- Department of Biological Sciences, University of Alberta, Edmonton, AB, Canada
Humans and other jawed vertebrates rely heavily on their craniofacial skeleton for eating, breathing, and communicating. As such, it is vital that the elements of the craniofacial skeleton develop properly during embryogenesis to ensure a high quality of life and evolutionary fitness. Indeed, craniofacial abnormalities, including cleft palate and craniosynostosis, represent some of the most common congenital abnormalities in newborns. Like many other organ systems, the development of the craniofacial skeleton is complex, relying on specification and migration of the neural crest, patterning of the pharyngeal arches, and morphogenesis of each skeletal element into its final form. These processes must be carefully coordinated and integrated. One way this is achieved is through the spatial and temporal deployment of cell signaling pathways. Recent studies conducted using the zebrafish model underscore the importance of the Transforming Growth Factor Beta (TGF-β) and Bone Morphogenetic Protein (BMP) pathways in craniofacial development. Although both pathways contain similar components, each pathway results in unique outcomes on a cellular level. In this review, we will cover studies conducted using zebrafish that show the necessity of these pathways in each stage of craniofacial development, starting with the induction of the neural crest, and ending with the morphogenesis of craniofacial elements. We will also cover human skeletal and craniofacial diseases and malformations caused by mutations in the components of these pathways (e.g., cleft palate, craniosynostosis, etc.) and the potential utility of zebrafish in studying the etiology of these diseases. We will also briefly cover the utility of the zebrafish model in joint development and biology and discuss the role of TGF-β/BMP signaling in these processes and the diseases that result from aberrancies in these pathways, including osteoarthritis and multiple synostoses syndrome. Overall, this review will demonstrate the critical roles of TGF-β/BMP signaling in craniofacial development and show the utility of the zebrafish model in development and disease.
Introduction
Craniofacial abnormalities, including malformations of the palate, lip, jaw, or cranium (skull), are the most common type of congenital disease, and can severely impact the quality of life of affected individuals by impairing speaking, breathing, eating, or, in some cases, cognition and brain function (Gorlin et al., 2001). The human craniofacial skeleton is generated during embryonic development from a transient population of embryonic cells known as neural crest cells (Jiang et al., 2002; Yoshida et al., 2008). Aberrations to the neural crest, caused by mutations in genes, perturbations to the intrauterine environment, or a combination of both, are known to cause craniofacial abnormalities in infants and children (Gorlin et al., 2001). However, the causative mutations, environmental insults, or interactions between the two remain incompletely understood. Additionally, many of the current treatments for craniofacial abnormalities (such as orofacial clefting and craniosynostosis) involve invasive surgeries with very few preventative measures existing (Shkoukani et al., 2014; Stanton et al., 2022). Therefore, understanding the underlying biology of craniofacial development will aid in understanding the pathogenesis of craniofacial abnormalities, which, in turn, can accelerate the development of strategies for identifying, diagnosing, and preventing craniofacial abnormalities in humans. Vertebrate model organisms, including the mouse and zebrafish, have been vital in understanding craniofacial development, and much of what we know about the underlying pathogenesis of craniofacial abnormalities has come from studies conducted in these organisms. Although many animal models have contributed to our understanding craniofacial development, studies conducted in zebrafish have provided important insights into the genetics and development of craniofacial biology and disease.
Craniofacial development is very complex and requires the coordinated deployment of many distinct cellular processes, including differentiation, migration, adhesion, shape changes, division, and programmed death. These processes must be controlled both spatially and temporally in the embryo to ensure proper development of the craniofacial skeleton; failure of proper temporo-spatial regulation causes abnormal craniofacial development. Signaling pathways are the primary way in which coordination between biological processes is achieved. In particular, the Transforming Growth Factor β (TGF-β) and Bone Morphogenetic Protein (BMP) signaling pathways have well-defined roles in craniofacial development and disease, and their roles in these processes have been illuminated by studies conducted using zebrafish.
In this review, we will examine the utility of using zebrafish as a model organism for understanding the role of BMP and TGF-β signaling in craniofacial development and disease. First, we will describe the components and regulation of TGF-β and BMP signaling pathways. Next, we will present an overview of zebrafish craniofacial development and highlight the advantages of using a zebrafish model for studying craniofacial development. We will then outline craniofacial and skeletal diseases in humans that are frequently caused by defects in BMP/TGF-β signaling. Finally, we will review the zebrafish studies of BMP and TGF-β signaling in craniofacial development and their impact on understanding the pathogenesis of craniofacial and skeletal disease.
Transforming growth factor Beta signaling
Ligands
TGF-β was first identified in the late 1970s/early 1980s as one of two factors (the other being TGF-α) that were able to “transform” anchorage-dependent fibroblasts into morphologically distinct cells able to grow in soft agar (De Larco and Todaro, 1978; Moses et al., 1981; Roberts et al., 1981). Shortly after the cDNAs for TGF-β1, 2, and 3 were cloned, several other proteins that shared sequence similarity with the C-terminal sequences of TGF-βs were identified (Derynck et al., 1985; Derynck et al., 1988; Mason et al., 1985; Cate et al., 1986; de Martin et al., 1987; Marquardt et al., 1987; Padgett et al., 1987; Weeks and Melton, 1987; Wozney et al., 1988). Currently, the TGF-β superfamily of signaling molecules contains at least 33 members in mammals, making it one of the largest families of signaling proteins. On the basis of structure and signaling activity, the TGF-β superfamily can be subdivided into a series of subfamilies. Six ligands comprise the TGF-β/Lefty/Inhibin family including the canonical TGF-βs (TGF-β1, 2, and 3), Lefty A and B, and Inhibin alpha (Hinck, 2012; Hinck et al., 2016). Activins comprise four additional ligands (Actβa, Actβb, Actβc, Actβe) (Hinck, 2012; Hinck et al., 2016). There are 22 members of the BMP/Growth Differentiation Factor (GDF)/Nodal/Mullerian Inhibiting Substance (MIS) family, which can be organized into four subfamilies based on structure (Newfeld et al., 1999; Hinck, 2012; Hinck et al., 2016). Eight ligands (BMP2, 4, 5, 6, 7, 8, and GDF1 and 3) comprise the canonical BMPs (Newfeld et al., 1999; Hinck, 2012; Hinck et al., 2016). A separable subfamily consists of GDF5, 6, and 7 together with BMP9 and 10. The three ligands Nodal, BMP3 and BMP10 (GDF2) are also structurally similar and represent a distinct subfamily of BMPs (Newfeld et al., 1999; Hinck, 2012; Hinck et al., 2016). More divergent from the above are the loosely grouped ligands BMP15, GDF15, GDF9, and MIS (Newfeld et al., 1999; Hinck, 2012; Hinck et al., 2016).
As alluded to previously, TGF-β superfamily ligands share a prototypical structure that is vital to their signaling activity and function. All ligands contain an N-terminal signal peptide, followed by a large (∼250 amino acid) prodomain that is necessary for protein folding, processing, and, in some cases, protein regulation and a smaller (∼110 amino acid) C-terminal mature growth factor domain (Figure 1A). TGF-β ligands form a structure known as a “cysteine knot” in their mature growth factor domain, where four distal polypeptide sequences are linked together by three disulfide bonds between six closely spaced pairs of cysteines (McDonald and Hendrickson, 1993) (Figure 1A). Ligand monomers are also covalently bound via their C-terminal signaling domains by an additional disulfide linkage, resulting in a ligand dimer with a structure that is frequently likened to a “butterfly” or two “hands” (Gentry et al., 1988). This “hand” structure consists of two sets of β-sheets that form “fingers” attached to a central stabilizing “wrist” or “palm” composed of an α-helix (Hinck et al., 2016). After transit out of the ER and into the Golgi, the prodomain is cleaved from the mature domain (Gentry et al., 1988). The prodomain typically remains associated with the mature signaling ligand and there is significant evidence that prodomains are critical for regulation of signaling activity in many instances, as suggested by 3D models of prodomain-mature ligand complexes (Gray and Mason, 1990).
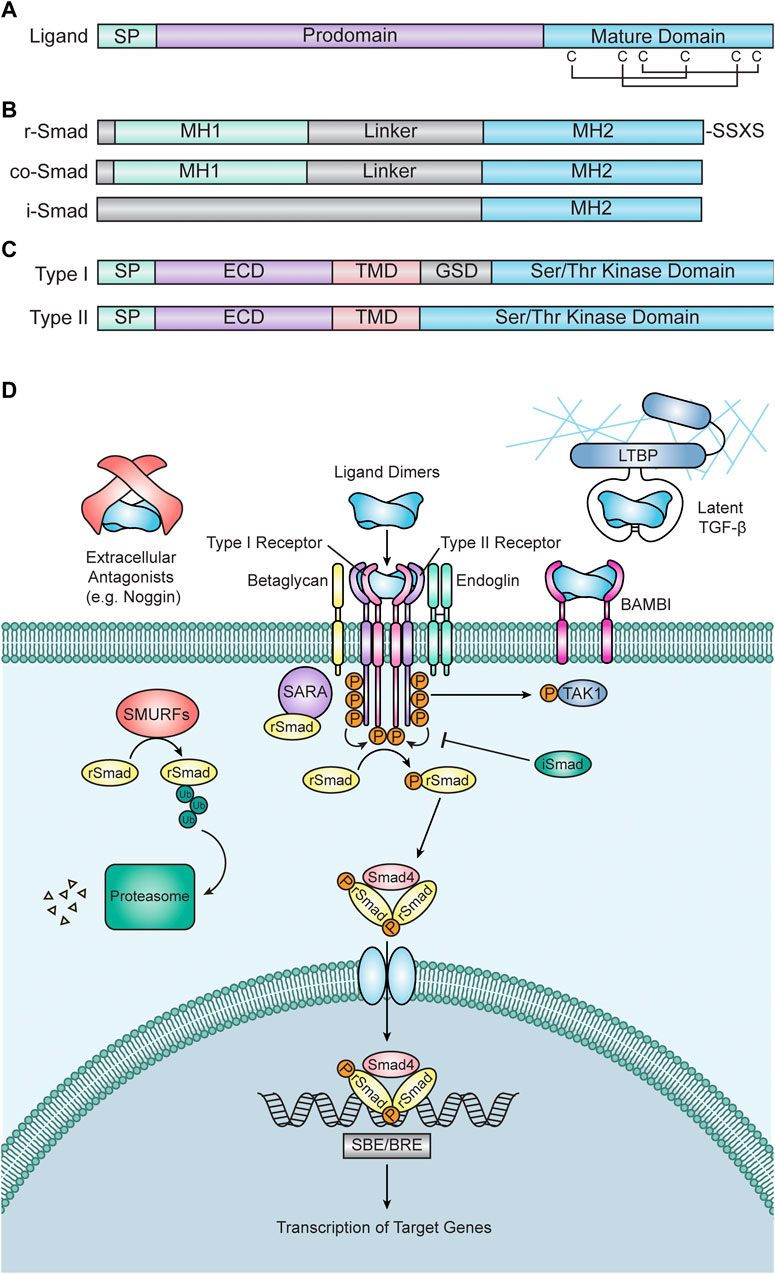
FIGURE 1. Transforming Growth Factor Beta (TGF-β) Superfamily Signaling. (A) Diagram of a prototypical TGF-β superfamily ligand. Each ligand consists of a short signal peptide (SP), followed by a prodomain and the mature signaling domain. The mature domain is stabilized by a “cysteine knot” motif, which consists of six cysteine residues connected via disulfide bonds. (B) Diagrams of Smad proteins. Receptor associated Smads (r-Smads, top) have an N-terminal Mad Homology 1 (MH1) domain and a C-terminal Mad Homology 2 (MH2) domain separated by a linker domain. R-Smads also contain an SXSS motif at their C-terminus, which is phosphorylated by receptors to initiate intracellular signal transduction. Common Smad4 (co-Smad, middle) has a similar structure to r-Smads but is lacking a C-terminal SXSS motif. Inhibitory Smads (i-Smads, bottom) contain an MH2 domain but lack an MH1 domain, allowing them to interact with r-Smad binding partners but preventing them from binding DNA and activating gene expression. (C) Diagrams of receptors. Type I receptors (top) contain a signal peptide (SP), an extracellular domain (ECD), a transmembrane domain (TMD), a glycine/serine-rich domain (GSD), and a serine/threonine kinase domain. Type II receptors (bottom) have a similar structure to Type I receptors, but they lack a GSD. The protein structures in (A–C) are highly conserved among vertebrates, including zebrafish. (D) TGF-β signal transduction. Signal transduction is initiated when TGF-β ligand dimers bind to two type II and two type I receptors. Ligand-receptor binding can be inhibited by extracellular antagonists (e.g., Noggin), TGF-β protein latency, or the BMP and Activin Membrane Bound Inhibitor (BAMBI). Ligand-receptor binding is also frequently facilitated by the coreceptors Endoglin or Betaglycan. Once the ligand-receptor complex is formed, constitutively active type II receptors phosphorylate type I receptors, thus activating them. Active type I receptors then phosphorylate r-Smads, which allow them to interact with co-Smad4 to create a trimeric Smad complex. R-Smad phosphorylation is facilitated by Smad Anchor for Receptor Activation (SARA) and inhibited by i-Smads. Type I receptors can also phosphorylate non-Smad targets, including TGF-β Activated Kinase (TAK1). Additionally, the degradation of r-Smads is promoted by Smad Ubiquitination Regulatory Factors (SMURFs). Once formed, Smad trimers enter the nucleus, bind to Smad Binding Elements (SBEs) or BMP Response Elements (BREs), and regulate the transcription of target genes.
Receptors
TGF-β signaling is initiated by binding of a ligand to its cognate type I and type II receptors. Receptors have two domains: an extracellular ligand-binding ectodomain and an intracellular kinase domain (Figure 1B). Unlike other receptor kinases, which typically phosphorylate tyrosine (e.g., fibroblast growth factor (FGF) receptors), TGF-β receptors are serine/threonine kinases (Wozney et al., 1988; Georgi et al., 1990; Mathews and Vale, 1991; Lin et al., 1992; Baarends et al., 1994; Kawabata et al., 1995; Yoshida et al., 2008). Receptors are categorized by which class of ligand they bind (Summarized in Table 1) (Hinck, 2012). Binding of a TGF-β ligand to its cognate type I and II receptors results in the creation of a receptor-ligand complex wherein a ligand dimer, via its receptor-binding domain, interacts with two type II receptors and two type I receptors (Wrana et al., 1992; Yamashita et al., 1994). This complex brings the type I and II receptors into proximity of one another, allowing the constitutively active serine/threonine kinase domain of the type II receptors to phosphorylate a glycine- and serine-rich domain (GS domain) in the cytoplasmic tail of the type I receptors, thus activating them and allowing them to phosphorylate downstream targets (Wrana et al., 1992; Wrana et al., 1994) (Figure 1D).
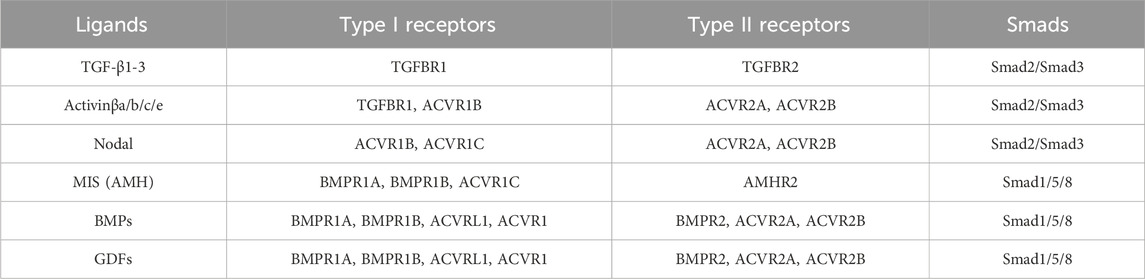
TABLE 1. Summary of TGF-β/BMP signaling pathway components. Listed below are each subgroup of ligands, followed by their cognate type I and type II receptors and the Smad proteins that they activate. Table adapted from information provided by Hinck (2012).
Smads
Once activated, type I receptors phosphorylate their intracellular target proteins, the receptor-associated Smads (r-Smads). Vertebrate Smads are homologous to (and named after) the Sma and Mad proteins, identified as regulators of TGF-β signaling in C. elegans and D. melanogaster, respectively (Raftery et al., 1995; Sekelsky et al., 1995; Derynck et al., 1996; Savage et al., 1996) Vertebrates possess five distinct r-Smads: Smad1, Smad2, Smad3, Smad5, and Smad8/9. Smad2/3 are typically activated by TGF-β, Activin, and Nodal ligands and receptors, whereas Smad1/5/8/9 are typically activated by BMP/GDF ligands and receptors (Table 1) (Graff et al., 1996). r-Smads have C-terminal and N-terminal Mad Homology (MH) domains, termed MH1 and MH2 respectively, which are connected by a linker domain that serves as a hub for facilitating cross talk between other pathways and regulating Smad protein activity (Fuentealba et al., 2007; Sapkota et al., 2007; Macias et al., 2015) (Figure 1C). Prior to phosphorylation, Smad2 interacts with the protein SARA (Smad Anchor for Receptor Activation), which anchors Smad2 to the cell membrane and facilitates the interaction between Smads and active Type I receptors (Tsukazaki et al., 1998) (Figure 1D). Similarly, the endosome-associated protein Endofin binds to Smad1 and enhances its phosphorylation by BMP receptors (Shi et al., 2007).
Active type I receptors interact with Smads via their MH1 domain and phosphorylate an SSXS motif on their C-terminus (Macías-Silva et al., 1996; Abdollah et al., 1997; Kretzschmar et al., 1997; Souchelnytskyi et al., 1997) (Figure 1C). This phosphorylation allows r-Smads to form a trimeric complex with common Smad4 (co-Smad4) consisting of two r-Smad subunits and one Smad4 subunit (Lagna et al., 1996; Kretzschmar et al., 1997; Wu et al., 1997; Zhang et al., 1997; Kawabata et al., 1998) (Figure 1D). Once formed, this Smad complex enters the nucleus and binds DNA elements to facilitate the transcription of target genes (Baker and Harland, 1996; Hoodless et al., 1996; Liu et al., 1996). Generally, Smad3/Smad4 complexes bind AGAC or GTCT sequences (termed Smad Binding Sequences or SBEs), whereas Smad1/Smad4 complexes bind SBEs weakly and preferentially bind GC-rich sequences (termed BMP Response Elements or BREs) (Zawel et al., 1998; Katagiri et al., 2002).
In addition to the r-Smads and co-Smad4, two additional Smad proteins exist: Smad6 and Smad7, also known as inhibitory Smads (i-Smads). As their name suggests, these Smad proteins inhibit signal transduction (Hayashi et al., 1997; Imamura et al., 1997; Nakao et al., 1997; Tsuneizumi et al., 1997; Hata et al., 1998) (Figure 1D). Smad6 is a potent inhibitor of BMP signaling, while Smad7 inhibits both TGF-β and BMP signaling (Hayashi et al., 1997; Hata et al., 1998). Although the MH2 domain of i-Smads is similar to other Smads, i-Smads differ significantly in their N-terminal MH1 and linker domains (Hanyu et al., 2001; Macias et al., 2015) (Figure 1C). i-Smads compete with r-Smads for type I receptor occupancy, thus preventing r-Smads from being phosphorylated and therefore inhibiting signal transduction (Nakao et al., 1997; Goto et al., 2007). Additionally, Smad7 can recruit other inhibitory proteins to type I receptors, including E3 ubiquitin ligases such as SMURFs, further attenuating signaling (Kavsak et al., 2000; Ebisawa et al., 2001). There is also evidence that i-Smads inhibit Smad-dependent transcription, suggesting that i-Smads interfere with signal transduction at multiple levels (Yan et al., 2016). The expression of i-Smads is induced by the pathways that they inhibit, thereby generating a negative feedback loop (Nagarajan et al., 1999; Denissova et al., 2000; Ishida et al., 2000; Benchabane and Wrana, 2003).
Distinct from the canonical Smad pathway, there is significant evidence that the TGF-β superfamily transduces its signal through non-Smad pathways. BMP/TGF-β signaling have been shown to induce the phosphorylation of mitogen activated protein kinases (MAPKs), including TGF-β Activated Kinase 1 (TAK1), which have been shown to activate kinases with downstream effects ranging from transcriptional regulation to cytoskeletal rearrangements (Yamaguchi et al., 1995) (Figure 1D). Additionally, several components of the TGF-β pathway have been shown to crosstalk with other signaling pathways, further adding complexity to these pathways and signaling effectors (Reviewed by Luo, 2017).
Regulators of TGF-β signaling
TGF-β proprotein latency
Unlike other superfamily members, TGF-β1, 2, and 3 are synthesized as latent protein complexes which only become activated after an activation cascade (Pircher et al., 1984; Lawrence et al., 1985; Wakefield et al., 1988; 1989; Lyons et al., 1990). After cleavage by proteases in the Golgi, TGF-β1-3 remain noncovalently associated with their prodomains (Gentry and Nash, 1990). In this context, the prodomain confers latency to the ligand and is referred to as Latency Associated Peptide (LAP). LAP masks the amino acids on the mature ligand that are critical for receptor binding, thus inhibiting interactions between the ligand and receptors (Shi et al., 2011). During synthesis and secretion, LAP becomes covalently linked to Latent TGF-β Binding Proteins (LTBP), which typically associate closely with components of the ECM such as fibrillin and fibronectin (Kanzaki et al., 1990) (Figure 1D). Secreted matrix metalloproteinases and plasmin degrade both the LAP and corresponding LTBP, thereby allowing the ligand to bind its cognate receptor (Sato and Rifkin, 1989; Yu and Stamenkovic, 2000). In addition, LAPs contain an Arg-Gly-Lys (RGD) motif, which is recognized by membrane-bound integrins. Upon binding of integrin dimers to LAPs, the mechanical force generated by the actin cytoskeleton induces a conformational change that releases the mature dimer from its LAP (Munger et al., 1999; Annes et al., 2004).
Extracellular BMP antagonists
While proprotein latency is unique to the TGF-β subfamily, inhibition of receptor binding by extracellular antagonists is unique to the BMP subfamily. BMP antagonists consist of subfamilies of secreted proteins and include Noggin, Chordin, Gremlin, DAN, Cerberus, and Follistatin (Zimmerman et al., 1996; Piccolo et al., 1999). Secreted BMP antagonists modulate binding of BMP dimers to their receptors (Groppe et al., 2002; Harrington et al., 2006; Zhang et al., 2008; Nolan et al., 2013). Like LAPs, BMP antagonists bind to BMP ligands and mask the residues that are critical for receptor binding, thus preventing binding of the ligand to its receptors (Groppe et al., 2002; Harrington et al., 2006; Zhang et al., 2008; Nolan et al., 2013) (Figure 1D). BMP antagonists tend to be expressed in very restricted temporal and spatial patterns, and the expression of antagonists is often regulated by other signaling pathways. Therefore, BMP antagonists are significant mediators of signaling crosstalk.
Co-receptors
In addition to the canonical type I and type II receptors that directly mediate signal transduction, there are also several co-receptors that modulate receptor activity. Some well-studied examples of these co-receptors are betaglycan and endoglin (Figure 1D). Betaglycan (sometimes referred to as the Type III TGF-β receptor or TGFBRIII) is a membrane-bound glycoprotein that binds TGF-β ligands, which can have a low affinity for their cognate type I and II receptors, and brings them into proximity of their receptors to form an active signaling complex (Wang et al., 1991; López-Casillas et al., 1993). Alternatively, the ectodomain of betaglycan can be cleaved and act as a sink for ligands, thereby preventing them from binding to receptors in a manner similar to extracellular BMP antagonists or LAP (López-Casillas et al., 1994). Endoglin, whose expression is primarily restricted to endothelial cells, is another well studied co-receptor for TGF-β (Cheifetz et al., 1992; Yamashita et al., 1994). Endoglin’s role in modulating signal transduction is complex; it can either antagonize signaling or promote ligand binding (Lastres et al., 1996). Like betaglycan, the ectodomain of endoglin can be cleaved and potentially regulate the availability of ligands in the extracellular space (Li et al., 1998; Castonguay et al., 2011). More recently, a BMP-specific co-receptor, Neogenin, was discovered; Neogenin binds BMP2, 4, 6, and 7, and enhances the ability of these ligands to activate both Smad1/5/8 phosphorylation and RhoA activity (Hagihara et al., 2011). Additionally, other membrane-bound or membrane-associated regulators of receptor activity exist, including GPI-anchored proteins such as Cripto, and have been shown to regulate TGF-β superfamily signaling in a cell-type specific manner (Minchiotti et al., 2000; Yeo and Whitman, 2001; Cheng et al., 2003; Gray et al., 2003; Garcia de Vinuesa et al., 2021).
BAMBI
Perhaps one of the most well-studied and potent negative regulators of TGF-β signaling is by BMP and Activin Membrane Bound Inhibitor (BAMBI). BAMBI expression is activated by TGF-β signaling, thereby creating a feedback loop where TGF-β signaling inhibits itself (Karaulanov et al., 2004; Sekiya et al., 2004). Structurally, BAMBI is very similar to TGF-β receptors, but lacks a GS domain in its cytoplasmic tail, thereby preventing it from being phosphorylated by Type II receptors and blocking signaling (Onichtchouk et al., 1999). BAMBI interacts with and acts as a sink for receptors, thereby preventing them from forming an active receptor complex (Onichtchouk et al., 1999) (Figure 1D). BAMBI also recruits i-Smads to the cell membrane, allowing them to interact with active receptors and inhibit signaling (Yan et al., 2009) (Figure 1D).
Regulation of Smads
Two main mechanisms exist to downregulate signaling at the level of Smads: dephosphorylation of activated r-Smads and proteasomal degradation of r-Smads. Once in their phosphorylated state, r-Smads can be dephosphorylated by phosphatases, which leads to the deactivation of the active Smad complex and, therefore, attenuation of signaling (Bruce and Sapkota, 2012). The second mechanism by which r-Smad activity is regulated is by degradation in the proteasome. Like other intracellular proteins, Smads can be covalently bound to ubiquitin by E3 ubiquitin ligases and targeted to the proteasome for degradation. Smad-specific E3 ubiquitin ligases, including SMURF1 and SMURF2, add ubiquitin groups to Smads, thereby targeting them for degradation (Zhu et al., 1999; Lin et al., 2000; Zhang et al., 2001) (Figure 1D). Although ubiquitination and phosphorylation represent the most extensively studied post-translational modifications of Smads, there is evidence that sumoylation, acetylation, and poly-ADP-ribosylation all play a role in regulating Smad activity.
Zebrafish TGF-β signaling
Although TGF-β/BMP pathways show a high degree of conservation between mammals and zebrafish, there are a few notable differences between the zebrafish and mammalian pathways. For example, the zebrafish homolog of BMP2 (Bmp2b) appears to be the functional equivalent to BMP4 in other systems (Kishimoto et al., 1997). Additionally, teleost fishes, including zebrafish, have undergone a complete genome duplication event, resulting in many genes (including those encoding components of the TGF-β/BMP pathways) having 2 paralogs (e.g., bmp2a and bmp2b, gdf6a, and gdf6b, etc.) (Glasauer and Neuhauss, 2014). In some instances, one paralog takes over the ancestral function, while the other undergoes non-functionalization (Glasauer and Neuhauss, 2014). For example, gdf6a appears to perform the same function as GDF6/Gdf6, whereas gdf6b appears to be, for all intents and purposes, non-functional (Glasauer and Neuhauss, 2014; Gramann et al., 2019). In other instances, the ancestral functions of the paralogs sub-functionalize, wherein each paralog performs regulates a slightly different process (Glasauer and Neuhauss, 2014). For instance, zebrafish have three paralogs of Noggin (nog1/2/3), each with completely different expression patterns and (presumably) different functions (Fürthauer et al., 1999).
Zebrafish craniofacial development
The cranial neural crest
The vertebrate head skeleton is made up of two components: the neurocranium, which houses the brain and sensory organs, and the viscerocranium, which includes the midface, jaw, and posterior pharyngeal structures (Schilling and Kimmel, 1994). Like other vertebrates, much of the zebrafish craniofacial skeleton is derived from a subpopulation of neural crest cells termed the cranial neural crest. During gastrulation, a region of ectoderm immediately lateral to the neural plate (the presumptive nervous system), termed the neural plate border, is specified by inductive signals that pattern the dorsal-ventral axis of the zebrafish embryo (Woo and Fraser, 1995). These inductive signals will activate the expression of neural plate border specifier genes, which predominantly encode transcription factors (e.g., pax3a, tfap2a, zic3, dlx5/6, msx1a/1b/3, etc.) (Seo et al., 1998; Knight et al., 2003; Knight et al., 2004; Barrallo-Gimeno et al., 2004; Phillips et al., 2006; Garnett et al., 2012; Narboux-Neme et al., 2019). NPB specifiers, in turn, will drive the expression of additional transcription factors that are required for NC identity (e.g., foxd3, snail1b, soz10, sox9a/b, twist, etc.) (Thisse et al., 1995; Kelsh and Eisen, 2000; Dutton et al., 2001; Li et al., 2002; Yan et al., 2002; 2005; Suazo et al., 2004; Carney et al., 2006; Lister et al., 2006; Montero-Balaguer et al., 2006; Stewart et al., 2006; Germanguz et al., 2007; Gestri et al., 2009; Das and Crump, 2012). During neurulation, neural crest cells will undergo an epithelial to mesenchymal transition (EMT), in which they detach from the ectoderm and initiate migration. Like other vertebrates, zebrafish EMT is a complex process that involves changes in the morphological and adhesive properties of neural crest cells; changes in these properties are primarily attained from upregulation of EMT genes by neural crest specifier genes (Berndt et al., 2008; Clay and Halloran, 2013; Clay and Halloran, 2014; Jimenez et al., 2016; Ahsan et al., 2019). After EMT, neural crest cells will migrate through the head mesenchyme and form the zebrafish craniofacial skeleton. A subpopulation of neural crest cells from the midbrain will migrate around the eyes and populate the frontonasal and maxillary prominences, where they will give rise to the ethmoid plate, trabeculae, and anterior skull vault of the neurocranium (Wada et al., 2005; Dougherty et al., 2013). Neural crest from the midbrain and rhombomeres of the hindbrain will migrate more ventrally and infiltrate the pharyngeal arches (PAs) (Wada et al., 2005; Dougherty et al., 2013). Neural crest cells from the midbrain and rhombomeres 1–3 populate PA1 (the mandibular arch), neural crest cells from rhombomeres 3–5 populate PA2 (the hyoid arch), and neural crest cells from rhombomeres 5–7 populate PA3-7 (the brachial arches) (Schilling and Kimmel, 1994). After migration, neural crest cells will condense and differentiate into cartilage and bone and ultimately form the zebrafish craniofacial skeleton (Schilling and Kimmel, 1994). During the larval stages, the craniofacial skeleton is relatively simple and primarily composed of cartilage with a few intramembranous bones (Cubbage and Mabee, 1996) (Figure 2A). Neural crest cells (and the subsequent cartilage and bone elements that they form) receive inductive signals along the anterior-posterior, dorsal-ventral, and proximal-distal axes, allowing each element to acquire a distinct shape that is dependent on their location (Minoux and Rijli, 2010; Medeiros and Crump, 2012). This, in turn, dictates the function of each element (Minoux and Rijli, 2010; Medeiros and Crump, 2012).
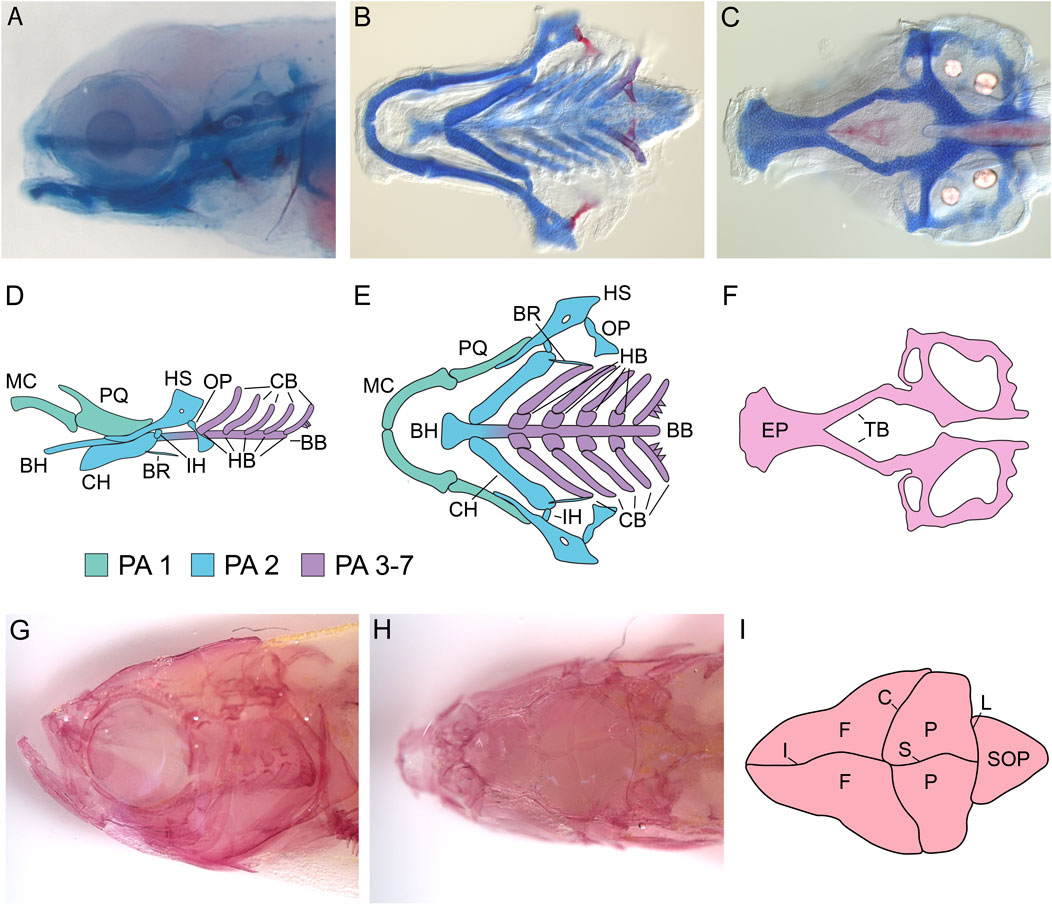
FIGURE 2. Zebrafish Craniofacial Anatomy. (A) Stereomicroscope image of a 5 dpf zebrafish larvae stained with Alcian blue (cartilage) and Alizarin red (mineralized bone). All cartilage elements and many of the early bone elements are present by 5 dpf in zebrafish, making analysis of craniofacial phenotypes relatively easy. (B,C) DIC microscopy image of the dissected, flat-mounted viscerocranium (B) and neurocranium (C) from a 5 dpf larval zebrafish stained with Alcian blue and Alizrin red. Flat-mounts of the larval craniofacial skeleton reveal the structural properties of cartilage/bone that are not visible by stereomicroscopy. (D,E) Schematics of the zebrafish viscerocranium at 5dpf. Schematics are shown in both a lateral (D) and ventral (E) orientation. At 5 dpf, the following cartilage structures are present in the viscerocranium: Meckel’s cartilage (MC) and the palatoquadrate (PQ) are derived from first pharyngeal arch (PA) neural crest, the basihyal (BH), ceratohyal (CH), hysoymplectic (HS), and the interhyal (IH) are derived from second PA neural crest, and the hypobranchials (HB), ceratobranchials (CB), and basibranchials (BB) are derived from third to seventh PA neural crest. At this stage, several mineralized membrane bones are also present, including the opercle (OP) and the branchiostegal ray (BR). (F) Schematic of the zebrafish neurocranium at 5dpf. At 5 dpf, both the ethmoid plate (EP) and the trabeculae (TB) are visible and the posterior part of the neurocranium is beginning to form. (G–I) Adult zebrafish craniofacial anatomy. (G) Lateral view of the adult zebrafish craniofacial skeleton stained with Alizarin red and visualized by stereomicroscopy. By adulthood, the larval craniofacial skeleton has been elaborated on significantly. (H) Dorsal view of the adult zebrafish craniofacial skeleton visualized by stereomicroscopy. Dorsal views reveal the zebrafish calvaria (skull bones) and sutures. (I) Schematic of adult zebrafish calvaria and sutures. Adult zebrafish possess two frontal bones (F), two parietal bones (P), and a supraoccipital bone (SOP) separated by the interfrontal suture (IF), the coronal suture (C), the sagittal suture (S), and the lamboid suture (L). All images are oriented with anterior facing left.
The zebrafish viscerocranium
Perhaps the best characterized component of the zebrafish craniofacial skeleton is the viscerocranium, which arises from the PAs (Figures 2A, B, D, E). Neural crest cells in the mandibular arch (PA1) give rise to Meckel’s cartilage, a pair of ventral cartilage rods that articulate at the midline via the mandibular symphysis. In larval zebrafish, Meckel’s cartilage acts as the lower jaw, and during adult craniofacial development, it acts as a template for the bones of the adult jaw to condense on (Cubbage and Mabee, 1996; Piotrowski et al., 1996; Schilling et al., 1996; Schilling and Kimmel, 1997). Located more dorsally in the mandibular arch is the palatoquadrate, a fan-shaped cartilage that articulates with Meckel’s cartilage via the mandibular jaw joint (Cubbage and Mabee, 1996; Piotrowski et al., 1996; Schilling et al., 1996; Schilling and Kimmel, 1997). The palatoquadrate is also attached to the ethmoid plate via the anteriorly positioned pterygoid processes to form the larval upper jaw (Cubbage and Mabee, 1996; Piotrowski et al., 1996; Schilling et al., 1996; Schilling and Kimmel, 1997). In the hyoid arch (PA2), ventral neural crest cells will form a pair of rod-shaped cartilage elements called the ceratohyals (Cubbage and Mabee, 1996; Piotrowski et al., 1996; Schilling et al., 1996; Schilling and Kimmel, 1997). Each ceratohyal is connected to the basihyal, an unpaired cartilage element positioned along the ventral midline (Cubbage and Mabee, 1996; Piotrowski et al., 1996; Schilling et al., 1996; Schilling and Kimmel, 1997). The ceratohyal serves to support the larval lower jaw and will progressively ossify via intramembranous ossification throughout larval development (Cubbage and Mabee, 1996; Piotrowski et al., 1996; Schilling et al., 1996; Schilling and Kimmel, 1997). The hyosymplectic is oriented more dorsally in the hyoid arch and articulates with the otic cartilage to connect skeletal elements from the hyoid arch to the cranium (Cubbage and Mabee, 1996; Piotrowski et al., 1996; Schilling et al., 1996; Schilling and Kimmel, 1997). The ceratohyal and hyosymplectic are joined to one another by the interhyal cartilage in the hyoid joint (Cubbage and Mabee, 1996; Piotrowski et al., 1996; Schilling et al., 1996; Schilling and Kimmel, 1997). The brachial arches (PA3-7) give rise to the ceratobranchials, which are paired, rod-shaped cartilages that are connected to the midline basibranchials via small cartilage elements called hypobrachials (Cubbage and Mabee, 1996; Piotrowski et al., 1996; Schilling et al., 1996; Schilling and Kimmel, 1997). These cartilage elements serve to support the gills and associated structures. The craniofacial skeleton undergoes many changes as the zebrafish proceeds through metamorphosis and into adulthood. New membrane bones are formed, and existing ones grow significantly (Cubbage and Mabee, 1996). Several of the cartilage elements ossify via endochondral ossification or are degraded after membrane bones form in their place (Cubbage and Mabee, 1996). The adult zebrafish craniofacial skeleton has 43 bones (compared to ∼22 in mammals), which represents a complex arrangement of highly specialized skeletal structures (Cubbage and Mabee, 1996) (Figure 2G).
The zebrafish neurocranium
The neurocranium, composed of the ethmoid plate, trabeculae, and the skull vault, also begins development in the embryonic and larval stages. The ethmoid plate forms from bilateral populations of neural crest cells that converge on the midline to produce a flat, fan-shaped sheet of cells (the ethmoid plate) that is connected to the neurocranium via two paired rods of cartilage termed the trabeculae (Wada et al., 2005; Eberhart et al., 2006; Dougherty et al., 2013) (Figures 2C, F). The ethmoid plate articulates with the retroarticular processes of the palatoquadrate to form the larval upper jaw (Cubbage and Mabee, 1996). The ethmoid also serves as the “roof” of the mouth and separates the larval mouth from the rest of the anterior head (Cubbage and Mabee, 1996). Later in development (∼2 months post-fertilization) the zebrafish skull vault develops (Topczewska et al., 2016; Kanther et al., 2019). Zebrafish, like mammals, have 5 calvaria: a pair of frontal bones, a pair of parietal bones, and one supraoccipital bone (Topczewska et al., 2016) (Figures 2H, I). The frontal and parietal bones develop from membranous ossification where mesenchymal cells ossify directly, whereas the supraoccipital bone must first pass through a cartilage template before ossifying via endochondral ossification (Topczewska et al., 2016; Kanther et al., 2019). Like mammals, the anterior-most region of the skull vault is derived from neural crest, whereas the more posterior regions are derived from mesoderm (Kague et al., 2012; Mongera et al., 2013). However, in contrast to mammals, where the demarcation between neural crest and mesoderm is represented by the coronal suture, zebrafish have a cryptic boundary between neural crest-derived and mesoderm-derived bones that occurs somewhere in the middle of the two frontal bones, making the frontal bones dual origin (Kague et al., 2012; Mongera et al., 2013). Calvaria are separated by fibrous joints called sutures (Topczewska et al., 2016; Kanther et al., 2019). The interfrontal suture separates the two frontal bones, the coronal suture separates the frontal and parietal bones, the sagittal suture separates the two parietal bones, and the lamboid sutures separate the parietal bones from the supraoccipital bone (Topczewska et al., 2016) (Figures 2H, I). Sutures contain osteoprogenitor cells, which act as a leading edge for calvaria osteogenesis as the brain grows, and mesenchymal stem cells, which are derived from both the mesoderm and neural crest and provide a niche for calvaria osteogenesis (Topczewska et al., 2016; Kanther et al., 2019). Complex signaling networks and cell behaviors are necessary for patterning suture formation and maintaining patency of the sutures (Topczewska et al., 2016; Kanther et al., 2019). Unlike human sutures, which fuse completely by adulthood, zebrafish sutures remain patent throughout life, allowing for lifelong brain growth (Topczewska et al., 2016).
Evolutionary conservation of the zebrafish craniofacial skeleton
A significant advantage of using zebrafish as a model to study craniofacial development and disease is the homology between several zebrafish and mammalian craniofacial structures. For instance, many elements in the larval/juvenile craniofacial skeleton, such as Meckel’s cartilage or the cranial sutures, are shared between the zebrafish and its mammalian, avian, and amphibian counterparts. Moreover, even when zebrafish do not have an obvious structure that is present in other animals, they frequently have structures that share an evolutionary origin with structures in mammals and are therefore considered homologous. Two examples of this are the ossicles of the middle ear and the mammalian hard palate. While zebrafish do not produce ossicles, the bones in the inner ear necessary for the conduction of sound, mammalian ossicles evolved from components of the teleost viscerocranium, and these components are homologous to mammalian ossicles (Anthwal et al., 2013). In zebrafish, the hyomandibula is homologous to the mammalian stapes, whereas the posterior of Meckel’s cartilage and the ventral portion of the palatoquadrate are homologous to the malleus and incus (Reichert, 1837). Furthermore, the connection of the hyomandibula to the otic cartilage in fish resembles the connection of the stapes to the oval window in mammals, further suggesting homology between these two structures (Reichert, 1837). The ethmoid plate has also been shown to be homologous to the mammalian hard palate. The ethmoid plate and the mammalian palate both have similar morphogenetic origins; like the mammalian palatal shelves, the neural crest cells that give rise to the ethmoid plate migrate towards the midline and fuse (Wada et al., 2005; Eberhart et al., 2006; Dougherty et al., 2013). Additionally, the genetic architecture that regulates palatogenesis in mammals has been shown to regulate ethmoid plate formation in zebrafish (Swartz et al., 2011). Therefore, these zebrafish structures can be used to model human craniofacial development and disease.
Zebrafish studies of TGF-β signaling and craniofacial development
As stated previously, the zebrafish model has been an invaluable resource for studying the role of signaling molecules in craniofacial development and disease and complement data generated in mouse models (for an excellent review on the use of mouse models to study craniofacial development and disease, we direct the reader to Ueharu and Mishina, 2023). In this section, we review studies conducted in zebrafish regarding BMP/TGF-β signaling in this process. The major details covered in this section are summarized in Table 2.
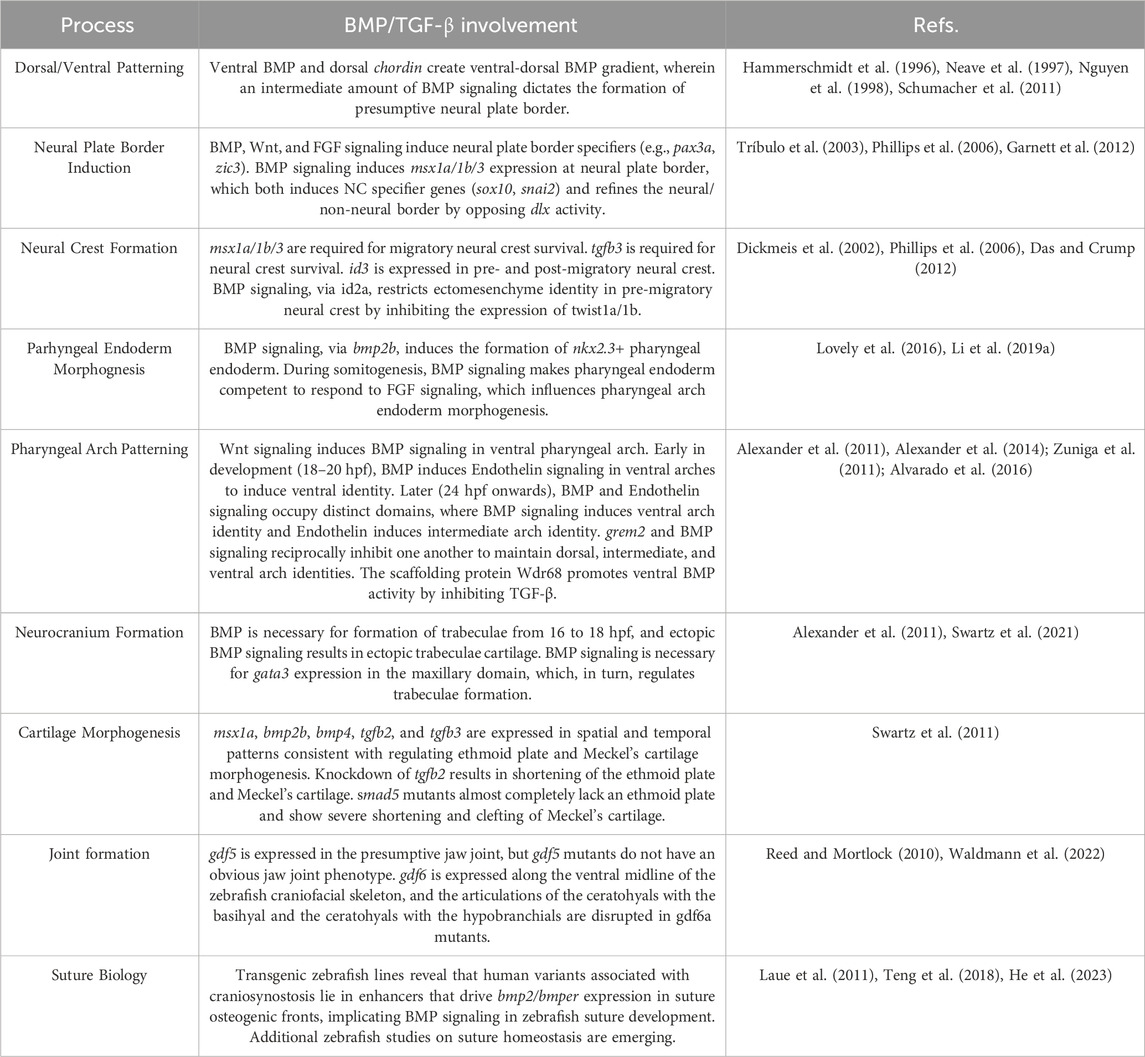
TABLE 2. Summary of Zebrafish Studies of TGF-β Signaling in Craniofacial Development. Listed below are the key findings generated in zebrafish regarding TGF-β superfamily signaling in craniofacial development organized by developmental processes with their corresponding references. Refs. = References.
Early development
Neural crest induction and migration
Neural crest cells, the precursors of the craniofacial skeleton, arise from the neural plate border (NPB). As is the case in other vertebrates, the presence and position of the zebrafish NPB is established by gradients of inductive signals that pattern the ectoderm during gastrulation. Forward genetic screens performed in zebrafish produced several mutants that were necessary for establishing dorsal-ventral patterning in the early embryo (Hammerschmidt et al., 1996; Mullins et al., 1996). Subsequently, the genes mutated in these mutants were found to encode components of the BMP signaling pathway, including swirl (bmp2b), snailhouse (bmp7a), somitabun (smad5) and dino (chordin/chd) (Kishimoto et al., 1997; Schulte-Merker et al., 1997; Hild et al., 1999; Schmid et al., 2000). Like other vertebrates, a gradient of BMP signaling is established in the early zebrafish gastrula; BMP ligands are expressed in the ventral gastrula, whereas chd is expressed in the dorsal side, creating a gradient of high to low BMP signaling activity along the ventral to dorsal axis of the embryo (Hammerschmidt et al., 1996; Neave et al., 1997) (Figure 3A). This gradient results in an intermediate amount of BMP activity in the region adjacent to the neural plate, which is sufficient to form the neural plate border and, thus, the neural crest (Schumacher et al., 2011) (Figure 3B). The formation of the neural plate border is highly sensitive to the amount of BMP activity: an absence in BMP signaling results in a failure of NPB establishment, whereas a mild reduction results in the expansion of the NPB (Nguyen et al., 1998).
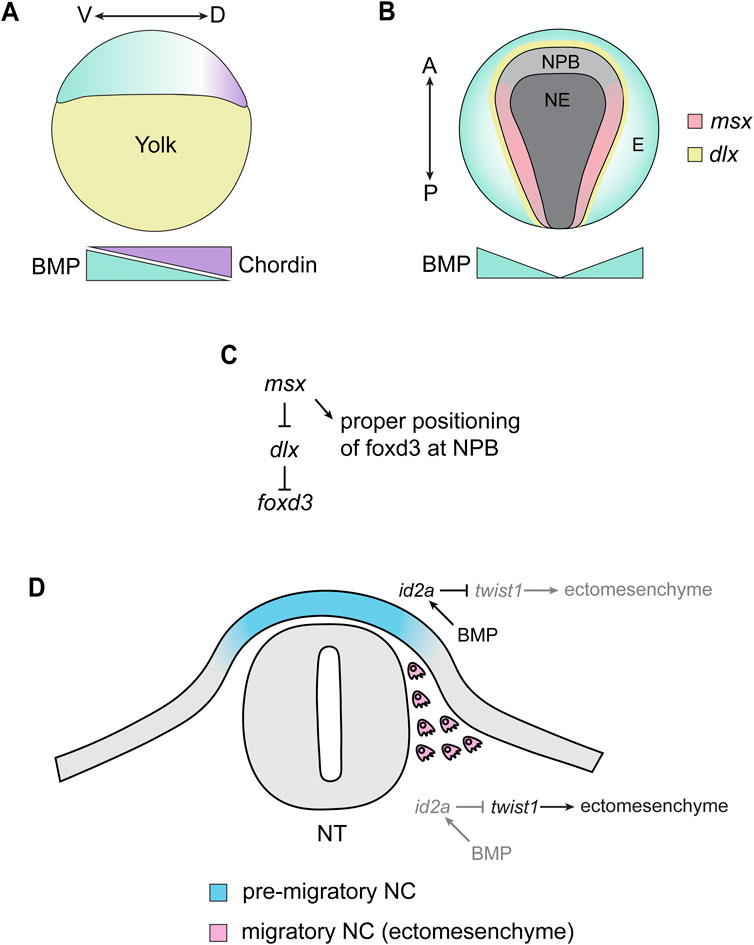
FIGURE 3. Summary of Zebrafish Studies on TGF-β Signaling and Neural Crest Induction/Migration. (A) During the late blastula stage (∼4 hpf), BMP ligands are expressed in the presumptive ventral side of the embryos (green). This is counteracted by chordin, which is expressed in the presumptive dorsal side of the embryo (purple). This creates a gradient of BMP signaling, where the presumptive ventral region of the embryo contains a high level of BMP signaling and the dorsal-most side of the embryo is absent of BMP signaling. V = ventral, D = dorsal. (B) The gradient of BMP signaling established at the blastula stage induces distinct tissue identities depending on the level of BMP signaling in that area, where the absence of BMP signaling allows for the development of neuroectoderm (NE), high BMP signaling induces epidermal (E) identity, and a moderate amount of BMP (together with Wnt and FGF signaling) induces the formation of the neural plate border (NPB). BMP signaling drives the expression of NPB transcription factors, including msx1a/1b/3, which are expressed within the NPB adjacent to the expression of dlx transcription factors. A = anterior, P = posterior. (C) In the NPB, msx transcription factors inhibit the activity of dlx transcription factors, which inhibit the expression of the neural crest specifier foxd3. This results in foxd3 being expressed in the neural plate border, allowing neural crest induction to proceed. (D) During the migratory phase of neural crest development, BMP signaling restricts the ectomesenchyme potential of neural crest cell. In dorsal, pre-migratory neural crest (NC) cells, ectodermal BMP signaling induces the expression of id2a in the NC. This, in turn, inhibits the activity of twist genes, preventing the acquisition of ectomesenchymal identity. As NC cells delaminate and migrate away from this ectodermal BMP, id2a expression is lost in NC cells, allowing twist genes to activate an ectomesenchymal gene expression program in the NC. All images are oriented with anterior facing left and dorsal facing up unless otherwise specified.
BMP signaling, together with Wnt and FGF, initiate the expression of transcription factors including pax3a and zic3, which confer NPB identity to the ectoderm (Garnett et al., 2012). BMP signaling seems particularly important for the expression of Muscle Segment Homeobox (msx) transcription factors. msx1a, msx1b, and msx3 are all expressed at the neural plate border and are necessary for the expression of the NPB specifier pax7 and the neural crest specifiers snai2 and sox10 (Tríbulo et al., 2003; Phillips et al., 2006) (Figure 3B). Although msx1a/1b/3 are not necessary for the expression of foxd3, the domains of foxd3 in the presumptive neural crest are closer to the midline in morphants compared to controls, suggesting that msx establishes and refines the neural-non neural border by opposing Distalless Homeobox (dlx) transcription factor activity (Phillips et al., 2006) (Figures 3B, C). msx gene expression is regulated by BMP signaling at the neural plate border; msx1b expression is eliminated in embryos that are BMP deficient, but in swirl, snailhouse, and somitabun mutants, where BMP signaling is present but reduced, the expression domain of msx1b is expanded, suggesting that msx1b is induced by a moderate amount of BMP and that the domain of msx1b is sensitive to gradients of BMP activation and inhibition (Tríbulo et al., 2003). In addition to their role in NPB patterning, msx1a/1b/3 also appear to be necessary for survival and induction of neural crest cells well after NPB induction (14–20 hpf) (Phillips et al., 2006). This results in a reduction of migratory neural crest and a disruption in neural crest-derivatives, including the craniofacial skeleton (Phillips et al., 2006). Therefore, msx transcription factors have multiple roles in neural crest development that extend well past NPB induction.
Near the end of zebrafish gastrulation (∼11 hpf), the cells of the neural plate border are specified to become neural crest. There is strong evidence that TGF-β/BMP modulates pre-migratory neural crest cell specification. tgfb3 is required for the survival of pre-migratory cranial neural crest cells in zebrafish, and knockdown or overexpression of tgfb3 results in cell death and abnormalities in the craniofacial skeleton (Cheah et al., 2010). id3, a member of the Inhibitor of Differentiation (id) family of transcription factors, is expressed in the pre-migratory and post-migratory cranial neural crest cells (Dickmeis et al., 2002). Given that ids are frequently targets of BMP signaling, this likely represents direct inductive signaling by BMP on pre- and post-migratory neural crest cells. Furthermore, BMP has also been shown to be critical for restricting the ectomesenchyme identity of neural crest cells in the head (Das and Crump, 2012). id2a is expressed in the pre-migratory neural crest, consistent with a high level of BMP activity in this region (Das and Crump, 2012) (Figure 3D). id2a becomes excluded from the migratory neural crest, and this exclusion of id2a was shown to be necessary for twist1a and twist1b activity (Figure 3D) (Das and Crump, 2012). twist1 and twist1b are critical for the switch from ectodermal to ectomesenchyme identity in cranial neural crest cells, and knockdown of twist1a/1b results in fewer ectomesenchyme neural crest cells (Das and Crump, 2012). Consistent with this, knockdown of twist1a/1b or forced activity of BMP in migratory neural crest results in profound loss of many elements of the craniofacial skeleton (Das and Crump, 2012). Thus, BMP signaling plays a critical role in restricting ectomesenchyme identity in the pre-migratory neural crest, thereby ensuring that the switch from ectoderm to ectomesenchyme is properly regulated.
Pharyngeal endoderm formation and signaling
After specification, neural crest cells undergo an epithelial to mesenchymal transition (EMT) that allows them to detach from the dorsal neural tube and initiate migration. As migratory cranial neural crest cells arrive in the PAs, they receive inductive signals from surrounding tissues that provide positional information, allowing the neural crest to form morphologically distinct structures based on their position in each arch. This includes diffusible inductive signals from the surrounding PA tissues, which encompass the mesoderm, the ectoderm (which forms the pharyngeal clefts), and the endoderm (which forms the pharyngeal pouches) (Graham, 2003). Studies performed in zebrafish have shown that signals from pharyngeal endoderm are particularly important for patterning and morphogenesis of the craniofacial skeleton, and proper morphogenesis of the endoderm itself is necessary for these processes (Piotrowski and Nüsslein-Volhard, 2000; David et al., 2002). BMP signaling has been identified as an inductive signal necessary for proper morphogenesis of the pharyngeal endoderm (Lovely et al., 2016; Li et al., 2019b). nkx2.3 is expressed in the prospective pharyngeal endoderm at the early somite stages and marks a subpopulation of the pharyngeal endoderm (Li et al., 2019b). Ablation of nkx2.3+ endoderm results in the loss or reduction of many cartilage elements in larval zebrafish, indicating that this subpopulation is necessary for craniofacial development (Li et al., 2019b). The specification of this subpopulation is dependent on BMP signaling; specifically, BMP signaling via bmp2b is necessary for the formation of these cells and proper pharyngeal endoderm development (Li et al., 2019b). In addition to its role in pharyngeal pouch specification, BMP signaling is also important for morphogenesis of the pharyngeal pouches during somitogenesis (Lovely et al., 2016). Blocking BMP signaling from 10 to 18 hpf results in a failure of pharyngeal pouch out-pocketing with no effect on endoderm formation, proliferation, or cell death, indicating that BMP signaling is necessary for the formation of the pharyngeal pouches (Lovely et al., 2016). Inhibition of BMP signaling during this period, in turn, influences craniofacial development, with many cartilage elements missing or reduced in BMP-deficient embryos (Lovely et al., 2016). BMP signaling is necessary for the expression of the FGF receptor fgfr4 in the PAs, and inhibiting BMP signaling lowers the response to FGF in the pharyngeal endoderm, suggesting that BMP signaling makes PA endoderm cells competent to respond to FGF signaling (Lovely et al., 2016). Taken together, these studies indicate that BMP signaling is necessary for both the induction and morphogenesis of the pharyngeal endoderm, which, in turn, regulates the formation of the craniofacial skeleton.
Pharyngeal arch patterning
Although neural crest cells receive anterior-posterior positional information from cues that pattern the dorsal ectoderm (e.g., the Hox code), the dorsal-ventral pattern of the viscerocranium is acquired after migratory neural crest cells infiltrate the PAs (Minoux and Rijli, 2010). Studies in zebrafish and mouse have revealed that BMP, Endothelin, and Notch signaling in the PAs regulate the nested expression of msx, hand, and dlx transcription factors (Clouthier et al., 1998; Clouthier et al., 2000; Miller et al., 2000; Clouthier et al., 2003; Miller et al., 2003; Charité et al., 2001; Beverdam et al., 2002; Depew et al., 2002; Ozeki et al., 2004; Kimmel et al., 2007; Nair et al., 2007; Sato et al., 2008; Talbot et al., 2010; Zuniga et al., 2010; Alexander et al., 2011; Zuniga et al., 2011; Alvarado et al., 2016). This, in turn, confers ventral, intermediate, and dorsal identity to the PAs and determines the shape of the individual elements and the placement of important structures such as the jaw joint (Miller et al., 2003; Barske et al., 2016). In this model, BMP and Endothelin promote the development of the ventral and intermediate domains, whereas Notch signaling promotes the formation of the dorsal domain by excluding Edn1 and BMP signaling from the dorsal domains of the arches (Miller et al., 2000; Miller et al., 2003; Kimmel et al., 2007; Nair et al., 2007; Zuniga et al., 2010; Alexander et al., 2011; Zuniga et al., 2011; Alvarado et al., 2016) (Figure 4). Although mouse studies have clearly identified BMP signaling as an important regulator of PA patterning, the necessity of BMP signaling in axis patterning, NPB formation, and neural crest induction has precluded analysis of this signaling pathway at later stages (Dudas et al., 2004; Liu et al., 2004; 2005; Bonilla-Claudio et al., 2012). Zebrafish studies have allowed for the analysis of BMP signaling in this later process, as transgenic lines designed to monitor, overexpress, and deplete BMP activity have allowed researchers to circumvent the need for BMP in early development. BMP, induced, in part, by Wnt signaling, is active in post-migratory ventral PA neural crest cells (Alexander et al., 2011; Alexander et al., 2014) (Figure 4A). Zebrafish studies have revealed that BMP signaling in the PAs is biphasic (Alexander et al., 2011; Zuniga et al., 2011). During early arch development (∼18–20 hpf), BMP is necessary for edn1 expression in the ventral arches, and depleting or ectopically activating BMP signaling during this time period reduces or increases edn1 expression, respectively (Alexander et al., 2011) (Figure 4A). Together, Endothelin and BMP signaling synergistically induce the expression of ventral (hand2) and intermediate (dlx5a/6a) PA transcription factors, thus imparting ventral identity to the ventral PA neural crest cells (Alexander et al., 2011) (Figure 4A). In contrast, later in development (24 hpf onwards), bmp4 and edn1 are expressed in different domains, with edn1 being slightly dorsal to bmp4, suggesting that these pathways pattern distinct domains at this timepoint (Zuniga et al., 2011). Consistent with this, ectopic activation of Endothelin or BMP signaling have distinct effects on the pharyngeal skeleton; while BMP activation induces the ventralization of many elements, Endothelin activation causes many elements to adopt an intermediate-like identity, indicating that BMP and Endothelin signaling promote ventral and intermediate identity, respectively (Zuniga et al., 2011). Accordingly, BMP promotes the induction of ventral-specifying factors (e.g., msx1a and hand2) while Endothelin signaling promotes the expression of intermediate domain-specifying factors, including signals required for positioning the jaw joint (e.g., dlx3b, dlx5a, dlx6a, and nkx3.2) (Alexander et al., 2011; Zuniga et al., 2011) (Figure 4A). Additionally, BMP signaling is no longer required for edn1 expression past 24 hpf, further indicating that the two pathways pattern distinct domains later in PA patterning (Alexander et al., 2011; Zuniga et al., 2011). Dorsal Notch signaling, aided by intermediate-domain Endothelin signaling, drives the expression of grem2 in the dorsal domain, thereby restricting BMP signaling to the ventral domain (Figure 4A) (Zuniga et al., 2011). BMP signaling, in turn, restricts grem2 expression to the dorsal domain, thereby generating domains of high BMP in the ventral-most domain of the PAs and low BMP in the dorsal PAs, which reciprocally inhibit one another to maintain these domains (Figure 4A) (Zuniga et al., 2011). These PA domains, established by these signaling pathways and their interactions, shape the pharyngeal skeleton by promoting and restricting cartilage formation in distinct domains; Notch and BMP signaling promote the expression of prrx1a/1b and restrict the expression of barx1 in the dorsal and ventral domains, whereas Endothelin signaling promotes the expression of barx1 and inhibits the expression of prrx1a/1b in the intermediate domain (Barske et al., 2016) (Figure 4B). This creates a heterochronic onset of chondrogenesis in these domains–barx1 in the intermediate domain will initiate chondrogenesis first, while prrx1a/1 dorsal and ventral domains will prevent chondrogenesis and hold neural crest cells in a skeletal progenitor state (Barske et al., 2016) (Figure 4B). This heterochrony of cartilage induction is thought to, in part, shape the pharyngeal skeleton along the dorsal-ventral axis (Barske et al., 2016). Therefore, interactions between signaling pathways, including BMP, define distinct dorsal-ventral domains within the PAs which, in turn, determines the shape of each element based on its dorsal-ventral position in the PAs. Furthermore, several signaling pathways, including BMP, position the expression F-box (fox) transcription factors in PAs. This results in sox9a+/fox+ domains in the PA, which promote a chondrogenic program, whereas dorsal sox9a+/fox- domains promote an osteogenic program that induce the formation of dermal bone (Xu et al., 2018) (Figure 4B). Therefore, BMP signaling, via the spatial deployment of fox genes, also patterns the PAs such that cartilage and bone are formed in distinct domains.
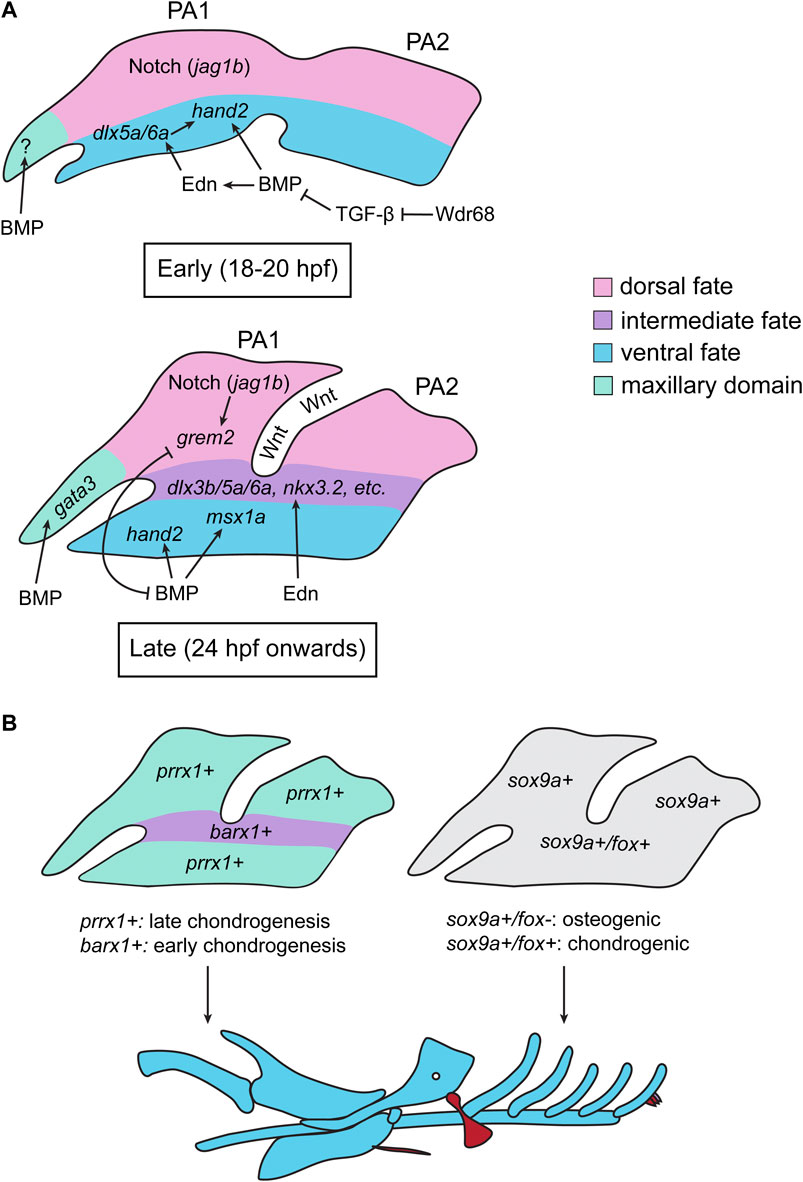
FIGURE 4. Summary of Zebrafish Studies on TGF-β Signaling and Pharyngeal Arch Patterning. (A) During the early stages of pharyngeal arch (PA) patterning (18–20 hpf), a ventral source of BMP activates Endothelin (Edn) signaling, which induces the expression of ventral-promoting transcription factors in the PAs (dlx5a/6a, hand2). BMP signaling also directly induced the expression of hand2 in the ventral PAs. Notch signaling (via jag1b) specifies the dorsal domain. There is also evidence that BMP signaling regulates the formation of the trabeculae in the maxillary domain (M) during this period, however how this is achieved in zebrafish in unclear. BMP is opposed by TGF-β signaling during this period, and the scaffolding protein Wdr68 permits BMP signaling by sequestering Smad2/3 and thereby inhibiting TGF-β signaling. In later stages of PA patterning (24 hpf onwards), the BMP (induced by Wnt signling) and Edn pathways act independently: BMP signaling activates the expression of ventral (hand2) and intermediate-ventral (msx1a) genes, whereas Edn induces the expression of intermediate identity specifiers (dlx3b/5a/6a, nkx3.2). Dorsal Notch signaling induces the expression of grem2, which inhibits BMP signaling and restricts hand2/msx1a to the ventral-most region of the arches. In turn, BMP signaling restricts the expression of grem2 to the dorsal region of each arch, creating distinct dorsal, intermediate, and ventral domains of gene expression. During this stage, BMP (together with Hedgehog signaling) also induces the expression of gata3 in the maxillary domain (B) The gene expression domains generated in (A) result in differential domains of prrx1 and barx1 expression, with barx1 being expressed in the intermediate domain and prrx1 being expressed in the dorsal and ventral domains (left). This, in turn, results in heterochronic onsets of chondrogenesis, where barx1+ cells initiate chondrogenesis earlier than prrx1+ cells. This heterochrony, in part, dictates the shape/identity of the resulting skeletal elements. The spatial gene expression domains in the PAs also create differential regions of fox gene expression, which dictate whether a cell will undergo osteogenesis or chondrogenesis; sox9a+/fox-regions will initiate an osteogenic gene expression program, whereas sox9a+/fox + cells will initiate a chondrogenic program (right). All images are oriented with anterior facing left.
Unlike BMP signaling, which plays an undoubtedly critical role in establishing the early dorsal-ventral pattern of the pharyngeal skeleton, there is limited evidence in zebrafish that TGF-β or Nodal signaling mediates early PA development. However, a 2016 study found that the scaffolding protein Wdr68 is necessary for the induction of BMP signaling in the early ventral PAs and, therefore, plays a role in ventral arch identity (Alvarado et al., 2016). The authors showed that TGF-β signaling inhibits BMP signaling during early arch patterning, and that this inhibition was enhanced in wdr68 mutants, suggesting that wdr68 indirectly promotes BMP signaling by inhibiting TGF-β signaling in the ventral arches (Alvarado et al., 2016) (Figure 4A). Although it is unclear if wdr68 modulates TGF-β signaling indirectly or directly, previous evidence has suggested that the Wdr68 protein binds and sequesters phosphorylated Smad2/3, indicating that this inhibition may occur at the level of Smads (Brown et al., 2008). Indeed, inhibitory interactions between BMP and TGF-β signaling is observed in other contexts, suggesting that this is a highly conserved mechanism of these two pathways (Reviewed by Hudnall et al. (2016)). It will be interesting to see if regulatory networks involving opposing functions of BMP and TGF-β signaling modulate other aspects of craniofacial and skeletal development.
Neurocranium formation
In addition to the pharyngeal skeleton, BMP signaling is also necessary for the early stages of neurocranium development, particularly the development of the trabeculae. Embryos lacking BMP signaling from 16 to 18 hpf have absent trabeculae, whereas ectopic BMP signaling results in malformation of the trabeculae and the formation of ectopic cartilage condensations on the trabeculae, indicating that BMP signaling likely induces the formation of trabeculae early in development of the neurocranium (Alexander et al., 2011). gata3 mutants display a spectrum of phenotypes affecting the trabeculae, ranging from mild malformations in the trabeculae resulting from altered cell arrangements to complete absence of both trabeculae, similar to what is observed in BMP-deficient embryos (Alexander et al., 2011; Sheehan-Rooney et al., 2013; Swartz et al., 2021). gata3 is expressed in the maxillary domain (the region that gives rise to the trabeculae) starting at 26 hpf, and gata3 expression and function in the maxillary domain at this timepoint is dependent on BMP signaling, further indicating that BMP signaling mediates trabeculae formation via gata3 (Swartz et al., 2021) (Figure 4A). However, attenuation of BMP signaling does not enhance the trabeculae phenotype in gata3 morphants, suggesting that BMP signaling is not responsible for the phenotypic variability observed in gata3 mutants (Swartz et al., 2021). Interestingly, treatment of gata3 mutant embryos with SAG, an agonist of Hedgehog (Hh) signaling, ameliorates the trabeculae phenotypes, whereas treatment with cyclopamine (a Hh inhibitor) enhances the trabeculae phenotype (Swartz et al., 2021). Shh components were found to be upregulated in gata3 mutants with a mild phenotype and downregulated in mutants with a severe phenotype, suggesting that Hh signaling, rather than BMP signaling, is a major contributor to the variability of phenotypes in gata3 mutants (Swartz et al., 2021). This, in part, may explain the phenotypic variability observed in human craniofacial diseases, including those observed in individuals with mutations in the human paralog, GATA3 (Swartz et al., 2021).
Late development
Cartilage formation and morphogenesis
Once the initial pattern for the craniofacial skeleton is established and the primordia of each cartilage element has formed, the presumptive cartilage cells undergo cellular rearrangements, allowing them to fine-tune the shaping of the cartilage elements. This, in turn, creates distinct shapes for the cartilage elements that correlates to their function. For example, many elements, such as Meckel’s cartilage, the ceratohyal, and the trabeculae of the neurocranium adopt rod-like shapes, where chondrocytes adopt a stacked morphology, whereas the palatoquadrate, hyosymplectic, and ethmoid plate adopt fan-like shapes (Kimmel et al., 1998; Eames et al., 2013). In zebrafish, some of the most striking cellular rearrangements occur in the ethmoid plate. Neural crest cells that populate the frontonasal and maxillary processes will converge towards the midline and form the ethmoid plate and trabeculae. From 36 to 48 hpf, significant cell rearrangements occur within this population of neural crest cells, and by 48 hpf, the primordia of the ethmoid plate is visible (Dougherty et al., 2013). A study analyzing the expression of genes encoding signalling pathway components has revealed that members of the TGF-β superfamily are likely involved in this process in zebrafish. msx1a, a downstream target of BMP signaling, is expressed in the maxillary domain from 36 to 60 hpf, consistent with a role in morphogenesis of the anterior neurocranium (Swartz et al., 2011). Accordingly, bmp2b and bmp4 are expressed in the anterior neural crest and oral ectoderm from 36 to 48 hpf (Swartz et al., 2011). Transcripts encoding the TGF-β ligands Tgfb2 and Tgfb3 are also expressed in regions and times relevant to ethmoid plate morphogenesis; tgfb2 is expressed in the maxillary neural crest from 36 to 48 hpf, whereas tgfb3 is expressed mainly in the oral epithelium surrounding the maxillary neural crest with only a small number of maxillary neural crest expressing tgfb3 from 36 to 44 hpf. However, from 44 to 72 hpf, tgfb3 is upregulated in the maxillary neural crest, suggesting that tgfb3 has distinct early and late roles in ethmoid plate morphogenesis (Swartz et al., 2011). Taken together, the expression patterns of tgfb2 and tgfb3 in zebrafish are consistent with their roles in palatogenesis in mice (Kaartinen et al., 1995; Proetzel et al., 1995; Sanford et al., 1997). Consistent with their expression patterns, zebrafish deficient for these signaling pathways display craniofacial defects consistent with aberrant morphogenesis. While knockdown of tgfb3 causes severe defects that preclude the analysis of the craniofacial skeleton, knockdown of tgfb2 results in shortening of the anterior neurocranium, wherein the ethmoid plate and trabeculae are malformed (Swartz et al., 2011). Additionally, smad5 mutant zebrafish, which have reduced BMP signaling activity, almost completely lack the ethmoid plate and trabeculae, which is consistent with mice lacking BMP signaling in palatogenic neural crest cells (Swartz et al., 2011; Kouskoura et al., 2013; Chen et al., 2019).
In addition to the ethmoid plate phenotypes observed in tgfb2 morphants and smad5 mutants, other cartilage elements also display aberrant morphology in these models. In tgfb2 morphants, Meckel’s cartilage is severely reduced, which is consistent with its expression in mandibular neural crest cells (Swartz et al., 2011). smad5 mutants have reduced Meckel’s and ceratohyal cartilages, with clefting occurring in the anterior of Meckel’s cartilage (Swartz et al., 2011). Therefore, it is likely that both TGF-β and BMP signaling play roles in shaping rod-shaped cartilage elements in addition to the trabeculae and ethmoid plate of the neurocranium (Swartz et al., 2011). However, how these pathways mediate cartilage morphogenesis in the zebrafish is currently uncharacterized. Previous studies in the zebrafish have indicated that two main processes fine-tune the shaping of cartilage elements during morphogenesis of the zebrafish craniofacial skeleton. The first is the acquisition of planar polarity, which mediates convergence-extension movements in the zebrafish craniofacial elements, allowing them to acquire their proper shapes. This is mediated by both Fat/Dchs signaling and noncanonical Wnt signaling, which are critical for the acquisition of chondrocyte polarity during morphogenesis of craniofacial cartilage (Kamel et al., 2013; Le Pabic et al., 2014; Sisson et al., 2015; Rochard et al., 2016; Ling et al., 2017; Dranow et al., 2023). The second is proper production and secretion of extracellular matrix proteins, which is intimately tied to chondrocyte identity. Mutations in sox9a, the zebrafish paralog of the cartilage master regulator Sox9, results in the formation of pre-cartilage condensations but prevents cartilage morphogenesis, indicating that cartilage identity is necessary for subsequent cartilage morphogenesis (Yan et al., 2002). Furthermore, mutations in components necessary for the synthesis or export of ECM components, particularly collagen, are necessary for cartilage morphogenesis, and aberration of these processes result in aberrant chondrocyte stacking and, thus, disrupted cartilage morphogenesis (Clément et al., 2008; Sarmah et al., 2010; Melville et al., 2011). One of the earliest identified biological outputs of TGF-β superfamily signaling is the regulation of ECM secretion, ECM modifying enzymes, and ECM interacting proteins (e.g., integrins) [Reviewed by Verrecchia and Mauviel (2002)]. Therefore, these pathways may regulate the timing of cartilage differentiation and/or cartilage ECM formation, thus regulating the shaping of these elements. Indeed, studies investigating the role of micro RNAs in zebrafish craniofacial development have provided interesting insights into the role of BMP signaling and cartilage morphogenesis: mir92a is necessary for the degradation of nog3 mRNA, thus permitting the activation of BMP signaling in the PAs, which is necessary for the formation and morphogenesis of pharyngeal cartilages (Ning et al., 2013).
Joint development
Although zebrafish are emerging as a useful model for joint development and disease, studies in murine models have greatly informed our understanding of synovial joint development and have demonstrated a critical role for BMP and TGF-β signaling in this process. Prior to joint formation, the skeleton is laid down as uninterrupted primordia of mesenchymal skeletal progenitors. The first morphological sign of a developing joint is the formation of a condensation within these mesenchymal progenitors at the presumptive joint called the interzone (Pacifici et al., 2006; Koyama et al., 2008). As joint development proceeds, the interzone adopts a tri-layered structure, where the presumptive joint capsule is flanked by chondrogenic outer layers that give rise to articular cartilage (Pacifici et al., 2006; Koyama et al., 2008). Cells in the joint capsule are specified into progenitors for many joint structures, and the remaining joint capsule then cavitates to form the joint space (Pacifici et al., 2006; Koyama et al., 2008). During embryogenesis and post-natal life, joint progenitors mature into ligaments, tendons, menisci, the synovium, and articular cartilage, forming a mature joint (Pacifici et al., 2006; Koyama et al., 2008). The joint cavity also becomes filled with synovial fluid, which contains macromolecules (e.g., Prg4/Lubricin) that provide lubrication to the joint (Rhee et al., 2005; Koyama et al., 2014). Histological analysis of joints demonstrates a conserved tissue organization between mammalian joints and zebrafish craniofacial joints, and live imaging of joint development in zebrafish suggests that joint specification and cavitation occurs similarly in zebrafish and mammals, suggesting a homologous origin for teleost and mammalian joints (Askary et al., 2016). Accordingly, several joints in the zebrafish craniofacial skeleton express prg4b during the larval and juvenile periods, including the jaw joint, the hyoid joint, and the articulation between the ceratohyals and the basial, indicating a conservation of lubricated joints throughout evolution (Askary et al., 2016). Zebrafish lacking prg4b display a progressive loss of jaw joint integrity, suggesting that lubrication is necessary for the maintenance of zebrafish joints (Askary et al., 2016). Given the similarities between zebrafish and mammalian joint development and the rapid formation of zebrafish craniofacial joints, zebrafish are a potentially useful model for studying the mechanism of joint formation. However, caution should be used when extrapolating findings in zebrafish to mammals, as the zebrafish jaw joint is more similar to the incudomalleolar joint (the articulation between the incus and malleus in the mammalian middle ear) rather than the joints of the appendicular skeleton (Askary et al., 2016).
Arguably, development of the zebrafish jaw joint begins during early patterning of the first and second PAs. BMP signaling emanating from the ventral PAs is necessary for proper positioning of the jaw joint; bmp4 overexpression or loss of grem2b abrogates bapx1 expression in the intermediate domains of the PAs, which marks the nascent joint (Zuniga et al., 2011). Therefore, early events in arch patterning are directly responsible for the correct position of joints in the zebrafish craniofacial skeleton. Additionally, BMP signaling has been extensively linked to joint formation in murine models. In mouse, Bmp2, Bmp4, Bmp7, Gdf5, Gdf6, Gdf7, Grem2, and Noggin are all expressed in or around the joint interzone, with Gdf5 being expressed in all joint interzones (Hogan, 1996; Wolfman et al., 1997; Brunet et al., 1998; Settle et al., 2003). Additionally, Noggin mutant mice show a widespread loss of joints throughout the body, indicating that inhibiting BMP signaling in the interzone is vital for joint formation (Brunet et al., 1998). This is consistent with zebrafish studies that show that overexpression of bmp4 blocks the formation of joints in the craniofacial skeleton (Askary et al., 2015). Therefore, gradients of BMP activity must be precisely regulated to ensure the proper patterning and development of the nascent joint. A subgroup of BMP ligands, termed the GDF5/6/7 subgroup, appear to have particularly important (but complicated) roles in joint development. In mice, one of the first transcripts expressed in the interzone is Gdf5, and Gdf5 is widely used as an early marker for the joint interzone (Storm et al., 1994). Interestingly, only a subset of joints are affected in mice mutant for Gdf5 or Gdf6, and Gdf5;Gdf6 double mutants do not phenocopy the widespread loss of joints observed in Noggin mutants, indicating that Gdf5/6 are not necessary for the induction of all joints (Storm et al., 1994; Storm and Kingsley, 1996; Settle et al., 2003). Rather, several lines of evidence seem to suggest that Gdf5/6/7, particularly Gdf5, regulate BMP signaling in and around the joint interzone. It has been suggested that Gdf5 competes with other BMPs for occupancy of Bmpr1a in the interzone but does not efficiently transduce a signal through this receptor, thereby preventing high BMP activity in the interzone (Lyons and Rosen, 2019). It has also been proposed that Gdf5 induces BMP signaling through Bmpr1b, which is expressed in the articular cartilage but not the joint interzone (Lyons and Rosen, 2019). Additionally, Gdf5 has been hypothesized to act as a “sink” for Noggin, preventing it from diffusing into the articular cartilage zone and thereby permitting BMP activity in this region (Lyons and Rosen, 2019). Investigations into gdf5 and gdf6a (the zebrafish homologs of Gdf5/GDF5 and Gdf6/GDF6, respectively) and their role in zebrafish skeletal and jaw development are emerging. In zebrafish, gdf5 is expressed in the primary jaw joint, the ceratohyal, and in the future basihyal cartilage at 77 hpf, suggesting some role in skeletal patterning and joint formation in zebrafish (Reed and Mortlock, 2010). However, gdf5 mutants do not display an overt craniofacial phenotype, suggesting either functional redundancy with other factors in these processes or a subtle phenotype that cannot be visualized with commonly employed histological methods (Waldmann et al., 2022). In contrast, gdf6a mutants do display a craniofacial phenotype; in gdf6a mutant zebrafish, the ceratohyal incorrectly articulates with the midline basihyal such that the ceratohyals appear offset rather than in-line with each other (Reed and Mortlock, 2010). Additionally, the hypobranchials are misshapen in gdf6a mutants, which results in lateral deviation of the ceratobranchials (Reed and Mortlock, 2010). In contrast to gdf5, which is expressed in laterally positioned joints in the craniofacial skeleton, gdf6a is expressed along the developing midline of the zebrafish craniofacial skeleton, suggesting potentially divergent roles for these signaling molecules relative to one another in zebrafish (Reed and Mortlock, 2010).
Calvaria development and suture homeostasis
Studies in murine models have implicated BMP signaling in regulating calvaria growth and suture homeostasis. Specifically, it is likely that BMP signaling plays a role in promoting osteogenesis in the suture. Msx2, a downstream target of BMP signaling, is expressed the osteogenic front of calvaria, and forced expression of both mutant and wild-type Msx2 in the sutures results in premature suture ossification, indicating that inhibition of BMP signaling in the sutures is vital for the proper maintenance of suture mesenchyme (Liu et al., 1995). Msx2 knockout mice display defects in the formation and ossification of calvaria, indicating that BMP signaling is necessary for ossification and must be regulated in the sutures to prevent premature fusion (Ishii et al., 2003; Han et al., 2007). Moreover, forced activation of BMP signaling in suture neural crest via expression of constitutively active Bmpr1a results in premature suture fusion, further suggesting that BMP signaling promotes ossification in the suture and must be precisely regulated to maintain suture homeostasis (Komatsu et al., 2013; Ueharu et al., 2023). Accordingly, the BMP antagonist Noggin is expressed in the suture mesenchyme, and misexpression of Noggin in the sutures or treatment of the sutures with recombinant Noggin protein delays suture closure (Warren et al., 2003; Shen et al., 2009). TGF-β also appears to be involved in this process; TGF-β, via Smad2/3, promotes suture closure and is regulated by Smad7 to prevent premature suture closure (Zhou et al., 2014). Deficiencies in other components of the BMP/TGF-β pathways (e.g., Gdf6) have been found to cause premature suture fusion, indicating that more components of the BMP/TGF-β pathways are likely involved in this complicated process (Settle et al., 2003). Although murine studies have been crucial for understanding the biology of cranial sutures and how suture closure is regulated, zebrafish are emerging as a model organism for understanding this process; zebrafish have been used to model Saethre-Chotzen syndrome (caused by mutations in TWIST1 and TCF12) and craniosynostosis caused by alterations to retinoic acid synthesis (Laue et al., 2011; Teng et al., 2018). A recently published zebrafish study identified putative enhancers of bmp2 and bmper (a regulator of BMP signaling) that drive reporter gene expression in the osteogenic fronts of transgenic zebrafish calvaria, suggesting that the function of BMP signaling in cranial development and suture homeostasis is conserved in zebrafish (He et al., 2023).
Human craniofacial diseases and TGF-β signaling
As discussed in the previous section, BMP/TGF-β signaling plays a critical role in the specification, migration, patterning, and late cell behaviors of neural crest. Genetic analyses in humans strongly support such findings and demonstrate conclusively that craniofacial, skeletal, and joint diseases are caused by aberrant TGF-β superfamily signaling. Here, we review selected craniofacial, skeletal, and joint diseases and their association with BMP/TGF-β signaling. The information presented in this section is summarized in Table 3.
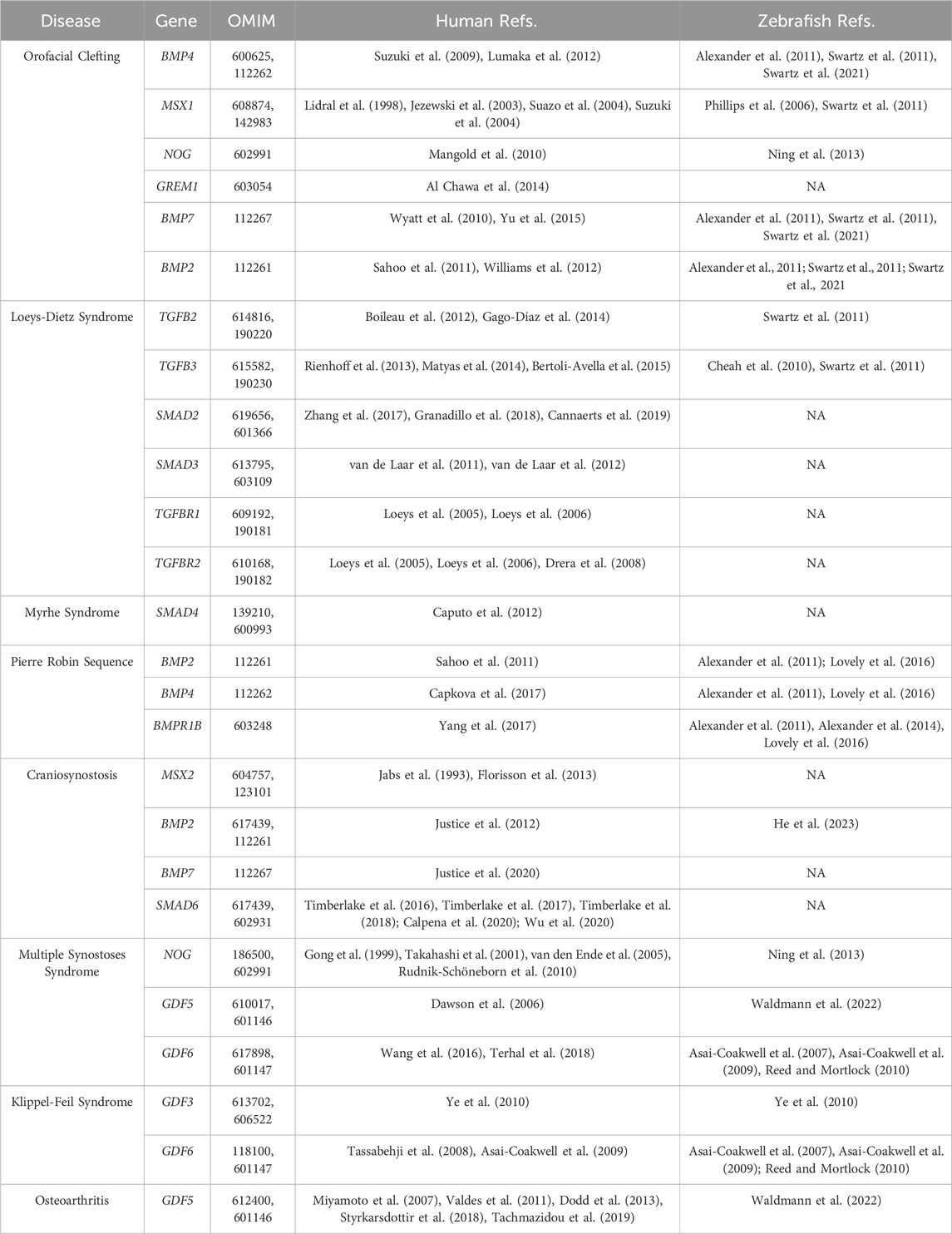
TABLE 3. Summary of Human Skeletal Conditions and their Associated Human Loci and Zebrafish Studies. Listed below are human craniofacial/skeletal diseases associated with aberrant TGF-β superfamily signaling, followed by their human gene symbol, their OMIM identifier, and references generated from human and zebrafish studies that implicate each gene/pathway in the associate disease. Refs. = References.
Orofacial clefting and Pierre Robin Sequence
Orofacial clefting (OFC) is the most common craniofacial abnormality and one of the most common congenital abnormalities in the world with an incidence of 1.2/1000 live births worldwide (Martin and Swan, 2023). OFC is characterized by gaps in the primary palate (the lip, alveolus, and small region of hard palate anterior to incisive foramen) or the secondary palate (the remainder of the hard palate posterior to the incisive foramen and the soft palate) (Martin and Swan, 2023). OFC is a common feature of many syndromes; 1/3 of all OFC cases are associated with a syndrome (Martin and Swan, 2023). While there is some variability in the classification system used during diagnosis, OFC is commonly categorized by whether the clefting affects the lip, palate, or both, whether the clefting is bilateral or unilateral, and the degree to which the clefting affects the lip, palate, or both (Martin and Swan, 2023). OFC can also be categorized as syndromic (i.e., being associated with other phenotypes) or non-syndromic (i.e., presenting alone) (Martin and Swan, 2023). OFC of the primary palate results when the anterior maxillary processes fail to fuse with the nasal processes along the midline of the developing embryo, whereas OFC of the secondary palate occurs when the palatine shelves (the posterior aspect of the maxillary processes) fail to rotate and fuse at the midline (Martin and Swan, 2023). Palatal fusion involves the coordination of many biological processes including cell identity, migration, adhesion, death, and proliferation, and requires the careful coordination of gene expression and signaling pathway regulation (Martin and Swan, 2023; Won et al., 2023). Additionally, OFC usually occurs from a combination of environmental and genetic causes (Martin and Swan, 2023). BMP and TGF-β have been strongly implicated in the etiology of orofacial clefting in humans. Pathogenic mutations in BMP4 have been associated with non-syndromic OFC, and microdeletions of 14q22-23, which encompasses BMP4, results in syndromic OFC (Suzuki et al., 2009; Lumaka et al., 2012). Moreover, mutations in MSX1, a downstream target of BMP signaling, have also been associated with OFC, further implicating this pathway in the etiology of OFC (Lidral et al., 1998; Jezewski et al., 2003; Suazo et al., 2004; Suzuki et al., 2004). Genome-wide association studies (GWAS) and burden analysis have implicated the BMP antagonists NOG and GREM1 as genetic risk factors for non-syndromic OFC (Mangold et al., 2010; Al Chawa et al., 2014). BMP7 mutations have also been associated with syndromic and non-syndromic OFC, and a deletion at 20p12.3 that encompasses BMP2 has been linked to syndromic OFC (Wyatt et al., 2010; Sahoo et al., 2011; Williams et al., 2012; Yu et al., 2015). While there is limited evidence that variants in the TGF-β pathway cause non-syndromic OFC, skeletal features including OFC and other craniofacial abnormalities are occasionally found in patients with Loeys-Dietz syndrome, which are caused by mutations in TGFB2, TGFB3, SMAD2, SMAD3, TGFBR1, and TGFBR2, suggesting that this pathway is important for craniofacial development and disease (Loeys et al., 2005; Loeys et al., 2006). Finally, Myhre syndrome, which is partially characterized by the presence of OFC, is caused by mutations in SMAD4, implicating the TGF-β superfamily as a whole in OFC (Caputo et al., 2012).
Given the morphogenetic conservation between the zebrafish ethmoid plate and the mammalian hard palate, zebrafish has served as a useful model for studying the genetic and developmental origins of OFC (Swartz et al., 2011). BMP signaling plays multiple roles in ethmoid plate development, with alterations to BMP signaling in both early and late ethmoid development resulting in OFC-like phenotypes (Alexander et al., 2011; Swartz et al., 2011; Swartz et al., 2021). Perhaps the most useful role for zebrafish in studying palate formation and OFC is studying gene-environment interactions [Reviewed by Raterman et al. (2020)]. The ex-utero development of zebrafish facilitates manipulation of the developmental environment and observation of the subsequent effects on development. This also presents a unique opportunity to study gene-environment interactions; mutants with mild/no phenotype can be exposed to an environmental condition and observed to determine whether the OFC phenotype is worsened or ameliorated, allowing for the delineation of gene-environment interactions. Indeed, a screen to identify loci that are sensitive to alcohol exposure revealed that several genes interact with ethanol to produce a more severe craniofacial phenotype (Swartz et al., 2014).
Pierre Robin Sequence (PRS) is a craniofacial abnormality related to OFC (specifically cleft palate). PRS is characterized by mandibular hypoplasia (underdevelopment of the mandible), which results in a mispositioned tongue, leading to airway obstruction (Robin, 1923). PRS has an incidence of 1/8500 to 1/14000 live births and can severely impact the quality of life of affected individuals due to airway obstruction (Bush and Williams, 1983; Printzlau and Andersen, 2004). While the etiology of PRS is incompletely understood, it is hypothesized that PRS is caused, in part, by a failure of Meckel’s cartilage to elongate during embryogenesis, leading to a hypoplastic mandible. This prevents the tongue from being drawn forward during embryogenesis, resulting in a smaller oral volume (Seegmiller and Fraser, 1977). This reduced oral volume is predicted to prevent the palatal shelves from migrating close enough together at the midline, thereby preventing them from fusing and causing cleft palate (Seegmiller and Fraser, 1977). While genetic causes of PRS have not been completely elucidated, some patients with microdeletions at 20p12.3 and 14q22.1-q32.1, which encompass BMP2 and BMP4, respectively, present with PRS, suggesting that BMP signaling plays a key role in mandible development and PRS (Sahoo et al., 2011; Capkova et al., 2017). Consistent with this model, patients with mutations in BMPR1B also present with PRS (Yang et al., 2017).
Studies in zebrafish suggest that aberrations to BMP signaling in several stages of craniofacial development may contribute to the development of PRS phenotypes and, therefore, OFC. Changes to neural crest induction can alter the number, migration, or survival of cranial neural crest cells can affect later craniofacial development, which can result in the reduction of Meckel’s cartilage and, therefore, a reduced jaw, suggesting that early events might contribute to the pathogenesis of this disease (Phillips et al., 2006; Cheah et al., 2010; Das and Crump, 2012). Additionally, formation of the pharyngeal endoderm, which requires BMP signaling, is necessary for the proper development of Meckel’s cartilage, suggesting that aberrant pharyngeal endoderm formation might explain the etiology of some cases of PRS (Lovely et al., 2016; Li et al., 2019b). Overactivation or elimination of BMP signaling during early PA patterning results in aberrant morphogenesis of several cartilage elements, including Meckel’s cartilage, implicating alterations to early PA patterning as a potential cause of PRS (Alexander et al., 2011; 2014; Zuniga et al., 2011). Knockdown and mutant studies have also demonstrated that both BMP and TGF-b signaling regulate fine-tuning of the shape of ventral elements, including the lower jaw. Determining how TGF-β and BMP signaling mediate this process will provide important insights into the pathogenesis of PRS (Swartz et al., 2011).
Craniosynostosis
Craniosynostosis is another relatively common craniofacial abnormality associated with BMP and TGF-β signaling. Craniosynostosis affects 1/2100-1/2500 live births in the United States and is a common feature of many syndromes (Lajeunie et al., 1995; Boulet et al., 2008). Craniosynostosis occurs when the sutures (the fibrous joints that separate the flat bones of the skull) ossify prematurely, leading to reduced shock absorption and increased pressure on the growing brain (Johnson and Wilkie, 2011). This, in turn, can cause neurocognitive deficits or intellectual disability, as well as increased pressure on the sensory organs that are housed in the skull such as the eyes and ears, resulting in deficits in sensory organ function (Johnson and Wilkie, 2011). Craniosynostosis is typically characterized by the sutures that are fused in an individual, and the cause of craniosynostosis can be genetic, environmental, or a combination of both (Johnson and Wilkie, 2011). Multiple signaling pathways have been found to regulate suture fusion, and mutations in these signaling components frequently cause craniosynostosis in humans. There is mounting evidence to suggest that BMP signaling regulates suture maintenance and closure. Mutations in MSX2 have been linked to Boston-type craniosynostosis (Jabs et al., 1993; Florisson et al., 2013). Additionally, haploinsufficiency of the BMP-specific inhibitory Smad SMAD6 is predicted to be a modifier of the penetrance of craniosynostosis, with mutations in SMAD6 increasing the risk of developing craniosynostosis (Timberlake et al., 2016; Timberlake et al., 2017; Timberlake et al., 2018; Calpena et al., 2020; Wu et al., 2020). GWAS has also identified BMP2 and BMP7 as susceptibility loci for craniosynostosis, further implicating this pathway as an important regulator of suture closure and craniosynostosis (Justice et al., 2012; Justice et al., 2020). Accordingly, whole exome sequencing of craniosynostosis patients reveals that potentially damaging mutations cluster in genes encoding components of the BMP signaling cascade (Timberlake et al., 2023). Although BMP signaling plays an undoubtedly important role in the etiology of craniosynostosis, many craniosynostosis cases are caused by mutations in components of the FGF signaling pathway (Johnson and Wilkie, 2011). Many of the variants in BMP signaling components (e.g., SMAD6, BMP2, BMP7) increase the risk of craniosynostosis rather than causing craniosynostosis directly, suggesting that interactions between pathways (namely, BMP and FGF) may contribute to the etiology of this disorder.
Studies have shown that zebrafish are a tractable model for studying suture biology and craniosynostosis, and the prospect of using zebrafish to study the suture biology and the pathogenesis of craniosynostosis is exciting (Laue et al., 2011; Teng et al., 2018). With respect to BMP signaling, a recent zebrafish study identified that GWAS variants associated with BMP2 lie in putative enhancer regions for BMP2 and BMPER, and that these enhancers likely drive the expression of these genes in the osteogenic fronts of the frontal bones, further implicating BMP signaling in suture homeostasis and craniosynostosis (Justice et al., 2012; He et al., 2023). Furthermore, yeast 2 hybrid assays indicated that the GWAS variants in these enhancers abrogate transcription factor binding ability, further suggesting that altered BMP signaling contributes to the pathogenesis of craniosynostosis (He et al., 2023). Moreover, unlike human sutures, which fuse by adulthood, zebrafish sutures remain patent throughout life; investigations into the mechanisms by which suture patency is maintained in zebrafish could inform the development of treatments or therapies for individuals affected by craniosynostosis and advance our understanding of stem cells in regenerative medicine (Topczewska et al., 2016). Indeed, recent studies in mouse have identified suture mesenchyme as a promising source of stem cells for regeneration of skeletal structures (particularly calvaria) and have demonstrated the role of BMP signaling in this process (Park et al., 2016; Vural et al., 2017). Complementing mouse studies with investigations into the mechanisms of zebrafish suture patency will surely advance our understanding of the etiology of craniosynostosis and provide insight into the use of suture stem cells as a therapeutic agent.
Disorders of joints
While not strictly a craniofacial disease, diseases of joints are commonly comorbid with craniofacial abnormalities. For example, ossicle abnormalities are a common feature of multiple synostosis syndrome and some individuals with Klippel-Feil syndrome have laryngeal cartilage abnormalities. Additionally, the joints in the zebrafish craniofacial skeleton are increasingly proving to be a tractable system to study joint formation and disease.
Multiple synostoses syndrome
Multiple synostoses syndrome (MSS), also referred to as WL syndrome, deafness-symphalangism syndrome, or facio-audio-symphalangism syndrome, is a congenital skeletal disease characterized by multiple joint fusions, which frequently occur in the phalanges (Takahashi et al., 2001). Other common features of this syndrome are conductive hearing loss caused by fusion of the articulations between the ossicles and characteristic facial features, suggesting that this disease also affects components of the craniofacial skeleton (Takahashi et al., 2001). While the incidence of MSS is not known, it is predicted to be very rare (<1/1 000 000 live births) (OMIM #186500). The first identified causes of MSS were mutations in the BMP antagonist NOG (Gong et al., 1999; Takahashi et al., 2001; van den Ende et al., 2005; Rudnik-Schöneborn et al., 2010). Given that mutations in NOG also cause other skeletal diseases, including proximal symphalangism and brachydactyly, it has been proposed that NOG mutations cause a spectrum of diseases termed NOG-related symphalangism spectrum disorder (Potti et al., 2011). Additionally, mutations in the NOG-responsive ligands GDF5 and GDF6 also cause disease, but patients with mutations in GDF5 lack the hearing loss observed in patients with mutations in GDF6 and NOG, suggesting that GDF5 does not regulate development of the ossicles (Dawson et al., 2006; Wang et al., 2016; Terhal et al., 2018). MSS is predicted to be caused by haploinsufficiency of NOG, which results in an overactivation of BMP signaling in the growth plates and joint primordia, resulting in the overgrowth of the growth plates and fusion of the joints (Brunet et al., 1998). Accordingly, mutations in GDF5 and GDF6 that cause MSS frequently result in the overactivation of BMP signaling. For example, mutations in GDF5 or GDF6 that make their corresponding protein resistant to inhibition by NOG cause MSS, suggesting that the inhibition of BMP signaling via NOG is vital for joint development (Schwaerzer et al., 2012; Wang et al., 2016; Yu et al., 2023).
Joint development requires a fine balance of BMP signaling and for gradient strength and position to be regulated; both the loss of BMP ligands and antagonists of BMP signaling cause joint fusions, suggesting that a carefully orchestrated balance of BMP is necessary during joint development. Zebrafish are a system that is well-suited for the study of signaling gradients and morphogenesis. In zebrafish, several tools exist for studying the temporal and spatial dynamics of BMP signaling, including transgenic lines that allow for the observation of BMP signaling output (e.g., Tg(BRE:GFP)) or the temporal activation or attenuation of BMP signaling (e.g., Tg(hsp70l:dnBmpr1a) or Tg(hsp70l:bmp4)), allowing for the study of BMP signaling during the window of time that craniofacial jaw development is occurring (Alexander et al., 2011; 2014; Zuniga et al., 2011). Additionally, given its utility as a model for live imaging transgenic strains, zebrafish can be used to visualize all stages of joint formation in-real time, allowing for the delineation of the role of BMP signaling at each step of joint specification and morphogenesis and, as a result, providing insight into joint development and the etiology of diseases of joint fusion such as MSS. Indeed, studies have already taken advantage of the zebrafish model for studying joint development and have provided novel insight into the molecular mechanisms of chondrocyte maturation as it relates to joint development (Askary et al., 2015).
Klippel-Feil Syndrome
Klippel-Feil syndrome (KFS) is a rare congenital abnormality characterized mainly by the fusion of the cervical vertebrae, which causes a shortened neck, a limited range of motion in the neck, and a low posterior hairline (Klippel, 1912). Patients with KFS have also been reported to have laryngeal cartilage abnormalities, craniofacial abnormalities, and scoliosis (Clarke et al., 1994). While the reported incidence of KFS suggests it is rare (1/40,000 live births worldwide), many cases are not identified at birth and go unnoticed until an injury results in radiographic imaging of the neck, suggesting that the actual incidence of KFS is higher (Gruber et al., 2018). While mutations in the TGF-β superfamily ligands GDF6 and GDF3 have been shown to cause KFS, KFS can be genetically heterogeneous (Tassabehji et al., 2008; Asai-Coakwell et al., 2009; Ye et al., 2010). Historically, KFS was thought to be caused by defective cervical somite segmentation and differentiation into cartilage and bone, resulting in fused cervical vertebrae. However, while KFS is not explicitly a craniofacial abnormality, it has been suggested that the pathogenesis of KFS is neural crest-derived in nature; the part of the cervical spine that is commonly affected in patients with KFS is derived from the post-otic neural crest, and KFS can arise from abnormalities in neural crest fate choices in the cervical spine (Matsuoka et al., 2005). Zebrafish gdf6a mutants and gdf3 morphants display phenotypes reminiscent of KFS, suggesting that zebrafish are a viable model for studying the role of these ligands in the etiology of KFS and their effect on post-otic neural crest activity (Asai-Coakwell et al., 2007; Asai-Coakwell et al., 2009; Ye et al., 2010). To date, Gdf3 and Gdf6 have yet to be studied regarding their potential roles in regulating of post-otic neural crest cell activity.
Osteoarthritis
Osteoarthritis (OA) is the most common joint disease worldwide, affecting up to 10% of men and 18% of women (Felson et al., 2000; Franke et al., 2023). OA is characterized by the breakdown of tissues in the joint, including articular cartilage, tendons and ligaments, the synovium (joint capsule), and bone, resulting in swelling of the joint, which leads to pain and reduced mobility (Brandt et al., 2009). OA is a major source of pain and disability worldwide, which, in turn, leads to increased socioeconomic burden (Woolf and Pfleger, 2003; Gupta et al., 2005; Schofield et al., 2013; Hunter et al., 2014). The main treatment courses for OA are pain management and surgical cartilage replacement, which, like any other invasive surgery, can result in complications (Nelson et al., 2014). OA is likely a complex disease, with the presence of multiple susceptibility alleles and environmental factors influencing the onset and severity of disease (Felson et al., 2000). Increased age and joint injury are the main risk factors for developing OA, but investigations into the causative factors of OA suggest that some individuals have a genetic predisposition to OA (Felson et al., 2000). Using several approaches, including GWAS, many loci have been identified as potentially conferring susceptibility to OA, including many members of the TGF-β superfamily. For example, GWAS have identified variants in the promoter of the key joint regulator GDF5 as risk factors for OA (Miyamoto et al., 2007; Valdes et al., 2011; Dodd et al., 2013; Styrkarsdottir et al., 2018; Tachmazidou et al., 2019). Interestingly, many variants associated with OA risk are frequently found in non-coding regulatory elements rather than the open reading frames of coding genes, suggesting that slight alterations to the expression of genes, rather than the activity/function of the gene itself, are responsible for increased OA risk (Aubourg et al., 2022). Moreover, loss of Grem1-expressing chondrogenic progenitor cells in mouse joints results in an OA phenotype, further implicating altered BMP signaling in the progression of this disease (Ng et al., 2023). Additionally, it has been hypothesized that slight variations to the way the joint develops during embryogenesis (via modulating GDF5 expression/activity) can influence how a joint functions in adulthood, which may influence the likelihood of developing OA later in life (Kiapour et al., 2018; Pregizer et al., 2018; Richard et al., 2020). Therefore, studying how joints develop during embryogenesis can provide insight into the risk factors for developing OA later in life. Additionally, many of the alterations in biological processes that lead to OA are developmentally relevant; understanding these developmental processes can increase our understanding of therapeutic strategies for OA.
While mammalian models have historically been used to study OA, zebrafish are gaining traction as a model for studying joint injury and disease. As stated previously, several of the joints in the zebrafish craniofacial skeleton are lubricated, and elimination of joint lubrication in zebrafish results in a progressive OA phenotype, demonstrating the tractability of zebrafish as a model for studying OA (Askary et al., 2016). The relatively short lifespan of zebrafish allows investigators to monitor joint health and integrity throughout their lifetime, allowing for relatively rapid analysis of joint maintenance under several conditions. Additionally, unlike mammals, zebrafish cartilage regenerates over the course of its lifetime; studying the molecular basis of this regeneration and injury repair can provide valuable insight into the development of preventative therapies and treatments for joint diseases like OA (Askary et al., 2016; Smeeton et al., 2022).
Conclusions and future perspectives
Zebrafish have proven to be an invaluable resource for dissecting the molecular pathways that regulate craniofacial development and unraveling the pathogenesis of craniofacial abnormalities. They have also proved to be particularly valuable for investigating the role of critical signaling pathways, including BMP and TGF-β, in craniofacial development, due to the ability to manipulate signaling temporally to bypass the early necessity of these signaling pathways in gastrulation and body axis patterning. In recent years, researchers have harnessed the extrauterine development of zebrafish to create single-cell atlases of craniofacial development using single-cell RNA sequencing and to generate transgenic zebrafish lines to optogenetically control Nodal and BMP signaling (Fabian et al., 2022; Rogers et al., 2023). These resources will undoubtedly be paramount for furthering our understanding of signaling and craniofacial morphogenesis and complement other recent optogenetic and transcriptomic studies performed in different systems (Li et al., 2019a; Holmes et al., 2020; Farmer et al., 2021; Humphreys et al., 2023; Siewert et al., 2023; Sun et al., 2023). Zebrafish are also increasingly being used to model human diseases such as OFC, craniosynostosis, and OA, and may therefore provide valuable insight into the pathogenesis, treatment, and prevention of these diseases (Laue et al., 2011; Askary et al., 2016; Teng et al., 2018; Smeeton et al., 2022). Although studies in zebrafish and mouse have provided a wealth of information about the mechanisms underlying craniofacial development and disease, there are still unanswered questions about TGF-β/BMP signaling and their role in these processes. Much of what we know about the role of TGF-β/BMP in craniofacial development is based on the “canonical” Smad pathway. However, there is relatively little work, especially in zebrafish, examining the role of non-canonical TGF-β/BMP signaling in craniofacial development and disease. TGF-β/BMP ligands have been shown to activate TGF-β Activated Kinase (TAK1), which has been shown to regulate cellular behavior independent of transcriptional activation of target genes (Yamaguchi et al., 1995). Indeed, mice with TAK1-deficient cranial neural crest cells develop OFC through both Smad and non-Smad pathways (Song et al., 2013; Yumoto et al., 2013; Liu et al., 2018). Therefore, more investigation into the role of these noncanonical pathways in craniofacial development is warranted. Additionally, while many craniofacial diseases can be caused by mutations in components of a variety of different signaling pathways, the crosstalk between these pathways is frequently overlooked (e.g., the interaction between BMP and FGF in craniosynostosis). Investigating the interactions between multiple signaling pathways can inform both the etiology of disease in humans and the development of therapies or preventative measures that take signaling crosstalk into consideration.
Author contributions
SF: Conceptualization, Writing–original draft, Writing–review and editing. AW: Conceptualization, Funding acquisition, Supervision, Writing–original draft, Writing–review and editing.
Funding
The author(s) declare financial support was received for the research, authorship, and/or publication of this article. This work was funded by a Natural Sciences and Engineering Research Council (NSERC) Discovery Grant (RGPIN 2022-03658).
Conflict of interest
The authors declare that the research was conducted in the absence of any commercial or financial relationships that could be construed as a potential conflict of interest.
Publisher’s note
All claims expressed in this article are solely those of the authors and do not necessarily represent those of their affiliated organizations, or those of the publisher, the editors and the reviewers. Any product that may be evaluated in this article, or claim that may be made by its manufacturer, is not guaranteed or endorsed by the publisher.
References
Abdollah, S., Macías-Silva, M., Tsukazaki, T., Hayashi, H., Attisano, L., and Wrana, J. L. (1997). TbetaRI phosphorylation of Smad2 on Ser465 and Ser467 is required for Smad2-Smad4 complex formation and signaling. J. Biol. Chem. 272 (44), 27678–27685. doi:10.1074/jbc.272.44.27678
Ahsan, K., Singh, N., Rocha, M., Huang, C., and Prince, V. E. (2019). Prickle1 is required for EMT and migration of zebrafish cranial neural crest. Dev. Biol. 448 (1), 16–35. doi:10.1016/j.ydbio.2019.01.018
Al Chawa, T., Ludwig, K. U., Fier, H., Pötzsch, B., Reich, R. H., Schmidt, G., et al. (2014). Nonsyndromic cleft lip with or without cleft palate: increased burden of rare variants within Gremlin-1, a component of the bone morphogenetic protein 4 pathway. Birth Defects Res. Part A Clin. Mol. Teratol. 100 (6), 493–498. doi:10.1002/bdra.23244
Alexander, C., Piloto, S., Le Pabic, P., and Schilling, T. F. (2014). Wnt signaling interacts with bmp and Edn1 to regulate dorsal-ventral patterning and growth of the craniofacial skeleton. PLOS Genet. 10 (7), e1004479. doi:10.1371/journal.pgen.1004479
Alexander, C., Zuniga, E., Blitz, I. L., Wada, N., Le Pabic, P., Javidan, Y., et al. (2011). Combinatorial roles for BMPs and Endothelin 1 in patterning the dorsal-ventral axis of the craniofacial skeleton. Development 138 (23), 5135–5146. doi:10.1242/dev.067801
Alvarado, E., Yousefelahiyeh, M., Alvarado, G., Shang, R., Whitman, T., Martinez, A., et al. (2016). Wdr68 mediates dorsal and ventral patterning events for craniofacial development. PLOS ONE 11 (11), e0166984. doi:10.1371/journal.pone.0166984
Annes, J. P., Chen, Y., Munger, J. S., and Rifkin, D. B. (2004). Integrin alphaVbeta6-mediated activation of latent TGF-beta requires the latent TGF-beta binding protein-1. J. Cell Biol. 165 (5), 723–734. doi:10.1083/jcb.200312172
Anthwal, N., Joshi, L., and Tucker, A. S. (2013). Evolution of the mammalian middle ear and jaw: adaptations and novel structures. J. Anat. 222 (1), 147–160. doi:10.1111/j.1469-7580.2012.01526.x
Asai-Coakwell, M., French, C. R., Berry, K. M., Ye, M., Koss, R., Somerville, M., et al. (2007). GDF6, a novel locus for a spectrum of ocular developmental anomalies. Am. J. Hum. Genet. 80 (2), 306–315. doi:10.1086/511280
Asai-Coakwell, M., French, C. R., Ye, M., Garcha, K., Bigot, K., Perera, A. G., et al. (2009). Incomplete penetrance and phenotypic variability characterize Gdf6-attributable oculo-skeletal phenotypes. Hum. Mol. Genet. 18 (6), 1110–1121. doi:10.1093/hmg/ddp008
Askary, A., Mork, L., Paul, S., He, X., Izuhara, A. K., Gopalakrishnan, S., et al. (2015). Iroquois proteins promote skeletal joint formation by maintaining chondrocytes in an immature state. Dev. Cell 35 (3), 358–365. doi:10.1016/j.devcel.2015.10.004
Askary, A., Smeeton, J., Paul, S., Schindler, S., Braasch, I., Ellis, N. A., et al. (2016). Ancient origin of lubricated joints in bony vertebrates. eLife 5, e16415. doi:10.7554/eLife.16415
Aubourg, G., Rice, S. J., Bruce-Wootton, P., and Loughlin, J. (2022). Genetics of osteoarthritis. Osteoarthr. Cartil. 30 (5), 636–649. doi:10.1016/j.joca.2021.03.002
Baarends, W. M., van Helmond, M. J., Post, M., van der Schoot, P. J., Hoogerbrugge, J. W., de Winter, J. P., et al. (1994). A novel member of the transmembrane serine/threonine kinase receptor family is specifically expressed in the gonads and in mesenchymal cells adjacent to the müllerian duct. Development 120 (1), 189–197. doi:10.1242/dev.120.1.189
Baker, J. C., and Harland, R. M. (1996). A novel mesoderm inducer, Madr2, functions in the activin signal transduction pathway. Genes & Dev. 10 (15), 1880–1889. doi:10.1101/gad.10.15.1880
Barrallo-Gimeno, A., Holzschuh, J., Driever, W., and Knapik, E. W. (2004). Neural crest survival and differentiation in zebrafish depends on mont blanc/tfap2a gene function. Development 131 (7), 1463–1477. doi:10.1242/dev.01033
Barske, L., Askary, A., Zuniga, E., Balczerski, B., Bump, P., Nichols, J. T., et al. (2016). Competition between jagged-notch and Endothelin1 signaling selectively restricts cartilage formation in the zebrafish upper face. PLOS Genet. 12 (4), e1005967. doi:10.1371/journal.pgen.1005967
Benchabane, H., and Wrana, J. L. (2003). GATA-and Smad1-dependent enhancers in the Smad7 gene differentially interpret bone morphogenetic protein concentrations. Mol. Cell. Biol. 23 (18), 6646–6661. doi:10.1128/mcb.23.18.6646-6661.2003
Berndt, J. D., Clay, M. R., Langenberg, T., and Halloran, M. C. (2008). Rho-kinase and myosin II affect dynamic neural crest cell behaviors during epithelial to mesenchymal transition in vivo. Dev. Biol. 324 (2), 236–244. doi:10.1016/j.ydbio.2008.09.013
Bertoli-Avella, A. M., Gillis, E., Morisaki, H., Verhagen, J. M. A., de Graaf, B. M., van de Beek, G., et al. (2015). Mutations in a TGF-β ligand, TGFB3, cause syndromic aortic aneurysms and dissections. J. Am. Coll. Cardiol. 65 (13), 1324–1336. doi:10.1016/j.jacc.2015.01.040
Beverdam, A., Merlo, G. R., Paleari, L., Mantero, S., Genova, F., Barbieri, O., et al. (2002). Jaw transformation with gain of symmetry after Dlx5/Dlx6 inactivation: mirror of the past? Genesis 34 (4), 221–227. doi:10.1002/gene.10156
Boileau, C., Guo, D. C., Hanna, N., Regalado, E. S., Detaint, D., Gong, L., et al. (2012). TGFB2 mutations cause familial thoracic aortic aneurysms and dissections associated with mild systemic features of Marfan syndrome. Nat. Genet. 44 (8), 916–921. doi:10.1038/ng.2348
Bonilla-Claudio, M., Wang, J., Bai, Y., Klysik, E., Selever, J., and Martin, J. F. (2012). Bmp signaling regulates a dose-dependent transcriptional program to control facial skeletal development. Development 139 (4), 709–719. doi:10.1242/dev.073197
Boulet, S. L., Rasmussen, S. A., and Honein, M. A. (2008). A population-based study of craniosynostosis in metropolitan Atlanta, 1989–2003. Am. J. Med. Genet. Part A 146 (8), 984–991. doi:10.1002/ajmg.a.32208
Brandt, K. D., Dieppe, P., and Radin, E. (2009). Etiopathogenesis of osteoarthritis. Med. Clin. N. Am. 93 (1), 1–24. doi:10.1016/j.mcna.2008.08.009
Brown, K. A., Ham, A. J. L., Clark, C. N., Meller, N., Law, B. K., Chytil, A., et al. (2008). Identification of novel Smad2 and Smad3 associated proteins in response to TGF-beta1. J. Cell. Biochem. 105 (2), 596–611. doi:10.1002/jcb.21860
Bruce, D. L., and Sapkota, G. P. (2012). Phosphatases in SMAD regulation. FEBS Lett. 586 (14), 1897–1905. doi:10.1016/j.febslet.2012.02.001
Brunet, L. J., McMahon, J. A., McMahon, A. P., and Harland, R. M. (1998). Noggin, cartilage morphogenesis, and joint formation in the mammalian skeleton. Science 280 (5368), 1455–1457. doi:10.1126/science.280.5368.1455
Bush, P. G., and Williams, A. J. (1983). Incidence of the Robin anomalad (pierre Robin syndrome). Br. J. Plastic Surg. 36 (4), 434–437. doi:10.1016/0007-1226(83)90123-6
Calpena, E., Cuellar, A., Bala, K., Swagemakers, S. M. A., Koelling, N., McGowan, S. J., et al. (2020). SMAD6 variants in craniosynostosis: genotype and phenotype evaluation. Genet. Med. 22 (9), 1498–1506. doi:10.1038/s41436-020-0817-2
Cannaerts, E., Kempers, M., Maugeri, A., Marcelis, C., Gardeitchik, T., Richer, J., et al. (2019). Novel pathogenic SMAD2 variants in five families with arterial aneurysm and dissection: further delineation of the phenotype. J. Med. Genet. 56 (4), 220–227. doi:10.1136/jmedgenet-2018-105304
Capkova, P., Santava, A., Markova, I., Stefekova, A., Srovnal, J., Staffova, K., et al. (2017). Haploinsufficiency of BMP4 and OTX2 in the Foetus with an abnormal facial profile detected in the first trimester of pregnancy. Mol. Cytogenet. 10 (1), 47. doi:10.1186/s13039-017-0351-3
Caputo, V., Cianetti, L., Niceta, M., Carta, C., Ciolfi, A., Bocchinfuso, G., et al. (2012). A restricted spectrum of mutations in the SMAD4 tumor-suppressor gene underlies Myhre syndrome. Am. J. Hum. Genet. 90 (1), 161–169. doi:10.1016/j.ajhg.2011.12.011
Carney, T. J., Dutton, K. A., Greenhill, E., Delfino-Machín, M., Dufourcq, P., Blader, P., et al. (2006). A direct role for Sox10 in specification of neural crest-derived sensory neurons. Development 133 (23), 4619–4630. doi:10.1242/dev.02668
Castonguay, R., Werner, E. D., Matthews, R. G., Presman, E., Mulivor, A. W., Solban, N., et al. (2011). Soluble endoglin specifically binds bone morphogenetic proteins 9 and 10 via its orphan domain, inhibits blood vessel formation, and suppresses tumor growth. J. Biol. Chem. 286 (34), 30034–30046. doi:10.1074/jbc.M111.260133
Cate, R. L., Mattaliano, R. J., Hession, C., Tizard, R., Farber, N. M., Cheung, A., et al. (1986). Isolation of the bovine and human genes for Müllerian inhibiting substance and expression of the human gene in animal cells. Cell 45 (5), 685–698. doi:10.1016/0092-8674(86)90783-x
Charité, J., McFadden, D. G., Merlo, G., Levi, G., Clouthier, D. E., Yanagisawa, M., et al. (2001). Role of Dlx6 in regulation of an endothelin-1-dependent, dHAND branchial arch enhancer. Genes & Dev. 15 (22), 3039–3049. doi:10.1101/gad.931701
Cheah, F. S. H., Winkler, C., Jabs, E. W., and Chong, S. S. (2010). Tgfbeta3 regulation of chondrogenesis and osteogenesis in zebrafish is mediated through formation and survival of a subpopulation of the cranial neural crest. Mech. Dev. 127 (7), 329–344. doi:10.1016/j.mod.2010.04.003
Cheifetz, S., Bellón, T., Calés, C., Vera, S., Bernabeu, C., Massagué, J., et al. (1992). Endoglin is a component of the transforming growth factor-beta receptor system in human endothelial cells. J. Biol. Chem. 267 (27), 19027–19030. doi:10.1016/s0021-9258(18)41732-2
Chen, Y., Wang, Z., and Zhang, Y. (2019). Conditional deletion of Bmp2 in cranial neural crest cells recapitulates Pierre Robin sequence in mice. Cell Tissue Res. 376, 199–210. doi:10.1007/s00441-018-2944-5
Cheng, S. K., Olale, F., Bennett, J. T., Brivanlou, A. H., and Schier, A. F. (2003). EGF-CFC proteins are essential coreceptors for the TGF-beta signals Vg1 and GDF1. Genes & Dev. 17 (1), 31–36. doi:10.1101/gad.1041203
Clarke, R. A., Davis, P. J., and Tonkin, J. (1994). Klippel-feil syndrome associated with malformed larynx: case report. Ann. Otology, Rhinology Laryngology 103 (3), 201–207. doi:10.1177/000348949410300306
Clay, M. R., and Halloran, M. C. (2013). Rho activation is apically restricted by Arhgap1 in neural crest cells and drives epithelial-to-mesenchymal transition. Development 140 (15), 3198–3209. doi:10.1242/dev.095448
Clay, M. R., and Halloran, M. C. (2014). Cadherin 6 promotes neural crest cell detachment via F-actin regulation and influences active Rho distribution during epithelial-to-mesenchymal transition. Development 141 (12), 2506–2515. doi:10.1242/dev.105551
Clément, A., Wiweger, M., von der Hardt, S., Rusch, M. A., Selleck, S. B., Chien, C. B., et al. (2008). Regulation of Zebrafish Skeletogenesis by ext2/dackel and papst1/pinscher. PLOS Genet. 4 (7), e1000136. doi:10.1371/journal.pgen.1000136
Clouthier, D. E., Hosoda, K., Richardson, J. A., Williams, S. C., Yanagisawa, H., Kuwaki, T., et al. (1998). Cranial and cardiac neural crest defects in endothelin-A receptor-deficient mice. Development 125 (5), 813–824. doi:10.1242/dev.125.5.813
Clouthier, D. E., Williams, S. C., Hammer, R. E., Richardson, J. A., and Yanagisawa, M. (2003). Cell-autonomous and nonautonomous actions of endothelin-A receptor signaling in craniofacial and cardiovascular development. Dev. Biol. 261 (2), 506–519. doi:10.1016/s0012-1606(03)00128-3
Clouthier, D. E., Williams, S. C., Yanagisawa, H., Wieduwilt, M., Richardson, J. A., and Yanagisawa, M. (2000). Signaling pathways crucial for craniofacial development revealed by endothelin-A receptor-deficient mice. Dev. Biol. 217 (1), 10–24. doi:10.1006/dbio.1999.9527
Cubbage, C. C., and Mabee, P. M. (1996). Development of the cranium and paired fins in the zebrafish Danio rerio (Ostariophysi, Cyprinidae). J. Morphol. 229 (2), 121–160. doi:10.1002/(SICI)1097-4687(199608)229:2<121::AID-JMOR1>3.0.CO;2-4
Das, A., and Crump, J. G. (2012). Bmps and id2a act upstream of Twist1 to restrict ectomesenchyme potential of the cranial neural crest. PLoS Genet. 8 (5), e1002710. doi:10.1371/journal.pgen.1002710
David, N. B., Saint-Etienne, L., Tsang, M., Schilling, T. F., and Rosa, F. M. (2002). Requirement for endoderm and FGF3 in ventral head skeleton formation. Development 129 (19), 4457–4468. doi:10.1242/dev.129.19.4457
Dawson, K., Seeman, P., Sebald, E., King, L., Edwards, M., Williams, J., et al. (2006). GDF5 is a second locus for multiple-synostosis syndrome. Am. J. Hum. Genet. 78 (4), 708–712. doi:10.1086/503204
De Larco, J. E., and Todaro, G. J. (1978). Growth factors from murine sarcoma virus-transformed cells. Proc. Natl. Acad. Sci. 75 (8), 4001–4005. doi:10.1073/pnas.75.8.4001
de Martin, R., Haendler, B., Hofer-Warbinek, R., Gaugitsch, H., Wrann, M., Schlüsener, H., et al. (1987). Complementary DNA for human glioblastoma-derived T cell suppressor factor, a novel member of the transforming growth factor-beta gene family. EMBO J. 6 (12), 3673–3677. doi:10.1002/j.1460-2075.1987.tb02700.x
Denissova, N. G., Pouponnot, C., Long, J., He, D., and Liu, F. (2000). Transforming growth factor beta -inducible independent binding of SMAD to the Smad7 promoter. Proc. Natl. Acad. Sci. 97 (12), 6397–6402. doi:10.1073/pnas.090099297
Depew, M. J., Lufkin, T., and Rubenstein, J. L. R. (2002). Specification of jaw subdivisions by Dlx genes. Science 298 (5592), 381–385. doi:10.1126/science.1075703
Derynck, R., Gelbart, W. M., Harland, R. M., Heldin, C. H., Kern, S. E., Massagué, J., et al. (1996). Nomenclature: vertebrate mediators of TGFβ family signals’, Cell. Elsevier 87 (2), 173. doi:10.1016/s0092-8674(00)81335-5
Derynck, R., Jarrett, J. A., Chen, E. Y., Eaton, D. H., Bell, J. R., Assoian, R. K., et al. (1985). Human transforming growth factor-beta complementary DNA sequence and expression in normal and transformed cells. Nature 316 (6030), 701–705. doi:10.1038/316701a0
Derynck, R., Lindquist, P. B., Lee, A., Wen, D., Tamm, J., Graycar, J. L., et al. (1988). A new type of transforming growth factor-beta, TGF-beta 3. EMBO J. 7 (12), 3737–3743. doi:10.1002/j.1460-2075.1988.tb03257.x
Dickmeis, T., Rastegar, S., Lam, C. S., Aanstad, P., Clark, M., Fischer, N., et al. (2002). Expression of the helix-loop-helix gene id3 in the zebrafish embryo. Mech. Dev. 113 (1), 99–102. doi:10.1016/s0925-4773(02)00006-0
Dodd, A. W., Syddall, C. M., and Loughlin, J. (2013). A rare variant in the osteoarthritis-associated locus GDF5 is functional and reveals a site that can be manipulated to modulate GDF5 expression. Eur. J. Hum. Genet. 21 (5), 517–521. doi:10.1038/ejhg.2012.197
Dougherty, M., Kamel, G., Grimaldi, M., Gfrerer, L., Shubinets, V., Ethier, R., et al. (2013). Distinct requirements for wnt9a and irf6 in extension and integration mechanisms during zebrafish palate morphogenesis. Development 140 (1), 76–81. doi:10.1242/dev.080473
Dranow, D. B., Le Pabic, P., and Schilling, T. F. (2023). The non-canonical Wnt receptor Ror2 is required for cartilage cell polarity and morphogenesis of the craniofacial skeleton in zebrafish. Development 150 (8), dev201273. doi:10.1242/dev.201273
Drera, B., Tadini, G., Barlati, S., and Colombi, M. (2008). Identification of a novel TGFBR1 mutation in a Loeys–Dietz syndrome type II patient with vascular Ehlers–Danlos syndrome phenotype. Clin. Genet. 73 (3), 290–293. doi:10.1111/j.1399-0004.2007.00942.x
Dudas, M., Sridurongrit, S., Nagy, A., Okazaki, K., and Kaartinen, V. (2004). Craniofacial defects in mice lacking BMP type I receptor Alk2 in neural crest cells’, Mechanisms of Development. Elsevier 121 (2), 173–182. doi:10.1016/j.mod.2003.12.003
Dutton, K. A., Pauliny, A., Lopes, S. S., Elworthy, S., Carney, T. J., Rauch, J., et al. (2001). Zebrafish colourless encodes sox10 and specifies non-ectomesenchymal neural crest fates. Development 128 (21), 4113–4125. doi:10.1242/dev.128.21.4113
Eames, B. F., DeLaurier, A., Ullmann, B., Huycke, T. R., Nichols, J. T., Dowd, J., et al. (2013). FishFace: interactive atlas of zebrafish craniofacial development at cellular resolution. BMC Dev. Biol. 13 (1), 23. doi:10.1186/1471-213X-13-23
Eberhart, J. K., Swartz, M. E., Crump, J. G., and Kimmel, C. B. (2006). Early Hedgehog signaling from neural to oral epithelium organizes anterior craniofacial development. Development 133 (6), 1069–1077. doi:10.1242/dev.02281
Ebisawa, T., Fukuchi, M., Murakami, G., Chiba, T., Tanaka, K., Imamura, T., et al. (2001). Smurf1 interacts with transforming growth factor-beta type I receptor through Smad7 and induces receptor degradation. J. Biol. Chem. 276 (16), 12477–12480. doi:10.1074/jbc.C100008200
Fabian, P., Tseng, K. C., Thiruppathy, M., Arata, C., Chen, H. J., Smeeton, J., et al. (2022). Lifelong single-cell profiling of cranial neural crest diversification in zebrafish. Nat. Commun. 13 (1), 13. doi:10.1038/s41467-021-27594-w
Farmer, D. T., Mlcochova, H., Zhou, Y., Koelling, N., Wang, G., Ashley, N., et al. (2021). The developing mouse coronal suture at single-cell resolution. Nat. Commun. 12 (1), 4797. doi:10.1038/s41467-021-24917-9
Felson, D. T., Lawrence, R. C., Dieppe, P. A., Hirsch, R., Helmick, C. G., Jordan, J. M., et al. (2000). Osteoarthritis: new insights. Part 1: the disease and its risk factors. Ann. Intern. Med. 133 (8), 635–646. doi:10.7326/0003-4819-133-8-200010170-00016
Florisson, J. M. G., Verkerk, A. J. M. H., Huigh, D., Hoogeboom, A. J. M., Swagemakers, S., Kremer, A., et al. (2013). Boston type craniosynostosis: report of a second mutation in MSX2. Am. J. Med. Genet. Part A 161 (10), 2626–2633. doi:10.1002/ajmg.a.36126
Franke, M., Mancino, C., and Taraballi, F. (2023). Reasons for the sex bias in osteoarthritis research: a review of preclinical studies. Int. J. Mol. Sci. 24 (12), 10386. doi:10.3390/ijms241210386
Fuentealba, L. C., Eivers, E., Ikeda, A., Hurtado, C., Kuroda, H., Pera, E. M., et al. (2007). Integrating patterning signals: wnt/GSK3 regulates the duration of the BMP/Smad1 signal. Cell 131 (5), 980–993. doi:10.1016/j.cell.2007.09.027
Fürthauer, M., Thisse, B., and Thisse, C. (1999). Three different noggin genes antagonize the activity of bone morphogenetic proteins in the zebrafish embryo. Dev. Biol. 214 (1), 181–196. doi:10.1006/dbio.1999.9401
Gago-Díaz, M., Blanco-Verea, A., Teixidó-Turà, G., Valenzuela, I., Del Campo, M., Borregan, M., et al. (2014). Whole exome sequencing for the identification of a new mutation in TGFB2 involved in a familial case of non-syndromic aortic disease. Clin. Chim. Acta 437, 88–92. doi:10.1016/j.cca.2014.07.016
Garcia de Vinuesa, A., Sanchez-Duffhues, G., Blaney-Davidson, E., van Caam, A., Lodder, K., Ramos, Y., et al. (2021). Cripto favors chondrocyte hypertrophy via TGF-β SMAD1/5 signaling during development of osteoarthritis. J. Pathology 255 (3), 330–342. doi:10.1002/path.5774
Garnett, A. T., Square, T. A., and Medeiros, D. M. (2012). BMP, Wnt and FGF signals are integrated through evolutionarily conserved enhancers to achieve robust expression of Pax3 and Zic genes at the zebrafish neural plate border. Development 139 (22), 4220–4231. doi:10.1242/dev.081497
Gentry, L. E., Lioubin, M. N., Purchio, A. F., and Marquardt, H. (1988). Molecular events in the processing of recombinant type 1 pre-pro-transforming growth factor beta to the mature polypeptide. Mol. Cell. Biol. 8 (10), 4162–4168. doi:10.1128/mcb.8.10.4162-4168.1988
Gentry, L. E., and Nash, B. W. (1990). The pro domain of pre-pro-transforming growth factor beta 1 when independently expressed is a functional binding protein for the mature growth factor. Biochemistry 29 (29), 6851–6857. doi:10.1021/bi00481a014
Georgi, L. L., Albert, P. S., and Riddle, D. L. (1990). daf-1, a C. elegans gene controlling dauer larva development, encodes a novel receptor protein kinase. Cell 61 (4), 635–645. doi:10.1016/0092-8674(90)90475-t
Germanguz, I., Lev, D., Waisman, T., Kim, C. H., and Gitelman, I. (2007). Four twist genes in zebrafish, four expression patterns. Dev. Dyn. 236 (9), 2615–2626. doi:10.1002/dvdy.21267
Gestri, G., Osborne, R. J., Wyatt, A. W., Gerrelli, D., Gribble, S., Stewart, H., et al. (2009). Reduced TFAP2A function causes variable optic fissure closure and retinal defects and sensitizes eye development to mutations in other morphogenetic regulators. Hum. Genet. 126 (6), 791–803. doi:10.1007/s00439-009-0730-x
Glasauer, S. M. K., and Neuhauss, S. C. F. (2014). Whole-genome duplication in teleost fishes and its evolutionary consequences. Mol. Genet. Genomics 289 (6), 1045–1060. doi:10.1007/s00438-014-0889-2
Gong, Y., Krakow, D., Marcelino, J., Wilkin, D., Chitayat, D., Babul-Hirji, R., et al. (1999). Heterozygous mutations in the gene encoding noggin affect human joint morphogenesis. Nat. Genet. 21 (3), 302–304. doi:10.1038/6821
Gorlin, R. J., Cohen, M. M., and Hennekam, R. C. M. (2001). Syndromes of the head and neck. Oxford, England: Oxford University Press.
Goto, K., Kamiya, Y., Imamura, T., Miyazono, K., and Miyazawa, K. (2007). Selective inhibitory effects of Smad6 on bone morphogenetic protein type I receptors. J. Biol. Chem. 282 (28), 20603–20611. doi:10.1074/jbc.M702100200
Graff, J. M., Bansal, A., and Melton, D. A. (1996). Xenopus Mad proteins transduce distinct subsets of signals for the TGF beta superfamily. Cell 85 (4), 479–487. doi:10.1016/s0092-8674(00)81249-0
Graham, A. (2003). Development of the pharyngeal arches. Am. J. Med. Genet. Part A 119A (3), 251–256. doi:10.1002/ajmg.a.10980
Gramann, A. K., Venkatesan, A. M., Guerin, M., and Ceol, C. J. (2019). Regulation of zebrafish melanocyte development by ligand-dependent BMP signaling. eLife 8, e50047. doi:10.7554/eLife.50047
Granadillo, J. L., Chung, W. K., Hecht, L., Corsten-Janssen, N., Wegner, D., Nij Bijvank, S. W. A., et al. (2018). Variable cardiovascular phenotypes associated with SMAD2 pathogenic variants. Hum. Mutat. 39 (12), 1875–1884. doi:10.1002/humu.23627
Gray, A. M., and Mason, A. J. (1990). Requirement for activin A and transforming growth factor-beta 1 pro-regions in homodimer assembly. Science 247 (4948), 1328–1330. doi:10.1126/science.2315700
Gray, P. C., Harrison, C. A., and Vale, W. (2003). Cripto forms a complex with activin and type II activin receptors and can block activin signaling. Proc. Natl. Acad. Sci. 100 (9), 5193–5198. doi:10.1073/pnas.0531290100
Groppe, J., Greenwald, J., Wiater, E., Rodriguez-Leon, J., Economides, A. N., Kwiatkowski, W., et al. (2002). Structural basis of BMP signalling inhibition by the cystine knot protein Noggin. Nature 420 (6916), 636–642. doi:10.1038/nature01245
Gruber, J., Saleh, A., Bakhsh, W., Rubery, P. T., and Mesfin, A. (2018). The prevalence of klippel-feil syndrome: a computed tomography–based analysis of 2,917 patients. Spine Deform. 6 (4), 448–453. doi:10.1016/j.jspd.2017.12.002
Gupta, S., Hawker, G. A., Laporte, A., Croxford, R., and Coyte, P. C. (2005). The economic burden of disabling hip and knee osteoarthritis (OA) from the perspective of individuals living with this condition. Rheumatology 44 (12), 1531–1537. doi:10.1093/rheumatology/kei049
Hagihara, M., Endo, M., Hata, K., Higuchi, C., Takaoka, K., Yoshikawa, H., et al. (2011). Neogenin, a receptor for bone morphogenetic proteins. J. Biol. Chem. 286 (7), 5157–5165. doi:10.1074/jbc.M110.180919
Hammerschmidt, M., Pelegri, F., Mullins, M. C., Kane, D. A., van Eeden, F. J., Granato, M., et al. (1996). Dino and mercedes, two genes regulating dorsal development in the zebrafish embryo. Development 123 (1), 95–102. doi:10.1242/dev.123.1.95
Han, J., Ishii, M., Bringas, P., Maas, R. L., Maxson, R. E., and Chai, Y. (2007). Concerted action of Msx1 and Msx2 in regulating cranial neural crest cell differentiation during frontal bone development. Mech. Dev. 124 (9), 729–745. doi:10.1016/j.mod.2007.06.006
Hanyu, A., Ishidou, Y., Ebisawa, T., Shimanuki, T., Imamura, T., and Miyazono, K. (2001). The N domain of Smad7 is essential for specific inhibition of transforming growth factor-β signaling. J. Cell Biol. 155 (6), 1017–1028. doi:10.1083/jcb.200106023
Harrington, A. E., Morris-Triggs, S. A., Ruotolo, B. T., Robinson, C. V., Ohnuma, S. I., and Hyvönen, M. (2006). Structural basis for the inhibition of activin signalling by follistatin. EMBO J. 25 (5), 1035–1045. doi:10.1038/sj.emboj.7601000
Hata, A., Lagna, G., Massagué, J., and Hemmati-Brivanlou, A. (1998). Smad6 inhibits BMP/Smad1 signaling by specifically competing with the Smad4 tumor suppressor. Genes & Dev. 12 (2), 186–197. doi:10.1101/gad.12.2.186
Hayashi, H., Abdollah, S., Qiu, Y., Cai, J., Xu, Y. Y., Grinnell, B. W., et al. (1997). The MAD-related protein Smad7 associates with the TGFbeta receptor and functions as an antagonist of TGFbeta signaling. Cell 89 (7), 1165–1173. doi:10.1016/s0092-8674(00)80303-7
He, X. A., Berenson, A., Bernard, M., Weber, C., Cook, L., Visel, A., et al. (2023). Identification of conserved skeletal enhancers associated with craniosynostosis risk genes. Hum. Mol. Genet. 26, ddad182. doi:10.1093/hmg/ddad182
Hild, M., Dick, A., Rauch, G. J., Meier, A., Bouwmeester, T., Haffter, P., et al. (1999). The smad5 mutation somitabun blocks Bmp2b signaling during early dorsoventral patterning of the zebrafish embryo. Development 126 (10), 2149–2159. doi:10.1242/dev.126.10.2149
Hinck, A. P. (2012). Structural studies of the TGF-βs and their receptors - insights into evolution of the TGF-β superfamily. FEBS Lett. 586 (14), 1860–1870. doi:10.1016/j.febslet.2012.05.028
Hinck, A. P., Mueller, T. D., and Springer, T. A. (2016). Structural biology and evolution of the TGF-β family. Cold Spring Harb. Perspect. Biol. 8 (12), a022103. doi:10.1101/cshperspect.a022103
Hogan, B. L. (1996). Bone morphogenetic proteins: multifunctional regulators of vertebrate development. Genes & Dev. 10 (13), 1580–1594. doi:10.1101/gad.10.13.1580
Holmes, G., Gonzalez-Reiche, A. S., Lu, N., Zhou, X., Rivera, J., Kriti, D., et al. (2020). Integrated transcriptome and network analysis reveals spatiotemporal dynamics of calvarial suturogenesis. Cell Rep. 32 (1), 107871. doi:10.1016/j.celrep.2020.107871
Hoodless, P. A., Haerry, T., Abdollah, S., Stapleton, M., O'Connor, M. B., Attisano, L., et al. (1996). MADR1, a MAD-related protein that functions in BMP2 signaling pathways. Cell 85 (4), 489–500. doi:10.1016/s0092-8674(00)81250-7
Hudnall, A. M., Arthur, J. W., and Lowery, J. W. (2016). Clinical relevance and mechanisms of antagonism between the BMP and activin/TGF-β signaling pathways. J. Osteopath. Med. 116 (7), 452–461. doi:10.7556/jaoa.2016.089
Humphreys, P. E. A., Woods, S., Bates, N., Rooney, K. M., Mancini, F. E., Barclay, C., et al. (2023). Optogenetic manipulation of BMP signaling to drive chondrogenic differentiation of hPSCs. Cell Rep. 42 (12), 113502. doi:10.1016/j.celrep.2023.113502
Hunter, D. J., Schofield, D., and Callander, E. (2014). The individual and socioeconomic impact of osteoarthritis. Nat. Rev. Rheumatol. 10 (7), 437–441. doi:10.1038/nrrheum.2014.44
Imamura, T., Takase, M., Nishihara, A., Oeda, E., Hanai, J., Kawabata, M., et al. (1997). Smad6 inhibits signalling by the TGF-beta superfamily. Nature 389 (6651), 622–626. doi:10.1038/39355
Ishida, W., Hamamoto, T., Kusanagi, K., Yagi, K., Kawabata, M., Takehara, K., et al. (2000). Smad6 is a smad1/5-induced smad inhibitor: characterization of bone morphogenetic protein-responsive element in the mouse Smad6 promoter. J. Biol. Chem. 275 (9), 6075–6079. doi:10.1074/jbc.275.9.6075
Ishii, M., Merrill, A. E., Chan, Y. S., Gitelman, I., Rice, D. P. C., Sucov, H. M., et al. (2003). Msx2 and Twist cooperatively control the development of the neural crest-derived skeletogenic mesenchyme of the murine skull vault. Development 130 (24), 6131–6142. doi:10.1242/dev.00793
Jabs, E. W., Müller, U., Li, X., Luo, W., Haworth, I. S., Klisak, I., et al. (1993). A mutation in the homeodomain of the human MSX2 gene in a family affected with autosomal dominant craniosynostosis. Cell 75 (3), 443–450. doi:10.1016/0092-8674(93)90379-5
Jezewski, P. A., Vieira, A. R., Nishimura, C., Ludwig, B., Johnson, M., O'Brien, S. E., et al. (2003). Complete sequencing shows a role for MSX1 in non-syndromic cleft lip and palate. J. Med. Genet. 40 (6), 399–407. doi:10.1136/jmg.40.6.399
Jiang, X., Iseki, S., Maxson, R. E., Sucov, H. M., and Morriss-Kay, G. M. (2002). Tissue origins and interactions in the mammalian skull vault. Dev. Biol. 241 (1), 106–116. doi:10.1006/dbio.2001.0487
Jimenez, L., Wang, J., Morrison, M. A., Whatcott, C., Soh, K. K., Warner, S., et al. (2016). Phenotypic chemical screening using a zebrafish neural crest EMT reporter identifies retinoic acid as an inhibitor of epithelial morphogenesis. Dis. Models Mech. 9 (4), 389–400. doi:10.1242/dmm.021790
Johnson, D., and Wilkie, A. O. M. (2011). Craniosynostosis. Eur. J. Hum. Genet. 19 (4), 369–376. doi:10.1038/ejhg.2010.235
Justice, C. M., Cuellar, A., Bala, K., Sabourin, J. A., Cunningham, M. L., Crawford, K., et al. (2020). A genome-wide association study implicates the BMP7 locus as a risk factor for nonsyndromic metopic craniosynostosis. Hum. Genet. 139 (8), 1077–1090. doi:10.1007/s00439-020-02157-z
Justice, C. M., Yagnik, G., Kim, Y., Peter, I., Jabs, E. W., Erazo, M., et al. (2012). A genome-wide association study identifies susceptibility loci for nonsyndromic sagittal craniosynostosis near BMP2 and within BBS9. Nat. Genet. 44 (12), 1360–1364. doi:10.1038/ng.2463
Kaartinen, V., Voncken, J. W., Shuler, C., Warburton, D., Bu, D., Heisterkamp, N., et al. (1995). Abnormal lung development and cleft palate in mice lacking TGF-beta 3 indicates defects of epithelial-mesenchymal interaction. Nat. Genet. 11 (4), 415–421. doi:10.1038/ng1295-415
Kague, E., Gallagher, M., Burke, S., Parsons, M., Franz-Odendaal, T., and Fisher, S. (2012). Skeletogenic fate of zebrafish cranial and trunk neural crest. PLOS ONE 7 (11), e47394. doi:10.1371/journal.pone.0047394
Kamel, G., Hoyos, T., Rochard, L., Dougherty, M., Kong, Y., Tse, W., et al. (2013). Requirement for frzb and fzd7a in cranial neural crest convergence and extension mechanisms during zebrafish palate and jaw morphogenesis. Dev. Biol. 381 (2), 423–433. doi:10.1016/j.ydbio.2013.06.012
Kanther, M., Scalici, A., Rashid, A., Miao, K., Van Deventer, E., and Fisher, S. (2019). Initiation and early growth of the skull vault in zebrafish. Mech. Dev. 160, 103578. doi:10.1016/j.mod.2019.103578
Kanzaki, T., Olofsson, A., Morén, A., Wernstedt, C., Hellman, U., Miyazono, K., et al. (1990). TGF-beta 1 binding protein: a component of the large latent complex of TGF-beta 1 with multiple repeat sequences. Cell 61 (6), 1051–1061. doi:10.1016/0092-8674(90)90069-q
Karaulanov, E., Knöchel, W., and Niehrs, C. (2004). Transcriptional regulation of BMP4 synexpression in transgenic Xenopus. EMBO J. 23 (4), 844–856. doi:10.1038/sj.emboj.7600101
Katagiri, T., Imada, M., Yanai, T., Suda, T., Takahashi, N., and Kamijo, R. (2002). Identification of a BMP-responsive element in Id1, the gene for inhibition of myogenesis. Genes Cells 7 (9), 949–960. doi:10.1046/j.1365-2443.2002.00573.x
Kavsak, P., Rasmussen, R. K., Causing, C. G., Bonni, S., Zhu, H., Thomsen, G. H., et al. (2000). Smad7 binds to Smurf2 to form an E3 ubiquitin ligase that targets the TGF beta receptor for degradation. Mol. Cell 6 (6), 1365–1375. doi:10.1016/s1097-2765(00)00134-9
Kawabata, M., Chytil, A., and Moses, H. L. (1995). Cloning of a novel type II serine/threonine kinase receptor through interaction with the type I transforming growth factor-beta receptor. J. Biol. Chem. 270 (10), 5625–5630. doi:10.1074/jbc.270.10.5625
Kawabata, M., Inoue, H., Hanyu, A., Imamura, T., and Miyazono, K. (1998). Smad proteins exist as monomers in vivo and undergo homo- and hetero-oligomerization upon activation by serine/threonine kinase receptors. EMBO J. 17 (14), 4056–4065. doi:10.1093/emboj/17.14.4056
Kelsh, R. N., and Eisen, J. S. (2000). The zebrafish colourless gene regulates development of non-ectomesenchymal neural crest derivatives. Development 127 (3), 515–525. doi:10.1242/dev.127.3.515
Kiapour, A. M., Cao, J., Young, M., and Capellini, T. D. (2018). The role of Gdf5 regulatory regions in development of hip morphology. PLOS ONE 13 (11), e0202785. doi:10.1371/journal.pone.0202785
Kimmel, C. B., Miller, C. T., Kruze, G., Ullmann, B., BreMiller, R. A., Larison, K. D., et al. (1998). The shaping of pharyngeal cartilages during early development of the zebrafish. Dev. Biol. 203 (2), 245–263. doi:10.1006/dbio.1998.9016
Kimmel, C. B., Walker, M. B., and Miller, C. T. (2007). Morphing the hyomandibular skeleton in development and evolution. J. Exp. Zoology Part B Mol. Dev. Evol. 308 (5), 609–624. doi:10.1002/jez.b.21155
Kishimoto, Y., Lee, K. H., Zon, L., Hammerschmidt, M., and Schulte-Merker, S. (1997). The molecular nature of zebrafish swirl: BMP2 function is essential during early dorsoventral patterning. Development 124 (22), 4457–4466. doi:10.1242/dev.124.22.4457
Klippel, M. (1912). ‘Un cas d’absence des vertebres cervicales avec cage thoracique remontent justu’a la base du crane’. Novelle Inconogr. las Salpetriere 25, 223–250.
Knight, R. D., Javidan, Y., Nelson, S., Zhang, T., and Schilling, T. (2004). Skeletal and pigment cell defects in the lockjaw mutant reveal multiple roles for zebrafish tfap2a in neural crest development. Dev. Dyn. 229 (1), 87–98. doi:10.1002/dvdy.10494
Knight, R. D., Nair, S., Nelson, S. S., Afshar, A., Javidan, Y., Geisler, R., et al. (2003). Lockjaw encodes a zebrafish tfap2a required for early neural crest development. Development 130 (23), 5755–5768. doi:10.1242/dev.00575
Komatsu, Y., Yu, P. B., Kamiya, N., Pan, H., Fukuda, T., Scott, G. J., et al. (2013). Augmentation of smad-dependent BMP signaling in neural crest cells causes craniosynostosis in mice. J. Bone Mineral Res. 28 (6), 1422–1433. doi:10.1002/jbmr.1857
Kouskoura, T., Kozlova, A., Alexiou, M., Blumer, S., Zouvelou, V., Katsaros, C., et al. (2013). The etiology of cleft palate formation in BMP7-deficient mice. PLoS ONE 8 (3), e59463. doi:10.1371/journal.pone.0059463
Koyama, E., Saunders, C., Salhab, I., Decker, R. S., Chen, I., Um, H., et al. (2014). Lubricin is required for the structural integrity and post-natal maintenance of TMJ. J. Dent. Res. 93 (7), 663–670. doi:10.1177/0022034514535807
Koyama, E., Shibukawa, Y., Nagayama, M., Sugito, H., Young, B., Yuasa, T., et al. (2008). A distinct cohort of progenitor cells participates in synovial joint and articular cartilage formation during mouse limb skeletogenesis. Dev. Biol. 316 (1), 62–73. doi:10.1016/j.ydbio.2008.01.012
Kretzschmar, M., Liu, F., Hata, A., Doody, J., and Massagué, J. (1997). The TGF-beta family mediator Smad1 is phosphorylated directly and activated functionally by the BMP receptor kinase. Genes & Dev. 11 (8), 984–995. doi:10.1101/gad.11.8.984
Lagna, G., Hata, A., Hemmati-Brivanlou, A., and Massagué, J. (1996). Partnership between DPC4 and SMAD proteins in TGF-beta signalling pathways. Nature 383 (6603), 832–836. doi:10.1038/383832a0
Lajeunie, E., Le Merrer, M., Bonaïti-Pellie, C., Marchac, D., and Renier, D. (1995). Genetic study of nonsyndromic coronal craniosynostosis. Am. J. Med. Genet. 55 (4), 500–504. doi:10.1002/ajmg.1320550422
Lastres, P., Letamendía, A., Zhang, H., Rius, C., Almendro, N., Raab, U., et al. (1996). Endoglin modulates cellular responses to TGF-beta 1. J. Cell Biol. 133 (5), 1109–1121. doi:10.1083/jcb.133.5.1109
Laue, K., Pogoda, H. M., Daniel, P. B., van Haeringen, A., Alanay, Y., von Ameln, S., et al. (2011). Craniosynostosis and multiple skeletal anomalies in humans and zebrafish result from a defect in the localized degradation of retinoic acid. Am. J. Hum. Genet. 89 (5), 595–606. doi:10.1016/j.ajhg.2011.09.015
Lawrence, D. A., Pircher, R., and Jullien, P. (1985). Conversion of a high molecular weight latent beta-TGF from chicken embryo fibroblasts into a low molecular weight active beta-TGF under acidic conditions. Biochem. Biophysical Res. Commun. 133 (3), 1026–1034. doi:10.1016/0006-291x(85)91239-2
Le Pabic, P., Ng, C., and Schilling, T. F. (2014). Fat-dachsous signaling coordinates cartilage differentiation and polarity during craniofacial development. PLOS Genet. 10 (10), e1004726. doi:10.1371/journal.pgen.1004726
Li, C. G., Wilson, P. B., Bernabeu, C., Raab, U., Wang, J. M., and Kumar, S. (1998). Immunodetection and characterisation of soluble CD105-TGFbeta complexes. J. Immunol. Methods 218 (1), 85–93. doi:10.1016/s0022-1759(98)00118-5
Li, H., Jones, K. L., Hooper, J. E., and Williams, T. (2019). The molecular anatomy of mammalian upper lip and primary palate fusion at single cell resolution. Development 146 (12), dev174888. doi:10.1242/dev.174888
Li, L., Ning, G., Yang, S., Yan, Y., Cao, Y., and Wang, Q. (2019). BMP signaling is required for nkx2.3-positive pharyngeal pouch progenitor specification in zebrafish. PLOS Genet. 15 (2), e1007996. doi:10.1371/journal.pgen.1007996
Li, M., Zhao, C., Wang, Y., Zhao, Z., and Meng, A. (2002). Zebrafish sox9b is an early neural crest marker. Dev. Genes Evol. 212, 203–206. doi:10.1007/s00427-002-0235-2
Lidral, A. C., Romitti, P. A., Basart, A. M., Doetschman, T., Leysens, N. J., Daack-Hirsch, S., et al. (1998). Association of MSX1 and TGFB3 with nonsyndromic clefting in humans. Am. J. Hum. Genet. 63 (2), 557–568. doi:10.1086/301956
Lin, H. Y., Wang, X. F., Ng-Eaton, E., Weinberg, R. A., and Lodish, H. F. (1992). Expression cloning of the TGF-beta type II receptor, a functional transmembrane serine/threonine kinase. Cell 68 (4), 775–785. doi:10.1016/0092-8674(92)90152-3
Lin, X., Liang, M., and Feng, X.-H. (2000). Smurf2 is a ubiquitin E3 ligase mediating proteasome-dependent degradation of Smad2 in transforming growth factor-beta signaling. J. Biol. Chem. 275 (47), 36818–36822. doi:10.1074/jbc.C000580200
Ling, I. T. C., Rochard, L., and Liao, E. C. (2017). Distinct requirements of wls, wnt9a, wnt5b and gpc4 in regulating chondrocyte maturation and timing of endochondral ossification. Dev. Biol. 421 (2), 219–232. doi:10.1016/j.ydbio.2016.11.016
Lister, J. A., Cooper, C., Nguyen, K., Modrell, M., Grant, K., and Raible, D. W. (2006). Zebrafish Foxd3 is required for development of a subset of neural crest derivatives. Dev. Biol. 290 (1), 92–104. doi:10.1016/j.ydbio.2005.11.014
Liu, F., Hata, A., Baker, J. C., Doody, J., Cárcamo, J., Harland, R. M., et al. (1996). A human Mad protein acting as a BMP-regulated transcriptional activator. Nature 381 (6583), 620–623. doi:10.1038/381620a0
Liu, W., Selever, J., Murali, D., Sun, X., Brugger, S. M., Ma, L., et al. (2005). Threshold-specific requirements for Bmp4 in mandibular development. Dev. Biol. 283 (2), 282–293. doi:10.1016/j.ydbio.2005.04.019
Liu, W., Selever, J., Wang, D., Lu, M. F., Moses, K. A., Schwartz, R. J., et al. (2004). Bmp4 signaling is required for outflow-tract septation and branchial-arch artery remodeling. Proc. Natl. Acad. Sci. 101 (13), 4489–4494. doi:10.1073/pnas.0308466101
Liu, X., Hayano, S., Pan, H., Inagaki, M., Ninomiya-Tsuji, J., Sun, H., et al. (2018). Compound mutations in Bmpr1a and Tak1 synergize facial deformities via increased cell death. genesis 56 (3), e23093. doi:10.1002/dvg.23093
Liu, Y. H., Kundu, R., Wu, L., Luo, W., Ignelzi, M. A., Snead, M. L., et al. (1995). Premature suture closure and ectopic cranial bone in mice expressing Msx2 transgenes in the developing skull. Proc. Natl. Acad. Sci. 92 (13), 6137–6141. doi:10.1073/pnas.92.13.6137
Loeys, B. L., Chen, J., Neptune, E. R., Judge, D. P., Podowski, M., Holm, T., et al. (2005). A syndrome of altered cardiovascular, craniofacial, neurocognitive and skeletal development caused by mutations in TGFBR1 or TGFBR2. Nat. Genet. 37 (3), 275–281. doi:10.1038/ng1511
Loeys, B. L., Schwarze, U., Holm, T., Callewaert, B. L., Thomas, G. H., Pannu, H., et al. (2006). Aneurysm syndromes caused by mutations in the TGF-beta receptor. N. Engl. J. Med. 355 (8), 788–798. doi:10.1056/NEJMoa055695
López-Casillas, F., Payne, H. M., Andres, J. L., and Massagué, J. (1994). Betaglycan can act as a dual modulator of TGF-beta access to signaling receptors: mapping of ligand binding and GAG attachment sites. J. Cell Biol. 124 (4), 557–568. doi:10.1083/jcb.124.4.557
López-Casillas, F., Wrana, J. L., and Massagué, J. (1993). Betaglycan presents ligand to the TGF beta signaling receptor. Cell 73 (7), 1435–1444. doi:10.1016/0092-8674(93)90368-z
Lovely, C. B., Swartz, M. E., McCarthy, N., Norrie, J. L., and Eberhart, J. K. (2016). Bmp signaling mediates endoderm pouch morphogenesis by regulating Fgf signaling in zebrafish. Development 143 (11), 2000–2011. doi:10.1242/dev.129379
Lumaka, A., Van Hole, C., Casteels, I., Ortibus, E., De Wolf, V., Vermeesch, J. R., et al. (2012). Variability in expression of a familial 2.79 Mb microdeletion in chromosome14q22.1–22.2. Am. J. Med. Genet. Part A 158A (6), 1381–1387. doi:10.1002/ajmg.a.35353
Luo, K. (2017). Signaling cross talk between TGF-β/Smad and other signaling pathways. Cold Spring Harb. Perspect. Biol. 9 (1), a022137. doi:10.1101/cshperspect.a022137
Lyons, K. M., and Rosen, V. (2019). BMPs, TGFβ, and border security at the interzone. Curr. Top. Dev. Biol. 133, 153–170. doi:10.1016/bs.ctdb.2019.02.001
Lyons, R. M., Gentry, L. E., Purchio, A. F., and Moses, H. L. (1990). Mechanism of activation of latent recombinant transforming growth factor beta 1 by plasmin. J. Cell Biol. 110 (4), 1361–1367. doi:10.1083/jcb.110.4.1361
Macias, M. J., Martin-Malpartida, P., and Massagué, J. (2015). Structural determinants of Smad function in TGF-β signaling. Trends Biochem. Sci. 40 (6), 296–308. doi:10.1016/j.tibs.2015.03.012
Macías-Silva, M., Abdollah, S., Hoodless, P. A., Pirone, R., Attisano, L., and Wrana, J. L. (1996). MADR2 is a substrate of the TGFbeta receptor and its phosphorylation is required for nuclear accumulation and signaling. Cell 87 (7), 1215–1224. doi:10.1016/s0092-8674(00)81817-6
Mangold, E., Ludwig, K. U., Birnbaum, S., Baluardo, C., Ferrian, M., Herms, S., et al. (2010). Genome-wide association study identifies two susceptibility loci for nonsyndromic cleft lip with or without cleft palate. Nat. Genet. 42 (1), 24–26. doi:10.1038/ng.506
Marquardt, H., Lioubin, M. N., and Ikeda, T. (1987). Complete amino acid sequence of human transforming growth factor type beta 2. J. Biol. Chem. 262 (25), 12127–12131. doi:10.1016/s0021-9258(18)45325-2
Martin, S. V., and Swan, M. C. (2023). An essential overview of orofacial clefting. Br. Dent. J. 234 (12), 937–942. doi:10.1038/s41415-023-6000-9
Mason, A. J., Hayflick, J. S., Ling, N., Esch, F., Ueno, N., Ying, S. Y., et al. (1985). Complementary DNA sequences of ovarian follicular fluid inhibin show precursor structure and homology with transforming growth factor-beta. Nature 318 (6047), 659–663. doi:10.1038/318659a0
Mathews, L. S., and Vale, W. W. (1991). Expression cloning of an activin receptor, a predicted transmembrane serine kinase. Cell 65 (6), 973–982. doi:10.1016/0092-8674(91)90549-e
Matsuoka, T., Ahlberg, P. E., Kessaris, N., Iannarelli, P., Dennehy, U., Richardson, W. D., et al. (2005). Neural crest origins of the neck and shoulder. Nature 436 (7049), 347–355. doi:10.1038/nature03837
Matyas, G., Naef, P., Tollens, M., and Oexle, K. (2014). De novo mutation of the latency-associated peptide domain of TGFB3 in a patient with overgrowth and Loeys–Dietz syndrome features. Am. J. Med. Genet. Part A 164 (8), 2141–2143. doi:10.1002/ajmg.a.36593
McDonald, N. Q., and Hendrickson, W. A. (1993). A structural superfamily of growth factors containing a cystine knot motif. Cell 73 (3), 421–424. doi:10.1016/0092-8674(93)90127-c
Medeiros, D. M., and Crump, J. G. (2012). New perspectives on pharyngeal dorsoventral patterning in development and evolution of the vertebrate jaw. Dev. Biol. 371 (2), 121–135. doi:10.1016/j.ydbio.2012.08.026
Melville, D. B., Montero-Balaguer, M., Levic, D. S., Bradley, K., Smith, J. R., Hatzopoulos, A. K., et al. (2011). The feelgood mutation in zebrafish dysregulates COPII-dependent secretion of select extracellular matrix proteins in skeletal morphogenesis. Dis. Models Mech. 4 (6), 763–776. doi:10.1242/dmm.007625
Miller, C. T., Schilling, T. F., Lee, K., Parker, J., and Kimmel, C. B. (2000). Sucker encodes a zebrafish Endothelin-1 required for ventral pharyngeal arch development. Development 127 (17), 3815–3828. doi:10.1242/dev.127.17.3815
Miller, C. T., Yelon, D., Stainier, D. Y. R., and Kimmel, C. B. (2003). Two endothelin 1 effectors, hand2 and bapx1, pattern ventral pharyngeal cartilage and the jaw joint. Development 130 (7), 1353–1365. doi:10.1242/dev.00339
Minchiotti, G., Parisi, S., Liguori, G., Signore, M., Lania, G., Adamson, E. D., et al. (2000). Membrane-anchorage of Cripto protein by glycosylphosphatidylinositol and its distribution during early mouse development. Mech. Dev. 90 (2), 133–142. doi:10.1016/s0925-4773(99)00235-x
Minoux, M., and Rijli, F. M. (2010). Molecular mechanisms of cranial neural crest cell migration and patterning in craniofacial development. Development 137 (16), 2605–2621. doi:10.1242/dev.040048
Miyamoto, Y., Mabuchi, A., Shi, D., Kubo, T., Takatori, Y., Saito, S., et al. (2007). A functional polymorphism in the 5′ UTR of GDF5 is associated with susceptibility to osteoarthritis. Nat. Genet. 39 (4), 529–533. doi:10.1038/2005
Mongera, A., Singh, A. P., Levesque, M. P., Chen, Y. Y., Konstantinidis, P., and Nüsslein-Volhard, C. (2013). Genetic lineage labeling in zebrafish uncovers novel neural crest contributions to the head, including gill pillar cells. Development 140 (4), 916–925. doi:10.1242/dev.091066
Montero-Balaguer, M., Lang, M. R., Sachdev, S. W., Knappmeyer, C., Stewart, R. A., De La Guardia, A., et al. (2006). The mother superior mutation ablates foxd3 activity in neural crest progenitor cells and depletes neural crest derivatives in zebrafish. Dev. Dyn. 235 (12), 3199–3212. doi:10.1002/dvdy.20959
Moses, H. L., Branum, E. L., Proper, J. A., and Robinson, R. A. (1981). Transforming growth factor production by chemically transformed cells. Cancer Res. 41 (7), 2842–2848.
Mullins, M. C., Hammerschmidt, M., Kane, D. A., Odenthal, J., Brand, M., van Eeden, F. J., et al. (1996). Genes establishing dorsoventral pattern formation in the zebrafish embryo: the ventral specifying genes. Development 123 (1), 81–93. doi:10.1242/dev.123.1.81
Munger, J. S., Huang, X., Kawakatsu, H., Griffiths, M. J., Dalton, S. L., Wu, J., et al. (1999). The integrin alpha v beta 6 binds and activates latent TGF beta 1: a mechanism for regulating pulmonary inflammation and fibrosis. Cell 96 (3), 319–328. doi:10.1016/s0092-8674(00)80545-0
Nagarajan, R. P., Zhang, J., Li, W., and Chen, Y. (1999). Regulation of Smad7 promoter by direct association with Smad3 and Smad4. J. Biol. Chem. 274 (47), 33412–33418. doi:10.1074/jbc.274.47.33412
Nair, S., Li, W., Cornell, R., and Schilling, T. F. (2007). Requirements for Endothelin type-A receptors and Endothelin-1 signaling in the facial ectoderm for the patterning of skeletogenic neural crest cells in zebrafish. Development 134 (2), 335–345. doi:10.1242/dev.02704
Nakao, A., Afrakhte, M., Morén, A., Nakayama, T., Christian, J. L., Heuchel, R., et al. (1997). Identification of Smad7, a TGFbeta-inducible antagonist of TGF-beta signalling. Nature 389 (6651), 631–635. doi:10.1038/39369
Narboux-Neme, N., Ekker, M., Levi, G., and Heude, E. (2019). Posterior axis formation requires Dlx5/Dlx6 expression at the neural plate border. PLOS ONE 14 (3), e0214063. doi:10.1371/journal.pone.0214063
Neave, B., Holder, N., and Patient, R. (1997). A graded response to BMP-4 spatially coordinates patterning of the mesoderm and ectoderm in the zebrafish. Mech. Dev. 62 (2), 183–195. doi:10.1016/s0925-4773(97)00659-x
Nelson, A. E., Allen, K. D., Golightly, Y. M., Goode, A. P., and Jordan, J. M. (2014). A systematic review of recommendations and guidelines for the management of osteoarthritis: the chronic osteoarthritis management initiative of the US bone and joint initiative. Seminars Arthritis Rheumatism 43 (6), 701–712. doi:10.1016/j.semarthrit.2013.11.012
Newfeld, S. J., Wisotzkey, R. G., and Kumar, S. (1999). Molecular evolution of a developmental pathway: phylogenetic analyses of transforming growth factor-beta family ligands, receptors and Smad signal transducers. Genetics 152 (2), 783–795. doi:10.1093/genetics/152.2.783
Ng, J. Q., Jafarov, T. H., Little, C. B., Wang, T., Ali, A. M., Ma, Y., et al. (2023). Loss of Grem1-lineage chondrogenic progenitor cells causes osteoarthritis. Nat. Commun. 14 (1), 6909. doi:10.1038/s41467-023-42199-1
Nguyen, V. H., Schmid, B., Trout, J., Connors, S. A., Ekker, M., and Mullins, M. C. (1998). Ventral and lateral regions of the zebrafish gastrula, including the neural crest progenitors, are established by a bmp2b/swirl pathway of genes. Dev. Biol. 199 (1), 93–110. doi:10.1006/dbio.1998.8927
Ning, G., Liu, X., Dai, M., Meng, A., and Wang, Q. (2013). MicroRNA-92a upholds bmp signaling by targeting noggin3 during pharyngeal cartilage formation. Dev. Cell 24 (3), 283–295. doi:10.1016/j.devcel.2012.12.016
Nolan, K., Kattamuri, C., Luedeke, D. M., Deng, X., Jagpal, A., Zhang, F., et al. (2013). Structure of protein related to Dan and Cerberus: insights into the mechanism of bone morphogenetic protein antagonism. Structure 21 (8), 1417–1429. doi:10.1016/j.str.2013.06.005
Onichtchouk, D., Chen, Y. G., Dosch, R., Gawantka, V., Delius, H., Massagué, J., et al. (1999). Silencing of TGF-beta signalling by the pseudoreceptor BAMBI. Nature 401 (6752), 480–485. doi:10.1038/46794
Ozeki, H., Kurihara, Y., Tonami, K., Watatani, S., and Kurihara, H. (2004). Endothelin-1 regulates the dorsoventral branchial arch patterning in mice. Mech. Dev. 121 (4), 387–395. doi:10.1016/j.mod.2004.02.002
Pacifici, M., Koyama, E., Shibukawa, Y., Wu, C., Tamamura, Y., Enomoto-Iwamoto, M., et al. (2006). Cellular and molecular mechanisms of synovial joint and articular cartilage formation. Ann. N. Y. Acad. Sci. 1068 (1), 74–86. doi:10.1196/annals.1346.010
Padgett, R. W., Johnston, R. D., and Gelbart, W. M. (1987). A transcript from a Drosophila pattern gene predicts a protein homologous to the transforming growth factor-beta family. Nature 325 (6099), 81–84. doi:10.1038/325081a0
Park, S., Zhao, H., Urata, M., and Chai, Y. (2016). Sutures possess strong regenerative capacity for calvarial bone injury. Stem Cells Dev. 25 (23), 1801–1807. doi:10.1089/scd.2016.0211
Phillips, B. T., Kwon, H. J., Melton, C., Houghtaling, P., Fritz, A., and Riley, B. B. (2006). Zebrafish msxB, msxC and msxE function together to refine the neural–nonneural border and regulate cranial placodes and neural crest development. Dev. Biol. 294 (2), 376–390. doi:10.1016/j.ydbio.2006.03.001
Piccolo, S., Agius, E., Leyns, L., Bhattacharyya, S., Grunz, H., Bouwmeester, T., et al. (1999). The head inducer Cerberus is a multifunctional antagonist of Nodal, BMP and Wnt signals. Nature 397 (6721), 707–710. doi:10.1038/17820
Piotrowski, T., and Nüsslein-Volhard, C. (2000). The endoderm plays an important role in patterning the segmented pharyngeal region in zebrafish (Danio rerio). Dev. Biol. 225 (2), 339–356. doi:10.1006/dbio.2000.9842
Piotrowski, T., Schilling, T. F., Brand, M., Jiang, Y. J., Heisenberg, C. P., Beuchle, D., et al. (1996). Jaw and branchial arch mutants in zebrafish II: anterior arches and cartilage differentiation. Development 123 (1), 345–356. doi:10.1242/dev.123.1.345
Pircher, R., Lawrence, D. A., and Jullien, P. (1984). Latent beta-transforming growth factor in nontransformed and Kirsten sarcoma virus-transformed normal rat kidney cells, clone 49F. Cancer Res. 44 (12), 5538–5543.
Potti, T. A., Petty, E. M., and Lesperance, M. M. (2011). A comprehensive review of reported heritable noggin-associated syndromes and proposed clinical utility of one broadly inclusive diagnostic term: NOG-related-symphalangism spectrum disorder (NOG-SSD). Hum. Mutat. 32 (8), 877–886. doi:10.1002/humu.21515
Pregizer, S. K., Kiapour, A. M., Young, M., Chen, H., Schoor, M., Liu, Z., et al. (2018). Impact of broad regulatory regions on Gdf5 expression and function in knee development and susceptibility to osteoarthritis. Ann. Rheumatic Dis. 77 (3), 450. doi:10.1136/annrheumdis-2017-212475
Printzlau, A., and Andersen, M. (2004). Pierre Robin sequence in Denmark: a retrospective population-based epidemiological study. Cleft Palate Craniofacial J. 41 (1), 47–52. doi:10.1597/02-055
Proetzel, G., Pawlowski, S. A., Wiles, M. V., Yin, M., Boivin, G. P., Howles, P. N., et al. (1995). Transforming growth factor-beta 3 is required for secondary palate fusion. Nat. Genet. 11 (4), 409–414. doi:10.1038/ng1295-409
Raftery, L. A., Twombly, V., Wharton, K., and Gelbart, W. M. (1995). Genetic screens to identify elements of the decapentaplegic signaling pathway in Drosophila. Genetics 139 (1), 241–254. doi:10.1093/genetics/139.1.241
Raterman, S. T., Metz, J. R., Wagener, F. A. D. T. G., and Von den Hoff, J. W. (2020). Zebrafish models of craniofacial malformations: interactions of environmental factors. Front. Cell Dev. Biol. 8, 600926. doi:10.3389/fcell.2020.600926
Reed, N. P., and Mortlock, D. P. (2010). Identification of a distant cis-regulatory element controlling pharyngeal arch-specific expression of zebrafish gdf6a/radar. Dev. Dyn. 239 (4), 1047–1060. doi:10.1002/dvdy.22251
Reichert, C. (1837). “Entwicklungsgeschichte der Gehoerknoechelchen. Der sogenannte Meckelsche Fortsatz des Hammers,” in Ueber die Visceralbogen der Wirbeltiere im Allgemeinen und deren Metamorphosen bei den Voegeln und Säugetieren’, Archiv für Anatomie und Physiologie. Editor C. Reichert, 120–222.
Rhee, D. K., Marcelino, J., Baker, M., Gong, Y., Smits, P., Lefebvre, V., et al. (2005). The secreted glycoprotein lubricin protects cartilage surfaces and inhibits synovial cell overgrowth. J. Clin. Investigation 115 (3), 622–631. doi:10.1172/JCI22263
Richard, D., Liu, Z., Cao, J., Kiapour, A. M., Willen, J., Yarlagadda, S., et al. (2020). Evolutionary selection and constraint on human knee chondrocyte regulation impacts osteoarthritis risk. Cell 181 (2), 362–381.e28. doi:10.1016/j.cell.2020.02.057
Rienhoff, H. Y., Yeo, C. Y., Morissette, R., Khrebtukova, I., Melnick, J., Luo, S., et al. (2013). A mutation in TGFB3 associated with a syndrome of low muscle mass, growth retardation, distal arthrogryposis and clinical features overlapping with marfan and loeys–dietz syndrome. Am. J. Med. Genet. Part A 161 (8), 2040–2046. doi:10.1002/ajmg.a.36056
Roberts, A. B., Anzano, M. A., Lamb, L. C., Smith, J. M., and Sporn, M. B. (1981). New class of transforming growth factors potentiated by epidermal growth factor: isolation from non-neoplastic tissues. Proc. Natl. Acad. Sci. 78 (9), 5339–5343. doi:10.1073/pnas.78.9.5339
Robin, P. (1923). A fall of the base of the tongue considered as a new cause of nasopharyngeal respiratory impairment. Bull. l'Académie Natl. Médecine 89, 37.
Rochard, L., Monica, S. D., Ling, I. T. C., Kong, Y., Roberson, S., Harland, R., et al. (2016). Roles of Wnt pathway genes wls, wnt9a, wnt5b, frzb and gpc4 in regulating convergent-extension during zebrafish palate morphogenesis. Development 143 (14), 2541–2547. doi:10.1242/dev.137000
Rogers, K. W., Saul, A. J., Rogers, C. E., Garmendia-Cedillos, M., and Pohida, T. (2023). Optogenetic signaling activation in zebrafish embryos. J. Vis. Exp. 200, e65733. doi:10.3791/65733
Rudnik-Schöneborn, S., Takahashi, T., Busse, S., Schmidt, T., Senderek, J., Eggermann, T., et al. (2010). Facioaudiosymphalangism syndrome and growth acceleration associated with a heterozygous NOG mutation. Am. J. Med. Genet. Part A 152A (6), 1540–1544. doi:10.1002/ajmg.a.33387
Sahoo, T., Theisen, A., Sanchez-Lara, P. A., Marble, M., Schweitzer, D. N., Torchia, B. S., et al. (2011). Microdeletion 20p12.3 involving BMP2 contributes to syndromic forms of cleft palate. Am. J. Med. Genet. Part A 155 (7), 1646–1653. doi:10.1002/ajmg.a.34063
Sanford, L. P., Ormsby, I., Gittenberger-de Groot, A. C., Sariola, H., Friedman, R., Boivin, G. P., et al. (1997). TGFbeta2 knockout mice have multiple developmental defects that are non-overlapping with other TGFbeta knockout phenotypes. Development 124 (13), 2659–2670. doi:10.1242/dev.124.13.2659
Sapkota, G., Alarcón, C., Spagnoli, F. M., Brivanlou, A. H., and Massagué, J. (2007). Balancing BMP signaling through integrated inputs into the Smad1 linker. Mol. Cell 25 (3), 441–454. doi:10.1016/j.molcel.2007.01.006
Sarmah, S., Barrallo-Gimeno, A., Melville, D. B., Topczewski, J., Solnica-Krezel, L., and Knapik, E. W. (2010). Sec24D-Dependent transport of extracellular matrix proteins is required for zebrafish skeletal morphogenesis. PLOS ONE 5 (4), e10367. doi:10.1371/journal.pone.0010367
Sato, T., Kurihara, Y., Asai, R., Kawamura, Y., Tonami, K., Uchijima, Y., et al. (2008). An endothelin-1 switch specifies maxillomandibular identity’, Proceedings of the National Academy of Sciences. Proc. Natl. Acad. Sci. 105 (48), 18806–18811. doi:10.1073/pnas.0807345105
Sato, Y., and Rifkin, D. B. (1989). Inhibition of endothelial cell movement by pericytes and smooth muscle cells: activation of a latent transforming growth factor-beta 1-like molecule by plasmin during co-culture. J. Cell Biol. 109 (1), 309–315. doi:10.1083/jcb.109.1.309
Savage, C., Das, P., Finelli, A. L., Townsend, S. R., Sun, C. Y., Baird, S. E., et al. (1996). Caenorhabditis elegans genes sma-2, sma-3, and sma-4 define a conserved family of transforming growth factor beta pathway components. Proc. Natl. Acad. Sci. 93 (2), 790–794. LP – 794. doi:10.1073/pnas.93.2.790
Schilling, T. F., and Kimmel, C. B. (1994). Segment and cell type lineage restrictions during pharyngeal arch development in the zebrafish embryo. Development 120 (3), 483–494. doi:10.1242/dev.120.3.483
Schilling, T. F., and Kimmel, C. B. (1997). Musculoskeletal patterning in the pharyngeal segments of the zebrafish embryo. Development 124 (15), 2945–2960. doi:10.1242/dev.124.15.2945
Schilling, T. F., Piotrowski, T., Grandel, H., Brand, M., Heisenberg, C. P., Jiang, Y. J., et al. (1996). Jaw and branchial arch mutants in zebrafish I: branchial arches. Development 123 (1), 329–344. doi:10.1242/dev.123.1.329
Schmid, B., Fürthauer, M., Connors, S. A., Trout, J., Thisse, B., Thisse, C., et al. (2000). Equivalent genetic roles for bmp7/snailhouse and bmp2b/swirl in dorsoventral pattern formation. Development 127 (5), 957–967. doi:10.1242/dev.127.5.957
Schofield, D. J., Shrestha, R. N., Percival, R., Passey, M. E., Callander, E. J., and Kelly, S. J. (2013). The personal and national costs of lost labour force participation due to arthritis: an economic study. BMC Public Health 13, 188. doi:10.1186/1471-2458-13-188
Schulte-Merker, S., Lee, K. J., McMahon, A. P., and Hammerschmidt, M. (1997). The zebrafish organizer requires chordino. Nature 387 (6636), 862–863. doi:10.1038/43092
Schumacher, J. A., Hashiguchi, M., Nguyen, V. H., and Mullins, M. C. (2011). An intermediate level of BMP signaling directly specifies cranial neural crest progenitor cells in zebrafish. PloS one 6 (11), e27403. doi:10.1371/journal.pone.0027403
Schwaerzer, G. K., Hiepen, C., Schrewe, H., Nickel, J., Ploeger, F., Sebald, W., et al. (2012). New insights into the molecular mechanism of multiple synostoses syndrome (SYNS): mutation within the GDF5 knuckle epitope causes noggin-resistance. J. Bone Mineral Res. 27 (2), 429–442. doi:10.1002/jbmr.532
Seegmiller, R. E., and Fraser, F. C. (1977). Mandibular growth retardation as a cause of cleft palate in mice homozygous for the chondrodysplasia gene. Development 38 (1), 227–238. doi:10.1242/dev.38.1.227
Sekelsky, J. J., Newfeld, S. J., Raftery, L. A., Chartoff, E. H., and Gelbart, W. M. (1995). Genetic characterization and cloning of mothers against dpp, a gene required for decapentaplegic function in Drosophila melanogaster. Genetics 139 (3), 1347–1358. doi:10.1093/genetics/139.3.1347
Sekiya, T., Oda, T., Matsuura, K., and Akiyama, T. (2004). Transcriptional regulation of the TGF-beta pseudoreceptor BAMBI by TGF-beta signaling. Biochem. Biophysical Res. Commun. 320 (3), 680–684. doi:10.1016/j.bbrc.2004.06.017
Seo, H.-C., Saetre, B. O., Håvik, B., Ellingsen, S., and Fjose, A. (1998). The zebrafish Pax3 and Pax7 homologues are highly conserved, encode multiple isoforms and show dynamic segment-like expression in the developing brain. Mech. Dev. 70 (1), 49–63. doi:10.1016/s0925-4773(97)00175-5
Settle, S. H., Rountree, R. B., Sinha, A., Thacker, A., Higgins, K., and Kingsley, D. M. (2003). Multiple joint and skeletal patterning defects caused by single and double mutations in the mouse Gdf6 and Gdf5 genes. Dev. Biol. 254 (1), 116–130. doi:10.1016/s0012-1606(02)00022-2
Sheehan-Rooney, K., Swartz, M. E., Zhao, F., Liu, D., and Eberhart, J. K. (2013). Ahsa1 and Hsp90 activity confers more severe craniofacial phenotypes in a zebrafish model of hypoparathyroidism, sensorineural deafness and renal dysplasia (HDR). Dis. Models Mech. 6 (5), 1285–1291. doi:10.1242/dmm.011965
Shen, K., Krakora, S. M., Cunningham, M., Singh, M., Wang, X., Hu, F. Z., et al. (2009). Medical treatment of craniosynostosis: recombinant Noggin inhibits coronal suture closure in the rat craniosynostosis model. Orthod. Craniofacial Res. 12 (3), 254–262. doi:10.1111/j.1601-6343.2009.01460.x
Shi, M., Zhu, J., Wang, R., Chen, X., Mi, L., Walz, T., et al. (2011). Latent TGF-β structure and activation. Nature 474 (7351), 343–349. doi:10.1038/nature10152
Shi, W., Chang, C., Nie, S., Xie, S., Wan, M., and Cao, X. (2007). Endofin acts as a Smad anchor for receptor activation in BMP signaling. J. Cell Sci. 120 (7), 1216–1224. doi:10.1242/jcs.03400
Shkoukani, M. A., Lawrence, L. A., Liebertz, D. J., and Svider, P. F. (2014). Cleft palate: a clinical review. Birth Defects Res. Part C 102 (4), 333–342. doi:10.1002/bdrc.21083
Siewert, A., Reiz, B., Krug, C., Heggemann, J., Mangold, E., Dickten, H., et al. (2023). Analysis of candidate genes for cleft lip ± cleft palate using murine single-cell expression data. Front. Cell Dev. Biol. 11, 1091666. doi:10.3389/fcell.2023.1091666
Sisson, B. E., Dale, R. M., Mui, S. R., Topczewska, J. M., and Topczewski, J. (2015). A role of glypican4 and wnt5b in chondrocyte stacking underlying craniofacial cartilage morphogenesis. Mech. Dev. 138, 279–290. doi:10.1016/j.mod.2015.10.001
Smeeton, J., Natarajan, N., Anderson, T., Tseng, K. C., Fabian, P., and Crump, J. G. (2022). Regeneration of jaw joint cartilage in adult zebrafish. Front. Cell Dev. Biol. 9, 777787. doi:10.3389/fcell.2021.777787
Song, Z., Liu, C., Iwata, J., Gu, S., Suzuki, A., Sun, C., et al. (2013). Mice with Tak1 deficiency in neural crest lineage exhibit cleft palate associated with abnormal tongue development. J. Biol. Chem. 288 (15), 10440–10450. doi:10.1074/jbc.M112.432286
Souchelnytskyi, S., Tamaki, K., Engström, U., Wernstedt, C., ten Dijke, P., and Heldin, C. H. (1997). Phosphorylation of Ser465 and Ser467 in the C terminus of Smad2 mediates interaction with Smad4 and is required for transforming growth factor-beta signaling. J. Biol. Chem. 272 (44), 28107–28115. doi:10.1074/jbc.272.44.28107
Stanton, E., Urata, M., Chen, J. F., and Chai, Y. (2022). The clinical manifestations, molecular mechanisms and treatment of craniosynostosis. Dis. Models Mech. 15 (4), dmm049390. doi:10.1242/dmm.049390
Stewart, R. A., Arduini, B. L., Berghmans, S., George, R. E., Kanki, J. P., Henion, P. D., et al. (2006). Zebrafish foxd3 is selectively required for neural crest specification, migration and survival. Dev. Biol. 292 (1), 174–188. doi:10.1016/j.ydbio.2005.12.035
Storm, E. E., Huynh, T. V., Copeland, N. G., Jenkins, N. A., Kingsley, D. M., and Lee, S. J. (1994). Limb alterations in brachypodism mice due to mutations in a new member of the TGF beta-superfamily. Nature 368 (6472), 639–643. doi:10.1038/368639a0
Storm, E. E., and Kingsley, D. M. (1996). Joint patterning defects caused by single and double mutations in members of the bone morphogenetic protein (BMP) family. Development 122 (12), 3969–3979. doi:10.1242/dev.122.12.3969
Styrkarsdottir, U., Lund, S. H., Thorleifsson, G., Zink, F., Stefansson, O. A., Sigurdsson, J. K., et al. (2018). Meta-analysis of Icelandic and UK data sets identifies missense variants in SMO, IL11, COL11A1 and 13 more new loci associated with osteoarthritis. Nat. Genet. 50 (12), 1681–1687. doi:10.1038/s41588-018-0247-0
Suazo, J., Santos, J. L., Carreño, H., Jara, L., and Blanco, R. (2004). Linkage disequilibrium between MSX1 and non-syndromic cleft lip/palate in the Chilean population. J. Dent. Res. 83 (10), 782–785. doi:10.1177/154405910408301009
Sun, J., Lin, Y., Ha, N., Zhang, J., Wang, W., Wang, X., et al. (2023). Single-cell RNA-Seq reveals transcriptional regulatory networks directing the development of mouse maxillary prominence. J. Genet. Genomics 50 (9), 676–687. doi:10.1016/j.jgg.2023.02.008
Suzuki, S., Marazita, M. L., Cooper, M. E., Miwa, N., Hing, A., Jugessur, A., et al. (2009). Mutations in BMP4 are associated with subepithelial, microform, and overt cleft lip. Am. J. Hum. Genet. 84 (3), 406–411. doi:10.1016/j.ajhg.2009.02.002
Suzuki, Y., Jezewski, P. A., Machida, J., Watanabe, Y., Shi, M., Cooper, M. E., et al. (2004). In a Vietnamese population, MSX1 variants contribute to cleft lip and palate. Genet. Med. 6 (3), 117–125. doi:10.1097/01.gim.0000127275.52925.05
Swartz, M. E., Lovely, C. B., and Eberhart, J. K. (2021). Variation in phenotypes from a Bmp-Gata3 genetic pathway is modulated by Shh signaling. PLOS Genet. 17 (5), e1009579. doi:10.1371/journal.pgen.1009579
Swartz, M. E., Sheehan-Rooney, K., Dixon, M. J., and Eberhart, J. K. (2011). Examination of a palatogenic gene program in zebrafish. Dev. Dyn. 240 (9), 2204–2220. doi:10.1002/dvdy.22713
Swartz, M. E., Wells, M. B., Griffin, M., McCarthy, N., Lovely, C. B., McGurk, P., et al. (2014). A screen of zebrafish mutants identifies ethanol-sensitive genetic loci. Alcohol. Clin. Exp. Res. 38 (3), 694–703. doi:10.1111/acer.12286
Tachmazidou, I., Hatzikotoulas, K., Southam, L., Esparza-Gordillo, J., Haberland, V., Zheng, J., et al. (2019). Identification of new therapeutic targets for osteoarthritis through genome-wide analyses of UK Biobank data. Nat. Genet. 51 (2), 230–236. doi:10.1038/s41588-018-0327-1
Takahashi, T., Takahashi, I., Komatsu, M., Sawaishi, Y., Higashi, K., Nishimura, G., et al. (2001). Mutations of the NOG gene in individuals with proximal symphalangism and multiple synostosis syndrome. Clin. Genet. 60 (6), 447–451. doi:10.1034/j.1399-0004.2001.600607.x
Talbot, J. C., Johnson, S. L., and Kimmel, C. B. (2010). hand2 and Dlx genes specify dorsal, intermediate and ventral domains within zebrafish pharyngeal arches. Development 137 (15), 2507–2517. doi:10.1242/dev.049700
Tassabehji, M., Fang, Z. M., Hilton, E. N., McGaughran, J., Zhao, Z., de Bock, C. E., et al. (2008). Mutations in GDF6 are associated with vertebral segmentation defects in Klippel-Feil syndrome. Hum. Mutat. 29 (8), 1017–1027. doi:10.1002/humu.20741
Teng, C. S., Ting, M. C., Farmer, D. T., Brockop, M., Maxson, R. E., and Crump, J. G. (2018). Altered bone growth dynamics prefigure craniosynostosis in a zebrafish model of Saethre-Chotzen syndrome. eLife 7, e37024. doi:10.7554/eLife.37024
Terhal, P. A., Verbeek, N. E., Knoers, N., Nievelstein, R. J. A. J., van den Ouweland, A., Sakkers, R. J., et al. (2018). Further delineation of the GDF6 related multiple synostoses syndrome. Am. J. Med. Genet. Part A 176 (1), 225–229. doi:10.1002/ajmg.a.38503
Thisse, C., Thisse, B., and Postlethwait, J. H. (1995). Expression of snail2, a second member of the zebrafish snail family, in cephalic mesendoderm and presumptive neural crest of wild-type and spadetail mutant embryos. Cephalic Mesendoderm Presumptive Neural Crest Wild-Type andspadetailMutant Embryos’ Dev. Biol. 172 (1), 86–99. doi:10.1006/dbio.1995.0007
Timberlake, A. T., Choi, J., Zaidi, S., Lu, Q., Nelson-Williams, C., Brooks, E. D., et al. (2016). Two locus inheritance of non-syndromic midline craniosynostosis via rare SMAD6 and common BMP2 alleles. eLife 5, e20125. doi:10.7554/eLife.20125
Timberlake, A. T., Furey, C. G., Choi, J., Nelson-Williams, C., Loring, E., Loring, E., et al. (2017). De novo mutations in inhibitors of Wnt, BMP, and Ras/ERK signaling pathways in non-syndromic midline craniosynostosis. Proc. Natl. Acad. Sci. 114 (35), 7341–7347. doi:10.1073/pnas.1709255114
Timberlake, A. T., Kiziltug, E., Jin, S. C., Nelson-Williams, C., Loring, E., Allocco, A., et al. (2023). De novo mutations in the BMP signaling pathway in lambdoid craniosynostosis. Hum. Genet. 142 (1), 21–32. doi:10.1007/s00439-022-02477-2
Timberlake, A. T., Wu, R., Nelson-Williams, C., Furey, C. G., Hildebrand, K. I., Elton, S. W., et al. (2018). Co-occurrence of frameshift mutations in SMAD6 and TCF12 in a child with complex craniosynostosis. Hum. Genome Var. 5 (1), 14. doi:10.1038/s41439-018-0014-x
Topczewska, J. M., Shoela, R. A., Tomaszewski, J. P., Mirmira, R. B., and Gosain, A. K. (2016). The morphogenesis of cranial sutures in zebrafish. PLOS ONE 11 (11), e0165775. doi:10.1371/journal.pone.0165775
Tríbulo, C., Aybar, M. J., Nguyen, V. H., Mullins, M. C., and Mayor, R. (2003). Regulation of Msx genes by a Bmp gradient is essential for neural crest specification. Development 130 (26), 6441–6452. doi:10.1242/dev.00878
Tsukazaki, T., Chiang, T. A., Davison, A. F., Attisano, L., and Wrana, J. L. (1998). SARA, a FYVE domain protein that recruits Smad2 to the TGFbeta receptor. Cell 95 (6), 779–791. doi:10.1016/s0092-8674(00)81701-8
Tsuneizumi, K., Nakayama, T., Kamoshida, Y., Kornberg, T. B., Christian, J. L., and Tabata, T. (1997). Daughters against dpp modulates dpp organizing activity in Drosophila wing development. Nature 389 (6651), 627–631. doi:10.1038/39362
Ueharu, H., and Mishina, Y. (2023). BMP signaling during craniofacial development: new insights into pathological mechanisms leading to craniofacial anomalies. Front. Physiology 14, 1170511. doi:10.3389/fphys.2023.1170511
Ueharu, H., Pan, H., Liu, X., Ishii, M., Pongetti, J., Kulkarni, A. K., et al. (2023). Augmentation of BMP signaling in cranial neural crest cells leads to premature cranial sutures fusion through endochondral ossification in mice. JBMR Plus 7 (4), e10716. doi:10.1002/jbm4.10716
Valdes, A. M., Evangelou, E., Kerkhof, H. J. M., Tamm, A., Doherty, S. A., Kisand, K., et al. (2011). The GDF5 rs143383 polymorphism is associated with osteoarthritis of the knee with genome-wide statistical significance. Ann. Rheumatic Dis. 70 (5), 873–875. doi:10.1136/ard.2010.134155
van de Laar, I. M. B. H., Oldenburg, R. A., Pals, G., Roos-Hesselink, J. W., de Graaf, B. M., Verhagen, J. M. A., et al. (2011). Mutations in SMAD3 cause a syndromic form of aortic aneurysms and dissections with early-onset osteoarthritis. Nat. Genet. 43 (2), 121–126. doi:10.1038/ng.744
van de Laar, I. M. B. H., van der Linde, D., Oei, E. H. G., Bos, P. K., Bessems, J. H., Bierma-Zeinstra, S. M., et al. (2012). Phenotypic spectrum of the SMAD3-related aneurysms–osteoarthritis syndrome. J. Med. Genet. 49 (1), 47–57. doi:10.1136/jmedgenet-2011-100382
van den Ende, J. J., Mattelaer, P., Declau, F., Vanhoenacker, F., Claes, J., Van Hul, E., et al. (2005). The facio-audio-symphalangism syndrome in a four generation family with a nonsense mutation in the NOG-gene. Clin. Dysmorphol. 14 (2), 73–80. doi:10.1097/00019605-200504000-00004
Verrecchia, F., and Mauviel, A. (2002). Transforming growth factor-beta signaling through the Smad pathway: role in extracellular matrix gene expression and regulation. J. Investigative Dermatology 118 (2), 211–215. doi:10.1046/j.1523-1747.2002.01641.x
Vural, A. C., Odabas, S., Korkusuz, P., Yar Sağlam, A. S., Bilgiç, E., Çavuşoğlu, T., et al. (2017). Cranial bone regeneration via BMP-2 encoding mesenchymal stem cells. Artif. Cells, Nanomedicine, Biotechnol. 45 (3), 544–550. doi:10.3109/21691401.2016.1160918
Wada, N., Javidan, Y., Nelson, S., Carney, T. J., Kelsh, R. N., and Schilling, T. F. (2005). Hedgehog signaling is required for cranial neural crest morphogenesis and chondrogenesis at the midline in the zebrafish skull. Development 132 (17), 3977–3988. doi:10.1242/dev.01943
Wakefield, L. M., Smith, D. M., Broz, S., Jackson, M., Levinson, A. D., and Sporn, M. B. (1989). Recombinant TGF-beta 1 is synthesized as a two-component latent complex that shares some structural features with the native platelet latent TGF-beta 1 complex. Growth Factors. 1 (3), 203–218. doi:10.3109/08977198908997997
Wakefield, L. M., Smith, D. M., Flanders, K. C., and Sporn, M. B. (1988). Latent transforming growth factor-beta from human platelets. A high molecular weight complex containing precursor sequences. J. Biol. Chem. 263 (16), 7646–7654. doi:10.1016/s0021-9258(18)68547-3
Waldmann, L., Leyhr, J., Zhang, H., Allalou, A., Öhman-Mägi, C., and Haitina, T. (2022). The role of Gdf5 in the development of the zebrafish fin endoskeleton. Dev. Dyn. 251 (9), 1535–1549. doi:10.1002/dvdy.399
Wang, J., Yu, T., Wang, Z., Ohte, S., Yao, R. E., Zheng, Z., et al. (2016). A new subtype of multiple synostoses syndrome is caused by a mutation in GDF6 that decreases its sensitivity to noggin and enhances its potency as a BMP signal. J. Bone Mineral Res. 31 (4), 882–889. doi:10.1002/jbmr.2761
Wang, X.-F., Lin, H. Y., Ng-Eaton, E., Downward, J., Lodish, H. F., and Weinberg, R. A. (1991). Expression cloning and characterization of the TGF-beta type III receptor. Cell 67 (4), 797–805. doi:10.1016/0092-8674(91)90074-9
Warren, S. M., Brunet, L. J., Harland, R. M., Economides, A. N., and Longaker, M. T. (2003). The BMP antagonist noggin regulates cranial suture fusion. Nature 422 (6932), 625–629. doi:10.1038/nature01545
Weeks, D. L., and Melton, D. A. (1987). A maternal mRNA localized to the vegetal hemisphere in Xenopus eggs codes for a growth factor related to TGF-beta. Cell 51 (5), 861–867. doi:10.1016/0092-8674(87)90109-7
Williams, E. S., Uhas, K. A., Bunke, B. P., Garber, K. B., and Martin, C. L. (2012). Cleft palate in a multigenerational family with a microdeletion of 20p12.3 involving BMP2. Am. J. Med. Genet. Part A 158A (10), 2616–2620. doi:10.1002/ajmg.a.35594
Wolfman, N. M., Hattersley, G., Cox, K., Celeste, A. J., Nelson, R., Yamaji, N., et al. (1997). Ectopic induction of tendon and ligament in rats by growth and differentiation factors 5, 6, and 7, members of the TGF-beta gene family. J. Clin. Investigation 100 (2), 321–330. doi:10.1172/JCI119537
Won, H.-J., Kim, J. W., and Shin, J. O. (2023). Gene regulatory networks and signaling pathways in palatogenesis and cleft palate: a comprehensive review. Cells 12 (15), 1954. doi:10.3390/cells12151954
Woo, K., and Fraser, S. E. (1995). Order and coherence in the fate map of the zebrafish nervous system. Development 121 (8), 2595–2609. doi:10.1242/dev.121.8.2595
Woolf, A. D., and Pfleger, B. (2003). Burden of major musculoskeletal conditions. Bull. World Health Organ. 81 (9), 646–656.
Wozney, J. M., Rosen, V., Celeste, A. J., Mitsock, L. M., Whitters, M. J., Kriz, R. W., et al. (1988). Novel regulators of bone formation: molecular clones and activities. Science 242 (4885), 1528–1534. doi:10.1126/science.3201241
Wrana, J. L., Attisano, L., Cárcamo, J., Zentella, A., Doody, J., Laiho, M., et al. (1992). TGF-beta signals through a heteromeric protein kinase receptor complex. Cell 71 (6), 1003–1014. doi:10.1016/0092-8674(92)90395-s
Wrana, J. L., Tran, H., Attisano, L., Arora, K., Childs, S. R., Massagué, J., et al. (1994). Two distinct transmembrane serine/threonine kinases from Drosophila melanogaster form an activin receptor complex. Mol. Cell. Biol. 14 (2), 944–950. doi:10.1128/mcb.14.2.944
Wu, R. T., Timberlake, A. T., Abraham, P. F., Gabrick, K. S., Lu, X., Peck, C. J., et al. (2020). SMAD6 genotype predicts neurodevelopment in nonsyndromic craniosynostosis. Plastic Reconstr. Surg. 145 (1), 117e–125e. doi:10.1097/PRS.0000000000006319
Wu, R.-Y., Zhang, Y., Feng, X. H., and Derynck, R. (1997). Heteromeric and homomeric interactions correlate with signaling activity and functional cooperativity of Smad3 and Smad4/DPC4. Mol. Cell. Biol. 17 (5), 2521–2528. doi:10.1128/mcb.17.5.2521
Wyatt, A. W., Osborne, R. J., Stewart, H., and Ragge, N. K. (2010). Bone morphogenetic protein 7 (BMP7) mutations are associated with variable ocular, brain, ear, palate, and skeletal anomalies. Hum. Mutat. 31 (7), 781–787. doi:10.1002/humu.21280
Xu, P., Balczerski, B., Ciozda, A., Louie, K., Oralova, V., Huysseune, A., et al. (2018). Fox proteins are modular competency factors for facial cartilage and tooth specification. Development 145 (12), dev165498. doi:10.1242/dev.165498
Yamaguchi, K., Shirakabe, K., Shibuya, H., Irie, K., Oishi, I., Ueno, N., et al. (1995). Identification of a member of the MAPKKK family as a potential mediator of TGF-beta signal transduction. Science 270 (5244), 2008–2011. doi:10.1126/science.270.5244.2008
Yamashita, H., Ichijo, H., Grimsby, S., Morén, A., ten Dijke, P., and Miyazono, K. (1994). Endoglin forms a heteromeric complex with the signaling receptors for transforming growth factor-beta. J. Biol. Chem. 269 (3), 1995–2001. doi:10.1016/s0021-9258(17)42126-0
Yan, X., Liao, H., Cheng, M., Shi, X., Lin, X., Feng, X. H., et al. (2016). Smad7 protein interacts with receptor-regulated smads (R-Smads) to inhibit transforming growth factor-β (TGF-β)/Smad signaling. J. Biol. Chem. 291 (1), 382–392. doi:10.1074/jbc.M115.694281
Yan, X., Lin, Z., Chen, F., Zhao, X., Chen, H., Ning, Y., et al. (2009). Human BAMBI cooperates with Smad7 to inhibit transforming growth factor-beta signaling. J. Biol. Chem. 284 (44), 30097–30104. doi:10.1074/jbc.M109.049304
Yan, Y.-L., Miller, C. T., Nissen, R. M., Singer, A., Liu, D., Kirn, A., et al. (2002). A zebrafish sox9 gene required for cartilage morphogenesis. Development 129 (21), 5065–5079. doi:10.1242/dev.129.21.5065
Yan, Y.-L., Willoughby, J., Liu, D., Crump, J. G., Wilson, C., Miller, C. T., et al. (2005). A pair of Sox: distinct and overlapping functions of zebrafish sox9 co-orthologs in craniofacial and pectoral fin development. Development 132 (5), 1069–1083. doi:10.1242/dev.01674
Yang, Y., Yuan, J., Yao, X., Zhang, R., Yang, H., Zhao, R., et al. (2017). BMPR1B mutation causes Pierre Robin sequence. Oncotarget 8 (16), 25864–25871. doi:10.18632/oncotarget.16531
Ye, M., Berry-Wynne, K. M., Asai-Coakwell, M., Sundaresan, P., Footz, T., French, C. R., et al. (2010). Mutation of the bone morphogenetic protein GDF3 causes ocular and skeletal anomalies. Hum. Mol. Genet. 19 (2), 287–298. doi:10.1093/hmg/ddp496
Yeo, C.-Y., and Whitman, M. (2001). Nodal signals to smads through cripto-dependent and cripto-independent mechanisms. Mol. Cell 7 (5), 949–957. doi:10.1016/s1097-2765(01)00249-0
Yoshida, T., Vivatbutsiri, P., Morriss-Kay, G., Saga, Y., and Iseki, S. (2008). Cell lineage in mammalian craniofacial mesenchyme. Mech. Dev. 125 (9), 797–808. doi:10.1016/j.mod.2008.06.007
Yu, Q., He, S., Zeng, N., Ma, J., Zhang, B., Shi, B., et al. (2015). BMP7 Gene involved in nonsyndromic orofacial clefts in Western han Chinese. Med. Oral, Patol. Oral Cirugia Bucal 20 (3), e298–e304. doi:10.4317/medoral.20335
Yu, Q., and Stamenkovic, I. (2000). Cell surface-localized matrix metalloproteinase-9 proteolytically activates TGF-β and promotes tumor invasion and angiogenesis. Genes & Dev. 14 (2), 163–176. doi:10.1101/gad.14.2.163
Yu, T., Li, G., Wang, C., Yao, R., and Wang, J. (2023). Defective joint development and maintenance in GDF6-related multiple synostoses syndrome. J. Bone Mineral Res. 38 (4), 568–577. doi:10.1002/jbmr.4785
Yumoto, K., Thomas, P. S., Lane, J., Matsuzaki, K., Inagaki, M., Ninomiya-Tsuji, J., et al. (2013). TGF-β-activated kinase 1 (Tak1) mediates agonist-induced Smad activation and linker region phosphorylation in embryonic craniofacial neural crest-derived cells. J. Biol. Chem. 288 (19), 13467–13480. doi:10.1074/jbc.M112.431775
Zawel, L., Dai, J. L., Buckhaults, P., Zhou, S., Kinzler, K. W., Vogelstein, B., et al. (1998). Human Smad3 and Smad4 are sequence-specific transcription activators. Mol. Cell 1 (4), 611–617. doi:10.1016/s1097-2765(00)80061-1
Zhang, J., Qiu, L. Y., Kotzsch, A., Weidauer, S., Patterson, L., Hammerschmidt, M., et al. (2008). Crystal structure analysis reveals how the Chordin family member crossveinless 2 blocks BMP-2 receptor binding. Dev. Cell 14 (5), 739–750. doi:10.1016/j.devcel.2008.02.017
Zhang, W., Zeng, Q., Xu, Y., Ying, H., Zhou, W., Cao, Q., et al. (2017). Exome sequencing identified a novel SMAD2 mutation in a Chinese family with early onset aortic aneurysms. Clin. Chim. Acta 468, 211–214. doi:10.1016/j.cca.2017.03.007
Zhang, Y., Chang, C., Gehling, D. J., Hemmati-Brivanlou, A., and Derynck, R. (2001). Regulation of Smad degradation and activity by Smurf2, an E3 ubiquitin ligase. Proc. Natl. Acad. Sci. 98 (3), 974–979. doi:10.1073/pnas.98.3.974
Zhang, Y., Musci, T., and Derynck, R. (1997). The tumor suppressor Smad4/DPC 4 as a central mediator of Smad function. Curr. Biol. 7 (4), 270–276. doi:10.1016/s0960-9822(06)00123-0
Zhou, H., Zou, S., Lan, Y., Fei, W., Jiang, R., and Hu, J. (2014). Smad7 modulates TGFβ signaling during cranial suture development to maintain suture patency. J. Bone Mineral Res. 29 (3), 716–724. doi:10.1002/jbmr.2066
Zhu, H., Kavsak, P., Abdollah, S., Wrana, J. L., and Thomsen, G. H. (1999). A SMAD ubiquitin ligase targets the BMP pathway and affects embryonic pattern formation. Nature 400 (6745), 687–693. doi:10.1038/23293
Zimmerman, L. B., De Jesús-Escobar, J. M., and Harland, R. M. (1996). The spemann organizer signal noggin binds and inactivates bone morphogenetic protein 4. Cell 86 (4), 599–606. doi:10.1016/s0092-8674(00)80133-6
Zuniga, E., Rippen, M., Alexander, C., Schilling, T. F., and Crump, J. G. (2011). Gremlin 2 regulates distinct roles of BMP and Endothelin 1 signaling in dorsoventral patterning of the facial skeleton. Development 138 (23), 5147–5156. doi:10.1242/dev.067785
Keywords: TGF-β, BMP, signal transduction, craniofacial development, disease, zebrafish
Citation: Fox SC and Waskiewicz AJ (2024) Transforming growth factor beta signaling and craniofacial development: modeling human diseases in zebrafish. Front. Cell Dev. Biol. 12:1338070. doi: 10.3389/fcell.2024.1338070
Received: 14 November 2023; Accepted: 18 January 2024;
Published: 07 February 2024.
Edited by:
Antionette Latrece Williams, Ann & Robert H. Lurie Children’s Hospital of Chicago, United StatesReviewed by:
Johannes W. Von den Hoff, Radboud University Medical Centre, NetherlandsSylvain Marcellini, University of Concepcion, Chile
Copyright © 2024 Fox and Waskiewicz. This is an open-access article distributed under the terms of the Creative Commons Attribution License (CC BY). The use, distribution or reproduction in other forums is permitted, provided the original author(s) and the copyright owner(s) are credited and that the original publication in this journal is cited, in accordance with accepted academic practice. No use, distribution or reproduction is permitted which does not comply with these terms.
*Correspondence: Andrew J. Waskiewicz, YXdAdWFsYmVydGEuY2E=