- GenPhySE, Université de Toulouse, INRAE, ENVT, Castanet Tolosan, France
Genomic imprinting is an epigenetically-regulated process of central importance in mammalian development and evolution. It involves multiple levels of regulation, with spatio-temporal heterogeneity, leading to the context-dependent and parent-of-origin specific expression of a small fraction of the genome. Genomic imprinting studies have therefore been essential to increase basic knowledge in functional genomics, evolution biology and developmental biology, as well as with regard to potential clinical and agrigenomic perspectives. Here we offer an overview on the contribution of livestock research, which features attractive resources in several respects, for better understanding genomic imprinting and its functional impacts. Given the related broad implications and complexity, we promote the use of such resources for studying genomic imprinting in a holistic and integrative view. We hope this mini-review will draw attention to the relevance of livestock genomic imprinting studies and stimulate research in this area.
1 Introduction
Controlled experiments in mice and investigation of placental pathologies in humans have made it possible to understand the need for a genome derived from specific male and female parental contributions, which must be spatio-temporally regulated for normal development (Hall, 1990; Reik and Walter, 2001). Such a conception of heredity, apparently contravening Mendel’s laws, has fueled the emergence of a specific field of research dedicated to characterizing the underlying phenomenon, known as genomic imprinting, and identifying the molecular mechanisms behind the non-equivalence of parental genomes (Ferguson-Smith and Bourchis, 2018; Tucci et al., 2019).
Our understanding of how regions affected by imprinting have evolved and function has expanded considerably (Llères et al., 2021; Kaneko-Ishino and Ishino, 2022; Richard Albert et al., 2023) and we today have increasingly sophisticated resources and tools (Hubert and Demars, 2022; Jima et al., 2022; Akbari et al., 2023) to identify and accurately report the links between nuclear architecture, Imprinting Control Regions (ICRs), imprinted genes, other genes including different RNA species and, finally, phenotypes. While cytosine methylation is key to almost all regulations that affect it, including transgenerational maintenance, imprinting involves multi-scale mechanisms (Monk et al., 2019), giving grounds for multi-omics approaches (Hubert and Demars, 2022). A diversity of non-exclusive patterns, such as the recruitment of CCCTC-Binding Factor (CTCF) (Noordermeer and Feil, 2020), the transcriptional interference of long non-coding RNA (lncRNA) (MacDonald and Mann, 2020) or the presence of microRNA (miRNA) clusters (Malnou et al., 2019), have, for example, been highlighted at imprinted loci. In addition, histone marks affect the methylation at ICRs, representing another layer of regulatory complexity (Sanli and Feil, 2015). Interestingly, the possibility of DNA methylation-independent, histone-based imprinting has been identified in rodents and termed as noncanonical imprinting (Raas et al., 2022; Inoue, 2023; Richard Albert et al., 2023). The rapid initial accumulation of knowledge on imprinting has been largely enabled by the mouse model (Swain et al., 1987; Li et al., 1993; Greally et al., 1998). However, livestock species have also led to very illuminating results (Cockett et al., 1996; Feil et al., 1998; Jeon et al., 1999; O'Doherty et al., 2015), due to their presence in many vertebrate clades, with a particular importance in the Cetartiodactyla order that includes ruminants and pigs, and the availability of experimental livestock resources suitable for research on imprinting. We therefore wish to emphasize livestock research on imprinting, as we believe it can provide valuable basic knowledge and models to link multi-level molecular variations to complex phenotypical changes (Magee et al., 2014; Daigneault, 2022).
In the present article, we first summarize some of the key contributions regarding imprinting in livestock species, primarily around the identification of imprinted genes, from a broad and historical perspective. We then discuss in more detail molecular mechanisms linking variability at imprinted domains, including IGF2–H19, CDKN1C–KCNQ1 and DLK1–GTL2, to developmental effects on muscle and growth phenotypes, as established in livestock species. We finally review several current topics in livestock research aiding deeper understanding of the role of imprinting in complex traits related to energy supply or intake, with broad significance for mammalian biology and health.
2 Livestock species occupy a pivotal place in research on genomic imprinting
Initially uncovered in the 80s through iconic murine pronuclear transplantation studies, the presence of imprinting in mammalian genomes led to new methodological developments to detect the parent-of-origin (PofO)-specific expression of genomic regions and their associated functions. They notably consisted of designing specific genetic constructs (Cattanach, 1986; Lee et al., 1993) then molecular biology approaches (Hatada et al., 1993) in mice, allowing the identification of the first imprinted genes in the early 90s (Igf2, Igf2r and H19). In humans, expression studies using in particular fetal tissues allowed determination of the imprinting status of many candidate genes for imprinting (Zhang and Tycko, 1992; Hatada et al., 1996; Blagitko et al., 2000; Murphy et al., 2001) leading to the identification of dozens of imprinted genes but also evidence for differences compared to mice (Kalscheuer et al., 1993; Monk et al., 2006).
In order to check theoretical predictions about the link between imprinting and viviparity, a subset of genes systematically identified as imprinted in placental mammals was found to be imprinted as well in different marsupial species (O'Neill et al., 2000; Suzuki et al., 2005), but not in monotremes (Killian et al., 2001) or in birds (O'Neill et al., 2000). While shedding light on the ancestral origin of genomic imprinting, such single-gene isolation studies were limited to highly conserved imprinted genes, such as in the IGF2 (Insulin Growth Factor 2) pathway. In 2002, humans and mice became the only mammals with a reference sequence, allowing early genome-wide studies for novel imprinted genes (Luedi et al., 2005; Luedi et al., 2007), in agreement with estimates suggesting that from one to a few percent of all mammalian genes could be subjected to imprinting. Some livestock species soon experienced comparable developments in sequencing technologies, improving the phylogenetic-scale knowledge on imprinting. With pig, cattle and sheep displaying more than 30 experimentally-validated imprinted genes, Cetartiodactyla is one of the most documented mammalian orders on imprinting, alongside primates (Cheong et al., 2015; Chu et al., 2021; Jima et al., 2022) and rodents (Raas et al., 2022; Richard Albert et al., 2023) (Figure 1). Not only do livestock species include representatives from various mammalian lineages but also from key outgroups, i.e., birds and fish, providing opportunities for testing hypotheses on imprinting and epigenomic evolution in vertebrates.
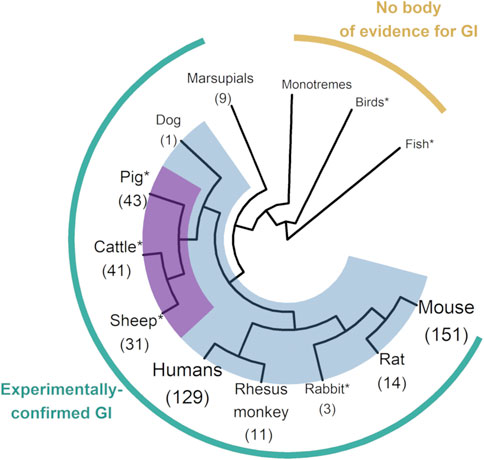
FIGURE 1. Phylogenetic tree of vertebrates with genomic imprinting research. The figure shows the clades in which the existence of imprinting has been tested. Where imprinted genes have been identified, their total number is reported between brackets under the corresponding clade or species name. Placental mammals, which gather almost all the imprinted genes identified to date, are highlighted in blue. The Cetartiodactyla superorder (including pig, cattle and sheep) is highlighted in magenta, since it forms one of the most documented clades for imprinting, with three branches above 30 imprinted genes identified from data available in livestock species. Size of branch labels is function of the number of currently known imprinted genes in the corresponding clade or species. This figure was produced using R base (v4.1.1) with the ggplot2 (v3.4.2), rotl (v3.0.14), magrittr (v2.0.3) and ggtree (v3.2.1) packages (https://cran.r-project.org/). Count data for imprinted genes come from the Geneimprint Database (http://www.geneimprint.com/). *Clade including livestock species. GI: Genomic imprinting.
Mainly driven by the needs of different agri-food sectors and the acceleration in the development of sequencing technologies in the 2000s, specific genomic resources began to emerge in animal species of economic importance. In 2015, the NCBI Reference Sequence Database reported at least a chromosome-level assembly reference sequence for a total of 14 of such species, with chicken (Wallis et al., 2004), ruminants (The Bovine Genome Sequencing and Analysis Consortium et al., 2009; The International Sheep Genomics Consortium et al., 2010; Dong et al., 2013) and pigs (Humphray et al., 2007) among the first. At the same time, these species have been subjected to the development of increasingly dense microarray platforms (Sellner et al., 2007). These early annotated genomes and high-throughput genotyping tools have promoted the use of genetic information for breeding, establishing species-specific genetic evaluation procedures (Hayes et al., 2009; Legarra et al., 2011; Ibáñez-Escriche et al., 2014) and enabling functional genomics approaches for increasingly fine and complex traits (Hayes et al., 2010; Do et al., 2013). This also brought higher-resolution analyses of imprinting (Bischoff et al., 2009; Chen et al., 2016; Kenny et al., 2022), leading to the identification of dozens of imprinted genes in pigs, cattle and sheep, including large conserved imprinted domains affecting early development, such as IGF2–H19 and MEST (Rutkowska and Lukaszewicz, 2019).
Combined with valuable pre-existing livestock resources, the increasing availability of Next-Generation Sequencing (NGS) platforms has resulted in diversified studies expanding several areas of our understanding of imprinting. Especially, results from non-mammalian livestock species with both adapted genomic and experimental resources made it possible to raise the question of the presence or absence of imprinting in egg-laying vertebrates (Frésard et al., 2014; Fan et al., 2016) at the genome level. As exemplified in chicken, several genome-scale studies across different tissues and developmental stages are advisable to better understand PofO-specific expression mechanisms among all possible forms of allelic imbalance (Zhuo et al., 2017). Robust tools and procedures are indeed essential when investigating potential imprinting patterns (Edwards et al., 2023), and transcriptomic studies involving reciprocal crosses have so far collectively concluded there were allele-specific expression rather than imprinting in chicken tissues (Frésard et al., 2014; Zhuo et al., 2017). In livestock mammals, NGS-based studies allowed identifying additional imprinted genes and started addressing different related regulatory processes (Congras et al., 2014; Chen et al., 2016; Duan et al., 2018). In addition, a growing body of research explores local mechanisms involved at imprinted loci with evo-devo or biomedical significance (Yu et al., 2018; Ahn et al., 2020; Li J. et al., 2022; Ahn et al., 2023).
Following the path taken by the biomedical research community, international initiatives promote the generation of increasingly comprehensive and accessible tools and resources in epigenomics and transcriptomics (Giuffra et al., 2019; Tixier-Boichard et al., 2021). Recent large-scale cattle studies show the interest of multi-omics not limited to coding regions to capture large proportions of heritability and better predict the phenome (Xiang et al., 2019; Xiang et al., 2023). Efforts in establishing large catalogs of functional elements has resulted in the public availability of datasets from adult, fetal tissues and single cells, including RNAseq, methylation, histone mark, CTCF occupancy and chromatin accessibility data in pigs, cattle and chicken (Kern et al., 2021; Pan et al., 2023). All this novel information on regulatory elements from a diversity of biological contexts (i.e., species, populations, developmental stages, tissues) provides an integrative framework that is particularly helpful to supporting new livestock studies on imprinting (Ahn et al., 2022; Bruscadin et al., 2022).
3 Livestock studies reveal a variety of molecular mechanisms at play in genomic imprinting
In livestock species, the major role played by imprinted genes is documented as they are part of the molecular architecture of some agronomic traits (O'Doherty et al., 2015). In pigs and sheep, two mutations affecting a production trait have been identified, and–along with a syndrome linked to imprinting disturbances in cattle–are examples of imprinting contribution to phenotypic variability.
3.1 The paternal mutation within IGF2 is responsible of hypermuscularity in pigs–a regulatory mutation unaffecting methylation
A Quantitative Trait Locus (QTL) with a major effect on muscle mass and fat deposition in pigs has been identified on chromosome 2 from different experimental crosses (Jeon et al., 1999; Nezer et al., 1999). This QTL is responsible for 30% of the variance observed for lean meat, 15%–30% of the variance for muscle mass and 10%–20% of the variance for increased backfat content. The conserved synteny between the porcine region of chromosome 2 and the human orthologous region suggested the paternally expressed IGF2 gene as a candidate gene given its major role in fetal and post-natal growth in humans. In order to test this hypothesis, an adapted statistical model testing for the presence of an imprinting effect has been developed. A paternal effect of this QTL has been demonstrated, reinforcing the interest of IGF2.
The causal mutation of this QTL corresponds to a point mutation in the third intron of the IGF2 gene (Van Laere et al., 2003). Only pigs homozygous for the mutated allele or heterozygous for the mutated allele inherited from their father show hypermuscularity. Animals carrying the mutated allele on their paternal chromosome produce three times more IGF2 messengers in skeletal muscle after birth (Van Laere et al., 2003). Although the mutation is located in an evolutionarily conserved CpG island that is hypomethylated in skeletal muscle, it does not affect its methylation pattern. However, the mutation abrogates binding to the transcriptional repressor ZBED6 (Zinc finger BED domain-containing protein 6), resulting in an increased expression of messengers from the IGF2 gene and hence muscle hypertrophy in animals that have received the mutated allele from their father (Markljung et al., 2009).
3.2 The DLK1-MEG3 mutation and the callipyge phenotype in sheep–a unique model of polar overdominance
The Callipyge phenotype, named after a Greek word meaning “beautiful buttocks”, is characterized in sheep by a 30% increase in hindquarters muscle associated with a 8% decrease in fat content and improved feed efficiency (Cockett et al., 1996). The hypermuscularity phenotype is only observed in heterozygous individuals carrying the mutated allele on their paternal chromosome. This atypical mode of non-Mendelian transmission represents a particular case of imprinting known as polar overdominance (Reik and Walter, 2001; Lawson et al., 2013).
The mutation is a substitution located on ovine chromosome 18 within a group of imprinted genes, between the paternally expressed gene coding for the DLK1 (Delta Like Non-Canonical Notch Ligand 1) protein and the maternally expressed MEG3 (Maternally Expressed 3) gene, which is a lncRNA (Freking et al., 2002; Smit et al., 2003). Callipyge animals, carrying the allele on the paternal chromosome, showed in comparison with non-Callipyge individuals i) an overexpression of transcripts from the DLK1 and PEG11 (Paternally Expressed 11) genes encoding proteins in skeletal muscle (Murphy et al., 2006), ii) a reduction in methylation in muscle throughout the region of the imprinted gene cluster (Takeda et al., 2006), and iii) a decrease in the expression of HDAC9 (Histone Deacetylase 9), an enzyme associated with chromatin condensation (Vuocolo et al., 2007). Although two transgenic mouse models for ectopic expression of DLK1 (Davis et al., 2004) and PEG11 (Xu et al., 2015) genes have suggested a synergistic action of the two genes to induce the Callipyge phenotype, it has recently been shown that DLK1 seems the primary effector of muscle hypertrophy (Yu et al., 2018).
3.3 The large offspring syndrome–a bovine model of human imprinting disorder
The main phenotype of Large Offspring Syndrome (LOS but also known as AOS, Abnormal Offspring Syndrome) is overgrowth, accompanied in particular with macroglossia, umbilical hernia, and limb and spinal cord anomalies; it can be identified during gestation (Rivera et al., 2022). This syndrome has also been observed in fetuses and newborns in ruminants (Walker et al., 1996; Chen et al., 2013). The first cases have been reported in the 90s following the use of assisted reproduction methods such as nuclear transfer (Willadsen et al., 1991) or in vitro embryo production (Behboodi et al., 1995). We now know that it can also be of natural origin, but its incidence increases with the use of assisted reproduction techniques (Rivera et al., 2021). In humans, Beckwith-Wiedemann Syndrome (BWS) has a similar etiology. This syndrome, induced in particular by assisted reproduction methods, may be genetic or epigenetic in origin and results in an alteration of imprinting (Filippi and Mckusick, 1970; Brioude et al., 2018).
Interestingly, it has been shown that LOS and BWS are the consequence of a loss of imprinting affecting orthologous genes between the two species (Mangiavacchi et al., 2021). This dysregulation of imprinting concerns the IGF2–H19 and CDKN1C–KCNQ1 domains, with their respective ICRs called ICR1 and ICR2 (Li et al., 2022). These alterations involve changes in DNA methylation of either ICR1 (gain of methylation of ICR1 leading to an increased expression of IGF2, an important growth factor (Mangiavacchi et al., 2021)) or ICR2 (loss of methylation of ICR2, leading to a dysregulation of imprinted genes located in the CDKN1C–KCNQ1 domain). Recent studies have also demonstrated the role of miRNAs (Li et al., 2019), tRNAs (Goldkamp et al., 2022) and changes in chromatin conformation (Li et al., 2022) in the manifestations of BWS. The phenotypic and molecular similarities between the two syndromes, bovine and human, make LOS an excellent model for studying human imprinting disorders.
4 Harnessing livestock resources for a fuller picture of the implications of genomic imprinting
Most of our understanding of the phenotypic consequences of imprinting comes from mouse models and human imprinting disorders (Peters, 2014). Imprinting disorders result in a range of clinical features including aberrant pre- and/or postnatal growth and abnormal feeding behaviours (Carli et al., 2020). Imprinted disorders are a group of congenital disorders with common underlying molecular alterations, including genetic abnormalities as well as aberrant epigenetic landscapes, which target imprinted genes. They show that imprinted genes are key loci involved in traits’ variability through their genetic and epigenetic PofO effects. Besides imprinted disorders, more and more studies pinpoint the role of imprinted genes and their PofO effects in the variability of complex traits (Lawson et al., 2013). In livestock species, some traits of major agronomic interest overlap with phenotypes affected in imprinting disorders such as birthweight, postnatal resources through mammary gland development or feeding behaviours, making livestock resources relevant for a better understanding of the role of imprinting in complex traits.
4.1 Birthweight is a trait of major importance in pig production
In pigs, birthweight is a trait with an important social impact given its significant association with stillbirth risk and pre-weaning mortality and a major economic interest since it significantly determines later growth and thus impacts economic outputs. Pig genetic selection for reproduction traits has been focused in the last decades on increasing litter size. However, the significant genetic gain obtained for this trait over 20 years of selection was coupled with a reduction of piglet survival from birth to weaning. Moreover, birthweight is considered as a maternal trait in most pig selective breeding neglecting both the direct and paternal effects in birthweight estimation, whereas in humans a paternal contribution to birthweight has been reported (Magnus et al., 2001). Recent epidemiological studies have confirmed that both maternal and paternal genes do participate to infant birthweight, with a greater influence from the maternal side than from the paternal side (Rice and Thapar, 2010). Moreover, estimates of direct heritability for individual birthweight in piglets are moderate (Banville et al., 2015), as also shown in humans (Momoko et al., 2016). As the birthweight of the offspring strongly depends on the maternal environment and genome, the placenta is the fetal tissue which allows the crosstalk between mother and fetus. Interestingly, it shows a tissue-specific imprinting pattern with many genes that are imprinted only in the placenta (Monk, 2015), which makes it a key tissue to better understand birthweight regulation in livestock. Moreover, the differences in placentation between humans, rodents, and pigs suggest that imprinting could serve in pig placenta an additional function to that of placental development and maternal resource allocation (Chavatte-Palmer and Tarrade, 2016).
Dedicated experimental designs have been set up to i) consider maternal, paternal and direct genetic effects and ii) identify genomic regions involved in the molecular architecture of birthweight and more recently maturity of piglets (Canario et al., 2022) (Figure 2A). Many studies have highlighted QTLs affecting birthweight in pigs in different breeds. Most of them are referenced in the Animal QTLdb (Hu et al., 2013). Remarkably, among all QTLs detected for birthweight, several regions encompass clusters of genes that are known to be imprinted in human such as QTLs overlapping with MEST (Mesoderm Specific Transcript) and DLK1 (Guo et al., 2008; Ai et al., 2012). These observations in pigs might mimic results reported in a recent study performed in a familial human dataset that showed an enrichment of associations with birthweight in imprinted regions (Juliusdottir et al., 2021).
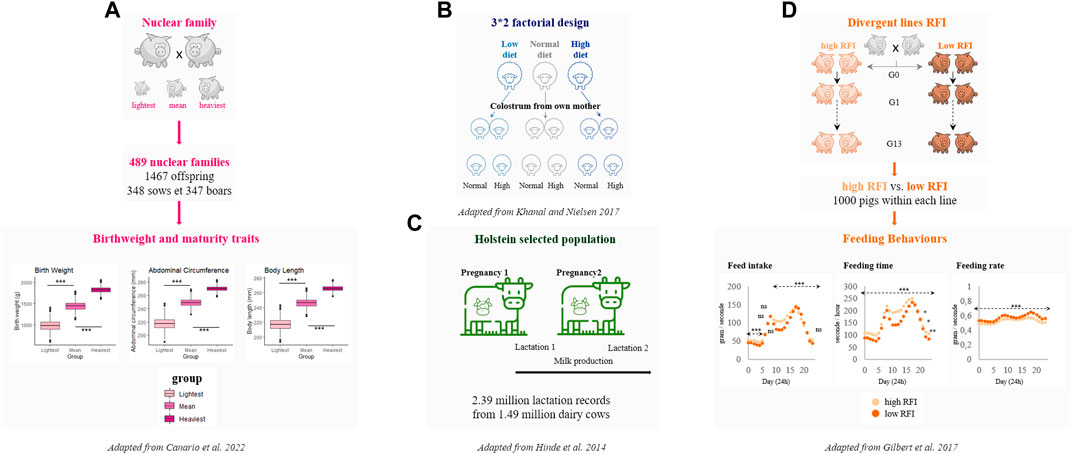
FIGURE 2. Experimental livestock populations measured for traits overlapping features of imprinted genes. (A)- An experimental design has been generated to quantify the different genetic components (paternal, maternal and offspring) of birthweight and maturity in piglets (Canario et al., 2022). (B)- The Copenhagen experimental design has been developed in sheep to evaluate impacts of prenatal and postnatal nutrition on animal production and performance (Khanal et al., 2017). (C)- The dairy Holstein cow population allow access to over 1 million of lactation records with their associated pedigree (Hinde et al., 2014). (D)- Divergent selected lines for Residual Feed Intake (RFI) in pigs over several generations have been also measured for feeding behaviours (Gilbert et al., 2017).
4.2 The mammary gland–an exocrine organ critical for postnatal growth of the offspring
Although few examples of specific patterns of imprinting in mammary gland have been highlighted (Curley et al., 2004; Andergassen et al., 2017; Xu et al., 2021), its role as a key imprinted tissue is still debated, as for the placenta and the brain. As an example, Grb10 has recently been shown to be maternally-expressed in lactating mammary glands in mice, with complementary functions in mother and offspring. While Grb10 suppresses growth in offspring, it increases milk production in mother (Cowley et al., 2014). Hanin and Ferguson-Smith (2020) support the idea suggested by Stringer et al. (2014) according to which mammary gland represents the functional equivalent of the placenta in the postnatal stage of eutherian mammals, contributing then to the development and growth of offspring. Indeed, mammary gland, by providing milk to the newborn, contributes further to maternal effects on offspring phenotypes, in addition to those that occur during fetal life. Milk provides the neonate with essential nutritional components and non-nutritional bio-active components, such as growth factors including IGF2 that has been shown to be an important biological regulator of milk production in dairy cattle (Berkowicz et al., 2011). Many studies, mainly in mice, showed that the maternal-offspring interface through lactation is a critical period, suggesting that genetic variation and maternal diets may affect milk composition, leading to lasting effects with alteration of lifelong health (Hanin and Ferguson-Smith, 2020; Rodríguez-González et al., 2020; Lean et al., 2022).
In ruminants, milk production is a major agronomic trait. Failure to produce quality and quantity of milk mainly due to diseases affecting mammary gland leads to high economic losses for breeders. In addition, a report from the Food and Agriculture Organization of the United Nations (FAO) pinpoints the importance of animal milk in the diets of children in populations with very low fat intakes and limited access to other animal source foods (https://www.fao.org/dairy-production-products/products/milk-composition/en/). Association genetics identified many genomic regions influencing different phenotypes of milk composition, showing in particular a major effect of the imprinted gene DGAT1 (Diacylglycerol O-Acyltransferase 1) and caseins on total yields of milk, protein and fat (Poulsen and Larsen, 2021). In addition, other candidate genes mostly affecting milk fat and protein content were shown to play a role in secretory functions in the mammary gland as well as mammary gland development (Cui et al., 2014). In consequence, ruminant species may represent powerful models (Figures 2B, C) to access high throughput information on genetic components of milk composition to fully understand the significance and the physiological role of imprinted genes in the mammary gland and in postnatal provisioning.
4.3 Feeding behaviours are indicators of animal welfare and health in livestock
In humans, the known role of imprinting mechanisms in feeding behaviours is mainly restricted to pathologies such as imprinted disorders (Ho-Shing and Dulac, 2019; Elbracht et al., 2020). Typical clinical features of imprinted disorders lies either in difficulties in feeding or hyperphagia. The increasing capacity to record behaviour parameters automatically for livestock precision farming makes it possible to monitor feeding behaviours of individual animals over time (Cellier et al., 2021). Nowadays, investigation of feeding behaviours in livestock species is merely used as a proxy of feed efficiency phenotypes that are major traits in breeding selection schemes (Cavani et al., 2022). For example, feed efficiency represents up to 50% of the breeding objectives in some European pig paternal lines (Gilbert et al., 2017). A divergent selection experiment on a measure of feed efficiency in pigs showed after more than 10 generations an alteration of feeding behaviours traits in feed efficiency-divergent individuals, with significant differences for feed intake (12%), daily eating time (22%) and number of visits and feeding rate (14%) (Gilbert et al., 2017) (Figure 2D).
Only few studies assessing the genomic basis of feeding behaviours and identifying several QTLs have been performed in livestock species so far (Ding et al., 2018; Fu et al., 2020). In an interesting way, among QTLs detected for feeding behaviours and available in Animal QTLdb (Hu et al., 2013), several regions encompass clusters of genes that are known to be imprinted in human such as DLK1 and PRKAG2 (Reiner et al., 2009). These observations highlight that livestock might significantly improve the understanding of imprinting in the variability of feeding behaviours, with implications for animal welfare and overall health in sight.
5 Discussion
This review aimed to show how livestock species could contribute to essential information on genomic imprinting, from evolutionary conservation to pathophysiological models for specific human disorders, including complex molecular imprinting patterns affecting trait variation.
Overall, there is very little imprinting studies on non-mammalian vertebrates, a first obstacle being the difficulty of accessing or funding adequate experimental resources. The scarcity of such studies may also be partly explained by the widespread possibility of parthenogenesis in these species, which is generally seen as incompatible with imprinting. Non-mammalian vertebrates however show a remarkable diversity of embryo-related adaptations, allowing detailed research on embryonic resource allocation (Furness et al., 2019; Whittington et al., 2022). In both birds and teleost fish, the absence of an orthologue of DNMT3L, a key methylation enzyme in mammals, supports the absence of imprinting. Analyses carried out on whole embryos, adult brains or embryonic tissues always concluded that imprinting was absent in chicken (Frésard et al., 2013). Conversely, studies performed in fish are more contradictory, with Ma et al. showing the ntl gene as differentially methylated between sperm and egg in goldfish and a differential timing of transcription between paternal and maternal alleles in the embryo (Ma et al., 2011). Recent data from both laboratory and cultured teleost fish suggest the possibility that some imprinted genes, such as IGF2 and DLK1, present homologues subjected to imprinting-like patterns outside mammals (Du et al., 2022; Zhang et al., 2023), highlighting the need for further research on this topic.
Improved annotation of livestock genomes supported by international initiatives such as FAANG (The FAANG Consortium et al., 2015) and FarmGTEx (https://www.farmgtex.org/) should allow building a more comprehensive phylogenetic tree of imprinting evolution, including investigating potential early differentiation in some clades. As an example, assessment of specific imprinted genes in cattle and pigs showed that SLC38A4 appeared to be species-specific, with a non-imprinted pattern in cattle in all adult tissues evaluated (Zaitoun and Khatib, 2006) compared to mice, which harbor tissue-specific imprinting (Zaitoun and Khatib, 2006). Moreover, a fine-tuned pattern of imprinting has been shown for paternally-expressed NAP1L5, with an expression distribution in pig tissues differing from those in mice and cattle (Jiang et al., 2011). Genome-wide comparative analyses suggest that changes in methylation are a potentially important source for shaping lineage- and species-specific innovations, as exemplified with imprinting (Hu et al., 2023). Livestock mammals such as cattle and pigs display epigenomic-specific features that make them particularly attractive to investigate the contribution of imprinting and epigenomic evolution to phenotypic variation (Lu et al., 2021).
Several traits of agronomic interest overlap with major features of imprinted genes, particularly due to their known role in growth, development and behavioral processes. Although molecular tools targeting imprinting in livestock still need to be developed (Hubert et al., 2024), experimental livestock populations may help to better understand how imprinted genes affect performance (Figure 2). Not only do these populations display a broad range of relevant phenotypes for imprinting (Hinde et al., 2014; Gilbert et al., 2017; Khanal et al., 2017; Canario et al., 2022), but also they allow access to a full pedigree with parental origins either through nuclear family datasets (Khanal et al., 2017; Canario et al., 2022) or divergent lines selected over several generations (Gilbert et al., 2017). Thus, considering parental origins in future genome-wide association studies performed in livestock would enable significant advances on the functional impact of imprinted genes in the variability of important traits.
Apart from the bovine LOS that mimics BWS (Rivera et al., 2022), other livestock species such as pigs might constitute relevant pathophysiological models for imprinted disorders. In mice, targeted mutagenesis has provided highly valuable clues on the mechanisms involved in the regulation of imprinting. However, mouse models and patient data show discrepancies on both growth traits and inheritance patterns (Shmela and Gicquel, 2013), suggesting that mice do not seem appropriate for further analysis of pathophysiology for therapeutic purposes, making pigs a potential alternative model for imprinted disorders. Indeed, pigs are considered as one of the best animal generators of human disease models, because they share similar features with humans in physiology, anatomy and genome organisation (Groenen et al., 2012). The emergence of the CRISPR/Cas9 genome editing technology is revolutionizing porcine genome engineering (Yao et al., 2016). Recent studies have introduced epigenome-editing strategies which target enzymatic activity to introduce or remove an epigenetic mark at a defined genomic site (Kungulovski and Jeltsch, 2016). Given the crucial role of ICRs into the regulation of local imprinted genes and the disruption of DNA methylation observed there in various imprinted disorders (Soellner et al., 2017), epigenome-editing technologies might be applied to perturb DNA methylation at a dedicated ICR in an allele-specific manner to mimic a specific disorder Supplementary Figure S1. Epigenome-edited pigs might be promising pathophysiological animal models to investigate the long-term dynamics of imprinted disorders.
Author contributions
J-NH: Visualization, Writing–original draft, Writing–review and editing. MP: Writing–review and editing. JR: Supervision, Writing–review and editing. JD: Funding acquisition, Supervision, Writing–original draft, Writing–review and editing, Visualization.
Funding
The author(s) declare financial support was received for the research, authorship, and/or publication of this article. J-NH is funded by ANR PIPETTE (ANR-18-CE20-0018) and the Animal Genetics division of INRAE. MP has a PhD grant funded by INRAE.
Conflict of interest
The authors declare that the research was conducted in the absence of any commercial or financial relationships that could be construed as a potential conflict of interest.
Publisher’s note
All claims expressed in this article are solely those of the authors and do not necessarily represent those of their affiliated organizations, or those of the publisher, the editors and the reviewers. Any product that may be evaluated in this article, or claim that may be made by its manufacturer, is not guaranteed or endorsed by the publisher.
Supplementary Material
The Supplementary Material for this article can be found online at: https://www.frontiersin.org/articles/10.3389/fcell.2024.1348036/full#supplementary-material
Supplementary Figure 1 | Pathophysiological pig model for Silver Russel Syndrome. Silver Russel Syndrome is an imprinted disorder mainly characterized by individuals being born small for gestational age, with relative macrocephaly at birth. The major molecular abnormality of Silver Russel Syndrome is a loss of methylation at the Imprinting Control Center 1 (ICR1) leading to a loss of IGF2 expression from the paternal allele and a biallelic expression of H19. (A) Principle of epigenome targeting technology applied to imprinting loci. Schematic representation of a catalytically inactive mutant Cas9 (dCas9) fused with Tet1 for erasing DNA methylation and with Dnmt3a for de novo methylation of specific sequences. (B) Schematic representation of the IGF2/H19 imprinting region associated with Beckwith-Wiedemann (excessive growth) and Silver-Russel (growth retardation). (C) The polymorphism is exploited to specifically target the paternal allele with the single guide RNA (sgRNA). The system coupled to Tet1 will remove the DNA methylation from the paternal ICR1. This will result to a loss of methylation at ICR1 which is the most common epimutation in Silver-Russell patients. (D) Generation of offspring with a loss of methylation at ICR1 that could mimic the Silver-Russel Syndrome. SNP: single nucleotide polymorphism.
References
Ahn, J., Hwang, I. S., Park, M. R., Cho, I. C., Hwang, S., and Lee, K. (2020). The landscape of genomic imprinting at the porcine SGCE/PEG10 locus from methylome and transcriptome of parthenogenetic embryos. G3 GenesGenomesGenetics 10, 4037–4047. doi:10.1534/g3.120.401425
Ahn, J., Hwang, I.-S., Park, M.-R., Hwang, S., and Lee, K. (2023). Imprinting at the KBTBD6 locus involves species-specific maternal methylation and monoallelic expression in livestock animals. J. Anim. Sci. Biotechnol. 14, 131. doi:10.1186/s40104-023-00931-3
Ahn, J., Lee, J., Kim, D. H., Hwang, I. S., Park, M. R., Cho, I. C., et al. (2022). Loss of monoallelic expression of IGF2 in the adult liver via alternative promoter usage and chromatin reorganization. Front. Genet. 13, 920641. doi:10.3389/fgene.2022.920641
Ai, H., Ren, J., Zhang, Z., Ma, J., Guo, Y., Yang, B., et al. (2012). Detection of quantitative trait loci for growth- and fatness-related traits in a large-scale White Duroc × Erhualian intercross pig population. Anim. Genet. 43, 383–391. doi:10.1111/j.1365-2052.2011.02282.x
Akbari, V., Hanlon, V. C. T., O'Neill, K., Lefebvre, L., Schrader, K. A., Lansdorp, P. M., et al. (2023). Parent-of-origin detection and chromosome-scale haplotyping using long-read DNA methylation sequencing and Strand-seq. Cell Genomics 3, 100233. doi:10.1016/j.xgen.2022.100233
Andergassen, D., Dotter, C. P., Wenzel, D., Sigl, V., Bammer, P. C., Muckenhuber, M., et al. (2017). Mapping the mouse Allelome reveals tissue-specific regulation of allelic expression. eLife 6, e25125. doi:10.7554/eLife.25125
Banville, M., Riquet, J., Bahon, D., Sourdioux, M., and Canario, L. (2015). Genetic parameters for litter size, piglet growth and sow’s early growth and body composition in the Chinese–European line Tai Zumu. J. Anim. Breed. Genet. 132, 328–337. doi:10.1111/jbg.12122
Behboodi, E., Anderson, G. B., BonDurant, R. H., Cargill, S. L., Kreuscher, B. R., Medrano, J. F., et al. (1995). Birth of large calves that developed from in vitro-derived bovine embryos. Theriogenology 44, 227–232. doi:10.1016/0093-691x(95)00172-5
Berkowicz, E. W., Magee, D. A., Sikora, K. M., Berry, D. P., Howard, D. J., Mullen, M. P., et al. (2011). Single nucleotide polymorphisms at the imprinted bovine insulin-like growth factor 2 (IGF2) locus are associated with dairy performance in Irish Holstein-Friesian cattle. J. Dairy Res. 78, 1–8. doi:10.1017/S0022029910000567
Bischoff, S. R., Tsai, S., Hardison, N., Motsinger-Reif, A. A., Freking, B. A., Nonneman, D., et al. (2009). Characterization of conserved and nonconserved imprinted genes in swine. Biol. Reprod. 81, 906–920. doi:10.1095/biolreprod.109.078139
Blagitko, N., Mergenthaler, S., Schulz, U., Wollmann, H. A., Craigen, W., Eggermann, T., et al. (2000). Human GRB10 is imprinted and expressed from the paternal and maternal allele in a highly tissue- and isoform-specific fashion. Hum. Mol. Genet. 9, 1587–1595. doi:10.1093/hmg/9.11.1587
Brioude, F., Kalish, J. M., Mussa, A., Foster, A. C., Bliek, J., Ferrero, G. B., et al. (2018). Expert consensus document: clinical and molecular diagnosis, screening and management of Beckwith-Wiedemann syndrome: an international consensus statement. Nat. Rev. Endocrinol. 14, 229–249. doi:10.1038/nrendo.2017.166
Bruscadin, J. J., Cardoso, T. F., da Silva Diniz, W. J., Afonso, J., de Souza, M. M., Petrini, J., et al. (2022). Allele-specific expression reveals functional SNPs affecting muscle-related genes in bovine. Biochim. Biophys. Acta BBA - Gene Regul. Mech. 1865, 194886. doi:10.1016/j.bbagrm.2022.194886
Canario, L., Billon, Y., Hébrard, W., and Demars, J. (2022). “Genetics of body weight and length of Large White piglets and relationship to maternal performance,” in Proceedings of 12th World Congress on Genetics Applied to Livestock Production (WCGALP) (Wageningen Academic Publishers), 532–535. doi:10.3920/978-90-8686-940-4_121
Carli, D., Riberi, E., Ferrero, G. B., and Mussa, A. (2020). Syndromic disorders caused by disturbed human imprinting. Jcrpe 12, 1–16. doi:10.4274/jcrpe.galenos.2019.2018.0249
Cattanach, B. M. (1986). Parental origin effects in mice. Development 97, 137–150. doi:10.1242/dev.97.supplement.137
Cavani, L., Brown, W. E., Parker Gaddis, K. L., Tempelman, R. J., VandeHaar, M. J., White, H. M., et al. (2022). Estimates of genetic parameters for feeding behavior traits and their associations with feed efficiency in Holstein cows. J. Dairy Sci. 105, 7564–7574. doi:10.3168/jds.2022-22066
Cellier, M., Duvaux-Ponter, C., and Nielsen, B. L. (2021). Inter- and intra-individual variability of feeding behaviour in group housed dairy goats. Appl. Anim. Behav. Sci. 234, 105167. doi:10.1016/j.applanim.2020.105167
Chavatte-Palmer, P., and Tarrade, A. (2016). Placentation in different mammalian species. Ann. Endocrinol. 77, 67–74. doi:10.1016/j.ando.2016.04.006
Chen, Z., Hagen, D. E., Wang, J., Elsik, C. G., Ji, T., Siqueira, L. G., et al. (2016). Global assessment of imprinted gene expression in the bovine conceptus by next generation sequencing. Epigenetics 11, 501–516. doi:10.1080/15592294.2016.1184805
Chen, Z., Robbins, K. M., Wells, K. D., and Rivera, R. M. (2013). Large offspring syndrome: a bovine model for the human loss-of-imprinting overgrowth syndrome Beckwith-Wiedemann. Epigenetics 8, 591–601. doi:10.4161/epi.24655
Cheong, C. Y., Chng, K., Ng, S., Chew, S. B., Chan, L., and Ferguson-Smith, A. C. (2015). Germline and somatic imprinting in the nonhuman primate highlights species differences in oocyte methylation. Genome Res. 25, 611–623. doi:10.1101/gr.183301.114
Chu, C., Zhang, W., Kang, Y., Ji, W., Niu, Y., and Zhang, Y. (2021). Analysis of developmental imprinting dynamics in primates using SNP-free methods to identify imprinting defects in cloned placenta. Dev. Cell 56, 2826–2840.e7. doi:10.1016/j.devcel.2021.09.012
Cockett, N. E., Jackson, S. P., Shay, T. L., Farnir, F., Berghmans, S., Snowder, G. D., et al. (1996). Polar overdominance at the ovine callipyge locus. Science 273, 236–238. doi:10.1126/science.273.5272.236
Congras, A., Yerle-Bouissou, M., Pinton, A., Vignoles, F., Liaubet, L., Ferchaud, S., et al. (2014). Sperm DNA methylation analysis in swine reveals conserved and species-specific methylation patterns and highlights an altered methylation at the GNAS locus in infertile boars. Biol. Reprod. 91, 137. doi:10.1095/biolreprod.114.119610
Cowley, M., Garfield, A. S., Madon-Simon, M., Charalambous, M., Clarkson, R. W., Smalley, M. J., et al. (2014). Developmental programming mediated by complementary roles of imprinted Grb10 in mother and pup. PLoS Biol. 12, e1001799. doi:10.1371/journal.pbio.1001799
Cui, X., Hou, Y., Yang, S., Xie, Y., Zhang, S., Zhang, Y., et al. (2014). Transcriptional profiling of mammary gland in Holstein cows with extremely different milk protein and fat percentage using RNA sequencing. BMC Genomics 15, 226. doi:10.1186/1471-2164-15-226
Curley, J. P., Barton, S., Surani, A., and Keverne, E. B. (2004). Coadaptation in mother and infant regulated by a paternally expressed imprinted gene. Proc. R. Soc. Lond B 271, 1303–1309. doi:10.1098/rspb.2004.2725
Daigneault, B. W. (2022). Insights to maternal regulation of the paternal genome in mammalian livestock embryos: a mini-review. Front. Genet. 13, 909804. doi:10.3389/fgene.2022.909804
Davis, E., Jensen, C. H., Schroder, H. D., Farnir, F., Shay-Hadfield, T., Kliem, A., et al. (2004). Ectopic expression of DLK1 protein in skeletal muscle of padumnal heterozygotes causes the callipyge phenotype. Curr. Biol. 14, 1858–1862. doi:10.1016/j.cub.2004.09.079
Ding, R., Yang, M., Wang, X., Quan, J., Zhuang, Z., Zhou, S., et al. (2018). Genetic architecture of feeding behavior and feed efficiency in a duroc pig population. Front. Genet. 9, 220. doi:10.3389/fgene.2018.00220
Do, D. N., Strathe, A. B., Ostersen, T., Jensen, J., Mark, T., and Kadarmideen, H. N. (2013). Genome-wide association study reveals genetic architecture of eating behavior in pigs and its implications for humans obesity by comparative mapping. PLoS ONE 8, e71509. doi:10.1371/journal.pone.0071509
Dong, Y., Xie, M., Jiang, Y., Xiao, N., Du, X., Zhang, W., et al. (2013). Sequencing and automated whole-genome optical mapping of the genome of a domestic goat (Capra hircus). Nat. Biotechnol. 31, 135–141. doi:10.1038/nbt.2478
Du, K., Pippel, M., Kneitz, S., Feron, R., da Cruz, I., Winkler, S., et al. (2022). Genome biology of the darkedged splitfin, Girardinichthys multiradiatus, and the evolution of sex chromosomes and placentation. Genome Res. 32, 583–594. doi:10.1101/gr.275826.121
Duan, J., Ellie, S., Flock, K., Seesi, S. A., Mandoiu, I., Jones, A., et al. (2018). Effects of maternal nutrition on the expression of genomic imprinted genes in ovine fetuses. Epigenetics 13, 793–807. doi:10.1080/15592294.2018.1503489
Edwards, C. A., Watkinson, W. M. D., Telerman, S. B., Hulsmann, L. C., Hamilton, R. S., and Ferguson-Smith, A. C. (2023). Reassessment of weak parent-of-origin expression bias shows it rarely exists outside of known imprinted regions. eLife 12, e83364. doi:10.7554/eLife.83364
Elbracht, M., Binder, G., Hiort, O., Kiewert, C., Kratz, C., and Eggermann, T. (2020). Clinical spectrum and management of imprinting disorders. Med. Genet. 32, 321–334. doi:10.1515/medgen-2020-2044
Fan, Z., Wu, Z., Wang, L., Zou, Y., Zhang, P., and You, F. (2016). Characterization of embryo transcriptome of gynogenetic olive flounder Paralichthys olivaceus. Mar. Biotechnol. 18, 545–553. doi:10.1007/s10126-016-9716-6
Feil, R., Khosla, S., Cappai, P., and Loi, P. (1998). Genomic imprinting in ruminants: allele-specific gene expression in parthenogenetic sheep. Mamm. Genome 9, 831–834. doi:10.1007/s003359900876
Ferguson-Smith, A. C., and Bourchis, D. (2018). The discovery and importance of genomic imprinting. eLife 7, e42368. doi:10.7554/eLife.42368
Filippi, G., and Mckusick, V. A. (1970). THE BECKWITH-WIEDEMANN SYNDROME: THE EXOMPHALOS-MACROGLOSSIA-GIGANTISM SYNDROME. Med. Baltim. 49, 279–298. doi:10.1097/00005792-197007000-00002
Freking, B. A., Murphy, S. K., Wylie, A. A., Rhodes, S. J., Keele, J. W., Leymaster, K. A., et al. (2002). Identification of the single base change causing the callipyge muscle hypertrophy phenotype, the only known example of polar overdominance in mammals. Genome Res. 12, 1496–1506. doi:10.1101/gr.571002
Frésard, L., Leroux, S., Servin, B., Gourichon, D., Dehais, P., Cristobal, M. S., et al. (2014). Transcriptome-wide investigation of genomic imprinting in chicken. Nucleic Acids Res. 42, 3768–3782. doi:10.1093/nar/gkt1390
Frésard, L., Morisson, M., Brun, J. M., Collin, A., Pain, B., Minvielle, F., et al. (2013). Epigenetics and phenotypic variability: some interesting insights from birds. Genet. Sel. Evol. 45, 16. doi:10.1186/1297-9686-45-16
Fu, L., Jiang, Y., Wang, C., Mei, M., Zhou, Z., Jiang, Y., et al. (2020). A genome-wide association study on feed efficiency related traits in landrace pigs. Front. Genet. 11, 692. doi:10.3389/fgene.2020.00692
Furness, A. I., Pollux, B. J. A., Meredith, R. W., Springer, M. S., and Reznick, D. N. (2019). How conflict shapes evolution in poeciliid fishes. Nat. Commun. 10, 3335. doi:10.1038/s41467-019-11307-5
Gilbert, H., Billon, Y., Brossard, L., Faure, J., Gatellier, P., Gondret, F., et al. (2017). Review: divergent selection for residual feed intake in the growing pig. Animal 11, 1427–1439. doi:10.1017/S175173111600286X
Giuffra, E., Tuggle, C. K., and Faang, C. (2019). Functional annotation of animal genomes (FAANG): current achievements and roadmap. Annu. Rev. Anim. Biosci. 7, 65–88. doi:10.1146/annurev-animal-020518-114913
Goldkamp, A. K., Li, Y., Rivera, R. M., and Hagen, D. E. (2022). Characterization of tRNA expression profiles in large offspring syndrome. BMC Genomics 23, 273. doi:10.1186/s12864-022-08496-7
Greally, J. M., Starr, D. J., Hwang, S., Song, L., Jaarola, M., and Zemel, S. (1998). The mouse H19 locus mediates a transition between imprinted and non-imprinted DNA replication patterns. Hum. Mol. Genet. 7, 91–95. doi:10.1093/hmg/7.1.91
Groenen, M. A. M., Archibald, A. L., Uenishi, H., Tuggle, C. K., Takeuchi, Y., Rothschild, M. F., et al. (2012). Analyses of pig genomes provide insight into porcine demography and evolution. Nature 491, 393–398. doi:10.1038/nature11622
Guo, Y. M., Lee, G. J., Archibald, A. L., and Haley, C. S. (2008). Quantitative trait loci for production traits in pigs: a combined analysis of two Meishan x Large White populations. Anim. Genet. 39, 486–495. doi:10.1111/j.1365-2052.2008.01756.x
Hall, J. G. (1990). Genomic imprinting: review and relevance to human diseases. Am. J. Hum. Genet. 46, 857–873.
Hanin, G., and Ferguson-Smith, A. C. (2020). The evolution of genomic imprinting: epigenetic control of mammary gland development and postnatal resource control. WIREs Mech. Dis. 12, e1476. doi:10.1002/wsbm.1476
Hatada, I., Inazawa, J., Abe, T., Nakayama, M., Kaneko, Y., Jinno, Y., et al. (1996). Genomic imprinting of human p57KIP2 and its reduced expression in Wilms’ tumors. Hum. Mol. Genet. 5, 783–788. doi:10.1093/hmg/5.6.783
Hatada, L., Sugama, T., and Mukai, T. (1993). A new imprinted gene cloned by a methylation-sensitive genome scanning method. Nucleic Acids Res. 21, 5577–5582. doi:10.1093/nar/21.24.5577
Hayes, B. J., Bowman, P. J., Chamberlain, A. J., and Goddard, M. E. (2009). Invited review: genomic selection in dairy cattle: progress and challenges. J. Dairy Sci. 92, 433–443. doi:10.3168/jds.2008-1646
Hayes, B. J., Pryce, J., Chamberlain, A. J., Bowman, P. J., and Goddard, M. E. (2010). Genetic architecture of complex traits and accuracy of genomic prediction: coat colour, milk-fat percentage, and type in Holstein cattle as contrasting model traits. PLoS Genet. 6, e1001139. doi:10.1371/journal.pgen.1001139
Hinde, K., Carpenter, A. J., Clay, J. S., and Bradford, B. J. (2014). Holsteins favor heifers, not bulls: biased milk production programmed during pregnancy as a function of fetal sex. PLoS ONE 9, e86169. doi:10.1371/journal.pone.0086169
Ho-Shing, O., and Dulac, C. (2019). Influences of genomic imprinting on brain function and behavior. Curr. Opin. Behav. Sci. 25, 66–76. doi:10.1016/j.cobeha.2018.08.008
Hu, Y., Yuan, S., Du, X., Liu, J., Zhou, W., and Wei, F. (2023). Comparative analysis reveals epigenomic evolution related to species traits and genomic imprinting in mammals. Innovation 4, 100434. doi:10.1016/j.xinn.2023.100434
Hu, Z.-L., Park, C. A., Wu, X.-L., and Reecy, J. M. (2013). Animal QTLdb: an improved database tool for livestock animal QTL/association data dissemination in the post-genome era. Nucleic Acids Res. 41, D871–D879. doi:10.1093/nar/gks1150
Hubert, J.-N., and Demars, J. (2022). Genomic imprinting in the new omics era: a model for systems-level approaches. Front. Genet. 13, 838534. doi:10.3389/fgene.2022.838534
Hubert, J.-N., Lannuccelli, N., Cabau, C., Jacomet, J.-E., Billon, Y., Serre, R.-F., et al. (2024). Detection of DNA methylation signatures through the lens of genomic imprinting. Scientific Reports 14, 1694. doi:10.1038/s41598-024-52114-3
Humphray, S. J., Scott, C. E., Clark, R., Marron, B., Bender, C., Camm, N., et al. (2007). A high utility integrated map of the pig genome. Genome Biol. 8, R139. doi:10.1186/gb-2007-8-7-r139
Ibáñez-Escriche, N., Forni, S., Noguera, J. L., and Varona, L. (2014). Genomic information in pig breeding: science meets industry needs. Livest. Sci. 166, 94–100. doi:10.1016/j.livsci.2014.05.020
Inoue, A. (2023). Noncanonical imprinting: intergenerational epigenetic inheritance mediated by Polycomb complexes. Curr. Opin. Genet. Dev. 78, 102015. doi:10.1016/j.gde.2022.102015
Jeon, J.-T., Carlborg, O., Törnsten, A., Giuffra, E., Amarger, V., Chardon, P., et al. (1999). A paternally expressed QTL affecting skeletal and cardiac muscle mass in pigs maps to the IGF2 locus. Nat. Genet. 21, 157–158. doi:10.1038/5938
Jiang, C. D., Li, S., and Deng, C. Y. (2011). Assessment of genomic imprinting of PPP1R9A, NAP1L5 and PEG3 in pigs. Russ. J. Genet. 47, 471–476. doi:10.1134/s1022795411040053
Jima, D. D., Skaar, D. A., Planchart, A., Motsinger-Reif, A., Cevik, S. E., Park, S. S., et al. (2022). Genomic map of candidate human imprint control regions: the imprintome. Epigenetics 17, 1920–1943. doi:10.1080/15592294.2022.2091815
Juliusdottir, T., Steinthorsdottir, V., Stefansdottir, L., Sveinbjornsson, G., Ivarsdottir, E. V., Thorolfsdottir, R. B., et al. (2021). Distinction between the effects of parental and fetal genomes on fetal growth. Nat. Genet. 53, 1135–1142. doi:10.1038/s41588-021-00896-x
Kalscheuer, V. M., Mariman, E. C., Schepens, M. T., Rehder, H., and Ropers, H.-H. (1993). The insulin–like growth factor type–2 receptor gene is imprinted in the mouse but not in humans. Nat. Genet. 5, 74–78. doi:10.1038/ng0993-74
Kaneko-Ishino, T., and Ishino, F. (2022). The evolutionary advantage in mammals of the complementary monoallelic expression mechanism of genomic imprinting and its emergence from a defense against the insertion into the host genome. Front. Genet. 13, 832983. doi:10.3389/fgene.2022.832983
Kenny, D., Sleator, R. D., Murphy, C. P., Evans, R. D., and Berry, D. P. (2022). Detection of genomic imprinting for carcass traits in cattle using imputed high-density genotype data. Front. Genet. 13, 951087. doi:10.3389/fgene.2022.951087
Kern, C., Wang, Y., Xu, X., Pan, Z., Halstead, M., Chanthavixay, G., et al. (2021). Functional annotations of three domestic animal genomes provide vital resources for comparative and agricultural research. Nat. Commun. 12, 1821. doi:10.1038/s41467-021-22100-8
Khanal, P., and Nielsen, M. O. (2017). Impacts of prenatal nutrition on animal production and performance: a focus on growth and metabolic and endocrine function in sheep. J. Anim. Sci. Biotechnol. 8, 75. doi:10.1186/s40104-017-0205-1
Killian, J. K., Nolan, C. M., Stewart, N., Munday, B. L., Andersen, N. A., Nicol, S., et al. (2001). Monotreme IGF2 expression and ancestral origin of genomic imprinting. J. Exp. Zool. 291, 205–212. doi:10.1002/jez.1070
Kungulovski, G., and Jeltsch, A. (2016). Epigenome editing: state of the art, concepts, and perspectives. Trends Genet. 32, 101–113. doi:10.1016/j.tig.2015.12.001
Lawson, H. A., Cheverud, J. M., and Wolf, J. B. (2013). Genomic imprinting and parent-of-origin effects on complex traits. Nat. Rev. Genet. 14, 609–617. doi:10.1038/nrg3543
Lean, S. C., Candia, A. A., Gulacsi, E., Lee, G. C. L., and Sferruzzi-Perri, A. N. (2022). Obesogenic diet in mice compromises maternal metabolic physiology and lactation ability leading to reductions in neonatal viability. Acta Physiol. 236, e13861. doi:10.1111/apha.13861
Lee, J. E., Tantravahi, U., Boyle, A. L., and Efstratiadis, A. (1993). Parental imprinting of an IGF-2 transgene. Mol. Reprod. Dev. 35, 382–390. doi:10.1002/mrd.1080350411
Legarra, A., Calenge, F., Mariani, P., Velge, P., and Beaumont, C. (2011). Use of a reduced set of single nucleotide polymorphisms for genetic evaluation of resistance to Salmonella carrier state in laying hens. Poult. Sci. 90, 731–736. doi:10.3382/ps.2010-01260
Li, E., Beard, C., and Jaenisch, R. (1993). Role for DNA methylation in genomic imprinting. Nature 366, 362–365. doi:10.1038/366362a0
Li, J., Yu, D., Wang, J., Li, C., Wang, Q., Wang, J., et al. (2022a). Identification of the porcine IG-DMR and abnormal imprinting of DLK1-DIO3 in cloned pigs. Front. Cell Dev. Biol. 10, 964045. doi:10.3389/fcell.2022.964045
Li, Y., Boadu, F., Highsmith, M. R., Hagen, D. E., Cheng, J., and Rivera, R. M. (2022b). Allele-specific aberration of imprinted domain chromosome architecture associates with large offspring syndrome. iScience 25, 104269. doi:10.1016/j.isci.2022.104269
Li, Y., Hagen, D. E., Ji, T., Bakhtiarizadeh, M. R., Frederic, W. M., Traxler, E. M., et al. (2019). Altered microRNA expression profiles in large offspring syndrome and Beckwith-Wiedemann syndrome. Epigenetics 14, 850–876. doi:10.1080/15592294.2019.1615357
Llères, D., Imaizumi, Y., and Feil, R. (2021). Exploring chromatin structural roles of non-coding RNAs at imprinted domains. Biochem. Soc. Trans. 49, 1867–1879. doi:10.1042/BST20210758
Lu, X., Zhang, Y., Wang, L., Wang, L., Wang, H., Xu, Q., et al. (2021). Evolutionary epigenomic analyses in mammalian early embryos reveal species-specific innovations and conserved principles of imprinting. Sci. Adv. 7, eabi6178. doi:10.1126/sciadv.abi6178
Luedi, P. P., Dietrich, F. S., Weidman, J. R., Bosko, J. M., Jirtle, R. L., and Hartemink, A. J. (2007). Computational and experimental identification of novel human imprinted genes. Genome Res. 17, 1723–1730. doi:10.1101/gr.6584707
Luedi, P. P., Hartemink, A. J., and Jirtle, R. L. (2005). Genome-wide prediction of imprinted murine genes. Genome Res. 15, 875–884. doi:10.1101/gr.3303505
Ma, S., Huang, W., Zhang, L., Zhao, S., Tong, Y., Liu, Z., et al. (2011). Germ cell-specific DNA methylation and genome diploidization in primitive vertebrates. Epigenetics 6, 1471–1480. doi:10.4161/epi.6.12.18177
MacDonald, W. A., and Mann, M. R. W. (2020). Long noncoding RNA functionality in imprinted domain regulation. PLOS Genet. 16, e1008930. doi:10.1371/journal.pgen.1008930
Magee, D. A., Spillane, C., Berkowicz, E. W., Sikora, K. M., and MacHugh, D. E. (2014). Imprinted loci in domestic livestock species as epigenomic targets for artificial selection of complex traits. Anim. Genet. 45 (Suppl. 1), 25–39. doi:10.1111/age.12168
Magnus, P., Gjessing, H. K., Skrondal, A., and Skjaerven, R. (2001). Paternal contribution to birth weight. J. Epidemiol. Community Health 55, 873–877. doi:10.1136/jech.55.12.873
Malnou, E. C., Umlauf, D., Mouysset, M., and Cavaillé, J. (2019). Imprinted MicroRNA gene clusters in the evolution, development, and functions of mammalian placenta. Front. Genet. 9, 706. doi:10.3389/fgene.2018.00706
Mangiavacchi, P. M., Caldas-Bussiere, M. C., Mendonça, M., Dias, A. J. B., and Rios, Á. F. L. (2021). Multi-locus imprinting disturbances of Beckwith-Wiedemann and Large offspring syndrome/Abnormal offspring syndrome: a brief review. Theriogenology 173, 193–201. doi:10.1016/j.theriogenology.2021.08.005
Markljung, E., Jiang, L., Jaffe, J. D., Mikkelsen, T. S., Wallerman, O., Larhammar, M., et al. (2009). ZBED6, a novel transcription factor derived from a domesticated DNA transposon regulates IGF2 expression and muscle growth. PLoS Biol. 7, e1000256. doi:10.1371/journal.pbio.1000256
Momoko, H., Robin, N. B., Felix, R. D., Nicole, M. W., Kooijman, M. N., Fernandez-Tajes, J., et al. (2016). Genome-wide associations for birth weight and correlations with adult disease. Nature 538, 248–252. doi:10.1038/nature19806
Monk, D. (2015). Genomic imprinting in the human placenta. Am. J. Obstet. Gynecol. 213, S152–S162. doi:10.1016/j.ajog.2015.06.032
Monk, D., Arnaud, P., Apostolidou, S., Hills, F. A., Kelsey, G., Stanier, P., et al. (2006). Limited evolutionary conservation of imprinting in the human placenta. Proc. Natl. Acad. Sci. U. S. A. 103, 6623–6628. doi:10.1073/pnas.0511031103
Monk, D., Mackay, D. J. G., Eggermann, T., Maher, E. R., and Riccio, A. (2019). Genomic imprinting disorders: lessons on how genome, epigenome and environment interact. Nat. Rev. Genet. 20, 235–248. doi:10.1038/s41576-018-0092-0
Murphy, S. K., Nolan, C. M., Huang, Z., Kucera, K. S., Freking, B. A., Smith, T. P. L., et al. (2006). Callipyge mutation affects gene expression in cis: a potential role for chromatin structure. Genome Res. 16, 340–346. doi:10.1101/gr.4389306
Murphy, S. K., Wylie, A. A., and Jirtle, R. L. (2001). Imprinting of PEG3, the human homologue of a mouse gene involved in nurturing behavior. Genomics 71, 110–117. doi:10.1006/geno.2000.6419
Nezer, C., Moreau, L., Brouwers, B., Coppieters, W., Detilleux, J., Hanset, R., et al. (1999). An imprinted QTL with major effect on muscle mass and fat deposition maps to the IGF2 locus in pigs. Nat. Genet. 21, 155–156. doi:10.1038/5935
Noordermeer, D., and Feil, R. (2020). Differential 3D chromatin organization and gene activity in genomic imprinting. Curr. Opin. Genet. Dev. 61, 17–24. doi:10.1016/j.gde.2020.03.004
O'Doherty, A. M., MacHugh, D. E., Spillane, C., and Magee, D. A. (2015). Genomic imprinting effects on complex traits in domesticated animal species. Front. Genet. 6, 156. doi:10.3389/fgene.2015.00156
O'Neill, M. J., Ingram, R. S., Vrana, P. B., and Tilghman, S. M. (2000). Allelic expression of IGF2 in marsupials and birds. Dev. Genes Evol. 210, 18–20. doi:10.1007/pl00008182
Pan, Z., Wang, Y., Wang, M., Wang, Y., Zhu, X., Gu, S., et al. (2023). An atlas of regulatory elements in chicken: a resource for chicken genetics and genomics. Sci. Adv. 9, eade1204. doi:10.1126/sciadv.ade1204
Peters, J. (2014). The role of genomic imprinting in biology and disease: an expanding view. Nat. Rev. Genet. 15, 517–530. doi:10.1038/nrg3766
Poulsen, N. A., and Bach Larsen, L. (2022). Genetic factors affecting the composition and quality of cow’s milk. Burleigh Dodds Ser. Agric. Sci., 501–532. doi:10.19103/AS.2022.0099.15
Raas, M. W. D., Zijlmans, D. W., Vermeulen, M., and Marks, H. (2022). There is another: H3K27me3-mediated genomic imprinting. Trends Genet. 38, 82–96. doi:10.1016/j.tig.2021.06.017
Reik, W., and Walter, J. (2001). Genomic imprinting: parental influence on the genome. Nat. Rev. Genet. 2, 21–32. doi:10.1038/35047554
Reiner, G., Köhler, F., Berge, T., Fischer, R., Hübner-Weitz, K., Scholl, J., et al. (2009). Mapping of quantitative trait loci affecting behaviour in swine. Anim. Genet. 40, 366–376. doi:10.1111/j.1365-2052.2008.01847.x
Rice, F., and Thapar, A. (2010). Estimating the relative contributions of maternal genetic, paternal genetic and intrauterine factors to offspring birth weight and head circumference. Early Hum. Dev. 86, 425–432. doi:10.1016/j.earlhumdev.2010.05.021
Richard Albert, J., Kobayashi, T., Inoue, A., Monteagudo-Sánchez, A., Kumamoto, S., Takashima, T., et al. (2023). Conservation and divergence of canonical and non-canonical imprinting in murids. Genome Biol. 24, 48. doi:10.1186/s13059-023-02869-1
Rivera, R. M., Donnelly, C. G., Patel, B. N., Li, Y., and Soto-Moreno, E. J. (2021). Abnormal offspring syndrome. Bov. Reprod., 876–895. doi:10.1002/9781119602484.ch71
Rivera, R. M., Goldkamp, A. K., Patel, B. N., Hagen, D. E., Soto-Moreno, E. J., Li, Y., et al. (2022). Identification of large offspring syndrome during pregnancy through ultrasonography and maternal blood transcriptome analyses. Sci. Rep. 12, 10540. doi:10.1038/s41598-022-14597-w
Rodríguez-González, G. L., Bautista, C. J., Rojas-Torres, K. I., Nathanielsz, P. W., and Zambrano, E. (2020). Importance of the lactation period in developmental programming in rodents. Nutr. Rev. 78, 32–47. doi:10.1093/nutrit/nuaa041
Rutkowska, K., and Lukaszewicz, M. (2019). Alterations to DNA structure as a cause of expression modifications of selected genes of known intrauterine-growth-restriction-association shared by chosen species — a review. Anim. Genet. 50, 613–620. doi:10.1111/age.12861
Sanli, I., and Feil, R. (2015). Chromatin mechanisms in the developmental control of imprinted gene expression. Int. J. Biochem. Cell Biol. 67, 139–147. doi:10.1016/j.biocel.2015.04.004
Sellner, E. M., Kim, J. W., McClure, M. C., Taylor, K. H., Schnabel, R. D., and Taylor, J. F. (2007). Board-invited review: applications of genomic information in livestock. J. Anim. Sci. 85, 3148–3158. doi:10.2527/jas.2007-0291
Shmela, M. E., and Gicquel, C. F. (2013). Human diseases versus mouse models: insights into the regulation of genomic imprinting at the human 11p15/mouse distal chromosome 7 region. J. Med. Genet. 50, 11–20. doi:10.1136/jmedgenet-2012-101321
Smit, M., Segers, K., Carrascosa, L. G., Shay, T., Baraldi, F., Gyapay, G., et al. (2003). Mosaicism of solid gold supports the causality of a noncoding A-to-G transition in the determinism of the callipyge phenotype. Genetics 163, 453–456. doi:10.1093/genetics/163.1.453
Soellner, L., Begemann, M., Mackay, D. J. G., Grønskov, K., Tümer, Z., Maher, E. R., et al. (2017). Recent advances in imprinting disorders: recent advances in imprinting disorders. Clin. Genet. 91, 3–13. doi:10.1111/cge.12827
Stringer, J. M., Pask, A. J., Shaw, G., and Renfree, M. B. (2014). Post-natal imprinting: evidence from marsupials. Heredity 113, 145–155. doi:10.1038/hdy.2014.10
Suzuki, S., Renfree, M. B., Pask, A. J., Shaw, G., Kobayashi, S., Kohda, T., et al. (2005). Genomic imprinting of IGF2, p57(KIP2) and PEG1/MEST in a marsupial, the tammar wallaby. Mech. Dev. 122, 213–222. doi:10.1016/j.mod.2004.10.003
Swain, J. L., Stewart, T. A., and Leder, P. (1987). Parental legacy determines methylation and expression of an autosomal transgene: a molecular mechanism for parental imprinting. Cell 50, 719–727. doi:10.1016/0092-8674(87)90330-8
Takeda, H., Caiment, F., Smit, M., Hiard, S., Tordoir, X., Cockett, N., et al. (2006). The callipyge mutation enhances bidirectional long-range DLK1-GTL2 intergenic transcription in cis. Proc. Natl. Acad. Sci. 103, 8119–8124. doi:10.1073/pnas.0602844103
The Bovine Genome Sequencing and Analysis Consortium, Elsik, C. G., Tellam, R. L., Worley, K. C., Gibbs, R. A., Muzny, D. M., Weinstock, G. M., et al. (2009). The genome sequence of taurine cattle: a window to ruminant biology and evolution. Science 324, 522–528. doi:10.1126/science.1169588
The FAANG Consortium, Archibald, A. L., Bottema, C. D., Brauning, R., Burgess, S. C., Burt, D. W., Casas, E., et al. (2015). Coordinated international action to accelerate genome-to-phenome with FAANG, the Functional Annotation of Animal Genomes project. Genome Biol. 16, 57. doi:10.1186/s13059-015-0622-4
The International Sheep Genomics Consortium, Archibald, A. L., Cockett, N. E., Dalrymple, B. P., Faraut, T., Kijas, J. W., Maddox, J. F., et al. (2010). The sheep genome reference sequence: a work in progress. Anim. Genet. 41, 449–453. doi:10.1111/j.1365-2052.2010.02100.x
Tixier-Boichard, M., Fabre, S., Dhorne-Pollet, S., Goubil, A., Acloque, H., Vincent-Naulleau, S., et al. (2021). Tissue resources for the functional annotation of animal genomes. Front. Genet. 12, 666265. doi:10.3389/fgene.2021.666265
Tucci, V., Isles, A. R., Kelsey, G., and Ferguson-Smith, A. C.Erice Imprinting Group (2019). Genomic imprinting and physiological processes in mammals. Cell 176, 952–965. doi:10.1016/j.cell.2019.01.043
Van Laere, A.-S., Nguyen, M., Braunschweig, M., Nezer, C., Collette, C., Moreau, L., et al. (2003). A regulatory mutation in IGF2 causes a major QTL effect on muscle growth in the pig. Nature 425, 832–836. doi:10.1038/nature02064
Vuocolo, T., Byrne, K., White, J., McWilliam, S., Reverter, A., Cockett, N. E., et al. (2007). Identification of a gene network contributing to hypertrophy in callipyge skeletal muscle. Physiol. Genomics 28, 253–272. doi:10.1152/physiolgenomics.00121.2006
Walker, S. K., Hartwich, K. M., and Seamark, R. F. (1996). The production of unusually large offspring following embryo manipulation: concepts and challenges. Theriogenology 45, 111–120. doi:10.1016/0093-691x(95)00360-k
Wallis, J. W., Aerts, J., Groenen, M. A. M., Crooijmans, R. P. M. A., Layman, D., Graves, T. A., et al. (2004). A physical map of the chicken genome. Nature 432, 761–764. doi:10.1038/nature03030
Whittington, C. M., Buddle, A. L., Griffith, O. W., and Carter, A. M. (2022). Embryonic specializations for vertebrate placentation. Philos. Trans. R. Soc. B Biol. Sci. 377, 20210261. doi:10.1098/rstb.2021.0261
Willadsen, S. M., Janzen, R., McAlister, R., Shea, B., Hamilton, G., and McDermand, D. (1991). The viability of late morulae and blastocysts produced by nuclear transplantation in cattle. Theriogenology 35, 161–170. doi:10.1016/0093-691x(91)90155-7
Xiang, R., Berg, I. v. d., MacLeod, I. M., Hayes, B. J., Prowse-Wilkins, C. P., Wang, M., et al. (2019). Quantifying the contribution of sequence variants with regulatory and evolutionary significance to 34 bovine complex traits. Proc. Natl. Acad. Sci. 116, 19398–19408. doi:10.1073/pnas.1904159116
Xiang, R., Fang, L., Liu, S., Macleod, I. M., Liu, Z., Breen, E. J., et al. (2023). Gene expression and RNA splicing explain large proportions of the heritability for complex traits in cattle. Cell Genomics 3, 100385. doi:10.1016/j.xgen.2023.100385
Xu, H., Zhao, L., Feng, X., Ma, Y., Chen, W., Zou, L., et al. (2021). Landscape of genomic imprinting and its functions in the mouse mammary gland. J. Mol. Cell Biol. 12, 857–869. doi:10.1093/jmcb/mjaa020
Xu, X., Ectors, F., Davis, E. E., Pirottin, D., Cheng, H., Farnir, F., et al. (2015). Ectopic expression of retrotransposon-derived PEG11/RTL1 contributes to the callipyge muscular hypertrophy. PLOS ONE 10, e0140594. doi:10.1371/journal.pone.0140594
Yao, J., Huang, J., and Zhao, J. (2016). Genome editing revolutionize the creation of genetically modified pigs for modeling human diseases. Hum. Genet. 135, 1093–1105. doi:10.1007/s00439-016-1710-6
Yu, H., Waddell, J. N., Kuang, S., Tellam, R. L., Cockett, N. E., and Bidwell, C. A. (2018). Identification of genes directly responding to DLK1 signaling in Callipyge sheep. BMC Genomics 19, 283. doi:10.1186/s12864-018-4682-1
Zaitoun, I., and Khatib, H. (2006). Assessment of genomic imprinting of SLC38A4, NNAT, NAP1L5, and H19 in cattle. BMC Genet. 7, 49. doi:10.1186/1471-2156-7-49
Zhang, S., Cheng, Y., Věchtová, P., Boryshpolets, S., Shazada, N. E., Alavi, S. M. H., et al. (2023). Potential implications of sperm DNA methylation functional properties in aquaculture management. Rev. Aquac. 15, 536–556. doi:10.1111/raq.12735
Zhang, Y., and Tycko, B. (1992). Monoallelic expression of the human H19 gene. Nat. Genet. 1, 40–44. doi:10.1038/ng0492-40
Keywords: parent-of-origin methylation, large offspring syndrome, callipyge, mammary gland, feeding behaviours, birthweight, epigenome evolution, pathophysiological models
Citation: Hubert J-N, Perret M, Riquet J and Demars J (2024) Livestock species as emerging models for genomic imprinting. Front. Cell Dev. Biol. 12:1348036. doi: 10.3389/fcell.2024.1348036
Received: 01 December 2023; Accepted: 19 January 2024;
Published: 15 February 2024.
Edited by:
Miguel Constancia, University of Cambridge, United KingdomReviewed by:
Yang Zhao, University of Michigan, United StatesJennifer M. Frost, Queen Mary University of London, United Kingdom
Copyright © 2024 Hubert, Perret, Riquet and Demars. This is an open-access article distributed under the terms of the Creative Commons Attribution License (CC BY). The use, distribution or reproduction in other forums is permitted, provided the original author(s) and the copyright owner(s) are credited and that the original publication in this journal is cited, in accordance with accepted academic practice. No use, distribution or reproduction is permitted which does not comply with these terms.
*Correspondence: Julie Demars, anVsaWUuZGVtYXJzQGlucmFlLmZy