- 1School of Mechanical Engineering and Automation, Northeastern University, Shenyang, China
- 2Department of Mechanical Engineering, Monash University, Melbourne, VIC, Australia
Endothelial cells, located on the surface of blood vessel walls, are constantly stimulated by mechanical forces from the blood flow. The mechanical forces, i.e., fluid shear stress, induced by the blood flow play a pivotal role in controlling multiple physiological processes at the endothelium and in regulating various pathways that maintain homeostasis and vascular function. In this review, research looking at different blood fluid patterns and fluid shear stress in the circulation system is summarized, together with the interactions between the blood flow and the endothelial cells. This review also highlights the flow profile as a response to the configurational changes of the endothelial glycocalyx, which is less revisited in previous reviews. The role of endothelial glycocalyx in maintaining endothelium health and the strategies for the restoration of damaged endothelial glycocalyx are discussed from the perspective of the fluid shear stress. This review provides a new perspective regarding our understanding of the role that blood flow plays in regulating endothelial functionality.
1 Introduction
The vascular system is one of the earliest recorded systems in human history (Slowey and Nyhan, 2022). Fluid shear stress induced by the blood flow plays a key part in maintaining homeostasis and is intimately related to vascular pathology (Traub and Berk, 1998; Tun et al., 2019). Endothelial cells, the cellular monolayer lining the inner surface of blood vessels, are in direct contact with the flowing blood and their response to hemodynamic forces directly affects the health of blood vessels (Baratchi et al., 2017). The intensive interactions between endothelial cells and fluid shear stress, therefore, are pivotal for controlling inflammatory responses, modulating the expression of adhesion molecules, cytokines and chemokines, and regulating the formation and destruction of thrombi (Chiu and Chien, 2011; Davis et al., 2015). The endothelial glycocalyx (EG), a layer of gel-like glycoproteins on the apical surface of endothelial cells, participates in maintaining the homeostasis of organs and blood vessels by fulfilling a variety of functions, including mechanotransduction, and by varying its configuration. The EG might be structurally and functionally different in distinct organs, thus revealing an unexpected level of structural complexity. Typically, an EG unit is composed of glycosaminoglycan (GAG) chains and core proteins which anchor the GAGs to the cell membrane (Jiang et al., 2021b). The GAGs in the EG include hyaluronic acid, heparan sulfate, chondroitin sulfate and sialic acid. The GAG chains feature a high density of negative charges and the electrostatic interactions between the GAG chains and some charged blood components allow the GAG chains to act as a microvascular barrier for the transport of ions (Jiang et al., 2019b; Jiang et al., 2020), macromolecules (Shi et al., 2021) and cells (Jiang et al., 2018c; Nishida et al., 2023). For example, the electrostatic repulsion causes albumin to move away from the vascular wall and towards the center of the vascular lumen (Suzuki et al., 2022). A previous review has summarized the mechanisms of endothelial permeability for transcellular and paracellular transport from the perspectives of molecular affinity, size and charge characteristics (Komarova and Malik, 2010).
Endothelial cells are exposed to flow of various patterns and hemodynamic forces throughout the vascular system (Chiu and Chien, 2011). As the “antennas” of endothelial cells, EG is an active mechano-sensor to transduce mechanical signals from the blood flow and transmit the signals to the endothelial cells. Such a process is often termed mechanotransduction. The mechanical forces from the blood flow drive the movements of endothelial cells, and the alignment of endothelial cells varies with the blood flow patterns. For example, porcine aortic valve endothelial cells elongate and align parallelly to the flow direction under continuous perfusion with a shear stress of 0.47 dyne/cm2, while they are perpendicular to the flow direction when porcine aortic valve endothelial cells are under stable or aortic pulsation blood flow with a shear stress of 8.9 dyne/cm2 (Lee et al., 2018). Indeed, from the viewpoint of fluid mechanists, the endothelial cell behavior in flow is a quintessential fluid-structure-interaction problem: the flow modifies the alignment of endothelial cell as well as the configuration of EG, and EG, in turn, changes the flow field and thereby the fluid shear stress (Jiang et al., 2018a; Jiang et al., 2018b; Jiang and Ventikos, 2022). Mechanotransduction is, therefore, an interactive process.
The normal expression of endothelial cell genes is essential for the maintenance of vascular homeostasis and integrity, whilst the malfunction of endothelial cells could lead to infectious diseases (such as sepsis), kidney disease, cardiovascular diseases (such as atherosclerosis, diabetes and hypertension) (Kalagara et al., 2018; Machin et al., 2019; Dogné and Flamion, 2020; Xu, 2020; Weinbaum et al., 2021; Mugerli et al., 2022). As endothelial cells are inherently exposed the blood and fluid shear stress, clarifying their sensing mechanisms for shear stress and the relationship between blood flow and homeostasis of the internal environment contributes to the understandings of the physiology and pathology of the vascular system.
In the past decades, researchers have investigated the relationship among endothelial cells, fluid shear stress and vascular physiology and pathology from various aspects, and review papers highlighting the up-to-date research progress have been published. Table 1 summarizes the recent review papers and labels their research of interest. The foci of these review papers are spotlighted by four factors relating to the functionality of endothelial cells, i.e., blood flow pattern, mechanotransduction, flow field modified by EG and vascular pathology if endothelial cells are malfunctioning. Here, the conventional definition of the mechanotransduction is used, i.e., mechanotransduction only refers to the process that EG responds to the flow.
According to Table 1, most review papers focus on the mechanotransduction of endothelial cells and the pathologies of EG-related diseases, whilst only a limited number of papers talk about blood flow patterns and the modified flow field as a response to EG configurational changes. In this review, we will summarize the latest findings about the effects of blood flow patterns on the physiological function expression of endothelial cells, and special focus will be given to the modified flow field by EG configurational changes. The vascular pathology due to the malfunction of EG is also reviewed. This paper will contribute to a comprehensive summarizing, and we hope understanding, of the role of flow in regulating endothelial functionality.
2 Flow patterns, fluid shear stress and endothelial cells
Blood flow can be laminar, transitional or turbulent, depending on the blood flow rate, the properties of blood, the blood vessel diameter, and other local geometric characteristics of the vessel. The characteristics of flow are determined by a dimensionless number called the Reynolds number (Re), which is calculated using Eq 1:
where ρ is the density of the blood,
Hemodynamic stimulation, including the fluid shear stress driven by pulsatile blood flow and the solid circumferential stress driven by pulsatile blood pressure and associated strain, acts simultaneously on endothelial cells and vascular smooth muscle cells, modulating numerous biological activities (Amaya et al., 2015). The fluid shear stress is a frictional force from the blood flow, parallel to the blood vessel walls, and exerted on the endothelium (Chien, 2007; Tarbell et al., 2014). The fluid shear stress is the product of the blood viscosity (μ) and the spatial gradient of blood velocity at the vascular wall (dU/dy), as shown in Eq 2 in simple unidirectional flow:
where F represents the fluid shear stress which is expressed in the unit of force per unit area (i.e., N/m2, Pascal, or dyne/cm2, with 1 N/m2 = 1 Pa = 10 dyne/cm2) (Chatzizisis et al., 2007). The shear stresses on endothelial cells have a wide range over the vasculature: the arterial shear stresses range from approximately 10 dyne/cm2 in the aorta to 50 dyne/cm2 in smaller arteries; the venous circulating fluid shear stress is relatively low, ranging from 1 to 20 dyne/cm2 (Givens and Tzima, 2016). Based on the flow pattern, fluid shear stress can be further categorized as laminar shear stress, oscillatory shear stress and pulsatile shear stress. Laminar shear stress is highly ordered and streamlined, which maintains the vascular homeostasis by activating mechanoreceptors, aligning endothelial cells along the longitudinal axis of blood vessels, and regulating the expression of intracellular signaling pathways, specific genes and proteins (Tresoldi et al., 2015). Oscillatory shear stress originates from the disturbed blood flow in irregular arterial regions, inducing pro-atherogenic phenotypes in endothelial cells and induces the development of plaques. In vitro experiments have confirmed that oscillatory shear stress can participate in cellular endothelial response, triggering hemostatic reactions and vascular remodeling (Storch et al., 2018). Periodic acceleration generated by motion can generate pulsatile shear stress on the vascular endothelium, and pulsatile shear stress could activate the production of a large number of cardiac and cellular protective mediators, such as endothelial nitric oxide, prostacyclin and tissue plasminogen activator (Martínez et al., 2008).
Abnormal shear stress generated by multidirectional or disturbed blood flow will lead to vascular dysfunction, inflammation and injury, thus inducing pathological processes such as atherosclerosis, thrombosis and arterial hypertension (Kwak et al., 2014; Min and Schwartz, 2019). When endothelium is cultured in wells swirled on an orbital shaker, multidirectional shear stresses act on ECs. The areas where multidirectional shear stresses are applied typically have a particularly high prevalence of lesions (Mohamied et al., 2015). At the center of the swirling well, low magnitude multidirectional shear stress induced by the swirling medium dominates; high magnitude and unidirectional flow is generated at the edge (Warboys et al., 2019). Previous studies suggest ECs cultured at the center of the well were influenced by the atherogenic flow and more pro-inflammatory and fewer homeostatic genes were expressed. Higher permeability, apoptosis and senescence together with more frequent endothelial-to-mesenchymal transition were observed on ECs at the center of the well compared to those at the edge (Ghim et al., 2021). The disturbed flow could trigger an increase in endothelial cell proliferation, which is an early symptom of site-specific atherogenesis (Joshi et al., 2020). Conversely, physiologically high laminar shear stresses are atheroprotective (Souilhol et al., 2020; Zeng et al., 2021). For example, in vitro studies verified that when the laminar shear stress is greater than or equal to 12 dyne/cm2 without oscillation, the protein expression level and activity of eNOS (the basic molecule for the synthesis and releases of the helpful vasodilator, antioxidant, and anti-inflammatory mediator—nitric oxide) in cultured endothelial cells and intact blood vessels are upregulated (Jin et al., 2003; Boo et al., 2006). Another study reported an increase in tumor suppressor gene in the bovine aortic endothelial cells after being exposed to laminar shear stresses of 3 dyne/cm2 or higher for 24 h, but when the shear stress was tuned down to 1.5 dyne/cm2 the gene level did not increase (Lin et al., 2000). Sustained laminar shear stress, together with the sparing endothelial cells from apoptosis, is capable of preventing the endothelial cells apoptosis induced by cytokines, oxidative stress, serum starvation, or cytoskeletal disruption. Pulsating and high laminar shear stress can exert adverse effects on the blood-brain barrier. As reported in an in vitro experiment (Garcia-Polite et al., 2017), the permeability of the brain endothelial monolayer increased and the expression of tight junction proteins decreased under pulsating flow and high laminar shear stress.
Flow adaptations can affect endothelial gene expression, and cells grown under flow conditions respond differently to changes in shear stress compared with those that are grown under static conditions (Raaz et al., 2014). For instance, unidirectional flow can affect the morphology of human umbilical vein endothelial cells more significantly than bidirectional flow or static culture. In a previous study, human umbilical vein endothelial cells were cultured in a static medium for 13 days and then subjected to stable shear stress stimulation at a rate of 12 dyne/cm2 for 24 h. After 24 h of shear exposure, human umbilical vein endothelial cells were elongated and their long axis aligned with the flow direction. However, after 24 h of recovery in the static medium, human umbilical vein endothelial cells returned to their original forms (Bai and Wang, 2014). Another study (Inglebert et al., 2020) reported that after long-term exposure to physiological shear stress, a reduction in fluid shear stress could cause endothelial cells to undergo actin cytoskeleton reorganization and phenotypic changes. Indeed, an increasing number of papers have evidenced the different behavior of endothelial cells under varying fluid shear stress stimulations. The recent papers reporting the roles of fluid shear stress in modifying endothelial cell behavior were summarised in Table 2.
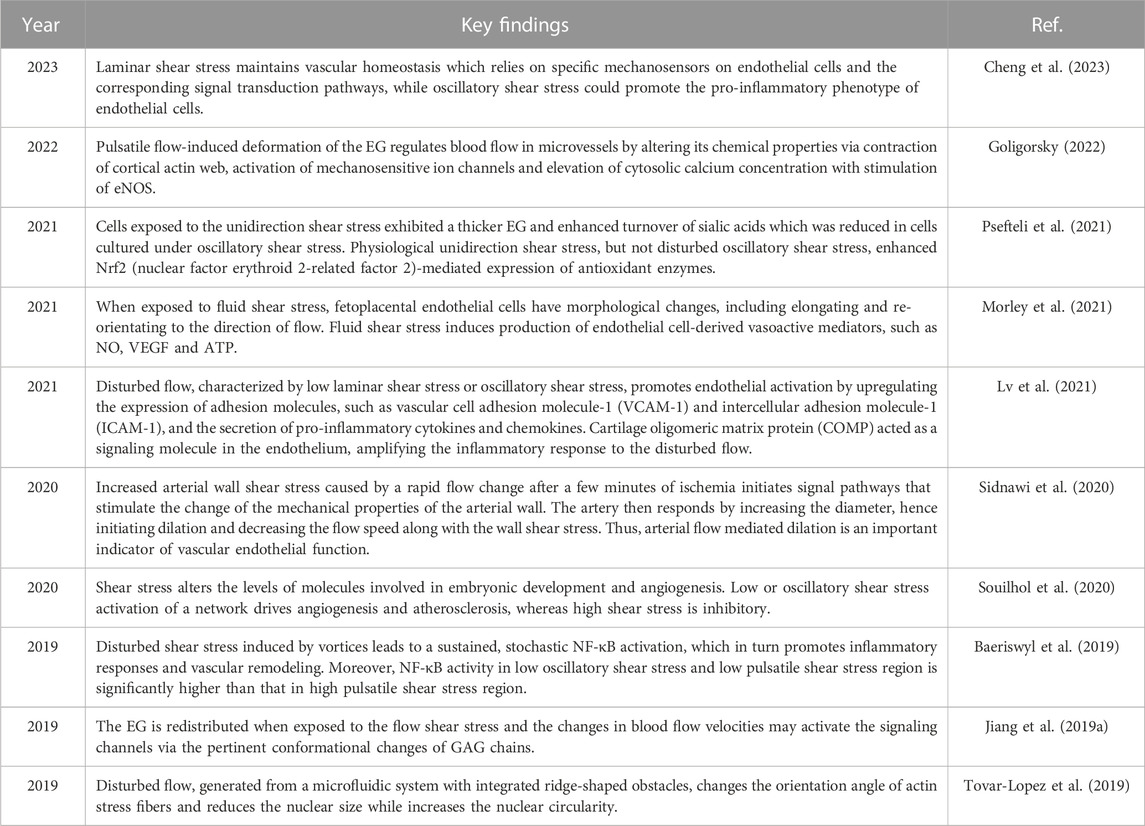
TABLE 2. Recent articles highlighting the function of fluid shear stress in modifying endothelial cell behavior.
The endothelial cells could sense changes upon shear stress and transit between quiescent and activated states (Collins and Tzima, 2011). As shown in Figure 1, mechanosensors first convert physical signals from different flow patterns into biochemical signals and then couple the signals with downstream signaling pathways, thereby inducing the expression of related genes and altering endothelial function and vascular remodeling.
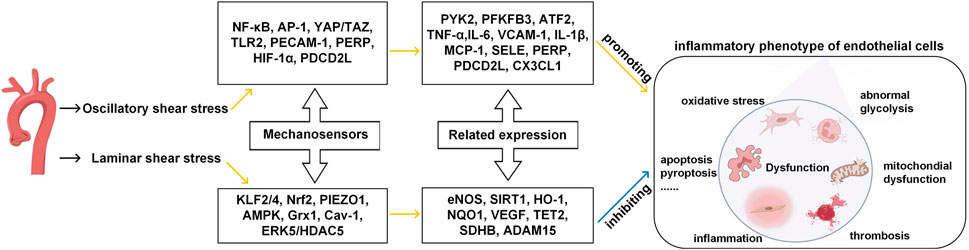
FIGURE 1. Effects of different patterns of shear stress on endothelial cell function in blood vessels. Yellow line indicates promotion, blue line indicates inhibition. (Modified from reference (Cheng et al., 2023) under a Creative Commons Attribution 4.0 International [CC BY 4.0) license].
Some of these mechanosensors can work as signal transduction molecules and transcription factors. For example, NF-κB (nuclear factor-κB), AP-1 (activator protein 1), YAP/TAZ (yes-associated protein/transcriptional co-activator with PDZ-binding motif), HIF-1α (hypoxia-inducible factor 1α), KLF2/4 (krüppel-like factor) and Nrf2 (nuclear factor-E2-related factor 2) can perceive extracellular signals such as oxygen levels and inflammatory cues, as they convert mechanical forces from the blood flow to cytoplasm. Specifically, when ECs are exposed to laminar shear stress, Nrf2 can protect ECs from oxidative stress by upregulating the expression of detoxifying enzymes, antioxidant enzymes, and proteins (Mann and Forman, 2015; Ishii et al., 2021). KLF2 is another important mediator of endothelial anti-inflammatory and anti-thrombotic properties (Boon and Horrevoets, 2009; Marin et al., 2013). In the OSS conditions, ECs can activate transcription factors (like NF-κB (Baeriswyl et al., 2019)), decrease smooth muscle cells contraction marker genes (such as smooth muscle α-actin and myocardin) and induce pro-inflammatory phenotype of the smooth muscle cells (such as the expressions of VCAM-1, IL-6, and MCP-1).
Some mechanosensors, including PIEZO1 (piezo-type mechanosensitive ion channel component 1), AMPK (AMP-activated protein kinase), TLR2 (toll-like receptors 2), Grx1 (glutenin 1), Cav-1 (caveolin-1), ERK5 (extracellular signal-regulated kinase), PECAM-1 (platelet/endothelial cell adhesion molecule-1), are signaling proteins, cell membrane proteins and enzymes which participate in intracellular signal transduction and regulation and maintain the stable structure and well-functioning of ECs. When ECs are exposed to laminar shear stress, the PIEZO1, a receptor ion channel anchored in the EC membrane, rapidly induces the activation of eNOS, thereby producing vasodilator NO through the upregulation and increased activity of eNOS, which has a profound impact on the proliferation and apoptosis of endothelial cells. When ECs are exposed to disturbed pulsatile flow, PECAM-1 senses the flow mechanical forces via the phosphorylation of two tyrosine residues of the cell-cell adhesion sites (Moriguchi and Sumpio, 2015). PECAM-1 can also interact with adhesion molecules such as ICAM-1 and VCAM-1 (Fu et al., 2023), and intensify the attachment of leukocyte to ECs. Molecular structures, signaling mechanisms, and the cellular environment can all influence the sensitivities of the mechanosensors in terms of signal perceiving accuracy and response speed to external stimuli. In the laminar shear stress environment, endothelial cells are anti-inflammatory, anti-oxidant and anti-thrombotic, the glycolysis process is improved, mitochondria are in homeostasis state, and the intracellular permeability is decreased. By contrast, in the oscillatory shear stress environment, endothelial cells are pro-inflammatory, pro-oxidative and pro-thrombotic, the glycolysis is abnormal, mitochondria are in dysfunction state, and the intracellular permeability is increased.
3 Interaction between EG and blood flow
The endothelial glycocalyx on the endothelial cell surface is the most inner layer of the endothelium and is in direct contact with circulating blood. Such a special location enables the EG to modulate blood flow by adjusting roughness via creating a layer of variable porosity and variable thickness. In an early in vivo study, the EG was demonstrated to exert extra resistance on the blood flow (Pries et al., 1997). To reveal the interactions between the EG and the blood flow, a series of large-scale molecular dynamics simulations in vitro were conducted to mimic the behavior of EG and the flow by tracking the trajectories of the atoms in the simulation system (Pikoula et al., 2018; Jiang et al., 2019a). By using such an atomic-scale method, the researchers were able to reproduce the microvascular events occurring on the endothelium, especially the flexible motions of GAG chains in response to the blood flow, which were difficult to observe by experimental methods. Scrutiny of velocity profile of the blood flow along the height of the GAG chains in vitro suggested that the blood velocity begins to drop particularly as the shape of the GAG chains becomes increasingly dendritic (Figure 2A) (Pikoula et al., 2018), and the loss or impairment of GAG chains could alter the surrounding blood flow field and fluid shear stress (Jiang et al., 2017; Jiang et al., 2021a). The alteration in the blood flow velocity profile occurs constantly (Figure 2B) as long as the conformational changes of the GAG chains by the flow happens, as reported in Ref. (Jiang et al., 2017). The passive modulation of the EG to the blood flow resistance maintains the blood homogeneity in the microvasculature. The shedding or loss of local EG could cause blood flow redistribution at the bifurcation (Machin et al., 2019), and the presence of heparinase results in glycocalyx degradation, thereby interfering with angiogenesis and perfusion (Ainslie et al., 2005).
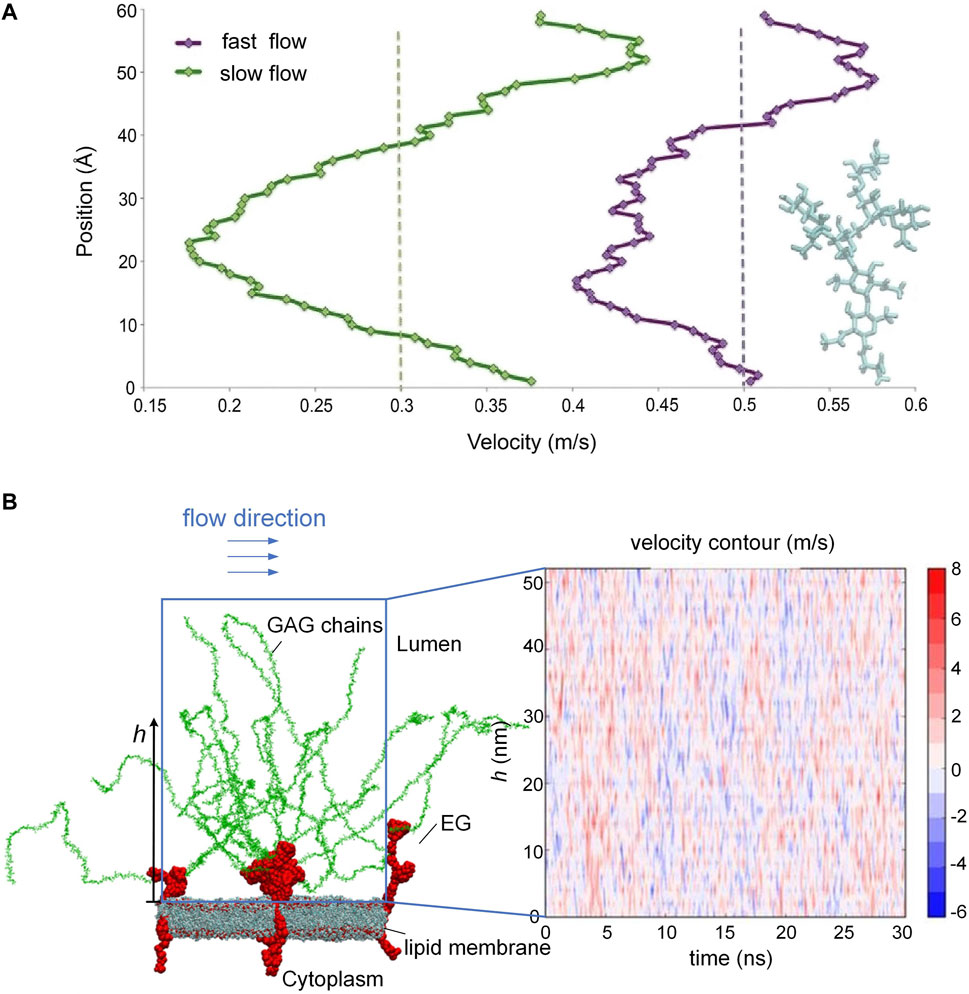
FIGURE 2. Blood flow velocity along the height of the GAG chains and oscillating velocity distributions. (A) Velocity drops as the shape of GAG chains becomes increasingly dendritic. (B) Alteration of blood flow velocity due to the conformational changes of the GAG chains. [Modified from references (Pikoula et al., 2018; Jiang et al, 2017) under a Creative Commons Attribution 4.0 International (CC BY 4.0) license].
The EG is a highly negatively charged brush-like structure with a thickness ranging from a few hundreds of nanometers to several micrometers (Squire et al., 2001). The distribution of endothelium could be modified or reorganized by the fluid shear stress via the EG. For example, Yao et al. (2007) reported that in cultured endothelial cells, heparan sulfate proteoglycans, a major component of the EG, redistribute from a uniform surface profile to a distinct peripheral pattern with most molecules detected above cell-cell junctions after 24-h exposure to the flow. The redistribution of the EG reduces the shear gradients that the cell surface experiences, helping the endothelial cells adapt to the forces from the blood flow. The strength of fluid shear stress also influences the synthesis of GAGs. For example, low laminar shear stress has been repeatedly shown to impair EG and in particular to lead to hyaluronic acid degradation in the EG (Koo et al., 2013). Giantsos-Adams et al. (2013) proved that shear stress enhanced heparan sulfate synthesis compared to static controls after enzymatic removal of heparan sulfate. A study with cultured endothelial cells by Koo et al. (2013) detected increased heparan sulfate expression and even spatial distribution on the apical surface under relatively high shear conditions without shear reversal but reduced heparan sulfate expression and irregular cell distribution under low shear conditions with shear reversal. It is noteworthy that the EG in vitro is thinner than its in vivo counterpart, as cultured endothelial cells are conventionally subjected to dehydration that would lead to glycocalyx collapse (Ebong et al., 2011).
4 Endothelium damage and restoration
The pathogenesis of vascular-related diseases is multifactorial, with most of them related to EG and influenced by integrity and functional expression the EG. EG is a fragile structure that can be damaged by various stimuli, leading to endothelial dysfunction characterized by enhanced vascular permeability, impaired vasodilation, increased leucocyte-endothelium interactions, thrombosis, and vascular inflammation. The existing results indicate that the mechanisms of EG degradation can be divided into two large categories, i.e., intrinsic and extrinsic, as detailed in Figure 3.
The interaction between the endothelial cells and the microenvironment determines the physiological status of cells. The degradation or other type of destruction mode causes the dysfunction or death of the endothelial cells and leads to a series of diseases. Disruption to the EG was observed under various pathological conditions, including infectious diseases (such as sepsis, dengue virus, flaviviruses, human corona viruses and malaria), cardiovascular diseases (such as atherosclerosis, stroke, chronic venous disease, hypertension and aging), diabetes, trauma and cancer (Weinbaum et al., 2021). The commonly used method to determine whether EG is damaged is to detect the concentrations of EG fragments, such as hyaluronic acid, heparan sulfate and syndecan-1, in the plasma. Such fragments are the biomarkers for EG shedding. For instance, in patients with sepsis caused by pneumonia, the concentration of plasma syndecan-1 increases over time, and the syndecan-1 is the only EG biomarker that is moderately correlated with inflammation (Smart et al., 2018). In rats, the circulating levels of syndecan-1 and hyaluronic acid are significantly reduced in the antithrombin-treated group (Iba et al., 2018).
In acute inflammation, the entire EG unit, including the core protein and the GAG chains, is completely destroyed, whereas, in chronic inflammation, the core protein is retained but the associated GAG chains are injured. Restoration of damaged EG and protection against further damage are promising targets for the treatment of chronic vascular diseases (Becker et al., 2010). As such, two therapeutic strategies can be formed to maintain the integrity of the EG: one is to prevent glycocalyx degradation (Zullo et al., 2016), and the other is to promote the glycocalyx biosynthesis (Henry and Duling, 1999; Coccheri and Mannello, 2013). To implement these therapies, agents with special functions can be used. For example, protective agents, such as heparin, heparanase antagonists, antithrombins and corticosteroids, can be used to fight inflammation and thereby prevent glycocalyx shedding (Rubio-Gayosso et al., 2006; Chappell et al., 2009; Brands et al., 2010). However, the clinical efficacy of using agents to prevent EG shedding remains unproven and may even be harmful, as the doses of the anti-inflammatory agents are hard to control and the anti-inflammatory agents may also cause unforeseen problems. Alternatively, as mentioned previously, endothelial cells behave differently under different blood flow patterns, which leaves the possibility to repair or maintain the endothelial cell functionality by applying flows with different flow characteristics. As demonstrated in previous in vitro experiments, when vascular smooth muscle cells and endothelial cells are co-cultured, flows with laminar shear stress can inhibit the dedifferentiation, migration and proliferation of vascular smooth muscle cells while maintaining the contractile phenotype (Hastings et al., 2007; Tsai et al., 2009; Sakamoto et al., 2011).
5 Discussion
Blood flow and the resulting fluid shear stress play a critical role in maintaining endothelial functionality. Endothelial cells covered by the EG are an important medium to convey and convert flow signals. In this review, we summarized the latest findings about the interactions between the blood flow and the endothelium, with special focus given to the modified flow field by EG configurational changes. The shear stress modulates the functionality of endothelial cells, from the activation of mechanosensors to the cellular phenotypes of vascular endothelial cells. To treat chronic vascular diseases, therapies focusing on restoring EG or preventing EG from further damage can be considered. The restoration and prevention could be realized via applying appropriate fluid shear stress to the endothelial cells. Mechanosensors like PECAM-1 and mechanosensitive ion channels, such as PIEZO1, can be targeted to modulate endothelial cell responses to flow (Ridone et al., 2019). Thus, drugs or therapies that could control the activities of these mechanosensors may become novel treatment options. Intervention of flow and shear stress patterns in vivo or in human bodies is no doubt a promising strategy to treat vascular diseases but also a challenging topic deserving significant additional effort.
Author contributions
HLZ: Investigation, Writing–original draft. XZJ: Conceptualization, Funding acquisition, Writing–review and editing. YV: Supervision, Writing–review and editing.
Funding
The author(s) declare financial support was received for the research, authorship, and/or publication of this article. This study was funded by “the Fundamental Research Funds for the Central Universities” (Grant No. N2303017).
Conflict of interest
The authors declare that the research was conducted in the absence of any commercial or financial relationships that could be construed as a potential conflict of interest.
Publisher’s note
All claims expressed in this article are solely those of the authors and do not necessarily represent those of their affiliated organizations, or those of the publisher, the editors and the reviewers. Any product that may be evaluated in this article, or claim that may be made by its manufacturer, is not guaranteed or endorsed by the publisher.
References
Ainslie, K. M., Garanich, J. S., Dull, R. O., and Tarbell, J. M. (2005). Vascular smooth muscle cell glycocalyx influences shear stress-mediated contractile response. J. Appl. Physiol. 98, 242–249. doi:10.1152/japplphysiol.01006.2003
Amaya, R., Pierides, A., and Tarbell, J. M. (2015). The interaction between fluid wall shear stress and solid circumferential strain affects endothelial gene expression. PLoS ONE 10, e0129952. doi:10.1371/journal.pone.0129952
Ashby, J. W., and Mack, J. J. (2021). Endothelial control of cerebral blood flow. Am. J. Pathol. 191, 1906–1916. doi:10.1016/j.ajpath.2021.02.023
Baeriswyl, D. C., Prionisti, I., Peach, T., Tsolkas, G., Chooi, K. Y., Vardakis, J., et al. (2019). Disturbed flow induces a sustained, stochastic NF-κB activation which may support intracranial aneurysm growth in vivo. Sci. Rep. 9, 4738. doi:10.1038/s41598-019-40959-y
Bai, K., and Wang, W. (2014). Shear stress-induced redistribution of the glycocalyx on endothelial cells in vitro. Biomech. Model. Mechanobiol. 13, 303–311. doi:10.1007/s10237-013-0502-3
Baratchi, S., Khoshmanesh, K., Woodman, O. L., Potocnik, S., and Peter, K., (2017). Molecular sensors of blood flow in endothelial cells. Trends Mol. Med. 23, 850–868. doi:10.1016/j.molmed.2017.07.007
Becker, B. F., Chappell, D., and Jacob, M. (2010). Endothelial glycocalyx and coronary vascular permeability: the fringe benefit. Basic Res. Cardiol. 105, 687–701. doi:10.1007/s00395-010-0118-z
Boo, Y. C., Kim, H. J., Song, H., Fulton, D., Sessa, W., and Jo, H. (2006). Coordinated regulation of endothelial nitric oxide synthase activity by phosphorylation and subcellular localization. Free Radic. Biol. Med. 41, 144–153. doi:10.1016/j.freeradbiomed.2006.03.024
Boon, R. A., and Horrevoets, A. J. G. (2009). Key transcriptional regulators of the vasoprotective effects of shear stress. Hamostaseologie 29, 39–43. doi:10.1055/s-0037-1616937
Brands, J., Spaan, J. A., Van Den Berg, B. M., Vink, H., and Vanteeffelen, J. W. (2010). Acute attenuation of glycocalyx barrier properties increases coronary blood volume independently of coronary flow reserve. Am. J. Physiol. Heart Circ. Physiol. 298, H515–H523. doi:10.1152/ajpheart.01306.2008
Chappell, D., Jacob, M., Hofmann-Kiefer, K., Rehm, M., Welsch, U., Conzen, P., et al. (2009). Antithrombin reduces shedding of the endothelial glycocalyx following ischaemia/reperfusion. Cardiovasc. Res. 83, 388–396. doi:10.1093/cvr/cvp097
Chatzizisis, Y. S., Coskun, A. U., Jonas, M., Edelman, E. R., Feldman, C. L., and Stone, P. H. (2007). Role of endothelial shear stress in the natural history of coronary atherosclerosis and vascular remodeling: molecular, cellular, and vascular behavior. J. Am. Coll. Cardiol. 49, 2379–2393. doi:10.1016/j.jacc.2007.02.059
Cheng, H., Zhong, W., Wang, L., Zhang, Q., Ma, X., Wang, Y., et al. (2023). Effects of shear stress on vascular endothelial functions in atherosclerosis and potential therapeutic approaches. Biomed. Pharmacother. 158, 114198. doi:10.1016/j.biopha.2022.114198
Chien, S. (2007). Mechanotransduction and endothelial cell homeostasis: the wisdom of the cell. Am. J. Physiol. Heart Circ. Physiol. 292, H1209–H1224. doi:10.1152/ajpheart.01047.2006
Chiu, J. J., and Chien, S. (2011). Effects of disturbed flow on vascular endothelium: pathophysiological basis and clinical perspectives. Physiol. Rev. 91, 327–387. doi:10.1152/physrev.00047.2009
Coccheri, S., and Mannello, F. (2013). Development and use of sulodexide in vascular diseases: implications for treatment. Drug Des. Devel. Ther. 8, 49–65. doi:10.2147/dddt.S6762
Collins, C., and Tzima, E. (2011). Hemodynamic forces in endothelial dysfunction and vascular aging. Exp. Gerontol. 46, 185–188. doi:10.1016/j.exger.2010.09.010
Cusack, R., Leone, M., Rodriguez, A. H., and Martin-Loeches, I. (2022). Endothelial damage and the microcirculation in critical illness. Biomedicines 10, 3150. doi:10.3390/biomedicines10123150
Davis, C. A., Zambrano, S., Anumolu, P., Allen, A. C., Sonoqui, L., and Moreno, M. R. (2015). Device-based in vitro techniques for mechanical stimulation of vascular cells: a review. J. Biomech. Eng. 137, 040801. doi:10.1115/1.4029016
Dogné, S., and Flamion, B. (2020). Endothelial glycocalyx impairment in disease: focus on hyaluronan shedding. Am. J. Pathol. 190, 768–780. doi:10.1016/j.ajpath.2019.11.016
Dominic, A., Banerjee, P., Hamilton, D. J., Le, N. T., and Abe, J. I. (2020). Time-dependent replicative senescence vs. disturbed flow-induced pre-mature aging in atherosclerosis. Redox Biol. 37, 101614. doi:10.1016/j.redox.2020.101614
Ebong, E. E., Macaluso, F. P., Spray, D. C., and Tarbell, J. M. (2011). Imaging the endothelial glycocalyx in vitro by rapid freezing/freeze substitution transmission electron microscopy. Arterioscler. Thromb. Vasc. Biol. 31, 1908–1915. doi:10.1161/atvbaha.111.225268
Fu, T., Sullivan, D. P., Gonzalez, A. M., Haynes, M. E., Dalal, P. J., Rutledge, N. S., et al. (2023). Mechanotransduction via endothelial adhesion molecule CD31 initiates transmigration and reveals a role for VEGFR2 in diapedesis. Immunity 56, 1–14. doi:10.1016/j.immuni.2023.08.001
Garcia-Polite, F., Martorell, J., Del Rey-Puech, P., Melgar-Lesmes, P., O'brien, C. C., Roquer, J., et al. (2017). Pulsatility and high shear stress deteriorate barrier phenotype in brain microvascular endothelium. J. Cereb. Blood Flow Metab. 37, 2614–2625. doi:10.1177/0271678X16672482
Ghim, M., Pang, K. T., Burnap, S. A., Baig, F., Yin, X., Arshad, M., et al. (2021). Endothelial cells exposed to atheroprotective flow secrete follistatin-like 1 protein which reduces transcytosis and inflammation. Atherosclerosis 333, 56–66. doi:10.1016/j.atherosclerosis.2021.08.025
Giantsos-Adams, K. M., Koo, A. J., Song, S., Sakai, J., Sankaran, J., Shin, J. H., et al. (2013). Heparan sulfate regrowth profiles under laminar shear flow following enzymatic degradation. Cell. Mol. Bioeng. 6, 160–174. doi:10.1007/s12195-013-0273-z
Givens, C., and Tzima, E. (2016). Endothelial mechanosignaling: does one sensor fit all? Antioxid. Redox Signal. 25, 373–388. doi:10.1089/ars.2015.6493
Goligorsky, M. S. (2022). Oscillators in the microvasculature: glycocalyx and beyond. Am. J. Pathol. 323, C432–C438. doi:10.1152/ajpcell.00170.2022
Goligorsky, M. S., and Sun, D. (2020). Glycocalyx in endotoxemia and sepsis. Am. J. Pathol. 190, 791–798. doi:10.1016/j.ajpath.2019.06.017
Hastings, N. E., Simmers, M. B., Mcdonald, O. G., Wamhoff, B. R., and Blackman, B. R. (2007). Atherosclerosis-prone hemodynamics differentially regulates endothelial and smooth muscle cell phenotypes and promotes pro-inflammatory priming. Am. J. Physiol. Cell Physiol. 293, C1824–C1833. doi:10.1152/ajpcell.00385.2007
Henry, C. B., and Duling, B. R. (1999). Permeation of the luminal capillary glycocalyx is determined by hyaluronan. Am. J. Physiol. 277, H508–H514. doi:10.1152/ajpheart.1999.277.2.H508
Iba, T., Levy, J. H., Hirota, T., Hiki, M., Sato, K., Murakami, T., et al. (2018). Protection of the endothelial glycocalyx by antithrombin in an endotoxin-induced rat model of sepsis. Thromb. Res. 171, 1–6. doi:10.1016/j.thromres.2018.09.042
Inglebert, M., Locatelli, L., Tsvirkun, D., Sinha, P., Maier, J. A., Misbah, C., et al. (2020). The effect of shear stress reduction on endothelial cells: a microfluidic study of the actin cytoskeleton. Biomicrofluidics 14, 024115. doi:10.1063/1.5143391
Ishii, T., Warabi, E., and Mann, G. E. (2021). Mechanisms underlying unidirectional laminar shear stress-mediated Nrf2 activation in endothelial cells: amplification of low shear stress signaling by primary cilia. Redox Biol. 46, 102103. doi:10.1016/j.redox.2021.102103
Jiang, X. Z., Feng, M., Luo, K. H., and Ventikos, Y. (2018a). Large-scale molecular dynamics simulation of flow under complex structure of endothelial glycocalyx. Comput. Fluids 173, 140–146. doi:10.1016/j.compfluid.2018.03.014
Jiang, X. Z., Feng, M., Ventikos, Y., and Luo, K. H. (2018b). Regimes of flow over complex structures of endothelial glycocalyx: a molecular dynamics simulation study. Sci. Rep. 8, 5732. doi:10.1038/s41598-018-24041-7
Jiang, X. Z., Goligorsky, M. S., and Luo, K. H. (2021a). Cross talk between endothelial and red blood cell glycocalyces via near-field flow. Biophys. J. 120, 3180–3191. doi:10.1016/j.bpj.2021.06.002
Jiang, X. Z., Gong, H., Luo, K. H., and Ventikos, Y. (2017). Large-scale molecular dynamics simulation of coupled dynamics of flow and glycocalyx: towards understanding atomic events on an endothelial cell surface. J. R. Soc. Interface 14, 20170780. doi:10.1098/rsif.2017.0780
Jiang, X. Z., Lu, Y., Luo, K. H., and Ventikos, Y. (2019a). Understanding endothelial glycocalyx function under flow shear stress from a molecular perspective. Biorheology 56, 89–100. doi:10.3233/bir-180193
Jiang, X. Z., Luo, K. H., and Ventikos, Y. (2018c). Reducing salt intake and exercising regularly: implications from molecular dynamics simulations of endothelial glycocalyx. Front. Physiol. 9, 1667. doi:10.3389/fphys.2018.01667
Jiang, X. Z., Luo, K. H., and Ventikos, Y. (2021b). Understanding the role of endothelial glycocalyx in mechanotransduction via computational simulation: a mini review. Front. Cell Dev. Biol. 9, 732815. doi:10.3389/fcell.2021.732815
Jiang, X. Z., Ventikos, Y., and Luo, K. H. (2019b). Microvascular ion transport through endothelial glycocalyx layer: new mechanism and improved Starling principle. Am. J. Physiol. Heart Circ. Physiol. 317, H104–H113. doi:10.1152/ajpheart.00794.2018
Jiang, X. Z., and Ventikos, Y. (2022). Molecular dynamics simulation: a new way to understand the functionality of the endothelial glycocalyx. Curr. Opin. Struct. Biol. 73, 102330. doi:10.1016/j.sbi.2022.102330
Jiang, X. Z., Yang, L., Ventikos, Y., and Luo, K. H. (2020). Sodium ion transport across the endothelial glycocalyx layer under electric field conditions: a molecular dynamics study. J. Chem. Phys. 153, 105102. doi:10.1063/5.0014177
Jin, Z. G., Ueba, H., Tanimoto, T., Lungu, A. O., Frame, M. D., and Berk, B. C. (2003). Ligand-independent activation of vascular endothelial growth factor receptor 2 by fluid shear stress regulates activation of endothelial nitric oxide synthase. Circ. Res. 93, 354–363. doi:10.1161/01.RES.0000089257.94002.96
Joshi, S., Jan, K. M., and Rumschitzki, D. (2020). Pre-atherosclerotic flow and oncotically active solute transport across the arterial endothelium. J. Theor. Biol. 499, 110275. doi:10.1016/j.jtbi.2020.110275
Kalagara, T., Moutsis, T., Yang, Y., Pappelbaum, K. I., Farken, A., Cladder-Micus, L., et al. (2018). The endothelial glycocalyx anchors von Willebrand factor fibers to the vascular endothelium. Blood Adv. 2, 2347–2357. doi:10.1182/bloodadvances.2017013995
Komarova, Y., and Malik, A. B. (2010). Regulation of endothelial permeability via paracellular and transcellular transport pathways. Annu. Rev. Physiol. 72, 463–493. doi:10.1146/annurev-physiol-021909-135833
Koo, A., Dewey, C. F., and García-Cardeña, G. (2013). Hemodynamic shear stress characteristic of atherosclerosis-resistant regions promotes glycocalyx formation in cultured endothelial cells. Am. J. Physiol. Cell Physiol. 304, C137–C146. doi:10.1152/ajpcell.00187.2012
Ku, D. N. (1997). Blood flow in arteries. Annu. Rev. Fluid Mech. 29, 399–434. doi:10.1146/annurev.fluid.29.1.399
Kwak, B. R., Bäck, M., Bochaton-Piallat, M. L., Caligiuri, G., Daemen, M. J., Davies, P. F., et al. (2014). Biomechanical factors in atherosclerosis: mechanisms and clinical implications. Eur. Heart J. 35, 3013–3020. doi:10.1093/eurheartj/ehu353
Lee, J., Estlack, Z., Somaweera, H., Wang, X., Lacerda, C. M. R., and Kim, J. (2018). A microfluidic cardiac flow profile generator for studying the effect of shear stress on valvular endothelial cells. Lab Chip 18, 2946–2954. doi:10.1039/c8lc00545a
Lin, K., Hsu, P.-P., Chen, B. P., Yuan, S., Usami, S., Shyy, J. Y.-J., et al. (2000). Molecular mechanism of endothelial growth arrest by laminar shear stress. Proc. Natl. Acad. Sci. U. S. A. 97, 9385–9389. doi:10.1073/pnas.170282597
Lv, H., Wang, H., Quan, M., Zhang, C., Fu, Y., Zhang, L., et al. (2021). Cartilage oligomeric matrix protein fine-tunes disturbed flow-induced endothelial activation and atherogenesis. Matrix Biol. 95, 32–51. doi:10.1016/j.matbio.2020.10.003
Machin, D. R., Phuong, T. T., and Donato, A. J. (2019). The role of the endothelial glycocalyx in advanced age and cardiovascular disease. Curr. Opin. Pharmacol. 45, 66–71. doi:10.1016/j.coph.2019.04.011
Mann, G. E., and Forman, H. J. (2015). Introduction to special issue on 'Nrf2 regulated redox signaling and metabolism in physiology and medicine. Free Radic. Biol. Med. 88, 91–92. doi:10.1016/j.freeradbiomed.2015.08.002
Marin, T., Gongol, B., Chen, Z., Woo, B., Subramaniam, S., Chien, S., et al. (2013). Mechanosensitive microRNAs-role in endothelial responses to shear stress and redox state. Free Radic. Biol. Med. 64, 61–68. doi:10.1016/j.freeradbiomed.2013.05.034
Martínez, A., Arias, J., Bassuk, J. A., Wu, H., Kurlansky, P., and Adams, J. A. (2008). Adrenomedullin is increased by pulsatile shear stress on the vascular endothelium via periodic acceleration (pGz). Peptides 29, 73–78. doi:10.1016/j.peptides.2007.10.021
Meng, F., Cheng, H., Qian, J., Dai, X., Huang, Y., and Fan, Y. (2022). In vitro fluidic systems: applying shear stress on endothelial cells. Med. Nov. Technol. Devices 15, 100143. doi:10.1016/j.medntd.2022.100143
Min, E., and Schwartz, M. A. (2019). Translocating transcription factors in fluid shear stress-mediated vascular remodeling and disease. Exp. Cell. Res. 376, 92–97. doi:10.1016/j.yexcr.2019.01.005
Mohamied, Y., Rowland, E. M., Bailey, E. L., Sherwin, S. J., Schwartz, M. A., and Weinberg, P. D. (2015). Change of direction in the biomechanics of atherosclerosis. Ann. Biomed. Eng. 43, 16–25. doi:10.1007/s10439-014-1095-4
Moriguchi, T., and Sumpio, B. E. (2015). PECAM-1 phosphorylation and tissue factor expression in HUVECs exposed to uniform and disturbed pulsatile flow and chemical stimuli. J. Vasc. Surg. 61, 481–488. doi:10.1016/j.jvs.2013.09.059
Morley, L. C., Debant, M., Walker, J. J., Beech, D. J., and Simpson, N. A. B. (2021). Placental blood flow sensing and regulation in fetal growth restriction. Placenta 113, 23–28. doi:10.1016/j.placenta.2021.01.007
Mugerli, S., Zupančič, D., Rok, R., and Lučovnik, M. (2022). Transmission electron microscopy demonstration of reduced endothelial glycocalyx in severe preeclampsia. Placenta 126, 64–69. doi:10.1016/j.placenta.2022.06.010
Nishida, R., Suzuki, D., Akimoto, Y., Matsubara, S., Hayakawa, J., Ushiyama, A., et al. (2023). Exploring the pathophysiological mechanism of interstitial edema focusing on the role of macrophages and their interaction with the glycocalyx. J. Oral Biosci. 65, 111–118. doi:10.1016/j.job.2023.01.001
Pikoula, M., Tessier, M. B., Woods, R. J., and Ventikos, Y. (2018). Oligosaccharide model of the vascular endothelial glycocalyx in physiological flow. Microfluid. Nanofluid. 22, 21. doi:10.1007/s10404-018-2037-5
Pries, A. R., Secomb, T. W., Jacobs, H., Sperandio, M., Osterloh, K., and Gaehtgens, P. (1997). Microvascular blood flow resistance: role of endothelial surface layer. Am. J. Physiol. 273, H2272–H2279. doi:10.1152/ajpheart.1997.273.5.H2272
Psefteli, P. M., Kitscha, P., Vizcay, G., Fleck, R., Chapple, S. J., Mann, G. E., et al. (2021). Glycocalyx sialic acids regulate Nrf2-mediated signaling by fluid shear stress in human endothelial cells. Redox Biol. 38, 101816. doi:10.1016/j.redox.2020.101816
Raaz, U., Toh, R., Maegdefessel, L., Adam, M., Nakagami, F., Emrich, F. C., et al. (2014). Hemodynamic regulation of reactive oxygen species: implications for vascular diseases. Antioxid. Redox Signal. 20, 914–928. doi:10.1089/ars.2013.5507
Reynolds, O. (1883). An experimental investigation of the circumstances which determine whether the motion of water shall Be direct or sinuous, and of the law of resistance in parallel channels. Proc. R. Soc. Lond. 174, 935–982. doi:10.1098/rstl.1883.0029
Ridone, P., Vassalli, M., and Martinac, B. (2019). Piezo1 mechanosensitive channels: what are they and why are they important. Biophys. Rev. 11, 795–805. doi:10.1007/s12551-019-00584-5
Rubio-Gayosso, I., Platts, S. H., and Duling, B. R. (2006). Reactive oxygen species mediate modification of glycocalyx during ischemia-reperfusion injury. Am. J. Physiol. Heart Circ. Physiol. 290, H2247–H2256. doi:10.1152/ajpheart.00796.2005
Sakamoto, N., Kiuchi, T., and Sato, M. (2011). Development of an endothelial-smooth muscle cell coculture model using phenotype-controlled smooth muscle cells. Ann. Biomed. Eng. 39, 2750–2758. doi:10.1007/s10439-011-0372-8
Shi, D., Sheng, A., and Chi, L. (2021). Glycosaminoglycan-protein interactions and their roles in human disease. Front. Mol. Biosci. 8, 639666. doi:10.3389/fmolb.2021.639666
Sidnawi, B., Chen, Z., Sehgal, C., and Wu, Q. (2020). Characterization of arterial flow mediated dilation via a physics-based model. J. Mech. Behav. Biomed. Mat. 107, 103756. doi:10.1016/j.jmbbm.2020.103756
Slowey, C., and Nyhan, D. (2022). The vascular system: anatomical, physiological, pathological, and aging considerations. Anesthesiol. Clin. 40, 557–574. doi:10.1016/j.anclin.2022.08.004
Smart, L., Bosio, E., Macdonald, S. P. J., Dull, R., Fatovich, D. M., Neil, C., et al. (2018). Glycocalyx biomarker syndecan-1 is a stronger predictor of respiratory failure in patients with sepsis due to pneumonia, compared to endocan. J. Crit. Care. 47, 93–98. doi:10.1016/j.jcrc.2018.06.015
Souilhol, C., Serbanovic-Canic, J., Fragiadaki, M., Chico, T. J., Ridger, V., Roddie, H., et al. (2020). Endothelial responses to shear stress in atherosclerosis: a novel role for developmental genes. Nat. Rev. Cardiol. 17, 52–63. doi:10.1038/s41569-019-0239-5
Squire, J. M., Chew, M., Nneji, G., Neal, C., Barry, J., and Michel, C. (2001). Quasi-periodic substructure in the microvessel endothelial glycocalyx: a possible explanation for molecular filtering? J. Struct. Biol. 136, 239–255. doi:10.1006/jsbi.2002.4441
Storch, A. S., Rocha, H. N. M., Garcia, V. P., Batista, G. M. D. S., Mattos, J. D., Campos, M. O., et al. (2018). Oscillatory shear stress induces hemostatic imbalance in healthy men. Thromb. Res. 170, 119–125. doi:10.1016/j.thromres.2018.08.019
Suzuki, A., Tomita, H., and Okada, H. (2022). Form follows function: the endothelial glycocalyx. Transl. Res. 247, 158–167. doi:10.1016/j.trsl.2022.03.014
Tarbell, J. M., Shi, Z. D., Dunn, J., and Jo, H. (2014). Fluid mechanics, arterial disease, and gene expression. Annu. Rev. Fluid Mech. 46, 591–614. doi:10.1146/annurev-fluid-010313-141309
Tovar-Lopez, F., Thurgood, P., Gilliam, C., Nguyen, N., Pirogova, E., Khoshmanesh, K., et al. (2019). A microfluidic system for studying the effects of disturbed flow on endothelial cells. Front. Bioeng. Biotechnol. 7, 81. doi:10.3389/fbioe.2019.00081
Traub, O., and Berk, B. C. (1998). Laminar shear stress: mechanisms by which endothelial cells transduce an atheroprotective force. Arterioscler. Thromb. Vasc. Biol. 18, 677–685. doi:10.1161/01.atv.18.5.677
Tresoldi, C., Pellegata, A. F., and Mantero, S. (2015). Cells and stimuli in small-caliber blood vessel tissue engineering. Regen. Med. 10, 505–527. doi:10.2217/rme.15.19
Tsai, M. C., Chen, L., Zhou, J., Tang, Z., Hsu, T. F., Wang, Y., et al. (2009). Shear stress induces synthetic-to-contractile phenotypic modulation in smooth muscle cells via peroxisome proliferator-activated receptor alpha/delta activations by prostacyclin released by sheared endothelial cells. Circ. Res. 105, 471–480. doi:10.1161/circresaha.109.193656
Tun, W. M., Yap, C. H., Saw, S. N., James, J. L., and Clark, A. R. (2019). Differences in placental capillary shear stress in fetal growth restriction may affect endothelial cell function and vascular network formation. Sci. Rep. 9, 9876. doi:10.1038/s41598-019-46151-6
Warboys, C. M., Ghim, M., and Weinberg, P. D. (2019). Understanding mechanobiology in cultured endothelium: a review of the orbital shaker method. Atherosclerosis 285, 170–177. doi:10.1016/j.atherosclerosis.2019.04.210
Weinbaum, S., Cancel, L. M., Fu, B. M., and Tarbell, J. M. (2021). The glycocalyx and its role in vascular physiology and vascular related diseases. Cardiovasc. Eng. Technol. 12, 37–71. doi:10.1007/s13239-020-00485-9
Xu, S. (2020). Therapeutic potential of blood flow mimetic compounds in preventing endothelial dysfunction and atherosclerosis. Pharmacol. Res. 155, 104737. doi:10.1016/j.phrs.2020.104737
Yao, Y., Rabodzey, A., and Dewey, C. F. (2007). Glycocalyx modulates the motility and proliferative response of vascular endothelium to fluid shear stress. Am. J. Physiol. Heart Circ. Physiol. 293, H1023–H1030. doi:10.1152/ajpheart.00162.2007
Zeng, Y., Du, X., Yao, X., Qiu, Y., Jiang, W., Shen, J., et al. (2021). Mechanism of cell death of endothelial cells regulated by mechanical forces. J. Biomech. 131, 110917. doi:10.1016/j.jbiomech.2021.110917
Keywords: flow shear stress, endothelial cells, mechanotransduction, endothelial functionality, endothelial glycocalyx
Citation: Zhou HL, Jiang XZ and Ventikos Y (2023) Role of blood flow in endothelial functionality: a review. Front. Cell Dev. Biol. 11:1259280. doi: 10.3389/fcell.2023.1259280
Received: 15 July 2023; Accepted: 04 October 2023;
Published: 13 October 2023.
Edited by:
Bingmei M. Fu, City College of New York (CUNY), United StatesReviewed by:
Yunfeng Chen, University of Texas Medical Branch at Galveston, United StatesMean Ghim, Yale University, United States
Copyright © 2023 Zhou, Jiang and Ventikos. This is an open-access article distributed under the terms of the Creative Commons Attribution License (CC BY). The use, distribution or reproduction in other forums is permitted, provided the original author(s) and the copyright owner(s) are credited and that the original publication in this journal is cited, in accordance with accepted academic practice. No use, distribution or reproduction is permitted which does not comply with these terms.
*Correspondence: Xi Zhuo Jiang, amlhbmd4ekBtYWlsLm5ldS5lZHUuY24=; Yiannis Ventikos, eWlhbm5pcy52ZW50aWtvc0Btb25hc2guZWR1