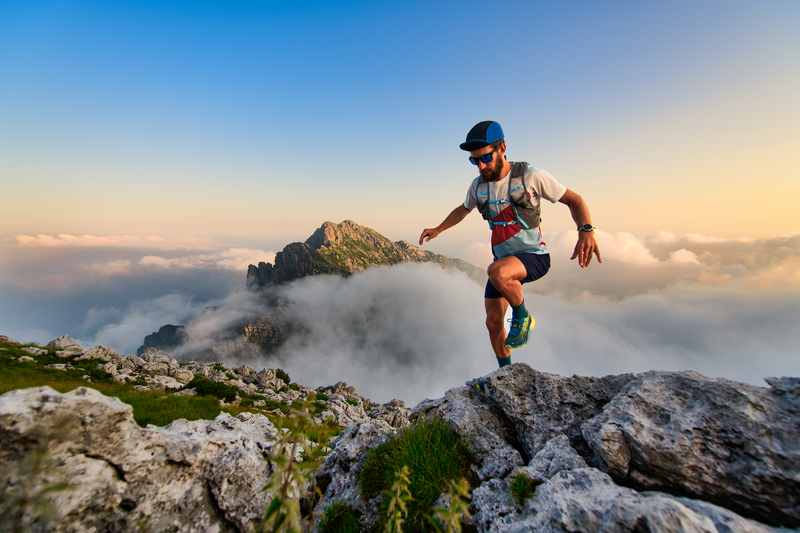
95% of researchers rate our articles as excellent or good
Learn more about the work of our research integrity team to safeguard the quality of each article we publish.
Find out more
MINI REVIEW article
Front. Cell Dev. Biol. , 13 December 2023
Sec. Membrane Traffic and Organelle Dynamics
Volume 11 - 2023 | https://doi.org/10.3389/fcell.2023.1334798
This article is part of the Research Topic Editors' Showcase 2023: Insights in Membrane Traffic View all 7 articles
The past six decades have seen major advances in our understanding of endocytosis, ranging from descriptive studies based on electron microscopy to biochemical and genetic characterization of factors required for vesicle formation. Most studies focus on clathrin as the major coat protein; indeed, clathrin-mediated endocytosis (CME) is the primary pathway for internalization. Clathrin-independent (CIE) pathways also exist, although mechanistic understanding of these pathways remains comparatively elusive. Here, we discuss how early studies of CME shaped our understanding of endocytosis and describe recent advances in CIE, including pathways in model organisms that are poised to provide key insights into endocytic regulation.
As we approach 2024, we mark 60 years of structural, biochemical, and genetic studies of endocytosis. While early work reported observations of phagocytosis and macropinocytosis, Roth and Porter capitalized on advances in electron microscopy to visualize mosquito oocytes forming pits at the plasma membrane (PM), resulting in intracellular vesicles (Roth and Porter, 1964). Their 1964 work suggested events leading to internalization: 1) assembly of a “bristle coat” at the PM, 2) invagination of bristle-coated structures into pits, 3) conversion of pits into vesicles, and 4) loss of the coat. Roth and Porter proposed mechanical functions for the coat that were later verified, including in a key 1969 study by Kanaseki and Kadota describing the coated structure as “The Vesicle in the Basket” (Kanaseki and Kadota, 1969). Here, electron micrographs of bristle-coated vesicles isolated from guinea pig brain demonstrated a polygonal coat surrounding the vesicle. Coat formation progressed from shallow, bristle-lined pits on the cytoplasmic face to the completed vesicle. Subsequently, Barbara Pearse isolated coated vesicles from pig brain in 1975, and described a polygonal structure consistent with findings from Kanaseki and Kadota (Pearse, 1975). Pearse purified a 180 kDa protein as the sole component of coats, and named it clathrin. These and other foundational papers accurately described the process of clathrin-mediated endocytosis (CME), identified the key coat protein, and formed the basis of our modern understanding of endocytosis.
CME is the main pathway for PM internalization (Kaksonen and Roux, 2018). It is currently the best-studied endocytic route, and involves sequential action of protein modules to recruit cargo, deform the PM, and generate a clathrin-coated vesicle (CCV) (Kaksonen et al., 2005; Newpher et al., 2005). During initial stages of clathrin-coated pit (CCP) formation, which may occur stochastically or from initiating cues, early-arriving proteins such as FCHo1/2 and the clathrin-binding adaptor complex AP-2 associate with endocytic sites by binding phosphoinositides, particularly phosphatidylinositol 4,5-bisphosphate [PI(4,5)P2] (Howard et al., 2002; Henne et al., 2010; Cocucci et al., 2012). Adaptors serve a dual function in cargo-binding and in providing an anchor point for clathrin assembly. The clathrin lattice consists of heavy and light chains that interact to form a triskelion (Unanue et al., 1981). As the CCP matures, scaffolding proteins recruit later-acting factors that facilitate invagination of endocytic pits, often through activation of Arp2/3 (Kübler and Riezman, 1993; Kaksonen et al., 2003; 2005; Sun et al., 2006; Goode et al., 2015). Finally, Bin/amphiphysin/Rvs (BAR)-domain proteins such as amphiphysin, syndapin and SNX9, and F-BAR proteins FBP17 and CIP4 interact with the GTPase dynamin at the CCP neck to facilitate scission (Bliek et al., 1993; Damke et al., 1994; Takei et al., 1995; Qualmann and Kelly, 2000; Kamioka et al., 2004; Soulet et al., 2005; Shimada et al., 2007; Yu and Yoshimura, 2022). Separated from the PM, the CCV quickly loses its coat following recruitment of auxilin/DnaJ, Hsp70, and synpatojanins (Ungewickell et al., 1995). Uncoated vesicles are then free to fuse to their target compartments. CME is conserved in eukaryotes, and many endocytic proteins perform similar functions in all species examined to date (Taylor et al., 2011).
Aside from CME, other pathways perform endocytosis without the clathrin coat. Collectively termed clathrin-independent endocytosis (CIE), these pathways offer additional routes for cargo entry (Figure 1). Indeed, early studies predating discovery of the bristle coat described phagocytosis and macropinocytosis, which are inherently clathrin-independent (Tauber, 2003; King and Kay, 2019). Intriguingly, molecular mechanisms of CIE remain poorly understood compared to CME. Recent studies advancing our understanding of CIE are filling gaps in our knowledge, with model organisms permitting identification of CIE genes that are likely conserved. In this review, we examine the history of CIE research, explore recent discoveries, and look toward new questions in the field.
FIGURE 1. Major Forms of Endocytosis. In addition to the well-studied clathrin-mediated endocytic pathway, a variety of clathrin-independent pathways exist in eukaryotes. Shown here, many of the known CIE pathways share overlapping machinery or classes of proteins with CME or with each other, while also possessing unique components and internalizing specific cargo proteins.
Although CCV purification permitted identification of CME machinery proteins, evidence of additional pathways predated the discovery of coated pits (Keen et al., 1979; Zaremba and Keen, 1983). For example, phagocytosis and micropinocytosis were first observed over 100 years ago, while caveolae were described nearly a decade before the discovery of coated vesicles but were not confirmed as endocytic structures until later (Tauber, 2003; King and Kay, 2019). Studies in the 1980s described formation of uncoated endocytic carriers, suggesting that CIE exists distinct from (and/or parallel to) CME (Moya et al., 1985). A clathrin-independent pathway was finally demonstrated in the 1990s with experiments that showed uptake of ricin persisted upon inhibition of transferrin internalization via CCVs (Sandvig and Deurs, 1990; Hansen et al., 1991). Additionally, genetic and biochemical studies showed that cells expressing dominant-negative mutants of CME proteins such as dynamin, epsinR, eps15 and AP180 still internalized fluid-phase and PM components (Damke et al., 1994; Benmerah et al., 1998; Chen et al., 1998; Ford et al., 2001). Identification of additional, mechanistically-distinct CIE pathways further demonstrated that endocytosis is far more complex than originally thought (Figure 1). In addition to phagocytic, macropinocytic and caveolar routes, these include clathrin-independent carriers and GPI-enriched endocytic compartments (CLIC/GEEC), small GTPase-mediated pathways, fast endophilin-mediated endocytosis (FEME) and ultrafast endocytosis (UFE) (Lamaze et al., 2001; Kirkham et al., 2005; Lundmark et al., 2008; Watanabe et al., 2013; Boucrot et al., 2015; Hak et al., 2018; Sathe et al., 2018; Casamento and Boucrot, 2020; Imoto et al., 2022).
Phagocytosis and macropinocytosis were amongst the earliest forms of endocytosis observed; both internalize large volumes in a single event. Phagocytosis occurs when cells extend the PM around particles to engulf them. This mechanism is highly conserved from protists such as Dictyostelium discoideum to immune cells (e.g., neutrophils and macrophages), and internalizes nutrients, clears debris and dead cells, and combats pathogens (Tauber, 2003; Vorselen et al., 2020). Initiation of a phagocytic cup occurs when surface receptors such as integrins recognize molecules on the foreign particle, triggering recruitment and activation of RhoA, Rac1 and Cdc42 along with actin modulators such as WASP and Arp2/3 (Caron and Hall, 1998; Jaumouillé et al., 2019). This machinery drives formation of pseudopodia through assembly of actin networks that provide force to drive PM protrusion. Similarly, macropinocytosis is actin-dependent, and relies on Rac1, Ras and Arf6 to induce PM ruffling that internalizes extracellular fluids, nutrients, growth factors and pathogens (Bar-Sagi and Feramisco, 1986; Fujii et al., 2013). Aside from bulk internalization, macropinocytosis allows cells to explore their environment and receive signals from other cells (Veltman et al., 2016). Closure and scission of phagosomes and macropinosomes remains poorly understood; however, myosins likely play a role through interactions with actin (Brzeska et al., 2016).
Caveolae are detergent-resistant, flask-shaped invaginations found in PM regions with high cholesterol and sphingolipid levels (Parton et al., 2006). The structure of caveolae is determined by the transmembrane proteins caveolin-1 (CAV1) and caveolin-2 (CAV2), which form a heterodimer that interacts with cytosolic cavins (Kiss and Botos, 2009). Caveolae are generally immobile, but binding of ligands such as simian virus 40, cholera toxin or albumin induces internalization (Pelkmans et al., 2002). Upon ligand binding, CAV1 recruits Src-family tyrosine kinases to specialized scaffolding domains. Src then phosphorylates CAV1/2, leading to endocytosis through currently-unclear mechanisms that depend on dynamin (Oh et al., 1998; Shajahan et al., 2004; Sverdlov et al., 2007).
To date, several CIE pathways involving Rho- and Arf-family GTPases have been identified but remain understudied. RhoA-dependent CIE internalizes the interleukin-2 receptor (IL2R); this pathway is dynamin-dependent, and requires Rac1 and its effector Pak1 (Lamaze et al., 2001). RhoA-dependent CIE is also responsible for compensatory endocytosis in bladder umbrella cells, which rapidly expand during bladder filling and contract during voiding. RhoA-dependent CIE allows for rapid PM internalization after voiding, likely in response to loss of membrane tension (Khandelwal et al., 2010). CIE in umbrella cells requires integrins but not Rac1, suggesting mechanistic distinctions from IL2R internalization.
Arf6 defines a dynamin-independent CIE route, that internalizes MHC1 as its major cargo, wherein the GTPase contributes to actin polymerization, activation of phosphatidylinositol-4-phosphate 5-kinase (PIP5K) and phospholipase D (PLD) (Naslavsky et al., 2004). PIP5K then generates PI(4,5)P2, which is required for vesicle formation (Grant and Donaldson, 2009). Subsequently, Arf6 inactivation promotes sorting to early endosomes or recycling pathways. Other CIE pathways, including FEME, UFE and CLIC/GEEC also utilize small GTPases, but are described separately due to the larger number of additional proteins involved.
Several CIE mechanisms operate independent of clathrin and dynamin, including CLIC/GEEC, which internalizes bulk fluid-phase material and glycosylphosphatidylinositol-anchored proteins (GPI-APs) in tubular carriers (Sabharanjak et al., 2002; Kirkham et al., 2005). At cholesterol-enriched PM microdomains, recruitment of the Arf1 guanine nucleotide exchange factor (GEF) GBF1 leads to Arf1 activation (Römer et al., 2010). Arf1-GTP subsequently recruits the Cdc42 GTPase-activating protein (GAP) ARGHGAP10/21, which regulates formation of endocytic carriers (Gupta et al., 2009). The Cdc42 effector GRAF1, which contains RhoGAP, SH3 and BAR-domains, also aids in membrane deformation while regulating Cdc42 activity (Lundmark et al., 2008). Additionally, CLIC/GEEC internalizes Shiga and cholera toxins, and may be activated to compensate for loss of membrane tension (Ferreira and Boucrot, 2018; Thottacherry et al., 2019).
FEME is a CIE pathway that relies on the BAR protein Endophilin to internalize cargos including G protein-coupled receptors (GPCRs), receptor tyrosine kinases (RTKs) and cytokine receptors (Boucrot et al., 2015). Upon ligand-receptor binding, Cdc42 initiates a signaling cascade via FBP17 and CIP4, which recruit the lipid phosphatases SHIP1/2; RhoA and Rac1 may also participate. Dephosphorylation of PI(3,4,5)P3 into PI(3,4)P2 permits anchoring of Lamellipodin, which binds to Endophilins A1 and A2 to facilitate membrane deformation (Hak et al., 2018). Finally, microtubules and microtubule-based motors are required for membrane tubule extension, while dynamin completes scission (Renard et al., 2015). Related to FEME is UFE, which involves endophilin-A3, synaptojanin-1, dynamin, and formin to mediate rapid recycling of synaptic membranes in neurons (Watanabe et al., 2013; 2018; Soykan et al., 2017; Imoto et al., 2022). FEME and UFE share some, but not all components, suggesting distinctions between the two pathways (Figure 1).
Genetically-tractable model organisms provide important opportunities to further our understanding of endocytosis and its regulation. Notably, budding and fission yeast (Saccharomyces cerevisiae and Schizosaccharomyces pombe, respectively) were extensively used to uncover conserved CME proteins and to identify the sequence of events required for CCV formation (Engqvist-Goldstein and Drubin, 2003; Kaksonen et al., 2005; Yarar et al., 2005; Basu and Chang, 2011). Metazoan models such as Drosophila melanogaster and Caenorhabditis elegans are valuable for characterizing roles for endocytosis during development and in the context of tissue and organ function.
While model organisms aid in understanding CME, their use in studying CIE pathways remains limited by comparison. For example, CIE in budding yeast was first described in 2011; all yeast were previously thought to utilize only CME, even though residual endocytosis still occurs in clathrin-null cells (Payne et al., 1988; Prosser et al., 2011). In the following section, we describe recent breakthroughs in CIE from the standpoint of model organisms and how they provide insight into mammalian pathways.
Similar to the mammalian RhoA-dependent pathway, CIE in budding yeast requires its homolog Rho1, as first described in CME-defective cells lacking functional adaptor proteins (Prosser et al., 2011). In numerous CME-deficient mutants, including clathrin-null cells, high-copy expression of RHO1, its GEF ROM1, and the integrin-like cell wall stress sensor MID2 improve cargo internalization, but do not correct aberrant dynamics of CME sites. Yeast CIE additionally requires the formin Bni1 (a Rho1 effector), polarisome proteins involved in Bni1 localization, and actin-stabilizing tropomyosins. Subsequent studies demonstrated CIE roles for select proteins involved in CME, including α-arrestins and Syp1, although their function in CME versus CIE may be mechanistically distinct (Prosser et al., 2015; Apel et al., 2017). Recent work demonstrated roles for the myosin Myo2, Myo2-dependent transport of cytoplasmic microtubules, microtubule-based motors (dynein/dynactin), and proteins involved in cortical microtubule capture (Num1) (Woodard et al., 2023). These findings suggest parallels between yeast CIE and mammalian pathways, including UFE (formin-dependent), FEME and uptake of cholera and Shiga toxins (microtubule and dynein/dynactin-dependent) (Day et al., 2015; Renard et al., 2015; Soykan et al., 2017).
Growing evidence suggests that CIE occurs in other fungi, although pathways remain poorly characterized. Fission yeast utilizes CME for endocytosis at cell tips, while formin (For3)-dependent actin polymerization facilitates internalization at the sides of cells (Gachet and Hyams, 2005); roles for clathrin in For3-dependent endocytosis have not yet been assessed. In Candida albicans, endocytosis persists in cells lacking Arp2/3, suggesting a likely CIE mechanism (Epp et al., 2013). RHO1 overexpression does not restore endocytosis in arp2/3 mutant Candida, suggesting differences from budding yeast CIE. Finally, in the filamentous fungus Aspergillus nidulans, an AP-2 and clathrin-independent endocytic pathway contributes to apical growth. Unlike budding yeast, α-arrestins are not involved in Aspergillus CIE, further suggesting mechanistic differences amongst fungi (Martzoukou et al., 2017).
Drosophila is widely used for understanding the genetic basis of metazoan development, which requires coordination of signaling events dependent on receptor internalization. One example is Delta-Notch signaling, wherein Notch is endocytosed and induces cell proliferation and differentiation. Some Notch and Delta internalize in clathrin mutant flies in a dynamin-dependent manner, suggesting a CIE pathway of unknown mechanism (Windler and Bilder, 2010; Hemalatha et al., 2016). Moreover, Drosophila utilizes phagocytic, macropinocytic and CLIC/GEEC pathways for internalization (Gupta et al., 2009).
Several CIE pathways exist in C. elegans, including an Arf6-mediated pathway similar to the mammalian mechanism described above. This pathway contributes to sorting and recycling using Rab10, Rab22, Rab35, Hook1, ALX1 and RME-1/EHD-1 (Chen et al., 2006; Glodowski et al., 2007; Shi et al., 2007). In addition, C. elegans is useful for studying UFE, which was first described in worms. Upon light stimulation of motor neurons expressing channelrhodopsin, endocytic events begin 50 ms after stimulation and are completed within one second, faster than occurs during CME (Watanabe et al., 2013). Further study of this pathway showed that dynamin, endophilin, and synaptojanin are required, while clathrin is not.
Characterization of endocytosis in plants is limited, but studies in Arabidopsis thaliana demonstrate that CIE functions during environmental stress and seedling development. Arabidopsis CIE relies on Flotillin1 (Flot1) at sterol- and sphingolipid-enriched membrane microdomains distinct from clathrin-containing PM regions (Li et al., 2012). Additionally, Latrunculin B-mediated actin depolymerization in root tip cells inhibits formation of Flot1-positive compartments, suggesting actin involvement. Knockdown of Flot1 impairs seedling development and growth, implying roles for Flot1 and CIE in these processes (Li et al., 2012). Arabidopsis offers a unique tool for studying endocytosis in a whole tissue context due to high levels of organization in root cells and ease of visualization by light microscopy. Analysis of root tips demonstrates that GPI-APs and FM4-64 are constitutively internalized through CME and CIE in epidermal cells, while inner cells only require CME. However, under high salinity, CIE is upregulated across all root cells to internalize GPI-APs, transmembrane proteins, and FM4-64. This pathway is distinct from constitutive CIE, and requires the Rab5 GEF Vps9a, and sterols (Baral et al., 2015).
In recent years, our understanding of CIE has expanded to reveal diverse pathways; this increased knowledge opens new questions and avenues for future exploration. First, what is the degree of overlap between pathways? While some proteins clearly participate in both CME and CIE, or in multiple CIE pathways, contributions to different pathways may be mechanistically distinct. Often, different pathways share several major components, but recruit unique proteins to work with the core machinery. For example, the CLIC/GEEC pathway, FEME and UFE all involve Cdc42 during early events to activate proteins that modulate phosphoinositides, which bind BAR-domain proteins. BAR proteins differ between pathways, with CLIC/GEEC relying on GRAF1, FEME on endophilin-A1/A2 and UFE on endophilin-A3 (Lundmark et al., 2008; Sathe et al., 2018; Casamento and Boucrot, 2020). Among these, only CLIC/GEEC is dynamin-independent, suggesting distinct scission mechanisms. This mosaic pattern of components is common in CIE, and provides targets to dissect mechanistic commonalities and differences.
The lack of defined coat in CIE raises questions about how forces are generated for membrane bending. BAR-domain proteins sense and induce membrane curvature and may thus contribute to deformation, although how these proteins function in CIE remains unclear. Newly-described roles for myosins, microtubules and other cytoskeletal components in budding yeast CIE could similarly contribute to membrane deformation. In CME, the type I myosins Myo3/5 generate force during endocytosis through interactions with actin (Sun et al., 2006). Myo2 may play a similar role in CIE; alternatively, Myo2-dependent transport of microtubules to the cell cortex may suggest involvement of dynein/dynactin (Woodard et al., 2023).
Sorting and selection mechanisms are also unresolved questions, in large part because of the lack of CIE cargos. While cargos are known for RhoA-mediated endocytosis (IL2R) and CLIC/GEEC (GPI-APs), they remain less defined for other routes such as FEME and UFE. In yeast, the pheromone receptor Ste3 was used to characterize CIE, but prefers CME as its primary route (Prosser et al., 2011). Ptr2 is another yeast cargo that prefers CME at low osmolarity, but is partially redirected into a CIE path under high osmolarity conditions (Apel et al., 2017). Aside from these, new studies continue to reveal additional cargos that will likely allow us to better understand selection mechanisms during CME and CIE. For example, amyloid precursor protein (APP) and low-density lipoprotein internalize when clathrin and dynamin are inhibited, suggesting roles for an as-yet undetermined CIE route (Aow et al., 2022). Similarly, uptake of L1CAM and CD166 through Endophilin-A3-mediated CIE may facilitate studies of pathways such as FEME or UFE (Renard et al., 2019; Lemaigre et al., 2022).
As our knowledge of CIE expands, we are only beginning to understand these unique and exciting pathways. Much work remains in characterizing the proteins involved, elucidating physiological roles for CIE in health and disease, and assessing relationships between distinct CIE routes or between CME and CIE. Addressing these knowledge gaps is critical to expanding our understanding of CIE, and will provide important insights into the multiple mechanisms that contribute to PM regulation.
DR: Conceptualization, Data curation, Visualization, Writing–original draft, Writing–review and editing. DP: Conceptualization, Data curation, Funding acquisition, Project administration, Supervision, Writing–original draft, Writing–review and editing.
The author(s) declare financial support was received for the research, authorship, and/or publication of this article. This work was supported by a National Science Foundation CAREER Award (MCB 1942395, to DP).
We would like to acknowledge the many excellent studies in the field of endocytosis that we were unable to discuss due to space constraints; we are also grateful to members of the DP lab for helpful discussions and feedback.
The authors declare that the research was conducted in the absence of any commercial or financial relationships that could be construed as a potential conflict of interest.
All claims expressed in this article are solely those of the authors and do not necessarily represent those of their affiliated organizations, or those of the publisher, the editors and the reviewers. Any product that may be evaluated in this article, or claim that may be made by its manufacturer, is not guaranteed or endorsed by the publisher.
Aow, J., Huang, T.-R., Goh, Y. T., Sun, A. X., Thinakaran, G., and Koo, E. H. (2022). Evidence for a clathrin-independent endocytic pathway for APP internalization in the neuronal somatodendritic compartment. Cell Rep. 42, 112774. doi:10.1016/j.celrep.2023.112774
Apel, A. R., Hoban, K., Chuartzman, S., Tonikian, R., Sidhu, S., Schuldiner, M., et al. (2017). Syp1 regulates the clathrin-mediated and clathrin-independent endocytosis of multiple cargo proteins through a novel sorting motif. Mol. Biol. Cell. 28, 2434–2448. doi:10.1091/mbc.e15-10-0731
Baral, A., Irani, N. G., Fujimoto, M., Nakano, A., Mayor, S., and Mathew, M. K. (2015). Salt-Induced remodeling of spatially restricted clathrin-independent endocytic pathways in Arabidopsis root. Plant Cell. 27, 1297–1315. doi:10.1105/tpc.15.00154
Bar-Sagi, D., and Feramisco, J. R. (1986). Induction of membrane ruffling and fluid-phase pinocytosis in quiescent fibroblasts by ras proteins. Science 233, 1061–1068. doi:10.1126/science.3090687
Basu, R., and Chang, F. (2011). Characterization of Dip1p reveals a switch in arp2/3-dependent actin assembly for fission yeast endocytosis. Curr. Biol. 21, 905–916. doi:10.1016/j.cub.2011.04.047
Benmerah, A., Lamaze, C., Bègue, B., Schmid, S. L., Dautry-Varsat, A., and Cerf-Bensussan, N. (1998). AP-2/Eps15 interaction is required for receptor-mediated endocytosis. J. Cell. Biol. 140, 1055–1062. doi:10.1083/jcb.140.5.1055
Bliek, A. V. D., Redelmeier, T., Damke, H., Tisdale, E., Meyerowitz, E., and Schmid, S. (1993). Mutations in human dynamin block an intermediate stage in coated vesicle formation. J. cell. Biol. 122, 553–563. doi:10.1083/jcb.122.3.553
Boucrot, E., Ferreira, A. P. A., Almeida-Souza, L., Debard, S., Vallis, Y., Howard, G., et al. (2015). Endophilin marks and controls a clathrin-independent endocytic pathway. Nature 517, 460–465. doi:10.1038/nature14067
Brzeska, H., Koech, H., Pridham, K. J., Korn, E. D., and Titus, M. A. (2016). Selective localization of myosin-I proteins in macropinosomes and actin waves. Cytoskeleton 73, 68–82. doi:10.1002/cm.21275
Caron, E., and Hall, A. (1998). Identification of two distinct mechanisms of phagocytosis controlled by different Rho GTPases. Science 282, 1717–1721. doi:10.1126/science.282.5394.1717
Casamento, A., and Boucrot, E. (2020). Molecular mechanism of fast endophilin-mediated endocytosis. Biochem. J. 477, 2327–2345. doi:10.1042/bcj20190342
Chen, C. C.-H., Schweinsberg, P. J., Vashist, S., Mareiniss, D. P., Lambie, E. J., and Grant, B. D. (2006). RAB-10 is required for endocytic recycling in the Caenorhabditis elegans intestine. Mol. Biol. Cell. 17, 1286–1297. doi:10.1091/mbc.e05-08-0787
Chen, H., Fre, S., Slepnev, V. I., Capua, M. R., Takei, K., Butler, M. H., et al. (1998). Epsin is an EH-domain-binding protein implicated in clathrin-mediated endocytosis. Nature 394, 793–797. doi:10.1038/29555
Cocucci, E., Aguet, F., Boulant, S., and Kirchhausen, T. (2012). The first five seconds in the Life of a clathrin-coated pit. Cell. 150, 495–507. doi:10.1016/j.cell.2012.05.047
Damke, H., Baba, T., Warnock, D. E., and Schmid, S. L. (1994). Induction of mutant dynamin specifically blocks endocytic coated vesicle formation. J. cell. Biol. 127, 915–934. doi:10.1083/jcb.127.4.915
Day, C. A., Baetz, N. W., Copeland, C. A., Kraft, L. J., Han, B., Tiwari, A., et al. (2015). Microtubule motors power plasma membrane tubulation in clathrin-independent endocytosis. Traffic (Cph., Den.) 16, 572–590. doi:10.1111/tra.12269
Engqvist-Goldstein, Å. E. Y., and Drubin, D. G. (2003). ACTIN assembly and endocytosis: from yeast to mammals. Annu. Rev. Cell. Dev. Biol. 19, 287–332. doi:10.1146/annurev.cellbio.19.111401.093127
Epp, E., Nazarova, E., Regan, H., Douglas, L. M., Konopka, J. B., Vogel, J., et al. (2013). Clathrin- and arp2/3-independent endocytosis in the fungal pathogen Candida albicans. mBio 4, 004766–e513. doi:10.1128/mbio.00476-13
Ferreira, A. P. A., and Boucrot, E. (2018). Mechanisms of carrier formation during clathrin-independent endocytosis. Trends Cell Biol. 28, 188–200. doi:10.1016/j.tcb.2017.11.004
Ford, M. G. J., Pearse, B. M. F., Higgins, M. K., Vallis, Y., Owen, D. J., Gibson, A., et al. (2001). Simultaneous binding of PtdIns(4,5)P2 and clathrin by AP180 in the nucleation of clathrin lattices on membranes. Science 291, 1051–1055. doi:10.1126/science.291.5506.1051
Fujii, M., Kawai, K., Egami, Y., and Araki, N. (2013). Dissecting the roles of Rac1 activation and deactivation in macropinocytosis using microscopic photo-manipulation. Sci. Rep. 3, 2385. doi:10.1038/srep02385
Gachet, Y., and Hyams, J. S. (2005). Endocytosis in fission yeast is spatially associated with the actin cytoskeleton during polarised cell growth and cytokinesis. J. Cell. Sci. 118, 4231–4242. doi:10.1242/jcs.02530
Glodowski, D. R., Chen, C. C.-H., Schaefer, H., Grant, B. D., and Rongo, C. (2007). RAB-10 regulates glutamate receptor recycling in a cholesterol-dependent endocytosis pathway. Mol. Biol. Cell. 18, 4387–4396. doi:10.1091/mbc.e07-05-0486
Goode, B. L., Eskin, J. A., and Wendland, B. (2015). Actin and endocytosis in budding yeast. Genetics 199, 315–358. doi:10.1534/genetics.112.145540
Grant, B. D., and Donaldson, J. G. (2009). Pathways and mechanisms of endocytic recycling. Nat. Rev. Mol. Cell. Biol. 10, 597–608. doi:10.1038/nrm2755
Gupta, G. D., Swetha, M. G., Kumari, S., Lakshminarayan, R., Dey, G., and Mayor, S. (2009). Analysis of endocytic pathways in Drosophila cells reveals a conserved role for GBF1 in internalization via GEECs. PLoS ONE 4, e6768. doi:10.1371/journal.pone.0006768
Hak, L. C. W., Khan, S., Meglio, I. D., Law, A.-L., Häsler, S. L.-A., Quintaneiro, L. M., et al. (2018). FBP17 and CIP4 recruit SHIP2 and lamellipodin to prime the plasma membrane for fast endophilin-mediated endocytosis. Nat. Cell. Biol. 20, 1023–1031. doi:10.1038/s41556-018-0146-8
Hansen, S. H., Sandvig, K., and Deurs, B. V. (1991). The preendosomal compartment comprises distinct coated and noncoated endocytic vesicle populations. J. cell. Biol. 113, 731–741. doi:10.1083/jcb.113.4.731
Hemalatha, A., Prabhakara, C., and Mayor, S. (2016). Endocytosis of Wingless via a dynamin-independent pathway is necessary for signaling in Drosophila wing discs. Proc. Natl. Acad. Sci. 113, E6993–E7002. doi:10.1073/pnas.1610565113
Henne, W. M., Boucrot, E., Meinecke, M., Evergren, E., Vallis, Y., Mittal, R., et al. (2010). FCHo proteins are nucleators of clathrin-mediated endocytosis. Science 328, 1281–1284. doi:10.1126/science.1188462
Howard, J. P., Hutton, J. L., Olson, J. M., and Payne, G. S. (2002). Sla1p serves as the targeting signal recognition factor for NPFX(1,2)D-mediated endocytosis. J. Cell. Biol. 157, 315–326. doi:10.1083/jcb.200110027
Imoto, Y., Raychaudhuri, S., Ma, Y., Fenske, P., Sandoval, E., Itoh, K., et al. (2022). Dynamin is primed at endocytic sites for ultrafast endocytosis. Neuron 110, 2815–2835.e13. doi:10.1016/j.neuron.2022.06.010
Jaumouillé, V., Cartagena-Rivera, A. X., and Waterman, C. M. (2019). Coupling of β2 integrins to actin by a mechanosensitive molecular clutch drives complement receptor-mediated phagocytosis. Nat. cell. Biol. 21, 1357–1369. doi:10.1038/s41556-019-0414-2
Kaksonen, M., and Roux, A. (2018). Mechanisms of clathrin-mediated endocytosis. Nat. Rev. Mol. Cell. Biol. 19, 313–326. doi:10.1038/nrm.2017.132
Kaksonen, M., Sun, Y., and Drubin, D. G. (2003). A pathway for association of receptors, adaptors, and actin during endocytic internalization. Cell. 115, 475–487. doi:10.1016/s0092-8674(03)00883-3
Kaksonen, M., Toret, C. P., and Drubin, D. G. (2005). A modular design for the clathrin- and actin-mediated endocytosis machinery. Cell. 123, 305–320. doi:10.1016/j.cell.2005.09.024
Kamioka, Y., Fukuhara, S., Sawa, H., Nagashima, K., Masuda, M., Matsuda, M., et al. (2004). A novel dynamin-associating molecule, formin-binding protein 17, induces tubular membrane invaginations and participates in endocytosis. J. Biol. Chem. 279, 40091–40099. doi:10.1074/jbc.m404899200
Kanaseki, T., and Kadota, K. (1969). The "vesicle in a basket". A morphological study of the coated vesicle isolated from the nerve endings of the Guinea pig brain, with special reference to the mechanism of membrane movements. J. Cell. Biol. 42, 202–220. doi:10.1083/jcb.42.1.202
Keen, J. H., Willingham, M. C., and Pastan, I. H. (1979). Clathrin-coated vesicles: isolation, dissociation and factor-dependent reassociation of clathrin baskets. Cell. 16, 303–312. doi:10.1016/0092-8674(79)90007-2
Khandelwal, P., Ruiz, W. G., and Apodaca, G. (2010). Compensatory endocytosis in bladder umbrella cells occurs through an integrin-regulated and RhoA- and dynamin-dependent pathway. EMBO J. 29, 1961–1975. doi:10.1038/emboj.2010.91
King, J. S., and Kay, R. R. (2019). The origins and evolution of macropinocytosis. Philos. Trans. R. Soc. B 374, 20180158. doi:10.1098/rstb.2018.0158
Kirkham, M., Fujita, A., Chadda, R., Nixon, S. J., Kurzchalia, T. V., Sharma, D. K., et al. (2005). Ultrastructural identification of uncoated caveolin-independent early endocytic vehicles. J. Cell. Biol. 168, 465–476. doi:10.1083/jcb.200407078
Kiss, A. L., and Botos, E. (2009). Endocytosis via caveolae: alternative pathway with distinct cellular compartments to avoid lysosomal degradation? J. Cell. Mol. Med. 13, 1228–1237. doi:10.1111/j.1582-4934.2009.00754.x
Kübler, E., and Riezman, H. (1993). Actin and fimbrin are required for the internalization step of endocytosis in yeast. EMBO J. 12, 2855–2862. doi:10.1002/j.1460-2075.1993.tb05947.x
Lamaze, C., Dujeancourt, A., Baba, T., Lo, C. G., Benmerah, A., and Dautry-Varsat, A. (2001). Interleukin 2 receptors and detergent-resistant membrane domains define a clathrin-independent endocytic pathway. Mol. Cell. 7, 661–671. doi:10.1016/s1097-2765(01)00212-x
Lemaigre, C., Ceuppens, A., Valades-Cruz, C. A., Ledoux, B., Vanbeneden, B., Hassan, M., et al. (2022). N-BAR and F-BAR proteins-endophilin-A3 and PSTPIP1-control clathrin-independent endocytosis of L1CAM. Traffic (Cph., Den.) 24, 190–212. doi:10.1111/tra.12883
Li, R., Liu, P., Wan, Y., Chen, T., Wang, Q., Mettbach, U., et al. (2012). A membrane microdomain-associated protein, Arabidopsis Flot1, is involved in a clathrin-independent endocytic pathway and is required for seedling development. Plant Cell. 24, 2105–2122. doi:10.1105/tpc.112.095695
Lundmark, R., Doherty, G. J., Howes, M. T., Cortese, K., Vallis, Y., Parton, R. G., et al. (2008). The GTPase-activating protein GRAF1 regulates the CLIC/GEEC endocytic pathway. Curr. Biol. 18, 1802–1808. doi:10.1016/j.cub.2008.10.044
Martzoukou, O., Amillis, S., Zervakou, A., Christoforidis, S., and Diallinas, G. (2017). The AP-2 complex has a specialized clathrin-independent role in apical endocytosis and polar growth in fungi. eLife 6, e20083. doi:10.7554/elife.20083
Moya, M., Dautry-Varsat, A., Goud, B., Louvard, D., and Boquet, P. (1985). Inhibition of coated pit formation in Hep2 cells blocks the cytotoxicity of diphtheria toxin but not that of ricin toxin. J. cell. Biol. 101, 548–559. doi:10.1083/jcb.101.2.548
Naslavsky, N., Weigert, R., and Donaldson, J. G. (2004). Characterization of a nonclathrin endocytic pathway: membrane cargo and lipid requirements. Mol. Biol. Cell. 15, 3542–3552. doi:10.1091/mbc.e04-02-0151
Newpher, T. M., Smith, R. P., Lemmon, V., and Lemmon, S. K. (2005). In vivo dynamics of clathrin and its adaptor-dependent recruitment to the actin-based endocytic machinery in yeast. Dev. Cell. 9, 87–98. doi:10.1016/j.devcel.2005.04.014
Oh, P., McIntosh, D. P., and Schnitzer, J. E. (1998). Dynamin at the neck of caveolae mediates their budding to form transport vesicles by GTP-driven fission from the plasma membrane of endothelium. J. Cell. Biol. 141, 101–114. doi:10.1083/jcb.141.1.101
Parton, R. G., Hanzal-Bayer, M., and Hancock, J. F. (2006). Biogenesis of caveolae: a structural model for caveolin-induced domain formation. J. Cell. Sci. 119, 787–796. doi:10.1242/jcs.02853
Payne, G. S., Baker, D., Tuinen, E. V., and Schekman, R. (1988). Protein transport to the vacuole and receptor-mediated endocytosis by clathrin heavy chain-deficient yeast. J. cell. Biol. 106, 1453–1461. doi:10.1083/jcb.106.5.1453
Pearse, B. M. F. (1975). Coated vesicles from pig brain: purification and biochemical characterization. J. Mol. Biol. 97, 93–98. doi:10.1016/s0022-2836(75)80024-6
Pelkmans, L., Puntener, D., and Helenius, A. (2002). Local actin polymerization and dynamin recruitment in SV40-induced internalization of caveolae. Science 296, 535–539. doi:10.1126/science.1069784
Prosser, D. C., Drivas, T. G., Maldonado-Báez, L., and Wendland, B. (2011). Existence of a novel clathrin-independent endocytic pathway in yeast that depends on Rho1 and formin. J. Cell. Biol. 195, 657–671. doi:10.1083/jcb.201104045
Prosser, D. C., Pannunzio, A. E., Brodsky, J. L., Thorner, J., Wendland, B., and O’Donnell, A. F. (2015). α-Arrestins participate in cargo selection for both clathrin-independent and clathrin-mediated endocytosis. J. Cell. Sci. 128, 4220–4234. doi:10.1242/jcs.175372
Qualmann, B., and Kelly, R. B. (2000). Syndapin isoforms participate in receptor-mediated endocytosis and actin organization. J. Cell Biol. 148, 1047–1062. doi:10.1083/jcb.148.5.1047
Renard, H.-F., Simunovic, M., Lemière, J., Boucrot, E., Garcia-Castillo, M. D., Arumugam, S., et al. (2015). Endophilin-A2 functions in membrane scission in clathrin-independent endocytosis. Nature 517, 493–496. doi:10.1038/nature14064
Renard, H.-F., Tyckaert, F., Giudice, C. L., Hirsch, T., Valades-Cruz, C. A., Lemaigre, C., et al. (2019). Endophilin-A3 and Galectin-8 control the clathrin-independent endocytosis of CD166. Nat. Commun. 11, 1457. doi:10.1038/s41467-020-15303-y
Römer, W., Pontani, L.-L., Sorre, B., Rentero, C., Berland, L., Chambon, V., et al. (2010). Actin dynamics drive membrane reorganization and scission in clathrin-independent endocytosis. Cell. 140, 540–553. doi:10.1016/j.cell.2010.01.010
Roth, T. F., and Porter, K. R. (1964). YOLK PROTEIN UPTAKE IN THE OOCYTE OF THE MOSQUITO AEDES AEGYPTI. L. J. Cell. Biol. 20, 313–332. doi:10.1083/jcb.20.2.313
Sabharanjak, S., Sharma, P., Parton, R. G., and Mayor, S. (2002). GPI-anchored proteins are delivered to recycling endosomes via a distinct cdc42-regulated, clathrin-independent pinocytic pathway. Dev. Cell. 2, 411–423. doi:10.1016/s1534-5807(02)00145-4
Sandvig, K., and Deurs, B. V. (1990). Selective modulation of the endocytic uptake of ricin and fluid phase markers without alteration in transferrin endocytosis. J. Biol. Chem. 265, 6382–6388. doi:10.1016/s0021-9258(19)39337-8
Sathe, M., Muthukrishnan, G., Rae, J., Disanza, A., Thattai, M., Scita, G., et al. (2018). Small GTPases and BAR domain proteins regulate branched actin polymerisation for clathrin and dynamin-independent endocytosis. Nat. Commun. 9, 1835. doi:10.1038/s41467-018-03955-w
Shajahan, A. N., Tiruppathi, C., Smrcka, A. V., Malik, A. B., and Minshall, R. D. (2004). Gbetagamma activation of Src induces caveolae-mediated endocytosis in endothelial cells. J. Biol. Chem. 279, 48055–48062. doi:10.1074/jbc.m405837200
Shi, A., Pant, S., Balklava, Z., Chen, C. C.-H., Figueroa, V., and Grant, B. D. (2007). A novel requirement for C. elegans alix/ALX-1 in RME-1-mediated membrane transport. Curr. Biol. 17, 1913–1924. doi:10.1016/j.cub.2007.10.045
Shimada, A., Niwa, H., Tsujita, K., Suetsugu, S., Nitta, K., Hanawa-Suetsugu, K., et al. (2007). Curved EFC/F-BAR-Domain dimers are joined end to end into a filament for membrane invagination in endocytosis. Cell. 129, 761–772. doi:10.1016/j.cell.2007.03.040
Soulet, F., Yarar, D., Leonard, M., and Schmid, S. L. (2005). SNX9 regulates dynamin assembly and is required for efficient clathrin-mediated endocytosis. Mol. Biol. Cell. 16, 2058–2067. doi:10.1091/mbc.e04-11-1016
Soykan, T., Kaempf, N., Sakaba, T., Vollweiter, D., Goerdeler, F., Puchkov, D., et al. (2017). Synaptic vesicle endocytosis occurs on multiple timescales and is mediated by formin-dependent actin assembly. Neuron 93, 854–866. doi:10.1016/j.neuron.2017.02.011
Sun, Y., Martin, A. C., and Drubin, D. G. (2006). Endocytic internalization in budding yeast requires coordinated actin nucleation and myosin motor activity. Dev. Cell. 11, 33–46. doi:10.1016/j.devcel.2006.05.008
Sverdlov, M., Shajahan, A. N., and Minshall, R. D. (2007). Tyrosine phosphorylation-dependence of caveolae-mediated endocytosis. J. Cell. Mol. Med. 11, 1239–1250. doi:10.1111/j.1582-4934.2007.00127.x
Takei, K., McPherson, P. S., Schmid, S. L., and De Camilli, P. (1995). Tubular membrane invaginations coated by dynamin rings are induced by GTP-γS in nerve terminals. Nature 374, 186–190. doi:10.1038/374186a0
Tauber, A. I. (2003). Metchnikoff and the phagocytosis theory. Nat. Rev. Mol. cell. Biol. 4, 897–901. doi:10.1038/nrm1244
Taylor, M. J., Perrais, D., and Merrifield, C. J. (2011). A high precision survey of the molecular dynamics of mammalian clathrin-mediated endocytosis. PLoS Biol. 9, e1000604. doi:10.1371/journal.pbio.1000604
Thottacherry, J. J., Kosmalska, A. J., Pradhan, S., Singh, P. P., Trepat, X., Vishwakarma, R., et al. (2019). Mechanochemical feedback control of dynamin independent endocytosis modulates membrane tension in adherent cells. Biophys. J. 116, 92a–93a. doi:10.1016/j.bpj.2018.11.542
Unanue, E. R., Ungewickell, E., and Branton, D. (1981). The binding of clathrin triskelions to membranes from coated vesicles. Cell. 26, 439–446. doi:10.1016/0092-8674(81)90213-0
Ungewickell, E., Ungewickell, H., Holstein, S. E. H., Lindner, R., Prasad, K., Barouch, W., et al. (1995). Role of auxilin in uncoating clathrin-coated vesicles. Nature 378, 632–635. doi:10.1038/378632a0
Veltman, D. M., Williams, T. D., Bloomfield, G., Chen, B.-C., Betzig, E., Insall, R. H., et al. (2016). A plasma membrane template for macropinocytic cups. eLife 5, e20085. doi:10.7554/elife.20085
Vorselen, D., Labitigan, R. L. D., and Theriot, J. A. (2020). A mechanical perspective on phagocytic cup formation. Curr. Opin. Cell. Biol. 66, 112–122. doi:10.1016/j.ceb.2020.05.011
Watanabe, S., Liu, Q., Davis, M. W., Hollopeter, G., Thomas, N., Jorgensen, N. B., et al. (2013). Ultrafast endocytosis at Caenorhabditis elegans neuromuscular junctions. eLife 2, e00723. doi:10.7554/elife.00723
Watanabe, S., Mamer, L. E., Raychaudhuri, S., Luvsanjav, D., Eisen, J., Trimbuch, T., et al. (2018). Synaptojanin and endophilin mediate neck formation during ultrafast endocytosis. Neuron 98, 1184–1197. doi:10.1016/j.neuron.2018.06.005
Windler, S. L., and Bilder, D. (2010). Endocytic internalization routes required for delta/notch signaling. Curr. Biol. 20, 538–543. doi:10.1016/j.cub.2010.01.049
Woodard, T. K., Rioux, D. J., and Prosser, D. C. (2023). Actin- and microtubule-based motors contribute to clathrin-independent endocytosis in yeast. Mol. Biol. Cell. 34, ar117. doi:10.1091/mbc.e23-05-0164
Yarar, D., Waterman-Storer, C. M., and Schmid, S. L. (2005). A dynamic actin cytoskeleton functions at multiple stages of clathrin-mediated endocytosis. Mol. Biol. Cell. 16, 964–975. doi:10.1091/mbc.e04-09-0774
Yu, Y., and Yoshimura, S. H. (2022). Self-assembly of CIP4 drives actin-mediated asymmetric pit-closing in clathrin-mediated endocytosis. Nat. Commun. 14, 4602. doi:10.1038/s41467-023-40390-y
Keywords: endocytosis, clathrin-independent, clathrin, model organism, actin, small GTPase
Citation: Rioux DJ and Prosser DC (2023) A CIE change in our understanding of endocytic mechanisms. Front. Cell Dev. Biol. 11:1334798. doi: 10.3389/fcell.2023.1334798
Received: 07 November 2023; Accepted: 04 December 2023;
Published: 13 December 2023.
Edited by:
Mitsuo Tagaya, Tokyo University of Pharmacy and Life Sciences, JapanReviewed by:
Wen Xiong, Baylor College of Medicine, United StatesCopyright © 2023 Rioux and Prosser. This is an open-access article distributed under the terms of the Creative Commons Attribution License (CC BY). The use, distribution or reproduction in other forums is permitted, provided the original author(s) and the copyright owner(s) are credited and that the original publication in this journal is cited, in accordance with accepted academic practice. No use, distribution or reproduction is permitted which does not comply with these terms.
*Correspondence: Derek C. Prosser, ZHByb3NzZXJAdmN1LmVkdQ==
Disclaimer: All claims expressed in this article are solely those of the authors and do not necessarily represent those of their affiliated organizations, or those of the publisher, the editors and the reviewers. Any product that may be evaluated in this article or claim that may be made by its manufacturer is not guaranteed or endorsed by the publisher.
Research integrity at Frontiers
Learn more about the work of our research integrity team to safeguard the quality of each article we publish.