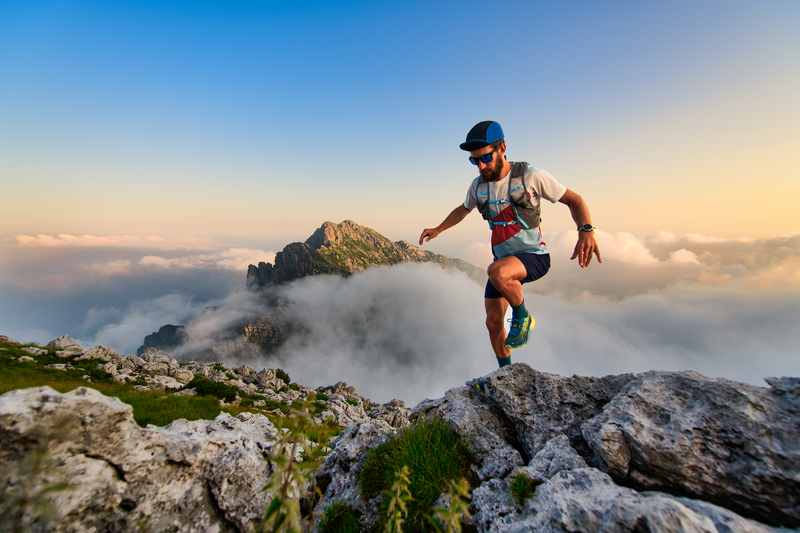
95% of researchers rate our articles as excellent or good
Learn more about the work of our research integrity team to safeguard the quality of each article we publish.
Find out more
ORIGINAL RESEARCH article
Front. Cell Dev. Biol. , 15 January 2024
Sec. Stem Cell Research
Volume 11 - 2023 | https://doi.org/10.3389/fcell.2023.1260019
This article is part of the Research Topic Editors Showcase 2023: Insights in Stem Cell Research View all 8 articles
Hypoxia signaling plays an important role in physiological and pathological conditions. Hypoxia in the heart tissue can produce different consequences depending on the duration of exposure to the hypoxic state. While acute hypoxic exposure leads to a reversible acclimatization in heart tissue with normal systemic oxygen supply, chronic hypoxia exacerbates cardiac dysfunction, leads to a destruction of the tissue. Extracellular vesicles (EVs) are small membrane vesicles that act as mediators of intercellular communication. EVs are secreted by different cell types and those produced by oral cavity-derived mesenchymal stem cells (MSCs), including human gingival MSCs (hGMSCs), have pro-angiogenic and anti-inflammatory effects and showed therapeutic role in tissue regeneration. The aim of the present work was to evaluate the potential protective and regenerative role of EVs produced by hGMSCs, in an in vitro model of hypoxia-conditioned HL-1 cardiomyocytes through the expression analysis of following inflammatory, oxidative stress, angiogenesis, cell survival and apoptotic markers: HIF-1α, P300, NFkB, CCL2, IL1B, IL6, NRF2, CASP-3, BAX and VEGF. Results showed that hGMSCs-derived EVs exerted protection HL-1 cardiomyocytes exposed to both pre and post hypoxic conditions. Moreover, modulation of CASP3 and BAX expression demonstrated that EVs reduced the apoptosis. The analysis of microRNAs in EVs derived from hGMSCs was performed to assess the epigenetic regulation of the presented markers. The following microRNAs: hsa-miR-138-5p, hsa-miR-17-5p, hsa-miR-18a-5p, hsa-miR-21-5p, hsa-miR-324-5p, hsa-miR-133a-3p, hsa-miR-150-5p, hsa-miR-199a-5p, hsa-miR-128-3p and hsa-miR-221-3p can directly or indirectly target the studied genes by determining their modulation obtained in our study. The data from this study suggested that EVs obtained from hGMSCs may be considered for the cell free treatment option in hypoxia-driven cardiac tissue dysfunction.
Molecular oxygen is an indispensable component in mammalian cells. In the condition of normal oxygen, mammalian cell consumes oxygen and nutrients to synthesize adenosine 5′-triphosphate (ATP). It is also involved in various key biochemical reactions in the cells. Therefore, mammalian cells keep oxygen balance to assure their physiological function. Decreased oxygen concentration stimulates a variety of downstream signal responses in the cells. In the presence of hypoxic pressure, mammalian cells will activate a series of downstream pathways, mainly including hypoxia-inducible factor (HIF) (Luo et al., 2022). Hypoxia is a condition in which the oxygen levels are lower than the normoxia’s oxygen level. The central pathway of cell response to a low oxygen environment involves HIF transcription factors, which are responsible for sensing the hypoxic environment in the cells, inducing metabolic changes, regulating cell proliferation, and controlling inflammatory response and other functions. HIF-1α transcription factor-mediated hypoxia signaling plays an important role in both physiological and pathological conditions (Della Rocca et al., 2022). At the same time, HIF signal has also displayed the link with different pathologies, such as cardiovascular, metabolic, inflammatory, and infection-related diseases. Tissue oxygenation is essential for the development of an ideal tissue microenvironment or the reconstitution of an altered microenvironment in the damaged tissue followed by hypoxic injury (Darby and Hewitson, 2016). A lower flow of oxygen caused by hypoxic state can be resulted in reversible or irreversible damage to an organ, depending on whether the hypoxic stimulus is of short duration or longer duration, thereby causing not only cell damage but also cell death with other different consequences in the affected tissue (Sies and Jones, 2020). Peripheral blood circulation is required to maintain physiological function in each tissue. Impaired blood circulation decreases oxygen delivery, leading to tissue hypoxia that occurs in several cardiovascular disorders including atherosclerosis, pulmonary arterial hypertension, and heart failure (Abe et al., 2017). Heart failure, also known as congestive heart failure (CHF), is a chronic and progressive clinical syndrome induced by structural or functional cardiac abnormalities and caused by an impairment of the heart’s blood pumping function (Arrigo et al., 2020). It is well known that oxidative stress plays an important role in the pathophysiology of cardiac remodeling and also in heart failure, since it determines molecular alterations of the intracellular pathways, causing cellular dysfunction (Tsutsui et al., 2011).
Formation of reactive oxygen species (ROS), nitric oxide (NO) and superoxide through NADPH in cardiac tissue may lead to mitochondrial damage, alteration of cardiac contractility and formation of atherosclerosis (Balligand et al., 1992; Babior, 2000). Recent studies indicated that hypoxia may play a key role in the progression of atherosclerotic plaques through lipid accumulation, ATP depletion and angiogenesis, which produce advanced lesions (Hulten and Levin, 2009).
Progression of heart diseases are sustained by the loss of cardiomyocyte. Cardiomyocytes damage can be caused by hypoxia state that induces oxidative stress and apoptosis (Giordano, 2005).
HIF has an impact in several cardiac phenotypes including heart failure (Murry et al., 1986). Following acute hypoxic exposure, the heart tissue responds with a reversible acclimatization due to an immediate increase in heart rate and an increase in lung function that allow maintaining optimal oxygen supply at the systemic level (Lu et al., 2015; Pearson, 2015).
Cardiovascular diseases are one of the main causes of death worldwide. Treatment through regenerative medicine approaches has emerged as a new platform for heart failure (Garbern and Lee, 2022). In particular, the use of extracellular vesicles (EVs) gained a lot of interest in the scientific community (Doyle and Wang, 2019).
EVs are natural nanoparticles containing biologically active molecules like lipids, proteins and different types of nucleic acids, which are enclosed by lipid membrane (Gupta et al., 2021).
EVs have been isolated from most bodily fluids and in the last years it is becoming increasingly clear that they have a central role not only in the regulation of normal physiological events, such as stem cell maintenance, tissue repair, immune surveillance and blood coagulation (Yanez-Mo et al., 2015). Accordingly, they represent important mediators of intercellular communication in both physiological and pathological conditions (Raposo and Stoorvogel, 2013; Wiklander et al., 2019).
EVs are released by almost all cell types which contribute to the correct intercellular communication, mediating the biological effects by transferring proteins, lipids, and nucleic acids (EL Andaloussi et al., 2013).
The most studied molecules included in the EVs content are the microRNAs (miRNAs). miRNAs are small non-coding RNAs which perform post-transcriptional regulation. Since the expression profile of miRNAs has specific characteristics for each physiological or pathological tissue condition, they could serve as pathological biomarkers and have therapeutic action (Liu et al., 2019).
Furthermore, recent data indicate that EVs can also be investigated directly as promising therapeutic agents for tissue repair and immune response modulation. For instance, EVs from mesenchymal stem cells (MSCs) have been utilized to induce tissue regeneration following myocardial infarction (Lai et al., 2011; Timmers et al., 2011).
EVs isolated from oral cavity-derived mesenchymal stem cells (MSCs) have shown to possess pro-angiogenic and anti-inflammatory effects, which contribute for their potential role in regenerative medicine (Diomede et al., 2022; Fonticoli et al., 2022). Indeed, EVs can be used as an alternative therapy for tissue regeneration either in their native form or as vehicles for the delivery of other therapeutic agents since they are able to cross biological barriers while also protecting the contents from degradation (Imafuku and Sjoqvist, 2021).
MSCs are largely described as multipotent non hematopoietic adult stem cells that showed a positive expression for CD73, CD90, and CD105, surface markers, and a negative expression for hematopoietic markers as CD14, CD34, and CD45 (Teixeira et al., 2013; Pagella et al., 2021). They can be isolated from different adult tissues, including oral cavity tissues as dental pulp, periodontal ligament and gingiva. MSCs showed some important features, like differentiation ability, self-renewal potential and immunomodulatory properties. To date the MSCs are considered a promising tool to promote the tissue regeneration in diverse pathological conditions. MSCs are able to migrate to the damaged site, engrafting, and subsequently differentiating into desired cells for tissue regeneration. Latest research demonstrated that the EVs are considered the key mechanism to release the biological factor and regulate the intercellular communication of MSCs action (Keshtkar et al., 2018; Harrell et al., 2019).
De Castro et al. reported that the transplantation of EVs derived from MSCs showed a therapeutic effect as well as the use of MSCs in asthma disease model (de Castro et al., 2017). The use of EVs is considered more advantageous than the transplantation of whole cells. EVs are easy to isolate, store and they showed less side effect in the in vivo study other than they maintain their biological properties (Chiricosta et al., 2020; Silvestro et al., 2020). Thus, the MSCs secrete factors represent a promising therapeutic strategy for use in the regenerative medicine clinical approach (Lai et al., 2015; Wu et al., 2020).
The present work aimed at evaluating the potential protective and regenerative role of EVs produced by human gingival MSCs (hGMSCs) against acute hypoxia in an in vitro model of HL-1 cardiomyocytes through the modulation of HIF-1α, P300, Nuclear Factor kappa B (NFkB), C-C Motif Chemokine Ligand 2 (CCL2), Interleukin 1 beta (IL1B), Interleukin 6 (IL6), nuclear factor erythroid 2–related factor 2 (NRF2), Caspase-3 (CASP3), Bcl-2-associated X protein (BAX) and Vascular Endothelial Growth Factor (VEGF), also from the point of view of the epigenetic modulation. In EVs derived from hGMSCs we have identified the following microRNAs: hsa-miR-138-5p, hsa-miR-17-5p, hsa-miR-18a-5p, hsa-miR-21-5p, hsa-miR-324-5p, hsa-miR-133a-3p, hsa-miR-150-5p, hsa-miR-199a-5p, hsa-miR-128-3p and hsa-miR-221-3p. These miRNAs may target some of the analyzed inflammatory, oxidative stress, angiogenesis, cell survival and apoptotic markers.
HL-1 cells (Sigma-Aldrich, Milan, Italy) were maintained in Claycomb medium completed with 10% fetal bovine serum (FBS, Euroclone, Milan, Italy), 2 mML-glutamine, 0.1 mM norepirephrine, and 100 μg/mL penicillin/streptomycin (Lonza, Basel, Switzerland). The cells were maintained at 37 °C in a humidified atmosphere of 5% of CO2 in air and subcultured until they reached 80% confluence (Marconi et al., 2022). Human gingival mesenchymal stem cells (hGMSCs) were isolated from gingival tissues of three patients, in good general health conditions and without oral disease, who underwent surgical procedure. The gingival tissues were placed in a culture dish, fragmented, washed several times in phosphate-buffered saline solution (PBS, Lonza, Basel, Switzerland) with 5% of gentamicin (Lonza) and transferred in the incubator at 37°C in a humidified atmosphere of 5% CO2 in air with mesenchymal stem cell growth medium-chemically defined (MSCGM-CD, Lonza). The medium was replaced every 2 days for approximately 2 weeks before cells reached 80% confluence and were passaged in culture (Marconi et al., 2021c).
The HL-1 cells were cultured in Claycomb medium com-pleted with 10% fetal bovine serum (Euroclone, Milan, Italy), 2 mM L-glutamine, 0.1 mM norepirephrine, and 100 μg/mL penicillin/streptomycin (Lonza, Basel, Switzerland) under 0.2% hypoxia for 24 h using ProOx Model P110 (BioSpherix, 25 Union Street, Parish, NY 13131) hypoxia chamber as per the referred manual of ProOx Model P110. In CTRL sample, after 24 h of acute hypoxia stimulus, the cells were successively cultured in normoxia for the next 24 h at 37°C in a humidified atmosphere of 5% of CO2 in air. In the sample entitled HL-1 + EVs-hGMSCs UN, the HL-1 cells were maintained under acute hypoxic stimulus at 0.2% for 24 h and then treated with EVs derived from hGMSCs under normoxia for 24 h. In the sample entitled HL-1 + EVs-hGMSCs UH, the HL-1 were first treated with EVs derived from hGMSCs and kept in hypoxia at 0.2% for 24 h and then in normoxia for 24 h.
Human GMSCs were seeded at confluence in 150 mm cell culture dishes. After 48 h of culture, EVs were isolated from the conditioned medium of hGMSCs. For EV extraction, the ExoQuick TC commercial agglutinant (System Biosciences, Euroclone SpA, Milan, Italy) was utilized. Briefly, 2 mL of ExoQuick TC solution was added to 10 mL of conditioned medium. The mix was incubated overnight at 4 °C without rotation; one centrifugation step was executed at 1,500× g for 30 min to sediment the EVs, and the pellets were re-suspended in 200 μL of Phosphate buffered saline (PBS). The EVs used in the current paper were isolated and characterized trough Western blotting as described by Pizzicannella et al. (Pizzicannella et al., 2019), from which data is evident EVs positivity for CD9, CD63 and CD81.
The study design is reported as follows:
CTRL: HL-1 cells were kept in hypoxia at 0.2% for 24 h and successively in normoxia for 24 h.
HL-1 + EVs-hGMSCs UN: HL-1 were kept in hypoxia at 0.2% for 24 h and then treated with hGMSCs EVs under normoxic stimulus for successive 24 h.
HL-1 + EVs-hGMSCs UH: HL-1 treated with hGMSCs EVs were kept in hypoxia at 0.2% for 24 h and then in normoxia for successive 24 h.
The HL-1 cells were seeded at 8,500/well on 8-well culture glass slides (Corning, Glendale, Arizona, United States) under hypoxia state for 24 h and treated with hGMSCs EVs both in EVs post hypoxia and EVs pre hypoxia conditions and processed for immunofluorescence analyses as previously described (Trubiani et al., 2012a). Then, the samples were fixed for 1 h with 4% paraformaldehyde in 0.1 M PBS (pH 7.4) (Lonza, Basel, Switzerland) at room temperature. After washing, cell samples were processed for the immunofluorescence staining: cells were permeabilized with 0.5% Triton X-100 in PBS (Lonza) for 10 min and blocked with 5% skimmed milk in PBS for 1 h. The HL-1 cells were incubated with the primary antibodies for1 h at room temperature using mouse monoclonal antibodies: HIF-1α (1:200) (sc-53546, Santa Cruz Biotechnology, Dallas, TX, United States), NFkB p65 (1:200) (sc-8008, Santa Cruz Biotechnology), p300 (1:200) (sc-32244, Santa Cruz Biotechnology), NRF2 (1:200) (sc-365949, Santa Cruz Biotechnology), VEGF (1:200) (sc-57496, Santa Cruz Biotechnology), and CASP3 (1:200) (sc-56052, Santa Cruz Biotechnology) (Diomede et al., 2019). Then, the HL-1 cells were incubated with secondary antibody Alexa Fluor 568 red fluorescence conjugated goat anti-mouse antibody (1:200; Molecular Probes, Invitrogen, Eugene, OR, United States), for 1 h at 1 h, 37°C; successively, the cells were incubated with Alexa Fluor 488 phalloidin green fluorescent conjugate (1:200; Molecular Probes), TOPRO (1:200; Molecular Probes) (1 h, 37°C). The Zeiss LSM800 confocal system (Carl Zeiss, Jena, Germany) was used to acquire microphotographs. The experiment was conducted in triplicate.
The proteins derived from HL-1 cell cultures of the three experimental points were used at the concentration of 50 μg for the electrophoresis and subsequent transfer on the membrane of polyvinylidene fluoride (PVDF) as previously described (Trubiani et al., 2012b). Successively, they were blocked in 5% of non-fat milk in PBS +0.1% Tween-20. Then, the blotted membranes were incubated overnight at 4°C with the primary antibodies of HIF-1α (1:500) (sc-53546, Santa Cruz Biotechnology), NFkB p65 (1:500) (sc-8008, Santa Cruz Biotechnology), p300 (1:500) (sc-32244, Santa Cruz Biotechnology), NRF2 (1:500) (sc-365949, Santa Cruz Biotechnology), VEGF (1:500) (sc-57496, Santa Cruz Biotechnology), and CASP3 (1:500) (sc-56052, Santa Cruz Biotechnology). GAPDH was used as loading control (1:750) (sc-69879, Santa Cruz Biotechnology). After washes in PBS containing 0.1% Tween-20, membranes were incubated for 1 h at room temperature with peroxidase-conjugated anti-mouse secondary antibody (A90-116 P Goat anti-mouse; Invitrogen; 1:5000 dilution in 2.5% milk made by 1X PBS and 0.1% Tween-20). The enhanced chemiluminescence detection method (ECL) was used to visualize protein expression through UVIband-1D gel analysis (Uvitec, Cambridge, United Kingdom) with photo documenter Alliance 2.7 (Uvitec, Cambridge, United Kingdom). The data obtained were normalized with GAPDH loading control. The experiment was conducted in triplicate.
The inflammatory markers expression IL1B, CCL2, IL6 and apoptotic-related genes BAX and CASP3 were analyzed by Real-Time PCR. Total RNA was extracted using PureLink RNA Mini Kit (Ambion, Thermo Fisher Scientific, Milan, Italy) according to the manufacturer’s instructions. Three independent biological replicates were analyzed for each sample. Two micrograms of total RNA was retrotranscribed using M-MLV Reverse Transcriptase (M1302 Sigma-Aldrich) to synthesize cDNA for 10 min at 70 °C, 50 min at 37 °C and 10 min at 90 °C according to the technical bulletin. Real-Time PCR was performed with Mastercycler ep real plex Real-Time PCR system (Eppendorf, Hamburg, Germany). The levels of mRNA expression of IL1B, CCL2, IL6,BAX, CASP3 and GAPDH (endogenous marker) were evaluated in CTRL, HL-1 + EVs-hGMSCs UN, and HL-1 + EVs-hGMSCs UH conditions. Commercially available PrimeTime™ Predesigned qPCR Assays of IL1B (Hs.PT.58.1518186, Tema Ricerca Srl, Castenaso, Italy); CCL2 (Hs.PT.58.45467977, Tema Ricerca Srl); IL6 (Hs.PT.58.40226675, Tema Ricerca Srl); BAX (Hs.PT.56a.19141193. g, Tema Ricerca Srl); CASP3 (Hs.PT.56a.25882379. g, Tema Ricerca Srl); GAPDH (Hs.PT.39a.22214836, Tema Ricerca Srl, Castenaso, Italy); and the PrimeTime™ Gene Expression Master Mix (cat.n°1055772, Tema Ricerca Srl) were utilized according to the protocol (Marconi, 2021a). Expression levels for each gene were performed according to the 2−ΔΔCT method. Real-Time PCR was performed in three independent experiments.
Total RNA, including microRNAs (miRNAs), was manually isolated from EVs of hGMSCs using the Total Exosome RNA and Protein Isolation Kit (Invitrogen, Thermo Fisher Scientific, Waltham, MA, United States) according to the manufacturer’s instructions. Quantity and quality of total RNA were assessed by microvolume UV–vis spectrophotometer NanoPhotometer (Implen, GmbH, Munich, Germany) (Marconi et al., 2021b).
MicroRNA detection analysis in EVs retrieved from hGMSCs was carried out by TaqMan™ Array Human MicroRNA A Cards v2.0 (Cat. 4398965, Applied Biosystems, Foster City, CA, United States). An initial Megaplex RT Reaction was performed in order to reverse-transcribe miRNAs, using the Megaplex RT primer Pool A and TaqMan MicroRNA Reverse Transcription kit (Applied Biosystems, Waltham, MA). Afterwards, a preamplification reaction of complementary DNAs (cDNAs) was also executed through use of the TaqMan PreAmp Master Mix 2X and Megaplex PreAmp Primers pool A (Applied Biosystems, Waltham, MA). The preamplified products were then diluted with 0.1X TE at pH 8.0 and subsequently mixed with the TaqMan™ universal Master Mix II, no UNG (Applied Biosystems, Waltham, MA) and nuclease-free water. Finally, they were loaded into the TaqMan™ Array Human MicroRNA A plates and processed by qRT- PCR, using a QuantStudioTM 7 Pro Real-Time PCR detection system (Life Technologies, Carlsbad, CA, United States).) Solely a characterization analysis of the small non-coding RNAs was carried out, taking into consideration exclusively amplification cycles (Cts) < 35 of detected miRNAs (Supplementary Figure S3). The TaqMan Array Human MicroRNA A Card v2.0 contains 384 TaqMan MicroRNA Assays of the most highly characterized miRNAs in humans, enabling accurate quantitation of 377 human microRNAs through Real-Time PCR technology. Three TaqMan MicroRNA Assay endogenous controls are included to aid in data normalization and one TaqMan MicroRNA Assay not related to human is included as a negative control.
Characterised miRNAs were analysed by Ingenuity Pathway Analysis (IPA) software in order to highlight the principal functions, cellular processes and molecular networks in which they actively participate. IPA-inferred network analysis was generated for the miRNAs and genes of interest HIF1Α, P300 (also known as EP300), NFkB (NFkB1), NRF2 (also known as NFE2L2), VEGF (VEGFA) and caspase 3 (CASP3)associating their functionality with the function of other small non-coding RNAs and genes, as well as with each other. The mechanistic network of these molecules was evidenced based on their connectivity and enrichment statistics.
One-Way ANOVA test and post hoc Tukey’s multiple comparisons test were performed through GraphPad Prism 5 software to evaluate the statistical differences. The value of p < 0.05 was set as a statistically significant value.
The immunofluorescence showed that the levels of hypoxia markers HIF-1α and P300 were significantly downregulated in EVs-hGMSCs UN and HL-1 + EVs-hGMSCs UH compared to the CTRL sample with greater evidence of HIF-1α decreased in EVs-hGMSCs UN (Figure 1). Moreover, inflammatory marker NFkB was found to be reduced in EVs-hGMSCs UN and HL-1 + EVs-hGMSCs UH compared to the CTRL sample (Figure 1).
FIGURE 1. (A) Immunofluorescence analysis of HIF-1α, p300, NFkB, NRF2, VEGF, and CASP3 expression in CTRL, HL-1 + EVs-hGMSCs UN and HL-1 + EVs-hGMSCs UH. MERGE (red fluorescence: markers; Green fluorescence: cytoskeleton actin; blue fluorescence TOPRO: nuclei. Scale bar: 10 μm. (B) CLSM quantitative analysis of HIF-1α, p300, NFkB, NRF2, VEGF, CASP3 expression calculated as arbitrary unit of fluorescence per cell surface unit ((F/μm2). Data are expressed as mean ± S.E.M. *p < 0.05; **p < 0.01; ***p < 0.001. Statistical analysis was performed by one-way ANOVA and a post hoc Tukey’s multiple comparison analysis.
The real-time evidenced that IL1B was found to be upregulated in CTRL compared to HL-1 + EVs-hGMSCs UN and HL-1 + EVs-hGMSCs UH samples. The gene expression of IL1B in HL-1 + EVs-hGMSCs UN showed a significant higher expression of IL1B compared to HL-1 + EVs-hGMSCs UH (Figure 3).
Moreover, the CCL2 gene expression was significant higher in CTRL compared to EVs-hGMSCs UN and EVs-hGMSCs UH. The CCL2 gene expression was higher in EVs-hGMSCs UH compared to EVs-hGMSCs UN (Figure 3).
In addition, the gene expression of IL6 was also evaluated evidencing a significant upregulation of the IL6 gene expression in CTRL compared to HL-1 + EVs-hGMSCs UN and HL-1 + EVs-hGMSCs UH samples. Additionally, the gene expression of IL6 in HL-1 + EVs-hGMSCs UN showed a significant higher expression of IL6 compared to HL-1 + EVs-hGMSCs UH (Figure 3).
The level of NRF2 immunofluorescence was found higher in HL-1 + EVs-hGMSCs UH compared to HL-1 + EVs-hGMSCs UN and CTRL sample (Figure 1). Similarly, VEGF, an angiogenic factor, was found to be significant overexpressed in HL-1 + EVs-hGMSCs UN compared to the CTRL and HL-1 + EVs-hGMSCs UH (Figure 1). CASP3 level expression showed a significant reduction in both HL-1 + EVs-hGMSCs UN and HL-1 + EVs-hGMSCs UH compared to CTRL (Figure 1).
The data obtained by Western blotting analysis to evaluate the protein expressions of HIF-1α, P300, NFkB, NRF2, VEGF and CASP3 confirmed the results obtained by Confocal Microscopy (Figure 2).
FIGURE 2. HIF-1α, p300, NFkB, NRF2, VEGF and CASP3 protein expression in CTRL, HL-1 + EVs-hGMSCs UN and HL-1 + EVs-hGMSCs analyzed by Western blotting. Each membrane was probed with GAPDH antibody to verify the loading consistency. Western blot data is representative of three different experiments. (A) Western blotting-specific bands of HIF-1α, p300, NFkB, NRF2, VEGF and CASP3 in CTRL, HL-1 + EVs-hGMSCs UN andHL-1 + EVs-hGMSCs UH. [(B)-(B5)] Histograms represent densitometric measurements of proteins bands expressed as the integrated optical intensity (IOI) mean of three separate experiments. The error bars show the standard deviation (±SD). Densitometric values analyzed by ANOVA (post hoc application of Tukey’s multiple comparison test) returned significant differences. *p < 0.05; **p < 0.01; ***p < 0.001.
The CASP3 results obtained by CLSM analysis were confirmed by qPCR analysis. The real-time PCR data for BAX gene expression analysis showed a significant downexpression of this marker in HL-1 + EVs-hGMSCs UN and HL-1 + EVs-hGMSCs UH compared to the CTRL sample. BAX gene expression was found to be significant downregulated in HL-1 + EVs-hGMSCs UN compared to HL-1 + EVs-hGMSCs UH. (Figure 3).
FIGURE 3. (A–E) Histograms of real-time PCR for IL1B, CCL2, IL6, BAX and CASP3 related gene expression in CTRL, HL-1 + EVs-hGMSCs UN and HL-1 + EVs-hGMSCs UH. *p < 0.05; **p < 0.01; ***p < 0.001.
Based on the IPA functional analysis, characterised miRNAs in our cohort of hGMSCs–derived EVs have been found to be involved in processes such as inflammatory response, cellular growth and proliferation, cardiovascular system development and function, as well as cardiovascular disease, cell death and survival and tissue development (Figure 4). A search of existing literature further contributed to select a subgroup of the most experimentally important miRNAs detected, coming up with the following 10 microRNAs, hsa-miR-138-5p (MIMAT0000430, miRBase), hsa-miR-17-5p (MIMAT0000070, miRBase), hsa-miR-18a-5p (MIMAT0000072, miRBase), hsa-miR-21-5p (MIMAT0000076, miRBase), hsa-miR-324-5p (MIMAT0000761, miRBase), hsa-miR-133a-3p (MIMAT0000427, miRBase), hsa-miR-150-5p (MI0000479, miRBase), hsa-miR-199a-5p (MI0000242, miRBase), hsa-miR-128-3p (MIMAT0000424, miRBase) and hsa-miR-221-3p (MIMAT0000278, miRBase). Alongside a further qualitative analysis performed by the IPA software, more light was shed on the networks in which these microRNAs and analysed markers HIF-1α, P300 (EP300), NFkB (NFkB1), NRF2 (NFE2L2), VEGF (VEGFA) and CASP3 are involved, as well as on their reciprocal relationship from a molecular point of view. As a result, it emerged that the reported 10 miRNAs can target directly or indirectly these genes, potentially silencing their expression and indicating the presence of a possible underlying epigenetic mechanism associated with their modulation. Top networks generated by IPA are provided in Figure 4 and are centered around the genes in question.
FIGURE 4. (A) IPA-generated bar chart of the principal biological functions modulated by the characterised microRNAs in hGMSCs–derived EVs. (B) IPA-inferred miRNAs and target genes networks in EVs isolated from hGMSCs. The networks are centered around 6 key node genes, which are known inflammatory, oxidative stress, angiogenesis, cell survival and apoptotic mediators. The central nodes in question are represented in turquoise by the markers (A) HIF1Α, (B) EP300, (C) NFkB1, (D) NFE2L2, (E) VEGFA and (F) CASP3. The 10 miRNAs of greater interest, based on their main biological functions, on the other hand, are provided by hsa-miR-138-5p, hsa-miR-17-5p, hsa-miR-18a-5p, hsa-miR-21-5p, hsa-miR-324-5p, hsa-miR-133a-3p, hsa-miR-150-5p, hsa-miR-199a-5p, hsa-miR-128-3p and hsa-miR-221-3p and are shown in yellow. In purple, HIF1Α is indicated, having been found present in some of the other networks centered around the remaining markers.
The heart needs oxygen to maintain effective contractility. Hypoxia inducible factor-1α (HIF-1α) controls oxygen supply, regulating angiogenesis, vascular remodeling and oxygen utilization, by controlling glucose metabolism and redox homeostasis. Previous work reported that in an in vivo study HIF-1, a transcription factor that functions as a master regulator of oxygen homeostasis, plays a fundamental role in the pathophysiology of ischemic heart disease and heart failure (Burgueno et al., 2013).
The present work analyzed the effect of the EVs produced by hGMSCs in cardiac patients under acute hypoxia stimulus. In detail, the HL-1 cells were maintained under acute hypoxic stimulus and then treated with EVs derived by hGMSCs under normoxia (HL-1 + EVs-hGMSCs UN) or the cells were treated with EVs derived by hGMSCs and kept in hypoxia and then in normoxia (HL-1 + EVs-hGMSCs UH). HIF-1α, stabilized under hypoxic conditions (Salceda and Caro, 1997), decreased with EVs treatment both in HL-1 + EVs-hGMSCs UH and HL-1 + EVs-hGMSCs UN suggesting a protective effect of EVs against hypoxia. The potential protective role of EVs derived by hGMSCs was further confirmed by the results obtained of p300 expression) which is a coactivator of HIF-α (Yu et al., 2020). Moreover, hypoxia-triggered oxidants activate the expression of NRF2 transcription factor that protects the cells against damage by controlling numerous downstream genes (Kensler et al., 2007). Interestingly, our data evidenced that EVs derived by hGMSCs increased NRF2 protein expression especially in HL-1 treated with EVs-hGMSCs UH, demonstrating their protective role against oxidative stress. The key transcription factor involved in the inflammation process is NFkB (Nennig and Schank, 2017). The chemokine CCL2 and the cytokines IL1B and IL6 are other proteins involved in inflammation (Ashton et al., 2013). The hypoxia can upregulate CCL2 in a HIF-1-dependent manner, since CCL2 in the promoter region has a HIF-1 binding motif (Stowe et al., 2012). Tissue injury is characterized by an upregulation in the gene expression of cytokines IL-6, IL-8, and chemokines CCL2, CXCL1, CXCL2. IL-6 induces the chemokines CCL2 and IL-6 secretion is determinated by many other inflammatory mediators including IL-1β (Wang et al., 2009). Our data showed NFkB, CCL2, IL1B and IL6 downexpression in treated samples UN and UH, that might indicate the potential anti-inflammatory properties of EVs derived by hGMSCs. In addition, our evidences reported an increase expression of VEGF, a key regulator of physiological angiogenesis, when the cells where treated with EVs exposed to acute hypoxic stimulus, which can promote the angiogenic process via its pro-angiogenic activity (Melincovici et al., 2018). Taken together these results may indicate a potential regenerative role of EVs derived by hGMSCs against acute hypoxia.
In parallel, cell apoptosis process was also investigated through the analysis of CASP3 and BAX expression.
The human caspase family is distributed into three principal groups, largely based on sequence likeness and biological properties. Group one includes the inflammatory such as caspases-1, -4, and -5 based on the commonalities of having a long caspase-recruitment domain and a preference for a large aromatic or hydrophobic residue at the P4 position. The Group two included the apoptotic effector such as caspases-3, -6, and -7 that share a similar short pro-domain, and are named ‘executors of apoptosis’. Among them, CASP3 is a frequently activated death protease, catalyzing the specific cleavage of many key cellular proteins (Lamkanfi et al., 2007). The B cell lymphoma 2 (Bcl-2) family plays a key role in the intrinsic apoptotic pathway triggered by mitochondrial dysfunction in the Bcl-2/Bax/Cleaved CASP3 signaling pathway regulating cell apoptosis and survival processes; CASP3 activation plays a key role to induce the apoptosis following genotoxic stress (Choudhary et al., 2015; Zhang et al., 2019). BAX gene is a member of the Bcl-2 gene family that has a pro-apoptotic effect in the intrinsic apoptosis regulation, inducing the release of cytochrome C from mitochondria and the cell death (Pawlowski and Kraft, 2000). CASP3 and BAX are directly related since BAX/BAK signaling activates the effector caspases caspase-3 and caspase-7 (Vince et al., 2018). In our study, the expression of intrinsic apoptotic markers CASP-3 and BAX followed the same trend in the three conditions analyzed. We found a significant decrease of CASP3 expression in HL-1 + EVs-hGMSCs UN and in HL-1 + EVs-hGMSCs UH in comparison with control sample. In detail, CASP3 was found downregulated in HL-1 + EVs-hGMSCs UN in comparison with HL-1 + EVs-hGMSCs UH condition. BAX qPCR analysis, showed a downregulation in HL-1 + EVs-hGMSCs UN and in HL-1 + EVs-hGMSCs UH conditions in comparison with CTRL. These data suggest a potential regenerative role of EVs derived by hGMSCs, which seem able to reverse the apoptotic events induced by acute hypoxic exposure.
Despite the limitations related to an in vitro study, these findings collectively expanded our knowledge regarding EVs derived by hGMSCs and their potential protective and regenerative role against inflammatory and oxidative stress stimulus induced by acute hypoxia. Our study provides interesting results regarding the role of EVs derived by hGMSCs under acute hypoxic exposure that may affect cardiac tissue as candidates for novel perspectives in regenerative medicine.
Summarily the current work suggested the protective role against inflammatory and oxidative stress stimulus generated by acute hypoxia, modulating HIF-1α, P300, NRF2, NFkB, IL1B, CCL2, IL6, and the regenerative role through the VEGF signaling pathway and through the reversion of the apoptotic stimuli by the modulation of CASP3 and BAX.
Some molecular data discussed which led to the observed biological effects can be also supported from an epigenetic point of view.
Among the miRNAs identified within the EVs derived by hGMSCs used as treatment in our experimental model, some of these were linked with the gene pathways investigated leading to the observed effects on protein expression levels.
The overexpression of miR-138-5 significantly suppresses the expression level of HIF-1α (Bai et al., 2022). Additionally, it has been demonstrated the protective effect of miR-138-5 on cardiovascular damage due to the inhibition of aldosterone synthase (CYP11B2) leading to reversal of cardiac fibrotic remodeling (Kim et al., 2023). This miR-138-5p was detected within EVs-hGMSCs used could support the down-expression of HIF-1α that was detected trough confocal microscopy and Western blotting in HL-1 + EVs-hGMSCs UN and HL-1 + EVs-hGMSCs UH conditions. The same effect is mediated by miR-18a-5p which has been identified as inversely correlated with HIF expression levels (Krutilina et al., 2014). This miRNA is contained in the EVs h-GMSCs and could therefore epigenetically contribute to the HIF-α downregulation demonstrated in the present work. Based on the literature, miR-17-5p plays a cytoprotective role in response to hypoxia (Yu et al., 2020). This miRNA is contained in the EVs derived by hGMSCs, thus could contribute to the obtained protective effect against hypoxia stimulus.
In parallel, in the hypoxia pathway, among the miRNAs within the EVs h-GMSCs was found to be miR-150-5p interacting with p300, which from our data appears to be downregulated in the same manner of HIF-1α, being p300 its coactivator. It is well known that exists an inversely proportional correlation between miR-150-5p and p300, as previously reported by Li Z et al., which demonstrated that the upregulation of miR-150-5p resulted in the decrease of P300 expression levels such as the inhibition of miR-150-5p resulted in overexpression of p300 (Li et al., 2018). We can therefore highlight the target action of miR-150-5p on p300 expression, which may have contributed to the decreased p300 expression shown in the data from HL-1 + EVs-hGMSCs UN and HL-1 + EVs-hGMSCs UH conditions.
Regarding the typical inflammation marker NFkB, we identified a miRNA within EVs-hGMSCs that could be involved in the decreased expression of NFkB in the epigenetic control. miR-324-5p was seen to have a suppressing effect on NFkB activation. Previous researchers demonstrated that transfection with miR-21 mimics inhibited the expression of intra-nuclear NFkB p65 and downregulation of miR-21 significantly increased the activation of NFkB p65 (Zhu et al., 2018).
Therefore, these results demonstrated that miR-21 could block the activation of NFkB which is decreased HL-1 + EVs-hGMSCs UN and HL-1 + EVs-hGMSCs UH conditions. The same function is mediated by miR-324-5p which has been seen to be involved in blocking NFkB activation through CUE domain-containing 2 (CUEDC2) (Chi and Zhou, 2016). miR-324-5p was also found within the EVs analyzed. Our data report an increase expression of VEGF. One of the miRNAs that appears to interact with VEGF expression is miR-133a-3p. Previous works suggests that miR-133a-3p is able to inhibit cardiomyocyte apoptosis, and increases angiogenesis through upregulation of VEGF expression levels (Zhu et al., 2021). miR-133a-3p was found within the EVs-hGMSCs that may be involved in the overexpression of VEGF in the conditions treated with the EVs derived from h-GMSCs.
Another miRNA that interacts with VEGF expression, already explained above in EVs h-GMSCs, is miR-21-5p. Hu H. et al., demonstrated that exosomes derived from bone marrow mesenchymal stem cells promoted angiogenesis in an in vivo ischemic models through upregulation of miR-21-5p (Hu et al., 2022). Since this miRNA is contained in EVs derived from hGMSCs, we may hypnotized that it can act as a promoter of angiogenesis in our in vitro model.
Earlier work demonstrated that in an in vitro model of pneumocytes, exosomes derived from hMSCSs showed antioxidant capacities that attenuated apoptosis. This property was associated with the identification of miR-199a-5p in vesicles, which protect against oxidative stress by activating the NRF2 signaling pathway through the reduction of Caveolin1 (CAV1) gene expression leading to activation of the antioxidant signaling pathway NRF2(Gong et al., 2023). Based on this knowledge, the potential protective role against oxidative stress shown in the present work through the up-expression of NRF2 can be also liked to miR-199a-5p found inside the EVs h-GMSCs.
Then, NRF2 overexpression found in HL-1 + EVs-hGMSCs UN and HL-1 + EVs-hGMSCs UH conditions, may suggest a potential role of miR-199a-5p in the protection of oxidative stress reported in our study.
Within EVs-hGMSCs there are three miRNAs that could play a role in CASP3 downexpression observed in HL-1 + EVs-hGMSCs UN and HL-1 + EVs-hGMSCs UH conditions: miR-128-3p, miR-138-5p, and miR-221-3p. Liu S et al. demonstrated that in an in vivo mouse model of sepsis, induction of miR-128-3p reduced apoptosis and the production of pro-inflammatory factors in lung tissues. They saw that overexpression of miR-128-3p led to a large decrease in CASP3 activity (Liu et al., 2021). In an ischemia model, neuroprotection of astrocytes was observed following treatment with exosomes derived from bone marrow-derived mesenchymal stem cells overexpressing microRNA-138-5p. This has been demonstrated using miR-138-5p inhibitors. The results suggested a lower astrocyte migration rate after treatment with the miR-138-5p inhibitor, as well as treatment with the miR-138-5p mimic resulted in a decrease of CASP3 (Deng et al., 2019). Overexpression of miR-138-5p promotes proliferation and inhibits apoptosis. Sun L et al. demonstrated that overexpression of miR-221-3p using mimics in a cardiomyoblast cell line model and one of human umbilical vein endothelial cells resulted in down-expression of the proapoptotic protein, CASP3 as well as upregulation of miR-221-3p VEGF protein. These data indicated that miR-221-3p inhibits cardiomyocyte apoptosis and promotes angiogenesis (Sun et al., 2020). These information on miRNAs associated with CASP3 downexpression support the assumed involvement of these miRNAs contained in the studied EVs on CASP3 downexpression obtained in our experimental model. The epigenetic data described above lay the foundations for further studies on the potential role of the described miRNAs in the analyzed functions of different physio/pathological conditions affecting cardiomyocytes.
In conclusion, the EVs-hGMSCs may have a double effect: from one side they have shown a protective role under hypoxia due to the decrease of NFkB, IL1B, CCL2, IL6, HIF-1α, p300 and an increase of NFR2; while, from the other side they have shown a regenerative role under normoxia due to the increase of VEGF and the decrease of CASP3 and BAX. As the next stage research study should be directed to provide effective, safe, and powerful novel strategies based on the EVs derived from MSCs in the in vivo scenario.
The raw data supporting the conclusion of this article will be made available by the authors, without undue reservation.
YDR: Conceptualization, Data curation, Investigation, Methodology, Software, Writing–original draft, Writing–review and editing. FD: Data curation, Formal Analysis, Funding acquisition, Methodology, Project administration, Resources, Software, Supervision, Validation, Visualization, Writing–review and editing. FK: Methodology, Validation, Visualization, Writing–review and editing. OT: Funding acquisition, Validation, Writing–review and editing. TSR: Data curation, Visualization, Writing–review and editing. SP: Supervision, Validation, Writing–review and editing. VG: Project administration, Supervision, Writing–review and editing. LS: Writing–review and editing. GDM: Conceptualization, Data curation, Formal Analysis, Funding acquisition, Investigation, Project administration, Resources, Supervision, Validation, Visualization, Writing–original draft, Writing–review and editing. JP: Conceptualization, Data curation, Formal Analysis, Project administration, Resources, Supervision, Validation, Visualization, Writing–review and editing.
The author(s) declare financial support was received for the research, authorship, and/or publication of this article. The APC was funded by GDM, FD, VG, LS, and OT from University “G. D’Annunzio” Chieti-Pescara, Italy. This work has been funded by the European Union—NextGenerationEU under the Italian Ministry of University and Research (MUR) National Innovation Ecosystem grant ECS00000041—VITALITY–CUP: D73C22000840006.
Author TS was employed by company Hoynoza Technologies Private Limited.
The remaining authors declare that the research was conducted in the absence of any commercial or financial relationships that could be construed as a potential conflict of interest.
All claims expressed in this article are solely those of the authors and do not necessarily represent those of their affiliated organizations, or those of the publisher, the editors and the reviewers. Any product that may be evaluated in this article, or claim that may be made by its manufacturer, is not guaranteed or endorsed by the publisher.
The Supplementary Material for this article can be found online at: https://www.frontiersin.org/articles/10.3389/fcell.2023.1260019/full#supplementary-material
Abe, H., Semba, H., and Takeda, N. (2017). The roles of hypoxia signaling in the pathogenesis of cardiovascular diseases. J. Atheroscler. Thrombosis 24, 884–894. doi:10.5551/jat.RV17009
Arrigo, M., Jessup, M., Mullens, W., Reza, N., Shah, A. M., Sliwa, K., et al. (2020). Acute heart failure. Nat. Rev. Dis. Prim. 6, 16. doi:10.1038/s41572-020-0151-7
Ashton, K. J., Tupicoff, A., Williams-Pritchard, G., Kiessling, C. J., See Hoe, L. E., Headrick, J. P., et al. (2013). Unique transcriptional profile of sustained ligand-activated preconditioning in pre- and post-ischemic myocardium. PLoS One 8, e72278. doi:10.1371/journal.pone.0072278
Babior, B. M. (2000). Phagocytes and oxidative stress. Am. J. Med. 109, 33–44. doi:10.1016/s0002-9343(00)00481-2
Bai, B., Liu, Y., Fu, X. M., Qin, H. Y., Li, G. K., Wang, H. C., et al. (2022). Dysregulation of EZH2/miR-138-5p Axis contributes to radiosensitivity in hepatocellular carcinoma cell by downregulating hypoxia-inducible factor 1 alpha (HIF-1α). Oxid. Med. Cell Longev. 2022, 7608712. doi:10.1155/2022/7608712
Balligand, J. L., Kelly, R. A., Michel, T., and Smith, T. W. (1992). Control of cardiac-muscle cell-function by an endogenous nitric-oxide signaling system. Clin. Res. 40, A200. doi:10.1073/pnas.90.1.347
Burgueno, A. L., Gianotti, T. F., Mansilla, N. G., Pirola, C. J., and Sookoian, S. (2013). Cardiovascular disease is associated with high-fat-diet-induced liver damage and up-regulation of the hepatic expression of hypoxia-inducible factor 1α in a rat model. Clin. Sci. 124, 53–63. doi:10.1042/CS20120151
Chi, Y., and Zhou, D. (2016). MicroRNAs in colorectal carcinoma--from pathogenesis to therapy. J. Exp. Clin. Cancer Res. 35, 43. doi:10.1186/s13046-016-0320-4
Chiricosta, L., Silvestro, S., Gugliandolo, A., Marconi, G. D., Pizzicannella, J., Bramanti, P., et al. (2020). Extracellular vesicles of human periodontal ligament stem cells contain MicroRNAs associated to proto-oncogenes: implications in cytokinesis. Front. Genet. 11, 582. doi:10.3389/fgene.2020.00582
Choudhary, G. S., Al-Harbi, S., and Almasan, A. (2015). Caspase-3 activation is a critical determinant of genotoxic stress-induced apoptosis. Methods Mol. Biol. 1219, 1–9. doi:10.1007/978-1-4939-1661-0_1
Darby, I. A., and Hewitson, T. D. (2016). Hypoxia in tissue repair and fibrosis. Cell Tissue Res. 365, 553–562. doi:10.1007/s00441-016-2461-3
De Castro, L. L., Xisto, D. G., Kitoko, J. Z., Cruz, F. F., Olsen, P. C., Redondo, P. a.G., et al. (2017). Human adipose tissue mesenchymal stromal cells and their extracellular vesicles act differentially on lung mechanics and inflammation in experimental allergic asthma. Stem Cell Res. Ther. 8, 151. doi:10.1186/s13287-017-0600-8
Della Rocca, Y., Fonticoli, L., Rajan, T. S., Trubiani, O., Caputi, S., Diomede, F., et al. (2022). Hypoxia: molecular pathophysiological mechanisms in human diseases. J. Physiol. Biochem. 78, 739–752. doi:10.1007/s13105-022-00912-6
Deng, Y., Chen, D., Gao, F., Lv, H., Zhang, G., Sun, X., et al. (2019). Exosomes derived from microRNA-138-5p-overexpressing bone marrow-derived mesenchymal stem cells confer neuroprotection to astrocytes following ischemic stroke via inhibition of LCN2. J. Biol. Eng. 13, 71. doi:10.1186/s13036-019-0193-0
Diomede, F., Fonticoli, L., Marconi, G. D., Della Rocca, Y., Rajan, T. S., Trubiani, O., et al. (2022). Decellularized dental pulp, extracellular vesicles, and 5-azacytidine: a new tool for endodontic regeneration. Biomedicines 10, 403. doi:10.3390/biomedicines10020403
Diomede, F., Marconi, G. D., Guarnieri, S., D'attilio, M., Cavalcanti, M., Mariggio, M. A., et al. (2019). A novel role of ascorbic acid in anti-inflammatory pathway and ROS generation in HEMA treated dental pulp stem cells. Mater. (Basel) 13, 130. doi:10.3390/ma13010130
Doyle, L. M., and Wang, M. Z. (2019). Overview of extracellular vesicles, their origin, composition, purpose, and methods for exosome isolation and analysis. Cells 8, 727. doi:10.3390/cells8070727
El Andaloussi, S., Maeger, I., Breakefield, X. O., and Wood, M. J. A. (2013). Extracellular vesicles: biology and emerging therapeutic opportunities. Nat. Rev. Drug Discov. 12, 348–358. doi:10.1038/nrd3978
Fonticoli, L., Della Rocca, Y., Rajan, T. S., Murmura, G., Trubiani, O., Oliva, S., et al. (2022). A narrative review: gingival stem cells as a limitless reservoir for regenerative medicine. Int. J. Mol. Sci. 23, 4135. doi:10.3390/ijms23084135
Garbern, J. C., and Lee, R. T. (2022). Heart regeneration: 20 years of progress and renewed optimism. Dev. Cell 57, 424–439. doi:10.1016/j.devcel.2022.01.012
Giordano, F. J. (2005). Oxygen, oxidative stress, hypoxia, and heart failure. J. Clin. Invest. 115, 500–508. doi:10.1172/JCI24408
Gong, C., Gu, Z., Zhang, X., Xu, Q., Mao, G., Pei, Z., et al. (2023). HMSCs exosome-derived miR-199a-5p attenuates sulfur mustard-associated oxidative stress via the CAV1/NRF2 signalling pathway. J. Cell Mol. Med. 27, 2165–2182. doi:10.1111/jcmm.17803
Gupta, D., Zickler, A. M., and El Andaloussi, S. (2021). Dosing extracellular vesicles. Adv. Drug Deliv. Rev. 178, 113961. doi:10.1016/j.addr.2021.113961
Harrell, C. R., Sadikot, R., Pascual, J., Fellabaum, C., Jankovic, M. G., Jovicic, N., et al. (2019). Mesenchymal stem cell-based therapy of inflammatory lung diseases: current understanding and future perspectives. Stem Cells Int. 2019, 4236973. doi:10.1155/2019/4236973
Hu, H., Hu, X., Li, L., Fang, Y., Yang, Y., Gu, J., et al. (2022). Exosomes derived from bone marrow mesenchymal stem cells promote angiogenesis in ischemic stroke mice via upregulation of MiR-21-5p. Biomolecules 12, 883. doi:10.3390/biom12070883
Hulten, L. M., and Levin, M. (2009). The role of hypoxia in atherosclerosis. Curr. Opin. Lipidol. 20, 409–414. doi:10.1097/MOL.0b013e3283307be8
Imafuku, A., and Sjoqvist, S. (2021). Extracellular vesicle therapeutics in regenerative medicine. Cell Biol. Transl. Med. 1312, 131–138. doi:10.1007/5584_2020_599
Kensler, T. W., Wakabayashi, N., and Biswal, S. (2007). Cell survival responses to environmental stresses via the Keap1-Nrf2-ARE pathway. Annu. Rev. Pharmacol. Toxicol. 47, 89–116. doi:10.1146/annurev.pharmtox.46.120604.141046
Keshtkar, S., Azarpira, N., and Ghahremani, M. H. (2018). Mesenchymal stem cell-derived extracellular vesicles: novel frontiers in regenerative medicine. Stem Cell Res. Ther. 9, 63. doi:10.1186/s13287-018-0791-7
Kim, I. K., Song, B. W., Lim, S., Kim, S. W., and Lee, S. (2023). The role of epicardial adipose tissue-derived MicroRNAs in the regulation of cardiovascular disease: a narrative review. Biol. (Basel) 12, 498. doi:10.3390/biology12040498
Krutilina, R., Sun, W., Sethuraman, A., Brown, M., Seagroves, T. N., Pfeffer, L. M., et al. (2014). MicroRNA-18a inhibits hypoxia-inducible factor 1α activity and lung metastasis in basal breast cancers. Breast Cancer Res. 16, R78. doi:10.1186/bcr3693
Lai, R. C., Chen, T. S., and Lim, S. K. (2011). Mesenchymal stem cell exosome: a novel stem cell-based therapy for cardiovascular disease. Regen. Med. 6, 481–492. doi:10.2217/rme.11.35
Lai, R. C., Yeo, R. W., and Lim, S. K. (2015). Mesenchymal stem cell exosomes. Semin. Cell Dev. Biol. 40, 82–88. doi:10.1016/j.semcdb.2015.03.001
Lamkanfi, M., Festjens, N., Declercq, W., Vanden Berghe, T., and Vandenabeele, P. (2007). Caspases in cell survival, proliferation and differentiation. Cell Death Differ. 14, 44–55. doi:10.1038/sj.cdd.4402047
Li, Z., Liu, Y., Guo, X., Sun, G., Ma, Q., Dai, Y., et al. (2018). Long noncoding RNA myocardial infarction-associated transcript is associated with the microRNA-150-5p/P300 pathway in cardiac hypertrophy. Int. J. Mol. Med. 42, 1265–1272. doi:10.3892/ijmm.2018.3700
Liu, S., Gao, S., Yang, Z., and Zhang, P. (2021). miR-128-3p reduced acute lung injury induced by sepsis via targeting PEL12. Open Med. (Wars) 16, 1109–1120. doi:10.1515/med-2021-0258
Liu, T., Zhang, Q., Zhang, J., Li, C., Miao, Y. R., Lei, Q., et al. (2019). EVmiRNA: a database of miRNA profiling in extracellular vesicles. Nucleic Acids Res. 47, D89–D93. doi:10.1093/nar/gky985
Lu, L., Liu, M., Sun, R. R., Zheng, Y., and Zhang, P. Y. (2015). Myocardial infarction: symptoms and treatments. Cell Biochem. Biophysics 72, 865–867. doi:10.1007/s12013-015-0553-4
Luo, Z., Tian, M., Yang, G., Tan, Q., Chen, Y., Li, G., et al. (2022). Hypoxia signaling in human health and diseases: implications and prospects for therapeutics. Signal Transduct. Target Ther. 7, 218. doi:10.1038/s41392-022-01080-1
Marconi, G. D., Della Rocca, Y., Fonticoli, L., Guarnieri, S., Carradori, S., Rajan, T. S., et al. (2022). The beneficial effect of carvacrol in HL-1 cardiomyocytes treated with LPS-G: anti-inflammatory pathway investigations. Antioxidants 11, 386. doi:10.3390/antiox11020386
Marconi, G. D., Fonticoli, L., Della Rocca, Y., Rajan, T. S., Piattelli, A., Trubiani, O., et al. (2021a). Human periodontal ligament stem cells response to titanium implant surface: extracellular matrix deposition. Biol. (Basel) 10, 931. doi:10.3390/biology10090931
Marconi, G. D., Fonticoli, L., Guarnieri, S., Cavalcanti, M., Franchi, S., Gatta, V., et al. (2021b). Ascorbic acid: a new player of epigenetic regulation in LPS-gingivalis treated human periodontal ligament stem cells. Oxid. Med. Cell Longev. 2021, 6679708. doi:10.1155/2021/6679708
Marconi, G. D., Fonticoli, L., Rajan, T. S., Lanuti, P., Della Rocca, Y., Pierdomenico, S. D., et al. (2021c). Transforming growth factor-beta1 and human gingival fibroblast-to-myofibroblast differentiation: molecular and morphological modifications. Front. Physiol. 12, 676512. doi:10.3389/fphys.2021.676512
Melincovici, C. S., Bosca, A. B., Susman, S., Marginean, M., Mihu, C., Istrate, M., et al. (2018). Vascular endothelial growth factor (VEGF) - key factor in normal and pathological angiogenesis. Rom. J. Morphol. Embryol. 59, 455–467.
Murry, C. E., Jennings, R. B., and Reimer, K. A. (1986). Preconditioning with ischemia - a delay of lethal cell injury in ischemic myocardium. Circulation 74, 1124–1136. doi:10.1161/01.cir.74.5.1124
Nennig, S. E., and Schank, J. R. (2017). The role of NFkB in drug addiction: beyond inflammation. Alcohol Alcohol 52, 172–179. doi:10.1093/alcalc/agw098
Pagella, P., Cordiale, A., Marconi, G. D., Trubiani, O., Rasponi, M., and Mitsiadis, T. A. (2021). Bioengineered tooth emulation systems for regenerative and pharmacological purposes. Eur. Cell Mater 41, 502–516. doi:10.22203/eCM.v041a32
Pawlowski, J., and Kraft, A. S. (2000). Bax-induced apoptotic cell death. Proc. Natl. Acad. Sci. U. S. A. 97, 529–531. doi:10.1073/pnas.97.2.529
Pearson, J. T. (2015). Cardiac responses to hypoxia and reoxygenation in Drosophila. New insights into evolutionarily conserved gene responses. Focus on "Cardiac responses to hypoxia and reoxygenation in Drosophila. Am. J. Physiology-Regulatory Integr. Comp. Physiology 309, R1344–R1346. doi:10.1152/ajpregu.00419.2015
Pizzicannella, J., Diomede, F., Gugliandolo, A., Chiricosta, L., Bramanti, P., Merciaro, I., et al. (2019). 3D printing PLA/gingival stem cells/EVs upregulate miR-2861 and-210 during osteoangiogenesis commitment. Int. J. Mol. Sci. 20, 3256. doi:10.3390/ijms20133256
Raposo, G., and Stoorvogel, W. (2013). Extracellular vesicles: exosomes, microvesicles, and friends. J. Cell Biol. 200, 373–383. doi:10.1083/jcb.201211138
Salceda, S., and Caro, J. (1997). Hypoxia-inducible factor 1alpha (HIF-1alpha) protein is rapidly degraded by the ubiquitin-proteasome system under normoxic conditions. Its stabilization by hypoxia depends on redox-induced changes. J. Biol. Chem. 272, 22642–22647. doi:10.1074/jbc.272.36.22642
Sies, H., and Jones, D. P. (2020). Reactive oxygen species (ROS) as pleiotropic physiological signalling agents. Nat. Rev. Mol. Cell Biol. 21, 363–383. doi:10.1038/s41580-020-0230-3
Silvestro, S., Chiricosta, L., Gugliandolo, A., Pizzicannella, J., Diomede, F., Bramanti, P., et al. (2020). Extracellular vesicles derived from human gingival mesenchymal stem cells: a transcriptomic analysis. Genes (Basel) 11, 118. doi:10.3390/genes11020118
Stowe, A. M., Wacker, B. K., Cravens, P. D., Perfater, J. L., Li, M. K., Hu, R., et al. (2012). CCL2 upregulation triggers hypoxic preconditioning-induced protection from stroke. J. Neuroinflammation 9, 33. doi:10.1186/1742-2094-9-33
Sun, L., Zhu, W., Zhao, P., Zhang, J., Lu, Y., Zhu, Y., et al. (2020). Down-regulated exosomal MicroRNA-221 - 3p derived from senescent mesenchymal stem cells impairs heart repair. Front. Cell Dev. Biol. 8, 263. doi:10.3389/fcell.2020.00263
Teixeira, F. G., Carvalho, M. M., Sousa, N., and Salgado, A. J. (2013). Mesenchymal stem cells secretome: a new paradigm for central nervous system regeneration? Cell Mol. Life Sci. 70, 3871–3882. doi:10.1007/s00018-013-1290-8
Timmers, L., Lim, S. K., Hoefer, I. E., Arslan, F., Lai, R. C., Van Oorschot, A. a.M., et al. (2011). Human mesenchymal stem cell-conditioned medium improves cardiac function following myocardial infarction. Stem Cell Res. 6, 206–214. doi:10.1016/j.scr.2011.01.001
Trubiani, O., Ballerini, P., Murmura, G., Pizzicannella, J., Giuliani, P., Buccella, S., et al. (2012a). Toll-like receptor 4 expression, interleukin-6,-8 and ccl-20 release, and nf-kb translocation in human periodontal ligament mesenchymal stem cells stimulated with lps-P-gingivalis. Eur. J. Inflamm. 10, 81–89. doi:10.1177/1721727x1201000109
Trubiani, O., Toniato, E., Di Iorio, D., Diomede, F., Merciaro, I., C, D. A., et al. (2012b). Morphological analysis and interleukin release in human gingival fibroblasts seeded on different denture base acrylic resins. Int. J. Immunopathol. Pharmacol. 25, 637–643. doi:10.1177/039463201202500310
Tsutsui, H., Kinugawa, S., and Matsushima, S. (2011). Oxidative stress and heart failure. Am. J. Physiology-Heart Circulatory Physiology 301, H2181–H2190. doi:10.1152/ajpheart.00554.2011
Vince, J. E., De Nardo, D., Gao, W., Vince, A. J., Hall, C., Mcarthur, K., et al. (2018). The mitochondrial apoptotic effectors BAX/BAK activate caspase-3 and -7 to trigger NLRP3 inflammasome and caspase-8 driven IL-1β activation. Cell Rep. 25, 2339–2353. doi:10.1016/j.celrep.2018.10.103
Wang, X. M., Hamza, M., Wu, T. X., and Dionne, R. A. (2009). Upregulation of IL-6, IL-8 and CCL2 gene expression after acute inflammation: correlation to clinical pain. Pain 142, 275–283. doi:10.1016/j.pain.2009.02.001
Wiklander, O. P. B., Brennan, M. A., Lotval, J., Breakefield, X. O., and El Andaloussi, S. (2019). Advances in therapeutic applications of extracellular vesicles. Sci. Transl. Med. 11, eaav8521. doi:10.1126/scitranslmed.aav8521
Wu, X. X., Wang, Y. W., Xiao, Y., Crawford, R., Mao, X. Z., and Prasadam, I. (2020). Extracellular vesicles: potential role in osteoarthritis regenerative medicine. J. Orthop. Transl. 21, 73–80. doi:10.1016/j.jot.2019.10.012
Yanez-Mo, M., Siljander, P. R. M., Andreu, Z., Zavec, A. B., Borras, F. E., Buzas, E. I., et al. (2015). Biological properties of extracellular vesicles and their physiological functions. J. Extracell. Vesicles 4, 27066. doi:10.3402/jev.v4.27066
Yu, X., Ruan, Y., Shen, T., Qiu, Q., Yan, M., Sun, S., et al. (2020a). Dexrazoxane protects cardiomyocyte from doxorubicin-induced apoptosis by modulating miR-17-5p. Biomed. Res. Int. 2020, 5107193. doi:10.1155/2020/5107193
Yu, Z., Liu, Y., Zhu, J., Han, J., Tian, X., Han, W., et al. (2020b). Insights from molecular dynamics simulations and steered molecular dynamics simulations to exploit new trends of the interaction between HIF-1α and p300. J. Biomol. Struct. Dyn. 38, 1–12. doi:10.1080/07391102.2019.1580616
Zhang, Y., Yang, X., Ge, X. H., and Zhang, F. Y. (2019). Puerarin attenuates neurological deficits via Bcl-2/Bax/cleaved caspase-3 and Sirt3/SOD2 apoptotic pathways in subarachnoid hemorrhage mice. Biomed. Pharmacother. 109, 726–733. doi:10.1016/j.biopha.2018.10.161
Zhu, W., Sun, L., Zhao, P., Liu, Y., Zhang, J., Zhang, Y., et al. (2021). Macrophage migration inhibitory factor facilitates the therapeutic efficacy of mesenchymal stem cells derived exosomes in acute myocardial infarction through upregulating miR-133a-3p. J. Nanobiotechnology 19, 61. doi:10.1186/s12951-021-00808-5
Keywords: extracellular vesicles, MicroRNAs, human gingival mesenchymal stem cells, cardiomyocytes, acute hypoxia
Citation: Della Rocca Y, Diomede F, Konstantinidou F, Trubiani O, Soundara Rajan T, Pierdomenico SD, Gatta V, Stuppia L, Marconi GD and Pizzicannella J (2024) Protective effect of oral stem cells extracellular vesicles on cardiomyocytes in hypoxia-reperfusion. Front. Cell Dev. Biol. 11:1260019. doi: 10.3389/fcell.2023.1260019
Received: 17 July 2023; Accepted: 21 December 2023;
Published: 15 January 2024.
Edited by:
Carmen Castro, University of Cádiz, SpainReviewed by:
Fabiola Marino, University of Magna Graecia, ItalyCopyright © 2024 Della Rocca, Diomede, Konstantinidou, Trubiani, Soundara Rajan, Pierdomenico, Gatta, Stuppia, Marconi and Pizzicannella. This is an open-access article distributed under the terms of the Creative Commons Attribution License (CC BY). The use, distribution or reproduction in other forums is permitted, provided the original author(s) and the copyright owner(s) are credited and that the original publication in this journal is cited, in accordance with accepted academic practice. No use, distribution or reproduction is permitted which does not comply with these terms.
*Correspondence: Jacopo Pizzicannella, amFjb3BvLnBpenppY2FubmVsbGFAdW5pY2guaXQ=
†These authors have contributed equally to this work
Disclaimer: All claims expressed in this article are solely those of the authors and do not necessarily represent those of their affiliated organizations, or those of the publisher, the editors and the reviewers. Any product that may be evaluated in this article or claim that may be made by its manufacturer is not guaranteed or endorsed by the publisher.
Research integrity at Frontiers
Learn more about the work of our research integrity team to safeguard the quality of each article we publish.