- 1Department of Molecular Medicine and Therapeutics, The Ohio State University Wexner Medical Center, Columbus, OH, United States
- 2Dorothy M. Davis Heart and Lung Research Institute, The Ohio State University Wexner Medical Center, Columbus, OH, United States
- 3Department of Internal Medicine, Division of Cardiovascular Health and Disease, University of Cincinnati College of Medicine, Cincinnati, OH, United States
RNA binding proteins (RBPs) play a central in the post-transcriptional regulation of gene expression, which can account for up to 50% of all variations in protein expression within a cell. Following their binding to target RNAs, RBPs most typically confer changes in gene expression through modulation of alternative spicing, RNA stabilization/degradation, or ribosome loading/translation rate. All of these post-transcriptional regulatory processes have been shown to play a functional role in pathological cardiac remodeling, and a growing body of evidence is beginning to identify the mechanistic contribution of individual RBPs and their cardiac RNA targets. This review highlights the mechanisms of RBP-dependent post-transcriptional gene regulation in cardiomyocytes and fibroblasts and our current understanding of how RNA binding proteins functionally contribute to pathological cardiac remodeling.
Introduction
An emerging body of literature is establishing a central role for RNA binding proteins (RBPs) in modulating pathological cardiac remodeling (Neumann et al., 2022; Robinson and Port, 2022; Kelaini et al., 2021; D’Antonio, et al., 2022). Approximately 3,000 genes in the human genome code for RNA binding proteins, and the diversity and functional distribution of many of the known RBPs has been previously reviewed in depth (Gerstberger et al., 2014). RBPs execute their regulatory functions by forming ribonucleoprotein complexes (or interactomes) by interacting with RNA in a dynamic and combinatorial manner. These interactomes may also contain RNA modifying enzymes which can influence RNA-protein interactions and ultimately drive RBP-dependent regulation of RNA stability, alternative polyadenylation and splicing, subcellular localization, and translation of mature mRNAs by ribosomes (Park et al., 2011; Gilbert et al., 2016; de Bruin et al., 2017; Hoernes and Erlacher, 2017; Hentze et al., 2018; Cornelius et al., 2022).
The cellular and molecular mechanisms of pathological cardiac remodeling encompass cardiomyocyte hypertrophy and enhanced fibroblast extracellular matrix (ECM) remodeling activity that ultimately lead to functional contractile deficiencies (Mishra and Kass, 2021; Chen and Peng, 2023; Guo et al., 2023). Fibroblasts comprise approximately 10%–20% of the total cell population in a healthy heart, but their proliferation, activation to a myofibroblast state, and increased ECM remodeling drives cardiac fibrosis, which is a ubiquitous hallmark of the failing heart. The activation of quiescent cardiac fibroblasts to pro-fibrotic myofibroblasts can occur in response to multiple stimuli including TGFβ, Wnt, Angiotensin II, or mechanotransduction (DeLeon-Pennell et al., 2020; Gibb Andrew et al., 2020). Regardless of the activating stimulus, cell differentiation to a myofibroblast and the resulting ECM remodeling activity are dependent on large scale transcriptomic and proteomic changes.
RBPs play a critical role in post-transcriptional regulation and the dynamic expression of both the transcriptome and proteome through RNA transcript-specific localization, stability, and translation. For example, it was recently demonstrated that roughly one-third of all TGFβ-dependent gene expression changes in cardiac fibroblasts are subject to translational regulation independent of RNA expression (Chothani et al., 2019). However, transcriptomic and proteomic analyses are rarely done in parallel, which can lead to key post-transcriptional regulatory processes being overlooked. This review will summarize the current knowledge of the field with regard to how RBP-dependent post-transcriptional gene regulation contributes to pathological cardiac remodeling, with a specific focus on their actions in cardiac myoyctes and fibroblasts.
To understand the mechanisms of actions of RBPs, it is essential to also briefly review the post-transcriptional processes they control. These mechanisms, which can be simplified into the three primary categories of alternative splicing, RNA stability/degradation, and translation, have been reviewed in depth elsewhere, but we will provide an overview here with a discussion of how they have been shown to impact pathological cardiac remodeling along with the individual RBPs that utilize these functions to modulate the cardiac transcriptome.
Alternative splicing
Pre-mRNAs are composed of introns and exons which can be included or excluded in a biologically regulated manner from the final mature mRNA sequence leading to multiple alternatively spliced transcripts (Sharp, 2005). Alternative splicing of these transcripts not only contributes to mRNA function and diversity, but also has downstream effects on RBP-dependent binding and functional modulation of the RNA transcript (Kelemen et al., 2013). Alternative splicing, mediated by several different RBPs, including RBM20/24, ASF/SF2, SRSF, CUGBP/CELF, MBNL, and Rbfox family members, has an established role in cardiac development and homeostasis as well as in response to pathological stimuli (Xu et al., 2005; Kalsotra et al., 2008; Kalsotra et al., 2010; Koshelev et al., 2010; Dasgupta and Ladd, 2012; Guo et al., 2012; Linke and Bucker, 2012; Blech-Hermoni and Ladd, 2013; Li et al., 2013; Wei et al., 2015; Gao et al., 2016). The best example of the importance of alternative splicing in cardiac physiology may be in the expression diversity of titin, one of the most abundant proteins in cardiomyocytes and a key component of the contractile sarcomere. Titin exists as a single gene with all expression variation manifesting as a result of alternative splicing, that is responsible for a developmental shift in titin isoform expression from fetal to adult hearts. Alternative splice variants of titin have been shown to confer different mechanical stiffness to the sarcomeres that directly impact contractility, and splicing mutants have been implicated in hereditary cardiomyopathies (LeWinter and Granzier, 2014).
RNA binding motif (RBM) proteins
Dozens of RBM protein family members have been annotated and shown to regulate RNA metabolism through splicing, stability, and translation in multiple tumor types (Li et al., 2010). However, RBM20 and RBM24 have been most studied in the heart as they have been shown to govern the alternative splicing of titin, and deletion of RBM20 results in the exclusive expression of the longer, fetal isoform of titin that impairs cardiac contractility in adult hearts (Guo et al., 2012). Unsurprisingly, mutations in RBM20 have been associated with dilated cardiomyopathies in humans (Brauch et al., 2009; Li et al., 2010). RBM20 has been shown to control the ratio of titin isoforms in rats following exercise, with exercise being associated be associated with an enriched expression ratio of a titin splice variant shown to be more mechanically compliant (Chung et al., 2020).
In addition to titin, RBM20 controls the splicing fates of crucial cardiac genes such as CamkIIδ, and RyR2, such that RBM20 gene mutation causes mis-splicing events of these cardiac genes, resulting in early onset dilated cardiomyopathy (Guo et al., 2012; van den Hoogenhof et al., 2018). The post-transcriptional processes controlled by RBM20 also extend to beyond alternative splicing of target RNA. For instance, RBM20 has been suggested to be at the forefront of ribonucleoprotein (RNP) granule regulation as well. RNP granules are intracellular condensates composed of RNA and protein, held together in a dynamic manner (Shin and Brangwynne, 2017; Schneider et al., 2020). The functional role of RNP granules are mostly unknown as they are akin to membrane-less organelles that comprise high localized RNP concentrations and are still being investigated (Ripin and Parker, 2023). However, RBM20 deficiency leads to dysregulated RNP granules that potentially drives myocardial pathobiology and heart failure (Schneider et al., 2020). A mutation in RBM20 has been identified to have a causal role in cardiac splicing alterations and re-distributes ribonucleoprotein granules within processing bodies (Fenix et al., 2021).
Similarly, RBM24, has also been shown to affect the alternative splicing of titin as well as other z-disc proteins in the sarcomere such as Nebl, Ablim1, and Enah (Liu et al., 2019). Knockdown of RBM24 also decreased the expression of genes encoding components of the contractile machinery and energy production (Liu et al., 2019). Rbm24 has been extensively assessed in zebrafish and adeno-associated virus (AAV9)-mediated RBM24 over the expressing mouse models (Poon et al., 2012; van den Hoogenhof et al., 2018). The findings in the zebrafish model reveal that RBM mutations can lead to sarcomere-related cardiomyopathy via regulation of sarcomere assembly and cardiomyocyte contractility (Poon et al., 2012). Overexpression of RBM24 can have a deleterious effect by inducing cardiac fibrosis, as seen through Rbm24-dependent splicing differences in cardiac genes such as PDZ and Lim domain 5, phospholamban, and Titin. This was accompanied with robust periostin expression, indicating Rbm24 mediates regulation of cardiac fibrosis, potentially via the regulation of TgfβR1 and TgfβR2 expression (van den Hoogenhof et al., 2018).
RNA binding Fox-1 homologs (Rbfox)
The Rbfox proteins, a conserved RBP family of alternative splicing mediators whose expression is enriched in both skeletal and cardiac muscle, has been suggested to play a role in both cardiac development and homeostatic maintenance in adult cardiomyocytes (Gallagher et al., 2011; Cao et al., 2021; Huang et al., 2022; Verma et al., 2022). Wei et al. (2015) reported decreased expression of Rbfox2 in mouse hearts in response to pressure overload (TAC)-induced heart failure and that cardiomyocyte-specific deletion of Rbfox2 is sufficient to induce contractile dysfunction and heart failure. Other groups have suggested that loss of Rbfox2 splicing function is an early pathological mediator in diabetic cardiomyopathy (Nutter et al., 2016). However, the mechanisms for this remain unclear as Rbfox2 has been shown to have potential splicing-independent roles as well and may contribute to pathological cardiac remodeling through transcriptional modulation of miRNAs (Hu et al., 2019).
Gao et al. (2016) also showed decreased cardiac expression of Rbfox1 in failing human hearts as well in mouse model of pressure overload-induced heart failure. They went on to show that Rbfox has a widespread effect on cardiac splice events during pathological progression, but Rbfox-mediated alternative splicing of MEF2, a key transcriptional regulator of hypertrophic gene expression in cardiomyocytes, plays a particularly important role. Importantly, they also showed that restoring Rbfox1 expression specifically in cardiomyocytes ameliorated pressure overload-induced cardiac dysfunction and pathology. Both Rbfox1 and two have also been suggested to contribute to myocyte hypertrophy and progression to heart failure through alternative splicing of the CaV1.2 L-type calcium channels (Wang et al., 2018; Li et al., 2023). Mutations in both Rbfox1 and Rbfox2 have also been linked to cardiac pathology in humans, but continued work is needed to fully understand the regulation of RNA targeting and functional contribution of Rbfox-mediated splice variants (Lale et al., 2011; Verma et al., 2016).
Muscleblind-like 1 (MBNL1)
MBNL1 has been suggested as a master regulator of the transformation of fibroblasts to myofibroblasts, which is a key step for wound healing and fibrotic remodeling, through direct binding and regulating a network of differentiation-specific and cytoskeletal/matrix-assembly transcripts which aids in myofibroblast differentiation (Davis et al., 2015; Stempien-Otero et al., 2016; Bugg et al., 2022). MBNL1 expression in the myocardium increases significantly within 4 days of myocardial infarction or in response to TGFβ-dependent stimulation of fibroblasts in vitro. Functionally, MBNL1 expression in fibroblasts appears to be dependent on their differentiation to myofibroblasts. Further, numerous MBNL1 target transcripts were identified in active myofibroblasts that play a role in several cell signaling pathways. For instance, mutations in MBNL1 lead to selective modulation of its target transcripts Calcineurin Aß, serum response factor (SRF), and p38 which can lead to fibrosis (Davis et al., 2015; Stempien-Otero et al., 2016). Furthermore, fibroblast state plasticity is also determined by MBNL1 as a post-transcriptional process which influences cardiac wound healing (Bugg et al., 2022). The pivotal regulatory position of MBNL1 in the myofibroblast differentiation signaling network makes it an attractive target to novel RNA-based therapeutic strategies, to slow down fibrosis and scarring during disease (Davis et al., 2015).
Additional work is needed to conclusively define the cell-type specific function of MBNL1 in cardiac remodeling, and MBNL1 may play an equally important role in cardiomyocytes. During early developmental stages, MBNL1 is a critical regulator of cardiomyocyte cell cycle entry and proliferation through altered cell cycle inhibitor transcript stability. MBNL1 is suggested to function as a transcriptome-wide switch between regenerative and mature myocyte states postnatally and throughout adulthood (Bailey et al., 2023). Deletion of MBNL1 results in the expression of multiple embryonic splice variants within the cardiac transcriptome, including genes regulating sodium and calcium currents as well as structural components of the sarcomere and cytoskeleton (Dixon et al., 2015). Many of these MBNL1-dependent splice variants, along with MBNL1 itself, have also been implicated in the disease progression of myotonic dystrophy type I (DM1), for which heart disease and sudden cardiac death are prominent causes of mortality (Philips et al., 1998). At the molecular level, DM1 is caused by expansion and expression of CUG repeats that accumulate and sequester MBNL proteins in the nucleus, reducing their native splicing activity (Miller et al., 2000; Fardaei et al., 2002; Mankodi et al., 2005). It was recently demonstrated that cardiomyocyte-specific deletion of both MBNL1 and MBNL2 recapitulates the cardiac pathology of DM1 and results in cardiac hypertrophy, fibrosis, and an increased prevalence of sudden cardiac death (Lee et al., 2022).
CUG-BP and ETR-3-like factors (CELF)
The alternative splicing function of CELF family proteins, of which CUG-BP1 and ETR-3 are the two most highly expressed in the heart, play a critical role in cardiac development. Cardiomyocyte-specific expression of a truncated dominant negative CELF protein was shown to specifically disrupt CELF-dependent splicing and leads to the development of cardiac hypertrophy and fibrosis in young (3–9 week-old) mice when expressed in vivo (Ladd et al., 2005a; Ladd et al., 2005b). However, the same group went on to show that this CELF-dependent cardiomyopathy was spontaneously overcome without intervention as the mice aged, with no remaining deficits in cardiac function detectable at 24 weeks of age in mice with a mild expression of the CELF dominant negative protein (Terenzi et al., 2009). Interestingly, CELF-dependent splice events were still found to be suppressed following functional recovery, suggesting either a threshold of CELF function required for homeostasis in the adult heart or a CELF-independent recovery of cardiac function in the adult heart. Indeed, the expression of CUG-BP1 and ETR-3 are both highest at birth and decrease with age, supporting a strong role for both in cardiac development. However, their functional role has not been investigated in the setting of cardiac pathology, but these results would suggest that they might play a key role in mediating expression of the fetal gene program that is associated with myocyte hypertrophy in the adult heart. The postnatal expression of MBNL and Fox proteins have been shown to increase as CELF protein expression decreases, and this transition in splicing protein expression pattern may regulate more than half of the development splicing pattern during cardiac development (Kalsotra et al., 2008; Gazzara et al., 2017).
Serine/arginine-rich splicing factor (SRSF) proteins
There are 12 SRSF family members (SRSF1-12), are traditional nuclear splicing factors that have also been shown to shuttle to the cytoplasm where thay can also regulate mRNA stability and translation (Twyffells et al., 2011). Deletion of SRSF1/2 or 10 disrupted postnatal and embryonic cardiac remodeling, in part through a dysregulation of contractility mediated by alternative splicing of CamKII and triadin, respectively, suggesting a role for these RBPs in cardiac development (Ding et al., 2004; Xu et al., 2005; Feng et al., 2009). In the adult heart, SRSF3 expression was shown to be reduced following ischemic injury, and its deletion in cardiomyocytes induces rapid (within 8 days) systolic failure and death that was contributed to alterations in mTOR splicing and subsequent mRNA decapping and reduced translation of contractile and calcium handling genes (Ortiz-Sanchez et al., 2019).
Alternative splicing as a posttranscriptional mechanism regulated by RBPs in fibroblasts is not limited to pathology, but also plays a role in the reprogramming of cells in the adult heart based on environmental cues. The involvement of RBPs in various cell lineages and pathways of cardiac reprogramming have also been studied using single cell genomics. These studies delineate that the downregulation of the RBP polypyrimidine tract binding protein (PTBP) allows cell transitions between cardiac fibroblasts (CFs) to induced cardiomyocytes (iCMs) (Liu et al., 2017). Accordingly, PTBP has been shown to play a role in cardiac development by its expression in both cardiomyocytes and endothelial cells through regulating the translation of apoptotic genes (Apaf-1 and Caspase-3) and splicing of β-arrestin-1, respectively (Zhang et al., 2009; Liu et al., 2023).
Additional RBPs are suggested to modulate cardiac physiology through splicing regulation, but with less understood about their mechanisms or targets in the heart. For example, RNA binding protein with multiple splicing (RBPMS), which significantly impacts myofibrillar organization and calcium handling through alternative splicing of regulators such as titin, Pdlim5 and nexilin (Akerberg et al., 2022; Gan et al., 2022). Similarly, the Quaking (QKI) family of RBPs function as alternative splicing factors of sarcomere and cytoskeletal component genes, calcium-handling genes, and post-transcriptional regulators (Kelaini et al., 2021; Cornelius et al., 2022). QKI isoforms themselves are products of alternative splicing with roles in angiogenesis, cell migration and adhesion senescence (Montañés-Agudo et al., 2023).
RBPs involved in mRNA processing and alternative splicing can also act as a molecular roadblock in generating the post-transcriptional splicing patterns needed to reprogram mouse fibroblasts into induced cardiomyocytes (Liu et al., 2017; Ricketts and Qian, 2022). Most investigations focus extensively on gene regulatory networks determining fibroblast transcriptomic fates, while overlooking the post-transcriptional regulation that controls the proteomic landscape. RBPs are at the forefront of the post-transcriptional regulation and have the potential to serve as a therapeutic target as their modulation can trigger changes in several genes in any cell type and disease model (Bretherton et al., 2020).
In addition to alternative splicing, eukaryotic RNA can also be regulated through alternative polyadenylation (APA) which can generate multiple variants of the same RNA with distinct 3′UTR sequence lengths (Tian and Manley, 2017). APA is highly prevalent in that 50%–70% of transcripts encoded by human genes are estimated to contain multiple alternative poly (A) sites in their 3′UTR (Tian et al., 2005; Derti et al., 2012). APA sites generate multiple mRNA transcripts, thereby altering mRNA coding potential; changing availability of RBP and microRNA binding sites, and thus determining mRNA fates (Di Giammartino et al., 2011). RBP mediated regulation of APA can occur by either directly constituting the cleavage and polyadenylation machinery or inhibiting the APA process or binding through adjacent region of the target poly (A) sites (Zheng and Tian, 2014).
The specific impact of APA on heart development and its dysregulation in cardiac pathology has been reviewed elsewhere (Cao and Kuyumcu-Martinez, 2023). mRNA isoform and microarray analyses of hypertrophic mouse hearts reveal genome-wide APA changes, wherein more than half of the 315 tandem APA events led to 3′UTR shortening. This was accompanied by embryonic-like APA patterns in the hypertrophic hearts, considering fetal gene expression is a hallmark of cardiac hypertrophy (Park et al., 2011; Cao and Kuyumcu-Martinez, 2023). Interestingly, most cardiac RBPs that are actively involved in alternative splicing, also display function as APA regulators. For instance, importance of MBNL1 in APA has been demonstrated through HITS-CLIP and minigene reporter analyses, and the loss of MBNL in mouse embryonic fibroblasts has been shown to dysregulate a lot of AP events (Batra et al., 2014; Davis et al., 2015). Many other important RBPs such as human antigen R (HuR) and PTBP, have also been implicated for their APA modulating activities on their target mRNA transcripts. HuR and PTB regulate cardioprotective genes like heat shock protein (HSP70.3) and cyclooxygenase (COX-2), respectively by interacting with the upstream sequence elements in the 3′-UTR that control the efficiency of polyadenylation (Tranter et al., 2011; Kraynik et al., 2015; Hall-Pogar et al., 2007).
RNA stability/degradation
Another key step modulated predominately by RBPs is control of mRNA stability, which directly determines the half-life of individual transcripts within the cells and thus impacts both the availability and capacity for translation of mRNAs, but also the function of other RNAs, such as circular or long non-coding RNAs (lncRNAs). lncRNA have themselves emerged as key modulators of mRNA stability, which can act either independently or synergistically with RBPs (Sebastian-delaCruz et al., 2021). mRNA stability is broadly determined by the combined actions of specific sequences within the RNA itself (cis-acting elements), typically within the 3′-untranslated region (UTR), and the interacting RBPs (trans-acting factors) that drive the stabilizing and destabilizing actions. These interactions occur in a cell type and disease dependent manner and are dependent on the expression and activation profile of RBPs as well as the transcriptome of available binding targets (Wu and Brewer, 2012). For instance, in the muscles of heart and other tissues, expression of sarcoplasmic reticulum calcium-ATPase 2 (SERCA2a) is influenced by RBPs that can have a destabilizing (e.g., AUF1) or stabilizing effect (e.g., HuR) on the mRNA stability, and even small changes in SERCA can result in direct functional effects on Ca2+ and ß-adrenergic signaling within contractile cells (Misquitta et al., 2006). Furthermore, the posttranscriptional regulation of G protein-coupled β adrenergic receptors (β-AR) in cardiomyocytes was also demonstrated to occur at the level of mRNA stability (Eberhardt et al., 2007). In addition, mRNA stability can also be heavily impacted by the degrading actions of miRNAs, such as miR-21, which has shown to be a major effector of cardiac fibrosis (Thum et al., 2008; Piccoli et al., 2016).
Many RBPs, such as ARE/poly(U)-binding/degradation factor 1 (AUF) and Human antigen R (HuR) have been demonstrated to regulate mRNA stability and degradation through specific binding to AU-rich elements (AREs), which are most typically found in the 3′untranslated region (3′UTR) of mRNAs (Gingerich et al., 2004). These AREs often form short RNA hairpin loops that facilitate RBP recognition through both a sequence and structural manner. AUF1 is a prominent example of an RBP that operates through ARE binding and subsequent recruitment of members of the mRNA degradation machinery. HuR on the other hand tends to promote stability of target transcripts following ARE recognition and binding (Eberhardt et al., 2007; Schultz et al., 2020). The balance of this regulation is dependent on expression, localization, and post-translational regulation of individual RBPs as well as the expression and relative stoichiometry of potentially available ARE-containing RNA targets. Additionally, RBPs may compete with RNA-RNA driven regulation of target transcripts, such as those mediated by lncRNA or miRNA (Engreitz et al., 2014; Xue, 2022). To this end, both HuR and AUF1 have been shown to compete with miRNA binding for mRNA target recognition and have even been shown to modulate the expression of some miRNAs (Srikantan et al., 2012). Much of this is governed by the pathophysiological state of the tissue and cell types, and while both HuR and AUF1 have been shown to play a role in the heart (discussed in detail in subsequent sections), the details of how these processes play out across distinct cell populations of the myocardium to mediate pathological cardiac remodeling remains poorly understood.
Human antigen R (HuR)
HuR is a nearly ubiquitously expressed RNA binding protein whose cardiac expression was first shown to be increased at 3 days post-MI (Krishnamurthy et al., 2010). Inhibition of HuR in the post-ischemic heart was subsequently shown to reduce pathological remodeling through suppression of inflammatory signaling, with a potential direct regulation of TGFβ and p53 mRNA stability (Krishnamurthy et al., 2010; Slone et al., 2023).
Our lab has shown that HuR is both necessary and sufficient for hypertrophic growth of cardiac myocytes (Slone et al., 2016). We have also shown that HuR expression and cytoplasmic translocation, an indicator of RNA binding activity, is increased in failing human hearts and that both cardiomyocyte-specific ablation or pharmacological inhibition of HuR reduces the progression of cardiac hypertrophy and fibrosis in a pressure overload (transverse aortic constriction; TAC) model of heart failure (Green et al., 2019). HuR has also been suggested to regulate the RNA stability of sodium channel (SCN5A) and calcium cycling modulator (phospholamban) genes during cardiac remodeling (Zhou et al., 2018; Hu et al., 2020).
In addition to its role in cardiomyocytes, we found HuR to be highly expressed in cardiac fibroblasts, and necessary for their pro-fibrotic response and phenotypic transformation to myofibroblasts (Green et al., 2023). Interestingly, our previous work in cardiomyocytes also identified a HuR-dependent regulation of TGFβ as well as secreted pro-inflammatory cytokines, suggesting that HuR may also orchestrate myoycte-centric signaling to other cardiac cell types (Green et al., 2019; Slone et al., 2023). To this end, Govindappa et al. (2020) showed that HuR-dependent extracellular vesicle (EV) signaling from macrophages may mediate fibroblast activity and fibrosis in diabetes associated cardiac remodeling. These results are consistent with other work showing HuR-dependent regulation of endocrine signaling from other cell types, including the modulation of extracellular vesicle secretion (Deng et al., 2009; Fabbiano, et al., 2020; Mukherjee, et al., 2016; Shi, et al., 2020; Deng, X., et al., 2020), and our additional work showing an adipose tissue-derived HuR-dependent endocrine impact on cardiac remodeling (Guarnieri et al., 2021).
ARE/polyU binding/degradation factor1 (AUF1)
AUF1, as the name suggests, is an RNA binding protein that binds to AU rich or poly U regions of mRNA and selects that RNA for rapid degradation. AUF1 itself does not have the ability to degrade the RNA, but the binding of AUF1 coordinates the recruitment and complex assembly of other trans-acting proteins to form the RNA degradation machinery. AUF1 expression was shown to be increased in failing human hearts, likely downstream of β-adrenergic receptor (β-AR) signaling (Pende et al., 1996). They also showed that AUF1 binds to the β1-AR mRNA and postulate that AUF1 contributes to the downregulation of β1-AR mRNA in cardiomyocytes observed in failing hearts (Pende et al., 1996).
AUF1 expression was shown to increase in response to angiotensin II (AngII) in cardiomyocytes to mediate the hypertrophic downregulation of myocyte voltage-gated Kv4 potassium channels (Zhou et al., 2008). They identified a specific AU-rich element in the 3′UTR of Kv4.3 that mediates AngII-dependent transcript destabilization, but showed that AngII-mediated binding of AUF1 had no effect on HuR to the Kv4.3 transcript (Zhou et al., 2008). AUF1 and HuR have been demonstrated to concurrently bind target mRNAs, with similar AU-rich target sequences, in a competitive and non-competitive manner (Lal et al., 2004).
AUF1 was also shown to bind to the 3′UTR of SERCA2a downstream of PKC activation in neonatal rat ventricular myocytes (Blum et al., 2005). Cardiac expression of SERCA2a is the result of alternative splicing that yields a differential exon inclusion and 3′UTR sequence between the SERCA2a isoform expressed in the heart and the more ubiquitously expressed SERCA2b isoform. The differential 3′UTR sequences between the two isoforms yields a difference in transcript stability that may contribute to the loss of SERCA2a expression in failing hearts, which exacerbates contractile dysfunction and contributes to pathological progression (Misquitta et al., 2002; Misquitta et al., 2005; Zhihao et al., 2020). Interestingly, the SERCA2a 3′UTR has five AU rich regions to which AUF1 and HuR are both predicted to bind, but Blum et al. were unable to detect HuR binding to SERCA2a in NRVMs (Blum et al., 2005).
However, both AUF1 and HuR have both been shown to exert post-transcriptional regulation via multiple regulatory mechanisms, including nuclear export, splicing, and translational control (Fan and Steitz, 1998; Doller et al., 2008; Izquierdo, 2008; Chang et al., 2014; Panda et al., 2014; Yoon et al., 2014). Similarly, HuR has been shown to act in concert with AUF1 to promote RNA destabilization (Chang et al., 2010). Another example of this was demonstrated in AUF1 and HuR independent regulation of Nrf2 expression, with HuR promoting nuclear export and AUF1 promoting RNA stability (Poganik et al., 2019). Nrf2 is a critical transcriptional mediator of cardiac I/R injury and response to oxidative stress (Chen and Maltagliati, 2018; Zang et al., 2020), but the potential significance of Nrf2 regulation by AUF1 or HuR has not yet been demonstrated in the failing heart. Similarly, AUF1 has been shown to regulate the expression of MEF2c, a key transcriptional regulator of cardiac hypertrophic signaling (Panda et al., 2014), but the importance of this interaction in pathological cardiac remodeling is unknown.
In addition to these tantalizing bits of data suggesting a prominent role for AUF1 in cardiomyocyte pathophysiology, there are also reports of AUF1 regulation of proliferation, migration, and cell senescence in skin and breast cancer associated fibroblasts that have gone unrealized in the cardiac field (Hendrayani et al., 2014; Wallis et al., 2015; Hendrayani et al., 2016; Yu et al., 2022).
Brain expressed X-linked protein 1 (Bex1)
Bex1 was identified to be increased in failing hearts where it associates with AU-rich mRNA targets, including many inflammatory gene products, as part of a larger ribonucleoprotein complex (Accornero et al., 2017). Cardiomyocyte-specific overexpression of Bex1 replicated a hypertrophic cardiomyopathy phenotype, while whole body deletion of Bex1 was protective against pressure overload-induced remodeling. Bex1 may play a more global role in muscle cell biology as the first report of Bex1-deficient mice showed an impairment in exercise performance and skeletal muscle regeneration (Koo et al., 2007). Interestingly, Bex1 may also bind to double stranded RNA and confer an antiviral role. Martens et al. recently showed that Bex1 plays a protective role against viral myocarditis and limits viral replication in both cardiomyocytes and fibroblasts (Martens et al., 2022).
Additional RBPs have been suggested to play a role in pathological remodeling through modulation of RNA stability, with much less known about the extent of their mechanisms or functional importance. For example, fused in sarcoma (FUS) is a ubiquitous and versatile protein involved in several cellular processes like DNA repair, gene transcription, oxidative stress, mitochondrial damage and cell apoptosis (Deng et al., 2015; Suzuki and Matsuoka, 2015; Bozzo et al., 2017; Singatulina et al., 2019; Birsa et al., 2020), and has been implicated in regulation of cardiomyocyte apoptosis in myocardial infarction models (Wu et al., 2018). More recently, FUS expression was shown to be induced downstream of AngII in cardiac fibroblasts and play a role in myofibroblast activity, but the mechanism or RNA targets of FUS remain largely unknown (Wang et al., 2021). More recently, PTBP was shown to also mediate cardiac fibrosis by promoting fibroblast proliferation and collagen deposition (Chen et al., 2023). This work also suggested a PTBP-dependent reduction in stability of the transcriptional regulator Nur77, highlighting the fact that many RBPs may act through multiple mechanisms as PTBP has already been mentioned mediate post-transcriptional regulation via splicing, translation, and alternative polyadenylation.
Translational control
Gene expression resulting from transcriptional regulation is the most widely studied as a fundamental phenomenon, but it is essential to acknowledge the contribution of translational regulation as an equally important mediator of protein expression. The translational regulation of cardiac gene expressions plays a key role in determining heart function and disease, as it is influenced by natural genetic variation, which could lead to inefficient translation termination. Findings from genome-wide RNA sequencing and ribosome profiling have shown that a large number of cardiac genes carry distinct signatures in 3′UTR variation, RNA-binding protein motifs and miRNA expression which are associated with translational regulation of gene expression (Schafer et al., 2015). RBPs commonly mediate RNA regulation at the translational level either to promote activation or, more commonly, repression of the initiation of translation (Abaza and Gebauer, 2008). The mechanism of action of RBPs on translation initiation occurs through competing with ribosome binding, modification of the RNA structure to prevent its ribosomal recognition, or via direct RBP complex-mediated protein-protein interaction with the ribosomes themselves (Babitzke et al., 2009). One of the major RBP families known to regulate translation is the CUG-BP, Elav-like family (CELF) proteins. RBPs like CELF have dual roles to play during RNA processing events in development and during disease pathogenesis either as a cause or consequence. However, CELF proteins have versatile mechanisms of action; in the nucleus, they can mediate alternative splicing through exon exclusion, and in the cytoplasm, they can also bind to the 3′ UTR of target mRNA and control the translation of stability (Brinegar and Cooper, 2016).
The multifunctional role of RBPs was recently highlighted in a comprehensive ribo-seq and RNAseq profiling of human hearts that identified 21 RBPs which control both mRNA abundance and translational efficiency (Schneider-Lunitz et al., 2021). These RBPs were further analyzed using published eCLIP and HITS-CLIP data, and G3BP1, PUM1, DDX3X, DDX6, and ELF proteins were identified for their translational regulation role in the human left ventricle (Chothani et al., 2019; Luo et al., 2020; Schneider-Lunitz et al., 2021). These new results merit further investigation of translational control by RBPs as a key mediator of cardiac homeostasis and pathological remodeling.
Novel roles of RBPs in HF
Our review of the mechanism of actions of RBPs that play a role in HF is mainly centered upon the most established mRNA modulatory actions like alternative splicing, mRNA degradation and translation. But newer investigations have shed light on the diverseness in their functionality in cardiovascular pathologies that extends beyond these mechanisms. As mentioned earlier RBPs can operate independently or in tandem with miRNAs, lncRNAs or circular RNAs and facilitate their actions to manifest effects on the heart. It’s also possible that RBPs may contribute to the generation of novel microproteins via stabilization, localization, or translational regulation of short ORFs identified within lncRNAs and circRNAs (Makarewich and Olson, 2017; van Heesch et al., 2019).
Studies conducted over the last decade have demonstrated that the regulatory effects of RBPs extend to beyond linear RNA transcripts, as RBPs have also been implicated in the biogenesis, expression and transport of circRNAs (Li et al., 2018). Most studies to date have explored the actions of circRNA as RBP-sequestrants or sponges and present evidence of competitive interaction between linear and circular RNA for regulatory RNA (e.g., miRNA) and RBP binding. Future work certainly needs to be aimed at increasing our understanding of circRNA-mediated mechanisms and determine whether specific targeting of circRNA-RBP complexes may modulate the initiation or progression of cardiac hypertrophy and fibrosis.
RBPs have also been shown to aid in the regulatory activities of mitochondrial non-coding RNA (ncRNA) and miRNAs also known as mitomiRs, by controlling their translocation from nucleus to mitochondria, by employing translocase-based sorting and assembly machinery (Wiedemann and Pfanner, 2017). RBPs execute this by associating themselves with a cytoplasmic multiprotein RNA-induced silencing complex (RISC), that consists of RBPs including protein kinase RNA activator, transactivation response RNA-binding protein (TRBP) and Dicer, which process pre-microRNAs into mature microRNAs. This overall mitochondrial regulation mediated by RISC has implications in HF owing to the energy dependence of cardiomyocytes on mitochondrial ATP (Jusic et al., 2020). Moreover, RBP contribution has also been documented in the active binding and transport of circulating cardiac miRNAs released by cardiac fibroblasts to be packaged into EVs, which in turn act as paracrine signaling mediators of cardiomyocyte hypertrophy (Tian et al., 2021).
In addition to transcriptional regulation, mRNA processing, and alternative processing, a cell can attain molecular complexity through post-transcriptional modification of RNA bases and gene editing. Through this cellular process RNA nucleotides in cell transcripts get altered in their sequence compared to the parent DNA they transcribed from (Gott and Emeson, 2000; Washburn and Hundley, 2016). The most well studied type of gene type of gene editing is the adenosine to inosine (A-I) editing in the dsRNA substrates, catalyzed by adenosine deaminase acting on RNA (ADAR) enzymes (Nishikura, 2010). The specific significance of A-I editing in growth and development, health and disease, various physiological processes, and relationship with RBPs has been reviewed elsewhere (Washburn and Hundley, 2016; Quinones-Valdez, 2019). ADARs are synonymous with RBPs and are either defined as a type of RBPs or known to act like RBPs, but additional RBPs influence ADAR-mediated gene editing by modifying certain RBP-binding sites to enhance editing or by altering the availability of dsRNA to suppress editing (Deffit, S. N., & Hundley, 2016). In the human heart, A-I editing is the predominant mechanism of gene editing, which is severely reduced during HF, accompanied by repressed ADAR2 expression and increased circRNA levels. Moreover, the potential importance of ADAR1 to cardiac remodeling was shown through augmented ventricular remodeling, cardiac dysfunction, unfolded protein response, and reduced miRNA expression following cardiomyocyte specific deletion of ADAR1 in adult mice (El Azzouzi et al., 2020).
RBPs also influence distinct post-transcriptional RNA modifications such as the N6-methyladenosine (m6A) methylation of mRNAs, rRNAs, tRNAs, long non-coding RNAs and microRNAs, that are vital for RNA splicing, transport, stability, and translation at the post-transcriptional level. Interestingly, cardiac hypertrophy and HF display aberrations in the m6A methylation of transcripts, thereby they can act as significant targets for deriving therapeutic strategies for managing CVD. This has also been further emphasized through the control of cardiac homeostasis and hypertrophy by The N6-methyladenosine mRNA methylase (METTL3) (Dorn et al., 2019). The cardiomyocyte RNA interactome in failing hearts was shown to contain 29 RBPs annotated for modifications like 5-methylcytosine, N6-methyladenosine and pseudouridine modifications, and adenosine-to-inosine editing (Liao et al., 2016; Hentze et al., 2018). m6A has been implicated to be an important target as repressed m6A levels have shown to attenuate fibrosis via altered RNA splicing, translation and degradation (Mathiyalagan et al., 2019; Chen et al., 2021).
Some examples of RBPs that recognize m6A-modified mRNA are YT521-B homology (YTH), heterogenous nuclear ribonucleoprotein A2/B1 (HNRNPA2B1) and insulin-like growth factor 2 mRNA-binding protein (IGF2BP) domain (Qin et al., 2020; Fan and Hu, 2022). Heterogenous nuclear ribonucleoprotein A2/B1 (HNRNPA2B1) is also suggested to regulate cardiac homeostasis and hypertrophy via N6 methyladenosine mRNA methylase (METTL3) and m6A switch recognition and alternative splicing events (Qin et al., 2020; Fan and Hu, 2022). RBPs also contribute to the regulation of RNA m5C methylation, dysregulation of which is closely associated with CVD (Balachander et al., 2023).
Conclusion
When cells of the myocardium are stressed or injured, they often initiate a functional remodeling of their transcriptome in order to minimize tissue damage and maintain cardiac structure and function. RBPs play a critical post-transcriptional regulatory role in this transcriptomic remodeling and their expression and activity are tightly coupled with dynamic changes in gene expression patterns that drive the pathophysiological response in cardiac remodeling (Table 1). As our understanding of the human genome has developed significantly, we have gained an increasingly comprehensive understanding of both protein-coding RNA transcripts and non-protein-coding RNA transcripts, as well as the RBPs that interact with them and coordinate their splicing, stability, and translation.
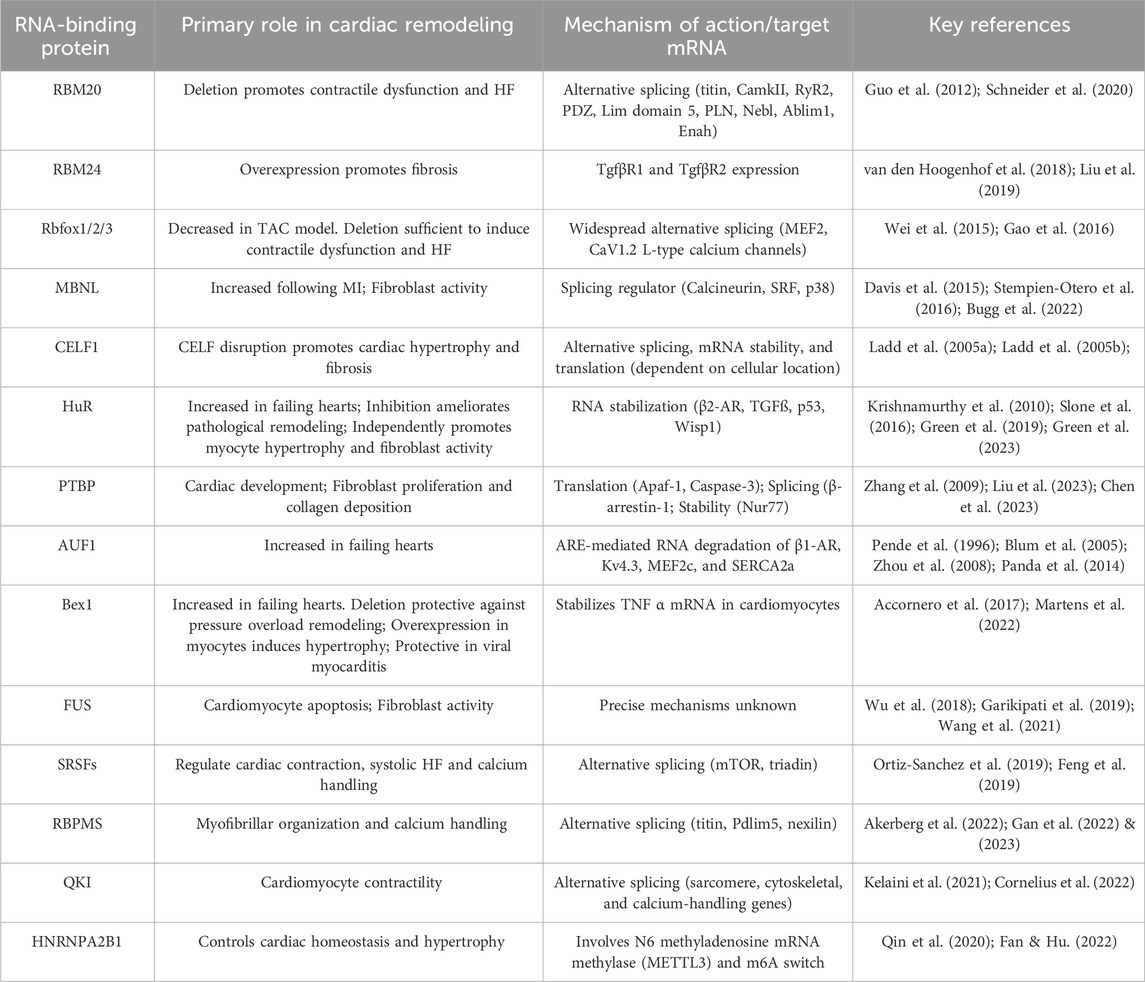
Table 1. An overview of the major cardiac RBPs that have been demonstrated to regulate pathological cardiac remodeling.
The interaction of RBPs with target mRNAs are often regulated by post-translational modifications, cofactor binding and protein-protein interactions. RBPs have historically been considered to be an undruggable class of proteins due to their high number of potential RNA targets, relatively weak protein-RNA binding interactions, and the resulting difficulties in targeting specific RBP-target RNA interactions. However, there have been recent developments in RNA-based therapeutics that act in an inhibitory manner by sponging specific RNA-binding proteins and reducing their binding and regulation of endogenous target RNAs (Schreiner et al., 2020; Wang and Liu, 2020). For example, AAV9-mediated expression of the circRNA circFndc3b was found to reduce pathological cardiac remodeling in a mouse myocardial infarction model, and the proposed mechanism is via circRNA binding and sponging of the RBP FUS to reduce FUS binding to endogenous targets (Garikipati et al., 2019). Similar approaches to target specific miRNAs via regRNAs, lncRNAs and circRNAs sponges have also been studied in oncology models, but the application of these approaches to the heart are still in very early stages (Zhang et al., 2019; Gomes et al., 2020; Jiang et al., 2021). As our mechanistic understanding of RBP-RNA interactions and their functional consequences to cardiac physiology, so too should the optimism that RBP-based therapeutics may hold promise as novel approaches to pathological cardiac remodeling.
Author contributions
PA: Conceptualization, Writing–original draft, Writing–review and editing. SP: Conceptualization, Writing–original draft, Writing–review and editing. MT: Conceptualization, Writing–original draft, Writing–review and editing.
Funding
The author(s) declare that financial support was received for the research, authorship, and/or publication of this article. This work was funded by NIH grants R01-HL158671 and R01-HL166326 (MT). SP is funded by NIH F31-HL170636.
Conflict of interest
The authors declare that the research was conducted in the absence of any commercial or financial relationships that could be construed as a potential conflict of interest.
Publisher’s note
All claims expressed in this article are solely those of the authors and do not necessarily represent those of their affiliated organizations, or those of the publisher, the editors and the reviewers. Any product that may be evaluated in this article, or claim that may be made by its manufacturer, is not guaranteed or endorsed by the publisher.
References
Abaza, I., and Gebauer, F. (2008). Trading translation with RNA-binding proteins. Rna 14 (3), 404–409. doi:10.1261/rna.848208
Accornero, F., Schips, T. G., Petrosino, J. M., Gu, S. Q., Kanisicak, O., Van Berlo, J. H., et al. (2017). BEX1 is an RNA-dependent mediator of cardiomyopathy. Nat. Commun. 8 (1), 1875. doi:10.1038/s41467-017-02005-1
Akerberg, A. A., Trembley, M., Butty, V., Schwertner, A., Zhao, L., Beerens, M., et al. (2022). RBPMS2 is a conserved regulator of alternative splicing that promotes myofibrillar organization and optimal calcium handling in cardiomyocytes. bioRxiv, 2021–2103. doi:10.1161/CIRCRESAHA.122.321728
Babitzke, P., Baker, C. S., and Romeo, T. (2009). Regulation of translation initiation by RNA binding proteins. Annu. Rev. Microbiol. 63, 27–44. doi:10.1146/annurev.micro.091208.073514
Bailey, L. R., Bugg, D., Reichardt, I. M., Ortaç, C. D., Gunaje, J., Johnson, R., et al. (2023). MBNL1 regulates programmed postnatal switching between regenerative and differentiated cardiac states. bioRxiv. doi:10.1101/2023.03.16.532974
Balachander, K., Priyadharsini, J. V., Roy, A., and Paramasivam, A. (2023). Emerging role of RNA m5C modification in cardiovascular diseases. J. Cardiovasc. Transl. Res. 16 (3), 598–605. doi:10.1007/s12265-022-10336-8
Batra, R., Charizanis, K., Manchanda, M., Mohan, A., Li, M., Finn, D. J., et al. (2014). Loss of MBNL leads to disruption of developmentally regulated alternative polyadenylation in RNA-mediated disease. Mol. Cell 56 (2), 311–322. doi:10.1016/j.molcel.2014.08.027
Birsa, N., Bentham, M. P., and Fratta, P. (2020). Cytoplasmic functions of TDP-43 and FUS and their role in ALS. Seminars Cell & Dev. Biol. 99, 193–201. Academic Press. doi:10.1016/j.semcdb.2019.05.023
Blech-Hermoni, Y., and Ladd, A. N. (2013). RNA binding proteins in the regulation of heart development. Int. J. Biochem. Cell Biol. 45 (11), 2467–2478. doi:10.1016/j.biocel.2013.08.008
Blum, J. L., Samarel, A. M., and Mestril, R. (2005). Phosphorylation and binding of AUF1 to the 3′-untranslated region of cardiomyocyte SERCA2a mRNA. Am. J. Physiology-Heart Circulatory Physiology 289 (6), H2543–H2550. doi:10.1152/ajpheart.00545.2005
Bozzo, F., Mirra, A., and Carrì, M. T. (2017). Oxidative stress and mitochondrial damage in the pathogenesis of ALS: new perspectives. Neurosci. Lett. 636, 3–8. doi:10.1016/j.neulet.2016.04.065
Brauch, K. M., Karst, M. L., Herron, K. J., de Andrade, M., Pellikka, P. A., Rodeheffer, R. J., et al. (2009). Mutations in ribonucleic acid binding protein gene cause familial dilated cardiomyopathy. J. Am. Coll. Cardiol. 54 (10), 930–941. doi:10.1016/j.jacc.2009.05.038
Bretherton, R., Bugg, D., Olszewski, E., and Davis, J. (2020). Regulators of cardiac fibroblast cell state. Matrix Biol. 91, 117–135. doi:10.1016/j.matbio.2020.04.002
Brinegar, A. E., and Cooper, T. A. (2016). Roles for RNA-binding proteins in development and disease. Brain Res. 1647, 1–8. doi:10.1016/j.brainres.2016.02.050
Bugg, D., Bailey, L. R., Bretherton, R. C., Beach, K. E., Reichardt, I. M., Robeson, K. Z., et al. (2022). MBNL1 drives dynamic transitions between fibroblasts and myofibroblasts in cardiac wound healing. Cell Stem Cell 29 (3), 419–433.e10. doi:10.1016/j.stem.2022.01.012
Cao, J., and Kuyumcu-Martinez, M. N. (2023). Alternative polyadenylation regulation in cardiac development and cardiovascular disease. Cardiovasc. Res. 119 (6), 1324–1335. doi:10.1093/cvr/cvad014
Cao, J., Verma, S. K., Jaworski, E., Mohan, S., Nagasawa, C. K., Rayavara, K., et al. (2021). RBFOX2 is critical for maintaining alternative polyadenylation patterns and mitochondrial health in rat myoblasts. Cell Rep. 37 (5), 109910. doi:10.1016/j.celrep.2021.109910
Chang, N., Yi, J., Guo, G., Liu, X., Shang, Y., Tong, T., et al. (2010). HuR uses AUF1 as a cofactor to promote p16INK4 mRNA decay. Mol. Cell. Biol. 30 (15), 3875–3886. doi:10.1128/MCB.00169-10
Chang, S. H., Elemento, O., Zhang, J., Zhuang, Z. W., Simons, M., and Hla, T. (2014). ELAVL1 regulates alternative splicing of eIF4E transporter to promote postnatal angiogenesis. Proc. Natl. Acad. Sci. 111 (51), 18309–18314. doi:10.1073/pnas.1412172111
Chen, Q. M., and Maltagliati, A. J. (2018). Nrf2 at the heart of oxidative stress and cardiac protection. Physiol. genomics 50 (2), 77–97. doi:10.1152/physiolgenomics.00041.2017
Chen, Y., and Peng, D. (2023). New insights into the molecular mechanisms of SGLT2 inhibitors on ventricular remodeling. Int. Immunopharmacol. 118, 110072. doi:10.1016/j.intimp.2023.110072
Chen, Y. S., Ouyang, X. P., Yu, X. H., Novák, P., Zhou, L., He, P. P., et al. (2021). N6-adenosine methylation (m 6 A) RNA modification: an emerging role in cardiovascular diseases. J. Cardiovasc. Transl. Res. 14, 857–872. doi:10.1007/s12265-021-10108-w
Chen, Z., He, C., Gao, Z., Li, Y., He, Q., Wang, Y., et al. (2023). Polypyrimidine tract binding protein 1 exacerbates cardiac fibrosis by regulating fatty acid-binding protein 5. Esc. Heart Fail. 10 (3), 1677–1688. doi:10.1002/ehf2.14318
Chothani, S., Schäfer, S., Adami, E., Viswanathan, S., Widjaja, A. A., Langley, S. R., et al. (2019). Widespread translational control of fibrosis in the human heart by RNA-binding proteins. Circulation 140 (11), 937–951. doi:10.1161/CIRCULATIONAHA.119.039596
Chung, C. S., Hiske, M. A., Chadha, A., and Mueller, P. J. (2020). Compliant titin isoform content is reduced in left ventricles of sedentary versus active rats. Front. Physiology 11, 15. doi:10.3389/fphys.2020.00015
Cornelius, V. A., Naderi-Meshkin, H., Kelaini, S., and Margariti, A. (2022). RNA-binding proteins: emerging therapeutics for vascular dysfunction. Cells 11 (16), 2494. doi:10.3390/cells11162494
D’Antonio, M., Nguyen, J. P., Arthur, T. D., Matsui, H., Donovan, M. K., D’Antonio-Chronowska, A., et al. (2022). In heart failure reactivation of RNA-binding proteins is associated with the expression of 1,523 fetal-specific isoforms. PLoS Comput. Biol. 18 (2), e1009918. doi:10.1371/journal.pcbi.1009918
Dasgupta, T., and Ladd, A. N. (2012). The importance of CELF control: molecular and biological roles of the CUG-BP, Elav-like family of RNA-binding proteins. Wiley Interdiscip. Rev. RNA 3 (1), 104–121. doi:10.1002/wrna.107
Davis, J., Salomonis, N., Ghearing, N., Lin, S. C. J., Kwong, J. Q., Mohan, A., et al. (2015). MBNL1-mediated regulation of differentiation RNAs promotes myofibroblast transformation and the fibrotic response. Nat. Commun. 6 (1), 10084. doi:10.1038/ncomms10084
de Bruin, R. G., Rabelink, T. J., van Zonneveld, A. J., and van der Veer, E. P. (2017). Emerging roles for RNA-binding proteins as effectors and regulators of cardiovascular disease. Eur. heart J. 38 (18), 1380–1388. doi:10.1093/eurheartj/ehw567
Deffit, S. N., and Hundley, H. A. (2016). To edit or not to edit: regulation of ADAR editing specificity and efficiency. Wiley Interdiscip. Rev. RNA 7 (1), 113–127. doi:10.1002/wrna.1319
DeLeon-Pennell, K. Y., Barker, T. H., and Lindsey, M. L. (2020). Fibroblasts: the arbiters of extracellular matrix remodeling. Matrix Biol. 91, 1–7. doi:10.1016/j.matbio.2020.05.006
Deng, J., Yang, M., Chen, Y., Chen, X., Liu, J., Sun, S., et al. (2015). FUS interacts with HSP60 to promote mitochondrial damage. PLoS Genet. 11 (9), e1005357. doi:10.1371/journal.pgen.1005357
Deng, Z. B., Poliakov, A., Hardy, R. W., Clements, R., Liu, C., Liu, Y., et al. (2009). Adipose tissue exosome-like vesicles mediate activation of macrophage-induced insulin resistance. Diabetes 58 (11), 2498–2505. doi:10.2337/db09-0216
Derti, A., Garrett-Engele, P., MacIsaac, K. D., Stevens, R. C., Sriram, S., Chen, R., et al. (2012). A quantitative atlas of polyadenylation in five mammals. Genome Res. 22 (6), 1173–1183. doi:10.1101/gr.132563.111
Di Giammartino, D. C., Nishida, K., and Manley, J. L. (2011). Mechanisms and consequences of alternative polyadenylation. Mol. Cell 43 (6), 853–866. doi:10.1016/j.molcel.2011.08.017
Ding, J. H., Xu, X., Yang, D., Pao-Hsien, C., Dalton, N. D., Ye, Z., et al. (2004). Dilated cardiomyopathy caused by tissue-specific ablation of SC35 in the heart. EMBO J. 23 (4), 885–896. doi:10.1038/sj.emboj.7600054
Dixon, D. M., Choi, J., El-Ghazali, A., Park, S. Y., Roos, K. P., Jordan, M. C., et al. (2015). Loss of muscleblind-like 1 results in cardiac pathology and persistence of embryonic splice isoforms. Sci. Rep. 5 (1), 9042. doi:10.1038/srep09042
Doller, A., Akool, E. S., Huwiler, A., Müller, R., Radeke, H. H., Pfeilschifter, J., et al. (2008). Posttranslational modification of the AU-rich element binding protein HuR by protein kinase Cdelta elicits angiotensin II-induced stabilization and nuclear export of cyclooxygenase 2 mRNA. Mol. Cell. Biol. 28 (8), 2608–2625. doi:10.1128/MCB.01530-07
Dorn, L. E., Lasman, L., Chen, J., Xu, X., Hund, T. J., Medvedovic, M., et al. (2019). The N6-methyladenosine mRNA methylase METTL3 controls cardiac homeostasis and hypertrophy. Circulation 139 (4), 533–545. doi:10.1161/CIRCULATIONAHA.118.036146
Eberhardt, W., Doller, A., Akool, E. S., and Pfeilschifter, J. (2007). Modulation of mRNA stability as a novel therapeutic approach. Pharmacol. Ther. 114 (1), 56–73. doi:10.1016/j.pharmthera.2007.01.002
El Azzouzi, H., Vilaça, A. P., Feyen, D. A., Gommans, W. M., De Weger, R. A., Doevendans, P. A., et al. (2020). Cardiomyocyte specific deletion of ADAR1 causes severe cardiac dysfunction and increased lethality. Front. Cardiovasc. Med. 7, 30. doi:10.3389/fcvm.2020.00030
Engreitz, J. M., Sirokman, K., McDonel, P., Shishkin, A. A., Surka, C., Russell, P., et al. (2014). RNA-RNA interactions enable specific targeting of noncoding RNAs to nascent Pre-mRNAs and chromatin sites. Cell 159 (1), 188–199. doi:10.1016/j.cell.2014.08.018
Fabbiano, F., Corsi, J., Gurrieri, E., Trevisan, C., Notarangelo, M., and D'Agostino, V. G. (2020). RNA packaging into extracellular vesicles: an orchestra of RNA-binding proteins? J. Extracell. vesicles 10 (2), e12043. doi:10.1002/jev2.12043
Fan, S., and Hu, Y. (2022). Role of m6A methylation in the occurrence and development of heart failure. Front. Cardiovasc. Med. 9, 892113. doi:10.3389/fcvm.2022.892113
Fan, X. C., and Steitz, J. A. (1998). HNS, a nuclear-cytoplasmic shuttling sequence in HuR. Proc. Natl. Acad. Sci. 95 (26), 15293–15298. doi:10.1073/pnas.95.26.15293
Fardaei, M., Rogers, M. T., Thorpe, H. M., Larkin, K., Hamshere, M. G., Harper, P. S., et al. (2002). Three proteins, MBNL, MBLL and MBXL, co-localize in vivo with nuclear foci of expanded-repeat transcripts in DM1 and DM2 cells. Hum. Mol. Genet. 11 (7), 805–814. doi:10.1093/hmg/11.7.805
Feng, Y., Valley, M. T., Lazar, J., Yang, A. L., Bronson, R. T., Firestein, S., et al. (2009). SRp38 regulates alternative splicing and is required for Ca2+ handling in the embryonic heart. Dev. Cell 16 (4), 528–538. doi:10.1016/j.devcel.2009.02.009
Fenix, A. M., Miyaoka, Y., Bertero, A., Blue, S. M., Spindler, M. J., Tan, K. K., et al. (2021). Gain-of-function cardiomyopathic mutations in RBM20 rewire splicing regulation and re-distribute ribonucleoprotein granules within processing bodies. Nat. Commun. 12 (1), 6324. doi:10.1038/s41467-021-26623-y
Gallagher, T. L., Arribere, J. A., Geurts, P. A., Exner, C. R., McDonald, K. L., Dill, K. K., et al. (2011). Rbfox-regulated alternative splicing is critical for zebrafish cardiac and skeletal muscle functions. Dev. Biol. 359 (2), 251–261. doi:10.1016/j.ydbio.2011.08.025
Gan, P., Wang, Z., Morales, M. G., Zhang, Y., Bassel-Duby, R., Liu, N., et al. (2022). RBPMS is an RNA-binding protein that mediates cardiomyocyte binucleation and cardiovascular development. Dev. Cell 57 (8), 959–973.e7. doi:10.1016/j.devcel.2022.03.017
Gao, C., Ren, S., Lee, J. H., Qiu, J., Chapski, D. J., Rau, C. D., et al. (2016). RBFox1-mediated RNA splicing regulates cardiac hypertrophy and heart failure. J. Clin. investigation 126 (1), 195–206. doi:10.1172/JCI84015
Garikipati, V. N. S., Verma, S. K., Cheng, Z., Liang, D., Truongcao, M. M., Cimini, M., et al. (2019). Circular RNA CircFndc3b modulates cardiac repair after myocardial infarction via FUS/VEGF-A axis. Nat. Commun. 10 (1), 4317. doi:10.1038/s41467-019-11777-7
Gazzara, M. R., Mallory, M. J., Roytenberg, R., Lindberg, J. P., Jha, A., Lynch, K. W., et al. (2017). Ancient antagonism between CELF and RBFOX families tunes mRNA splicing outcomes. Genome Res. 27 (8), 1360–1370. doi:10.1101/gr.220517.117
Gerstberger, S., Hafner, M., and Tuschl, T. (2014). A census of human RNA-binding proteins. Nat. Rev. Genet. 15 (12), 829–845. doi:10.1038/nrg3813
Gibb Andrew, A., Lazaropoulos Michael, P., and Elrod John, W. (2020). Myofibroblasts and fibrosis: mitochondrial and metabolic control of cellular differentiation. Circ. Res. 127, 427–447. doi:10.1161/CIRCRESAHA.120.316958
Gilbert, W. V., Bell, T. A., and Schaening, C. (2016). Messenger RNA modifications: form, distribution, and function. Science 352 (6292), 1408–1412. doi:10.1126/science.aad8711
Gingerich, T. J., Feige, J. J., and LaMarre, J. (2004). AU-rich elements and the control of gene expression through regulated mRNA stability. Animal health Res. Rev. 5 (1), 49–63. doi:10.1079/ahr200460
Gomes, C. P. D. C., Schroen, B., Kuster, G. M., Robinson, E. L., Ford, K., Squire, I. B., et al. (2020). Regulatory RNAs in heart failure. Circulation 141 (4), 313–328. doi:10.1161/circulationaha.119.042474
Gott, J. M., and Emeson, R. B. (2000). Functions and mechanisms of RNA editing. Annu. Rev. Genet. 34 (1), 499–531. doi:10.1146/annurev.genet.34.1.499
Govindappa, P. K., Patil, M., Garikipati, V. N. S., Verma, S. K., Saheera, S., Narasimhan, G., et al. (2020). Targeting exosome-associated human antigen R attenuates fibrosis and inflammation in diabetic heart. FASEB J. official Publ. Fed. Am. Soc. Exp. Biol. 34 (2), 2238–2251. doi:10.1096/fj.201901995R
Green, L. C., Anthony, S. R., Slone, S., Lanzillotta, L., Nieman, M. L., Wu, X., et al. (2019). Human antigen R as a therapeutic target in pathological cardiac hypertrophy. JCI insight 4 (4), e121541. doi:10.1172/jci.insight.121541
Green, L. C., Slone, S., Anthony, S. R., Guarnieri, A. R., Parkins, S., Shearer, S. M., et al. (2023). HuR-dependent expression of Wisp1 is necessary for TGFβ-induced cardiac myofibroblast activity. J. Mol. Cell. Cardiol. 174, 38–46. doi:10.1016/j.yjmcc.2022.10.007
Guarnieri, A. R., Anthony, S. R., Gozdiff, A., Green, L. C., Fleifil, S. M., Slone, S., et al. (2021). Adipocyte-specific deletion of HuR induces spontaneous cardiac hypertrophy and fibrosis. Am. J. Physiology-Heart Circulatory Physiology 321 (1), H228–H241. doi:10.1152/ajpheart.00957.2020
Guo, Q. Y., Yang, J. Q., Feng, X. X., and Zhou, Y. J. (2023). Regeneration of the heart: from molecular mechanisms to clinical therapeutics. Mil. Med. Res. 10 (1), 18–16. doi:10.1186/s40779-023-00452-0
Guo, W., Schafer, S., Greaser, M. L., Radke, M. H., Liss, M., Govindarajan, T., et al. (2012). RBM20, a gene for hereditary cardiomyopathy, regulates titin splicing. Nat. Med. 18 (5), 766–773. doi:10.1038/nm.2693
Hall-Pogar, T., Liang, S., Hague, L. K., and Lutz, C. S. (2007). Specific trans-acting proteins interact with auxiliary RNA polyadenylation elements in the COX-2 3′-UTR. Rna 13 (7), 1103–1115. doi:10.1261/rna.577707
Hendrayani, S. F., Al-Harbi, B., Al-Ansari, M. M., Silva, G., and Aboussekhra, A. (2016). The inflammatory/cancer-related IL-6/STAT3/NF-κB positive feedback loop includes AUF1 and maintains the active state of breast myofibroblasts. Oncotarget 7 (27), 41974–41985. doi:10.18632/oncotarget.9633
Hendrayani, S. F., Al-Khalaf, H. H., and Aboussekhra, A. (2014). The cytokine IL-6 reactivates breast stromal fibroblasts through transcription factor STAT3-dependent up-regulation of the RNA-binding protein AUF1. J. Biol. Chem. 289 (45), 30962–30976. doi:10.1074/jbc.M114.594044
Hentze, M. W., Castello, A., Schwarzl, T., and Preiss, T. (2018). A brave new world of RNA-binding proteins. Nat. Rev. Mol. Cell Biol. 19 (5), 327–341. doi:10.1038/nrm.2017.130
Hoernes, T. P., and Erlacher, M. D. (2017). Translating the epitranscriptome. Wiley Interdiscip. Rev. RNA 8 (1), e1375. doi:10.1002/wrna.1375
Hu, H., Jiang, M., Cao, Y., Zhang, Z., Jiang, B., Tian, F., et al. (2020). HuR regulates phospholamban expression in isoproterenol-induced cardiac remodelling. Cardiovasc. Res. 116 (5), 944–955. doi:10.1093/cvr/cvz205
Hu, J., Gao, C., Wei, C., Xue, Y., Shao, C., Hao, Y., et al. (2019). RBFox2-miR-34a-Jph2 axis contributes to cardiac decompensation during heart failure. Proc. Natl. Acad. Sci. 116 (13), 6172–6180. doi:10.1073/pnas.1822176116
Huang, M., Akerberg, A. A., Zhang, X., Yoon, H., Joshi, S., Hallinan, C., et al. (2022). Intrinsic myocardial defects underlie an Rbfox-deficient zebrafish model of hypoplastic left heart syndrome. Nat. Commun. 13 (1), 5877. doi:10.1038/s41467-022-32982-x
Izquierdo, J. M. (2008). Hu antigen R (HuR) functions as an alternative pre-mRNA splicing regulator of Fas apoptosis-promoting receptor on exon definition. J. Biol. Chem. 283 (27), 19077–19084. doi:10.1074/jbc.M800017200
Jiang, M. P., Xu, W. X., Hou, J. C., Xu, Q., Wang, D. D., and Tang, J. H. (2021). The emerging role of the interactions between circular RNAs and RNA-binding proteins in common human cancers. J. Cancer 12 (17), 5206–5219. doi:10.7150/jca.58182
Jusic, A., and Devaux, Y.EU-CardioRNA COST Action (CA17129) (2020). Mitochondrial noncoding RNA-regulatory network in cardiovascular disease. Basic Res. Cardiol. 115 (3), 23. doi:10.1007/s00395-020-0783-5
Kalsotra, A., Wang, K., Li, P. F., and Cooper, T. A. (2010). MicroRNAs coordinate an alternative splicing network during mouse postnatal heart development. Genes & Dev. 24 (7), 653–658. doi:10.1101/gad.1894310
Kalsotra, A., Xiao, X., Ward, A. J., Castle, J. C., Johnson, J. M., Burge, C. B., et al. (2008). A postnatal switch of CELF and MBNL proteins reprograms alternative splicing in the developing heart. Proc. Natl. Acad. Sci. 105 (51), 20333–20338. doi:10.1073/pnas.0809045105
Kelaini, S., Chan, C., Cornelius, V. A., and Margariti, A. (2021). RNA-binding proteins hold key roles in function, dysfunction, and disease. Biology 10 (5), 366. doi:10.3390/biology10050366
Kelemen, O., Convertini, P., Zhang, Z., Wen, Y., Shen, M., Falaleeva, M., et al. (2013). Function of alternative splicing. Gene 514 (1), 1–30. doi:10.1016/j.gene.2012.07.083
Koo, J. H., Smiley, M. A., Lovering, R. M., and Margolis, F. L. (2007). Bex1 knock out mice show altered skeletal muscle regeneration. Biochem. biophysical Res. Commun. 363 (2), 405–410. doi:10.1016/j.bbrc.2007.08.186
Koshelev, M., Sarma, S., Price, R. E., Wehrens, X. H., and Cooper, T. A. (2010). Heart-specific overexpression of CUGBP1 reproduces functional and molecular abnormalities of myotonic dystrophy type 1. Hum. Mol. Genet. 19 (6), 1066–1075. doi:10.1093/hmg/ddp570
Kraynik, S. M., Gabanic, A., Anthony, S. R., Kelley, M., Paulding, W., Roessler, A., et al. (2015). The stress-induced heat shock protein 70.3 expression is regulated by a dual-component mechanism involving alternative polyadenylation and HuR. BBA Gene Regul. Mech. 1849 (6), 688–696. doi:10.1016/j.bbagrm.2015.02.004
Krishnamurthy, P., Lambers, E., Verma, S., Thorne, T., Qin, G., Losordo, D. W., et al. (2010). Myocardial knockdown of mRNA-stabilizing protein HuR attenuates post-MI inflammatory response and left ventricular dysfunction in IL-10-null mice. FASEB J. 24 (7), 2484–2494. doi:10.1096/fj.09-149815
Ladd, A. N., Stenberg, M. G., Swanson, M. S., and Cooper, T. A. (2005a). Dynamic balance between activation and repression regulates pre-mRNA alternative splicing during heart development. Dev. Dyn. official Publ. Am. Assoc. Anatomists 233 (3), 783–793. doi:10.1002/dvdy.20382
Ladd, A. N., Taffet, G., Hartley, C., Kearney, D. L., and Cooper, T. A. (2005b). Cardiac tissue-specific repression of CELF activity disrupts alternative splicing and causes cardiomyopathy. Mol. Cell. Biol. 25 (14), 6267–6278. doi:10.1128/MCB.25.14.6267-6278.2005
Lal, A., Mazan-Mamczarz, K., Kawai, T., Yang, X., Martindale, J. L., and Gorospe, M. (2004). Concurrent versus individual binding of HuR and AUF1 to common labile target mRNAs. EMBO J. 23 (15), 3092–3102. doi:10.1038/sj.emboj.7600305
Lale, S., Yu, S., and Ahmed, A. (2011). Complex congenital heart defects in association with maternal diabetes and partial deletion of the A2BP1 gene. Fetal Pediatr. Pathology 30 (3), 161–166. doi:10.3109/15513815.2010.547555
Lee, K. Y., Seah, C., Li, C., Chen, Y. F., Chen, C. Y., Wu, C. I., et al. (2022). Mice lacking MBNL1 and MBNL2 exhibit sudden cardiac death and molecular signatures recapitulating myotonic dystrophy. Hum. Mol. Genet. 31 (18), 3144–3160. doi:10.1093/hmg/ddac108
LeWinter, M. M., and Granzier, H. L. (2014). Cardiac titin and heart disease. J. Cardiovasc. Pharmacol. 63 (3), 207–212. doi:10.1097/FJC.0000000000000007
Li, D., Morales, A., Gonzalez-Quintana, J., Norton, N., Siegfried, J. D., Hofmeyer, M., et al. (2010). Identification of novel mutations in RBM20 in patients with dilated cardiomyopathy. Clin. Transl. Sci. 3 (3), 90–97. doi:10.1111/j.1752-8062.2010.00198.x
Li, M., Ding, W., Sun, T., Tariq, M. A., Xu, T., Li, P., et al. (2018). Biogenesis of circular RNA s and their roles in cardiovascular development and pathology. FEBS J. 285 (2), 220–232. doi:10.1111/febs.14191
Li, P., Qin, D., Chen, T., Hou, W., Song, X., Yin, S., et al. (2023). Dysregulated Rbfox2 produces aberrant splicing of CaV1. 2 calcium channel in diabetes-induced cardiac hypertrophy. Cardiovasc. Diabetol. 22 (1), 168. doi:10.1186/s12933-023-01894-5
Li, S., Guo, W., Dewey, C. N., and Greaser, M. L. (2013). Rbm20 regulates titin alternative splicing as a splicing repressor. Nucleic acids Res. 41 (4), 2659–2672. doi:10.1093/nar/gks1362
Liao, Y., Castello, A., Fischer, B., Leicht, S., Föehr, S., Frese, C. K., et al. (2016). The cardiomyocyte RNA-binding proteome: links to intermediary metabolism and heart disease. Cell Rep. 16 (5), 1456–1469. doi:10.1016/j.celrep.2016.06.084
Linke, W. A., and Bucker, S. (2012). King of hearts: a splicing factor rules cardiac proteins. Nat. Med. 18 (5), 660–661. doi:10.1038/nm.2762
Liu, H., Duan, R., He, X., Qi, J., Xing, T., Wu, Y., et al. (2023). Endothelial deletion of PTBP1 disrupts ventricular chamber development. Nat. Commun. 14 (1), 1796. doi:10.1038/s41467-023-37409-9
Liu, J., Kong, X., Zhang, M., Yang, X., and Xu, X. (2019). RNA binding protein 24 deletion disrupts global alternative splicing and causes dilated cardiomyopathy. Protein Cell 10, 405–416. doi:10.1007/s13238-018-0578-8
Liu, Z., Wang, L., Welch, J. D., Ma, H., Zhou, Y., Vaseghi, H. R., et al. (2017). Single-cell transcriptomics reconstructs fate conversion from fibroblast to cardiomyocyte. Nature 5, 100–104. doi:10.1038/nature24454
Luo, E. C., Nathanson, J. L., Tan, F. E., Schwartz, J. L., Schmok, J. C., Shankar, A., et al. (2020). Large-scale tethered function assays identify factors that regulate mRNA stability and translation. Nat. Struct. Mol. Biol. 27 (10), 989–1000. 51(7678), 100-104. doi:10.1038/s41594-020-0477-6
Makarewich, C. A., and Olson, E. N. (2017). Mining for micropeptides. Trends Cell Biol. 27 (9), 685–696. doi:10.1016/j.tcb.2017.04.006
Mankodi, A., Lin, X., Blaxall, B. C., Swanson, M. S., and Thornton, C. A. (2005). Nuclear RNA foci in the heart in myotonic dystrophy. Circulation Res. 97 (11), 1152–1155. doi:10.1161/01.RES.0000193598.89753.e3
Martens, C. R., Dorn, L. E., Kenney, A. D., Bansal, S. S., Yount, J. S., and Accornero, F. (2022). BEX1 is a critical determinant of viral myocarditis. PLoS Pathog. 18 (2), e1010342. doi:10.1371/journal.ppat.1010342
Mathiyalagan, P., Adamiak, M., Mayourian, J., Sassi, Y., Liang, Y., Agarwal, N. N., et al. (2019). FTO-dependent N6-methyladenosine regulates cardiac function during remodeling and repair. Circulation 139 (4), 518–532. doi:10.1161/CIRCULATIONAHA.118.033794
Miller, J. W., Urbinati, C. R., Teng-umnuay, P., Stenberg, M. G., Byrne, B. J., Thornton, C. A., et al. (2000). Recruitment of human muscleblind proteins to (CUG) n expansions associated with myotonic dystrophy. EMBO J. 19 (17), 4439–4448. doi:10.1093/emboj/19.17.4439
Mishra, S., and Kass, D. A. (2021). Cellular and molecular pathobiology of heart failure with preserved ejection fraction. Nat. Rev. Cardiol. 18 (6), 400–423. doi:10.1038/s41569-020-00480-6
Misquitta, C. M., Chen, T., and Grover, A. K. (2006). Control of protein expression through mRNA stability in calcium signalling. Cell calcium 40 (4), 329–346. doi:10.1016/j.ceca.2006.04.004
Misquitta, C. M., Ghosh, P., Mwanjewe, J., and Grover, A. K. (2005). Role of cis-acting elements in the control of SERCA2b Ca2+ pump mRNA decay by nuclear proteins. Biochem. J. 388 (1), 291–297. doi:10.1042/BJ20041568
Misquitta, C. M., Mwanjewe, J., Nie, L., and Grover, A. K. (2002). Sarcoplasmic reticulum Ca2+ pump mRNA stability in cardiac and smooth muscle: role of the 3′-untranslated region. Am. J. Physiology-Cell Physiology 283 (2), C560–C568. doi:10.1152/ajpcell.00527.2001
Montañés-Agudo, P., Aufiero, S., Schepers, E. N., van der Made, I., Cócera-Ortega, L., Ernault, A. C., et al. (2023). The RNA-binding protein QKI governs a muscle-specific alternative splicing program that shapes the contractile function of cardiomyocytes. Cardiovasc. Res. 119 (5), 1161–1174. doi:10.1093/cvr/cvad007
Mukherjee, K., Ghoshal, B., Ghosh, S., Chakrabarty, Y., Shwetha, S., Das, S., et al. (2016). Reversible HuR-micro RNA binding controls extracellular export of miR-122 and augments stress response. EMBO Rep. 17 (8), 1184–1203. doi:10.15252/embr.201541930
Neumann, D. P., Goodall, G. J., and Gregory, P. A. (2022). The Quaking RNA-binding proteins as regulators of cell differentiation. Wiley Interdiscip. Rev. RNA 13 (6), e1724. doi:10.1002/wrna.1724
Nishikura, K. (2010). Functions and regulation of RNA editing by ADAR deaminases. Annu. Rev. Biochem. 79, 321–349. doi:10.1146/annurev-biochem-060208-105251
Nutter, C. A., Jaworski, E. A., Verma, S. K., Deshmukh, V., Wang, Q., Botvinnik, O. B., et al. (2016). Dysregulation of RBFOX2 is an early event in cardiac pathogenesis of diabetes. Cell Rep. 15 (10), 2200–2213. doi:10.1016/j.celrep.2016.05.002
Ortiz-Sanchez, P., Villalba-Orero, M., López-Olañeta, M. M., Larrasa-Alonso, J., Sánchez-Cabo, F., Martí-Gómez, C., et al. (2019). Loss of SRSF3 in cardiomyocytes leads to decapping of contraction-related mRNAs and severe systolic dysfunction. Circulation Res. 125 (2), 170–183. doi:10.1161/CIRCRESAHA.118.314515
Panda, A. C., Abdelmohsen, K., Yoon, J. H., Martindale, J. L., Yang, X., Curtis, J., et al. (2014). RNA-binding protein AUF1 promotes myogenesis by regulating MEF2C expression levels. Mol. Cell. Biol. 34 (16), 3106–3119. doi:10.1128/MCB.00423-14
Park, J. Y., Li, W., Zheng, D., Zhai, P., Zhao, Y., Matsuda, T., et al. (2011). Comparative analysis of mRNA isoform expression in cardiac hypertrophy and development reveals multiple post-transcriptional regulatory modules. PloS one 6 (7), e22391. doi:10.1371/journal.pone.0022391
Pende, A., Tremmel, K. D., DeMaria, C. T., Blaxall, B. C., Minobe, W. A., Sherman, J. A., et al. (1996). Regulation of the mRNA-binding protein AUF1 by activation of the beta-adrenergic receptor signal transduction pathway. J. Biol. Chem. 271 (14), 8493–8501. doi:10.1074/jbc.271.14.8493
Philips, A. V., Timchenko, L. T., and Cooper, T. A. (1998). Disruption of splicing regulated by a CUG-binding protein in myotonic dystrophy. Science 280 (5364), 737–741. doi:10.1126/science.280.5364.737
Piccoli, M. T., Bär, C., and Thum, T. (2016). Non-coding RNAs as modulators of the cardiac fibroblast phenotype. J. Mol. Cell. Cardiol. 92, 75–81. doi:10.1016/j.yjmcc.2015.12.023
Poganik, J. R., Long, M. J., Disare, M. T., Liu, X., Chang, S. H., Hla, T., et al. (2019). Post-transcriptional regulation of Nrf2-mRNA by the mRNA-binding proteins HuR and AUF1. FASEB J. 33 (12), 14636–14652. doi:10.1096/fj.201901930R
Poon, K. L., Tan, K. T., Wei, Y. Y., Ng, C. P., Colman, A., Korzh, V., et al. (2012). RNA-binding protein RBM24 is required for sarcomere assembly and heart contractility. Cardiovasc. Res. 94 (3), 418–427. doi:10.1093/cvr/cvs095
Qin, Y., Li, L., Luo, E., Hou, J., Yan, G., Wang, D., et al. (2020). Role of m6A RNA methylation in cardiovascular disease (Review). Int. J. Mol. Med. 46 (6), 1958–1972. doi:10.3892/ijmm.2020.4746
Quinones-Valdez, G., Tran, S. S., Jun, H. I., Bahn, J. H., Yang, E. W., Zhan, L., et al. (2019). Regulation of RNA editing by RNA-binding proteins in human cells. Commun. Biol. 2 (1), 19. doi:10.1038/s42003-018-0271-8
Ricketts, S. N., and Qian, L. (2022). The heart of cardiac reprogramming: the cardiac fibroblasts. J. Mol. Cell. Cardiol. 172, 90–99. doi:10.1016/j.yjmcc.2022.08.004
Ripin, N., and Parker, R. (2023). Formation, function, and pathology of RNP granules. Cell 186 (22), 4737–4756. doi:10.1016/j.cell.2023.09.006
Robinson, E. L., and Port, J. D. (2022). Utilization and potential of RNA-based therapies in cardiovascular disease. Basic Transl. Sci. 7 (9), 956–969. doi:10.1016/j.jacbts.2022.02.003
Schafer, S., Adami, E., Heinig, M., Rodrigues, K. E. C., Kreuchwig, F., Silhavy, J., et al. (2015). Translational regulation shapes the molecular landscape of complex disease phenotypes. Nat. Commun. 6 (1), 7200. doi:10.1038/ncomms8200
Schneider, J. W., Oommen, S., Qureshi, M. Y., Goetsch, S. C., Pease, D. R., Sundsbak, R. S., et al. (2020). Dysregulated ribonucleoprotein granules promote cardiomyopathy in RBM20 gene-edited pigs. Nat. Med. 26 (11), 1788–1800. doi:10.1038/s41591-020-1087-x
Schneider-Lunitz, V., Ruiz-Orera, J., Hubner, N., and van Heesch, S. (2021). Multifunctional RNA-binding proteins influence mRNA abundance and translational efficiency of distinct sets of target genes. PLoS Comput. Biol. 17 (12), e1009658. doi:10.1371/journal.pcbi.1009658
Schreiner, S., Didio, A., Hung, L. H., and Bindereif, A. (2020). Design and application of circular RNAs with protein-sponge function. Nucleic acids Res. 48 (21), 12326–12335. doi:10.1093/nar/gkaa1085
Schultz, C. W., Preet, R., Dhir, T., Dixon, D. A., and Brody, J. R. (2020). Understanding and targeting the disease-related RNA binding protein human antigen R (HuR). Wiley Interdiscip. Rev. RNA 11 (3), e1581. doi:10.1002/wrna.1581
Sebastian-delaCruz, M., Gonzalez-Moro, I., Olazagoitia-Garmendia, A., Castellanos-Rubio, A., and Santin, I. (2021). The role of lncRNAs in gene expression regulation through mRNA stabilization. Non-coding RNA 7 (1), 3. doi:10.3390/ncrna7010003
Sharp, P. A. (2005). The discovery of split genes and RNA splicing. Trends Biochem. Sci. 30 (6), 279–281. doi:10.1016/j.tibs.2005.04.002
Shi, X., Cheng, Q., and Zhang, Y. (2020). Reprogramming extracellular vesicles with engineered proteins. Methods 177, 95–102. doi:10.1016/j.ymeth.2019.09.017
Shin, Y., and Brangwynne, C. P. (2017). Liquid phase condensation in cell physiology and disease. Science 357 (6357), eaaf4382. doi:10.1126/science.aaf4382
Singatulina, A. S., Hamon, L., Sukhanova, M. V., Desforges, B., Joshi, V., Bouhss, A., et al. (2019). PARP-1 activation directs FUS to DNA damage sites to form PARG-reversible compartments enriched in damaged DNA. Cell Rep. 27 (6), 1809–1821. doi:10.1016/j.celrep.2019.04.031
Slone, S., Anthony, S. R., Green, L. C., Nieman, M. L., Alam, P., Wu, X., et al. (2023). HuR inhibition reduces post-ischemic cardiac remodeling by dampening acute inflammatory gene expression and the innate immune response. bioRxiv, 2023.01.17.524420–01. doi:10.1101/2023.01.17.524420
Slone, S., Anthony, S. R., Wu, X., Benoit, J. B., Aube, J., Xu, L., et al. (2016). Activation of HuR downstream of p38 MAPK promotes cardiomyocyte hypertrophy. Cell. Signal. 28 (11), 1735–1741. doi:10.1016/j.cellsig.2016.08.005
Srikantan, S., Tominaga, K., and Gorospe, M. (2012). Functional interplay between RNA-binding protein HuR and microRNAs. Curr. Protein Peptide Sci. 13 (4), 372–379. doi:10.2174/138920312801619394
Stempien-Otero, A., Kim, D. H., and Davis, J. (2016). Molecular networks underlying myofibroblast fate and fibrosis. J. Mol. Cell. Cardiol. 97, 153–161. doi:10.1016/j.yjmcc.2016.05.002
Suzuki, H., and Matsuoka, M. (2015). Overexpression of nuclear FUS induces neuronal cell death. Neuroscience 287, 113–124. doi:10.1016/j.neuroscience.2014.12.007
Terenzi, F., Brimacombe, K. R., Penn, M. S., and Ladd, A. N. (2009). CELF-mediated alternative splicing is required for cardiac function during early, but not later, postnatal life. J. Mol. Cell. Cardiol. 46 (3), 395–404. doi:10.1016/j.yjmcc.2008.10.030
Thum, T., Gross, C., Fiedler, J., Fischer, T., Kissler, S., Bussen, M., et al. (2008). MicroRNA-21 contributes to myocardial disease by stimulating MAP kinase signalling in fibroblasts. Nature 456 (7224), 980–984. doi:10.1038/nature07511
Tian, B., Hu, J., Zhang, H., and Lutz, C. S. (2005). A large-scale analysis of mRNA polyadenylation of human and mouse genes. Nucleic acids Res. 33 (1), 201–212. doi:10.1093/nar/gki158
Tian, B., and Manley, J. L. (2017). Alternative polyadenylation of mRNA precursors. Nat. Rev. Mol. Cell Biol. 18 (1), 18–30. doi:10.1038/nrm.2016.116
Tian, C., Gao, L., and Zucker, I. H. (2021). Regulation of Nrf2 signaling pathway in heart failure: role of extracellular vesicles and non-coding RNAs. Free Radic. Biol. Med. 167, 218–231. doi:10.1016/j.freeradbiomed.2021.03.013
Tranter, M., Helsley, R. N., Paulding, W. R., McGuinness, M., Brokamp, C., Haar, L., et al. (2011). Coordinated post-transcriptional regulation of Hsp70. 3 gene expression by microRNA and alternative polyadenylation. J. Biol. Chem. 286 (34), 29828–29837. doi:10.1074/jbc.M111.221796
Twyffels, L., Gueydan, C., and Kruys, V. (2011). Shuttling SR proteins: more than splicing factors. FEBS J. 278 (18), 3246–3255. doi:10.1111/j.1742-4658.2011.08274.x
van den Hoogenhof, M. M., van der Made, I., de Groot, N. E., Damanafshan, A., van Amersfoorth, S. C., Zentilin, L., et al. (2018). AAV9-mediated Rbm24 overexpression induces fibrosis in the mouse heart. Sci. Rep. 8 (1), 11696. doi:10.1038/s41598-018-29552-x
van Heesch, S., Witte, F., Schneider-Lunitz, V., Schulz, J. F., Adami, E., Faber, A. B., et al. (2019). The translational landscape of the human heart. Cell 178 (1), 242–260. doi:10.1016/j.cell.2019.05.010
Verma, S. K., Deshmukh, V., Nutter, C. A., Jaworski, E., Jin, W., Wadhwa, L., et al. (2016). Rbfox2 function in RNA metabolism is impaired in hypoplastic left heart syndrome patient hearts. Sci. Rep. 6 (1), 30896. doi:10.1038/srep30896
Verma, S. K., Deshmukh, V., Thatcher, K., Belanger, K. K., Rhyner, A. M., Meng, S., et al. (2022). RBFOX2 is required for establishing RNA regulatory networks essential for heart development. Nucleic acids Res. 50 (4), 2270–2286. doi:10.1093/nar/gkac055
Wallis, J. L., Irvine, M. W., Jane, D. E., Lodge, D., Collingridge, G. L., and Bortolotto, Z. A. (2015). An interchangeable role for kainate and metabotropic glutamate receptors in the induction of rat hippocampal mossy fiber long-term potentiation in vivo. Hippocampus 25 (11), 1407–1417. doi:10.1002/hipo.22460
Wang, G., Wu, H., Liang, P., He, X., and Liu, D. (2021). Fus knockdown inhibits the profibrogenic effect of cardiac fibroblasts induced by angiotensin II through targeting Pax3 thereby regulating TGF-β1/Smad pathway. Bioengineered 12 (1), 1415–1425. doi:10.1080/21655979.2021.1918522
Wang, J., Li, G., Yu, D., Wong, Y. P., Yong, T. F., Liang, M. C., et al. (2018). Characterization of CaV1. 2 exon 33 heterozygous knockout mice and negative correlation between Rbfox1 and CaV1. 2 exon 33 expressions in human heart failure. Channels 12 (1), 51–57. doi:10.1080/19336950.2017.1381805
Wang, Y., and Liu, B. (2020). Circular RNA in diseased heart. Cells 9 (5), 1240. doi:10.3390/cells9051240
Washburn, M. C., and Hundley, H. A. (2016). Controlling the editor: the many roles of RNA-binding proteins in regulating A-to-I RNA editing. RNA Process. Dis. Genome-wide Probing 907, 189–213. doi:10.1007/978-3-319-29073-7_8
Wei, C., Qiu, J., Zhou, Y., Xue, Y., Hu, J., Ouyang, K., et al. (2015) Repression of the central splicing regulator RBFox2 is functionally linked to pressure.
Wiedemann, N., and Pfanner, N. (2017). Mitochondrial machineries for protein import and assembly. Annu. Rev. Biochem. 86, 685–714. doi:10.1146/annurev-biochem-060815-014352
Wu, H., Zhao, Z. A., Liu, J., Hao, K., Yu, Y., Han, X., et al. (2018). Long noncoding RNA Meg3 regulates cardiomyocyte apoptosis in myocardial infarction. Gene Ther. 25 (8), 511–523. doi:10.1038/s41434-018-0045-4
Wu, X., and Brewer, G. (2012). The regulation of mRNA stability in mammalian cells: 2.0. Gene 500 (1), 10–21. doi:10.1016/j.gene.2012.03.021
Xu, X., Yang, D., Ding, J. H., Wang, W., Chu, P. H., Dalton, N. D., et al. (2005). ASF/SF2-regulated CaMKIIdelta alternative splicing temporally reprograms excitation-contraction coupling in cardiac muscle. Cell 120 (1), 59–72. doi:10.1016/j.cell.2004.11.036
Xue, Y., Sirokman, K., McDonel, P., Shishkin, A. A., Surka, C., Russell, P., et al. (2022). Architecture of RNA–RNA interactions. Current opinion in genetics & development, 72, 138-144. As to nascent Pre-mRNAs and chromatin sites. Cell 159 (1), 188–199. doi:10.1016/j.cell.2014.08.018
Yoon, J. H., De, S., Srikantan, S., Abdelmohsen, K., Grammatikakis, I., Kim, J., et al. (2014). PAR-CLIP analysis uncovers AUF1 impact on target RNA fate and genome integrity. Nat. Commun. 5 (1), 5248. doi:10.1038/ncomms6248
Yu, D., Li, X., Wang, Z., Jiang, S., Yan, T., Fang, K., et al. (2022). Role of AUF1 in modulating the proliferation, migration and senescence of skin cells. Exp. Ther. Med. 23 (1), 45–12. doi:10.3892/etm.2021.10967
Zang, H., Mathew, R. O., and Cui, T. (2020). The dark side of Nrf2 in the heart. Front. physiology 11, 722. doi:10.3389/fphys.2020.00722
Zhang, J., Bahi, N., Llovera, M., Comella, J. X., and Sanchis, D. (2009). Polypyrimidine tract binding proteins (PTB) regulate the expression of apoptotic genes and susceptibility to caspase-dependent apoptosis in differentiating cardiomyocytes. Cell Death Differ. 16 (11), 1460–1468. doi:10.1038/cdd.2009.87
Zhang, X., Zhou, Y., Chen, S., Li, W., Chen, W., and Gu, W. (2019). LncRNA MACC1-AS1 sponges multiple miRNAs and RNA-binding protein PTBP1. Oncogenesis 8 (12), 73. doi:10.1038/s41389-019-0182-7
Zheng, D., and Tian, B. (2014). RNA-binding proteins in regulation of alternative cleavage and polyadenylation. Syst. Biol. RNA Bind. Proteins 825, 97–127. doi:10.1007/978-1-4939-1221-6_3
Zhihao, L., Jingyu, N., Lan, L., Michael, S., Rui, G., Xiyun, B., et al. (2020). SERCA2a: a key protein in the Ca 2+ cycle of the heart failure. Heart Fail. Rev. 25, 523–535. doi:10.1007/s10741-019-09873-3
Zhou, A., Xie, A., Kim, T. Y., Liu, H., Shi, G., Kang, G. J., et al. (2018). HuR-mediated SCN5A messenger RNA stability reduces arrhythmic risk in heart failure. Heart rhythm. 15 (7), 1072–1080. doi:10.1016/j.hrthm.2018.02.018
Keywords: RNA binding proteins (RBPs), post-transcriptional gene regulation, cardiac, hypertrophy, fibrosis
Citation: Acharya P, Parkins S and Tranter M (2024) RNA binding proteins as mediators of pathological cardiac remodeling. Front. Cell Dev. Biol. 12:1368097. doi: 10.3389/fcell.2024.1368097
Received: 09 January 2024; Accepted: 01 May 2024;
Published: 16 May 2024.
Edited by:
Adrián Ruiz Villalba, Junta de Andalucía-Universidad de Málaga, SpainReviewed by:
Carlos García Padilla, University of Jaén, SpainMaarten M. G. van den Hoogenhof, Heidelberg University Hospital, Germany
Lucia Cócera Ortega, Maastricht University, Netherlands
Copyright © 2024 Acharya, Parkins and Tranter. This is an open-access article distributed under the terms of the Creative Commons Attribution License (CC BY). The use, distribution or reproduction in other forums is permitted, provided the original author(s) and the copyright owner(s) are credited and that the original publication in this journal is cited, in accordance with accepted academic practice. No use, distribution or reproduction is permitted which does not comply with these terms.
*Correspondence: Michael Tranter, bWljaGFlbC50cmFudGVyQG9zdW1jLmVkdQ==