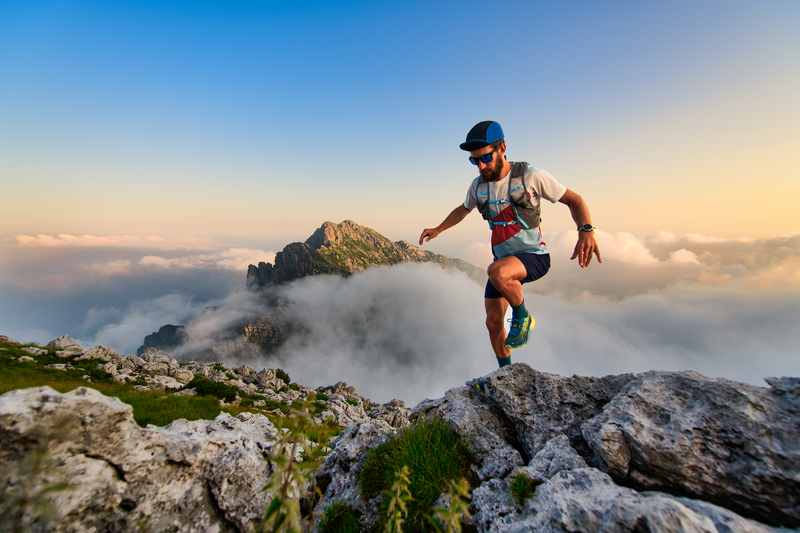
95% of researchers rate our articles as excellent or good
Learn more about the work of our research integrity team to safeguard the quality of each article we publish.
Find out more
REVIEW article
Front. Cell Dev. Biol. , 10 October 2023
Sec. Stem Cell Research
Volume 11 - 2023 | https://doi.org/10.3389/fcell.2023.1245872
The innate and adaptive immune systems rely on the skin for various purposes, serving as the primary defense against harmful environmental elements. However, skin lesions may lead to undesirable consequences such as scarring, accelerated skin aging, functional impairment, and psychological effects over time. The rising popularity of mesenchymal stromal cells (MSCs) for skin wound treatment is due to their potential as a promising therapeutic option. MSCs offer advantages in terms of differentiation capacity, accessibility, low immunogenicity, and their central role in natural wound-healing processes. To accelerate the healing process, MSCs promote cell migration, angiogenesis, epithelialization, and granulation tissue development. Oxygen plays a critical role in the formation and expansion of mammalian cells. The term “normoxia” refers to the usual oxygen levels, defined at 20.21 percent oxygen (160 mm of mercury), while “hypoxia” denotes oxygen levels of 2.91 percent or less. Notably, the ambient O2 content (20%) in the lab significantly differs from the 2%–9% O2 concentration in their natural habitat. Oxygen regulation of hypoxia-inducible factor-1 (HIF-1) mediated expression of multiple genes plays a crucial role in sustaining stem cell destiny concerning proliferation and differentiation. This study aims to elucidate the impact of normoxia and hypoxia on MSC biology and draw comparisons between the two. The findings suggest that expanding MSC-based regenerative treatments in a hypoxic environment can enhance their growth kinetics, genetic stability, and expression of chemokine receptors, ultimately increasing their effectiveness.
The skin’s ability to heal wounds results from the body’s innate ability to repair and regenerate damaged tissues (Lau et al., 2017). It is a common occurrence in people due to accidents, surgeries, burns, and long-term illnesses. Various wound therapies have been suggested, such as pharmacotherapy (Jackson et al., 2012; Abdullahi and Jeschke, 2014), cellular therapy (Nauta et al., 2013; Morimoto et al., 2015), extracorporeal shock wave therapy (Mittermayr et al., 2012; Webster et al., 2012), negative pressure wound therapy (NPWT) (Webster et al., 2012), electrical stimulation therapy (Thakral et al., 2013; Baleg et al., 2015), and light therapy (Haubner et al., 2012; Lau et al., 2015).
The use of mesenchymal stromal/stem cells (MSCs) to treat skin wounds has gained popularity. MSCs offer a reasonable, safe, and successful therapeutic method due to their differential competency and other features, including ease of collection, low immunogenicity, and their essential role in native wound healing physiology (Zou et al., 2012; Markov et al., 2021; Hassan et al., 2014). In fact, MSCs promote the migration of skin cells, the development of blood vessels, the re-epithelialization of wounds, and the production of granulation tissue, thereby accelerating the healing process. Remarkably, they foster a regenerative wound-healing environment instead of a fibrotic one (Zahorec et al., 2015; Yao et al., 2016). While clinical studies have provided evidence suggesting the safety, practicality, and efficacy of MSC-based therapies (Huang et al., 2020; Gentile and Garcovich, 2021; Mahjoor et al., 2023), more evidence is needed due to small sample sizes and a lack of long-term follow-up. Multipotent stem cells (MSCs) exert therapeutic effects through various cell type-specific and wound-healing phase-specific functions, from hemostasis to remodeling (Hocking and Gibran, 2010).
Many adult organs, including bone marrow, adipose tissue, and umbilical cords (UCs), contain mesenchymal stem cells (MSCs) (Pereira et al., 2013). MSCs typically undergo two distinct environments along their journey from isolation to engraftment. Both the in vivo or physiological setting and the in vitro culture setting (from isolation to transplanting) are considered (before isolation and after transplantation). Cells are exposed to 20% O2 during most MSC growth techniques nowadays, which is around 4–10 times higher than the concentration of O2 in their natural habitats (Chow et al., 2001; Antoniou et al., 2004). MSCs cultivated in vitro may be stressed by the increased oxygen levels. Moreover, various studies have provided significant evidence in recent years, indicating the deleterious effect of ambient O2 concentration on MSCs, including early senescence, prolonged population doubling time, DNA damage (Fehrer et al., 2007; Estrada et al., 2012), and poor engraftment upon transplantation (Sch¨achinger et al., 2006a). These findings highlight the critical influence of O2 concentration on the biology of MSCs. Over the past 2 decades, in vitro research examining the pathways involved in stem cell preservation has proliferated. However, the effects of normoxic (often 20%–21% O2 concentration) and hypoxic (typically 2%–9% O2 concentration) environments on stem cell development have been largely overlooked (Simon and Keith, 2008). This article compares and contrasts MSC culture under normoxic and hypoxic in vitro settings. Lastly, this research discusses how MSCs cultivated in vitro under hypoxic settings might serve as a therapeutic option.
Wounds on the skin can be classified into two categories: those requiring tissue regeneration and those that do not. Open wound edges are brought together, and the wound is mostly closed through localized matrix remodeling after surgery or a minor incision. However, a complicated chain reaction can occur when tissue loss is significant in wounds, such as burns, abrasions, and traumatic wounds.
The first phase involves hemostasis and inflammation, which helps rebuild a sterile barrier and reduces exposure to the outside world. Subsequently, the wound undergoes tissue replacement (scar formation). If difficulties arise during the early phases of healing, such as an inadequate immune response or impaired angiogenesis, wounds can become chronic or non-healing (Lazarus et al., 1994; Yates et al., 2011; Frykberg and Banks, 2015; Mahmoudvand et al., 2023). Chronic wounds encompass vascular ulcers, pressure ulcers, and diabetic ulcers, and each patient requires a customized treatment plan based on the severity of their condition. Lack of wound healing progress poses risks of infection, tissue spread, and potential amputation.
To combat the severe consequences of chronic wounds, scientists and doctors are diligently developing and implementing innovative biological treatments. Abnormalities in the last two phases of wound healing, such as keloid or hypertrophic scarring, may result in extensive scarring in contrast to chronic wounds. Although the epithelium may regenerate, and the wound may appear healed, the underlying dermis in these scars is damaged and dysfunctional. Improperly healed wounds tend to be structurally weaker and more susceptible to re-ulceration.
Healing a wound involves a complex synchronization of several cell types, signaling pathways, and microenvironmental adjustments across three overlapping stages of repair: hemostasis/inflammation, tissue replacement, and resolution. The initial step is the hemostasis/inflammation phase, during which the focus is on stopping bleeding from broken blood vessels and preventing the spread of pathogenic infection. Platelets in the bloodstream join with enzymatically transformed fibrin to create a clot that covers the wound and acts as a temporary matrix (Barker and Engler, 2017). This fibrin clot is physiologically active and recruits pro-inflammatory macrophages (M1) and leukocytes, triggering a localized immune response in addition to preventing infection and clearing the wound of cellular and extracellular debris. The tissue replacement phase begins when specialized cells, such as fibroblasts, endothelial cells, epidermal cells, and progenitor cells, enter the wound bed. Fibroblasts produce collagen III-rich granulation tissue to temporarily replace the missing extracellular matrix (ECM), endothelial cells generate new blood vessels through angiogenesis, and epidermal cells migrate beneath the scab to permanently seal the wound surface and restore the epidermis. At this stage, pro-inflammatory macrophages (M1) decrease and are replaced by wound-healing macrophages, categorizing the lesion as sterile (M2). These M2 macrophages facilitate additional vascular healing by producing and modulating the granulated ECM.
The resolution phase involves the permanent replacement of the wound bed with mature ECM, removal of up to 90% of the surplus blood vessels, and cessation of any remaining proliferation and migration signals, replaced with stop signals, such as those operating via CXCR3 (Bodnar et al., 2013; Martins-Green et al., 2013; Mirshekar et al., 2023). Transdifferentiation of fibroblasts into myofibroblasts causes the wound bed to contract by reorganizing and restructuring juvenile collagen III into mature collagen I, thereby restoring the skin’s tensile strength (Sahota et al., 2003; Mahjoor et al., 2021). If everything proceeds as expected, the final result will be little more than a barely noticeable scar.
Various types of stem cells, including bone marrow mesenchymal stem cells (BM-MSCs), umbilical cord MSCs, adipose-derived stem cells (ASCs), molar MSCs, and amniotic fluid ASCs, have been investigated as potential sources of MSCs, which hold significant promise in the field of stem cell therapies. These MSCs, regardless of their source, facilitate the healing process through two main processes: paracrine effects and direct development into skin cells (fibroblasts and keratinocytes) (Martins-Green et al., 2013; Kinnaird et al., 2004).
Miao et al. conducted a comparison between placental-derived MSCs and bone marrow-derived MSCs, assessing their morphology, growth, membrane markers, and differentiation potential (Yao et al., 2016; Lau et al., 2017). Placental MSCs exhibited similar morphology and growth characteristics, as well as the presence of markers CD105, CD29, and CD44. Notably, they lacked expression of hematopoietic markers CD34, CD45, and HLA-DR. Furthermore, the study demonstrated that placental MSCs could differentiate into endothelial and neuronal cells (Tsai et al., 2012). In another recent study focusing on venous leg ulcer treatment, allogeneic AM transplantation proved highly beneficial for serious wounds, showing antiadhesive properties, wound protection, and effective re-epithelialization (anti-scarring) (Martin-Rendon et al., 2007a).
MSCs secrete a myriad of active compounds, such as insulin-like growth factor, hepatocyte growth factor, transforming growth factor beta-1, vascular endothelial growth factor (VEGF), keratinocyte growth factor, fibroblast growth factor 2, platelet-derived growth factor, and collagen I9, which play critical roles in wound healing by stimulating angiogenesis, skin cell proliferation, and ECM formation (Fui et al., 2019). The precise mechanism of direct differentiation of MSCs into specific skin cells remains incompletely understood. However, it is well-established that MSCs have the capacity to differentiate into various types of resident cells when introduced into a wound site. The primary mechanism through which stem cells differentiate into specialized cell types, such as endothelial cells, fibroblasts, and keratinocytes, is through the local release of multiple chemicals. Thus, strategies aimed at augmenting the paracrine MSC secretion within the wound microenvironment hold the potential to enhance the efficiency of wound healing. One promising approach could involve modifying the composition of the suspension by utilizing bubbles encapsulating a thoughtfully selected secretome, which can then be applied to the wound to potentially enhance wound healing outcomes. However, randomized trials confirming the effectiveness and safety of MSC use in wound treatment are still needed. Clinical trials involving MSCs are currently underway, and their findings are awaited to further validate the effectiveness and safety of MSC-based treatments for wound healing (Kyung-Chul et al., 2019).
In the process of wound healing, MSCs originating from subcutaneous fat tissue and the blood supply play a role in regulating early and mid-phase inflammation, as well as contributing to the restoration of dermal tissue. Upon infiltrating the wound, MSCs initiate the secretion of proinflammatory cytokines, which recruit neutrophils and M1 converted macrophages, aiding in the degradation of damaged tissue during the hemostasis/inflammation phase. Additionally, MSCs help in controlling the overall inflammatory response by reducing the number of activated T cells, neutrophils, and macrophages. During the tissue replacement phase, MSCs guide the polarization of monocytes into proreparative M2 macrophages, facilitating the clearance of cellular debris and modifying the temporary matrix. In the resolution phase, MSCs continue to regulate the matrix by releasing matrix metalloproteinases (MMPs) and tissue inhibitors of metalloproteinases (TIMPs), while also maintaining a balance between transforming growth factor-b1 and transforming growth factor-b3 to prevent hypertrophic scarring (Yates et al., 2011).
A unique delivery system employing fibrin glue was investigated in both acute and chronic wounds by Falanga et al. (Sch¨achinger et al., 2006b). They topically applied bone marrow-derived MSCs combined with a fibrin spray up to three times. The study observed that surgical defects from excision of nonmelanoma skin cancers healed within 8 weeks, suggesting that MSCs contributed to accelerated resurfacing. Additionally, chronic lower-extremity wounds present for longer than 1 year significantly decreased in size or healed completely by 20 weeks. The study also found a correlation between the surface density of MSCs and the reduction in ulcer size. Another study by Yoshikawa et al. included 20 patients with various nonhealing wounds who were treated with autologous bone marrow-derived MSCs cultured on a collagen sponge (Chavakis et al., 2010). It was observed that 90% of the wounds healed completely when treated with the cell composite graft, and the addition of MSCs facilitated tissue regeneration.
In 1958, Cooper et al. and Zwartouw & West-wood noticed that certain cells grew more quickly at low O2 tension levels compared to standard air levels (Cooper et al., 1958; Zwartouw and Westwood, 1958). This prompted them to conduct the first experiments to evaluate the effects of varied O2 tension levels on MSC growth. Hypoxia has been identified as a key regulator of MSC recruitment, migration, and differentiation (Raheja et al., 2010a; Raheja et al., 2011). A study by Rochefort et al. demonstrated that hypoxia mobilized MSCs from the bone marrow into the peripheral blood but not hematopoietic progenitor cells (Rochefort et al., 2006). Additionally, stem-cell quiescence and plasticity have been linked to low O2 tension levels (D’Ippolito et al., 2006; Liu et al., 2011). Researchers have been interested in finding the appropriate O2 tension levels for in vitro cultivation (Das et al., 2010). Raheja et al. (2010) conducted an experiment in which they seeded and stimulated MSCs for differentiation in different oxygen concentrations. Results indicated that 21% oxygen was optimal for MSC development into osteoblasts, whereas oxygen levels below 5% significantly slowed the differentiation process. At 5% and 21% O2, however, no significant change in osteogenic markers was detected (Raheja et al., 2010b). Early passaged (P2) MSCs at 5% O2 concentration showed enhanced osteoblastic and adipogenic differentiation capacity (Basciano et al., 2011a). Recent studies have shown that MSCs may survive in an oxygen-depleted (1%–5% O2) environment (Grayson et al., 2007a; Nekanti et al., 2010; Holzwarth et al., 2010a; L´opez et al., 2011). It has also been observed that MSCs isolated from adipose tissue that has been precultured in a hypoxic environment have enhanced adipogenic and osteogenic differentiation potentials (Valorani et al., 2012) (Table 1). Nevertheless, when MSCs were maintained and driven for differentiation in 1% O2 concentration, only a small number of studies demonstrated a loss in differentiation potential (Yang et al., 2011; Hung S.-P. et al., 2012). Several clinical trial data indicate that transplantation of MSCs cultivated under an ambient environment has a limited ability for engraftment (Mohamadnejad et al., 2010; Sch¨achinger et al., 2006b).
Clinical studies utilizing MSCs have shown some promising therapeutic results, as documented in various review papers and meta-analyses (Abdel-Latif et al., 2007; Lipinski et al., 2007; Chavakis et al., 2010; Song et al., 2011). The engraftment and differentiation capacity to cardiomyocytes in vivo of first-passage mouse BM-MSCs was shown to be higher than that of fifth-passage mouse BM-MSCs (Jin et al., 2011). Moreover, murine MSCs pre-conditioned in a hypoxic environment demonstrated increased blood flow and vascular development at day 7 compared to MSCs maintained in a normoxic condition (Leroux et al., 2010). Additionally, hypoxia or a reagent that mimics the reaction to hypoxia increased the expression of the chemokine receptors CXCR4, CXCR7, and CX3CR1 (Hung S.-C. et al., 2007; Liu et al., 2012a; Saller et al., 2012; Tsai et al., 2012). Survival of MSCs in hypoxic environments depends on their capacity, shared by all cells, to switch efficiently from aerobic to anaerobic metabolic pathways (Das et al., 2010). An experimental investigation done with rats on the alterations in MSCs under serum-deprivation and hypoxia settings indicated that serum deprivation was the critical factor leading to ischemia-induced death of MSCs. Nevertheless, it also indicated that prolonged exposure to hypoxia led to mitochondrial malfunction and Caspase-3 activation, a crucial factor in apoptosis (Zhu et al., 2006). The buildup of lactate from glycolysis may become an inhibiting factor over the long term (Lord-Dufour et al., 2009; Das et al., 2010), despite the fact that MSCs have been proven to resist hypoxia (e.g., O2 1%) for at least 48 h (Martin-Rendon et al., 2007a). Hypoxia-inducible factor-1 alpha (HIF-1α), when stabilized, migrates into the cell nucleus and joins with hypoxia-inducible factor-1 beta (HIF-1β) to promote intracellular signaling pathways linked with cell survival. To regulate gluconeogenesis, hypoxia-responsive genes such as glucose-6-phosphate transporter (G6PT) bind to their promoter regions as dimeric complexes. There is evidence that the higher glucose level from gluconeogenesis aids MSC survival in hypoxic or serum-depleted environments (Ren et al., 2006a; Martin-Rendon et al., 2007a; Hung S. C. et al., 2007). In addition to HIF-1 upregulation, a rise in erythropoietin receptors and anti-apoptotic proteins Bcl-2 and Bcl-XL, followed by lower Caspase-3 levels, all contribute to a higher survival rate under hypoxia compared to normoxia. In addition, hypoxia stimulates the production of the proangiogenic molecules interleukin IL-6 and VEGF (Wang Y. et al., 2008) (Table 1). Several signaling pathways, such as the Akt and ERK pathways, control these positive effects.
The Akt signaling pathway is phosphorylated in response to hypoxia and HIF-1 stabilization but is destroyed in the presence of normal oxygen levels. The expression of the pro-apoptotic protein Bax is downregulated, while the expression of the anti-apoptotic factor Bcl-2 is upregulated when Akt is active. Apoptosis or additional stabilization of HIF-1, leading to its translocation into the cell nucleus and activation of hypoxia-responsive genes like G6PT and angiogenesis-related proteins like VEGF and IL-6, may result from such overexpression interacting with the Bax accumulating in the mitochondria (Hill et al., 2009). The ideal culture time of MSCs in hypoxic circumstances, like the optimal O2 tension level, is yet unknown. After just 10 min in culture, Wang et al. found that MSCs subjected to short-term hypoxia pre-conditioning showed improved cell survival and angiogenic characteristics (Wang J. A. et al., 2008). Cell growth was shown to be 30 times greater in low O2 conditions (2% for 7 passes) compared to normoxic cultures by Grayson et al. (Grayson et al., 2007b). After 7 days in culture, Hung et al. found that MSCs could proliferate more effectively when exposed to hypoxia (1% O2) (Hung et al., 2012b). An in vitro migration experiment was also carried out in this work, and the results revealed that MSCs’ migratory potential was improved by hypoxia (Hung et al., 2012c). Matin-Rendon et al. found that MSC proliferation increased even after just 24 h of exposure to 1.5% O2 (Martin-Rendon et al., 2007b). The number of cells in the G2/S/M phase was shown to rise during hypoxia (Ren et al., 2006b), and D'Ippolito et al. demonstrated that a low O2 tension level shortened the time required for the cell population to double when cultivated at 3% O2 (D’Ippolito et al., 2006).
The opposite was found in research by Holzwarth et al., which compared the proliferation rates of MSCs after 7 days of culture at 21%, 5%, 3%, and 1% O2. Strong proliferation rates were seen in their research when MSCs were cultured at 21% O2, which is hyperoxic compared to the physiological environment in which MSCs dwell. After 7 days in culture, a significantly lower percentage of MSCs (1.37%) reached the G2/M phase in hypoxic cell cultures (1% O2) than in cultures with 21% O2. With fewer cells in the G2/M phase, the authors conclude that cell proliferation is inhibited under low- O2 conditions (Holzwarth et al., 2010b). Via nuclear factor kappa-dependent processes (Crisostomo P. R. et al., 2008), hypoxia seems to control the concentrations of soluble factors (including VEGF, fibroblast growth factor-2 (FGF2), hepatocyte growth factor, and insulin-like growth factor 1 (IGF-1)). Results indicate that hypoxia may have an effect on the immunoregulatory capacities of MSCs. After 24 h of exposure to hypoxia, Wu et al. found that most genes were regulated using human MSCs. Nevertheless, matrix metalloproteinase-2 (MMP2) levels dropped after less than 4 h of hypoxia, but VEGF and membrane type 1-matrix metalloproteinase (MT1-MMP) levels increased (Wu et al., 2007). Increased VEGF expression was also reported by Muir et al. in hypoxic circumstances (Muir et al., 2006). After 48 h of cultivation with FBS under hypoxia, Potier et al. found that the expression of TGF-3, but not FGF2 or VEGF, was reduced. The levels of IL-6, IL-8, and MPC1 were not altered in that investigation (Potier et al., 2007). In contrast, Hung et al. reported that under hypoxia, the expression of IL-6, macrophage chemotactic protein (MCP1), and VEGF was elevated in MSCs grown in an FBS-free media (Hung S. C. et al., 2007).
Nakagawa et al. suggested that MSCs, together with bFGF in a skin defect model, accelerate wound healing and showed that the human MSCs transdifferentiated into the epithelium in rats (Valorani et al., 2012). Shumakov et al. showed that the transplantation of MSCs on the surface of deep burn wounds in rats decreased inflammatory cell infiltration and accelerated the formation of new vessels and granulation tissue (Hung S.-P. et al., 2012). The cells were also shown to produce bioactive substances that seemed to accelerate the regeneration process. Collectively, these data demonstrate that MSC treatment impacts all phases of wound repair, including inflammation, epithelialization, granulation tissue formation, and tissue remodeling.
The chemokine receptors CX3CR1 and CXCR4 and the hepatocyte growth factor receptor cMet are all regulated by hypoxia, which also affects the production of soluble factors. These receptors enhance MSCs’ ability to migrate to and home in on injured cells (Das et al., 2010). Hepatocyte growth factor and its receptor cMet, the expression of which is elevated during hypoxia, may have a role, as discovered by Rosova et al. (Rosova et al., 2008a). Hung et al. demonstrated that MSCs’ migratory ability was enhanced by hypoxia, and Wang et al. demonstrated the same thing in the context of a brain injury (Hung et al., 2012d). The CXCR4 receptor was implicated in the capacity of these cells to move to injured tissue in the latter investigation (Wang Y. et al., 2008). In animal models, MSCs cultured under hypoxia appear to have improved immune regulatory performance, suggesting a possible role of hypoxia in cellular therapy strategies (Mao et al., 2004; Hu et al., 2008). Hypoxia has also been shown to influence the secretion of trophic factors and membrane markers associated with MSC migration and homing. Several cytokines, chemokines, and integrins may all play a role in migration. One chemokine that is widely expressed is stromal cell-derived factor-1 (SDF-1/CXCR4 receptor) (Liu et al., 2012b). Thus, the expression of CXCR4 on the surface of MSCs cultivated under hypoxia has a significant role in the migration of transplanted cells (Martin-Rendon et al., 2007b).
Others defined a hypoxia pretreatment of tissue-engineered substitutes, which are 3D biomimetic collagen-chitosan sponge scaffolds (CCSS), as biocompatible and biodegradable supports for the effective delivery, favorable adhesion, and survival of BM-MSCs to accelerate chronic wound healing by inhibiting inflammation and improving angiogenesis (Lazarus et al., 1994; Yates et al., 2012). 3D biomimetic tissue-engineered substrates have a great effect on the development of scaffolds for tissue engineering in chronic wound healing, particularly in combination with cells such as MSCs, fibroblasts, keratinocytes, and endothelial cells. Co-transplantation of CD341 cells with CD341-derived endothelial cells in a 3D fibrin scaffold improved wound healing by decreasing the inflammatory reaction and increasing neovascularization in diabetic rats. The relative secreted factors include VEGF, PDGF, bFGF, IL-10, and IL-17 (Lau et al., 2015). In addition, a similar tissue-engineered substitute using autologous fibroblasts and keratinocytes seeded in Hyaff-11, an ester of hyaluronic acid, has been reported to enhance wound healing in diabetics and is associated with enhanced revascularization (Barker and Engler, 2017).
Others found that the major inflammatory cell infiltrated the CCSS in the wound site, and the expression of TNF-a and IL-6 was significantly higher at early implantation. However, BMMSCs efficiently improved this effect and significantly suppressed TNF-a and IL-6 levels in the wound site, thereby contributing to improved wound healing, particularly, hypoxia pretreatment of the tissue-engineered substitute. They found that the expression of IL-10 was enhanced at the wound site and that IL-10 levels in the tissue-engineered substitute with hypoxia pretreatment were higher compared with other conditions. In addition, normoxic pretreatment of the tissue-engineered substitute also caused a significant increase in IL-10 levels when compared with CCSS only in the wound site.
The molecular signaling molecule oxygen profoundly influences the formation and behavior of mammalian cells. Oxygen deprivation (hypoxia) exerts diverse effects on different cell types, leading to alterations in proliferation (Packer and Fuehr, 1977), adhesion (Lash et al., 2001), apoptosis (Carmeliet et al., 1998), metabolism (Loike et al., 1992), ECM secretion (Horino et al., 2002), growth factor expression (Minchenko et al., 1994), and differentiation phenotypes (Lennon et al., 2001) (Graphical Abstract). The paracrine activity of MSCs induces the overexpression of several secretable factors, including VEGF, transforming growth factor-beta 1 (TGF-1), among others, which may result in apoptosis under hypoxic conditions (Crisostomo P. R. et al., 2008; Lee E. Y. et al., 2009). In comparison to AD-MSCs cultured in normoxic conditioned medium (norCM), AD-MSCs cultured in a hypoxic conditioned medium (hypoCM) have been shown to enhance human dermal fibroblast migration and decrease wound area in an in vivo model (Table 2) (Lee E. Y. et al., 2009). Many MSCs reside in a low oxygen tension environment (between 3% and 9%), depending on the tissue source, and hypoxia within the stem cell niche appears to sustain stem cells’ ability to self-renew, proliferate, and migrate, all of which are essential for their therapeutic efficacy (Rosova et al., 2008b; Ivanovic, 2009; Basciano et al., 2011b; Kimura and Sadek, 2012; Ejtehadifar et al., 2015; Zhou et al., 2022). However, when oxygen levels drop to deficient levels (1.5% O2), as is often the case in a wound bed, MSCs may become overstressed and undergo apoptosis (Ejtehadifar et al., 2015).
Hypoxic BM-MSCs secrete conditioned medium (hypoCM) that promotes the proliferation and migration of keratinocytes and fibroblasts in vitro, as well as the migration of CD14+ monocytes (Table 2). In contrast, norCM hardly influences the growth of these cells in any significant manner. HypoCM can also boost endothelial cell proliferation and migration, thus stimulating the initial stages of angiogenesis (Table 2). Angiogenesis, cell proliferation at the injury site, and recruitment of local macrophages were significantly increased by hypoCM compared to vehicle control medium and norCM, leading to expedited wound closure in vivo. HypoCM likely contains increased amounts of secreted molecules, including bFGF and VEGF-A, induced by HIF, which contributes to the acceleration of wound healing by increasing angiogenesis, cell proliferation, and the recruitment of resident macrophages to the wound. HypoCM also regulates collagen synthesis/degradation and alters collagen composition at the injury site, potentially acting synergistically with bFGF and VEGFA (Table 2). The quality of wound healing was shown to be higher in the hypoCM group. This occurrence may be due to the alternative and regulatory actions of bFGF (Xie et al., 2008), which are brought about by hypoxia when considering the unchanging gene expression level of MMP-1 and the undifferentiated or low protein expression and/or secretion of TGF-b1-3. Based on these findings, regenerative medicine procedures using hypoxic BM-MSCs and their released products may be employed to improve tissue healing after subcutaneous damage (Chen et al., 2014a). It was evident that ASCs cultured under hypoxic circumstances released substances that promoted wound healing in vitro using primary keratinocytes. This impact was amplified compared to normoxic settings. Wound repair mediated by keratinocytes is greatly aided by ASCs and other MSCs (Table 2) (Walter et al., 2010; Lee et al., 2012). Primary human keratinocytes, which are more representative of the human body than immortalized cell lines, show the same impact. It is interesting to note that the irregular cellular migration seen during wound closure may be an indicator of epithelial-to-mesenchymal transition in keratinocytes (Moreno-Bueno et al., 2009).
Hypoxia can alter the production of inflammatory and vasculogenic cytokines in mono- and co-culture, and the conditioned media acquired under hypoxia can stimulate wound healing and vascular development in vitro. Hypoxia-inducible factor-1 (HIF-1) is ubiquitinated and destroyed when oxygen levels are normal, preventing it from enacting its transcriptional activity. To counteract this, HIF-1 accumulates under hypoxic circumstances, forming a heterodimer with HIF-1 and triggering the transcription of genes involved in angiogenic processes, cell proliferation, survival, migration, and death (Table 2) (Semenza, 2003). The conditioned media (CM) obtained from MSC cultivation in vitro includes many cytokines, chemokines, and growth factors (Burdon et al., 2011), and several studies have shown that the paracrine actions of MSC have regenerative potential (Burdon et al., 2011). Angiogenic factors, including VEGF-A, MCP-1, and angiogenin, have been found in the CM from MSC cultivated in hypoxia (Hung SC. et al., 2007). Researchers have also looked into the paracrine effect of MSC. Their findings suggest that MSC grown in normoxia and hypoxia release different soluble factors like KGF, EGF, IGF-1, VEGF-A, PDGF, EPO, and TPO that increase fibroblast, keratinocyte, and endothelial cell migration and increase extracellular matrix, which speeds up wound healing (Table 2). The normoxic co-culture group had higher levels of the pro-inflammatory cytokines IL-1, IL-6, and IL-8 than the NMSC group. However, HMSCs had lower levels of IL-1 and IL-8 than NMSCs did. Pre-conditioning MSC with hypoxia may decrease their immunoreactivity by lowering the expression of pro-inflammatory genes.
Conversely, in both the normoxia and hypoxia groups, the addition of EC boosted the short-term expression of pro-inflammatory cytokines, indicating more significant immunoreactivity from co-culturing the two cell types. Hypoxic groups showed increased VEGF-A gene and protein expression. Several investigations have shown the same (Chen et al., 2008), and it is well established that MSC expresses considerably more VEGF-A than EC in vitro (Pedersen et al., 2014). While the paracrine impact of MSC has been demonstrated to trigger the sprouting of EC through VEGF signaling (Beckermann et al., 2008; Sordi et al., 2005), our results imply that VEGF-A production is enhanced by hypoxia regardless of the presence of EC.
In in vitro and animal experiments, hypoxia was shown to boost ADSC proliferation and improve ADSC wound-healing capability. Hypoxia-induced upregulation of growth factors VEGF and bFGF in ADSCs may be involved in the process (Lee EY. et al., 2009; Li et al., 2023). Because of their ability to stimulate angiogenesis, cell migration, proliferation, and other processes necessary for wound healing, hypoxic BM-MSCs release various growth factors and cytokines. Moreover, IL-6 and IL-8, produced by BM-MSCs, should also be involved in the enhanced wound healing caused by hypoCM (Table 2) (Chen et al., 2014c), due to their promotive effects on the function of fibroblasts and skin cell migration. The angiogenic growth factor ANG-1 has been linked to healthy blood vessel function and integrity (Suri et al., 1996). Several researchers found that hypoxia suppressed ANG-1 expression while elevating ANG-2 expression in co-cultures maintained under normal and lowered oxygen levels. Hypoxia increases ANG-2 expression in endothelial cells (Simon et al., 2008), while reducing ANG-1 expression (Enholm et al., 1997). The ANG-1/ANG-2 ratio is downregulated by hypoxia, which may result in immature vessel development during angiogenesis (Lund et al., 2004). The oxygen tension of the tissue around a wound plays a mediating role in the healing process. As a wound forms, hypoxia occurs as a normal part of the healing process. Resident MSCs move to the injury site after being drawn there by the favorable microenvironment, aiding in wound healing by releasing paracrine factors in this low-oxygen setting (Chen et al., 2014b; Lee et al., 2016). Vascularization is another critical factor in the healing and regenerative processes. MSCs release vasculogenic cytokines at higher rates, which boosts angiogenesis (Boomsma and Geenen, 2012). When EC and MSC conditioned media were tested under hypoxic conditions, the EC group showed significantly lower levels of vascular formation and VEGF expression than the MSC group (Zhang et al., 2012).
In conclusion, the potential of mesenchymal stromal cells (MSCs) in advancing wound healing therapies is truly remarkable. Their paracrine interactions play a pivotal role in expediting the healing process, promoting angiogenesis, and facilitating skin regeneration, making them a promising solution for cutaneous wounds. As researchers strive to enhance the therapeutic efficacy of MSC-based treatments, inducing hypoxia has emerged as a powerful tool to further unleash their capabilities.
By subjecting MSCs to hypoxic pre-conditioning, we can better equip them to withstand the challenges of the wound microenvironment, minimizing cell death and maximizing their beneficial effects on tissue repair. The future of wound healing appears brighter than ever as we delve deeper into the transformative potential of MSCs under hypoxic conditions. Embracing this approach not only holds promise for improving patient outcomes but also paves the way for more effective and efficient wound healing strategies.
By harnessing the power of MSCs in the context of hypoxia, we are forging a path towards advanced regenerative therapies that will enhance the lives of patients and promote overall skin health. The journey to harnessing the full regenerative potential of MSCs in wound healing has just begun, and its impact on medical practice is bound to be profound.
MM, AF: wrote the manuscript, SF: edited manuscript and designed figures of manuscript, HN: English teacher, checked grammar and structure of manuscript English, HA: design and supervision, FH: Scientific revision of the manuscript. All authors read and approved the final manuscript.
The authors declare that the research was conducted in the absence of any commercial or financial relationships that could be construed as a potential conflict of interest.
All claims expressed in this article are solely those of the authors and do not necessarily represent those of their affiliated organizations, or those of the publisher, the editors and the reviewers. Any product that may be evaluated in this article, or claim that may be made by its manufacturer, is not guaranteed or endorsed by the publisher.
Abdel-Latif, A., Bolli, R., Tleyjehet al., I. M., Montori, V. M., Perin, E. C., Hornung, C. A., et al. (2007). Adultbonemarrowderived cells for cardiac repair: A systematic review and metaanalysis. Archives Intern. Med. 167 (10), 989–997. doi:10.1001/archinte.167.10.989
Abdullahi, A., and Jeschke, M. G. (2014). Nutrition and anabolic pharmacotherapies in the care of burn patients. Nutr. Clin. Pract. 29, 621–630. doi:10.1177/0884533614533129
Antoniou, E. S., Sund, S., Homsi, E. N., Challenger, L. F., and Rameshwar, P. (2004). A theoretical simulation of hematopoietic stem cells during oxygen fluctuations: prediction of bone marrow responses during hemorrhagic shock. Shock 22 (5), 415–422. doi:10.1097/01.shk.0000142185.88094.88
Baleg, A., Mohammed, S., Bidin, N., Suan, L. P., Ahmad, S., Fakarruddin, M., et al. (2015). Combination of Er: YAG laser and CO2 laser treatment on skin tissue. Photochem Photobiol. 91 (1), 134–138. doi:10.1111/php.12369
Barker, T. H., and Engler, A. J. (2017). The provisional matrix: setting the stage for tissue repair outcomes. Matrix Biol. 60-61, 1–4. doi:10.1016/j.matbio.2017.04.003
Basciano, L., Nemos, C., Foliguet, B., de Isla, N., de Carvalho, M., Tran, N., et al. (2011b). Long term culture of mesenchymal stem cells in hypoxia promotes a genetic program maintaining their undifferentiated and multipotent status. BMC Cell Biol. 12, 12. doi:10.1186/1471-2121-12-12
Basciano, L., Nemos, C., Foliguet, B., de Isla, N., de Carvalho, M., Tran, N., et al. (2011a). Long term culture of mesenchymal stem cells in hypoxia promotes a genetic program maintaining their undifferentiated and multipotent status. BMC Cell Biol. 12, 12. doi:10.1186/1471-2121-12-12
Beckermann, B. M., Kallifatidis, G., Groth, A., Frommhold, D., Apel, A., Mattern, J., et al. (2008). VEGF expression by mesenchymal stem cells contributes to angiogenesis in pancreatic carcinoma. Br. J. Cancer 99, 622–631. doi:10.1038/sj.bjc.6604508
Bodnar, R. J., Rodgers, M. E., Chen, W. C., and Wells, A. (2013). Pericyte regulation of vascular remodeling through the CXC receptor 3. Arterioscler. Thromb. Vasc. Biol. 33, 2818–2829. doi:10.1161/ATVBAHA.113.302012
Boomsma, R. A., and Geenen, D. L. (2012). Mesenchymal stem cells secrete multiple cytokines that promote angiogenesis and have contrasting effects on chemotaxis and apoptosis. PLoS One 7, e35685. doi:10.1371/journal.pone.0035685
Burdon, T. J., Paul, A., Noiseux, N., Prakash, S., and Shum-Tim, D. (2011). Bone marrow stem cell derived paracrine factors for regenerative medicine: current perspectives and therapeutic potential. Bone Marrow Res. 2011, 207326. doi:10.1155/2011/207326
Carmeliet, P., Dor, Y., Herbert, J.-M., Fukumura, D., Brusselmans, K., Dewerchin, M., et al. (1998). Role of HIF-1alpha in hypoxia-mediated apoptosis, cell proliferation and tumour angiogenesis. Nature 394, 485–490. doi:10.1038/28867
Chavakis, E., Koyanagi, M., and Dimmeler, S. (2010). Enhancing the outcome of cell therapy for cardiac repair: progress from bench to bedside and back. Circulation 121 (2), 325–335. doi:10.1161/CIRCULATIONAHA.109.901405
Chen, L., Tredget, E. E., Wu, P. Y., and Wu, Y. (2008). Paracrine factors of mesenchymal stem cells recruit macrophages and endothelial lineage cells and enhance wound healing. PLoS One 3, e1886. doi:10.1371/journal.pone.0001886
Chen, L., Xu, Y., Zhao, J., Zhang, Z., Yang, R., Xie, J., et al. (2014a). Conditioned medium from hypoxic bone marrow-derived mesenchymal stem cells enhances wound healing in mice. PloS one 9 (4), e96161. doi:10.1371/journal.pone.0096161
Chen, L., Xu, Y., Zhao, J., Zhang, Z., Yang, R., Xie, J., et al. (2014b). Conditioned medium from hypoxic bone marrow-derived mesenchymal stem cells enhances wound healing in mice. PLoS One 9, e96161. doi:10.1371/journal.pone.0096161
Chen, L., Xu, Y., Zhao, J., Zhang, Z., Yang, R., Xie, J., et al. (2014c). Conditioned medium from hypoxic bone marrow-derived mesenchymal stem cells enhances wound healing in mice. PloS one 9 (4), e96161. doi:10.1371/journal.pone.0096161
Chow, D. C., Wenning, L. A., Miller, W. M., and Papoutsakis, E. T. (2001). Modeling pO2 distributions in the bone marrow hematopoietic compartment. II. Modified Kroghian models. Biophysical J. 81 (2), 685–696. doi:10.1016/S0006-3495(01)75733-5
Cooper, P. D., Burt, A. M., and Wilson, J. N. (1958). Critical ef-fect of oxygen tension on rate of growth of animal cells in continuous suspended culture. Nature 182 (4648), 1508–1509. doi:10.1038/1821508b0
Crisostomo, P. R., Wang, Y., Markel, T. A., Wang, M., Lahm, T., and Meldrum, D. R. (2008a). Human mesenchymal stem cells stimulated by TNF-alpha, LPS, or hypoxia pro-duce growth factors by an NF kappa B, but not JNK- dependent mechanism. Am. J. Physiology Cell Physiology 294 (3), C675–C682. doi:10.1152/ajpcell.00437.2007
Crisostomo, P. R., Wang, Y., Markel, T. A., Wang, M., Lahm, T., and Meldrum, D. R. (2008b). Human mesenchymal stem cells stimulated by TNF-alpha, LPS, or hypoxia produce growth factors by an NF kappa B- but not JNK-dependent mechanism. Am. J. Physiol. Cell Physiol. 294, C675–C682. doi:10.1152/ajpcell.00437.2007
Das, R., Jahr, H., van Osch, G. J., and Farrell, E. (2010). The role of hypoxia in bone marrow-derived mesenchymal stem cells: considerations for regenerative medicine ap-proaches. Tissue Eng. Part B Rev. 16 (2), 159–168. doi:10.1089/ten.teb.2009.0296
D’Ippolito, G., Diabira, S., Howard, G. A., Roos, B. A., and Schiller, P. C. (2006). Low oxygen tension inhibits osteogenic differentiation and enhances stemness of human MI- AMI cells. Bone 39 (3), 513–522. doi:10.1016/j.bone.2006.02.061
Ejtehadifar, M., Shamsasenjan, K., Movassaghpour, A., Akbarzadehlaleh, P., Dehdilani, N., Abbasi, P., et al. (2015). The effect of hypoxia on mesenchymal stem cell biology. Adv. Pharm. Bull. 5, 141–149. doi:10.15171/apb.2015.021
Enholm, B., Paavonen, K., Ristimaki, A., Kumar, V., Gunji, Y., Klefstrom, J., et al. (1997). Comparison of VEGF, VEGF-B, VEGF-C and Ang-1 mRNA regulation by serum, growth factors, oncoproteins and hypoxia. Oncogene 14, 2475–2483. doi:10.1038/sj.onc.1201090
Estrada, J. C., Albo, C., Bengur´ıa, A., Dopazo, A., López-Romero, P., Carrera-Quintanar, L., et al. (2012). Culture of human mesenchymal stem cells at low oxygen tension improves growth and genetic stability by activating glycolysis. Cell Death Differ. 19 (5), 743–755. doi:10.1038/cdd.2011.172
Fehrer, C., Brunauer, R., Laschober, G., Unterluggauer, H., Reitinger, S., Kloss, F., et al. (2007). Reduced oxygen tension attenuates differentiation capacity of humanmesenchymal stemcells and prolongs their lifespan. Aging Cell 6 (6), 745–757. doi:10.1111/j.1474-9726.2007.00336.x
Frykberg, R. G., and Banks, J. (2015). Challenges in the treatment of chronic wounds. Adv. Wound Care (New Rochelle) 4, 560–582. doi:10.1089/wound.2015.0635
Fui, L., Lok, M., Govindasamy, V., Yong, T. K., and Lek, T. K. (2019). Understanding the multifaceted mechanisms of diabetic wound healing and therapeutic application of stem cells conditioned medium in the healing process. J. Tissue Eng. Regen. Med. 13 (12), 2218–2233. doi:10.1002/term.2966
Gentile, P., and Garcovich, S. (2021). Systematic review: adipose-derived mesenchymal stem cells, platelet-rich plasma and biomaterials as new regenerative strategies in chronic skin wounds and soft tissue defects. Int. J. Mol. Sci. 22 (4), 1538. doi:10.3390/ijms22041538
Grayson, W. L., Zhao, F., Bunnell, B., and Ma, T. (2007a). Hypoxia enhances proliferation and tissue formation of human mesenchymal stem cells. Biochem. Biophysical Res. Commun. 358 (3), 948–953. doi:10.1016/j.bbrc.2007.05.054
Grayson, W. L., Zhao, F., Bunnell, B., and Ma, T. (2007b). Hypoxia enhances proliferation and tissue formation of human mesenchymal stem cells. Biochem. Biophysical Res. Commun. 358 (3), 948–953. doi:10.1016/j.bbrc.2007.05.054
Hassan, W., Greiser, U., and Wang, W. (2014). Role of adipose-derived stem cells in wound healing. Wound Repair Regen. 22 (3), 313–325. doi:10.1111/wrr.12173
Haubner, F., Ohmann, E., Pohl, F., Strutz, J., and Gassner, H. G. (2012). Wound healing after radiation therapy: review of the literature. Radiat. Oncol. 7 (1), 162. doi:10.1186/1748-717X-7-162
Hill, R. P., Marie-Egyptienne, D. T., and Hedley, D. W. (2009). Cancer stem cells, hypoxia and metastasis. Seminars Radiat. Oncol. 19 (2), 106–111. doi:10.1016/j.semradonc.2008.12.002
Hocking, A. M., and Gibran, N. S. (2010). Mesenchymal stem cells: paracrine signaling and differentiation during cutaneous wound repair. Exp. Cell Res. 316 (14), 2213–2219. doi:10.1016/j.yexcr.2010.05.009
Holzwarth, C., Vaegler, M., Gieseke, F., Pfister, S. M., Handgretinger, R., Kerst, G., et al. (2010a). Low physiologic oxygen tensions reduce proliferation and differentiation of human multipotent mesenchymal stromal cells. BMC Cell Biol. 11, 11. doi:10.1186/1471-2121-11-11
Holzwarth, C., Vaegler, M., Gieseke, F., Pfister, S. M., Handgretinger, R., Kerst, G., et al. (2010b). Low physiologic oxygen tensions reduce proliferation and differentia-tion of human multipotent mesenchymal stromal cells. BMC Cell Biol. 11, 11. doi:10.1186/1471-2121-11-11
Horino, Y., Takahashi, S., Miura, T., and Takahashi, Y. (2002). Prolonged hypoxia accelerates the posttranscriptional process of collagen synthesis in cultured fibroblasts. Life Sci. 71, 3031–3045. doi:10.1016/s0024-3205(02)02142-2
Hu, X., Yu, S. P., Fraser, J. L., Lu, Z., Ogle, M. E., Wang, J. A., et al. (2008). Transplantation of hypoxia-preconditioned mesenchymal stem cells improves infarcted heart function via enhanced survival of implanted cells and angiogenesis. J. Thorac. Cardiovasc. Surg. 135 (4), 799–808. doi:10.1016/j.jtcvs.2007.07.071
Huang, Y-Z., Gou, M., Da, L-C., Zhang, W-Q., and Xie, H-Q. (2020). Mesenchymal stem cells for chronic wound healing: current status of preclinical and clinical studies. Tissue Eng. Part B Rev. 26 (6), 555–570. doi:10.1089/ten.TEB.2019.0351
Hung, S.-C., Pochampally, R. R., Hsu, S.-C., Sanchez, C., Chen, S. C., Spees, J., et al. (2007a). Short-term exposure of multipotent stromal cells to low oxygen increases their expression of CX3CR1 and CXCR4 and their engraftment in vivo. PLoS ONE 2 (5), e416. doi:10.1371/journal.pone.0000416
Hung, S.-P., Ho, J. H., Shih, Y.-R. V., Lo, T., and Lee, O. K. (2012a). Hypoxia promotes proliferation and osteogenic differentiation potentials of human mesenchymal stem cells. J. Orthop. Res. 30 (2), 260–266. doi:10.1002/jor.21517
Hung, S. C., Pochampally, R. R., Chen, S. C., Hsu, S. C., and Prockop, D. J. (2007c). Angiogenic effects of human multipotent stromal cell conditioned medium activate the PI3K-Akt pathway in hypoxic endothelial cells to inhibit apoptosis, increase survival, and stimulate angiogenesis. Stem Cells 25, 2363–2370. doi:10.1634/stemcells.2006-0686
Hung, S. C., Pochampally, R. R., Hsu, S. C., Sanchez, C., Chen, S. C., Spees, J., et al. (2007b). Short-term expo-sure of multipotent stromal cells to low oxygen in-creases their expression of CX3CR1 and CXCR4 and their engraftment in vivo. PLoS One 2 (5), e416. doi:10.1371/journal.pone.0000416
Hung, S. P., Ho, J. H., Shih, Y. R., Lo, T., and Lee, O. K. (2012d). Hypoxia promotes proliferation and osteogenic differ-entiation potentials of human mesenchymal stem cells. J. Orthop. Res. 30 (2), 260–266. doi:10.1002/jor.21517
Hung, S. P., Ho, J. H., Shih, Y. R., Lo, T., and Lee, O. K. (2012c). Hypoxia promotes proliferation and osteogenic differ-entiation potentials of human mesenchymal stem cells. J. Orthop. Res. 30 (2), 260–266. doi:10.1002/jor.21517
Hung, S. P., Ho, J. H., Shih, Y. R., Lo, T., and Lee, O. K. (2012b). Hypoxia promotes proliferation and osteogenic differ-entiation potentials of human mesenchymal stem cells. J. Orthop. Res. 30 (2), 260–266. doi:10.1002/jor.21517
Ivanovic, Z. (2009). Hypoxia or in situ normoxia: the stem cell paradigm. J. Cell Physiol. 219, 271–275. doi:10.1002/jcp.21690
Jackson, W. M., Nesti, L. J., and Tuan, R. S. (2012). Mesenchymal stem cell therapy for attenuation of scar formation during wound healing. Stem Cell Res. Ther. 3 (3), 20. doi:10.1186/scrt111
Jin, J., Zhao, Y., Tan, X., Guo, C., Yang, Z., and Miao, D. (2011). An improved transplantation strategy for mouse mesenchymal stem cells in an acutemyocardial infarctionmodel. PLoS ONE 6 (6), e21005. doi:10.1371/journal.pone.0021005
Kimura, W., and Sadek, H. A. (2012). The cardiac hypoxic niche: emerging role of hypoxic microenvironment in cardiac progenitors. Cardiovasc Diagn Ther. 2, 278–289. doi:10.3978/j.issn.2223-3652.2012.12.02
Kinnaird, T., Stabile, E., Burnett, M. S., Shou, M., Lee, C. W., Barr, S., et al. (2004). Local delivery of marrow-derived stromal cells augments collateral perfusion through paracrine mechanisms. Circulation 109, 1543–1549. doi:10.1161/01.CIR.0000124062.31102.57
Kyung-Chul, Moon, Hyun-Suk, Suh, Ki-Bum, Kim, Han, S. K., Young, K. W., Lee, J. W., et al. (2019). Potential of allogeneic adipose-derived stem cell-hydrogel complex for treating diabetic foot ulcers. Diabetes 68, 837–846. doi:10.2337/db18-0699
Lash, G. E., Fitzpatrick, T. E., and Graham, C. H. (2001). Effect of hypoxia on cellular adhesion to vitronectin and fibronectin. Biochem. Biophys. Res. Commun. 287, 622–629. doi:10.1006/bbrc.2001.5618
Lau, P., Bidin, N., Islam, S., Shukri, W. N., Zakaria, N., Musa, N., et al. Influence of gold nanoparticles on wound healing treatment in rat model: photobiomodulation therapy. Lasers Surg. Med., 2017;49(4):380–386. doi:10.1002/lsm.22614
Lau, P. S., Bidin, N., Krishnan, G., AnaybBaleg, S. M., Marsin, F. M., Sum, M. B. M., et al. (2015). Wound treatment on a diabetic rat model by a 808nm diode laser. Laser Phys. 25 (7), 075601. doi:10.1088/1054-660x/25/7/075601
Lazarus, G. S., Cooper, D. M., Knighton, D. R., Percoraro, R. E., Rodeheaver, G., Robson, M. C., et al. (1994). Definitions and guidelines for assessment of wounds and evaluation of healing. Wound Repair Regen. 2, 165–170. doi:10.1046/j.1524-475X.1994.20305.x
Lee, D. E., Ayoub, N., and Agrawal, D. K. (2016). Mesenchymal stem cells and cutaneous wound healing: novel methods to increase cell delivery and therapeutic efficacy. Stem Cell Res. Ther. 7, 37. doi:10.1186/s13287-016-0303-6
Lee, E. Y., Xia, Y., Kim, W. S., Kim, M. H., Kim, T. H., Kim, K. J., et al. (2009b). Hypoxia-enhanced wound-healing function of adipose-derived stem cells: increase in stem cell proliferation and up-regulation of VEGF and bFGF. Wound Repair Regen. 17 (4), 540–547. doi:10.1111/j.1524-475X.2009.00499.x
Lee, E. Y., Xia, Y., Kim, W. S., Kim, M. H., Kim, T. H., Kim, K. J., et al. (2009a). Hypoxia-enhanced wound-healing function of adipose-derived stem cells: increase in stem cell proliferation and up-regulation of VEGF and bFGF. Wound Repair Regen. 17, 540–547. doi:10.1111/j.1524-475X.2009.00499.x
Lee, S. H., Jin, S. Y., Song, J. S., Seo, K. K., and Cho, K. H. (2012). Paracrine effects of adipose-derived stem cells on keratinocytes and dermal fibroblasts. Ann. Dermatol 24, 136–143. doi:10.5021/ad.2012.24.2.136
Lennon, D. P., Edmison, J. M., and Caplan, A. I. (2001). Cultivation of rat marrow-derived mesenchymal stem cells in reduced oxygen tension: effects on in vitro and in vivo osteochondrogenesis. J. Cell. Physiol. 187, 345–355. doi:10.1002/jcp.1081
Leroux, L., Descamps, B., Tojais, N. F., Séguy, B., Oses, P., Moreau, C., et al. (2010). Hypoxia preconditioned mesenchymal stem cells improve vascular and skeletal muscle fiber regeneration after ischemia through a wnt4-dependent pathway. MolecularTherapy 18 (8), 1545–1552. doi:10.1038/mt.2010.108
Li, S., Sun, J., Yang, J., Yang, Y., Ding, H., Yu, B., et al. (2023). Gelatin methacryloyl (GelMA) loaded with concentrated hypoxic pretreated adipose-derived mesenchymal stem cells (ADSCs) conditioned medium promotes wound healing and vascular regeneration in aged skin. Biomaterials Res. 27 (1), 11. doi:10.1186/s40824-023-00352-3
Lipinski, M. J., Biondi-Zoccai, G. G. L., Abbate, A., Khianey, R., Sheiban, I., Bartunek, J., et al. (2007). Impact of intracoronary cell therapy on left ventricular function in the setting of acute myocardial infarction: A collaborative systematic review and meta-analysis of controlled clinical trials. J. theAmericanCollege Cardiol. 50 (18), 1761–1767. doi:10.1016/j.jacc.2007.07.041
Liu, H., Liu, S., Li, Y., Wang, X., Xue, W., Ge, G., et al. (2012a). The role of SDF-1-CXCR4/CXCR7 axis in the therapeutic effects of hypoxia-preconditioned mesenchymal stem cells for renal ischemia/reperfusion injury. PloS ONE 7 (4), e34608. doi:10.1371/journal.pone.0034608
Liu, H., Liu, S., Li, Y., Wang, X., Xue, W., Ge, G., et al. (2012b). The role of SDF-1-CXCR4/CXCR7 Axis in the therapeutic effects of hypoxia-preconditioned mesen-chymal stem cells for renal ischemia/reperfusion in- jury. PLoS One 7 (4), e34608. doi:10.1371/journal.pone.0034608
Liu, L., Yu, Q., Lin, J., Lai, X., Cao, W., Du, K., et al. (2011). Hypoxia-inducible factor-1α is essential for hypoxia-induced mesenchymal stem cell mobilization into the peripheral blood. Stem Cells Dev. 20 (11), 1961–1971. doi:10.1089/scd.2010.0453
Loike, J., Cao, L., Brett, J., Ogawa, S., Silverstein, S., and Stern, D. (1992). Hypoxia induces glucose transporter expression in endothelial cells. Am. J. Physiol. Cell Physiol. 263, C326–C333. doi:10.1152/ajpcell.1992.263.2.C326
Lord-Dufour, S., Copland, I. B., Levros, L. C., Post, M., Das, A., Khosla, C., et al. (2009). Evidence for transcriptional regulation of the glu-cose-6-phosphate transporter by HIF-1Alpha: targeting G6PT with mumbaistatin analogs in hypoxic mesen-chymal stromal cells. Stem Cells 27 (3), 489–497. doi:10.1634/stemcells.2008-0855
Lund, E. L., Høg, A., Olsen, M. W., Hansen, L. T., Engelholm, S. A., and Kristjansen, P. E. G. (2004). Differential regulation of VEGF, HIF1alpha and angiopoietin-1, -2 and -4 by hypoxia and ionizing radiation in human glioblastoma. Int. J. Cancer 108, 833–838. doi:10.1002/ijc.11662
L´opez, Y., Seshareddy, K., Trevino, E., Cox, J., and Weiss, M. L. (2011). Evaluating the impact of oxygen concentration and plating density on human wharton’s jelly-derived mesenchymal stromal cells. Open Tissue Eng. Regen. Med. J. 4 (1), 82–94. doi:10.2174/1875043501104010082
Mahjoor, M., Afkhami, H., Mollaei, M., Nasr, A., Shahriary, S., and Khorrami, S. (2021). MicroRNA-30c delivered by bone marrow-mesenchymal stem cells induced apoptosis and diminished cell invasion in U-251 glioblastoma cell line. Life Sci. [Internet] 279, 119643. Available from: https://www.sciencedirect.com/science/article/pii/S0024320521006299
Mahjoor, M., Afkhami, H., Najafi, M., Nasr, A., and Khorrami, S. (2023). The role of microRNA-30c in targeting interleukin 6, as an inflammatory cytokine, in the mesenchymal stem cell: a therapeutic approach in colorectal cancer. J Cancer Res. Clin. Oncol. 149 (7), 3149–60.
Mahmoudvand, G., Karimi Rouzbahani, A., Razavi, Z. S., Mahjoor, M., and Afkhami, H. (2023). Mesenchymal stem cell therapy for non-healing diabetic foot ulcer infection: new insight. Front. Bioeng. Biotechnol. 11, 1158484.
Mao, X., Zeng, Q., Wang, X., Cao, L., and Bai, Z. (2004). Angio-genic potency of bone marrow stromal cells improved by ex vivo hypoxia prestimulation. J. Hua- zhong Univ. Sci. Technol. Med. Sci. 24 (6), 566–568. doi:10.1007/BF02911356
Markov, A., Thangavelu, L., Aravindhan, S., Zekiy, A. O., Jarahian, M., Chartrand, M. S., et al. (2021). Mesenchymal stem/stromal cells as a valuable source for the treatment of immune-mediated disorders. Stem Cell Res. Ther. 12 (1), 192–230. doi:10.1186/s13287-021-02265-1
Martin-Rendon, E., Hale, S. J., Ryan, D., Baban, D., Forde, S. P., Roubelakis, M., et al. (2007b). Transcriptional pro-filing of human cord blood CD133+ and cultured bone marrow mesenchymal stem cells in response to hy-poxia. Stem Cells 25 (4), 1003–1012. doi:10.1634/stemcells.2006-0398
Martin-Rendon, E., Hale, S. J., Ryan, D., Baban, D., Forde, S. P., Roubelakis, M., et al. (2007a). Transcriptional pro-filing of human cord blood CD133+ and cultured bone marrow mesenchymal stem cells in response to hy-poxia. Stem Cells 25 (4), 1003–1012. doi:10.1634/stemcells.2006-0398
Martins-Green, M., Petreaca, M., and Wang, L. (2013). Chemokines and their receptors are key players in the orchestra that regulates wound healing. Adv. Wound Care 2, 327–347. doi:10.1089/wound.2012.0380
Minchenko, A., Salceda, S., Bauer, T., and Caro, J. (1994). Hypoxia regulatory elements of the human vascular endothelial growth factor gene. Cell. Mol. Biol. Res. 40, 35–39.
Mirshekar, M., Afkhami, H., Razavi, S., Masjedian Jazi, F., Darban-Sarokhalil, D., Ohadi, E., et al. (2023). Potential antibacterial activity and healing effect of topical administration of bone marrow and adipose mesenchymal stem cells encapsulated in collagen-fibrin hydrogel scaffold on full-thickness burn wound infection caused by Pseudomonas aeruginosa. Burns [Internet]. Available from: https://www.sciencedirect.com/science/article/pii/S0305417923000062
Mittermayr, R., Antonic, V., Hartinger, J., Kaufmann, H., Redl, H., Teot, L., et al. (2012). Extracorporeal shock wave therapy (ESWT) for wound healing: technology, mechanisms, and clinical efficacy. Wound Repair Regen. 20 (4), 456–465. doi:10.1111/j.1524-475X.2012.00796.x
Mohamadnejad, M., Pournasr, B., Bagheri, M., Aghdami, N., Shahsavani, M., Hosseini, L. A., et al. (2010). Transplantation of allogeneic bone marrow mesenchymal stromal cellderived hepatocyte-like cells in homozygous familial hypercholesterolemia. Cytotherapy 12 (4), 566–568. doi:10.3109/14653240903511143
Moreno-Bueno, G., Peinado, H., Molina, P., Olmeda, D., Cubillo, E., Santos, V., et al. (2009). The morphological and molecular features of the epithelial-to-mesenchymal transition. Nat. Protoc. 4, 1591–1613. doi:10.1038/nprot.2009.152
Morimoto, N., Kakudo, N., Matsui, M., Ogura, T., Hara, T., Suzuki, K., et al. (2015). Exploratory clinical trial of combination wound therapy with a gelatin sheet and platelet-rich plasma in patients with chronic skin ulcers: study protocol. BMJ Open 5 (5), e007733. doi:10.1136/bmjopen-2015-007733
Muir, C., Chung, L. W., Carson, D. D., and Farach- Carson, M. C. (2006). Hypoxia increases VEGF-A production by prostate cancer and bone marrow stromal cells and ini-tiates paracrine activation of bone marrow endothelial cells. Clin. Exp. Metastasis 23 (1), 75–86. doi:10.1007/s10585-006-9021-2
Nauta, A., Seidel, C., Deveza, L., Montoro, D., Grova, M., Ko, S. H., et al. (2013). Adipose-derived stromal cells overexpressing vascular endothelial growth factor accelerate mouse excisional wound healing. Mol. Ther. 21 (1), 445–455. doi:10.1038/mt.2012.234
Nekanti, U., Dastidar, S., Venugopal, P., Totey, S., and Ta, M. (2010). Increased proliferation and analysis of differential gene expression in human Wharton’s jelly-derived mesenchymal stromal cells under hypoxia. Int. J. Biol. Sci. 6 (5), 499–512. doi:10.7150/ijbs.6.499
Packer, L., and Fuehr, K. (1977). Low oxygen concentration extends the lifespan of cultured human diploid cells. Nature 267, 423–425. doi:10.1038/267423a0
Pedersen, T. O., Blois, A. L., Xue, Y., Xing, Z., Sun, Y., Finne-Wistrand, A., et al. (2014). Mesenchymal stem cells induce endothelial cell quiescence and promote capillary formation. Stem Cell Res. Ther. 5, 23. doi:10.1186/scrt412
Pereira, R. F., Barrias, C. C., Granja, P. L., and Bartolo, P. J. (2013). Advanced biofabrication strategies for skin regeneration and repair. Nanomedicine (Lond.) 8, 603–621. doi:10.2217/nnm.13.50
Potier, E., Ferreira, E., Andriamanalijaona, R., Pujol, J. P., Oudina, K., Logeart-Avramoglou, D., et al. (2007). Hy-poxia affects mesenchymal stromal cell osteogenic differentiation and angiogenic factor expression. Bone 40 (4), 1078–1087. doi:10.1016/j.bone.2006.11.024
Raheja, L. F., Genetos, D. C., Wong, A., and Yellow- ley, C. E. (2011). Hypoxic regulation of mesenchymal stem cell migration: the role of RhoA and HIF-1α. Cell Bi- ology Int. 35 (10), 981–989. doi:10.1042/CBI20100733
Raheja, L. F., Genetos, D. C., and Yellowley, C. E. (2010a). The effect of oxygen tension on the long-term osteogenic differentiation and MMP/TIMP expression of human mesenchymal stem cells. Cells Tissues Organs 191 (3), 175–184. doi:10.1159/000235679
Raheja, L. F., Genetos, D. C., and Yellowley, C. E. (2010b). The effect of oxygen tension on the long-termosteogenic differentiation and MMP/TIMP expression of human mesenchymal stem cells. Cells Tissues Organs 191 (3), 175–184. doi:10.1159/000235679
Ren, H., Cao, Y., Zhao, Q., Li, J., Zhou, C., Liao, L., et al. (2006a). Proliferation and differentiation of bone marrow stro-mal cells under hypoxic conditions. Biochem. Biophysical Res. Commun. 347 (1), 12–21. doi:10.1016/j.bbrc.2006.05.169
Ren, H., Cao, Y., Zhao, Q., Li, J., Zhou, C., Liao, L., et al. (2006b). Proliferation and differentiation of bone marrow stro-mal cells under hypoxic conditions. Biochem. Biophysical Res. Commun. 347 (1), 12–21. doi:10.1016/j.bbrc.2006.05.169
Rochefort, G. Y., Delorme, B., Lopez, A., Herault, O., Bonnet, P., Charbord, P., et al. (2006). Multi-potential mesenchymal stem cells are mobilized into peripheral blood by hypoxia. Stem Cells 24 (10), 2202–2208. doi:10.1634/stemcells.2006-0164
Rosova, I., Dao, M., Capoccia, B., Link, D., and Nolta, J. A. (2008b). Hypoxic preconditioning results in increased motility and improved therapeutic potential of human mesenchymal stem cells. Stem Cells 26, 2173–2182. doi:10.1634/stemcells.2007-1104
Rosova, I., Dao, M., Capoccia, B., Link, D., and Nolta, J. A. (2008a). Hypoxic preconditioning results in increased motility and improved therapeutic potential of human mesen-chymal stem cells. Stem Cells 26 (8), 2173–2182. doi:10.1634/stemcells.2007-1104
Sahota, P. S., Burn, J. L., Heaton, M., Freedlander, E., Suvarna, S. K., Brown, N. J., et al. (2003). Development of a reconstructed human skin model for angiogenesis. Wound Repair Regen. 11, 275–284. doi:10.1046/j.1524-475x.2003.11407.x
Saller, M. M., Prall, W. C., Docheva, D., Schönitzer, V., Popov, T., Anz, D., et al. (2012). Increased stemness and migration of human mesenchymal stem cells in hypoxia is associated with altered integrin expression. Biochem. Biophysical Res. Commun. 423 (2), 379–385. doi:10.1016/j.bbrc.2012.05.134
Sch¨achinger, V., Erbs, S., Els¨asser, A., Haberbosch, W., Hambrecht, R., Hölschermann, H., et al. (2006a). Intracoronary bone marrow-derived progenitor cells in acute myocardial infarction. Newengl. J. ofMedicine 355 (12), 1210–1221. doi:10.1056/nejmoa060186
Sch¨achinger, V., Erbs, S., Els¨asser, A., Haberbosch, W., Hambrecht, R., Hölschermann, H., et al. (2006b). Intracoronary bone marrow-derived progenitor cells in acute myocardial infarction. Newengl. J. ofMedicine 355 (12), 1210–1221. doi:10.1056/nejmoa060186
Semenza, G. L. (2003). Targeting HIF-1 for cancer therapy. Nat. Rev. Cancer 3, 721–732. doi:10.1038/nrc1187
Simon, M. C., and Keith, B. (2008). The role of oxygen availability in embryonic development and stem cell function. Nat. Rev. Mol. Cell Biol. 9 (4), 285–296. doi:10.1038/nrm2354
Simon, M. P., Tournaire, R., and Pouyssegur, J. (2008). The angiopoietin-2 gene of endothelial cells is up-regulated in hypoxia by a HIF binding site located in its first intron and by the central factors GATA-2 and Ets-1. J. Cell Physiol. 217, 809–818. doi:10.1002/jcp.21558
Song, C.-H., Honmou, O., Furuoka, H., and Horiuchi, M. (2011). Identification of chemoattractive factors involved in the migration of bone marrow-derived mesenchymal stem cells to brain lesions caused by prions. J. Virology 85 (21), 11069–11078. doi:10.1128/JVI.05318-11
Sordi, V., Malosio, M. L., Marchesi, F., Mercalli, A., Melzi, R., Giordano, T., et al. (2005). Bone marrow mesenchymal stem cells express a restricted set of functionally active chemokine receptors capable of promoting migration to pancreatic islets. Blood 106 (2), 419–427. doi:10.1182/blood-2004-09-3507
Suri, C., Jones, P. F., Patan, S., Bartunkova, S., Maisonpierre, P. C., Davis, S., et al. (1996). Requisite role of angiopoietin-1, a ligand for the TIE2 receptor, during embryonic angiogenesis. Cell 87, 1171–1180. doi:10.1016/s0092-8674(00)81813-9
Thakral, G., LaFontaine, J., Najafi, B., Talal, T. K., Kim, P., and Lavery, L. A. (2013). Electrical stimulation to accelerate wound healing. Diabet. Foot Ankle 4, 22081. doi:10.3402/dfa.v4i0.22081
Tsai, C.-C., Yew, T.-L., Yang, D.-C., Huang, W.-H., and Hung, S.-C. (2012). Benefits of hypoxic culture on bone marrow multipotent stromal cells. Am. J. Blood Res. 2 (3), 148–159.
Valorani, M. G., Montelatici, E., Germani, A., Biddle, A., D'Alessandro, D., Strollo, R., et al. (2012). Pre-culturing human adipose tissue mesenchymal stem cells under hypoxia increases their adipogenic and osteogenic differentiation potentials. Cell Prolif. 45 (3), 225–238. doi:10.1111/j.1365-2184.2012.00817.x
Walter, M. N., Wright, K. T., Fuller, H. R., MacNeil, S., and Johnson, W. E. (2010). Mesenchymal stem cell-conditioned medium accelerates skin wound healing: an in vitro study of fibroblast and keratinocyte scratch assays. Exp. Cell Res. 316, 1271–1281. doi:10.1016/j.yexcr.2010.02.026
Wang, J. A., Chen, T. L., Jiang, J., Shi, H., Gui, C., Luo, R. H., et al. (2008b). Hypoxic preconditioning attenuates hypoxia/reoxygenation-in- duced apoptosis in mesenchymal stem cells. Acta Pharmacol. Sin. 29 (1), 74–82. doi:10.1111/j.1745-7254.2008.00716.x
Wang, Y., Deng, Y., and Zhou, G. Q. (2008a). SDF-1Alpha/CXCR4-mediated migration of systemically transplant-ed bone marrow stromal cells towards ischemic brain lesion in a rat model. Brain Res. 1195, 104–112. doi:10.1016/j.brainres.2007.11.068
Webster, J., Scuffham, P., Sherriff, K. L., Stankiewicz, M., and Chaboyer, W. P. (2012). Negative pressure wound therapy for skin grafts and surgical wounds healing by primary intention. Cochrane Libr. 4, CD0092612012. doi:10.1002/14651858.CD009261.pub2
Wu, E. H., Li, H. S., Zhao, T., Fan, J. D., Ma, X., Xiong, L., et al. (2007). Effect of hypoxia on the gene profile of human bone marrow-derived mesenchy-mal stem cells. Acta Physiol. Sin. 59 (2), 227–232.
Xie, J. L., Bian, H. N., Qi, S. H., Chen, H. D., Li, H. D., Xu, Y. B., et al. (2008). Basic fibroblast growth factor (bFGF) alleviates the scar of the rabbit ear model in wound healing. Wound Repair Regen. 16, 576–581. doi:10.1111/j.1524-475X.2008.00405.x
Yang, D.-C., Yang, M.-H., Tsai, C.-C., Huang, T.-F., Chen, Y.-H., and Hung, S.-C. (2011). Hypoxia inhibits osteogenesis in human mesenchymal stem cells through direct regulation of RUNX2 by TWIST. PLoS ONE 6 (9), e23965. doi:10.1371/journal.pone.0023965
Yao, B., Huang, S., Gao, D., Xie, J., Liu, N., and Fu, X. (2016). Age-associated changes in regenerative capabilities of mesenchymal stem cell: impact on chronic wounds repair. Int. Wound J. 13 (6), 1252–1259. doi:10.1111/iwj.12491
Yates, C. C., Bodnar, R., and Wells, A. (2011). Matrix control of scarring. Cell Mol. Life Sci. 68, 1871–1881. doi:10.1007/s00018-011-0663-0
Yates, C. C., Hebda, P., and Wells, A. (2012). Skin wound healing and scarring: fetal wounds and regenerative restitution. Birth Defects Res. C Embryo Today 96, 325–333. doi:10.1002/bdrc.21024
Zahorec, P., Koller, J., Danisovic, L., and Bohac, M. (2015). Mesenchymal stem cells for chronic wounds therapy. Cell Tissue Bank. 16 (1), 19–26. doi:10.1007/s10561-014-9440-2
Zhang, B., Yang, S., Zhang, Y., Sun, Z., Xu, W., and Ye, S. (2012). Co-culture of mesenchymal stem cells with umbilical vein endothelial cells under hypoxic condition. J. Huazhong Univ. Sci. Technol. Med. Sci. 32, 173–180. doi:10.1007/s11596-012-0031-9
Zhou, W., Zhao, X., Shi, X., Chen, C., Cao, Y., and Liu, J. (2022). Constructing tissue-engineered dressing membranes with adipose-derived stem cells and acellular dermal matrix for diabetic wound healing: A comparative study of hypoxia-or normoxia-culture modes. Stem Cells Int. 2022, 2976185. doi:10.1155/2022/2976185
Zhu, W., Chen, J., Cong, X., Hu, S., and Chen, X. (2006). Hypoxia and serum deprivation-induced apoptosis in mesen-chymal stem cells. Stem Cells 24 (2), 416–425. doi:10.1634/stemcells.2005-0121
Zou, J-P., Huang, S., Peng, Y., Liu, H-W., Cheng, B., Fu, X-B., et al. (2012). Mesenchymal stem cells/multipotent mesenchymal stromal cells (MSCs) potential role in healing cutaneous chronic wounds. Int. J. Low. Extrem Wounds 11 (4), 244–253. doi:10.1177/1534734612463935
Keywords: hypoxic MSCs, mesenchymal stromal/stem cells (MSCs), normoxic MSCs, wound, wound healing
Citation: Mahjoor M, Fakouri A, Farokhi S, Nazari H, Afkhami H and Heidari F (2023) Regenerative potential of mesenchymal stromal cells in wound healing: unveiling the influence of normoxic and hypoxic environments. Front. Cell Dev. Biol. 11:1245872. doi: 10.3389/fcell.2023.1245872
Received: 23 June 2023; Accepted: 11 August 2023;
Published: 10 October 2023.
Edited by:
Francesca Diomede, University of Studies G d'Annunzio Chieti and Pescara, ItalyReviewed by:
MIchael Sorkin, The Ohio State University, United StatesCopyright © 2023 Mahjoor, Fakouri, Farokhi, Nazari, Afkhami and Heidari. This is an open-access article distributed under the terms of the Creative Commons Attribution License (CC BY). The use, distribution or reproduction in other forums is permitted, provided the original author(s) and the copyright owner(s) are credited and that the original publication in this journal is cited, in accordance with accepted academic practice. No use, distribution or reproduction is permitted which does not comply with these terms.
*Correspondence: Hamed Afkhami, aGFtZWRhZmtoYW1pNzBAZ21haWwuY29t; Fatemeh Heidari, aGVpZGFyaS5hbmF0b215QGdtYWlsLmNvbQ==
†These authors share first authorship
Disclaimer: All claims expressed in this article are solely those of the authors and do not necessarily represent those of their affiliated organizations, or those of the publisher, the editors and the reviewers. Any product that may be evaluated in this article or claim that may be made by its manufacturer is not guaranteed or endorsed by the publisher.
Research integrity at Frontiers
Learn more about the work of our research integrity team to safeguard the quality of each article we publish.