- 1Department of Gastric and Colorectal Surgery, General Surgery Center, The First Hospital of Jilin University, Changchun, China
- 2Division of Clinical Epidemiology, The First Hospital of Jilin University, Changchun, China
The occurrence and progression of tumors are inseparable from glucose metabolism. With the development of tumors, the volume increases gradually and the nutritional supply of tumors cannot be fully guaranteed. The tumor microenvironment changes and glucose deficiency becomes the common stress environment of tumors. Here, we discuss the mutual influences between glucose deprivation and other features of the tumor microenvironment, such as hypoxia, immune escape, low pH, and oxidative stress. In the face of a series of stress responses brought by glucose deficiency, different types of tumors have different coping mechanisms. We summarize the tumor studies on glucose deficiency in the last decade and review the genes and pathways that determine the fate of tumors under harsh conditions. It turns out that most of these genes help tumor cells survive in glucose-deprivation conditions. The development of related inhibitors may bring new opportunities for the treatment of tumors.
1 Background
In eukaryotes, metabolic pathways occur within the cytoplasm and mitochondria of cells and most of the energy in animal cells is provided by glucose or fatty acids (Judge and Dodd, 2020). Large amounts of nutrients can provide energy, reductive equivalents, and biosynthetic precursors to support the survival, proliferation, and malignant progression of cancer cells (Brunner and Finley, 2021). However, due to the unlimited proliferation of the cancer cells, the nutrients, such as glucose and glutamine, were always in shortage in the environment, inducing energy stress. And metabolic reprogramming has been widely observed during cancer development under such energy stress, which enables cancer cells to survive and proliferate (Li and Zhang, 2016) and is now recognized as a hallmark of cancer (Hanahan and Weinberg, 2011).
Glucose is the most available nutrient for cancer cells. Cancer cells require a higher glucose supply than normal cells to maintain their rapid proliferation (Yue et al., 2021). Normal tissues use glycolysis to produce about 10% of cellular ATP, of which mitochondria account for 90%. However, more than 50% of the tumor’s cellular energy is produced by glycolysis, with the rest produced by mitochondria. Interestingly, this transition occurs even when the oxygen is enough to support mitochondrial function, which is called Warburg effect (Warburg, 1956). Tumor cells rely on glycolysis for energy production, causing them to consume more glucose, which then accelerates the energy stress (Denko, 2008).
The tumor microenvironment is significant for the proliferation, metastasis of tumor cells. Low glucose, hypoxia, immune escape, low pH, and oxidative stress were the main characteristics of the tumor microenvironment. In this paper, we reviewed the crosstalk among the above characteristics and the effects of glucose deprivation on tumorigenesis and development in the past decade.
2 The main characteristics of the physical tumor microenvironment (TME)
TME is complicated, including a social microenvironment and a physical microenvironment. The social microenvironment includes all non-cancerous host cells in the tumor, including fibroblasts, adipocytes, endothelial cells, neurons, adaptive and innate immune cells, as well as its non-cellular components, including extracellular matrix (ECM), and soluble products, such as chemokines, cytokines, growth factors, and extracellular vesicles. All organisms living in the social microenvironment will encounter hypoxia, immune surveillance, low pH, oxidative stress, nutrient deprivation and competition, and physical pressure which together make up the physical microenvironment (Sun et al., 2018; Xiao and Yu, 2021).
As we know, cancer-associated fibroblasts (CAFs) are considered to be key mediators of interactions between malignant tumor cells and their microenvironment (Barron and Rowley, 2012; Franco and Hayward, 2012). Quiescent or resting fibroblasts are inert, spindle-shaped single cells embedded in the interstitial space of the ECM. When the quiescent fibroblasts are activated, they gain further secretory phenotypes, such as generating cytokines and chemokines, recruiting immune cells, synthesizing ECM, and exerting physical forces to modify tissue structure (Kalluri, 2016). Then ECM is remodeled and the interstitial pressure rises, impeding the activity of the vascular system (Jain et al., 2014; DuFort et al., 2016). In addition, tumors have dilated and tortuous blood vessels with uneven vascular density and diameter (Katayama et al., 2019). Therefore, poor vascular development and vascular leakage lead to the failure of nutrient delivery, metabolic waste removal difficulties, and obstruction of gas exchange. This causes nutrient deficiency such as glucose, metabolic waste deposits such as lactate, and a state of hypoxia, as shown in Figure 1.
2.1 Hypoxia
In primary tumors, hypoxia may occur within the mass due to impaired vascularization. Hypoxia is common in locally advanced solid tumors and has become an important factor in tumor physiology due to its ability to promote tumor initiation, progression, and treatment resistance (Carnero and Lleonart, 2016). Hypoxia will further increase the dependence of tumor cells on glycolysis, upregulating glucose transporters such as GLUT1 and enzymes associated with glycolytic pathways (Buono and Longo, 2018; Al Tameemi et al., 2019). Hypoxia also increases the levels of hypoxia-inducible transcription factors 1α (HIF-1α) and HIF-2α, thereby upregulating glycolysis (Shaw, 2006; Semenza, 2010; Kierans and Taylor, 2021). As a result, more glucose is consumed, which can lead to glucose deficiency. In addition, the expression of HIF-mediated gene products promotes vascular network regeneration to reverse hypoxia. However, the newly formed blood vessels are often irregular and distorted, which are inefficient in material transport and eventually lead to nutrient limitation (Brahimi-Horn et al., 2007; Rey and Semenza, 2010), including glucose limitation.
2.2 Immune escape
Immune cells in TME include early immune infiltrating cells such as lymphocytes, natural killer cells (NK), macrophages, and dendritic cells (DCs). These cells are suppressed by the action of immunosuppressive cells, such as regulatory T cells (Tregs), myeloid-derived suppressor cells (MDSCs), and type 2-polarized macrophages (M2) (Pitt et al., 2016). Nutritional competition and metabolic interactions between cancer cells and T cells are thought to be key drivers of tumorigenesis. Fast-growing cancer cells will consume most of the nutrients, and immune cells must metabolically adapt to these changes to perform necessary functions when subjected to local nutrient deprivation (Chang et al., 2015). On the other hand, metabolic changes occurring in cancer cells will affect the function of immune cells and promote immune evasion of tumors (Cassim and Pouyssegur, 2019). Glucose-deficient tumor microenvironment limited aerobic glycolysis, and altered the production of IFN-γ, thereby impairing proliferation, cytokine production, and cytolysis of tumor-infiltrating T cells (Cham and Gajewski, 2005; Cham et al., 2008; Chang et al., 2015; Ho et al., 2015).
AMP-activated protein kinase (AMPK) is an indirect glucose sensor and mTOR complex 1 (mTORC1) is an important metabolic regulator controlling NK cell differentiation, shaping T-cell differentiation, and regulating the function of antigen-presenting DCs. The altered AMPK-mTORC1 signaling pathway due to glucose limitation would suppress NK cell and inflammatory T cell responses, promoted Treg differentiation, and increased DC pro-inflammatory output (Kedia-Mehta and Finlay, 2019). Taken together, glucose is of great importance to immune surveillance.
2.3 Acidic environment
Acidosis, a constant stressor of most tumor cells, is formed by the fermentation of glucose into lactate in normoxic or hypoxic regions (Ordway et al., 2021). Cancer cells undergo a high rate of glycolysis despite aerobic conditions, leading to glucose consumption and increased lactate production in tumor cells (Gwangwa et al., 2018). To maintain the homeostasis of intracellular pH levels, cancer cells need to actively transport lactate into the extracellular space (Yan et al., 2019). The major players in cancer extracellular acidification are the monocarboxylate transport (MCT) proteins, specifically MCT1/4, whose expressions are elevated to move the accumulation of lactic acid and H+(Ordway et al., 2021).
H+ ions flow from the tumor into adjacent normal tissue along a concentration gradient, causing tissue remodeling. The resulting acidic environment is also toxic to normal cells and promotes the proteinase degradation of ECM. However, cancer cells invaded and occupied the degrading matrix of normal cells (Gottfried et al., 2012; Estrella et al., 2013), increased angiogenesis through the release of VEGF, and suppressed the immune response to tumor antigens. It has been suggested that an acidic pH is essential for tumorigenesis, invasion, and metastasis (Gwangwa et al., 2018).
2.4 Oxidative stress
The glucose deprivation and hypoxia reduced ATP production and accelerated the production and accumulation of reactive oxygen species (ROS) (Ren and Shen, 2019). Glucose deprivation can also induce oxidative stress and mitochondrial dysfunction in rat pheochromocytoma (PC12) cells and human cancer cells, with cytotoxic effects due to ATP depletion and ROS accumulation (Liu et al., 2003; Ahmad et al., 2005). It has been suggested that oxidative stress induced by glucose deprivation activates gene expression and signal transduction in tumors (Blackburn et al., 1999). Glucose depletion can regulate multiple cellular processes by activating miRNA expression through oxidative stress and inhibition of histone deacetylation (Druz et al., 2012).
Under chronic metabolic oxidative stress conditions, cancer cells may upregulate glucose metabolism and produce more NADPH and pyruvate to prevent their toxicity (Spitz et al., 2000; Simons et al., 2009). Nicotinamide phosphoribosyltransferase (NAMPT), a rate-limiting enzyme involved in NAD+ biosynthesis, protects tumor cells from glucose deprivation-induced oxidative stress by maintaining NADPH levels (Hong et al., 2016). It has been suggested that oxidative stress induced by glucose deprivation is associated with aggresome formation and autophagy in cultured cardiomyocytes (Marambio et al., 2010). In addition, oxidative stress induced by glucose starvation triggers the LKB1-AMPK signaling pathway to facilitate selective autophagy, thereby enhancing Keap1 degradation and the Nrf2 activation (Endo et al., 2018). Hexokinase-II (HK-II) regulates glucose starvation-induced autophagy by binding to and inhibiting the autophagy suppressor, mTORC1, switching cells from an energy-sufficient metabolic economy to a conserved economy under starvation (Roberts et al., 2014).
2.5 Crosstalk among the physical tumor microenvironment
Low glucose, hypoxia, immune escape, low pH, and oxidative stress were the main characteristics of the physical tumor microenvironment, and they influence each other mutually. Low glucose will promote the proliferation of vascular endothelial cells by inhibiting VEGFR2 O-GlcNAcylation and its proteasomal degradation (Deng, 2023). Hypoxia not only promotes angiogenesis through HIF-1-dependent processes (Augustin et al., 2020) but also through lactic acidosis (Singh et al., 2023). At the same time, the hypoxic response will also cause the enhancement of tumor glycolysis and lactic acid deposition. The buildup of lactic acid acidifies the TME and then affects the recognition and response of the immune system to the tumor. Under the condition of nutrition restriction, tumor immunity will also be influenced (Lyssiotis and Kimmelman, 2017). And the crosstalk among different constituents in the physical microenvironment was shown in Figure 2.
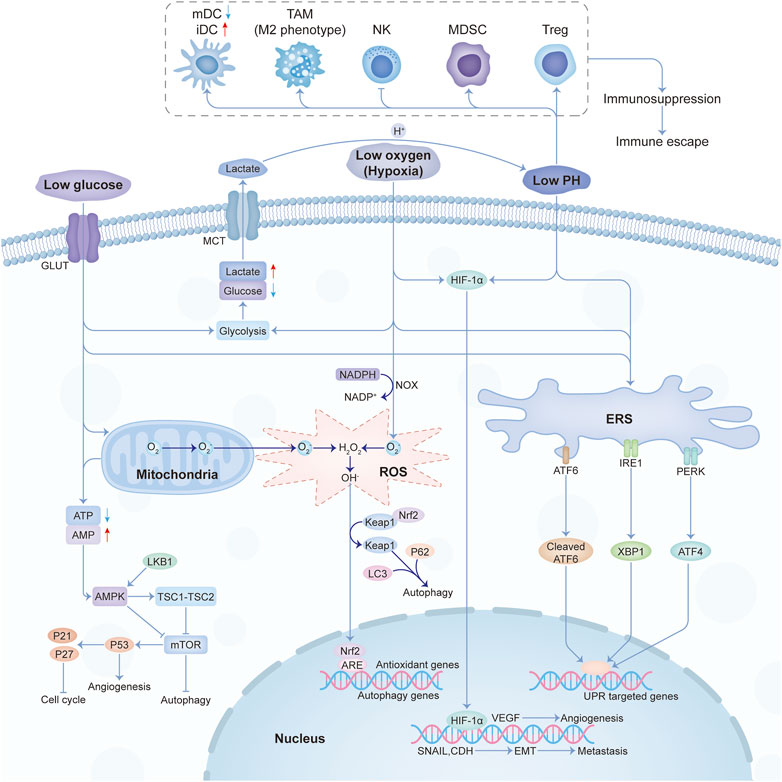
FIGURE 2. The crosstalk among the main constituents of the physical tumor microenvironment. Abbreviations: mDC, mature dendritic cell; iDC, immature dendritic cell; TAM, tumor-associated macrophages; NK, natural killer; MDSC, myeloid-derived suppressor cell; Treg, T regulatory.
2.6 Physical tumor microenvironment derived important biological processes
2.6.1 Endoplasmic reticulum stress (ERS)
The protein-folding capacity of the endoplasmic reticulum (ER) in tumor cells and infiltrating immune cells are altered under harsh microenvironmental conditions, which promotes the accumulation of misfolded or unfolded proteins, leading to ERS (Chen and Cubillos-Ruiz, 2021). Hypoxia will trigger ERS by affecting disulfide bond formation and protein folding to different degrees (May et al., 2005; Koumenis and Wouters, 2006). Glucose restriction interrupts the hexosamine biosynthetic pathway (HBP), which affects protein glycosylation and protein folding (Ricciardiello et al., 2020; Lam et al., 2021). Intracellular ROS accumulation (Shimizu and Hendershot, 2009) and acidosis (Maeyashiki et al., 2020) also readily inhibit the protein-folding capacity of the ER, thus triggering a sustained ERS response. Then intracellular signal transduction pathways are activated, which is called the unfolded protein response (UPR) (Ron and Walter, 2007).
Three different types of ER stress transducers were identified to be involved in this process, including activating transcription factor 6 (ATF6), inositol requiring protein 1 (IRE1), and protein kinase RNA (PKR)-like ER kinase (PERK). To alleviate the accumulation of misfolded proteins, signal transduction events are induced by these transducers. A process called ERAD (ER-associated degradation), which stimulates the retrograde transport of misfolded proteins from the ER into the cytosol for ubiquitination and destruction, is involved (Sano and Reed, 2013). In addition, four protein kinases, including PKR, PERK, general control nonderepressible 2 (GCN2), and heme-regulated inhibitor (HRI), can phosphorylate eIF2α in response to stressors (Nakagawa and Ohta, 2019). PKR, GCN2, and HRI can be independent of the ERS pathway, and this part of the UPR is called the integrated stress response (ISR) (Ron and Walter, 2007). In addition to coordinating ER function to restore homeostasis, this series of reactions also alter immune cell function in TME to influence tumor malignant progression. ER-stressed tumor cells alter NK cell-mediated tumor recognition, release other factors to recruit or alter myeloid cell function, as well as regulating T cell-mediated tumor growth, metastasis, and response to immunotherapy. Moreover, activation of UPR may help promote a dormant state for stressed tumor cells and maintain their initial survival (Chen and Cubillos-Ruiz, 2021).
2.6.2 Autophagy
Autophagy plays a dynamic inhibitory or promoting role in different stages of tumor development. As a survival pathway and quality control mechanism, autophagy can prevent tumorigenesis and inhibit cancer progression in early tumorigenesis. Once a tumor progresses to an advanced stage and is subjected to environmental stress, autophagy will act as a dynamic degradation and recycling system that contributes to the survival and growth of established tumors while enhancing cancer aggressiveness by promoting metastasis (Li et al., 2020a). Under metabolic stress conditions such as glucose deficiency, tumor cells in TME promote cell survival by activating autophagy, which is closely related to the mTOR and AMPK pathways (Kimmelman and White, 2017). Hypoxia can activate AMPK, HIF-1α, or ATF4, and induce downstream gene expression to activate autophagy to mediate cell survival (Rouschop et al., 2010; Hu et al., 2012; Di Conza et al., 2017a). Oxidative stress can induce autophagy through nuclear factor-κb (NF-κB) or LKB1-AMPK, and activated autophagy can also promote antioxidant response through Keap1-Nrf2 to alleviate oxidative stress (Alexander et al., 2010; Taguchi et al., 2012; Song et al., 2017). Moreover, autophagy is involved in the survival, apoptosis, and differentiation of immune cell subsets (Xia et al., 2021). Targeting the autophagy pathway to improve the efficacy of immunotherapy will be a promising area.
3 The effects of glucose deprivation on tumorigenesis and development
In the past decade, there have been a lot of cancer studies on glucose deprivation. Among these studies, researchers have found many important molecules for tumor survival or death, which play a direct or indirect role in response to energy stress, as shown in Figures 3, 4. Several tumor types with a large number of studies are shown in Supplementary Table S1.
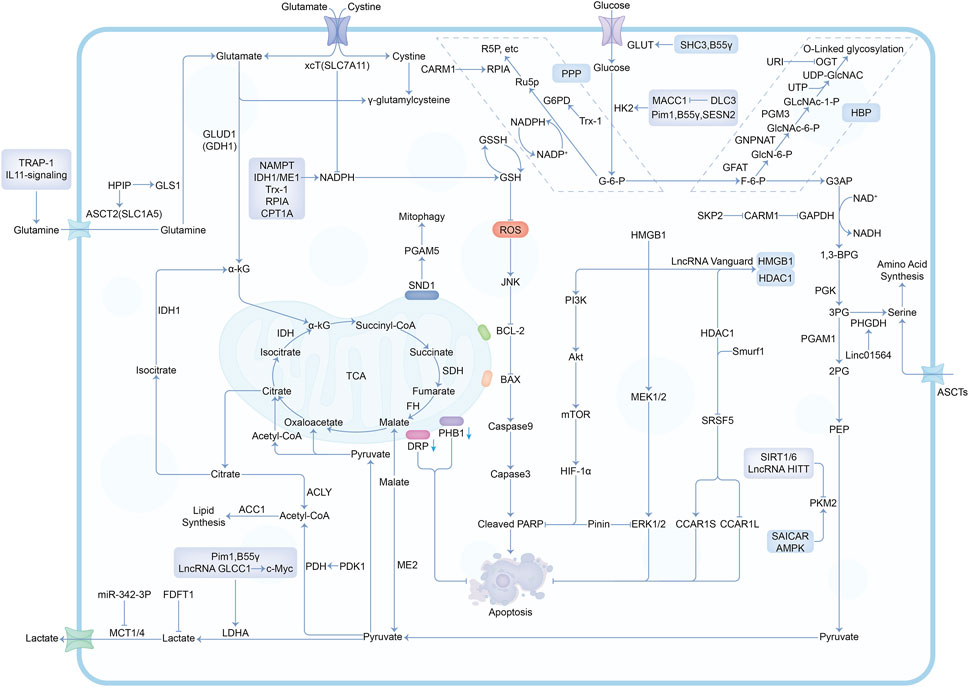
FIGURE 4. The mechanisms and molecules related to metabolic pathways under glucose deprivation conditions. Abbreviations: G-6-P, Glucose-6-phosphate; F-6-P, Fructose-6-phosphate; G3AP, Glyceraldehyde-3-phosphate; 1,3-BPG, 1,3-Biphosphoglycerate; 3PG, 3-Phosphoglycerate; 2PG, 2-Phosphoglycerate; PEP, Phosphoenolpyruvate; Ru5P, Ribulose-5-phosphate; R5P, Ribose-5-phosphate; GlcN-6-P, Glucosamine-6-phosphate; GlcNAc-6-P, N-acetylglucosamine-6-phosphate; GlcNAc-1-P, N-acetylglucosamine-1-phosphate; PPP, Pentose Phosphate Pathway; HBP, Hexosamine Biosynthesis Pathway.
3.1 Pan-cancer
Studies involving a variety of tumors have suggested that many molecules participated in the response to energy stress. Glucose deficiency necessarily affects metabolic homeostasis and the enzymes involved in glycolysis. SRSF5 (Serine and arginine-rich splicing factor 5), a member of the serine/arginine (SR)-rich family of pre-mRNA splicing factors, promotes the production of CCAR1S proteins by alternative splicing CCAR1 which enhances glucose consumption and acetyl-coA production, thus promoting tumor growth (Chen et al., 2018). SAICAR can alter cellular energy levels, glucose uptake, and lactate production. SAICAR-PKM2 interaction promoted cancer cell survival under glucose limitation conditions (Keller et al., 2012). In addition, many molecules related to AMPK as well as the production of GSH, NADPH, and ATP are associated with glucose deprivation. For example, MDK inhibits both basal and stress-induced activation of AMPK by disrupting the LKB1-STRAD-Mo25 complex (Xia et al., 2022). AMPK will also phosphorylate the CHK1 domain for subsequent ubiquitination and degradation, which causes DNA mutagenesis and affects the cell cycle and tumor progression (Ma et al., 2019). ME1 produces NADPH in the cytoplasm, which acts as a reducing agent and affects macromolecular biosynthesis and redox homeostasis (Murai et al., 2017). PLD1 inhibition will block fatty acid oxidation (FAO) and inhibits ATP production, increasing ROS and leading to cancer cell death (Cai et al., 2016). PKA can regulate different genes involved in glutaminolysis by coordinating their transcription (Palorini et al., 2016). Such a coordinated regulation applies to oncogenes such as c-myc (Sun et al., 2015). Besides, some molecules are also involved in cell death-related pathways, including TRAIL receptor-related apoptosis (Iurlaro et al., 2017), necrosis (Khan et al., 2017), and disulfidptosis (Liu et al., 2023).
3.2 Liver cancer and pancreatic cancer
In liver cancer, many molecules respond to energy stress by regulating proteins related to glycolysis and glutaminolysis. SESN2 affects glycolysis by destabilizing HK2 mRNA (Kumar et al., 2018; Li et al., 2023a). GDH1 can drive glutamine-derived carbon into the TCA cycle in response to glucose starvation (Zhou et al., 2022). RHOF promotes the Warburg effect by upregulating the expression and function of several glycolytic enzymes, including GLUT1, HK2, PDK1, and LDH (Li et al., 2021a). Meanwhile, there are also some regulatory pathways related to AMPK, such as autophagy and FAO (fatty acid oxidation), which not only affect cell fate but also cause changes in ATP production. SKP2 promotes HCC (hepatocellular carcinoma) progression and its autophagy-induced nuclear function via CARM1 and AMPK(Wei et al., 2018). GPx1 induces protective autophagy in PDA (pancreatic ductal adenocarcinoma) cells under extreme glucose starvation (Meng et al., 2018). HBx promotes FAO in HCC cells in the absence of glucose, thereby maintaining NADPH and ATP levels (Wang et al., 2016a). Upregulation of CPT-1A increases intracellular ATP required for PDA cell survival (Luo et al., 2016). Besides, some stress responses are involved in EMT (epithelial-mesenchymal transition) and angiogenesis, which are undoubtedly another interpretation of metastasis to facilitate tumor escape from harsh energy stress conditions. HSF1 is required for EMT-related migration of HCC cells under low glucose conditions (Liu et al., 2016). In human hepatoblastoma HepG2 cells, the AhR pathway will induce VEGF expression (Terashima et al., 2013). In addition, the researchers examined non-polar and lipid metabolites in the microenvironment. By characterizing polar small molecule nutrients in PDAC and LUAD tumors, we can identify the metabolic liabilities of cancer cells, which ultimately translates into more effective treatments (Sullivan et al., 2019). For example, one study suggests that forced hyperglycemia may provide a new treatment strategy for pancreatic cancer sensitization to chemotherapy (Vaziri-Gohar et al., 2023). Cell metabolism can respond to and adapt to environmental nutrient levels. In different nutritional environments, cancer cells can change their metabolic needs and response to drugs (Schug et al., 2015; Muir et al., 2017; Vaziri-Gohar et al., 2018).
3.3 Breast cancer and lung cancer
When faced with energy stress, the metabolism of the tumor is altered. Breast cancer cells induce the expression of proto-oncogene HPIP through the AMPK-FOXO3a pathway. HPIP reconnects glutaminolysis by controlling the expression of solute carrier family 1 member 5 (SLC1A5) and glutaminase (GLS) genes (Penugurti et al., 2021). KAP1 Ser473 phosphorylation in glucose-starved breast cancer cells restricts mitochondrial hyperfusion, leading to reduced oxidative phosphorylation (OXPHOS) and ROS production (Cheng et al., 2016). In addition to mechanisms related to metabolism, the stress mechanisms also include ERS and oxidative stress. Glucose-regulated protein 78 (GRP78) responds to ERS by inducing the unfolded protein response (UPR) to support cellular homeostasis and survival under stress conditions (Xiao et al., 2019). PPARδ regulates breast cancer cell survival under harsh microenvironmental conditions by reducing oxidative stress (Wang et al., 2016b). Nrf2 is a major regulator of antioxidant responses, and its increased activity protects breast cancer cells during glucose deprivation (Walker et al., 2018). Meanwhile, a vicious cycle between AMPK inactivation and ROS exists in LKB1-mutant non-small cell lung cancer (NSCLC) cells, which are susceptible to glucose starvation leading to cell death (Ren et al., 2021). Similarly, we noticed that many molecules were strongly associated with various types of cell death in these studies. Entosis is a cannibalistic process between cells that can resist metabolic stress, PCK2 affects the entosis process by controlling protein glycosylation (Hyroššová et al., 2022). Glucose deprivation evokes ERS and induces ORP150 expression to inhibit apoptosis and senescence of breast cancer cells (Krętowski et al., 2013). Glucose deprivation can also trigger ZBP1-dependent necroptosis in breast cancer (Baik et al., 2021). Apart from PHB1 (Raut et al., 2019) and NDRG2 (Kim et al., 2014) are associated with apoptosis under energy stress conditions in breast cancer, 4EBP1 can sense extracellular glucose deprivation and initiates lung cancer cell death (Wang et al., 2022).
3.4 Gastric cancer and colorectal cancer
In gastrointestinal cancer, many molecules affect cell fate under energy stress by regulating metabolism. The molecules, including lncRNA GLCC1(Tang et al., 2019), Pim1 (Zhang et al., 2018), and JMJD2B (Fu et al., 2018), are upregulated under glucose starvation in colorectal cancer (CRC) cells to support cell survival and proliferation by enhancing glycolysis. In gastric cancer, glucose starvation inhibits the malignant behavior through the miR-216a-5p/FDFT1 axis (Zhao et al., 2021). Meanwhile, gastric cancer cells can escape metabolic stress through DLC3/MACC1 axis (Lin et al., 2019). In this process, DLC3 expression is decreased and MACC1 expression is increased. MACC1 then promotes the Warburg effect by upregulating a series of glycolytic enzymes (Lin et al., 2015). Tumor cells can also regulate migration and invasion to escape from energy stress. HMGB1 is involved in the stimulation of colonic myofibroblast migration and invasion under glucose deprivation (Sharma et al., 2016). Acss2/HIF-2 signaling is activated by glucose deprivation in colon cancer cells and is essential for cell migration and invasion (Garcia et al., 2023). In addition, some molecules are also associated with apoptosis. For example, HAP1 not only inhibits gastric cancer cell migration and invasion but also promotes cell death during glucose deprivation (Qu et al., 2023). In contrast, HIF-1α signaling was activated to acquire anti-apoptotic properties in colon cancer (Nishimoto et al., 2014). Another way to promote cell survival under energy stress is to enhance NADPH production, through which Trx-1 (Lu et al., 2022) and RPIA (Guo et al., 2020) support ROS clearance.
3.5 Glioma and other types of cancer
When glioma undergoes glucose deprivation, in addition to maintaining metabolic homeostasis by regulating glycolysis (Azzalin et al., 2020) and glutaminolysis (Stuart et al., 2023), many molecules mediate oxidative stress and apoptosis pathways to affect the outcome. ID2 inhibits ROS production, reduces mitochondrial damage, and enhances tumor cell survival (Zhang et al., 2017b). Meanwhile, overexpression of xCT (Yamaguchi et al., 2020; Yamamoto et al., 2021) or SIRT6(Sheikh et al., 2018) can induce ROS accumulation and cell death. In addition, there are many pathways involved in other types of cancer. MAT2A can promote the growth of cervical cancer cells under glucose deprivation by mediating the methylation of programmed cell death protein 6 (PDCD6) (Luo et al., 2022). LSR promotes ovarian cancer cell survival and tumor growth through the LKB1-AMPK pathway (Takahashi et al., 2021). GLUT1 protects prostate cancer cells from oxidative stress induced by glucose deprivation (Gonzalez-Menendez et al., 2018). GPX4-dependent ferroptosis was significantly enhanced by AMPK activation in renal cancer cells upon glucose deprivation. Moreover, in the presence of glucose deficiency, ASNS (Fang et al., 2020), and stathmin1 (Wang et al., 2021) promote the migration and invasion of esophageal squamous cell carcinoma (ESCC) cells and gallbladder carcinoma (GBC) cells, respectively.
4 Promising direction: Drug research
The tumor microenvironment is very complex and contains a variety of cells and components. Different cell subpopulations in the tumor microenvironment have different functions and influence tumor development through multiple mechanisms. Therapies that directly act on tumor cells have many shortcomings, such as tumor heterogeneity, genetic instability of cancer cells, drug resistance of cancer cells, etc. Targeting other components of the tumor microenvironment has gradually become the focus of research. This includes all non-cancerous host cells in the tumor, such as endothelial cells, fibroblasts, fat cells, immune cells, as well as its non-cellular components, including the extracellular matrix (ECM) and soluble products, such as cytokines, chemokines, growth factors, etc. Immune cells typically include T and B lymphocytes, tumor-associated macrophages (TAM), dendritic cells (DC), natural killer (NK) cells, neutrophils, and myeloid suppressor cells (MDSC).
There are many kinds of drugs. For example, for CAF and ECM, there are FAP monoclonal antibody, vitamin D analogue, PDGFR inhibitor, CXCR4 receptor antagonist and so on. Drugs that target the monocyte/macrophage populations are CCR2 blocking antibodies or antagonists, PI3K-γ inhibitor, CXCR1/2 antagonist, CD40 agonist antibodies, etc. Drugs targeting tumor vasculature include VEGF/VEGFR inhibitors, ANG inhibitors and so on. Despite the multitude of targets, many clinical trials targeting TME have failed to show promising efficacy in cancer patients. The only exception is immunotherapy, including immune checkpoint blocking therapy, such as anti-PD1/PDL1 therapy, have shown significantly higher efficacy in treating tumors with pre-existing anti-tumor immunity. Moreover, people are beginning to realize that combination therapy may have greater value. With the development of cutting-edge technologies such as single-cell multi-omics and artificial intelligence, we will decipher TME through multi-omics profiling to further improve our understanding of TME. (Bejarano et al., 2021; Xiao and Yu, 2021).
Glucose deprivation, one of the characteristics of the tumor physical microenvironment that we focused on, we learned there are some preclinical and clinical studies in which combinations of drugs are tested in which the effect due to glucose deprivation is exploited. Under nutritional restrictions, allosteric inhibitors of wild-type isocitrate dehydrogenase 1 (wtIDH1) can be lethal to cancer cells (Vaziri-Gohar et al., 2022). Buformin, a UPR (unfolded protein response) inhibitor, effectively reduces the survival of kidney cancer cells with sensitive and resistant phenotypes under glucose deprivation conditions (Isono et al., 2014). Metformin, a drug used in the treatment of type II diabetes, works synergistically with glucose deprivation to inhibit triple-negative breast cancer cell proliferation by activating pro-apoptotic molecules through UPR (Li et al., 2023c). One study suggested that the use of insulin to treat hyperglycemia improved skeletal muscle protein and amino acid metabolism in cancer patients after major surgery (Biolo et al., 2008). Moreover, it has been suggested that a drug combination of niclosamide and quinacrine can inhibit melanoma proliferation and glucose intake (Li et al., 2023c). SCIC2.1, an activator of SIRT1, can promote energy homeostasis and alleviate metabolic stress in liver cancer cells subjected to glucose deprivation (Varghese et al., 2023). GBS-01, an extract from the fruit of Arctium lappa L, has been reported to attenuate the tolerance of cancer cells to glucose deprivation and exert anti-tumor activity. It plays a role in patients with gemcitabine refractory advanced pancreatic cancer (Ikeda et al., 2016). LKB1 is a key sensor of metabolic stress, including hypoxia and glucose deprivation, which are common in the tumor microenvironment exacerbated by antiangiogenic therapy. LKB1 has the potential to predict the sensitivity of advanced non-small cell lung cancer to bevacizumab (Bonanno et al., 2017). In addition, in liver cancer, another option other than surgery to kill the tumor is to block the blood supply through a process called embolization. This process deprives tumor cells of oxygen and nutrients such as glucose (Chao et al., 2016). This is actually related to the effects of glucose deprivation. anti-angiogenic therapy can also cause harsh hypoxia and glucose limiting microenvironments (Boso et al., 2023), and GLUT inhibitors can mimic glucose starvation (Yang et al., 2023).
5 Conclusion
The tumor microenvironment is closely related to the external conditions faced by tumors, and it has a complex and huge regulatory network. Starting with glucose deprivation, we can see the correlation between several features of the tumor microenvironment. At the same time, we have also learned many genes and pathways that determine the fate of tumor cells under glucose deprivation conditions. In addition to cell death due to stress, there are many mechanisms mediating tumor survival. Targeting these molecules and designing corresponding inhibitors may bring new opportunities for the treatment of tumors.
Author contributions
YC: Conceptualization, Visualization, Writing–original draft, Data curation. YS: Data curation, Visualization, Writing–review and editing. DL: Data curation, Visualization, Writing–review and editing. YuZ: Data curation, Visualization, Writing–review and editing. YaZ: Data curation, Visualization, Writing–review and editing. DC: Conceptualization, Writing–review and editing. XC: Conceptualization, Writing–review and editing.
Funding
The author(s) declare financial support was received for the research, authorship, and/or publication of this article. This work was supported by grants from the National Natural Science Foundation of China (82002932), the Jilin Province Department of Finance (JLSWSRCZX2021-073); and the Scientific and Technological Development Program of Jilin Province (20210101333JC).
Conflict of interest
The authors declare that the research was conducted in the absence of any commercial or financial relationships that could be construed as a potential conflict of interest.
Publisher’s note
All claims expressed in this article are solely those of the authors and do not necessarily represent those of their affiliated organizations, or those of the publisher, the editors and the reviewers. Any product that may be evaluated in this article, or claim that may be made by its manufacturer, is not guaranteed or endorsed by the publisher.
Supplementary material
The Supplementary Material for this article can be found online at: https://www.frontiersin.org/articles/10.3389/fcell.2023.1275543/full#supplementary-material
References
Ahmad, I. M., Aykin-Burns, N., Sim, J. E., Walsh, S. A., Higashikubo, R., Buettner, G. R., et al. (2005). Mitochondrial O2*- and H2O2 mediate glucose deprivation-induced stress in human cancer cells. J. Biol. Chem. 280 (6), 4254–4263. doi:10.1074/jbc.M411662200
Alexander, A., Cai, S. L., Kim, J., Nanez, A., Sahin, M., MacLean, K. H., et al. (2010). ATM signals to TSC2 in the cytoplasm to regulate mTORC1 in response to ROS. Proc. Natl. Acad. Sci. U. S. A. 107 (9), 4153–4158. doi:10.1073/pnas.0913860107
Al Tameemi, W., Dale, T. P., Al-Jumaily, R. M. K., and Forsyth, N. R. (2019). Hypoxia-Modified cancer cell metabolism. Front. Cell Dev. Biol. 7, 4. doi:10.3389/fcell.2019.00004
Augustin, R. C., Delgoffe, G. M., and Najjar, Y. G. (2020). Characteristics of the tumor microenvironment that influence immune cell functions: hypoxia, oxidative stress, metabolic alterations. Cancers (Basel) 12 (12), 3802. doi:10.3390/cancers12123802
Azzalin, A., Brambilla, F., Arbustini, E., Basello, K., Speciani, A., Mauri, P., et al. (2020). A new pathway promotes adaptation of human glioblastoma cells to glucose starvation. Cells 9 (5), 1249. doi:10.3390/cells9051249
Baik, J. Y., Liu, Z., Jiao, D., Kwon, H. J., Yan, J., Kadigamuwa, C., et al. (2021). ZBP1 not RIPK1 mediates tumor necroptosis in breast cancer. Nat. Commun. 12 (1), 2666. doi:10.1038/s41467-021-23004-3
Bao, Y., Qian, C., Liu, M. Y., Jiang, F., Jiang, X., Liu, H., et al. (2021). PRKAA/AMPKα phosphorylation switches the role of RASAL2 from a suppressor to an activator of autophagy. Autophagy 17 (11), 3607–3621. doi:10.1080/15548627.2021.1886767
Barron, D. A., and Rowley, D. R. (2012). The reactive stroma microenvironment and prostate cancer progression. Endocr. Relat. Cancer 19 (6), R187–R204. doi:10.1530/erc-12-0085
Bejarano, L., Jordāo, M. J. C., and Joyce, J. A. (2021). Therapeutic targeting of the tumor microenvironment. Cancer Discov. 11 (4), 933–959. doi:10.1158/2159-8290.cd-20-1808
Belo do Nascimento, I., Verfaillie, M., Ates, G., Beckers, P., Joris, V., Desmet, N., et al. (2022). AMPK modulates the metabolic adaptation of C6 glioma cells in glucose-deprived conditions without affecting glutamate transport. Cells 11 (11), 1800. doi:10.3390/cells11111800
Biolo, G., De Cicco, M., Lorenzon, S., Dal Mas, V., Fantin, D., Paroni, R., et al. (2008). Treating hyperglycemia improves skeletal muscle protein metabolism in cancer patients after major surgery. Crit. Care Med. 36 (6), 1768–1775. doi:10.1097/CCM.0b013e318174de32
Blackburn, R. V., Spitz, D. R., Liu, X., Galoforo, S. S., Sim, J. E., Ridnour, L. A., et al. (1999). Metabolic oxidative stress activates signal transduction and gene expression during glucose deprivation in human tumor cells. Free Radic. Biol. Med. 26 (3-4), 419–430. doi:10.1016/s0891-5849(98)00217-2
Bonanno, L., De Paoli, A., Zulato, E., Esposito, G., Calabrese, F., Favaretto, A., et al. (2017). LKB1 expression Correlates with increased survival in patients with advanced non-small cell lung cancer treated with chemotherapy and bevacizumab. Clin. Cancer Res. 23 (13), 3316–3324. doi:10.1158/1078-0432.ccr-16-2410
Boso, D., Tognon, M., Curtarello, M., Minuzzo, S., Piga, I., Brillo, V., et al. (2023). Anti-VEGF therapy selects for clones resistant to glucose starvation in ovarian cancer xenografts. J. Exp. Clin. Cancer Res. 42 (1), 196. doi:10.1186/s13046-023-02779-x
Brahimi-Horn, M. C., Chiche, J., and Pouysségur, J. (2007). Hypoxia and cancer. J. Mol. Med. Berl. 85 (12), 1301–1307. doi:10.1007/s00109-007-0281-3
Brucker, D. P., Maurer, G. D., Harter, P. N., Rieger, J., and Steinbach, J. P. (2016). FOXO3a orchestrates glioma cell responses to starvation conditions and promotes hypoxia-induced cell death. Int. J. Oncol. 49 (6), 2399–2410. doi:10.3892/ijo.2016.3760
Brunner, J. S., and Finley, L. W. S. (2021). SnapShot: cancer metabolism. Mol. Cell 81 (18), 3878–3878.e1. doi:10.1016/j.molcel.2021.06.021
Buono, R., and Longo, V. D. (2018). Starvation, stress resistance, and cancer. Trends Endocrinol. Metab. 29 (4), 271–280. doi:10.1016/j.tem.2018.01.008
Burén, S., Gomes, A. L., Teijeiro, A., Fawal, M. A., Yilmaz, M., Tummala, K. S., et al. (2016). Regulation of OGT by URI in response to glucose confers c-MYC-dependent survival mechanisms. Cancer Cell 30 (2), 290–307. doi:10.1016/j.ccell.2016.06.023
Cai, H., Jiang, D., Qi, F., Xu, J., Yu, L., and Xiao, Q. (2015). HRP-3 protects the hepatoma cells from glucose deprivation-induced apoptosis. Int. J. Clin. Exp. Pathol. 8 (11), 14383–14391.
Cai, M., He, J., Xiong, J., Tay, L. W., Wang, Z., Rog, C., et al. (2016). Phospholipase D1-regulated autophagy supplies free fatty acids to counter nutrient stress in cancer cells. Cell Death Dis. 7 (11), e2448. doi:10.1038/cddis.2016.355
Carnero, A., and Lleonart, M. (2016). The hypoxic microenvironment: a determinant of cancer stem cell evolution. Bioessays 38, S65–S74. doi:10.1002/bies.201670911
Cassim, S., and Pouyssegur, J. (2019). Tumor microenvironment: a metabolic player that Shapes the immune response. Int. J. Mol. Sci. 21 (1), 157. doi:10.3390/ijms21010157
Cham, C. M., Driessens, G., O'Keefe, J. P., and Gajewski, T. F. (2008). Glucose deprivation inhibits multiple key gene expression events and effector functions in CD8+ T cells. Eur. J. Immunol. 38 (9), 2438–2450. doi:10.1002/eji.200838289
Cham, C. M., and Gajewski, T. F. (2005). Glucose availability regulates IFN-gamma production and p70S6 kinase activation in CD8+ effector T cells. J. Immunol. 174 (8), 4670–4677. doi:10.4049/jimmunol.174.8.4670
Chang, C. H., Qiu, J., O'Sullivan, D., Buck, M. D., Noguchi, T., Curtis, J. D., et al. (2015). Metabolic competition in the tumor microenvironment is a driver of cancer progression. Cell 162 (6), 1229–1241. doi:10.1016/j.cell.2015.08.016
Chao, M., Wu, H., Jin, K., Li, B., Wu, J., Zhang, G., et al. (2016). A nonrandomized cohort and a randomized study of local control of large hepatocarcinoma by targeting intratumoral lactic acidosis. Elife 5, e15691. doi:10.7554/eLife.15691
Chen, J., Zou, L., Lu, G., Grinchuk, O., Fang, L., Ong, D. S. T., et al. (2022a). PFKP alleviates glucose starvation-induced metabolic stress in lung cancer cells via AMPK-ACC2 dependent fatty acid oxidation. Cell Discov. 8 (1), 52. doi:10.1038/s41421-022-00406-1
Chen, K. J., Hsu, J. W., and Lee, F. S. (2022b). AMPK promotes Arf6 activation in a kinase-independent manner upon glucose starvation. J. Cell Sci. 135 (18), jcs259609. doi:10.1242/jcs.259609
Chen, M. C., Hsu, L. L., Wang, S. F., Hsu, C. Y., Lee, H. C., and Tseng, L. M. (2020). ROS mediate xCT-dependent cell death in human breast cancer cells under glucose deprivation. Cells 9 (7), 1598. doi:10.3390/cells9071598
Chen, X., and Cubillos-Ruiz, J. R. (2021). Endoplasmic reticulum stress signals in the tumour and its microenvironment. Nat. Rev. Cancer 21 (2), 71–88. doi:10.1038/s41568-020-00312-2
Chen, Y., Huang, Q., Liu, W., Zhu, Q., Cui, C. P., Xu, L., et al. (2018). Mutually exclusive acetylation and ubiquitylation of the splicing factor SRSF5 control tumor growth. Nat. Commun. 9 (1), 2464. doi:10.1038/s41467-018-04815-3
Cheng, C. T., Kuo, C. Y., Ouyang, C., Li, C. F., Chung, Y., Chan, D. C., et al. (2016). Metabolic stress-induced phosphorylation of KAP1 Ser473 blocks mitochondrial Fusion in breast cancer cells. Cancer Res. 76 (17), 5006–5018. doi:10.1158/0008-5472.can-15-2921
Choi, H. N., Jin, H. O., Kim, J. H., Hong, S. E., Kim, H. A., Kim, E. K., et al. (2013). Inhibition of S6K1 enhances glucose deprivation-induced cell death via downregulation of anti-apoptotic proteins in MCF-7 breast cancer cells. Biochem. Biophys. Res. Commun. 432 (1), 123–128. doi:10.1016/j.bbrc.2013.01.074
Deng, Z. (2023). A relatively low glucose promotes the proliferation of vascular endothelial cells by suppressing VEGFR2 O-GlcNAcylation and its proteasome degradation. Int. Ophthalmol. 43 (3), 899–914. doi:10.1007/s10792-022-02492-2
Deng, Z., Li, X., Blanca Ramirez, M., Purtell, K., Choi, I., Lu, J. H., et al. (2021). Selective autophagy of AKAP11 activates cAMP/PKA to fuel mitochondrial metabolism and tumor cell growth. Proc. Natl. Acad. Sci. U. S. A. 118 (14), e2020215118. doi:10.1073/pnas.2020215118
Denko, N. C. (2008). Hypoxia, HIF1 and glucose metabolism in the solid tumour. Nat. Rev. Cancer 8 (9), 705–713. doi:10.1038/nrc2468
Dharaskar, S. P., and Amere Subbarao, S. (2023). The mitochondrial chaperone TRAP-1 regulates the glutamine metabolism in tumor cells. Mitochondrion 69, 159–170. doi:10.1016/j.mito.2023.02.011
Di Conza, G., Trusso Cafarello, S., Loroch, S., Mennerich, D., Deschoemaeker, S., Di Matteo, M., et al. (2017a). The mTOR and PP2A pathways regulate PHD2 phosphorylation to Fine-Tune HIF1α levels and colorectal cancer cell survival under hypoxia. Cell Rep. 18 (7), 1699–1712. doi:10.1016/j.celrep.2017.01.051
Di Conza, G., Trusso Cafarello, S., Zheng, X., Zhang, Q., and Mazzone, M. (2017b). PHD2 targeting overcomes breast cancer cell death upon glucose starvation in a PP2A/B55α-mediated manner. Cell Rep. 18 (12), 2836–2844. doi:10.1016/j.celrep.2017.02.081
Ding, B., Parmigiani, A., Divakaruni, A. S., Archer, K., Murphy, A. N., and Budanov, A. V. (2016). Sestrin2 is induced by glucose starvation via the unfolded protein response and protects cells from non-canonical necroptotic cell death. Sci. Rep. 6, 22538. doi:10.1038/srep22538
Druz, A., Betenbaugh, M., and Shiloach, J. (2012). Glucose depletion activates mmu-miR-466h-5p expression through oxidative stress and inhibition of histone deacetylation. Nucleic Acids Res. 40 (15), 7291–7302. doi:10.1093/nar/gks452
DuFort, C. C., DelGiorno, K. E., Carlson, M. A., Osgood, R. J., Zhao, C., Huang, Z., et al. (2016). Interstitial pressure in pancreatic ductal adenocarcinoma is Dominated by a Gel-Fluid Phase. Biophys. J. 110 (9), 2106–2119. doi:10.1016/j.bpj.2016.03.040
Endo, H., Owada, S., Inagaki, Y., Shida, Y., and Tatemichi, M. (2018). Glucose starvation induces LKB1-AMPK-mediated MMP-9 expression in cancer cells. Sci. Rep. 8 (1), 10122. doi:10.1038/s41598-018-28074-w
Estrella, V., Chen, T., Lloyd, M., Wojtkowiak, J., Cornnell, H. H., Ibrahim-Hashim, A., et al. (2013). Acidity generated by the tumor microenvironment drives local invasion. Cancer Res. 73 (5), 1524–1535. doi:10.1158/0008-5472.can-12-2796
Fang, K., Chu, Y., Zhao, Z., Li, Q., Li, H., Chen, T., et al. (2020). Enhanced expression of asparagine synthetase under glucose-deprived conditions promotes esophageal squamous cell carcinoma development. Int. J. Med. Sci. 17 (4), 510–516. doi:10.7150/ijms.39557
Fang, X., Lu, G., Ha, K., Lin, H., Du, Y., Zuo, Q., et al. (2018). Acetylation of TIP60 at K104 is essential for metabolic stress-induced apoptosis in cells of hepatocellular cancer. Exp. Cell Res. 362 (2), 279–286. doi:10.1016/j.yexcr.2017.11.028
Ferretti, A. C., Tonucci, F. M., Hidalgo, F., Almada, E., Larocca, M. C., and Favre, C. (2016). AMPK and PKA interaction in the regulation of survival of liver cancer cells subjected to glucose starvation. Oncotarget 7 (14), 17815–17828. doi:10.18632/oncotarget.7404
Franco, O. E., and Hayward, S. W. (2012). Targeting the tumor stroma as a novel therapeutic approach for prostate cancer. Adv. Pharmacol. 65, 267–313. doi:10.1016/b978-0-12-397927-8.00009-9
Fu, L. N., Wang, Y. Q., Tan, J., Xu, J., Gao, Q. Y., Chen, Y. X., et al. (2018). Role of JMJD2B in colon cancer cell survival under glucose-deprived conditions and the underlying mechanisms. Oncogene 37 (3), 389–402. doi:10.1038/onc.2017.345
Garcia, J. A., Chen, R., Xu, M., Comerford, S. A., Hammer, R. E., Melton, S. D., et al. (2023). Acss2/HIF-2 signaling facilitates colon cancer growth and metastasis. PLoS One 18 (3), e0282223. doi:10.1371/journal.pone.0282223
Gonzalez-Menendez, P., Hevia, D., Alonso-Arias, R., Alvarez-Artime, A., Rodriguez-Garcia, A., Kinet, S., et al. (2018). GLUT1 protects prostate cancer cells from glucose deprivation-induced oxidative stress. Redox Biol. 17, 112–127. doi:10.1016/j.redox.2018.03.017
Gottfried, E., Kreutz, M., and Mackensen, A. (2012). Tumor metabolism as modulator of immune response and tumor progression. Semin. Cancer Biol. 22 (4), 335–341. doi:10.1016/j.semcancer.2012.02.009
Guo, J., Zhang, Q., Su, Y., Lu, X., Wang, Y., Yin, M., et al. (2020). Arginine methylation of ribose-5-phosphate isomerase A senses glucose to promote human colorectal cancer cell survival. Sci. China Life Sci. 63 (9), 1–12. doi:10.1007/s11427-019-1562-y
Gwangwa, M. V., Joubert, A. M., and Visagie, M. H. (2018). Crosstalk between the Warburg effect, redox regulation and autophagy induction in tumourigenesis. Cell Mol. Biol. Lett. 23, 20. doi:10.1186/s11658-018-0088-y
Hanahan, D., and Weinberg, R. A. (2011). Hallmarks of cancer: the next generation. Cell 144 (5), 646–674. doi:10.1016/j.cell.2011.02.013
Ho, P. C., Bihuniak, J. D., Macintyre, A. N., Staron, M., Liu, X., Amezquita, R., et al. (2015). Phosphoenolpyruvate is a metabolic checkpoint of anti-tumor T cell responses. Cell 162 (6), 1217–1228. doi:10.1016/j.cell.2015.08.012
Hong, S. M., Park, C. W., Kim, S. W., Nam, Y. J., Yu, J. H., Shin, J. H., et al. (2016). NAMPT suppresses glucose deprivation-induced oxidative stress by increasing NADPH levels in breast cancer. Oncogene 35 (27), 3544–3554. doi:10.1038/onc.2015.415
Hu, Y. L., DeLay, M., Jahangiri, A., Molinaro, A. M., Rose, S. D., Carbonell, W. S., et al. (2012). Hypoxia-induced autophagy promotes tumor cell survival and adaptation to antiangiogenic treatment in glioblastoma. Cancer Res. 72 (7), 1773–1783. doi:10.1158/0008-5472.can-11-3831
Hu, Y. L., Yin, Y., Liu, H. Y., Feng, Y. Y., Bian, Z. H., Zhou, L. Y., et al. (2016). Glucose deprivation induces chemoresistance in colorectal cancer cells by increasing ATF4 expression. World J. Gastroenterol. 22 (27), 6235–6245. doi:10.3748/wjg.v22.i27.6235
Hyroššová, P., Aragó, M., Muñoz-Pinedo, C., Viñals, F., García-Rovés, P. M., Escolano, C., et al. (2022). Glycosylation defects, offset by PEPCK-M, drive entosis in breast carcinoma cells. Cell Death Dis. 13 (8), 730. doi:10.1038/s41419-022-05177-x
Ikeda, M., Sato, A., Mochizuki, N., Toyosaki, K., Miyoshi, C., Fujioka, R., et al. (2016). Phase I trial of GBS-01 for advanced pancreatic cancer refractory to gemcitabine. Cancer Sci. 107 (12), 1818–1824. doi:10.1111/cas.13086
Isono, T., Chano, T., Kitamura, A., and Yuasa, T. (2014). Glucose deprivation induces G2/M transition-arrest and cell death in N-GlcNAc2-modified protein-producing renal carcinoma cells. PLoS One 9 (5), e96168. doi:10.1371/journal.pone.0096168
Iurlaro, R., Püschel, F., León-Annicchiarico, C. L., O'Connor, H., Martin, S. J., Palou-Gramón, D., et al. (2017). Glucose deprivation induces ATF4-mediated apoptosis through TRAIL death receptors. Mol. Cell Biol. 37 (10), e00479-16. doi:10.1128/mcb.00479-16
Jain, R. K., Martin, J. D., and Stylianopoulos, T. (2014). The role of Mechanical forces in tumor growth and therapy. Annu. Rev. Biomed. Eng. 16 (1), 321–346. doi:10.1146/annurev-bioeng-071813-105259
Judge, A., and Dodd, M. S. (2020). Metabolism. Essays Biochem. 64 (4), 607–647. doi:10.1042/ebc20190041
Kalimuthu, K., Kim, J. H., Park, Y. S., Luo, X., Zhang, L., Ku, J. L., et al. (2021). Glucose deprivation-induced endoplasmic reticulum stress response plays a pivotal role in enhancement of TRAIL cytotoxicity. J. Cell Physiol. 236 (9), 6666–6677. doi:10.1002/jcp.30329
Kalluri, R. (2016). The biology and function of fibroblasts in cancer. Nat. Rev. Cancer 16 (9), 582–598. doi:10.1038/nrc.2016.73
Kang, Y., Zhu, X., Xu, Y., Tang, Q., Huang, Z., Zhao, Z., et al. (2018). Energy stress-induced lncRNA HAND2-AS1 represses HIF1α-mediated energy metabolism and inhibits osteosarcoma progression. Am. J. Cancer Res. 8 (3), 526–537.
Kanska, J., Aspuria, P. P., Taylor-Harding, B., Spurka, L., Funari, V., Orsulic, S., et al. (2017). Glucose deprivation elicits phenotypic plasticity via ZEB1-mediated expression of NNMT. Oncotarget 8 (16), 26200–26220. doi:10.18632/oncotarget.15429
Katayama, Y., Uchino, J., Chihara, Y., Tamiya, N., Kaneko, Y., Yamada, T., et al. (2019). Tumor Neovascularization and developments in therapeutics. Cancers (Basel) 11 (3), 316. doi:10.3390/cancers11030316
Kedia-Mehta, N., and Finlay, D. K. (2019). Competition for nutrients and its role in controlling immune responses. Nat. Commun. 10 (1), 2123. doi:10.1038/s41467-019-10015-4
Keller, K. E., Tan, I. S., and Lee, Y. S. (2012). SAICAR stimulates pyruvate kinase isoform M2 and promotes cancer cell survival in glucose-limited conditions. Science 338 (6110), 1069–1072. doi:10.1126/science.1224409
Khan, M. R., Xiang, S., Song, Z., and Wu, M. (2017). The p53-inducible long noncoding RNA TRINGS protects cancer cells from necrosis under glucose starvation. Embo J. 36 (23), 3483–3500. doi:10.15252/embj.201696239
Kierans, S. J., and Taylor, C. T. (2021). Regulation of glycolysis by the hypoxia-inducible factor (HIF): implications for cellular physiology. J. Physiol. 599 (1), 23–37. doi:10.1113/jp280572
Kim, H. S., Kim, M. J., Lim, J., Yang, Y., Lee, M. S., and Lim, J. S. (2014). NDRG2 overexpression enhances glucose deprivation-mediated apoptosis in breast cancer cells via inhibition of the LKB1-AMPK pathway. Genes Cancer 5 (5-6), 175–185. doi:10.18632/genesandcancer.17
Kimmelman, A. C., and White, E. (2017). Autophagy and tumor metabolism. Cell Metab. 25 (5), 1037–1043. doi:10.1016/j.cmet.2017.04.004
Koppula, P., Olszewski, K., Zhang, Y., Kondiparthi, L., Liu, X., Lei, G., et al. (2021). KEAP1 deficiency drives glucose dependency and sensitizes lung cancer cells and tumors to GLUT inhibition. iScience 24 (6), 102649. doi:10.1016/j.isci.2021.102649
Koumenis, C., and Wouters, B. G. (2006). Translating" tumor hypoxia: unfolded protein response (UPR)-dependent and UPR-independent pathways. Mol. Cancer Res. 4 (7), 423–436. doi:10.1158/1541-7786.mcr-06-0150
Krętowski, R., Stypułkowska, A., and Cechowska-Pasko, M. (2013). Low-glucose medium induces ORP150 expression and exerts inhibitory effect on apoptosis and senescence of human breast MCF7 cells. Acta Biochim. Pol. 60 (2), 167–173. doi:10.18388/abp.2013_1967
Kumar, A., Giri, S., and Shaha, C. (2018). Sestrin2 facilitates glutamine-dependent transcription of PGC-1α and survival of liver cancer cells under glucose limitation. Febs J. 285 (7), 1326–1345. doi:10.1111/febs.14406
Lam, C., Low, J. Y., Tran, P. T., and Wang, H. (2021). The hexosamine biosynthetic pathway and cancer: current knowledge and future therapeutic strategies. Cancer Lett. 503, 11–18. doi:10.1016/j.canlet.2021.01.010
Lee, J. H., Park, S. A., Park, I. G., Yoon, B. K., Lee, J. S., and Lee, J. M. (2023). Stem cell properties of gastric cancer stem-like cells under stress conditions are regulated via the c-Fos/UCH-L3/β-Catenin Axis. Mol. Cells 46 (8), 476–485. doi:10.14348/molcells.2023.0011
Lee, Y., Itahana, Y., Ong, C. C., and Itahana, K. (2022). Redox-dependent AMPK inactivation disrupts metabolic adaptation to glucose starvation in xCT-overexpressing cancer cells. J. Cell Sci. 135 (15), jcs259090. doi:10.1242/jcs.259090
Li, M., Thorne, R. F., Wang, R., Cao, L., Cheng, F., Sun, X., et al. (2023a). Sestrin2-mediated disassembly of stress granules dampens aerobic glycolysis to overcome glucose starvation. Cell Death Discov. 9 (1), 127. doi:10.1038/s41420-023-01411-3
Li, S., Liu, Y., Bai, Y., Chen, M., Cheng, D., Wu, M., et al. (2021a). Ras Homolog family member F, Filopodia associated promotes hepatocellular carcinoma metastasis by altering the metabolic Status of cancer cells through RAB3D. Hepatology 73 (6), 2361–2379. doi:10.1002/hep.31641
Li, S., Wang, D., Zheng, X., Li, Y., Ding, C., Wang, M., et al. (2023b). Combination of niclosamide and quinacrine inactivates Akt/HK2/Cyclin D1 axis mediated by glucose deprivation towards the inhibition of melanoma cell proliferation. Biomed. Pharmacother. 163, 114865. doi:10.1016/j.biopha.2023.114865
Li, W., Liu, C., Huang, Z., Shi, L., Zhong, C., Zhou, W., et al. (2021b). AKR1B10 negatively regulates autophagy through reducing GAPDH upon glucose starvation in colon cancer. J. Cell Sci. 134 (8), jcs255273. doi:10.1242/jcs.255273
Li, X., Deng, S., Liu, M., Jin, Y., Zhu, S., Deng, S., et al. (2018). The responsively decreased PKM2 facilitates the survival of pancreatic cancer cells in hypoglucose. Cell Death Dis. 9 (2), 133. doi:10.1038/s41419-017-0158-5
Li, X., He, S., and Ma, B. (2020a). Autophagy and autophagy-related proteins in cancer. Mol. Cancer 19 (1), 12. doi:10.1186/s12943-020-1138-4
Li, Y., Liang, R., Sun, M., Li, Z., Sheng, H., Wang, J., et al. (2020b). AMPK-dependent phosphorylation of HDAC8 triggers PGM1 expression to promote lung cancer cell survival under glucose starvation. Cancer Lett. 478, 82–92. doi:10.1016/j.canlet.2020.03.007
Li, Y., Zhang, Q., Yang, J., He, W., Jiang, Y., Chen, Y., et al. (2023c). Metformin combined with glucose starvation synergistically suppress triple-negative breast cancer by enhanced unfolded protein response. Biochem. Biophys. Res. Commun. 675, 146–154. doi:10.1016/j.bbrc.2023.07.029
Li, Y., Zhang, Y., Qiu, Q., Wang, L., Mao, H., Hu, J., et al. (2022). Energy-stress-mediated AMPK activation promotes GPX4-dependent ferroptosis through the JAK2/STAT3/P53 Axis in renal cancer. Oxid. Med. Cell Longev. 2022, 2353115. doi:10.1155/2022/2353115
Li, Y. N., Cao, Y. Q., Wu, X., Han, G. S., Wang, L. X., Zhang, Y. H., et al. (2015). The association between Salt-inducible kinase 2 (SIK2) and gamma isoform of the regulatory subunit B55 of PP2A (B55gamma) contributes to the survival of glioma cells under glucose depletion through inhibiting the phosphorylation of S6K. Cancer Cell Int. 15, 21. doi:10.1186/s12935-015-0164-6
Li, Z., and Zhang, H. (2016). Reprogramming of glucose, fatty acid and amino acid metabolism for cancer progression. Cell Mol. Life Sci. 73 (2), 377–392. doi:10.1007/s00018-015-2070-4
Liang, S., Zhu, C., Suo, C., Wei, H., Yu, Y., Gu, X., et al. (2022). Mitochondrion-localized SND1 promotes Mitophagy and liver cancer progression through PGAM5. Front. Oncol. 12, 857968. doi:10.3389/fonc.2022.857968
Lin, L., Huang, H., Liao, W., Ma, H., Liu, J., Wang, L., et al. (2015). MACC1 supports human gastric cancer growth under metabolic stress by enhancing the Warburg effect. Oncogene 34 (21), 2700–2710. doi:10.1038/onc.2014.204
Lin, L., Liu, Y., Pan, C., Zhang, J., Zhao, Y., Shao, R., et al. (2019). Gastric cancer cells escape metabolic stress via the DLC3/MACC1 axis. Theranostics 9 (7), 2100–2114. doi:10.7150/thno.29538
Liu, D., Sun, L., Qin, X., Liu, T., Zhang, S., Liu, Y., et al. (2016). HSF1 promotes the inhibition of EMT-associated migration by low glucose via directly regulating Snail1 expression in HCC cells. Discov. Med. 22 (120), 87–96.
Liu, M., Zhang, Z., Wang, H., Chen, X., and Jin, C. (2019). Activation of AMPK by metformin promotes renal cancer cell proliferation under glucose deprivation through its interaction with PKM2. Int. J. Biol. Sci. 15 (3), 617–627. doi:10.7150/ijbs.29689
Liu, X., Nie, L., Zhang, Y., Yan, Y., Wang, C., Colic, M., et al. (2023). Actin cytoskeleton vulnerability to disulfide stress mediates disulfidptosis. Nat. Cell Biol. 25 (3), 404–414. doi:10.1038/s41556-023-01091-2
Liu, Y., Song, X. D., Liu, W., Zhang, T. Y., and Zuo, J. (2003). Glucose deprivation induces mitochondrial dysfunction and oxidative stress in PC12 cell line. J. Cell Mol. Med. 7 (1), 49–56. doi:10.1111/j.1582-4934.2003.tb00202.x
Liu, Y., Tu, C. E., Guo, X., Wu, C., Gu, C., Lai, Q., et al. (2021). Tumor-suppressive function of EZH2 is through inhibiting glutaminase. Cell Death Dis. 12 (11), 975. doi:10.1038/s41419-021-04212-7
Loong, J. H., Wong, T. L., Tong, M., Sharma, R., Zhou, L., Ng, K. Y., et al. (2021). Glucose deprivation-induced aberrant FUT1-mediated fucosylation drives cancer stemness in hepatocellular carcinoma. J. Clin. Invest 131 (11), e143377. doi:10.1172/jci143377
Lu, F., Fang, D., Li, S., Zhong, Z., Jiang, X., Qi, Q., et al. (2022). Thioredoxin 1 supports colorectal cancer cell survival and promotes migration and invasion under glucose deprivation through interaction with G6PD. Int. J. Biol. Sci. 18 (14), 5539–5553. doi:10.7150/ijbs.71809
Lu, Y. F., Xu, X. P., Lu, X. P., Zhu, Q., Liu, G., Bao, Y. T., et al. (2020). SIRT7 activates p53 by enhancing PCAF-mediated MDM2 degradation to arrest the cell cycle. Oncogene 39 (24), 4650–4665. doi:10.1038/s41388-020-1305-5
Luo, H., Song, Y., Zhang, J. A., Liu, Y., Chen, F., Wang, Z., et al. (2022). MAT2A facilitates PDCD6 methylation and promotes cell growth under glucose deprivation in cervical cancer. Cell Death Discov. 8 (1), 176. doi:10.1038/s41420-022-00987-6
Luo, J., Hong, Y., Tao, X., Wei, X., Zhang, L., and Li, Q. (2016). An indispensable role of CPT-1a to survive cancer cells during energy stress through rewiring cancer metabolism. Tumour Biol. 37, 15795–15804. doi:10.1007/s13277-016-5382-6
Lyssiotis, C. A., and Kimmelman, A. C. (2017). Metabolic interactions in the tumor microenvironment. Trends Cell Biol. 27 (11), 863–875. doi:10.1016/j.tcb.2017.06.003
Ma, Y., Cui, D., Wang, L., Wang, Y., Yang, F., Pan, H., et al. (2022). P90 ribosomal S6 kinase confers cancer cell survival by mediating checkpoint kinase 1 degradation in response to glucose stress. Cancer Sci. 113 (1), 132–144. doi:10.1111/cas.15168
Ma, Y., Cui, D., Xiong, X., Inuzuka, H., Wei, W., Sun, Y., et al. (2019). SCFβ-TrCP ubiquitinates CHK1 in an AMPK-dependent manner in response to glucose deprivation. Mol. Oncol. 13 (2), 307–321. doi:10.1002/1878-0261.12403
Ma, Z., Chen, H., Xia, Z., You, J., Han, C., Wang, S., et al. (2023). Energy stress-induced circZFR enhances oxidative phosphorylation in lung adenocarcinoma via regulating alternative splicing. J. Exp. Clin. Cancer Res. 42 (1), 169. doi:10.1186/s13046-023-02723-z
Maeyashiki, C., Melhem, H., Hering, L., Baebler, K., Cosin-Roger, J., Schefer, F., et al. (2020). Activation of pH-Sensing receptor OGR1 (GPR68) induces ER stress via the IRE1α/JNK pathway in an Intestinal epithelial cell Model. Sci. Rep. 10 (1), 1438. doi:10.1038/s41598-020-57657-9
Marambio, P., Toro, B., Sanhueza, C., Troncoso, R., Parra, V., Verdejo, H., et al. (2010). Glucose deprivation causes oxidative stress and stimulates aggresome formation and autophagy in cultured cardiac myocytes. Biochim. Biophys. Acta 1802 (6), 509–518. doi:10.1016/j.bbadis.2010.02.002
May, D., Itin, A., Gal, O., Kalinski, H., Feinstein, E., and Keshet, E. (2005). Ero1-L alpha plays a key role in a HIF-1-mediated pathway to improve disulfide bond formation and VEGF secretion under hypoxia: implication for cancer. Oncogene 24 (6), 1011–1020. doi:10.1038/sj.onc.1208325
Meng, Q., Xu, J., Liang, C., Liu, J., Hua, J., Zhang, Y., et al. (2018). GPx1 is involved in the induction of protective autophagy in pancreatic cancer cells in response to glucose deprivation. Cell Death Dis. 9 (12), 1187. doi:10.1038/s41419-018-1244-z
Muir, A., Danai, L. V., Gui, D. Y., Waingarten, C. Y., Lewis, C. A., and Vander Heiden, M. G. (2017). Environmental cystine drives glutamine anaplerosis and sensitizes cancer cells to glutaminase inhibition. Elife 6, e27713. doi:10.7554/eLife.27713
Murai, S., Ando, A., Ebara, S., Hirayama, M., Satomi, Y., and Hara, T. (2017). Inhibition of malic enzyme 1 disrupts cellular metabolism and leads to vulnerability in cancer cells in glucose-restricted conditions. Oncogenesis 6 (5), e329. doi:10.1038/oncsis.2017.34
Nakagawa, T., and Ohta, K. (2019). Quercetin regulates the integrated stress response to improve memory. Int. J. Mol. Sci. 20 (11), 2761. doi:10.3390/ijms20112761
Nishimoto, A., Kugimiya, N., Hosoyama, T., Enoki, T., Li, T. S., and Hamano, K. (2014). HIF-1α activation under glucose deprivation plays a central role in the acquisition of anti-apoptosis in human colon cancer cells. Int. J. Oncol. 44 (6), 2077–2084. doi:10.3892/ijo.2014.2367
Ordway, B., Gillies, R. J., and Damaghi, M. (2021). Extracellular acidification induces Lysosomal Dysregulation. Cells 10 (5), 1188. doi:10.3390/cells10051188
Owada, S., Shimoda, Y., Tsuchihara, K., and Esumi, H. (2013). Critical role of H2O2 generated by NOX4 during cellular response under glucose deprivation. PLoS One 8 (3), e56628. doi:10.1371/journal.pone.0056628
Palorini, R., Votta, G., Pirola, Y., De Vitto, H., De Palma, S., Airoldi, C., et al. (2016). Protein kinase A activation promotes cancer cell resistance to glucose starvation and Anoikis. PLoS Genet. 12 (3), e1005931. doi:10.1371/journal.pgen.1005931
Pan, T., Zhang, M., Zhang, F., Yan, G., Ru, Y., Wang, Q., et al. (2017). NDRG2 overexpression suppresses hepatoma cells survival during metabolic stress through disturbing the activation of fatty acid oxidation. Biochem. Biophys. Res. Commun. 483 (2), 860–866. doi:10.1016/j.bbrc.2017.01.018
Park, K. C., Kim, J. M., Kim, S. Y., Kim, S. M., Lim, J. H., Kim, M. K., et al. (2023). PMCA inhibition reverses drug resistance in clinically refractory cancer patient-derived models. BMC Med. 21 (1), 38. doi:10.1186/s12916-023-02727-8
Park, S. C., and Lee, J. M. (2022). Ezh2 promotes TRβ lysine methylation-mediated degradation in hepatocellular carcinoma. Genes Genomics 44 (3), 369–377. doi:10.1007/s13258-021-01196-8
Parker, A. L., Turner, N., McCarroll, J. A., and Kavallaris, M. (2016). βIII-Tubulin alters glucose metabolism and stress response signaling to promote cell survival and proliferation in glucose-starved non-small cell lung cancer cells. Carcinogenesis 37 (8), 787–798. doi:10.1093/carcin/bgw058
Penugurti, V., Khumukcham, S. S., Padala, C., Dwivedi, A., Kamireddy, K. R., Mukta, S., et al. (2021). HPIP protooncogene differentially regulates metabolic adaptation and cell fate in breast cancer cells under glucose stress via AMPK and RNF2 dependent pathways. Cancer Lett. 518, 243–255. doi:10.1016/j.canlet.2021.07.027
Pitt, J. M., Marabelle, A., Eggermont, A., Soria, J. C., Kroemer, G., and Zitvogel, L. (2016). Targeting the tumor microenvironment: removing obstruction to anticancer immune responses and immunotherapy. Ann. Oncol. 27 (8), 1482–1492. doi:10.1093/annonc/mdw168
Qu, Y. M., Chen, A., Zhao, X., Wang, Z., Guo, D., Shao, S. L., et al. (2023). Huntingtin-associated protein 1 is a potential tumor suppressor for gastric cancer. Mol. Biol. Rep. 50 (2), 1517–1531. doi:10.1007/s11033-022-08090-w
Raut, G. K., Chakrabarti, M., Pamarthy, D., and Bhadra, M. P. (2019). Glucose starvation-induced oxidative stress causes mitochondrial dysfunction and apoptosis via Prohibitin 1 upregulation in human breast cancer cells. Free Radic. Biol. Med. 145, 428–441. doi:10.1016/j.freeradbiomed.2019.09.020
Ren, Y., Chen, J., Chen, P., Hao, Q., Cheong, L. K., Tang, M., et al. (2021). Oxidative stress-mediated AMPK inactivation determines the high susceptibility of LKB1-mutant NSCLC cells to glucose starvation. Free Radic. Biol. Med. 166, 128–139. doi:10.1016/j.freeradbiomed.2021.02.018
Ren, Y., and Shen, H. M. (2019). Critical role of AMPK in redox regulation under glucose starvation. Redox Biol. 25, 101154. doi:10.1016/j.redox.2019.101154
Rey, S., and Semenza, G. L. (2010). Hypoxia-inducible factor-1-dependent mechanisms of vascularization and vascular remodelling. Cardiovasc Res. 86 (2), 236–242. doi:10.1093/cvr/cvq045
Ricciardiello, F., Gang, Y., Palorini, R., Li, Q., Giampà, M., Zhao, F., et al. (2020). Hexosamine pathway inhibition overcomes pancreatic cancer resistance to gemcitabine through unfolded protein response and EGFR-Akt pathway modulation. Oncogene 39 (20), 4103–4117. doi:10.1038/s41388-020-1260-1
Roberts, D. J., Tan-Sah, V. P., Ding, E. Y., Smith, J. M., and Miyamoto, S. (2014). Hexokinase-II positively regulates glucose starvation-induced autophagy through TORC1 inhibition. Mol. Cell 53 (4), 521–533. doi:10.1016/j.molcel.2013.12.019
Romero-Cordoba, S. L., Rodriguez-Cuevas, S., Bautista-Pina, V., Maffuz-Aziz, A., D'Ippolito, E., Cosentino, G., et al. (2018). Loss of function of miR-342-3p results in MCT1 over-expression and contributes to oncogenic metabolic reprogramming in triple negative breast cancer. Sci. Rep. 8 (1), 12252. doi:10.1038/s41598-018-29708-9
Ron, D., and Walter, P. (2007). Signal integration in the endoplasmic reticulum unfolded protein response. Nat. Rev. Mol. Cell Biol. 8 (7), 519–529. doi:10.1038/nrm2199
Rouschop, K. M., van den Beucken, T., Dubois, L., Niessen, H., Bussink, J., Savelkouls, K., et al. (2010). The unfolded protein response protects human tumor cells during hypoxia through regulation of the autophagy genes MAP1LC3B and ATG5. J. Clin. Invest 120 (1), 127–141. doi:10.1172/jci40027
Saggese, P., Pandey, A., Fung, E., Hall, A., Yanagawa, J., Rodriguez, E. F., et al. (2023). Glucose deprivation promotes pseudo-hypoxia and de-differentiation in lung adenocarcinoma. bioRxiv, 2023.01.30.526207. doi:10.1101/2023.01.30.526207
Sano, R., and Reed, J. C. (2013). ER stress-induced cell death mechanisms. Biochim. Biophys. Acta 1833 (12), 3460–3470. doi:10.1016/j.bbamcr.2013.06.028
Schug, Z. T., Peck, B., Jones, D. T., Zhang, Q., Grosskurth, S., Alam, I. S., et al. (2015). Acetyl-CoA synthetase 2 promotes acetate utilization and maintains cancer cell growth under metabolic stress. Cancer Cell 27 (1), 57–71. doi:10.1016/j.ccell.2014.12.002
Semenza, G. L. (2010). Defining the role of hypoxia-inducible factor 1 in cancer biology and therapeutics. Oncogene 29 (5), 625–634. doi:10.1038/onc.2009.441
Shao, Y., Ren, W., Dai, H., Yang, F., Li, X., Zhang, S., et al. (2023). SKP2 contributes to AKT activation by ubiquitination degradation of PHLPP1, Impedes autophagy, and facilitates the survival of thyroid carcinoma. Mol. Cells 46, 360–373. doi:10.14348/molcells.2022.2242
Sharma, S., Evans, A., and Hemers, E. (2016). Mesenchymal-epithelial signalling in tumour microenvironment: role of high-mobility group Box 1. Cell Tissue Res. 365 (2), 357–366. doi:10.1007/s00441-016-2389-7
Shaw, R. J. (2006). Glucose metabolism and cancer. Curr. Opin. Cell Biol. 18 (6), 598–608. doi:10.1016/j.ceb.2006.10.005
Sheikh, T., Gupta, P., Gowda, P., Patrick, S., and Sen, E. (2018). Hexokinase 2 and nuclear factor erythroid 2-related factor 2 transcriptionally coactivate xanthine oxidoreductase expression in stressed glioma cells. J. Biol. Chem. 293 (13), 4767–4777. doi:10.1074/jbc.M117.816785
Shimizu, Y., and Hendershot, L. M. (2009). Oxidative folding: cellular strategies for dealing with the resultant equimolar production of reactive oxygen species. Antioxid. Redox Signal 11 (9), 2317–2331. doi:10.1089/ars.2009.2501
Simons, A. L., Mattson, D. M., Dornfeld, K., and Spitz, D. R. (2009). Glucose deprivation-induced metabolic oxidative stress and cancer therapy. J. Cancer Res. Ther. 5, S2–S6. doi:10.4103/0973-1482.55133
Singh, L., Nair, L., Kumar, D., Arora, M. K., Bajaj, S., Gadewar, M., et al. (2023). Hypoxia induced lactate acidosis modulates tumor microenvironment and lipid reprogramming to sustain the cancer cell survival. Front. Oncol. 13, 1034205. doi:10.3389/fonc.2023.1034205
Song, C., Mitter, S. K., Qi, X., Beli, E., Rao, H. V., Ding, J., et al. (2017). Oxidative stress-mediated NFκB phosphorylation upregulates p62/SQSTM1 and promotes retinal pigmented epithelial cell survival through increased autophagy. PLoS One 12 (2), e0171940. doi:10.1371/journal.pone.0171940
Spitz, D. R., Sim, J. E., Ridnour, L. A., Galoforo, S. S., and Lee, Y. J. (2000). Glucose deprivation-induced oxidative stress in human tumor cells. A fundamental defect in metabolism? Ann. N. Y. Acad. Sci. 899, 349–362. doi:10.1111/j.1749-6632.2000.tb06199.x
Stuart, S. F., Bezawork-Geleta, A., Areeb, Z., Gomez, J., Tsui, V., Zulkifli, A., et al. (2023). The Interleukin-11/IL-11 receptor promotes glioblastoma survival and invasion under glucose-starved conditions through enhanced glutaminolysis. Int. J. Mol. Sci. 24 (4), 3356. doi:10.3390/ijms24043356
Su, X., He, X., Ben, Q., Wang, W., Song, H., Ye, Q., et al. (2017). Effect of p53 on pancreatic cancer-glucose tolerance abnormalities by regulating transglutaminase 2 in resistance to glucose metabolic stress. Oncotarget 8 (43), 74299–74311. doi:10.18632/oncotarget.19402
Sudhagar, S., Sathya, S., Gokulapriya, G., and Lakshmi, B. S. (2016). AKT-p53 axis protect cancer cells from autophagic cell death during nutrition deprivation. Biochem. Biophys. Res. Commun. 471 (4), 396–401. doi:10.1016/j.bbrc.2016.02.064
Sullivan, M. R., Danai, L. V., Lewis, C. A., Chan, S. H., Gui, D. Y., Kunchok, T., et al. (2019). Quantification of microenvironmental metabolites in murine cancers reveals determinants of tumor nutrient availability. Elife 8, e44235. doi:10.7554/eLife.44235
Sun, L., Song, L., Wan, Q., Wu, G., Li, X., Wang, Y., et al. (2015). cMyc-mediated activation of serine biosynthesis pathway is critical for cancer progression under nutrient deprivation conditions. Cell Res. 25 (4), 429–444. doi:10.1038/cr.2015.33
Sun, L., Suo, C., Li, S. T., Zhang, H., and Gao, P. (2018). Metabolic reprogramming for cancer cells and their microenvironment: beyond the Warburg Effect. Biochim. Biophys. Acta Rev. Cancer 1870 (1), 51–66. doi:10.1016/j.bbcan.2018.06.005
Taguchi, K., Fujikawa, N., Komatsu, M., Ishii, T., Unno, M., Akaike, T., et al. (2012). Keap1 degradation by autophagy for the maintenance of redox homeostasis. Proc. Natl. Acad. Sci. U. S. A. 109 (34), 13561–13566. doi:10.1073/pnas.1121572109
Takahashi, Y., Serada, S., Ohkawara, T., Fujimoto, M., Hiramatsu, K., Ueda, Y., et al. (2021). LSR promotes epithelial ovarian cancer cell survival under energy stress through the LKB1-AMPK pathway. Biochem. Biophys. Res. Commun. 537, 93–99. doi:10.1016/j.bbrc.2020.12.079
Tan, J., Wang, H. L., Yang, J., Liu, Q. Q., Li, C. M., Wang, Y. Q., et al. (2020). JMJD2B-induced amino acid alterations enhance the survival of colorectal cancer cells under glucose-deprivation via autophagy. Theranostics 10 (13), 5763–5777. doi:10.7150/thno.38087
Tang, J., Yan, T., Bao, Y., Shen, C., Yu, C., Zhu, X., et al. (2019). LncRNA GLCC1 promotes colorectal carcinogenesis and glucose metabolism by stabilizing c-Myc. Nat. Commun. 10 (1), 3499. doi:10.1038/s41467-019-11447-8
Teramoto, K., and Katoh, H. (2019). The cystine/glutamate antiporter xCT is a key regulator of EphA2 S897 phosphorylation under glucose-limited conditions. Cell Signal 62, 109329. doi:10.1016/j.cellsig.2019.05.014
Terashima, J., Tachikawa, C., Kudo, K., Habano, W., and Ozawa, S. (2013). An aryl hydrocarbon receptor induces VEGF expression through ATF4 under glucose deprivation in HepG2. BMC Mol. Biol. 14, 27. doi:10.1186/1471-2199-14-27
Umapathy, D., Karthikeyan, M. C., Ponnuchamy, K., Kannan, M. K., Ganeshan, M., and Arockiam, A. J. V. (2022). The absence of cellular glucose triggers oncogene AEG-1 that instigates VEGFC in HCC: a possible genetic root cause of angiogenesis. Gene 826, 146446. doi:10.1016/j.gene.2022.146446
Varghese, B., Chianese, U., Capasso, L., Sian, V., Bontempo, P., Conte, M., et al. (2023). SIRT1 activation promotes energy homeostasis and reprograms liver cancer metabolism. J. Transl. Med. 21 (1), 627. doi:10.1186/s12967-023-04440-9
Vaziri-Gohar, A., Cassel, J., Mohammed, F. S., Zarei, M., Hue, J. J., Hajihassani, O., et al. (2022). Limited nutrient availability in the tumor microenvironment renders pancreatic tumors sensitive to allosteric IDH1 inhibitors. Nat. Cancer 3 (7), 852–865. doi:10.1038/s43018-022-00393-y
Vaziri-Gohar, A., Hue, J. J., Abbas, A., Graor, H. J., Hajihassani, O., Zarei, M., et al. (2023). Increased glucose availability sensitizes pancreatic cancer to chemotherapy. Nat. Commun. 14 (1), 3823. doi:10.1038/s41467-023-38921-8
Vaziri-Gohar, A., Zarei, M., Brody, J. R., and Winter, J. M. (2018). Metabolic Dependencies in pancreatic cancer. Front. Oncol. 8, 617. doi:10.3389/fonc.2018.00617
Walker, A., Singh, A., Tully, E., Woo, J., Le, A., Nguyen, T., et al. (2018). Nrf2 signaling and autophagy are complementary in protecting breast cancer cells during glucose deprivation. Free Radic. Biol. Med. 120, 407–413. doi:10.1016/j.freeradbiomed.2018.04.009
Wang, J., Ni, X., Shen, S., Zhang, D., Ni, X., Suo, T., et al. (2021). Phosphorylation at Ser10 triggered p27 degradation and promoted gallbladder carcinoma cell migration and invasion by regulating stathmin1 under glucose deficiency. Cell Signal 80, 109923. doi:10.1016/j.cellsig.2021.109923
Wang, M. D., Wu, H., Huang, S., Zhang, H. L., Qin, C. J., Zhao, L. H., et al. (2016a). HBx regulates fatty acid oxidation to promote hepatocellular carcinoma survival during metabolic stress. Oncotarget 7 (6), 6711–6726. doi:10.18632/oncotarget.6817
Wang, R., Cheng, Y., Su, D., Gong, B., He, X., Zhou, X., et al. (2017). Cpt1c regulated by AMPK promotes papillary thyroid carcinomas cells survival under metabolic stress conditions. J. Cancer 8 (18), 3675–3681. doi:10.7150/jca.21148
Wang, X., Wang, G., Shi, Y., Sun, L., Gorczynski, R., Li, Y. J., et al. (2016b). PPAR-delta promotes survival of breast cancer cells in harsh metabolic conditions. Oncogenesis 5 (6), e232. doi:10.1038/oncsis.2016.41
Wang, Y., Lei, J., Zhang, S., Wang, X., Jin, J., Liu, Y., et al. (2022). 4EBP1 senses extracellular glucose deprivation and initiates cell death signaling in lung cancer. Cell Death Dis. 13 (12), 1075. doi:10.1038/s41419-022-05466-5
Warburg, O. (1956). On the Origin of cancer cells. Science 123(3191), 309–314. doi:10.1126/science.123.3191.309
Wei, S., Zhao, Q., Zheng, K., Liu, P., Sha, N., Li, Y., et al. (2022). GFAT1-linked TAB1 glutamylation sustains p38 MAPK activation and promotes lung cancer cell survival under glucose starvation. Cell Discov. 8 (1), 77. doi:10.1038/s41421-022-00423-0
Wei, X., Li, X., Yan, W., Zhang, X., Sun, Y., and Zhang, F. (2018). SKP2 promotes hepatocellular carcinoma progression through nuclear AMPK-SKP2-CARM1 signaling transcriptionally regulating nutrient-deprived autophagy induction. Cell Physiol. Biochem. 47 (6), 2484–2497. doi:10.1159/000491622
Wei, Z., Xia, J., Li, J., Cai, J., Shan, J., Zhang, C., et al. (2023). SIRT1 promotes glucolipid metabolic conversion to facilitate tumor development in colorectal carcinoma. Int. J. Biol. Sci. 19 (6), 1925–1940. doi:10.7150/ijbs.76704
Xia, H., Green, D. R., and Zou, W. (2021). Autophagy in tumour immunity and therapy. Nat. Rev. Cancer 21 (5), 281–297. doi:10.1038/s41568-021-00344-2
Xia, T., Chen, D., Liu, X., Qi, H., Wang, W., Chen, H., et al. (2022). Midkine noncanonically suppresses AMPK activation through disrupting the LKB1-STRAD-Mo25 complex. Cell Death Dis. 13 (4), 414. doi:10.1038/s41419-022-04801-0
Xiang, J., Chen, C., Liu, R., Gou, D., Chang, L., Deng, H., et al. (2021). Gluconeogenic enzyme PCK1 deficiency promotes CHK2 O-GlcNAcylation and hepatocellular carcinoma growth upon glucose deprivation. J. Clin. Invest 131 (8), e144703. doi:10.1172/jci144703
Xiao, X., Li, S., Zhang, X., Lu, J., Wang, W., Zhou, S., et al. (2019). HHQ-4, a quinoline derivate, preferentially inhibits proliferation of glucose-deprived breast cancer cells as a GRP78 down-regulator. Toxicol. Appl. Pharmacol. 373, 10–25. doi:10.1016/j.taap.2019.04.017
Xiao, Y., and Yu, D. (2021). Tumor microenvironment as a therapeutic target in cancer. Pharmacol. Ther. 221, 107753. doi:10.1016/j.pharmthera.2020.107753
Yamaguchi, I., Yoshimura, S. H., and Katoh, H. (2020). High cell density increases glioblastoma cell viability under glucose deprivation via degradation of the cystine/glutamate transporter xCT (SLC7A11). J. Biol. Chem. 295 (20), 6936–6945. doi:10.1074/jbc.RA119.012213
Yamamoto, M., Teramoto, K., and Katoh, H. (2021). Epidermal growth factor promotes glioblastoma cell death under glucose deprivation via upregulation of xCT (SLC7A11). Cell Signal 78, 109874. doi:10.1016/j.cellsig.2020.109874
Yan, L., Raj, P., Yao, W., and Ying, H. (2019). Glucose metabolism in pancreatic cancer. Cancers (Basel) 11 (10), 1460. doi:10.3390/cancers11101460
Yang, B., Li, Y., Zhang, R., Liu, L., Miao, H., Li, Y., et al. (2020). MOB1A regulates glucose deprivation-induced autophagy via IL6-STAT3 pathway in gallbladder carcinoma. Am. J. Cancer Res. 10 (11), 3896–3910.
Yang, H., Zhu, R., Zhao, X., Liu, L., Zhou, Z., Zhao, L., et al. (2019). Sirtuin-mediated deacetylation of hnRNP A1 suppresses glycolysis and growth in hepatocellular carcinoma. Oncogene 38 (25), 4915–4931. doi:10.1038/s41388-019-0764-z
Yang, R., Zhang, M., Gustafson, A. R., Wang, E., Cole, M. P., Tooley, C. E., et al. (2015). Loss of protein targeting to glycogen sensitizes human hepatocellular carcinoma cells towards glucose deprivation mediated oxidative stress and cell death. Biosci. Rep. 35 (3), e00207. doi:10.1042/bsr20150090
Yang, S. M., Kim, J., Lee, J. Y., Lee, J. S., and Lee, J. M. (2023). Regulation of glucose and glutamine metabolism to overcome cisplatin resistance in intrahepatic cholangiocarcinoma. BMB Rep., 5877. doi:10.5483/bmbrep.2023-0029
Yang, X., Sun, D., Dong, C., Tian, Y., Gao, Z., and Wang, L. (2016). Pinin associates with prognosis of hepatocellular carcinoma through promoting cell proliferation and suppressing glucose deprivation-induced apoptosis. Oncotarget 7 (26), 39694–39704. doi:10.18632/oncotarget.9233
Ying, M., You, D., Zhu, X., Cai, L., Zeng, S., and Hu, X. (2021). Lactate and glutamine support NADPH generation in cancer cells under glucose deprived conditions. Redox Biol. 46, 102065. doi:10.1016/j.redox.2021.102065
Yoshikawa, N., Saito, Y., Manabe, H., Nakaoka, T., Uchida, R., Furukawa, R., et al. (2019). Glucose depletion enhances the stem cell phenotype and gemcitabine resistance of cholangiocarcinoma Organoids through AKT phosphorylation and reactive oxygen species. Cancers (Basel) 11 (12), 1993. doi:10.3390/cancers11121993
Yu, H., Xie, Y., Zhou, Z., Wu, Z., Dai, X., and Xu, B. (2019). Curcumin regulates the progression of colorectal cancer via LncRNA NBR2/AMPK pathway. Technol. Cancer Res. Treat. 18, 1533033819870781. doi:10.1177/1533033819870781
Yue, L., Sun, T., Yang, K., Cheng, Q., Li, J., Pan, Y., et al. (2021). Supramolecular nanovesicles for synergistic glucose starvation and hypoxia-activated gene therapy of cancer. Nanoscale 13 (21), 9570–9576. doi:10.1039/d1nr02159a
Zhang, B., Thorne, R. F., Zhang, P., Wu, M., and Liu, L. (2022a). Vanguard is a glucose deprivation-Responsive long non-Coding RNA essential for Chromatin Remodeling-Reliant DNA Repair. Adv. Sci. (Weinh) 9 (30), e2201210. doi:10.1002/advs.202201210
Zhang, F. K., Ni, Q. Z., Wang, K., Cao, H. J., Guan, D. X., Zhang, E. B., et al. (2022b). Targeting USP9X-AMPK Axis in ARID1A-deficient hepatocellular carcinoma. Cell Mol. Gastroenterol. Hepatol. 14 (1), 101–127. doi:10.1016/j.jcmgh.2022.03.009
Zhang, G., Yang, Y., Hu, H., Liu, K., Li, B., Zhu, Y., et al. (2021). Energy stress-induced linc01564 activates the serine synthesis pathway and facilitates hepatocellular carcinogenesis. Oncogene 40 (16), 2936–2951. doi:10.1038/s41388-021-01749-x
Zhang, H. L., Wang, M. D., Zhou, X., Qin, C. J., Fu, G. B., Tang, L., et al. (2017a). Blocking preferential glucose uptake sensitizes liver tumor-initiating cells to glucose restriction and sorafenib treatment. Cancer Lett. 388, 1–11. doi:10.1016/j.canlet.2016.11.023
Zhang, M., Liu, T., Sun, H., Weng, W., Zhang, Q., Liu, C., et al. (2018). Pim1 supports human colorectal cancer growth during glucose deprivation by enhancing the Warburg effect. Cancer Sci. 109 (5), 1468–1479. doi:10.1111/cas.13562
Zhang, Z., Rahme, G. J., Chatterjee, P. D., Havrda, M. C., and Israel, M. A. (2017b). ID2 promotes survival of glioblastoma cells during metabolic stress by regulating mitochondrial function. Cell Death Dis. 8 (2), e2615. doi:10.1038/cddis.2017.14
Zhao, K., Wang, X., Zhao, D., Lin, Q., Zhang, Y., and Hu, Y. (2022). lncRNA HITT inhibits lactate production by Repressing PKM2 Oligomerization to reduce tumor growth and macrophage Polarization. Res. (Wash D C) 2022, 9854904. doi:10.34133/2022/9854904
Zhao, R., Cao, B., Li, H., Li, T., Xu, X., Cui, H., et al. (2021). Glucose starvation suppresses gastric cancer through targeting miR-216a-5p/Farnesyl-Diphosphate Farnesyltransferase 1 axis. Cancer Cell Int. 21 (1), 704. doi:10.1186/s12935-021-02416-7
Zhong, X. Y., Yuan, X. M., Xu, Y. Y., Yin, M., Yan, W. W., Zou, S. W., et al. (2018). CARM1 Methylates GAPDH to regulate glucose metabolism and is suppressed in liver cancer. Cell Rep. 24 (12), 3207–3223. doi:10.1016/j.celrep.2018.08.066
Zhou, Y., Yu, H., Cheng, S., Chen, Y., He, L., Ren, J., et al. (2022). Glutamate dehydrogenase 1 mediated glutaminolysis sustains HCC cells survival under glucose deprivation. J. Cancer 13 (3), 1061–1072. doi:10.7150/jca.64195
Zhu, C., He, X., Chen, K., Huang, Z., Yao, A., Tian, X., et al. (2021). LncRNA NBR2 aggravates hepatoblastoma cell malignancy and promotes cell proliferation under glucose starvation through the miR-22/TCF7 axis. Cell Cycle 20 (5-6), 575–590. doi:10.1080/15384101.2021.1885236
Zhu, M., Wei, C., Wang, H., Han, S., Cai, L., Li, X., et al. (2023). SIRT1 mediated gastric cancer progression under glucose deprivation through the FoxO1-Rab7-autophagy axis. Front. Oncol. 13, 1175151. doi:10.3389/fonc.2023.1175151
Zhu, W., Chen, X., Guo, X., Liu, H., Ma, R., Wang, Y., et al. (2022). Low glucose-induced overexpression of HOXC-AS3 promotes metabolic reprogramming of breast cancer. Cancer Res. 82 (5), 805–818. doi:10.1158/0008-5472.can-21-1179
Keywords: glucose deprivation, cancer, tumor microenvironment, cell death, cell survival
Citation: Cui Y, Sun Y, Li D, Zhang Y, Zhang Y, Cao D and Cao X (2023) The crosstalk among the physical tumor microenvironment and the effects of glucose deprivation on tumors in the past decade. Front. Cell Dev. Biol. 11:1275543. doi: 10.3389/fcell.2023.1275543
Received: 10 August 2023; Accepted: 18 October 2023;
Published: 01 November 2023.
Edited by:
Ponti Donatella, Sapienza University of Rome, ItalyReviewed by:
Simona D’Aguanno, Hospital Physiotherapy Institutes (IRCCS), ItalyAli Vaziri-Gohar, Loyola University Chicago, United States
Copyright © 2023 Cui, Sun, Li, Zhang, Zhang, Cao and Cao. This is an open-access article distributed under the terms of the Creative Commons Attribution License (CC BY). The use, distribution or reproduction in other forums is permitted, provided the original author(s) and the copyright owner(s) are credited and that the original publication in this journal is cited, in accordance with accepted academic practice. No use, distribution or reproduction is permitted which does not comply with these terms.
*Correspondence: Donghui Cao, Y2FvZGgxMjEyQGpsdS5lZHUuY24=; Xueyuan Cao, amQzZDJ1YkBqbHUuZWR1LmNu