- 1Jilin Provincial Key Laboratory of Animal Model, College of Animal Science, Jilin University, Changchun, China
- 2Animal Genome Editing Technology Innovation Center, College of Animal Science, Jilin University, Changchun, China
- 3Department of Animal Science, Division of Applied Life Science (BK21 Four), Gyeongsang National University, Jinju, Republic of Korea
Introduction: Methoxychlor (MXC) is an organochlorine pesticide (OCP) that was formerly used worldwide as an insecticide against pests and mosquitoes. However, MXC is not biodegradable and has lipophilic characteristics; thus, it accumulates in organisms and affects reproductive function. MXC, as an estrogenic compound, promotes oxidative stress, induces oxidative stress damage to ovarian follicles, and causes miscarriages and stillbirths in females. In this research endeavor, our primary objective was to explore the ramifications of MXC regarding the developmental processes occurring during the initial stages of embryogenesis in pigs.
Methods: In this study, we counted the blastocyst rate of early embryos cultured in vitro. We also examined the reactive oxygen species level, glutathione level, mitochondrial membrane potential, mitochondrial copy number and ATP level in four-cell stage embryos. Finally, apoptosis and DNA damage in blastocyst cells, as well as pluripotency-related and apoptosis-related genes in blastocyst cells were detected. The above experiments were used to evaluate the changes of MXC damage on early parthenogenetic embryo development.
Results and Discussion: The results showed that early embryos exposed to MXC had a significantly lower cleavage rate, blastocyst rate, hatching rate, and total cell count compared with the control group. It was also of note that MXC not only increased the levels of reactive oxygen species (ROS), but also decreased the mitochondrial membrane potential (ΔΨm) and mitochondrial copy number during the development of early embryos. In addition, after MXC treatment, blastocyst apoptosis and DNA damage were increased, decreased cell proliferation, and the expression of pluripotency-related genes SOX2, NANOG, and OCT4 was down-regulated, while the expression of apoptosis-related genes BAX/BCL-2 and Caspase9 was up-regulated. Our results clearly show that MXC can have deleterious effects on the developmental processes of early porcine embryos, establishing the toxicity of MXC to the reproductive system. In addition, the study of this toxic effect may lead to greater concern about pesticide residues in humans and the use of safer pesticides, thus potentially preventing physiological diseases caused by chemical exposure.
1 Introduction
Organochlorine pesticides (OCPs) constitute a category of persistent organic pollutants (POPs) that can persist for long periods and be transported over long distances during the degradation process, and can also result in the accumulation of toxic chemical substances in animals and humans (Zhang et al., 2003; Maskaoui et al., 2005; Ali et al., 2014). The accumulation of OCPs in the tissues of organisms positioned at higher trophic levels in the food chain surpasses that observed in organisms occupying lower trophic levels (Snedeker, 2001; El-Shahawi et al., 2010).
OCPs may have adverse effects on the endocrine system and reproduction (Safe, 2004; Toft et al., 2004; Bretveld et al., 2006; Li et al., 2008). China, as a major agricultural country, employed widespread use of dichlorodiphenyltrichloroethane (DDT) between 1940 and 1980. Since 1983, DDT has been banned in China and there has been an increase in the use of methoxychlor (MXC), which is an alternative to DDT. However, MXC has been banned in developed countries, although it is still used in some developing countries. However, in recent years, the toxic effects of MXC and its metabolites have been discovered; as previously reported, in utero exposure to high doses of MXC could decrease the levels of testosterone in rat fetuses, consequently leading to malformations of the genital tract in male offspring (Liu et al., 2016a). MXC and its metabolites also inhibit steroidogenesis by decreasing the activity of rat neurosteroidogenic 3α-hydroxysteroid de-hydrogenase (AKR1C14) and retinol dehydrogenase 2 (RDH2) (Mao et al., 2018). MXC causes oxidative stress in adult rat spermatozoa, reduces sperm viability (Aly and Azhar, 2013), and induces testicular cell apoptosis via mitochondrial and FasL-mediated pathways in testicular cells (Vaithinathan et al., 2010).
In addition to its toxicity to the male reproductive system, MXC has an even greater effect on the female reproductive system because it is an insecticide with estrogen-like activity. MXC induces oxidative stress both in vivo and in vitro within the oocyte, impairing mitochondrial respiration and inducing oxidative damage to proteins and DNA. When exposed to MXC in an aqueous environment, the hepatopancreas and ovarian tissues of the Chinese mitten crab (Eriocheir sinensis) were seriously damaged, with the ovary identified as the target organ of MXC (Cheng et al., 2019). MXC causes a significant increase in the proportion of atretic sinus follicles in adult female mice (Paulose et al., 2012). Within the human environment, the analysis of follicular fluid from 127 infertile women in Hubei Province, China, revealed the presence of seventeen distinct types of OCPs. Remarkably, 13.4% of these detected OCPs featured MXC as the primary compound, with an average concentration of 167.9 ± 33.9 ng/g lipid weight (lw) (Zhu et al., 2015).
The effects of oxidative stress on embryonic development are critical. The addition of several exogenous substances may lead to the generation of reactive oxygen species (ROS) (Ghosh et al., 2012), which will make the oxidant antioxidant level in the cell unbalanced and produce oxidative stress. Cell biomolecules suffer severe oxidative damage and may pass through mitochondria-dependent and mitochondria-independent pathways, causing cell apoptosis (Sinha et al., 2013). Many pesticides have been evaluated for reproductive toxicity risk in laboratory animals (Koç et al., 2009; Bhardwaj and Saraf, 2014). Some of these pesticides can cause oxidative stress in reproductive tissues, leading to ovarian follicle atresia (Symonds et al., 2008; Koç et al., 2009; Banerjee et al., 2014). Adding MXC can induce oxidative stress in mouse ovarian follicles, leading to slow growth and atresia of the follicles (Gupta et al., 2006a). At the same time, MXC has the potential to induce mitochondrial dysfunction and provoke oxidative damage within the mouse ovaries (Gupta et al., 2006b). MXC can induce oxidative stress in rat granulosa cells to induce apoptosis, which leads to follicular atresia. Follicular atresia has a bad influence on female reproductive function (Bhardwaj and Saraf, 2017). The IGF signaling pathway could potentially play a role in mediating MXC-induced disturbances in ovarian function and fertility (Ozden-Akkaya et al., 2017).
The primary cause of human female reproductive toxicity induced by MXC predominantly stems from the induction of oxidative stress, with the ovary being identified as the target organ. However, the impact of MXC on early mammalian embryos is not clear. Therefore, this work aims to directly verify the hypothesis that MXC induces porcine embryo development reduction through the oxidative stress pathway. In this study, the toxic effects of MXC-induced oxidative stress were studied by quantifying ROS levels and mitochondrial membrane potential.
2 Materials and methods
All reagents, unless otherwise specified, were procured from Sigma Aldrich (St. Louis, Mo, United States).
2.1 Collection of oocytes and cultivation of early embryos
Ovaries were obtained from a slaughterhouse, preserved in a saline solution containing penicillin-streptomycin at 37°C, and promptly transported to the laboratory within a 2-h timeframe by the designated staff. The ovaries were washed two or three times with 0.9% saline at 37°C and then placed into a water bath at 37°C. Follicles of 3–8 mm were obtained by manually pressing the ovaries, creating negative pressure, and inserting a 10 mL syringe with a No. 12 needle on one side. The fluid extracted from the follicles was kept in a 10 mL centrifuge tube and was allowed to precipitate for 10 min. The supernatant was removed using a Pasteur pipette, and the rest of the liquid was cleaned three times with HEPES, by mixing it in the tube and precipitating for 10 min. The oocytes in HEPES were placed in a culture dish. The oocytes with 2-3 layers of cumulus cells were viewed under a stereomicroscope with a hot plate (37°C) and selected using a pipette.
Oocytes were matured in vitro by placement in a 4-well dish. To each well, 600 μL of growth medium and 50 oocytes were added and then covered with mineral oil. M199 medium was chosen as the basal culture medium with 10% porcine follicular fluid, 0.01 mg/mL sodium pyruvate, 0.01 μg/mL EGF, also containing PMSG (10 IU/mL) and HCG (10 IU/mL). The oocytes were cultured for a duration of 44 h within a CO2 incubator set at 38.5°C, under an atmosphere consisting of 5% CO2, 95% air, and a humidity level of 95%.
The cumulus cells of mature oocytes were removed with 0.1% hyaluronidase. The electroactivation of oocytes with the first polar body was performed in order to produce a parthenogenetic embryo. They were kept in 7.5 μg/mL cytochalasin B for 3 h to inhibit the draining of the second polar body. The activated oocytes were transferred to a culture medium (PZM-5) containing different concentrations of MXC. The embryos were then cultivated in a CO2 incubator at 38.5°C in an atmosphere of 5% CO2, 95% air, and 95% humidity. The oocyte cleavage rate was counted 30 h after activation, and hatching rates were counted after 8 days of incubation. The blastocysts were collected for experiments on day 7.
2.2 Treatment with MXC
A stock solution containing 500 μM MXC in DMSO was initially prepared. Subsequently, this solution was further diluted with IVC to yield concentrations of 50 μM, 100 μM, 200 μM, and 500 μM, while maintaining a final DMSO concentration of 1/1,000. These solutions corresponded to four groups, three of which were experimental groups with different concentrations of MXC, and one which was a control group containing 1/1,000 of DMSO. Each experimental group consisted of an average of 50 oocytes. The experiment was replicated three times.
2.3 Counting the number of cells in blastocysts
For the purpose of blastocyst cell counting, day 7 blastocysts were gathered from each experimental group. These collected blastocysts were subsequently immersed in a 4% paraformaldehyde solution at room temperature for a duration of 30 min. Following this fixation step, the blastocysts were subjected to three successive washes using PBS-PVA (0.1% PVA). The blastocysts were then transferred to PBS-PVA (0.1% PVA) containing 0.1% TritonX-100 and left to stand for 20 min. Ultimately, the blastocysts underwent an additional three washes with PBS-PVA. Subsequently, they were incubated in a solution containing 5 ng/mL Hoechst33342 for 10 min at room temperature. After the incubation, the blastocysts were carefully transferred onto slides, sealed using an anti-fluorescence quencher, and subjected to visualization under a fluorescence microscope. The counting of cells within the blastocysts was conducted with the aid of ImageJ software.
2.4 ROS and GSH assay
Embryos at the 4-cell stage were chosen for further analysis. These embryos underwent a thorough washing process, involving three cycles of rinsing with PBS/PVA. Subsequently, they were subjected to incubation with a ROS detection dye obtained from Invitrogen (NY, United States), along with 10 mM 2′,7′-dichlorofluorescein diacetate (DCFH) sourced from the same manufacturer. After incubation in the dark at 38°C for 30 min, the embryos were imaged using a fluorescence microscope with a digital camera (green fluorescence, UV filters, 490 nm). The embryos were immersed in a GSH dye solution containing 10 mM 4-chloromethyl-6,8-difluoro-7-hydroxycoumarin, which was obtained from Invitrogen (NY, United States). Subsequently, they were incubated at a temperature of 38°C for a duration of 30 min. Images were captured using appropriate filters, including blue fluorescence and UV filters with a wavelength of 370 nm. Subsequently, ImageJ software was employed to quantitatively assess the fluorescence intensity of both ROS and GSH.
2.5 Mitochondrial membrane potential assay
The 4-cell stage embryos underwent a thorough washing procedure, involving three rinses with PBS/PVA. Subsequently, they were subjected to incubation with JC-1 dye (5,5′,6,6′-tetrachloro-1,1′,3,3′-tetraethylbenzimidazolocarbocyanine iodide) sourced from Beyotime, China, at a temperature of 38°C for a duration of 30 min. Following this incubation, the embryos were once again washed with PBS/PVA. Images capturing the red and green fluorescence signals were acquired through the use of a fluorescence microscope equipped with a digital camera. The quantification of the ratio between red fluorescence intensity (representing JC-1 aggregates and activated mitochondria) and green fluorescence intensity (corresponding to JC-1 monomers and inactive mitochondria) was conducted using ImageJ software.
2.6 TUNEL assay
The day 7 blastocysts were washed three times with PBS/PVA, fixed in 4% paraformaldehyde at room temperature for 30 min, and then washed again three times with PBS/PVA. After washing, the embryos were transferred to a solution of PBS/PVA containing 0.5% Triton X-100 and permeabilized for 30 min, washed again three times with PBS/PVA, and then incubated in TUNEL dye in the dark for 60 min (In Situ Cell Death Detection Kit; Roche, Mannheim, Germany). The embryos were stained with 10 ng/mL Hoechst 33342 for a duration of 10 min, followed by three sequential washes with PBS/PVA. Subsequently, they were affixed to microscope slides and subjected to observation using a fluorescence microscope.
2.7 EDU cell proliferation stain
Day 6 blastocysts are proliferation stained (38.5°C, 5% CO2) using EDU proliferation dye (Beyotime, C0071S). At the end of staining the nucleus was stained using Hoechst 33,342 (5 ng/mL) (Beyotime, C1022) and incubated for 10 min in the dark room, after which the blastocysts were aspirated and washed, sealed with slides and coverslips, photographed using a fluorescence microscope (E179168; Nikon). Amounts of proliferating cells were counted using NIH ImageJ software.
2.8 Immunofluorescence staining
Blastocysts were washed 3 times in PBS-PVA and fixed in 4% paraformaldehyde for 30 min after washing. After fixation, they were washed with a solution of PBS containing 0.05% Tween20 (PBST) and treated with 0.1% TritonX-100 at room temperature for 20 min. Blastocysts were washed with PBST three times for 5 min, and immediately after washing, they were treated with 0.1 M, PH = 8 Tris-Hcl for 15 min. Wash again with PBST and close the solution with 2% BSA in PBST for 1 h. Transfer the blastocysts into antibody containing γ-H2AX (2% BSA preparation) overnight at 4 °C. Wash the blastocysts three times with PBST for 5 min and incubate with the secondary antibody (Abbkine, A23420) for 1 h at room temperature. The blastocysts were washed with PBST for 5 min each and incubated with Hoechst 33,342 (5 ng/mL) for 10 min. Finally, the blastocysts were transferred to antifade mounting medium (Abbkine, BMU104-CN) on a slide. Photographs (TIFF format) were taken using a fluorescence microscope (E179168; Nikon), and the NIH ImageJ software was used to analyze and calculate the fluorescence intensity.
2.9 Mitochondrial copy number assay
Sixty stage 4-cell stage embryos were collected and DNA was extracted using a DNA extraction kit (QIAGEN, Germany, 80284) according to the instructions. Fluorescence quantification was employed to detect the mitochondrial gene ND1. Simultaneously, the expression levels of the nuclear single-copy gene GCG were measured. To determine the mitochondrial copy number, the relative concentrations of ND1 and GCG were calculated. Specific primer sequences (Table 1) were designed using the National Center of Biotechnology Information (NCBI) database. The specific calculation formula is as follows: N = 2−Ctmt/2−Ctn
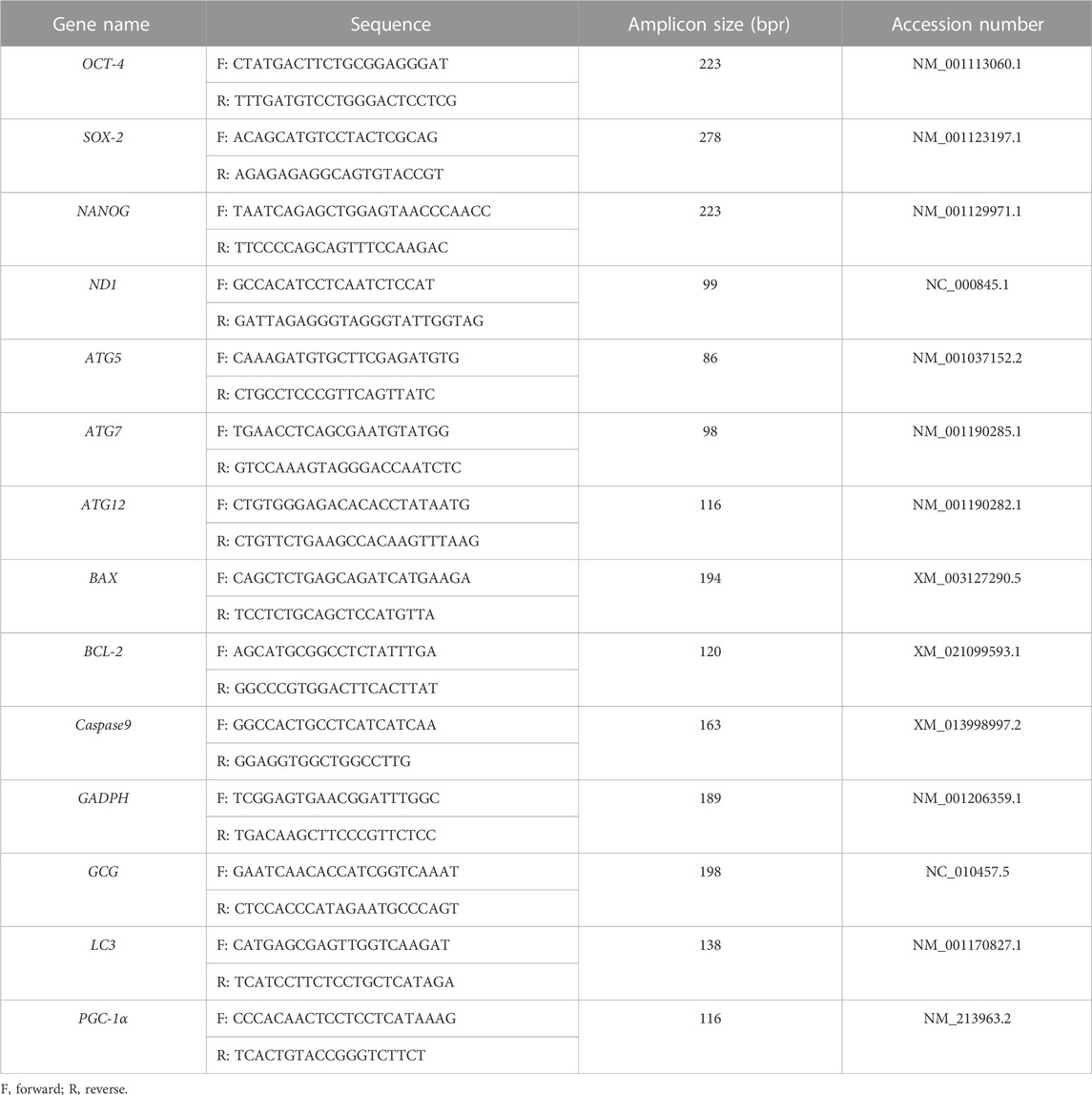
TABLE 1. The names of the tested genes, sequences of primers, sizes of PCR products, and accession numbers for RT-qPCR experiments.
N: mtDNA copy number; Ctmt: Ct values for mitochondrial genes; Ctn: Ct values for nuclear single-copy genes.
2.10 ATP level detection
ATP levels in 4-cell stage embryos were measured using an ATP assay kit (Beyotime, S0027), using 50 4-cell stage embryos in each group. Measurements were made using ELISA reader (TECAN, Infinite M200, pro).
2.11 Quantitative RT-PCR (qRT-PCR)
Blastocysts were collected on day 7, and the extraction of RNA was carried out using an RNA mini kit (Qiagen, Germany, 80284), following the protocol outlined in the provided manual. mRNA was collected from each group of 60 blastocysts. Complementary DNA (cDNA) was synthesized through the reverse transcription of mRNA, employing a reverse transcription kit (Tiangen, China). Quantitative real-time PCR was carried out utilizing a fluorescent quantitative assay kit (Tiangen, China). The PCR protocol involved an initial heating step at 95°C for 5 min, followed by 40 cycles of denaturation at 95°C for 15 s, annealing at 60°C for 25 s, and extension at 72°C for 20 s. The final extension steps were performed at 72°C. The target genes were NANOG, OCT4, SOX2, BCL-2, BAX and Caspase9. The primer sequences utilized in this investigation are detailed in Table 1.
2.12 Statistical analysis
In this study, all experiments were independently repeated three times. Statistical analysis was performed using GraphPad Prism 9 software (GraphPad Software, San Diego, CA, United States). The assessment of fluorescence intensities was conducted using ImageJ software (National Institutes of Health in Bethesda, MD, United States). The normality of the data was assessed using the Shapiro-Wilk test, and outliers exceeding ±2 times the standard error of the mean (SEM) were excluded. Experimental data were compared using Student’s t-test, with each group compared to the control group. p < 0.05 is considered statistically significant and the results were presented as mean ± SEM.
3 Results
3.1 MXC inhibited early embryonic development in pigs
After parthenogenetic activation, the oocytes were placed in IVC medium containing different concentrations of MXC. We examined the blastocyst rates and the hatching rates of embryos exposed to different concentrations of MXC (control group, 50 μM, 100 μM, 200 μM, and 500 μM MXC). The blastocyst rates were significantly different among groups (58.25% ± 2.83%, 43.65% ± 1.28%, 40.10% ± 1.01%, 35.49% ± 2.57%, 9.94% ± 1.95%, p < 0.05, Figure 1A). Compared with other concentrations, 200 μM and 500 μM MXC significantly reduced the hatching rate (21.68% ± 3.57%, 20.12% ± 3.07%, 18.29% ± 1.28%, 8.18% ± 1.09%, 1.31% ± 0.85%, p < 0.05, Figure 1B). Compared with those treated with 200 μM MXC, almost no early pig embryos treated with 500 μM MXC demonstrated development. Hence, 200 μM MXC was selected for the subsequent experiments (Figure 1C). The exposure of parthenogenetic embryos to 200 μM MXC significantly reduced the cleavage rate to lower than in the control group (92.30 ± 1.06% vs. 80.11% ± 1.02%, p < 0.05, Figure 1D). The rate of blastocyst was notably lower when compared to that observed in the control group (58.93 ± 2.49% vs. 37.57% ± 3.00%, p < 0.05, Figure 1E). The hatching rate was also significantly lower than that in the control group (20.44 ± 3.26% vs. 7.29% ± 1.27%, p < 0.05, Figure 1F). Therefore, 200 μM MXC was shown to result in significant inhibition of early embryonic development.
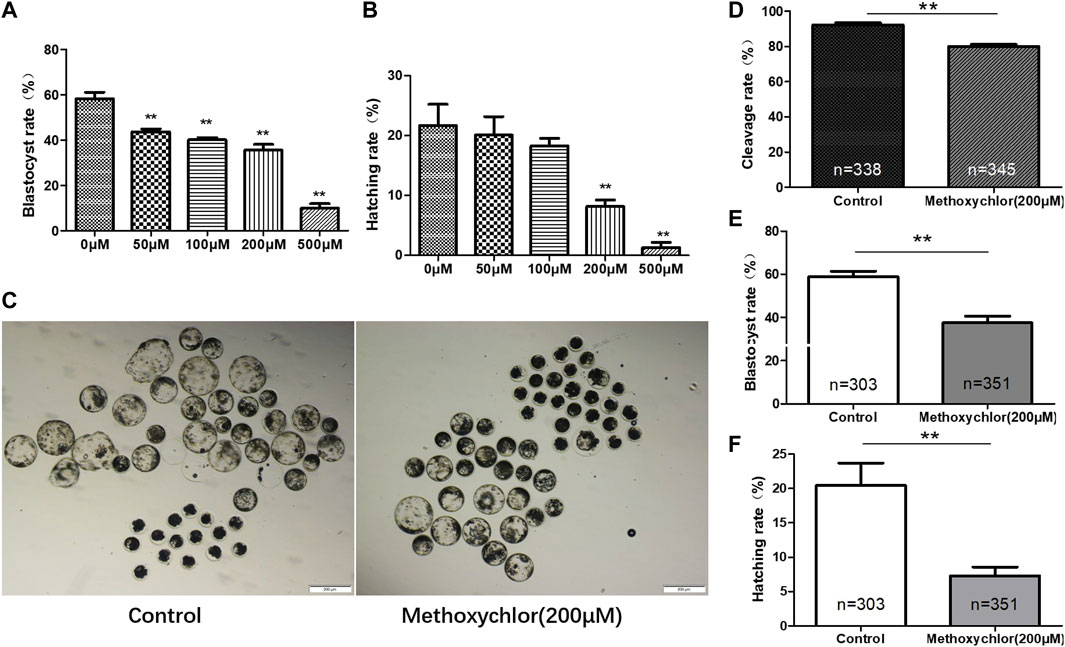
FIGURE 1. Impact of MXC on parthenogenetic pig embryos. The data from each group were compared only to the control group. (A) The blastocyst rates of early pig embryos at different concentrations of MXC (magnified 40 times, scale bar = 200 µm). (B) The hatching rates of early pig embryos exposed to different concentrations of MXC. (C) Blastocyst formation in the control group and the 200 μM MXC group. (D) Cleavage rates in the control group and the 200 μM MXC group. (E) Blastocyst rate in the control group and the 200 μM MXC group. (F) Hatching rate in the control group and the 200 μM MXC group. They were expressed as mean ± SEM, significance difference is denoted by **p < 0.01.
3.2 MXC induced oxidative stress and increased ROS levels in embryos
Changes in reactive oxygen species (ROS) can have a significant impact on oocytes, and an excess of ROS can lead to damage in oocytes (Agarwal and Allamaneni, 2004). Glutathione (GSH), an essential antioxidant molecule involved in the removal of ROS, has been found to protect oocytes from oxidative stress (Barros et al., 2019). The excessive production of ROS can result in oxidative damage to various macromolecules, including lipids, proteins, and DNA. To determine if MXC induced ROS production, the formation of ROS in 4-cell stage embryos treated with MXC was examined using dichlorodihydrofluorescein diacetate (DCFH-DA). As illustrated in Figure 2, the relative fluorescence intensity of ROS in embryos subjected to MXC treatment exhibited a notable increase compared to that of the control group at the 4-cell stage. (1.00 ± 0.05, n = 94 vs. 1.15 ± 0.03, n = 86, p < 0.01, Figures 2A, B). In the subsequent analysis of fluorescence intensity, the glutathione (GSH) fluorescence intensity in embryos treated with MXC was lower than that of the control group (1.00 ± 0.01, n = 94 vs. 0.71 ± 0.02, n = 86, p < 0.05, Figures 2C, D). The results showed that MXC could increase oxidative stress in early pig embryos.
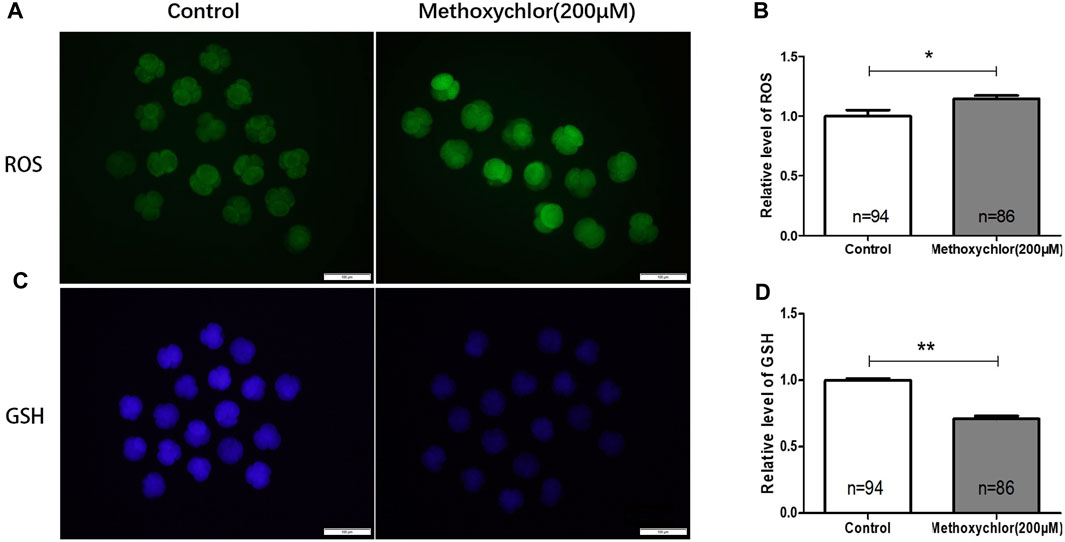
FIGURE 2. The influence of MXC on the levels of ROS and GSH in early pig embryos. (A) The ROS fluorescence intensity in 4-cell stage early embryos (magnified 100 times, scale bar = 100 µm). (B) The relative levels of ROS in 4-cell stage embryos. A significant difference is denoted by * (p < 0.05). (C) The GSH fluorescence intensity in 4-cell stage early embryos (magnified 100 times, scale bar = 100 µm). (D) The relative levels of GSH in 4-cell stage embryos. A highly significant difference is denoted by **(p < 0.01). They were expressed as mean ± SEM, significant difference is denoted by *(p < 0.05), **(p < 0.01).
3.3 Impact of MXC on mitochondrial function during early embryonic development in pigs
Mitochondria provide the energy required for early embryonic development, and are thus essential. The mitochondrial membrane potential stands as one of the foremost critical indicators used to assess mitochondrial function. To clarify the mechanism by which MXC affected early embryonic development in pigs, the ΔΨm of the 4-cell stage embryos was measured. ΔΨm is shown in Figure 3. As revealed through JC-1 staining, it became evident that embryos exposed to 200 mM MXC exhibited a significantly reduced ΔΨm in comparison to the control group. This decline in ΔΨm signifies a disturbance in mitochondrial function (3.15 ± 0.17, n = 122 vs. 2.35 ± 0.12, n = 88, p < 0.01, Figures 3A, B). We then examined the mitochondrial copy number in 4-cell stage embryos and found that the mitochondrial copy number was significantly reduced in the MXC-treated group (1.00 ± 0.035 vs. 0.764 ± 0.036, p < 0.01, Figure 3C). Mitochondria are an important site of ATP synthesis, and a large amount of ATP is required for embryo development. Our assay of ATP levels in embryos at the 4-cell stage showed that MXC reduced ATP content during embryo development (1.00 ± 0.021, vs. 0.743 ± 0.030, n = 50, p < 0.01, Figure 3D). The expression of the mitochondria-related gene PGC-1α was examined and found to be significantly reduced by MXC treatment (Figure 3E). The above results suggest that MXC caused mitochondrial dysfunction during early embryonic development.
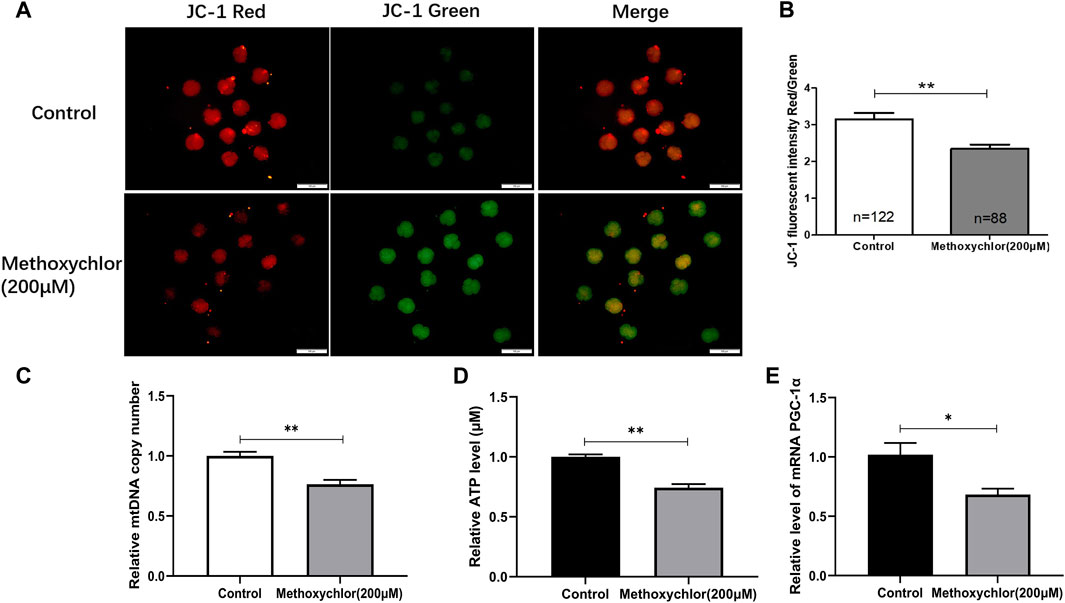
FIGURE 3. MXC may induce mitochondrial dysfunction in 4-cell stage early embryos. (A) Embryos at the 4-cell stage subjected to JC-1 staining (magnified 100 times, scale bar = 100 µm). (B) The relative levels of JC-1 fluorescence intensity. (C) Relative copy number of mitochondria in 4-cell stage embryos (n = 80). (D) Relative ATP level in 4-cell stage embryos (n = 50). (E) Relative expression of mitochondria-related gene PGC-1α in 4-cell stage embryos. They were expressed as mean ± SEM, significant is denoted by *(p < 0.05), **(p < 0.01).
3.4 Impact of MXC on apoptosis in early pig embryos
To confirm the negative impact of MXC on the embryos, the apoptosis of MXC-treated blastocyst cells was examined using the TUNEL assay. As shown in Figure 4, there was a greater extent of cellular apoptosis in the group treated with MXC (5.4 ± 0.48, n = 75 vs. 10.26 ± 1.07, n = 67, p < 0.01, Figures 4A, B). At the same time, the number of blastocyst cell was also reduced (53.61 ± 2.43, n = 75 vs. 40.96 ± 2.39, n = 67, p < 0.01, Figure 4C). We also examined the expression of apoptosis-related genes BAX, BCL-2 and Caspase9 in blastocyst cells and found that the expression of apoptosis-related genes was increased in the MXC-treated group (Figure 4D). Autophagy plays a role in cells to remove damaged cells and maintain cellular homeostasis and embryonic development (Mizushima and Komatsu, 2011). We subsequently examined the expression of autophagy-related genes in blastocyst cells to investigate whether the increase in the level of apoptosis in blastocyst cells led to an increase in the level of autophagy, and the results showed that no significant changes in the expression of autophagy-related genes were detected in blastocyst cells after MXC treatment (Figure 4E). The results show that treating embryos with MXC increases the level of apoptosis but autophagy is not activated.
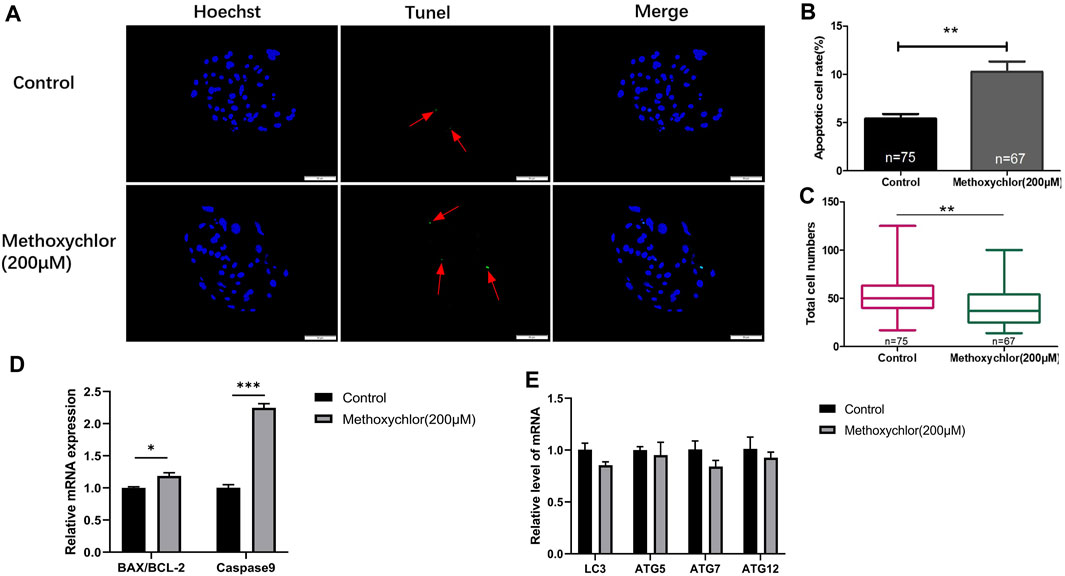
FIGURE 4. Impact of MXC on apoptosis. (A) Blastocysts on Day 6 dyed stained with Hoechst 33342 and TUNEL (magnified 200 times, scale bar = 50 µm). The arrows point to the nucleus that were stained positively for apoptosis. (B) The apoptotic blastocyst cell rates in the control group and the MXC group. (C) The total cell numbers of the control group and the MXC group on Day 6. (D) Relative expression of apoptosis-related genes BAX/BCL-2 and Caspase9. (E) Relative expression of autophagy-related gene expression LC3, ATG5, ATG7 and ATG12. They were expressed as mean ± SEM, significant is denoted by *(p < 0.05), **(p < 0.01), ***(p < 0.001).
3.5 Impact of MXC on DNA damage in early porcine embryos
To determine the effect of MXC on DNA damage in blastocyst cells. We examined DNA damage in blastocyst cells by immunofluorescence staining using the DNA break marker γH2AX. We found a significant increase in the number of DNA breaks produced by cells in the MXC-treated group (12.79% ± 1.79%, n = 25 vs. 25.89% ± 4.47%, n = 27, p < 0.01, Figures 5A, B). The results showed that MXC increased DNA damage in blastocyst cells.
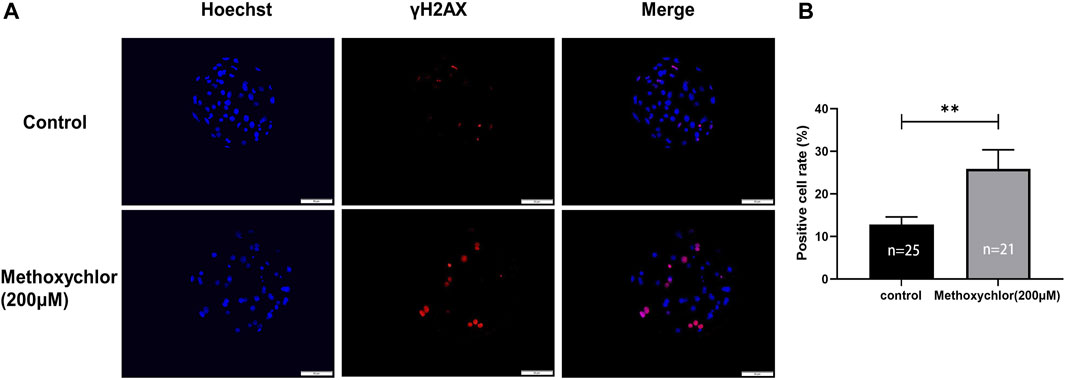
FIGURE 5. Impact of MXC on DNA damage. (A) Representative fluorescence images of day seven blastocyst γH2AX immunofluorescence staining (magnified 200 times, scale bar = 50 µm). (B) Ratio of the number of cells producing DNA breaks in blastocyst cells. They were expressed as mean ± SEM, significant differences are indicated by **(p < 0.01).
3.6 Impact of MXC on the proliferative capacity of porcine embryos
To determine whether MXC affects the proliferative capacity of early parthenogenetic embryos, we have stained day six blastocysts for EDU cell proliferation. In the MXC group, a significant reduction in the number of proliferating cells was observed compared to the control group (47.334 ± 8.474, n = 15 vs. 30.777 ± 6.096, n = 12, p < 0.01, Figures 6A, B). We then examined day seven blastocysts the expression of totipotency-related genes NANOG, SOX2 and OCT4. The results showed that MXC treatment affected the expression of pluripotency genes in early embryos (Figure 6C). The results indicated that MXC reduced the proliferative capacity of early parthenogenetic embryos and affected the quality of embryonic development.
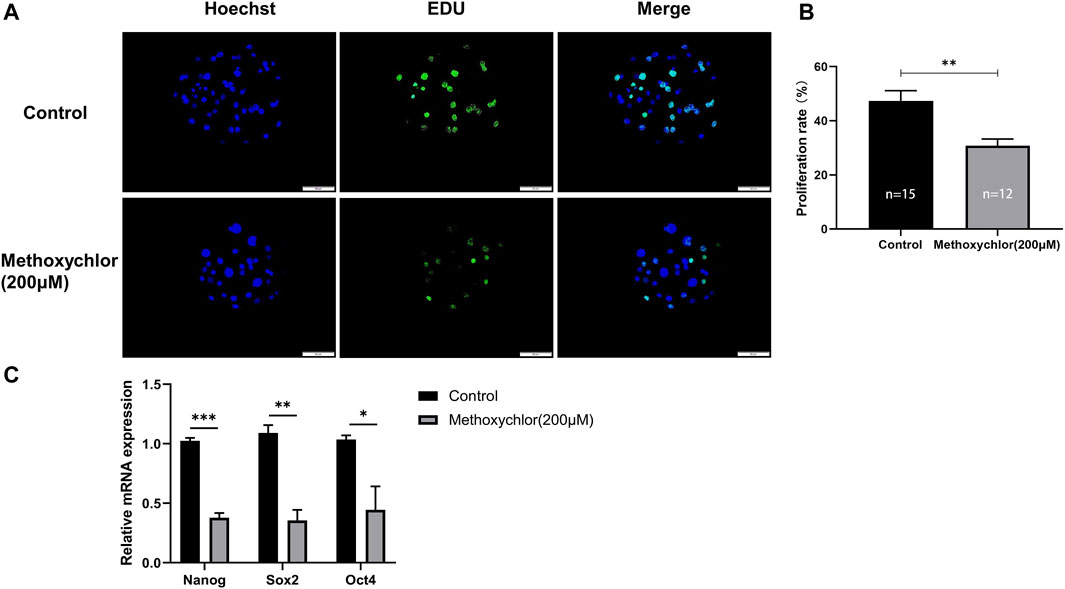
FIGURE 6. Impact of MXC on the proliferative capacity of embryos. (A) Representative fluorescence images of day six blastocyst EDU staining (magnified 200 times, scale bar = 50 µm). (B) Proliferation rate of blastocyst cells in each group. (C) Pluripotency-related gene expression in day seven blastocysts in control and MXC (200 μM) group (n = 60 per group). They were expressed as mean ± SEM, significant difference is denoted by *(p < 0.05), **(p < 0.01), ***(p < 0.001).
4 Discussion
In the past few years, food security and environmental pollution have emerged as two of the most popular research topics related to human health and are future challenges facing the Earth. Aside from workers in the agricultural industry, the general public may also be exposed to OCPs through various routes, including food and water. OCPs have been identified in various environmental compartments such as air, soil, water, and sediment, as well as in commonly consumed items such as food, vegetables, fish, and poultry. Furthermore, these compounds have also been found in biological samples including blood, adipose tissue, breastmilk, and cord blood within the general population in China (Nakata et al., 2002; Qiu et al., 2004; Nakata et al., 2005; Poon et al., 2005; Yang et al., 2005; Yang et al., 2006; Zhou et al., 2006; Wang et al., 2007; Tao et al., 2009; Luo et al., 2016). MXC-induced oxidative changes also occur in other reproductive organs (Gangadharan et al., 2001; Latchoumycandane et al., 2002). We studied the effects of MXC on early pig embryos using methods described in a paper that investigated the impact of MXC on oxidative stress in oocytes and their meiosis in mice (Liu et al., 2016b). We found that MXC increased the level of superoxide free radicals and other ROS in early pig embryos, increased the percentage of apoptotic blastocysts and DNA damage, and decreased the mitochondrial membrane potential, mitochondrial copy number, cell proliferation capacity, total number of blastocysts, and the blastocyst rate. Therefore, it can be concluded that MXC adversely affects the development of early pig embryos.
The persistence of MXC as an environmental contaminant in the environment affects embryonic development. Blastocyst rate and hatching rate are important reference standards for judging the quality of in vitro embryo development. MXC inhibits embryo development and reduces the number of cells in blastocysts (Amstislavsky et al., 2003). This is consistent with the results of the present study in which MXC exposure reduced the rate of blastocysts in parthenogenesis, and the number of blastocyst cells and their hatching rate.
Free radicals are generated as part of routine metabolic processes, and they play integral roles in normal cellular functions, including cell signal transduction, gene expression, and the regulation of cell apoptosis (Ghosh and Myers, 1998). However, in addition to the endogenous free radicals produced by normal metabolism, there are also exogenous sources. The primary external origins of free radicals include smoking, exposure to air pollution, ultraviolet and ionizing radiation, as well as suboptimal food quality (Valko et al., 2007). GSH is an important antioxidant molecule involved in the elimination of ROS and has been shown to reduce oxidative stress in cells (Barros et al., 2019). MXC caused a significant increase in ROS levels in mouse oocytes, contributing to oxidative stress (Liu et al., 2016b). This is consistent with the results in this study, where MXC led to an overproduction of ROS and a decrease in GSH content during early embryonic development, which exerted oxidative stress on early embryos. We believe that this is the main cause of MXC-induced developmental damage to early pig embryos.
Mitochondria are important organelles that provide ATP for most cellular energy-demanding processes through the oxidative phosphorylation pathway in the early embryo (Niu et al., 2020). Mitochondria are also the main source of ROS production, and the electron transport chain in mitochondrial respiration is accompanied by ROS production. The number of mitochondria in an embryo is one of the markers of embryo quality (Kobayashi et al., 2020). Mitochondrial membrane potential provides the foundation for the respiration function of mitochondria, converting ADP into ATP under the action of enzymes. The depolarization of ΔΨm can disrupt the transfer of electrons to oxygen receptors, leading to the overproduction of ROS (Lenaz, 2001; St-Pierre et al., 2002; Djavaheri-Mergny et al., 2003; Pelicano et al., 2003; Hagen et al., 2004), and excessive ROS will induce cell apoptosis (Valko et al., 2007). Due to the high reactivity of free radicals, the excess production of free radicals can induce oxidative stress damage to macromolecules (such as lipids, proteins and DNA) by affecting their functions (Goutzourelas et al., 2018). It has been shown that MXC exposure increases hydrogen peroxide production in rat brain cells, decreased mitochondrial membrane potential, and inhibits mitochondrial respiration in mice (Schuh et al., 2005). MXC also causes excessive production of ROS in the mouse ovary, leading to mitochondrial dysfunction in the ovary and thus reproductive dysfunction (Gupta et al., 2006b). The decrease in mitochondrial copy number is also evidence of a decrease in embryo quality (Gil et al., 2012). This is consistent with the findings of this study that exposure to MXC may lead to the depolarization of mitochondrial membrane potential, reduced mitochondrial copy number, decreased ATP levels, causing the overproduction of ROS.
The delicate equilibrium between ROS levels and endogenous antioxidants, when disrupted, can culminate in oxidative stress and, in more severe scenarios, trigger apoptosis (Soga et al., 2012). Mitochondria are involved in the regulation of cell death pathways (Green and Reed, 1998; Kaufmann et al., 2000) and occupy a pivotal role in the integration and transmission of cell death signals, encompassing factors like oxidative stress and DNA damage (Green and Kroemer, 2004). Mitochondria have important functions in apoptosis, and changes in the mitochondrial membrane potential are a critical mediator of apoptosis induction (Liu et al., 2000). It has been shown that MXC significantly increases the rate of apoptosis in mouse preimplantation embryos and affects embryonic development (Amstislavsky et al., 2003). The TUNEL assay shows that compared with the control group, the blastocyst rate of the experimental group exposed to MXC was decreased, while the extent of apoptosis was increased. The results of the detection of DNA damage in blastocysts showed that MXC resulted in increased DNA damage in embryos. The data from this study show that the exposure of early pig embryos to MXC induced apoptosis and increases DNA damage.
The proliferation and orderly differentiation of embryonic cells play crucial role in mammalian embryonic development, and the expression of pluripotency genes is closely related to the quality of early embryonic development (Suwinska and Ciemerych, 2011). Our results suggest that MXC does have a reducing effect on the proliferative capacity of early porcine embryos. Previous studies have shown that MXC can reduce embryo totipotent gene expression, and the oxidative stress induced by MXC may cause changes in Bcl-2 family members (Gupta et al., 2006a). This in turn leads to the expression of Caspase9, which induces apoptosis. We examined gene expression in day 7 parthenogenetic blastocysts. The qRT-PCR result revealed a notable elevation in the expression of blastocyst apoptosis-related genes, namely, BAX/BCL-2 and Caspase9, within the experimental group. Conversely, the expression levels of pluripotency genes, including NANOG, Oct4, and Sox2, displayed a discernible reduction in the experimental group. Therefore, these results show that the exposure of early pig embryos to MXC may reduce their development potential.
Using pig in vitro fertilized (IVF) embryos to study the effects of MXC on early-stage embryos is more meaningful. While IVF embryos and parthenogenetic embryos (PA) show similar patterns in mitochondrial activity during development (Du et al., 2021), their gene expression profiles differ at various time points (Razmi et al., 2023). However, compared to cattle and mice, pigs exhibit the unique characteristic of polyspermy during IVF, where multiple sperm can fertilize a single oocyte, leading to different gene expression patterns in embryos that result from polyspermy (Funahashi, 2003). This can introduce potential errors in experiments.
Parthenogenetic embryos, on the other hand, are widely used in early embryonic development research due to their stability and ease of acquisition (Chen et al., 2023). The focus of this study is the impact of the environmental pollutant MXC on early embryo development, and therefore, using more stable PA embryos for experimentation is justified. Given the distinct developmental patterns of IVF embryos and PA embryos, we plan to investigate the effects of MXC on IVF embryo development and in vivo embryo development in future experiments.
In summary, our results have unequivocally demonstrated that MXC elicits detrimental impacts on the developmental processes of early pig embryos, thereby reinforcing MXC’s toxicity to the reproductive system. In addition, research on this toxic effect may cause humans to pay more attention to pesticide residues, use safer pesticides, thus potentially preventing the physiological diseases caused by chemical exposure.
5 Conclusion
Our results show that MXC has reproductive toxicity, which impedes the development of early embryos by increasing ROS, decreasing mitochondrial function, promoting embryonic apoptosis and DNA damage.
Data availability statement
The original contributions presented in the study are included in the article/Supplementary material, further inquiries can be directed to the corresponding authors.
Ethics statement
Ethical approval was not required for the studies on animals in accordance with the local legislation and institutional requirements because only commercially available established cell lines were used.
Author contributions
ZG: Validation, Writing–original draft. YJ: Resources, Funding acquisition, Writing–review and editing. FQ: Software, Visualization, Writing–review and editing. SH: Data curation, Writing–original draft. SS: Writing–original draft, Investigation. BH: Formal Analysis, Writing–original draft. ZC: Methodology, Writing–original draft. IK: Writing–review and editing. MZ: Supervision, Funding acquisition, Writing–review and editing. XY: Conceptualization, Funding acquisition, Project administration, Writing–review and editing.
Funding
The author(s) declare financial support was received for the research, authorship, and/or publication of this article. This research was funded by the earmarked fund for Jilin Provincial Natural Science Foundation of China (20210101014JC). International cooperation project (3D5205987203).
Conflict of interest
The authors declare that the research was conducted in the absence of any commercial or financial relationships that could be construed as a potential conflict of interest.
The author(s) declared that they were an editorial board member of Frontiers, at the time of submission. This had no impact on the peer review process and the final decision.
Publisher’s note
All claims expressed in this article are solely those of the authors and do not necessarily represent those of their affiliated organizations, or those of the publisher, the editors and the reviewers. Any product that may be evaluated in this article, or claim that may be made by its manufacturer, is not guaranteed or endorsed by the publisher.
References
Agarwal, A., and Allamaneni, S. S. (2004). Role of free radicals in female reproductive diseases and assisted reproduction. Reprod. Biomed. Online 9 (3), 338–347. doi:10.1016/s1472-6483(10)62151-7
Ali, U., Syed, J. H., Malik, R. N., Katsoyiannis, A., Li, J., Zhang, G., et al. (2014). Organochlorine pesticides (OCPs) in South Asian region: a review. Sci. Total Environ. 476-477, 705–717. doi:10.1016/j.scitotenv.2013.12.107
Aly, H. A., and Azhar, A. S. (2013). Methoxychlor induced biochemical alterations and disruption of spermatogenesis in adult rats. Reprod. Toxicol. 40, 8–15. doi:10.1016/j.reprotox.2013.05.002
Amstislavsky, S. Y., Kizilova, E. A., Eroschenko, V. P., and Amstislavksy, S. Y. (2003). Preimplantation mouse embryo development as a target of the pesticide methoxychlor. Reprod. Toxicol. 17 (1), 79–86. doi:10.1016/s0890-6238(02)00095-3
Banerjee, S., Banerjee, S., Saraswat, G., Bandyopadhyay, S. A., and Kabir, S. N. (2014). Female reproductive aging is master-planned at the level of ovary. PLoS One 9 (5), e96210. doi:10.1371/journal.pone.0096210
Barros, F. D. A., Adona, P. R., Guemra, S., and Damião, B. C. M. (2019). Oxidative homeostasis in oocyte competence for in vitro embryo development. Anim. Sci. J. 90 (10), 1343–1349. doi:10.1111/asj.13256
Bhardwaj, J. K., and Saraf, P. (2014). Malathion-induced granulosa cell apoptosis in caprine antral follicles: an ultrastructural and flow cytometric analysis. Microsc. Microanal. 20 (6), 1861–1868. doi:10.1017/S1431927614013452
Bhardwaj, J. K., and Saraf, P. (2017). N-acetyl cysteine-mediated effective attenuation of methoxychlor-induced granulosa cell apoptosis by counteracting reactive oxygen species generation in caprine ovary. Environ. Toxicol. 32 (1), 156–166. doi:10.1002/tox.22221
Bretveld, R. W., Thomas, C. M., Scheepers, P. T., Zielhuis, G. A., and Roeleveld, N. (2006). Pesticide exposure: the hormonal function of the female reproductive system disrupted? Reprod. Biol. Endocrinol. 4, 30. doi:10.1186/1477-7827-4-30
Chen, F., Li, M. G., Hua, Z. D., Ren, H. Y., Gu, H., Luo, A. F., et al. (2023). TET family members are integral to porcine oocyte maturation and parthenogenetic pre-implantation embryogenesis. Int. J. Mol. Sci. 24 (15), 12455. doi:10.3390/ijms241512455
Cheng, L., Song, W., Rao, Q., Zhou, J., and Zhao, Z. (2019). Bioaccumulation and toxicity of methoxychlor on Chinese mitten crab (Eriocheir sinensis). Comp. Biochem. Physiol. C Toxicol. Pharmacol. 221, 89–95. doi:10.1016/j.cbpc.2019.04.002
Djavaheri-Mergny, M., Wietzerbin, J., and Besançon, F. (2003). 2-Methoxyestradiol induces apoptosis in Ewing sarcoma cells through mitochondrial hydrogen peroxide production. Oncogene 22 (17), 2558–2567. doi:10.1038/sj.onc.1206356
Du, Z. Q., Liang, H., Liu, X. M., Liu, Y. H., Wang, C., and Yang, C. X. (2021). Single cell RNA-seq reveals genes vital to in vitro fertilized embryos and parthenotes in pigs. Sci. Rep. 11 (1), 14393. doi:10.1038/s41598-021-93904-3
El-Shahawi, M. S., Hamza, A., Bashammakh, A. S., and Al-Saggaf, W. T. (2010). An overview on the accumulation, distribution, transformations, toxicity and analytical methods for the monitoring of persistent organic pollutants. Talanta 80 (5), 1587–1597. doi:10.1016/j.talanta.2009.09.055
Funahashi, H. (2003). Polyspermic penetration in porcine IVM-IVF systems. Reprod. Fertil. Dev. 15 (3), 167–177. doi:10.1071/rd02076
Gangadharan, B., Murugan, M. A., and Mathur, P. P. (2001). Effect of methoxychlor on antioxidant system of goat epididymal sperm in vitro. Asian J. Androl. 3 (4), 285–288.
Ghosh, J., and Myers, C. E. (1998). Inhibition of arachidonate 5-lipoxygenase triggers massive apoptosis in human prostate cancer cells. Proc. Natl. Acad. Sci. U. S. A. 95 (22), 13182–13187. doi:10.1073/pnas.95.22.13182
Ghosh, M., Das, J., and Sil, P. C. (2012). D(+) galactosamine induced oxidative and nitrosative stress-mediated renal damage in rats via NF-κB and inducible nitric oxide synthase (iNOS) pathways is ameliorated by a polyphenol xanthone, mangiferin. Free Radic. Res. 46 (2), 116–132. doi:10.3109/10715762.2011.644240
Gil, M. A., Maside, C., Cuello, C., Parrilla, I., Vazquez, J. M., Roca, J., et al. (2012). Effects of Hoechst 33342 staining and ultraviolet irradiation on mitochondrial distribution and DNA copy number in porcine oocytes and preimplantation embryos. Mol. Reprod. Dev. 79 (9), 651–663. doi:10.1002/mrd.22071
Goutzourelas, N., Orfanou, M., Charizanis, I., Leon, G., Spandidos, D. A., and Kouretas, D. (2018). GSH levels affect weight loss in individuals with metabolic syndrome and obesity following dietary therapy. Exp. Ther. Med. 16 (2), 635–642. doi:10.3892/etm.2018.6204
Green, D. R., and Kroemer, G. (2004). The pathophysiology of mitochondrial cell death. Science 305 (5684), 626–629. doi:10.1126/science.1099320
Green, D. R., and Reed, J. C. (1998). Mitochondria and apoptosis. Science 281 (5381), 1309–1312. doi:10.1126/science.281.5381.1309
Gupta, R. K., Miller, K. P., Babus, J. K., and Flaws, J. A. (2006a). Methoxychlor inhibits growth and induces atresia of antral follicles through an oxidative stress pathway. Toxicol. Sci. 93 (2), 382–389. doi:10.1093/toxsci/kfl052
Gupta, R. K., Schuh, R. A., Fiskum, G., and Flaws, J. A. (2006b). Methoxychlor causes mitochondrial dysfunction and oxidative damage in the mouse ovary. Toxicol. Appl. Pharmacol. 216 (3), 436–445. doi:10.1016/j.taap.2006.06.013
Hagen, T., D'amico, G., Quintero, M., Palacios-Callender, M., Hollis, V., Lam, F., et al. (2004). Inhibition of mitochondrial respiration by the anticancer agent 2-methoxyestradiol. Biochem. Biophys. Res. Commun. 322 (3), 923–929. doi:10.1016/j.bbrc.2004.07.204
Kaufmann, S. H., Mesner, P. W., Samejima, K., Toné, S., and Earnshaw, W. C. (2000). Detection of DNA cleavage in apoptotic cells. Methods Enzymol. 322, 3–15. doi:10.1016/s0076-6879(00)22003-x
Kobayashi, M., Ito, J., Shirasuna, K., Kuwayama, T., and Iwata, H. (2020). Comparative analysis of cell-free DNA content in culture medium and mitochondrial DNA copy number in porcine parthenogenetically activated embryos. J. Reprod. Dev. 66 (6), 539–546. doi:10.1262/jrd.2020-097
Koç, N. D., Kayhan, F. E., Sesal, C., and Muşlu, M. N. (2009). Dose-dependent effects of endosulfan and malathion on adult Wistar albino rat ovaries. Pak J. Biol. Sci. 12 (6), 498–503. doi:10.3923/pjbs.2009.498.503
Latchoumycandane, C., Chitra, K. C., and Mathur, P. P. (2002). The effect of methoxychlor on the epididymal antioxidant system of adult rats. Reprod. Toxicol. 16 (2), 161–172. doi:10.1016/s0890-6238(02)00002-3
Lenaz, G. (2001). The mitochondrial production of reactive oxygen species: mechanisms and implications in human pathology. IUBMB Life 52 (3-5), 159–164. doi:10.1080/15216540152845957
Li, J., Li, N., Ma, M., Giesy, J. P., and Wang, Z. (2008). In vitro profiling of the endocrine disrupting potency of organochlorine pesticides. Toxicol. Lett. 183 (1-3), 65–71. doi:10.1016/j.toxlet.2008.10.002
Liu, L., Trimarchi, J. R., and Keefe, D. L. (2000). Involvement of mitochondria in oxidative stress-induced cell death in mouse zygotes. Biol. Reprod. 62 (6), 1745–1753. doi:10.1095/biolreprod62.6.1745
Liu, S., Li, C., Wang, Y., Hong, T., Song, T., Li, L., et al. (2016a). In utero methoxychlor exposure increases rat fetal Leydig cell number but inhibits its function. Toxicology 370, 31–40. doi:10.1016/j.tox.2016.09.009
Liu, Y., Wang, Y. L., Chen, M. H., Zhang, Z., Xu, B. H., Liu, R., et al. (2016b). Methoxychlor exposure induces oxidative stress and affects mouse oocyte meiotic maturation. Mol. Reprod. Dev. 83 (9), 768–779. doi:10.1002/mrd.22683
Luo, D., Pu, Y., Tian, H., Cheng, J., Zhou, T., Tao, Y., et al. (2016). Concentrations of organochlorine pesticides in umbilical cord blood and related lifestyle and dietary intake factors among pregnant women of the Huaihe River Basin in China. Environ. Int. 92-93, 276–283. doi:10.1016/j.envint.2016.04.017
Mao, B., Wu, C., Zheng, W., Shen, Q., Wang, Y., Wang, Q., et al. (2018). Methoxychlor and its metabolite HPTE inhibit rat neurosteroidogenic 3α-hydroxysteroid dehydrogenase and retinol dehydrogenase 2. Neurosci. Lett. 684, 169–174. doi:10.1016/j.neulet.2018.08.008
Maskaoui, K., Zhou, J. L., Zheng, T. L., Hong, H., and Yu, Z. (2005). Organochlorine micropollutants in the jiulong river estuary and western xiamen sea, China. Mar. Pollut. Bull. 51 (8-12), 950–959. doi:10.1016/j.marpolbul.2004.11.018
Mizushima, N., and Komatsu, M. (2011). Autophagy: renovation of cells and tissues. Cell 147 (4), 728–741. doi:10.1016/j.cell.2011.10.026
Nakata, H., Kawazoe, M., Arizono, K., Abe, S., Kitano, T., Shimada, H., et al. (2002). Organochlorine pesticides and polychlorinated biphenyl residues in foodstuffs and human tissues from China: status of contamination, historical trend, and human dietary exposure. Arch. Environ. Contam. Toxicol. 43 (4), 473–480. doi:10.1007/s00244-002-1254-8
Nakata, H., Nasu, T., Abe, S., Kitano, T., Fan, Q., Li, W., et al. (2005). Organochlorine contaminants in human adipose tissues from China: mass balance approach for estimating historical Chinese exposure to DDTs. Environ. Sci. Technol. 39 (13), 4714–4720. doi:10.1021/es050493d
Niu, Y. J., Zhou, W., Nie, Z. W., Shin, K. T., and Cui, X. S. (2020). Melatonin enhances mitochondrial biogenesis and protects against rotenone-induced mitochondrial deficiency in early porcine embryos. J. Pineal Res. 68 (2), e12627. doi:10.1111/jpi.12627
Ozden-Akkaya, O., Altunbas, K., and Yagcı, A. (2017). Effects of methoxychlor on IGF-I signaling pathway in rat ovary. Biotech. Histochem 92 (3), 230–242. doi:10.1080/10520295.2017.1303193
Paulose, T., Tannenbaum, L. V., Borgeest, C., and Flaws, J. A. (2012). Methoxychlor-induced ovarian follicle toxicity in mice: dose and exposure duration-dependent effects. Birth Defects Res. B Dev. Reprod. Toxicol. 95 (3), 219–224. doi:10.1002/bdrb.21007
Pelicano, H., Feng, L., Zhou, Y., Carew, J. S., Hileman, E. O., Plunkett, W., et al. (2003). Inhibition of mitochondrial respiration: a novel strategy to enhance drug-induced apoptosis in human leukemia cells by a reactive oxygen species-mediated mechanism. J. Biol. Chem. 278 (39), 37832–37839. doi:10.1074/jbc.M301546200
Poon, B. H., Leung, C. K., Wong, C. K., and Wong, M. H. (2005). Polychlorinated biphenyls and organochlorine pesticides in human adipose tissue and breast milk collected in Hong Kong. Arch. Environ. Contam. Toxicol. 49 (2), 274–282. doi:10.1007/s00244-004-0111-3
Qiu, X., Zhu, T., Li, J., Pan, H., Li, Q., Miao, G., et al. (2004). Organochlorine pesticides in the air around the Taihu Lake, China. Environ. Sci. Technol. 38 (5), 1368–1374. doi:10.1021/es035052d
Razmi, K., Mousavi, S. E., and Patil, J. G. (2023). Paternal source of germ plasm determinants in the viviparous teleost, Gambusia holbrooki; dads do matter. Dev. Biol. 502, 14–19. doi:10.1016/j.ydbio.2023.06.018
Safe, S. (2004). Endocrine disruptors and human health: is there a problem. Toxicology 205 (1-2), 3–10. doi:10.1016/j.tox.2004.06.032
Schuh, R. A., Kristián, T., Gupta, R. K., Flaws, J. A., and Fiskum, G. (2005). Methoxychlor inhibits brain mitochondrial respiration and increases hydrogen peroxide production and CREB phosphorylation. Toxicol. Sci. 88 (2), 495–504. doi:10.1093/toxsci/kfi334
Sinha, K., Das, J., Pal, P. B., and Sil, P. C. (2013). Oxidative stress: the mitochondria-dependent and mitochondria-independent pathways of apoptosis. Arch. Toxicol. 87 (7), 1157–1180. doi:10.1007/s00204-013-1034-4
Snedeker, S. M. (2001). Pesticides and breast cancer risk: a review of DDT, DDE, and dieldrin. Environ. Health Perspect. 109 (Suppl. 1), 35–47. doi:10.1289/ehp.01109s135
Soga, M., Matsuzawa, A., and Ichijo, H. (2012). Oxidative stress-induced diseases via the ASK1 signaling pathway. Int. J. Cell Biol. 2012, 439587. doi:10.1155/2012/439587
St-Pierre, J., Buckingham, J. A., Roebuck, S. J., and Brand, M. D. (2002). Topology of superoxide production from different sites in the mitochondrial electron transport chain. J. Biol. Chem. 277 (47), 44784–44790. doi:10.1074/jbc.M207217200
Suwinska, A., and Ciemerych, M. A. (2011). Factors regulating pluripotency and differentiation in early mammalian embryos and embryo-derived stem cells. Vitam. Horm. 87, 1–37. doi:10.1016/B978-0-12-386015-6.00022-6
Symonds, D. A., Merchenthaler, I., and Flaws, J. A. (2008). Methoxychlor and estradiol induce oxidative stress DNA damage in the mouse ovarian surface epithelium. Toxicol. Sci. 105 (1), 182–187. doi:10.1093/toxsci/kfn100
Tao, S., Liu, W. X., Li, X. Q., Zhou, D. X., Li, X., Yang, Y. F., et al. (2009). Organochlorine pesticide residuals in chickens and eggs at a poultry farm in Beijing, China. Environ. Pollut. 157 (2), 497–502. doi:10.1016/j.envpol.2008.09.005
Toft, G., Hagmar, L., Giwercman, A., and Bonde, J. P. (2004). Epidemiological evidence on reproductive effects of persistent organochlorines in humans. Reprod. Toxicol. 19 (1), 5–26. doi:10.1016/j.reprotox.2004.05.006
Vaithinathan, S., Saradha, B., and Mathur, P. P. (2010). Methoxychlor induces apoptosis via mitochondria- and FasL-mediated pathways in adult rat testis. Chem. Biol. Interact. 185 (2), 110–118. doi:10.1016/j.cbi.2010.03.014
Valko, M., Leibfritz, D., Moncol, J., Cronin, M. T., Mazur, M., and Telser, J. (2007). Free radicals and antioxidants in normal physiological functions and human disease. Int. J. Biochem. Cell Biol. 39 (1), 44–84. doi:10.1016/j.biocel.2006.07.001
Wang, F., Jiang, X., Bian, Y. R., Yao, F. X., Gao, H. J., Yu, G. F., et al. (2007). Organochlorine pesticides in soils under different land usage in the Taihu Lake region, China. J. Environ. Sci. (China) 19 (5), 584–590. doi:10.1016/s1001-0742(07)60097-7
Yang, N., Matsuda, M., Kawano, M., and Wakimoto, T. (2006). PCBs and organochlorine pesticides (OCPs) in edible fish and shellfish from China. Chemosphere 63 (8), 1342–1352. doi:10.1016/j.chemosphere.2005.09.029
Yang, R. Q., Lv, A. H., Shi, J. B., and Jiang, G. B. (2005). The levels and distribution of organochlorine pesticides (OCPs) in sediments from the Haihe River, China. Chemosphere 61 (3), 347–354. doi:10.1016/j.chemosphere.2005.02.091
Zhang, Z. L., Hong, H. S., Zhou, J. L., Huang, J., and Yu, G. (2003). Fate and assessment of persistent organic pollutants in water and sediment from Minjiang River Estuary, Southeast China. Chemosphere 52 (9), 1423–1430. doi:10.1016/S0045-6535(03)00478-8
Zhou, R., Zhu, L., Yang, K., and Chen, Y. (2006). Distribution of organochlorine pesticides in surface water and sediments from Qiantang River, East China. J. Hazard Mater 137 (1), 68–75. doi:10.1016/j.jhazmat.2006.02.005
Keywords: methoxychlor, ROS, embryo, apoptosis, pig
Citation: Geng Z, Jin Y, Quan F, Huang S, Shi S, Hu B, Chi Z, Kong I, Zhang M and Yu X (2023) Methoxychlor induces oxidative stress and impairs early embryonic development in pigs. Front. Cell Dev. Biol. 11:1325406. doi: 10.3389/fcell.2023.1325406
Received: 21 October 2023; Accepted: 20 November 2023;
Published: 01 December 2023.
Edited by:
Jae Yong Han, Seoul National University, Republic of KoreaReviewed by:
Shao-Chen Sun, Nanjing Agricultural University, ChinaHyuk-Joon Jeon, University of Pennsylvania, United States
Copyright © 2023 Geng, Jin, Quan, Huang, Shi, Hu, Chi, Kong, Zhang and Yu. This is an open-access article distributed under the terms of the Creative Commons Attribution License (CC BY). The use, distribution or reproduction in other forums is permitted, provided the original author(s) and the copyright owner(s) are credited and that the original publication in this journal is cited, in accordance with accepted academic practice. No use, distribution or reproduction is permitted which does not comply with these terms.
*Correspondence: Mingjun Zhang, bWp6aGFuZ0BqbHUuZWR1LmNu; Xianfeng Yu, eGlhbmZlbmc3OUBqbHUuZWR1LmNu
†These authors have contributed equally to this work