- Department of Biological Sciences, Center for Stem Cells and Regenerative Medicine, and Center for Zebrafish Research, Galvin Life Sciences Building, University of Notre Dame, Notre Dame, IN, United States
Zebrafish possess the ability to regenerate dying neurons in response to retinal injury, with both Müller glia and microglia playing integral roles in this response. Resident Müller glia respond to damage by reprogramming and undergoing an asymmetric cell division to generate a neuronal progenitor cell, which continues to proliferate and differentiate into the lost neurons. In contrast, microglia become reactive, phagocytose dying cells, and release inflammatory signals into the surrounding tissue following damage. In recent years, there has been increased attention on elucidating the role that microglia play in regulating retinal regeneration. Here we demonstrate that inflammatory cytokines are differentially expressed during retinal regeneration, with the expression of a subset of pro-inflammatory cytokine genes upregulated shortly after light damage and the expression of a different subset of cytokine genes subsequently increasing. We demonstrate that both cytokine IL-1β and IL-10 are essential for Müller glia proliferation in the light-damaged retina. While IL-1β is sufficient to induce Müller glia proliferation in an undamaged retina, expression of IL-10 in undamaged retinas only induces Müller glia to express gliotic markers. Together, these findings demonstrate the essential role of inflammatory cytokines IL-1β and IL-10 on Müller glia proliferation following light damage in adult zebrafish.
1 Introduction
Zebrafish are a widely recognized model organism in the vertebrate vision research field, with a highly conserved retinal structure and common cell types shared between zebrafish and mammalian retinas (Goldsmith and Harris, 2003; Gestri et al., 2012; Malicki et al., 2016; Angueyra and Kindt, 2018). Zebrafish are also a valuable model for understanding human ocular diseases (Phillips and Westerfield, 2014; Richardson et al., 2017; Patton and Tobin, 2019; Rosa et al., 2023). Unlike mammals, however, zebrafish have a remarkable capability to regenerate retinal neurons following retinal damage. Acute retinal neuronal loss induces resident Müller glia to reprogram and undergo an asymmetric cell division to produce a neuronal progenitor cell (NPC; Bernardos et al., 2007; Gorsuch and Hyde, 2014; Lahne et al., 2020; Powell et al., 2016). The NPCs continue to proliferate and migrate to the proper retinal layer, where they differentiate into the missing retinal neurons (Gemberling et al., 2013; Lenkowski and Raymond, 2014; Lahne et al., 2021). This mechanism enables the zebrafish retina to not only regenerate dying retinal neurons, but ultimately restore lost visual responses (Sherpa et al., 2008; 2014). A large number of studies have identified genes that are expressed within the Müller glia that are required for Müller glia reprogramming and reentry into the cell cycle (Hoang et al., 2020; Lahne et al., 2020). However, much less is known about extrinsic signals that regulate Müller glia-dependent neuronal regeneration in the adult retina, Recently, there has been increasing interest in the role of microglia in regulating neuronal regeneration following retinal damage in zebrafish (White et al., 2017; Mitchell et al., 2019; Van Dyck et al., 2021; Iribarne and Hyde, 2022). Microglia are the resident immune cells of the nervous system and play a vital role during neurogenesis, synaptic maintenance, and surveillance for abnormalities (Paolicelli et al., 2011; Parkhurst et al., 2013; Gogoleva et al., 2019; Casali and Reed-Geaghan, 2021; Wang and Li, 2021). Under pathological insults, microglia become activated and phagocytose dying cells and release inflammatory signals within the surrounding tissue (Fricker et al., 2012; Tanaka et al., 2013; Fu et al., 2014; Wolf et al., 2017; Muzio et al., 2021). In adult zebrafish, retinal damage induces resident microglia and infiltrating macrophages to proliferate and migrate to the site of damage, where they actively phagocytose cellular debris (Mitchell et al., 2018). In addition, microglia are essential to properly regulate the Müller glia-dependent neuronal regeneration response. Depleting microglia, either by pharmacological treatment or ablation prior to neuronal damage, inhibits Müller glia reprogramming and proliferation following retinal damage (White et al., 2017; Conedera et al., 2019; Zhang et al., 2020; Van Dyck et al., 2021; Iribarne and Hyde, 2022). This reduced regenerative response by the Müller glia is likely due to the loss of secreted signals from the depleted microglia to the Müller glia.
Activated microglia can release both pro- and anti-inflammatory cytokines in response to neuronal damage (Frank et al., 2007; Rodríguez-Gómez et al., 2020; Jesudasan et al., 2021). Cytokines that commonly exert a pro-inflammatory effect such as IL-1β, IL-6, and Ifng1 induce inflammation, which is intended to prevent further neuronal damage (Frank et al., 2007; Smith et al., 2012; Rodríguez-Gómez et al., 2020; Jesudasan et al., 2021). However, prolonged and excessive exposure to these cytokines can be detrimental due to their inherent neurotoxicity. In the adult zebrafish retina, one pro-inflammatory cytokine, TNFα, was shown to be necessary and sufficient to induce the Müller glia-dependent neuronal regeneration response (Nelson et al., 2013; Conner et al., 2014). However, TNFα is not initially produced by the microglia, rather it is released from the dying retinal neurons (Nelson et al., 2013). In contrast, cytokines that are often associated with an anti-inflammatory effect, such as IL-4, IL-10, and IL-13, attenuate the inflammatory response over time to focus on tissue repair and homeostasis (Lively et al., 2018; Sun et al., 2019; Bottiglione et al., 2020; Miao et al., 2020; Shemer et al., 2020; Chen et al., 2022). A successful tissue recovery is achieved only when the appropriate balance of inflammatory cytokines is expressed (Cicchese et al., 2018; Soliman and Barreda, 2023).
In this manuscript, we explored the expression and role of the inflammatory response following light damage of the zebrafish retina. We confirmed that a subset of inflammatory cytokines increased shortly after retinal damage, followed by increased expression of three cytokines that possess anti-inflammatory effects. We then demonstrated that the pro-inflammatory cytokine IL-1β is both necessary and sufficient for Müller glia proliferation. Additionally, IL-1β expression induced the expression of cytokine IL-10. We then demonstrated that IL-10 was also required for Müller glia proliferation in the light-damaged retina but was not sufficient to induce Müller glia proliferation in undamaged retinas. However, in the absence of retinal damage, IL-10 induced Müller glia to exhibit a hypertrophic morphology and express several gliotic genes, which are essential for Müller glia reprogramming (Thomas et al., 2016; Hoang et al., 2020). This work demonstrates that the dynamic expression of at least two inflammatory cytokines is essential to induce Müller glia proliferation at the outset of retinal regeneration in zebrafish.
2 Materials and methods
2.1 Fish maintenance
Female and male zebrafish (Danio rerio) were used in this study and maintained at 28°C under normal light conditions (14 h light:10 h dark) in the Freimann Life Science Center at the University of Notre Dame as described previously (Vihtelic and Hyde, 2000). Fish were 6–12 months old and 4–5 cm in length. The fish lines utilized in this study include albino, and albino;Tg(gfap:EGFP)nt11. All animal care protocols were approved by the University of Notre Dame Animal Care and Use Committee and in compliance with the Association of Research in Vision and Ophthalmology for the use of animal in vision research.
2.2 Light damage paradigm
Adult albino and albino;Tg(gfap:EGFP)nt11 zebrafish were dark-adapted for 2 weeks and exposed to constant intense light for up to 96 h as previously described (Vihtelic and Hyde, 2000; Kassen et al., 2006; Lahne et al., 2021). The temperature of the tanks was maintained at 32°C, which is optimal to induce maximal photoreceptor cell death. Fish were euthanized in a 1:500 dilution of 2-phenoxyethanol (2-PE; 77699; Sigma-Aldrich, St. Louis, MO) in system water after each collection timepoint.
2.3 Protein and drug injections
2.3.1 Injection of microglia drugs pexidartinib and dexamethasone
To inhibit microglia during light treatment, a combined treatment of Pexidartinib, also known as PLX3397, (PLX; S78181; Selleck Chemicals, Houston, TX) and Dexamethasone (Dex; D1756; Sigma-Aldrich) were used. PLX3397 reduces the number of microglia and Dexamethasone will reduce the inflammatory response of any remaining microglia. Dark-adapted albino:Tg(gfap:EGFP)nt11 fish were anesthetized in 1:1000 2-PE and a small incision was made in the cornea with a double-edged sapphire blade (504077; World Precision Instruments, Sarasota, FL). Zebrafish were then intravitreally injected with either 0.5 μL of DMSO (vehicle control) or a combination of 250 µM of Pexidartinib and 250 µM of Dexamethasone using a 2.5 μL Hamilton syringe with 33-gauge rounded needle (7762-06; Hamilton, Reno, NV) every 24 h from the start of light treatment to 72 h of light treatment (LT). Fish were removed from either the constant light treatment room or the standard light treatment room for approximately 15 min to perform intravitreal injections and then returned to the corresponding light conditions.
2.3.2 Intravitreal injection of caspase-1 inhibitor, Ac-YVAD-cmk
To investigate the role of inflammatory cytokine IL-1β, dark-adapted albino;Tg(gfap:EGFP)nt11 fish were anesthetized in 1:000 2-PE and intravitreally injected with 0.5 μL of either DMSO (vehicle control) or 1 mM of Ac-YVAD-cmk (YVAD; SML0429; Sigma-Aldrich). Fish were injected 24 h prior to the start of light treatment and subsequently every 24 h until 36 h LT. Injections were performed as described in Section 2.3.1.
2.3.3 Injection of recombinant proteins IL-1β and IL-10
Adult albino and albino; Tg (gfap:EGFP)nt11 were used for undamaged retinal injections. Fish were anesthetized and intravitreally injected with 0.5 μL of either PBS (vehicle control), recombinant zebrafish IL-1β protein (AB236204; Abcam, Cambridge, United Kingdom) or IL-10 protein (RP1023Z; Kingfisher Biotech, St. Paul, MN) every 24 h. After each injection, fish were placed in a dark incubator at 32°C, which corresponds to the tank temperature that the light-treated fish are kept at and enables the initiation of maximal Müller glia and NPC proliferation in a short time frame (Conner et al., 2014). Fish were euthanized and collected at 2- and 3-days post injections (dpi). Injections were performed as described in Section 2.3.1.
2.4 Morpholino-mediated knockdown and electroporation
Morpholino oligos were synthesized by GeneTools LLC (Philomath, OR) and contained a positively charged lissamine tag. Dark-adapted albino; Tg(gfap:EGFP)nt11 zebrafish were intravitreally injected with 0.5 μL of either 1 mM Standard Control, il-1β or il-10 translation-blocking morpholinos. Electroporation were then performed as previously described (Thummel et al., 2011; Campbell et al., 2021) and immediately placed in constant light treatment following electroporation. The following morpholinos were used: Standard Control: 5′-CCTCTTACCTCAGTTACAATTTATA-3’ (Nasevicius and Ekker, 2000), il-1β: 5′- CCCACAAACTGCAAAATATCAGCTT -3' (López-Muñoz et al., 2011; Frame et al., 2020; Wattrus et al., 2022), il-10: 5′- AATCAGTGGCACTTACGTTTATGTT- 3′, tnfa: 5′- AGCTTCATAATTGCTGTATGTCTTA- 3’ (Nelson et al., 2013).
2.5 Immunohistochemistry
Immunohistochemistry was performed as previously described (Lahne et al., 2021; Boyd et al., 2023). Eyes were collected and fixed in a 9:1 ethanolic formaldehyde solution overnight at 4°C. Eyes were rehydrated in a series of ethanol washes and incubated in 30% sucrose overnight at 4°C. Eyes were then cryoprotected in a mixture of 2:1 Tissue Freezing Media:30% sucrose overnight at 4°C. Retinas were then frozen in Tissue Freezing Media, sectioned at 14 μm thickness, and stored at −80°C until immunostaining.
Slides were rehydrated in PBS for 30 min at room temperature and blocked in 2% DMSO, 2% normal goat serum, 1% Tween-20, and 0.4% Triton X in PBS for 1 h at room temperature. Primary antibodies diluted in blocking solution were applied on the slide and incubated overnight at room temperature. Primary antibodies used in this study included: chicken anti-GFP (1:1000; AB13970; Abcam), mouse anti-PCNA (1:1000; P8825; Sigma-Aldrich) and rabbit anti-Lcp1 (1:400; GTX134687; GeneTex, Irvine, CA). Slides were washed with PBS/0.05% Tween-20 (PBS-T) and incubated in secondary antibodies diluted in blocking solution for 1 h at room temperature. Fluorescent-tagged secondary antibodies (Life Technologies, Carlsbad, CA) used in this study included: Alexa Fluor goat anti-chicken 488 (1:1000; A11039), Alexa Fluor goat anti-mouse 594 (1:1000; A11032), and Alexa Fluor goat anti-rabbit 647 (1:1000; A21245). 4,6-diamidino-2phenylindol (DAPI; 1:1000; D1306, Thermo Fisher Scientific; Waltham, MA) was also applied for nuclear localization. Slides were washed in PBS-T and mounted in Prolong Gold Antifade Reagent (P36930; Life Technologies).
2.6 RNA isolation and quantitative real-time polymerase chain reaction
Dorsal retinas from adult albino zebrafish were isolated at specific time points of constant light and RNA isolation was performed with Trizol (15596-026; Thermo Fisher) as previously described (Campbell et al., 2021; Lahne et al., 2021). For each time point, 6-7 dorsal retinas were collected and RNA concentrations were measured. The cDNA samples were synthesized using LunaScript RT SuperMix kit (E3101; New England Biolabs, Ipswich, MA) and stored at −80°C. Sample reactions were assembled using Luna universal qPCR Master Mix (M3003, New England Biolabs) following the manufacturer’s instructions with TaqMan primers listed in Table 1 (Applied Biosystem). StepOnePlus Real-Time PCR system (4276600; Thermo Fisher) was used to perform qRT-PCR reactions with the following conditions: 1 min at 95°C, followed by 40 cycles of 15 s at 95°C and 30 s at 60°C. qRT-PCR reactions were analyzed using the comparative ΔΔCT method as previously described (Gorsuch et al., 2017; Lahne et al., 2021) using 18 s rRNA as the reference gene. The CT values for 18 s rRNA are consistent across all rounds of qRT-PCR experiments. Light-treated retinas used 0 h LT to generate a log2-fold change in gene expression levels (Kassen et al., 2006) and undamaged retinas used PBS (vehicle) control to generate a log2-fold change in gene expression levels. Three biological replicates were conducted with each sample.
2.7 RNAscope in situ hybridization
RNAscope Multiplex Fluorescent v2 Assay (Advance Cell Diagnostics; Newark, CA) was utilized for RNA in situ hybridization. Sample fixation and immunohistochemistry with in situ hybridization were performed according to a protocol described previously (Campbell et al., 2021; Boyd et al., 2023). Briefly, frozen tissue sections were washed in PBS for 5 min before baking at 60°C for 1 h. Sections were then post-fixed in 4% paraformaldehyde at room temperature for 1 h. Sections were dehydrated in sequential 50%, 70%, 100% ethanol washes for 5 min each and twice in 100% before baking at 60°C for 1 h. Slides were given a hydrogen peroxide treatment provided by the manufacturer (Advance Cell Diagnostic) at room temperature for 1 h. The slides were washed in distilled water and immediately submerged into a mildly boiling Target Retrieval Reagent solution (Advance Cell Diagnostic) for 15 min. Sections were placed in distilled water at room temperature and dehydrated in 100% ethanol. A hydrophobic barrier (ImmEdge Hydrophobic pen; H-4000; Vector Laboratories; Burlingame, CA) was applied on the slides and baked at 60°C for 1 h before drying overnight at room temperature.
Sections were given a Protease III treatment at 40°C for 30 min in the HybEZ™ Oven (Advance Cell Diagnostic). Slides were washed with distilled water followed by probe hybridization at 40°C for 2 h. The probe used in this study was Dr-il1b-C1 (432971; Advance Cell Diagnostic), which was taken from NCBI accession of D. rerio il-1β: NM_212,844.2. A 3-plex negative control probe, provided by the manufacturer, was applied simultaneously with experiment slides. Following probe hybridization, sections were incubated with a probe amplification AMP1 solution at 40°C for 30 min followed by a buffer wash. Signal development with HRP was based on the manufacturer’s protocol with Opal 570 (1:1000; FP1488001KT; Akoya Biosciences; Menlo Park, CA). Once signal development was complete, slides were washed in PBS-T for 5 min at room temperature before proceeding with immunohistochemistry. Primary antibodies used were chicken anti-GFP (1:1000; AB13970; Abcam) and rabbit anti-Lcp1 (1:400, GTX134697; Genetex, Irvine, CA). Fluorescent-tagged secondary antibodies used were Alexa Fluor goat anti-chicken 488 (1:1000; A11039; Thermo Fisher Scientific) and Alexa Fluor goat anti-rabbit 647 (1:1000; A21245; Thermo Fisher Scientific) and DAPI (1:1000; D1306; Thermo Fisher Scientific) was applied to stain nuclei.
2.8 Image acquisition and data analysis
A Nikon A1 confocal microscope and Leica Stellaris eight confocal microscope with a 40x oil-immersion objective were used to acquire approximately 10 µm z-stack with 1.0 µm step size images of the central dorsal retina. Cell counts were manually performed using FIJI/ImageJ software and normalized to a 300 µm length of the retina. Scale bars for images are either 20, 10, or 5 μm and are indicated in the figures and figure legends.
2.9 Single-cell RNA-Seq analysis
We used single-cell RNA-Seq (scRNA-Seq) data from whole-light-damaged retinas that was previously published (Hoang et al., 2020). scRNA-seq analysis was performed using Seurat (Hao et al., 2021). Violin plots were generated using cell clusters identified and maintained from Hoang et al. (2020). Differential expression analyses were performed with microglia and Müller glia.
2.10 Statistical analyses
The data in this study were analyzed with at least three independent trials. The sample size and the mean ± SEM are stated in the text and each figure legend. Statistical analysis was performed using GraphPad Prism 10 (San Diego, CA). Statistical significance was determined using either a Student’s t-test, a one-way ANOVA followed by Bonferroni’s pos-hoc test, or a two-way ANOVA followed by Bonferroni’s post hoc test. A p-value less than 0.05 indicated statistical significance, with graphs displaying * for p ≤ 0.05, ** for p ≤ 0.01, *** for p ≤ 0.001, **** for p ≤ 0.0001, and n. s. for no significance.
3 Results
3.1 Cotreatment with pexidartinib and dexamethasone reduces the number of microglia and proliferating Müller glia in light-damaged retinas
It was previously demonstrated that microglia are required for Müller glia proliferation following retinal damage (White et al., 2017; Conedera et al., 2019; Silva et al., 2020; Iribarne and Hyde, 2022). To confirm that microglia are required for retinal regeneration in light-damaged adult zebrafish retinas, we utilized two pharmacological treatments to reduce the number of activated microglia. Pexidartinib, or PLX3397 (PLX), is a small molecule that targets the colony-stimulating factor 1 receptor (CSF1R), which blocks CSF1 binding, a regulator of microglia and macrophage survival, production, and differentiation (Elmore et al., 2014; Conedera et al., 2019; Han et al., 2022). Dexamethasone (Dex) is an anti-inflammatory glucocorticoid that has immunosuppressive effects on microglia (Park et al., 2019; Hui et al., 2020; Iribarne and Hyde, 2022). Together, these drugs should reduce the number of microglia and the inflammatory response of any remaining microglia. Dark-adapted albino;Tg(gfap:EGFP)nt11 zebrafish were intravitreally injected with a combination of PLX and Dex every 24 h until 72 h of constant light treatment (LT). Retinas were collected at 0, 24, 36, and 72 h LT and immunolabeled for GFP and PCNA to label proliferating Müller glia and Lcp1, a marker commonly used for microglia, macrophages, and other leukocyte cells (Figures 1A–H). Because macrophages and other leukocyte cells are typically uncommon in the retina, unless the blood-brain barrier is compromised, Lcp1+ retinal cells are predominantly microglia.
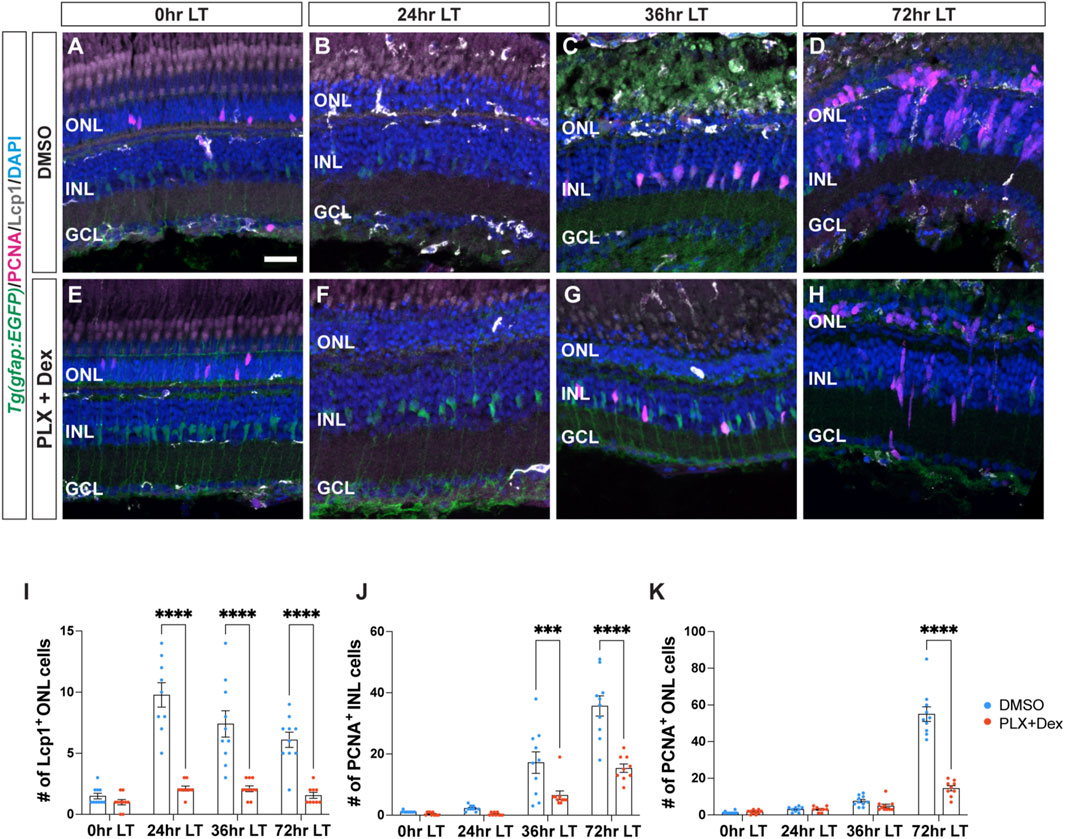
Figure 1. Treatment with PLX3397 and Dexamethasone reduces the number of microglia and proliferating Müller glia in light-damaged retinas. (A–H) Confocal images of albino;Tg(gfap:EGFP)nt11 retinas that were light-damaged and collected at 0, 24, 36, and 72 h of light treatment (LT). Eyes were intravitreally injected with either DMSO (A–D) or PLX3397 and Dexamethasone (PLX + Dex, E-H) at 0 h LT. Sections were immunostained to detect GFP (Müller glia, green), PCNA (proliferating cells, magenta), and Lcp1 (microglia, white), with DAPI counterstain (nuclei, blue). (I) Quantifications of the numbers of Lcp1+ ONL cells under the different conditions described above. (J,K) The numbers of PCNA+ cells were quantified in the INL (J) and ONL (K). Quantifications were normalized to 300 μm along the length of the central-dorsal retina. Statistical analyses were performed using two-way ANOVA with Bonferroni’s post hoc test. Graphs represent the Mean ± SEM and n ≥ 9. ***, p < 0.001, ****, p < 0.0001. ONL, outer nuclear layer; INL, inner nuclear layer; GCL, ganglion cell layer. Scale bar in A is 20 µm and is the same for (B–H).
The PLX + Dex coinjected retinas possessed significantly fewer Lcp1+ cells compared to the DMSO (vehicle) control group at all time points of constant light (Figure 1I; DMSO: 24 h LT: 9.78 ± 0.99, 36 h LT: 7.40 ± 1.08, 72 h LT: 6.10 ± 0.62; PLX + Dex: 24 h LT: 2.11 ± 0.20, p < 0.0001, 36 h LT: 2.10 ± 0.23, p < 0.0001, 72 h LT: 1.56 ± 0.24, p < 0.0001). The reduced number of microglia during LT also correlated with significantly fewer PCNA+ Müller glia in the INL at 36 h LT (Figure 1J; DMSO: 17.6 ± 3.50; PLX + Dex: 6.50 ± 1.41, p = 0.0005). At 72 h LT, when Müller glia-derived neuronal progenitor cells (NPCs) are present in both the INL and ONL, there were significantly fewer proliferating NPCs in the drug-treated retinas relative to the controls (Figure 1K; DMSO INL: 35.70 ± 3.29, ONL: 55.00 ± 3.98; PLX + Dex INL: 15.33 ± 1.36, p < 0.0001, ONL: 14.56 ± 1.42, p < 0.0001). These findings suggest that the combined treatment of PLX and Dex effectively reduced the number of microglia and proliferating Müller glia in light-damaged retinas.
3.2 Cytokines are differentially expressed following light damage
In pathological conditions, activated microglia play a critical role in regulating inflammatory signaling, which attracts other cells to the site of injury and modulates the surrounding microenvironment (Streit et al., 2004; Bosak et al., 2018; Mitchell et al., 2018; Iribarne and Hyde, 2022). To examine the expression of a subset of inflammatory cytokines during LT, we used quantitative real-time PCR (qRT-PCR). Dark-adapted albino zebrafish were light-damaged, and retinas were collected and RNA isolated at 0, 6, 12, 24, 36, and 72 h LT, as well as 1-day (120 h) and 7-days (264 h) after terminating the constant light treatment at 96 h LT. These time points were selected based on established events observed during light-induced damage and regeneration of the adult zebrafish retina, including: 1) photoreceptor cell death between 12 and 24 h, 2) Müller glia proliferation 31–36 h, and 3) amplification and migration of NPCs to the ONL between 68 and 96 h (Vihtelic and Hyde, 2000; Gorsuch and Hyde, 2014). Two additional time points were included: 1-day (120 h) and 7-days (264 h) after terminating the constant light treatment at 96 h LT, which represent time points that correspond to the regeneration of retinal neurons (Lyu et al., 2023). We investigated the temporal expression of three cytokine genes commonly associated with pro-inflammatory effects: interleukin-1β (il-1β), interleukin-6 (il-6), and interferon gamma one (ifng1) and three cytokine genes usually associated with anti-inflammatory effects: interleukin-4 (il-4), interleukin-10 (il-10), and interleukin-13 (il-13). The expression of all three cytokine genes in the first group rapidly increased during the first 24 h after initiating the LT and then decreased over the next several days (Figure 2A). In contrast, the three cytokine genes in the latter group peaked in their expressions between 72 and 120 h after the start of constant light. (Figure 2A). Thus, these six cytokines exhibit temporally distinct expression profiles following light damage.
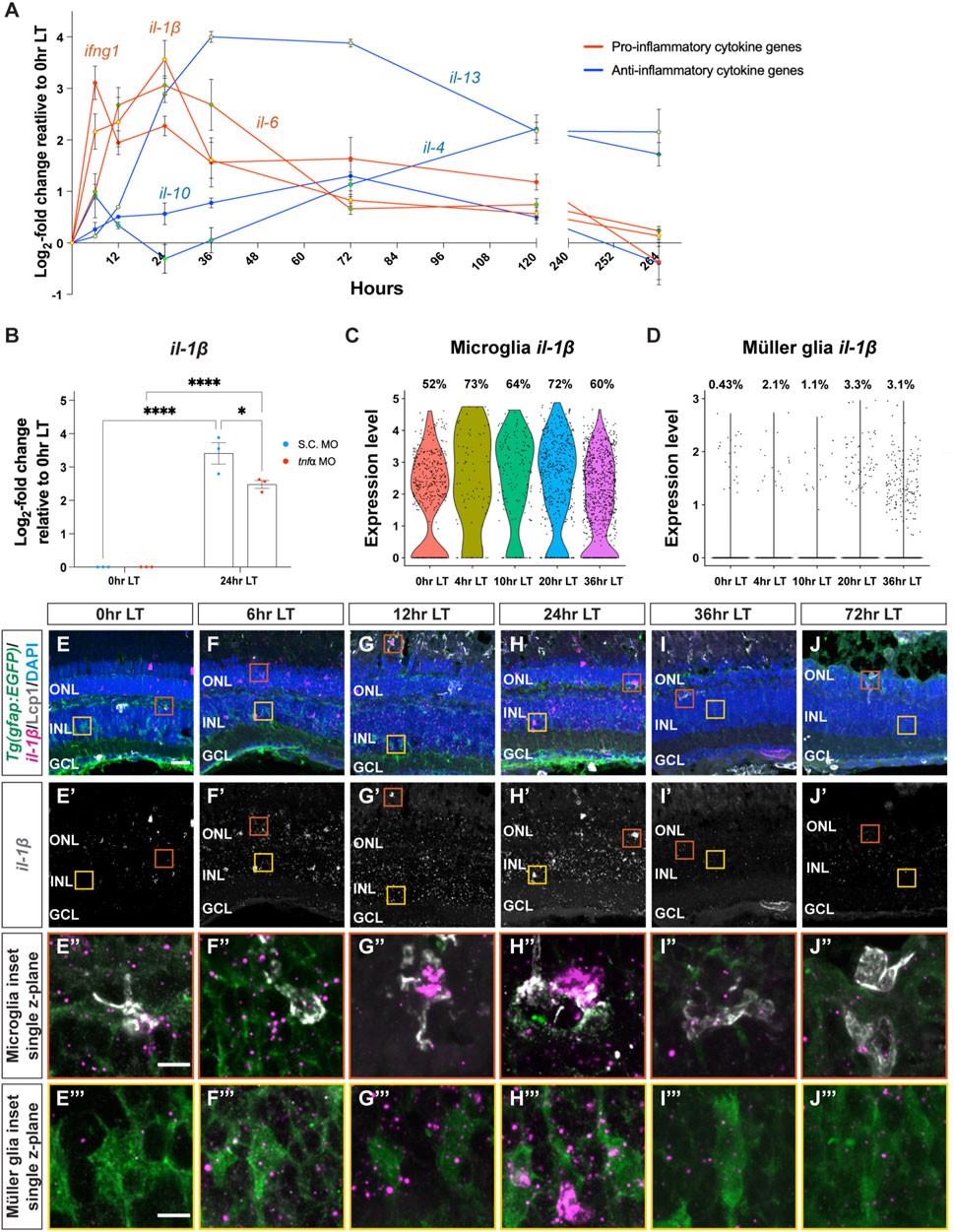
Figure 2. Cytokine genes are dynamically expressed following light damage. (A) qRT-PCR analysis of three cytokine genes, which are commonly associated with inflammatory effects: il-1β (red line, yellow dot), il-6 (red line, green dot), and ifng1 (red line, red dot) and three cytokine genes, which are usually associated with inhibiting inflammation: il-10 (blue line, blue dot), il-13 (blue line, yellow dot), il-4 (blue line, green dot), across light treatment (LT) time course (0, 6, 12, 24, 36, and 72 h LT) and 1-day (120 h) and 7-days (264 h) recovery (after completing 96 h constant LT). All values are normalized to 18 s rRNA reference gene. The mRNA expression levels are displayed as log2-fold change relative to 0 h LT from three independent replicates with a pool of 6-7 dorsal retinas for each replicate. (B) qRT-PCR analysis of il-1β expression at 0 h and 24 h LT in Standard Control morphants (S.C. MO, blue circles) and tnfa morphants (red circles). Data was normalized to 18 s rRNA reference gene and displayed as log2-fold change relative to the S.C. MO control group. For the qRT-PCR, three independent replicates were performed with a pool of 6-7 dorsal retinas for each replicate. (C,D) Previously published single-cell RNA-Seq data (Hoang et al., 2020) were analyzed by violin plots for il-1β expression in microglia (C) and Müller glia (D). The time points included in the snRNA-Seq dataset correspond to 0, 4, 10, 20, and 36 h LT. (E–J‴) Confocal images of dark-adapted albino;Tg(gfap:EGFP)nt11 zebrafish retinas that were isolated at 0 (E–E’’’), 6 (F–F’’’), 12 (G–G’’’), 24 (H–H’’’), 36 (I–I’’’), and 72 h LT (J–J’’’). Retinal sections were labeled by in situ hybridization with a probe for il-1β magenta: (E–J,E’’–J’’’), grayscale: (E’–J’). Sections were also immunostained to detect GFP Müller glia, green: (E–J,E’’-J’’’) and Lcp1 microglia, grayscale: (E–J, E’’–J’’’), with DAPI counterstain nuclei, blue: (E–J, E′′–J‴). Orange boxes in (E–J) were magnified to better portray il-1β signal in microglia (E’’–J’’) and yellow boxes in E-J were magnified to better portray il-1β signal in Müller glia (E’’’–J’’’). The magnified images in (E’’–J’’’) represent single z-plane images. ONL, outer nuclear layer; INL, inner nuclear layer; GCL, ganglion cell layer. Scale bar in E is 20 µm and is the same for F-J’ and scale bars in E’’ and E’’’ are 5 µm and are the same for (F’’–J’’) and (F’’’–J’’’), respectively. Graphs represent the Mean ± SEM and n = 3. *, p < 0.05, ****, p < 0.0001.
We previously demonstrated that tumor necrosis factor-alpha (tnfa) expression increases in damaged/dying retinal neurons and is required and sufficient to induce Müller glia proliferation (Nelson et al., 2013). We hypothesized that TNFα expression from the dying photoreceptors might induce il-1β in the microglia. To test this, we intravitreally injected and electroporated either the Standard Control morpholino (S.C. MO), which is not complementary to any known sequence in the zebrafish genome, or the tnfa MO (Nelson et al., 2013) into dark-adapted albino;Tg(gfap:EGFP)nt11 zebrafish. Fish were then placed in constant bright light for either 0 h or 24 h and then retinas were collected, mRNA was purified from dorsal retinas, and subjected to qRT-PCR using primers for the il-1β mRNA. As expected, there was a significant increase in il-1β expression at 24 h LT relative to 0 h LT (Figure 2B). In addition, the tnfa morphant expressed significantly lower amounts of il-1β relative to the S.C. morphant (Figure 2B). This is consistent with TNFα being required to induce the increased expression of il-1β in the light-damaged retina.
We analyzed il-1β further because it had the highest level of expression of the three early onset expressing cytokines that we tested. To identify what cells expressed il-1β during constant light treatment, we analyzed il-1β expression in our previously published scRNA-Seq dataset (Hoang et al., 2020). The time points that were analyzed in this dataset were 0, 4, 10, 20, and 36 h LT. Expression of il-1β increased rapidly, peaking at 20 h LT and gradually declining (Figure 2C). Within this dataset of microglia from 0 to 36 h LT, the average il-1β expression peaked at 4 h LT (2.20), as did the percentage of microglia expressing il-1β (Figure 2C, 73%). We then examined if the Müller glia expressed il-1β and found that, while il-1β expression was significantly lower in the Müller glia relative to the microglia, the average il-1β expression peaked at 36 h LT (0.044) and the percentage Müller glia expressing il-1β peaked at 20 h LT (Figure 2D, 3.3%). Thus, il-1β expression is rapidly expressed in microglia after the start of constant light damage and then followed by expression in the Müller glia.
To confirm the spatial pattern of il-1β expression during LT, we utilized RNAscope in situ hybridization with immunohistochemistry (Figures 2E–J’’’). Low levels of il-1β probe signal were present at 0 h and increased through 24 h LT (Figures 2E’–H’), before decreasing in expression at 36 and 72 h LT (Figures 2I’, J’), consistent with the qRT-PCR results. Over the time course, the il-1β probe colocalized with both the Lcp1+ microglia (Figures 2E’’–J’’) and GFAP+ Müller glia cell populations (Figures 2E’’’–J’’’). Taken together, these data reveal that inflammatory cytokines are expressed in a dynamic temporal manner during LT, and the pro-inflammatory cytokine gene il-1β is expressed sequentially in both microglia and Müller glia.
3.3 Pro-inflammatory cytokine IL-1β is both necessary and sufficient for Müller glia proliferation in light-damaged retinas
To investigate how pro-inflammatory cytokine IL-1β may affect Müller glia proliferation following light damage, we inhibited IL-1β secretion by using caspase-1 inhibitor, Ac-YVAD-cmk (YVAD), which is a tetrapeptide that blocks caspase-1 cleavage of the pro-IL-1β precursor and obstructs the secretion of the mature IL-1β from the cell (Gemma et al., 2007; Vojtech et al., 2012; Lin et al., 2018; Liang et al., 2019). Dark-adapted albino;Tg(gfap:EGFP)nt11 zebrafish were intravitreally injected with YVAD 24 h prior to the start of LT and every 24 h afterward. Retinas were collected at 36 h LT and immunolabelled for GFP and PCNA (Figures 3A–D). YVAD-treated retinas showed a significant decrease in the number of PCNA+ Müller glia relative to the DMSO (vehicle) control group (Figure 3E; DMSO: 23.3 ± 2.29; YVAD: 8.83 ± 1.30, p < 0.0001), suggesting that reducing the amount of mature IL-1β resulted in fewer proliferating Müller glia during LT.
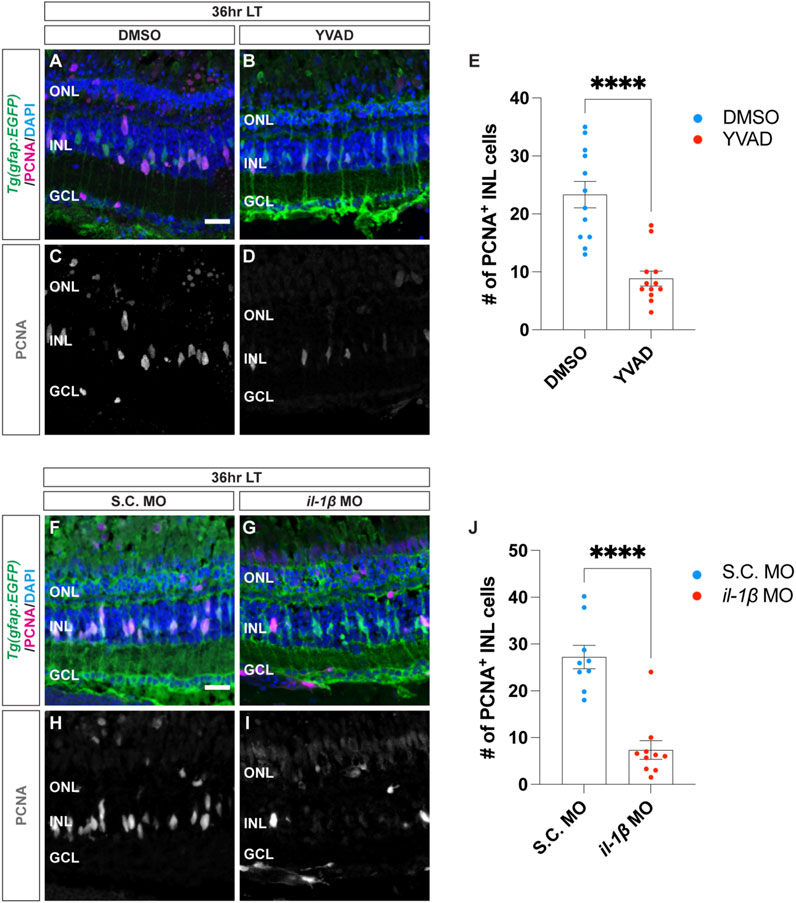
Figure 3. Cytokine IL-1β is necessary for Müller glia proliferation in light-damaged retinas. (A–D) Confocal images from albino;Tg(gfap:EGFP)nt11 retinas that were intravitreally injected with a caspase-1 inhibitor Ac-YVAD-cmk (YVAD) 24 h prior to the start of light treatment and continued to inject every 24 h until 36 h LT. At 0 h LT, retinas were intravitreally injected with either DMSO vehicle control: (A,C) or YVAD (B,D). Retinal sections were immunostained to detect GFP Müller glia, green: (A,B) ande PCNA proliferating cells, magenta in (A,B), grayscale in (C,D), with DAPI counterstain (nuclei, blue: (A,B). (E) The numbers of PCNA+ INL cells in the YVAD group (red circles) relative to the DMSO control group (blue circles). (F–I) Confocal images of retinas from albino;Tg(gfap:EGFP)nt11 zebrafish electroporated with either Standard Control (S.C.; (F,H) or il-1β morpholino (MO; (G,I) to knockdown IL-1β protein expression and immediately placed in constant light treatment. Retinas were collected at 36 h LT and immunostained to detect GFP Müller glia, green: (F,G) and PCNA proliferating cells, magenta in (F,G), grayscale in (H,I), with DAPI counterstain (nuclei, blue: (F,G). (J) Quantification of the numbers of PNCA+ INL cells in the il-1β morphant (red circles) and the S.C. MO (blue circles). Quantifications were normalized to 300 μm along the length of the central-dorsal retina. Statistical analyses were performed using Student’s t-test. Graphs represent the Mean ± SEM and n ≥ 7. ****, p < 0.0001. ONL, outer nuclear layer; INL, inner nuclear layer; GCL, ganglion cell layer. Scale bars in (A,F) are 20 μm and are the same for (B–D) and (G–I), respectively.
To independently confirm this result, we also used morpholinos to knockdown IL-1β expression. Dark-adapted albino;Tg(gfap:EGFP)nt11 zebrafish were electroporated with either a Standard Control morpholino (S.C. MO) or il-1β MO (Figures 3F–I). Similar to the YVAD-treated zebrafish, the il-1β morphants possessed significantly fewer number of PCNA+ Müller glia relative to the S.C. morphants (Figure 3J; DMSO: 27.2 ± 2.48; il-1β: 7.34 ± 2.00, p < 0.0001). Because the il-1β morphant did not possess significantly fewer pyknotic nuclei at 36 h LT relative to the S.C. morphant (Supplementary Figures S1A, B, D, E), the reduced number of proliferating Müller glia in the il-1β morphant relative to the S.C. morphant was not due to decreased cell death. These data confirm that the pro-inflammatory cytokine IL-1β is required for Müller glia proliferation in light-damaged retinas.
We next examined if the morpholino specifically knocked down IL-1β expression by testing if Müller glia proliferation in light-damaged albino;Tg(gfap:EGFP)nt11 il-1β morphant retinas was rescued by intravitreal injection of zebrafish IL-1β protein. Standard Control and il-1β morphant albino;Tg(gfap:EGFP)nt11 fish were intravitreally injected with recombinant zebrafish IL-1β protein every 24 h beginning at the start of constant light treatment (Figures 4A–F). As expected, fish that were electroporated with either il-1β MO alone or il-1β MO with PBS (vehicle) possessed significantly fewer PCNA+ Müller glia at 36 h LT relative to S.C. morphants (Figures 4A, B, D, E, G; S.C. MO alone: 28.70 ± 3.54, il-1β MO alone: 11.50 ± 1.88, p = 0.001; S.C. MO with PBS: 26.50 ± 2.62, il-1β MO with PBS: 10.1 ± 1.62, p = 0.002). In contrast, there was no significant difference in the number of PCNA+ Müller glia between the il-1β morphants injected with IL-1β protein and S.C. morphants injected with IL-1β protein (Figures 4C, F, G; S.C. MO with IL-1β protein: 22.70 ± 3.28, il-1β MO with IL-1β protein: 27.80 ± 3.61, p > 0.99). This data suggests that IL-1β protein rescued the Müller glia proliferation in il-1β morphants following light damage.
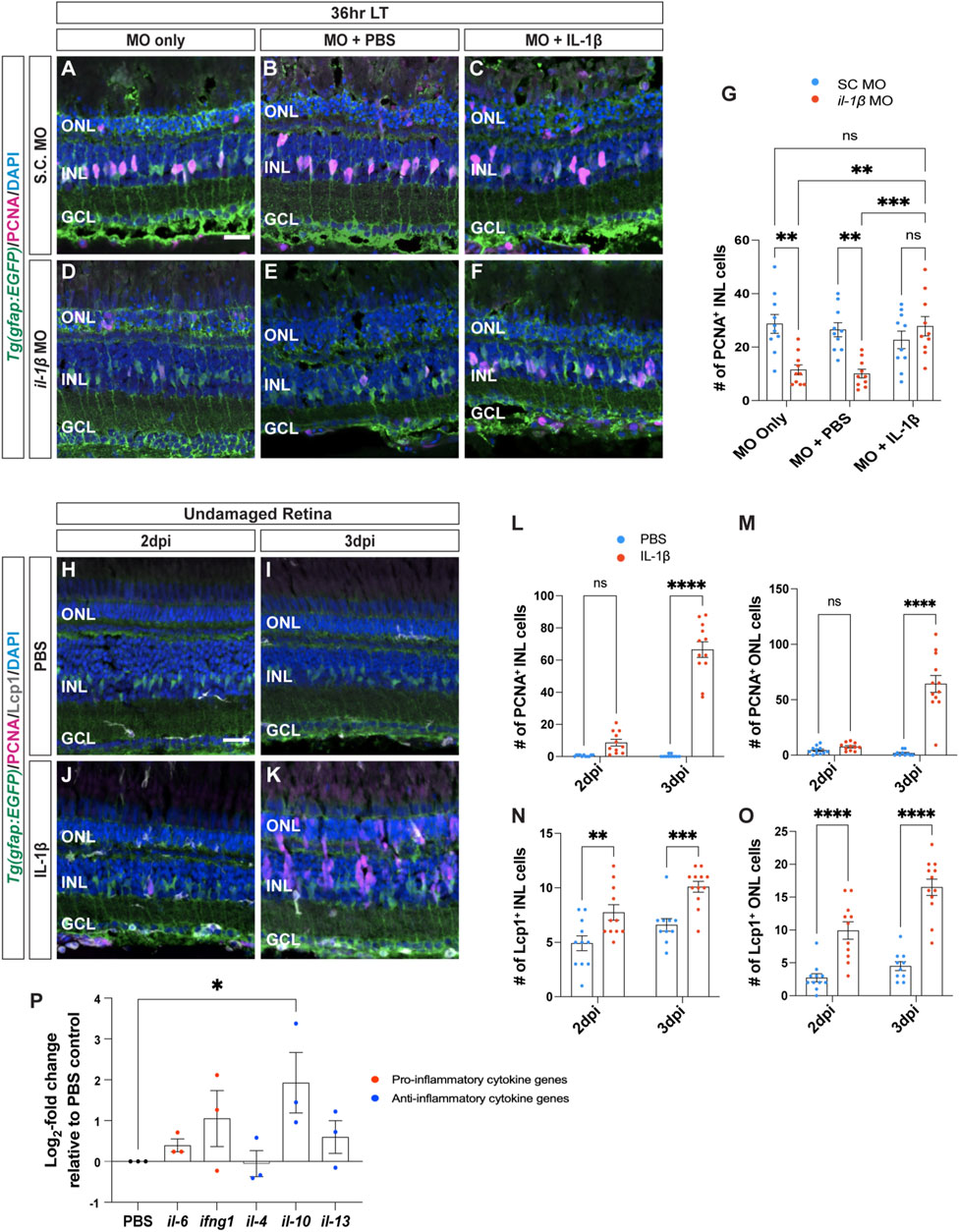
Figure 4. Recombinant IL-1β is sufficient to induce Müller glia proliferation in undamaged retinas. (A–F) Confocal images of albino;Tg(gfap:EGFP)nt11 retinas that were electroporated with Standard Control (S.C.; (A–C) and il-1β morpholinos (D–F) prior to the start of constant light treatment and intravitreally injected with either MO only (A,D), PBS (B,E) or recombinant IL-1β protein (C,F), and placed in LT. Retinal sections were collected at 36 h LT and immunostained to detect GFP (Müller glia, green) and PCNA (proliferating cells, magenta), with DAPI counterstain (nuclei, blue). (G) Quantification of the numbers of PCNA+ INL cells in il-1β morphants alone and injected with PBS (vehicle, blue circles) relative to S.C. morphants (red circles). Müller glia proliferation was rescued in il-1β morphants injected with IL-1β protein. (H–K) Confocal images of retinas from albino;Tg(gfap:EGFP)nt11 zebrafish that were injected with either PBS (H,I) or recombinant IL-1β protein (J,K) in undamaged retinas every 24 h. Retinal sections were collected at 2- and 3-days following the first injection (dpi) and immunolabeled for GFP (Müller glia, green) and PCNA (proliferating cells, magenta), with DAPI counterstain (nuclei, blue). (L,M) Quantifications showing the numbers of PCNA+ cells in the INL (L) and ONL (M) under different conditions described above. (N,O) Quantifications of the numbers of Lcp1+ cells in the INL (N) and ONL (O) under different conditions. (P) qRT-PCR analysis of pro-inflammatory (red circles) and anti-inflammatory (blue circles) cytokine gene expression profiles in undamaged retinas injected with IL-1β protein and collected at 3dpi. Data was normalized to 18 s rRNA reference gene and displayed as log2-fold change relative to the PBS (vehicle) control group. For the qRT-PCR, three independent replicates were performed with a pool of 6-7 dorsal retinas for each replicate. Cell count quantifications (G,L–O) were normalized to 300 μm along the length of the central-dorsal retina. Statistical analyses were performed using either a two-way ANOVA (G,L–O) or one-way ANOVA (P) both followed by Bonferroni’s post hoc test. Graphs represent the Mean ± SEM and n ≥ 10, *, p < 0.05, **, p < 0.01; ***, p < 0.001; ****, p < 0.0001; ns, no significance. ONL, outer nuclear layer; INL, inner nuclear layer; GCL, ganglion cell layer. Scale bar in A and H is 20 μm and is the same for (B–F) and (I–K), respectively.
Because IL-1β is required for Müller glia proliferation, we examined if IL-1β is also sufficient to induce Müller glia proliferation in undamaged retinas. We intravitreally injected recombinant zebrafish IL-1β protein into undamaged albino;Tg(gfap:EGFP)nt11 zebrafish every 24 h and collected at 2- and 3-days following the initial injection (dpi) (Figures 4H–K). At 2dpi, there were no significant differences in the number of proliferating Müller glia between retinas injected with PBS and IL-1β (Figure 4L; PBS: 0.55 ± 0.16, IL-1β: 8.64 ± 2.06, p = 0.105). By 3dpi, retinas treated with IL-1β protein had significantly greater number of PCNA+ Müller glia and NPCs in the INL compared to the PBS (vehicle) control (Figure 4L; PBS: 0.40 ± 0.27, IL-1β: 66.50 ± 4.86, p < 0.0001) and in the ONL (Figure 4M; PBS: 1.7 ± 0.76, IL-1β: 64.33 ± 7.66, p < 0.0001). Furthermore, the number of Lcp1+ cells also significantly increased in retinas injected with IL-1β protein in both the INL and ONL at 2dpi (Figures 4N, O; PBS INL: 4.91 ± 0.68, IL-1β INL: 7.73 ± 0.72, p = 0.0054; PBS ONL: 2.73 ± 0.62, IL-1β ONL: 9.91 ± 1.32, p < 0.0001) and 3dpi (Figure N–O; PBS INL: 6.60 ± 0.58, IL-1β INL: 10.08 ± 0.50, p = 0.0006; PBS ONL: 4.50 ± 0.69, IL-1β ONL: 16.50 ± 1.25, p < 0.0001). However, we did not observe any signs of cell loss (TUNEL+ cells, Supplementary Figure S2) or pyknotic nuclei in the IL-1β-injected retinas (Figures 4J, K). This demonstrates that the pro-inflammatory cytokine IL-1β protein is sufficient to induce Müller glia proliferation even without retinal damage.
We next examined if injections of IL-1β affected the gene expression profiles of other inflammatory cytokines in the undamaged retinas. We performed qRT-PCR on albino zebrafish that were intravitreally injected with IL-1β protein for 3 days (Figure 4P). Only one of the five cytokines examined showed a significant increase in gene expression, il-10 (Figure 4P; il-10: 2.90 ± 1.1, p = 0.0420) relative to the PBS control. Thus, not only is IL-1β necessary and sufficient to induce Müller glia proliferation following light damage, but it also likely activates the expression of il-10 and possibly other cytokines.
3.4 Cytokine IL-10 is required for Müller glia proliferation following light damage
Cytokine IL-10 often possesses anti-inflammatory effects that can regulate the expression of pro-inflammatory cytokines released by immune cells, such as microglia (O’Garra and Vieira, 2007; Lobo-Silva et al., 2017; Shemer et al., 2020). To determine if IL-10 is required for Müller glia proliferation following light damage, Standard Control and il-10 morpholinos were intravitreally injected alone or with PBS every 24 h until 36 h LT (Figures 5A–F). Similar to the il-1β morphants, the il-10 morphants had significantly fewer proliferating Müller glia in the INL compared to the S.C. morphants either MO alone or with PBS groups (Figures 5A, B, D, E, G; S.C. MO alone: 28 ± 2.56, il-10 MO alone: 12.07 ± 2.24, p = 0.0002; S.C. MO with PBS: 25.40 ± 3.00, il-10 MO with PBS: 12.44 ± 1.29, p = 0.007). Because the il-10 morphant did not possess significantly fewer pyknotic nuclei at 36 h LT relative to the S.C. morphant (Supplementary Figures S1A, B, D, E), the reduced number of proliferating Müller glia in the il-10 morphant relative to the S.C. morphant was not due to decreased cell death. Additionally, Müller glia proliferation was rescued when IL-10 protein was intravitreally injected every 24 h into the il-10 morphants (Figures 5C, F, G; S.C. MO with IL-10: 21.56 ± 2.45. il-10 MO with IL-10: 27.22 ± 2.91, p > 0.99). This demonstrated that the il-10 morpholino specifically knocked down IL-10 expression, which is required for Müller glia proliferation following light damage.
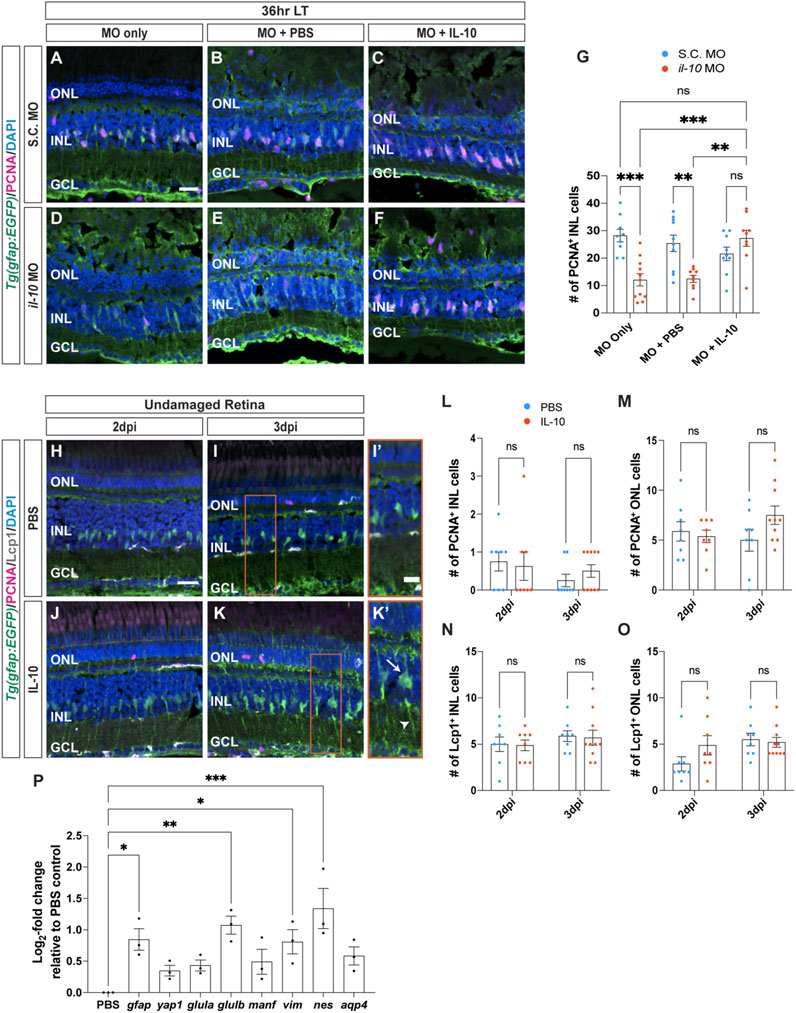
Figure 5. Cytokine IL-10 is necessary, but not sufficient, for Müller glia proliferation. (A–F) Confocal images of retinas from albino;Tg(gfap:EGFP)nt11 zebrafish that were electroporated with Standard Control (S.C.; (A–C) or il-10 morpholinos (D–F) prior to the start of constant light treatment and intravitreally injected with either MO only (A,D), PBS (B,E) or recombinant IL-10 protein (C,F), and placed in LT. Retinal sections were collected at 36 h LT and immunolabeled for GFP (Müller glia, green) and PCNA (proliferating cells, magenta), with DAPI counterstain (nuclei, blue). (G) Quantifications of the numbers of PCNA+ INL cells under different conditions. Müller glia proliferation was rescued in il-10 morphants injected with IL-10 protein. (H–K) Confocal images of albino;Tg(gfap:EGFP)nt11 retinas that were injected with either PBS (H,I) or recombinant IL-10 protein (J,K) in undamaged retinas every 24 h. Retinal sections were collected at 2- and 3-days following the first injection (dpi) and immunolabeled to detect GFP (Müller glia, green) and PCNA (proliferating cells, magenta), with DAPI counterstain (nuclei, blue). Red boxes in I and K were magnified to better portray the EGFP signal in the hypertrophied Müller glia (I’,K’). Arrow and arrowhead mark a hypertrophied cell body and process, respectively. (L–O) Quantifications of the numbers of PCNA+ cells (L, M) and Lcp1+ cells (N,O) in the INL (L,N) and ONL (M,O). (P) qRT-PCR analysis of gliotic-associated gene expression in undamaged retinas injected with IL-10 protein and collected at 3dpi. Data was normalized to 18 s rRNA reference gene and displayed as log2-fold change relative to the PBS (vehicle) control group. For the qRT-PCR, three independent replicates were performed with a pool of 6-7 dorsal retinas for each replicate. Cell count quantifications (G,L–O) were normalized to 300 μm along the length of the central-dorsal retina. Statistical analyses were performed using either a two-way ANOVA (G,L–O) or one-way ANOVA (P) both followed by Bonferroni’s post hoc test. Graphs represent the Mean ± SEM and n ≥ 8. *, p < 0.05, **, p < 0.01; ***, p < 0.001; ns, no significance. ONL, outer nuclear layer; INL, inner nuclear layer; GCL, ganglion cell layer. Scale bars in (A,H) are 20 μm and are the same for (B–F) and (I–K), respectively. Scale bar in (I′) is 10 μm and is the same for (K’).
To determine if IL-10 is sufficient to induce Müller glia proliferation, IL-10 protein was intravitreally injected into undamaged retinas every 24 h and collected at 2dpi and 3dpi (Figures 5H–K). There was no significant difference in the number of PCNA+ Müller glia between the PBS and IL-10 protein injected groups in either the INL or ONL at 2dpi (Figures 5L, M; PBS INL: 0.75 ± 0.25, IL-10 INL: 0.63 ± 0.38, p > 0.99; PBS ONL: 5.88 ± 0.97, IL-10 ONL: 5.38 ± 0.63, p > 0.99) and 3dpi (Figures 5L, M; PBS INL: 0.25 ± 0.16, IL-10 INL: 0.50 ± 0.17, p > 0.99; PBS ONL: 5.00 ± 1.10, IL-10 ONL: 7.50 ± 0.92, p > 0.99). There were also no significant difference in the number of Lcp1+ cells relative to the PBS controls in either the INL or ONL at 2dpi (Figures 5N, O; PBS INL: 5.00 ± 0.78, IL-10 INL: 4.88 ± 0.58, p > 0.99; PBS ONL: 2.88 ± 0.77, IL-10 ONL: 4.88 ± 1.04, p > 0.99) and at 3dpi (Figures 5N, O; PBS INL: 5.88 ± 0.58, IL-10 INL: 5.70 ± 0.82, p > 0.99; PBS ONL: 5.50 ± 0.68, IL-10 ONL: 5.20 ± 0.53, p > 0.99). Thus, IL-10 is necessary, but not sufficient to induce Müller glia proliferation.
Upon closer examination, undamaged retinas injected with IL-10 possessed Müller glia that displayed a gliotic morphology after 3dpi, with hypertrophied soma and processes (Figures 5I’, K’, arrows and arrowhead, respectively). To confirm that these Müller glia were gliotic, we performed qRT-PCR on various gliotic markers including gfap, yap1, glutamine synthase (glula/b), mesencephalic astrocyte-derived neurotrophic factor (manf), vimentin (vim), nestin (nes), and aquaporin 4 (aqp4) (Figure 5P). The gfap, glulb, vim, and nes gliotic marker genes were significantly upregulated in expression in the IL-10 injected retinas relative to the PBS (vehicle) control group after 3dpi (Figure 5P; gfap: 0.85 ± 0.30, p = 0.021; yap1: 0.35 ± 0.15, p > 0.99; glula: 0.43 ± 0.15, p = 0.73; glulb: 1.10 ± 0.25, p = 0.0026; manf: 0.49 ± 0.34, p = 0.46; vim: 0.81 ± 0.33, p = 0.030; nes: 1.3 ± 0.55, p = 0.0002; aqp4: 0.58 ± 0.25, p = 0.22). Thus, IL-10 is necessary for Müller glia proliferation in damaged retinas, while injection of IL-10 in undamaged retinas stimulated the Müller glia to exhibit a gliotic phenotype.
3.5 IL-1β can stimulate Müller glia proliferation, independent of IL-10 expression in undamaged retinas
We demonstrated that il-1β expression is induced before il-10 and both genes are required for Müller glia proliferation in light-damaged retinas. Additionally, injection of IL-1β into undamaged retinas induced il-10 expression and stimulated Müller glia proliferation. However, injection of IL-10 into the undamaged retina did not induce Müller glia proliferation. Thus, IL-1β may be required to induce il-10 expression in the undamaged retina in order to stimulate Müller glia and/or NPC proliferation. To test this hypothesis, we injected IL-1β protein into undamaged retinas with and without the il-10 morpholino. The albino;Tg(gfap:EGFP)nt11 zebrafish were electroporated with il-10 morpholino at 0dpi and then injected with recombinant zebrafish IL-1β protein every 24 h and collected at 3dpi (Figures 6B–D). These were compared to undamaged albino;Tg(gfap:EGFP)nt11 zebrafish that were injected with only recombinant zebrafish IL-1β protein every 24 h and collected at 3dpi (Figure 6A). The albino;Tg(gfap:EGFP)nt11 fish that were only injected with IL-1β possessed a large number of PCNA+ Müller glia and NPCs at 3dpi in both the INL and ONL (Figures 6A, E, F; INL: 56.44 ± 7.52, ONL: 58 ± 9.65). The il-10 morphants alone and the il-10 morphants injected with PBS (vehicle) control possessed very low numbers of PCNA+ Müller glia and NPCs in the INL (Figures 6B, C, E; il-10 MO:1.78 ± 0.68; il-10 MO with PBS: 5.40 ± 1.07) and ONL (Figures 6B, C, F; il-10 MO: 11.56 ± 2.19, il-10 MO with PBS: 11.40 ± 1.27). However, il-10 morphants injected with IL-1β protein possessed similar numbers of PCNA+ Müller glia and NPCs to zebrafish injected with only IL-1β protein in the INL (Figures 6A, D, E; IL-1β: 56.44 ± 7.52, il-10 MO with IL-1β: 64.73 ± 10.50, p > 0.99), but not in the ONL (Figures 6A, D, F; IL-1β: 58.33 ± 9.65, il-10 MO with IL-1β: 27.36 ± 5.92, p = 0.0027). This demonstrates that IL-1β induces Müller glia proliferation independent of IL-10 expression in the undamaged retina.
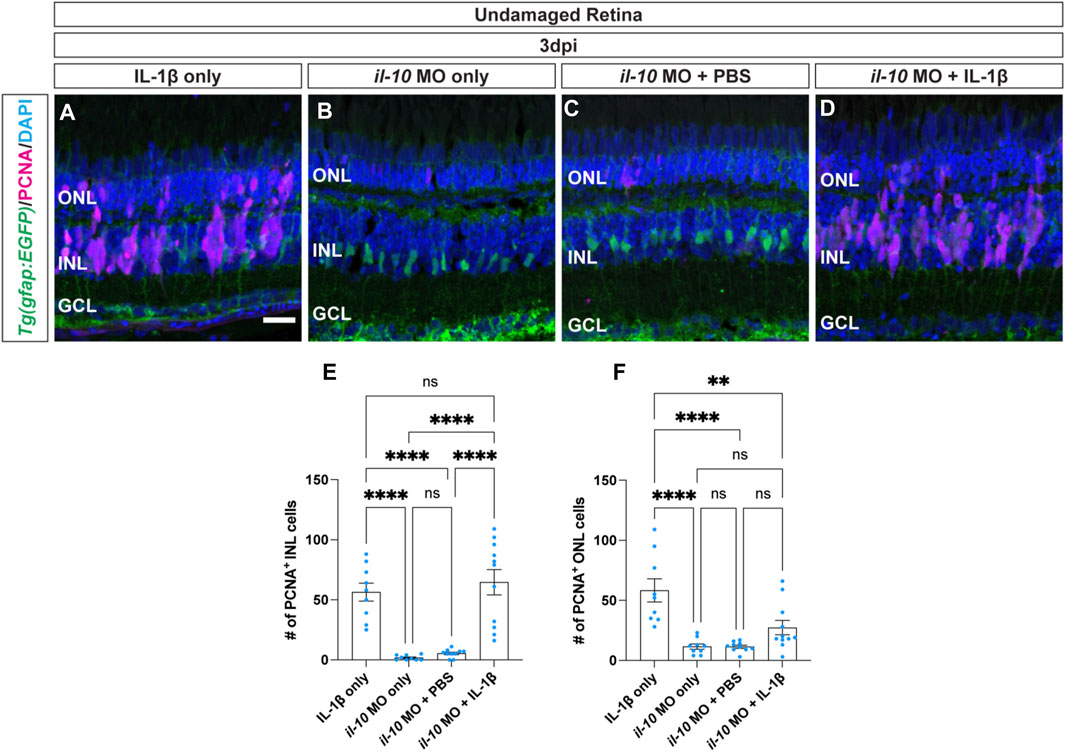
Figure 6. Cytokine IL-1β induction of Müller glia proliferation does not require IL-10. (A–D) Confocal images of undamaged albino;Tg(gfap:EGFP)nt11 zebrafish retinas that were intravitreally injected with IL-1β alone (A), or electroporated with il-10 morpholino and intravitreally injected with either MO only (B), PBS (C), or IL-1β protein (D). Sections were collected at 3 days post-injection (dpi) and immunostained for GFP (Müller glia, green) and PCNA (proliferating cells, magenta), with DAPI counterstain (nuclei, blue). (E) Quantifications of the numbers of PCNA+ INL cells under different conditions. (F) Quantifications of the numbers of PCNA+ ONL cells under different conditions. Quantifications were normalized to 300 μm along the central-dorsal retina. Statistical analyses were performed using one-way ANOVA followed by Tukey’s post hoc test. Mean ± SEM and n ≥ 9, **, p < 0.01; ****, p < 0.0001; ns, not significant. ONL, outer nuclear layer, INL, inner nuclear layer, GCL, ganglion cell layer. Scale bar in A is 20 μm and is the same for (B–D).
4 Discussion
In recent years, it was demonstrated that inflammation plays a critical role in regulating regeneration of zebrafish retinal neurons (Nagashima and Hitchcock, 2021). In this study, we focused on the expression of cytokines and their role in inducing Müller glia proliferation following light damage at the onset of retinal regeneration in adult zebrafish. We found that a subset of cytokines, which are usually known to induce inflammation, increases in expression shortly after the start of light-induced retinal damage. As their expression begins to decrease, a subset of cytokines, which are often associated with inhibiting inflammation, increases in expression, suggesting that this dynamic temporal pattern of expression is necessary for Müller glia proliferation and regeneration of retinal neurons following light damage. We then demonstrated that expression of the pro-inflammatory cytokine IL-1β is both necessary and sufficient to induce Müller glia proliferation. In contrast, expression of the cytokine IL-10 is necessary, but not sufficient, for Müller glia proliferation. Thus, the expression of various cytokines plays a key role in stimulating Müller glia proliferation following damage in the adult zebrafish retina.
We examined the expression of a subset of cytokines in light-damaged retinas. The expression of three cytokine genes, which usually exhibit pro-inflammatory effects: il-1β, il-6, and ifng1 were upregulated shortly after starting the constant light treatment, while three additional cytokine genes, which often possess anti-inflammatory effects: il-4, il-10, and il-13 were delayed before increasing their expression. These findings aligned with our previous study, which revealed differential expression of inflammatory genes following NMDA-damage in the chronic gosh cone degeneration mutant retinas (Iribarne and Hyde, 2022). This dynamic expression of cytokines can be generated in several different ways, with one possibility being that one or more pro-inflammatory cytokines induce the expression of cytokines, which are commonly associated with inhibiting inflammation. Consistent with this model, we found that intravitreal injection of recombinant IL-1β was sufficient to stimulate il-10 expression in undamaged retinas. This is similar to what was observed in rodent models of experimental autoimmune encephalomyelitis (EAE), where expression of IL-1β activated and translocated NF-ΚB to the nucleus and induced expression of inflammatory cytokines, IL-1β and TNF-α, as well as the IL-10 (Tomczak et al., 2006; Lawrence, 2009; Goldmann et al., 2013; Zhou et al., 2020). Whether other inflammatory cytokines induce the expression of additional cytokines associated with inhibiting inflammation, or if other regulatory mechanisms are at work here, remains to be determined.
Our finding that IL-1β is necessary to induce Müller glia proliferation in the damaged retina and sufficient to stimulate a similar response in undamaged retinas is similar to the ability of the inflammatory cytokine TNF-α inducing Müller glia proliferation in undamaged retinas (Nelson et al., 2013). However, these two inflammatory cytokines differ in the source of their initial expression, with TNF-α initially being expressed in dying retinal neurons (Nelson et al., 2013) and IL-1β expressed in microglia. However, tnfa is also expressed in microglia, but peaks in only 14% of the microglia at 20 h LT (Hoang et al., 2020). It is unlikely that both IL-1β and TNF-α induce Müller glia proliferation through redundant pathways because morpholino-mediated knockdown of either IL-1β or TNF-α is sufficient to significantly reduce Müller glia proliferation. Additionally, it is unlikely that they each stimulate an independent pathway because intravitreal injection of either IL-1β or TNF-α is sufficient to stimulate Müller glia proliferation. It is more likely that TNF-α expression from the dying retinal neurons stimulates the microglia, which in turn expresses IL-1β, and possibly also induces tnfa expression in the microglia, to induce the reprogramming and proliferation of the Müller glia (Figure 7). Alternatively, TNF-α stimulates both the microglia and Müller glia, although at a low level, and the stimulation of the microglia induces IL-1β expression that amplifies the induction of the Müller glia to reprogram and proliferate. In either case, IL-1β would function downstream of TNF-α in stimulating the maximal number of Müller glia to reprogram and proliferate.
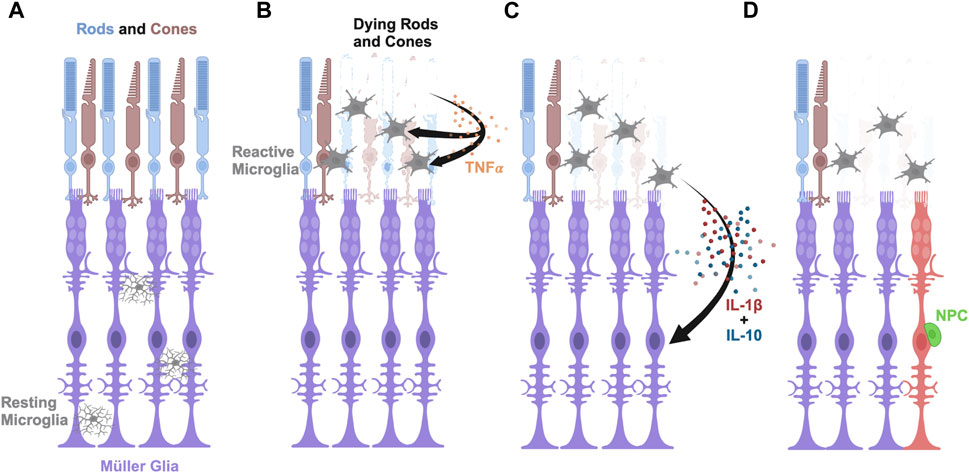
Figure 7. Model of pro-inflammatory cytokine IL-1β and anti-inflammatory cytokine IL-10 inducing Müller glia proliferation in light-damaged retinas. (A) Schematic depicting rod and cone photoreceptors (blue and brown, respectively), Müller glia (purple), and resting microglia (gray) in an undamaged retina. (B) Upon light damage, the resting microglia move from the locations in the plexiform layers to the outer retina where they become activated and begin phagocytosing dying rods and cones. The dying photoreceptors express TNFα. (C) The activated microglia express IL-1β and IL-10 in a dynamic fashion that signal the Müller glia to reprogram. (D) The reprogrammed Müller glia divide asymmetrically to produce a neuronal progenitor cell (NPC, green), which will continue to proliferate and differentiate into the missing retinal neurons.
We also examined the potential role of the IL-10 on Müller glia proliferation in the light-damaged adult zebrafish retina. Previous studies examined the role of anti-inflammatory cytokines, like IL-10, on ocular diseases, but the specific role of IL-10 on Müller glia reprogramming and proliferation has not been previously examined (Nakamura et al., 2015; Eastlake et al., 2016; Nikoopour et al., 2019). We found that IL-10 was necessary for Müller glia proliferation in light-damaged retinas, but it was not sufficient to induce Müller glia proliferation in undamaged retinas. Further, we demonstrated that intravitreal injection of IL-1β into undamaged retinas was sufficient to significantly induce IL-10 expression. Taken together, we propose that TNFα is produced by the dying photoreceptors (Nelson et al., 2013), which stimulates the expression of il-1β and il-10 in the microglia (Figures 7B, C). IL-1β further induces il-10 expression, which is required for Müller glia reprogramming and proliferation in the adult zebrafish retina to produce neuronal progenitor cells (Figure 7D). Thus, Müller glia proliferation in response to light damage requires the expression of both pro-inflammatory and anti-inflammatory cytokines. This would account for both IL-1β and IL-10 being required for Müller glia proliferation in the light-damaged retina, the ability of intravitreal injection of IL-1β to induce Müller glia proliferation in undamaged retinas (due to IL-1β inducing il-10 expression), and the inability of intravitreal injection of IL-10 to induce Müller glia proliferation in the undamaged retinas (due to the absence of increased il-1β expression).
Because IL-10 is necessary for Müller glia proliferation, it was unexpected that intravitreal injection of IL-10 into undamaged retinas induced Müller glia to enter a gliotic-like state rather than either stimulating Müller glia proliferation or having no discernible effect. It was previously demonstrated that Müller glia enter a transient gliotic state before reprogramming and proliferation, with a gliotic cell morphology, including cell hypertrophy, as well as increased expression of several gliotic genes (Thomas et al., 2018; Hoang et al., 2020). However, il-10 expression peaks several hours after the peak of il-1β expression in light-damaged retinas (Figure 2A). There are two possible explanations for how IL-10 could induce a gliotic state in undamaged retinas. First, there is a small, but significant increase in il-10 expression that coincides with increasing il-1β expression (Figure 2A). It is possible that a low level of IL-10 expression leads to the transient gliotic state before increasing IL-1β expression induces the Müller glia to reprogram and reenter the cell cycle. In this model, the application of a high level of IL-10 to the undamaged retina, without increased IL-1β expression, would lead to gliosis rather than Müller glia proliferation. In the second model, IL-10 does not induce the transient gliotic state in the light-damaged retina. However, in the undamaged retina, where regeneration signals (TNFα and IL-1β) are absent and IL-10 is exogenously applied, the IL-10 protein may induce the gliotic state through an unrelated mechanism. Differentiating between these two models will require precisely altering the dynamic timing and expression levels of these two cytokines.
What this model fails to account for is that intravitreal injection of IL-1β is sufficient to induce Müller glia proliferation in undamaged il-10 morphant retinas. Based on our model, intravitreal injection of IL-1β would require increased il-10 expression to stimulate Müller glia proliferation. It is possible that the morpholino-mediated knockdown does not entirely abolish il-10 expression. There are numerous examples where the morpholino does not entirely abolish expression of the target protein (Nelson et al., 2012; Gorsuch et al., 2017). In this case, the intravitreal injection of IL-1β could have been sufficiently large that it induced a greater level of expression of il-10 than present in the light-damaged retina, such that it induced a level of il-10 transcript was large enough to allow a sufficient amount of IL-10 protein even though the morpholino blocked a large amount of Il-10 protein translation. Alternatively, the intravitreal injection of IL-1β into undamaged retinas was greater than what is normally present in the light-damaged retina and this unusually high level of IL-1β had altered effects on other cytokines and this resulted in the nontraditional mechanism to induce Müller glia proliferation. Given the dynamic nature of cytokine expression following light damage (Figure 2A), it is possible that precise timing and level of individual cytokine expression are the critical components in Müller glia proliferation.
Although this study focused on the roles of two major cytokines, IL-1β and IL-10 in the light-damaged adult zebrafish retina, we cannot overlook the potential contributions of other cytokines involved in Müller glia proliferation and neuronal regeneration. IL-6 family cytokines, along with p-Stat3 signaling, were shown to stimulate zebrafish Müller glia reprogramming (Zhao et al., 2014). Similarly, TNF-α is required for Müller glia proliferation in the zebrafish retina, although it is produced in dying retinal neurons (Nelson et al., 2013). Outside the retina, IL-4 suppressed inflammation following zebrafish gill tissue damage (Bottiglione et al., 2020), while IL-10 and IL-4 signaling worked synergistically together to induce synaptogenesis in sensory hair cells and motor neurons in zebrafish (Denans et al., 2022). As numerous signaling events take place during damage and initiation of neuronal regeneration, the dynamic timing and interaction between these cytokines and both neuronal and non-neuronal cells found in the retina may play a critical role in determining the successful regeneration of neurons in the zebrafish retina following injury. While other cytokines and signaling molecules are likely involved in this mechanism to initiate and regulate retinal regeneration, the identification of IL-1β and IL-10 as being necessary for Müller glia proliferation in the regeneration process provides a strong foundation to build future investigations.
Data availability statement
The original contributions presented in the study are included in the article/Supplementary Material, further inquiries can be directed to the corresponding author. All scRNA-seq data and source codes are available at GitHub https://github.com/jiewwwang/Single-cell-retinal-regeneration. The scRNA-seq data can be queried interactively at https://proteinpaint.stjude.org/F/2019.retina.scRNA.html.
Ethics statement
The animal study was approved by University of Notre Dame Animal Care and Use Committee. The study was conducted in accordance with the local legislation and institutional requirements.
Author contributions
CL: Conceptualization, Writing–review and editing, Data curation, Formal Analysis, Investigation, Methodology, Validation, Visualization, Writing–original draft. DH: Conceptualization, Writing–review and editing, Funding acquisition, Project administration, Resources, Supervision.
Funding
The author(s) declare that financial support was received for the research, authorship, and/or publication of this article. This work was funded by the National Institutes of Health/National Eye Institute grants (U01-EY027267 and R01-EY034493), the Hiller Family Endowment for Excellence in Stem Cell Research, and the Center for Zebrafish Research at the University of Notre Dame.
Acknowledgments
We thank the staff of the Freimann Life Science Center technicians for zebrafish care and husbandry, the University of Notre Dame Integrated Imaging Facility, and the Optical Microscopy Core for support with imaging, and members of the Hyde lab for thoughtful discussion.
Conflict of interest
The authors declare that the research was conducted in the absence of any commercial or financial relationships that could be construed as a potential conflict of interest.
Publisher’s note
All claims expressed in this article are solely those of the authors and do not necessarily represent those of their affiliated organizations, or those of the publisher, the editors and the reviewers. Any product that may be evaluated in this article, or claim that may be made by its manufacturer, is not guaranteed or endorsed by the publisher.
Supplementary material
The Supplementary Material for this article can be found online at: https://www.frontiersin.org/articles/10.3389/fcell.2024.1406330/full#supplementary-material
SUPPLEMENTARY FIGURE S1 | Morpholino-mediated knockdown of either IL-1β or IL-10 does not significantly alter the extent of photoreceptor cell death following light damage. (A–C) Confocal images of albino;Tg(gfap:EGFP)nt11 zebrafish retinas that were electroporated with either Standard Control S.C; (A), il-1β (B), or il-10 (C) morpholinos prior to the start of constant light treatment. Retinas were collected at 36hr LT and counterstained with DAPI nuclei, blue; (A–C). Quantifications showing the number of pyknotic cells in the INL (D) and ONL (E) at 36hr LT. Cell counts were normalized to 300 μm along the length of the central-dorsal retina. Statistical analyses were performed using one-way ANOVA followed by Bonferroni’s post-hoc test. Graphs represent the Mean ± SEM and n ≥ 5. ns represents no significance. ONL, outer nuclear layer; INL, inner nuclear layer; GCL, ganglion cell layer. Scale Bar in A is 20 μm and is the same for (B,C).
SUPPLEMENTARY FIGURE S2 | Intravitreal injection of IL-1β protein does not induce cell death in the undamaged retina. (A–D) Confocal images of albino;Tg(gfap:EGFP)nt11 zebrafish retinas that were intravitreally injected with recombinant IL-1β protein in the undamaged retinas every 24 hours for 3 days. Sections were collected at 2- and 3- days following the first injection (dpi). TUNEL assay was performed to identify apoptotic cells (magenta) and retinal sections were immunostained with GFP (Müller glia, green) and counterstained with DAPI (nuclei, blue). (E,F) Quantifications showing the number of TUNEL+ cells in the INL (E) and ONL (F) at 2- and 3-dpi. Cell counts were normalized to 300 μm along the length of the central-dorsal retina. Statistical analyses were performed using two-way ANOVA followed by Bonferroni’s post-hoc test. Mean ± SEM, n ≥ 5. ns no significance. ONL, outer nuclear layer; INL, inner nuclear layer; GCL, ganglion cell layer. Scale bar in A is 20 μm and is the same for (B–D).
References
Angueyra, J. M., and Kindt, K. S. (2018). Leveraging zebrafish to study retinal degenerations. Front. Cell Dev. Biol. 6, 110–119. doi:10.3389/fcell.2018.00110
Bernardos, R. L., Barthel, L. K., Meyers, J. R., and Raymond, P. A. (2007). Late-stage neuronal progenitors in the retina are radial Müller glia that function as retinal stem cells. J. Neurosci. 27, 7028–7040. doi:10.1523/JNEUROSCI.1624-07.2007
Bosak, V., Murata, K., Bludau, O., and Brand, M. (2018). Role of the immune response in initiating central nervous system regeneration in vertebrates: learning from the fish. Int. J. Dev. Biol. 62, 403–417. doi:10.1387/ijdb.180033vb
Bottiglione, F., Dee, C. T., Lea, R., Zeef, L. A. H., Badrock, A. P., Wane, M., et al. (2020). Zebrafish IL-4–like cytokines and IL-10 suppress inflammation but only IL-10 is essential for gill homeostasis. J. Immunol. 205, 994–1008. doi:10.4049/jimmunol.2000372
Boyd, P., Campbell, L. J., and Hyde, D. R. (2023). Clcf1/Crlf1a-mediated signaling is neuroprotective and required for Müller glia proliferation in the light-damaged zebrafish retina. Front. Cell Dev. Biol. 11, 1142586–1142614. doi:10.3389/fcell.2023.1142586
Campbell, L. J., Hobgood, J. S., Jia, M., Boyd, P., Hipp, R. I., and Hyde, D. R. (2021). Notch3 and DeltaB maintain Müller glia quiescence and act as negative regulators of regeneration in the light-damaged zebrafish retina. Glia 69, 546–566. doi:10.1002/glia.23912
Casali, B. T., and Reed-Geaghan, E. G. (2021). Microglial function and regulation during development, homeostasis and alzheimer’s disease. Cells 10, 957–1028. doi:10.3390/cells10040957
Chen, D., Li, J., Huang, Y., Wei, P., Miao, W., Yang, Y., et al. (2022). Interleukin 13 promotes long-term recovery after ischemic stroke by inhibiting the activation of STAT3. J. Neuroinflammation 19, 112–128. doi:10.1186/s12974-022-02471-5
Cicchese, J. M., Evans, S., Hult, C., Joslyn, L. R., Wessler, T., Millar, J. A., et al. (2018). Dynamic balance of pro- and anti-inflammatory signals controls disease and limits pathology. Immunol. Rev. 285, 147–167. doi:10.1111/imr.12671
Conedera, F. M., Maria, A., Pousa, Q., Mercader, N., Tschopp, M., and Enzmann, V. (2019). Retinal microglia signaling affects Müller cell behavior in the zebrafish following laser injury induction. Glia 67, 1150–1166. doi:10.1002/glia.23601
Conner, C., Ackerman, K. M., Lahne, M., Hobgood, J. S., and Hyde, D. R. (2014). Repressing notch signaling and expressing TNFα are sufficient to mimic retinal regeneration by inducing Müller glial proliferation to generate committed progenitor cells. J. Neurosci. 34, 14403–14419. doi:10.1523/JNEUROSCI.0498-14.2014
Denans, N., Tran, N. T. T., Swall, M. E., Diaz, D. C., Blanck, J., and Piotrowski, T. (2022). An anti-inflammatory activation sequence governs macrophage transcriptional dynamics during tissue injury in zebrafish. Nat. Commun. 13, 5356. doi:10.1038/s41467-022-33015-3
Eastlake, K., Banerjee, P. J., Angbohang, A., Charteris, D. G., Khaw, P. T., and Limb, G. A. (2016). Müller glia as an important source of cytokines and inflammatory factors present in the gliotic retina during proliferative vitreoretinopathy. Glia 64, 495–506. doi:10.1002/glia.22942
Elmore, M. R. P., Najafi, A. R., Koike, M. A., Dagher, N. N., Spangenberg, E. E., Rice, R. A., et al. (2014). Colony-stimulating factor 1 receptor signaling is necessary for microglia viability, unmasking a microglia progenitor cell in the adult brain. Neuron 82, 380–397. doi:10.1016/j.neuron.2014.02.040
Frame, J. M., Kubaczka, C., Long, T. L., Esain, V., Soto, R. A., Hachimi, M., et al. (2020). Metabolic regulation of inflammasome activity controls embryonic hematopoietic stem and progenitor cell production. Dev. Cell 55, 133–149. doi:10.1016/j.devcel.2020.07.015
Frank, M. G., Baratta, M. V., Sprunger, D. B., Watkins, L. R., and Maier, S. F. (2007). Microglia serve as a neuroimmune substrate for stress-induced potentiation of CNS pro-inflammatory cytokine responses. Brain. Behav. Immun. 21, 47–59. doi:10.1016/j.bbi.2006.03.005
Fricker, M., Oliva-Martín, M. J., and Brown, G. C. (2012). Primary phagocytosis of viable neurons by microglia activated with LPS or Aβ is dependent on calreticulin/LRP phagocytic signalling. J. Neuroinflammation 9, 196–212. doi:10.1186/1742-2094-9-196
Fu, R., Shen, Q., Xu, P., Luo, J. J., and Tang, Y. (2014). Phagocytosis of microglia in the central nervous system diseases. Mol. Neurobiol. 49, 1422–1434. doi:10.1007/s12035-013-8620-6
Gemberling, M., Bailey, T. J., Hyde, D. R., and Poss, K. D. (2013). The zebrafish as a model for complex tissue regeneration. Trends Genet. 29, 611–620. doi:10.1016/j.tig.2013.07.003
Gemma, C., Bachstetter, A. D., Cole, M. J., Fister, M., Hudson, C., and Bickford, P. C. (2007). Blockade of caspase-1 increases neurogenesis in the aged hippocampus. Eur. J. Neurosci. 26, 2795–2803. doi:10.1111/j.1460-9568.2007.05875.x
Gestri, G., Link, B. A., and Neuhauss, S. C. F. (2012). The visual system of zebrafish and its use to model human ocular Diseases. Dev. Neurobiol. 72, 302–327. doi:10.1002/dneu.20919
Gogoleva, V. S., Drutskaya, M. S., and Atretkhany, K. S. N. (2019). The role of microglia in the homeostasis of the central nervous system and neuroinflammation. Mol. Biol. 53, 790–798. doi:10.1134/S0026898419050057
Goldmann, T., Wieghofer, P., Müller, P. F., Wolf, Y., Varol, D., Yona, S., et al. (2013). A new type of microglia gene targeting shows TAK1 to be pivotal in CNS autoimmune inflammation. Nat. Neurosci. 16, 1618–1626. doi:10.1038/nn.3531
Goldsmith, P., and Harris, W. A. (2003). The zebrafish as a tool for understanding the biology of visual disorders. Semin. Cell Dev. Biol. 14, 11–18. doi:10.1016/S1084-9521(02)00167-2
Gorsuch, R. A., and Hyde, D. R. (2014). Regulation of Müller glial dependent neuronal regeneration in the damaged adult zebrafish retina. Exp. Eye Res. 123, 131–140. doi:10.1016/j.exer.2013.07.012
Gorsuch, R. A., Lahne, M., Yarka, C. E., Petravick, M. E., Li, J., and Hyde, D. R. (2017). Sox2 regulates Müller glia reprogramming and proliferation in the regenerating zebrafish retina via Lin28 and Ascl1a. Exp. Eye Res. 161, 174–192. doi:10.1016/j.exer.2017.05.012
Han, J., Chitu, V., Stanley, E. R., Wszolek, Z. K., Karrenbauer, V. D., and Harris, R. A. (2022). Inhibition of colony stimulating factor-1 receptor (CSF-1R) as a potential therapeutic strategy for neurodegenerative diseases: opportunities and challenges. Cell. Mol. Life Sci. 79, 219–315. doi:10.1007/s00018-022-04225-1
Hao, Y., Hao, S., Andersen-Nissen, E., Mauck, W. M., Zheng, S., Butler, A., et al. (2021). Integrated analysis of multimodal single-cell data. Cell 184, 3573–3587.e29. doi:10.1016/j.cell.2021.04.048
Hoang, T., Wang, J., Boyd, P., Wang, F., Santiago, C., Jiang, L., et al. (2020). Gene regulatory networks controlling vertebrate retinal regeneration. Science 370, eabb8598. doi:10.1126/science.abb8598
Hui, B., Yao, X., Zhang, L., and Zhou, Q. (2020). Dexamethasone sodium phosphate attenuates lipopolysaccharide-induced neuroinflammation in microglia BV2 cells. Naunyn. Schmiedeb. Arch. Pharmacol. 393, 1761–1768. doi:10.1007/s00210-019-01775-3
Iribarne, M., and Hyde, D. R. (2022). Different inflammation responses modulate Müller glia proliferation in the acute or chronically damaged zebrafish retina. Front. Cell Dev. Biol. 10, 892271–892319. doi:10.3389/fcell.2022.892271
Jesudasan, S. J. B., Gupta, S. J., Churchward, M. A., Todd, K. G., and Winship, I. R. (2021). Inflammatory cytokine profile and plasticity of brain and spinal microglia in response to ATP and glutamate. Front. Cell. Neurosci. 15, 634020–634029. doi:10.3389/fncel.2021.634020
Kassen, S. C., Ramanan, V., Montgomery, J. E., Burket, C. T., Liu, C.-G., Vihtelic, T. S., et al. (2006). Time course analysis of gene expression during light-induced photoreceptor cell death and regeneration in albino zebrafish. Dev. Neurobiol. 67, 1009–1031. doi:10.1002/dneu.20362
Kyritsis, N., Kizil, C., Zocher, S., Kroehne, V., Kaslin, J., Freudenreich, D., et al. (2012). Acute inflammation initiates the regenerative response in the adult. Science 338, 1353–1357. doi:10.1126/science.1228773
Lahne, M., Brecker, M., Jones, S. E., and Hyde, D. R. (2021). The regenerating adult zebrafish retina recapitulates developmental fate specification programs. Dev. Neurobiol. 8, 617923. doi:10.3389/fcell.2020.617923
Lahne, M., Nagashima, M., Hyde, D. R., and Hitchcock, P. F. (2020). Reprogramming Müller glia to regenerate retinal neurons. Annu. Rev. Vis. Sci. 6, 171–193. doi:10.1146/annurev-vision-121219-081808
Lawrence, T. (2009). The nuclear factor NF-kappaB pathway in inflammation. Cold Spring Harb. Perspect. Biol. 1, 0016511–a1710. doi:10.1101/cshperspect.a001651
Lenkowski, J. R., and Raymond, P. A. (2014). Müller glia: stem cells for generation and regeneration of retinal neurons in teleost fish. Prog. Retin. Eye Res. 40, 94–123. doi:10.1016/j.preteyeres.2013.12.007
Liang, H., Sun, Y., Gao, A., Zhang, N., Jia, Y., Yang, S., et al. (2019). Ac-YVAD-cmk improves neurological function by inhibiting caspase-1- mediated in fl ammatory response in the intracerebral hemorrhage of rats. Int. Immunopharmacol. 75, 105771. doi:10.1016/j.intimp.2019.105771
Lin, X., Ye, H., Siaw-Debrah, F., Pan, S., He, Z., Ni, H., et al. (2018). Ac-YVAD-CMK inhibits pyroptosis and improves functional outcome after intracerebral hemorrhage. Biomed. Res. Int. 2018, 3706047. doi:10.1155/2018/3706047
Lively, S., Schlichter, L. C., and Symes, A. J. (2018). Microglia responses to pro-inflammatory stimuli (LPS, IFN γ + TNF α) and reprogramming by resolving cytokines (IL-4, IL-10). Front. Cell Neurosci. 12, 1–19. doi:10.3389/fncel.2018.00215
Lobo-Silva, D., Carriche, G. M., Castro, A. G., Roque, S., and Saraiva, M. (2017). Interferon-β regulates the production of IL-10 by toll-like receptor-activated microglia. Glia 65, 1439–1451. doi:10.1002/glia.23172
López-Muñoz, A., Sepulcre, M. P., Roca, F. J., Figueras, A., Meseguer, J., and Mulero, V. (2011). Evolutionary conserved pro-inflammatory and antigen presentation functions of zebrafish IFNγ revealed by transcriptomic and functional analysis. Mol. Immunol. 48, 1073–1083. doi:10.1016/j.molimm.2011.01.015
Lyu, P., Iribarne, M., Serjanov, D., Zhai, Y., Hoang, T., Campbell, L. J., et al. (2023). Common and divergent gene regulatory networks control injury-induced and developmental neurogenesis in zebrafish retina. Nat. Commun. 4, 8477. doi:10.1038/s41467-023-44142-w
Malicki, J., Pooranachandran, N., Nikolaev, A., Fang, X., and Avanesov, A. (2016) Analysis of the retina in the zebrafish model. Elsevier Ltd. doi:10.1016/bs.mcb.2016.04.017
Miao, W., Zhao, Y., Huang, Y., Chen, D., Luo, C., Su, W., et al. (2020). IL-13 ameliorates neuroinflammation and promotes functional recovery after traumatic brain injury. J. Immunol. 204, 1486–1498. doi:10.4049/jimmunol.1900909
Mitchell, D. M., Lovel, A. G., and Stenkamp, D. L. (2018). Dynamic changes in microglial and macrophage characteristics during degeneration and regeneration of the zebrafish retina. J. Neuroinflammation 15, 163–220. doi:10.1186/s12974-018-1185-6
Mitchell, D. M., Sun, C., Hunter, S. S., New, D. D., and Stenkamp, D. L. (2019). Regeneration associated transcriptional signature of retinal microglia and macrophages. Sci. Rep. 9, 4768–4817. doi:10.1038/s41598-019-41298-8
Muzio, L., Viotti, A., and Martino, G. (2021). Microglia in neuroinflammation and neurodegeneration: from understanding to therapy. Front. Neurosci. 15, 742065–742125. doi:10.3389/fnins.2021.742065
Nagashima, M., and Hitchcock, P. F. (2021). Inflammation regulates the multi-step process of retinal regeneration in Zebrafish. Cells 10, 783. doi:10.3390/cells10040783
Nakamura, R., Sene, A., Santeford, A., Gdoura, A., Kubota, S., Zapata, N., et al. (2015). IL10-driven STAT3 signalling in senescent macrophages promotes pathological eye angiogenesis. Nat. Commun. 6, 7847. doi:10.1038/ncomms8847
Nasevicius, A., and Ekker, S. C. (2000). Effective targeted gene “knockdown” in zebrafish. Nat. Genet. 26, 216–220. doi:10.1038/79951
Nelson, C. M., Ackerman, K. M., Hayer, P. O., Bailey, T. J., Gorsuch, R. A., and Hyde, D. R. (2013). Tumor necrosis factor-alpha is produced by dying retinal neurons and is required for Müller glia proliferation during zebrafish retinal regeneration. J. Neurosci. 33, 6524–6539. doi:10.1523/JNEUROSCI.3838-12.2013
Nelson, C. M., Gorsuch, R. A., Bailey, T. J., Ackerman, K. M., Kassen, S. C., and Hyde, D. R. (2012). Stat3 defines three populations of Müller glia and is required for initiating maximal Müller glia proliferation in the regenerating zebrafish retina. J. Comp. Neurol. 520, 4294–4311. doi:10.1002/cne.23213
Nikoopour, E., Lin, C., Sheskey, S., Heckenlively, J. R., and Lundy, S. K. (2019). Immune cell infiltration into the eye is controlled by IL-10 in recoverin-induced autoimmune retinopathy. J. Immunol. 202, 1057–1068. doi:10.4049/jimmunol.1800574
O’Garra, A., and Vieira, P. (2007). TH1 cells control themselves by producing interleukin-10. Nat. Rev. Immunol. 7, 425–428. doi:10.1038/nri2097
Paolicelli, R. C., Bolasco, G., Pagani, F., Maggi, L., Scianni, M., Panzanelli, P., et al. (2011). Synaptic pruning by microglia is necessary for normal brain development. Science 333, 1456–1458. doi:10.1126/science.1202529
Park, M. J., Park, H. S., You, M. J., Yoo, J., Kim, S. H., and Kwon, M. S. (2019). Dexamethasone induces a specific form of ramified dysfunctional microglia. Mol. Neurobiol. 56, 1421–1436. doi:10.1007/s12035-018-1156-z
Parkhurst, C. N., Yang, G., Ninan, I., Savas, J. N., Yates, J. R., Lafaille, J. J., et al. (2013). Microglia promote learning-dependent synapse formation through brain-derived neurotrophic factor. Cell 155, 1596–1609. doi:10.1016/j.cell.2013.11.030
Patton, E. E., and Tobin, D. M. (2019). Spotlight on zebrafish: the next wave of translational research. DMM Dis. Model. Mech. 12, dmm039370–2020. doi:10.1242/dmm.039370
Phillips, J. B., and Westerfield, M. (2014). Zebrafish models in translational research: tipping the scales toward advancements in human health. DMM Dis. Model. Mech. 7, 739–743. doi:10.1242/dmm.015545
Powell, C., Cornblath, E., Elsaeidi, F., Wan, J., and Goldman, D. (2016). Zebrafish Müller glia-derived progenitors are multipotent, exhibit proliferative biases and regenerate excess neurons. Sci. Rep. 6, 24851–24910. doi:10.1038/srep24851
Richardson, R., Webster, A., and Moosajee, M. (2017). The zebrafish eye — a paradigm for investigating human ocular genetics. Eye 31, 68–86. doi:10.1038/eye.2016.198
Rodríguez-Gómez, J. A., Kavanagh, E., Engskog-vlachos, P., Engskog, M. K., Herrera, A. J., Espinosa-Oliva, A. M., et al. (2020). Microglia: agents of the CNS pro-inflammatory response. Cells 9, 1717. doi:10.3390/cells9071717
Rosa, J. G. S., Lopes-Ferreira, M., and Lima, C. (2023). An overview towards zebrafish larvae as a model for ocular diseases. Int. J. Mol. Sci. 24, 5387. doi:10.3390/ijms24065387
Shemer, A., Scheyltjens, I., Frumer, G. R., Kim, J. S., Grozovski, J., Ayanaw, S., et al. (2020). Interleukin-10 prevents pathological microglia hyperactivation following peripheral endotoxin challenge. Immunity 53, 1033–1049. doi:10.1016/j.immuni.2020.09.018
Sherpa, T., Fimbel, S. M., Mallory, D. E., Maaswinkel, H., Spritzer, S. D., Sand, J. A., et al. (2008). Ganglion cell regeneration following whole-retina destruction in zebrafish. Dev. Neurobiol. 68, 166–181. doi:10.1002/dneu.20568
Sherpa, T., Lankford, T., Mcginn, T. E., Hunter, S. S., Frey, R. A., Sun, C., et al. (2014). Retinal regeneration is facilitated by the presence of surviving neurons. Dev. Neurobiol. 74, 851–876. doi:10.1002/dneu.22167
Silva, N. J., Nagashima, M., Li, J., Kakuk-Atkins, L., Ashrafzadeh, M., Hyde, D. R., et al. (2020). Inflammation and matrix metalloproteinase 9 (Mmp-9) regulate photoreceptor regeneration in adult zebrafish. Glia 68, 1445–1465. doi:10.1002/glia.23792
Smith, J. A., Das, A., Ray, S. K., and Banik, N. L. (2012). Role of pro-inflammatory cytokines released from microglia in neurodegenerative diseases. Brain Res. Bull. 87, 10–20. doi:10.1016/j.brainresbull.2011.10.004
Soliman, A. M., and Barreda, D. R. (2023). Acute inflammation in tissue healing. Int. J. Mol. Sci. 24, 641. doi:10.3390/ijms24010641
Streit, W. J., Mrak, R. E., and Griffin, W. S. T. (2004). Microglia and neuroinflammation: a pathological perspective. J. Neuroinflammation 1, 14–4. doi:10.1186/1742-2094-1-14
Sun, Y., Ma, J., Li, D., Li, P., Zhou, X., Li, Y., et al. (2019). Interleukin-10 inhibits interleukin-1β production and inflammasome activation of microglia in epileptic seizures. J. Neuroinflammation 16, 66–13. doi:10.1186/s12974-019-1452-1
Tanaka, Y., Matsuwaki, T., Yamanouchi, K., and Nishihara, M. (2013). Exacerbated inflammatory responses related to activated microglia after traumatic brain injury in progranulin-deficient mice. Neuroscience 231, 49–60. doi:10.1016/j.neuroscience.2012.11.032
Thomas, J. L., Morgan, G. W., Dolinski, K. M., and Thummel, R. (2018). Characterization of the pleiotropic roles of Sonic Hedgehog during retinal regeneration in adult zebrafish. Exp. Eye Res. 166, 106–115. doi:10.1016/j.exer.2017.10.003
Thomas, J. L., Ranski, A. H., Morgan, G. W., and Thummel, R. (2016). Reactive gliosis in the adult zebra fi sh retina. Exp. Eye Res. 143, 98–109. doi:10.1016/j.exer.2015.09.017
Thummel, R., Bailey, T. J., and Hyde, D. R. (2011). In vivo electroporation of morpholinos into the adult zebrafish retina. J. Vis. Exp., e3603–e3607. doi:10.3791/3603
Tomczak, M. F., Erdman, S. E., Davison, A., Wang, Y. Y., Nambiar, P. R., Rogers, A. B., et al. (2006). Inhibition of Helicobacter hepaticus-induced colitis by IL-10 requires the p50/p105 subunit of NF-kappa B. J. Immunol. 177, 7332–7339. doi:10.4049/jimmunol.177.10.7332
Van Dyck, A., Bollaerts, I., Beckers, A., Vanhunsel, S., Glorian, N., van houcke, J., et al. (2021). Müller glia–myeloid cell crosstalk accelerates optic nerve regeneration in the adult zebrafish. Glia 69, 1444–1463. doi:10.1002/glia.23972
Vihtelic, T. S., and Hyde, D. R. (2000). Light-induced rod and cone cell death and regeneration in the adultalbino zebrafish (Danio rerio) retina. J. Neurobiol. 44, 289–307. doi:10.1002/1097-4695(20000905)44:3<289::AID-NEU1>3.0.CO;2-H
Vojtech, L. N., Scharping, N., Woodson, J. C., and Hansen, J. D. (2012). Roles of inflammatory caspases during processing of zebrafish interleukin-1β in Francisella noatunensis infection. Infect. Immun. 80, 2878–2885. doi:10.1128/IAI.00543-12
Wang, X.-L., and Li, L. (2021). Microglia regulate neuronal circuits in homeostatic and high-fat diet-induced inflammatory conditions. Front. Cell. Neurosci. 15, 722028–722114. doi:10.3389/fncel.2021.722028
Wattrus, S. J., Smith, M. L., Rodrigues, C. P., Hagedorn, E. J., Kim, J. W., Budnik, B., et al. (2022). Quality assurance of hematopoietic stem cells by macrophages determines stem cell clonality. Science 377, 1413–1419. doi:10.1126/science.abo4837
White, D. T., Sengupta, S., Saxena, M. T., Xu, Q., Hanes, J., Ding, D., et al. (2017). Immunomodulation-accelerated neuronal regeneration following selective rod photoreceptor cell ablation in the zebrafish retina. Proc. Natl. Acad. Sci. 114, E3719–E3728. doi:10.1073/pnas.1617721114
Wolf, S. A., Boddeke, H. W. G. M., and Kettenmann, H. (2017). Microglia in physiology and disease. Annu. Rev. Physiol. 79, 619–643. doi:10.1146/annurev-physiol-022516-034406
Zhang, Z., Hou, H., Yu, S., Zhou, C., Zhang, X., Li, N., et al. (2020). Inflammation-induced mammalian target of rapamycin signaling is essential for retina regeneration. Glia 68, 111–127. doi:10.1002/glia.23707
Zhao, X. F., Wan, J., Powell, C., Ramachandran, R., Myers, M. G., and Goldman, D. (2014). Leptin and IL-6 family cytokines synergize to stimulate Müller Glia reprogramming and retina regeneration. Cell Rep. 9, 272–284. doi:10.1016/j.celrep.2014.08.047
Keywords: zebrafish, retina, regeneration, Müller glia, microglia, cytokines, IL-1β, IL-10
Citation: Lu C and Hyde DR (2024) Cytokines IL-1β and IL-10 are required for Müller glia proliferation following light damage in the adult zebrafish retina. Front. Cell Dev. Biol. 12:1406330. doi: 10.3389/fcell.2024.1406330
Received: 24 March 2024; Accepted: 16 May 2024;
Published: 13 June 2024.
Edited by:
Francisco Javier Diaz Corrales, Spanish National Research Council (CSIC), SpainReviewed by:
Muriel PERRON, Centre National de la Recherche Scientifique (CNRS), FranceDiana Marie Mitchell, University of Idaho, United States
Copyright © 2024 Lu and Hyde. This is an open-access article distributed under the terms of the Creative Commons Attribution License (CC BY). The use, distribution or reproduction in other forums is permitted, provided the original author(s) and the copyright owner(s) are credited and that the original publication in this journal is cited, in accordance with accepted academic practice. No use, distribution or reproduction is permitted which does not comply with these terms.
*Correspondence: David R. Hyde, ZGh5ZGVAbmQuZWR1