., Gauthier, N. C., Biais, N., and Sheetz, M. P. (2013). Integrin-dependent force transmission to the extracellular matrix by α-actinin triggers adhesion maturation. Proc. Natl. Acad. Sci. U. S. A. 110, E1361–E1370. doi:10.1073/PNAS.1220723110
Roshanzadeh, A., Nguyen, T. T., Nguyen, K. D., Kim, D.-S., Lee, B.-K., Lee, D.-W., et al. (2020). Mechanoadaptive organization of stress fiber subtypes in epithelial cells under cyclic stretches and stretch release. Sci. Rep. 10, 18684. doi:10.1038/s41598-020-75791-2
Sasaki, E., Byrne, A. T., Phelan, E., Cox, D. W., and Reardon, W. (2019). A review of filamin A mutations and associated interstitial lung disease. Eur. J. Pediatr. 178, 121–129. doi:10.1007/s00431-018-3301-0
Sastry, S. K., and Burridge, K. (2000). Focal adhesions: a nexus for intracellular signaling and cytoskeletal dynamics. Exp. Cell Res. 261, 25–36. doi:10.1006/EXCR.2000.5043
Sato, K., Ogawa, Y., Ito, S., Fujisawa, S., and Minami, K. (2015). Strain magnitude dependent intracellular calcium signaling response to uniaxial stretch in osteoblastic cells. J. Biomech. Sci. Eng. 10, 15-00242–242. doi:10.1299/jbse.15-00242
Schäfer, A., and Radmacher, M. (2005). Influence of myosin II activity on stiffness of fibroblast cells. Acta Biomater. 1, 273–280. doi:10.1016/j.actbio.2005.02.004
Schmoller, K. M., Fernández, P., Arevalo, R. C., Blair, D. L., and Bausch, A. R. (2010). Cyclic hardening in bundled actin networks. Nat. Commun. 1, 134. doi:10.1038/ncomms1134
Schmoller, K. M., Lieleg, O., and Bausch, A. R. (2009). Structural and viscoelastic properties of actin/filamin networks: cross-linked versus bundled networks. Biophys. J. 97, 83–89. doi:10.1016/j.bpj.2009.04.040
Schoumacher, M., El-Marjou, F., Laé, M., Kambou, N., Louvard, D., Robine, S., et al. (2014). Conditional expression of fascin increases tumor progression in a mouse model of intestinal cancer. Eur. J. Cell Biol. 93, 388–395. doi:10.1016/j.ejcb.2014.08.002
Senger, F., Pitaval, A., Ennomani, H., Kurzawa, L., Blanchoin, L., and Théry, M. (2019). Spatial integration of mechanical forces by α-actinin establishes actin network symmetry. J. Cell Sci. 132, jcs236604. doi:10.1242/JCS.236604
Shao, H., Wang, J. H.-C., Pollak, M. R., and Wells, A. (2010a). α-actinin-4 is essential for maintaining the spreading, motility and contractility of fibroblasts. PLoS One 5, e13921. doi:10.1371/journal.pone.0013921
Shao, H., Wu, C., and Wells, A. (2010b). Phosphorylation of alpha-actinin 4 upon epidermal growth factor exposure regulates its interaction with actin. J. Biol. Chem. 285, 2591–2600. doi:10.1074/jbc.M109.035790
Shellard, A., and Mayor, R. (2021). Collective durotaxis along a self-generated stiffness gradient in vivo. Nature 600, 690–694. doi:10.1038/s41586-021-04210-x
Shibue, T., and Weinberg, R. A. (2009). Integrin beta1-focal adhesion kinase signaling directs the proliferation of metastatic cancer cells disseminated in the lungs. Proc. Natl. Acad. Sci. 106, 10290–10295. doi:10.1073/pnas.0904227106
Shiu, J.-Y., Aires, L., Lin, Z., and Vogel, V. (2018). Nanopillar force measurements reveal actin-cap-mediated YAP mechanotransduction. Nat. Cell Biol. 20, 262–271. doi:10.1038/s41556-017-0030-y
Skutek, M., Griensven, M., Zeichen, J., Brauer, N., and Bosch, U. (2001). Cyclic mechanical stretching modulates secretion pattern of growth factors in human tendon fibroblasts. Eur. J. Appl. Physiol. 86, 48–52. doi:10.1007/s004210100502
Smith, M. A., Blankman, E., Gardel, M. L., Luettjohann, L., Waterman, C. M., and Beckerle, M. C. (2010). A zyxin-mediated mechanism for actin stress fiber maintenance and repair. Dev. Cell 19, 365–376. doi:10.1016/j.devcel.2010.08.008
Smith, M. A., Hoffman, L. M., and Beckerle, M. C. (2014). LIM proteins in actin cytoskeleton mechanoresponse. Trends Cell Biol. 24, 575–583. doi:10.1016/J.TCB.2014.04.009
Spencer, V. A., Costes, S., Inman, J. L., Xu, R., Chen, J., Hendzel, M. J., et al. (2011). Depletion of nuclear actin is a key mediator of quiescence in epithelial cells. J. Cell Sci. 124, 123–132. doi:10.1242/jcs.073197
Stamenović, D. (2008). Rheological behavior of mammalian cells. Cell. Mol. Life Sci. 65, 3592–3605. doi:10.1007/s00018-008-8292-y
Tanner, K., Boudreau, A., Bissell, M. J., and Kumar, S. (2010). Dissecting regional variations in stress fiber mechanics in living cells with laser nanosurgery. Biophys. J. 99, 2775–2783. doi:10.1016/J.BPJ.2010.08.071
Thomas, D. G., and Robinson, D. N. (2017). The fifth sense: mechanosensory regulation of alpha-actinin-4 and its relevance for cancer metastasis. Semin. Cell Dev. Biol. 71, 68–74. doi:10.1016/j.semcdb.2017.05.024
Thomas, D. G., Yenepalli, A., Denais, C. M., Rape, A., Beach, J. R., Wang, Y. li, et al. (2015). Non-muscle myosin IIB is critical for nuclear translocation during 3D invasion. J. Cell Biol. 210, 583–594. doi:10.1083/JCB.201502039
Tojkander, S., Gateva, G., and Lappalainen, P. (2012). Actin stress fibers--assembly, dynamics and biological roles. J. Cell Sci. 125, 1855–1864. doi:10.1242/jcs.098087
Totsukawa, G., Yamakita, Y., Yamashiro, S., Hosoya, H., Hartshorne, D. J., and Matsumura, F. (1999). Activation of myosin phosphatase targeting subunit by mitosis-specific phosphorylation. J. Cell Biol. 144, 735–744. doi:10.1083/jcb.144.4.735
Trichet, L., Le Digabel, J., Hawkins, R. J., Vedula, S. R. K., Gupta, M., Ribrault, C., et al. (2012). Evidence of a large-scale mechanosensing mechanism for cellular adaptation to substrate stiffness. Proc. Natl. Acad. Sci. U. S. A. 109, 6933–6938. doi:10.1073/PNAS.1117810109
Tseng, Y., An, K. M., Esue, O., and Wirtz, D. (2004). The bimodal role of filamin in controlling the architecture and mechanics of F-actin networks. J. Biol. Chem. 279, 1819–1826. doi:10.1074/jbc.M306090200
Tseng, Y., Kole, T. P., and Wirtz, D. (2002). Micromechanical mapping of live cells by multiple-particle-tracking microrheology. Biophys. J. 83, 3162–3176. doi:10.1016/S0006-3495(02)75319-8
Uemura, A., Nguyen, T.-N., Steele, A. N., and Yamada, S. (2011). The LIM domain of zyxin is sufficient for force-induced accumulation of zyxin during cell migration. Biophys. J. 101, 1069–1075. doi:10.1016/j.bpj.2011.08.001
Vale, R. D., and Milligan, R. A. (2000). The way things move: looking under the hood of molecular motor proteins. Science 288, 88–95. doi:10.1126/science.288.5463.88
Vignjevic, D., Schoumacher, M., Gavert, N., Janssen, K.-P., Jih, G., Laé, M., et al. (2007). Fascin, a novel target of beta-catenin-TCF signaling, is expressed at the invasive front of human colon cancer. Cancer Res. 67, 6844–6853. doi:10.1158/0008-5472.CAN-07-0929
Wachsstock, D. H., Schwarz, W. H., and Pollard, T. D. (1994). Cross-linker dynamics determine the mechanical properties of actin gels. Biophys. J. 66, 801–809. doi:10.1016/S0006-3495(94)80856-2
Walcott, S., Kim, D. H., Wirz, D., and Sun, S. X. (2011). Nucleation and decay initiation are the stiffness-sensitive phases of focal adhesion maturation. Biophys. J. 101, 2919–2928. doi:10.1016/J.BPJ.2011.11.010
Wang, J. G., Miyazu, M., Matsushita, E., Sokabe, M., and Naruse, K. (2001). Uniaxial cyclic stretch induces focal adhesion kinase (FAK) tyrosine phosphorylation followed by mitogen-activated protein kinase (MAPK) activation. Biochem. Biophys. Res. Commun. 288, 356–361. doi:10.1006/bbrc.2001.5775
Wang, Y., Sherrard, A., Zhao, B., Melak, M., Trautwein, J., Kleinschnitz, E.-M., et al. (2019). GPCR-induced calcium transients trigger nuclear actin assembly for chromatin dynamics. Nat. Commun. 10, 5271. doi:10.1038/s41467-019-13322-y
Wei, C., Wang, X., Zheng, M., and Cheng, H. (2012). Calcium gradients underlying cell migration. Curr. Opin. Cell Biol. 24, 254–261. doi:10.1016/j.ceb.2011.12.002
Weins, A., Schlondorff, J. S., Nakamura, F., Denker, B. M., Hartwig, J. H., Stossel, T. P., et al. (2007). Disease-associated mutant alpha-actinin-4 reveals a mechanism for regulating its F-actin-binding affinity. Proc. Natl. Acad. Sci. U. S. A. 104, 16080–16085. doi:10.1073/pnas.0702451104
Weißenbruch, K., Grewe, J., Hippler, M., Fladung, M., Tremmel, M., Stricker, K., et al. (2021). Distinct roles of nonmuscle myosin ii isoforms for establishing tension and elasticity during cell morphodynamics. Elife 10, e71888. doi:10.7554/ELIFE.71888
Wirtz, D. (2009). Particle-tracking microrheology of living cells: principles and applications. Annu. Rev. Biophys. 38, 301–326. doi:10.1146/annurev.biophys.050708.133724
Wolfenson, H., Meacci, G., Liu, S., Stachowiak, M. R., Iskratsch, T., Ghassemi, S., et al. (2015). Tropomyosin controls sarcomere-like contractions for rigidity sensing and suppressing growth on soft matrices. Nat. Cell Biol. 18, 33–42. doi:10.1038/ncb3277
Woll, K. A., and Van Petegem, F. (2021). Calcium-release channels: structure and function of IP3 receptors and ryanodine receptors. Physiol. Rev. 102, 209–268. doi:10.1152/physrev.00033.2020
Wu, J., Dickinson, R. B., and Lele, T. P. (2012). Investigation of in vivo microtubule and stress fiber mechanics with laser ablation. Integr. Biol. 4, 471–479. doi:10.1039/c2ib20015e
Xu, J., Tseng, Y., and Wirtz, D. (2000). Strain hardening of actin filament networks: REGULATION by the dynamic CROSS-LINKING protein α-ACTININ. J. Biol. Chem. 275, 35886–35892. doi:10.1074/jbc.M002377200
Yamaguchi, N., and Knaut, H. (2022). Focal adhesion-mediated cell anchoring and migration: from in vitro to in vivo. Development 149, dev200647. doi:10.1242/dev.200647
Yang, C., and Glass, W. F. (2008). Expression of alpha-actinin-1 in human glomerular mesangial cells in vivo and in vitro. Exp. Biol. Med. 233, 689–693. doi:10.3181/0710-RM-279
Yao, M., Goult, B. T., Chen, H., Cong, P., Sheetz, M. P., and Yan, J. (2014). Mechanical activation of vinculin binding to talin locks talin in an unfolded conformation. Sci. Rep. 4, 4610–4617. doi:10.1038/srep04610
Yoshigi, M., Hoffman, L. M., Jensen, C. C., Yost, H. J., and Beckerle, M. C. (2005). Mechanical force mobilizes zyxin from focal adhesions to actin filaments and regulates cytoskeletal reinforcement. J. Cell Biol. 171, 209–215. doi:10.1083/JCB.200505018
Zhao, B., Li, L., Lei, Q., and Guan, K.-L. (2010). The Hippo-YAP pathway in organ size control and tumorigenesis: an updated version. Genes Dev. 24, 862–874. doi:10.1101/gad.1909210
Zhou, D. W., Lee, T. T., Weng, S., Fu, J., and García, A. J. (2017). Effects of substrate stiffness and actomyosin contractility on coupling between force transmission and vinculin-paxillin recruitment at single focal adhesions. Mol. Biol. Cell 28, 1901–1911. doi:10.1091/MBC.E17-02-0116
Keywords: stress fiber, focal adhesion, viscoelasticity, crosslinking protein, rigidity sensing, extracellular matrix, cell migration, mechanotransduction
Citation: Katsuta H, Sokabe M and Hirata H (2024) From stress fiber to focal adhesion: a role of actin crosslinkers in force transmission. Front. Cell Dev. Biol. 12:1444827. doi: 10.3389/fcell.2024.1444827
Received: 06 June 2024; Accepted: 01 August 2024;
Published: 13 August 2024.
Edited by:
Akiko Mammoto, Medical College of Wisconsin, United StatesReviewed by:
Yifan Yuan, Yale University, United StatesKaustabh Ghosh, Department of Ophthalmology at UCLA, and Doheny Eye Institute, United States
Copyright © 2024 Katsuta, Sokabe and Hirata. This is an open-access article distributed under the terms of the Creative Commons Attribution License (CC BY). The use, distribution or reproduction in other forums is permitted, provided the original author(s) and the copyright owner(s) are credited and that the original publication in this journal is cited, in accordance with accepted academic practice. No use, distribution or reproduction is permitted which does not comply with these terms.
*Correspondence: Hiroaki Hirata, aGlyYXRhQG5lcHR1bmUua2FuYXphd2EtaXQuYWMuanA=