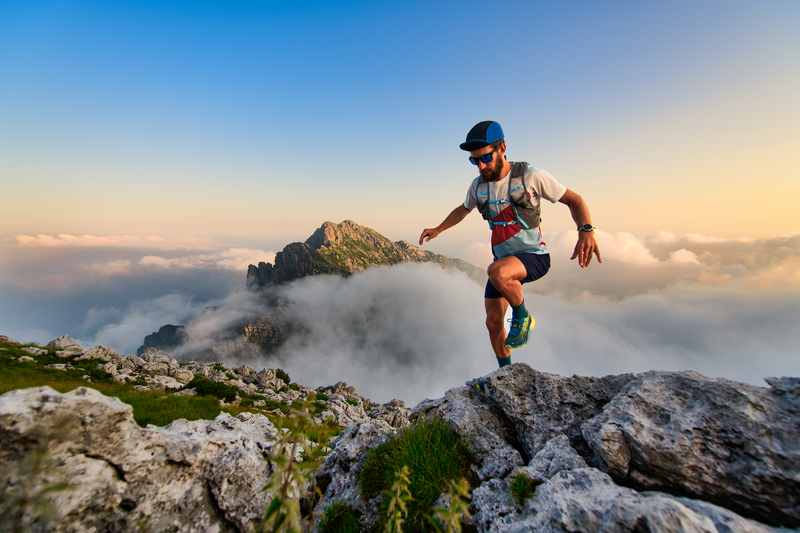
95% of researchers rate our articles as excellent or good
Learn more about the work of our research integrity team to safeguard the quality of each article we publish.
Find out more
OPINION article
Front. Cell Dev. Biol. , 24 May 2024
Sec. Membrane Traffic and Organelle Dynamics
Volume 12 - 2024 | https://doi.org/10.3389/fcell.2024.1392810
This article is part of the Research Topic Autophagy Beyond Degradation: Unraveling the Secretory Function of the Endo-lysosomal System View all 4 articles
Autophagic degradation of mitochondria, or mitophagy, has been widely studied as an important process for mitochondrial quality control (see review (Picca et al., 2023)). Intriguingly, recent studies reveal that mitochondrial clearance relies on extracellular vesicles (EVs) in the absence of the mATG8-conjugation system (Tan et al., 2022) or functional lysosomes (Liang et al., 2023), indicating the emerging role of secretory autophagy in organelle secretion. Moreover, mitochondria can be packed into newly identified types of EVs, such as cardiac exophers during heterophagy for damaged mitochondria release from cardiomyocytes (Nicolas-Avila et al., 2020; Nicolas-Avila et al., 2022), mitosomes (specialized migrasomes) during mitocytosis for damaged mitochondria release from migrating cells (Jiao et al., 2021), and mitophers during mitopherogenesis for healthy mitochondria release from worm spermatids (Liu et al., 2023). Mitochondria-containing EVs (hereinafter referred as to Mito-EVs) can later be internalized by macrophages for elimination (Nicolas-Avila et al., 2020; Liang et al., 2023) or stimulate innate immune response in other recipient cells (Tan et al., 2022).
In this opinion piece, we compare the molecular mechanisms underlying the formation of five types of recently reported Mito-EVs and discuss the potential roles of secretory autophagy in releasing both damaged and healthy mitochondria.
Mitochondria have been reported to transfer across cells through tunneling nanotubes, within extracellular vesicles, or in a carrier-free manner (see review (Borcherding and Brestoff, 2023)). In the past two decades, mitochondrial-derived vesicles (MDVs) containing mitochondrial components and Mito-EVs containing fragmented or intact mitochondria have been observed in many cell types like mesenchymal stem cells (Spees et al., 2006; Islam et al., 2012), endothelial cells (Tramontano et al., 2010), platelets (Boudreau et al., 2014), and iPSC-derived cardiomyocytes (Ikeda et al., 2021). MDVs and Mito-EVs are formed under physiological and pathological conditions and may exert therapeutic effects in various diseases (see review (Zhou et al., 2023; Hazan Ben-Menachem et al., 2024)). Here, we compare five recently reported processes that produce Mito-EVs (Table 1) in depths.
Mito-EVs vary in size and markers. Cardiac exophers are the largest, ranging from 3.5 ± 0.1 μm in diameter, positive for the “eat-me” signal externalized phosphatidylserine, and the autophagosomal marker LC3 (Nicolas-Avila et al., 2020), while lysosomal impairment-induced Mito-EVs are the smallest, ranging from 115 to 550 nm in diameter, positive for multivesicular body (MVB) and EV markers CD81, ALIX, and TSG101 but not CD63, as well as autophagosomal markers LC3 and SQSTM1/p62 (Liang et al., 2023). Mitosomes contain multiple small mitochondria of 240 nm size on average, positive for the migrasomal marker TSPAN4 (Jiao et al., 2021). During the autophagic secretion of mitochondria (ASM), the secreted mitochondria are bigger than 0.22 μm, negative for the EV markers CD63 and CD9 (Tan et al., 2022). The mitochondria in the abovementioned processes are mostly damaged, assessed by morphology, mitochondrial membrane potential (ΔΨm), or other markers (Nicolas-Avila et al., 2020; Jiao et al., 2021; Tan et al., 2022). In contrast, mitophers are 720 nm in diameter, positive for externalized phosphatidylserine, embedding a single healthy mitochondrion of 600 nm size on average with normal morphology and ΔΨm (Liu et al., 2023).
Mito-EVs function in the damaged mitochondria quality control or healthy mitochondria quantity control. Cardiac exophers and lysosomal impairment-induced CD81-positive Mito-EVs can be secreted from cardiomyocytes and taken up by macrophages in the neighborhood for degradation to prevent inflammation (Nicolas-Avila et al., 2020; Liang et al., 2023), a process called heterophagy (Nicolas-Avila et al., 2022). Cardiac-resident macrophages (cMacs) play an important role in maintaining mitochondrial homeostasis in the heart: short-term depletion of cMacs results in an accumulation of cardiac exophers, while the long-term depletion of cMacs reduces the formation of cardiac exophers through inflammasome activation and autophagy block in cardiomyocytes (Nicolas-Avila et al., 2020). ASM takes place upon both mATG8-lipidation deficiency and mitochondrial stress in donor cells and stimulates cGAS–STING-mediated innate immune response in recipient cells of the same type (Tan et al., 2022). Mitosomes are a kind of migrasomes, released by various migrating cells upon low doses of mitochondrial stressors (Jiao et al., 2021). Mitophers bud off C. elegans spermatids to reduce around 1/3 quantity of mitochondria to maintain sperm motility and fertility (Liu et al., 2023). It is not clear how mitosomes and mitophers are ultimately cleared; we speculate that they can be internalized by other cells, for elimination or for material and/or signal transfer, since several kinds of migrasomes have been implicated to regulate lateral transfer of cellular contents (Yu and Yu, 2022; Zhang et al., 2023) and worm exophers produced from body wall muscles, a small population of which contains intact mitochondria and can transport yolk proteins to oocytes (Turek et al., 2021).
The molecular mechanisms underlying the formation of Mito-EVs are characterized at different stages, including upstream signaling transduction, mitochondria translocation, cargo recognition, vesicle fusion, or budding. Mitophers are triggered by a signaling cascade consisting of extracellular protease TRY-5, transmembrane protein SPE-12, and tyrosine kinase SPE-8 (Liu et al., 2023). Microtubules, actin cytoskeleton, and motor proteins play a vital role in mitochondria translocation. Actin polymerization is required and is sufficient to promote mitopher formation. Myosin VI SPE-15 negatively regulates mitochondrial outward transport on actin filaments (Liu et al., 2023). During mitocytosis, the microtubule outward motor KIF5B and the actin motor Myo19 facilitate the transport of tubular mitochondria to the bottom of the cells to form mitosomes, while microtubule inward motor dynein inhibits the process; the tips of tubular mitochondria undergo Drp1-mediated fission before the mitochondrial fragments on the retraction fibers are packed into migrasomes, whose formation requires cell migration and tetraspanin proteins (Jiao et al., 2021). Autophagy proteins exhibit complex functions in cargo recognition and vesicle formation during cardiac exophergenesis, ASM, and lysosomal impairment-induced mitochondrial secretion, which are discussed in details in the later section. To release Mito-EVs, RAB27A mediates the docking and fusion of MVB to the plasma membrane (Liang et al., 2023), whereas SNAP23 mediates the fusion of mitophagosome to the plasma membrane, and the authors suggest that the secreted mitochondria are non-enveloped during ASM (Tan et al., 2022). Mitophers are released by direct budding from the plasma membrane, although the molecular mechanism remains elusive (Liu et al., 2023). It is not examined how cardiac exophers get released (Nicolas-Avila et al., 2020). Since cardiac exophers contain mitophagosomes, a fusion step between MVB or amphisomes to the plasma membrane may occur.
Mito-EVs show different dependencies on the autophagic pathway. Cardiac exophers, positive for LC3, require the mATG8-conjugation system as ATG7 knockdown in cardiomyocytes reduces the number of cardiac exophers, and autophagy induction by rapamycin is sufficient to induce cardiac exopher production (Nicolas-Avila et al., 2020). The mATG8-conjugation system is dispensable for lysosomal impairment-induced CD81-positive Mito-EV secretion since ATG5 or ATG7 knockdown shows no effect; interestingly, LC3 and p62 are secreted in CD81-positive EVs upon the same treatment (Liang et al., 2023), suggesting that Mito-EVs may carry such cargos as ubiquitinated proteins, and LC3/p62-dependent and independent EV cargo sorting pathways may co-exist. Consistent with this thought, LIR-containing RNA-binding proteins have been reported to secrete in LC3-positive EVs through a process named LC3-dependent EV loading and secretion (LDELS) (Leidal et al., 2020). When the mATG8-conjugation system is defective (ATG3-, ATG5-, and ATG7-knockout), PINK1–Parkin and the mitophagy cargo receptor NDP52 mediate the sorting of damaged mitochondria; upstream ATG proteins such as ATG9A, ULK1 complex, and PI3KC3-C1 complex control the formation of mitophagosomes, which later fuse with the plasma membrane instead of lysosomes (Tan et al., 2022). These results indicate an inhibitory role of mATG8-conjugation machinery and a positive role of upstream ATG proteins on secretory autophagy. Notably, not all studies have evaluated ATG proteins upstream of mATG8 conjugation, and it remains elusive whether ATG proteins mediating autophagosome initiation and elongation regulate the formation of CD81-positive Mito-EVs, mitosomes, and mitophers, although the authors claim they are autophagy-independent.
Mito-EV formation barely needs lysosomal function. Worm spermatids that generate mitophers lack lysosomes (Liu et al., 2023). When lysosomal function is compromised by pharmacological inhibitors (bafilomycin A1 and chloroquine), RAB7A depletion or dysregulation (inactive mutation T22N), LAMP2 depletion or Danon disease-causing mutations, or aging, CD81-positive Mito-EVs take charge to remove mitochondria (Liang et al., 2023). Pharmacological inhibition of lysosomes does not affect ASM, and the colocalization of mitochondria and LAMP2 is reduced during ASM (Tan et al., 2022). It is worth noting that after Mito-EVs are captured by recipient cells, especially macrophages, the clearance of Mito-EVs still involves lysosomal degradation (Nicolas-Avila et al., 2020; Liang et al., 2023). This suggests that when mitophagy cannot occur in donor cells, Mito-EV secretion and heterophagy in recipient cells are compensatory processes.
In the past decade, an accumulating body of evidence has uncovered the role of secretory autophagy in conventional and unconventional secretions of a wide range of cargos for cellular homeostasis or intercellular communications (Ponpuak et al., 2015; Piletic et al., 2023). However, the definition of secretory autophagy is still vague. For a cargo secretion process to be considered secretory autophagy, how many autophagy proteins, which may function at different stages of the autophagic pathway, shall be required? Shall autophagy proteins be on or in the cargo carriers? Shall autophagy proteins be sufficient to promote cargo secretion upon activation? It is urgent for the field to reach a consensus. Furthermore, the relationship between degradative and secretory autophagy needs in-depth investigation. It is intuitive to assume that the divergence point of degradative and secretory autophagy is the fusion step of autophagosomes. When autophagosome fuses with lysosome, cargos undergo degradative autophagy; when autophagosome fuses with late endosome, MVB, and/or the plasma membrane, cargos undergo secretory autophagy. However, as discussed above, neither ASM (Tan et al., 2022) nor lysosomal impairment-induced CD81-positive Mito-EV secretion (Liang et al., 2023) requires the mATG8-conjugation system, suggesting that the divergence point of degradative and secretory autophagy is rather upstream, likely the formation step of autophagosomes.
Mitochondria are well-known cargos of degradative autophagy (see review (Picca et al., 2023)), and they could be cargos of secretory autophagy as well (Table 1). The secretion of cardiac exophers sets a good example of secretory autophagy because (1) autophagy is necessary for the Mito-EV secretion (ATG7-dependent); (2) autophagy protein is on the Mito-EV (LC3-positive); and (3) autophagy is sufficient for the Mito-EV secretion (rapamycin-induced). ASM shares the first two features with the secretion of cardiac exophers, the necessity of autophagy (ATG9A, ULK1 complex, and PI3KC3-C1 complex-dependent) and the presence of autophagy protein in the carriers (NDP52-positive), while the sufficiency of autophagy has not been tested. We propose that the following steps are involved in the secretory autophagy of mitochondria, applicable to different types of Mito-EVs: cargo sorting via autophagy receptor-cargo recognition, autophagosome formation, and the plasma membrane fusion or budding (Figure 1). Future studies shall evaluate more ATG proteins than the mATG8-conjugation system to determine whether secretory autophagy occurs.
Figure 1. Secretory autophagy may mediate the secretion of mitochondria and signaling molecules. The first step is cargo sorting where autophagy receptors recognize such cargos as damaged mitochondria (“waste”) and healthy mitochondria (“food”). The second step is the formation of mitophagosomes, resembling waste storage and food packing. The last step is plasma membrane fusion or budding, resembling waste disposal and food delivery. In the five recent studies on Mito-EVs discussed in this opinion, the autophagy receptor NDP52 regulates the sorting of damaged mitochondria (Tan et al., 2022), and the autophagy receptor SQSTM1/p62 regulates the sorting of ubiquitinated proteins to CD81-positive Mito-EVs (Liang et al., 2023). ATG9A, ULK1 complex, and PI3KC3-C1 complex control the formation of mitophagosomes (autophagosomes that contain mitochondria) (Tan et al., 2022), while the mATG8-conjugation system may also be required (Nicolas-Avila et al., 2020). The fusion to the plasma membrane is mediated by SNAP23 for mitophagosomes (Tan et al., 2022) or by RAB27A for MVB containing CD81-positive Mito-EVs (Liang et al., 2023). The direct budding from the plasma membrane is regulated by actin cytoskeleton and myosin VI motor to form mitophers (each contains a healthy mitochondrion) (Liu et al., 2023) or mediated by the tetraspanin protein TSPAN9 and myosin II motor to form mitosomes (specialized migrasomes) (Jiao et al., 2021), although it is not clear if direct budding is involved in secretory autophagy. Whether healthy mitochondria are sorted, packed, and delivered via similar mechanisms require further investigation (open arrows and question marks).
In addition, we propose that both damaged and healthy mitochondria could be the cargos of secretory autophagy (Figure 1, “waste” vs. “food”): different cargos are sorted via distinct receptor–cargo pairs and packed into mitophagosomes. EVs that contain healthy mitochondria can be transferred from bone marrow-derived stromal cells to alveolar epithelial cells in injured lungs, restoring bioenergetics and improving survival (Islam et al., 2012). Healthy mitochondria-containing EVs can also be isolated from stem cell-derived cardiomyocytes, taken up by cardiomyocytes in failing hearts, restoring bioenergetics and improving cardiac function (Ikeda et al., 2021). Although the role of secretory autophagy in healthy mitochondria release has not been reported, we think worm exophers which are produced from body wall muscles and delivered to oocytes may be the case. Muscular exophers contain organelles like intact mitochondria and large protein complexes, and the production is autophagy-dependent (ATG7 and ATG8-dependent) (Turek et al., 2021). The secretory autophagosomes may encapsulate various cargos and signaling molecules, which is common for degradative autophagosomes. After fusing with or budding off the plasma membrane, the cargos can be disposed to the extracellular space or delivered to recipient cells. Future studies shall focus on the cargo sorting mechanisms of mitochondria and identify additional cargos on the same ride.
In summary, Mito-EVs are novel structures that cells generate for mitochondrial quality or quantity control, some of which rely on secretory autophagy. Future research on autophagy proteins and Mito-EVs will not only shed light on the role of secretory autophagy in mitochondrial homeostasis but also on the mechanistic distinctions between degradative and secretory autophagy.
YG: writing–original draft. YZu: writing–original draft. LF: writing–original draft. YZo: writing–original draft and writing–review and editing.
The author(s) declare that financial support was received for the research, authorship, and/or publication of this article. This work was supported by the National Natural Science Foundation of China (32270804), Northwest A&F University Start-up Funding (2190021004), and Shaanxi Province Undergraduate Training Programs for Innovation and Entrepreneurship (S202310712442).
The authors declare that the research was conducted in the absence of any commercial or financial relationships that could be construed as a potential conflict of interest.
All claims expressed in this article are solely those of the authors and do not necessarily represent those of their affiliated organizations, or those of the publisher, the editors, and the reviewers. Any product that may be evaluated in this article, or claim that may be made by its manufacturer, is not guaranteed or endorsed by the publisher.
Borcherding, N., and Brestoff, J. R. (2023). The power and potential of mitochondria transfer. Nature 623, 283–291. doi:10.1038/s41586-023-06537-z
Boudreau, L. H., Duchez, A. C., Cloutier, N., Soulet, D., Martin, N., Bollinger, J., et al. (2014). Platelets release mitochondria serving as substrate for bactericidal group IIA-secreted phospholipase A2 to promote inflammation. Blood 124, 2173–2183. doi:10.1182/blood-2014-05-573543
Hazan Ben-Menachem, R., Pines, O., and Saada, A. (2024). Mitochondrial derived vesicles- quo vadis? FEBS J. doi:10.1111/febs.17103
Ikeda, G., Santoso, M. R., Tada, Y., Li, A. M., Vaskova, E., Jung, J. H., et al. (2021). Mitochondria-rich extracellular vesicles from autologous stem cell-derived cardiomyocytes restore energetics of ischemic myocardium. J. Am. Coll. Cardiol. 77, 1073–1088. doi:10.1016/j.jacc.2020.12.060
Islam, M. N., Das, S. R., Emin, M. T., Wei, M., Sun, L., Westphalen, K., et al. (2012). Mitochondrial transfer from bone-marrow-derived stromal cells to pulmonary alveoli protects against acute lung injury. Nat. Med. 18, 759–765. doi:10.1038/nm.2736
Jiao, H., Jiang, D., Hu, X., Du, W., Ji, L., Yang, Y., et al. (2021). Mitocytosis, a migrasome-mediated mitochondrial quality-control process. Cell 184, 2896–2910.e13. doi:10.1016/j.cell.2021.04.027
Leidal, A. M., Huang, H. H., Marsh, T., Solvik, T., Zhang, D., Ye, J., et al. (2020). The LC3-conjugation machinery specifies the loading of RNA-binding proteins into extracellular vesicles. Nat. Cell Biol. 22, 187–199. doi:10.1038/s41556-019-0450-y
Liang, W., Sagar, S., Ravindran, R., Najor, R. H., Quiles, J. M., Chi, L., et al. (2023). Mitochondria are secreted in extracellular vesicles when lysosomal function is impaired. Nat. Commun. 14, 5031. doi:10.1038/s41467-023-40680-5
Liu, P., Shi, J., Sheng, D., Lu, W., Guo, J., Gao, L., et al. (2023). Mitopherogenesis, a form of mitochondria-specific ectocytosis, regulates sperm mitochondrial quantity and fertility. Nat. Cell Biol. 25, 1625–1636. doi:10.1038/s41556-023-01264-z
Nicolas-Avila, J. A., Lechuga-Vieco, A. V., Esteban-Martinez, L., Sanchez-Diaz, M., Diaz-Garcia, E., Santiago, D. J., et al. (2020). A network of macrophages supports mitochondrial homeostasis in the heart. Cell 183, 94–109. doi:10.1016/j.cell.2020.08.031
Nicolas-Avila, J. A., Pena-Couso, L., Munoz-Canoves, P., and Hidalgo, A. (2022). Macrophages, metabolism and heterophagy in the heart. Circ. Res. 130, 418–431. doi:10.1161/CIRCRESAHA.121.319812
Picca, A., Faitg, J., Auwerx, J., Ferrucci, L., and D'amico, D. (2023). Mitophagy in human health, ageing and disease. Nat. Metab. 5, 2047–2061. doi:10.1038/s42255-023-00930-8
Piletic, K., Alsaleh, G., and Simon, A. K. (2023). Autophagy orchestrates the crosstalk between cells and organs. EMBO Rep. 24, e57289. doi:10.15252/embr.202357289
Ponpuak, M., Mandell, M. A., Kimura, T., Chauhan, S., Cleyrat, C., and Deretic, V. (2015). Secretory autophagy. Curr. Opin. Cell Biol. 35, 106–116. doi:10.1016/j.ceb.2015.04.016
Spees, J. L., Olson, S. D., Whitney, M. J., and Prockop, D. J. (2006). Mitochondrial transfer between cells can rescue aerobic respiration. Proc. Natl. Acad. Sci. U. S. A. 103, 1283–1288. doi:10.1073/pnas.0510511103
Tan, H. W. S., Lu, G., Dong, H., Cho, Y. L., Natalia, A., Wang, L., et al. (2022). A degradative to secretory autophagy switch mediates mitochondria clearance in the absence of the mATG8-conjugation machinery. Nat. Commun. 13, 3720. doi:10.1038/s41467-022-31213-7
Tramontano, A. F., Lyubarova, R., Tsiakos, J., Palaia, T., Deleon, J. R., and Ragolia, L. (2010). Circulating endothelial microparticles in diabetes mellitus. Mediat. Inflamm. 2010, 250476. doi:10.1155/2010/250476
Turek, M., Banasiak, K., Piechota, M., Shanmugam, N., Macias, M., Sliwinska, M. A., et al. (2021). Muscle-derived exophers promote reproductive fitness. EMBO Rep. 22, e52071. doi:10.15252/embr.202052071
Yu, S., and Yu, L. (2022). Migrasome biogenesis and functions. FEBS J. 289, 7246–7254. doi:10.1111/febs.16183
Zhang, X., Yao, L., Meng, Y., Li, B., Yang, Y., and Gao, F. (2023). Migrasome: a new functional extracellular vesicle. Cell Death Discov. 9, 381. doi:10.1038/s41420-023-01673-x
Keywords: mitochondria, secretory autophagy, extracellular vesicles, mitophagosome, membrane fusion, mitophagy, autophagosome formation
Citation: Gong Y, Zhou Y, Feng L and Zhao Y (2024) The roles of secretory autophagy in mitochondria release via extracellular vesicles: waste disposal and food delivery?. Front. Cell Dev. Biol. 12:1392810. doi: 10.3389/fcell.2024.1392810
Received: 28 February 2024; Accepted: 16 April 2024;
Published: 24 May 2024.
Edited by:
Lorena Urbanelli, University of Perugia, ItalyReviewed by:
Chiara Ciardiello, G. Pascale National Cancer Institute Foundation (IRCCS), ItalyCopyright © 2024 Gong, Zhou, Feng and Zhao. This is an open-access article distributed under the terms of the Creative Commons Attribution License (CC BY). The use, distribution or reproduction in other forums is permitted, provided the original author(s) and the copyright owner(s) are credited and that the original publication in this journal is cited, in accordance with accepted academic practice. No use, distribution or reproduction is permitted which does not comply with these terms.
*Correspondence: Yuting Zhao, eXV0aW5nX3poYW9AbndhZnUuZWR1LmNu
†These authors have contributed equally to this work
Disclaimer: All claims expressed in this article are solely those of the authors and do not necessarily represent those of their affiliated organizations, or those of the publisher, the editors and the reviewers. Any product that may be evaluated in this article or claim that may be made by its manufacturer is not guaranteed or endorsed by the publisher.
Research integrity at Frontiers
Learn more about the work of our research integrity team to safeguard the quality of each article we publish.