- Department of Hepatobiliary Surgery, Beijing Chao-Yang Hospital Affiliated to Capital Medical University, Beijing, China
Succinate serves as an essential circulating metabolite within the tricarboxylic acid (TCA) cycle and functions as a substrate for succinate dehydrogenase (SDH), thereby contributing to energy production in fundamental mitochondrial metabolic pathways. Aberrant changes in succinate concentrations have been associated with pathological states, including chronic inflammation, ischemia/reperfusion (IR) injury, and cancer, resulting from the exaggerated response of specific immune cells, thereby rendering it a central area of investigation. Recent studies have elucidated the pivotal involvement of succinate and SDH in immunity beyond metabolic processes, particularly in the context of cancer. Current scientific endeavors are concentrated on comprehending the functional repercussions of metabolic modifications, specifically pertaining to succinate and SDH, in immune cells operating within a hypoxic milieu. The efficacy of targeting succinate and SDH alterations to manipulate immune cell functions in hypoxia-related diseases have been demonstrated. Consequently, a comprehensive understanding of succinate’s role in metabolism and the regulation of SDH is crucial for effectively targeting succinate and SDH as therapeutic interventions to influence the progression of specific diseases. This review provides a succinct overview of the latest advancements in comprehending the emerging functions of succinate and SDH in metabolic processes. Furthermore, it explores the involvement of succinate, an intermediary of the TCA cycle, in chronic inflammation, IR injury, and cancer, with particular emphasis on the mechanisms underlying succinate accumulation. This review critically assesses the potential of modulating succinate accumulation and metabolism within the hypoxic milieu as a means to combat various diseases. It explores potential targets for therapeutic interventions by focusing on succinate metabolism and the regulation of SDH in hypoxia-related disorders.
1 Introduction
Succinate and succinate dehydrogenase (SDH) are essential components of the tricarboxylic acid (TCA) cycle and are integral to the production of adenosine triphosphate (ATP) within mitochondria (Murphy and O'Neill, 2018). Additionally, succinate serves as a critical participant in various metabolic pathways, contributing to the regulation of numerous catabolic and anabolic processes within different stages of the cycle (Mills and O'Neill, 2014).
Over the past decade, there has been a growing recognition of the capacity of immune cells to adapt their metabolic processes in order to maintain immune homeostasis in hypoxic environments. These metabolic alterations have been found to have significant implications for the immune response to chronic inflammation, ischemia/reperfusion (IR) injury, and tumors (Fulcher and Katelaris, 1991; Murphy and Chouchani, 2022). Recent literature has increasingly highlighted the non-canonical functions of succinate levels and the regulation of SDH beyond their traditional role in metabolism. For instance, succinate has been found to stimulate dendritic cells (DCs) and stabilize hypoxia-inducible factor-1α (HIF-1α) via the succinate receptor, G protein-coupled receptor 91 (GPR91), in distinct tumors and activated macrophages (Mills and O'Neill, 2014; O'Neill and Pearce, 2016; Huang and Millar, 2013; Dalla Pozza et al., 2020). Furthermore, mutations in the SDH gene have been detected in various cancer types, suggesting potential mechanisms that lead to abnormal succinate accumulation (Peti-Peterdi, 2010). Succinate can function as an oncometabolite in tumorigenesis and cancer progression, and it can also induce post-translational modifications (PTMs) of proteins through succinylation (Mills and O'Neill, 2014; Mills et al., 2021). This review offers a comprehensive analysis of the roles played by succinate and the changes in SDH expression or activity that are linked to modifications in immune cell function during hypoxic conditions. Alongside summarizing the pathways leading to succinate accumulation, our objective is to elucidate potential therapeutic targets by focusing on succinate metabolism in the context of inflammation, IR injury, and cancer.
2 Succinate and SDH in metabolism
2.1 Biogenesis of succinate
Succinate, or butanedioic acid, was initially isolated and identified from amber through the process of dry distillation by Georgius Agricola, a German chemist, in 1,546 (Tretter et al., 2016). One of the most significant scientific breakthroughs of the 20th century was the elucidation of the TCA cycle, also known as the citric acid cycle, by Hans Adolf Krebs. In 1937, Krebs established that the succinate-fumarate-malate-oxaloacetate pathway constituted a vital component of the TCA cycle, with the inclusion of citrate, isocitrate, and α-ketoglutarate (α-KG) (Raju, 1999). For decades, the TCA cycle has been extensively employed in the microbial synthesis of citrates, glutamates, and succinates (Vuoristo et al., 2016). In the TCA cycle, succinate is synthesized through the catalytic action of succinyl-CoA synthetase from succinyl-CoA. Subsequently, succinate is promptly converted to fumarate by the enzyme SDH. In summary, succinate and SDH are of paramount importance in ATP generation and serve as pivotal cyclic pathways in metabolism, functioning as a reservoir for catabolic processes and as a point of origin for various anabolic processes (Chinopoulos, 2013).
2.2 Roles of SDH in the TCA cycle and ETC
Succinate and SDH exhibit a robust interconnection within the TCA cycle and electron transport chain (ETC.). SDH, referred to as complex II, facilitates the integration of two significant pathways within mitochondria, namely, the oxidation of succinate to fumarate as a vital constituent of the TCA cycle and the transformation of ubiquinone to ubiquinol in the mitochondrial, ETC. Both processes are indispensable for oxidative phosphorylation, which is responsible for ATP generation to sustain biogenesis (Gill, 2012; Tretter et al., 2016).
The structure of SDH is characterized by its intricate composition of multiple subunits, namely, SDHA, SDHB, SDHC, and SDHD. In addition, SDHAF1 and SDHAF2 function as assembly factors for the associated assembly process (Moosavi et al., 2019). Notably, SDHC and SDHD possess hydrophobic properties that enable their anchoring in the inner mitochondrial membrane (Her and Maher, 2015). Conversely, SDHA and SDHB extend into the matrix. SDHA is covalently linked to a flavin adenine dinucleotide (FAD) prosthetic group and harbors the binding site for succinate. In the presence of succinate, SDHA facilitates the oxidation of succinate to fumarate by bringing succinate into close proximity with the isoalloxazine ring of FAD (Hagerhall, 1997). SDHB serves as a connecting link between SDHA and SDHC and SDHD. Within SDHB, three Fe-S centers are present, which facilitate the transfer of electrons from succinate to ubiquinone for utilization in the aerobic and energy-generating respiratory chain in eukaryotic mitochondria and various prokaryotes (Yankovskaya et al., 2003). The Fe-S centers, as subunit components of SDH, offer new perspectives for the development of candidate vaccines aimed at inducing anti-embryonation and anti-fecundity immunity (Yu et al., 2007). The SDH complex is comprised of two ubiquinone-binding sites (Yankovskaya et al., 2003; Sun et al., 2005). Residues originating from SDHB, SDHC, and SDHD, which are situated in close proximity to the matrix side of the inner mitochondrial membrane, contribute to the formation of the high-affinity binding sites, thereby enhancing their efficiency (Silkin et al., 2007; Szeto et al., 2007). Conversely, the low-affinity binding site, located nearer to the intermembrane space of the inner mitochondrial membrane, is formed by SDHC and SDHD (Her and Maher, 2015). Consequently, the oxidation of succinate to fumarate by SDH results in the generation of flavin adenine dinucleotide, reduced (FADH2). In the, ETC., only SDH (complex II) is completely encoded in nuclear deoxyribonucleic acid (DNA), and electrons from succinate oxidation are transferred to ubiquinone in the, ETC (Vuoristo et al., 2016).
Moreover, ATP can also be generated through substrate-level phosphorylation (SLP). Succinyl-CoA and adenosine diphosphate (ADP) [or guanosine diphosphate (GDP)] undergo catalysis by succinyl-CoA synthetase, resulting in the production of succinate, CoASH, and ATP [or guanosine triphosphate (GTP)]. This metabolic process, referred to as SLP, is predominantly utilized in mitochondria to generate ATP (Johnson et al., 1998). Notably, SLP in the TCA cycle, facilitated by succinyl-CoA ligase (SUCL), operates independently of the respiratory chain and the mitochondrial proton motive force. This ATP production mechanism is in addition to oxidative phosphorylation (OXPHOS) and adenylate kinase (AK) reactions (Bruns and Regina, 1977; Johnson et al., 1998; Nobumoto et al., 1998; Panayiotou et al., 2014; Komlodi and Tretter, 2017). In hence, comprehending the significance of SDH and succinate in metabolic processes can offer novel perspectives for the formulation of therapeutic approaches aimed at addressing inflammation, IR injury, and cancer (Hagerhall, 1997).
3 Succinate as a metabolic signal in immuno-inflammatory response
Significant research findings intriguingly indicate that succinate may serve as a novel category of regulators in inflammation, acting as crucial signals to modulate the inflammatory process (Mills and O'Neill, 2014; Tannahill et al., 2013; Grimolizzi and Arranz, 2018; Palsson-McDermott and O'Neill, 2013). Succinate is increasingly recognized as a significant signal in the immuno-inflammatory response.
3.1 Stabilization of HIF-1α in inflammation
In hypoxic inflammatory microenvironments, the activation of immune cells, including macrophages and DCs, induces a metabolic shift towards glycolysis (Mills and O'Neill, 2014; Tannahill and O'Neill, 2011). This metabolic shift towards glycolysis in activated immune cells is thought to have a significant impact in low oxygen conditions, such as hypoxic inflammatory sites. The accumulation of succinate has the potential to enhance inflammatory signaling and greatly influence the immuno-inflammatory response. Various potential sources may contribute to the accumulation of succinate. Firstly, these studies have provided confirmation that succinate accumulation resulting from SDH mutations has the ability to stabilize HIF-1α in activated macrophages, particularly when the activity of the prolyl hydroxylase domain (PHD) enzyme is inhibited. This stabilization subsequently leads to the production of the proinflammatory cytokine interleukin 1β (IL-1β) through the signaling of HIF-1α (Mills and O'Neill, 2014; O'Neill and Pearce, 2016; Tannahill et al., 2013; Zhang et al., 2021; Peace and O'Neill, 2022; Williams and O'Neill, 2018) (Figure 1). In addition, it has been observed that succinate accumulation can also occur in lipopolysaccharide (LPS)-activated macrophages through the process of glutamine metabolism, which facilitates the anaplerosis of α-KG into the TCA cycle (Rubic et al., 2008) (Figure 1). Subsequently, the “GABA shunt” pathway can lead to the accumulation of succinate, as it is stimulated by the increased levels of γ-aminobutyric acid (GABA) and its transporters induced by LPS (Figure 1). This pathway can be hindered by vigabatrin which inhibits an essential enzyme of the GABA shunt, resulting in a reduction in succinate accumulation (Figure 1). Additionally, the inhibition of GABA transaminase (GABA-T), a critical enzyme of the GABA shunt, with vigabatrin can effectively prevent succinate accumulation (Tannahill et al., 2013; Ren et al., 2019) (Figure 1). Finally, succinate accumulation can arise from the inhibition of SDH activity during hypoxia and various other pathways. In addition to hypoxia, FAD-induced decrease in nicotinamide adenine dinucleotide (NAD+) levels caused by LPS also hampers SDH activity (Wang et al., 2021) (Figure 1). NAD+ demonstrates multiple anti-inflammatory properties through the activation of sirtuins, a group of NAD+-dependent deacetylases. Specifically, sirtuin 1 (SIRT1) suppresses glycolysis and the activity of proinflammatory transcription factors such as nuclear factor-κB (NF-κB) and HIF-1α (Schug et al., 2010; Misawa et al., 2013), while also promoting autophagy-related gene function and oxidative metabolism. Conversely, the inactivation of SIRT3 has been observed to heighten the activation of the NOD-like receptor family, pyrin domain containing 3 (NLRP3) inflammasome (Schug et al., 2010; Misawa et al., 2013) (Figure 1).
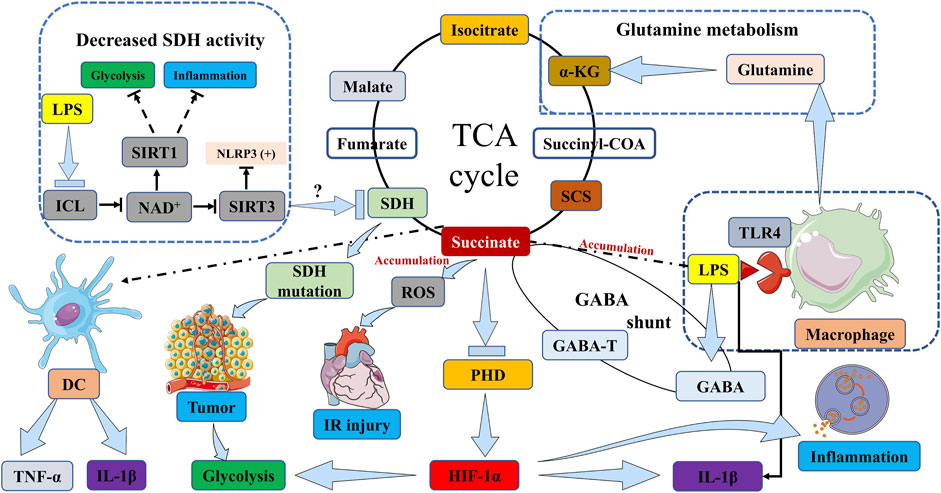
FIGURE 1. Succinate accumulation pathways in immune cells, inflammation, IR injury and tumor. The activation of macrophages by LPS induces TLR4 signaling, which subsequently initiates the accumulation of succinate. This accumulation of succinate in activated macrophages stabilizes HIF-1α, particularly in the presence of inhibited PHD enzyme activity, thereby resulting in the production of IL-1β through HIF-1α signaling. In DCs, the stimulation of succinate and LPS leads to an increase in the expression of IL-1β and TNF-α. In the context of tumorigenesis, mutations in the SDH gene lead to the accumulation of succinate, which in turn stabilizes HIF-1α, thereby promoting tumor growth and exacerbating inflammation through the upregulation of glycolytic enzymes and IL-1β. Simultaneously, succinate accumulation inhibits tumor cell growth and survival. This accumulation of succinate can occur through two pathways: glutamine metabolism, which promotes the replenishment of α-KG in the TCA cycle, or the “GABA shunt” pathway, which is activated by elevated levels of GABA and its transporters induced by LPS. The inhibition of GABA-T can effectively prevent the accumulation of succinate. The transformation of isocitrate into succinate within the glyoxylate shunt is facilitated by ICL, and this process can lead to an increase in succinate levels. Furthermore, the accumulation of succinate can be attributed to the suppression of SDH activity, which can be triggered by various factors including hypoxia or reduced levels of NAD+ induced by LPS. SIRT1 suppresses glycolysis and inflammation. Conversely, the inactivation of SIRT3 has been observed to heighten the activation of NLRP3 inflammasome. ICL, isocitrate lyase; NAD+, nicotinamide adenine dinucleotide; SIRT, Sirtuin; LPS, lipopolysaccharide; SDH, succinate dehydrogenase; TCA cycle, tricarboxylic acid cycle; α-KG, α-ketoglutarate; DC, dendritic cell; TNF-α, tumor necrosis factor α; IL-1β, interleukin 1β; PHD, prolyl hydroxylase domain; HIF-1α, hypoxia-inducible factor-1α; SCS, succinyl-CoA synthetase; GABA, γ-aminobutyric acid; GABA-T, γ-aminobutyric acid transaminase; TLR4, toll-like receptor 4. IR injury, ischemia/reperfusion injury; NLRP3, NOD-like receptor family, pyrin domain containing 3.
Succinate additionally governs the immune functions of immune cells, specifically pertaining to immune cell migration, cytokine production, and augmentation of the ability of antigen-presenting cells (APCs) to elicit adaptive immune responses (Peace and O'Neill, 2022). For instance, succinate has been found to induce migration of monocyte-derived dendritic cells (MoDCs) in vitro and act as a chemokine, thereby promoting migration to draining lymph nodes in succinate-treated DCs (Rubic et al., 2008; Haffke et al., 2019). Succinate has been found to facilitate the production of cytokines through synergistic interactions with certain ligands, including tumor necrosis factor-α (TNF-α) (Rubic et al., 2008). Furthermore, succinate stimulation with LPS has been shown to increase the expression of IL-1β in murine bone marrow-derived dendritic cells (BMDCs), in addition to TNF-α (Williams and O'Neill, 2018) (Figure 1). In addition to promoting cytokine generation, succinate can also enhance the ability of APCs to induce immune responses that result in inflammatory outcomes. Multiple studies have provided evidence of succinate’s capacity to hinder tumor growth, which will be further expounded upon in the subsequent section (Dalla Pozza et al., 2020). Of greater significance, the manipulation of succinate metabolism holds potential as a microbiological therapy in host-microbe interactions (Wei et al., 2023). These findings suggest that the manipulation of succinate metabolism could be employed to devise innovative therapeutics for the prevention and treatment of inflammation.
3.2 Succinate and SUCNR1 signaling during inflammation
There have been numerous studies demonstrating the interaction between the ligand-receptor pair succinate receptor 1 (SUCNR1) (formerly known as GPR91) and succinate, beyond its role as a metabolic signal in inflammation by stabilizing HIF-1α (de Castro Fonseca et al., 2016; Ristic et al., 2017). Wittenberger et al. initially discovered several G-protein coupled receptors, including SUCNR1 (Wittenberger et al., 2001). He et al. later identified succinate as a selective ligand of SUCNR1 (He et al., 2004). SUCNR1 was initially identified and studied in the renal system, subsequently revealing significant expression levels in the hepatic, splenic, and intestinal tissues (He et al., 2004; de Castro Fonseca et al., 2016; Gilissen et al., 2016). SUCNR1 expression has been observed in white adipocytes, hematopoietic progenitor cells (Rubic et al., 2008), as well as various blood and immune cell types (Macaulay et al., 2007; Hakak et al., 2009; de Castro Fonseca et al., 2016; Krzak et al., 2021). Succinate induces the activation of SUCNR1, which subsequently initiates signaling cascades via multiple pathways. Comparable to Gβ and Gγ signaling cascades, succinate stimulation results in the mobilization of calcium ions (Ca2+), accumulation of inositol trisphosphate (IP3), and phosphorylation involving extracellular regulated kinase (ERK) (He et al., 2004; Robben et al., 2009; Gilissen et al., 2016) (Figure 2). Gaining a comprehensive understanding of the signaling pathways triggered by the interaction between SUCNR1 and succinate can offer novel perspectives for the advancement of therapeutic approaches targeting specific diseases, particularly those associated with chronic inflammation.
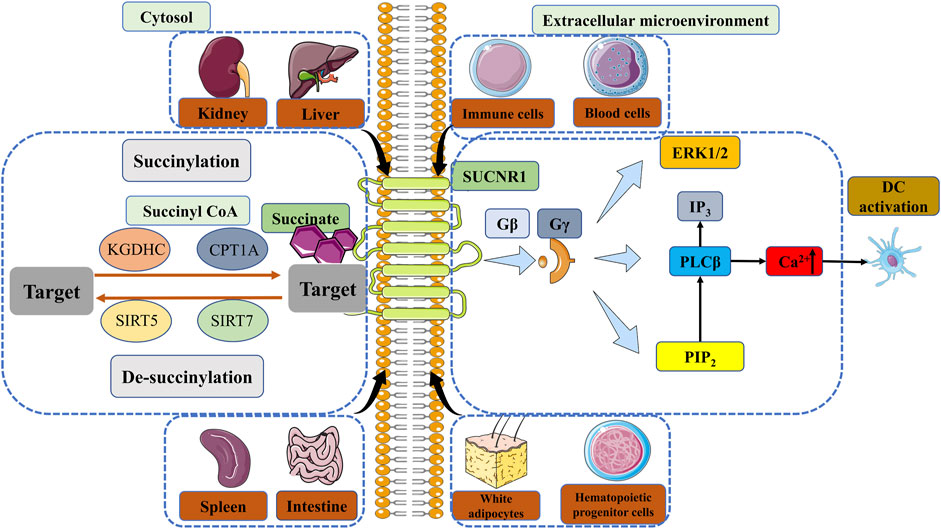
FIGURE 2. The regulation of succinylation, desuccinylation and the signal of SUCNR1. Enzymatic succinylation is facilitated by succinyl CoA and can be enhanced by KGDHC in the cytosol. CPT1A has the ability to augment lysine succinylation without altering succinyl CoA levels. The levels of SIRT5 have an impact on desuccinylase activity, resulting in modifications in succinylation. Desuccinylation catalyzed by SIRT7 primarily occurs in the nucleus and plays a crucial role in the response to DNA damage and cell survival. Succinate in the extracellular microenvironment can transmit signals through GPR91, thereby sustaining cytokine production and activating DCs. The comprehension of enzymatic succinylation and desuccinylation pathways and mechanisms elucidates the potential of succinate metabolism as a therapeutic approach for the treatment of diverse diseases, such as inflammation, IR injury and cancer. KGDHC, α-ketoglutarate dehydrogenase complex; CPT1A, carnitine palmitoyltransferase 1A; SIRT, Sirtuin; SUCNR1, succinate receptor 1; GPR91, G protein-coupled receptor 91; ERK, extracellular regulated kinase; IP3, inositol trisphosphate; PIP2, phosphatidylinositol (Fulcher and Katelaris, 1991; O'Neill and Pearce, 2016) bisphosphate; PLCβ, phospholipase Cβ; Ca2+, calcium ion; DC, dendritic cell.
Recent studies have provided evidence indicating the involvement of SUCNR1 in multiple succinate-dependent inflammatory processes in vivo. Firstly, it was observed that SUCNR1-deficient mice exhibited heightened migration of DCs in comparison to their wild-type counterparts. Secondly, the absence of SUCNR1 in DCs resulted in the absence of cytokine elevation, thereby supporting the notion that succinate functions as a conventional signal to enhance the antigen-presenting function of APCs. Lastly, solid organ transplantation from SUCNR1-deficient mice exhibited prolonged graft survival when compared to that from wild-type mice (Rubic et al., 2008). Peruzzotti-Jametti et al. have presented significant evidence demonstrating that the inhibition of SUCNR1 leads to a reduction in carrier-mediated succinate uptake. This suggests that the signaling of SUCNR1 promotes the expression of plasma membrane Na+-dependent dicarboxylic acid transporters, which facilitate the transportation of succinate across the membrane. These findings introduce intriguing possibilities regarding the involvement of succinate in the paracrine/autocrine regulation of disease development (Peruzzotti-Jametti et al., 2018). To sum up, the aforementioned findings have indicated that SUCNR1 plays a pivotal role in the immune-inflammatory response triggered by succinate. Consequently, the modulation of SUCNR1 signaling presents a promising therapeutic strategy for the management of inflammatory disorders.
3.3 Succinate and succinylation in signal transduction
Succinylation, a PTM mechanism, entails the incorporation of a succinyl group onto a protein residue, predominantly lysine residues, thereby influencing the functionality of amino groups (Hirschey and Zhao, 2015; Alleyn et al., 2018; Sreedhar et al., 2020; Chinopoulos, 2021). Recent investigations have concentrated on the succinylation modification, wherein succinyl CoA can form an amide bond with protein lysine (Zhao et al., 2023). The identification of elevated succinate levels in numerous proteins involved in the regulation of diverse cellular and biological processes underscores the significance of succinylation as a crucial biological function (Xie et al., 2012; Park et al., 2013; Hirschey and Zhao, 2015). The catalytic processes involved in succinylation mediated by multiple enzymes, remain poorly understood. Consequently, there is ongoing research aimed at elucidating these fundamental mechanisms in order to facilitate the development of innovative therapeutic interventions and associated pharmaceuticals. Numerous investigations have demonstrated that succinylation can occur in organisms through both enzymatic and non-enzymatic means. In fact, the majority of is known to occur through non-enzymatic processes, as extensively documented in the literature (Sreedhar et al., 2020; Shen et al., 2023; Zhao et al., 2023), particularly by Matthew D Hirschey et al., who have demonstrated the higher chemical reactivity of succinyl-CoA compared to other acyl-CoA species (Wagner et al., 2017). Non-enzymatic succinylation appears to be influenced by various factors, including the concentration-dependent levels of reactants within mitochondria, while the catalysis of enzymatic succinylation may be regulated by succinyl CoA. Enzymatic succinylation may be promoted via α-ketoglutarate dehydrogenase complex (KGDHC) and E1k [α-Ketoglutarate Dehydrogenase (KGDH)] beyond succinyl CoA (Yang and Gibson, 2019) (Figure 2). Succinylation is consistently mediated by KGDHC in the cytosol and nucleus. Enzymatic TCA cycle steps like succinyl-CoA synthetase (SCS) can also regulate succinyl-CoA levels, which then non-enyzmatically drive succinylation (Li et al., 2015; Carrico et al., 2018; Gut et al., 2020). The carnitine palmitoyltransferase 1A (CPT1A) G710E mutant has been observed to enhance cell proliferation in the presence of metabolic stress, while CPT1A increases lysine succinylation without any changes in succinyl CoA levels in mammalian cells (Kurmi et al., 2018; Wang et al., 2019). This finding suggests that the lysine succinyltransferase activity of CPT1A may succinylate downstream substrate proteins, thereby facilitating proliferation (Kurmi et al., 2018; Sreedhar et al., 2020). In contrast to the established mechanism of succinyl-CoA non-enzymatic lysine succinylation, the mechanism promoted by CPT1A remains controversial and has not been shown in vivo (Sreedhar et al., 2020; Shen et al., 2023). At best, this mechanism would account for a minor fraction of succinylation (Sreedhar et al., 2020). The potential impact of concentration-dependent acyl-CoA distribution on succinylation in certain tissues has been identified (Weinert et al., 2013). This finding implies that the tissue-specific regulation of acyl-CoA concentrations offers novel therapeutic strategies for modulating succinylation in certain diseases. Furthermore, the regulation of de-succinylation plays a crucial role in controlling various catalytic processes across all cellular compartments, with succinylation potentially exhibiting a balanced reciprocal relationship. Notably, the levels of NAD+-dependent SIRT5 have been found to influence dynamic changes in desuccinylase activity (Figure 2). Certain studies have provided evidence indicating that the inhibition of SIRT5 results in an elevation of succinylation in particular proteins, thereby contributing to the development and progression of cancer (Park et al., 2013). SIRT7, on the other hand, acts as a histone desuccinylase that is functionally associated with chromatin compaction and the maintenance of genome stability (Li et al., 2016) (Figure 2). The process of desuccinylation catalyzed by SIRT7 predominantly occurs within the nucleus and plays a crucial role in the DNA damage response and the survival of cells (Li et al., 2016).
As previously stated, the maintenance of a delicate equilibrium between succinylation and desuccinylation processes is of utmost importance for the regulation of physiological functions (Wang and Lin, 2021). Disruption of this equilibrium can lead to the development of various diseases, including inflammatory disorders, IR injury, and cancer (Choudhary et al., 2014; Sabari et al., 2017; Yang et al., 2017). The intricate involvement of SIRT5 in the process of carcinogenesis is currently under investigation by researchers (Yang et al., 2017) (Figure 2). It has been proposed that desuccinylation events facilitated by SIRT5 may play a role in the initiation and progression of tumorigenesis. The inhibition of SIRT5 has demonstrated a reduction in cell proliferation and tumor growth, implying that hyper-succinylation may exert similar effects on tumor growth by modulating SIRT5 (Li et al., 2015; Xiangyun et al., 2017). Furthermore, mounting evidence suggests that lysine succinylation is abnormally elevated during cancer development, indicating that succinylation regulates tumor energy metabolism (Song et al., 2017). Mutations in isocitrate dehydrogenase isoform 1 that impede SDH result in an elevated level of succinyl-CoA, thereby promoting cancerous metabolism (Li et al., 2015). The augmentation of succinylation under hypoxic conditions may potentially counteract the effects of IR injury in both cardiac and cerebral tissues. The proficient regulation of succinylation holds promise for unveiling novel therapeutic strategies for managing IR injury and potentially facilitating transplantation procedures (Murphy and O'Neill, 2018). In summary, comprehending the tissue-specific mechanisms implicated is imperative for the advancement of pharmacological interventions targeting succinylation and de-succinylation in hypoxia-related ailments such as inflammation, IR injury, and cancer.
4 Succinate and SDH in IR injury
IR injury is commonly observed when the blood supply to a solid organ, such as the heart, brain, lung, or kidney, is disrupted, subsequently followed by reperfusion in specific pathological conditions like heart attack, ischemic stroke, kidney IR injury, or organ transplantation (Kula-Alwar et al., 2019). The underlying mechanism of this injury primarily involves the generation of ROS by mitochondria, which triggers a cascade of events including aberrant immune responses, accumulation of succinate, and cellular harm (Yellon and Hausenloy, 2007; Murphy and Steenbergen, 2008; Burwell et al., 2009; Eltzschig and Eckle, 2011; Timmers et al., 2012) (Figure 1). During the state of ischemia, the absence of oxygen induces a reduction in mitochondrial respiration and a rise in the accumulation of succinate. Subsequent reperfusion prompts the swift oxidation of the accumulated succinate by SDH. Reverse electron transport (RET) through mitochondrial complex I allows some of the electrons in the mitochondria to break away from, ETC, leading to an inadequate reduction reaction of oxygen, which in turn drives the production of ROS that inflict oxidative harm upon cellular constituents such as lipids, proteins, and DNA (Figure 1). This oxidative damage serves to intensify the inflammatory response, thereby precipitating tissue injury and dysfunction. The significance of succinate and SDH’s involvement in the pathogenesis of IR injury underscores the potential of targeting succinate metabolism as a therapeutic strategy for the prevention and treatment of this condition (Panconesi et al., 2022). The development of pharmaceuticals that specifically target succinate metabolism may offer a novel approach to address the clinical challenge posed by IR injury.
SDH is a key enzyme involved in succinate formation during ischemia and its oxidation upon reperfusion (Davidson et al., 2019). Malonate, a competitive inhibitor of SDH, has emerged as a candidate therapy for selective SDH inhibition to reduce reperfusion injury. The protective effect of malonate was demonstrated using the malonate prodrug dimethyl malonate, which was effective when administered before and throughout ischemia (Chouchani et al., 2014). Adequate studies have shown that IR injury can be regulated through the accumulation of succinate during ischemia, which is subsequently re-oxidized by SDH to generate mitochondrial ROS (Chouchani et al., 2014). Succinate, therefore, acts as a metabolic signal of IR injury, responsible for generating mitochondrial ROS (Figure 1). Blocking ischemic succinate accumulation resulting from oxidation can abolish IR injury (Pell et al., 2016). Similar studies have demonstrated that preventing succinate from oxidation may decrease IR injury and increase tolerance in the process of liver transplantation (LT) from steatosis liver donors (Evans et al., 2008). By targeting succinate metabolism, malonate may provide additional therapeutic strategies for heart damage underlying chronic heart failure (Pell et al., 2016). In addition to malonate, it has been determined that ginsenoside Rb1 possesses the ability to mitigate myocardial IR injury by inhibiting the production of ROS originating from mitochondrial complex I according to a proteomic analysis (Jiang et al., 2021). The cerebral IR injury results in the ischemic accumulation of succinate, which subsequently induces Cdc42 succinylation and inhibits the proliferation of neural stem cells (Huang et al., 2023).
However, despite promising results in vitro and animal models, translation to human trials has proved challenging, with high failure rates due to low drug exposure at the target site or clinical safety issues. Translation failure in IR injury is likely caused by delivery difficulties or insufficient knowledge of the pathological mechanisms, leading to inappropriate drug targets. Therefore, further research is needed to develop effective and safe therapeutic strategies for targeting succinate metabolism in IR injury (Dambrova et al., 2021).
5 Succinate and SDH in tumor
Metabolites originating from the TCA cycle and respiratory enzymes, specifically succinate and SDH, have been found to have a significant impact on the initiation and progression of tumorigenesis (Mullen and DeBerardinis, 2012; Dalla Pozza et al., 2020; Kes et al., 2020). Notably, alterations in SDH activity leading to the accumulation of succinate, such as SDH mutations, have been observed (Dalla Pozza et al., 2020). Furthermore, extensive investigation has been conducted to elucidate the role of succinate in the development of cancer, encompassing its capacity to induce epigenetic and metabolic modifications, as well as its influence on cell migration, invasion, and angiogenesis.
5.1 Succinate and SDH in cancer-immune cycle
Succinate has garnered growing recognition for its multifaceted involvement in both immune and cancer-related processes, in addition to its established role in immuno-inflammatory responses. The cumulative evidence from numerous interconnected studies has consistently demonstrated that succinate possesses the capacity to regulate tumorigenesis within specific intrinsic microenvironments. Consequently, succinate is now acknowledged as a classical tumorigenic signal, alongside its well-established association with inflammation (Huang and Millar, 2013; Jiang and Yan, 2017).
SDH mutations and associated succinate pathways have been demonstrated to contribute to tumorigenesis (Bardella et al., 2011). Specifically, mutations in the SDH gene have been observed to expedite tumor development and can be detected in tumor tissues and cells (Pasini and Stratakis, 2009; Gaude and Frezza, 2014). The SDH enzyme plays a crucial role in the TCA cycle by facilitating the conversion of succinate to fumarate. Mutations in the SDH gene have been identified in various tumors, establishing SDH as a recognized tumor suppressor (Bardella et al., 2011; Wallace, 2012; Wu and Zhao, 2013; Jiang and Yan, 2017). However, mutations in this gene can result in succinate accumulation, which in turn stabilizes HIF-1α and fosters tumor growth (Selak et al., 2005) (Figure 1). Hence, it is imperative to engage in a comprehensive examination of succinate accumulation in the context of cancer. Succinate accumulation can manifest through diverse pathways that contribute to the development of tumors and immune responses. The regulation of SDH activity plays a crucial role in the buildup of succinate. Notably, the presence of a mutation in the gene responsible for encoding SDH in certain cancer types has been observed to diminish SDH activity, resulting in succinate accumulation and subsequent augmentation of mitochondrial ROS generation (Drose, 2013) (Figure 1). Hence, the functional deficiency of subunits in SDH resulting from mutation and carcinogenesis has garnered significant attention in numerous studies on metabolism (Tomitsuka et al., 2010). Furthermore, the function of SDH is reliant on the presence of oxidized FAD and NAD+ as cofactors (Gill, 2012). However, under conditions of hypoxia where these cofactors are predominantly in their reduced forms, SDH function is hindered, leading to the accumulation of succinate in the cancer-immunity cycle (Jiang and Yan, 2017). It is noteworthy to mention that the accumulation of succinate in M1 macrophages is closely associated with the activation of toll-like receptor 4 (TLR4) signaling. Upon activation by LPS, TLR4 signaling is triggered, resulting in the disruption of the Krebs cycle (O'Neill and Pearce, 2016) (Figure 1). This disruption subsequently leads to metabolic reprogramming and the accumulation of succinate. Previous research has demonstrated that LPS-activated macrophages induce succinate accumulation by inhibiting the activity of the PHD enzyme, thereby stabilizing HIF-1α (Figure 1). The stabilization of target genes responsible for encoding glycolytic enzymes and the pro-inflammatory cytokine IL-1β, which can impede tumor cell growth, enhance tumor survival, and intensify inflammation, is facilitated by this process (O'Neill and Pearce, 2016; Selak et al., 2005; Liu et al., 2016; Aspuria et al., 2014) (Figure 1). Succinate, in aggregate, may possess two distinct functions in the development of tumors, either promoting or hindering tumor growth. Nevertheless, thus far, SDH has exclusively demonstrated its involvement in suppressing tumor growth and advancement.
In the context of the cancer-immune cycle, succinate has the potential to exert an influence on the immune responses of various immune cells, including APCs and T cells. Notably, succinate has been observed to enhance the antigen presentation capacity of DCs by eliciting an adaptive response and impeding tumor growth (Palucka and Banchereau, 2012). Furthermore, upon encountering a sequence of stimuli originating from antigens, heightened activation of antigen-specific T cells can result in the secretion of cytokines such as TNF-α and interferon-γ (IFN-γ) during immune activation (Cortese et al., 2014) (Figure 1). These cytokines possess the ability to impede the progression of cancer cells and extend the survival of patients (Hadrup et al., 2013; Jiang and Yan, 2016).
5.2 Succinate and SDH in tumorigenesis
Po-Lin Tseng et al. have provided evidence indicating an inverse correlation between the malignancy of hepatocellular carcinoma (HCC) and the expression level of SDHB. Specifically, a higher degree of malignancy in HCC is associated with a lower expression of SDHB, which is significantly associated with advanced tumor stage and unfavorable prognosis (Tseng et al., 2018). Furthermore, in mouse models, experimental interventions involving the silencing and overexpression of SDHB have demonstrated the potential feasibility of regulating HCC growth and metastasis. Thus, the downregulation of SDHB expression in human HCC leads to a metabolic shift from aerobic respiration to glycolysis and the induction of the Warburg effect, ultimately facilitating tumor malignancy (Tseng et al., 2018). Studies have demonstrated that the reduction in SDH activity, resulting from the silencing of one of its subunits, promotes HCC cell proliferation and metastasis both in vitro and in vivo by increasing ROS and subsequently activating NF-κB signaling (Li et al., 2019). These findings suggest that SDH could potentially be targeted for therapeutic intervention in the treatment of HCC due to its tumor-suppressive role.
In addition to its association with HCC, the most closely correlated association with SDH is hereditary paraganglioma/phaeochromocytoma syndrome (HPGL/PCC), which is characterized by the presence of germline loss-of-function mutations in SDH genes (Brière et al., 2005). Various mechanisms of carcinogenesis have been suggested, as patients with HPGL/PCC have been found to harbor germline mutations in SDHB, SDHC, and SDHD (Eniafe and Jiang, 2021; Takács-Vellai et al., 2021). In addition to HPGL/PCC, a multitude of neuroendocrine and non-neuroendocrine neoplasms, such as gastrointestinal stromal tumors (GISTs), renal tumors, and thyroid tumors, have been discovered to exhibit a significant correlation with SDH gene mutations, alongside HPGL/PCC. The growing body of research highlights the tumor suppressor function of SDH and the oncogenic role of succinate as a metabolite in the progression of cancer (Neppala et al., 2019; Ibrahim and Chopra, 2020; Yong et al., 2020). The current research focuses on investigating the genetic and molecular mechanisms that lead to succinate accumulation as a result of SDH mutations, and how these mutations initiate neoplasm invasion and metastasis. Additionally, variants of SDH genes have been identified in thyroid C-cell hyperplasia and papillary thyroid cancers, independent of SDH mutations. Nevertheless, the importance of succinate and SDH in the development of cancer has been firmly established, resulting in the classification of succinate as an oncometabolite and SDH as a tumor suppressor.
6 Succinate and SDH as cancer biomarkers and treatment strategies
As previously stated, succinate serves as an oncometabolite, suggesting that the identification of these oncometabolites in cancer specimens from patients holds the potential to facilitate cancer detection, tumor screening, and comprehensive follow-up during the early phases of cancer (Semeraro et al., 2018). Furthermore, mass spectrometry and nuclear magnetic resonance technologies present promising methodologies that clinical practitioners can use to identify the buildup of succinate. Moreover, the utilization of immunohistochemistry (IHC) presents an opportunity to assess oncometabolites as potential cancer biomarkers in individuals afflicted with cancer (Yong et al., 2020). The abnormal expression of SDH, specifically the loss of the B subunit (SDHB), is implicated in the pathogenesis of neuroendocrine tumors as a prognostic factor, likely due to the accumulation of succinate in cancer (Hwang et al., 2014; Milione et al., 2017). A 2020 review by Dalla Pozza et al. provided a comprehensive summary of the detection of succinate and SDH as cancer biomarkers across various tissues (Dalla Pozza et al., 2020).
The deregulation of Complex II has the potential to result in an overabundance of ROS, which can induce apoptotic cell death in a manner specific to tumors. Consequently, numerous compounds that impact SDH activity have been examined for their potential as anticancer agents (Kluckova et al., 2013; Hwang et al., 2014) (Table 1). These compounds exhibit the ability to selectively target cancer cells by promoting apoptosis mediated by ROS, while preserving normal cells. Nevertheless, further investigation is necessary to comprehensively comprehend the underlying mechanisms and ascertain the effectiveness and safety of these compounds as potential anticancer treatments.
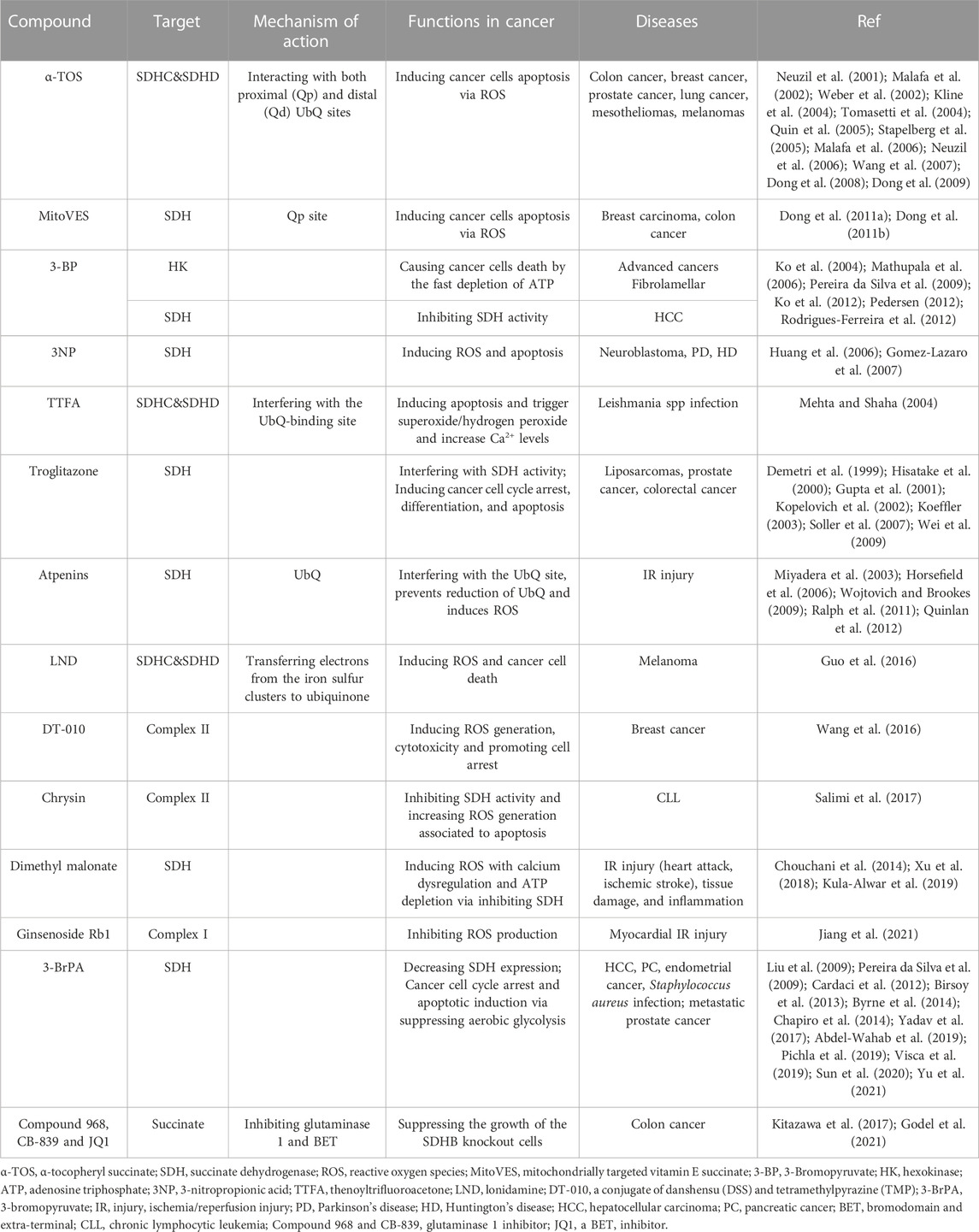
TABLE 1. Several compounds affecting SDH activity have been tested for their anticancer properties, IR injury and anti-inflammatory effects.
The identification of diverse pathways contributing to succinate accumulation presents promising avenues for the regulation of succinate levels and the modulation of tumorigenesis. The manipulation of succinate levels holds potential for the development of innovative cancer therapies. By comprehending the underlying molecular mechanisms governing succinate accumulation and its consequential impact on tumorigenesis, it becomes feasible to devise novel therapeutic approaches that specifically target succinate metabolism in malignant cells. These therapeutic interventions have the potential to enhance patient prognoses and offer efficacious treatment alternatives for diverse cancer types. Further investigation is warranted to comprehensively elucidate the precise involvement of succinate in cancer and to devise targeted therapeutic approaches that proficiently modulate its concentrations.
7 Conclusion and future perspectives
Historically, succinate and SDH have been recognized as pivotal contributors to ATP generation in the context of mitochondrial energy metabolism. Nevertheless, it has become evident that comprehending their extensive involvement in IR injury, immuno-inflammatory responses, and tumorigenesis could offer innovative and potent insights into disease control (Grimolizzi and Arranz, 2018). The diverse mechanisms involving succinate and SDH in the process of neoplasm invasion and metastasis imply that the identification of succinate as an oncometabolite could serve as a valuable diagnostic indicator for tracking tumors, whereas SDH, acting as a tumor suppressor, may present a promising target for anticancer treatment. Nevertheless, despite the potential of succinate accumulation and abnormal succinylation expression as diagnostic markers, the majority of existing studies have been conducted in laboratory settings, and it is crucial to validate ideal and efficacious molecular markers in vivo. On the other hand, despite the evident effectiveness of numerous compounds in vitro and animal models in targeting succinate to prevent ischemia-reperfusion injury and tumorigenesis, the translation of these findings from laboratory experiments to clinical application is fraught with challenges. Primarily, the attainment of the necessary concentrations of these compounds for protective and preventive purposes may present difficulties. Additionally, the development of strategies to enhance the delivery of succinate metabolism-targeting therapies to cells will be crucial in overcoming these obstacles (Gupta et al., 2021). The precise timing of targeted therapy administration holds significant importance in mitigating adverse reactions and ensuring optimal drug concentration within the affected tissue. Additionally, comprehending the pharmacokinetics of succinate-based medications during administration is crucial for establishing appropriate drug delivery schedules. Moreover, attaining selectivity towards specific tissues is imperative to prevent off-target effects. Overcoming these challenges is indispensable for the successful transition of succinate-based therapeutics from experimental settings to clinical application in the foreseeable future.
To summarize, there has been a comprehensive discourse on the role of succinate and SDH in the aforementioned areas of interest. Future investigations should persist in exploring potential diagnostic and therapeutic strategies that focus on succinate metabolism for inflammation, ischemia-reperfusion injury, and tumors. Additional research is imperative to validate the associations and underlying mechanisms between succinate and SDH in metabolic alterations as signaling pathways in the regulation of immune cells, potentially paving the way for innovative therapeutic interventions.
Author contributions
WZ: Investigation, Supervision, Visualization, Writing–original draft, Writing–review and editing. RL: Validation, Visualization, Writing–original draft, Writing–review and editing, Conceptualization, Funding acquisition, Investigation, Resources, Supervision.
Funding
The author(s) declare that no financial support was received for the research, authorship, and/or publication of this article.
Acknowledgments
We acknowledge use of the drawing platform “Figuredraw” for the production of figures in our manuscript.
Conflict of interest
The authors declare that the research was conducted in the absence of any commercial or financial relationships that could be construed as a potential conflict of interest.
Publisher’s note
All claims expressed in this article are solely those of the authors and do not necessarily represent those of their affiliated organizations, or those of the publisher, the editors and the reviewers. Any product that may be evaluated in this article, or claim that may be made by its manufacturer, is not guaranteed or endorsed by the publisher.
References
Abdel-Wahab, A. F., Mahmoud, W., and Al-Harizy, R. M. (2019). Targeting glucose metabolism to suppress cancer progression: prospective of anti-glycolytic cancer therapy. Pharmacol. Res. 150, 104511. doi:10.1016/j.phrs.2019.104511
Alleyn, M., Breitzig, M., Lockey, R., and Kolliputi, N. (2018). The dawn of succinylation: a posttranslational modification. Am. J. Physiol. Cell Physiol. 314 (2), C228–c232. doi:10.1152/ajpcell.00148.2017
Aspuria, P. P., Lunt, S. Y., Varemo, L., Vergnes, L., Gozo, M., Beach, J. A., et al. (2014). Succinate dehydrogenase inhibition leads to epithelial-mesenchymal transition and reprogrammed carbon metabolism. Cancer Metab. 2, 21. doi:10.1186/2049-3002-2-21
Bardella, C., Pollard, P. J., and Tomlinson, I. (2011). SDH mutations in cancer. Biochim. Biophys. Acta 1807 (11), 1432–1443. doi:10.1016/j.bbabio.2011.07.003
Birsoy, K., Wang, T., Possemato, R., Yilmaz, O. H., Koch, C. E., Chen, W. W., et al. (2013). MCT1-mediated transport of a toxic molecule is an effective strategy for targeting glycolytic tumors. Nat. Genet. 45 (1), 104–108. doi:10.1038/ng.2471
Brière, J. J., Favier, J., El Ghouzzi, V., Djouadi, F., Bénit, P., Gimenez, A. P., et al. (2005). Succinate dehydrogenase deficiency in human. Cell Mol. Life Sci. 62 (19-20), 2317–2324. doi:10.1007/s00018-005-5237-6
Bruns, G. A., and Regina, V. M. (1977). Adenylate kinase 2, a mitochondrial enzyme. Biochem. Genet. 15 (5-6), 477–486. doi:10.1007/bf00520192
Burwell, L. S., Nadtochiy, S. M., and Brookes, P. S. (2009). Cardioprotection by metabolic shut-down and gradual wake-up. J. Mol. Cell Cardiol. 46 (6), 804–810. doi:10.1016/j.yjmcc.2009.02.026
Byrne, F. L., Poon, I. K., Modesitt, S. C., Tomsig, J. L., Chow, J. D., Healy, M. E., et al. (2014). Metabolic vulnerabilities in endometrial cancer. Cancer Res. 74 (20), 5832–5845. doi:10.1158/0008-5472.Can-14-0254
Cardaci, S., Rizza, S., Filomeni, G., Bernardini, R., Bertocchi, F., Mattei, M., et al. (2012). Glutamine deprivation enhances antitumor activity of 3-bromopyruvate through the stabilization of monocarboxylate transporter-1. Cancer Res. 72 (17), 4526–4536. doi:10.1158/0008-5472.Can-12-1741
Carrico, C., Meyer, J. G., He, W., Gibson, B. W., and Verdin, E. (2018). The mitochondrial acylome emerges: proteomics, regulation by sirtuins, and metabolic and disease implications. Cell Metab. 27 (3), 497–512. doi:10.1016/j.cmet.2018.01.016
Chapiro, J., Sur, S., Savic, L. J., Ganapathy-Kanniappan, S., Reyes, J., Duran, R., et al. (2014). Systemic delivery of microencapsulated 3-bromopyruvate for the therapy of pancreatic cancer. Clin. Cancer Res. 20 (24), 6406–6417. doi:10.1158/1078-0432.Ccr-14-1271
Chinopoulos, C. (2021). The mystery of extramitochondrial proteins lysine succinylation. Int. J. Mol. Sci. 22 (11), 6085. doi:10.3390/ijms22116085
Chinopoulos, C. (2013). Which way does the citric acid cycle turn during hypoxia? The critical role of alpha-ketoglutarate dehydrogenase complex. J. Neurosci. Res. 91 (8), 1030–1043. doi:10.1002/jnr.23196
Chouchani, E. T., Pell, V. R., Gaude, E., Aksentijevic, D., Sundier, S. Y., Robb, E. L., et al. (2014). Ischaemic accumulation of succinate controls reperfusion injury through mitochondrial ROS. Nature 515 (7527), 431–435. doi:10.1038/nature13909
Choudhary, C., Weinert, B. T., Nishida, Y., Verdin, E., and Mann, M. (2014). The growing landscape of lysine acetylation links metabolism and cell signalling. Nat. Rev. Mol. Cell Biol. 15 (8), 536–550. doi:10.1038/nrm3841
Cortese, M., Sinclair, C., and Pulendran, B. (2014). Translating glycolytic metabolism to innate immunity in dendritic cells. Cell Metab. 19 (5), 737–739. doi:10.1016/j.cmet.2014.04.012
Dalla Pozza, E., Dando, I., Pacchiana, R., Liboi, E., Scupoli, M. T., Donadelli, M., et al. (2020). Regulation of succinate dehydrogenase and role of succinate in cancer. Semin. Cell Dev. Biol. 98, 4–14. doi:10.1016/j.semcdb.2019.04.013
Dambrova, M., Zuurbier, C. J., Borutaite, V., Liepinsh, E., and Makrecka-Kuka, M. (2021). Energy substrate metabolism and mitochondrial oxidative stress in cardiac ischemia/reperfusion injury. Free Radic. Biol. Med. 165, 24–37. doi:10.1016/j.freeradbiomed.2021.01.036
Davidson, S. M., Ferdinandy, P., Andreadou, I., Bøtker, H. E., Heusch, G., Ibáñez, B., et al. (2019). Multitarget strategies to reduce myocardial ischemia/reperfusion injury: JACC review topic of the week. J. Am. Coll. Cardiol. 73 (1), 89–99. doi:10.1016/j.jacc.2018.09.086
de Castro Fonseca, M., Aguiar, C. J., da Rocha Franco, J. A., Gingold, R. N., and Leite, M. F. (2016). GPR91: expanding the frontiers of Krebs cycle intermediates. Cell Commun. Signal 14, 3. doi:10.1186/s12964-016-0126-1
Demetri, G. D., Fletcher, C. D., Mueller, E., Sarraf, P., Naujoks, R., Campbell, N., et al. (1999). Induction of solid tumor differentiation by the peroxisome proliferator-activated receptor-gamma ligand troglitazone in patients with liposarcoma. Proc. Natl. Acad. Sci. U. S. A. 96 (7), 3951–3956. doi:10.1073/pnas.96.7.3951
Dong, L. F., Freeman, R., Liu, J., Zobalova, R., Marin-Hernandez, A., Stantic, M., et al. (2009). Suppression of tumor growth in vivo by the mitocan alpha-tocopheryl succinate requires respiratory complex II. Clin. Cancer Res. 15 (5), 1593–1600. doi:10.1158/1078-0432.Ccr-08-2439
Dong, L. F., Jameson, V. J., Tilly, D., Cerny, J., Mahdavian, E., Marín-Hernández, A., et al. (2011a). Mitochondrial targeting of vitamin E succinate enhances its pro-apoptotic and anti-cancer activity via mitochondrial complex II. J. Biol. Chem. 286 (5), 3717–3728. doi:10.1074/jbc.M110.186643
Dong, L. F., Jameson, V. J., Tilly, D., Prochazka, L., Rohlena, J., Valis, K., et al. (2011b). Mitochondrial targeting of α-tocopheryl succinate enhances its pro-apoptotic efficacy: a new paradigm for effective cancer therapy. Free Radic. Biol. Med. 50 (11), 1546–1555. doi:10.1016/j.freeradbiomed.2011.02.032
Dong, L. F., Low, P., Dyason, J. C., Wang, X. F., Prochazka, L., Witting, P. K., et al. (2008). alpha-tocopheryl succinate induces apoptosis by targeting ubiquinone-binding sites in mitochondrial respiratory complex II. Oncogene 27 (31), 4324–4335. doi:10.1038/onc.2008.69
Drose, S. (2013). Differential effects of complex II on mitochondrial ROS production and their relation to cardioprotective pre- and postconditioning. Biochim. Biophys. Acta 1827 (5), 578–587. doi:10.1016/j.bbabio.2013.01.004
Eltzschig, H. K., and Eckle, T. (2011). Ischemia and reperfusion-from mechanism to translation. Nat. Med. 17 (11), 1391–1401. doi:10.1038/nm.2507
Eniafe, J., and Jiang, S. (2021). The functional roles of TCA cycle metabolites in cancer. Oncogene 40 (19), 3351–3363. doi:10.1038/s41388-020-01639-8
Evans, Z. P., Ellett, J. D., Fariss, M. W., Schnellmann, R. G., Schmidt, M. G., and Chavin, K. (2008). Vitamin E succinate reduces ischemia/reperfusion injury in steatotic livers. Transpl. Proc. 40 (10), 3327–3329. doi:10.1016/j.transproceed.2008.06.076
Fulcher, D. A., and Katelaris, C. H. (1991). Anaphylactoid reaction to intravenous hydrocortisone sodium succinate: a case report and literature review. Med. J. Aust. 154 (3), 210–214. doi:10.5694/j.1326-5377.1991.tb121038.x
Gaude, E., and Frezza, C. (2014). Defects in mitochondrial metabolism and cancer. Cancer Metab. 2, 10. doi:10.1186/2049-3002-2-10
Gilissen, J., Jouret, F., Pirotte, B., and Hanson, J. (2016). Insight into SUCNR1 (GPR91) structure and function. Pharmacol. Ther. 159, 56–65. doi:10.1016/j.pharmthera.2016.01.008
Gill, A. J. (2012). Succinate dehydrogenase (SDH) and mitochondrial driven neoplasia. Pathology 44 (4), 285–292. doi:10.1097/PAT.0b013e3283539932
Godel, M., Ortone, G., Anobile, D. P., Pasino, M., Randazzo, G., Riganti, C., et al. (2021). Targeting mitochondrial oncometabolites: A new approach to overcome drug resistance in cancer. Pharmaceutics 13 (5), 762. doi:10.3390/pharmaceutics13050762
Gomez-Lazaro, M., Galindo, M. F., Melero-Fernandez de Mera, R. M., Fernandez-Gómez, F. J., Concannon, C. G., Segura, M. F., et al. (2007). Reactive oxygen species and p38 mitogen-activated protein kinase activate Bax to induce mitochondrial cytochrome c release and apoptosis in response to malonate. Mol. Pharmacol. 71 (3), 736–743. doi:10.1124/mol.106.030718
Grimolizzi, F., and Arranz, L. (2018). Multiple faces of succinate beyond metabolism in blood. Haematologica 103 (10), 1586–1592. doi:10.3324/haematol.2018.196097
Guo, L., Shestov, A. A., Worth, A. J., Nath, K., Nelson, D. S., Leeper, D. B., et al. (2016). Inhibition of mitochondrial complex II by the anticancer agent lonidamine. J. Biol. Chem. 291 (1), 42–57. doi:10.1074/jbc.M115.697516
Gupta, P. K., Gahtori, R., Govarthanan, K., Sharma, V., Pappuru, S., Pandit, S., et al. (2021). Recent trends in biodegradable polyester nanomaterials for cancer therapy. Mater Sci. Eng. C Mater Biol. Appl. 127, 112198. doi:10.1016/j.msec.2021.112198
Gupta, R. A., Brockman, J. A., Sarraf, P., Willson, T. M., and DuBois, R. N. (2001). Target genes of peroxisome proliferator-activated receptor gamma in colorectal cancer cells. J. Biol. Chem. 276 (32), 29681–29687. doi:10.1074/jbc.M103779200
Gut, P., Matilainen, S., Meyer, J. G., Pällijeff, P., Richard, J., Carroll, C. J., et al. (2020). SUCLA2 mutations cause global protein succinylation contributing to the pathomechanism of a hereditary mitochondrial disease. Nat. Commun. 11 (1), 5927. doi:10.1038/s41467-020-19743-4
Hadrup, S., Donia, M., and Thor Straten, P. (2013). Effector CD4 and CD8 T cells and their role in the tumor microenvironment. Cancer Microenviron. 6 (2), 123–133. doi:10.1007/s12307-012-0127-6
Haffke, M., Fehlmann, D., Rummel, G., Boivineau, J., Duckely, M., Gommermann, N., et al. (2019). Structural basis of species-selective antagonist binding to the succinate receptor. Nature 574 (7779), 581–585. doi:10.1038/s41586-019-1663-8
Hagerhall, C. (1997). Succinate: quinone oxidoreductases. Variations on a conserved theme. Biochim. Biophys. Acta 1320 (2), 107–141. doi:10.1016/s0005-2728(97)00019-4
Hakak, Y., Lehmann-Bruinsma, K., Phillips, S., Le, T., Liaw, C., Connolly, D. T., et al. (2009). The role of the GPR91 ligand succinate in hematopoiesis. J. Leukoc. Biol. 85 (5), 837–843. doi:10.1189/jlb.1008618
He, W., Miao, F. J., Lin, D. C., Schwandner, R. T., Wang, Z., Gao, J., et al. (2004). Citric acid cycle intermediates as ligands for orphan G-protein-coupled receptors. Nature 429 (6988), 188–193. doi:10.1038/nature02488
Her, Y. F., and Maher, L. J. (2015). Succinate dehydrogenase loss in familial paraganglioma: biochemistry, genetics, and epigenetics. Int. J. Endocrinol. 2015, 296167. doi:10.1155/2015/296167
Hirschey, M. D., and Zhao, Y. (2015). Metabolic regulation by lysine malonylation, succinylation, and glutarylation. Mol. Cell Proteomics 14 (9), 2308–2315. doi:10.1074/mcp.R114.046664
Hisatake, J. I., Ikezoe, T., Carey, M., Holden, S., Tomoyasu, S., and Koeffler, H. P. (2000). Down-Regulation of prostate-specific antigen expression by ligands for peroxisome proliferator-activated receptor gamma in human prostate cancer. Cancer Res. 60 (19), 5494–5498.
Horsefield, R., Yankovskaya, V., Sexton, G., Whittingham, W., Shiomi, K., Omura, S., et al. (2006). Structural and computational analysis of the quinone-binding site of complex II (succinate-ubiquinone oxidoreductase): a mechanism of electron transfer and proton conduction during ubiquinone reduction. J. Biol. Chem. 281 (11), 7309–7316. doi:10.1074/jbc.M508173200
Huang, L. S., Sun, G., Cobessi, D., Wang, A. C., Shen, J. T., Tung, E. Y., et al. (2006). 3-nitropropionic acid is a suicide inhibitor of mitochondrial respiration that, upon oxidation by complex II, forms a covalent adduct with a catalytic base arginine in the active site of the enzyme. J. Biol. Chem. 281 (9), 5965–5972. doi:10.1074/jbc.M511270200
Huang, L. Y., Ma, J. Y., Song, J. X., Xu, J. J., Hong, R., Fan, H. D., et al. (2023). Ischemic accumulation of succinate induces Cdc42 succinylation and inhibits neural stem cell proliferation after cerebral ischemia/reperfusion. Neural Regen. Res. 18 (5), 1040–1045. doi:10.4103/1673-5374.355821
Huang, S., and Millar, A. H. (2013). Succinate dehydrogenase: the complex roles of a simple enzyme. Curr. Opin. Plant Biol. 16 (3), 344–349. doi:10.1016/j.pbi.2013.02.007
Hwang, M. S., Rohlena, J., Dong, L. F., Neuzil, J., and Grimm, S. (2014). Powerhouse down: complex II dissociation in the respiratory chain. Mitochondrion 19 Pt A, 20–28. doi:10.1016/j.mito.2014.06.001
Ibrahim, A., and Chopra, S. (2020). Succinate dehydrogenase-deficient gastrointestinal stromal tumors. Arch. Pathol. Lab. Med. 144 (5), 655–660. doi:10.5858/arpa.2018-0370-RS
Jiang, L., Yin, X., Chen, Y. H., Chen, Y., Jiang, W., Zheng, H., et al. (2021). Proteomic analysis reveals ginsenoside Rb1 attenuates myocardial ischemia/reperfusion injury through inhibiting ROS production from mitochondrial complex I. Theranostics 11 (4), 1703–1720. doi:10.7150/thno.43895
Jiang, S., and Yan, W. (2017). Succinate in the cancer-immune cycle. Cancer Lett. 390, 45–47. doi:10.1016/j.canlet.2017.01.019
Jiang, S., and Yan, W. (2016). T-cell immunometabolism against cancer. Cancer Lett. 382 (2), 255–258. doi:10.1016/j.canlet.2016.09.003
Johnson, J. D., Mehus, J. G., Tews, K., Milavetz, B. I., and Lambeth, D. O. (1998). Genetic evidence for the expression of ATP- and GTP-specific succinyl-CoA synthetases in multicellular eucaryotes. J. Biol. Chem. 273 (42), 27580–27586. doi:10.1074/jbc.273.42.27580
Kes, M. M. G., Van den Bossche, J., Griffioen, A. W., and Huijbers, E. J. M. (2020). Oncometabolites lactate and succinate drive pro-angiogenic macrophage response in tumors. Biochim. Biophys. Acta Rev. Cancer 1874 (2), 188427. doi:10.1016/j.bbcan.2020.188427
Kitazawa, S., Ebara, S., Ando, A., Baba, Y., Satomi, Y., Soga, T., et al. (2017). Succinate dehydrogenase B-deficient cancer cells are highly sensitive to bromodomain and extra-terminal inhibitors. Oncotarget 8 (17), 28922–28938. doi:10.18632/oncotarget.15959
Kline, K., Yu, W., and Sanders, B. G. (2004). Vitamin E and breast cancer. J. Nutr. 134 (12), 3458S–3462s. doi:10.1093/jn/134.12.3458S
Kluckova, K., Bezawork-Geleta, A., Rohlena, J., Dong, L., and Neuzil, J. (2013). Mitochondrial complex II, a novel target for anti-cancer agents. Biochim. Biophys. Acta 1827 (5), 552–564. doi:10.1016/j.bbabio.2012.10.015
Ko, Y. H., Smith, B. L., Wang, Y., Pomper, M. G., Rini, D. A., Torbenson, M. S., et al. (2004). Advanced cancers: eradication in all cases using 3-bromopyruvate therapy to deplete ATP. Biochem. Biophys. Res. Commun. 324 (1), 269–275. doi:10.1016/j.bbrc.2004.09.047
Ko, Y. H., Verhoeven, H. A., Lee, M. J., Corbin, D. J., Vogl, T. J., and Pedersen, P. L. (2012). A translational study "case report" on the small molecule "energy blocker" 3-bromopyruvate (3BP) as a potent anticancer agent: from bench side to bedside. J. Bioenerg. Biomembr. 44 (1), 163–170. doi:10.1007/s10863-012-9417-4
Koeffler, H. P. (2003). Peroxisome proliferator-activated receptor gamma and cancers. Clin. Cancer Res. 9 (1), 1–9.
Komlodi, T., and Tretter, L. (2017). Methylene blue stimulates substrate-level phosphorylation catalysed by succinyl-CoA ligase in the citric acid cycle. Neuropharmacology 123, 287–298. doi:10.1016/j.neuropharm.2017.05.009
Kopelovich, L., Fay, J. R., Glazer, R. I., and Crowell, J. A. (2002). Peroxisome proliferator-activated receptor modulators as potential chemopreventive agents. Mol. Cancer Ther. 1 (5), 357–363.
Krzak, G., Willis, C. M., Smith, J. A., Pluchino, S., and Peruzzotti-Jametti, L. (2021). Succinate receptor 1: an emerging regulator of myeloid cell function in inflammation. Trends Immunol. 42 (1), 45–58. doi:10.1016/j.it.2020.11.004
Kula-Alwar, D., Prag, H. A., and Krieg, T. (2019). Targeting succinate metabolism in ischemia/reperfusion injury. Circulation 140 (24), 1968–1970. doi:10.1161/circulationaha.119.042791
Kurmi, K., Hitosugi, S., Wiese, E. K., Boakye-Agyeman, F., Gonsalves, W. I., Lou, Z., et al. (2018). Carnitine palmitoyltransferase 1A has a lysine succinyltransferase activity. Cell Rep. 22 (6), 1365–1373. doi:10.1016/j.celrep.2018.01.030
Li, F., He, X., Ye, D., Lin, Y., Yu, H., Yao, C., et al. (2015). NADP(+)-IDH mutations promote hypersuccinylation that impairs mitochondria respiration and induces apoptosis resistance. Mol. Cell 60 (4), 661–675. doi:10.1016/j.molcel.2015.10.017
Li, J., Liang, N., Long, X., Zhao, J., Yang, J., Du, X., et al. (2019). SDHC-related deficiency of SDH complex activity promotes growth and metastasis of hepatocellular carcinoma via ROS/NFκB signaling. Cancer Lett. 461, 44–55. doi:10.1016/j.canlet.2019.07.001
Li, L., Shi, L., Yang, S., Yan, R., Zhang, D., Yang, J., et al. (2016). SIRT7 is a histone desuccinylase that functionally links to chromatin compaction and genome stability. Nat. Commun. 7, 12235. doi:10.1038/ncomms12235
Liu, L., Lu, Y., Martinez, J., Bi, Y., Lian, G., Wang, T., et al. (2016). Proinflammatory signal suppresses proliferation and shifts macrophage metabolism from Myc-dependent to HIF1α-dependent. Proc. Natl. Acad. Sci. U. S. A. 113 (6), 1564–1569. doi:10.1073/pnas.1518000113
Liu, X. H., Zheng, X. F., and Wang, Y. L. (2009). Inhibitive effect of 3-bromopyruvic acid on human breast cancer MCF-7 cells involves cell cycle arrest and apoptotic induction. Chin. Med. J. Engl. 122 (14), 1681–1685.
Macaulay, I. C., Tijssen, M. R., Thijssen-Timmer, D. C., Gusnanto, A., Steward, M., Burns, P., et al. (2007). Comparative gene expression profiling of in vitro differentiated megakaryocytes and erythroblasts identifies novel activatory and inhibitory platelet membrane proteins. Blood 109 (8), 3260–3269. doi:10.1182/blood-2006-07-036269
Malafa, M. P., Fokum, F. D., Andoh, J., Neitzel, L. T., Bandyopadhyay, S., Zhan, R., et al. (2006). Vitamin E succinate suppresses prostate tumor growth by inducing apoptosis. Int. J. Cancer 118 (10), 2441–2447. doi:10.1002/ijc.21689
Malafa, M. P., Fokum, F. D., Mowlavi, A., Abusief, M., and King, M. (2002). Vitamin E inhibits melanoma growth in mice. Surgery 131 (1), 85–91. doi:10.1067/msy.2002.119191
Mathupala, S. P., Ko, Y. H., and Pedersen, P. L. (2006). Hexokinase II: cancer's double-edged sword acting as both facilitator and gatekeeper of malignancy when bound to mitochondria. Oncogene 25 (34), 4777–4786. doi:10.1038/sj.onc.1209603
Mehta, A., and Shaha, C. (2004). Apoptotic death in leishmania donovani promastigotes in response to respiratory chain inhibition: complex II inhibition results in increased pentamidine cytotoxicity. J. Biol. Chem. 279 (12), 11798–11813. doi:10.1074/jbc.M309341200
Milione, M., Maisonneuve, P., Pellegrinelli, A., Pusceddu, S., Centonze, G., Dominoni, F., et al. (2017). Loss of succinate dehydrogenase subunit B (SDHB) as a prognostic factor in advanced ileal well-differentiated neuroendocrine tumors. Endocrine 57 (3), 512–517. doi:10.1007/s12020-016-1180-6
Mills, E. L., Harmon, C., Jedrychowski, M. P., Xiao, H., Garrity, R., Tran, N. V., et al. (2021). UCP1 governs liver extracellular succinate and inflammatory pathogenesis. Nat. Metab. 3 (5), 604–617. doi:10.1038/s42255-021-00389-5
Mills, E., and O'Neill, L. A. (2014). Succinate: a metabolic signal in inflammation. Trends Cell Biol. 24 (5), 313–320. doi:10.1016/j.tcb.2013.11.008
Misawa, T., Takahama, M., Kozaki, T., Lee, H., Zou, J., Saitoh, T., et al. (2013). Microtubule-driven spatial arrangement of mitochondria promotes activation of the NLRP3 inflammasome. Nat. Immunol. 14 (5), 454–460. doi:10.1038/ni.2550
Miyadera, H., Shiomi, K., Ui, H., Yamaguchi, Y., Masuma, R., Tomoda, H., et al. (2003). Atpenins, potent and specific inhibitors of mitochondrial complex II (succinate-ubiquinone oxidoreductase). Proc. Natl. Acad. Sci. U. S. A. 100 (2), 473–477. doi:10.1073/pnas.0237315100
Moosavi, B., Berry, E. A., Zhu, X. L., Yang, W. C., and Yang, G. F. (2019). The assembly of succinate dehydrogenase: a key enzyme in bioenergetics. Cell Mol. Life Sci. 76 (20), 4023–4042. doi:10.1007/s00018-019-03200-7
Mullen, A. R., and DeBerardinis, R. J. (2012). Genetically-defined metabolic reprogramming in cancer. Trends Endocrinol. Metab. 23 (11), 552–559. doi:10.1016/j.tem.2012.06.009
Murphy, E., and Steenbergen, C. (2008). Mechanisms underlying acute protection from cardiac ischemia-reperfusion injury. Physiol. Rev. 88 (2), 581–609. doi:10.1152/physrev.00024.2007
Murphy, M. P., and Chouchani, E. T. (2022). Why succinate? Physiological regulation by a mitochondrial coenzyme Q sentinel. Nat. Chem. Biol. 18 (5), 461–469. doi:10.1038/s41589-022-01004-8
Murphy, M. P., and O'Neill, L. A. J. (2018). Krebs cycle reimagined: the emerging roles of succinate and itaconate as signal transducers. Cell 174 (4), 780–784. doi:10.1016/j.cell.2018.07.030
Neppala, P., Banerjee, S., Fanta, P. T., Yerba, M., Porras, K. A., Burgoyne, A. M., et al. (2019). Current management of succinate dehydrogenase-deficient gastrointestinal stromal tumors. Cancer Metastasis Rev. 38 (3), 525–535. doi:10.1007/s10555-019-09818-0
Neuzil, J., Wang, X. F., Dong, L. F., Low, P., and Ralph, S. J. (2006). Molecular mechanism of 'mitocan'-induced apoptosis in cancer cells epitomizes the multiple roles of reactive oxygen species and Bcl-2 family proteins. FEBS Lett. 580 (22), 5125–5129. doi:10.1016/j.febslet.2006.05.072
Neuzil, J., Weber, T., Terman, A., Weber, C., and Brunk, U. T. (2001). Vitamin E analogues as inducers of apoptosis: implications for their potential antineoplastic role. Redox Rep. 6 (3), 143–151. doi:10.1179/135100001101536247
Nobumoto, M., Yamada, M., Song, S., Inouye, S., and Nakazawa, A. (1998). Mechanism of mitochondrial import of adenylate kinase isozymes. J. Biochem. 123 (1), 128–135. doi:10.1093/oxfordjournals.jbchem.a021899
O'Neill, L. A., and Pearce, E. J. (2016). Immunometabolism governs dendritic cell and macrophage function. J. Exp. Med. 213 (1), 15–23. doi:10.1084/jem.20151570
Palsson-McDermott, E. M., and O'Neill, L. A. (2013). The Warburg effect then and now: from cancer to inflammatory diseases. Bioessays 35 (11), 965–973. doi:10.1002/bies.201300084
Palucka, K., and Banchereau, J. (2012). Cancer immunotherapy via dendritic cells. Nat. Rev. Cancer 12 (4), 265–277. doi:10.1038/nrc3258
Panayiotou, C., Solaroli, N., and Karlsson, A. (2014). The many isoforms of human adenylate kinases. Int. J. Biochem. Cell Biol. 49, 75–83. doi:10.1016/j.biocel.2014.01.014
Panconesi, R., Widmer, J., Carvalho, M. F., Eden, J., Dondossola, D., Dutkowski, P., et al. (2022). Mitochondria and ischemia reperfusion injury. Curr. Opin. Organ Transpl. 27 (5), 434–445. doi:10.1097/mot.0000000000001015
Park, J., Chen, Y., Tishkoff, D. X., Peng, C., Tan, M. J., Dai, L. Z., et al. (2013). SIRT5-Mediated lysine desuccinylation impacts diverse metabolic pathways. Mol. Cell 50 (6), 919–930. doi:10.1016/j.molcel.2013.06.001
Pasini, B., and Stratakis, C. A. (2009). SDH mutations in tumorigenesis and inherited endocrine tumours: lesson from the phaeochromocytoma-paraganglioma syndromes. J. Intern. Med. 266 (1), 19–42. doi:10.1111/j.1365-2796.2009.02111.x
Peace, C. G., and O'Neill, L. A. (2022). The role of itaconate in host defense and inflammation. J. Clin. Invest. 132 (2), e148548. doi:10.1172/jci148548
Pedersen, P. L. (2012). 3-Bromopyruvate (3BP) a fast acting, promising, powerful, specific, and effective "small molecule" anti-cancer agent taken from labside to bedside: introduction to a special issue. J. Bioenerg. Biomembr. 44 (1), 1–6. doi:10.1007/s10863-012-9425-4
Pell, V. R., Chouchani, E. T., Frezza, C., Murphy, M. P., and Krieg, T. (2016). Succinate metabolism: a new therapeutic target for myocardial reperfusion injury. Cardiovasc Res. 111 (2), 134–141. doi:10.1093/cvr/cvw100
Pereira da Silva, A. P., El-Bacha, T., Kyaw, N., dos Santos, R. S., da-Silva, W. S., Almeida, F. C., et al. (2009). Inhibition of energy-producing pathways of HepG2 cells by 3-bromopyruvate. Biochem. J. 417 (3), 717–726. doi:10.1042/bj20080805
Peruzzotti-Jametti, L., Bernstock, J. D., Vicario, N., Costa, A. S. H., Kwok, C. K., Leonardi, T., et al. (2018). Macrophage-derived extracellular succinate licenses neural stem cells to suppress chronic neuroinflammation. Cell Stem Cell 22 (3), 355–368. doi:10.1016/j.stem.2018.01.020
Peti-Peterdi, J. (2010). High glucose and renin release: the role of succinate and GPR91. Kidney Int. 78 (12), 1214–1217. doi:10.1038/ki.2010.333
Pichla, M., Sroka, J., Pienkowska, N., Piwowarczyk, K., Madeja, Z., Bartosz, G., et al. (2019). Metastatic prostate cancer cells are highly sensitive to 3-bromopyruvic acid. Life Sci. 227, 212–223. doi:10.1016/j.lfs.2019.03.066
Quin, J., Engle, D., Litwiller, A., Peralta, E., Grasch, A., Boley, T., et al. (2005). Vitamin E succinate decreases lung cancer tumor growth in mice. J. Surg. Res. 127 (2), 139–143. doi:10.1016/j.jss.2005.01.014
Quinlan, C. L., Orr, A. L., Perevoshchikova, I. V., Treberg, J. R., Ackrell, B. A., and Brand, M. D. (2012). Mitochondrial complex II can generate reactive oxygen species at high rates in both the forward and reverse reactions. J. Biol. Chem. 287 (32), 27255–27264. doi:10.1074/jbc.M112.374629
Raju, T. N. (1999). The nobel chronicles. 1953: hans Adolf Krebs (1900-81) and fritz albert lipmann (1899-1986). Lancet 353 (9164), 1628. doi:10.1016/s0140-6736(05)75758-5
Ralph, S. J., Moreno-Sánchez, R., Neuzil, J., and Rodríguez-Enríquez, S. (2011). Inhibitors of succinate: quinone reductase/complex II regulate production of mitochondrial reactive oxygen species and protect normal cells from ischemic damage but induce specific cancer cell death. Pharm. Res. 28 (11), 2695–2730. doi:10.1007/s11095-011-0566-7
Ren, W., Xia, Y., Chen, S., Wu, G., Bazer, F. W., Zhou, B., et al. (2019). Glutamine metabolism in macrophages: A novel target for obesity/type 2 diabetes. Adv. Nutr. 10 (2), 321–330. doi:10.1093/advances/nmy084
Ristic, B., Bhutia, Y. D., and Ganapathy, V. (2017). Cell-surface G-protein-coupled receptors for tumor-associated metabolites: A direct link to mitochondrial dysfunction in cancer. Biochimica Biophysica Acta-Reviews Cancer 1868 (1), 246–257. doi:10.1016/j.bbcan.2017.05.003
Robben, J. H., Fenton, R. A., Vargas, S. L., Schweer, H., Peti-Peterdi, J., Deen, P. M., et al. (2009). Localization of the succinate receptor in the distal nephron and its signaling in polarized MDCK cells. Kidney Int. 76 (12), 1258–1267. doi:10.1038/ki.2009.360
Rodrigues-Ferreira, C., da Silva, A. P., and Galina, A. (2012). Effect of the antitumoral alkylating agent 3-bromopyruvate on mitochondrial respiration: role of mitochondrially bound hexokinase. J. Bioenerg. Biomembr. 44 (1), 39–49. doi:10.1007/s10863-012-9413-8
Rubic, T., Lametschwandtner, G., Jost, S., Hinteregger, S., Kund, J., Carballido-Perrig, N., et al. (2008). Triggering the succinate receptor GPR91 on dendritic cells enhances immunity. Nat. Immunol. 9 (11), 1261–1269. doi:10.1038/ni.1657
Sabari, B. R., Zhang, D., Allis, C. D., and Zhao, Y. (2017). Metabolic regulation of gene expression through histone acylations. Nat. Rev. Mol. Cell Biol. 18 (2), 90–101. doi:10.1038/nrm.2016.140
Salimi, A., Roudkenar, M. H., Seydi, E., Sadeghi, L., Mohseni, A., Pirahmadi, N., et al. (2017). Chrysin as an anti-cancer agent exerts selective toxicity by directly inhibiting mitochondrial complex II and V in CLL B-lymphocytes. Cancer Invest. 35 (3), 174–186. doi:10.1080/07357907.2016.1276187
Schug, T. T., Xu, Q., Gao, H., Peres-da-Silva, A., Draper, D. W., Fessler, M. B., et al. (2010). Myeloid deletion of SIRT1 induces inflammatory signaling in response to environmental stress. Mol. Cell Biol. 30 (19), 4712–4721. doi:10.1128/mcb.00657-10
Selak, M. A., Armour, S. M., MacKenzie, E. D., Boulahbel, H., Watson, D. G., Mansfield, K. D., et al. (2005). Succinate links TCA cycle dysfunction to oncogenesis by inhibiting HIF-alpha prolyl hydroxylase. Cancer Cell 7 (1), 77–85. doi:10.1016/j.ccr.2004.11.022
Semeraro, M., Boenzi, S., Carrozzo, R., Diodato, D., Martinelli, D., Olivieri, G., et al. (2018). The urinary organic acids profile in single large-scale mitochondrial DNA deletion disorders. Clin. Chim. Acta 481, 156–160. doi:10.1016/j.cca.2018.03.002
Shen, R., Ruan, H., Lin, S., Liu, B., Song, H., Li, L., et al. (2023). Lysine succinylation, the metabolic bridge between cancer and immunity. Genes Dis. 10 (6), 2470–2478. doi:10.1016/j.gendis.2022.10.028
Silkin, Y., Oyedotun, K. S., and Lemire, B. D. (2007). The role of Sdh4p Tyr-89 in ubiquinone reduction by the Saccharomyces cerevisiae succinate dehydrogenase. Biochim. Biophys. Acta 1767 (2), 143–150. doi:10.1016/j.bbabio.2006.11.017
Soller, M., Dröse, S., Brandt, U., Brüne, B., and von Knethen, A. (2007). Mechanism of thiazolidinedione-dependent cell death in Jurkat T cells. Mol. Pharmacol. 71 (6), 1535–1544. doi:10.1124/mol.107.034371
Song, Y., Wang, J., Cheng, Z., Gao, P., Sun, J., Chen, X., et al. (2017). Quantitative global proteome and lysine succinylome analyses provide insights into metabolic regulation and lymph node metastasis in gastric cancer. Sci. Rep. 7, 42053. doi:10.1038/srep42053
Sreedhar, A., Wiese, E. K., and Hitosugi, T. (2020). Enzymatic and metabolic regulation of lysine succinylation. Genes Dis. 7 (2), 166–171. doi:10.1016/j.gendis.2019.09.011
Stapelberg, M., Gellert, N., Swettenham, E., Tomasetti, M., Witting, P. K., Procopio, A., et al. (2005). Alpha-tocopheryl succinate inhibits malignant mesothelioma by disrupting the fibroblast growth factor autocrine loop: mechanism and the role of oxidative stress. J. Biol. Chem. 280 (27), 25369–25376. doi:10.1074/jbc.M414498200
Sun, F., Huo, X., Zhai, Y. J., Wang, A. J., Xu, J. X., Su, D., et al. (2005). Crystal structure of mitochondrial respiratory membrane protein complex II. Cell 121 (7), 1043–1057. doi:10.1016/j.cell.2005.05.025
Sun, X., Sun, G., Huang, Y., Hao, Y., Tang, X., Zhang, N., et al. (2020). 3-Bromopyruvate regulates the status of glycolysis and BCNU sensitivity in human hepatocellular carcinoma cells. Biochem. Pharmacol. 177, 113988. doi:10.1016/j.bcp.2020.113988
Szeto, S. S. W., Reinke, S. N., Sykes, B. D., and Lemire, B. D. (2007). Ubiquinone-binding site mutations in the Saccharomyces cerevisiae succinate dehydrogenase generate superoxide and lead to the accumulation of succinate. J. Biol. Chem. 282 (37), 27518–27526. doi:10.1074/jbc.M700601200
Takács-Vellai, K., Farkas, Z., Ősz, F., and Stewart, G. W. (2021). Model systems in SDHx-related pheochromocytoma/paraganglioma. Cancer Metastasis Rev. 40 (4), 1177–1201. doi:10.1007/s10555-021-10009-z
Tannahill, G., Curtis, A., Adamik, J., Palsson-McDermott, E., McGettrick, A., Goel, G., et al. (2013). Succinate is an inflammatory signal that induces IL-1β through HIF-1α. HIF-1α 496 (7444), 238–242. doi:10.1038/nature11986
Tannahill, G. M., and O'Neill, L. A. (2011). The emerging role of metabolic regulation in the functioning of Toll-like receptors and the NOD-like receptor Nlrp3. FEBS Lett. 585 (11), 1568–1572. doi:10.1016/j.febslet.2011.05.008
Timmers, L., Pasterkamp, G., de Hoog, V. C., Arslan, F., Appelman, Y., and de Kleijn, D. P. (2012). The innate immune response in reperfused myocardium. Cardiovasc Res. 94 (2), 276–283. doi:10.1093/cvr/cvs018
Tomasetti, M., Gellert, N., Procopio, A., and Neuzil, J. (2004). A vitamin E analogue suppresses malignant mesothelioma in a preclinical model: a future drug against a fatal neoplastic disease? Int. J. Cancer 109 (5), 641–642. doi:10.1002/ijc.20062
Tomitsuka, E., Kita, K., and Esumi, H. (2010). The NADH-fumarate reductase system, a novel mitochondrial energy metabolism, is a new target for anticancer therapy in tumor microenvironments. Ann. N. Y. Acad. Sci. 1201, 44–49. doi:10.1111/j.1749-6632.2010.05620.x
Tretter, L., Patocs, A., and Chinopoulos, C. (2016). Succinate, an intermediate in metabolism, signal transduction, ROS, hypoxia, and tumorigenesis. Biochim. Biophys. Acta 1857 (8), 1086–1101. doi:10.1016/j.bbabio.2016.03.012
Tseng, P. L., Wu, W. H., Hu, T. H., Chen, C. W., Cheng, H. C., Li, C. F., et al. (2018). Decreased succinate dehydrogenase B in human hepatocellular carcinoma accelerates tumor malignancy by inducing the Warburg effect. Sci. Rep. 8 (1), 3081. doi:10.1038/s41598-018-21361-6
Visca, P., Pisa, F., and Imperi, F. (2019). The antimetabolite 3-bromopyruvate selectively inhibits Staphylococcus aureus. Int. J. Antimicrob. Agents 53 (4), 449–455. doi:10.1016/j.ijantimicag.2018.11.008
Vuoristo, K. S., Mars, A. E., Sanders, J. P. M., Eggink, G., and Weusthuis, R. A. (2016). Metabolic engineering of TCA cycle for production of chemicals. Trends Biotechnol. 34 (3), 191–197. doi:10.1016/j.tibtech.2015.11.002
Wagner, G. R., Bhatt, D. P., O'Connell, T. M., Thompson, J. W., Dubois, L. G., Backos, D. S., et al. (2017). A class of reactive acyl-CoA species reveals the non-enzymatic origins of protein acylation. Cell Metab. 25 (4), 823–837. doi:10.1016/j.cmet.2017.03.006
Wallace, D. C. (2012). Mitochondria and cancer. Nat. Rev. Cancer 12 (10), 685–698. doi:10.1038/nrc3365
Wang, C., Wang, Y., and Shen, L. (2021). Mitochondrial proteins in heart failure: the role of deacetylation by SIRT3. Pharmacol. Res. 172, 105802. doi:10.1016/j.phrs.2021.105802
Wang, C., Zhang, C., Li, X., Shen, J., Xu, Y., Shi, H., et al. (2019). CPT1A-mediated succinylation of S100A10 increases human gastric cancer invasion. J. Cell Mol. Med. 23 (1), 293–305. doi:10.1111/jcmm.13920
Wang, L., Zhang, X., Cui, G., Chan, J. Y., Wang, L., Li, C., et al. (2016). A novel agent exerts antitumor activity in breast cancer cells by targeting mitochondrial complex II. Oncotarget 7 (22), 32054–32064. doi:10.18632/oncotarget.8410
Wang, M., and Lin, H. (2021). Understanding the function of mammalian sirtuins and protein lysine acylation. Annu. Rev. Biochem. 90, 245–285. doi:10.1146/annurev-biochem-082520-125411
Wang, X. F., Birringer, M., Dong, L. F., Veprek, P., Low, P., Swettenham, E., et al. (2007). A peptide conjugate of vitamin E succinate targets breast cancer cells with high ErbB2 expression. Cancer Res. 67 (7), 3337–3344. doi:10.1158/0008-5472.Can-06-2480
Weber, T., Lu, M., Andera, L., Lahm, H., Gellert, N., Fariss, M. W., et al. (2002). Vitamin E succinate is a potent novel antineoplastic agent with high selectivity and cooperativity with tumor necrosis factor-related apoptosis-inducing ligand (Apo2 ligand) in vivo. Clin. Cancer Res. 8 (3), 863–869.
Wei, S., Yang, J., Lee, S. L., Kulp, S. K., and Chen, C. S. (2009). PPARgamma-independent antitumor effects of thiazolidinediones. Cancer Lett. 276 (2), 119–124. doi:10.1016/j.canlet.2008.08.008
Wei, Y. H., Ma, X., Zhao, J. C., Wang, X. Q., and Gao, C. Q. (2023). Succinate metabolism and its regulation of host-microbe interactions. Gut Microbes 15 (1), 2190300. doi:10.1080/19490976.2023.2190300
Weinert, B. T., Scholz, C., Wagner, S. A., Iesmantavicius, V., Su, D., Daniel, J. A., et al. (2013). Lysine succinylation is a frequently occurring modification in prokaryotes and eukaryotes and extensively overlaps with acetylation. Cell Rep. 4 (4), 842–851. doi:10.1016/j.celrep.2013.07.024
Williams, N. C., and O'Neill, L. A. J. (2018). A role for the Krebs cycle intermediate citrate in metabolic reprogramming in innate immunity and inflammation. Front. Immunol. 9, 141. doi:10.3389/fimmu.2018.00141
Wittenberger, T., Schaller, H. C., and Hellebrand, S. (2001). An expressed sequence tag (EST) data mining strategy succeeding in the discovery of new G-protein coupled receptors. J. Mol. Biol. 307 (3), 799–813. doi:10.1006/jmbi.2001.4520
Wojtovich, A. P., and Brookes, P. S. (2009). The complex II inhibitor atpenin A5 protects against cardiac ischemia-reperfusion injury via activation of mitochondrial KATP channels. Basic Res. Cardiol. 104 (2), 121–129. doi:10.1007/s00395-009-0001-y
Wu, W., and Zhao, S. (2013). Metabolic changes in cancer: beyond the Warburg effect. Acta Biochim. Biophys. Sin. (Shanghai) 45 (1), 18–26. doi:10.1093/abbs/gms104
Xiangyun, Y., Xiaomin, N., Linping, G., Yunhua, X., Ziming, L., Yongfeng, Y., et al. (2017). Desuccinylation of pyruvate kinase M2 by SIRT5 contributes to antioxidant response and tumor growth. Oncotarget 8 (4), 6984–6993. doi:10.18632/oncotarget.14346
Xie, Z., Dai, J., Dai, L., Tan, M., Cheng, Z., Wu, Y., et al. (2012). Lysine succinylation and lysine malonylation in histones. Mol. Cell Proteomics 11 (5), 100–107. doi:10.1074/mcp.M111.015875
Xu, J., Pan, H., Xie, X., Zhang, J., Wang, Y., and Yang, G. (2018). Inhibiting succinate dehydrogenase by dimethyl malonate alleviates Brain damage in a rat model of cardiac arrest. Neuroscience 393, 24–32. doi:10.1016/j.neuroscience.2018.09.041
Yadav, S., Pandey, S. K., Kumar, A., Kujur, P. K., Singh, R. P., and Singh, S. M. (2017). Antitumor and chemosensitizing action of 3-bromopyruvate: implication of deregulated metabolism. Chem. Biol. Interact. 270, 73–89. doi:10.1016/j.cbi.2017.04.015
Yang, L., Ma, X., He, Y., Yuan, C., Chen, Q., Li, G., et al. (2017). Sirtuin 5: a review of structure, known inhibitors and clues for developing new inhibitors. Sci. China Life Sci. 60 (3), 249–256. doi:10.1007/s11427-016-0060-7
Yang, Y., and Gibson, G. E. (2019). Succinylation links metabolism to protein functions. Neurochem. Res. 44 (10), 2346–2359. doi:10.1007/s11064-019-02780-x
Yankovskaya, V., Horsefield, R., Tornroth, S., Luna-Chavez, C., Miyoshi, H., Leger, C., et al. (2003). Architecture of succinate dehydrogenase and reactive oxygen species generation. Science 299 (5607), 700–704. doi:10.1126/science.1079605
Yellon, D. M., and Hausenloy, D. J. (2007). Myocardial reperfusion injury. N. Engl. J. Med. 357 (11), 1121–1135. doi:10.1056/NEJMra071667
Yong, C., Stewart, G. D., and Frezza, C. (2020). Oncometabolites in renal cancer. Nat. Rev. Nephrol. 16 (3), 156–172. doi:10.1038/s41581-019-0210-z
Yu, H., Zhu, J., Chang, L., Liang, C., Li, X., and Wang, W. (2021). 3-Bromopyruvate decreased kidney fibrosis and fibroblast activation by suppressing aerobic glycolysis in unilateral ureteral obstruction mice model. Life Sci. 272, 119206. doi:10.1016/j.lfs.2021.119206
Yu, J., Wang, S., Li, W., Dai, G., Xu, S., He, Z., et al. (2007). Cloning, expression and protective immunity evaluation of the full-length cDNA encoding succinate dehydrogenase iron-sulfur protein of Schistosoma japonicum. Sci. China C Life Sci. 50 (2), 221–227. doi:10.1007/s11427-007-0035-z
Zhang, W., Liu, Z., and Xu, X. (2021). Navigating immune cell immunometabolism after liver transplantation. Crit. Rev. Oncol. Hematol. 160, 103227. doi:10.1016/j.critrevonc.2021.103227
Keywords: succinate, succinate dehydrogenase (SDH), immune cells, inflammation, ischemia/reperfusion (IR) injury, cancer
Citation: Zhang W and Lang R (2023) Succinate metabolism: a promising therapeutic target for inflammation, ischemia/reperfusion injury and cancer. Front. Cell Dev. Biol. 11:1266973. doi: 10.3389/fcell.2023.1266973
Received: 07 August 2023; Accepted: 15 September 2023;
Published: 22 September 2023.
Edited by:
De-Li Shi, Sorbonne Université, FranceReviewed by:
Thomas Blacker, University College London, United KingdomEric Stephen Goetzman, University of Pittsburgh, United States
Copyright © 2023 Zhang and Lang. This is an open-access article distributed under the terms of the Creative Commons Attribution License (CC BY). The use, distribution or reproduction in other forums is permitted, provided the original author(s) and the copyright owner(s) are credited and that the original publication in this journal is cited, in accordance with accepted academic practice. No use, distribution or reproduction is permitted which does not comply with these terms.
*Correspondence: Ren Lang, ZHJfbGFuZ3JlbkAxMjYuY29t