1 Introduction
Cells need to adapt constantly to internal and environmental changes ranging from normal physiological fluctuations to pathological alterations. Changes in the cellular demand can cause perturbations in different cellular compartments that in turn activate distinct signaling pathways to elicit transcriptional programs aiming at resolving the perturbation at the site of origin. In line, distinct adaptive responses to different types of “stresses” have been described for mitochondria. However, while responses to oxidative stress and hypoxia as well as events resulting in apoptosis are well understood, knowledge on signals, mediators, and targets employed in the response to disturbed mitochondrial proteostasis is still rudimentary (Ryan and Hoogenraad, 2007; Vogtle, 2021). Yet, protein aggregation has a significant impact on mitochondrial function and consequently, imbalances in mitochondrial proteostasis are implicated in ageing and are associated with a plethora of human diseases (Rath et al., 2018; Suomalainen and Battersby, 2018). The mitochondrial unfolded protein response (mtUPR or UPRmt) evoked by insufficient protein-folding capacity, accumulation of misfolded proteins or nondegradable protein aggregates in mitochondria, is a protective response to restore proteostasis. Upregulating nuclear-encoded mitochondrial chaperones and proteases as well as controlling mitochondrial RNA translation, mtUPR improves the mitochondrial folding environment, thus maintaining mitochondrial integrity (Munch, 2018). Although a growing number of players in mtUPR has been identified in the recent years, many open questions remain, including the identity of the initial signal, as well as unidentified molecular components to sense and mediate the retrograde signal to the nucleus (Vogtle, 2021). In 2011, we identified the double-stranded RNA (dsRNA)-activated protein kinase (PKR) as a signaling component of the mammalian mtUPR and demonstrated its disease-relevance for inflammatory bowel diseases (Rath et al., 2011), findings that have been confirmed by us and others (Jackson et al., 2020; Khaloian et al., 2020). However, we were not able to identify the signal that leads to PKR activation upon induction of mtUPR by expression of a mutant protein, ornithine transcarbamylase (OTC)Δ, that accumulates in a misfolded state in the mitochondrial matrix (Ryan and Hoogenraad, 2007; Rath et al., 2011). New findings by Kim et al. now indicate that PKR can be activated by mitochondrial RNA that exist as intermolecular dsRNA, in particular under stress conditions (Kim et al., 2018). These results contribute to a more comprehensive understanding of mitochondrial stress signaling and make it tempting to speculate that mtUPR-associated PKR activation is mediated via mitochondrial dsRNA.
2 Signaling disturbed mitochondrial proteostasis
Mitochondrial proteostasis can be used as a sensitive measure for cellular functionality, as it faces the unique challenge of coordinating import and processing of mitochondrial precursor proteins from the cytosol with the mitochondrial transcriptional and translational machinery to ensure the stoichiometric assembly of respiratory chain complexes (Ryan and Hoogenraad, 2007). Physiological triggers like fluctuating cellular energy demands, oxidative stress, and infections, can impair protein folding (Ron and Walter, 2007; Ryan and Hoogenraad, 2007), highlighted by the fact that mtUPR contributes to the dynamically regulated mitochondrial biogenesis program (Hood et al., 2006).
The quest to identify the initial sensor or signal activated by disrupted proteostasis has been complicated by the use of different model systems (yeast, Caenorhabditis elegans, different mammalian cell lines) and a large number of “stressors” used to evoke mtUPR signaling (Vogtle, 2021). Responses seem to be highly specific for organisms and stress triggers, and comparing the same stress trigger in metabolically different cells furthermore demonstrates that the initial metabolic state of the cell modulates mtUPR signaling (Mick et al., 2020). Depending on the stressor used (mainly chemical inhibitors of oxidative phosphorylation, and inhibitors or knockdown of proteases and chaperones), the following signals are mainly implicated in the initiation of mtUPR with different contributions: ROS and metabolites synthesized within mitochondria, peptide fragments derived from protease-mediated protein degradation and altered protein transport across the mitochondrial membranes (Figure 1) (Munch, 2018; Song et al., 2021). For example, ROS generated in yeast cells treated with respiratory chain inhibitors induce the generation of oxidized lipids (ergosterol peroxide). These serve as interaction partners of Vms1 in the outer mitochondrial membrane and recruit cofactors for the proteasome-mediated cytoplasmatic degradation of ubiquitylated outer membrane proteins (Nielson et al., 2017). In contrast, a proposed sensor for unspecific mitochondria-released peptides due to stress-induced proteolysis or the overall rate of efflux is still missing (Yano, 2017). However, the targeted cleavage of DELE1, a protein associated with the mitochondrial inner membrane, by proteases has been shown to give rise to a protein fragments activating the cytoplasmic kinase HRI (Fessler et al., 2020; Guo et al., 2020). Supporting the notion on differences and also convergence of mtUPR signaling, two different mitochondrial proteases were implicated in DELE1 cleave after CCCP (proton ionophore) or oligomycin (ATP synthase inhibitor) treatment, HTRA2 and OMA1, respectively (Fessler et al., 2020; Guo et al., 2020; Bi et al., 2023). The probably most famous signal for mitochondrial stress is blocked protein import via the TIM/TOM complexes, although vice versa the translocation of a nuclear protein (Rox1) to the mitochondrial matrix to protect the mtDNA and sustain translation upon mitochondrial perturbation has also been described (Poveda-Huertes et al., 2020). One current paradigm is that the mtUPR-associated transcription factor ATF5 (analogue to C. elegans ATFS-1) is regulated by dual localization (Nargund et al., 2012; Fiorese et al., 2016). During homeostasis, ATF5 is imported and degraded within mitochondria, but upon stress-induced impaired protein import into mitochondria, it translocates to the nucleus to induce the transcriptional mtUPR program. Consistent with a model of sensing mitochondrial protein import in a general way, pharmacological inhibition of the mitochondrial chaperone HSP90 leads to accumulation of mitochondrial protein precursors in the cytosol and a parallel release of mtROS into the cytosol, activating a cytosolic signaling cascade involving HSF1 (Sutandy et al., 2023). Similarly, it has been proposed that accumulation of newly synthesized PINK1 into the outer mitochondrial membrane as a consequence of collapsed mitochondrial protein import serves as a trigger for mitophagy (Jin and Youle, 2013; Fiesel et al., 2017) (Figure 1). Of note, the defects in mitochondrial protein import and quality control in these studies were not associated with mitochondrial depolarization. A proposed outcome of mtUPR signaling induced by impaired protein import into mitochondria is increased expression of mitochondrial chaperones and proteases that need to be imported into the mitochondrial matrix to fulfil their functions (Vogtle, 2021). This apparent contradiction suggests either that parallel, faster signals (partly) restore the mitochondrial protein import capacity before new nuclear-encoded mitochondrial precursor proteins are translated or a prioritized import of certain proteins into the mitochondria.
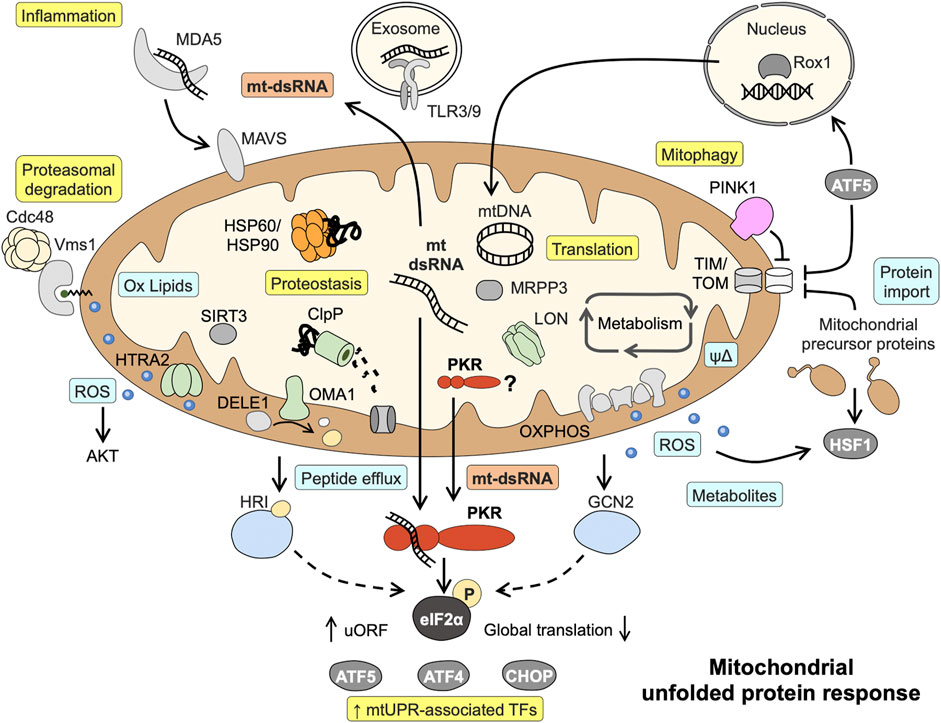
FIGURE 1. Known mitochondrial responses to disturbed proteostasis and proposed role of mitochondrial dsRNA and PKR in mtUPR signaling. Mitochondrial dsRNA might play a central role in activating the eIF2α kinase PKR and acts as danger signal capable of inducing inflammatory pathways. Light blue boxes = initial signals mediating mtUPR; Yellow boxes = endpoints of signaling. Details and abbreviation are given in the main text.
3 The mitochondrial unfolded protein response
Several axes of the mtUPR have been characterized that in part share components with other stress signaling pathways such as the integrated stress response (ISR), the endoplasmic reticulum unfolded protein response (erUPR), and the cytosolic heat shock response (HSR) (Rath et al., 2011; Munch, 2018; Sutandy et al., 2023). Most likely, the different mtUPR signaling axes act synergistically and with some redundancy. The current paradigm also comprises a cascade of events, beginning with local responses including translational regulation escalating to cell-wide responses comprising nuclear gene regulation if disturbances are more severe or affect a larger proportion of the cell´s mitochondria. Consequently, starting off as a protective response aiming to alleviate the protein-folding burden, mtUPR can result mitophagy in the case of severe or irreversible dysfunction (Munch, 2018; Samluk et al., 2019). With the exception of the intermembrane space (IMS) UPR axis, all axes are activated upon mitochondrial protein misfolding/aggregation in the mitochondrial matrix [reviewed in (Munch, 2018; Rath et al., 2018)]. (I) IMS mtUPR employs ROS-activated AKT to phosphorylate estrogen receptor- α (ERα) leading to transcriptional induction of NRF1 and the IMS-localized protease HTRA2. (II) The sirtuin axis acts via SIRT3, a protein deacetylase targeting FOXO3A causing its translocation to the nucleus to enhance transcription of ROS detoxification and mitophagy-associated genes. (III) The axis first described, the canonical mtUPR, results in upregulation of mitochondrial chaperones and proteases involving the transcription factors CHOP, CEBPβ, AP1, ATF4, and ATF5. (IV) A local translational mtUPR diminishes mitochondrial translation by LON-mediated degradation of the mitochondrial pre-RNA processing nuclease MRPP3 (Figure 1).
4 Eif2α phosphorylation and kinases in mtUPR
Various mitochondrial defects result in phosphorylation of eIF2α, a hallmark of the ISR, and also the canonical axis of mtUPR entails eIF2α phosphorylation (Rath et al., 2011; Quiros et al., 2017; Zurita Rendon and Shoubridge, 2018; Fessler et al., 2020; Mick et al., 2020). Phosphorylation of eIF2α results in global attenuation of cytosolic protein translation and selective translation of mRNAs containing upstream open reading frames (uORFs) such as CHOP, ATF4, and ATF5, thus underlining the central role of this event in mtUPR signaling. Four different kinases are known to phosphorylate eIF2α, the ER membrane-associated kinase PERK and the cytosolic kinases GCN2, HRI and PKR. With the exception of PERK, all kinases have been shown to be directly involved in mtUPR signaling in different experimental model systems. GCN2 responds to amino acid or glucose deprivation by binding to uncharged tRNAs as well as ROS, and ROS are required for GCN2 activation under mitochondrial stress (Baker et al., 2012). HRI is classically activated by heme deficiency, but an alternative way of activation by mtUPR signaling has been described (see above) (Fessler et al., 2020; Guo et al., 2020). Last but not least, PKR has first been characterized as kinase initiating immune response during infection by binding viral double-stranded RNAs (Gal-Ben-Ari et al., 2018). However, PKR has broad functions in sensing challenging cellular conditions and can be alternatively activated by its cellular protein activator of PKR (PACT) or endogenous dsRNAs such as small nucleolar RNAs during cell cycle or metabolic stress (Youssef et al., 2015; Chukwurah et al., 2021). Downstream, PKR posses several substrates including p53 and can modulate inflammatory and metabolic pathways including TNF signaling, JNK and NFκB activation, as well as insulin sensitivity (Gal-Ben-Ari et al., 2018). Consequently, PKR expression is induced by chemical inhibition of OXPHOS (Lee et al, 2020) and PKR activation is not only associated with IBD but also a hallmark of osteoarthritis and neurodegenerative diseases such as Alzheimer’s disease, Parkinson’s disease and Huntington’s disease (Bando et al., 2005; Rath et al., 2011; Ohno, 2014).
5 The riddle on PKR activation
Characterizing mtUPR signaling upon transfection of murine cells with OTCΔ in 2011, we unexpectedly discovered eIF2α phosphorylation as part of the signaling cascade. Importantly, we identified PKR to be responsible for phosphorylation of eIF2α. In this system, PKR is not only activated by disturbed mitochondrial proteostasis, but also a transcriptional target of the signaling. In line with previous publications showing the involvement of the MEK/JNK2 pathway and subsequent activation of AP1 in the model of OTCΔ-mediated mitochondrial stress induction (Horibe and Hoogenraad, 2007), we detected AP1 recruitment to the PKR promotor. Furthermore, we confirmed a role for the mitochondrial protease ClpP in the signaling pathway (Rath et al., 2011). Yet, searching for potential signals leading to PKR phosphorylation and thus activation we were only able to exclude several proposed mechanisms. Neither calcium signaling nor PACT were required for PKR activation/eIF2α phosphorylation. Additionally, using two different PKR knockout MEF cell lines with deletions in either the catalytic domain (C-PKR−/−) or the dsRNA-binding domain (N-PKR−/−), we found both domains to be required for mtUPR-induced phosphorylation of eIF2α (Rath et al., 2011; Rath, 2012).
6 Mitochondrial dsRNA and PKR activation
In contrast to mtDNA, a well-known danger-associated molecular pattern (DAMP) activating TLR and cGAS-STING pathways, mtRNA has only recently gained attention as cellular danger signal (Dhir et al., 2018; Lee J. H. et al, 2020; Grochowska et al., 2022). The circular mitochondrial genome is bidirectional transcribed as long polycistronic precursor transcript from both strands prior to processing into individual RNAs (Ojala et al., 1981). Thus, mtRNA from both strands of mtDNA can bind each other to form intermolecular dsRNA, that in turn can act as mtDAMP if released into the cytosol or the extracellular space (Kim et al., 2022). Consequently, mitochondrial dsRNAs (mt-dsRNAs) are implicated in triggering innate immune responses via MDA5 and TLR3 (Dhir et al., 2018; Lee J. H. et al, 2020; Kim et al., 2022) and also in disease-associated PKR activation (Kim et al., 2022; Yoon et al., 2022; Zhu et al., 2023). Actually, applying formaldehyde crosslinking, Kim et al. revealed that the majority of endogenous RNAs interacting with PKR is mt-dsRNA. Additionally, Kim et al. demonstrated that the abundance of mt-dsRNA and PKR activation is tightly regulated during cell cycle progression and under severe stress conditions (Kim et al., 2018; Kim et al., 2022).
The abundance of mt-(ds)RNA is determined by mtRNA synthesis and degradation and correlates with efflux into cytoplasm and PKR activation (Kim et al., 2018; Kim et al., 2022; Yoon et al., 2022; Zhu et al., 2023). With regard to disturbed mitochondrial proteostasis, two mechanisms linking mtUPR and mt-dsRNA generation/leakage into the cytoplasm seem likely, regulation of protease activity and/or ROS generation. Using an inhibitor of the mitochondrial chaperone HSP90 resulting in mitochondrial protein aggregate formation, it was shown that rapid degradation of the mitochondrial pre-RNA processing nuclease MRPP3 by the protease LON leads to defective pre-RNA processing and a stall in translation (Munch and Harper, 2016). These reversible processes could transiently increase the abundance of mtRNA and are in line with our results showing a role for mitochondrial proteases in mtUPR-induced PKR activation. Of note, the mtRNA encoding the ND5 locus is a preferred binding partner of PKR, and ND5 was shown to be a target of mitochondrial translational inhibition in the course of mtUPR (Munch and Harper, 2016; Kim et al., 2018). Furthermore, the transcription factor AP1/cJun, that we found to be activated by OTCΔ expression (Rath et al., 2011) has been shown to decrease mtDNA transcription by direct binding to mtDNA (Chae et al., 2013), indicating a potential feedback mechanism. On the other hand, mt-(ds)RNA decay might be affected by disturbances of mitochondrial proteostasis. MtRNA degradation takes place in the mitochondrial matrix and the IMS and involves, among others, the helicase SUV3 and the ribonuclease PNPase (encoded by PNPT1) (Luna-Sanchez et al., 2021). Loss of each of the proteins results in mt-dsRNA accumulation (Dhir et al., 2018; Pajak et al., 2019), but only PNPase seems to be involved in preventing mt-dsRNA efflux from mitochondria and downstream signaling including PKR activation (Dhir et al., 2018; Zhu et al., 2023). Next to PNPase, the release of mtRNA into the cytosol involves BAX/BAK pores, particularly upon mtDNA damage (Dhir et al., 2018; Tigano et al., 2021). Similarly, under severe stress conditions causing apoptosis, mitochondrial outer membrane permeabilization (MOMP) or disruption of mitochondrial membranes may lead to release of mtRNA to the cytosol and subsequent PKR activation (Kim et al., 2022). Vice versa, the mitochondrial chaperone HSP60, a target gene of mtUPR, is implicated in the retention of mt-dsRNAs in mitochondria, thereby reducing inflammatory signaling (Huang et al., 2022). Yet, PKR activation might also take place inside mitochondria, as a fraction of PKR is present in the mitochondrial matrix (Kim et al., 2018). In line, a proteomic study showed that PKR interacts with mitochondrial proteins, including HSP60 (Nakamura et al., 2015).
Overall, these findings suggest a model in which mt-dsRNA serves as signal sensed by PKR to integrate mitochondrial stress signaling into global cellular responses (Figure 1).
7 Conclusion and future directions
Some important questions remain, for example, how exactly disturbances in mitochondrial proteostasis might account for increased abundance and release of mt-dsRNA, if mt-dsRNA efflux involves active transport processes, or where exactly PKR activation takes place in the cell. It is likely that several signals are required for mt-dsRNA signalling, resembling other axes of mtUPR activation that have been shown to be dependent on multiple factors (Sutandy et al., 2023).
However, the findings by Kim et al. already shed new light on mitochondrial signaling and are a step towards a mechanistic and more holistic understanding of cellular responses toward mitochondrial disturbances (Monzel et al., 2023). It will be exciting to validate if mtUPR involves mt-dsRNA-initiated signaling and PKR activation. These data could be a framework to explore new targets for intervention in pathology-associated mitochondrial dysfunction.
Author contributions
ER: Writing–original draft, Writing–review and editing.
Funding
The author(s) declare financial support was received for the research, authorship, and/or publication of this article.
Acknowledgments
The author would like to thank her friends and colleagues Nadine Waldschmitt and Emanuel Berger for 15 years of intense discussions on mtUPR signaling and PKR activation and her family for support during writing of the article.
Conflict of interest
The author declares that the research was conducted in the absence of any commercial or financial relationships that could be construed as a potential conflict of interest.
Publisher’s note
All claims expressed in this article are solely those of the authors and do not necessarily represent those of their affiliated organizations, or those of the publisher, the editors and the reviewers. Any product that may be evaluated in this article, or claim that may be made by its manufacturer, is not guaranteed or endorsed by the publisher.
References
Baker, B. M., Nargund, A. M., Sun, T., and Haynes, C. M. (2012). Protective coupling of mitochondrial function and protein synthesis via the eIF2α kinase GCN-2. PLoS Genet. 8 (6), e1002760. doi:10.1371/journal.pgen.1002760
Bando, Y., Onuki, R., Katayama, T., Manabe, T., Kudo, T., Taira, K., et al. (2005). Double-strand RNA dependent protein kinase (PKR) is involved in the extrastriatal degeneration in Parkinson's disease and Huntington's disease. Neurochem. Int. 46 (1), 11–18. doi:10.1016/j.neuint.2004.07.005
Bi, P. Y., Killackey, S. A., Schweizer, L., Arnoult, D., Philpott, D. J., and Girardin, S. E. (2023). Cytosolic retention of the mitochondrial protease HtrA2 during mitochondrial protein import stress (MPIS) triggers the DELE1-HRI pathway. bioRxiv 2023. doi:10.1101/2023.02.26.530105
Chae, S., Ahn, B. Y., Byun, K., Cho, Y. M., Yu, M. H., Lee, B., et al. (2013). A systems approach for decoding mitochondrial retrograde signaling pathways. Sci. Signal 6 (264), rs4. doi:10.1126/scisignal.2003266
Chukwurah, E., Farabaugh, K. T., Guan, B. J., Ramakrishnan, P., and Hatzoglou, M. (2021). A tale of two proteins: PACT and PKR and their roles in inflammation. FEBS J. 288 (22), 6365–6391. doi:10.1111/febs.15691
Dhir, A., Dhir, S., Borowski, L. S., Jimenez, L., Teitell, M., Rotig, A., et al. (2018). Mitochondrial double-stranded RNA triggers antiviral signalling in humans. Nature 560 (7717), 238–242. doi:10.1038/s41586-018-0363-0
Fessler, E., Eckl, E. M., Schmitt, S., Mancilla, I. A., Meyer-Bender, M. F., Hanf, M., et al. (2020). A pathway coordinated by DELE1 relays mitochondrial stress to the cytosol. Nature 579 (7799), 433–437. doi:10.1038/s41586-020-2076-4
Fiesel, F. C., James, E. D., Hudec, R., and Springer, W. (2017). Mitochondrial targeted HSP90 inhibitor Gamitrinib-TPP (G-TPP) induces PINK1/Parkin-dependent mitophagy. Oncotarget 8 (63), 106233–106248. doi:10.18632/oncotarget.22287
Fiorese, C. J., Schulz, A. M., Lin, Y. F., Rosin, N., Pellegrino, M. W., and Haynes, C. M. (2016). The transcription factor ATF5 mediates a mammalian mitochondrial UPR. Curr. Biol. 26 (15), 2037–2043. doi:10.1016/j.cub.2016.06.002
Gal-Ben-Ari, S., Barrera, I., Ehrlich, M., and Rosenblum, K. (2018). Pkr: A kinase to remember. Front. Mol. Neurosci. 11, 480. doi:10.3389/fnmol.2018.00480
Grochowska, J., Czerwinska, J., Borowski, L. S., and Szczesny, R. J. (2022). Mitochondrial RNA, a new trigger of the innate immune system. Wiley Interdiscip. Rev. RNA 13 (3), e1690. doi:10.1002/wrna.1690
Guo, X., Aviles, G., Liu, Y., Tian, R., Unger, B. A., Lin, Y. T., et al. (2020). Mitochondrial stress is relayed to the cytosol by an OMA1-DELE1-HRI pathway. Nature 579 (7799), 427–432. doi:10.1038/s41586-020-2078-2
Hood, D. A., Irrcher, I., Ljubicic, V., and Joseph, A. M. (2006). Coordination of metabolic plasticity in skeletal muscle. J. Exp. Biol. 209 (12), 2265–2275. doi:10.1242/jeb.02182
Horibe, T., and Hoogenraad, N. J. (2007). The chop gene contains an element for the positive regulation of the mitochondrial unfolded protein response. PLoS ONE 2 (9), e835. doi:10.1371/journal.pone.0000835
Huang, Y. H., Wang, F. S., Wang, P. W., Lin, H. Y., Luo, S. D., and Yang, Y. L. (2022). Heat shock protein 60 restricts release of mitochondrial dsRNA to suppress hepatic inflammation and ameliorate non-alcoholic fatty liver disease in mice. Int. J. Mol. Sci. 23 (1), 577. doi:10.3390/ijms23010577
Jackson, D. N., Panopoulos, M., Neumann, W. L., Turner, K., Cantarel, B. L., Thompson-Snipes, L., et al. (2020). Mitochondrial dysfunction during loss of prohibitin 1 triggers Paneth cell defects and ileitis. Gut 69 (11), 1928–1938. doi:10.1136/gutjnl-2019-319523
Jin, S. M., and Youle, R. J. (2013). The accumulation of misfolded proteins in the mitochondrial matrix is sensed by PINK1 to induce PARK2/Parkin-mediated mitophagy of polarized mitochondria. Autophagy 9 (11), 1750–1757. doi:10.4161/auto.26122
Khaloian, S., Rath, E., Hammoudi, N., Gleisinger, E., Blutke, A., Giesbertz, P., et al. (2020). Mitochondrial impairment drives intestinal stem cell transition into dysfunctional Paneth cells predicting Crohn’s disease recurrence. Gut 69 (11), 1939–1951. doi:10.1136/gutjnl-2019-319514
Kim, S., Lee, K., Choi, Y. S., Ku, J., Kim, H., Kharbash, R., et al. (2022). Mitochondrial double-stranded RNAs govern the stress response in chondrocytes to promote osteoarthritis development. Cell Rep. 40 (6), 111178. doi:10.1016/j.celrep.2022.111178
Kim, Y., Park, J., Kim, S., Kim, M., Kang, M. G., Kwak, C., et al. (2018). PKR senses nuclear and mitochondrial signals by interacting with endogenous double-stranded RNAs. Mol. Cell 71 (6), 1051–1063. doi:10.1016/j.molcel.2018.07.029
Lee, H., Fenster, R. J., Pineda, S. S., Gibbs, W. S., Mohammadi, S., Davila-Velderrain, J., et al. (2020a). Cell type-specific transcriptomics reveals that mutant huntingtin leads to mitochondrial RNA release and neuronal innate immune activation. Neuron 107 (5), 891–908. doi:10.1016/j.neuron.2020.06.021
Lee, J. H., Shim, Y. R., Seo, W., Kim, M. H., Choi, W. M., Kim, H. H., et al. (2020b). Mitochondrial double-stranded RNA in exosome promotes interleukin-17 production through toll-like receptor 3 in alcohol-associated liver injury. Hepatology 72 (2), 609–625. doi:10.1002/hep.31041
Luna-Sanchez, M., Bianchi, P., and Quintana, A. (2021). Mitochondria-induced immune response as a trigger for neurodegeneration: A pathogen from within. Int. J. Mol. Sci. 22 (16), 8523. doi:10.3390/ijms22168523
Mick, E., Titov, D. V., Skinner, O. S., Sharma, R., Jourdain, A. A., and Mootha, V. K. (2020). Distinct mitochondrial defects trigger the integrated stress response depending on the metabolic state of the cell. Elife 9, e49178. doi:10.7554/eLife.49178
Monzel, A. S., Enriquez, J. A., and Picard, M. (2023). Multifaceted mitochondria: moving mitochondrial science beyond function and dysfunction. Nat. Metab. 5 (4), 546–562. doi:10.1038/s42255-023-00783-1
Munch, C., and Harper, J. W. (2016). Mitochondrial unfolded protein response controls matrix pre-RNA processing and translation. Nature 534 (7609), 710–713. doi:10.1038/nature18302
Munch, C. (2018). The different axes of the mammalian mitochondrial unfolded protein response. BMC Biol. 16 (1), 81. doi:10.1186/s12915-018-0548-x
Nakamura, T., Kunz, R. C., Zhang, C., Kimura, T., Yuan, C. L., Baccaro, B., et al. (2015). A critical role for PKR complexes with TRBP in Immunometabolic regulation and eIF2α phosphorylation in obesity. Cell Rep. 11 (2), 295–307. doi:10.1016/j.celrep.2015.03.021
Nargund, A. M., Pellegrino, M. W., Fiorese, C. J., Baker, B. M., and Haynes, C. M. (2012). Mitochondrial import efficiency of ATFS-1 regulates mitochondrial UPR activation. Science 337 (6094), 587–590. doi:10.1126/science.1223560
Nielson, J. R., Fredrickson, E. K., Waller, T. C., Rendon, O. Z., Schubert, H. L., Lin, Z., et al. (2017). Sterol oxidation mediates stress-responsive Vms1 translocation to mitochondria. Mol. Cell 68 (4), 673–685. doi:10.1016/j.molcel.2017.10.022
Ohno, M. (2014). Roles of eIF2α kinases in the pathogenesis of Alzheimer's disease. Front. Mol. Neurosci. 7, 22. doi:10.3389/fnmol.2014.00022
Ojala, D., Montoya, J., and Attardi, G. (1981). tRNA punctuation model of RNA processing in human mitochondria. Nature 290 (5806), 470–474. doi:10.1038/290470a0
Pajak, A., Laine, I., Clemente, P., El-Fissi, N., Schober, F. A., Maffezzini, C., et al. (2019). Defects of mitochondrial RNA turnover lead to the accumulation of double-stranded RNA in vivo. PLoS Genet. 15 (7), e1008240. doi:10.1371/journal.pgen.1008240
Poveda-Huertes, D., Matic, S., Marada, A., Habernig, L., Licheva, M., Myketin, L., et al. (2020). An early mtUPR: redistribution of the nuclear transcription factor Rox1 to mitochondria protects against intramitochondrial proteotoxic aggregates. Mol. Cell 77 (1), 180–188. doi:10.1016/j.molcel.2019.09.026
Quiros, P. M., Prado, M. A., Zamboni, N., D'Amico, D., Williams, R. W., Finley, D., et al. (2017). Multi-omics analysis identifies ATF4 as a key regulator of the mitochondrial stress response in mammals. J. Cell Biol. 216 (7), 2027–2045. doi:10.1083/jcb.201702058
Rath, E., Berger, E., Messlik, A., Nunes, T., Liu, B., Kim, S. C., et al. (2011). Induction of dsRNA-activated protein kinase links mitochondrial unfolded protein response to the pathogenesis of intestinal inflammation. Gut 61, 1269–1278. doi:10.1136/gutjnl-2011-300767
Rath, E. B. (2012). Mitochondrial unfolded protein Response in the epithelium: relevance to intestinal InflammationVerlag. Dr. Hut.
Rath, E., Moschetta, A., and Haller, D. (2018). Mitochondrial function - gatekeeper of intestinal epithelial cell homeostasis. Nat. Rev. Gastroenterol. Hepatol. 15 (8), 497–516. doi:10.1038/s41575-018-0021-x
Ron, D., and Walter, P. (2007). Signal integration in the endoplasmic reticulum unfolded protein response. Nat. Rev. Mol. Cell Biol. 8 (7), 519–529. doi:10.1038/nrm2199
Ryan, M. T., and Hoogenraad, N. J. (2007). Mitochondrial-nuclear communications. Annu. Rev. Biochem. 76, 701–722. doi:10.1146/annurev.biochem.76.052305.091720
Samluk, L., Urbanska, M., Kisielewska, K., Mohanraj, K., Kim, M. J., Machnicka, K., et al. (2019). Cytosolic translational responses differ under conditions of severe short-term and long-term mitochondrial stress. Mol. Biol. Cell 30 (15), 1864–1877. doi:10.1091/mbc.E18-10-0628
Song, J., Herrmann, J. M., and Becker, T. (2021). Quality control of the mitochondrial proteome. Nat. Rev. Mol. Cell Biol. 22 (1), 54–70. doi:10.1038/s41580-020-00300-2
Suomalainen, A., and Battersby, B. J. (2018). Mitochondrial diseases: the contribution of organelle stress responses to pathology. Nat. Rev. Mol. Cell Biol. 19 (2), 77–92. doi:10.1038/nrm.2017.66
Sutandy, F. X. R., Gossner, I., Tascher, G., and Munch, C. (2023). A cytosolic surveillance mechanism activates the mitochondrial UPR. Nature 618 (7966), 849–854. doi:10.1038/s41586-023-06142-0
Tigano, M., Vargas, D. C., Tremblay-Belzile, S., Fu, Y., and Sfeir, A. (2021). Nuclear sensing of breaks in mitochondrial DNA enhances immune surveillance. Nature 591 (7850), 477–481. doi:10.1038/s41586-021-03269-w
Vogtle, F. N. (2021). Open questions on the mitochondrial unfolded protein response. FEBS J. 288 (9), 2856–2869. doi:10.1111/febs.15569
Yano, M. (2017). ABCB10 depletion reduces unfolded protein response in mitochondria. Biochem. Biophys. Res. Commun. 486 (2), 465–469. doi:10.1016/j.bbrc.2017.03.063
Yoon, J., Lee, M., Ali, A. A., Oh, Y. R., Choi, Y. S., Kim, S., et al. (2022). Mitochondrial double-stranded RNAs as a pivotal mediator in the pathogenesis of Sjӧgren's syndrome. Mol. Ther. Nucleic Acids 30, 257–269. doi:10.1016/j.omtn.2022.09.020
Youssef, O. A., Safran, S. A., Nakamura, T., Nix, D. A., Hotamisligil, G. S., and Bass, B. L. (2015). Potential role for snoRNAs in PKR activation during metabolic stress. Proc. Natl. Acad. Sci. U. S. A. 112 (16), 5023–5028. doi:10.1073/pnas.1424044112
Zhu, Y., Zhang, M., Wang, W., Qu, S., Liu, M., Rong, W., et al. (2023). Polynucleotide phosphorylase protects against renal tubular injury via blocking mt-dsRNA-PKR-eIF2α axis. Nat. Commun. 14 (1), 1223. doi:10.1038/s41467-023-36664-0
Zurita Rendon, O., and Shoubridge, E. A. (2018). LONP1 is required for maturation of a subset of mitochondrial proteins, and its loss elicits an integrated stress response. Mol. Cell Biol. 38 (20), e00412-17. doi:10.1128/MCB.00412-17
Glossary
Keywords: mitochondrial unfolded protein response, inflammatory bowel diseases, doublestranded RNA, proteostasis, double-stranded RNA-activated protein kinase, integrated stress response, mitochondria, stress signaling
Citation: Rath E (2023) PKR activation in mitochondrial unfolded protein response-mitochondrial dsRNA might do the trick. Front. Cell Dev. Biol. 11:1270341. doi: 10.3389/fcell.2023.1270341
Received: 31 July 2023; Accepted: 14 August 2023;
Published: 29 August 2023.
Edited by:
Tadahiro Nagaoka, Fujita Health University, JapanReviewed by:
Chiwei Xu, The Rockefeller University, United StatesCopyright © 2023 Rath. This is an open-access article distributed under the terms of the Creative Commons Attribution License (CC BY). The use, distribution or reproduction in other forums is permitted, provided the original author(s) and the copyright owner(s) are credited and that the original publication in this journal is cited, in accordance with accepted academic practice. No use, distribution or reproduction is permitted which does not comply with these terms.
*Correspondence: Eva Rath, ZXZhLnJhdGhAdHVtLmRl