- 1Division of Biological Science, Graduate School of Science and Technology, Nara Institute of Science and Technology, Nara, Japan
- 2Institut Curie, PSL Research University, Centre national de la recherche scientifique (CNRS), Paris, France
- 3Data Science Center, Nara Institute of Science and Technology, Nara, Japan
- 4Center for Digital Green-innovation, Nara Institute of Science and Technology, Nara, Japan
Extracellular vesicles (EVs) are crucial for transferring bioactive materials between cells and play vital roles in both health and diseases. Cellular protrusions, including filopodia and microvilli, are generated by the bending of the plasma membrane and are considered to be rigid structures facilitating various cellular functions, such as cell migration, adhesion, and environment sensing. Compelling evidence suggests that these protrusions are dynamic and flexible structures that can serve as sources of a new class of EVs, highlighting the unique role they play in intercellular material transfer. Cytonemes are specialized filopodia protrusions that make direct contact with neighboring cells, mediating the transfer of bioactive materials between cells through their tips. In some cases, these tips fuse with the plasma membrane of neighboring cells, creating tunneling nanotubes that directly connect the cytosols of the adjacent cells. Additionally, virus particles can be released from infected cells through small bud-like of plasma membrane protrusions. These different types of protrusions, which can transfer bioactive materials, share common protein components, including I-BAR domain-containing proteins, actin cytoskeleton, and their regulatory proteins. The dynamic and flexible nature of these protrusions highlights their importance in cellular communication and material transfer within the body, including development, cancer progression, and other diseases.
1 Introduction
Extracellular vesicles (EVs) play a crucial role in exchanging bioactive materials, such as proteins, nucleic acids, metabolites, and lipids, between cells (Thery et al., 2018) (Figure 1A). These EVs are naturally secreted by most cells and can be found in various biological fluids, including blood, urine, saliva, cerebrospinal fluid, amniotic fluid, and seminal fluid (Yáñez-Mó et al., 2015). As studies on EVs expand under various conditions, different nomenclatures are used depending on different experimental models, molecular markers, biological conditions, and discovered roles. Efforts are being made to standardize terminology among researchers working in the field of EVs (Welsh et al., 2024). The exact process of EV biogenesis is not completely understood, but one major source of EVs is endosomes, which are the membrane organelles for material transport to/from the plasma membrane and to lysosomes. Intraluminal vesicles (ILVs) in endosomes are secreted by the fusion of endosomes with the plasma membrane. While the term “exosomes” is often used interchangeably with EVs, exosomes specifically refer to EVs derived from endosomes (Welsh et al., 2024). On the other hand, EVs can also be derived directly from the plasma membrane and are known as microvesicles or ectosomes (D’Angelo et al., 2023; Rilla, 2021). Importantly, the name, microvesicles, implies that these microvesicles might be larger than the endosome-derived EVs or exosomes. However, there are overlaps in their sizes, and they cannot be completely separated using ultra-centrifugal separation, which gives the “small EV” and “large EV” fractions (Hu et al., 2021; Welsh et al., 2024) (Figure 1B). Cells continuously reshape their plasma membrane, extending various kinds of membrane protrusions (Chhabra and Higgs, 2007). Membrane protrusions were traditionally considered to be stiff and rigid structures used for cell migration and adhesion, serving as substrate anchors. However, emerging studies suggest that some cellular protrusions are dynamic and flexible, can exist in diverse forms, and can be transformed into EVs (D’Angelo et al., 2023; Rilla, 2021).
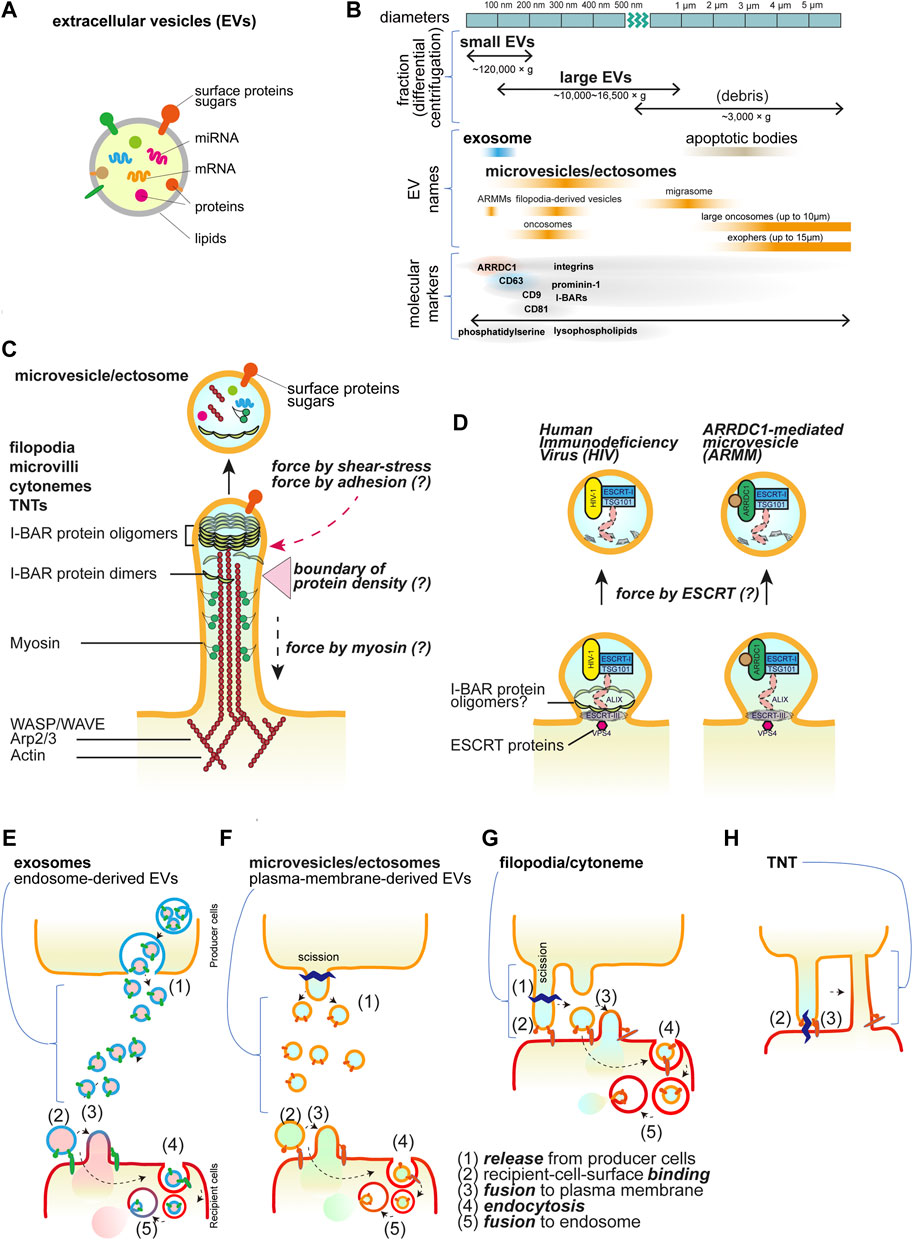
Figure 1. Extracellular vesicles and cellular protrusions. (A) The schematic illustration of the extracellular vesicles. (B) The EV nomenclature in fractionation and the others. The approximate sizes in diameter are shown with the names in the centrifugal separation of EVs as well as the commonly used names, i.e., exosomes and microvesicles/ectosomes. The names dependent on the origins are also shown. ARRDC1-mediated microvesicles (ARRMs) (Nabhan et al., 2012), filopodia-derived vesicles (FDVs) (Nishimura et al., 2021), migrasomes from the cellular retraction fibers (Ma et al., 2015), oncosomes from cancer cells (Meehan et al., 2016), exophers that contain the subcellular organelles and protein aggregates (Melentijevic et al., 2017), and apoptotic bodies that are the fragments of the apoptotic cells (Kakarla et al., 2020). The representative proteins that are analyzed for EVs are shown, though there is incomplete correspondence to the fractionation and the names. (C) The core proteins of the cellular protrusions of filopodia, cytonemes, microvilli, and tunneling nanotubes (TNTs). The actin filaments and motor protein myosins on the filaments, their regulators, and the I-BAR proteins are the key core proteins. The protrusions can release the vesicles by cutting, which occurs by the friction force of the shear flow and presumably by the pulling force associated with the adhesion. The differential localization of the core proteins will make a boundary for cutting. (D) Small buds of the plasma membrane for vesicle release. The HIV and ARMM are generated by the ESCRT protein complex to mediate the cutting. The I-BAR protein is involved in HIV release. (E-H) Schematics of transfer of the bioactive material inside vesicles or by TNTs. Vesicles are released and then bind to the recipient cells, followed by the fusion with the plasma membrane or by the endocytosis of the vesicles. The endocytosed vesicles are then fused to the endosomal membrane to release the materials inside the vesicles. Each step is marked by the numbers: (1) release from producer cells, (2) recipient-cell-surface binding, (3) fusion to plasma membrane, (4) endocytosis, and (5) fusion to endosome. (E) Exosomes, (F) microvesicles or ectosomes, (G) filopodia/cytonemes, which are the same as (F), but the vesicles are generated after binding to the recipient cells, i.e., no free travel of vesicles occurs, and (H) TNTs, which have the tip of the protrusions fused to the recipient cells, facilitate the connection of the cytoplasm.
In this review, we will discuss the similarities between different types of plasma membrane protrusions, including filopodia, cytoneme, microvilli, tunneling nanotubes (TNTs), cilia, pseudopods (pro platelets) from megakaryocytes, and small protrusions of viral budding, and their role as sources of EVs.
2 The building blocks of the protrusions
2.1 Cytoplasmic proteins
Most membrane protrusions, including filopodia at the cell periphery and microvilli at the apical surface of cells, share similar proteins (Figure 1C). Actin filaments are the cellular cytoskeleton that provides mechanical stuffiness. The actin filaments are generated through actin polymerization, which is dependent on the Arp2/3 complex and WASP/WAVE proteins, together with the barbed end nucleation factors of mDia and Ena/VASP family proteins and the barbed end-capping proteins of Eps8 (Mattila and Lappalainen, 2008; Jacquemet et al., 2015; Blake and Gallop, 2023). Myosin motor proteins also cooperate in the formation of filopodia and microvilli (Tokuo and Ikebe, 2004; McConnell et al., 2009; Meenderink et al., 2019; Gaeta et al., 2021). The proteins with the inverse Bin-Amphiphysin-Rvs (I-BAR) domain-containing proteins (I-BAR proteins), such as IRSp53, IRTKS, and MIM, connect the actin cytoskeleton to the protrusion membrane (Suetsugu et al., 2006; Scita et al., 2008). These I-BAR proteins can induce protrusion formation directly and are associated with the production of EVs (Hu et al., 2020; Nishimura et al., 2021; de Poret et al., 2022). The I-BAR domain alone can remodel the membrane into protrusions directly, and there is a zone with fewer actin filaments at the tip of the protrusions (Suetsugu et al., 2006; Sudhaharan et al., 2019). The simulation indicates that I-BAR protein recruitment or membrane bending ability can spontaneously induce the pearl/beads-on-a-string-like structure, which can be the precursors of the EVs (Suetsugu et al., 2006; Ravid et al., 2023). The production of phosphatidic acid by the cytoplasmic phospholipase D2 enhances protrusion formation, highlighting the involvement of lipid metabolism (Shen et al., 2002). The I-BAR-dependent EVs are enriched in one acyl-chain lysophospholipids, which potentially makes membrane fragile (Nishimura et al., 2021). Therefore, the tip of the membrane, lacking actin filaments, is potentially a fragile structure that may be permissive to fission and serves as the source of EVs.
The physiologically relevant friction force exerted on the protrusions by the flow of the medium plays a key role in the cutting of the tip (Nishimura et al., 2021). Shear stress induced by fluid flow has been shown to trigger vesiculation from the cells cultured under constant agitation (Mohieldin et al., 2021; Nishimura et al., 2021). Several studies have also suggested the possibility that shear force from blood flow in capillaries influences EV production (Hyenne et al., 2019; Verweij et al., 2019). Additionally, the pulling force that is applied to protrusions upon contact with neighbor cells or the substratum is also considered to provide the force for tip scission as well as friction due to cellular contractility or tissue deformation.
The endosomal sorting complex required for transport (ESCRT) machinery is a sequential cytoplasmic protein assembly involved in deforming and cutting lipid membranes. Plasma membrane budding and the release for the human immunodeficiency virus-1 (HIV-1) particles and small ectosomes ARRDC1-mediated microvesicles (ARRMs) are under the control of ESCRT machinery (Nabhan et al., 2012; Votteler and Sundquist, 2013). Furthermore, HIV-1 and Pseudorabies virus utilize IRSp53 for their budding (Yu et al., 2019; Inamdar et al., 2021) (Figure 1D), although, the involvement of ESCRT in cellular protrusions is still not fully understood.
It is unclear how the length of the protrusion can be determined. External cues, including growth factor stimulation, enhance protrusion formation through the activation of cellular signaling proteins, including tyrosine kinases and small GTPases. The above-mentioned actin regulators and I-BAR proteins are all cytoplasmic proteins, lacking transmembrane regions, and can be assembled through multivalent protein interactions downstream of these signaling proteins (Feng et al., 2022; Wan Mohamad Noor et al., 2023).
2.2 Transmembrane proteins
Representative transmembrane proteins at protrusions include receptor tyrosine kinases, cell adhesion proteins such as cadherins for cell-cell interactions, and integrins for cell-substratum adhesion proteins with the extracellular matrix (Rabinovitz and Mercurio, 1997; Vasioukhin et al., 2000; Robles et al., 2005). The cell adhesion status, including the area that forms the adhesion contact, greatly affects filopodia formation (Mukherjee et al., 2023). Furthermore, a pentaspan transmembrane glycoprotein, Prominin-1/CD133 (Weigmann et al., 1997) and tetraspan transmembrane proteins, tetraspanin, including CD9 and CD81 (Peñas et al., 2000) are enriched at plasma membrane protrusions. Tetraspanin CD63 is especially enriched in endosome membranes but can also localize to the plasma membrane through endosome fusion with the plasma membrane (Welsh et al., 2024; D’Angelo et al., 2023) (Figure 1E). Endosome-derived EV formation depends on the ESCRT, while plasma-membrane-derived vesicles can serve as an alternative pathway when ESCRT is inhibited (Nishimura et al., 2021). Hyaluronan synthases, including HAS3, are also enriched in protrusions (Rilla et al., 2013). These proteins often serve as marker proteins for EVs. However, the causality of these proteins in the formation of EVs has been enigmatic.
3 Protrusions in the inter-cellular material transfer
3.1 Filopodia
Filopodia are finger-like protrusions of the cell membrane, having 0.1–0.3 microns in diameter, and with varying lengths. Filopodia can participate in a broad range of cellular processes, including cell migration and adhesion to the extracellular matrix (Arjonen et al., 2011), cue-sensing (Heckman and Plummer, 2013), tissue morphogenesis (Fairchild and Barna, 2014), and pathogen invasion (Chang et al., 2016). Filopodia are well characterized at the leading edge of migrating cells, dendrite and growth cone of neuronal cells.
Emerging studies demonstrating that filopodia transmit signaling molecules were mostly conducted in Drosophila (Hsiung et al., 2005; Callejo et al., 2011; Roy et al., 2011; Bilioni et al., 2013; Bischoff et al., 2013; Fereres et al., 2019; Patel et al., 2022; Clements et al., 2024), human cell lines (Stanganello et al., 2015; Mattes et al., 2018; Hu et al., 2020; Brunt et al., 2021; Nishimura et al., 2021; de Poret et al., 2022), and vertebrates such as chick embryos (Sanders et al., 2013), zebrafish (Stanganello et al., 2015; Mattes et al., 2018; Brunt et al., 2021), and mice (Mattes et al., 2018; Brunt et al., 2021; Hall et al., 2021) (Table 1). The maximum recorded length of filopodia in Drosophila wing imaginal disc is surprisingly longer than those reported in vertebrates, which are 700 µm and 150 μm, respectively (Ramirez-Weber and Kornberg, 1999; Sanders et al., 2013), implying the variety dependent on experimental models. Filopodia in vertebrates and invertebrates are able to transfer signaling molecules, such as Decapentaplegic (Dpp) (Hsiung et al., 2005; Roy et al., 2011; Fereres et al., 2019), Hedgehog (Hh) (Callejo et al., 2011; Bilioni et al., 2013; Bischoff et al., 2013; Gradilla et al., 2014), Sonic Hedgehog (Shh) (Sanders et al., 2013; Hall et al., 2021; Hall et al., 2024), Notch (de Joussineau et al., 2003; Cohen et al., 2010; Clements et al., 2024), and Wnt (Stanganello et al., 2015; Mattes et al., 2018; Brunt et al., 2021; Hall et al., 2024) to distant cells through physical contact or vesicle release (Table 1). Several of the above-mentioned filopodia and vesicle are dependent on the I-BAR proteins (Table 1). In several studies, plasma-membrane-derived vesicles do not seem to travel through the body fluid or the culture medium. Rather, the tip appears to bind to the recipient cell, after which the tip is excised to form the vesicle. Alternately, if recipient cells are in a close vicinity, the vesicles will travel a very short distance before being immediately captured. These observations suggest that the transfer by plasma-membrane-derived EVs and by filopodia are similar (Figures 1F, G).
The recipient cells receive these EVs presumably through their surface receptors. Subsequently, the EVs either fuse with the plasma membrane or are endocytosed and then fuse with endosomes to release their content. Although the mechanisms underlying the release of the bioactive cargos from EVs are largely unknown, they are supposed to be common to those for the endosome-derived EVs (Figures 1E–G).
3.2 Cytonemes
The potential role of filopodia in intercellular signaling was indicated nearly 25 years ago, when long, slender cellular protrusions emerging from Drosophila wing imaginal disc, named “cytonemes,” were shown to orient toward morphogen (Ramirez-Weber and Kornberg, 1999). In the term “cytoneme,” “cyto” refers to the presence of cytoplasmic materials, and “neme” denotes the finger-like appearance, which is the feature of filopodia. Although it is still unclear whether all filopodia are capable of exchanging signals between cells, cytoneme (Hsiung et al., 2005; Mattes et al., 2018), signaling filopodia (Clements et al., 2024), and specialized filopodia (Sanders et al., 2013) are considered to refer the bona fide filopodia.
3.3 Microvilli
Microvilli and filopodia share a similar molecular architecture, consisting of dense, parallel actin bundles and contain I-BAR proteins and myosin (Figure 1C). Microvilli are often found on the apical surface of epithelial cells, where they provide a larger surface area for nutrient absorption. However, new research suggests a novel role for microvilli as a source of EVs. Studies have shown that the neuroepithelium microvilli in the mouse brain can release prominin-1-containing EVs, which function in tissue differentiation (Marzesco et al., 2005). These EVs appear to preferentially bind to protrusion sites on both epithelial and non-epithelial cells (Weigmann et al., 1997; Marzesco et al., 2005; Karbanová et al., 2008). The shedding of EVs from the distal tips of microvilli has also been reported in various tissues, including Drosophila wing imaginal disc epithelium (Hurbain et al., 2022), rat enterocytes (McConnell et al., 2009), and placenta (Davies et al., 2022). Prominin-1 has a cholesterol-binding domain, and the removal of cholesterol has been shown to alter the distribution of prominin at the microvilli (Röper et al., 2000; Marzesco et al., 2009). The depletion of cholesterol from microvilli renders the microvilli unstable and triggers the release of EVs from the barbed end of protrusions (Marzesco et al., 2009). Myosins, known for transporting proteins to the tips of filopodia, have also been implicated in driving the release of EVs from microvilli, such as myosin-1a in the intestinal lumen (McConnell et al., 2009). The involvement of cytoskeletal proteins and BAR protein in the shedding of microvilli EVs is still unknown, and it will be an interesting topic for future research.
3.4 Tunneling nanotubes
Tunneling nanotubes (TNTs) were first identified in rat pheochromocytoma PC12 cells as the actin-rich membrane protrusions that connect distant cells and transport membranous vesicles (Rustom et al., 2004). Emerging studies revealed the presence of TNTs in various cell types, including immune cell line (Onfelt et al., 2006; Chinnery et al., 2008; Sowinski et al., 2008; Dupont et al., 2020), epithelial cells (Gurke et al., 2008; Wang et al., 2010; Wang and Gerdes, 2012), neuronal cells (Gousset et al., 2009; Wang X. et al., 2012; Wang and Gerdes, 2012), adenocarcinoma (Wang Z.-G. et al., 2012; Wang and Gerdes, 2012), vascular endothelial cells (Wang and Gerdes, 2012), rat cardiomyocytes (Koyanagi et al., 2005), and myoblast cell (He et al., 2010). The feature of TNTs is different from filopodia or cytoneme, where TNTs are open-ended structures that transport the cargo along the long tubules to the distant cells without exocytosis and touching the substratum (Rustom et al., 2004; Abounit and Zurzolo, 2012; Dupont et al., 2018) (Figure 1H). The interconnected tubes are transient and sensitive to stresses such as light exposure and frictional force (Rustom et al., 2004). The reported lengths of TNTs vary, from a few micrometers to a few hundred micrometers, and the maximum recorded length is 300 µm (Rustom et al., 2004; Chinnery et al., 2008; He et al., 2010; Wang Z.-G. et al., 2012).
Some studies reported that there are two subtypes of TNTs, which are constituted of both actin and microtubules or actin alone, with different diameters (Onfelt et al., 2006; Wang X. et al., 2012; Wang Z.-G. et al., 2012). IRSp53 and Eps8 are involved in the TNT formation (Henderson et al., 2023). Driven by actin polymerization, TNTs extend from a cell, and the distal tip fuses with the plasma membrane of another cell. The biological roles of TNTs include transporting organelle in between cells (Onfelt et al., 2006; Gurke et al., 2008; Wang and Gerdes, 2015), transferring receptor complexes to mediate cell immune responses (Chinnery et al., 2008), and HIV-1 transmission (Sowinski et al., 2008; Dupont et al., 2020). The mechanism of transportation was reported to be facilitated by Myosin Va, a protein involved in organelle transport (Rustom et al., 2004).
3.5 Viral budding
One of the prerequisites for the budding of viral particles, such as those of HIV-1, is the formation of membrane curvature. The membrane budding was thought to be solely dependent on the virus Gag protein, but studies revealed that the I-BAR protein, IRSp53, plays a crucial role in the assembly and budding of the viral particles (Thomas et al., 2015; Inamdar et al., 2021). Both Gag and IRSp53 can interact with phosphatidylinositol 4,5-bisphosphate (PIP2) in the inner leaflet of plasma membrane (Prévost et al., 2015; Favard et al., 2019; Sengupta et al., 2019), and the activation of small GTPase, Rac1, at the IRSp53-Gag localized membrane drives the viral particle release (Thomas et al., 2015). The involvement of actin cytoskeleton in the virus budding was also reported, where the IRSp53 recruits WAVE2 and Arp2/3 for actin polymerization in the budding site (Thomas et al., 2015). A recent finding in neuronal cells reported that IRSp53 can interact with Arc, which is an intrinsic protein that has similarity with retroviral Gag (Alicia et al., 2024). IRSp53 can facilitate the oligomerization of Arc into capsid at the membrane protrusion site and release EVs that contain the Arc capsid and mRNA (Alicia et al., 2024). Other than IRSp53, Gag, and Arc, the budding and release of viral particles were dependent on the ESCRT machinery (Votteler and Sundquist, 2013). Nevertheless, the interplay between these proteins in the membrane site for virus budding is still yet to be fully discovered.
3.6 Proplatelet protrusions from megakaryocytes
Platelets and microvesicles/ectosomes have similar origins, as both are generated through the scission of proplatelets, which are the pseudopod protrusions of megakaryocytes (Behnke, 1968; Machlus and Italiano, 2013). Furthermore, platelets were among the first to demonstrate secretion of EVs. The activated platelets have abundant protrusions on their surface (Sorrentino et al., 2021; Zheng et al., 2021), and the first discovery of EVs arose from particles derived from the platelets, known as “platelet dust,” where initially perceived as cellular waste (Wolf, 1967). The later research revealed that the formation of EVs from most cell types was not always a random process of excretion of waste but actively driven by intricate cellular mechanisms (Raposo and Stoorvogel, 2013; van Niel et al., 2018).
Megakaryocytes are mainly present in bone marrow and are responsible for platelet biogenesis (Ogawa, 1993; Morita et al., 2011). The plasma membrane of megakaryocytes is expandable to 10-fold to serve as the reservoir for cytoskeleton proteins and membrane lipids to generate thousands of platelets. The scission of proplatelets, i.e., the generation of platelets, also relies on shear stress (Ito et al., 2018), which might be reminiscent of the filopodia-derived vesicles.
In proplatelets shedding, the microtubules and actin filaments play crucial roles (Patel et al., 2005; Thon et al., 2010; Machlus and Italiano, 2013). The polymerization of microtubules powers the elongation of proplatelets in cooperation with a microtubule minus end-associated protein, dynein (Lecine et al., 2000; Patel et al., 2005; Machlus et al., 2019). The lack of functional tubulin affects human platelet production as the tubulin mutation was identified in the patient with macrothrombocytopenia (Kunishima et al., 2009).
3.7 Cilia
There are two types of cilia, called motile cilia and non-motile cilia. Motile cilia are hair-like protrusions on the surface of epithelial cells underlying the respiratory tract, oviduct, and brain ventricular system, whereas non-motile cilia, such as primary cilium, is the solitary sensory organelle projected from the apical surface of differentiated, non-dividing-cells. Motile cilia facilitate the transport of substances along a passage through wave-like beating motion (Zhou and Roy, 2015), whereas primary cilium has abundant receptors at the tip, and acts as the antenna that transmits signals between cells (Anvarian et al., 2019). Both the motile-cilia and primary cilia on epithelial cells have been evidenced to release EVs from the distal tips (Dubreuil et al., 2007; Kesimer et al., 2009) as well as from the base of the protrusions (Wang et al., 2021).
Contrary to filopodia and microvilli, the core of cilia is composed of microtubule filaments. Therefore, cilia are not generally considered to be similar to filopodia. However, there are several molecular similarities in the EV release. The mechanism of EV secretion from cilia involves the cooperation of actin and myosin components (Nager et al., 2017; Phua et al., 2017). EV secretion from cilia can be triggered by the actin regulatory protein, drebrin, myosin 6 (Nager et al., 2017), as well as phospholipid PIP2 (Phua et al., 2017). Similar to the EVs from microvilli, the EVs released from the neuroepithelial primary cilium contain prominin-1 (Dubreuil et al., 2007), and the shedding of EVs from cilia serves in transmitting Hh signaling (Nager et al., 2017).
4 Membrane protrusions and EVs in cancer cells
Studies have shown that the abundance of membrane protrusive structures, such as filopodia, is highly related to cancer progression and metastasis. In cancer, increased filopodia density often correlates with cell migration and metastasis of cancer cells (Jacquemet et al., 2015; Jacquemet et al., 2017). Several studies have shown that cancer cells secrete more EVs as observed in cancer-patient samples in comparison with healthy patients-derived samples (Khan et al., 2012; Puhka et al., 2017), and in vitro comparison of cancer cells with their non-cancer cellular counterparts (Riches et al., 2014).
Furthermore, EVs from cancer cells exhibit a unique profile compared to those of normal cells (György et al., 2011). Likewise, commonly used chemotherapeutic regimen affects EV distribution and function (Tzoran et al., 2015; Aharon et al., 2017) by enhancing the release of EVs with pro-metastatic (Keklikoglou et al., 2019; Wills et al., 2021), pro-chemotherapeutic resistance (Wang et al., 2019), and pro-angiogenic (Zarfati et al., 2019) abilities. The EVs derived from cancer cell membrane protrusions have also been reported to promote tumorigenesis and metastasis (Muralidharan-Chari et al., 2009; Härkönen et al., 2019; Hu et al., 2020).
Although these studies have not completely examined the protrusions as their EV sources, these findings strongly suggest the importance of membrane protrusions as a platform for the generation of cancer cell-derived EVs, which will be useful as prognostic markers or therapeutic targets.
5 Conclusion and perspective
The plasma membrane serves as a versatile platform that can generate various forms of protrusive structures. These protrusions play a crucial role in the generation of EVs that are important for cell-to-cell communication. Given the diverse varieties of protrusions and the understanding of their molecular mechanisms, protrusion-derived EVs can be a powerful tool in disease prognosis, especially in cancer. By elucidating the components involved in EV secretion from protrusive structures, it is possible to utilize a protrusive structure as a personalized system for EV secretion in cancer therapy.
Author contributions
HTH: Writing–original draft, Writing–review and editing, Investigation. TN: Writing–review and editing. HK: Writing–review and editing. RD: Writing–original draft. GD’A: Writing–original draft, Conceptualization, Writing–review and editing. SS: Conceptualization, Writing–review and editing, Writing–original draft.
Funding
The author(s) declare that financial support was received for the research, authorship, and/or publication of this article. Japan Science and Technology Corporation (JPMJCR1863), Japan Society for the Promotion of Science (JP20KK0341, JP20H03252, 24H01286, and 24K02024), the European Union, EVCA Twining project (Horizon GA no. 101079264).
Conflict of interest
The authors declare that the research was conducted in the absence of any commercial or financial relationships that could be construed as a potential conflict of interest.
The author(s) declared that they were an editorial board member of Frontiers, at the time of submission. This had no impact on the peer review process and the final decision.
Publisher’s note
All claims expressed in this article are solely those of the authors and do not necessarily represent those of their affiliated organizations, or those of the publisher, the editors and the reviewers. Any product that may be evaluated in this article, or claim that may be made by its manufacturer, is not guaranteed or endorsed by the publisher.
References
Abounit, S., and Zurzolo, C. (2012). Wiring through tunneling nanotubes–from electrical signals to organelle transfer. J. Cell Sci. 125, 1089–1098. doi:10.1242/jcs.083279
Aharon, A., Sabbah, A., Ben-Shaul, S., Berkovich, H., Loven, D., Brenner, B., et al. (2017). Chemotherapy administration to breast cancer patients affects extracellular vesicles thrombogenicity and function. Oncotarget 8, 63265–63280. doi:10.18632/oncotarget.18792
Alicia, R., Kaelan, S., Jenifer, E., Sevnur Kömürlü, K., Tom, K., Mitali, T., et al. (2024). Arc mediates intercellular synaptic plasticity via IRSp53-dependent extracellular vesicle biogenesis. bioRxiv 2024.01.30.578027.
Anvarian, Z., Mykytyn, K., Mukhopadhyay, S., Pedersen, L. B., and Christensen, S. T. (2019). Cellular signalling by primary cilia in development, organ function and disease. Nat. Rev. Nephrol. 15, 199–219. doi:10.1038/s41581-019-0116-9
Arjonen, A., Kaukonen, R., and Ivaska, J. (2011). Filopodia and adhesion in cancer cell motility. Cell Adhesion Migr. 5, 421–430. doi:10.4161/cam.5.5.17723
Behnke, O. (1968). An electron microscope study of the megacaryocyte of the rat bone marrow: I. The development of the demarcation membrane system and the platelet surface coat. J. Ultrastruct. Res. 24, 412–433. doi:10.1016/s0022-5320(68)80046-2
Bilioni, A., Sánchez-Hernández, D., Callejo, A., Gradilla, A.-C., Ibáñez, C., Mollica, E., et al. (2013). Balancing Hedgehog, a retention and release equilibrium given by Dally, Ihog, Boi and shifted/DmWif. Dev. Biol. 376, 198–212. doi:10.1016/j.ydbio.2012.12.013
Bischoff, M., Gradilla, A.-C., Seijo, I., Andrés, G., Rodríguez-Navas, C., González-Méndez, L., et al. (2013). Cytonemes are required for the establishment of a normal Hedgehog morphogen gradient in Drosophila epithelia. Nat. Cell Biol. 15, 1269–1281. doi:10.1038/ncb2856
Blake, T. C. A., and Gallop, J. L. (2023). Filopodia in vitro and in vivo. Annu. Rev. Cell Dev. Biol. 39, 307–329. doi:10.1146/annurev-cellbio-020223-025210
Brunt, L., Greicius, G., Rogers, S., Evans, B. D., Virshup, D. M., Wedgwood, K. C. A., et al. (2021). Vangl2 promotes the formation of long cytonemes to enable distant Wnt/β-catenin signaling. Nat. Commun. 12, 2058. doi:10.1038/s41467-021-22393-9
Callejo, A., Bilioni, A., Mollica, E., Gorfinkiel, N., Andres, G., Ibanez, C., et al. (2011). Dispatched mediates Hedgehog basolateral release to form the long-range morphogenetic gradient in the Drosophila wing disk epithelium. Proc. Natl. Acad. Sci. U. S. A. 108, 12591–12598. doi:10.1073/pnas.1106881108
Chang, K., Baginski, J., Hassan, S. F., Volin, M., Shukla, D., and Tiwari, V. (2016). Filopodia and viruses: an analysis of membrane processes in entry mechanisms. Front. Microbiol. 7, 300–313. doi:10.3389/fmicb.2016.00300
Chhabra, E. S., and Higgs, H. N. (2007). The many faces of actin: matching assembly factors with cellular structures. Nat. Cell Biol. 9, 1110–1121. doi:10.1038/ncb1007-1110
Chinnery, H. R., Pearlman, E., and McMenamin, P. G. (2008). Cutting edge: membrane nanotubes in vivo: a feature of MHC class II+ cells in the mouse cornea. J. Immunol. 180, 5779–5783. doi:10.4049/jimmunol.180.9.5779
Clements, R., Smith, T., Cowart, L., Zhumi, J., Sherrod, A., Cahill, A., et al. (2024). Myosin XV is a negative regulator of signaling filopodia during long-range lateral inhibition. Dev. Biol. 505, 110–121. doi:10.1016/j.ydbio.2023.11.002
Cohen, M., Georgiou, M., Stevenson, N. L., Miodownik, M., and Baum, B. (2010). Dynamic filopodia transmit intermittent delta-notch signaling to drive pattern refinement during lateral inhibition. Dev. Cell 19, 78–89. doi:10.1016/j.devcel.2010.06.006
D’Angelo, G., Raposo, G., Nishimura, T., and Suetsugu, S. (2023). Protrusion-derived vesicles: new subtype of EVs? Nat. Rev. Mol. Cell Biol. 24, 81–82. doi:10.1038/s41580-022-00555-x
Davies, R., Griffiths, C., Askelund, K., Palaiologou, E., Cleal, J. K., Page, A., et al. (2022). Microvillous tip vesicles may be an origin of placental extracellular vesicles. Placenta 123, 24–30. doi:10.1016/j.placenta.2022.04.003
de Joussineau, C., Soulé, J., Martin, M., Anguille, C., Montcourrier, P., and Alexandre, D. (2003). Delta-promoted filopodia mediate long-range lateral inhibition in Drosophila. Nature 426, 555–559. doi:10.1038/nature02157
de Poret, A., Dibsy, R., Merida, P., Trausch, A., Inamdar, K., and Muriaux, D. (2022). Extracellular vesicles containing the I-BAR protein IRSp53 are released from the cell plasma membrane in an Arp2/3 dependent manner. Biol. Cell 114, 259–275. doi:10.1111/boc.202100095
Dubreuil, V., Marzesco, A. M., Corbeil, D., Huttner, W. B., and Wilsch-Brauninger, M. (2007). Midbody and primary cilium of neural progenitors release extracellular membrane particles enriched in the stem cell marker prominin-1. J. Cell Biol. 176, 483–495. doi:10.1083/jcb.200608137
Dupont, M., Souriant, S., Balboa, L., Vu Manh, T. P., Pingris, K., Rousset, S., et al. (2020). Tuberculosis-associated IFN-I induces Siglec-1 on tunneling nanotubes and favors HIV-1 spread in macrophages. Elife 9, e52535. doi:10.7554/eLife.52535
Dupont, M., Souriant, S., Lugo-Villarino, G., Maridonneau-Parini, I., and Verollet, C. (2018). Tunneling nanotubes: intimate communication between myeloid cells. Front. Immunol. 9, 43. doi:10.3389/fimmu.2018.00043
Fairchild, C. L., and Barna, M. (2014). Specialized filopodia: at the ’tip' of morphogen transport and vertebrate tissue patterning. Curr. Opin. Genet. Dev. 27, 67–73. doi:10.1016/j.gde.2014.03.013
Favard, C., Chojnacki, J., Merida, P., Yandrapalli, N., Mak, J., Eggeling, C., et al. (2019). HIV-1 Gag specifically restricts PI(4,5)P2 and cholesterol mobility in living cells creating a nanodomain platform for virus assembly. Sci. Adv. 5, eaaw8651. doi:10.1126/sciadv.aaw8651
Feng, Z., Lee, S., Jia, B., Jian, T., Kim, E., and Zhang, M. (2022). IRSp53 promotes postsynaptic density formation and actin filament bundling. J. Cell Biol. 221, e202105035. doi:10.1083/jcb.202105035
Fereres, S., Hatori, R., Hatori, M., and Kornberg, T. B. (2019). Cytoneme-mediated signaling essential for tumorigenesis. PLoS Genet. 15, e1008415. doi:10.1371/journal.pgen.1008415
Gaeta, I. M., Meenderink, L. M., Postema, M. M., Cencer, C. S., and Tyska, M. J. (2021). Direct visualization of epithelial microvilli biogenesis. Curr. Biol. 31, 2561–2575.e6. doi:10.1016/j.cub.2021.04.012
Gousset, K., Schiff, E., Langevin, C., Marijanovic, Z., Caputo, A., Browman, D. T., et al. (2009). Prions hijack tunnelling nanotubes for intercellular spread. Nat. Cell Biol. 11, 328–336. doi:10.1038/ncb1841
Gradilla, A.-C., González, E., Seijo, I., Andrés, G., Bischoff, M., González-Mendez, L., et al. (2014). Exosomes as Hedgehog carriers in cytoneme-mediated transport and secretion. Nat. Commun. 5, 5649. doi:10.1038/ncomms6649
Gurke, S., Barroso, J. F., and Gerdes, H. H. (2008). The art of cellular communication: tunneling nanotubes bridge the divide. Histochem Cell Biol. 129, 539–550. doi:10.1007/s00418-008-0412-0
György, B., Szabó, T. G., Pásztói, M., Pál, Z., Misják, P., Aradi, B., et al. (2011). Membrane vesicles, current state-of-the-art: emerging role of extracellular vesicles. Cell. Mol. Life Sci. 68, 2667–2688. doi:10.1007/s00018-011-0689-3
Hall, E. T., Dillard, M. E., Cleverdon, E. R., Zhang, Y., Daly, C. A., Ansari, S. S., et al. (2024). Cytoneme signaling provides essential contributions to mammalian tissue patterning. Cell 187, 276–293 e23. doi:10.1016/j.cell.2023.12.003
Hall, E. T., Dillard, M. E., Stewart, D. P., Zhang, Y., Wagner, B., Levine, R. M., et al. (2021). Cytoneme delivery of Sonic Hedgehog from ligand-producing cells requires Myosin 10 and a Dispatched-BOC/CDON co-receptor complex. eLife 10, e61432. doi:10.7554/eLife.61432
Härkönen, K., Oikari, S., Kyykallio, H., Capra, J., Hakkola, S., Ketola, K., et al. (2019). CD44s assembles hyaluronan coat on filopodia and extracellular vesicles and induces tumorigenicity of MKN74 gastric carcinoma cells. Cells 8, 276. doi:10.3390/cells8030276
He, K., Luo, W., Zhang, Y., Liu, F., Liu, D., Xu, L., et al. (2010). Intercellular transportation of quantum dots mediated by membrane nanotubes. ACS Nano 4, 3015–3022. doi:10.1021/nn1002198
Heckman, C. A., and Plummer, H. K. (2013). Filopodia as sensors. Cell. Signal. 25, 2298–2311. doi:10.1016/j.cellsig.2013.07.006
Henderson, J. M., Ljubojevic, N., Belian, S., Chaze, T., Castaneda, D., Battistella, A., et al. (2023). Tunnelling nanotube formation is driven by Eps8/IRSp53-dependent linear actin polymerization. EMBO J. 42, e113761. doi:10.15252/embj.2023113761
Hsiung, F., Ramirez-Weber, F. A., Iwaki, D. D., and Kornberg, T. B. (2005). Dependence of Drosophila wing imaginal disc cytonemes on Decapentaplegic. Nature 437, 560–563. doi:10.1038/nature03951
Hu, H. T., Nishimura, T., and Suetsugu, S. (2021). Ultracentrifugal separation, characterization, and functional study of extracellular vesicles derived from serum-free cell culture. Star. Protoc. 2, 100625. doi:10.1016/j.xpro.2021.100625
Hu, H. T., Sasakura, N., Matsubara, D., Furusawa, N., Mukai, M., Kitamura, N., et al. (2020). Involvement of I-BAR protein IRSp53 in tumor cell growth via extracellular microvesicle secretion. bioRxiv 2020.04.20.050492.
Hurbain, I., Macé, A.-S., Romao, M., Prince, E., Sengmanivong, L., Ruel, L., et al. (2022). Microvilli-derived extracellular vesicles carry Hedgehog morphogenic signals for Drosophila wing imaginal disc development. Curr. Biol. 32, 361–373.e6. doi:10.1016/j.cub.2021.11.023
Hyenne, V., Ghoroghi, S., Collot, M., Bons, J., Follain, G., Harlepp, S., et al. (2019). Studying the fate of tumor extracellular vesicles at high spatiotemporal resolution using the zebrafish embryo. Dev. Cell 48, 554–572. doi:10.1016/j.devcel.2019.01.014
Inamdar, K., Tsai, F.-C., Dibsy, R., de Poret, A., Manzi, J., Merida, P., et al. (2021). Full assembly of HIV-1 particles requires assistance of the membrane curvature factor IRSp53. eLife 10, e67321. doi:10.7554/eLife.67321
Ito, Y., Nakamura, S., Sugimoto, N., Shigemori, T., Kato, Y., Ohno, M., et al. (2018). Turbulence activates platelet biogenesis to enable clinical scale ex vivo production. Cell 174, 636–648. doi:10.1016/j.cell.2018.06.011
Jacquemet, G., Hamidi, H., and Ivaska, J. (2015). Filopodia in cell adhesion, 3D migration and cancer cell invasion. Curr. Opin. Cell Biol. 36, 23–31. doi:10.1016/j.ceb.2015.06.007
Jacquemet, G., Paatero, I., Carisey, A. F., Padzik, A., Orange, J. S., Hamidi, H., et al. (2017). FiloQuant reveals increased filopodia density during breast cancer progression. J. Cell Biol. 216, 3387–3403. doi:10.1083/jcb.201704045
Kakarla, R., Hur, J., Kim, Y. J., Kim, J., and Chwae, Y.-J. (2020). Apoptotic cell-derived exosomes: messages from dying cells. Exp. Mol. Med. 52, 1–6. doi:10.1038/s12276-019-0362-8
Karbanová, J., Missol-Kolka, E., Fonseca, A. V., Lorra, C., Janich, P., Hollerová, H., et al. (2008). The stem cell marker CD133 (Prominin-1) is expressed in various human glandular epithelia. J. Histochem Cytochem 56, 977–993. doi:10.1369/jhc.2008.951897
Keklikoglou, I., Cianciaruso, C., Güç, E., Squadrito, M. L., Spring, L. M., Tazzyman, S., et al. (2019). Chemotherapy elicits pro-metastatic extracellular vesicles in breast cancer models. Nat. Cell Biol. 21, 190–202. doi:10.1038/s41556-018-0256-3
Kesimer, M., Scull, M., Brighton, B., DeMaria, G., Burns, K., O’Neal, W., et al. (2009). Characterization of exosome-like vesicles released from human tracheobronchial ciliated epithelium: a possible role in innate defense. FASEB J. 23, 1858–1868. doi:10.1096/fj.08-119131
Khan, S., Jutzy, J. M. S., Valenzuela, M. M. A., Turay, D., Aspe, J. R., Ashok, A., et al. (2012). Plasma-derived exosomal survivin, a plausible biomarker for early detection of prostate cancer. PLoS ONE 7, e46737. doi:10.1371/journal.pone.0046737
Koyanagi, M., Brandes, R. P., Haendeler, J., Zeiher, A. M., and Dimmeler, S. (2005). Cell-to-cell connection of endothelial progenitor cells with cardiac myocytes by nanotubes: a novel mechanism for cell fate changes? Circulation Res. 96, 1039–1041. doi:10.1161/01.RES.0000168650.23479.0c
Kunishima, S., Kobayashi, R., Itoh, T. J., Hamaguchi, M., and Saito, H. (2009). Mutation of the beta1-tubulin gene associated with congenital macrothrombocytopenia affecting microtubule assembly. Blood 113, 458–461. doi:10.1182/blood-2008-06-162610
Lecine, P., Italiano, J. E., Kim, S.-W., Villeval, J.-L., and Shivdasani, R. (2000). Hematopoietic-specific β1 tubulin participates in a pathway of platelet biogenesis dependent on the transcription factor NF-E2. Blood 96, 1366–1373. doi:10.1182/blood.v96.4.1366.h8001366_1366_1373
Ma, L., Li, Y., Peng, J., Wu, D., Zhao, X., Cui, Y., et al. (2015). Discovery of the migrasome, an organelle mediating release of cytoplasmic contents during cell migration. Cell Res. 25, 24–38. doi:10.1038/cr.2014.135
Machlus, K. R., and Italiano, J. E. (2019). “2 - megakaryocyte development and platelet formation,” in Platelets. Editor A. D. Michelson Fourth Edition (Academic Press), 25–46.
Machlus, K. R., and Italiano, J. E. (2013). The incredible journey: from megakaryocyte development to platelet formation. J. Cell Biol. 201, 785–796. doi:10.1083/jcb.201304054
Marzesco, A. M., Janich, P., Wilsch-Bräuninger, M., Dubreuil, V., Langenfeld, K., Corbeil, D., et al. (2005). Release of extracellular membrane particles carrying the stem cell marker prominin-1 (CD133) from neural progenitors and other epithelial cells. J. Cell Sci. 118, 2849–2858. doi:10.1242/jcs.02439
Marzesco, A. M., Wilsch-Bräuninger, M., Dubreuil, V., Janich, P., Langenfeld, K., Thiele, C., et al. (2009). Release of extracellular membrane vesicles from microvilli of epithelial cells is enhanced by depleting membrane cholesterol. FEBS Lett. 583, 897–902. doi:10.1016/j.febslet.2009.01.048
Mattes, B., Dang, Y., Greicius, G., Kaufmann, L. T., Prunsche, B., Rosenbauer, J., et al. (2018). Wnt/PCP controls spreading of Wnt/β-catenin signals by cytonemes in vertebrates. eLife 7, e36953. doi:10.7554/eLife.36953
Mattila, P. K., and Lappalainen, P. (2008). Filopodia: molecular architecture and cellular functions. Nat. Rev. Mol. Cell Biol. 9, 446–454. doi:10.1038/nrm2406
McConnell, R. E., Higginbotham, J. N., Shifrin, D. A., Tabb, D. L., Coffey, R. J., and Tyska, M. J. (2009). The enterocyte microvillus is a vesicle-generating organelle. J. Cell Biol. 185, 1285–1298. doi:10.1083/jcb.200902147
Meehan, B., Rak, J., and Di Vizio, D. (2016). Oncosomes - large and small: what are they, where they came from? J. Extracell. Vesicles 5, 33109. doi:10.3402/jev.v5.33109
Meenderink, L. M., Gaeta, I. M., Postema, M. M., Cencer, C. S., Chinowsky, C. R., Krystofiak, E. S., et al. (2019). Actin dynamics drive microvillar motility and clustering during brush border assembly. Dev. Cell 50, 545–556. doi:10.1016/j.devcel.2019.07.008
Melentijevic, I., Toth, M. L., Arnold, M. L., Guasp, R. J., Harinath, G., Nguyen, K. C., et al. (2017). C. elegans neurons jettison protein aggregates and mitochondria under neurotoxic stress. Nature 542, 367–371. doi:10.1038/nature21362
Mohieldin, A. M., Pala, R., Beuttler, R., Moresco, J. J., Yates, J. R., and Nauli, S. M. (2021). Ciliary extracellular vesicles are distinct from the cytosolic extracellular vesicles. J. Extracell. Vesicles 10, e12086. doi:10.1002/jev2.12086
Morita, Y., Iseki, A., Okamura, S., Suzuki, S., Nakauchi, H., and Ema, H. (2011). Functional characterization of hematopoietic stem cells in the spleen. Exp. Hematol. 39, 351–359. doi:10.1016/j.exphem.2010.12.008
Mukherjee, A., Ron, J. E., Hu, H. T., Nishimura, T., Hanawa-Suetsugu, K., Behkam, B., et al. (2023). Actin filaments couple the protrusive tips to the nucleus through the I-BAR domain protein IRSp53 during the migration of cells on 1D fibers. Adv. Sci. 10, 2207368. doi:10.1002/advs.202207368
Muralidharan-Chari, V., Clancy, J., Plou, C., Romao, M., Chavrier, P., Raposo, G., et al. (2009). ARF6-regulated shedding of tumor cell-derived plasma membrane microvesicles. Curr. Biol. 19, 1875–1885. doi:10.1016/j.cub.2009.09.059
Nabhan, J. F., Hu, R., Oh, R. S., Cohen, S. N., and Lu, Q. (2012). Formation and release of arrestin domain-containing protein 1-mediated microvesicles (ARMMs) at plasma membrane by recruitment of TSG101 protein. Proc. Natl. Acad. Sci. U. S. A. 109, 4146–4151. doi:10.1073/pnas.1200448109
Nager, A. R., Goldstein, J. S., Herranz-Pérez, V., Portran, D., Ye, F., Garcia-Verdugo, J. M., et al. (2017). An actin network dispatches ciliary GPCRs into extracellular vesicles to modulate signaling. Cell 168, 252–263. doi:10.1016/j.cell.2016.11.036
Nishimura, T., Oyama, T., Hu, H. T., Fujioka, T., Hanawa-Suetsugu, K., Ikeda, K., et al. (2021). Filopodium-derived vesicles produced by MIM enhance the migration of recipient cells. Dev. Cell 56, 842–859 e8. doi:10.1016/j.devcel.2021.02.029
Ogawa, M. (1993). Differentiation and proliferation of hematopoietic stem cells. Blood 81, 2844–2853. doi:10.1182/blood.v81.11.2844.bloodjournal81112844
Onfelt, B., Nedvetzki, S., Benninger, R. K., Purbhoo, M. A., Sowinski, S., Hume, A. N., et al. (2006). Structurally distinct membrane nanotubes between human macrophages support long-distance vesicular traffic or surfing of bacteria. J. Immunol. 177, 8476–8483. doi:10.4049/jimmunol.177.12.8476
Patel, A., Wu, Y., Han, X., Su, Y., Maugel, T., Shroff, H., et al. (2022). Cytonemes coordinate asymmetric signaling and organization in the Drosophila muscle progenitor niche. Nat. Commun. 13, 1185. doi:10.1038/s41467-022-28587-z
Patel, S. R., Richardson, J. L., Schulze, H., Kahle, E., Galjart, N., Drabek, K., et al. (2005). Differential roles of microtubule assembly and sliding in proplatelet formation by megakaryocytes. Blood 106, 4076–4085. doi:10.1182/blood-2005-06-2204
Peñas, P. F., García-Díez, A., Sánchez-Madrid, F., and Yáñez-Mó, M. (2000). Tetraspanins are localized at motility-related structures and involved in normal human keratinocyte wound healing migration. J. Invest. Dermatol 114, 1126–1135. doi:10.1046/j.1523-1747.2000.00998.x
Phua, S. C., Chiba, S., Suzuki, M., Su, E., Roberson, E. C., Pusapati, G. V., et al. (2017). Dynamic remodeling of membrane composition drives cell cycle through primary cilia excision. Cell 168, 264–279. doi:10.1016/j.cell.2016.12.032
Prévost, C., Zhao, H., Manzi, J., Lemichez, E., Lappalainen, P., Callan-Jones, A., et al. (2015). IRSp53 senses negative membrane curvature and phase separates along membrane tubules. Nat. Commun. 6, 8529. doi:10.1038/ncomms9529
Puhka, M., Takatalo, M., Nordberg, M. E., Valkonen, S., Nandania, J., Aatonen, M., et al. (2017). Metabolomic profiling of extracellular vesicles and alternative normalization methods reveal enriched metabolites and strategies to study prostate cancer-related changes. Theranostics 7, 3824–3841. doi:10.7150/thno.19890
Rabinovitz, I., and Mercurio, A. M. (1997). The integrin alpha6beta4 functions in carcinoma cell migration on laminin-1 by mediating the formation and stabilization of actin-containing motility structures. J. Cell Biol. 139, 1873–1884. doi:10.1083/jcb.139.7.1873
Ramirez-Weber, F. A., and Kornberg, T. B. (1999). Cytonemes: cellular processes that project to the principal signaling center in Drosophila imaginal discs. Cell 97, 599–607. doi:10.1016/s0092-8674(00)80771-0
Raposo, G., and Stoorvogel, W. (2013). Extracellular vesicles: exosomes, microvesicles, and friends. J. Cell Biol. 200, 373–383. doi:10.1083/jcb.201211138
Ravid, Y., Penič, S., Mimori-Kiyosue, Y., Suetsugu, S., Iglič, A., and Gov, N. S. (2023). Theoretical model of membrane protrusions driven by curved active proteins. Front. Mol. Biosci. 10, 1153420. doi:10.3389/fmolb.2023.1153420
Riches, A., Campbell, E., Borger, E., and Powis, S. (2014). Regulation of exosome release from mammary epithelial and breast cancer cells-A new regulatory pathway. Eur. J. Cancer 50, 1025–1034. doi:10.1016/j.ejca.2013.12.019
Rilla, K. (2021). Diverse plasma membrane protrusions act as platforms for extracellular vesicle shedding. J. Extracell. Vesicles 10, e12148. doi:10.1002/jev2.12148
Rilla, K., Pasonen-Seppänen, S., Deen, A. J., Koistinen, V. V. T., Wojciechowski, S., Oikari, S., et al. (2013). Hyaluronan production enhances shedding of plasma membrane-derived microvesicles. Exp. Cell Res. 319, 2006–2018. doi:10.1016/j.yexcr.2013.05.021
Robles, E., Woo, S., and Gomez, T. M. (2005). Src-dependent tyrosine phosphorylation at the tips of growth cone filopodia promotes extension. J. Neurosci. 25, 7669–7681. doi:10.1523/JNEUROSCI.2680-05.2005
Röper, K., Corbeil, D., and Huttner, W. B. (2000). Retention of prominin in microvilli reveals distinct cholesterol-based lipid micro-domains in the apical plasma membrane. Nat. Cell Biol. 2, 582–592. doi:10.1038/35023524
Roy, S., Hsiung, F., and Kornberg, T. B. (2011). Specificity of Drosophila cytonemes for distinct signaling pathways. Science 332, 354–358. doi:10.1126/science.1198949
Rustom, A., Saffrich, R., Markovic, I., Walther, P., and Gerdes, H. H. (2004). Nanotubular highways for intercellular organelle transport. Science 303, 1007–1010. doi:10.1126/science.1093133
Sanders, T. A., Llagostera, E., and Barna, M. (2013). Specialized filopodia direct long-range transport of SHH during vertebrate tissue patterning. Nature 497, 628–632. doi:10.1038/nature12157
Scita, G., Confalonieri, S., Lappalainen, P., and Suetsugu, S. (2008). IRSp53: crossing the road of membrane and actin dynamics in the formation of membrane protrusions. Trends Cell Biol. 18, 52–60. doi:10.1016/j.tcb.2007.12.002
Sengupta, P., Seo, A. Y., Pasolli, H. A., Song, Y. E., Johnson, M. C., and Lippincott-Schwartz, J. (2019). A lipid-based partitioning mechanism for selective incorporation of proteins into membranes of HIV particles. Nat. Cell Biol. 21, 452–461. doi:10.1038/s41556-019-0300-y
Shen, Y., Zheng, Y., and Foster, D. A. (2002). Phospholipase D2 stimulates cell protrusion in v-Src-transformed cells. Biochem. Biophys. Res. Commun. 293, 201–206. doi:10.1016/S0006-291X(02)00204-8
Sorrentino, S., Conesa, J. J., Cuervo, A., Melero, R., Martins, B., Fernandez-Gimenez, E., et al. (2021). Structural analysis of receptors and actin polarity in platelet protrusions. Proc. Natl. Acad. Sci. U. S. A. 118, e2105004118. doi:10.1073/pnas.2105004118
Sowinski, S., Jolly, C., Berninghausen, O., Purbhoo, M. A., Chauveau, A., Köhler, K., et al. (2008). Membrane nanotubes physically connect T cells over long distances presenting a novel route for HIV-1 transmission. Nat. Cell Biol. 10, 211–219. doi:10.1038/ncb1682
Stanganello, E., Hagemann, A. I. H., Mattes, B., Sinner, C., Meyen, D., Weber, S., et al. (2015). Filopodia-based Wnt transport during vertebrate tissue patterning. Nat. Commun. 6, 5846. doi:10.1038/ncomms6846
Sudhaharan, T., Hariharan, S., Lim, J. S. Y., Liu, J. Z., Koon, Y. L., Wright, G. D., et al. (2019). Superresolution microscopy reveals distinct localisation of full length IRSp53 and its I-BAR domain protein within filopodia. Sci. Rep. 9, 2524. doi:10.1038/s41598-019-38851-w
Suetsugu, S., Murayama, K., Sakamoto, A., Hanawa-Suetsugu, K., Seto, A., Oikawa, T., et al. (2006). The RAC binding domain/IRSp53-MIM homology domain of IRSp53 induces RAC-dependent membrane deformation. J. Biol. Chem. 281, 35347–35358. doi:10.1074/jbc.M606814200
Thery, C., Witwer, K. W., Aikawa, E., Alcaraz, M. J., Anderson, J. D., Andriantsitohaina, R., et al. (2018). Minimal information for studies of extracellular vesicles 2018 (MISEV2018): a position statement of the International Society for Extracellular Vesicles and update of the MISEV2014 guidelines. J. Extracell. Vesicles 7, 1535750. doi:10.1080/20013078.2018.1535750
Thomas, A., Mariani-Floderer, C., López-Huertas, M. R., Gros, N., Hamard-Péron, E., Favard, C., et al. (2015). Involvement of the rac1-IRSp53-wave2-arp2/3 signaling pathway in HIV-1 Gag particle release in CD4 T cells. J. virology 89, 8162–8181. doi:10.1128/JVI.00469-15
Thon, J. N., Montalvo, A., Patel-Hett, S., Devine, M. T., Richardson, J. L., Ehrlicher, A., et al. (2010). Cytoskeletal mechanics of proplatelet maturation and platelet release. J. Cell Biol. 191, 861–874. doi:10.1083/jcb.201006102
Tokuo, H., and Ikebe, M. (2004). Myosin X transports Mena/VASP to the tip of filopodia. Biochem. Biophysical Res. Commun. 319, 214–220. doi:10.1016/j.bbrc.2004.04.167
Tzoran, I., Rebibo-Sabbah, A., Brenner, B., and Aharon, A. (2015). Disease dynamics in patients with acute myeloid leukemia: new biomarkers. Exp. Hematol. ISEH - Int. Soc. Exp. Hematol. 43, 936–943. doi:10.1016/j.exphem.2015.07.004
van Niel, G., D’Angelo, G., and Raposo, G. (2018). Shedding light on the cell biology of extracellular vesicles. Nat. Rev. Mol. Cell Biol. 19, 213–228. doi:10.1038/nrm.2017.125
Vasioukhin, V., Bauer, C., Yin, M., and Fuchs, E. (2000). Directed actin polymerization is the driving force for epithelial cell–cell adhesion. Cell 100, 209–219. doi:10.1016/s0092-8674(00)81559-7
Verweij, F. J., Revenu, C., Arras, G., Dingli, F., Loew, D., Pegtel, D. M., et al. (2019). Live tracking of inter-organ communication by endogenous exosomes in vivo. Dev. Cell 48, 573–589. doi:10.1016/j.devcel.2019.01.004
Votteler, J., and Sundquist, W. I. (2013). Virus budding and the ESCRT pathway. Cell host microbe 14, 232–241. doi:10.1016/j.chom.2013.08.012
Wang, J., Nikonorova, I. A., Silva, M., Walsh, J. D., Tilton, P. E., Gu, A., et al. (2021). Sensory cilia act as a specialized venue for regulated extracellular vesicle biogenesis and signaling. Curr. Biol. CB 31, 3943–3951.e3. doi:10.1016/j.cub.2021.06.040
Wang, X., Bukoreshtliev, N. V., and Gerdes, H. H. (2012a). Developing neurons form transient nanotubes facilitating electrical coupling and calcium signaling with distant astrocytes. PLoS One 7, e47429. doi:10.1371/journal.pone.0047429
Wang, X., and Gerdes, H. H. (2012). Long-distance electrical coupling via tunneling nanotubes. Biochimica biophysica acta 1818, 2082–2086. doi:10.1016/j.bbamem.2011.09.002
Wang, X., and Gerdes, H. H. (2015). Transfer of mitochondria via tunneling nanotubes rescues apoptotic PC12 cells. Cell Death Differ. 22, 1181–1191. doi:10.1038/cdd.2014.211
Wang, X., Qiao, D., Chen, L., Xu, M., Chen, S., Huang, L., et al. (2019). Chemotherapeutic drugs stimulate the release and recycling of extracellular vesicles to assist cancer cells in developing an urgent chemoresistance. Mol. Cancer 18, 182–218. doi:10.1186/s12943-019-1114-z
Wang, X., Veruki, M. L., Bukoreshtliev, N. V., Hartveit, E., and Gerdes, H.-H. (2010). Animal cells connected by nanotubes can be electrically coupled through interposed gap-junction channels. Proc. Natl. Acad. Sci. U. S. A. 107, 17194–17199. doi:10.1073/pnas.1006785107
Wang, Z.-G., Liu, S.-L., Tian, Z.-Q., Zhang, Z.-L., Tang, H.-W., and Pang, D.-W. (2012b). Myosin-driven intercellular transportation of wheat germ agglutinin mediated by membrane nanotubes between human lung cancer cells. ACS Nano 6, 10033–10041. doi:10.1021/nn303729r
Wan Mohamad Noor, W. N. I., Nguyen, N. T. H., Cheong, T. H., Chek, M. F., Hakoshima, T., Inaba, T., et al. (2023). Small GTPase Cdc42, WASP, and scaffold proteins for higher-order assembly of the F-BAR domain protein. Sci. Adv. 9, eadf5143. doi:10.1126/sciadv.adf5143
Weigmann, A., Corbeil, D., Hellwig, A., and Huttner, W. B. (1997). Prominin, a novel microvilli-specific polytopic membrane protein of the apical surface of epithelial cells, is targeted to plasmalemmal protrusions of non-epithelial cells. Proc. Natl. Acad. Sci. U. S. A. 94, 12425–12430. doi:10.1073/pnas.94.23.12425
Welsh, J. A., Goberdhan, D. C. I., O’Driscoll, L., Buzas, E. I., Blenkiron, C., Bussolati, B., et al. (2024). Minimal information for studies of extracellular vesicles (MISEV2023): from basic to advanced approaches. J. Extracell. Vesicles 13, e12404. doi:10.1002/jev2.12404
Wills, C. A., Liu, X., Chen, L., Zhao, Y., Dower, C. M., Sundstrom, J., et al. (2021). Chemotherapy-induced upregulation of small extracellular vesicle-associated PTX3 accelerates breast cancer metastasis. Cancer Res. 81, 452–463. doi:10.1158/0008-5472.CAN-20-1976
Wolf, P. (1967). The nature and significance of platelet products in human plasma. Br. J. Haematol. 13, 269–288. doi:10.1111/j.1365-2141.1967.tb08741.x
Yáñez-Mó, M., Siljander, P. R., Andreu, Z., Zavec, A. B., Borràs, F. E., Buzas, E. I., et al. (2015). Biological properties of extracellular vesicles and their physiological functions. J. Extracell. Vesicles 4, 27066. doi:10.3402/jev.v4.27066
Yu, F. L., Miao, H., Xia, J., Jia, F., Wang, H., Xu, F., et al. (2019). Proteomics analysis identifies IRSp53 and fascin as critical for PRV egress and direct cell-cell transmission. Proteomics 19, e1900009. doi:10.1002/pmic.201900009
Zarfati, M., Avivi, I., Brenner, B., Katz, T., and Aharon, A. (2019). Extracellular vesicles of multiple myeloma cells utilize the proteasome inhibitor mechanism to moderate endothelial angiogenesis. Angiogenesis 22, 185–196. doi:10.1007/s10456-018-9649-y
Zheng, Y., Montague, S. J., Lim, Y. J., Xu, T., Xu, T., Gardiner, E. E., et al. (2021). Label-free multimodal quantitative imaging flow assay for intrathrombus formation in vitro. Biophys. J. 120, 791–804. doi:10.1016/j.bpj.2021.01.015
Keywords: filopodia, cytoneme, microvilli, cilia, nanotube tunneling, virus, cancer, platelet
Citation: Hu HT, Nishimura T, Kawana H, Dante RAS, D’Angelo G and Suetsugu S (2024) The cellular protrusions for inter-cellular material transfer: similarities between filopodia, cytonemes, tunneling nanotubes, viruses, and extracellular vesicles. Front. Cell Dev. Biol. 12:1422227. doi: 10.3389/fcell.2024.1422227
Received: 23 April 2024; Accepted: 17 June 2024;
Published: 05 July 2024.
Edited by:
Stephane Gasman, Université de Strasbourg, FranceReviewed by:
Alistair Hume, University of Nottingham, United KingdomCopyright © 2024 Hu, Nishimura, Kawana, Dante, D’Angelo and Suetsugu. This is an open-access article distributed under the terms of the Creative Commons Attribution License (CC BY). The use, distribution or reproduction in other forums is permitted, provided the original author(s) and the copyright owner(s) are credited and that the original publication in this journal is cited, in accordance with accepted academic practice. No use, distribution or reproduction is permitted which does not comply with these terms.
*Correspondence: Shiro Suetsugu, c3VldHN1Z3VAYnMubmFpc3QuanA=