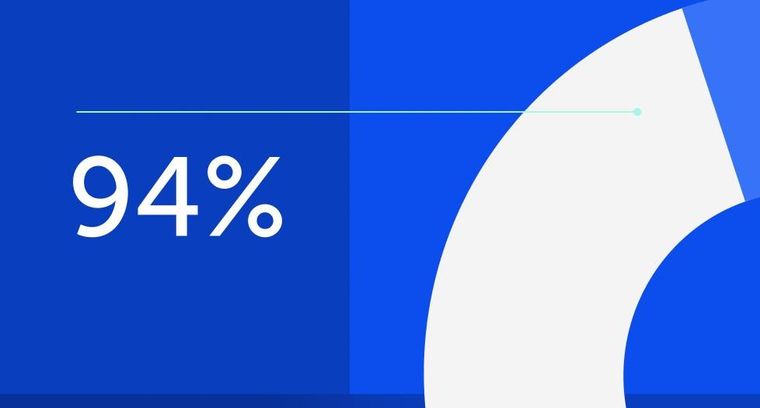
94% of researchers rate our articles as excellent or good
Learn more about the work of our research integrity team to safeguard the quality of each article we publish.
Find out more
ORIGINAL RESEARCH article
Front. Cell Dev. Biol., 04 December 2023
Sec. Nuclear Organization and Dynamics
Volume 11 - 2023 | https://doi.org/10.3389/fcell.2023.1285695
This article is part of the Research TopicMolecular Architecture and Dynamics of Meiotic ChromosomesView all 16 articles
Nuclear Pore Complexes (NPCs) are embedded in the nuclear envelope (NE), regulating macromolecule transport and physically interacting with chromatin. The NE undergoes dramatic breakdown and reformation during plant cell division. In addition, this structure has a specific meiotic function, anchoring and positioning telomeres to facilitate the pairing of homologous chromosomes. To elucidate a possible function of the structural components of the NPCs in meiosis, we have characterized several Arabidopsis lines with mutations in genes encoding nucleoporins belonging to the outer ring complex. Plants defective for either SUPPRESSOR OF AUXIN RESISTANCE1 (SAR1, also called NUP160) or SAR3 (NUP96) present condensation abnormalities and SPO11-dependent chromosome fragmentation in a fraction of meiocytes, which is increased in the double mutant sar1 sar3. We also observed these meiotic defects in mutants deficient in the outer ring complex protein HOS1, but not in mutants affected in other components of this complex. Furthermore, our findings may suggest defects in the structure of NPCs in sar1 and a potential link between the meiotic role of this nucleoporin and a component of the RUBylation pathway. These results provide the first insights in plants into the role of nucleoporins in meiotic chromosome behavior.
The nuclear pore complex (NPC) is one of the largest non-polymeric protein complexes in eukaryotic cells (Knockenhauer and Schwartz, 2016). The main function of the NPC is to mediate the selective nucleocytoplasmic transport of macromolecules while allowing the free diffusion of molecules smaller than 40 kDa (Raíces and D’Angelo, 2012; Meier et al., 2017). Most of the proteins forming the NPCs, known as nucleoporins, are evolutionarily conserved, as well as the octagonal symmetry of these complexes (Gall, 1967; Hoelz et al., 2011; Lin and Hoelz, 2019). The NPC is organized in different subcomplexes composed of more than 30 different nucleoporins (Tamura and Hara-Nishimura, 2013; Li and Gu, 2020). In addition to their main role as trafficking channels, NPCs also act as hubs for relevant processes such as chromatin organization, gene transcription, replication stress resolution or DNA repair (Blobel, 1985; Beck and Hurt, 2017; Lamm et al., 2021). In these cases, NPCs could act as membrane-bound sliding platforms to associate the underlying chromatin with other protein complexes localized in the nucleus (Strambio-De-Castilla et al., 2010; Raices and D’Angelo, 2012).
The overall organization of the NPCs is highly conserved among evolutionarily distant eukaryotes, although there is a significant variability in the composition of nucleoporins (Fiserova et al., 2009; Tamura et al., 2011; 2013; Fernandez-Martinez and Rout, 2021). Nucleoporins assemble into different subcomplexes forming the inner, outer, and membrane rings. Moreover, the central channel is filled by phenylalanine-glycine-rich (FG) nucleoporins, and a nuclear basket and cytoplasmic filaments are anchored to the nuclear and cytoplasmic outer rings, respectively (Alber et al., 2007; Beck and Hurt, 2017; Meier et al., 2017). The outer ring complex nucleoporins form Y-shaped complexes, and accordingly, this NPC module is also known as the Y-complex (Stuwe et al., 2015). This complex is also called NUP107-160 in plants and vertebrates and Nup84 (or Nup84-Nup133) in yeast (Lutzmann et al., 2002; Walther et al., 2003; Meier et al., 2017; Nordeen et al., 2020). The outer ring complex plays essential roles in NPC assembly, microtubule polymerization at kinetochores, and DNA repair (Walther et al., 2003; Nagai et al., 2008; Mishra et al., 2010). In Arabidopsis thaliana, members of this complex are involved in flowering time regulation, abiotic stress and immune responses, as well as in hormone signaling (Gu, 2018; Zhang A. et al., 2020; Cheng et al., 2020; Nie et al., 2023). In this species, defective mutants for members of this complex, such as nup160 and nup96, were identified in a screening for suppression of auxin resistance phenotypes. For this reason, these mutants are also called suppressor of auxin resistance1 (sar1) and sar3, respectively, and exhibit pleiotropic growth defects including an early flowering phenotype (Cernac et al., 1997; Parry et al., 2006; Wiermer et al., 2012).
Meiosis is a specialized cell division required to generate haploid gametes from diploid parent cells. During early prophase I, homologous chromosomes pair, synapse and exchange genetic information. These processes are facilitated by the movement of telomeres along the nuclear envelope (NE) (Koszul and Kleckner, 2009). The transmission of cytoplasmic forces to telomeres is mediated by the LINC (LInker of the Nucleoskeleton and Cytoskeleton) complexes (Starr, 2009; Klutstein and Cooper, 2014). These complexes consist of SUN and KASH proteins that span the inner and outer nuclear membranes (INM and ONM), connecting nuclear and cytoplasmic structures. Disrupting the function of the LINC complex impairs chromosome movements, leading to defects in synapsis and meiotic recombination (Ding et al., 2007; Murphy et al., 2014; Varas et al., 2015; Zhang F. et al., 2020). This role for LINC complexes seems to be conserved in yeast, animals, and plants (Burke, 2018). It has been suggested that LINC complexes and NPCs could be functionally related. Indeed, in HeLa cells SUN1 interacts with NPCs being important for their distribution (Liu et al., 2007). However, studies showing a possible meiotic function for NPCs are scarce and mostly focused on yeast. Several of these studies have associated the function of certain nucleoporins with kinetochores and chromosome segregation (Yang et al., 2015; Hattersley et al., 2016; 2022). In Saccharomyces cerevisiae, the nuclear basket nucleoporins Nup2 and Nup60 transiently detach from the NPC core during the first meiotic division and promote chromosome dynamics during meiosis (Chu et al., 2017; Komachi and Burgess, 2022; King et al., 2023). Until now, no work in plants has linked NPCs to chromatin organization and chromosome behavior during meiosis. In this regard, it is important to note that in contrast to the situation in yeast, in plants, the NE breaks down to allow the connection between the chromosomes and the cytoplasmic spindle (Meier et al., 2017).
In this study, we focus on the meiotic role of nucleoporins belonging to the outer ring complex of the NPC, in particular NUP160 (SAR1) and NUP96 (SAR3). Analysis of the meiotic process in pollen mother cells (PMCs) has revealed that SAR1 and SAR3 are essential for ensuring proper chromatin condensation and meiotic repair of double-strand breaks (DSBs). Additionally, the findings may suggest that SAR1 is important for preserving the integrity of NPCs in prophase I and that its meiotic function could be linked to that of AXR1, a subunit of the RUB1 activating enzyme, which regulates the protein degradation activity of SKP1-CULLIN1-F-BOX (SCF) complexes. Our work provides, for the first time, important insights into the function of NPCs in plant meiosis.
All plants used in this study were Arabidopsis thaliana (L.) Heynh, Columbia (Col-0) accession. Mutant lines were obtained from the European Arabidopsis Stock Centre: sar1-4 (SALK_126801), sar3-4 (SALK_117966), hos1-3 (SALK_069312), nup85-2 (SALK_113274), and seh1-1 (SALK_022717). Double mutants were built using: spo11-1-5 (SALK_009440) (Pradillo et al., 2007), and axr1-31 (SALK_013238) (Martínez-García et al., 2020). Seeds were sown on soil directly or after transfer from MS plates (Murashige and Skoog, 1962). Plants were grown under long-day conditions (16 h light/8 h dark) at 19°C. Homozygous plants were identified by PCR screening using primers listed in Supplementary Table S1.
Fixation of flower buds and spreading of male meiocytes were performed according to Ross et al. (1996). The fluorescent in situ hybridization (FISH) technique was carried out as described by Sanchez-Moran et al. (2001) with minor modifications. The DNA probes used in the analysis of chromosomal configurations at metaphase I were 45S rDNA (pTa71; Gerlach and Bedbrook, 1979) and 5S rDNA (pCT4.2; Campell et al., 1992). At least three plants of each genotype were analyzed. To analyze pollen grains, we used fresh material and transferred anthers to a 1% solution of carmine in 45% acetic acid, we heated the slide slowly over an alcohol burner (∼30 s) and used the squash method. A Nikon Eclipse E400 phase-contrast microscope with a Nikon DMX-12005-E400 digital camera were used for image acquisition.
To detect meiotic recombination proteins, we performed the spreading technique described by Armstrong et al. (2009) with the modifications included in Varas and Pradillo (2018). To detect histone modifications and NE proteins, we applied the squash technique detailed in Oliver et al. (2013). Information about the dilution and source of primary antibodies is included in Supplementary Table S2. The secondary antibodies used were anti-rabbit Alexa Fluor 555-conjugated (Invitrogen, Molecular probes; 1:500), anti-rat Alexa Fluor 555-conjugated (Invitrogen, Molecular probes; 1:500), anti-mouse FITC-conjugated (Agrisera; 1:100), and anti-rabbit FITC-conjugated (Merck; 1:50). Cells were imaged using an Olympus BX61 epifluorescence microscope with an Olympus DP71 digital camera. Quantification of foci was performed using ImageJ. We scored all images blind to genotype.
To evaluate mRNA nuclear accumulation, we followed the protocol described in Parry (2014) using samples from roots and flower buds. The samples were incubated with a Cy3 labelled oligo(dT) probe. For quantification, we compared pixel intensity between the nucleus and the cytoplasm (ImageJ) and calculated the fold change (ratio nucleus/cytoplasm) in each cell respect to Col-0. Cells from at least three different slides were analyzed for each tissue.
Microsoft Office Excel and GraphPad Prism were used for data organization and statistical analysis, respectively. Mann-Whitney U test and one-way ANOVA with post hoc Tukey test were performed to compare independent samples. Fisher’s Exact test and Chi-square test were used to compare frequencies.
The mutants analyzed in this study were previously characterized by Cernac et al. (1997) and Parry et al. (2006). These authors revealed that sar1 and sar3 show a pleiotropic phenotype with early flowering, dwarfism, and abnormal auxin response. These mutants also have altered expression of certain defense genes and nuclear mRNA accumulation (Meier and Brkljacic, 2009; Wiermer et al., 2012). In addition, the pleiotropic defects in the single mutants were exacerbated in the double mutant sar1 sar3, suggesting the disruption of an NPC specific function associated to the loss of several subunits (Parry et al., 2006). The reduced production of seeds in these plants was attributed to their developmental defects and abnormal inflorescences (Parry et al., 2006).
In this work, we have confirmed the fertility defects in sar1 and sar3 (Supplementary Figure S1) and observed that this phenotype is aggravated in the double mutant sar1 sar3, which is completely sterile. In addition, this double mutant has viability problems, as only 2% of sar1 sar3 double mutant plants were obtained in the offspring of double heterozygous plants. The detailed cytological characterization of pollen mother cells (PMCs) that we conducted in these mutants revealed that these fertility defects are due to abnormalities during meiosis. In the single mutants sar1 and sar3, most of the meiocytes were apparently indistinguishable from the control: homologous chromosomes were associated along their entire length in pachynema; five bivalents, with no alterations in chromosome condensation, were observed at metaphase I; and chromosomes and sister-chromatids segregated correctly at anaphase I and II, respectively, giving rise to balanced tetrads with the same amount of genetic material in each nucleus (Supplementary Figure S2). However, alterations in chromatin condensation and chromosome fragmentation appeared at different stages corresponding to both meiotic divisions in a percentage of meiocytes (sar1: 13.52%, n = 636; sar3: 19.08%, n = 1,373). No anomalies were observed at leptonema, but from zygonema onwards some PMCs showed aberrant chromatin condensation. In metaphase I, entangled bivalents were observed and segregation problems, as well as chromosome fragments, were detected in both meiosis I and II. All these defects led to the formation of tetrads and polyads with differentially condensed and unbalanced nuclei (Figure 1A). Abnormal meiocytes were not limited to specific flower buds or anthers but appeared alongside populations of normal cells (Figure 1B; Supplementary Figure S1). The percentage of abnormal meiocytes was much higher in the double mutant (57.02%, n = 114) than in the single mutants, which also differed from each other (Figure 1C; Supplementary Tables S3, S4). In addition, the proportion of meiocytes with chromosomal fragmentation was increased in the double mutant compared to the single mutants (Supplementary Table S5).
FIGURE 1. Cytological analysis of meiotic defects in sar mutants. (A) Chromosome spreads of male meiocytes from WT, sar1-4, sar3-4 and the double mutant sar1-4 sar3-4. Mutants show hypercondensed meiocytes at pachynema, entangled chromosomes at metaphase I, chromosome fragments and unbalanced segregations at telophase I, unbalanced nuclei and chromosome fragments at metaphase II, and polyads with nuclei displaying unevenly condensed chromatin. (B) Examples from sar1-4 showing abnormal meiocytes (arrowheads) surrounded by normal meiocytes in both meiotic divisions. (C) Proportion of abnormal and normal meiocytes in sar1-4, sar3-4 and the double mutant sar1-4 sar3-4. Fisher’s exact test was performed to analyze differences between mutants (p-value: ns–non-significant, *p < 0.05, **p < 0.01, ***p < 0.001). I: Meiosis I; II: Meiosis II. Scale bars = 5 µm.
In order to elucidate whether these defects were a consequence of the alteration of any component of the outer ring complex, we analyzed other mutants defective for this subunit. HIGH EXPRESSION OF OSMOTICALLY RESPONSIVE GENES1 (HOS1) functions in the regulation of flowering through controlling the protein level of CONSTANT, like SAR1 and SAR3 (Cheng et al., 2020; Li et al., 2020). The cytological analysis of male meiosis in hos1 revealed the same type of alterations as those observed in sar1 and sar3 mutants (Supplementary Figure S2). Problems in chromatin condensation and chromosomal fragmentation were detected, although meiocytes without any abnormalities could also be observed. Interestingly, these meiotic alterations do not appear when other components of the outer ring, such as NUP85 (n = 166) or SEH1 (n = 36), are absent (Supplementary Figure S2). Both sar1 (mutant in which we detected meiosis abnormalities) and nup85 (mutant in which we did not detect any meiosis abnormalities) show mRNA accumulation in the nucleus, not only in root cells, but also in flower bud cells (Supplementary Figure S3). Therefore, these results show that the different outer ring components are not equally important during meiosis, and that the meiotic defects do not appear to arise as a consequence of the mRNA accumulation.
In order to conduct a more exhaustive study of the meiotic process, the sar1 mutant was chosen as representative of the meiotic problems observed in the outer ring complex mutants. Since the meiotic problems began to be detected in zygonema-pachynema, we carried out an analysis of the synaptonemal complex (SC) formation by immunolocalization of ASY1 (protein associated to the axial/lateral element) and ZYP1 (transverse filament protein) on prophase I chromosome spreads (Armstrong et al., 2002; Higgins et al., 2005). We also detected two cell populations with respect to SC formation. In all meiocytes in which the chromosome morphology was indistinguishable to the WT (Supplementary Figure S2), the pattern corresponding to the ASY1+ZYP1 proteins revealed normal behavior: bright linear signals of ASY1 in the unsynapsed regions during zygonema and short stretches of ZYP1 that extended until full synapsis at pachynema (Figure 2; Supplementary Figure S4). In the case of meiocytes with extreme chromosomal condensation, we observed a continuous ASY1 signal in zygonema, despite the small size of these nuclei (Figure 2A). However, in these abnormal meiocytes we could not detect a continuous ZYP1 signal, observing only some aggregates without a defined pattern (Figure 2B). Thus, chromosomal axes appear to form correctly in sar1 meiocytes, and although synapsis is normal in most sar1 meiocytes, some have problems achieving synapsis.
FIGURE 2. Immunolocalization of meiotic chromosome axes and synaptonemal complex in sar1-4. Squash preparations of male meiocytes showing the meiotic chromosome axis protein ASY1 (green) and the synaptonemal complex transverse filament protein ZYP1 (magenta). (A) Zygonema. Chromosome axes appear to be normal despite condensation defects in sar1-4. (B) Pachynema. Although full synapsis is observed in normal-looking sar1-4 meiocytes, ZYP1 forms numerous chromosomic aberrant aggregates in hypercondensed sar1-4 meiocytes, revealing problems in synaptonemal complex formation in these cells. Scale bars = 5 µm.
To determine whether, despite not detecting problems in synapsis and bivalent formation, there is any defect in meiotic recombination in normal-looking meiocytes in sar1, the frequency of chiasmata per cell at metaphase I was analyzed. To facilitate the interpretation of bivalent morphology and the localization of chiasmata, we performed 45S and 5S rDNA FISH (Sanchez-Moran et al., 2001) (Figure 3A). This analysis could not be applied to meiocytes with aberrant chromatin condensation, although we observed an arrangement of the FISH signals indicating some level of pairing, since homologous chromosomes were close together in the nucleus and presented some co-orientation at metaphase I (Figure 3B). The mean cell chiasma frequency in the WT was 10.20 ± 0.14 (n = 69), with a range of variation from 8 to 13. In sar1 no significant differences were found with respect to this value, since the mean was 10.07 ± 0.21 (n = 43), varying from 7 to 14 (U = 1,393; p = 0.579) (Figure 3C).
FIGURE 3. Cytological analysis of chiasma frequency in sar1-4. 45S rDNA (green) and 5S rDNA (magenta) probes were used for chromosome identification. DAPI is showed in gray. (A) WT and normal-looking sar1-4 meiocytes at metaphase I. Numbers identify each bivalent. (B) Abnormal sar1-4 meiocytes. The position of the FISH signals indicates pairing in prophase I and some co-orientation of homologous chromosomes in metaphase I. (C) Quantification of chiasma frequency per cell in WT and sar1-4 (see the text for more details). Scale bars = 5 µm.
Histone post-translational modifications are thought to play a pivotal role in chromosome condensation during meiosis (Fuchs et al., 2006; Xu et al., 2009). To test whether there was any variation in the epigenetic pattern of abnormally condensed meiocytes, we immunolocalized histone modifications associated to euchromatin and heterochromatin, as well as a modification specific of the chromosomal condensation process. Specifically, we analyzed the pattern corresponding to H3K4me3 (euchromatin-specific methylation), H3K9me2 (heterochromatin-specific methylation), and H3S10ph (phosphorylation associated to chromosome condensation) (Supplementary Figure S5). H3K4me3 is observed in all chromosomal regions except pericentromeric heterochromatin (Oliver et al., 2013). No variations from the WT were detected in sar1 prophase I meiocytes. In the case of sar1 hypercondensed meiocytes, H3K4me3 also had a similar pattern, appearing in most of the chromatin area. On the other hand, H3K9me2, which is restricted to pericentromeric regions throughout meiosis (Oliver et al., 2013), showed no variations in sar1 meiocytes compared to WT, since signals were always observed in the chromocenters or brightest DAPI regions. Surprisingly, no changes were observed in the H3S10ph pattern either. This mark appears in Arabidopsis from diplonema onwards, a stage in which the chromatin is more condensed (Oliver et al., 2013). We could expect the presence of this modification in sar1 meiocytes with hypercondensation. However, no signal corresponding to this epigenetic mark was detected despite chromatin compaction. Therefore, the condensation abnormalities observed in sar1 meiocytes are not due to alterations in these histone modifications, at least at the cytological level.
Analysis of PMCs from sar1 plants revealed fragmented chromosomes from anaphase I onwards in a percentage of meiocytes, leading to the formation of polyads containing microspores with unequal amounts of DNA (Figure 1). To ascertain whether DSBs formed by SPO11 could be the source of the chromosome fragmentation observed in SAR1-deficient plants, we generated sar1 spo11-1 double mutants. Meiosis in spo11 mutants is characterized by the presence of ten univalents at metaphase I, which segregate randomly during anaphase I (Grelon et al., 2001). In the absence of either SPO11-1 or SPO11-2, no DSBs occur at the onset of meiosis, therefore the integrity of the chromosomes in spo11 mutants is intact.
The meiotic phenotype of the double mutant sar1 spo11-1 was very similar to that observed in the spo11-1 single mutant, and no formation of SC or bivalents was detected. Ten univalents were invariably observed at metaphase I (n = 42) and no evidence of chromosomal fragmentation was found in any of the successive stages of meiosis (Figure 4A). Therefore, sar1 chromosomal fragmentation problems are caused by the failure to repair SPO11-induced DSBs.
FIGURE 4. Cytological analysis of PMCs from the double mutant sar1-4 spo11-1-5. (A) Chromosome spreads of male meiocytes in spo11-1-5 and sar1-4 spo11-1-5. Homologous chromosomes fail to undergo synapsis and ten univalents are observed at metaphase I in both mutants. The presence of univalents leads to mis-segregations of chromosomes at anaphase I, unbalanced nuclei during second meiotic division and polyads. (B) Example of hypercondensed (arrowhead) and normal-looking (arrow) meiocyte in the double mutant sar1-4 spo11-1-5. (C) Proportion of abnormal and normal meiocytes in sar1-4 (blue) and sar1-4 spo11-1-5 (orange). Fisher’s exact test was performed to analyze differences between the mutants (p-value: ns–non-significant, *p < 0.05, **p < 0.01, ***p < 0.001). I: Meiosis I; II: Meiosis II. Scale bars = 5 µm.
Detailed analysis of sar1 spo11-1 PMCs revealed that although chromosomal fragmentation disappears, this double mutant still shows alterations in chromatin condensation (Figure 4B). In fact, the percentage of abnormal meiocytes (with alterations in chromatin condensation and/or chromosome fragmentation) during the first division did not vary significantly from that observed in the sar1 single mutant (Figure 4C; Supplementary Table S6). In contrast, we detected a reduction in the frequency of abnormal meiocytes observed during the second division in the double mutant. The differences become apparent at the second division because at this division most of the abnormal meiocytes quantified in the sar1 single mutant have chromosomal breaks, whereas at the first division most of the abnormal sar1 meiocytes have chromatin condensation problems. Therefore, the chromosome condensation abnormalities observed in the sar1 single mutant do not appear to arise from a specific meiotic alteration or at least from defects in the processing of DSBs. There is no evidence to suggest that hypercondensation has a pre-meiotic nature, as we did not identify any issues with chromatin condensation in somatic cells (Supplementary Figure S5). Furthermore, as mentioned before, all the observed leptotene meiocytes appeared to be normal-looking.
To further examine the meiotic homologous recombination (HR) process in sar1 meiocytes, we detected phosphorylated histone H2AX (γH2AX) and RAD51 foci by immunolocalization. γH2AX is deposited at DNA damage sites and is commonly used as a DSB-marker (Lowndes and Toh, 2005), and the recombinase RAD51 is loaded on ssDNA during meiotic recombination (Kurzbauer et al., 2012). The number of foci corresponding to both proteins was quantified in both normal appearing and hypercondensed sar1 meiocytes (Figure 5; Supplementary Figure S6). We used ASY1 protein as a prophase I progression marker (Armstrong et al., 2002).
FIGURE 5. Immunolocalization of γH2AX and RAD51 in sar1-4. Chromosome spreads of male meiocytes showing γH2AX (magenta) and the recombinase RAD51 (magenta). ASY1 (green) has been used as a cytological marker to identify the meiotic chromosome axes. (A) WT, normal-looking and hypercondensed sar1-4 zygotene cells showing ASY1 (green) and γH2AX foci (magenta). (B) Quantification of γH2AX foci in zygotene cells. (C) WT, normal-looking and hypercondensed sar1-4 zygotene cells showing ASY1 (green) and RAD51 foci (magenta). (D) Quantification of RAD51 foci in zygotene cells. One-way ANOVA with a Tukey’s post hoc test was performed in both cases (p-value: ns–non-significant, p *** < 0.001). Scale bars = 5 µm.
The number of γH2AX foci in sar1 normal-looking meiocytes (196.61 ± 6.76; n = 46) was comparable as that observed in WT meiocytes (200.04 ± 6.02; n = 47), whereas we detected a significant reduction in the number of γH2AX foci in sar1 hypercondensed meiocytes (66,00 ± 10,96; n = 15) (Figures 5A, B; Supplementary Table S7). The results for the quantification of the number of RAD51 foci were very similar, as no differences were found between normal-looking sar1 (152.71 ± 7.49; n = 14) and WT meiocytes (149.42 ± 7.03; n = 36), whereas the number of RAD51 foci was drastically reduced in sar1 hypercondensed cells (44.8 ± 5.03; n = 5) (Figures 5C, D; Supplementary Table S7). These results confirm that the meiotic recombination process, in line with the results obtained for synapsis, is severely compromised in sar1 hypercondensed meiocytes.
Since the absence of the outer ring complex nucleoporins may compromise the integrity of the NPCs, we decided to analyze the distribution of these complexes, as well as that of the LINC complexes in the NE of sar1 meiocytes. For the study of LINC complexes, we applied an immunolocalization to detect SUN proteins (Figure 6; Supplementary Figure S7). In WT meiocytes these proteins present a distribution pattern along the entire NE during prophase I, disappearing at the end of this stage (n = 107). In the case of sar1, no differences were found in the pattern of these proteins with respect to WT, with a continuous signal also appearing around the entire NE, both in normal-looking meiocytes (n = 25) and in hypercondensed meiocytes (n = 9). As expected, we observed a reduction of the NE surface in the latter, in line with their hypercondensed chromatin state. Thus, the distribution of LINC complexes is apparently not affected by the absence of a structural nucleoporin.
FIGURE 6. Immunolocalization of SUN proteins and NPCs in sar1-4. Squash preparations of WT, normal-looking and hypercondensed sar1-4 zygotene cells. (A) SUN proteins (magenta) combined with DAPI (gray). A continuous signal around the entire NE is present in both normal-looking and hypercondensed sar1-4 meiocytes, as well as in the WT. (B) NPCs (green) combined with DAPI (gray). Normal-looking sar1-4 meiocytes are indistinguishable from WT cells, whereas in hypercondensed sar1-4 meiocytes NPCs appear to be absent. Scale bars = 5 µm.
Regarding NPCs, in WT cells the distribution pattern is similar to that of the LINC complexes, with a signal appearing along the entire NE during prophase I (Figure 6). We confirmed that normal-looking sar1 meiocytes (n = 103) do not display variations in the distribution pattern of NPCs with respect to WT meiocytes (n = 83). However, in sar1 hypercondensed meiocytes, no trace of the signal corresponding to NPCs was detected at any location in the nucleus (n = 76), revealing that these cells present severe structural abnormalities in the NPCs.
Nucleoporins SAR1 and SAR3 are called by these names because they were firstly identified in a screening for suppression of the axr1 resistance to auxin phenotype (Cernac et al., 1997; Parry et al., 2006). The axr1 mutation produces a dramatic effect on plant morphology (Lincoln et al., 1990) and, interestingly, meiotic defects consisting of abnormal synapsis at prophase I, univalents at metaphase I, unequal chromosome segregation at anaphase I, and unbalanced tetrads or polyads (Jahns et al., 2014). The origin of these meiotic abnormalities is poorly understood, although it has been suggested that they could be related to the protein modifications associated to the RUBylation pathway (Jahns et al., 2014).
Since mutations in either SAR1 or SAR3 suppress most aspects of the phenotype conferred by axr1 (Cernac et al., 1997; Parry et al., 2006; Supplementary Figure S8), we wondered if this suppression also affects axr1 meiotic defects. To find out if this was the case, we generated the double mutant sar1 axr1 and analyzed its meiotic phenotype. The results showed that the characteristic meiotic problems associated with axr1 disappear in sar1 axr1 (Figure 7A). In the double mutant, we observed full synapsis at pachynema, five bivalents at metaphase I, equal distribution of chromosomes during both meiotic divisions, and balanced tetrads. This means that in the double mutant the asynaptic phenotype of axr1 disappears, as well as the problems in bivalent formation.
FIGURE 7. Cytological analysis of PMCs from the double mutant sar1-4 axr1-31. (A) Chromosome spreads of male meiocytes in axr1-31 and sar1-4 axr1-31. The single mutant axr1-31 shows defects in synapsis, presence of univalents at metaphase I, unbalanced nuclei during the second meiotic division, and tetrads with micronuclei. These meiotic defects are suppressed in the double mutant sar1-4 axr1-31. (B) Example of hypercondensed (arrowhead) and normal-looking (arrow) meiocyte in the double mutant sar1-4 axr1-31. (C) Proportion of abnormal and normal meiocytes in sar1-4 (blue) and sar1-4 axr1-31 (orange). Fisher’s exact test was performed to analyze differences between the mutants (p-value: ns–non-significant, ***p < 0.001). I: Meiosis I; II: Meiosis II. Scale bars = 5 µm.
To analyze the different chromosome configurations in more detail, the FISH technique was applied (Supplementary Figure S8). In WT meiocytes most of the bivalents are closed (ring bivalents), with at least one chiasma in each arm (76.2%; n = 69), and the same occurs in sar1 (85.5%; n = 43), in which no univalents are detected either. In axr1 most of the bivalents are open (rod bivalents), without chiasmata in one of the arms (47.2%; n = 72), although there are also closed bivalents (24.2%) and univalents (28.6%). In the double mutant sar1 axr1, most bivalents appeared in closed configuration (70.4%; n = 50), recovering the WT phenotype (p = 0.155). Obligatory chiasma formation is almost restored in this double mutant, as univalents disappear. We detected only 4% of the cells with a single pair of univalents (2/50). To further analyze recombination events, we conducted an immunolocalization to detect MLH1, a marker of most crossovers in Arabidopsis (Jackson et al., 2006). This analysis showed no significant differences between the WT (8.09 ± 0.40), sar1 (8.03 ± 0.38), and sar1 axr1 (8.42 ± 0.25) (F = 0.469, p = 0.627) concerning MLH1 foci (Supplementary Figure S8).
Regarding to the presence of abnormal meiocytes, in the double mutant sar1 axr1 there was a decrease in the percentage of these meiocytes, as well as in sar1 spo11 (Figures 7B, C). We observed fewer abnormal meiocytes than in the sar1 single mutant in the first meiotic division, although the difference between both mutants was not significant. The reduction was statistically significant in the second meiotic division (Supplementary Table S8). Furthermore, in sar1 axr1, the proportion of hypercondensed meiocytes was higher than that of fragmented meiocytes in the second division, in contrast to the sar1 single mutant (Supplementary Table S5).
The results of this work have revealed that some of the structural nucleoporins that belong to the outer ring complex of the NPC are necessary for proper meiosis progression. Several of the nucleoporin-defective mutants of this complex show a pleiotropic phenotype, including developmental deficiencies and reduced fertility (Cernac et al., 1997; Parry et al., 2006). We have demonstrated that the semi-sterile phenotype is due to failures in meiosis, highlighting the importance of the NPCs in this cell division.
The cytological analysis of PMCs in sar mutants has determined the presence of abnormal meiocytes, both in first and second division. The altered meiotic phenotype is characterized by the presence of cells with extreme chromatin condensation, especially during the first division, in addition to the appearance of chromosomal fragments from anaphase I onwards, which generates unbalanced tetrads and even polyads at the end of meiosis. These abnormal meiocytes appear along with others in which meiosis is properly achieved. The percentage of meiocytes with alterations varies between the mutants sar1 and sar3 and increases considerably in the double mutant sar1 sar3 (Figure 1), which might suggest a certain degree of independence in their functions. In the double mutant, problems in vegetative development and fertility are also exacerbated respect to the single mutants, suggesting that the loss of both nucleoporins produce a severe defect in NPC function (Parry et al., 2006). We also found similar meiotic alterations in hos1 (Supplementary Figure S2). HOS1 is an outer ring complex nucleoporin that functions as an E3 ubiquitin ligase preventing precocious flowering. Interestingly, SAR1 and SAR3 also contribute to flowering time regulation by ensuring the stability and association of HOS1 with the NPC (Cheng et al., 2020; Li et al., 2020). It is possible that these nucleoporins affect a common regulatory mechanism between flowering and meiosis, since we have not detected meiotic problems in other mutants defective in the outer ring complex (nup85, seh1), which, unlike the previous ones, do not exhibit a significant flowering phenotype (Li and Gu, 2020). These mutations also do not aggravate the somatic abnormalities observed in sar1, suggesting some functional diversity between these nucleoporins that belong to the same NPC subcomplex (Wiermer et al., 2012; Parry, 2014). On the other hand, an accumulation of polyadenylated mRNA was found in all outer ring complex mutants in which RNA export was analyzed (Parry, 2015). Therefore, the observed meiotic alterations do not seem to be related to this mRNA accumulation (Supplementary Figure S3). It is likely that these nucleoporins do not only function in the context of NPCs. Indeed, NPCs undergo large-scale structural rearrangements during cell division and, for example, the nuclear basket transiently dissociate from the NPC core during meiosis in budding yeast (King et al., 2023). It is not known whether something similar occurs in Arabidopsis, but in any case, HOS1, apart from its E3-ubiquitin ligase activity, is associated with chromatin to influence gene expression in this species (Lazaro et al., 2012; Jung et al., 2014). In the case of SAR1 and SAR3, no such association has been confirmed. Further experiments will be required to confirm whether the meiotic functions of these nucleoporins are related to their structural function within the NPC.
sar1 and sar3 present abnormalities in mRNA accumulation or nuclear morphology in all somatic cells (Cernac et al., 1997; Parry et al., 2006; Parry, 2014). However, they only show meiotic alterations in a percentage of meiocytes. Although there is no clear explanation for this result, it is possible that in some meiocytes other nucleoporins cannot supply the function of SAR proteins. Alternatively, it could be a timing problem, with defects occurring in meiocytes in which meiosis is slower. In any case, the presence of normal-looking meiocytes together with abnormal meiocytes has been described in other mutants. For example, mutations in BQT1, a gene encoding a protein that tether telomeres to the spindle-pole body during prophase I, affect spindle formation in about half of meiotic cells in fission yeasts (Klutstein et al., 2015). In Arabidopsis, mutants lacking JASON, a protein essential for proper spindle orientation, or NSE2, a protein belonging to the SMC5/6 complex, generate normal and unreduced meiotic products (Erilova et al., 2009; De Storme and Geelen, 2011; Yang et al., 2021). Mutants affected in CYCA2 genes or CYCB3;1 also show alterations in a fraction of meiocytes (Bulankova et al., 2013).
The suppression of chromosome fragmentation in sar1 spo11 has evidenced that fragments produced by the absence of the nucleoporin are generated by the inability to properly repair the recombination intermediates formed from meiotic DSBs (Figure 4). These defects in DNA repair may be due to failures in the recruitment of proteins involved in the early stages of HR, as evidenced by a reduction in the number of γH2AX and RAD51 foci (Figure 5). Another possibility is that, due to the absence of the nucleoporin, HR proceeds more slowly in some meiocytes and this triggers failures in the repair of DSBs. In any case, despite these problems in HR, the chromosome axes seem to form correctly, even in the abnormal meiocytes, according to the results obtained for ASY1. However, the process of synapsis is compromised (Figure 2). The absence of synapsis is most likely caused by problems in DNA homology search during DSB repair, which is a prerequisite for the progression of synapsis (Osman et al., 2011). In addition, the entangled chromosomes observed at metaphase I in sar1, sar3, and hos1 (Figures 1, 3; Supplementary Figure S2) are reminiscent of those observed in recombination-defective mutants such as rad51, xrcc3, rad51c or mnd1 (Kerzendorfer et al., 2006; Pradillo et al., 2012; 2014). Interestingly, SUMOylation in plants, as well as in yeast, appears to be linked to the NPC, and SUMOylated proteins accumulate in mutants defective for NUA (structural component of the nuclear basket) and SAR1 (Muthuswamy and Meier, 2011). In fission yeast, the Y-complex nucleoporin Nup132 is involved in the regulation of SUMOylation during meiosis, and mutants deficient for this nucleoporin exhibit upregulated SUMOylated proteins including Pim1, Top1, and Top2 (Yang et al., 2023). Hyper-SUMOylation of Top2 alters meiotic chromosome architecture (Li et al., 2013). In Arabidopsis, topII mutants show condensation defects, entangled chromosomes, and high levels of DNA fragmentation (Martinez-Garcia et al., 2018). It is tempting to speculate that the meiotic phenotype observed in Arabidopsis Y-complex deficient mutants is somehow related to alterations in the SUMOylation of proteins with meiotic function.
The excessive chromatin condensation observed in sar1 does not seem to originate from the problems in HR, as it does not disappear in the sar1 spo11 double mutant (Figure 4). These abnormally condensed meiocytes have a morphology similar to that of cells undergoing cell death. This process is characterized by cell shrinkage, chromatin condensation, and DNA fragmentation (Kerr et al.,1972; Reape et al., 2008). Hendzel et al. (1998) pointed out that during cell death, chromatin condensation is not an active process associated with histone phosphorylation as occurs in mitosis or meiosis (Houben et al., 1999; Manzanero et al., 2000; Oliver et al., 2013). In this case, condensation would be the result of the degradation of euchromatin, nuclear matrix and lamin, in addition to the aggregation of heterochromatin. However, we have not detected appreciable variations in euchromatin- or heterochromatin-specific epigenetic marks in the abnormally condensed cells, at least at the cytological level, as variations at the molecular level cannot be ruled out (Supplementary Figure S5). Chromosome condensation problems in meiosis have been described in mutants defective for the condensin complex (Siddiqui et al., 2003; Smith et al., 2014) or mmd1 (male meiocyte death1) mutants (Yang et al., 2003; Wang et al., 2016). Nevertheless, unlike sar mutants, these mutants do not show any defects in the appearance of chromosomes during early prophase I and present chromatin decondensation at later stages (Yang et al., 2003; Smith et al., 2014).
The altered distribution of NPCs in the NE could be the source of the problems in chromatin compaction, since in abnormally condensed meiocytes there is no defined pattern for NPCs, unlike in normal-looking meiocytes (Figure 6). This phenotype reveals the importance of SAR1 in the structure of NPCs. The absence of signal corresponding to NPCs in these meiocytes is not due to NE disintegration, as the signal corresponding to SUN proteins is observed around the chromatin. Perhaps the apparent collapse of NPCs could have some reversibility, and this explains why meiotic changes only occur in a percentage of cells. Indeed, in HeLa cells depletion of the Nup107-160 complex results in nuclei with a continuous NE but no NPCs, although the defect in NPC assembly could be reversed by adding Nup107-160 complex containing fractions (Walther et al., 2003). In addition, depletion of this complex also induces cell death following a spindle checkpoint-dependent delay during mitosis (Rieder and Maiato, 2004; Zuccolo et al., 2007). In plants, the spindle checkpoint is not as tightly regulated as in yeast or animals, and it could even not function or be much relaxed during meiosis (Komaki and Schnittger, 2016). In this sense, mutants with severe recombination problems can complete meiosis, although no gamete is functional (Wijnker and Schnittger, 2013). In sar mutants the hypercondensed meiocytes appear to progress through meiosis, giving rise to the masses of entangled chromosomes observed at metaphase I (Figure 1). This is of particular interest because studying meiosis in these mutants may provide information on the possible meiotic function of these nucleoporins that cannot be obtained from studies using other model organisms.
AXR1 is a component of the RUBylation pathway targeting, among others, cullin proteins (Mergner and Schwechheimer, 2014). axr1 plants display auxin-related growth defects that are suppressed by eliminating the function of SAR1 or SAR3 (Cernac et al., 1997; Parry et al., 2006). In the present work, we have shown that the sar1 mutation also reverses the altered meiotic phenotype of axr1, which is completely different from that of sar1 (Jahns et al., 2014). In sar1 axr1 the synapsis problems disappear and the formation of the obligatory chiasma, required for bivalent formation, is restored (Figure 7). In the case of the somatic phenotype, it has been suggested that sar mutants delay the nuclear import of Aux/IAA negative regulators, thus ameliorating the defect in axr1, which initially inhibits auxin gene expression (Parry et al., 2006). The reversal of the axr1 meiotic phenotype in sar1 axr1 may also be explained in this way. On the other hand, neither sar1 nor sar3 exhibit auxin hypersensitivity, revealing a complex relationship between the NPC and the auxin response (Parry et al., 2006; Robles et al., 2012). Surprisingly, there are no studies showing a clear link between auxins and meiosis. This deserves further investigation in the future.
Alternatively, the reversal of the meiotic phenotype in sar1 axr1 might be related to the RUBylation pathway. It has been suggested that AXR1 functions during meiotic recombination through the activation of a CRL4 (CULLIN RING LIGASE4) complex involved in the ubiquitylation of specific protein targets, since a cul4 mutant exhibit a meiotic phenotype reminiscent of that observed in axr1 (Jahns et al., 2014). Curiously, the SUMO and ubiquitin-proteasome systems function coordinately in meiotic chromosome organization and the regulation of meiotic recombination in mouse (Prasada Rao et al., 2017). The presence of upregulated SUMOylated proteins in sar1 may somehow compensate for the lack of CRL4 activity in axr1. Further analyses will be required to connect the function of these post-translational modifications to the NPCs.
During meiosis, LINC complexes contribute to promote telomere-driven chromosome movement at prophase I and this function is highly conserved in evolution (Kim et al., 2022). Strikingly, these complexes are also required for the distribution of NPCs in the NE (Liu et al., 2007). Surprisingly, few studies have analyzed the distribution of NPCs in plants, although they appear to have a non-homogeneous distribution during the early stages of meiosis (Holm, 1977; Zickler and Kleckner, 1998; Cowan et al., 2002). Similarly, little is known about how NPCs can influence chromosome behavior during meiosis. This study reveals a meiotic role for SAR1 and SAR3, scaffold nucleoporins belonging to the outer ring complex, in plant meiosis. These findings will lead to new lines of research to better understand how NE organization is modulated in the dynamic chromosome events during meiosis and the specific function of NPCs in this type of cell division.
The original contributions presented in the study are included in the article/Supplementary Material, further inquiries can be directed to the corresponding author.
NF-J: Conceptualization, Data curation, Formal Analysis, Investigation, Methodology, Writing–original draft, Writing–review and editing. MM-G: Data curation, Formal Analysis, Investigation, Writing–review and editing. JV: Conceptualization, Investigation, Writing–review and editing. FG-D: Data curation, Investigation, Writing–review and editing. JS: Formal Analysis, Writing–review and editing. MP: Conceptualization, Formal Analysis, Funding acquisition, Supervision, Writing–original draft, Writing–review and editing.
The author(s) declare financial support was received for the research, authorship, and/or publication of this article. This research was supported by the Ministry of Science and Innovation (PID2020-118038GB-I00/AEI/10.13039/501100011033) to MP and by the PhD fellow funded by the FPU program of Spanish Ministry of Education (FPU16/02772) to NF-J. MP also acknowledges the current support of the European Union (TED2021-131852B-I00/AEI/10.13039/501100011033/Unión Europea NextGenerationEU/PRTR).
We thank B. Martín and M. C. Moreno for excellent technical assistance, Prof. C. Franklin (University of Birmingham, United Kingdom) for anti-RAD51, anti-MLH1, anti-ASY1, and anti-ZYP1 antibodies, and Spanish Meiosis Community and members of the COST Action n° CA 16212 “INDEPTH” for helpful discussions about this research.
Author JV was employed by GlaxoSmithKline Spain.
The remaining authors declare that the research was conducted in the absence of any commercial or financial relationships that could be construed as a potential conflict of interest.
The reviewer ODI declared a past coauthorship with the author MP to the handling editor.
All claims expressed in this article are solely those of the authors and do not necessarily represent those of their affiliated organizations, or those of the publisher, the editors and the reviewers. Any product that may be evaluated in this article, or claim that may be made by its manufacturer, is not guaranteed or endorsed by the publisher.
The Supplementary Material for this article can be found online at: https://www.frontiersin.org/articles/10.3389/fcell.2023.1285695/full#supplementary-material
Alber, F., Dokudovskaya, S., Veenhoff, L. M., Zhang, W., Kipper, J., Devos, D., et al. (2007). The molecular architecture of the nuclear pore complex. Nature 450, 695–701. doi:10.1038/nature06405
Armstrong, S. J., Caryl, A. P., Jones, G. H., and Franklin, F. C. H. (2002). Asy1, a protein required for meiotic chromosome synapsis, localizes to axis-associated chromatin in Arabidopsis and Brassica. J. Cell Sci. 115, 3645–3655. doi:10.1242/jcs.00048
Armstrong, S. J., Sanchez-Moran, E., Chris, F., and Franklin, H. (2009). Cytological Analysis of Arabidopsis thaliana meiotic chromosomes. Methods Mol. Biol. 558, 131–145. doi:10.1007/978-1-60761-103-5_9
Beck, M., and Hurt, E. (2017). The nuclear pore complex: understanding its function through structural insight. Nat. Rev. Mol. Cell Biol. 18, 73–89. doi:10.1038/nrm.2016.147
Blobel, G. (1985). Gene gating: a hypothesis. Proc. Natl. Acad. Sci. 82, 8527–8529. doi:10.1073/pnas.82.24.8527
Bulankova, P., Akimcheva, S., Fellner, N., and Riha, K. (2013). Identification of Arabidopsis meiotic cyclins reveals functional diversification among plant cyclin Genes. PLoS Genet. 9, e1003508. doi:10.1371/journal.pgen.1003508
Burke, B. (2018). LINC complexes as regulators of meiosis. Curr. Opin. Cell Biol. 52, 22–29. doi:10.1016/j.ceb.2018.01.005
Campell, B. R., Song, Y., Posch, T. E., Cullis, C. A., and Town, C. D. (1992). Sequence and organization of 5S ribosomal RNA-encoding genes of Arabidopsis thaliana. Gene 112, 225–228. doi:10.1016/0378-1119(92)90380-8
Cernac, A., Lincoln, C., Lammer, D., and Estelle, M. (1997). The SAR1 gene of Arabidopsis acts downstream of the AXR1 gene in auxin response. Development 124, 1583–1591. doi:10.1242/dev.124.8.1583
Cheng, Z., Zhang, X., Huang, P., Huang, G., Zhu, J., Chen, F., et al. (2020). NUP96 and HOS1 are mutually stabilized and gate constans protein level, conferring long-day photoperiodic flowering regulation in Arabidopsis. Plant Cell 32, 374–391. doi:10.1105/tpc.19.00661
Chu, D. B., Gromova, T., Newman, T. A. C., and Burgess, S. M. (2017). The nucleoporin Nup2 contains a meiotic-autonomous region that promotes the dynamic chromosome events of meiosis. Genetics 206, 1319–1337. doi:10.1534/genetics.116.194555
Cowan, C. R., Carlton, P. M., and Cande, W. Z. (2002). Reorganization and polarization of the meiotic bouquet-stage cell can be uncoupled from telomere clustering. J. Cell Sci. 115, 3757–3766. doi:10.1242/jcs.00054
De Storme, N., and Geelen, D. (2011). The Arabidopsis mutant jason produces unreduced first division restitution male gametes through a parallel/fused spindle mechanism in meiosis II. Plant Physiol. 155, 1403–1415. doi:10.1104/pp.110.170415
Ding, X., Xu, R., Yu, J., Xu, T., Zhuang, Y., and Han, M. (2007). SUN1 is required for telomere attachment to nuclear envelope and gametogenesis in mice. Dev. Cell 12, 863–872. doi:10.1016/j.devcel.2007.03.018
Erilova, A., Brownfield, L., Exner, V., Rosa, M., Twell, D., Scheid, O. M., et al. (2009). Imprinting of the Polycomb group gene MEDEA serves as a ploidy sensor in Arabidopsis. PLoS Genet. 5, e1000663. doi:10.1371/journal.pgen.1000663
Fernandez-Martinez, J., and Rout, M. P. (2021). One ring to rule them all? structural and functional diversity in the nuclear pore complex. Trends biochem. Sci. 46, 595–607. doi:10.1016/j.tibs.2021.01.003
Fiserova, J., Kiseleva, E., and Goldberg, M. W. (2009). Nuclear envelope and nuclear pore complex structure and organization in tobacco BY-2 cells. Plant J. 59, 243–255. doi:10.1111/j.1365-313X.2009.03865.x
Fuchs, J., Demidov, D., Houben, A., and Schubert, I. (2006). Chromosomal histone modification patterns - from conservation to diversity. Trends Plant Sci. 11, 199–208. doi:10.1016/j.tplants.2006.02.008
Gerlach, W. L., and Bedbrook, J. R. (1979). Cloning and characterization of ribosomal RNA genes from wheat and barley. Nucleic Acids Res. 7, 1869–1885. doi:10.1093/nar/7.7.1869
Grelon, M., Vezon, D., Gendrot, G., and Pelletier, G. (2001). AtSPO11-1 is necessary for efficient meiotic recombination in plants. EMBO J. 20, 589–600. doi:10.1093/emboj/20.3.589
Gu, Y. (2018). The nuclear pore complex: a strategic platform for regulating cell signaling. New Phytol. 219, 25–30. doi:10.1111/nph.14756
Hattersley, N., Cheerambathur, D., Moyle, M., Stefanutti, M., Richardson, A., Lee, K. Y., et al. (2016). A nucleoporin docks protein phosphatase 1 to direct meiotic chromosome segregation and nuclear assembly. Dev. Cell 38, 463–477. doi:10.1016/j.devcel.2016.08.006
Hattersley, N., Schlientz, A. J., Prevo, B., Oegema, K., and Desai, A. (2022). MEL-28/ELYS and CENP-C coordinately control outer kinetochore assembly and meiotic chromosome-microtubule interactions. Curr. Biol. 32, 2563–2571.e4. doi:10.1016/j.cub.2022.04.046
Hendzel, M. J., Nishioka, W. K., Raymond, Y., Allis, C. D., Bazett-Jones, D. P., and Th’ng, J. P. H. (1998). Chromatin condensation is not associated with apoptosis. J. Biol. Chem. 273, 24470–24478. doi:10.1074/jbc.273.38.24470
Higgins, J. D., Sanchez-Moran, E., Armstrong, S. J., Jones, G. H., and Franklin, F. C. H. (2005). The Arabidopsis synaptonemal complex protein ZYP1 is required for chromosome synapsis and normal fidelity of crossing over. Genes Dev. 19, 2488–2500. doi:10.1101/gad.354705
Hoelz, A., Debler, E. W., and Blobel, G. (2011). The Structure of the nuclear pore complex. Annu. Rev. Biochem. 80, 613–643. doi:10.1146/annurev-biochem-060109-151030
Holm, P. B. (1977). Three-dimensional reconstruction of chromosome pairing during the zygotene stage of meiosis in Lilium longiflorum (Thunb.). Carlsb. Res. Commun. 42, 103–151. doi:10.1007/bf02906489
Houben, A., Wako, T., Furushima-Shimogawara, R., Presting, G., Künzel, G., Schubert, I., et al. (1999). Short communication: the cell cycle dependent phosphorylation of histone H3 is correlated with the condensation of plant mitotic chromosomes. Plant J. 18, 675–679. doi:10.1046/j.1365-313X.1999.00496.x
Jackson, N., Sanchez-Moran, E., Buckling, E., Armstrong, S. J., Jones, G. H., and Franklin, F. C. H. (2006). Reduced meiotic crossovers and delayed prophase I progression in AtMLH3-deficient Arabidopsis. EMBO J. 25, 1315–1323. doi:10.1038/sj.emboj.7600992
Jahns, M. T., Vezon, D., Chambon, A., Pereira, L., Falque, M., Martin, O. C., et al. (2014). Crossover localisation is regulated by the neddylation posttranslational regulatory pathway. PLoS Biol. 12, e1001930. doi:10.1371/journal.pbio.1001930
Jung, J. H., Lee, H. J., Park, M. J., and Park, C. M. (2014). Beyond ubiquitination: proteolytic and nonproteolytic roles of HOS1. Trends Plant Sci. 19, 538–545. doi:10.1016/j.tplants.2014.03.012
Kerr, J. F. R., Wyllie, A. H., and Currie, A. R. (1972). Apoptosis: a basic biological phenomenon with wideranging implications in tissue kinetics. Br. J. Cancer 26, 239–257. doi:10.1038/bjc.1972.33
Kerzendorfer, C., Vignard, J., Pedrosa-Harand, A., Siwiec, T., Akimcheva, S., Jolivet, S., et al. (2006). The Arabidopsis thaliana MND1 homologue plays a key role in meiotic homologous pairing, synapsis and recombination. J. Cell Sci. 119, 2486–2496. doi:10.1242/jcs.02967
Kim, H. J., Liu, C., and Dernburg, A. F. (2022). How and why chromosomes interact with the cytoskeleton during meiosis. Genes (Basel). 13, 901. doi:10.3390/genes13050901
King, G. A., Wettstein, R., Varberg, J. M., Chetlapalli, K., Walsh, M. E., Gillet, L. C. J., et al. (2023). Meiotic nuclear pore complex remodeling provides key insights into nuclear basket organization. J. Cell Biol. 222, e202204039. doi:10.1083/jcb.202204039
Klutstein, M., and Cooper, J. P. (2014). The chromosomal courtship dance-homolog pairing in early meiosis. Curr. Opin. Cell Biol. 26, 123–131. doi:10.1016/j.ceb.2013.12.004
Klutstein, M., Fennell, A., Fernández-Álvarez, A., and Cooper, J. P. (2015). The telomere bouquet regulates meiotic centromere assembly. Nat. Cell Biol. 17, 458–469. doi:10.1038/ncb3132
Knockenhauer, K. E., and Schwartz, T. U. (2016). The nuclear pore complex as a flexible and dynamic gate. Cell 164, 1162–1171. doi:10.1016/j.cell.2016.01.034
Komachi, K., and Burgess, S. M. (2022). The Nup2 meiotic-autonomous region relieves inhibition of Nup60 to promote progression of meiosis and sporulation in Saccharomyces cerevisiae. Genetics 221, iyac045. doi:10.1093/genetics/iyac045
Komaki, S., and Schnittger, A. (2016). The spindle checkpoint in plants — a green variation over a conserved theme? Curr. Opin. Plant Biol. 34, 84–91. doi:10.1016/j.pbi.2016.10.008
Koszul, R., and Kleckner, N. (2009). Dynamic chromosome movements during meiosis: a way to eliminate unwanted connections? Trends Cell Biol. 19, 716–724. doi:10.1016/j.tcb.2009.09.007
Kurzbauer, M. T., Uanschou, C., Chen, D., and Schlögelhofer, P. (2012). The recombinases DMC1 and RAD51 are functionally and spatially separated during meiosis in Arabidopsis. Plant Cell 24, 2058–2070. doi:10.1105/tpc.112.098459
Lamm, N., Rogers, S., and Cesare, A. J. (2021). Chromatin mobility and relocation in DNA repair. Trends Cell Biol. 31, 843–855. doi:10.1016/j.tcb.2021.06.002
Lazaro, A., Valverde, F., Pineiro, M., and Jarillo, J. A. (2012). The Arabidopsis E3 ubiquitin ligase HOS1 negatively regulates CONSTANS abundance in the photoperiodic control of flowering. Plant Cell 24, 982–999. doi:10.1105/tpc.110.081885
Li, C., Liu, L., Teo, Z. W. N., Shen, L., and Yu, H. (2020). Nucleoporin 160 regulates flowering through anchoring HOS1 for destabilizing CO in Arabidopsis. Plant Commun. 1, 100033. doi:10.1016/j.xplc.2020.100033
Li, X., and Gu, Y. (2020). Structural and functional insight into the nuclear pore complex and nuclear transport receptors in plant stress signaling. Curr. Opin. Plant Biol. 58, 60–68. doi:10.1016/j.pbi.2020.10.006
Li, X. M., Yu, C., Wang, Z. W., Zhang, Y. L., Liu, X. M., Zhou, D., et al. (2013). DNA topoisomerase II is dispensable for oocyte meiotic resumption but is essential for meiotic chromosome condensation and separation in mice. Biol. Reprod. 89, 118–211. doi:10.1095/biolreprod.113.110692
Lin, D. H., and Hoelz, A. (2019). The structure of the nuclear pore complex (An Update). Annu. Rev. Biochem. 88, 725–783. doi:10.1146/annurev-biochem-062917-011901
Lincoln, C., Britton, J. H., and Estelle, M. (1990). Growth and development of the axr1 mutants of Arabidopsis. Plant Cell 2, 1071–1080. doi:10.1105/tpc.2.11.1071
Liu, Q., Pante, N., Misteli, T., Elsagga, M., Crisp, M., Hodzic, D., et al. (2007). Functional association of Sun1 with nuclear pore complexes. J. Cell Biol. 178, 785–798. doi:10.1083/jcb.200704108
Lowndes, N. F., and Toh, G. W.-L. (2005). DNA repair: the importance of phosphorylating histone H2AX. Curr. Biol. 15, R99–R102. doi:10.1016/j.cub.2005.01.029
Lutzmann, M., Kunze, R., Buerer, A., Aebi, U., and Hurt, E. (2002). Modular self-assembly of a Y-shaped multiprotein complex from seven nucleoporins. EMBO J. 21, 387–397. doi:10.1093/emboj/21.3.387
Manzanero, S., Arana, P., Puertas, M. J., and Houben, A. (2000). The chromosomal distribution of phosphorylated histone H3 differs between plants and animals at meiosis. Chromosoma 109, 308–317. doi:10.1007/s004120000087
Martinez-Garcia, M., Fernández-Jiménez, N., Santos, J. L., and Pradillo, M. (2020). Duplication and divergence: new insights into AXR1 and AXL functions in DNA repair and meiosis. Sci. Rep. 10, 8860–8913. doi:10.1038/s41598-020-65734-2
Martinez-Garcia, M., Schubert, V., Osman, K., Darbyshire, A., Sanchez-Moran, E., Franklin, F. C. H., et al. (2018). TOP II and chromosome movement help remove interlocks between entangled chromosomes during meiosis. J. Cell Biol. 217, 4070–4079. doi:10.1083/jcb.201803019
Meier, I., and Brkljacic, J. (2009). The nuclear pore and plant development. Curr. Opin. Plant Biol. 12, 87–95. doi:10.1016/j.pbi.2008.09.001
Meier, I., Richards, E. J., and Evans, D. E. (2017). Cell biology of the plant nucleus. Annu. Rev. Plant Biol. 68, 139–172. doi:10.1146/annurev-arplant-042916-041115
Mergner, J., and Schwechheimer, C. (2014). The NEDD8 modification pathway in plants. Front. Plant Sci. 5, 103. doi:10.3389/fpls.2014.00103
Mishra, R. K., Chakraborty, P., Arnaoutov, A., Fontoura, B. M. A., and Dasso, M. (2010). The Nup107-160 complex and γ-TuRC regulate microtubule polymerization at kinetochores. Nat. Cell Biol. 12, 164–169. doi:10.1038/ncb2016
Murashige, T., and Skoog, F. (1962). A revised medium for rapid growth and bio assays with Tobacco tissue cultures. Physiol. Plant. 15, 473–497. doi:10.1111/j.1399-3054.1962.tb08052.x
Murphy, S. P., Gumber, H. K., Mao, Y., and Bass, H. W. (2014). A dynamic meiotic SUN belt includes the zygotene-stage telomere bouquet and is disrupted in chromosome segregation mutants of maize (Zea mays L.). Front. Plant Sci. 5, 314–411. doi:10.3389/fpls.2014.00314
Muthuswamy, S., and Meier, I. (2011). Genetic and environmental changes in SUMO homeostasis lead to nuclear mRNA retention in plants. Planta 233, 201–208. doi:10.1007/s00425-010-1278-7
Nagai, S., Dubrana, K., Tsai-Pflugfelder, M., Davidson, M. B., Roberts, T. M., Brown, G. W., et al. (2008). Functional targeting of DNA damage to a nuclear pore-associated SUMO-dependent ubiquitin ligase. Science 322, 597–602. doi:10.1126/science.1162790
Nie, Y., Li, Y., Liu, M., Ma, B., Sui, X., Chen, J., et al. (2023). The nucleoporin NUP160 and NUP96 regulate nucleocytoplasmic export of mRNAs and participate in ethylene signaling and response in Arabidopsis. Plant Cell Rep. 42, 549–559. doi:10.1007/s00299-022-02976-6
Nordeen, S. A., Turman, D. L., and Schwartz, T. U. (2020). Yeast Nup84-Nup133 complex structure details flexibility and reveals conservation of the membrane anchoring ALPS motif. Nat. Commun. 11, 6060–6112. doi:10.1038/s41467-020-19885-5
Oliver, C., Pradillo, M., Corredor, E., and Cuñado, N. (2013). The dynamics of histone H3 modifications is species-specific in plant meiosis. Planta 238, 23–33. doi:10.1007/s00425-013-1885-1
Osman, K., Higgins, J. D., Sanchez-Moran, E., Armstrong, S. J., and Franklin, F. C. H. (2011). Pathways to meiotic recombination in Arabidopsis thaliana. New Phytol. 190, 523–544. doi:10.1111/j.1469-8137.2011.03665.x
Parry, G. (2014). Components of the Arabidopsis nuclear pore complex play multiple diverse roles in control of plant growth. J. Exp. Bot. 65, 6057–6067. doi:10.1093/jxb/eru346
Parry, G. (2015). The plant nuclear envelope and regulation of gene expression. J. Exp. Bot. 66, 1673–1685. doi:10.1093/jxb/erv023
Parry, G., Ward, S., Cernac, A., Dharmasiri, S., and Estelle, M. (2006). The Arabidopsis SUPPRESSOR OF AUXIN RESISTANCE proteins are nucleoporins with an important role in hormone signaling and development. Plant Cell 18, 1590–1603. doi:10.1105/tpc.106.041566
Pradillo, M., López, E., Linacero, R., Romero, C., Cuñado, N., Sanchez-Moran, E., et al. (2012). Together yes, but not coupled: new insights into the roles of RAD51 and DMC1 in plant meiotic recombination. Plant J. 69, 921–933. doi:10.1111/j.1365-313X.2011.04845.x
Pradillo, M., López, E., Romero, C., Sanchez-Moran, E., Cuñado, N., and Santos, J. L. (2007). An analysis of univalent segregation in meiotic mutants of Arabidopsis thaliana: a possible role for synaptonemal complex. Genetics 175, 505–511. doi:10.1534/genetics.106.067595
Pradillo, M., Varas, J., Oliver, C., and Santos, J. L. (2014). On the role of AtDMC1, AtRAD51 and its paralogs during Arabidopsis meiosis. Front. Plant Sci. 5, 23. doi:10.3389/fpls.2014.00023
Prasada Rao, H. B. D., Qiao, H., Bhatt, S. K., Bailey, L. R. J., Tran, H. D., Bourne, S. L., et al. (2017). A SUMO-ubiquitin relay recruits proteasomes to chromosome axes to regulate meiotic recombination. Science 355, 403–407. doi:10.1126/science.aaf6407
Raices, M., and D’Angelo, M. A. (2012). Nuclear pore complex composition: a new regulator of tissue-specific and developmental functions. Nat. Rev. Mol. Cell Biol. 13, 687–699. doi:10.1038/nrm3461
Reape, T. J., Molony, E. M., and McCabe, P. F. (2008). Programmed cell death in plants: distinguishing between different modes. J. Exp. Bot. 59, 435–444. doi:10.1093/jxb/erm258
Rieder, C. L., and Maiato, H. (2004). Stuck in division or passing through: what happens when cells cannot satisfy the spindle assembly checkpoint. Dev. Cell 7, 637–651. doi:10.1016/j.devcel.2004.09.002
Robles, L. M., Deslauriers, S. D., Alvarez, A. A., and Larsen, P. B. (2012). A loss-of-function mutation in the nucleoporin AtNUP160 indicates that normal auxin signalling is required for a proper ethylene response in Arabidopsis. J. Exp. Bot. 63, 2231–2241. doi:10.1093/jxb/err424
Ross, K. J., Fransz, P., and Jones, G. H. (1996). A light microscopic atlas of meiosis in Arabidopsis thaliana. Chromosom. Res. 4, 507–516. doi:10.1007/BF02261778
Sanchez-Moran, E. S., Armstrong, S. J., Santos, J. L., Franklin, F. C. H., and Jones, G. H. (2001). Chiasma formation in Arabidopsis thaliana accession Wassileskija and in two meiotic mutants. Chromosom. Res. 9, 121–128. doi:10.1023/A:1009278902994
Siddiqui, N. U., Stronghill, P. E., Dengler, R. E., Hasenkampf, C. A., and Riggs, C. D. (2003). Mutations in Arabidopsis condensin genes disrupt embryogenesis, meristem organization and segregation of homologous chromosomes during meiosis. Development 130, 3283–3295. doi:10.1242/dev.00542
Smith, S. J., Osman, K., and Franklin, F. C. H. (2014). The condensin complexes play distinct roles to ensure normal chromosome morphogenesis during meiotic division in Arabidopsis. Plant J. 80, 255–268. doi:10.1111/tpj.12628
Starr, D. A. (2009). A nuclear-envelope bridge positions nuclei and moves chromosomes. J. Cell Sci. 122, 577–586. doi:10.1242/jcs.037622
Strambio-De-Castillia, C., Niepel, M., and Rout, M. P. (2010). The nuclear pore complex: bridging nuclear transport and gene regulation. Nat. Rev. Mol. Cell Biol. 11, 490–501. doi:10.1038/nrm2928
Stuwe, T., Correia, A. R., Lin, D. H., Paduch, M., Lu, V. T., Kossiakoff, A. A., et al. (2015). Nuclear pores. Architecture of the nuclear pore complex coat. Sci. (80-. ) 347, 1148–1152. doi:10.1126/science.aaa4136
Tamura, K., Fukao, Y., Iwamoto, M., Haraguchi, T., and Hara-Nishimura, I. (2011). Identification and characterization of nuclear pore complex components in Arabidopsis thaliana. Plant Cell 22, 4084–4097. doi:10.1105/tpc.110.079947
Tamura, K., and Hara-Nishimura, I. (2013). The molecular architecture of the plant nuclear pore complex. J. Exp. Bot. 64, 823–832. doi:10.1093/jxb/ers258
Varas, J., Graumann, K., Osman, K., Pradillo, M., Evans, D. E., Santos, J. L., et al. (2015). Absence of SUN1 and SUN2 proteins in Arabidopsis thaliana leads to a delay in meiotic progression and defects in synapsis and recombination. Plant J. 81, 329–346. doi:10.1111/tpj.12730
Varas, J., and Pradillo, M. (2018). Immunolabeling protocols for studying meiosis in plant mutants defective for nuclear envelope components. Methods Mol. Biol. 1840, 237–247. doi:10.1007/978-1-4939-8691-0_17
Walther, T. C., Alves, A., Pickersgill, H., Loïodice, I., Hetzer, M., Galy, V., et al. (2003). The conserved Nup107-160 complex is critical for nuclear pore complex assembly. Cell 113, 195–206. doi:10.1016/S0092-8674(03)00235-6
Wang, J., Niu, B., Huang, J., Wang, H., Yang, X., Dong, A., et al. (2016). The PHD finger protein MMD1/DUET ensures the progression of male meiotic chromosome condensation and directly regulates the expression of the condensin gene CAP-D3. Plant Cell 28, 1894–1909. doi:10.1105/tpc.16.00040
Wiermer, M., Cheng, Y. T., Imkampe, J., Li, M., Wang, D., Lipka, V., et al. (2012). Putative members of the Arabidopsis Nup107-160 nuclear pore sub-complex contribute to pathogen defense. Plant J. 70, 796–808. doi:10.1111/j.1365-313X.2012.04928.x
Wijnker, E., and Schnittger, A. (2013). Control of the meiotic cell division program in plants. Plant Reprod. 26, 143–158. doi:10.1007/s00497-013-0223-x
Xu, D., Bai, J., Duan, Q., Costa, M., and Dai, W. (2009). Covalent modifications of histones during mitosis and meiosis. Cell Cycle 8, 3688–3694. doi:10.4161/cc.8.22.9908
Yang, F., Fernández-Jiménez, N., Tučková, M., Vrána, J., Cápal, P., Díaz, M., et al. (2021). Defects in meiotic chromosome segregation lead to unreduced male gametes in Arabidopsis SMC5/6 complex mutants. Plant Cell 33, 3104–3119. doi:10.1093/plcell/koab178
Yang, H., Asakawa, H., Li, F., Haraguchi, T., Shih, H., and Hiraoka, Y. (2023). A nuclear pore complex-associated regulation of SUMOylation in meiosis. Genes Cells 28, 188–201. doi:10.1111/gtc.13003
Yang, H. J., Asakawa, H., Haraguchi, T., and Hiraoka, Y. (2015). Nup132 modulates meiotic spindle attachment in fission yeast by regulating kinetochore assembly. J. Cell Biol. 211, 295–308. doi:10.1083/jcb.201501035
Yang, X., Makaroff, C. A., and Ma, H. (2003). The Arabidopsis MALE MEIOCYTE DEATH1 gene encodes a PHD-finger protein that is required for male meiosis. Plant Cell 15, 1281–1295. doi:10.1105/tpc.010447
Zhang, A., Wang, S., Kim, J., Yan, J., Yan, X., Pang, Q., et al. (2020a). Nuclear pore complex components have temperature-influenced roles in plant growth and immunity. Plant Cell Environ. 43, 1452–1466. doi:10.1111/pce.13741
Zhang, F., Ma, L., Zhang, C., Du, G., Shen, Y., Tang, D., et al. (2020b). The SUNdomain proteins OsSUN1 and OsSUN2 play critical but partially redundant roles in meiosis. Plant Physiol. 183, 1517–1530. doi:10.1104/pp.20.00140
Zickler, D., and Kleckner, N. (1998). The leptotene-zygotene transition of meiosis. Annu. Rev. Genet. 32, 619–697. doi:10.1146/annurev.genet.32.1.619
Keywords: Arabidopsis, meiosis, nuclear pore complex, NUP96, NUP160, SAR1, SAR3
Citation: Fernández-Jiménez N, Martinez-Garcia M, Varas J, Gil-Dones F, Santos JL and Pradillo M (2023) The scaffold nucleoporins SAR1 and SAR3 are essential for proper meiotic progression in Arabidopsis thaliana. Front. Cell Dev. Biol. 11:1285695. doi: 10.3389/fcell.2023.1285695
Received: 30 August 2023; Accepted: 21 November 2023;
Published: 04 December 2023.
Edited by:
Alexei Arnaoutov, Eunice Kennedy Shriver National Institute of Child Health and Human Development (NIH), United StatesReviewed by:
Inna Lermontova, Leibniz Institute of Plant Genetics and Crop Plant Research (IPK), GermanyCopyright © 2023 Fernández-Jiménez, Martinez-Garcia, Varas, Gil-Dones, Santos and Pradillo. This is an open-access article distributed under the terms of the Creative Commons Attribution License (CC BY). The use, distribution or reproduction in other forums is permitted, provided the original author(s) and the copyright owner(s) are credited and that the original publication in this journal is cited, in accordance with accepted academic practice. No use, distribution or reproduction is permitted which does not comply with these terms.
*Correspondence: Mónica Pradillo, cHJhZGlsbG9AYmlvLnVjbS5lcw==
Disclaimer: All claims expressed in this article are solely those of the authors and do not necessarily represent those of their affiliated organizations, or those of the publisher, the editors and the reviewers. Any product that may be evaluated in this article or claim that may be made by its manufacturer is not guaranteed or endorsed by the publisher.
Research integrity at Frontiers
Learn more about the work of our research integrity team to safeguard the quality of each article we publish.