- 1CRTD—Center for Regenerative Therapies, and PoL—Cluster of Excellence Physics of Life, Dresden, Germany
Inflammation can lead to persistent and irreversible loss of retinal neurons and photoreceptors in mammalian vertebrates. In contrast, in the adult zebrafish brain, acute neural inflammation is both necessary and sufficient to stimulate regeneration of neurons. Here, we report on the critical, positive role of the immune system to support retina regeneration in adult zebrafish. After sterile ablation of photoreceptors by phototoxicity, we find rapid response of immune cells, especially monocytes/microglia and neutrophils, which returns to homeostatic levels within 14 days post lesion. Pharmacological or genetic impairment of the immune system results in a reduced Müller glia stem cell response, seen as decreased reactive proliferation, and a strikingly reduced number of regenerated cells from them, including photoreceptors. Conversely, injection of the immune stimulators flagellin, zymosan, or M-CSF into the vitreous of the eye, leads to a robust proliferation response and the upregulation of regeneration-associated marker genes in Müller glia. Our results suggest that neuroinflammation is a necessary and sufficient driver for retinal regeneration in the adult zebrafish retina.
Introduction
Immune system activation is one of the first responses to tissue damage, e.g., by infection, disease or injury. Cells of the immune system (leukocytes) can recognize invading pathogens or factors that are secreted by damaged or dying cells (Ferrero-Miliani et al., 2007). Subsequently, leukocytes accumulate at the affected area, removing pathogens and clearing cellular debris, thus supporting the reestablishment of a physiological balance (Nathan and Ding, 2010). Conversely, if inflammation cannot be resolved, a detrimental chronic inflammation can occur causing progressive tissue damage and pathology (Zhou et al., 2016; Akhtar-Schäfer et al., 2018).
In the lesioned mammalian central nervous system (CNS), accumulation of reactive astrocytes often results in a glial scar that acts as a barrier for successful regeneration (Buffo et al., 2008; Fitch and Silver, 2008; Sofroniew, 2009). Similarly, neurodegenerative diseases like Parkinson’s or Alzheimer’s show characteristics of chronic inflammation, causing subsequent neuronal death (Amor et al., 2010; Herrero et al., 2015; Kinney et al., 2018).
In contrast to mammals, injuries of the zebrafish CNS, such as brain, retina, spinal chord or optic nerve, result in a strong regenerative response despite an initial inflammation (Fausett and Goldman, 2006; Bernardos et al., 2007; Fimbel et al., 2007; Kroehne et al., 2011; Kyritsis et al., 2012; Kizil et al., 2015; White et al., 2017; Bosak et al., 2018; Mitchell et al., 2018; Tsarouchas et al., 2018; Bollaerts et al., 2019; Silva et al., 2020; Zhang et al., 2020). Whereas immune system activation in the mammalian CNS is typically detrimental for regeneration, studies in zebrafish demonstrated a strong beneficial link between an immune response and neural stem cell reactivity (Kyritsis et al., 2012; Aurora and Olson, 2014; Zhao et al., 2014; Bosak et al., 2018; Tsarouchas et al., 2018; Van Dyck et al., 2021; Fogerty et al., 2022; Iribarne and Hyde, 2022). Remarkably, in the zebrafish adult telencephalon, inflammation is required to initiate a successful regenerative response, and a lipid inflammatory cue, leukotriene-C4, is sufficient to stimulate proliferation of radial glia-type stem cells (Kyritsis et al., 2012; Kizil et al., 2015).
In the retina, zebrafish Müller glial cells similarly act as stem cells, and generate neuronal precursor cells (NPCs) in response to retinal lesion (Fausett and Goldman, 2006; Bernardos et al., 2007; Fimbel et al., 2007; Goldman, 2014; Gorsuch and Hyde, 2014; Lenkowski and Raymond, 2014). These cells then amplify, migrate to the lesion site, and differentiate into the lost neuronal subtypes and thus gradually restore vision (Raymond et al., 2006; Hammer et al., 2022). Dying neurons release the proinflammatory cytokine TNF-α, triggering the regenerative response of Müller glia (Nelson et al., 2013; Conner et al., 2014). Likewise, the inflammation-associated factors Interleukin-11 and TGF-ß stimulate Müller glia cell cycle re-entry and NPC generation (Lenkowski et al., 2013; Zhao et al., 2014). Furthermore, microglia—the CNS tissue resident macrophages—support this initial regenerative response by secreting proinflammatory factors (Kizil et al., 2015; Conedera et al., 2019; Zhang et al., 2020; Iribarne and Hyde, 2022). To date the molecular pathways involved in damage recognition, stem cell proliferation, neuronal precursor cell amplification and differentiation during retinal regeneration are poorly understood; in particular, the role of inflammation is unclear (Lenkowski and Raymond, 2014; Mitchell et al., 2018; 2019; Lahne et al., 2020; Iribarne and Hyde, 2022).
Here, we analyze the contribution of inflammation to regeneration using a non-invasive, sterile phototoxic ablation model of photoreceptor cells, as the key cell type affected by retinal disease, in the adult zebrafish retina (Weber et al., 2013; Mitchell et al., 2018; Silva et al., 2020; Zhang et al., 2020). Following light lesion, we observe strong convergence of tissue resident microglia to the lesion site. Blocking inflammation pharmacologically caused reduced reactive proliferation of Müller glia stem cells and impaired photoreceptor regeneration. Similarly, in a genetic model of microglia deficiency, we find that microglia support Müller cell proliferation. Conversely, when the immune stimulators flagellin, zymosan, or M-CSF are injected into the vitreous of the eye, Müller glial cells are triggered to undergo reactive proliferation and regeneration-associated marker gene expression. Taken together, our results show that, in the regeneration-competent adult zebrafish retina, acute inflammation is an important positive regulator of retina regeneration.
Results
Leukocytes react to sterile ablation of photoreceptor cells
The immune system of vertebrates rapidly responds to retinal damage. In larval zebrafish, mpeg1:mCherry positive monocytes react to photoreceptor ablation by rapid migration towards the site of lesion (White et al., 2017). Similarly, microglia respond by accumulation and phagocytosis of debris in response to neurotoxic ablation of inner retinal cells in adult zebrafish (Mitchell et al., 2018). To investigate if leukocytes are recruited in an injury model at adult stages, we used intense diffuse light that causes sterile ablation of all photoreceptor subtypes by phototoxicity (Weber et al., 2013). In this model, due to the refractive properties of the adult zebrafish visual system, the photoreceptors in a central stripe of the retina are ablated, whereas ventral and dorsal retina is much less affected (Weber et al., 2013; Figure 1A). In flatmounts of Tg(mpeg1:mCherry) x Tg(opn1sw1:GFP) double transgenic zebrafish that express GFP as a marker in the entire UV cone population, the central lesion is marked by the absence of GFP, and microglia can be nicely observed to accumulate in the central region at 2 days post lesion (dpl; Figure 1A). In sham conditions, microglia display an equal distribution and a ramified structure in the inner retina, and only few cells are detected around the rod outer segments; whereas following a light lesion, they accumulate in the central lesion area, and disappear from the unlesioned peripheral regions and from the rod outer segments (Supplementary Figure S1). We analyzed the accumulation and appearance of leukocytes in the central retina further on sections by immunohistochemistry for L-Plastin, a pan-leukocyte marker (Redd et al., 2006; Kroehne et al., 2011) in Tg(mpeg1:mCherry) reporter animals, labeling the monocyte lineage, including macrophages and microglia (Figure 1A) and - as recently reported—a subset of B- and NK-cells (Ferrero et al., 2020; Moyse and Richardson, 2020). In comparison to unlesioned (sham) controls, L-Plastin positive cells accumulated in the central lesion zone already at 2 dpl (Figures 1A,B). Whereas leukocytes showed a ramified morphology in sham control retinae, their appearance changed to an amoeboid and swollen shape at 2 dpl (insets in Figures 1A,D), as described by Mitchell et al., 2018 upon neurotoxic lesion. At 2 dpl an increased number of L-Plastin positive leukocytes at the outer nuclear layer (ONL) in comparison to sham controls is revealed (Figures 1A,B). In a time course, the number of L-Plastin positive cells peaked at 2 dpl and subsequently declined to sham levels at 14 dpl (Figure 1C). To further determine the identity of retinal leukocytes, we analyzed immunoreactivity of L-Plastin in transgenic Tg(mpeg1:mCherry) animals. In sham control retinae, L-Plastin-positive cells always co-expressed mpeg1:mCherry, indicating that all tissue resident homeostatic leukocytes were of the monocyte lineage, namely, microglia (Figure 1B). In contrast, additional L-Plastin positive, but mpeg1:mCherry negative cells could be detected upon injury at 2 dpl (insets in Figure 1B). Subsequent quantification of the number of L-Plastin and mpeg1:mCherry double positive cells revealed a decrease from 99% to approximately 83%, indicating the presence of another cell type of the leukocyte lineage at the lesion site (Supplementary Figure S2A). Here, neutrophils are known to rapidly respond to tissue damage and are labeled by transgenic Tg(mpo:GFP) (Renshaw et al., 2016; Kurimoto et al., 2013; Figures 1D, Supplementary Figure S2B). In contrast to sham controls, which never showed any mpo:GFP positive cells in the homeostatic retina, we observed rapid accumulation of neutrophils after light lesion. mpo:GFP positive cells first appearing in the retina by 12 hpl, and a diffuse GFP positive pattern (referred to as matrix), in the outer segment layer and the inner nuclear layer (INL) was present from 15 hpl onwards (Figure 1C), presumably reflecting neutrophil NETosis (Zhu et al., 2021). Analyses of Tg(mpeg1:mCherry) and Tg(mpo:GFP) double transgenic animals revealed that mpeg1:mCherry positive cells co-localized with the GFP positive matrix, suggesting an uptake of the matrix material by monocytes (Supplementary Figure S2B). T cells of the lymphoid lineage were reported to stimulate Müller glia proliferation, and to augment retina regeneration in a stab wound assay (Hui et al., 2017). To examine if T cells are also recruited to the lesion site, we used transgenic Tg(lck:NLS-DsRed) animals, but could not detect any lck positive T cells, neither in the homeostatic nor in the regenerating retina up to 7 days post lesion (Supplementary Figure S3).
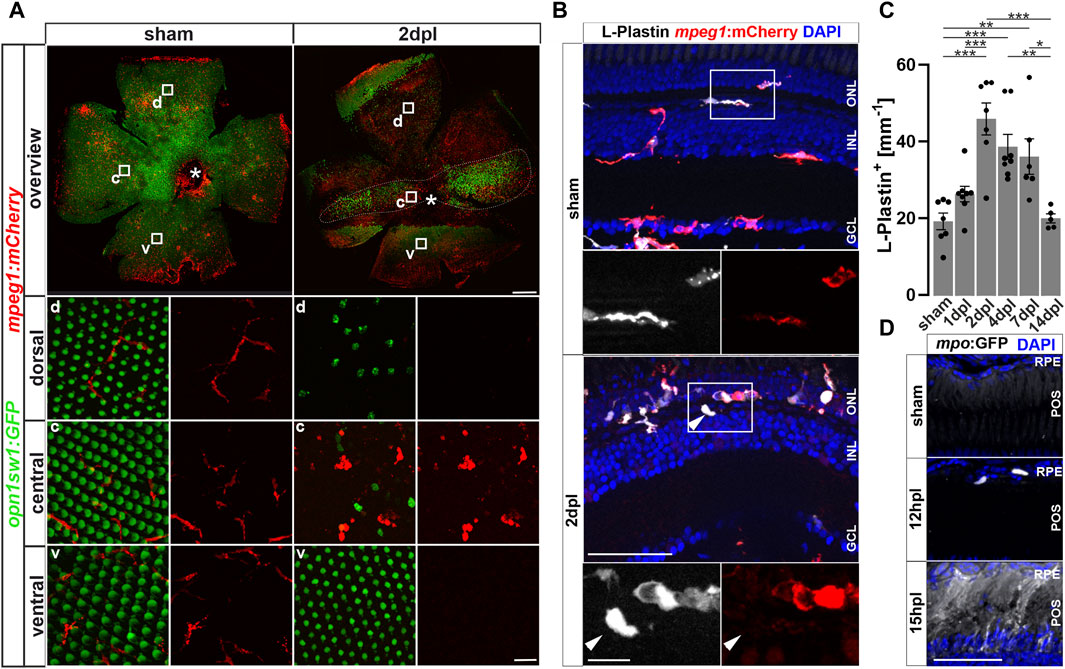
Figure 1. Sterile phototoxic ablation of photoreceptors triggers leukocyte accumulation. (A) Retinal flat mounts of Tg(opn1sw1:GFP) x Tg(mpeg1:mCherry) show the ramified structure of leukocytes in a UV-cone-specific reporter line and their reaction to lesion. Upon light lesion, leukocytes increase in number at the lesion site and display an amoeboid, activated morphology and accumulate in the central part of the lesion, while unharmed areas appear devoid of mpeg1:mCherry positive cells. A central stripe of autofluorescence can be seen (dashed lines) at 2 dpl. In the corresponding inset a few remaining UV-cones are visible. The optic nerve head is indicated by an asterisk. (B) Retinal sections show that the majority of L-Plastin+ cells are Tg(mpeg1:mCherry)+, display morphological changes after lesion, and accumulate at the outer nuclear layer (ONL) in response to lesion. However, L-Plastin+ Tg(mpeg1:mCherry) negative cells were also observed upon injury (arrowhead). (C) Quantification on sections of L-Plastin+ cells in sham and regenerating retinae at 1, 2, 4, 7 and 14 days post lesion (dpl) shows an increase of cells within 2 dpl that is resolved within 14 dpl. (D) In contrast to sham, Tg(mpo:GFP)+ neutrophils are detected upon lesion. They gather at the lesion site (12 hpl) and a diffuse GFP positive pattern (matrix) is formed from 15hpl onwards. Scale bars in A: overview 200 μm, Insets 10 µm; Scale Bars in D: overview 50µm, insets 10µm; error bars indicate SEM; * = p ≤ 0.05; ** = p ≤ 0.01; *** = p < 0.001; N ≥ 6; one-way ANOVA Tukey’s post hoc analysis; ONL = outer nuclear layer, INL = inner nuclear layer; GCL = ganglion cell layer; POS = photoreceptor outer segments; RPE = retina pigment epithelium; d = dorsal; c = central; v = ventral.
Taken together, our data show a strong accumulation and activation of innate immune cells following a sterile light lesion that is resolved by 14 dpl, consistent with the mounting of an acute inflammatory response after retinal injury.
Müller glia activate NF-κB signaling in response to injury
In zebrafish, Müller glia have key functions in the regulation of retinal homeostasis as well as during regeneration (Lenkowski and Raymond, 2014). To study how Müller glia react to injury during regeneration and inflammation, we analyzed the activation of the proinflammatory signaling pathway NF-κB using Tg(NF-κB:GFP) x Tg(gfap:NLS-mCherry) transgenic animals. The Tg(NF-κB:GFP) line reports canonical NF-κB activity via the expression of GFP under the control of six human NF-κB binding motifs driving a c-fos minimal promoter (Kanther et al., 2011). Of note, the newly generated Tg(gfap:NLS-mCherry) reporter line shows mCherry leakage into the cytoplasm (Figure 2A), probably due to a passive mCherry leakage from the nucleus to the cytosol that is not fully compensated by the nuclear import, as observed also for our Tg(gfap:NLS-GFP) reporter (Semmelink et al., 2021). In sham control retinae, NF-κB:GFP expression was only found in the retinal vasculature and microglia (Figures 2A, Supplementary Figure S4). In sharp contrast, robust activation of NF-κB:GFP was present in numerous gfap:NLS-mCherry positive Müller glia already at 1 dpl (Figures 2A, Supplementary Figure S4). Moreover, NF-κB:GFP expression was present in cells located in the ONL showing the characteristic morphology of photoreceptor cells. Both cell types continued to express NF-κB:GFP at 2 dpl. At 4 dpl, NF-κB:GFP expression was strongly decreased in the ONL but remained detectable in Müller glia. To determine if NF-κB activation might be functionally relevant, we investigated the expression of matrix metallopeptidase 9 (mmp9), a known downstream target of NF-κB with important functions in degrading extracellular matrix and chemokines (Cheng et al., 2012; LeBert et al., 2015; Yang et al., 2017; Xu et al., 2018). Thus, we performed mmp9 in situ hybridization in combination with immunohistochemistry against glial fibrillary acidic protein (GFAP/Zrf-1) labeling Müller glia and proliferating cell nuclear antigen (PCNA) labeling cells in S-phase and shortly after. In contrast to sham controls that never showed mmp9 expression in the homeostatic retina, mmp9 was strongly expressed by Zrf-1 positive Müller glia at 1 dpl, indicating de novo mmp9 expression in these cells (Figure 2B). Moreover, numerous mmp9 and Zrf-1 expressing cells were also positive for PCNA, identifying them as reactively proliferating Müller glia in response to injury. Expression of mmp9 remained strong at 2 dpl, but dropped below detection levels at 4 dpl, consistent with transcriptome data for mmp9 (Silva et al., 2020; Kramer et al., 2021; Celotto et al., 2023). Taken together, our results show that the NF-κB signaling pathway is transiently activated in Müller glia in response to a sterile ablation of photoreceptor cells, indicative of an inflammatory response by Müller glia.
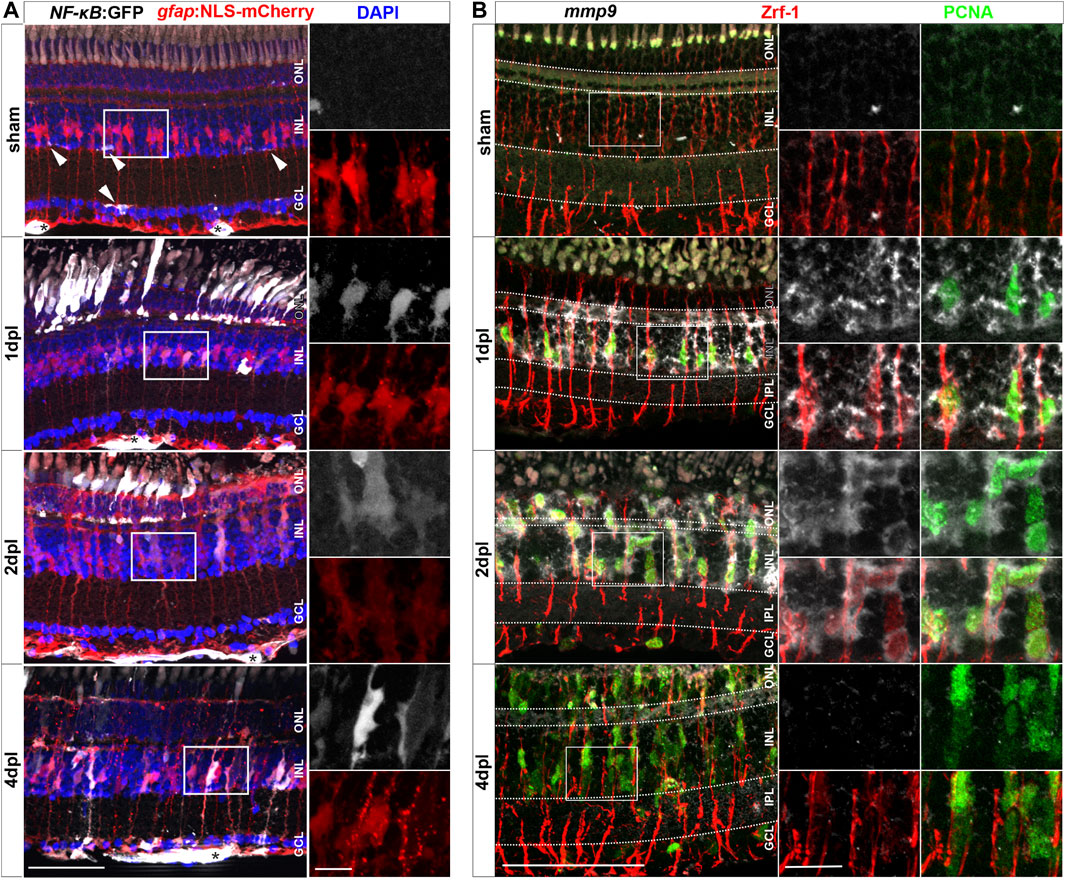
Figure 2. Müller glia transiently activate the NF-κB:GFP reporter and express the NF-κB target metalloproteinase mmp9 in response to injury. (A) In homeostatic retina the NF-κB:GFP reporter is expressed in the vasculature residing below the ganglion cell layer (asterisk) and in microglial cells (arrowheads). In comparison to sham, the NF-κB:GFP reporter is activated in response to lesion in gfap:NLS-mCherry labeled Müller glia, and in photoreceptors at the outer nuclear layer (ONL), and is predominantly active in Müller cells at 4 dpl. (B) In situ hybridization of mmp9, in combination with immunohistochemistry for proliferating cell nuclear antigen (PCNA) and glial fibrillary acidic protein (GFAP/Zrf-1) labeling Müller glia, shows that mmp9 is not expressed in sham retinae. In contrast, mmp9 is transiently expressed at one and 2 dpl and returns to undetectable levels at 4 dpl. Scale bar: 50 µm, insets 10 µm. INL = inner nuclear layer; GCL = ganglion cell layer.
Dexamethasone-mediated immunosuppression reduces retinal regeneration
To study the role of the immune system during retinal regeneration, we used Dexamethasone (Dex), a potent immunosuppressant (Coutinho and Chapman, 2011; Kyritsis et al., 2012; Gallina et al., 2015; Silva et al., 2020; Zhang et al., 2020). Experimental zebrafish were treated with Dex from 10 days prior to lesion until the time point of analysis (Figure 3A). Vehicle control experiments were carried out with the respective amount of the solvent methanol (MeOH). In contrast to MeOH-treated controls, Dex-treatment for 10 consecutive days resulted in an overall reduction of retinal microglia (Figure 3B). Similarly, leukocyte recruitment upon light lesion was significantly reduced in Dex-treated animals at 2 dpl, compared to control (Figure 3B). Quantification of L-Plastin positive cells corroborated a significant reduction at all indicated time points (Figure 3C). In addition, Dex-treatment reduced the number of resident retinal microglia during homeostasis, and no increase in the overall number of leukocytes was noted after lesion. To address if the observed changes might be caused indirectly by the known neuroprotective properties of Dex (Gallina et al., 2015), we analyzed cell death using the TdT-mediated dUTP-digoxigenin nick end labeling (TUNEL) assay; however, we did not observe any difference in the number of TUNEL positive cells after light lesion in control MeOH- or Dex-treated animals (Supplementary Figure S5).
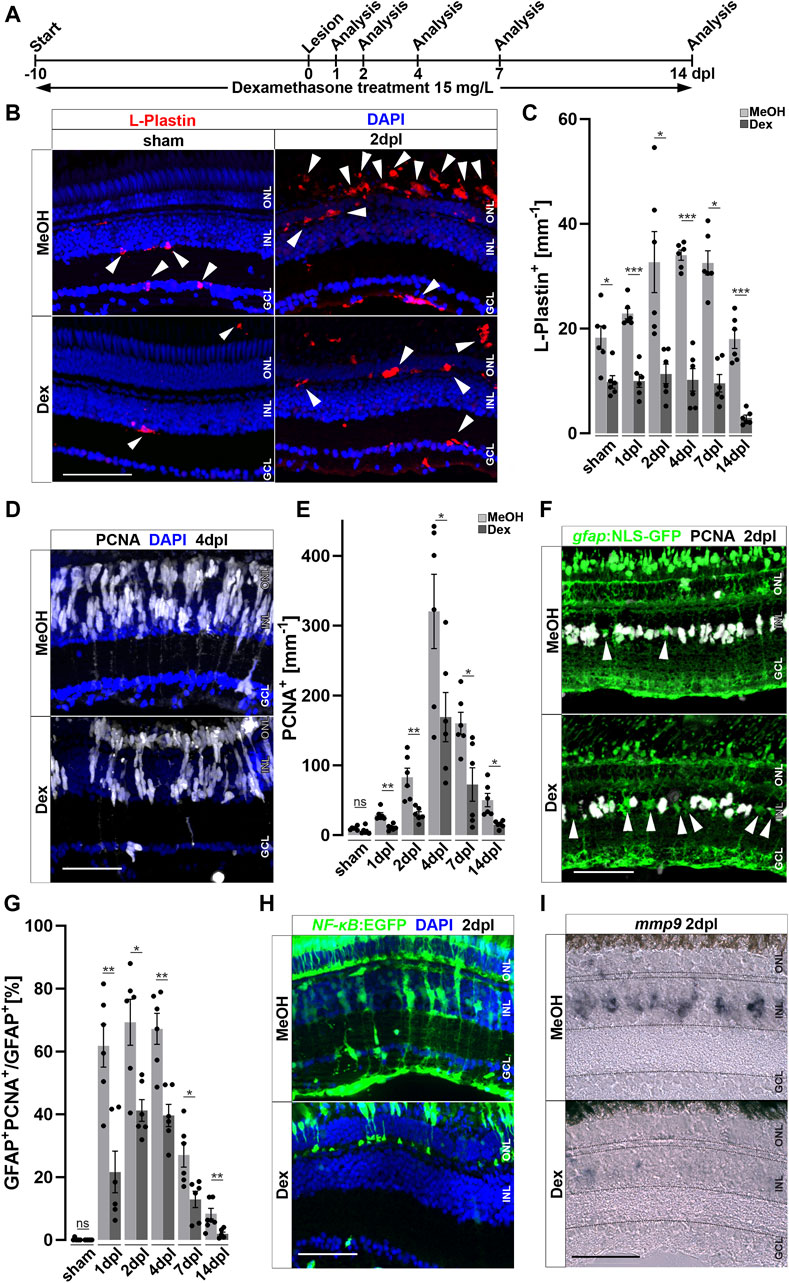
Figure 3. Immunosuppression interferes with leukocyte accumulation and Müller glia reactivity. (A) Scheme of experimental outline. Fish were treated with Dexamethasone (Dex) or vehicle (Methanol; MeOH) from 10 days prior to injury until the day of analysis (sham, 1, 2, 4, 7 and 14 days post lesion; dpl). (B) Dex-treatment reduces the number of L-Plastin+ leukocytes (arrowheads) in sham (left panel) and regenerating retinae at 2 dpl (right panel). (C) Quantification of L-Plastin + cells in MeOH- and Dex-treated sham or lesioned animals at 1, 2, 4, 7 and 14 dpl. (D) Immunohistochemistry for proliferating cell nuclear antigen (PCNA) reveals impaired proliferation in Dex-treated animals at 4 dpl. (E) Quantification of PCNA + cells in MeOH- and Dex-treated sham and regenerating retinae at 1, 2, 4, 7 and 14 dpl indicates impaired proliferative response in the Dex-treated group. (F) Immunohistochemistry for PCNA in gfap:NLS-GFP labeled Müller glia shows reduced numbers of proliferating Müller glia in the Dex treated group at 2 dpl compared to MeOH controls (arrowheads indicating non proliferative Müller cells). (G) Quantification of proliferating Müller glia in vehicle and Dex-treated sham and regenerating retinae at 1, 2, 4, 7, and 14 dpl, indicating that Dex hinders Müller glia proliferation. (H) In comparison to vehicle, the NFκB:GFP reporter is not activated in the inner nuclear layer (INL) of Dex-treated retinae at 2 dpl. (I) Injury-induced expression of mmp9 is strongly reduced in Dex-treated retinae at 2 dpl. Retinal layering is indicated by dashed lines. Scale bar: 50 μm, Error bars indicate SEM; ns = p > 0.05, * = p ≤ 0.05; ** = p ≤ 0.01; *** = p < 0.001; N = 6; two-tailed t-Test, ONL = outer nuclear layer, INL = inner nuclear layer GCL = ganglion cell layer.
Reactive proliferation of Müller glia is a hallmark of retinal regeneration; we therefore further analyzed if Dex-treatment influences proliferation after sterile light lesion using immunolabeling of the proliferation marker PCNA (Figure 3D). In contrast to MeOH-treated controls, Dex-treated animals showed a significant reduction in PCNA positive cells at 4 dpl. Quantification of PCNA positive cells during the course of regeneration revealed that reactive proliferation, driven by Müller cells and neuronal progenitors, is significantly reduced in Dex-treated animals at all time-points examined in comparison to MeOH controls. Furthermore, homeostatic proliferation (most likely by cells of the ciliary margin) in unlesioned retinae is not affected (Figure 3E). To investigate if Dex-treatment specifically affects Müller glia proliferation, we analyzed the number of PCNA positive cells in transgenic Tg(gfap:NLS-GFP) animals, which express strong nuclear GFP in all Müller glia (with some leakage of GFP to the cytoplasm, identifying the characteristic Müller glia cell shape, Figure 3F). Indeed, in comparison to MeOH-treated animals, the number of PCNA and gfap:NLS-GFP double positive cells was significantly reduced after Dex-treatment. Quantification showed that the percentage of Müller glia co-localizing with PCNA is reduced to less than ∼50% at all analyzed time points (Figure 3G). This suggests that Müller glia reactive proliferation is impaired upon Dex-treatment. Consistent with this possibility, NF-κB activation is reduced in Müller glia after Dex-treatment, as seen in the Tg(NF-κB:GFP) reporter line indicating impaired Müller cell reactivity (Figure 3H). As expected, cells in the ONL and Müller glia show a robust NF-κB:GFP expression in MeOH-treated control animals at 2 dpl, whereas a reduced number of cells in the ONL activated the NF-κB:GFP transgene and no GFP positive Müller glia could be detected at 2 dpl after Dex-treatment. Consistently, activation of the NF-κB downstream target mmp9 was almost absent in Dex-treated animals at 2 dpl, compared to MeOH controls (Figure 3I). Taken together, these results show that Dex-mediated immunosuppression efficiently reduces the accumulation of leukocytes and impairs Müller glia reactivity, as well as reactive proliferation and regeneration-associated marker gene expression, during retinal regeneration.
Immunosuppression reduces regeneration of photoreceptors
To examine if the reduced cell proliferation has consequences for the regenerative outcome, we probed the effect of Dex-immunosuppression on restoration of photoreceptors after light lesion. Thus, we performed Dex-treatment and light lesions on Tg(opn1sw1:GFP) animals expressing GFP in all UV cones, followed by repeated EdU injections to label newborn cells, and analyzed the animals at 28 dpl when cellular regeneration is completed (Figure 4A). Consistent with the afore-mentioned decrease of retinal leukocytes after Dex-treatment, we observed an almost complete loss of retinal leukocytes after 38 days of continuous immunosuppression/28 dpl (Supplementary Figure S6). In comparison to MeOH-treated control animals, the overall number of EdU positive cells was significantly lower after Dex-treatment at 28 dpl (Figure 4B). Similarly, the number of EdU positive UV-cones, as a subpopulation for the regenerated photoreceptors (Figure 4C), was severely decreased in Dex-treated animals compared to MeOH-treated controls. Moreover, in addition to a reduction in number, EdU positive UV cones appeared malformed with improperly shaped outer segments in Dex-treated animals (insets Figure 4B). Despite the fact that the diffuse light lesion only affects photoreceptor cells, EdU positive nuclei are also found in the ganglion and inner nuclear layer. Quantifications show that the number of EdU positive nuclei were similarly reduced in all retinal layers in the dexamethasone treated group (Figure 4C). Of note, a regenerative bias in non-ablated nucleated layers in different lesion paradigms of the zebrafish retina is described by Powell et al., 2016. In conclusion, Dex-mediated immunosuppression interferes with reactive proliferation and reactive neurogenesis, indicating that the injury-induced immune response is essential for proper retina regeneration.
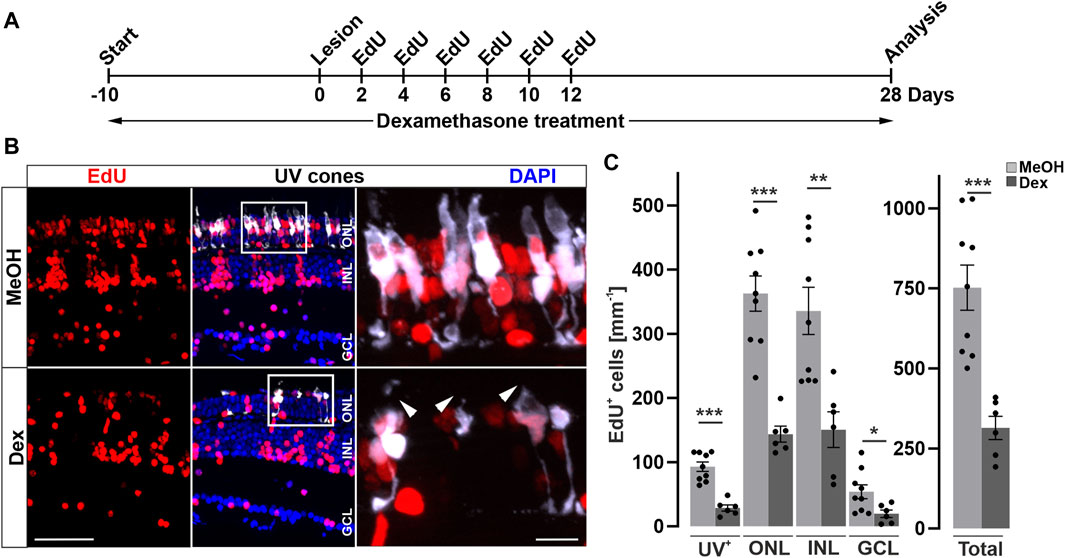
Figure 4. Long-term immune suppression impairs photoreceptor regeneration. (A) Scheme of experimental outline. opn1sw1:GFP transgenic animals (labeling UV-cones) were treated with Dexamethasone (Dex) or vehicle (Methanol; MeOH) from 10 days prior to lesion until the day of analysis (28 days post lesion; dpl; 38 days post treatment; dpt). EdU pulses were applied at 2, 4, 6, 8, 10, and 12 dpl. (B) In comparison to MeOH-treated animals, the number of regenerated EdU+ UV-cones is significantly reduced after Dex-treatment (compare upper panel with lower panel). Furthermore, the morphology of photoreceptor cells is disrupted in comparison to MeOH controls (arrowheads). (C) Quantification of EdU+ cells with respect to retinal layers show significant reduction of EdU positive nuclei in the Dex-treated group. Scale bars: 50µm, insets 10 µm. Error bars indicate SEM; * = p ≤ 0.05; ** = p ≤ 0.01; *** = p < 0.001; N ≥ 6; two-tailed t-Test. ONL = outer nuclear layer; INL = inner nuclear layer; GCL = ganglion cell layer.
Retinal microglia appear to support reactive Müller glia proliferation
Activated microglia and macrophages clear debris from dead cells, and they interact with Müller glia and influence their cellular response (Wang et al., 2011; Keightley et al., 2014; Aslanidis et al., 2015; Palazzo et al., 2020). Consistently, our results show that Dex-mediated immunosuppression interferes with reactive proliferation and neurogenesis, indicating that the injury-induced immune response is essential for successful retina regeneration. To independently test this notion, we investigated whether microglia positively contribute to reactive proliferation during retina regeneration, using Interferon regulatory factor 8 (irf8) myeloid-defective mutants to genetically deplete microglia in embryonic and juvenile fish (Shiau et al., 2015). To verify leukocyte deficiency at adult stages, we analyzed L-Plastin immunoreactivity in homozygous adult irf8 mutants and heterozygous control siblings, and found that the number of leukocytes in the homeostatic retina was significantly reduced in irf8 mutants, but not completely absent (Figures 5A,B). Next, we asked whether the microglia deficiency also affects the proliferation of Müller cells and NPCs during regeneration. We therefore combined the light lesion paradigm with EdU-labeling at 3 dpl prior to analysis at 4 dpl (Figure 5C). Compared to heterozygous control siblings, the number of accumulated L-Plastin positive cells was clearly reduced in irf8 mutant animals at 4 dpl (Figure 5D). Similarly, the number of EdU positive cells is lower in irf8 mutants at 4 dpl compared to heterozygous controls (Figure 5D). Quantification of the L-Plastin positive cells highlights that the irf8 mutants have strong deficits in the recruitment/accumulation of leukocytes towards the lesion. Additionally, the number of EdU positive cells indicates a reduction in the proliferative response to damage (Figure 5E). Thus, consistent with our above findings using Dex-mediated immunosuppression, these results indicate that genetic depletion of leukocytes correlates with decreased reactive proliferation upon injury, supporting the notion of an important role of immune cells during zebrafish retina regeneration.
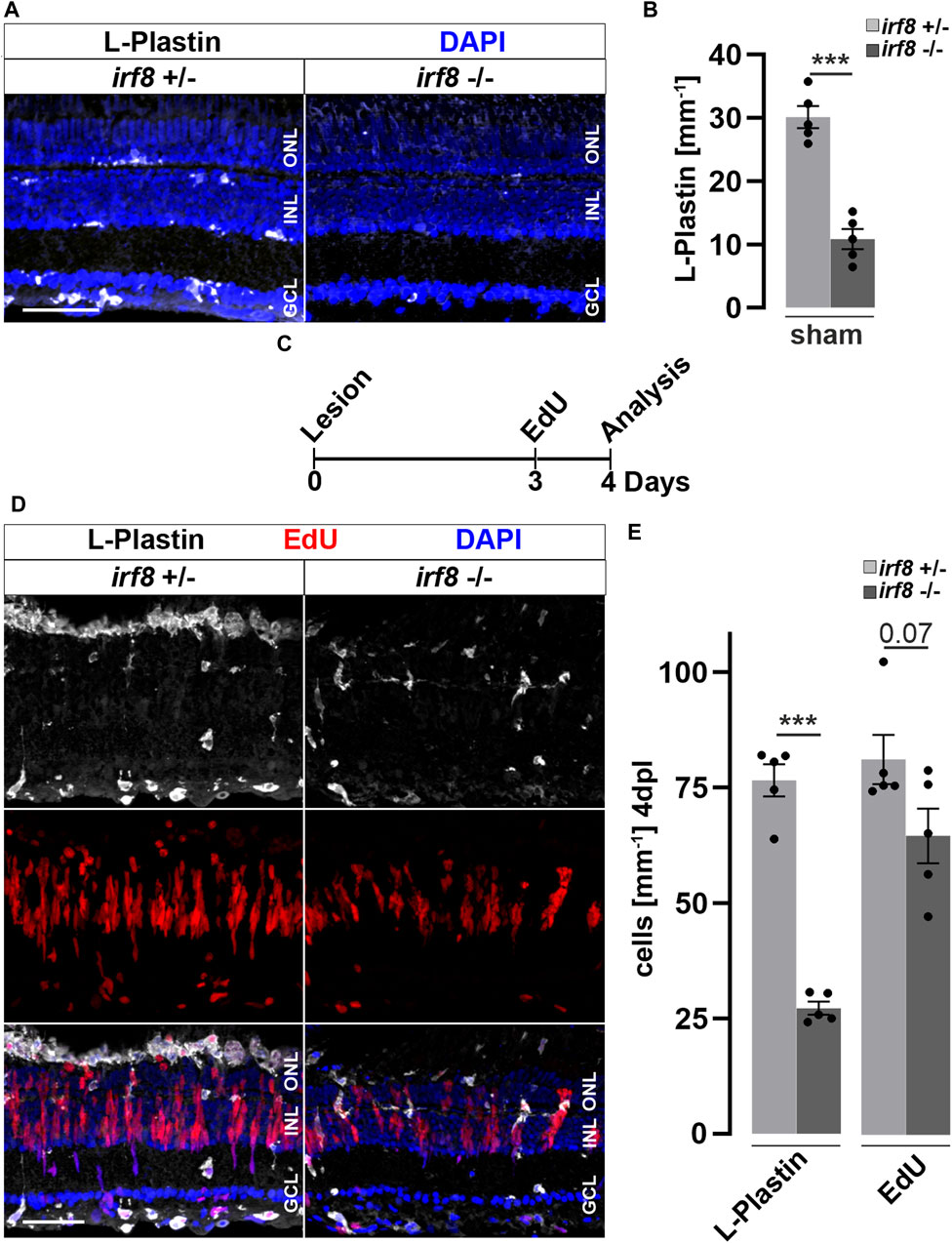
Figure 5. Genetic reduction of microglia impairs retinal reactive proliferation in response to injury. (A) In contrast to heterozygous control siblings (irf8+/−), interferon regulatory factor 8 (irf8) homozygous myeloid-deficient mutant retinae (irf8−/−) display a decreased number of L-Plastin+ leukocytes in homeostasis. (B) Quantifications of L-Plastin+ cells in irf8−/− and control heterozygous siblings irf8+/−. (C) Scheme of experimental outline. irf8−/− and irf8+/− animals received an EdU pulse at 3 days post lesion (dpl) and were analyzed at 4 dpl. (D) During regeneration, the accumulation of L-Plastin+ cells at the outer nuclear layer (ONL) is impaired in irf8−/− but not in control irf8+/− siblings. Moreover, the number of EdU+ cells appears decreased in irf8−/− retinae. (E) Quantification of L-Plastin+ cells and EdU+ nuclei in irf8−/− and control irf8+/− animals at 4 dpl, showing reduced amounts of positive cells, respectively. Scale bar: 50 µm. Error bars indicate SEM; *** = p < 0.001; N = 5; two-tailed t-Test; INL = inner nuclear layer; GCL ganglion cell layer.
Inflammatory stimuli trigger Müller glia reactivity and reactive proliferation
We hypothesized that inflammation might not only be necessary, but also sufficient, to trigger reactive proliferation of Müller glia and the generation of NPCs. To address this notion, we injected different immune activators into the vitreous of the eye—thus leaving the retina unlesioned (Figure 6A) - and analyzed the response of Müller glia at 2 and 4 days post injections (dpi; Figure 6). The toll-like receptor (TLR) agonist zymosan has previously been shown to stimulate an inflammatory response both in the zebrafish and mouse eye, and in the zebrafish telencephalon (Kyritsis et al., 2012; Kurimoto et al., 2013; Zhang et al., 2020). Next, we tested injections of flagellin, the principal structural protein of bacterial flagella, which is known to cause sepsis in zebrafish larvae (Barber et al., 2016). As a control, to ensure that injection of both zymosan and flagellin did not cause retinal cell death and thereby trigger a regenerative response indirectly, we performed TUNEL assays at two dpi. Neither zymosan nor flagellin injection resulted in a significant increase of TUNEL positive cells in comparison to control PBS-injected eyes, indicating that compounds injected do not cause cell death per se (Figure 6B). Additionally, the number of DAPI positive nuclei as well as pyknotic nuclei among these groups are similar, although a small decrease from sham (uninjected) retinae can be detected (Supplementary Figure S7), indicating that some neuronal loss is caused by the injection into the vitreous. Interestingly, the number of L-Plastin positive cells also did not significantly increase after injecting zymosan or flagellin (Figure 6C). In contrast, both zymosan as well as flagellin-injected retinae show a strong cell proliferation marked by PCNA immunohistochemistry which appears to be almost absent in PBS-injected controls (Figure 6D). Quantification of PCNA positive nuclei confirmed a significant increase in zymosan and flagellin-injected animals at four dpi, but not at two dpi (Figure 6E). Furthermore, we analyzed activity of NF-κB after injections of PBS, zymosan and flagellin using the NF-κB:GFP reporter line. Consistent with the afore-mentioned expression in non-injected retinae, PBS-injected controls show NF-κB:GFP expression in blood vessels, and microglia (Figure 6F). In contrast, NF-κB:GFP is strongly activated in additional cells of the inner and outer nuclear layer in zymosan and flagellin-injected animals at two dpi. Again, GFP positive cells in the ONL showed mostly the characteristic morphology of photoreceptor cells (see also above, Figures 2B, 3H), in particular in the zymosan-injected animals. Consistent with the activation of the NF-κB:GFP reporter, retinal expression of mmp9 is also found in zymosan and flagellin-injected animals, but not in PBS-injected controls at two dpi (Figure 6G). Finally, we analyzed the expression of the transcription factor her4.1, a downstream target of the Notch signaling pathway that is upregulated in NPCs generated in response to photoreceptor damage (Wan et al., 2012). No expression of her4.1 is detected in PBS-injected controls, but her4.1 expression is activated in zymosan and flagellin-injected samples at four dpi (Figure 6H). These results show that activators of the immune system can strongly stimulate Müller glia reactivity and proliferation, even in the absence of tissue damage.
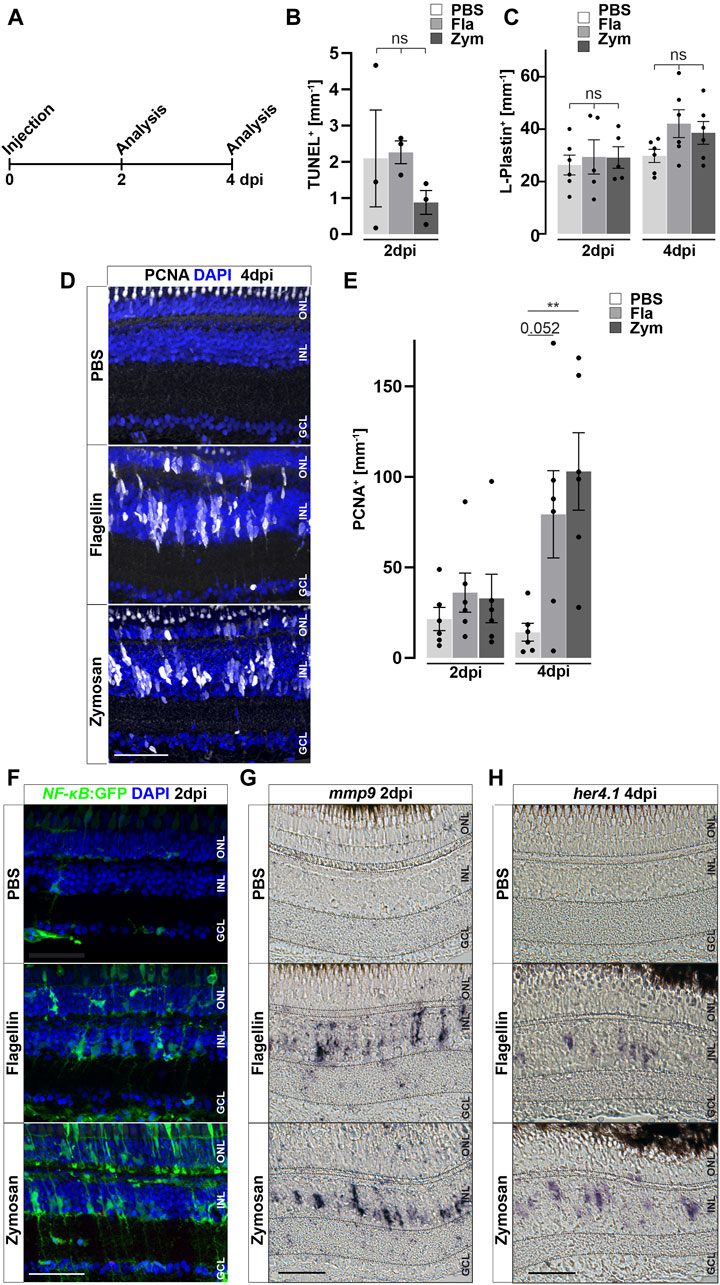
Figure 6. Inflammatory stimuli trigger Müller glia reactivity. (A) Scheme of experimental outline. Wild type or NF-κB:GFP reporter animals were injected with flagellin or zymosan into the vitreous of the eye and analyzed at 2 and 4 days post injection (dpi). (B) Quantification of TUNEL+ nuclei in control and injected retinae at two dpi revealing no increase in cell death. See also Supplementary Figure S7 for counts of pyknotic nuclei. (C) Quantification of L-Plastin+ cells in control (PBS) and flagellin or zymosan injected retinae at two and four dpi display no significant increase in leukocytes due to the injection of factors. (D) Immunohistochemistry for proliferating cell nuclear antigen (PCNA) reveals proliferation in flagellin or zymosan injected but not in control animals at four dpi. Signal in the outer nuclear layer (ONL) appears to be a non-specific labeling of photoreceptor cells. (E) Quantification of PCNA+ nuclei in injected retinae at two and four dpi. (F) In comparison to PBS injected animals, injection of flagellin or zymosan results in strong activation of the NFκB:GFP reporter at two dpi. (G & H) In situ hybridization of mmp9 and her4.1 show expression of both genes in flagellin or zymosan injected, but not in PBS injected, eyes at two and four dpi, respectively. Retinal layering is indicated by dashed lines. Scale bar: 50 μm. Error bars indicate standard error; * * = p≤ 0.01 B: N = 3 Fish; C&E: N = 6 Fish; One-tailed ANOVA; ONL = outer nuclear layer; INL = inner nuclear layer; GCL = ganglion cell layer.
M-CSF injection stimulates leukocyte and Müller glia reactivity in the absence of a lesion
In order to identify the stimulatory potential of specific individual inflammatory mediators, we focused on macrophage colony-stimulating-factor (M-CSF/CSF-1), based on preliminary data from our recent single-cell RNAseq dataset of regenerating retina (Celotto et al., 2023), and using the same injection paradigm as before (Figure 7A). In mammals, M-CSF stimulates CSF-1 receptor signaling, which is involved in monocyte colonization and stimulation (Chitu et al., 2016; Wu et al., 2018). Injection of human M-CSF induced an increased cell proliferation at four dpi compared to PBS controls (Figures 7B,C), despite the absence of unspecific damage to the retina, as seen by a lack of an increase in TUNEL positive cells at two dpi and the absence of an elevated amount of pyknotic nuclei (Supplementary Figure S7). Quantification corroborated a significant increase of proliferating cells, scored as PCNA positive nuclei, in M-CSF-injected retinae, compared to PBS-injected controls (Figure 7D). To address if M-CSF causes an increase in the number of retinal leukocytes, we performed immunolabeling of L-Plastin in M-CSF- and PBS-injected specimens. We observed a significant accumulation of L-Plastin positive cells in M-CSF, but not in PBS-injected, animals at four dpi (Figures 7E,F). Further, we found augmented NF-κB activity after M-CSF injection in NF-κB:GFP reporter animals. PBS-injected controls showed the homeostatic NF-κB:GFP expression described above (Figure 7G). In sharp contrast, additional NF-κB:GFP positive cells were found in the M-CSF-injected animals at two dpi, with the characteristic morphology of Müller glia. Interestingly, photoreceptor cells in the ONL did not activate the NF-κB:GFP reporter following M-CSF injection whereas they do so upon lesion (see above, Figures 2B, 3H), which we tentatively suggest to reflect a more selective role of M-CSF signaling for Müller glia. Consistent with the activation of NF-κB:GFP, mmp9 was also found to be expressed in M-CSF injected animals, but not in PBS-injected controls at two dpi (Figure 7H). Taken together, these data demonstrate that human recombinant M-CSF can induce leukocyte accumulation in the neuronal retina of zebrafish, and can stimulate Müller glia reactive proliferation and alter gene expression even in the absence of a lesion.
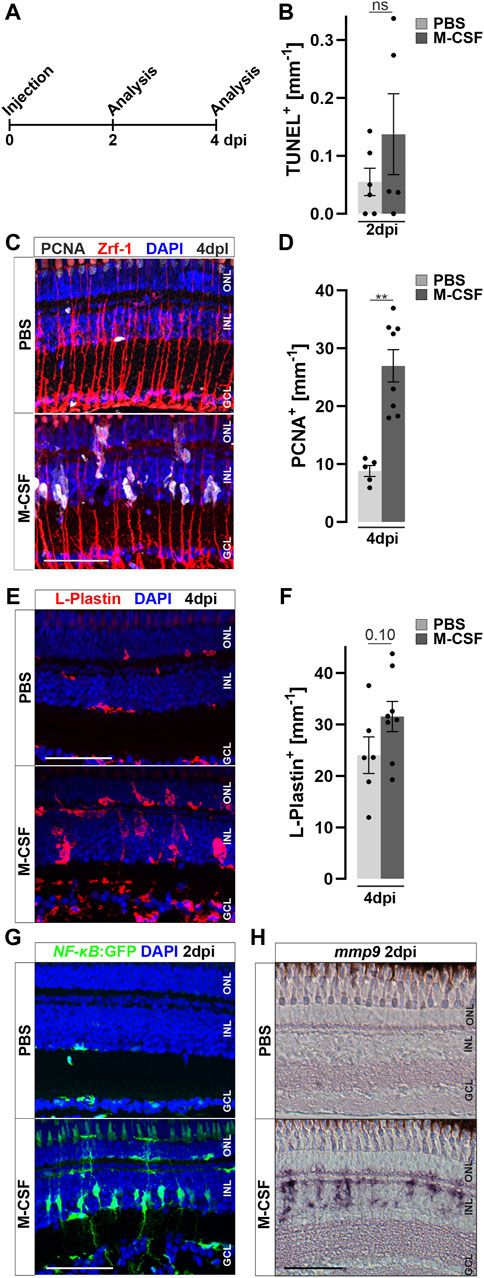
Figure 7. M-CSF stimulates an inflammatory response and initiates Müller glia cell cycle re-entry in the absence of a lesion. (A) Scheme of experimental outline. Wild type or NFκB:GFP reporter animals were injected with M-CSF or PBS into the vitreous of the eye and analyzed at 2 and 4 days post injection (dpi). (B) Quantification of TUNEL+ nuclei in control and M-CSF-injected retinae at two dpi reveals no chance of cell death. See also Supplementary Figure S7 for counts of pyknotic nuclei. (C) Immunohistochemistry for proliferating cell nuclear antigen (PCNA) reveals proliferation in close proximity to Zrf-1 (GFAP) positive Müller cells in M-CSF injected but not in control injected animals at four dpi. n both conditions a slight nonspecific labeling can be seen in the ONL. (D) Quantification of PCNA+ cells in control (PBS) and M-CSF injected retinae at four dpi. (E) L-Plastin staining shows more positive cells in M-CSF injected retinae at four dpi compared to controls. The location in the inner nuclear layer (INL) and morphology of the leukocytes indicates reactivity. (F) Quantification of L-Plastin+ cells in control and M-CSF injected retinae at four dpi, show accumulation of L-Plastin cells in M-CSF injected specimen. (G) In comparison to PBS, injection of M-CSF results in strong activation of the NFκB:GFP reporter at two dpi. (H) In situ hybridization of mmp9 shows transcriptional activation of this gene in M-CSF injected but not in control-injected animals at two dpi. Scale bars = 50µm, Error bars indicate SEM; ns > 0.05; ** = p ≤ 0.01; *** = p < 0.001; N ≥ 5; two-tailed Student’s t-test. ONL = outer nuclear layer; GCL = ganglion cell layer.
Discussion
In contrast to mammals, the zebrafish retina readily regenerates photoreceptor cells that are lost following a phototoxic lesion. Several signaling pathways have been implicated in the restoration of lost neurons (Hochmann et al., 2012; Goldman, 2014; Lenkowski and Raymond, 2014; Lahne et al., 2020). A role for the immune system in regeneration of the adult zebrafish retina has previously been suggested (Zhao et al., 2014; Sifuentes et al., 2016; White et al., 2017; Mitchell et al., 2018; Silva et al., 2020; Zhang et al., 2020; Iribarne and Hyde, 2022), but it is not understood in detail. Here, we confirm and extend these previous studies on the role of inflammation, and its impact on Müller glia reactivity, by systemically modulating immune activity during regeneration of the adult zebrafish retina after a sterile phototoxic ablation of photoreceptor cells. We find that microglia and neutrophils infiltrate and accumulate at damaged sites of the retina. Importantly, Müller glia themselves react by activating the proinflammatory NF-κB signaling pathway in response to retinal damage. Our functional studies show that Dexamethasone-mediated immunosuppression reduces 1) leukocyte accumulation, and 2) the reactive Müller glia response at the proliferative and gene expression level, and thus 3) reduces the regeneration of photoreceptors. 4) Conversely, in gain-of-function assays, injection of flagellin, zymosan or M-CSF as inflammatory factors that are thought to facilitate the immune system, some Müller glia are stimulated and reactive proliferation is induced (Gorsuch and Hyde, 2014; Lenkowski et al., 2013; Nelson et al., 2013; Figure 8). Overall, we suggest that cells of the innate immune system act as necessary and sufficient positive regulators for successful regeneration of the adult zebrafish retina. Regulators of the inflammatory state, such as Dexamethasone and the M-CSF that stimulates Müller glia-based photoreceptor regeneration in our injection assays, might therefore provide advanced options for clinical treatments of retinal disease, as has also been suggested for corticosteroid management of early phases of spinal cord injury (Nelson et al., 2019).
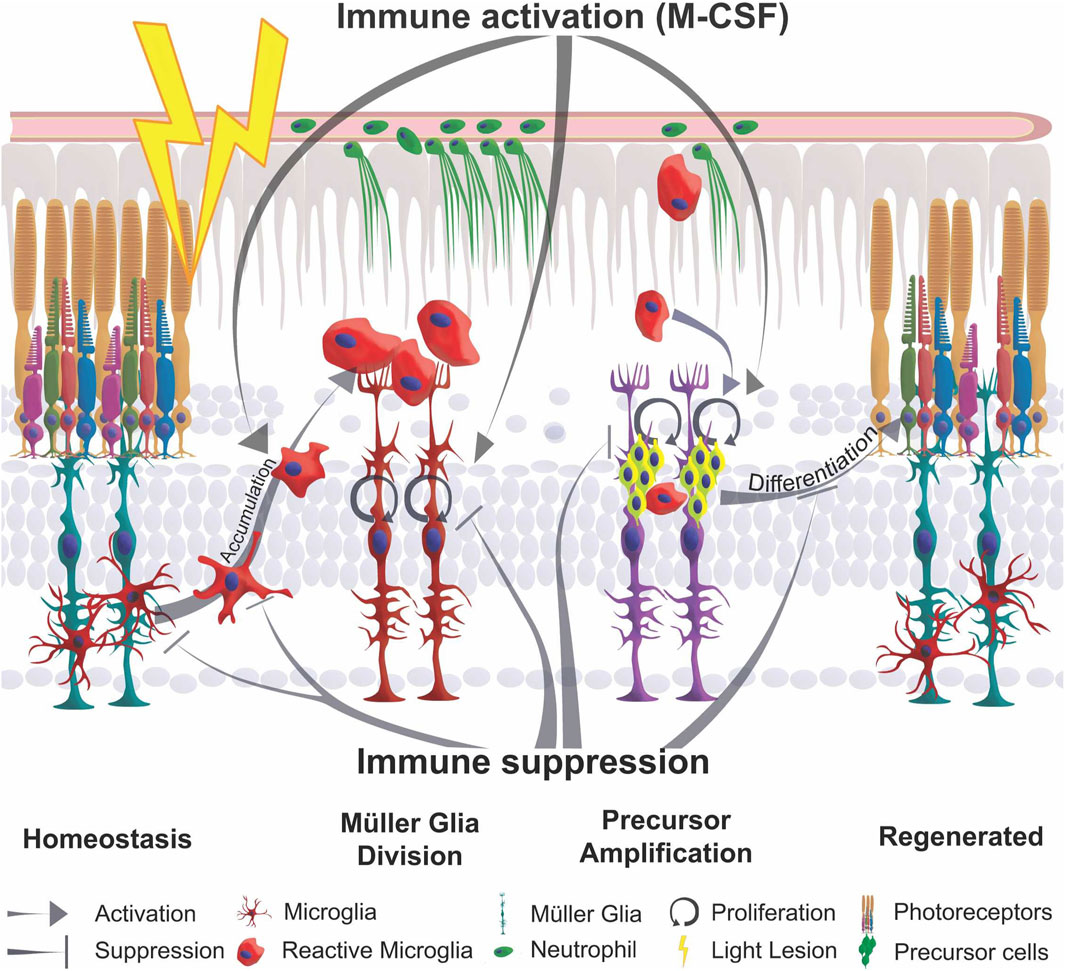
Figure 8. Schematic summary of the impact of inflammation in retinal regeneration. In response to injury, microglia undergo a phenotypic change from ramified to amoeboid shape and accumulate at the lesion site. This process is inhibited by immune suppression. The reactive proliferation of Müller glia during regeneration is also impaired upon immunosuppression. Leukocytes stimulate the proliferation of neuronal precursor cells. M-CSF as inflammatory mediator triggers Müller glia proliferation, leukocyte accumulation and the generation of neuronal precursor cells. This process may also act indirectly via the stimulation of immune cells. Overall, the innate immune system, via mediators such as M-CSF, supports the differentiation into photoreceptor cells and thereby acts as a regenerative cue for retina regeneration.
Sterile ablation of photoreceptors recruits innate immune cells
Consistent with previous studies, we find that retinal mpeg1:mCherry positive tissue-resident microglia display a ramified morphology in homeostasis, but change to an activated amoeboid cell shape upon a sterile light lesion, similar to what is observed after CNS lesion (Karlstetter et al., 2010; Kroehne et al., 2011; Kyritsis et al., 2012; Mitchell et al., 2018). Along with activation, we find a strong accumulation of microglia at the lesion site, which is resolved over a period of 14 days. The underlying dynamic events indicate an acute inflammatory response of leukocytes that is tightly controlled in a spatiotemporal manner. We assume that it is mostly tissue-resident microglia that mediate the observed immune response. Although we did not examine this further, these microglia population might be recruited from the less affected ventral and dorsal retinal territories (Weber et al., 2013), and from the rod outer segment layer/subretinal space (Supplementary Figure S1), where a subset of microglia appears to be resident (Martins et al., 2019; Song et al., 2020). Further, peripheral macrophages might also be recruited from the bloodstream by extravasation, and thus contribute to the observed reaction, similar to the neutrophils which we observed to enter the retina upon lesion. Consistent with our assumption, live imaging in larval zebrafish revealed that peripheral macrophages do not enter the developing retina during drug-mediated ablation of rod photoreceptors (Oosterhof et al., 2017; White et al., 2017). In contrast, neurotoxic ablation of the ganglion cells and neurons of the inner nuclear layer using ouabain triggers proliferation of adult retinal microglia, and it has been suggested that the observed increase in cell number might also involve peripheral macrophages entering the retina from circulation (Mitchell et al., 2018). The Tg(mpeg1:mCherry) reporter line we employed also has been reported to label a small subpopulation of B and/or Natural killer cells that therefore may additionally enter the retina upon lesion (Ferrero et al., 2020; Moyse and Richardson, 2020). The observation of mpo:GFP positive neutrophils in the vasculature, as well as neutrophil accumulation in the photoreceptor outer segment layer upon light lesion, indicates a certain permeabilization of the blood-retina-barrier, which may also allow additional leukocytes to invade the damaged retina (Sim et al., 2015; Eshaq et al., 2017; McMenamin et al., 2019). Further studies of the cell-permeability of the blood-retina-barrier during regeneration, e.g., by in vivo imaging approaches, and specific labeling of either peripheral macrophage or microglia populations, will likely be interesting in this regard.
Our observation that neutrophils arrive in the retina following non-invasive light lesion suggests an interesting aspect of retina regeneration, with parallels to observations after neurotoxic ablation of retinal ganglion cells (Mitchell et al., 2018). Neutrophils have different functions at the site of injury, and they are among the first cells to react to injury. It has been proposed that they participate in clearing cellular debris, by secretion of factors influencing angiogenesis and regeneration, but they may also support resolution of inflammation (Wang, 2018). In mice, neutrophils promote the regeneration of the optic nerve by expression of oncomodulin, and can influence the response of glial cells and axonal outgrowth after spinal cord injury (Stirling et al., 2009; Kurimoto et al., 2013; Tsarouchas et al., 2018; Andries et al., 2023). Furthermore, neutrophils are indicated to resolve inflammation by a localized O2 depletion (Campbell et al., 2014). Thus, neutrophils could be involved in the mediation of the immune response in adult retina regeneration in zebrafish upon lesion. Further investigation of the observed GFP positive matrices may therefore be interesting. We speculate that these structures might serve as “extracellular-traps” that could prevent cellular debris from spreading further into the surrounding tissues, protecting the latter from secondary cell death (Walker et al., 2007; Branzk and Papayannopoulos, 2013), which might help suppress a systemic inflammatory/regenerative reaction in the retina. The observation of GFP positive inclusions in mpeg1 positive macrophages furthermore suggests degradation of the trap-like structures by macrophage uptake (Farrera and Fadeel, 2013).
While microglia and neutrophils seem to play important roles in retina regeneration, we did not observe any infiltration of lck:NLS-dsRed positive T cells into the retina after lesion, arguing that T cells may be dispensable at least for the initial regeneration response. A recent report suggests that T cells can positively influence the regenerative response after an invasive mechanical lesion of the retina (Hui et al., 2017); this difference might be due to differences in the lesion paradigm employed, and/or timing of the regeneration events. As mentioned above, the non-invasive light lesion that we employed results in a specific ablation of photoreceptors without physically disrupting the blood-retina barrier. In contrast, the needle stab employed by Hui et al., 2017 disrupts the blood-retina barrier, thereby allowing T cells to access the lesion.
Müller glia react to photoreceptor cell death in an inflammatory manner
Upon retinal injury and progressive dystrophies, mammalian Müller glia undergo physiological changes and show transcriptional alterations as a hallmark of their gliotic response (Bringmann et al., 2009; Bringmann and Wiedemann, 2011). Despite the regenerative response of zebrafish Müller glia, they exhibit initial signs of reactive gliosis following retinal damage (Thomas et al., 2016). Here, we report that ablation of photoreceptors induces an acute inflammatory response at the molecular level. Importantly, we observed an inflammatory-like response directly in the lesion-responding Müller glia. Using NF-κB:GFP reporter fish (Kanther et al., 2011), we find that canonical NF-κB becomes activated in Müller glia upon damage, in keeping with transcriptomic changes of Müller glia upon light lesion (Sifuentes et al., 2016; Celotto et al., 2023). In the regenerating chick retina, NF-κB is active in Müller glia and acts as an important pathway controlled by factors that are secreted by microglia (Palazzo et al., 2020). A potential activator of NF-κB may be TNFα; indeed, several studies suggested that dying neurons secrete this factor and thus trigger Müller glia proliferation and the generation of NPCs (Kumar and Shamsuddin, 2012; Nelson et al., 2013).
Consistent with previous reports, we also found the NF-κB target gene, matrix metallopeptidase mmp9, to be expressed in reactive Müller glia, displaying a highly dynamic and strictly spatiotemporally controlled activation profile (Takada et al., 2004; Kim et al., 2007; Kaur et al., 2018; Silva et al., 2020). In mmp9 knockout zebrafish, Müller glia-derived progenitors show a hyperproliferative response after injury, but reduced survival of regenerated photoreceptors (Silva et al., 2020). The exact function of mmp9 is not completely clear yet; we speculate that mmp9, as a metalloproteinase, might be involved in the degradation of extracellular matrix (ECM), thus “opening” the retina for incoming immune or neuronal precursor cells, and/or modulating ECM interacting cytokines (Yoong et al., 2007; Gong et al., 2008; LeBert et al., 2015; Xu et al., 2018). Interestingly mmp9 is a key marker gene known to be differentially expressed between wet and dry forms of age-related macular degeneration, providing further evidence for a possibly critical role during inflammatory processes (Fritsche et al., 2016). Understanding the contribution and molecular control of mmp9 in zebrafish retina regeneration may therefore provide further insights into the mechanisms and molecules that are required to restore lost neural tissues.
Immunosuppression impairs the regenerative response of the zebrafish retina
Immunosuppression with the glucocorticoid dexamethasone drastically decreases the amount of retinal microglia and blocks accumulation of reactive leukocytes in the retina after lesion (Gallina et al., 2014; White et al., 2017). In addition, activation of NF-κB signaling was also successfully blocked in Müller glia, as shown by the loss of NF-κB:GFP and expression of its target gene. NF-κB activation has been described in a plethora of cellular functions such as cell survival, cell death and mediation of inflammation by the regulation of cytokine expression, as well as cell proliferation and migration (Liu et al., 2017). In Müller glia, NF-κB signaling could mediate an inflammatory-like response, consistent with their expression of pro- and anti-inflammatory cytokines (Karin and Lin, 2002; Nelson et al., 2013; Zhao et al., 2014). Consistently, following Dex-treatment, we find that the reactive proliferation of Müller glia was strongly reduced, indicating that inflammatory-like signaling promotes Müller glia cell cycle reentry. Interestingly, homeostatic proliferation, which mainly occurs in the ciliary marginal zone and only sporadically in the central retina, remains unaffected, similar to our findings in the zebrafish telencephalon (Kyritsis et al., 2012). Moreover, Dex-treatment is not only affecting reactive proliferation, as is also confirmed by other research groups (White et al., 2017; Silva et al., 2020; Iribarne and Hyde, 2022). Regenerated UV-cones show dysmorphic outer segments, indicating that Dex-treatment also impairs the proper differentiation of photoreceptor cells. On the other hand, in mmp9 mutants, initial Dex-treatment supports the restoration of the photoreceptors and thus can act positively (Silva et al., 2020). Collectively, we propose that inflammatory signaling is required for cell cycle reentry of Müller glia during regeneration, but might also play additional roles during maturation of regenerated photoreceptors (Figure 8). An incomplete regenerative outcome was also detected in larval retinal pigment epithelium (RPE) regeneration in irf8-mutants deficient in microglia. Upon ablation of RPE, the neural retina showed an increased reactive proliferation in mutants, but the amount of regenerated RPE cells is lower than in wild type siblings (Hanovice et al., 2019).
To further understand the impact of resident microglia on the regeneration process in adult retina, we addressed the proliferative potential of retinae in response to injury in irf8 mutant fish, as a genetic model for myeloid deficiency in larval and juvenile zebrafish (Shiau et al., 2015). We confirm microglia deficiency at adult stages (>6 months), indicating that irf8 is required throughout lifetime in primitive as well as a definitive wave of monocyte populations of the eye (Shiau et al., 2015; Ferrero et al., 2018). However, a reduced number of leukocytes is still found to populate the adult retina. These could be tissue-specific macrophages that are irf8-independent, as previously described for the gut and retina (Earley et al., 2018; Fogerty et al., 2022). Upon lesion, we observed that the number of cells that are in cell cycle is significantly reduced in irf8 mutants in comparison to heterozygous siblings in response to lesion. Interestingly, the number of L-Plastin + leukocytes in irf8 mutants increases upon injury, but never reaches levels comparable to heterozygous control siblings. In line with other reports, these findings indicate that microglia are supportive for Müller cell proliferation and NPC amplification and thereby support initial steps of regeneration (Conedera et al., 2019; Zhang et al., 2020; Iribarne and Hyde, 2022). The necessity of (tissue-resident) macrophages is also observed in other tissues, e.g., telencephalon, spinal cord, caudal fin and heart, showing the contribution of the innate immune system to tissue regeneration (Kyritsis et al., 2012; Petrie et al., 2015; De Preux Charles et al., 2016; Portillo et al., 2017; Roche et al., 2018; Tsarouchas et al., 2018). This supportive role of macrophages in zebrafish is unlike that of the rodent retina, where microglia appear to inhibit Müller-glia-mediated tissue regeneration by suppression of ascl1 expression. This interesting species difference might be explained by distinct expression profiles of zebrafish vs mouse microglia during the time course of regeneration (Mitchell et al., 2019; Issaka Salia and Mitchell, 2020; Todd et al., 2020).
Immune stimulation triggers Müller glia reactivity and proliferation
Retinal pathology is often coupled to neuronal loss, activation of the immune system and Müller glia reactivity (Iribarne and Hyde, 2022). In particular, mammalian Müller glia react to injury mostly by (proliferative) gliosis, which contributes to glial scarring, thus enhancing loss of vision (Bringmann et al., 2006). In zebrafish, retinal injury also results in an acute immune reaction; however, in contrast to mammals, this is followed by a regenerative response.
Importantly, inflammatory signals can indeed initiate reactive proliferation, even in the absence of injury, as seen by our vitreous injections of toll-like receptor (TLR) agonists zymosan and flagellin to trigger an inflammatory response (Akira and Takeda, 2004; Barber et al., 2016; Zhang et al., 2020). Leukocyte number was not increased following these injections, which might be explained by three possible scenarios: 1) Zymosan and flagellin do not stimulate leukocyte proliferation 2) recruitment from the bloodstream is not induced; while in both scenarios a local leukocyte activation can occur. 3) The accumulation could already be resolved within 48 h, since the response of immune cells is rapid and highly dynamic. Furthermore, the concentration of zymosan injected in a rodent and zebrafish model, in which a strong accumulation of leukocytes is observed, is much higher than the concentration used in our injections and may reflect a dose-dependency (Kurimoto et al., 2013; Van Dyck et al., 2021).
In contrast to the more generic inflammatory stimuli, M-CSF-injected retinae show an increased number of leukocytes. This may be the consequence of both proliferation and recruitment from the bloodstream, since M-CSF receptor (CSF-1R) is thought to regulate the population of microglia in neural tissue during development (Wu et al., 2018). Following M-CSF injection, we find that Müller glia activate NF-κB signaling, which fits well with studies reporting TLR and CSF-1 receptor expression in Müller glia and microglia (Bsibsi et al., 2002; Olson and Miller, 2004; Kumar and Shamsuddin, 2012; Oosterhof et al., 2017; Rocío Nieto-Arellano and Sánchez-Iranzo, 2019). Most importantly, we found that in the absence of additional injury, stimulation of the immune system activates the regenerative response program of Müller glia, as shown by upregulation of mmp9 and her4.1, as well as an increase in proliferation; consistently, expression of her4.1 also indicates the generation of NPCs from Müller glia. The expression of the CSF-1 receptor in neural progenitor cells is also associated with proliferation, survival and differentiation, supporting the theory that M-CSF stimulates a proliferative/regenerative response in Müller glia (Chitu et al., 2016). Of note, also in csf1ra/b mutants an abnormal proliferation of Müller cells was described (van Dyck et al., 2021). Even in the absence of microglia, Müller cells tend to proliferate in the native retina, which is not described for systems lacking microglia in the adult retina (Conedera et al., 2019; Forgerty et al., 2022). This is suggesting that indeed CSF receptor signaling might influence Müller cell behavior directly. Nevertheless, whether Müller glia reactivity is a direct consequence of CSF/TLR-agonist treatment, or an indirect consequence of potentially altered microglia signaling, is so far not clear, because receptors for CSF/TLR signaling appear to be expressed in both cell populations (Letiembre et al., 2007; Kochan et al., 2012; Kumar and Shamsuddin, 2012; Lin et al., 2013; Tsarouchas et al., 2018; Wu et al., 2018; Mitchell et al., 2019). Another possible trigger of the Müller cell response to M-CSF injection could be that this factor stimulates microglia that in turn become reactive and secrete factors and/or could harm retinal neurons and thus stimulate a secondary Müller cell response that we did not address in our experimental design. Hence, it will be interesting to test whether CSF-1R or TLR agonists alone are sufficient to drive increased Müller glia proliferation, using a model that completely lacks microglial cells in the retina. Moreover, it will be interesting to determine if the observed effects of the CSF1R/TLR-agonists are mediated via NF-κB signaling, or via other pathways, such as the MAP kinase pathway, or combinations thereof (Wan et al., 2012; Kawasaki and Kawai, 2014; Chen et al., 2018).
Conclusion
Our work strongly supports the notion that acute inflammatory signaling provides a beneficial contribution during adult zebrafish retina regeneration, akin to findings in the telencephalon (Kyritsis et al., 2012; Kizil et al., 2015). Reactivity of retinal leukocytes appears to be tightly controlled after injury, and the presence of leukocytes positively influences reactive proliferation of Müller glial cells, as well as the downstream differentiation of Müller glia progeny into mature photoreceptor cells. Importantly, immune stimulation through TLRs, or via M-CSF injection, was sufficient, in the absence of injury caused by these factors, to trigger Müller glia proliferation and NPC formation. Hence, we conclude that inflammatory signaling plays a critical role during zebrafish retina regeneration.
Further investigations on possible interactions between leukocytes and Müller glia will help to understand the balance between the inflammatory reaction and its resolution during regeneration of neural tissue in zebrafish. In addition, it will be important to identify critical factors secreted by leukocytes in an acute reaction to lesion that are -directly or indirectly-involved in triggering Müller glia reactivity, and thus, regeneration. Finally, this study provides a framework for investigating potentially beneficial effects of an acute immune response, also in comparison with failed regeneration and chronic inflammatory outcome in mammalian models.
Materials and methods
Zebrafish maintenance
Zebrafish (Danio rerio) were kept under standard housing conditions as previously described (Brand et al., 2002). All fish used in this study were adults, both males and females, and 6–12 months of age. Transgenic fish used are: Tg(−5.5opn1sw1:EGFP)kj9 (Takechi et al., 2003); Tg(mpeg1:mCherry) (Ellett et al., 2011); Tg(mpo:GFP) (Renshaw et al., 2016); Tg(gfap:NLS-GFP) (described here); Tg(gfap:NLS-mCherry) (Lange et al., 2020), Tg(lck:NLS-mCherry)sd31 (Butko et al., 2015), Tg(NF-kB:GFP) (Kanther et al., 2011), Δirf8st95 (Shiau et al., 2015).
Generation of Tg(gfap:nls-GFP)
The Tg(gfap:NLS-GFP) reporter line with its NLS-tag shows highly nuclear localization of GFP, but also some leaky cytoplasmic expression in Müller glial cells, and thus serves as an ideal marker for MGCs in our experiments. To generate this line, the GFP reporter was PCR amplified and flanked by restriction sites using GFP-for (atatGGCCGGCCgccaccatggctccaaagaagaagcgtaaggt) and GFP-rev (ggtgtgcatgttttgacgttgatggc) primers. By PCR, the nuclear localization sequence (NLS) was added as a 5′ overhang to the GFP. The PCR product was subcloned into the p2.1 Topo Vector. Next, Topo vector with the reporters and the pTol(gfap:mcherry-T2A-CreERT2) construct (SH and MB, unpublished) were digested using the enzymes Asc and FseI and ligated to replace the mCherry-CreERT2 cassette with the NLS-reporter. For germline transformation, either linearized plasmid DNA or plasmid DNA with transposase mRNA were injected into fertilized eggs (F0) in E3 medium (Brand et al., 2002), raised to adulthood and crossed to AB wild-type fish as previously described (Kawakami et al., 2004).
Diffuse light lesion
To ablate photoreceptor cells, diffuse light lesion was performed as described in Weber et al., 2013. Briefly, dark-adapted fish were transferred to a beaker containing 250 mL system water and exposed to the light of an EXFO X-Cite 120 W metal halide lamp (−200,000 lux) for 30′. Fish were connected to the system and kept under standard light and housing conditions until sacrificed. For control, untreated non-dark adapted fish were used.
Drug treatment
Dexamethasone (Dex, Sigma-Aldrich, Germany) was diluted from a 25 mg/mL stock solution in methanol to 15 mg/L in autoclaved system water. For the treatment, adult fish were kept individually in 250 mL solution in sterilized tanks with lits. Control groups were treated with the corresponding amount of methanol (MeOH) only, Both groups did not show retinal cell death in the absence of lesion (Supplementary Figure S3). Solutions were renewed daily, and tanks were exchanged every 5 days. Fish were fed with brine shrimp 1 h prior to solution exchange. Animals were pretreated for 10 days and respective conditions were maintained until sacrificed. Light lesions were performed in corresponding solutions.
Tissue preparation and sectioning
For retinal flat mounts, eyes were removed and a small incision in the cornea was made using a sapphire blade scalpel (ZT215 W0.5 mm A60°). Eyes were prefixed in 4% PFA in calcium-free ringer solution (PFAR) for 30′ with slow agitation. Cornea, sclera and the lens were removed in calcium-free ringer solution and the retinae were cut at four sides. Flat mounts were fixed in 4% PFAR at 4°C overnight with gentle agitation. For storage, samples were transferred to 100% methanol and stored at −20°C.
For sections, the lens was removed and fish heads were fixed at 4°C in 4% PFA in 0.1 M phosphate buffer (PB). Following that, samples were decalcified and cryo-protected with 20% sucrose/20% EDTA in 0.1 M PB. Tissues were embedded in 7.5% gelatine/20% sucrose in 0.1% PB, stored at −80°C and sectioned into 14 µm cryo-sections using a Microm HM560. Sections were stored at −20°C.
In situ hybridization
In situ hybridization on sections was performed as described in Ganz et al., 2015. Hybridization was carried out overnight at 62°C. Probes were generated using mmp9 (primer forward: CTTGGAGTCCTGGCGTTTCT; primer reverse: GCCCGTCCTTGAAGAAGTGA) and her4.1 (Takke et al., 1999) as targets. For detection, NBT/BCIP or SIGMAFAST™ Fast Red TR/Naphtanol AS-MX was used. For combination with immunohistochemistry, the primary antibody was incubated with anti-digoxigenin-AP. Secondary antibody was applied after staining development for 2 h and washed with PBS with 0.3% Triton-X100 (PBSTx). Sections were mounted in glycerol.
Immunohistochemistry & TUNEL-staining
Sections were dried for 2 h at 50°C and rehydrated with PBSTx. Following this, sections were incubated with primary antibody: anti-Zrf-1 ZIRC mouse IgG1 1:200 (Fausett and Goldman, 2006), anti-Zpr-3 ZIRC IgG1 1:200 (Zou et al., 2008), anti-GFP Abcam chicken 1:3000 (Leinninger et al., 2009), anti-DsRed Clontech rabbit 1:500 (Glass et al., 2005), anti-PCNA mouse IgG2a DACO PC10 1:500 (Grandel et al., 2006), anti-L-Plastin rabbit 1:5000 (Redd et al., 2006), and incubated overnight at 4°C. Excess antibody was washed off using PBSTx followed by incubation with secondary antibody against the respective primary antibody host (Molecular Probes; Alexa 488, Alexa 555, Alexa 635; 1:750) containing 1 μg/mL 4′,6-Diamidin-2-phenylindol (DAPI). Antibody-solution was washed off and slides were mounted with glycerol.
To retrieve the PCNA antigen, sections were incubated for 8’ in 50 mM Tris buffer pH 8.0 at 99°C followed by 10 min PBS prior to primary antibody incubation.
Modifications for retinal flat mounts are prolonged incubation times for primary and secondary antibody solution for 48 h respectively at 4°C with slow agitation and extensive washing 10 × 30′ after each antibody incubation. Flat mounts were mounted on slides in glycerol.
TUNEL (TdT-mediated dUTP-biotin nick end labeling) assays were performed on sections using ApopTag® Red in Situ Apoptosis Detection Kit (Merk) according to manufacturer’s instructions.
EdU labeling and detection
To trace proliferating cells, EdU (5-ethynyl-2′-deoxyuridine) pulses were given intraperitoneally. Fish were anesthetized in 0.024% Tricaine and EdU was injected intraperitoneally (20 µL of 2.5 mg/mL EdU in PBS per pulse). Detection was performed on cryosections using “Click-iT® Plus EdU Alexa Fluor® 555 Imaging Kit” (Thermo Fischer Scientific) according to manufacturer’s instructions.
Intravitreal injections
Fish were anesthetized in 0.024% tricaine and covered with tricaine-moistened paper towel, leaving the eye exposed. The outer cornea was removed. Subsequently, a small incision in the cornea was made using a sapphire blade scalpel (ZT215 W0.5 mm A60°). A Hamilton syringe equipped with a 33 gauge, blunt-end needle was inserted and 0.5 µL of solution (flagellin from S. typhimurium, 0.1 μg/μL, Sigma; zymosan from S. cerevisiae, 0.2 μg/μL BioParticles; Human M-CSF, 50 ng/μL, PreproTech; PBS) was injected into the vitreous of the eye without touching the retina. The incision was covered with Histoacryl® (Braun) to avoid leakage.
Image acquisition
Images were acquired using a Zeiss Axio Imager, equipped with ApoTome. EC Plan-Neofluar 5x/0.16, Plan-Apochromat 20x/0.8 and LD C-Apochromat 40x/1.1 W Korr UV VIS IR objectives were used for magnification. For detection either a monochromatic Axiocam HR (1388 × 1040 pixels, 6.45*6.45) for fluorescence or a polychromatic Axiocam MR Rev3 (1388 × 1040 pixels, 6.45*6.45) for bright-field imaging was used. Sequential image acquisition was used in co-stained samples with multiple fluorophores. Images were acquired in AxioVision Rel. 4.8 and processed using Fiji (Schindelin et al., 2012). Figure panels were assembled in Adobe Photoshop CS6 and CorelDraw 2020.
Cell counting and statistical analysis
For cell quantification, retinal sections with a thickness of 14 µm were used. For single fluorophore cell quantification, the first 3-5 consecutive retinal sections rostral to the optic nerve were selected and manually counted. For normalization, the length of the retina was determined for every section by measuring a snapshot of the retina at the level of the inner nuclear layer. For quantification of multiple fluorophores, 20x images were acquired. Cells were manually quantified on images displayed in Fiji. Length measurements were applied on the same images for normalization. For statistical analyses, ≥3 fish were used for calculations. Counted cell numbers were normalized to the respective parameter (reference cell population or length) and the counts per fish were averaged (N = 1 fish ≙ n = 2 retinae). The average number for every fish was used as an analytical unit. For statistical analysis, GraphPad Prism 10.0.3 was used to determine p-values with a one-way ANOVA test with Tukey’s post hoc analysis or two-tailed t-Test. Significance levels are displayed as: not significant (ns) = p > 0.05, * = p ≤ 0.5, ** = p ≤ 0.01, *** = p ≤ 0.001. Graphs shown in bar charts with single data points were created using in GraphPad Prison 10.0.3, error bars represent standard error of the mean (SEM). For small sample sizes (N = 3-5 fish), e.g., in Figure 6B (TUNEL staining in Flagellin and Zymosan injected eyes) we conducted an additional power analysis using GPower3.1 to determine if sample size is of sufficient experimental power given our sample size. The additional quantification of pyknotic nuclei in Supplementary Figure S7 was conducted on optical sections of our available image data from one experimental run.
Data availability statement
The original contributions presented in the study are included in the article/Supplementary Material, further inquiries can be directed to the corresponding author.
Ethics statement
The animal experiments were performed in strict accordance with the European Union and German law (Tierschutzgesetz). Experimental procedures were approved by the animal ethics committee of the TU Dresden and the Landesdirektion Sachsen (permits AZ: 24-9168.11-1/2013-5; AZ: TV A 1/2017; TVV 21/2018; AZ: TVV 55/2018).
Author contributions
OB: Investigation, Visualization, Writing–original draft, Writing–review and editing. AW: Investigation, Writing–review and editing. VB: Investigation, Writing–review and editing. VK: Investigation, Writing–review and editing. KD: Investigation, Writing–review and editing. SH: Investigation, Writing–review and editing. MB: Conceptualization, Funding acquisition, Investigation, Methodology, Project administration, Resources, Supervision, Validation, Visualization, Writing–original draft, Writing–review and editing.
Funding
The author(s) declare that financial support was received for the research, authorship, and/or publication of this article. This work was supported by project grants of the German Research Foundation (DFG, project numbers BR 1746/3, BR 1746/11-1) and an ERC advanced grant (Zf-BrainReg) to MB.
Acknowledgments
We are very grateful to Marika Fischer, Jitka Michling, Daniela Mögel, and Claudia Meyer who provided outstanding fish care, and Dr. Judith Konantz for managing the fish facility. We also thank Michaela Geffarth and Nathalie Franke for excellent technical support and John F Rawls for sharing the Tg(NF-kB:GFP) reporter line. Further, we thank past and present members of the Brand lab for many interesting discussions, and Thomas Quail, Judith Konantz, Christian Lange, Thomas Becker, Catherina Becker, Marius Ader, Ecem Çayıroğlu and Susanne Koch for comments on earlier versions of this manuscript. We also gratefully acknowledge support of OB by a pre-doctoral fellowship of the ProRetina foundation.
Conflict of interest
The authors declare that the research was conducted in the absence of any commercial or financial relationships that could be construed as a potential conflict of interest.
Publisher’s note
All claims expressed in this article are solely those of the authors and do not necessarily represent those of their affiliated organizations, or those of the publisher, the editors and the reviewers. Any product that may be evaluated in this article, or claim that may be made by its manufacturer, is not guaranteed or endorsed by the publisher.
Supplementary material
The Supplementary Material for this article can be found online at: https://www.frontiersin.org/articles/10.3389/fcell.2024.1332347/full#supplementary-material
References
Akhtar-Schäfer, I., Wang, L., Krohne, T. U., Xu, H., and Langmann, T. (2018). Modulation of three key innate immune pathways for the most common retinal degenerative diseases. EMBO Mol. Med. 10 (10), e8259. doi:10.15252/emmm.201708259
Akira, S., and Takeda, K. (2004). Toll-like receptor signalling. Nat. Rev. Immunol. 4 (7), 499–511. doi:10.1038/nri1391
Amor, S., Puentes, F., Baker, D., and Van Der Valk, P. (2010). Inflammation in neurodegenerative diseases. Immunology 129 (2), 154–169. doi:10.1111/j.1365-2567.2009.03225.x
Andries, L., Kancheva, D., Masin, L., Scheyltjens, I., Van Hove, H., De Vlaminck, K., et al. (2023). Immune stimulation recruits a subset of pro-regenerative macrophages to the retina that promotes axonal regrowth of injured neurons. acta neuropathol. Commun. 11, 85. doi:10.1186/s40478-023-01580-3
Aslanidis, A., Karlstetter, M., Scholz, R., Fauser, S., Neumann, H., Fried, C., et al. (2015). Activated microglia/macrophage whey acidic protein (AMWAP) inhibits NFΚB signaling and induces a neuroprotective phenotype in microglia. J. Neuroinflammation 12 (1), 77–14. doi:10.1186/s12974-015-0296-6
Aurora, A. B., and Olson, E. N. (2014). Immune modulation of stem cells and regeneration. Cell Stem Cell 15 (1), 14–25. doi:10.1016/j.stem.2014.06.009
Barber, A. E., Fleming, B. A., and Mulvey, M. A. (2016). Similarly lethal strains of extraintestinal pathogenic Escherichia coli trigger markedly diverse host responses in a zebrafish model of sepsis. MSphere 1 (2), 000622–e119. doi:10.1128/msphere.00062-16
Bernardos, R. L., Barthel, L. K., Meyers, J. R., and Raymond, P. A. (2007). Late-stage neuronal progenitors in the retina are radial Müller glia that function as retinal stem cells. J. Neurosci. official J. Soc. Neurosci. 27 (26), 7028–7040. doi:10.1523/JNEUROSCI.1624-07.2007
Bollaerts, I., Van Houcke, J., Beckers, A., Lemmens, K., Vanhunsel, S., De Groef, L., et al. (2019). Prior exposure to immunosuppressors sensitizes retinal microglia and accelerates optic nerve regeneration in zebrafish. Mediat. Inflamm. 2019, 6135795. doi:10.1155/2019/6135795
Bosak, V., Murata, K., Bludau, O., and Brand, M. (2018). Role of the immune response in initiating central nervous system regeneration in vertebrates: learning from the fish. Int. J. Dev. Biol. 417, 403–417. doi:10.1387/ijdb.180033vb
Brand, M., Granato, M., and Nüsslein-Volhard, C. (2002). “Keeping and raising zebrafish,” in Zebrafish: a practical approach. Editors C. Nüsslein-Volhard, and R. Dahm (Oxford: Oxford University Press), 7–38. doi:10.1093/oso/9780199638086.003.0002
Branzk, N., and Papayannopoulos, V. (2013). Molecular mechanisms regulating NETosis in infection and disease. Seminars Immunopathol. 35 (4), 513–530. doi:10.1007/s00281-013-0384-6
Bringmann, A., Pannicke, T., Biedermann, B., Francke, M., Iandiev, I., Grosche, J., et al. (2009). Role of retinal glial cells in neurotransmitter uptake and metabolism. Neurochem. Int. 54 (3–4), 143–160. doi:10.1016/J.NEUINT.2008.10.014
Bringmann, A., Pannike, T., Grosche, J., Francke, M., Wiedemann, P., Skatchkov, S., et al. (2006). Müller cells in the healthy and diseased retina. Prog. Retin. Eye Res. 25 (4), 397–424. doi:10.1016/j.preteyeres.2006.05.003
Bringmann, A., and Wiedemann, P. (2011). Müller glial cells in retinal disease. Ophthalmologica 227 (1), 1–19. doi:10.1159/000328979
Bsibsi, M., Ravid, R., Gveric, D., and Van Noort, J. M. (2002). Broad expression of Toll-like receptors in the human central nervous system. J. Neuropathology Exp. Neurology 61 (11), 1013–1021. doi:10.1093/jnen/61.11.1013
Buffo, A., Rite, I., Tripathi, P., Lepier, A., Colak, D., Horn, A.-P., et al. (2008). Origin and progeny of reactive gliosis: a source of multipotent cells in the injured brain. Proc. Natl. Acad. Sci. 105 (9), 3581–3586. doi:10.1073/pnas.0709002105
Butko, E., Distel, M., Pouget, C., Weijts, B., Kobayashi, I., Ng, K., et al. (2015). Gata2b is a restricted early regulator of hemogenic endothelium in the zebrafish embryo. Development 142 (6), 1050–1061. doi:10.1242/dev.119180
Campbell, E. L., Bruyninckx, W. J., Kelly, C. J., Glover, L. E., McNamee, E. N., Bowers, B. E., et al. (2014). Transmigrating neutrophils shape the mucosal microenvironment through localized oxygen depletion to influence resolution of inflammation. Immunity 40 (1), 66–77. doi:10.1016/j.immuni.2013.11.020
Celotto, L., Rost, F., Machate, A., Bläsche, J., Dahl, A., Weber, A., et al. (2023). Single cell RNA sequencing unravels the transcriptional network underlying zebrafish retina regeneration. eLife 12, RP86507. doi:10.7554/eLife.86507
Chen, L., Deng, H., Cui, H., Fang, J., Zuo, Z., Deng, J., et al. (2018). Inflammatory responses and inflammation-associated diseases in organs. Oncotarget 9 (6), 7204–7218. doi:10.18632/oncotarget.23208
Cheng, C. Y., Hsieh, H. L., Hsiao, L. D., and Yang, C. M. (2012). PI3-K/Akt/JNK/NF-κB is essential for MMP-9 expression and outgrowth in human limbal epithelial cells on intact amniotic membrane. Stem Cell Res. 9 (1), 9–23. doi:10.1016/j.scr.2012.02.005
Chitu, V., Gokhan, Ş., Nandi, S., Mehler, M. F., and Stanley, E. R. (2016). Emerging roles for CSF-1 receptor and its ligands in the nervous system. Trends Neurosci. 39 (6), 378–393. doi:10.1016/J.TINS.2016.03.005
Conedera, F. M., Pousa, A. M. Q., Mercader, N., Tschopp, M., and Enzmann, V. (2019). Retinal microglia signaling affects Müller cell behavior in the zebrafish following laser injury induction. Glia 6 (November 2018), 1150–1166. doi:10.1002/glia.23601
Conner, C., Ackerman, K. M., Lahne, M., Hobgood, J. S., and Hyde, D. R. (2014). Repressing Notch signaling and expressing TNFα are sufficient to mimic retinal regeneration by inducing muller glial proliferation to generate committed progenitor cells. J. Neurosci. 34 (43), 14403–14419. doi:10.1523/JNEUROSCI.0498-14.2014
Coutinho, A. E., and Chapman, K. E. (2011). The anti-inflammatory and immunosuppressive effects of glucocorticoids, recent developments and mechanistic insights. Mol. Cell. Endocrinol. 335 (1), 2–13. doi:10.1016/j.mce.2010.04.005
De Preux Charles, A. S., Bise, T., Baier, F., Marro, J., and Jaźwińska, A. (2016). Distinct effects of inflammation on preconditioning and regeneration of the adult zebrafish heart. Open Biol. 6 (7), 160102. doi:10.1098/rsob.160102
Earley, A. M., Graves, C. L., and Shiau, C. E. (2018). Critical role for a subset of intestinal macrophages in shaping gut microbiota in adult zebrafish. Cell Rep. 25 (2), 424–436. doi:10.1016/j.celrep.2018.09.025
Ellett, F., Pase, L., Hayman, J. W., Andrianopoulos, A., and Lieschke, G. J. (2011). Phagocytes, Granulocytes, and Myelopoiesis mpeg1 promoter transgenes direct macrophage-lineage expression in zebrafish. Blood, 27(4), e49–e56. doi:10.1182/blood-2010-10-314120
Eshaq, R. S., Aldalati, A. M. Z., Alexander, J. S., and Harris, N. R. (2017). Diabetic retinopathy: breaking the barrier. Pathophysiology 24 (4), 229–241. doi:10.1016/J.PATHOPHYS.2017.07.001
Farrera, C., and Fadeel, B. (2013). Macrophage clearance of neutrophil extracellular traps is a silent process. J. Immunol. 191 (5), 2647–2656. doi:10.4049/jimmunol.1300436
Fausett, B. V., and Goldman, D. (2006). A role for alpha1 tubulin-expressing Müller glia in regeneration of the injured zebrafish retina. J. Neurosci. 26 (23), 6303–6313. doi:10.1523/jneurosci.0332-06.2006
Ferrero, G., Gomez, E., lyer, S., Rovira, M., Misweocchi, M., Langenau, D. M., et al. (2020). The macrophage-expressed gene (mpeg) 1 identifies a subpopulation of B cells in the adult zebrafish. J. Leukoc. Biol. 107, 431–443. doi:10.1002/JLB.1A1119-223R
Ferrero, G., Mahony, C. B., Dupuis, E., Yvernogeau, L., Di Ruggiero, E., Miserocchi, M., et al. (2018). Embryonic microglia derive from primitive macrophages and are replaced by cmyb-dependent definitive microglia in zebrafish. Cell Rep. 24 (1), 130–141. doi:10.1016/j.celrep.2018.05.066
Ferrero-Miliani, L., Nielsen, O. H., Andersen, P. S., and Girardin, S. E. (2007). Chronic inflammation: importance of NOD2 and NALP3 in interleukin-1beta generation. Clin. Exp. Immunol. 147 (2), 227–235. doi:10.1111/j.1365-2249.2006.03261.x
Fimbel, S. M., Montgomery, J. E., Burket, C. T., and Hyde, D. R. (2007). Regeneration of inner retinal neurons after intravitreal injection of ouabain in zebrafish. J. Neurosci. official J. Soc. Neurosci. 27 (7), 1712–1724. doi:10.1523/JNEUROSCI.5317-06.2007
Fitch, M. T., and Silver, J. (2008). CNS injury, glial scars, and inflammation: inhibitory extracellular matrices and regeneration failure. Exp. Neurol. 209 (2), 294–301. doi:10.1016/j.expneurol.2007.05.014
Fogerty, J., Song, P., Boyd, P., Grabinski, S. E., Hoang, T., Reich, A., et al. (2022). Notch inhibition promotes regeneration and immunosuppression supports cone survival in a zebrafish model of inherited retinal dystrophy. J. Neurosci. official J. Soc. Neurosci. 42 (26), 5144–5158. doi:10.1523/JNEUROSCI.0244-22.2022
Fritsche, L. G., Igl, W., Bailey, J. N. C., Grassmann, F., Sengupta, S., Bragg-Gresham, J. L., et al. (2016). A large genome-wide association study of age-related macular degeneration highlights contributions of rare and common variants. Nat. Genet. 48 (2), 134–143. doi:10.1038/ng.3448
Gallina, D., Zelinka, C., and Fischer, A. J. (2014). Glucocorticoid receptors in the retina, Muller glia and the formation of Muller glia-derived progenitors. Development 141 (17), 3340–3351. doi:10.1242/dev.109835
Gallina, D., Zelinka, C. P., Cebulla, C. M., and Fischer, A. J. (2015). Activation of glucocorticoid receptors in Müller glia is protective to retinal neurons and suppresses microglial reactivity. Exp. Neurol. 273, 114–125. doi:10.1016/j.expneurol.2015.08.007
Ganz, J., Kroehne, V., Freudenreich, D., Machate, A., Geffarth, M., Braasch, I., et al. (2015). Subdivisions of the adult zebrafish pallium based on molecular marker analysis. F1000Research 3 (3), 308. doi:10.12688/f1000research.5595.2
Glass, R., Synowitz, M., Kronenberg, G., Walzlein, J., Markovic, D., Wang, L., et al. (2005). Glioblastoma-induced attraction of endogenous neural precursor cells is associated with improved survival. J. Neurosci. 25 (10), 2637–2646. doi:10.1523/jneurosci.5118-04.2005
Goldman, D. (2014). Müller glial cell reprogramming and retina regeneration. Nat. Rev. Neurosci. 15 (7), 431–442. doi:10.1038/nrn3723
Gong, Y., Hart, E., Shchurin, A., and Hoover-Plow, J. (2008). Inflammatory macrophage migration requires MMP-9 activation by plasminogen in mice. J. Clin. Investigation 118 (9), 3012–3024. doi:10.1172/JCI32750
Gorsuch, R. A., and Hyde, D. R. (2014). Regulation of Müller glial dependent neuronal regeneration in the damaged adult zebrafish retina. Exp. Eye Res. 123, 131–140. doi:10.1016/j.exer.2013.07.012
Grandel, H., Kaslin, J., Ganz, J., Wenzel, I., and Brand, M. (2006). Neural stem cells and neurogenesis in the adult zebrafish brain: origin, proliferation dynamics, migration and cell fate. Dev. Biol. 295 (1), 263–277. doi:10.1016/j.ydbio.2006.03.040
Hammer, J., Röppenack, P., Yousuf, S., Schnabel, C., Weber, A., Zöller, D., et al. (2022). Visual function is gradually restored during retina regeneration in adult zebrafish. Front. Cell Dev. Biol. 9 (February), 831322–831418. doi:10.3389/fcell.2021.831322
Hanovice, N. J., Leach, L. L., Slater, K., Gabriel, A. E., Romanovicz, D., Shao, E., et al. (2019). Regeneration of the zebrafish retinal pigment epithelium after widespread genetic ablation. PLoS Genet. 15 (Issue 1), e1007939. doi:10.1371/journal.pgen.1007939
Herrero, M.-T., Estrada, C., Maatouk, L., and Vyas, S. (2015). Inflammation in Parkinsons disease: role of glucocorticoids. Front. Neuroanat. 9 (April), 32–12. doi:10.3389/fnana.2015.00032
Hochmann, S., Kaslin, J., Hans, S., Weber, A., Machate, A., Geffarth, M., et al. (2012). Fgf signaling is required for photoreceptor maintenance in the adult zebrafish retina. PLoS One 7 (1), e30365. doi:10.1371/journal.pone.0030365
Hui, S. P., Sheng, D. Z., Sugimoto, K., Gonzalez-Rajal, A., Nakagawa, S., Hesselson, D., et al. (2017). Zebrafish regulatory T cells mediate organ-specific regenerative programs. Dev. Cell 43 (6), 659–672. doi:10.1016/j.devcel.2017.11.010
Iribarne, M., and Hyde, D. R. (2022). Different inflammation responses modulate Müller glia proliferation in the acute or chronically damaged zebrafish retina. Front. Cell Dev. Biol. 10 (August), 892271–892319. doi:10.3389/fcell.2022.892271
Issaka Salia, O., and Mitchell, D. M. (2020). Bioinformatic analysis and functional predictions of selected regeneration-associated transcripts expressed by zebrafish microglia. BMC Genomics 21 (1), 870–917. doi:10.1186/s12864-020-07273-8
Kanther, M., Sun, X., Mühlbauer, M., MacKey, L. C., Flynn, E. J., Bagnat, M., et al. (2011). Microbial colonization induces dynamic temporal and spatial patterns of NF-κB activation in the zebrafish digestive tract. Gastroenterology 141 (1), 197–207. doi:10.1053/j.gastro.2011.03.042
Karlstetter, M., Ebert, S., and Langmann, T. (2010). Microglia in the healthy and degenerating retina: insights from novel mouse models. Immunobiology 215 (9–10), 685–691. doi:10.1016/j.imbio.2010.05.010
Karin, M., and Lin, A. (2002). NF-kappaB at the crossroads of life and death. Nat Immunol. 3 (30), 221–7. doi:10.1038/ni0302-221
Kaur, S., Gupta, S., Chaudhary, M., Khursheed, M. A., Mitra, S., Kurup, A. J., et al. (2018). let-7 MicroRNA-mediated regulation of shh signaling and the gene regulatory network is essential for retina regeneration. Cell Rep. 23 (5), 1409–1423. doi:10.1016/j.celrep.2018.04.002
Kawakami, K., Kobayashi, M., Matsuda, N., Takeda, H., Mishina, M., and Kawakami, N. (2004). A transposon-mediated gene trap approach identifies developmentally regulated genes in zebrafish. Dev. Cell 7 (1), 133–144. doi:10.1016/j.devcel.2004.06.005
Kawasaki, T., and Kawai, T. (2014). Toll-like receptor signaling pathways. Front. Immunol. 300 (5625), 1524–1525. doi:10.3389/fimmu.2014.00461
Keightley, M. C., Wang, C. H., Pazhakh, V., and Lieschke, G. J. (2014). Delineating the roles of neutrophils and macrophages in zebrafish regeneration models. Int. J. Biochem. Cell Biol. 56, 92–106. doi:10.1016/j.biocel.2014.07.010
Kim, S. D., Yang, S.-I., Kim, H.-C., Shin, C. Y., and Ko, K. H. (2007). Inhibition of GSK-3beta mediates expression of MMP-9 through ERK1/2 activation and translocation of NF-kappaB in rat primary astrocyte. Brain Res. 1186, 12–20. doi:10.1016/J.BRAINRES.2007.10.018
Kinney, J. W., Bemiller, S. M., Murtishaw, A. S., Leisgang, A. M., Salazar, A. M., and Lamb, B. T. (2018). Inflammation as a central mechanism in Alzheimer’s disease. Alzheimer’s Dementia Transl. Res. Clin. Interventions 4, 575–590. doi:10.1016/j.trci.2018.06.014
Kizil, C., Kyritsis, N., and Brand, M. (2015). Effects of inflammation on stem cells: together they strive? EMBO Rep. 16 (4), 416–426. doi:10.15252/embr.201439702
Kochan, T., Singla, A., Tosi, J., and Kumar, A. (2012). Toll-like receptor 2 ligand pretreatment attenuates retinal microglial inflammatory response but enhances phagocytic activity toward Staphylococcus aureus. Infect. Immun. 80 (6), 2076–2088. doi:10.1128/iai.00149-12
Kramer, A. C., Gurdziel, K., and Thummel, R. (2021). A comparative analysis of gene and protein expression throughout a full 28-day retinal regeneration time-course in adult zebrafish. Front. Cell Dev. Biol. 9, 741514. doi:10.3389/fcell.2021.741514
Kroehne, V., Freudenreich, D., Hans, S., Kaslin, J., and Brand, M. (2011). Regeneration of the adult zebrafish brain from neurogenic radial glia-type progenitors. Development 138 (22), 4831–41. doi:10.1242/dev.072587
Kumar, A., and Shamsuddin, N. (2012). Retinal muller glia initiate innate response to infectious stimuli via toll-like receptor signaling. PLoS ONE 7 (1), e29830. doi:10.1371/journal.pone.0029830
Kurimoto, T., Yin, Y., Habboub, G., Gilbert, H.-Y., Li, Y., Nakao, S., et al. (2013). Neutrophils express oncomodulin and promote optic nerve regeneration. J. Neurosci. 33 (37), 14816–14824. doi:10.1523/JNEUROSCI.5511-12.2013
Kyritsis, N., Kizil, C., Zocher, S., Kroehne, V., Kaslin, J., Freudenreich, D., et al. (2012). Acute inflammation initiates the regenerative response in the adult zebrafish brain. Science 338 (6112), 1353–1356. doi:10.1126/science.1228773
Lahne, M., Nagashima, M., Hyde, D. R., and Hitchcock, P. F. (2020). Reprogramming müller glia to regenerate retinal neurons. Annu. Rev. Vis. Sci. 6, 171–193. doi:10.1146/annurev-vision-121219-081808
Lange, C., Rost, F., MacHate, A., Reinhardt, S., Lesche, M., Weber, A., et al. (2020). Single cell sequencing of radial glia progeny reveals the diversity of newborn neurons in the adult zebrafish brain. Dev. Camb. 147 (1), dev185595. doi:10.1242/dev.185595
LeBert, D. C., Squirrell, J. M., Rindy, J., Broadbridge, E., Lui, Y., Zakrzewska, A., et al. (2015). Matrix metalloproteinase 9 modulates collagen matrices and wound repair. Development 142 (12), 2136–2146. doi:10.1242/dev.121160
Leinninger, G. M., Jo, Y. H., Leshan, R. L., Louis, G. W., Yang, H., Barrera, J. G., et al. (2009). Leptin acts via leptin receptor-expressing lateral hypothalamic neurons to modulate the mesolimbic dopamine system and suppress feeding. Cell Metab. 10 (2), 89–98. doi:10.1016/j.cmet.2009.06.011
Lenkowski, J. R., Qin, Z., Sifuentes, C. J., Thummel, R., Soto, C. M., Moens, C. B., et al. (2013). Retinal regeneration in adult zebrafish requires regulation of TGFβ signaling. Glia 61 (10), 1687–1697. doi:10.1002/glia.22549
Lenkowski, J. R., and Raymond, P. A. (2014). Müller glia: stem cells for generation and regeneration of retinal neurons in teleost fish. Prog. Retin. Eye Res. 40, 94–123. doi:10.1016/j.preteyeres.2013.12.007
Letiembre, M., Hao, W., Liu, Y., Walter, S., Mihaljevic, I., Rivest, S., et al. (2007). Innate immune receptor expression in normal brain aging. Neuroscience 146 (1), 248–254. doi:10.1016/J.NEUROSCIENCE.2007.01.004
Lin, X., Fang, D., Zhou, H., and Su, S. B. (2013). The expression of Toll-like receptors in murine Müller cells, the glial cells in retina. Neurol. Sci. 34 (8), 1339–1346. doi:10.1007/s10072-012-1236-1
Liu, T., Zhang, L., Joo, D., and Sun, S. C. (2017). NF-κB signaling in inflammation. Signal Transduct. Target. Ther. 2 (March), 17023. doi:10.1038/sigtrans.2017.23
Martins, R. R., Ellis, P. S., MacDonald, R. B., Richardson, R. J., and Henriques, C. M. (2019). Resident immunity in tissue repair and maintenance: the zebrafish model coming of age. Front. Cell Dev. Biol. 7, 12. doi:10.3389/fcell.2019.00012
McMenamin, P. G., Saban, D. R., and Dando, S. J. (2019). Immune cells in the retina and choroid: two different tissue environments that require different defenses and surveillance. Prog. Retin. Eye Res. 70, 85–98. doi:10.1016/j.preteyeres.2018.12.002
Mitchell, D. M., Lovel, A. G., and Stenkamp, D. L. (2018). Dynamic changes in microglial and macrophage characteristics during degeneration and regeneration of the zebrafish retina. J. Neuroinflammation 15 (1), 163–220. doi:10.1186/s12974-018-1185-6
Mitchell, D. M., Sun, C., Hunter, S. S., New, D. D., and Stenkamp, D. L. (2019). Regeneration associated transcriptional signature of retinal microglia and macrophages. Sci. Rep. 9 (1), 4768. doi:10.1038/s41598-019-41298-8
Moyse, B. R., and Richardson, R. J. (2020). A population of injury-responsive lymphoid cells expresses mpeg1.1 in the adult zebrafish heart. ImmunoHorizons 4 (8), 464–474. doi:10.4049/immunohorizons.2000063
Nathan, C., and Ding, A. (2010). Nonresolving inflammation. Cell 140 (6), 871–882. doi:10.1016/j.cell.2010.02.029
Nelson, C. M., Ackerman, K. M., O’Hayer, P., Bailey, T. J., Gorsuch, R. A., and Hyde, D. R. (2013). Tumor necrosis factor-alpha is produced by dying retinal neurons and is required for muller glia proliferation during zebrafish retinal regeneration. J. Neurosci. 33 (15), 6524–6539. doi:10.1523/JNEUROSCI.3838-12.2013
Nelson, C. M., Lennon, V. A., Lee, H., Krug, R. G. 2nd, Kamalova, A., Madigan, N. N., et al. (2019). Glucocorticoids Target Ependymal Glia and Inhibit Repair of the Injured Spinal Cor. Front. Cell Dev. Biol. 24 (7), 56. doi:10.3389/fcell.2019.00056
Olson, J. K., and Miller, S. D. (2004). Microglia initiate central nervous system innate and adaptive immune responses through multiple TLRs. J. Immunol. 173 (6), 3916–3924. doi:10.4049/jimmunol.173.6.3916
Oosterhof, N., Holtman, I. R., Kuil, L. E., van der Linde, H. C., Boddeke, E. W. G. M., Eggen, B. J. L., et al. (2017). Identification of a conserved and acute neurodegeneration-specific microglial transcriptome in the zebrafish. Glia 65 (1), 138–149. doi:10.1002/glia.23083
Palazzo, I., Deistler, K., Hoang, T. V., Blackshaw, S., and Fischer, A. J. (2020). NF-κB signaling regulates the formation of proliferating Müller glia-derived progenitor cells in the avian retina. Development 147 (10), dev183418. doi:10.1242/dev.183418
Petrie, T. A., Strand, N. S., Yang, C.-T., Rabinowitz, J. S., and Moon, R. T. (2015). Macrophages modulate adult zebrafish tail fin regeneration. Development 142 (2), 406. doi:10.1242/dev.120642
Polager, S., and Ginsberg, D. (2002). NF-kappaB at the crossroads of life and death. Trends Cell Biol. 3 (3), 528–535. Available at: http://www.ncbi.nlm.nih.gov/pubmed/18805009.
Portillo, J. A. C., Corcino, Y. L., Miao, Y., Tang, J., Sheibani, N., Kern, T. S., et al. (2017). CD40 in retinal müller cells induces P2X7-dependent cytokine expression in macrophages/microglia in diabetic mice and development of early experimental diabetic retinopathy. Diabetes 66 (2), 483–493. doi:10.2337/db16-0051
Powell, C., Cornblath, E., Elsaeidi, F., Wan, J., and Goldman, D. (2016). Zebrafish Müller glia-derived progenitors are multipotent, exhibit proliferative biases and regenerate excess neurons. Sci. Rep. 6, 24851. doi:10.1038/srep24851
Raymond, P. A., Barthel, L. K., Bernardos, R. L., and Perkowski, J. J. (2006). Molecular characterization of retinal stem cells and their niches in adult zebrafish. BMC Dev. Biol. 6 (36), 36–17. doi:10.1186/1471-213X-6-36
Redd, M. J., Kelly, G., Dunn, G., Way, M., and Martin, P. (2006). Imaging macrophage chemotaxis in vivo: studies of microtubule function in zebrafish wound inflammation. Cell Motil. Cytoskelet. 63 (7), 415–422. doi:10.1002/cm.20133
Renshaw, S. A., Loynes, C. A., Trushell, D. M. I., Elworthy, S., Ingham, P. W., and Whyte, M. K. B. (2016). Plenary paper A transgenic zebrafish model of neutrophilic inflammation. Blood J. 108 (13), 3976–3979. doi:10.1182/blood-2006-05-024075
Roche, S. L., Ruiz-Lopez, A. M., Moloney, J. N., Byrne, A. M., and Cotter, T. G. (2018). Microglial-induced Müller cell gliosis is attenuated by progesterone in a mouse model of retinitis pigmentosa. Glia 66 (2), 295–310. doi:10.1002/glia.23243
Rocío Nieto-Arellano, H. S.-I., and Sánchez-Iranzo, H. (2019). zfRegeneration: a database for gene expression profiling during regeneration. Bioinformatics 35 (4), 703–705. doi:10.1093/bioinformatics/bty659
Schindelin, J., Arganda-Carreras, I., Frise, E., Kaynig, V., Longair, M., Pietzsch, T., et al. (2012). Fiji: an open-source platform for biological-image analysis. Nat. Methods 9 (7), 676–682. doi:10.1038/nmeth.2019
Semmelink, M. F. W., Steen, A., and Veenhoff, L. M. (2021). Measuring and interpreting nuclear transport in neurodegenerative disease—the example of C9orf72 ALS. Int. J. Mol. Sci. 22 (17), 9217. doi:10.3390/ijms22179217
Shiau, C. E., Kaufman, Z., Meireles, A. M., and Talbot, W. S. (2015). Differential requirement for irf8 in formation of embryonic and adult macrophages in zebrafish. PLOS ONE 10 (1), e0117513. doi:10.1371/journal.pone.0117513
Sifuentes, C. J., Kim, J. W., Swaroop, A., and Raymond, P. A. (2016). Rapid, dynamic activation of Müller Glial stem cell responses in Zebrafish. Investigative Ophthalmol. Vis. Sci. 57 (13), 5148–5160. doi:10.1167/iovs.16-19973
Silva, N. J., Nagashima, M., Li, J., Kakuk-Atkins, L., Ashrafzadeh, M., Hyde, D. R., et al. (2020). Inflammation and matrix metalloproteinase 9 (Mmp-9) regulate photoreceptor regeneration in adult zebrafish. Glia 68 (7), 1445–1465. doi:10.1002/glia.23792
Sim, D. A., Chu, C. J., Selvam, S., Powner, M. B., Liyanage, S., Copland, D. A., et al. (2015). A simple method for in vivo labelling of infiltrating leukocytes in the mouse retina using indocyanine green dye. Dis. Models Mech. 8 (11), 1479–1487. doi:10.1242/dmm.019018
Sofroniew, M. V. (2009). Molecular dissection of reactive astrogliosis and glial scar formation. Trends Neurosci. 32 (12), 638–647. doi:10.1016/j.tins.2009.08.002
Song, P., Fogerty, J., Cianciolo, L. T., Stupay, R., and Perkins, B. D. (2020). Cone photoreceptor degeneration and neuroinflammation in the zebrafish bardet-biedl syndrome 2 (bbs2) mutant does not lead to retinal regeneration. Front. Cell Dev. Biol. 8, 578528. doi:10.3389/fcell.2020.578528
Stirling, D. P., Liu, S., Kubes, P., and Yong, V. W. (2009). Depletion of Ly6G/Gr-1 leukocytes after spinal cord injury in mice alters wound healing and worsens neurological outcome. J. Neurosci. 29 (3), 753–764. doi:10.1523/JNEUROSCI.4918-08.2009
Takada, Y., Khuri, F. R., and Aggarwal, B. B. (2004). Protein farnesyltransferase inhibitor (SCH 66336) abolishes NF-kappaB activation induced by various carcinogens and inflammatory stimuli leading to suppression of NF-kappaB-regulated gene expression and up-regulation of apoptosis. J. Biol. Chem. 279 (25), 26287–26299. doi:10.1074/jbc.M400963200
Takechi, M., Hamaoka, T., and Kawamura, S. (2003). Fluorescence visualization of ultraviolet-sensitive cone photoreceptor development in living zebrafish. FEBS Lett. 553 (1–2), 90–94. doi:10.1016/S0014-5793(03)00977-3
Takke, C., Dornseifer, P., v Weizsäcker, E., and Campos-Ortega, J. A. (1999). her4, a zebrafish homologue of the Drosophila neurogenic gene E(spl), is a target of NOTCH signalling. Dev. Camb. Engl. 126 (9), 1811–1821. doi:10.1242/dev.126.9.1811
Thomas, J. L., Ranski, A. H., Morgan, G. W., and Thummel, R. (2016). Reactive gliosis in the adult zebrafish retina. Exp. Eye Res. 143, 98–109. doi:10.1016/j.exer.2015.09.017
Todd, L., Finkbeiner, C., Wong, C. K., Hooper, M. J., and Reh, T. A. (2020). Microglia suppress ascl1-induced retinal regeneration in mice. Cell Rep. 33 (11), 108507. doi:10.1016/j.celrep.2020.108507
Tsarouchas, T. M., Wehner, D., Cavone, L., Munir, T., Keatinge, M., Lambertus, M., et al. (2018). Dynamic control of proinflammatory cytokines Il-1β and Tnf-α by macrophages in zebrafish spinal cord regeneration. Nat. Commun. 9 (1), 4670. doi:10.1038/s41467-018-07036-w
Van Dyck, A., Bollaerts, I., Beckers, A., Vanhunsel, S., Glorian, N., van Houcke, J., et al. (2021). Müller glia-myeloid cell crosstalk accelerates optic nerve regeneration in the adult zebrafish. Glia 69 (6), 1444–1463. doi:10.1002/glia.23972
Walker, M. J., Hollands, A., Sanderson-Smith, M. L., Cole, J. N., Kirk, J. K., Henningham, A., et al. (2007). DNase Sda1 provides selection pressure for a switch to invasive group A streptococcal infection. Nat. Med. 13 (8), 981–985. doi:10.1038/nm1612
Wan, J., Ramachandran, R., and Goldman, D. (2012). HB-EGF is necessary and sufficient for müller glia dedifferentiation and retina regeneration. Dev. Cell 22 (2), 334–347. doi:10.1016/j.devcel.2011.11.020
Wang, J. (2018). Neutrophils in tissue injury and repair. Cell Tissue Res. 371 (3), 531–539. doi:10.1007/s00441-017-2785-7
Wang, M., Ma, W., Zhao, L., Fariss, R. N., and Wong, W. T. (2011). Adaptive Müller cell responses to microglial activation mediate neuroprotection and coordinate inflammation in the retina. J. Neuroinflammation 8 (1), 173. doi:10.1186/1742-2094-8-173
Weber, A., Hochmann, S., Cimalla, P., Gärtner, M., Kuscha, V., Hans, S., et al. (2013). Characterization of light lesion paradigms and optical coherence tomography as tools to study adult retina regeneration in zebrafish. PLOS ONE 8 (11), e80483. doi:10.1371/journal.pone.0080483
White, D. T., Sengupta, S., Saxena, M. T., Xu, Q., Hanes, J., Ding, D., et al. (2017). Immunomodulation-accelerated neuronal regeneration following selective rod photoreceptor cell ablation in the zebrafish retina. Proc. Natl. Acad. Sci. 114 (18), E3719–E3728. doi:10.1073/pnas.1617721114
Wu, S., Xue, R., Hassan, S., Nguyen, T. M. L., Wang, T., Pan, H., et al. (2018). Il34-Csf1r pathway regulates the migration and colonization of microglial precursors. Dev. Cell 46 (5), 552–563. doi:10.1016/j.devcel.2018.08.005
Xu, S., Webb, S. E., Lau, T. C. K., and Cheng, S. H. (2018). Matrix metalloproteinases (MMPs) mediate leukocyte recruitment during the inflammatory phase of zebrafish heart regeneration. Sci. Rep. 8 (1), 7199–7214. doi:10.1038/s41598-018-25490-w
Yang, J.-S., Lin, C.-W., Hsieh, Y.-H., Chien, M.-H., Chuang, C.-Y., and Yang, S.-F. (2017). Overexpression of carbonic anhydrase IX induces cell motility by activating matrix metalloproteinase-9 in human oral squamous cell carcinoma cells. Oncotarget 8 (47), 83088–83099. doi:10.18632/oncotarget.20236
Yoong, S., O’Connell, B., Soanes, A., Crowhurst, M. O., Lieschke, G. J., and Ward, A. C. (2007). Characterization of the zebrafish matrix metalloproteinase 9 gene and its developmental expression pattern. Gene Expr. Patterns 7 (1–2), 39–46. doi:10.1016/j.modgep.2006.05.005
Zhang, Z., Hou, H., Yu, S., Zhou, C., Zhang, X., Li, N., et al. (2020). Inflammation-induced mammalian target of rapamycin signaling is essential for retina regeneration. Glia 68 (1), 111–127. doi:10.1002/glia.23707
Zhao, X. F., Wan, J., Powell, C., Ramachandran, R., Myers, M. G., and Goldman, D. (2014). Leptin and IL-6 family cytokines synergize to stimulate Müller Glia reprogramming and retina regeneration. Cell Rep. 9 (1), 272–284. doi:10.1016/j.celrep.2014.08.047
Zhou, Y., Hong, Y., and Huang, H. (2016). Triptolide attenuates inflammatory response in membranous glomerulo-nephritis rat via downregulation of NF-κB signaling pathway. Kidney Blood Press. Res. 41 (6), 901–910. doi:10.1159/000452591
Zhu, S., Yu, Y., Ren, Y., Xu, L., Wang, H., Ling, X., et al. (2021). The emerging roles of neutrophil extracellular traps in wound healing. Cell Death Dis. 12 (October), 984. doi:10.1038/s41419-021-04294-3
Keywords: immune suppression, M-CSF, microglia, Müller glia, NF-κB pathway, regeneration, retina, zebrafish
Citation: Bludau O, Weber A, Bosak V, Kuscha V, Dietrich K, Hans S and Brand M (2024) Inflammation is a critical factor for successful regeneration of the adult zebrafish retina in response to diffuse light lesion. Front. Cell Dev. Biol. 12:1332347. doi: 10.3389/fcell.2024.1332347
Received: 02 November 2023; Accepted: 17 June 2024;
Published: 12 July 2024.
Edited by:
Enrique Martin-Blanco, Spanish National Research Council (CSIC), SpainReviewed by:
David R. Hyde, University of Notre Dame, United StatesRyan Thummel, Wayne State University, United States
Copyright © 2024 Bludau, Weber, Bosak, Kuscha, Dietrich, Hans and Brand. This is an open-access article distributed under the terms of the Creative Commons Attribution License (CC BY). The use, distribution or reproduction in other forums is permitted, provided the original author(s) and the copyright owner(s) are credited and that the original publication in this journal is cited, in accordance with accepted academic practice. No use, distribution or reproduction is permitted which does not comply with these terms.
*Correspondence: Michael Brand, bWljaGFlbC5icmFuZEB0dS1kcmVzZGVuLmRl
†ORCID: Michael Brand, orcid.org/0000-0001-5711-6512
‡Present address: Oliver Bludau,Department of Physiological Genomics, Ludwig-Maximilians-Universität München, Planegg-Martinsried, Germany