- 1Department of Anatomy, College of Medicine and Health Sciences, United Arab Emirates University, Al Ain, United Arab Emirates
- 2Department of Genetics and Genomics, United Arab Emirates University, Al Ain, United Arab Emirates
- 3Department of Nutrition and Health, College of Medicine and Health Sciences, United Arab Emirates University, Al Ain, United Arab Emirates
Proper growth and branching of dendrites are crucial for adequate central nervous system (CNS) functioning. The neuronal dendritic geometry determines the mode and quality of information processing. Any defects in dendrite development will disrupt neuronal circuit formation, affecting brain function. Besides cell-intrinsic programmes, extrinsic factors regulate various aspects of dendritic development. Among these extrinsic factors are extracellular molecular signals which can shape the dendrite architecture during early development. This review will focus on extrinsic factors regulating dendritic growth during early neuronal development, including neurotransmitters, neurotrophins, extracellular matrix proteins, contact-mediated ligands, and secreted and diffusible cues. How these extracellular molecular signals contribute to dendritic growth has been investigated in developing nervous systems using different species, different areas within the CNS, and different neuronal types. The response of the dendritic tree to these extracellular molecular signals can result in growth-promoting or growth-limiting effects, and it depends on the receptor subtype, receptor quantity, receptor efficiency, the animal model used, the developmental time windows, and finally, the targeted signal cascade. This article reviews our current understanding of the role of various extracellular signals in the establishment of the architecture of the dendrites.
1 Introduction
The dendrites of neurons represent the main input compartment, and the proper growth and arborisation of dendrites are crucial for the proper functioning of the central nervous system. The dendrites of a neuron contain a variety of receptors designed to receive signal input from other cells for communication, differentiation, and maturation. In neurons, axon generation (axogenesis) and specification precede dendritogenesis (Barnes and Polleux, 2009). The neurites are specified to become axon or dendrites. Generally, dendrites differ from axons at the molecular, functional, and morphological levels. The initial polarization of the neuron is regulated by both extrinsic factors and intrinsic cues (see review by Horton and Ehlers, 2003). In vitro and in vivo studies have shown that few nascent processes extend from the soma, and one of these will differentiate into the axon and grow out of the soma, whereas the remaining neurites are destined to become dendrites (Dotti and Banker, 1987; Dotti et al., 1988) (Figure 1). Most studies on dendritic growth have shown that dendritic growth process is extremely dynamic (Cline, 2001; Prigge and Kay, 2018). The new dendritic branches extend and retract undergoing constant remodeling. In most neuronal types, dendrites grow away from the cell body using guidance molecules, so they increase in length and diameter and, thereafter, they start to bifurcate to increase their complexity. Both extrinsic and intrinsic factors are involved in dendritic growth process but for the scope of this review we will focus only on extrinsic factors that regulate dendritic growth. There are mechanisms that ensure that dendritic arbors maximise their spread across a territory while minimising the redundancy of the innervated area between dendrites of different neurons, a process known as dendritic tilling (Grueber and Sagasti, 2010). Meanwhile, dendrites develop specialized structures named dendritic spines (spinogenesis), which are one of the important contact sites for communication between neurons to ensure proper network maturation (Ben-Ari, 2001). At this point, neurons refine and modify their dendritic tree through retraction and elimination of dendritic parts (dendritic pruning). It is now well recognized that pruning of dendrites as a mean to refine neuronal networks is a widespread phenomenon required for the normal development of vertebrate and invertebrate nervous systems (for a review, see Schuldiner and Yaron, 2015).
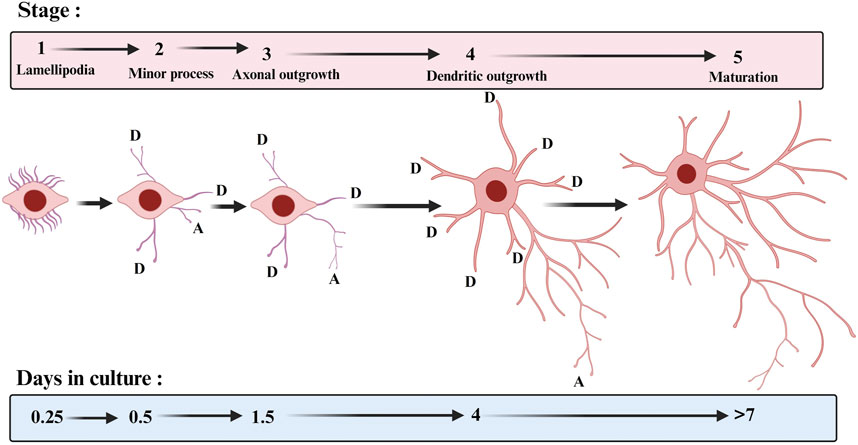
FIGURE 1. Stages of hippocampal neuronal development in culture. The approximate times when cells enter each of the stages are indicated. D: dendrite and A: axon. The template for this figure was (Dotti et al., 1988).
During early development, dendritic growth is regulated by both cell-intrinsic programmes and extrinsic factors that regulate various aspects of dendritic development (Jan and Jan, 2003; Lin et al., 2020). It was always believed that dendritic growth is intrinsically determined. However, in the past 2 decades, numerous studies have shown that the dendritic growth process is remarkably responsive to extrinsic factors, influencing local and global mechanisms of dendrite development (Valnegri et al., 2015). The extrinsic factors include neurotransmitters, neurotrophins, extracellular matrix proteins, contact-mediated ligands, and secreted and diffusible cues. On the other hand, the intrinsic factors that play a key role in dendritic development are mediated by specific transcription factors. They play a key role in determining the neuronal identity, morphological features, positioning and functional electrical properties of the neuronal cells; comprehensive reviews on the role of the transcription factor in dendritic growth development appear elsewhere (McAllister, 2000; Jan and Jan, 2003; Schuldiner and Yaron, 2015; Ledda and Paratcha, 2017). The neuronal membrane is involved in neuronal preservation and functioning. For a neuron to respond to an external stimulus, it is necessary to have sufficient active membrane receptors. Therefore, manipulating the neuronal receptor quantity or efficiency can affect the dendritic growth process. The Ca2+ set-point hypothesis for dendritic growth suggests that too little or too much intracellular Ca2+ inhibits neurite growth, whereas maintaining a moderate intracellular Ca2+ level is essential for proper dendritic development (Lohmann and Wong, 2005). Ca2+ signaling impacts dendritic growth by triggering specific signalling cascades. In addition, Ca2+ signalling influences dendrite dynamics through modulation of the cytoskeleton near the Ca2+ entry site, whereas Ca2+−dependent dendritic growth involves activating specific transcription factors (Konur and Ghosh, 2005). Ca2+ signalling through the intracellular CaMKII also has been implicated in dendritic growth in early development. CaMKII has two different isoforms, CaMKIIα which restrict dendritic growth (Wu and Cline, 1998; Redmond et al., 2002) and CaMKIIβ which promote dendritic growth (Fink et al., 2003), suggesting that CaMKs are receptor downstream Ca2+−dependent dendritic growth regulators.
Neuronal activity has been shown to shape synaptic connectivity early in the development of the central nervous system (Katz and Shatz, 1996; Kirischuk et al., 2017). One remarkable feature of the developing brain is the appearance of spontaneous neuronal activity (Khazipov and Luhmann, 2006; Luhmann et al., 2016). The early network spontaneous activity patterns coincide with the period of rapid dendritic growth dependent on Ca2+ signalling (Ben-Ari, 2001). Blocking the synaptic activity in optic tectum neurons delayed dendritic development in vivo (Rajan and Cline, 1998). Enhanced visual activity driven by a light stimulus has been shown to promote dendritic growth (Sin et al., 2002). Visual deprivation from dark rearing in the visual system decreases the dendritic growth of stellate neurons (Coleman and Riesen, 1968). In addition, the overexpression or the elimination of certain receptors that increase neuronal excitability affects the dendritic growth process (Haas et al., 2006; Hamad et al., 2011; Jack et al., 2019). Therefore, neuronal activity is key in dendritic growth during early development. This review summarises and critically evaluates the extracellular molecular signals that control dendrite architecture. Additionally, we present our perspectives on the implications of abnormal dendritic morphology for nervous system diseases.
2 Extracellular molecular signals regulating dendritic growth: Classes and mechanisms of action
2.1 Neurotransmitters
2.1.1 Glutamate
Glutamate is a major neurotransmitter that regulates dendritic growth, and glutamatergic transmission convergence with Ca2+ signalling plays a key role in dendritic differentiation. Based on pharmacology and structural homology, the Glutamate receptors are grouped into four distinct classes, including the AMPA receptors (GluA1–GluA4), the kainate receptors (GluK1–GluK5), the NMDA receptors (GluN1, GluN2A–GluN2D, GluN3A, and GluN3B), and the δ receptors (GluD1 and GluD2) (Traynelis et al., 2010). Among these classes, the AMPAR-type glutamate receptor (AMPAR) mediates the fastest excitatory synaptic transmission in the brain. Studies investigating the role of glutamate and its receptors in dendritic growth are summarised in Table 1. The efficacy of AMPARs in enhancing dendritic growth depends on the overexpressed subunit editing site and splice variant. Regarding the editing variants, the overexpression of the Ca2+-permeable AMPAR subunit containing the unedited (Q) variant was reported to enhance dendritic growth (Inglis et al., 2002; Jeong et al., 2006; Prithviraj et al., 2008; Hamad et al., 2011). On the other hand, the overexpression of the edited Ca2+-impermeable variant (R) frequently did not affect dendritic growth (Inglis et al., 2002; Prithviraj et al., 2008; Hamad et al., 2011) (Figure 2). The presence of the edited variant (R) in heteromeric AMPARs renders the channel impermeable to Ca2+ and influences channel kinetics and single-channel conductance, whereas the Ca2+-permeable variant (Q) renders the channel permeable to Ca2+ (Burnashev et al., 1992). Thus, the Ca2+ permeability of the overexpressed subunit is a determining factor in promoting dendrite growth. Splice variants of AMPARs can also control dendritic growth. All four AMPAR subunits occur in two spliced versions, named flip-and-flop. The flip-flop change occurs in the proximity of the ligand-binding domain in the extracellular loop (Sommer et al., 1990). In neurons, flip variants are usually predominant in early developmental stages and are replaced by flop variants in adulthood (Monyer et al., 1991). The flip variants have slower desensitisation time than the flop variant, keeping the channel open longer, and thereby allowing more Ca2+ influx into the cell. Expectedly, overexpression of the AMPAR subunits GluA1, GluA2, and GluA3 harbouring the flip variant improved dendritic growth (Inglis et al., 2002; Jeong et al., 2006; Prithviraj et al., 2008; Hamad et al., 2011), while overexpression of the flop variant did not affect dendritic growth in neocortical neurons (Hamad et al., 2011). On the other hand, the overexpression of AMPARs GluA1 and GluA2 C-terminal domains in optic tectal neurons reduced dendritic growth (Haas et al., 2006). However, in P28, the overexpression of the GluA1 subunit did not alter the dendritic length (Jeong et al., 2006). Generally, blocking glutamate receptors in rat neocortical organotypic cultures reduced the dendritic growth of these neurons (Baker et al., 1997). Furthermore, the stimulation with the positive allosteric modulators of AMPARs (ampakines) increased the dendritic growth of hippocampal pyramidal neurons in adult rats (Lauterborn et al., 2016). Although chronic treatment with ampakines did not disturb basic synaptic physiology, it positively affected long-term potentiation. Taken together, these studies suggest that AMPARs play a key role in dendritic development and such effect relies on these receptors editing and splice variants. Generally, the Ca2+ permeability of AMPARs is a key factor for promoting dendritic growth.
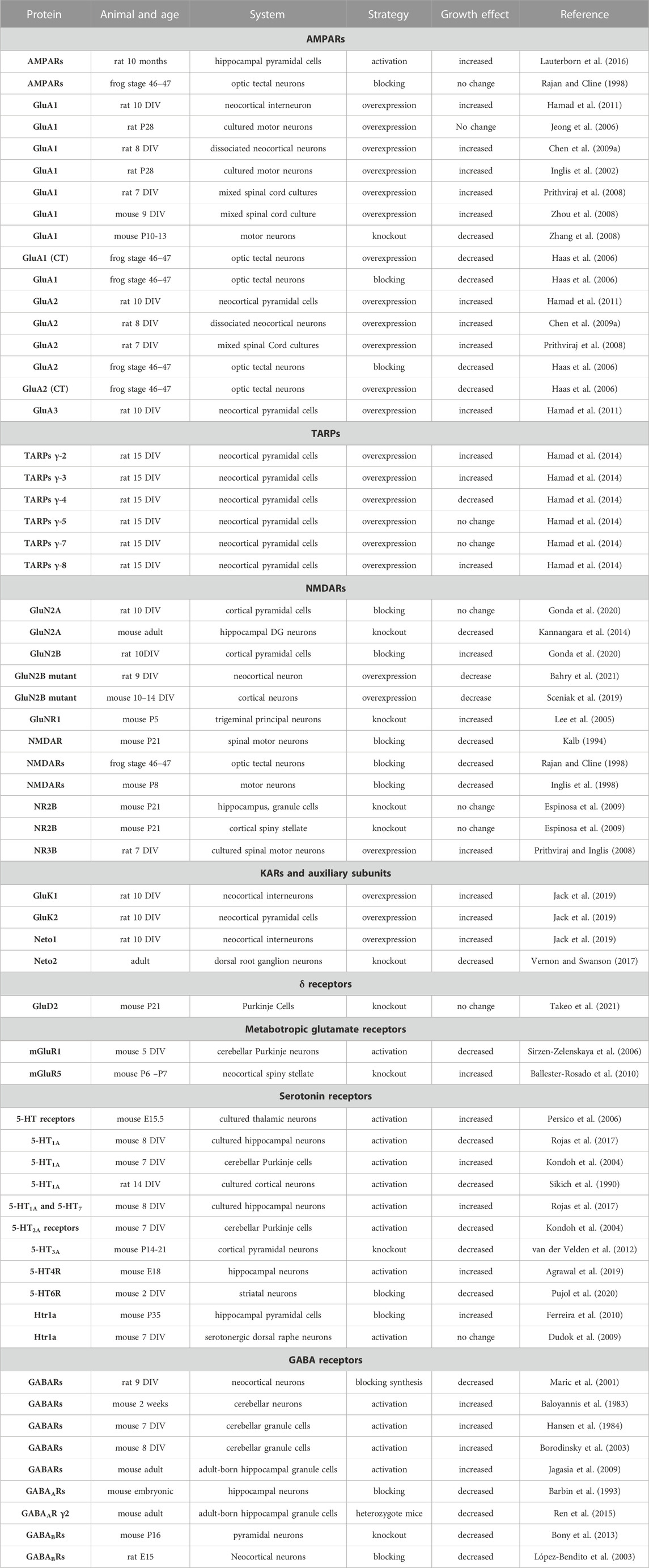
TABLE 1. Summary of the studies on the role of neurotransmitters and their receptors in dendritic growth. Days in vitro (DIV). Postnatal day (P). Embryonic (E).
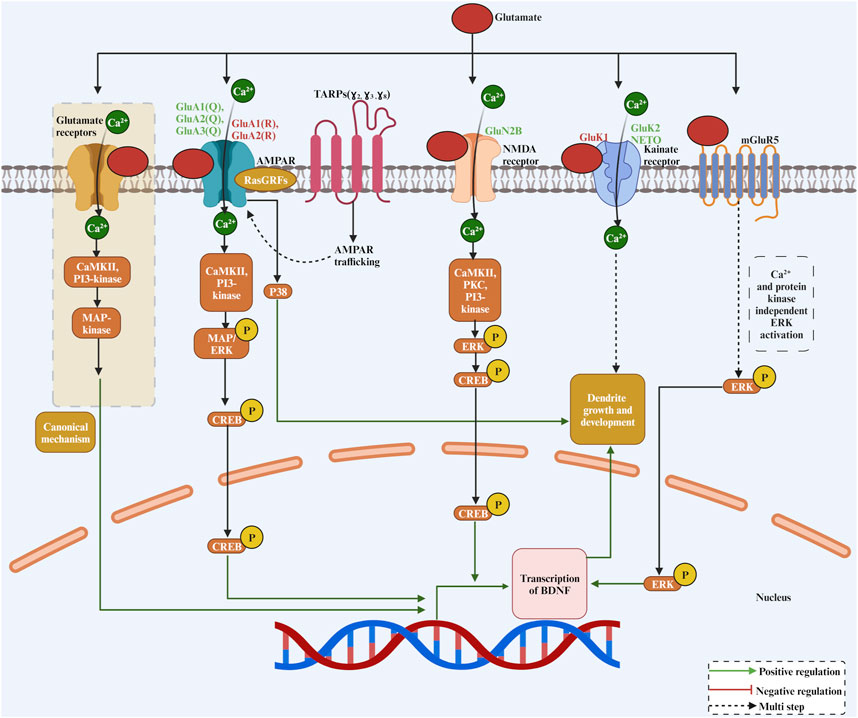
FIGURE 2. The complex role of glutamate as a neurotransmitter and its receptors in dendritic growth. Glutamate binds to a variety of receptors, including AMPAR, NMDA, Kainate and mGluR5, activating CREB in a Ca2+ and kinase-dependent manner except for mGluR5 for transcriptional activation of genes required for dendritic growth and development.
The glutamate kainate receptors (KARs) have also been involved in dendritic growth. Overexpression of the GluK1 kainate subunit promoted inhibitory interneuron dendritic growth, while overexpression of the GluK2 kainate receptor promotes dendritic growth of excitatory pyramidal cells in the neocortex (Jack et al., 2019). The glutamate receptors NMDA type are also involved in dendritic development. In vivo, injection of NMDAR antagonists MK-801 or APV reduced dendritic growth of spinal cord motoneurons (Kalb, 1994). Furthermore, pharmacological antagonising of the GluN2B but not the GluN2A receptor reduced the basal dendrites of neocortical pyramidal cells (Gonda et al., 2020). On the other hand, a single-cell knockout of the glutamate receptor GluN2B in hippocampal granule cells and cortical spiny stellate cells did not alter the dendritic size of these cells (Espinosa et al., 2009). A reduction in dendritic growth has also been reported in glutamate receptor GluN2A knockout hippocampal neurons (Kannangara et al., 2014). Furthermore, the barrelette neurons in the principal trigeminal nucleus had an increased dendritic size in GluNR1 knockout mice (Lee et al., 2005). Blocking NMDARs with APV reduced dendritic length and branching of optic tectal neurons (Rajan and Cline, 1998) and spinal cord motoneurons (Inglis et al., 1998). Overexpression of the NMDARs subunit GluN3B increased dendritic complexity in spinal cord culture (Prithviraj and Inglis, 2008), and overexpression of the GluN2B mutant caused a decrease in dendritic length in neocortical neurons (Sceniak et al., 2019). These studies suggest a role for NMDARs in shaping dendritic trees.
How do glutamate receptors regulate dendritic growth? Generally, glutamate receptors regulate gene expression in neurons by activating various intracellular signalling cascades that phosphorylate transcription factors within the nucleus (Figure 2). On the other hand, it has been reported that glutamate can induce elongation of dendritic protrusions through group I mGluRs within minutes, suggesting that glutamate-induced dendritic growth is often way too rapid to be mediated by slow transcriptional events (Cruz-Martín et al., 2012). However, the exact mechanism by which glutamate induces rapid dendritic growth through group I mGluRs is unknown. One of the best-characterized signal cascades for glutamate-mediated growth regulatory process is the mitogen-activated protein kinase (MAPK) (Wang et al., 2007). MAPK is usually activated following sustained plasma membrane depolarisation, which triggers Ca2+ entry to the cell through glutamate receptors. Activity-dependent activation of the MAPK pathway in hippocampal neurons has been shown to elicit stable dendritic protrusion of new filopodia (Wu et al., 2001). Furthermore, the MAPK pathway is important for Ca2+ influx-mediated dendritic growth in neocortical neurons (Redmond et al., 2002). The Ca2+ permeable ionotropic glutamate receptor activates MAPKs through a signalling route involving the Ca2+-sensitive Ras-guanine nucleotide releasing factor, Ca2+/calmodulin-dependent protein kinase II (CaMKII), and phosphoinositide 3-kinase.
For the NMDARs, the activation of NMDARs triggers Ca2+ influx to neurons. Ca2+ influx to the cell activates Ca2+-dependent kinases to increase ERK phosphorylation (Figure 2). Among these kinases, the CaMKII was identified as a main Ca2+-sensitive kinase at the postsynaptic site. Protein kinase C (PKC) and phosphatidylinositol (phosphoinositide) 3-kinase (PI3-kinase) are also other candidate kinases that have been involved in NMDAR-mediated ERK phosphorylation. The high affinity of the calmodulin-binding sequence in PI3-kinase facilitates the association of calmodulin with PI3-kinase. Furthermore, ERK phosphorylation has been implemented in gene regulation. Interestingly, the phosphorylated form of ERK can regulate c-AMP response element binding protein (CREB). There is a strong connection between Ca2+ permeable glutamate receptor activation and CREB phosphorylation (Shaywitz and Greenberg, 1999; West et al., 2002). Activation of ERK through Ca2+ permeability of glutamate receptors has been shown to increase CREB phosphorylation (Mao et al., 2004). One of the targeted growth-promoting genes of CREB is the BDNF. Interestingly, in cortical neurons, Ca2+ influx triggers phosphorylation of CREB, which, by binding to a critical Ca2+ response element (CRE) within the BDNF gene, activates BDNF transcription (Tao et al., 1998). Mutation of BDNF CRE, an adjacent novel regulatory element, and a blockade of CREB function resulted in a dramatic loss of BDNF transcription (Tao et al., 1998). For the AMPARs, signalling for dendritic growth looks like the NMDARs. The AMPARs can induce MAPK/ERK phosphorylation (Wang et al., 2004). It has been shown that CaMKII and PI3-kinase are the two kinases that connect a pathway between AMPARs and ERK (Perkinton et al., 1999). Another mechanism by which AMPARs regulate dendrite growth is mediated through RasGRFs, which can form a complex with Ca2+-permeable AMPARs and subsequently guarantee a quick response of RasGRFs to Ca2+ signals derived from AMPARs to activate Ras-ERK (Tian and Feig, 2006). It is also important to mention that AMPARs have been coupled with p38 signaling, which could represent a determinant mechanism to decide whether nerve cells survive or die (Rivera-Cervantes et al., 2004). Kainate receptor-mediated Ca2+ influx has also been shown to trigger ERK phosphorylation in striatal neurons, and the active ERK1/2 cascade activates the downstream transcription factor CREB to participate in the regulation of gene expression (Mao et al., 2004). Taken together, these studies suggested that glutamate receptors ability to induce dendritic growth relies on Ca2+ permeability of the receptor and the subsequent increase in intracellular Ca2+, which triggers a dendritic growth-promoting signal cascade.
The trafficking of glutamate receptors is involved in dendritic growth. The discovery of the transmembrane AMPA receptor regulatory proteins (TARPs) paved the way to understand how AMPARs traffic to the plasma membrane and how the function of AMPARs is modulated (Straub and Tomita, 2012). By increasing trafficking and modulating the function of AMPARs, TARPS has also been implicated in dendritic growth (Hamad et al., 2014). In summary, overexpression of type I TARPs γ-2, γ-3, and γ-8, known to enhance AMPAR trafficking and insertion of AMPARs into the synaptic membrane, promoted dendritic growth (Figure 2). On the other hand, the overexpression of the TARP γ-4 subunit reduced dendritic growth (Hamad et al., 2014), possibly because it slows the channel opening and closing rates of AMPARs (Pierce and Niu, 2019). Type II TARPs γ-5 and γ-7 (also called non-TARPs), which potentiate the function of AMPARs but do not participate in the trafficking of AMPARs, did not affect the dendritic growth of neocortical neurons (Hamad et al., 2014). Interestingly, the TARP subunits, which increase the trafficking and insertion of new AMPAR subunits in the plasma membrane had elicited dendritic growth, whereas the TARP subunits, which only potentiate the existing receptors, did not affect dendritic growth. Furthermore, overexpression of the kainate receptor auxiliary subunit NETO1 but not NETO2 has been shown to promote dendritic growth of inhibitory interneurons (Jack et al., 2019). Furthermore, in neurons of the dorsal root ganglion, NETO2 plays a crucial and dynamic role in neurite outgrowth during early development and adulthood (Vernon and Swanson, 2017). Thus, these studies suggest that enhancing the AMPARs or kainate receptor density on the plasma membrane could also be another mechanism by which neurons regulate their dendritic growth.
Metabotropic glutamate receptors are also involved in dendrite growth (Figure 2). For instance, in group I metabotropic glutamate receptor 5 (mGluR5) knockout mice, dendritic growth of neocortical spiny stellate neurons was increased (Ballester-Rosado et al., 2010). On the other side, the activation of the metabotropic glutamate receptor mGluR1 decreased cerebellar Purkinje cells dendritic growth, and it does not require activation of phospholipase C or protein kinase C (Sirzen-Zelenskaya et al., 2006). It has been suggested that mGluR1 and mGluR5 receptor coupling to ERK occurred via mechanisms independent of PI3K activity and intracellular and/or extracellular Ca2+ concentration (Thandi et al., 2002). Therefore, it has been shown that these two closely related mGluR receptors utilize different G proteins to cause ERK activation and may recruit different tyrosine kinases to facilitate this response (Thandi et al., 2002).
2.1.2 GABA
The GABA excitation/inhibition shift occurs during brain development between the first and second postnatal developmental week (Ben-Ari et al., 2007; Kirmse et al., 2015; Luhmann, 2021). The depolarizing effect of GABA, which occurs only in early development in developing neurons, is due to the expression of the cotransporter NKCC1, which causes an efflux of Cl− ions following the activation of GABAARs (Blaesse et al., 2009). Thus, the activation of GABAARs in early development depolarises the plasma membrane, leading to Ca2+ influx through the voltage-dependent Ca2+ channels (VDCCs) (Leinekugel et al., 1997). This will raise intracellular Ca2+ levels (Tozuka et al., 2005) and thereby promote dendritic growth (Figure 3). Studies investigating the role of GABA and its receptors in dendritic growth are summarised in Table 1. GABA treatment of rat cortical neurons increased dendritic length and branching (Maric et al., 2001). Furthermore, blocking GABAARs with bicuculline decreased dendrite length and branching of hippocampal neurons (Barbin et al., 1993). In cerebellar culture neurons, feeding medium enriched with GABA enhanced dendritic growth and dendritic arborization (Baloyannis et al., 1983). Furthermore, it has been shown that GABA serves as a trophic factor for cerebellar granule cells (CGCs) (Hansen et al., 1984). The effect of GABA on CGCc dendrite growth was induced by Ca2+ influx through the activation of L-type VDCCs, as it was prevented by the blocker nifedipine (Borodinsky et al., 2003). Interestingly, GABA-induced dendritic growth of CGCs was mediated by GABAARs activation and requires Ca2+ influx and subsequent activation of CaMKII and ERK1/2 pathways (Borodinsky et al., 2003). Moreover, the adult hippocampal newly born granule cells dendrites were smaller in the γ2 GABAARs heterozygote mice (Ren et al., 2015), as GABAAR-mediates depolarisation of neurons and activate a Ca2+-dependent signalling cascade that promotes the expression of the BDNF gene and BDNF release (Baldelli et al., 2002). Furthermore, depolarising GABA is involved in the phosphorylation of CREB during the second postnatal week of newly generated neurons in the adult hippocampus, and that results in an enhancement of dendritic growth (Jagasia et al., 2009). This occurs because CREB signalling converges onto the BDNF pathway for promoting dendritic growth (Tao et al., 1998). Together, these studies suggest that GABAAR activation induces Ca2+ influx, which triggers the activation of the CaMKII and ERK1/2 pathways and this results in CREB phosphorylation and the subsequent transcription of BDNF to promote dendritic growth (Figure 3). However, this effect is restricted only to early postnatal development when GABA is still excitatory. At the end of the postnatal week, the dendritic growth-prompting action of GABA will reach an end as GABA becomes hyperpolarizing (inhibitory), and that inhibitory action is indispensable for the control of network activity.
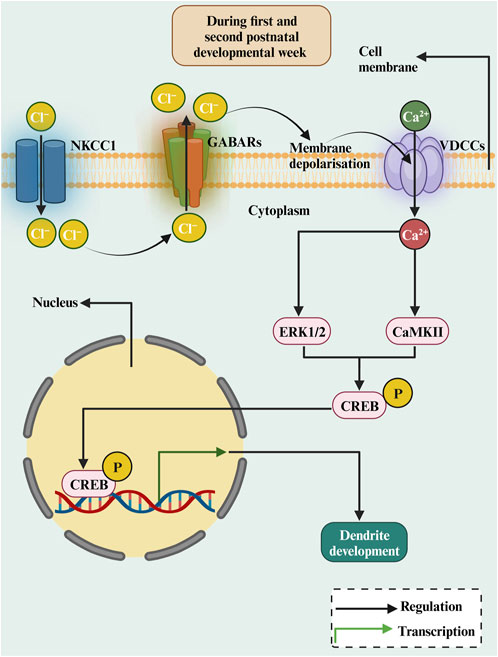
FIGURE 3. The diagrammatic representation of the roles of depolarizing GABA in dendritic growth during early development. In the immature brain, the intracellular Cl− concentration is relatively high, as Cl− transport over the membrane is dominated by NKCC1. Activation of GABAARs results in an outflow of Cl− resulting in membrane depolarization. This results in Ca2+ inflow and activating CREB by ERK1/2 or CaMKII, stimulating transcription of the BDNF gene, which is crucial for dendritic growth and development.
The role of GABABRs in dendritic growth is still not well-documented. However, some studies have reported an effect of the GABABRs on dendrite growth. For example, blocking the GABABR in organotypic cultures reduced the dendritic growth of migratory inhibitory GABAergic neurons (López-Bendito et al., 2003). The removal of GABABR in utero resulted in smaller dendrites of neocortical pyramidal cells due to disruption of cAMP/LKB1 signalling (Bony et al., 2013). These studies suggest that GABABRs are also involved in dendritic growth in early development because, during the GABABRs activation, they lack coupling to potassium channels and thus exert a non-hyperpolarizing action.
2.1.3 Serotonin
The neurotransmitter serotonin has also been involved in the dendritic growth process (Figure 4; Table 1). Serotonin application to rat cortical neurons has been shown to inhibit dendritic growth and branching (Sikich et al., 1990). On the other hand, serotonin has been shown to increase dendritic growth and branching of cultured thalamic neurons (Persico et al., 2006). Moreover, in organotypic cultures, cerebellar Purkinje cells dendritic growth was promoted by serotonin through 5-HT1A receptors but inhibited by serotonin through 5-HT2A receptors (Kondoh et al., 2004). However, the inhibition of serotonin synthesis, using DL-P-chlorophenylalanine (PCPA), a reversible inhibitor of 5-HT synthesis, during embryonic developmental stage, reduced dendritic growth of adult cortical pyramidal neurons (Vitalis et al., 2007). Serotonergic receptors mediate the dendritic growth process. Activating the serotonin receptor Htr1A with 8-OH-DPAT increased dendritic length and branching in cultured embryonic thalamic neurons in mice (Persico et al., 2006). Later, activation of Htr1A by 8-OH-DPAT for 7 days did not affect dendritic growth in mouse dorsal raphe nuclei slice cultures (Dudok et al., 2009). Similarly, no morphological changes have been revealed in Htr1A knockout mice (Heisler et al., 1998). In addition, the pharmacological blockade of serotonin receptor 1A (Htr1a) increased hippocampal pyramidal dendritic growth at P35 (Ferreira et al., 2010). The Htr1a activation reduces the cAMP levels by inhibiting adenylyl cyclase and increasing autophosphorylation of CaMKIIα, which restricts dendritic growth. The Htr1B receptor seems not to be involved in dendritic growth since Htr1B knockout mice presented with no morphological abnormality (Saudou et al., 1994). Furthermore, activating the 5-HT1a and 5-HT7 receptors increased the dendritic growth of cultured hippocampal neurons (Rojas et al., 2017). The activation of 5-HT1AR and 5-HT7R promotes the growth of short secondary dendrites and triggers ERK1/2 and AKT phosphorylation through MEK and PI3K activation, respectively. Studies on the Htr2A/C receptor have shown different effects on neurite growth. For example, activation of the Htr2A/C receptor decreases the total dendritic length of cerebellar Purkinje cells (Kondoh et al., 2004). However, activation of the Htr2A/C enhanced dendritic growth of cultured embryonic mouse thalamic neurons in mice (Persico et al., 2006). Among the serotonin receptors, Htr3 is the only ionotropic receptor subtype. The activation of the Htr3 receptor with m-CPBG enhanced the dendritic growth of cultured rat cortical neurons (Vitalis and Parnavelas, 2003). No abnormalities were found in Htr3A knockout mice (Walstab et al., 2010), however, an enhancement of apical dendrite length has been reported in the neocortical neurons of Htr3A knockouts (van der Velden et al., 2012). Therefore, how do serotonin receptors modulate dendritic growth? First of all, as the studies above suggested, serotonin has a different growth action for dendritic growth, and that effect depends on the nature of the receptor (Figure 4). For instance, the Htr1 and Htr5 receptor families are inhibitory. On the other hand, the Htr2, Htr3, Htr4, Htr6, and Htr7 receptor families are excitatory. Furthermore, the stimulation of 5-HT4R with the agonist RS67333 had increased hippocampal neurons dendritic growth (Agrawal et al., 2019) as it upregulates the expression of the neurotrophic factors BDNF, NT-3, NGF as also the TRK-A receptor. Moreover, Htr3 is ionotropic and could depolarize the plasma membrane, resulting in cations influx. The other serotonin receptors are G protein-coupled (GPCR). For example, Htr1, Htr4, Htr5, Htr6, and Htr7 modulate cAMP activity, and by increasing cAMP activity, CREB can be phosphorylated, targeting BDNF transcription. For the Htr3 receptor, serotonin-induced increases in intracellular Ca2+, which are mediated by 5-HT (3) receptors and L-type Ca2+ channels, and subsequent activation of calmodulin and calcineurin have been shown to enhance NGF-induced neurite outgrowth (Homma et al., 2006). The serotonin receptors activating the G protein have a dendritic growth signalling pathway (Figure 4). For instance, the serotonin receptor Htr1A activation leads to a number of neurotrophic events by activating a series of signal transduction molecules and these effect s has been shown to be mediated through G protein (Fricker et al., 2005). Finally, serotonin has been shown to induce the expression of growth factors, in particular BDNF (Vaidya et al., 1997). Taken together, these studies suggest that serotonin modulate dendritic growth. The action of serotonin depends on the nature of the activated receptor as well as the activated signaling cascade.
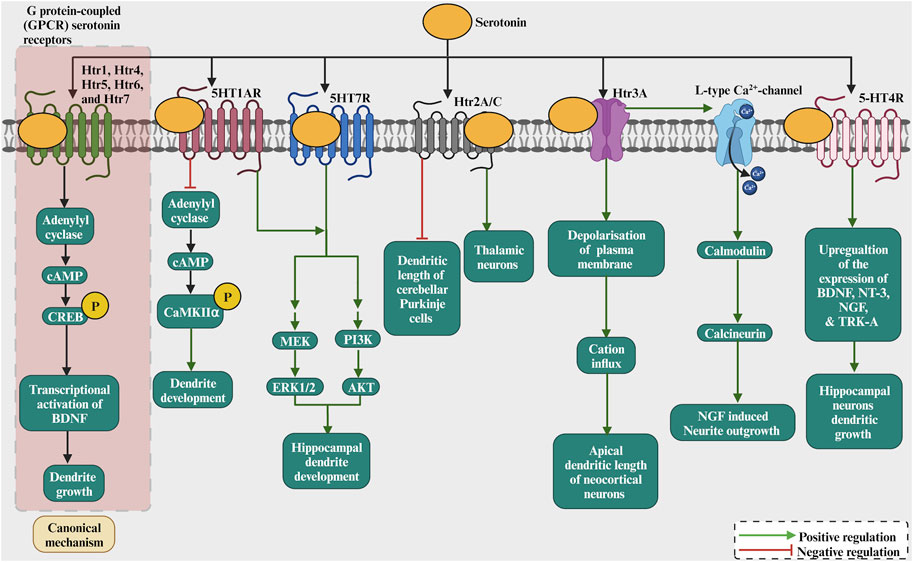
FIGURE 4. The role of serotonin as a neurotransmitter by binding to receptors such as 5HT1R, Htr2A/C, Htr3A, 5HT4R, which modulates adenylate cyclase and phosphorylating CREB/CaMKII. This results in the transcription of BDNF/NT3/NGF/Trk-A and activation of CREB by ERK1/2 or others that regulate dendritic growth and development. Serotonin induces the increase in intracellular Ca2+ via activation of 5-HT3A receptors and voltage-gated Ca2+ channels and subsequent activation of calmodulin and calcineurin which enhances NGF-induced neurite outgrowth. The 5-HT4R stimulation increased hippocampal neurons dendritic growth as it upregulates the expression of the neurotrophic factors BDNF, NT-3, NGF and also the TRK-A receptor.
2.2 Neurotrophins
Neurotrophins are a family of closely related proteins initially identified as survival factors for sensory and sympathetic neurons and have since been shown to regulate many aspects of neuronal survival, development, and function in the peripheral and the central nervous systems. Neurotrophins consist of nerve growth factor (NGF), brain-derived growth factor (BDNF), neurotrophin 3 (NT-3), and neurotrophin 4 (NT-4). Individual neurotrophins have been shown to activate the p75 neurotrophin receptor (p75NTR), a member of the tumour necrosis factor receptor superfamily (McAllister et al., 1999). In addition, neurotrophins have been shown to activate one or more of the three known members of the tropomyosin-related kinase (Trk) family of receptor tyrosine kinases (TrkA, TrkB and TrkC). Neurotrophins have been shown to play a key role in regulating dendritic architecture in neurons. Studies investigating the role of neurotrophins in dendritic growth are summarised in Table 2. BDNF is perhaps the most extensively studied molecule known to influence cortical neurons dendritic development and its receptor, TrkB, is broadly expressed in the developing and adult mammalian brain. Early studies carried out in organotypic cultures showed the contribution of BDNF to dendritic development, where is has been shown to increase the dendrite complexity of cortical pyramidal neurons by increasing total dendritic length, the number of dendritic segments, and the number of primary dendrites (McAllister et al., 1995; Niblock et al., 2000). Interestingly, BDNF exerted a growth effect after 1 day of treatment. Furthermore, the effect of BDNF on dendritic growth was specific as it was pronounced in basal but not apical dendrites. The overexpression of BDNF in neocortical pyramidal neurons elicited dramatic sprouting of basal dendrites, accompanied by a regression of dendritic spines (Horch et al., 1999). In early development, the overexpression of BDNF or NT4 in neocortical pyramidal cells increased dendritic length and number of segments without affecting maximum branch order and number of primary dendrites, suggesting that early in development BDNF probably accelerates dendritogenesis in an autocrine fashion (Wirth et al., 2003). NT-4 and NT-3 could, in part, mediate dendritic growth and branching of cortical pyramidal neurons in earlier developmental stages as it is expressed at an early age during cortical development and also signals through the TrkB receptor (Timmusk et al., 1993). In addition, in the visual cortex, BDNF released from dendrites and cell bodies acted directly on nearby recipient neurons to increase dendritic branching in a distance-dependent manner (Horch and Katz, 2002), suggesting that BDNF can control dendritic structure in a spatially restricted manner. Furthermore, using a conditional mouse mutant essentially lacking BDNF, it has been shown that BDNF remarkably enhanced dendritic growth of cultured striatal neurons but not of those of hippocampal neurons, suggesting that the differential responsiveness to BDNF is part of a neurone-intrinsic program (Rauskolb et al., 2010). Furthermore, the overexpression of BDNF in the dendritic structure of granule cells of the hippocampal dentate gyrus increases their dendritic complexity (Tolwani et al., 2002). BDNF has also been shown to modulate the dendritic architecture in cortex and hippocampus in a cell type-specific manner, suggesting that diverse levels of responsiveness to BDNF among different populations of hippocampal and cortical neurons within the same brain area (Zagrebelsky et al., 2018). Taken together, these studies suggest that neurotrophins exert their growth-promoting effect in a cell-type-specific and spatially restricted manner.
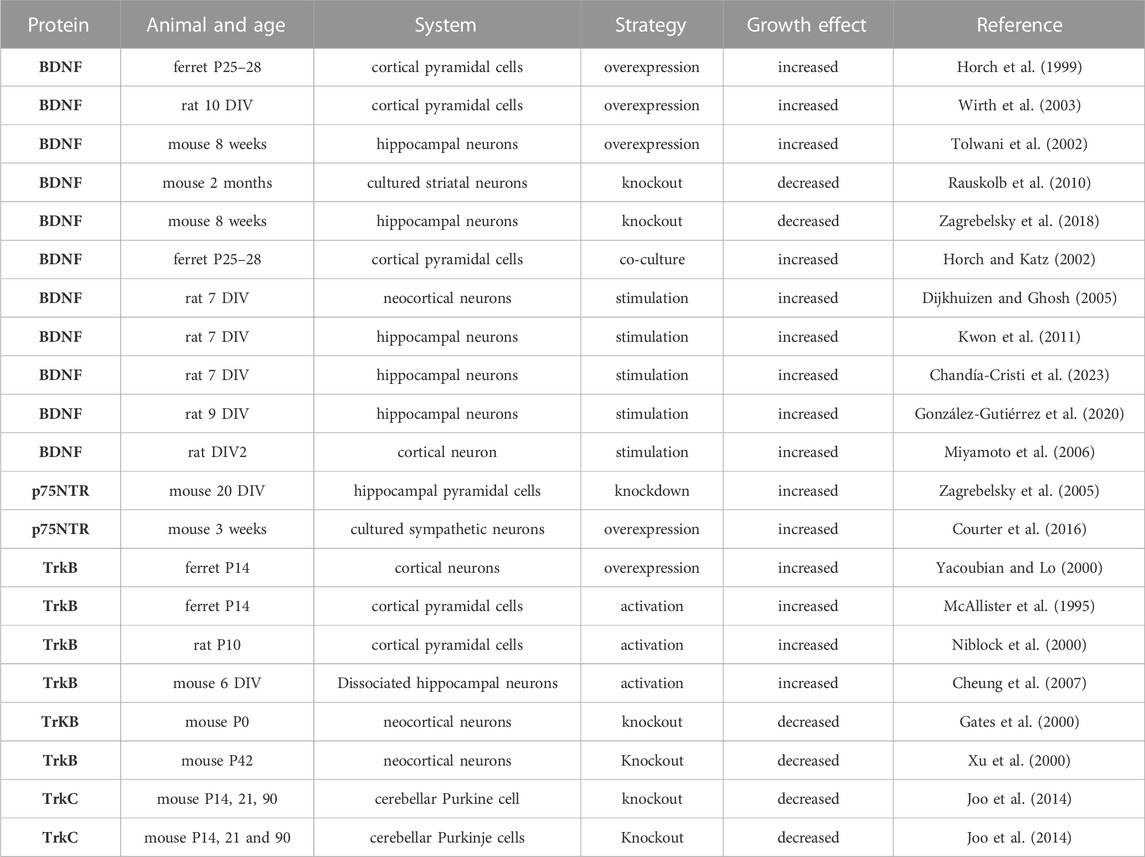
TABLE 2. Summary of the studies conducted on the role of neurotrophins and their receptors in dendritic growth. Days in vitro (DIV). Postnatal day (P).
Interestingly, altering TrkB expression has also been shown to influence dendrite growth. For instance, conditional deletion of TrkB in cortical pyramidal neurons induced dendritic retraction and neuronal loss, suggesting that BDNF/TrkB signalling is required for the development of neocortical neurons and maintenance of certain neuronal subtypes in the adult neocortex (Gates et al., 2000). Furthermore, in the ferret visual cortical slices, the overexpression of full-length and truncated TrkB receptors exerted differential effects on dendritic branching, with the full-length TrkB increasing proximal dendritic branching, whereas truncated TrkB promoted net elongation of distal dendrites (Yacoubian and Lo, 2000). Thus, the morphological effects of each receptor isoform were complementary to one another. A study using TrkB knockout mice indicated that TrkB signaling is important for the regulation of dendritic branching of cortical pyramidal neurons in vivo (Xu et al., 2000). The effects of p75NTR on dendrite growth depend on the cell type investigated with its overexpression in mouse-cultured sympathetic neurons increasing dendritic length and branching (Courter et al., 2016). In contrast, in hippocampal pyramidal cells, dendritic growth was increased in p75NTR knockout organotypic cultures (Zagrebelsky et al., 2005). It has been shown that p75NTR constitutively activated RhoA and negatively modulated the length of neurites and filopodia (Gehler et al., 2004). Furthermore, in TrkC knockout mice, the dendritic complexity of cerebellar Purkinje cells was reduced, suggesting that NT-3/TrkC levels are critical for their signaling (Joo et al., 2014). These data suggest that neurotrophin and its receptors play a pivotal role in dendritic growth, and their effect depends on the cell type.
Hence, how do neurotrophins binding to their receptors affect dendritic growth? Trk receptors cytoplasmic domains contain several tyrosine phosphorylation sites that recruit intermediates in intracellular signalling cascades (Figure 5). For instance, neurotrophin signalling has been shown to regulate actin filament dynamics by modulation of Rho GTPase family proteins and other actin remodelling proteins (Miyamoto et al., 2006; Cheung et al., 2007; Shirazi Fard et al., 2010; Hedrick et al., 2016). In addition, BDNF leads to the rapid activation of PI3-kinase, MAP kinase, and PLC-gamma in cortical neurons, and pharmacological inhibition of PI3-kinase and MAP kinase in dissociated cell cultures and cortical slice cultures suppresses the ability of BDNF to induce dendrite formation, suggesting that BDNF induces primary dendrite formation via activation of the PI3-kinase and MAP kinase pathways (Dijkhuizen and Ghosh, 2005). Applying BDNF to dissociated hippocampal neurons has increased neuronal cypin expression. The cypin promoter region contains putative conserved cAMP response element (CRE) regions, which have been found to be recognized and activated by the CRE-binding protein (CREB). It has been shown that BDNF promoted increases in proximal dendrites via CREB-dependent transcriptional regulation of cypin (Kwon et al., 2011). A more specific mechanism in which TrkB receptor signalling regulates dendrite growth has recently been suggested, with the non-receptor tyrosine kinase c-Abl as a possible candidate regulator of this process, as it has been implicated in tyrosine kinase receptors signalling and trafficking and consequently the regulation of dendritogenesis (Figure 5). BDNF/TrkB-dependent activation of c-Abl has been shown to be a novel and essential mechanism in TrkB signalling, which is required for branching and growth of dendrites (Chandía-Cristi et al., 2023). Furthermore, BDNF-promoted c-Abl activation did not require the signaling pathways ERK1/2, phosphoinositide 3-kinase (PI3K) and PLC-γ signalling pathways, suggesting that c-Abl acts through an independent pathway downstream of TrkB (Chanda-Cristi et al., 2023). Furthermore, following TrkB activation, the receptor undergoes endocytosis and transport to the neuronal soma. Surprisingly, in hippocampal neurons, the internalised neurotrophin bound to its Trk receptor continues to signal in endosomes and trigger the activation of Ras-MAP kinase, PLC-γ, and PI3K pathways (González-Gutiérrez et al., 2020). Therefore, the endosomal pathway is required for the signaling cascade initiated by BDNF and its receptors on the plasma membrane to modulate BDNF-dependent genes and neuronal dendritic growth mediated by the CREB transcription factor (González-Gutiérrez et al., 2020). Consequently, the TrkB receptor can be recycled into the plasma membrane or degraded by lysosomes (Gonzalez et al., 2016). It has been proposed that Rab11-dependent dendritic recycling provides a mechanism to retain TrkB in dendrites and to increase local signalling to regulate arborization (Lazo et al., 2013). Recent work has shown that the recycled TrkB can be required for long-distance induction of dendritic growth mediated through dynein-dependent BDNF-TrkB-containing endosomal transport mechanism (Moya-Alvarado et al., 2023). Furthermore, BDNF-induced dendritic growth leads to activation of the PI3K/AKT and ERK1/2 signaling pathways, as well as activation of local protein translation by mTOR (mammalian target of rapamycin) kinase (Schratt et al., 2004; Jaworski et al., 2005; Kumar et al., 2005; see also Figure 5). The local translation of the Homer2 cytoskeleton-associated protein has been shown to have important implications for growth cone dynamics and dendritic development (Schratt et al., 2004). Other studies demonstrated Trk receptors engagement with specific Leucine-rich repeat (LRR) transmembrane proteins to modulate dendritic growth. For instance, the LRR protein Lrig1 was found to interact physically with TrkB and attenuate BDNF signalling, thereby promoting hippocampal pyramidal neuronal growth (Alsina et al., 2016). In addition, Slitrk5 mediates BDNF-dependent TrkB receptor trafficking and signalling, suggesting that Slitrk5 acts as a TrkB co-receptor (Song et al., 2015, see also Figure 5). Thus, LRR proteins interaction with Trk signalling delivered further mechanisms by which neurotrophins regulate dendrites development.
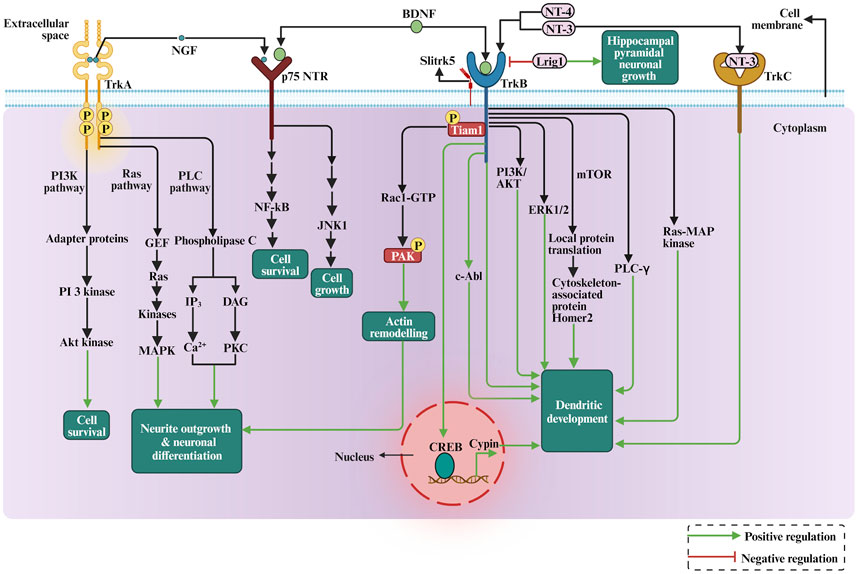
FIGURE 5. The depiction of the role of neurotrophins in dendritic growth and development involves the cellular signalling pathways, PI3K pathway, RAS-MAP kinase pathway, mTOR signalling, and actin cytoskeleton rearrangement via Rho GTPases.
The neurotrophin function appears to be linked to TRPC signaling in several ways. The TRPC is a family of transient receptor potential cation channels in animals and they regulate brain development, including neural progenitor proliferation, neurogenesis, neuron survival, axon guidance, and dendritic morphology (Tai and Jia, 2017). Some TRPC family members have been shown to influence dendritic growth (Tai et al., 2008; Puram et al., 2011; Jeon et al., 2013). Interestingly, the TRPC3 channels are necessary for BDNF to increase dendritic spine density. The activation of TrkB through BDNF leads to a TRPC3-dependent cation influx in CNS neurons in vivo (Li et al., 1999). Thus, TRPC channels emerge as novel mediators of BDNF-mediated dendritic remodeling through the activation of a slowly developing and sustained membrane depolarization (Amaral and Pozzo-Miller, 2007). Furthermore, the Ca2+ influx through TRPC5 induced by NT-3 inhibited neuronal dendritic growth by activating CaMKIIα. In contrast, the Ca2+ influx through TRPC6 induced by NT-4 promoted the dendritic growth (He et al., 2012). Thus, TRPC5 acts as a novel and specific mediator for NT-3 to regulate dendrite development through CaMKIIα. Besides regulating the sensory function of the nervous system, some TRPC family members are necessary for neurotrophins to promote dendritic growth.
2.3 Extracellular matrix proteins
In early development, the ECM regulates neuronal morphology, cell shape, differentiation, migration, and morphogenesis of neural tissues (Long and Huttner, 2019). Most well-known ECM proteins influence neurite outgrowth and axon pathfinding by integrin-ECM interaction mechanisms (Myers et al., 2011). Studies investigating the role of the ECM in dendritic growth are summarised in Table 3. Laminin was found to promote neurite outgrowth in cultured neurons (Lander et al., 1985), and it regulates neurite growth and neuronal migration via integrin signalling through the AKT/GSK-3beta pathway (Chen et al., 2009b). Moreover, the activation of integrins has also been shown to regulate dendrite growth in retinal neurons (Ivins et al., 2000). Furthermore, a selective loss of integrin β1 in excitatory hippocampal neurons reduces dendritic size and complexity (Warren et al., 2012). Moreover, the integrin α3 stabilizes the dendritic tree of hippocampal neurons through an Arg nonreceptor tyrosine kinase, which activates p190RhoGAP, and that, in turn, inhibits the RhoA GTPase and thereby stabilizes the dendritic tree (Kerrisk et al., 2013). The classical example of ECM regulating neuronal growth is the role of reelin in neocortical development (Figure 6). Reelin is a well-known ECM protein that controls several aspects of mammalian brain development and function. It controls neuronal migration and layer formation in the developing cerebral cortex (Caviness, 1976). Reelin signaling, through its membrane receptors (Bock and May, 2016), guides the migration of newborn neurons and orchestrates the development of cortical layers. The canonical Reelin signaling cascade requires direct binding of reelin to ApoER2 and VLDLR and activation of the intracellular adapter protein disabled-1 (DAB1) by tyrosine phosphorylation (D’Arcangelo et al., 1999; Trommsdorff et al., 1999). The most important function of reelin in early development is to guide the migration of newborn neurons and to control proper cortical layer development (for a review, see (Förster et al., 2010; Jossin, 2020)). Reelin exerts an opposing effect on dendritic growth depending on the developmental stage. During the embryonic developmental stage (Figure 6), reelin promotes dendritic growth and branching of embryonic pyramidal cells in dissociated hippocampal cell cultures of reeler mutant mice (Niu et al., 2004), and it increases dendritic growth of hippocampal neurons (Jossin and Goffinet, 2007; Matsuki et al., 2008). Moreover, neocortical pyramidal cell apical dendrites failed to extend and contact the marginal zone when reelin signalling was suppressed (Olson et al., 2006) and reorganized their dendrites in a tangential orientation to the marginal zone (O’Dell et al., 2015). Furthermore, reelin-induced branching of the leading processes of migrating neurons and the apical processes of radial glial cells (Chai et al., 2015). Furthermore, deletion of the reelin’s C-terminal region around E14.5 reduced the apical dendritic length of pyramidal cells around P7 (Kohno et al., 2015). Finally, reelin was found to specify the molecular identity of the pyramidal neuron distal dendritic compartment through enrichment of HCN1 and in the distal tuft dendrites of hippocampal CA1 neurons (Kupferman et al., 2014), however, conditional reelin knockout mice did not show an altered HCN1 distribution in CA1 neurons (Meseke et al., 2018). In turn, during postnatal development, an opposite effect of reelin on dendritic growth was reported. The N-terminal region of reelin restricted dendritic growth of neocortical apical pyramidal neurons (Chameau et al., 2009), and morphometric analysis of interneurons in the adult reeler neocortex and hippocampus revealed hypertrophic growth with longer dendritic branches in reeler when compared with wild-type interneurons (Yabut et al., 2007). Furthermore, newly generated neurons in the adult hippocampus respond to reelin overexpression with faster development of the dendritic tree within the first 2 weeks, while the complexity of the dendritic was reduced after 8 weeks (Teixeira et al., 2012). Finally, knocking out reelin conditionally in early postnatal development increased inhibitory interneuron dendritic growth (Hamad et al., 2021b) due to the absence of GABABR signalling (Hamad et al., 2021a). These data suggest that reelin has opposing effects on morphological neuronal maturation during embryonic when compared with postnatal dendritic development. Differential effects of the different proteolytic fragments of reelin explain the discrepancy between the roles of reelin during embryonic versus postnatal development. During early embryonal development, reelin is secreted by Cajal-Retzius (CR) cells, which disappear around P14 by undergoing selective cell death through apoptosis, and it remains exclusively expressed by inhibitory interneurons (Pohlkamp et al., 2014). There is an important biochemical basis for the function of reelin proteolysis in brain development and function (Kohno et al., 2009). After secretion from CR cells, reelin cleavage produces at least five fragments that could be detected using antibodies against N-terminal, central, and C-terminal reelin epitopes (Jossin et al., 2004). The secreted reelin fragments containing the C-terminal fragment appeared to remain localized within the marginal zone (Jossin et al., 2007). Because the C-terminus of reelin is necessary for promoting dendritic growth (Kohno et al., 2015), pyramidal cell apical dendrites, localized in the vicinity of the marginal zone, benefit from locally deposited C-terminal fragments to promote their dendritic growth. It has been shown that the cleaved N-terminal fragment is transported to distant regions of the superficial layers (Koie et al., 2014), and acts oppositely to the C-terminal fragment, i.e., as a growth-limiting signal for dendritic growth (Chameau et al., 2009). Thus, the observed discrepancies of the reelin effects might be due to the differential action of the various reelin proteolytic fragments. Hence, which signal mechanism does reelin activate to promote dendritic growth? The main component of the reelin signalling pathway which were documented for dendritic growth are the VLDLR, ApoER2, Dab1 and SFK (Niu et al., 2004; Olson et al., 2006; Jossin and Goffinet, 2007; see also Figure 6). In cultured hippocampal neurons, it has been shown that Crk family proteins are important downstream components of the reelin signalling pathway in regulating postnatal hippocampal dendritic growth (Matsuki et al., 2008). Furthermore, it has been shown that reelin signalling requires PI3-kinase, Akt and mTor for proper neocortical dendritic development (Jossin and Goffinet, 2007; see also Figure 6). Furthermore, reelin regulates the extension of the Golgi along the dendritic shaft in the cortex and hippocampus (Matsuki et al., 2010; O’Dell et al., 2012). The Cdc42/Rac1 guanine nucleotide exchange factor αPIX/Arhgef6 promoted Golgi cisternae into developing dendrites of hippocampal neurons, and reelin treatment further increased the αPIX-dependent effect, suggesting that αPIX may promote dendritic Golgi translocation, as a downstream component of a reelin-modulated signaling pathway (Meseke et al., 2013). Furthermore, there is a connection between endocytic trafficking and reelin receptor signalling. When the SNX17 (an ApoER2 binding partner of sorting) interacts with the ApoER2 cytoplasmic domain, it facilitates receptor exit from a Rab5 to a Rab11 endosomal compartment and its recycling to the cell surface (Sotelo et al., 2014). This mechanism influences ApoER2 cell surface levels and reelin-induced signalling for dendritic growth (Sotelo et al., 2014). Recently, it has been shown that there is an interaction between reelin and neuropilin-1 (Nrp1) which is required for normal dendritic development in superficial-layer neurons (Kohno et al., 2020). Taken together, these studies suggest that reelin regulates dendritic growth by using different signalling pathways to guarantee proper neuronal maturation.
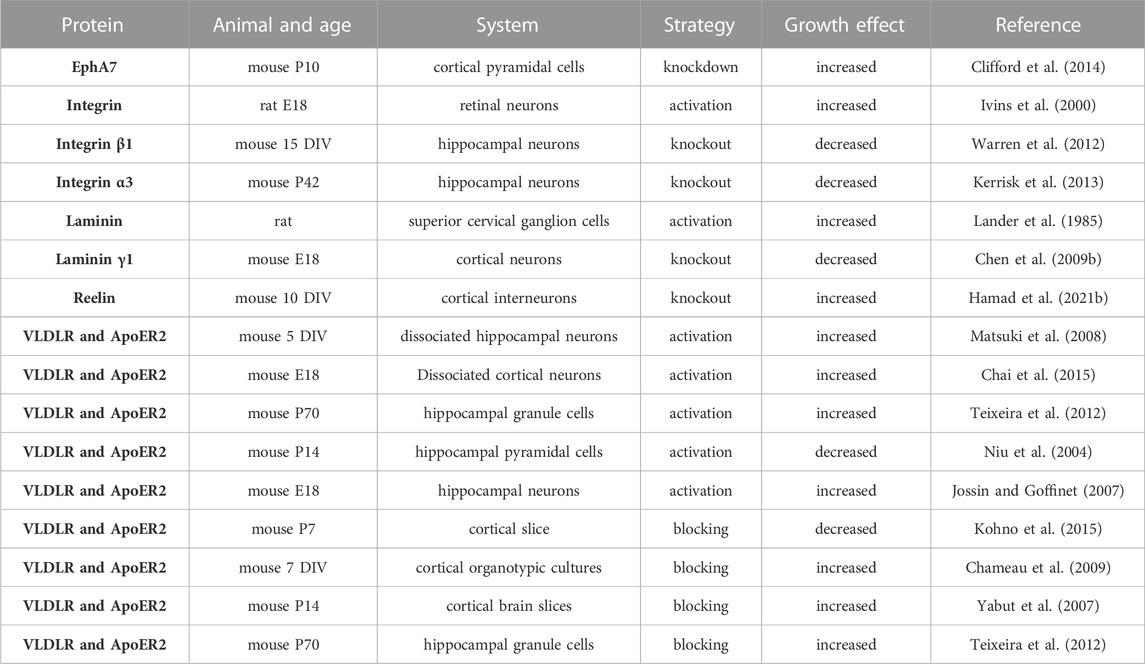
TABLE 3. Summary of the studies on the role of ECM proteins and their receptors in dendritic growth. Days in vitro (DIV). Postnatal day (P).
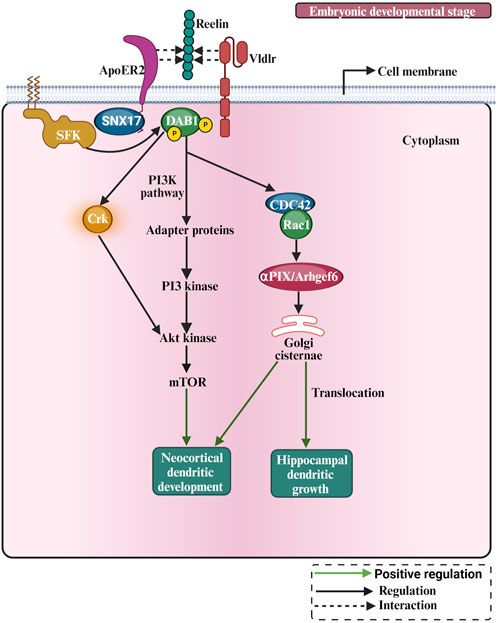
FIGURE 6. The pictorial representation of reelin involved in dendritic growth and development. Reelin is involved in cellular pathways such as the PI3K pathway. During early postnatal development, the Crk adaptor proteins and the PI3K-Akt-mTOR pathway contribute to Reelin activity by promoting protein translation, and dendrite outgrowth.
2.4 Contact-mediated ligands
2.4.1 Cadherins and protocadherins
Cadherins are transmembrane proteins that mediate cell–cell adhesion. Cadherins play a crucial role in tissue morphogenesis and homeostasis by regulating contact formation and stability. Several cadherin/protocadherins have been implicated in dendrite growth. The studies on the role of cadherins and protocadherins in dendritic growth are summarised in Table 4. For instance, conditionally knocking out the γ-protocadherins (γ-pcdhs) in adult olfactory bulb granule cells decreased dendritic growth (Ledderose et al., 2013), as they control cortical dendrite arborization by regulating the activity of a FAK/PKC/MARCKS signalling pathway (Garrett et al., 2012; see also Figure 7). Eliminating Celsr2 seven-pass transmembrane cadherins decreased dendritic growth in neocortical pyramidal cells (Shima et al., 2007; see also Figure 7). The effect of Celsr2 on dendritic growth depends on the activation of CaMKIIβ, which promotes dendritic growth. Furthermore, eliminating Celsr3 seven-pass transmembrane cadherins in neocortical pyramidal neurons increased dendritic growth (Shima et al., 2007). The effect of Celsr3 on dendritic growth depends on calcineurin activation. Furthermore, γ-Pcdhs act locally to promote dendrite arborization via homophilic matching, and that depends on molecular interactions between neurons and between neurons and astrocytes (Molumby et al., 2016). Moreover, retinal amacrine cells (ACs) project primary dendrites into a discrete synaptic layer called the inner plexiform layer and rarely extend processes into other retinal layers. The atypical cadherin Fat3 ensures that ACs develop this unipolar morphology (Deans et al., 2011; see also Figure 7). AC precursors are initially multipolar but lose neurites as they migrate through the neuroblastic layer. In cadherin fat3 mutants, dendritic growth is enhanced (Deans et al., 2011; Krol et al., 2016). These studies suggest that cadherins and protocadherins are critical in dendritic development.
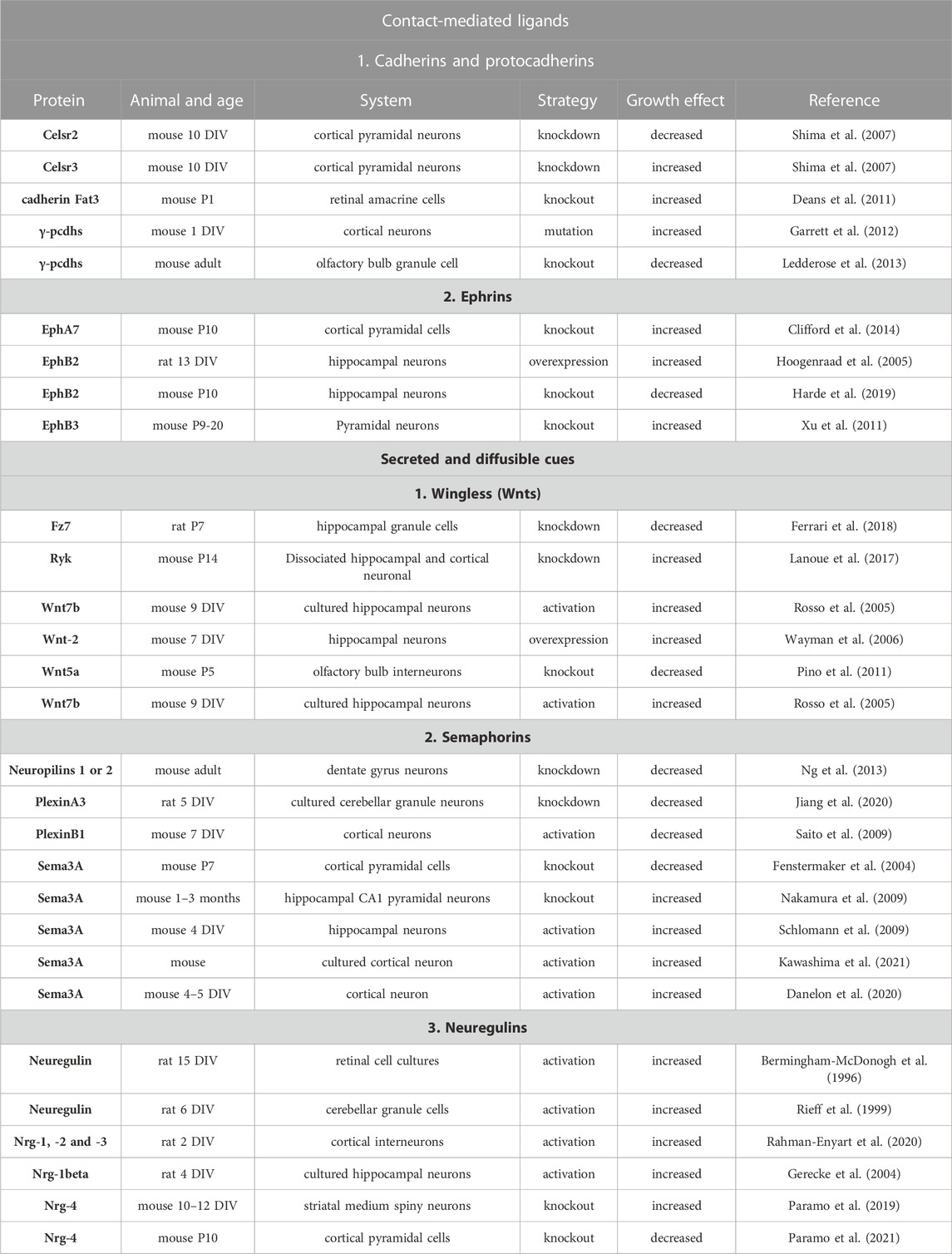
TABLE 4. Summary of the studies on the role of contact-mediated ligands and secreted and diffusible cues, and their receptors in dendritic growth. Days in vitro (DIV). Postnatal day (P).
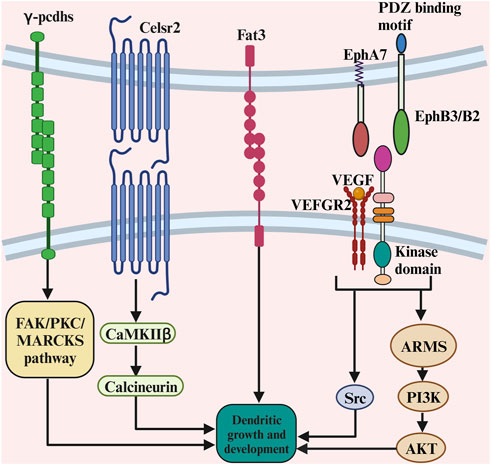
FIGURE 7. The complex role of contact-mediated ligands and its receptors in dendritic growth. The γ-protocadherins control cortical dendrite arborization by regulating the activity of a FAK/PKC/MARCKS signalling pathway. The effect of Celsr2 on dendritic growth depends on the activation of CaMKIIβ, which promotes dendritic growth. The ephrin-B3, functions postsynaptically as a receptor to transduce reverse signals into developing dendrites of mouse hippocampal neurons. The PI3K and Akt-mediated signalling pathway are crucial for ARMS-dependent dendrite development. There is a functional crosstalk of VEGFR2 and ephrinB2 in vivo to control dendritic growth, as VEGF activates the signalling partner of the Src family kinases to promote dendritic growth.
2.4.2 Ephrins
The Eph receptors represent mammals largest known family of receptor tyrosine kinases. These receptors are critical for a variety of normal cellular processes during development. The studies on the role of ephrins in dendritic growth are summarised in Table 4. It has been shown that hippocampal neurons showed a reduced dendritic size in EphB receptor (EphBR) knockout mice (Hoogenraad et al., 2005). A possible explanation is that the extracellular domain of EphBR is involved in the clustering and functional enhancement of NMDA receptor expression, which plays a role in dendritic development (Grunwald et al., 2001). Moreover, cortical pyramidal cells showed increased dendritic size in EphA7 knockout mice compared to wild-type mice (Clifford et al., 2014). Furthermore, the ephrin-B3, a transmembrane ligand for Eph receptors, functions postsynaptically as a receptor to transduce reverse signals into developing dendrites of mouse hippocampal neurons (Figure 7). Both tyrosine phosphorylation-dependent GRB4 SH2/SH3 adaptor-mediated signals and PSD-95-discs large-zona occludens-1 (PDZ) domain-dependent signals are required for inhibition of dendrite branching (Xu et al., 2011). Furthermore, suppressing ankyrin repeat-rich membrane-spanning protein (ARMS) using in utero electroporation reduced cortical neurons dendritic growth, as ankyrin is required for neurotrophin and ephrin receptor-dependent dendrite development (Chen et al., 2012). The PI3K and Akt-mediated signalling pathways are crucial for ARMS-dependent dendrite development (Figure 7). Recently, it has been shown that there is a functional crosstalk of VEGFR2 and ephrinB2 in vivo to control dendritic arborization (Harde et al., 2019). Hippocampal pyramidal cells showed reduced dendritic growth in the vascular endothelial growth factor receptor 2 (VEGFR2) knockout mice (Harde et al., 2019), as VEGF activates the signalling partner of the Src family kinases to promote dendritic growth (Figure 7). Taken together, these studies suggest the role of ephrins in dendritic growth.
2.5 Secreted and diffusible cues
2.5.1 Wingless (Wnts)
Wingless/Wnts are signalling molecules which play a role in dendritic development. WNTs signal through their Frizzled (FZ) receptors to be able to activate the cytoplasmic scaffolding protein Dishevelled (DVL). WNTs can also signal through the tyrosine kinase-related receptor RYK, which interacts with FZ and DVL (Figure 8). Downstream of DVL, the WNT pathway diverges into at least three branches: the canonical or WNT/β-catenin pathway (Figure 8), the planar cell polarity (PCP) pathway and the WNT/Ca2+ pathway (Ciani and Salinas, 2005). The studies on the role of Wnts in dendritic growth are summarised in Table 4. Eliminating the Wnt Ryk receptor in dissociated hippocampal and cortical neurons also increased dendritic growth (Lanoue et al., 2017). Ryk is known to signal through the PCP pathway, and the depletion of Ryk expands the dendritic tree. Furthermore, when overexpressed, the Wnt5a-Frizzled4 signalling pathway has increased the dendritic growth of the developing hippocampal neurons. The growth effect of Fzd4 was mediated by the downstream signalling cascade of the distal PDZ-binding motif of Fzd4 (Bian et al., 2015). The elimination of Frizzled-7 (Fz7) reduced the dendritic growth of granule cells (Ferrari et al., 2018). The Fz7 induces the phosphorylation of CaMKIIβ, which mediates dendritic growth. Furthermore, Wnt7b, expressed in the mouse hippocampus, increases dendritic branching in cultured hippocampal neurons through Dishevelled, Rac and JNK (Rosso et al., 2005). Moreover, Wnt-2 increased dendritic growth and branching of primary hippocampal neurons (Wayman et al., 2006). Finally, the non-canonical Wnt pathway induced by Wnt5a is important for the morphological development of olfactory bulb interneurons both in vitro and in vivo (Pino et al., 2011). Together. these studies suggest that the Wnt signalling molecules are important for dendritic development.
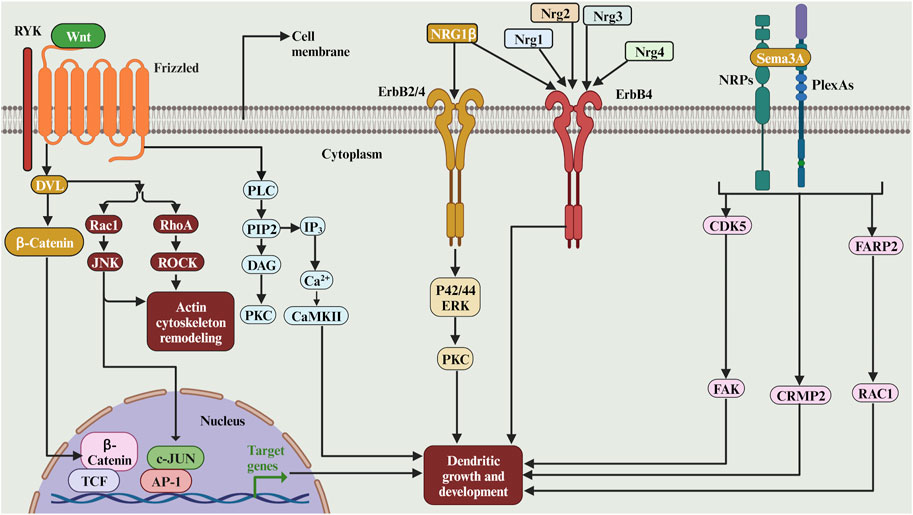
FIGURE 8. The complex role of secreted and diffusible cues and its receptors in dendritic growth. WNTs can signal through the tyrosine kinase-related receptor RYK, which interacts with FZ and DVL. Downstream of DVL, the WNT pathway diverges into at least three branches: the canonical or WNT/β-catenin pathway, the planar cell polarity (PCP) pathway and the WNT/Ca2+ pathway. The Sema3A facilitate the initial growth of CA1 apical dendrites via the activation of p35/Cdk5, which may in turn signal hippocampal development. The Sema3A regulates basal pyramidal dendritic length on cortical neurons via Sema3A-Nrp1/PlxnA4/FARP2/Rac1 signalling pathway that specifically controls dendritic morphogenesis. The neuregulin NRG-1beta stimulates hippocampal dendrite extension and arborization via a signalling pathway that involves erbB membrane tyrosine kinases (erbB2 and/or erbB4), p42/44 ERK, and PKC. The Nrg4 binds to its receptor ErbB4 and promotes dendritic growth of the striatal medium spiny neurons. In cortical GABAergic inhibitory interneurons, the neuregulins 1, 2, and 3 promote early dendritic growth in ErbB4-expressing cells.
2.5.2 Semaphorins
Semaphorins comprise a large family of conserved proteins that act as guidance cues and dendritic development and guidance in many organisms. The studies on the role of semaphorins in dendritic growth are summarised in (Table 4). Semaphorin 3A (Sema3A), a class III semaphorin, has been shown to regulate the radial orientation of pyramidal neurons in the developing neocortex (Figure 8). It is implicated in the elaborating second and third-order dendritic branches in pyramidal neurons in the neocortex (Fenstermaker et al., 2004). In Sema3A mutant mice, atypical proximal bifurcation of CA1 pyramidal apical dendrites was increased (Nakamura et al., 2009). The proximal bifurcation of CA1 pyramidal neurons was also increased in the mutant mice of p35, an activator of cyclin-dependent kinase 5 (Cdk5), suggesting that Sema3A may facilitate the initial growth of CA1 apical dendrites via the activation of p35/Cdk5, which may in turn signal hippocampal development (Nakamura et al., 2009). Interestingly, the Sema3A controls cortical development through remote signalling. It has been proposed that retrograde Sema3A signalling regulates the glutamate receptor localization through the trafficking of AMPAR GluA2 along dendrites; this remote signalling may be an alternative mechanism to local adhesive contacts for neural network formation (Goshima et al., 2016). Moreover, the neutralisation of plexin-B1, the semaphorin 4D (Sema4D) receptor, with an antibody resulted in a reduction in the dendritic growth of dissociated neocortical neurons (Saito et al., 2009) since Plexin B1, which is a dual functional GAP for R-Ras and M-Ras, remodels dendrite morphology. Moreover, silencing of semaphorin receptors neuropilins (NRP) 1 or 2 in neural progenitors at the adult mouse dentate gyrus resulted in newly differentiated neurons with shorter dendrites and simpler branching in vivo through the activation of Cdk5-FAK signalling pathway (Ng et al., 2013). Moreover, Sema3A promotes the extension of hippocampal dendrites by a pathway that requires focal adhesion kinase (FAK). The stimulation of dendrite growth and FAK phosphorylation by Sema3A depends on integrin engagement (Schlomann et al., 2009). Moreover, the Sema3A-regulated dendritic development of cortical pyramidal neurons required phosphorylation of collapsin response mediator proteins (CRMP1) by Fyn is an essential step (Kawashima et al., 2021). Furthermore, Sema3A regulates basal pyramidal dendritic length on cortical neurons via Sema3A-Nrp1/PlxnA4/FARP2/Rac1 signalling pathway that specifically controls dendritic morphogenesis (Danelon et al., 2020; see also Figure 8). Moreover, Sema3A signalling during dendritic growth in cerebellar granule neurons requires the PlexinA3/CRMP2 pathway (Jiang et al., 2020, see also Figure 8). Taken together, these studies suggest the role of the Sema3A in modulating dendritic architecture in early development.
2.5.3 Neuregulins
Neuregulins are a family of four structurally related proteins making part of the EGF family. These neuregulins have been shown to have multiple functions during the development of the nervous system. Many studies have shown that neuregulins can regulate dendritic development. The studies on the role of neuregulins in dendritic growth are summarised in (Table 4). For instance, in rat retinal cell cultures, when added to cultures of embryonic or neonatal rat retinal cells, neuregulin (rhGGF2) promotes survival and neurite extension from retinal neurons in a dose-dependent manner (Bermingham-McDonogh et al., 1996). Moreover, the neuregulin Ig-NRG plays a crucial role in cerebellar granule cell dendrite outgrowth by increasing the expression of the GABAAR beta2 subunit (Rieff et al., 1999). Furthermore, it has been shown that the neuregulin NRG-1beta stimulates hippocampal dendrite extension and arborization via a signalling pathway that involves erbB membrane tyrosine kinases (erbB2 and/or erbB4), p42/44 ERK, and PKC (Gerecke et al., 2004; see also Figure 8). Moreover, it has been shown that neuregulin-4 (Nrg4) binds to its receptor ErbB4 and promotes dendritic growth of the striatal medium spiny neurons (Paramo et al., 2019). Indeed, in the motor cortex, the Nrg4 is required to maintain the pyramidal cells soma size (Paramo et al., 2021). Finally, in cortical GABAergic inhibitory interneurons, the neuregulins 1, 2, and 3 promote early dendritic growth in ErbB4-expressing cells (Rahman-Enyart et al., 2020; see also Figure 8). Thus, the neuregulin plays a key role in early dendritic development.
3 Conclusion and perspectives
Over the past 3 decades, studies of dendrite development and its associated signalling cascade mechanisms that regulate dendrite outgrowth have enormously expanded. We have focused in this review on receptor control of dendrite development. Most of these studies have been performed in an early developmental time window because dendrites in early development undergo massive growth and retraction. In early development, the neuronal network starts to mature and become spontaneously active, which coincides with the period of rapid dendritic growth dependent on Ca2+ signalling (Garaschuk et al., 2000; Ben-Ari, 2001). Moreover, in the early developmental stage, neurons start to express a subset of receptors to facilitate neurone-neurone contact and to promote the activation of a certain signal cascade, which may be an important signal for neuronal differentiation. The outcome of the overexpression of receptors studies has been inconclusive. Some studies reported increased dendritic growth, while others reported a suppression in dendritic growth. Ca2+ permeability is a crucial factor in promoting dendritic growth. In fact, most of the overexpressed Ca2+-impermeable glutamate receptors did not alter dendritic morphology, indicating that Ca2+ signalling is indispensable for dendritic development. Depending on the activated signal cascade downstream of the receptor, the dendrite might grow or stop growing. Thus, overexpressing or activating a certain receptor is not guaranteed for neurons to grow unless that receptor is coupled to a growth-promoting signal cascade.
Similarly, eliminating or inhibiting certain receptors does not imply that the cell will stop growing unless that receptor is coupled to a growth-promoting signal cascade. Neuronal hypotrophy will not allow neurons to establish and receive enough contact from neighbouring cells, resulting in poor synaptic transmission. On the other hand, hypertrophic dendrites are also pathological. Neuronal hypertrophy can lead to pathological hyperexcitability of neuronal circuits, which can contribute to disorders such as epilepsy, schizophrenia or bipolar disorder, autism spectrum disorder (Courchesne and Pierce, 2005; Ishii et al., 2016; Hu et al., 2017). Therefore, it is necessary to have ‘stop growth signals’ to transform dynamic, immature dendrites into more stable mature dendrites and establish balanced neuronal network connectivity. Any disruption of the dendritic development process can contribute to neurological disorders associated with cognitive deficits. Exploring the mechanisms that regulate dendritic growth will pave the way for future studies to improve understanding of neurological diseases.
Author contributions
MH: Conceptualization, Funding acquisition, Supervision, Visualization, Writing–original draft, Writing–review and editing. BE: Conceptualization, Writing–review and editing. KK: Writing–review and editing. MI: Writing–original draft. BA: Writing–review and editing. MB: Conceptualization, Writing–review and editing.
Funding
The author(s) declare financial support was received for the research, authorship, and/or publication of this article. This work was supported by the United Arab Emirates University startup grant numbers 12M142 and 31M525 to MH.
Conflict of interest
The authors declare that the research was conducted in the absence of any commercial or financial relationships that could be construed as a potential conflict of interest.
Publisher’s note
All claims expressed in this article are solely those of the authors and do not necessarily represent those of their affiliated organizations, or those of the publisher, the editors and the reviewers. Any product that may be evaluated in this article, or claim that may be made by its manufacturer, is not guaranteed or endorsed by the publisher.
References
Agrawal, L., Vimal, S. K., and Shiga, T. (2019). Role of serotonin 4 receptor in the growth of hippocampal neurons during the embryonic development in mice. Neuropharmacology 158, 107712. doi:10.1016/j.neuropharm.2019.107712
Alsina, F. C., Hita, F. J., Fontanet, P. A., Irala, D., Hedman, H., Ledda, F., et al. (2016). Lrig1 is a cell-intrinsic modulator of hippocampal dendrite complexity and BDNF signaling. Rep 17, 601–616. doi:10.15252/embr.201541218
Amaral, M. D., and Pozzo-Miller, L. (2007). TRPC3 channels are necessary for brain-derived neurotrophic factor to activate a nonselective cationic current and to induce dendritic spine formation. J Neurosci 27, 5179–5189. doi:10.1523/JNEUROSCI.5499-06.2007
Bahry, J. A., Fedder-Semmes, K. N., Sceniak, M. P., and Sabo, S. L. (2021). An utism-ssociated de novo utation in GluN2B estabilizes rowing endrites by romoting etraction and runing. Front Neurosci 15, 692232. doi:10.3389/fncel.2021.692232
Baker, R. E., Wolters, P., and van Pelt, J. (1997). Chronic blockade of glutamate-mediated bioelectric activity in long-term organotypic neocortical explants differentially effects pyramidal/non-pyramidal dendritic morphology. Res Dev Res 104, 31–39. doi:10.1016/s0165-3806(97)00133-8
Baldelli, P., Novara, M., Carabelli, V., Hernández-Guijo, J. M., and Carbone, E. (2002). BDNF up-regulates evoked GABAergic transmission in developing hippocampus by potentiating presynaptic N- and P/Q-type Ca2+ channels signalling. Eur J Neurosci 16, 2297–2310. doi:10.1046/j.1460-9568.2002.02313.x
Ballester-Rosado, C. J., Albright, M. J., Wu, C.-S., Liao, C.-C., Zhu, J., Xu, J., et al. (2010). mGluR5 in cortical excitatory neurons exerts both cell-autonomous and -nonautonomous influences on cortical somatosensory circuit formation. J Neurosci 30, 16896–16909. doi:10.1523/JNEUROSCI.2462-10.2010
Baloyannis, S. J., Karakatsanis, K., Karathanasis, J., Apostolakis, M., and Diacoyannis, A. (1983). Effects of GABA, glycine, and sodium barbiturate on dendritic growth in vitro. Acta Neuropathol 59, 171–182. doi:10.1007/BF00703201
Barbin, G., Pollard, H., Gaïarsa, J. L., and Ben-Ari, Y. (1993). Involvement of GABAA receptors in the outgrowth of cultured hippocampal neurons. Neurosci Lett 152, 150–154. doi:10.1016/0304-3940(93)90505-f
Barnes, A. P., and Polleux, F. (2009). Establishment of axon-dendrite polarity in developing neurons. Annu Rev Neurosci 32, 347–381. doi:10.1146/annurev.neuro.31.060407.125536
Ben-Ari, Y. (2001). Developing networks play a similar melody. Trends Neurosci 24, 353–360. doi:10.1016/s0166-2236(00)01813-0
Ben-Ari, Y., Gaiarsa, J.-L., Tyzio, R., and Khazipov, R. (2007). GABA: a pioneer transmitter that excites immature neurons and generates primitive oscillations. Physiol Rev 87, 1215–1284. doi:10.1152/physrev.00017.2006
Bermingham-McDonogh, O., McCabe, K. L., and Reh, T. A. (1996). Effects of GGF/neuregulins on neuronal survival and neurite outgrowth correlate with erbB2/neu expression in developing rat retina. Development 122, 1427–1438. doi:10.1242/dev.122.5.1427
Bian, W.-J., Miao, W.-Y., He, S.-J., Wan, Z.-F., Luo, Z.-G., and Yu, X. (2015). A novel Wnt5a-Frizzled4 signaling pathway mediates activity-independent dendrite morphogenesis via the distal PDZ motif of Frizzled 4. Dev Neurobiol 75, 805–822. doi:10.1002/dneu.22250
Blaesse, P., Airaksinen, M. S., Rivera, C., and Kaila, K. (2009). Cation-chloride cotransporters and neuronal function. Neuron 61, 820–838. doi:10.1016/j.neuron.2009.03.003
Bock, H. H., and May, P. (2016). Canonical and on-canonical eelin ignaling. Front Neurosci 10, 166. doi:10.3389/fncel.2016.00166
Bony, G., Szczurkowska, J., Tamagno, I., Shelly, M., Contestabile, A., and Cancedda, L. (2013). Non-hyperpolarizing GABAB receptor activation regulates neuronal migration and neurite growth and specification by cAMP/LKB1. Nat Commun 4, 1800. doi:10.1038/ncomms2820
Borodinsky, L. N., O’Leary, D., Neale, J. H., Vicini, S., Coso, O. A., and Fiszman, M. L. (2003). GABA-induced neurite outgrowth of cerebellar granule cells is mediated by GABA(A) receptor activation, calcium influx and CaMKII and erk1/2 pathways. J Neurochem 84, 1411–1420. doi:10.1046/j.1471-4159.2003.01638.x
Burnashev, N., Monyer, H., Seeburg, P. H., and Sakmann, B. (1992). Divalent ion permeability of AMPA receptor channels is dominated by the edited form of a single subunit. Neuron 8, 189–198. doi:10.1016/0896-6273(92)90120-3
Caviness, V. S. (1976). Patterns of cell and fiber distribution in the neocortex of the reeler mutant mouse. J Comp Neurol 170, 435–447. doi:10.1002/cne.901700404
Chai, X., Fan, L., Shao, H., Lu, X., Zhang, W., Li, J., et al. (2015). Reelin nduces ranching of eurons and adial lial ells during orticogenesis. Cereb25, 3640–3653. doi:10.1093/cercor/bhu216
Chameau, P., Inta, D., Vitalis, T., Monyer, H., Wadman, W. J., and van Hooft, J. A. (2009). The N-terminal region of reelin regulates postnatal dendritic maturation of cortical pyramidal neurons. Proc Natl Acad Sci U A 106, 7227–7232. doi:10.1073/pnas.0810764106
Chandía-Cristi, A., Stuardo, N., Trejos, C., Leal, N., Urrutia, D., Bronfman, F. C., et al. (2023). c-Abl yrosine inase s equired for BDNF-nduced endritic ranching and rowth. Int J Mol Sci 24, 1944. doi:10.3390/ijms24031944
Chen, W., Prithviraj, R., Mahnke, A. H., McGloin, K. E., Tan, J. W., Gooch, A. K., et al. (2009a). AMPA glutamate receptor subunits 1 and 2 regulate dendrite complexity and spine motility in neurons of the developing neocortex. Neuroscience 159, 172–182. doi:10.1016/j.neuroscience.2008.11.038
Chen, Y., Fu, W.-Y., Ip, J. P. K., Ye, T., Fu, A. K. Y., Chao, M. V., et al. (2012). Ankyrin repeat-rich membrane spanning protein (kidins220) is required for neurotrophin and ephrin receptor-dependent dendrite development. J Neurosci 32, 8263–8269. doi:10.1523/JNEUROSCI.1264-12.2012
Chen, Z.-L., Haegeli, V., Yu, H., and Strickland, S. (2009b). Cortical deficiency of laminin gamma1 impairs the AKT/GSK-3beta signaling pathway and leads to defects in neurite outgrowth and neuronal migration. Dev Biol 327, 158–168. doi:10.1016/j.ydbio.2008.12.006
Cheung, Z. H., Chin, W. H., Chen, Y., Ng, Y. P., and Ip, N. Y. (2007). Cdk5 is involved in BDNF-stimulated dendritic growth in hippocampal neurons. Biol 5, e63. doi:10.1371/journal.pbio.0050063
Ciani, L., and Salinas, P. C. (2005). WNTs in the vertebrate nervous system: from patterning to neuronal connectivity. Nat Rev Neurosci 6, 351–362. doi:10.1038/nrn1665
Clifford, M. A., Athar, W., Leonard, C. E., Russo, A., Sampognaro, P. J., Van der Goes, M.-S., et al. (2014). EphA7 signaling guides cortical dendritic development and spine maturation. Proc Natl Acad Sci U A 111, 4994–4999. doi:10.1073/pnas.1323793111
Cline, H. (2001). Dendritic arbor development and synaptogenesis. Curr Opin Neurobiol 11, 118–126. doi:10.1016/s0959-4388(00)00182-3
Coleman, P. D., and Riesen, A. H. (1968). Evironmental effects on cortical dendritic fields. I. Rearing in the dark. J Anat 102, 363–374.
Courchesne, E., and Pierce, K. (2005). Brain overgrowth in autism during a critical time in development: implications for frontal pyramidal neuron and interneuron development and connectivity. Int J Dev Neurosci 23, 153–170. doi:10.1016/j.ijdevneu.2005.01.003
Courter, L. A., Shaffo, F. C., Ghogha, A., Parrish, D. J., Lorentz, C. U., Habecker, B. A., et al. (2016). BMP7-induced dendritic growth in sympathetic neurons requires p75(NTR) signaling. Dev Neurobiol 76, 1003–1013. doi:10.1002/dneu.22371
Cruz-Martín, A., Crespo, M., and Portera-Cailliau, C. (2012). Glutamate induces the elongation of early dendritic protrusions via mGluRs in wild type mice, but not in fragile X mice. PLoS One 7, e32446. doi:10.1371/journal.pone.0032446
Danelon, V., Goldner, R., Martinez, E., Gokhman, I., Wang, K., Yaron, A., et al. (2020). Modular and istinct lexin-A4/FARP2/ac1 ignaling ontrols endrite orphogenesis. J Neurosci 40, 5413–5430. doi:10.1523/JNEUROSCI.2730-19.2020
D’Arcangelo, G., Homayouni, R., Keshvara, L., Rice, D. S., Sheldon, M., and Curran, T. (1999). Reelin is a ligand for lipoprotein receptors. Neuron 24, 471–479. doi:10.1016/s0896-6273(00)80860-0
Deans, M. R., Krol, A., Abraira, V. E., Copley, C. O., Tucker, A. F., and Goodrich, L. V. (2011). Control of neuronal morphology by the atypical cadherin Fat3. Neuron 71, 820–832. doi:10.1016/j.neuron.2011.06.026
Dijkhuizen, P. A., and Ghosh, A. (2005). BDNF regulates primary dendrite formation in cortical neurons via the PI3-kinase and MAP kinase signaling pathways. J Neurobiol 62, 278–288. doi:10.1002/neu.20100
Dotti, C. G., and Banker, G. A. (1987). Experimentally induced alteration in the polarity of developing neurons. Nature 330, 254–256. doi:10.1038/330254a0
Dotti, C. G., Sullivan, C. A., and Banker, G. A. (1988). The establishment of polarity by hippocampal neurons in culture. J Neurosci 8, 1454–1468. doi:10.1523/JNEUROSCI.08-04-01454.1988
Dudok, J. J., Groffen, A. J. A., Witter, M. P., Voorn, P., and Verhage, M. (2009). Chronic activation of the 5-HT(2) receptor reduces 5-HT neurite density as studied in organotypic slice cultures. Res 1302, 1–9. doi:10.1016/j.brainres.2009.08.071
Espinosa, J. S., Wheeler, D. G., Tsien, R. W., and Luo, L. (2009). Uncoupling dendrite growth and patterning: single-cell knockout analysis of NMDA receptor 2B. Neuron 62, 205–217. doi:10.1016/j.neuron.2009.03.006
Fenstermaker, V., Chen, Y., Ghosh, A., and Yuste, R. (2004). Regulation of dendritic length and branching by semaphorin 3A. J Neurobiol 58, 403–412. doi:10.1002/neu.10304
Ferrari, M. E., Bernis, M. E., McLeod, F., Podpolny, M., Coullery, R. P., Casadei, I. M., et al. (2018). Wnt7b signalling through Frizzled-7 receptor promotes dendrite development by coactivating CaMKII and JNK. J Sci 131, jcs216101. doi:10.1242/jcs.216101
Ferreira, T. A., Iacono, L. L., and Gross, C. T. (2010). Serotonin receptor 1A modulates actin dynamics and restricts dendritic growth in hippocampal neurons. Eur J Neurosci 32, 18–26. doi:10.1111/j.1460-9568.2010.07283.x
Fink, C. C., Bayer, K.-U., Myers, J. W., Ferrell, J. E., Schulman, H., and Meyer, T. (2003). Selective regulation of neurite extension and synapse formation by the beta but not the alpha isoform of CaMKII. Neuron 39, 283–297. doi:10.1016/s0896-6273(03)00428-8
Förster, E., Bock, H. H., Herz, J., Chai, X., Frotscher, M., and Zhao, S. (2010). Emerging topics in Reelin function. Eur J Neurosci 31, 1511–1518. doi:10.1111/j.1460-9568.2010.07222.x
Fricker, A. D., Rios, C., Devi, L. A., and Gomes, I. (2005). Serotonin receptor activation leads to neurite outgrowth and neuronal survival. Res Mol Res 138, 228–235. doi:10.1016/j.molbrainres.2005.04.016
Garaschuk, O., Linn, J., Eilers, J., and Konnerth, A. (2000). Large-scale oscillatory calcium waves in the immature cortex. Nat Neurosci 3, 452–459. doi:10.1038/74823
Garrett, A. M., Schreiner, D., Lobas, M. A., and Weiner, J. A. (2012). γ-protocadherins control cortical dendrite arborization by regulating the activity of a FAK/PKC/MARCKS signaling pathway. Neuron 74, 269–276. doi:10.1016/j.neuron.2012.01.028
Gates, M. A., Tai, C. C., and Macklis, J. D. (2000). Neocortical neurons lacking the protein-tyrosine kinase B receptor display abnormal differentiation and process elongation in vitro and in vivo. Neuroscience 98, 437–447. doi:10.1016/s0306-4522(00)00106-8
Gehler, S., Gallo, G., Veien, E., and Letourneau, P. C. (2004). p75 neurotrophin receptor signaling regulates growth cone filopodial dynamics through modulating RhoA activity. J Neurosci 24, 4363–4372. doi:10.1523/JNEUROSCI.0404-04.2004
Gerecke, K. M., Wyss, J. M., and Carroll, S. L. (2004). Neuregulin-1beta induces neurite extension and arborization in cultured hippocampal neurons. Mol Neurosci 27, 379–393. doi:10.1016/j.mcn.2004.08.001
Gonda, S., Giesen, J., Sieberath, A., West, F., Buchholz, R., Klatt, O., et al. (2020). GluN2B but ot GluN2A for asal endritic rowth of ortical yramidal eurons. Front Neuroanat 14, 571351. doi:10.3389/fnana.2020.571351
Gonzalez, A., Moya-Alvarado, G., Gonzalez-Billaut, C., and Bronfman, F. C. (2016). Cellular and molecular mechanisms regulating neuronal growth by brain-derived neurotrophic factor. Cytoskelet73, 612–628. doi:10.1002/cm.21312
González-Gutiérrez, A., Lazo, O. M., and Bronfman, F. C. (2020). The ab5-ab11 ndosomal athway is equired for BDNF-nduced CREB ranscriptional egulation in ippocampal eurons. J Neurosci 40, 8042–8054. doi:10.1523/JNEUROSCI.2063-19.2020
Goshima, Y., Yamashita, N., Nakamura, F., and Sasaki, Y. (2016). Regulation of dendritic development by semaphorin 3A through novel intracellular remote signaling. Migr 10, 627–640. doi:10.1080/19336918.2016.1210758
Grueber, W. B., and Sagasti, A. (2010). Self-avoidance and tiling: echanisms of dendrite and axon spacing. Spring Harb Perspect Biol 2, a001750. doi:10.1101/cshperspect.a001750
Grunwald, I. C., Korte, M., Wolfer, D., Wilkinson, G. A., Unsicker, K., Lipp, H. P., et al. (2001). Kinase-independent requirement of EphB2 receptors in hippocampal synaptic plasticity. Neuron 32, 1027–1040. doi:10.1016/s0896-6273(01)00550-5
Haas, K., Li, J., and Cline, H. T. (2006). AMPA receptors regulate experience-dependent dendritic arbor growth in vivo. Proc Natl Acad Sci U A 103, 12127–12131. doi:10.1073/pnas.0602670103
Hamad, M. I. K., Jack, A., Klatt, O., Lorkowski, M., Strasdeit, T., Kott, S., et al. (2014). Type I TARPs promote dendritic growth of early postnatal neocortical pyramidal cells in organotypic cultures. Development 141, 1737–1748. doi:10.1242/dev.099697
Hamad, M. I. K., Jbara, A., Rabaya, O., Petrova, P., Daoud, S., Melliti, N., et al. (2021a). Reelin signaling modulates GABAB receptor function in the neocortex. J Neurochem 156, 589–603. doi:10.1111/jnc.14990
Hamad, M. I. K., Ma-Högemeier, Z.-L., Riedel, C., Conrads, C., Veitinger, T., Habijan, T., et al. (2011). Cell class-specific regulation of neocortical dendrite and spine growth by AMPA receptor splice and editing variants. Development 138, 4301–4313. doi:10.1242/dev.071076
Hamad, M. I. K., Petrova, P., Daoud, S., Rabaya, O., Jbara, A., Melliti, N., et al. (2021b). Reelin restricts dendritic growth of interneurons in the neocortex. Development 148, dev199718. doi:10.1242/dev.199718
Hansen, G. H., Meier, E., and Schousboe, A. (1984). GABA influences the ultrastructure composition of cerebellar granule cells during development in culture. Int J Dev Neurosci 2, 247–257. doi:10.1016/0736-5748(84)90019-4
Harde, E., Nicholson, L., Furones Cuadrado, B., Bissen, D., Wigge, S., Urban, S., et al. (2019). EphrinB2 regulates VEGFR2 during dendritogenesis and hippocampal circuitry development. Elife 8, e49819. doi:10.7554/eLife.49819
He, Z., Jia, C., Feng, S., Zhou, K., Tai, Y., Bai, X., et al. (2012). TRPC5 channel is the mediator of neurotrophin-3 in regulating dendritic growth via CaMKIIα in rat hippocampal neurons. J Neurosci 32, 9383–9395. doi:10.1523/JNEUROSCI.6363-11.2012
Hedrick, N. G., Harward, S. C., Hall, C. E., Murakoshi, H., McNamara, J. O., and Yasuda, R. (2016). Rho GTPase complementation underlies BDNF-dependent homo- and heterosynaptic plasticity. Nature 538, 104–108. doi:10.1038/nature19784
Heisler, L. K., Chu, H. M., Brennan, T. J., Danao, J. A., Bajwa, P., Parsons, L. H., et al. (1998). Elevated anxiety and antidepressant-like responses in serotonin 5-HT1A receptor mutant mice. Proc Natl Acad Sci U A 95, 15049–15054. doi:10.1073/pnas.95.25.15049
Homma, K., Kitamura, Y., Ogawa, H., and Oka, K. (2006). Serotonin induces the increase in intracellular Ca2+ that enhances neurite outgrowth in PC12 cells via activation of 5-HT3 receptors and voltage-gated calcium channels. J Neurosci Res 84, 316–325. doi:10.1002/jnr.20894
Hoogenraad, C. C., Milstein, A. D., Ethell, I. M., Henkemeyer, M., and Sheng, M. (2005). GRIP1 controls dendrite morphogenesis by regulating EphB receptor trafficking. Nat Neurosci 8, 906–915. doi:10.1038/nn1487
Horch, H. W., and Katz, L. C. (2002). BDNF release from single cells elicits local dendritic growth in nearby neurons. Nat Neurosci 5, 1177–1184. doi:10.1038/nn927
Horch, H. W., Krüttgen, A., Portbury, S. D., and Katz, L. C. (1999). Destabilization of cortical dendrites and spines by BDNF. Neuron 23, 353–364. doi:10.1016/s0896-6273(00)80785-0
Horton, A. C., and Ehlers, M. D. (2003). Neuronal polarity and trafficking. Neuron 40, 277–295. doi:10.1016/s0896-6273(03)00629-9
Hu, J. S., Vogt, D., Sandberg, M., and Rubenstein, J. L. (2017). Cortical interneuron development: a tale of time and space. Development 144, 3867–3878. doi:10.1242/dev.132852
Inglis, F. M., Crockett, R., Korada, S., Abraham, W. C., Hollmann, M., and Kalb, R. G. (2002). The AMPA receptor subunit GluR1 regulates dendritic architecture of motor neurons. J Neurosci 22, 8042–8051. doi:10.1523/JNEUROSCI.22-18-08042.2002
Inglis, F. M., Furia, F., Zuckerman, K. E., Strittmatter, S. M., and Kalb, R. G. (1998). The role of nitric oxide and NMDA receptors in the development of motor neuron dendrites. J Neurosci 18, 10493–10501. doi:10.1523/JNEUROSCI.18-24-10493.1998
Ishii, K., Kubo, K.-I., and Nakajima, K. (2016). Reelin and europsychiatric isorders. Front Neurosci 10, 229. doi:10.3389/fncel.2016.00229
Ivins, J. K., Yurchenco, P. D., and Lander, A. D. (2000). Regulation of neurite outgrowth by integrin activation. J Neurosci 20, 6551–6560. doi:10.1523/JNEUROSCI.20-17-06551.2000
Jack, A., Hamad, M. I. K., Gonda, S., Gralla, S., Pahl, S., Hollmann, M., et al. (2019). Development of ortical yramidal ell and nterneuronal endrites: a ole for ainate eceptor ubunits and NETO1. Mol Neurobiol 56, 4960–4979. doi:10.1007/s12035-018-1414-0
Jagasia, R., Steib, K., Englberger, E., Herold, S., Faus-Kessler, T., Saxe, M., et al. (2009). GABA-cAMP response element-binding protein signaling regulates maturation and survival of newly generated neurons in the adult hippocampus. J Neurosci 29, 7966–7977. doi:10.1523/JNEUROSCI.1054-09.2009
Jan, Y.-N., and Jan, L. Y. (2003). The control of dendrite development. Neuron 40, 229–242. doi:10.1016/s0896-6273(03)00631-7
Jaworski, J., Spangler, S., Seeburg, D. P., Hoogenraad, C. C., and Sheng, M. (2005). Control of dendritic arborization by the phosphoinositide-3’-kinase-Akt-mammalian target of rapamycin pathway. J Neurosci 25, 11300–11312. doi:10.1523/JNEUROSCI.2270-05.2005
Jeon, J.-P., Roh, S.-E., Wie, J., Kim, J., Kim, H., Lee, K.-P., et al. (2013). Activation of TRPC4β by Gαi subunit increases Ca2+ selectivity and controls neurite morphogenesis in cultured hippocampal neuron. Calcium 54, 307–319. doi:10.1016/j.ceca.2013.07.006
Jeong, G.-B., Werner, M., Gazula, V.-R., Itoh, T., Roberts, M., David, S., et al. (2006). Bi-directional control of motor neuron dendrite remodeling by the calcium permeability of AMPA receptors. Mol Neurosci 32, 299–314. doi:10.1016/j.mcn.2006.04.008
Jiang, T., Zhang, G., Liang, Y., Cai, Z., Liang, Z., Lin, H., et al. (2020). PlexinA3 nteracts with CRMP2 to ediate Sema3A ignalling uring endritic rowth in ultured erebellar ranule eurons. Neuroscience 434, 83–92. doi:10.1016/j.neuroscience.2020.02.008
Joo, W., Hippenmeyer, S., and Luo, L. (2014). Neurodevelopment. Dendrite morphogenesis depends on relative levels of NT-3/TrkC signaling. Science 346, 626–629. doi:10.1126/science.1258996
Jossin, Y. (2020). Reelin unctions, echanisms of ction and ignaling athways uring rain evelopment and aturation. Biomolecules 10, 964. doi:10.3390/biom10060964
Jossin, Y., and Goffinet, A. M. (2007). Reelin signals through phosphatidylinositol 3-kinase and Akt to control cortical development and through mTor to regulate dendritic growth. Mol Biol 27, 7113–7124. doi:10.1128/MCB.00928-07
Jossin, Y., Gui, L., and Goffinet, A. M. (2007). Processing of Reelin by embryonic neurons is important for function in tissue but not in dissociated cultured neurons. J Neurosci 27, 4243–4252. doi:10.1523/JNEUROSCI.0023-07.2007
Jossin, Y., Ignatova, N., Hiesberger, T., Herz, J., Lambert de Rouvroit, C., and Goffinet, A. M. (2004). The central fragment of Reelin, generated by proteolytic processing in vivo, is critical to its function during cortical plate development. J Neurosci 24, 514–521. doi:10.1523/JNEUROSCI.3408-03.2004
Kalb, R. G. (1994). Regulation of motor neuron dendrite growth by NMDA receptor activation. Development 120, 3063–3071. doi:10.1242/dev.120.11.3063
Kannangara, T. S., Bostrom, C. A., Ratzlaff, A., Thompson, L., Cater, R. M., Gil-Mohapel, J., et al. (2014). Deletion of the NMDA receptor GluN2A subunit significantly decreases dendritic growth in maturing dentate granule neurons. PLoS One 9, e103155. doi:10.1371/journal.pone.0103155
Katz, L. C., and Shatz, C. J. (1996). Synaptic activity and the construction of cortical circuits. Science 274, 1133–1138. doi:10.1126/science.274.5290.1133
Kawashima, T., Jitsuki-Takahashi, A., Takizawa, K., Jitsuki, S., Takahashi, T., Ohshima, T., et al. (2021). Phosphorylation of ollapsin esponse ediator rotein 1 (CRMP1) at yrosine 504 residue regulates emaphorin 3A-induced cortical dendritic growth. J Neurochem 157, 1207–1221. doi:10.1111/jnc.15304
Kerrisk, M. E., Greer, C. A., and Koleske, A. J. (2013). Integrin α3 is required for late postnatal stability of dendrite arbors, dendritic spines and synapses, and mouse behavior. J Neurosci 33, 6742–6752. doi:10.1523/JNEUROSCI.0528-13.2013
Khazipov, R., and Luhmann, H. J. (2006). Early patterns of electrical activity in the developing cerebral cortex of humans and rodents. Trends Neurosci 29, 414–418. doi:10.1016/j.tins.2006.05.007
Kirischuk, S., Sinning, A., Blanquie, O., Yang, J.-W., Luhmann, H. J., and Kilb, W. (2017). Modulation of eocortical evelopment by arly euronal ctivity: hysiology and athophysiology. Front Neurosci 11, 379. doi:10.3389/fncel.2017.00379
Kirmse, K., Kummer, M., Kovalchuk, Y., Witte, O. W., Garaschuk, O., and Holthoff, K. (2015). GABA depolarizes immature neurons and inhibits network activity in the neonatal neocortex in vivo. Nat Commun 6, 7750. doi:10.1038/ncomms8750
Kohno, S., Kohno, T., Nakano, Y., Suzuki, K., Ishii, M., Tagami, H., et al. (2009). Mechanism and significance of specific proteolytic cleavage of Reelin. Biochem Biophys Res Commun 380, 93–97. doi:10.1016/j.bbrc.2009.01.039
Kohno, T., Honda, T., Kubo, K.-I., Nakano, Y., Tsuchiya, A., Murakami, T., et al. (2015). Importance of Reelin C-terminal region in the development and maintenance of the postnatal cerebral cortex and its regulation by specific proteolysis. J Neurosci 35, 4776–4787. doi:10.1523/JNEUROSCI.4119-14.2015
Kohno, T., Ishii, K., Hirota, Y., Honda, T., Makino, M., Kawasaki, T., et al. (2020). Reelin-Nrp1 nteraction egulates eocortical endrite evelopment in a ontext-pecific anner. J Neurosci 40, 8248–8261. doi:10.1523/JNEUROSCI.1907-20.2020
Koie, M., Okumura, K., Hisanaga, A., Kamei, T., Sasaki, K., Deng, M., et al. (2014). Cleavage within Reelin repeat 3 regulates the duration and range of the signaling activity of Reelin protein. J Biol Chem 289, 12922–12930. doi:10.1074/jbc.M113.536326
Kondoh, M., Shiga, T., and Okado, N. (2004). Regulation of dendrite formation of Purkinje cells by serotonin through serotonin1A and serotonin2A receptors in culture. Neurosci Res 48, 101–109. doi:10.1016/j.neures.2003.10.001
Konur, S., and Ghosh, A. (2005). Calcium signaling and the control of dendritic development. Neuron 46, 401–405. doi:10.1016/j.neuron.2005.04.022
Krol, A., Henle, S. J., and Goodrich, L. V. (2016). Fat3 and Ena/VASP proteins influence the emergence of asymmetric cell morphology in the developing retina. Development 143, 2172–2182. doi:10.1242/dev.133678
Kumar, V., Zhang, M.-X., Swank, M. W., Kunz, J., and Wu, G.-Y. (2005). Regulation of dendritic morphogenesis by Ras-PI3K-Akt-mTOR and Ras-MAPK signaling pathways. J Neurosci 25, 11288–11299. doi:10.1523/JNEUROSCI.2284-05.2005
Kupferman, J. V., Basu, J., Russo, M. J., Guevarra, J., Cheung, S. K., and Siegelbaum, S. A. (2014). Reelin signaling specifies the molecular identity of the pyramidal neuron distal dendritic compartment. Cell 158, 1335–1347. doi:10.1016/j.cell.2014.07.035
Kwon, M., Fernández, J. R., Zegarek, G. F., Lo, S. B., and Firestein, B. L. (2011). BDNF-promoted increases in proximal dendrites occur via CREB-dependent transcriptional regulation of cypin. J Neurosci 31, 9735–9745. doi:10.1523/JNEUROSCI.6785-10.2011
Lander, A. D., Fujii, D. K., and Reichardt, L. F. (1985). Purification of a factor that promotes neurite outgrowth: isolation of laminin and associated molecules. J Biol 101, 898–913. doi:10.1083/jcb.101.3.898
Lanoue, V., Langford, M., White, A., Sempert, K., Fogg, L., and Cooper, H. M. (2017). The Wnt receptor Ryk is a negative regulator of mammalian dendrite morphogenesis. Sci Rep 7, 5965. doi:10.1038/s41598-017-06140-z
Lauterborn, J. C., Palmer, L. C., Jia, Y., Pham, D. T., Hou, B., Wang, W., et al. (2016). Chronic mpakine reatments timulate endritic rowth and romote earning in iddle-ged ats. J Neurosci 36, 1636–1646. doi:10.1523/JNEUROSCI.3157-15.2016
Lazo, O. M., Gonzalez, A., Ascaño, M., Kuruvilla, R., Couve, A., and Bronfman, F. C. (2013). BDNF regulates Rab11-mediated recycling endosome dynamics to induce dendritic branching. J Neurosci 33, 6112–6122. doi:10.1523/JNEUROSCI.4630-12.2013
Ledda, F., and Paratcha, G. (2017). Mechanisms regulating dendritic arbor patterning. Mol Life Sci 74, 4511–4537. doi:10.1007/s00018-017-2588-8
Ledderose, J., Dieter, S., and Schwarz, M. K. (2013). Maturation of postnatally generated olfactory bulb granule cells depends on functional γ-protocadherin expression. Sci Rep 3, 1514. doi:10.1038/srep01514
Lee, L.-J., Lo, F.-S., and Erzurumlu, R. S. (2005). NMDA receptor-dependent regulation of axonal and dendritic branching. J Neurosci 25, 2304–2311. doi:10.1523/JNEUROSCI.4902-04.2005
Leinekugel, X., Medina, I., Khalilov, I., Ben-Ari, Y., and Khazipov, R. (1997). Ca2+ oscillations mediated by the synergistic excitatory actions of GABA(A) and NMDA receptors in the neonatal hippocampus. Neuron 18, 243–255. doi:10.1016/s0896-6273(00)80265-2
Li, H. S., Xu, X. Z., and Montell, C. (1999). Activation of a TRPC3-dependent cation current through the neurotrophin BDNF. Neuron 24, 261–273. doi:10.1016/s0896-6273(00)80838-7
Lin, T.-Y., Chen, P.-J., Yu, H.-H., Hsu, C.-P., and Lee, C.-H. (2020). Extrinsic actors egulating endritic atterning. Front Neurosci 14, 622808. doi:10.3389/fncel.2020.622808
Lohmann, C., and Wong, R. O. L. (2005). Regulation of dendritic growth and plasticity by local and global calcium dynamics. Calcium 37, 403–409. doi:10.1016/j.ceca.2005.01.008
Long, K. R., and Huttner, W. B. (2019). How the extracellular matrix shapes neural development. Open Biol 9, 180216. doi:10.1098/rsob.180216
López-Bendito, G., Luján, R., Shigemoto, R., Ganter, P., Paulsen, O., and Molnár, Z. (2003). Blockade of GABA(B) receptors alters the tangential migration of cortical neurons. Cereb13, 932–942. doi:10.1093/cercor/13.9.932
Luhmann, H. J. (2021). Neurophysiology of the eveloping erebral ortex: hat e ave earned and hat e eed to now. Front Neurosci 15, 814012. doi:10.3389/fncel.2021.814012
Luhmann, H. J., Sinning, A., Yang, J.-W., Reyes-Puerta, V., Stüttgen, M. C., Kirischuk, S., et al. (2016). Spontaneous euronal ctivity in eveloping eocortical etworks: rom ingle ells to arge-cale nteractions. Front Neural10, 40. doi:10.3389/fncir.2016.00040
Mao, L., Tang, Q., Samdani, S., Liu, Z., and Wang, J. Q. (2004). Regulation of MAPK/ERK phosphorylation via ionotropic glutamate receptors in cultured rat striatal neurons. Eur J Neurosci 19, 1207–1216. doi:10.1111/j.1460-9568.2004.03223.x
Maric, D., Liu, Q. Y., Maric, I., Chaudry, S., Chang, Y. H., Smith, S. V., et al. (2001). GABA expression dominates neuronal lineage progression in the embryonic rat neocortex and facilitates neurite outgrowth via GABA(A) autoreceptor/Cl-channels. J Neurosci 21, 2343–2360. doi:10.1523/JNEUROSCI.21-07-02343.2001
Matsuki, T., Matthews, R. T., Cooper, J. A., van der Brug, M. P., Cookson, M. R., Hardy, J. A., et al. (2010). Reelin and stk25 have opposing roles in neuronal polarization and dendritic Golgi deployment. Cell 143, 826–836. doi:10.1016/j.cell.2010.10.029
Matsuki, T., Pramatarova, A., and Howell, B. W. (2008). Reduction of Crk and CrkL expression blocks reelin-induced dendritogenesis. J Sci 121, 1869–1875. doi:10.1242/jcs.027334
McAllister, A. (2000). Cellular and molecular mechanisms of dendrite growth. Cereb10, 963–973. doi:10.1093/cercor/10.10.963
McAllister, A. K., Katz, L. C., and Lo, D. C. (1999). Neurotrophins and synaptic plasticity. Annu Rev Neurosci 22, 295–318. doi:10.1146/annurev.neuro.22.1.295
McAllister, A. K., Lo, D. C., and Katz, L. C. (1995). Neurotrophins regulate dendritic growth in developing visual cortex. Neuron 15, 791–803. doi:10.1016/0896-6273(95)90171-x
Meseke, M., Neumüller, F., Brunne, B., Li, X., Anstötz, M., Pohlkamp, T., et al. (2018). Distal endritic nrichment of HCN1 hannels in ippocampal CA1 s romoted by strogen, but oes ot equire eelin. eNeuro 5, ENEURO.0258–18. doi:10.1523/ENEURO.0258-18.2018
Meseke, M., Rosenberger, G., and Förster, E. (2013). Reelin and the Cdc42/Rac1 guanine nucleotide exchange factor αPIX/Arhgef6 promote dendritic Golgi translocation in hippocampal neurons. Eur J Neurosci 37, 1404–1412. doi:10.1111/ejn.12153
Miyamoto, Y., Yamauchi, J., Tanoue, A., Wu, C., and Mobley, W. C. (2006). TrkB binds and tyrosine-phosphorylates Tiam1, leading to activation of Rac1 and induction of changes in cellular morphology. Proc Natl Acad Sci U A 103, 10444–10449. doi:10.1073/pnas.0603914103
Molumby, M. J., Keeler, A. B., and Weiner, J. A. (2016). Homophilic rotocadherin ell-ell nteractions romote endrite omplexity. Rep 15, 1037–1050. doi:10.1016/j.celrep.2016.03.093
Monyer, H., Seeburg, P. H., and Wisden, W. (1991). Glutamate-operated channels: developmentally early and mature forms arise by alternative splicing. Neuron 6, 799–810. doi:10.1016/0896-6273(91)90176-z
Moya-Alvarado, G., Tiburcio-Felix, R., Ibáñez, M. R., Aguirre-Soto, A. A., Guerra, M. V., Wu, C., et al. (2023). BDNF/TrkB signaling endosomes in axons coordinate CREB/mTOR activation and protein synthesis in the cell body to induce dendritic growth in cortical neurons. Elife 12, e77455. doi:10.7554/eLife.77455
Myers, J. P., Santiago-Medina, M., and Gomez, T. M. (2011). Regulation of axonal outgrowth and pathfinding by integrin-ECM interactions. Dev Neurobiol 71, 901–923. doi:10.1002/dneu.20931
Nakamura, F., Ugajin, K., Yamashita, N., Okada, T., Uchida, Y., Taniguchi, M., et al. (2009). Increased proximal bifurcation of CA1 pyramidal apical dendrites in sema3A mutant mice. J Comp Neurol 516, 360–375. doi:10.1002/cne.22125
Ng, T., Ryu, J. R., Sohn, J. H., Tan, T., Song, H., Ming, G.-L., et al. (2013). Class 3 semaphorin mediates dendrite growth in adult newborn neurons through Cdk5/FAK pathway. PLoS One 8, e65572. doi:10.1371/journal.pone.0065572
Niblock, M. M., Brunso-Bechtold, J. K., and Riddle, D. R. (2000). Insulin-like growth factor I stimulates dendritic growth in primary somatosensory cortex. J Neurosci 20, 4165–4176. doi:10.1523/JNEUROSCI.20-11-04165.2000
Niu, S., Renfro, A., Quattrocchi, C. C., Sheldon, M., and D’Arcangelo, G. (2004). Reelin promotes hippocampal dendrite development through the VLDLR/ApoER2-Dab1 pathway. Neuron 41, 71–84. doi:10.1016/s0896-6273(03)00819-5
O’Dell, R. S., Cameron, D. A., Zipfel, W. R., and Olson, E. C. (2015). Reelin revents pical eurite etraction during erminal ranslocation and endrite nitiation. J Neurosci 35, 10659–10674. doi:10.1523/JNEUROSCI.1629-15.2015
O’Dell, R. S., Ustine, C. J. M., Cameron, D. A., Lawless, S. M., Williams, R. M., Zipfel, W. R., et al. (2012). Layer 6 cortical neurons require Reelin-Dab1 signaling for cellular orientation, Golgi deployment, and directed neurite growth into the marginal zone. Neural Dev 7, 25. doi:10.1186/1749-8104-7-25
Olson, E. C., Kim, S., and Walsh, C. A. (2006). Impaired neuronal positioning and dendritogenesis in the neocortex after cell-autonomous Dab1 suppression. J Neurosci 26, 1767–1775. doi:10.1523/JNEUROSCI.3000-05.2006
Paramo, B., Bachmann, S. O., Baudouin, S. J., Martinez-Garay, I., and Davies, A. M. (2021). Neuregulin-4 s equired for aintaining oma ize of yramidal eurons in the otor ortex. eNeuro 8, ENEURO.0288–20. doi:10.1523/ENEURO.0288-20.2021
Paramo, B., Wyatt, S., and Davies, A. M. (2019). Neuregulin-4 s equired for the rowth and laboration of triatal edium piny euron endrites. J Neuropathol Exp Neurol 78, 725–734. doi:10.1093/jnen/nlz046
Perkinton, M. S., Sihra, T. S., and Williams, R. J. (1999). Ca(2+)-permeable AMPA receptors induce phosphorylation of cAMP response element-binding protein through a phosphatidylinositol 3-kinase-dependent stimulation of the mitogen-activated protein kinase signaling cascade in neurons. J Neurosci 19, 5861–5874. doi:10.1523/JNEUROSCI.19-14-05861.1999
Persico, A. M., Di Pino, G., and Levitt, P. (2006). Multiple receptors mediate the trophic effects of serotonin on ventroposterior thalamic neurons in vitro. Res 1095, 17–25. doi:10.1016/j.brainres.2006.04.006
Pierce, V. D., and Niu, L. (2019). Stargazin and γ4 slow the channel opening and closing rates of GluA4 AMPA receptors. Sci Rep 9, 9570. doi:10.1038/s41598-019-45870-0
Pino, D., Choe, Y., and Pleasure, S. J. (2011). Wnt5a controls neurite development in olfactory bulb interneurons. PLoS One 3, e00059. doi:10.1042/AN20100038
Pohlkamp, T., Dávid, C., Cauli, B., Gallopin, T., Bouché, E., Karagiannis, A., et al. (2014). Characterization and distribution of Reelin-positive interneuron subtypes in the rat barrel cortex. Cereb24, 3046–3058. doi:10.1093/cercor/bht161
Prigge, C. L., and Kay, J. N. (2018). Dendrite morphogenesis from birth to adulthood. Curr Opin Neurobiol 53, 139–145. doi:10.1016/j.conb.2018.07.007
Prithviraj, R., and Inglis, F. M. (2008). Expression of the N-methyl-D-aspartate receptor subunit NR3B regulates dendrite morphogenesis in spinal motor neurons. Neuroscience 155, 145–153. doi:10.1016/j.neuroscience.2008.03.089
Prithviraj, R., Kelly, K. M., Espinoza-Lewis, R., Hexom, T., Clark, A. B., and Inglis, F. M. (2008). Differential regulation of dendrite complexity by AMPA receptor subunits GluR1 and GluR2 in motor neurons. Dev Neurobiol 68, 247–264. doi:10.1002/dneu.20590
Pujol, C. N., Dupuy, V., Séveno, M., Runtz, L., Bockaert, J., Marin, P., et al. (2020). Dynamic interactions of the 5-HT6 receptor with protein partners control dendritic tree morphogenesis. Sci Signal 13, eaax9520. doi:10.1126/scisignal.aax9520
Puram, S. V., Riccio, A., Koirala, S., Ikeuchi, Y., Kim, A. H., Corfas, G., et al. (2011). A TRPC5-regulated calcium signaling pathway controls dendrite patterning in the mammalian brain. Dev 25, 2659–2673. doi:10.1101/gad.174060.111
Rahman-Enyart, A., Lai, C., and Prieto, A. L. (2020). Neuregulins 1, 2, and 3 romote arly eurite utgrowth in ErbB4-xpressing ortical GABAergic nterneurons. Mol Neurobiol 57, 3568–3588. doi:10.1007/s12035-020-01966-7
Rajan, I., and Cline, H. T. (1998). Glutamate receptor activity is required for normal development of tectal cell dendrites in vivo. J Neurosci 18, 7836–7846. doi:10.1523/JNEUROSCI.18-19-07836.1998
Rauskolb, S., Zagrebelsky, M., Dreznjak, A., Deogracias, R., Matsumoto, T., Wiese, S., et al. (2010). Global deprivation of brain-derived neurotrophic factor in the CNS reveals an area-specific requirement for dendritic growth. J Neurosci 30, 1739–1749. doi:10.1523/JNEUROSCI.5100-09.2010
Redmond, L., Kashani, A. H., and Ghosh, A. (2002). Calcium regulation of dendritic growth via CaM kinase IV and CREB-mediated transcription. Neuron 34, 999–1010. doi:10.1016/s0896-6273(02)00737-7
Ren, Z., Sahir, N., Murakami, S., Luellen, B. A., Earnheart, J. C., Lal, R., et al. (2015). Defects in dendrite and spine maturation and synaptogenesis associated with an anxious-depressive-like phenotype of GABAA receptor-deficient mice. Neuropharmacology 88, 171–179. doi:10.1016/j.neuropharm.2014.07.019
Rieff, H. I., Raetzman, L. T., Sapp, D. W., Yeh, H. H., Siegel, R. E., and Corfas, G. (1999). Neuregulin induces GABA(A) receptor subunit expression and neurite outgrowth in cerebellar granule cells. J Neurosci 19, 10757–10766. doi:10.1523/JNEUROSCI.19-24-10757.1999
Rivera-Cervantes, M. C., Torres, J. S., Feria-Velasco, A., Armendariz-Borunda, J., and Beas-Zárate, C. (2004). NMDA and AMPA receptor expression and cortical neuronal death are associated with p38 in glutamate-induced excitotoxicity in vivo. J Neurosci Res 76, 678–687. doi:10.1002/jnr.20103
Rojas, P. S., Aguayo, F., Neira, D., Tejos, M., Aliaga, E., Muñoz, J. P., et al. (2017). Dual effect of serotonin on the dendritic growth of cultured hippocampal neurons: nvolvement of 5-HT1A and 5-HT7 receptors. Mol Neurosci 85, 148–161. doi:10.1016/j.mcn.2017.09.009
Rosso, S. B., Sussman, D., Wynshaw-Boris, A., and Salinas, P. C. (2005). Wnt signaling through Dishevelled, Rac and JNK regulates dendritic development. Nat Neurosci 8, 34–42. doi:10.1038/nn1374
Saito, Y., Oinuma, I., Fujimoto, S., and Negishi, M. (2009). Plexin-B1 is a GTPase activating protein for M-Ras, remodelling dendrite morphology. Rep 10, 614–621. doi:10.1038/embor.2009.63
Saudou, F., Amara, D. A., Dierich, A., LeMeur, M., Ramboz, S., Segu, L., et al. (1994). Enhanced aggressive behavior in mice lacking 5-HT1B receptor. Science 265, 1875–1878. doi:10.1126/science.8091214
Sceniak, M. P., Fedder, K. N., Wang, Q., Droubi, S., Babcock, K., Patwardhan, S., et al. (2019). An autism-associated mutation in GluN2B prevents NMDA receptor trafficking and interferes with dendrite growth. J Sci 132, jcs232892. doi:10.1242/jcs.232892
Schlomann, U., Schwamborn, J. C., Müller, M., Fässler, R., and Püschel, A. W. (2009). The stimulation of dendrite growth by Sema3A requires integrin engagement and focal adhesion kinase. J Sci 122, 2034–2042. doi:10.1242/jcs.038232
Schratt, G. M., Nigh, E. A., Chen, W. G., Hu, L., and Greenberg, M. E. (2004). BDNF regulates the translation of a select group of mRNAs by a mammalian target of rapamycin-phosphatidylinositol 3-kinase-dependent pathway during neuronal development. J Neurosci 24, 7366–7377. doi:10.1523/JNEUROSCI.1739-04.2004
Schuldiner, O., and Yaron, A. (2015). Mechanisms of developmental neurite pruning. Mol Life Sci 72, 101–119. doi:10.1007/s00018-014-1729-6
Shaywitz, A. J., and Greenberg, M. E. (1999). CREB: a stimulus-induced transcription factor activated by a diverse array of extracellular signals. Annu Rev Biochem 68, 821–861. doi:10.1146/annurev.biochem.68.1.821
Shima, Y., Kawaguchi, S., Kosaka, K., Nakayama, M., Hoshino, M., Nabeshima, Y., et al. (2007). Opposing roles in neurite growth control by two seven-pass transmembrane cadherins. Nat Neurosci 10, 963–969. doi:10.1038/nn1933
Shirazi Fard, S., Kele, J., Vilar, M., Paratcha, G., and Ledda, F. (2010). Tiam1 as a signaling mediator of nerve growth factor-dependent neurite outgrowth. PLoS One 5, e9647. doi:10.1371/journal.pone.0009647
Sikich, L., Hickok, J. M., and Todd, R. D. (1990). 5-HT1A receptors control neurite branching during development. Res Dev Res 56, 269–274. doi:10.1016/0165-3806(90)90092-d
Sin, W. C., Haas, K., Ruthazer, E. S., and Cline, H. T. (2002). Dendrite growth increased by visual activity requires NMDA receptor and Rho GTPases. Nature 419, 475–480. doi:10.1038/nature00987
Sirzen-Zelenskaya, A., Zeyse, J., and Kapfhammer, J. P. (2006). Activation of class I metabotropic glutamate receptors limits dendritic growth of Purkinje cells in organotypic slice cultures. Eur J Neurosci 24, 2978–2986. doi:10.1111/j.1460-9568.2006.05196.x
Sommer, B., Keinänen, K., Verdoorn, T. A., Wisden, W., Burnashev, N., Herb, A., et al. (1990). Flip and flop: a cell-specific functional switch in glutamate-operated channels of the CNS. Science 249, 1580–1585. doi:10.1126/science.1699275
Song, M., Giza, J., Proenca, C. C., Jing, D., Elliott, M., Dincheva, I., et al. (2015). Slitrk5 ediates BDNF-ependent TrkB eceptor rafficking and ignaling. Dev33, 690–702. doi:10.1016/j.devcel.2015.04.009
Sotelo, P., Farfán, P., Benitez, M. L., Bu, G., and Marzolo, M.-P. (2014). Sorting nexin 17 regulates ApoER2 recycling and reelin signaling. PLoS One 9, e93672. doi:10.1371/journal.pone.0093672
Straub, C., and Tomita, S. (2012). The regulation of glutamate receptor trafficking and function by TARPs and other transmembrane auxiliary subunits. Curr Opin Neurobiol 22, 488–495. doi:10.1016/j.conb.2011.09.005
Tai, Y., Feng, S., Ge, R., Du, W., Zhang, X., He, Z., et al. (2008). TRPC6 channels promote dendritic growth via the CaMKIV-CREB pathway. J Sci 121, 2301–2307. doi:10.1242/jcs.026906
Tai, Y., and Jia, Y. (2017). TRPC hannels and euron evelopment, lasticity, and ctivities. Adv Exp Med Biol 976, 95–110. doi:10.1007/978-94-024-1088-4_9
Takeo, Y. H., Shuster, S. A., Jiang, L., Hu, M. C., Luginbuhl, D. J., Rülicke, T., et al. (2021). GluD2-and Cbln1-mediated competitive interactions shape the dendritic arbors of cerebellar Purkinje cells. Neuron 109, 629–644.e8. doi:10.1016/j.neuron.2020.11.028
Tao, X., Finkbeiner, S., Arnold, D. B., Shaywitz, A. J., and Greenberg, M. E. (1998). Ca2+ influx regulates BDNF transcription by a CREB family transcription factor-dependent mechanism. Neuron 20, 709–726. doi:10.1016/s0896-6273(00)81010-7
Teixeira, C. M., Kron, M. M., Masachs, N., Zhang, H., Lagace, D. C., Martinez, A., et al. (2012). Cell-autonomous inactivation of the reelin pathway impairs adult neurogenesis in the hippocampus. J Neurosci 32, 12051–12065. doi:10.1523/JNEUROSCI.1857-12.2012
Thandi, S., Blank, J. L., and Challiss, R. A. J. (2002). Group-I metabotropic glutamate receptors, mGlu1a and mGlu5a, couple to extracellular signal-regulated kinase (ERK) activation via distinct, but overlapping, signalling pathways. J Neurochem 83, 1139–1153. doi:10.1046/j.1471-4159.2002.01217.x
Tian, X., and Feig, L. A. (2006). Age-dependent participation of Ras-GRF proteins in coupling calcium-permeable AMPA glutamate receptors to Ras/Erk signaling in cortical neurons. J Biol Chem 281, 7578–7582. doi:10.1074/jbc.M512060200
Timmusk, T., Belluardo, N., Metsis, M., and Persson, H. (1993). Widespread and developmentally regulated expression of neurotrophin-4 mRNA in rat brain and peripheral tissues. Eur J Neurosci 5, 605–613. doi:10.1111/j.1460-9568.1993.tb00526.x
Tolwani, R. J., Buckmaster, P. S., Varma, S., Cosgaya, J. M., Wu, Y., Suri, C., et al. (2002). BDNF overexpression increases dendrite complexity in hippocampal dentate gyrus. Neuroscience 114, 795–805. doi:10.1016/s0306-4522(02)00301-9
Tozuka, Y., Fukuda, S., Namba, T., Seki, T., and Hisatsune, T. (2005). GABAergic excitation promotes neuronal differentiation in adult hippocampal progenitor cells. Neuron 47, 803–815. doi:10.1016/j.neuron.2005.08.023
Traynelis, S. F., Wollmuth, L. P., McBain, C. J., Menniti, F. S., Vance, K. M., Ogden, K. K., et al. (2010). Glutamate receptor ion channels: structure, regulation, and function. Pharmacol Rev 62, 405–496. doi:10.1124/pr.109.002451
Trommsdorff, M., Gotthardt, M., Hiesberger, T., Shelton, J., Stockinger, W., Nimpf, J., et al. (1999). Reeler/Disabled-like disruption of neuronal migration in knockout mice lacking the VLDL receptor and ApoE receptor 2. Cell 97, 689–701. doi:10.1016/s0092-8674(00)80782-5
Vaidya, V. A., Marek, G. J., Aghajanian, G. K., and Duman, R. S. (1997). 5-HT2A receptor-mediated regulation of brain-derived neurotrophic factor mRNA in the hippocampus and the neocortex. J Neurosci 17, 2785–2795. doi:10.1523/JNEUROSCI.17-08-02785.1997
Valnegri, P., Puram, S. V., and Bonni, A. (2015). Regulation of dendrite morphogenesis by extrinsic cues. Trends Neurosci 38, 439–447. doi:10.1016/j.tins.2015.05.003
van der Velden, L., van Hooft, J. A., and Chameau, P. (2012). Altered dendritic complexity affects firing properties of cortical layer 2/3 pyramidal neurons in mice lacking the 5-HT3A receptor. J Neurophysiol 108, 1521–1528. doi:10.1152/jn.00829.2011
Vernon, C. G., and Swanson, G. T. (2017). Neto2 ssembles with ainate eceptors in DRG eurons during evelopment and odulates eurite utgrowth in dult ensory eurons. J Neurosci 37, 3352–3363. doi:10.1523/JNEUROSCI.2978-16.2017
Vitalis, T., Cases, O., Passemard, S., Callebert, J., and Parnavelas, J. G. (2007). Embryonic depletion of serotonin affects cortical development. Eur J Neurosci 26, 331–344. doi:10.1111/j.1460-9568.2007.05661.x
Vitalis, T., and Parnavelas, J. G. (2003). The role of serotonin in early cortical development. Dev Neurosci 25, 245–256. doi:10.1159/000072272
Walstab, J., Rappold, G., and Niesler, B. (2010). 5-HT(3) receptors: role in disease and target of drugs. Pharmacol Ther 128, 146–169. doi:10.1016/j.pharmthera.2010.07.001
Wang, J. Q., Fibuch, E. E., and Mao, L. (2007). Regulation of mitogen-activated protein kinases by glutamate receptors. J Neurochem 100, 1–11. doi:10.1111/j.1471-4159.2006.04208.x
Wang, J. Q., Tang, Q., Parelkar, N. K., Liu, Z., Samdani, S., Choe, E. S., et al. (2004). Glutamate signaling to Ras-MAPK in striatal neurons: mechanisms for inducible gene expression and plasticity. Mol Neurobiol 29, 1–14. doi:10.1385/MN:29:1:01
Warren, M. S., Bradley, W. D., Gourley, S. L., Lin, Y.-C., Simpson, M. A., Reichardt, L. F., et al. (2012). Integrin β1 signals through Arg to regulate postnatal dendritic arborization, synapse density, and behavior. J Neurosci 32, 2824–2834. doi:10.1523/JNEUROSCI.3942-11.2012
Wayman, G. A., Impey, S., Marks, D., Saneyoshi, T., Grant, W. F., Derkach, V., et al. (2006). Activity-dependent dendritic arborization mediated by CaM-kinase I activation and enhanced CREB-dependent transcription of Wnt-2. Neuron 50, 897–909. doi:10.1016/j.neuron.2006.05.008
West, A. E., Griffith, E. C., and Greenberg, M. E. (2002). Regulation of transcription factors by neuronal activity. Nat Rev Neurosci 3, 921–931. doi:10.1038/nrn987
Wirth, M. J., Brun, A., Grabert, J., Patz, S., and Wahle, P. (2003). Accelerated dendritic development of rat cortical pyramidal cells and interneurons after biolistic transfection with BDNF and NT4/5. Development 130, 5827–5838. doi:10.1242/dev.00826
Wu, G. Y., and Cline, H. T. (1998). Stabilization of dendritic arbor structure in vivo by CaMKII. Science 279, 222–226. doi:10.1126/science.279.5348.222
Wu, G. Y., Deisseroth, K., and Tsien, R. W. (2001). Spaced stimuli stabilize MAPK pathway activation and its effects on dendritic morphology. Nat Neurosci 4, 151–158. doi:10.1038/83976
Xu, B., Zang, K., Ruff, N. L., Zhang, Y. A., McConnell, S. K., Stryker, M. P., et al. (2000). Cortical degeneration in the absence of neurotrophin signaling: dendritic retraction and neuronal loss after removal of the receptor TrkB. Neuron 26, 233–245. doi:10.1016/s0896-6273(00)81153-8
Xu, N.-J., Sun, S., Gibson, J. R., and Henkemeyer, M. (2011). A dual shaping mechanism for postsynaptic ephrin-B3 as a receptor that sculpts dendrites and synapses. Nat Neurosci 14, 1421–1429. doi:10.1038/nn.2931
Yabut, O., Renfro, A., Niu, S., Swann, J. W., Marín, O., and D’Arcangelo, G. (2007). Abnormal laminar position and dendrite development of interneurons in the reeler forebrain. Res 1140, 75–83. doi:10.1016/j.brainres.2005.09.070
Yacoubian, T. A., and Lo, D. C. (2000). Truncated and full-length TrkB receptors regulate distinct modes of dendritic growth. Nat Neurosci 3, 342–349. doi:10.1038/73911
Zagrebelsky, M., Gödecke, N., Remus, A., and Korte, M. (2018). Cell type-specific effects of BDNF in modulating dendritic architecture of hippocampal neurons. Struct Funct 223, 3689–3709. doi:10.1007/s00429-018-1715-0
Zagrebelsky, M., Holz, A., Dechant, G., Barde, Y.-A., Bonhoeffer, T., and Korte, M. (2005). The p75 neurotrophin receptor negatively modulates dendrite complexity and spine density in hippocampal neurons. J Neurosci 25, 9989–9999. doi:10.1523/JNEUROSCI.2492-05.2005
Zhang, L., Schessl, J., Werner, M., Bonnemann, C., Xiong, G., Mojsilovic-Petrovic, J., et al. (2008). Role of GluR1 in activity-dependent motor system development. J Neurosci 28, 9953–9968. doi:10.1523/JNEUROSCI.0880-08.2008
Keywords: dendritic development, neurotransmitters, extracellular matrix, neurotrophins, neuregulins, cadherins and protocadherins, ephrin, reelin
Citation: Hamad MIK, Emerald BS, Kumar KK, Ibrahim MF, Ali BR and Bataineh MF (2023) Extracellular molecular signals shaping dendrite architecture during brain development. Front. Cell Dev. Biol. 11:1254589. doi: 10.3389/fcell.2023.1254589
Received: 10 July 2023; Accepted: 28 November 2023;
Published: 07 December 2023.
Edited by:
Josef P. Kapfhammer, University of Basel, SwitzerlandReviewed by:
Roland Andreas Bender, University of Hamburg, GermanyEric C. Olson, Upstate Medical University, United States
Copyright © 2023 Hamad, Emerald, Kumar, Ibrahim, Ali and Bataineh. This is an open-access article distributed under the terms of the Creative Commons Attribution License (CC BY). The use, distribution or reproduction in other forums is permitted, provided the original author(s) and the copyright owner(s) are credited and that the original publication in this journal is cited, in accordance with accepted academic practice. No use, distribution or reproduction is permitted which does not comply with these terms.
*Correspondence: Mohammad I. K. Hamad, bS5oYW1hZEB1YWV1LmFjLmFl