- 1School of Clinical Medical, Changchun University of Chinese Medicine, Jilin, China
- 2Cardiology Department, Affiliated Hospital of Changchun University of Chinese Medicine, Jilin, China
- 3Department of Massage, Affiliated Hospital of Changchun University of Chinese Medicine, Jilin, China
- 4Internal Medicine of Chinese Medicine, Affiliated Hospital of Changchun University of Chinese Medicine, Jilin, China
- 5School of Pharmacy, Changchun University of Chinese Medicine, Jilin, China
- 6Orthopedics Department, The Third Affiliated Hospital of Changchun University of Chinese Medicine, Jilin, China
Cardiovascular disease (CVD) is the primary cause of death in humans. Atherosclerosis (AS) is the most common CVD and a major cause of many CVD-related fatalities. AS has numerous risk factors and complex pathogenesis, and while it has long been a research focus, most mechanisms underlying its progression remain unknown. Noncoding RNAs (ncRNAs) represent an important focus in epigenetics studies and are critical biological regulators that form a complex network of gene regulation. Abnormal ncRNA expression disrupts the normal function of tissues or cells, leading to disease development. A large body of evidence suggests that ncRNAs are involved in all stages of atherosclerosis, from initiation to progression, and that some are significantly differentially expressed during AS development, suggesting that they may be powerful markers for screening AS or potential treatment targets. Here, we review the role of ncRNAs in AS development and recent developments in the use of ncRNAs for AS-targeted therapy, providing evidence for ncRNAs as diagnostic markers and therapeutic targets.
Introduction
Cardiovascular disease (CVD) is the leading cause of death in humans, with over 17 million CVD-related deaths occurring in 2020 (2020; Acosta et al., 2021). CVD mainly includes atherosclerosis (AS), congenital heart disease, arrhythmia, and heart failure, among which AS is the most common (Virani et al., 2021). AS is a chronic inflammatory disease caused by endothelial dysfunction and abnormal lipid metabolism (Ross, 1999; Zhao et al., 2022). In the early stages of AS, intimal low-density lipoprotein (LDL) accumulation triggers inflammation, and macrophages phagocytose the lipids, forming foam cells that trigger the inflammatory response. During this process, the migration and proliferation capacity of many types of cells, such as vascular smooth muscle cells and macrophages, are abnormal (Moore et al., 2013; Wolf and Ley, 2019; Mushenkova et al., 2020). Subsequently, atherosclerotic plaques are formed by inflammatory cells and smooth muscle cell apoptosis, angiogenesis, and thrombogenesis. The prevalence of AS is high and underlies most CVDs, leading to high-mortality-rate manifestations, such as acute coronary syndrome and myocardial infarction (Falk, 2006; Xie et al., 2020). Early AS has no obvious symptoms; thus, early screening for AS is necessary to effectively prevent high-risk CVD (Liu et al., 2018). Imaging remains the primary means for early AS diagnosis, and due to advances in medical technology, some molecular markers shown promise for improving AS screening (Meng H. et al., 2022). Notably, although aging is a major risk factor for AS, with changes in lifestyle habits and dietary structure, there is now a trend toward the development of AS in younger individuals (Libby, 2021; Tyrrell and Goldstein, 2021). Due to the harm of AS to human health, substantial research has been conducted on the mechanisms underlying its occurrence and development to improve the current status of AS diagnosis and treatment. Indeed, epigenetic modifications contribute to AS, such as an abnormal DNA methylation status, altered histone modification levels, and disordered ncRNA expression levels (Xu et al., 2018). NcRNAs are not involved in protein synthesis but can regulate gene expression (Zhang et al., 2016; Bonilauri and Dallagiovanna, 2022; Sandmann et al., 2023).
Long noncoding RNAs (lncRNAs), microRNAs (miRNAs), circular RNAs (circRNAs), ribosomal RNAs, and tRNAs are the most common ncRNAs(Palazzo and Lee, 2015; Zhang Y. et al., 2022; Dragomir et al., 2022). There is increasing evidence that ncRNAs are important in vascular biology, maintaining human health (Jinn et al., 2015; Håkansson et al., 2019; He et al., 2021; Sletten et al., 2021; Farina et al., 2023). Abnormal ncRNA expression can give rise to numerous diseases, including CVD, various cancers, neurodegenerative diseases, and diabetes (Anastasiadou et al., 2018; Mehta et al., 2020; Zhao et al., 2021). NcRNAs have been shown to regulate biological features, biological mechanisms, and phenotypes, such as cell proliferation, apoptosis, and migration capabilities, and have demonstrated potential as therapeutic targets in various diseases (Zhao W. et al., 2018; Ji et al., 2022). In recent years, abnormally expressed ncRNAs have been found to play an important role in various stages of AS progression, including endothelial damage, plaque formation, and plaque instability (Simion et al., 2020; Cui et al., 2022).
Here, we review the abnormal expression of ncRNAs in the occurrence and development of AS and the specific role of key ncRNAs in these processes. We then introduce several ncRNA-targeted treatment methods for AS, exploring the potential of ncRNAs as diagnostic and therapeutic targets in this disease.
miRNAs participate in AS development and are potential treatment targets
MiRNAs are ncRNAs approximately 20 nucleotides in length. MiRNA is first transcribed into pre-miRNA, which moves from the nucleus to the cytoplasm, undergoes progressive processing, and ultimately matures. Mature miRNAs participate in the formation of RNA silencing complexes (RISCs) and impart RISC targeting by loosely pairing with mRNAs with corresponding miRNA responsive elements (MREs) (Kobayashi and Tomari, 2016; Zealy et al., 2017). Then, miRNAs use RISCs to degrade target mRNA or inhibit its translation, representing a key form of post-transcriptional regulation (Lu and Rothenberg, 2018).
Abnormal miRNA expression has been observed frequently in AS in recent years. Indeed, miRNAs can be used as therapeutic and diagnostic biomarkers and drug targets in AS. The regulatory roles of an increasing number of miRNAs in the occurrence and development of AS are becoming clear (Table 1).
Differentially expressed miRNAs in AS progression can now be more efficiently identified from tissue or blood samples due to advances in sequencing technologies, enabling the analysis of potential key miRNAs in AS and their downstream mechanisms (Moreau et al., 2021; Dragomir et al., 2022; Santovito and Weber, 2022). Albuminuria is a marker of endothelial dysfunction. Screening of miRNAs in the plasma of hypertensive patients with albuminuria symptoms revealed that miR-126-3p was abnormally elevated and associated with cardiovascular events; thus, this miRNA is a potential molecular marker for AS (Martinez-Arroyo et al., 2023). miR-126-5p is also involved in endothelial cell protection via autophagy in AS (Santovito et al., 2020a). In a recent study of AS in 16 baboons, blood and common iliac artery (CIA) samples were collected before and after 2 years of high cholesterol and high-fat diets, and miRNA expression levels were analyzed to identify potential molecular markers of human AS. miR-17-5p and miR-146a-5p, which are upregulated in both fatty streak and fibrous plaque lesion types, were revealed as possible key players in AS development (Karere et al., 2023). Another previous report indicated that miR-17-5p and miR-146a-5p demonstrate potential in the clinical diagnosis of AS (Sharma et al., 2022). miR-342-5p is significantly overexpressed in damaged endothelial cells and significantly affects apoptosis levels in oxidatively damaged endothelial cells by regulating PPP1R12B (Xing et al., 2020). miR-19b expression was found to be elevated in the endothelial cells and arterial tissues of an ox-LDL-induced AS mouse model and promoted inflammation, thus participating in AS development by inhibiting the ubiquitination of NF-κB/p65 by PPARγ(Wang et al., 2021). Abnormally elevated levels of miR-202-5p have been found in AS tissues and to induce macrophage apoptosis by inhibiting Bcl-2 expression levels, thereby promoting AS plaque formation and reducing fibrous cap thickness in vivo, which in turn increased the risk of plaque rupture (Xu et al., 2023). In addition, reduced expression of some miRNAs has been observed during the AS process, suggesting that these miRNAs may have a potential inhibitory effect on AS. Reduced miR-330-3p expression levels and increased AQP9 expression levels have been observed in an AS mouse model. Further experimental results indicated that miR-330-3p could reduce endothelial cell apoptosis and promote endothelial cell proliferation and migration by inhibiting AQP9 (Shan et al., 2023). The recruitment of inflammatory cells is an important event in AS plaque formation. Monocytes adhere to the vessel wall and gradually migrate to the subendothelial layer in the inflammatory state, during which IL-1β upregulates the expression level of cell adhesion molecules; this effect is achieved by inhibiting miR-1914-5p (Toriuchi et al., 2023). When miR-1914-5p was overexpressed, the migration ability of monocytes induced by IL-1β across the endothelial cell layer was significantly inhibited. Ox-LDL-induced AS resulted in a significant downregulation of miR-147a expression levels during AS, which led to elevated ZEB2 levels that mediated monocyte adhesion to endothelial cells, exacerbating lipid accumulation and AS plaque formation; in contrast, elevated miR-147a expression could stabilize AS (Chen et al., 2023).
Numerous studies have focused on new drugs for AS and their key underlying mechanisms, revealing the importance of miRNA expression regulation in the associated pathways. Sodium butyrate, a product of intestinal flora, has a therapeutic effect on AS. A previous report revealed that sodium butyrate in an AS model increased the expression levels of 29 miRNAs, including miR-7a-5p, and reduced the expression of 24 (Ma et al., 2023). This finding suggests that sodium butyrate can regulate miRNA expression in AS. Icariside (ICA), the main active ingredient of Epimedium, can produce significant therapeutic effects in animal models of AS, reducing lipid accumulation and plaque formation in blood vessels. Further studies demonstrated that ICA elevated miR-205-5p expression (Zhu and Ren, 2022), promoted endothelial cell apoptosis, and inhibited their migration; silencing miR-205-5p reversed this inhibitory effect, demonstrating miR-205-5p upregulation is a potential approach for AS treatment (Huang et al., 2023). There is evidence that miR-217 can inhibit apoptosis through the TLR4/PI3K/Akt/NF-κB pathway in atherosclerotic endothelial cells in a rat model (Zhang et al., 2020). Moreover, miR-124-3p overexpression inhibits macrophage proliferation and apoptosis by downregulating MEKK3 expression in a mouse model of AS (Zhai et al., 2020). In addition, numerous studies have indicated that miRNAs are strongly expressed cell lines associated with AS; for example, miR-126-5p in human endothelial cells promotes cell proliferation and reduces apoptosis by inhibiting caspase-3 and Dlk1 (Schober et al., 2014; Santovito et al., 2020a); miR-133a and miR-145 in human VSMCs inhibit cell migration and promote contractile phenotype (Cipollone et al., 2011; Gao et al., 2014; Li et al., 2018; Shi et al., 2019); miR-21 and miR-155 are present in human and mouse monocytes/macrophages, and the former regulates circadian apoptosis in macrophages, whereas the latter exerts inhibitory in early AS but promotional effects in advanced AS (Raitoharju et al., 2011; Wei et al., 2015; Schober et al., 2021). miR-144 knockdown has been shown to attenuate intimal hyperplasia in VSMCs(Lian et al., 2022). The above findings indicate that miRNAs play a role in AS.
MiRNAs, key post-transcriptional regulation factors, change during AS development, likely affecting downstream gene expression homeostasis and AS. Thus, drugs could be developed to target miRNAs that regulate gene expression in AS. In conclusion, abnormally expressed miRNAs are potential diagnostic biomarkers and potential therapeutic targets in AS, and reversing abnormal miRNA expression levels may be a powerful AS prevention or treatment modality.
lncRNAs act as molecular sponges in the AS process
Single-stranded RNAs greater than 200 nucleotides in length are classified as lncRNAs. Some lncRNAs are processed similarly to mRNAs, transcribed by RNA pol III, or capped/polyadenylated (Simion et al., 2019). The competing endogenous RNA (ceRNA) hypothesis posits that lncRNAs can competitively bind to miRNA and regulate the expression of miRNA target genes (Salmena et al., 2011). Research on lncRNAs continues to advance, and our knowledge of their functions has been enriched, suggesting that many diseases are closely related to abnormal lncRNA expression (Table 2).
The lncRNA CARMN, upstream of miR-143 and miR-145, has been found to be downregulated in late AS plaques. CARMN knockdown downregulated miR-143 and miR-145 expression and accelerated AS progression in mice, increasing the area and volume of AS plaques and producing a late AS phenotype (Dong et al., 2021; Ni et al., 2021; Vacante et al., 2021). Moreover, CARMN has been considered a regulator of mouse and human VSMC plasticity in AS (Dong et al., 2021; Ni et al., 2021). In these studies, CARMN promoted the contractile phenotype of VSMCs through miR-143/145, whereas regulation of VSMCs proliferation was independent.
NcRNAs are known to be involved in cellular biology processes in AS, such as cell apoptosis (Kockx and Herman, 2000). MALAT1 can mediate cell autophagy and affects plaque inflammation in AS (Zhu Y. et al., 2019; Cremer et al., 2019). LncRNA RASSF8-AS1 is highly expressed in the serum of AS patients and can promote ATG7-mediated autophagy through the competitive combination of miR-188-3p, promote the proliferation ability and anti-apoptotic activity of smooth muscle cells, and play an important role in the development of AS plaques (Song et al., 2023). A recent study identified the macrophage-specific lncRNA MAARS and observed a 270-fold increase in its expression level in the aortic intima during AS progression and a 60% decrease during AS regression, indicating that this lncRNA has high clinical value as a biomarker for AS diagnosis (Simion et al., 2020). In the aforementioned study, knocking out MAARS resulted in a 52% reduction in AS lesions in LDLR−/− mice; MAARS can interact with the RNA binding protein HuR and thus regulate macrophage apoptosis levels. Knocking down MAARS increases the exocytosis of macrophages and reduces their apoptosis level, thus reducing AS plaque necrosis.
In addition, the lncRNA AI662270 has been observed to be specifically enriched in macrophages during AS. AI662270 overexpression promotes lipid accumulation, reduces the cholesterol outflow of macrophages, and accelerates the formation of foam cells, yielding an AS-promoting effect. AI662270 knockdown exerts a therapeutic effect on AS (Hong et al., 2023). Moreover, there is evidence that MIAT can affect lesion formation and plaque destabilization in AS. MIAT regulates smooth muscle cell proliferation and apoptosis as well as contributes to the transformation of smooth muscle cells into inflammatory macrophage-like cells (Fasolo et al., 2021).
Due to the broad regulatory role of lncRNAs, their abnormal expression often affects the expression levels of multiple downstream genes. LncRNAs may be upstream of other factors in the complex mechanism of AS development and have greater potential as a diagnostic marker for this disease. In addition, a large body of evidence suggests that regulating lncRNA expression can have therapeutic effects on AS and that targeting lncRNAs in AS treatment may produce therapeutic effects through multiple downstream pathways. Further exploration of these specific mechanisms will enrich the theoretical basis of AS.
circRNAs play a functional role in AS
Unlike other ncRNAs, circRNAs exhibit a loop structure. The closed-loop structure of circRNAs provides stability in the presence of RNA nucleic acid exonucleases (Zhao K. et al., 2018). Similar to lncRNAs, circRNAs regulate target mRNA expression levels and influence protein levels by competitively binding to miRNAs(Abdelmohsen et al., 2017). As research has progressed, understanding of circRNAs has gradually increased; however, most circRNAs’ functions remain unclear (Li et al., 2019). Multiple circRNAs have been shown to be differentially expressed during AS progression and, similar to miRNAs and lncRNAs, are involved in various stages of AS occurrence and development (Figure 1). The expression of circRNAs demonstrates temporal and spatial specificity, which, combined with their stable expression characteristics, affords them good potential as molecular markers of AS (Singh et al., 2022). In addition, circRNAs play an important role in gene regulatory networks, and reversing the abnormal expression of key circRNAs in AS may be a potential therapeutic option. Therefore, exploring the key circRNAs in AS and analyzing their role is important for developing diagnostic and treatment approaches for this disease (Table 3).
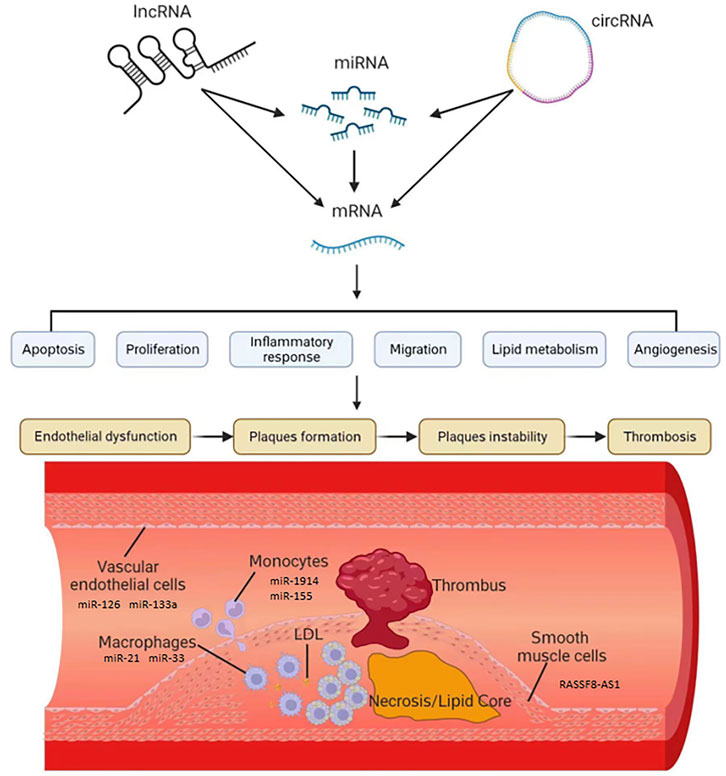
FIGURE 1. The role of ncRNAs in the development of AS. The expression of mRNA which was related to AS was regulated by lncRNA, miRNA and circRNA. These ncRNAs, such as miR-126, miR-133a, miR-21, miR-33, miR-1914,miR-155 and lncRNA RASSF8-AS1, could influence apoptosis, proliferation, inflammatory, migration, lipid metabolism and angiogenesis in vascular endothelial cells, macrophages, monocytes and smooth muscle cells.
The potential role of certain circRNAs in AS has been recognized, and sequencing has been used to directly screen differentially expressed circRNAs to explore AS diagnostic markers. Abnormally elevated circRNA has_circ_0126672 was found in the gene expression profile associated with coronary artery disease. This circRNA can competitively adsorb numerous miRNAs, including miR-145-5p, and is closely associated with AS (Rafiq et al., 2023). Feng Zhang et al. prepared AS rabbit models using a high-fat diet, screened the differentially expressed circRNAs, miRNAs, and mRNAs by RNA-seq and mapped the ceRNA network, concluding that seven circRNAs, including ocu-cirR-novel-18038, were associated with AS by gene function enrichment analysis (Zhang et al., 2018). In addition, circZNF292 in endothelial cells and circLRP6 in VSMCs have been demonstrated to influence endothelial cell shape and vascular diseases in both humans and mice (Hall et al., 2019; Heumüller et al., 2022). CircZNF292 regulates endothelial cell flow responses, while circLRP6 participates in AS by acting as a molecular sponge of miR-145.
After screening for differentially expressed circRNAs in the AS process, further analysis of the role of these circRNAs in AS facilitates the development of therapeutics. In an AS cell model prepared using ox-LDL treatment, circ_0090231 was found to promote the progression of AS by competitively adsorbing miR-635, increasing NLRP3 expression levels and triggering higher levels of cell damage and cell scorching (Ge et al., 2021). In addition, ox-LDL treatment significantly reduced the expression level of circ_0026218, and overexpression of this circRNA increased the expression level of SIRT6 by adsorbing miR-338-3P, enhancing endothelial cell viability and inhibiting the inflammatory response and apoptosis. In contrast, miR-338-3p overexpression reversed the protective effect of circ_0026218 on endothelial cells. Thus, the circ_0026218/miR-338-3p/SIRT6 axis is closely associated with endothelial injury in the early stages of AS (Yang L. et al., 2023).
Angiogenesis is a key event in AS progression and increases AS plaque instability, further elevating AS-related CVD risk. A recent study identified a novel circRNA_06206, circSCRG1, and found that its expression level decreased after ox-LDL treatment and increased after treatment with drugs blocking endothelial cell angiogenesis. Further studies revealed that circSCRG1 has a role in stabilizing AS plaques by competitively adsorbing miR-1268b to regulate NR4A1 expression levels and thereby inhibit angiogenesis (Yuan et al., 2023).
Some circRNAs are expressed at higher levels during the AS process, and further mechanistic exploration has revealed that they could play a role in promoting AS; in contrast, certain circRNAs show decreased expression levels during AS and perform several functions, including the protection of endothelial cells and inhibition of apoptosis. In addition, the stability of circRNAs increases their potential for use as biomarkers versus other ncRNAs. In conclusion, as research on circRNAs in AS continues to unfold, circRNAs as diagnostic markers and therapeutic targets may help alleviate the burden associated with AS.
AS-targeted therapeutic approaches using ncRNAs as targets
With the development of medical technology, precision and personalized medicine approaches are increasingly becoming the main development trend in clinical medicine (Wang et al., 2018). Some drugs have been demonstrated to regulate ncRNA expression related to AS. Furthermore, as potential drugs, lncRNAs and miRNAs might play a role in AS treatment (Saenz-Pipaon and Dichek, 2022). Regarding ncRNAs in precision and personalized medicine, carriers should have the characteristics of low cytotoxicity, non-immunogenicity, and ease of mass production (Nam et al., 2015). Thus, peptides, micelles, liposomes, exosomes, and microbubbles have been assessed as carriers to deliver ncRNAs for AS therapy (Terashima et al., 2018; Aoki et al., 2020; Scholz et al., 2022) (Figure 2).
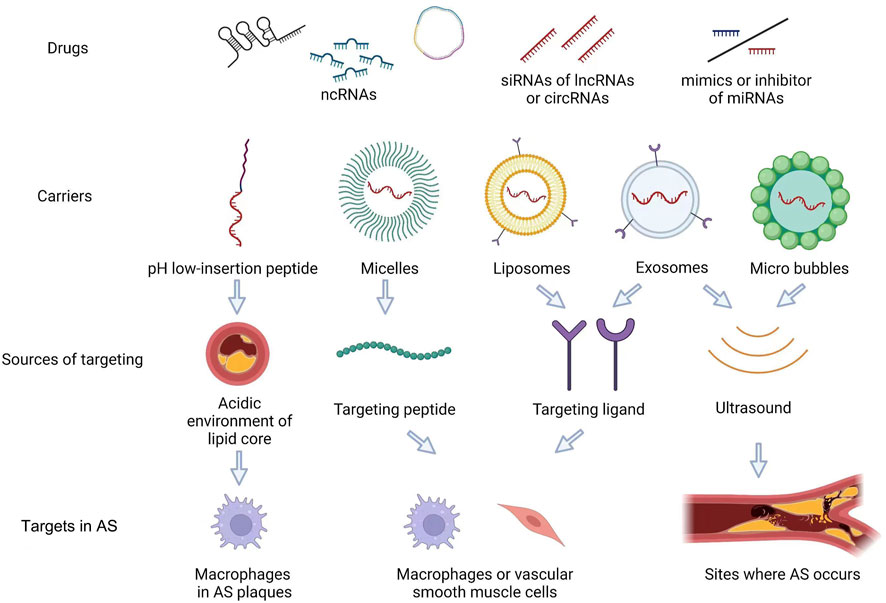
FIGURE 2. AS-targeted therapeutic approaches using ncRNAs as targets. As potential drugs, a few of siRNA of lncRNAs or circRNAs, mimics or inhibitor of miRNAs, can be used in AS. Furtherly, the carriers which were micelles, liposomes, exosomes and micro bubbles, can deliver ncRNAs for AS therapy. These carries can target lipid core, peptide, ligand in Macrophages and VSMCs. Exosome and micro bubbles can used in the sites where the AS occurs by ultrasound.
Exosomes are cell-derived vesicles, typically 50–200 nm, containing rich RNA, DNA, protein, and lipid content. Exosome content secreted by different types of cells varies in composition (Shao et al., 2018). Exosomes play a dual role in AS, on the one hand worsening AS through the contents they carry. For example, exosomes secreted by nicotine-stimulated macrophages contain large amounts of miR-21-3p and increase the migration and proliferation ability of vascular smooth muscle cells through miR-21-3p, thus accelerating the progression of AS (Zhu J. et al., 2019). On the other hand, a fraction of exosomes can have therapeutic effects on AS. For example, adipose-derived MSC exosomes can inhibit miR-342-5p expression and may thereby reduce endothelial cell damage in AS (Xing et al., 2020). Since exosomes themselves may contain therapeutic ncRNAs, nucleotide drugs, such as siRNAs, can be further artificially piggybacked by electroporation to enhance their therapeutic effects (Alvarez-Erviti et al., 2011; Familtseva et al., 2019). With the development of molecular engineering technology, exosomes with higher targeting capabilities can be generated by adding ligands for the targeted treatment of AS (Liang et al., 2021). For example, a recent trial evaluated prepared engineered M2 macrophage-derived exosomes with higher inflammatory tropism and anti-inflammatory effects that could better carry the contents for the treatment of AS (Wu et al., 2020).
Liposomes are lipid bilayer particles with good biocompatibility and bioavailability and allow for modifications on their surface to increase stability and targeting within the plasma (Yan et al., 2019; Scheideler et al., 2020). Liposomes are now widely used to deliver ncRNA drugs; in a recent study miR-146a encapsulated into liposomes demonstrated increased stability that could be preserved for over 2 months, reducing inflammation and decreasing foam cell production (Ho et al., 2023).
Several other types of nanocarriers can be used to deliver ncRNAs for targeted therapy in AS. A novel water-soluble membrane molecule pH low-insertion peptide (pHLIP) was recently proposed as a carrier for AS-targeted therapy based on the acidic environment of the AS lipid core. pHLIP was used to carry antisense oligonucleotides of miR-33-5p to target macrophages in AS plaques without the side effects of systemically reducing miR-33-5p. This study demonstrated that pHLIP could be an excellent vector for miRNA-targeted therapy in vivo and successfully reduced lipid accumulation in macrophages by inhibiting miR-33-5p expression, promoting AS regression and increasing AS plaque stability (Zhang X. et al., 2022).
In contrast to the pH-responsive carriers mentioned above, carriers actively targeting AS also exist. In one study, monocyte chemotactic protein-1 (MCP-1) peptide was used to synthesize peptide amphiphile micelle (PAM), which can target monocytes in AS, and MCP-1/C-C motif chemokine ligand 2 (CCL2) was added to it to target miR-145 to vascular smooth muscle cells through its interaction with C-C chemokine receptor-2 (CCR2), which is enriched in vascular smooth muscle cells (Chin et al., 2021). In the aforementioned study, intravenous miR-145 micelles successfully inhibited nearly half of the lesion growth in an early AS model and inhibited AS plaque growth 35% more than free miR-145 in a mid-stage AS model. In a follow-up study, miR-145 micelles were shown to be effective in the long-term treatment of AS in vivo (Chin et al., 2023).
Ultrasound-targeted microbubble destruction (UTMD) is another a promising technique for targeted drug delivery that uses ultrasound to induce the rupture of drug-carrying microbubbles at the target site, and this approach allows for perforation of the cell membrane to increase the efficiency of drug delivery (Zhou et al., 2022). Yu Wu et al. synthesized cationic microbubbles and encapsulated miR-145 within them to target its release in vascular smooth muscle cells using ultrasound. The in vitro findings demonstrated that this approach significantly increased the transfection efficiency of miR-145; in vivo, this approach reduced AS plaque size by nearly half that achieved by direct treatment with free miR-145 (Wu et al., 2023). In addition, UTMD technology can be applied to exosomal vectors to achieve targeted release of the mounted ncRNA (Sun et al., 2020).
Medical developments have revealed that non-targeted therapeutic drugs sometimes result in side effects that cannot be ignored. Especially in gene therapy, the overall regulation of certain genes may lead to more serious consequences for patients. As a result, there has been a concerted effort to develop targeted therapies for a wide range of diseases, including gene therapy. As the number of specific molecules in key cells in AS grows, theoretically, non-viral vectors for AS targeting could also be gradually improved, allowing the more precise delivery of nucleotide drugs to regulate ncRNA expression levels in AS treatment.
Conclusion
AS is the most common disease of the cardiovascular system, and its ability to induce a variety of fatal CVDs makes AS a constant threat to patients’ life and health. Current AS treatments are pharmacological or surgical, and improving lifestyle habits can help patients with less severe AS. Statins are commonly used to treat AS and have cholesterol-lowering, anti-thrombotic, and anti-inflammatory effects (Min et al., 2013; Vadali and Post, 2014). Antiplatelet agents, hypotensive drugs, and hypoglycemic drugs are also often used in the treatment of AS, and some plant extracts have also demonstrated therapeutic effects; however, despite the continuous development of AS therapies, none are currently curative (Ruan et al., 2022; Shen et al., 2022). Thus, it is important to continue exploring the mechanisms underlying AS development. Currently known risk factors for AS include age, unhealthy diet, smoking, and lack of exercise (Lechner et al., 2020; Tyrrell and Goldstein, 2021). The aging-related miR-217 has been found to be overexpressed in the plasma of patients with CVD. A recent study specifically knocked in miR-217 into endothelial cells in an AS-promoting mouse model, demonstrating the role of miR-217 in reducing NO and promoting endothelial dysfunction, thus exacerbating the AS process, explaining the cause of aging-induced AS to some extent (de Yébenes et al., 2020). miR-124-3p expression levels were significantly higher in smokers than in nonsmokers and past smokers, and increased miR-124-3p expression levels were associated with AS due to the altered monocyte phenotype caused by miR-124-3p overexpression (de Ronde et al., 2017). Thus, more detailed screening of smokers for AS risk may be possible based on ncRNA expression levels.
Sex is also considered a major factor for AS, possibly due to hormone levels. Young women have a much lower risk of developing AS than men, and the risk of AS in postmenopausal women gradually increases or even exceeds that of men (Mathur et al., 2015). A study in a mouse AS model showed that miR-144 silencing prevented the development of AS in male mice but had no effect on female mice (Cheng et al., 2020). Thus, ncRNAs may play different roles in AS patients of different sexes, which is important to consider in the clinical treatment of AS. Strategies exist to improve lifestyle habits to prevent AS, and evidence suggests that ncRNA regulation is a key aspect of these strategies. Exercise is an effective measure to prevent AS, and exercise has been shown to downregulate lncRNA NEAT1 expression, protecting the endothelium from early AS (Yang Q. et al., 2023). In the aforementioned study, NEAT1 was found to induce apoptosis in endothelial cells by binding to KLF4, promoting the expression of the cellular focal death protein NLRP3. Exercise reduced the expression level of NEAT1 through N6-methyladenosine modification. There is a close relationship between diet and AS, and there is evidence that the intake of specific foods can reduce AS risk (Riccardi et al., 2022). Astaxanthin is a common nutrient that has been reported to have AS-protective effects, and a recent study demonstrated that the protective effect of astaxanthin on AS arises through the CircTPP2/miR-3073b-5p/ABCA1 axis that promotes macrophage cholesterol efflux and thus reduces foam cell formation (Zhang Z. et al., 2023). In addition, the side effects of some drugs used to treat other diseases may contribute to AS, and ncRNAs may be crucial regulators of these processes. The exploration of such mechanisms has the potential to improve the use of these drugs and identify new targets for treating AS. For example, after treatment with doxorubicin, a common chemotherapeutic agent with cardiac side effects that limit its application to some extent, miR-33 expression levels increased and consequently inhibited the expression level of ATP-binding cassette transporter protein A1 (ABCA1), promoting lipid accumulation in macrophages and exhibiting the hallmarks of early AS (Zhu et al., 2023). Another study demonstrated that inhibiting miR-33-5p can reduce plaque necrosis by regulating macrophage autophagy in AS, confirming the feasibility of targeting miR-33-5p in AS therapy (Ouimet et al., 2017). However, caution is needed on how to utilize miR-33 as a target for AS therapy, as silencing it for long periods of time may cause additional metabolic abnormalities (Goedeke et al., 2014). This is because miR-33 also plays an important role in cholesterol homeostasis (Marquart et al., 2010; Rayner et al., 2010). It is also noteworthy that two members of miR-33, miR-33a and miR-33b, showed different trends after statin treatment (Allen et al., 2012; Santovito et al., 2020b). Thus the formal application of miRNAs to the clinical treatment of AS has many challenges to overcome.
In summary, changes in ncRNA levels were observed in the presence of common risk factors for AS, further emphasizing the role of ncRNAs in AS development. Evidence suggests that aberrant ncRNA expression has substantial potential as a diagnostic basis for AS and that targeting aberrant ncRNAs to reverse such aberrant expression may have therapeutic effects on AS in the clinical setting.
AS is a non-fatal chronic disease that, without intervention, can easily worsen and lead to more serious CVDs. Abnormal ncRNA expression affects AS development. Many miRNAs, such as miR-217 and miR-124, regulate target mRNAs involved in AS development. As molecular sponges, lncRNAs and circRNAs can influence miRNA and mRNA expression through ceRNA in AS. These ncRNAs are potential biomarkers for the diagnostic screening and therapy of AS. Moreover, with the development of nanomolecular carriers, ncRNAs have become more effective as targeted therapies for AS.
Author contributions
ZY, JY, ZT, and TH participated in writing, editing, and making figures. ZW, YC, TL, and WZ read and approved the final manuscript. All authors contributed to the article and approved the submitted version.
Funding
This work was supported by Scientific Research Project of Education Department of Jilin Province (Grant No. JJKH20220865KJ; JJKH20230983KJ).
Conflict of interest
The authors declare that the research was conducted in the absence of any commercial or financial relationships that could be construed as a potential conflict of interest.
Publisher’s note
All claims expressed in this article are solely those of the authors and do not necessarily represent those of their affiliated organizations, or those of the publisher, the editors and the reviewers. Any product that may be evaluated in this article, or claim that may be made by its manufacturer, is not guaranteed or endorsed by the publisher.
Abbreviations
ABCA1, ATP-binding cassette transporter protein A1; AS, atherosclerosis; CCL2, C-C motif chemokine ligand 2; CCR2, C-C chemokine receptor-2; ceRNA, competing endogenous RNA; CIA, common iliac artery; circRNA, circular RNA; CVD, Cardiovascular disease; DOX, doxorubicin; ICA, Icariside; LDL, low-density lipoprotein; lncRNA, long chain non coding RNA; MCP-1, monocyte chemotactic protein-1; miRNA, microRNA; MREs, miRNA responsive elements; ncRNA, non-coding RNA; PAM, peptide amphiphile micelle; pHLIP, pH low-insertion peptide; RISCs, RNA silencing complexes; UTMD, ultrasound-targeted microbubble destruction.
References
Abdelmohsen, K., Panda, A. C., Munk, R., Grammatikakis, I., Dudekula, D. B., De, S., et al. (2017). Identification of HuR target circular RNAs uncovers suppression of PABPN1 translation by CircPABPN1. RNA Biol. 14 (3), 361–369. doi:10.1080/15476286.2017.1279788
Acosta, S., Johansson, A., and Drake, I. (2021). Diet and lifestyle factors and risk of atherosclerotic cardiovascular disease-A prospective cohort study. Nutrients 13 (11), 3822. doi:10.3390/nu13113822
Allen, R. M., Marquart, T. J., Albert, C. J., Suchy, F. J., Wang, D. Q., Ananthanarayanan, M., et al. (2012). miR-33 controls the expression of biliary transporters, and mediates statin- and diet-induced hepatotoxicity. EMBO Mol. Med. 4 (9), 882–895. doi:10.1002/emmm.201201228
Alvarez-Erviti, L., Seow, Y., Yin, H., Betts, C., Lakhal, S., and Wood, M. J. (2011). Delivery of siRNA to the mouse brain by systemic injection of targeted exosomes. Nat. Biotechnol. 29 (4), 341–345. doi:10.1038/nbt.1807
Anastasiadou, E., Jacob, L. S., and Slack, F. J. (2018). Non-coding RNA networks in cancer. Nat. Rev. Cancer 18 (1), 5–18. doi:10.1038/nrc.2017.99
Aoki, M., Matsumoto, N. M., Dohi, T., Kuwahawa, H., Akaishi, S., Okubo, Y., et al. (2020). Direct delivery of apatite nanoparticle-encapsulated siRNA targeting TIMP-1 for intractable abnormal scars. Mol. Ther. Nucleic Acids 22, 50–61. doi:10.1016/j.omtn.2020.08.005
Bonilauri, B., and Dallagiovanna, B. (2022). Microproteins in skeletal muscle: hidden keys in muscle physiology. J. Cachexia Sarcopenia Muscle 13 (1), 100–113. doi:10.1002/jcsm.12866
Chen, B., Luo, L., Wei, X., Gong, D., and Jin, L. (2018). Altered plasma miR-144 as a novel biomarker for coronary artery disease. Ann. Clin. Lab. Sci. 48 (4), 440–445.
Chen, F., Ning, Y., Liu, J., Lian, M., Wang, J., and Dan, H. (2023). miR-147a targets ZEB2 to regulate ox-LDL-induced monocyte adherence to HUVECs, atherosclerotic plaque formation, and stability in atherosclerosis. J. Biol. Chem. 299, 104657. doi:10.1016/j.jbc.2023.104657
Cheng, J., Cheng, A., Clifford, B. L., Wu, X., Hedin, U., Maegdefessel, L., et al. (2020). MicroRNA-144 silencing protects against atherosclerosis in male, but not female mice. Arterioscler. Thromb. Vasc. Biol. 40 (2), 412–425. doi:10.1161/atvbaha.119.313633
Chin, D. D., Patel, N., Lee, W., Kanaya, S., Cook, J., and Chung, E. J. (2023). Long-term, in vivo therapeutic effects of a single dose of miR-145 micelles for atherosclerosis. Bioact. Mater 27, 327–336. doi:10.1016/j.bioactmat.2023.04.001
Chin, D. D., Poon, C., Wang, J., Joo, J., Ong, V., Jiang, Z., et al. (2021). miR-145 micelles mitigate atherosclerosis by modulating vascular smooth muscle cell phenotype. Biomaterials 273, 120810. doi:10.1016/j.biomaterials.2021.120810
Cipollone, F., Felicioni, L., Sarzani, R., Ucchino, S., Spigonardo, F., Mandolini, C., et al. (2011). A unique microRNA signature associated with plaque instability in humans. Stroke 42 (9), 2556–2563. doi:10.1161/strokeaha.110.597575
Cremer, S., Michalik, K. M., Fischer, A., Pfisterer, L., Jaé, N., Winter, C., et al. (2019). Hematopoietic deficiency of the long noncoding RNA MALAT1 promotes atherosclerosis and plaque inflammation. Circulation 139 (10), 1320–1334. doi:10.1161/circulationaha.117.029015
Cui, Y., Zhou, Y., Gan, N., Xiang, Q., Xia, M., Liao, W., et al. (2022). The role of extracellular non-coding RNAs in atherosclerosis. J. Cardiovasc Transl. Res. 15 (3), 477–491. doi:10.1007/s12265-022-10218-z
de Ronde, M. W. J., Kok, M. G. M., Moerland, P. D., Van den Bossche, J., Neele, A. E., Halliani, A., et al. (2017). High miR-124-3p expression identifies smoking individuals susceptible to atherosclerosis. Atherosclerosis 263, 377–384. doi:10.1016/j.atherosclerosis.2017.03.045
de Yébenes, V. G., Briones, A. M., Martos-Folgado, I., Mur, S. M., Oller, J., Bilal, F., et al. (2020). Aging-associated miR-217 aggravates atherosclerosis and promotes cardiovascular dysfunction. Arterioscler. Thromb. Vasc. Biol. 40 (10), 2408–2424. doi:10.1161/atvbaha.120.314333
Ding, J., Li, H., Liu, W., Wang, X., Feng, Y., Guan, H., et al. (2022). miR-186-5p dysregulation in serum exosomes from patients with AMI aggravates atherosclerosis via targeting LOX-1. Int. J. Nanomedicine 17, 6301–6316. doi:10.2147/ijn.S383904
Dong, K., Shen, J., He, X., Hu, G., Wang, L., Osman, I., et al. (2021). CARMN is an evolutionarily conserved smooth muscle cell-specific LncRNA that maintains contractile phenotype by binding myocardin. Circulation 144 (23), 1856–1875. doi:10.1161/circulationaha.121.055949
Dragomir, M. P., Knutsen, E., and Calin, G. A. (2022). Classical and noncanonical functions of miRNAs in cancers. Trends Genet. 38 (4), 379–394. doi:10.1016/j.tig.2021.10.002
Falk, E. (2006). Pathogenesis of atherosclerosis. J. Am. Coll. Cardiol. 47, C7–C12. doi:10.1016/j.jacc.2005.09.068
Familtseva, A., Jeremic, N., and Tyagi, S. C. (2019). Exosomes: cell-created drug delivery systems. Mol. Cell Biochem. 459 (1-2), 1–6. doi:10.1007/s11010-019-03545-4
Fan, Y., Zhang, Y., Zhao, H., Liu, W., Xu, W., Jiang, L., et al. (2023). lncR-GAS5 upregulates the splicing factor SRSF10 to impair endothelial autophagy, leading to atherogenesis. Front. Med. 17, 317–329. doi:10.1007/s11684-022-0931-4
Farina, F. M., Weber, C., and Santovito, D. (2023). The emerging landscape of non-conventional RNA functions in atherosclerosis. Atherosclerosis 374, 74–86. doi:10.1016/j.atherosclerosis.2023.01.009
Fasolo, F., Jin, H., Winski, G., Chernogubova, E., Pauli, J., Winter, H., et al. (2021). Long noncoding RNA MIAT controls advanced atherosclerotic lesion formation and plaque destabilization. Circulation 144 (19), 1567–1583. doi:10.1161/circulationaha.120.052023
Gao, S., Wassler, M., Zhang, L., Li, Y., Wang, J., Zhang, Y., et al. (2014). MicroRNA-133a regulates insulin-like growth factor-1 receptor expression and vascular smooth muscle cell proliferation in murine atherosclerosis. Atherosclerosis 232 (1), 171–179. doi:10.1016/j.atherosclerosis.2013.11.029
GBD (2020). Global burden of 369 diseases and injuries in 204 countries and territories, 1990-2019: A systematic analysis for the global burden of disease study 2019. Lancet 396, 1204–1222. doi:10.1016/s0140-6736(2030925-9)
Ge, Y., Liu, W., Yin, W., Wang, X., Wang, J., Zhu, X., et al. (2021). Circular RNA circ_0090231 promotes atherosclerosis in vitro by enhancing NLR family pyrin domain containing 3-mediated pyroptosis of endothelial cells. Bioengineered 12 (2), 10837–10848. doi:10.1080/21655979.2021.1989260
Goedeke, L., Salerno, A., Ramírez, C. M., Guo, L., Allen, R. M., Yin, X., et al. (2014). Long-term therapeutic silencing of miR-33 increases circulating triglyceride levels and hepatic lipid accumulation in mice. EMBO Mol. Med. 6 (9), 1133–1141. doi:10.15252/emmm.201404046
Håkansson, K. E. J., Goossens, E. A. C., Trompet, S., van Ingen, E., de Vries, M. R., van der Kwast, R., et al. (2019). Genetic associations and regulation of expression indicate an independent role for 14q32 snoRNAs in human cardiovascular disease. Cardiovasc Res. 115 (10), 1519–1532. doi:10.1093/cvr/cvy309
Hall, I. F., Climent, M., Quintavalle, M., Farina, F. M., Schorn, T., Zani, S., et al. (2019). Circ_Lrp6, a circular RNA enriched in vascular smooth muscle cells, acts as a sponge regulating miRNA-145 function. Circ. Res. 124 (4), 498–510. doi:10.1161/circresaha.118.314240
He, X., Yang, Y., Wang, Q., Wang, J., Li, S., Li, C., et al. (2021). Expression profiles and potential roles of transfer RNA-derived small RNAs in atherosclerosis. J. Cell Mol. Med. 25 (14), 7052–7065. doi:10.1111/jcmm.16719
Heumüller, A. W., Jones, A. N., Mourão, A., Klangwart, M., Shi, C., Wittig, I., et al. (2022). Locus-conserved circular RNA cZNF292 controls endothelial cell flow responses. Circ. Res. 130 (1), 67–79. doi:10.1161/circresaha.121.320029
Ho, D., Lynd, T. O., Jun, C., Shin, J., Millican, R. C., Estep, B. K., et al. (2023). MiR-146a encapsulated liposomes reduce vascular inflammatory responses through decrease of ICAM-1 expression, macrophage activation, and foam cell formation. Nanoscale 15 (7), 3461–3474. doi:10.1039/d2nr03280e
Hong, Y., Zhang, Y., Chen, H., Tang, X., Zhao, H., Meng, Z., et al. (2023). Genetic dissection of the impact of lncRNA AI662270 during the development of atherosclerosis. J. Transl. Med. 21 (1), 97. doi:10.1186/s12967-023-03962-6
Huang, P., Wang, F., Zhang, Y., Zhang, Y., Qin, M., Ji, J., et al. (2023). Icariin alleviates atherosclerosis by regulating the miR-205-5p/ERBB4/AKT signaling pathway. Int. Immunopharmacol. 114, 109611. doi:10.1016/j.intimp.2022.109611
Ji, C. Y., Yuan, M. X., Chen, L. J., Gao, H. Q., Zhang, T., and Yin, Q. (2022). miR-92a represses the viability and migration of nerve cells in Hirschsprung's disease by regulating the KLF4/PI3K/AKT pathway. Acta Neurobiol. Exp. (Wars) 82 (3), 336–346. doi:10.55782/ane-2022-032
Jiang, M., Song, Y., Ren, M. X., He, R. C., Dong, X. H., Li, X. H., et al. (2023). LncRNA NIPA1-SO confers atherosclerotic protection by suppressing the transmembrane protein NIPA1. J. Adv. Res. doi:10.1016/j.jare.2023.01.017
Jinn, S., Brandis, K. A., Ren, A., Chacko, A., Dudley-Rucker, N., Gale, S. E., et al. (2015). snoRNA U17 regulates cellular cholesterol trafficking. Cell Metab. 21 (6), 855–867. doi:10.1016/j.cmet.2015.04.010
Karere, G. M., Glenn, J. P., Li, G., Konar, A., VandeBerg, J. L., and Cox, L. A. (2023). Potential miRNA biomarkers and therapeutic targets for early atherosclerotic lesions. Sci. Rep. 13 (1), 3467. doi:10.1038/s41598-023-29074-1
Kobayashi, H., and Tomari, Y. (2016). RISC assembly: coordination between small RNAs and argonaute proteins. Biochim. Biophys. Acta 1859 (1), 71–81. doi:10.1016/j.bbagrm.2015.08.007
Kockx, M. M., and Herman, A. G. (2000). Apoptosis in atherosclerosis: beneficial or detrimental? Cardiovasc Res. 45 (3), 736–746. doi:10.1016/s0008-6363(99)00235-7
Lechner, K., von Schacky, C., McKenzie, A. L., Worm, N., Nixdorff, U., Lechner, B., et al. (2020). Lifestyle factors and high-risk atherosclerosis: pathways and mechanisms beyond traditional risk factors. Eur. J. Prev. Cardiol. 27 (4), 394–406. doi:10.1177/2047487319869400
Li, H. M., Ma, X. L., and Li, H. G. (2019). Intriguing circles: conflicts and controversies in circular RNA research. Wiley Interdiscip. Rev. RNA 10 (5), e1538. doi:10.1002/wrna.1538
Li, P., Hong, J., Liang, C., Li, Y., Gao, L., Wu, L., et al. (2023). Endothelial cell-released extracellular vesicles trigger pyroptosis and vascular inflammation to induce atherosclerosis through the delivery of HIF1A-AS2. Faseb J. 37 (6), e22942. doi:10.1096/fj.202201399RRR
Li, S., Sun, W., Zheng, H., and Tian, F. (2018). Microrna-145 accelerates the inflammatory reaction through activation of NF-κB signaling in atherosclerosis cells and mice. Biomed. Pharmacother. 103, 851–857. doi:10.1016/j.biopha.2018.03.173
Lian, X., Lv, M., and Shi, B. (2022). MicroRNA-144 silencing attenuates intimal hyperplasia by directly targeting PTEN. Clin. Exp. Hypertens. 44, 678–686. doi:10.1080/10641963.2022.2123923
Liang, Y., Duan, L., Lu, J., and Xia, J. (2021). Engineering exosomes for targeted drug delivery. Theranostics 11 (7), 3183–3195. doi:10.7150/thno.52570
Libby, P. (2021). The changing landscape of atherosclerosis. Nature 592 (7855), 524–533. doi:10.1038/s41586-021-03392-8
Liu, A., Pirastehfar, M., Yu, D., and Linares, G. (2018). Phenotypic ASCOD characterisations of ischaemic stroke in the young at an urban tertiary care centre. Stroke Vasc. Neurol. 3 (4), 209–214. doi:10.1136/svn-2017-000139
Liu, L., Liu, Y., and Zhao, Y. (2023). Circular RNA circ_0008896 contributes to oxidized low-density lipoprotein-induced aortic endothelial cell injury via targeting miR-188-3p/NOD2 axis. Cell Stress Chaperones 28 (3), 275–287. doi:10.1007/s12192-023-01336-x
Lu, T. X., and Rothenberg, M. E. (2018). MicroRNA. J. Allergy Clin. Immunol. 141 (4), 1202–1207. doi:10.1016/j.jaci.2017.08.034
Ma, H., Yang, L., Liu, Y., Yan, R., Wang, R., Zhang, P., et al. (2023). Butyrate suppresses atherosclerotic inflammation by regulating macrophages and polarization via GPR43/HDAC-miRNAs axis in ApoE-/- mice. PLoS One 18 (3), e0282685. doi:10.1371/journal.pone.0282685
Ma, J., Liu, J., Li, T., and Ren, J. (2022). Hsa_circ_0030042 facilitates the proliferation and migration of vascular smooth muscle cells via the miR-514a-3p/FOXO1 Axis. J. Endovasc. Ther. 29 (4), 611–622. doi:10.1177/15266028211057086
Marquart, T. J., Allen, R. M., Ory, D. S., and Baldán, A. (2010). miR-33 links SREBP-2 induction to repression of sterol transporters. Proc. Natl. Acad. Sci. U. S. A. 107 (27), 12228–12232. doi:10.1073/pnas.1005191107
Martinez-Arroyo, O., Ortega, A., Flores-Chova, A., Sanchez-Garcia, B., Garcia-Garcia, A. B., Chaves, F. J., et al. (2023). High miR-126-3p levels associated with cardiovascular events in a general population. Eur. J. Intern Med. 113, 49–56. doi:10.1016/j.ejim.2023.04.013
Mathur, P., Ostadal, B., Romeo, F., and Mehta, J. L. (2015). Gender-related differences in atherosclerosis. Cardiovasc Drugs Ther. 29 (4), 319–327. doi:10.1007/s10557-015-6596-3
Mehta, S. L., Dempsey, R. J., and Vemuganti, R. (2020). Role of circular RNAs in brain development and CNS diseases. Prog. Neurobiol. 186, 101746. doi:10.1016/j.pneurobio.2020.101746
Meng, F., Han, L., Liang, Q., Lu, S., Huang, Y., and Liu, J. (2022a). The lnc-RNA APPAT suppresses human aortic smooth muscle cell proliferation and migration by interacting with MiR-647 and FGF5 in atherosclerosis. J. Endovasc. Ther., 152660282211122. doi:10.1177/15266028221112247
Meng, H., Ruan, J., Yan, Z., Chen, Y., Liu, J., Li, X., et al. (2022b). New progress in early diagnosis of atherosclerosis. Int. J. Mol. Sci. 23 (16), 8939. doi:10.3390/ijms23168939
Min, L., Shao, S., Wu, X., Cong, L., Liu, P., Zhao, H., et al. (2013). Anti-inflammatory and anti-thrombogenic effects of atorvastatin in acute ischemic stroke. Neural Regen. Res. 8 (23), 2144–2154. doi:10.3969/j.issn.1673-5374.2013.23.004
Moore, K. J., Sheedy, F. J., and Fisher, E. A. (2013). Macrophages in atherosclerosis: A dynamic balance. Nat. Rev. Immunol. 13 (10), 709–721. doi:10.1038/nri3520
Moreau, P. R., Tomas Bosch, V., Bouvy-Liivrand, M., Õunap, K., Örd, T., Pulkkinen, H. H., et al. (2021). Profiling of primary and mature miRNA expression in atherosclerosis-associated cell types. Arterioscler. Thromb. Vasc. Biol. 41 (7), 2149–2167. doi:10.1161/atvbaha.121.315579
Mushenkova, N. V., Summerhill, V. I., Zhang, D., Romanenko, E. B., Grechko, A. V., and Orekhov, A. N. (2020). Current advances in the diagnostic imaging of atherosclerosis: insights into the pathophysiology of vulnerable plaque. Int. J. Mol. Sci. 21 (8), 2992. doi:10.3390/ijms21082992
Nam, J. P., Nam, K., Jung, S., Nah, J. W., and Kim, S. W. (2015). Evaluation of dendrimer type bio-reducible polymer as a siRNA delivery carrier for cancer therapy. J. Control Release 209, 179–185. doi:10.1016/j.jconrel.2015.04.039
Ni, H., Haemmig, S., Deng, Y., Chen, J., Simion, V., Yang, D., et al. (2021). A smooth muscle cell-enriched long noncoding RNA regulates cell plasticity and atherosclerosis by interacting with serum response factor. Arterioscler. Thromb. Vasc. Biol. 41 (9), 2399–2416. doi:10.1161/atvbaha.120.315911
Ouimet, M., Ediriweera, H., Afonso, M. S., Ramkhelawon, B., Singaravelu, R., Liao, X., et al. (2017). microRNA-33 regulates macrophage autophagy in atherosclerosis. Arterioscler. Thromb. Vasc. Biol. 37 (6), 1058–1067. doi:10.1161/atvbaha.116.308916
Palazzo, A. F., and Lee, E. S. (2015). Non-coding RNA: what is functional and what is junk? Front. Genet. 6, 2. doi:10.3389/fgene.2015.00002
Rafiq, M., Dandare, A., Javed, A., Liaquat, A., Raja, A. A., Awan, H. M., et al. (2023). Competing endogenous RNA regulatory networks of hsa_circ_0126672 in pathophysiology of coronary heart disease. Genes (Basel) 14 (3), 550. doi:10.3390/genes14030550
Raitoharju, E., Lyytikäinen, L. P., Levula, M., Oksala, N., Mennander, A., Tarkka, M., et al. (2011). miR-21, miR-210, miR-34a, and miR-146a/b are up-regulated in human atherosclerotic plaques in the Tampere Vascular Study. Atherosclerosis 219 (1), 211–217. doi:10.1016/j.atherosclerosis.2011.07.020
Rayner, K. J., Suárez, Y., Dávalos, A., Parathath, S., Fitzgerald, M. L., Tamehiro, N., et al. (2010). MiR-33 contributes to the regulation of cholesterol homeostasis. Science 328 (5985), 1570–1573. doi:10.1126/science.1189862
Riccardi, G., Giosuè, A., Calabrese, I., and Vaccaro, O. (2022). Dietary recommendations for prevention of atherosclerosis. Cardiovasc Res. 118 (5), 1188–1204. doi:10.1093/cvr/cvab173
Ross, R. (1999). Atherosclerosis--an inflammatory disease. N. Engl. J. Med. 340 (2), 115–126. doi:10.1056/nejm199901143400207
Ruan, C., Mao, X., Chen, S., Wu, S., and Wang, W. (2022). Subclinical atherosclerosis could increase the risk of hearing impairment in males: A community-based cross-sectional survey of the Kailuan study. Front. Neurosci. 16, 813628. doi:10.3389/fnins.2022.813628
Saenz-Pipaon, G., and Dichek, D. A. (2022). Targeting and delivery of microRNA-targeting antisense oligonucleotides in cardiovascular diseases. Atherosclerosis 374, 44–54. doi:10.1016/j.atherosclerosis.2022.12.003
Salmena, L., Poliseno, L., Tay, Y., Kats, L., and Pandolfi, P. P. (2011). A ceRNA hypothesis: the rosetta stone of a hidden RNA language? Cell 146 (3), 353–358. doi:10.1016/j.cell.2011.07.014
Sandmann, C. L., Schulz, J. F., Ruiz-Orera, J., Kirchner, M., Ziehm, M., Adami, E., et al. (2023). Evolutionary origins and interactomes of human, young microproteins and small peptides translated from short open reading frames. Mol. Cell 83 (6), 994–1011.e18. doi:10.1016/j.molcel.2023.01.023
Santovito, D., Egea, V., Bidzhekov, K., Natarelli, L., Mourão, A., Blanchet, X., et al. (2020a). Noncanonical inhibition of caspase-3 by a nuclear microRNA confers endothelial protection by autophagy in atherosclerosis. Sci. Transl. Med. 12 (546), eaaz2294. doi:10.1126/scitranslmed.aaz2294
Santovito, D., Marcantonio, P., Mastroiacovo, D., Natarelli, L., Mandolini, C., De Nardis, V., et al. (2020b). High dose rosuvastatin increases ABCA1 transporter in human atherosclerotic plaques in a cholesterol-independent fashion. Int. J. Cardiol. 299, 249–253. doi:10.1016/j.ijcard.2019.07.094
Santovito, D., and Weber, C. (2022). Non-canonical features of microRNAs: paradigms emerging from cardiovascular disease. Nat. Rev. Cardiol. 19 (9), 620–638. doi:10.1038/s41569-022-00680-2
Scheideler, M., Vidakovic, I., and Prassl, R. (2020). Lipid nanocarriers for microRNA delivery. Chem. Phys. Lipids 226, 104837. doi:10.1016/j.chemphyslip.2019.104837
Schober, A., Blay, R. M., Saboor Maleki, S., Zahedi, F., Winklmaier, A. E., Kakar, M. Y., et al. (2021). MicroRNA-21 controls circadian regulation of apoptosis in atherosclerotic lesions. Circulation 144 (13), 1059–1073. doi:10.1161/circulationaha.120.051614
Schober, A., Nazari-Jahantigh, M., Wei, Y., Bidzhekov, K., Gremse, F., Grommes, J., et al. (2014). MicroRNA-126-5p promotes endothelial proliferation and limits atherosclerosis by suppressing Dlk1. Nat. Med. 20 (4), 368–376. doi:10.1038/nm.3487
Scholz, J., Weil, P. P., Pembaur, D., Koukou, G., Aydin, M., Hauert, D., et al. (2022). An adenoviral vector as a versatile tool for delivery and expression of miRNAs. Viruses 14 (9), 1952. doi:10.3390/v14091952
Shan, E., Yu, Y., Tang, W., Wang, W., Wang, X., Zhou, S., et al. (2023). miR-330-3p alleviates the progression of atherosclerosis by downregulating AQP9. Funct. Integr. Genomics 23 (2), 77. doi:10.1007/s10142-023-01001-7
Shao, H., Im, H., Castro, C. M., Breakefield, X., Weissleder, R., and Lee, H. (2018). New technologies for analysis of extracellular vesicles. Chem. Rev. 118 (4), 1917–1950. doi:10.1021/acs.chemrev.7b00534
Sharma, A. R., Sharma, G., Bhattacharya, M., Lee, S. S., and Chakraborty, C. (2022). Circulating miRNA in atherosclerosis: A clinical biomarker and early diagnostic tool. Curr. Mol. Med. 22 (3), 250–262. doi:10.2174/1566524021666210315124438
Shen, J., Li, X., Zhang, X., Li, Z., Abulaiti, G., Liu, Y., et al. (2022). Effects of Xinjiang wild cherry plum (Prunus divaricata Ledeb) anthocyanin-rich extract on the plasma metabolome of atherosclerotic apoE-deficient mice fed a high-fat diet. Front. Nutr. 9, 923699. doi:10.3389/fnut.2022.923699
Shi, L., Yu, C., Tian, X., Ma, C., Wang, L., Xia, D., et al. (2019). Effect of microRNA-133a-3p/matrix metalloproteinase-9 axis on the growth of atherosclerotic vascular smooth muscle cells. Exp. Ther. Med. 18 (6), 4356–4362. doi:10.3892/etm.2019.8070
Simion, V., Haemmig, S., and Feinberg, M. W. (2019). LncRNAs in vascular biology and disease. Vasc. Pharmacol. 114, 145–156. doi:10.1016/j.vph.2018.01.003
Simion, V., Zhou, H., Haemmig, S., Pierce, J. B., Mendes, S., Tesmenitsky, Y., et al. (2020). A macrophage-specific lncRNA regulates apoptosis and atherosclerosis by tethering HuR in the nucleus. Nat. Commun. 11 (1), 6135. doi:10.1038/s41467-020-19664-2
Singh, D., Rai, V., and Agrawal, D. K. (2022). Non-Coding RNAs in regulating plaque progression and remodeling of extracellular matrix in atherosclerosis. Int. J. Mol. Sci. 23 (22), 13731. doi:10.3390/ijms232213731
Sletten, A. C., Davidson, J. W., Yagabasan, B., Moores, S., Schwaiger-Haber, M., Fujiwara, H., et al. (2021). Loss of SNORA73 reprograms cellular metabolism and protects against steatohepatitis. Nat. Commun. 12 (1), 5214. doi:10.1038/s41467-021-25457-y
Song, Z., Han, Q., Wen, Z., Lv, Q., Pan, C., and Pan, Y. (2023). LncRNA RASSF8-AS1 knockdown displayed antiproliferative and proapoptotic effects through miR-188-3p/ATG7 pathway in ox-LDL-treated vascular smooth muscle cells. Ann. Transl. Med. 11 (3), 143. doi:10.21037/atm-22-6457
Sun, W., Zhao, P., Zhou, Y., Xing, C., Zhao, L., Li, Z., et al. (2020). Ultrasound targeted microbubble destruction assisted exosomal delivery of miR-21 protects the heart from chemotherapy associated cardiotoxicity. Biochem. Biophys. Res. Commun. 532 (1), 60–67. doi:10.1016/j.bbrc.2020.05.044
Tang, Y., Yan, J. H., Ge, Z. W., Fei, A. H., and Zhang, Y. C. (2022). LncRNA Gaplinc promotes the pyroptosis of vascular endothelial cells through SP1 binding to enhance NLRP3 transcription in atherosclerosis. Cell Signal 99, 110420. doi:10.1016/j.cellsig.2022.110420
Terashima, T., Ogawa, N., Nakae, Y., Sato, T., Katagi, M., Okano, J., et al. (2018). Gene therapy for neuropathic pain through siRNA-IRF5 gene delivery with homing peptides to microglia. Mol. Ther. Nucleic Acids 11, 203–215. doi:10.1016/j.omtn.2018.02.007
Toriuchi, K., Kihara, T., Aoki, H., Kakita, H., Takeshita, S., Ueda, H., et al. (2023). Monocyte-derived miRNA-1914-5p attenuates IL-1β-induced monocyte adhesion and transmigration. Int. J. Mol. Sci. 24 (3), 2829. doi:10.3390/ijms24032829
Tyrrell, D. J., and Goldstein, D. R. (2021). Ageing and atherosclerosis: vascular intrinsic and extrinsic factors and potential role of IL-6. Nat. Rev. Cardiol. 18 (1), 58–68. doi:10.1038/s41569-020-0431-7
Vacante, F., Rodor, J., Lalwani, M. K., Mahmoud, A. D., Bennett, M., De Pace, A. L., et al. (2021). CARMN loss regulates smooth muscle cells and accelerates atherosclerosis in mice. Circ. Res. 128 (9), 1258–1275. doi:10.1161/circresaha.120.318688
Vadali, S., and Post, S. R. (2014). Lipid rafts couple class A scavenger receptors to phospholipase A2 activation during macrophage adhesion. J. Leukoc. Biol. 96 (5), 873–881. doi:10.1189/jlb.2A0414-214R
Virani, S. S., Alonso, A., Aparicio, H. J., Benjamin, E. J., Bittencourt, M. S., Callaway, C. W., et al. (2021). Heart disease and stroke statistics-2021 update: A report from the American heart association. Circulation 143 (8), e254–e743. doi:10.1161/cir.0000000000000950
Wang, J., Xu, X., Li, P., Zhang, B., and Zhang, J. (2021). HDAC3 protects against atherosclerosis through inhibition of inflammation via the microRNA-19b/PPARγ/NF-κB axis. Atherosclerosis 323, 1–12. doi:10.1016/j.atherosclerosis.2021.02.013
Wang, K., Huang, X. T., Miao, Y. P., Bai, X. L., and Jin, F. (2022a). MiR-148a-3p attenuates apoptosis and inflammation by targeting CNTN4 in atherosclerosis. Ann. Transl. Med. 10 (22), 1201. doi:10.21037/atm-22-3768
Wang, X. S., Ding, X. Z., Li, X. C., He, Y., Kong, D. J., Zhang, L., et al. (2018). A highly integrated precision nanomedicine strategy to target esophageal squamous cell cancer molecularly and physically. Nanomedicine 14 (7), 2103–2114. doi:10.1016/j.nano.2018.06.008
Wang, Z., Wang, H., Guo, C., Yu, F., Zhang, Y., Qiao, L., et al. (2022b). Role of hsa_circ_0000280 in regulating vascular smooth muscle cell function and attenuating neointimal hyperplasia via ELAVL1. Cell Mol. Life Sci. 80 (1), 3. doi:10.1007/s00018-022-04602-w
Wei, Y., Zhu, M., Corbalán-Campos, J., Heyll, K., Weber, C., and Schober, A. (2015). Regulation of Csf1r and Bcl6 in macrophages mediates the stage-specific effects of microRNA-155 on atherosclerosis. Arterioscler. Thromb. Vasc. Biol. 35 (4), 796–803. doi:10.1161/atvbaha.114.304723
Wolf, D., and Ley, K. (2019). Immunity and inflammation in atherosclerosis. Circ. Res. 124 (2), 315–327. doi:10.1161/circresaha.118.313591
Wu, G., Zhang, J., Zhao, Q., Zhuang, W., Ding, J., Zhang, C., et al. (2020). Molecularly engineered macrophage-derived exosomes with inflammation tropism and intrinsic heme biosynthesis for atherosclerosis treatment. Angew. Chem. Int. Ed. Engl. 59 (10), 4068–4074. doi:10.1002/anie.201913700
Wu, Y., Deng, C., Xu, J., Wang, W., Chen, Y., Qin, X., et al. (2023). Enhanced local delivery of microRNA-145a-5P into mouse aorta via ultrasound-targeted microbubble destruction inhibits atherosclerotic plaque formation. Mol. Pharm. 20 (2), 1086–1095. doi:10.1021/acs.molpharmaceut.2c00799
Xie, X., Urabe, G., Marcho, L., Williams, C., Guo, L. W., and Kent, K. C. (2020). Smad3 regulates neuropilin 2 transcription by binding to its 5' untranslated region. J. Am. Heart Assoc. 9 (8), e015487. doi:10.1161/jaha.119.015487
Xing, X., Li, Z., Yang, X., Li, M., Liu, C., Pang, Y., et al. (2020). Adipose-derived mesenchymal stem cells-derived exosome-mediated microRNA-342-5p protects endothelial cells against atherosclerosis. Aging (Albany NY) 12 (4), 3880–3898. doi:10.18632/aging.102857
Xiu, J., Yang, Z., Sui, Y., Zhang, L., and Zhou, Y. (2022). CircNMD3 relieves endothelial cell injury induced by oxidatively modified low-density lipoprotein through regulating miR-498/BMP and activin membrane-bound inhibitor (BAMBI) axis. Bioengineered 13 (5), 12558–12571. doi:10.1080/21655979.2022.2065813
Xu, F., Yao, F., and Ning, Y. (2023). MicroRNA-202-5p-dependent inhibition of Bcl-2 contributes to macrophage apoptosis and atherosclerotic plaque formation. Gene 867, 147366. doi:10.1016/j.gene.2023.147366
Xu, S., Pelisek, J., and Jin, Z. G. (2018). Atherosclerosis is an epigenetic disease. Trends Endocrinol. Metab. 29 (11), 739–742. doi:10.1016/j.tem.2018.04.007
Yan, Y., Li, X. Q., Duan, J. L., Bao, C. J., Cui, Y. N., Su, Z. B., et al. (2019). Nanosized functional miRNA liposomes and application in the treatment of TNBC by silencing Slug gene. Int. J. Nanomedicine 14, 3645–3667. doi:10.2147/ijn.S207837
Yang, L., Chen, W., Li, B., Hu, Y., Lu, H., Zhang, P., et al. (2023a). Circular RNA circ_0026218 suppressed atherosclerosis progression via miR-338-3p/SIRT6 Axis. Biomed. Res. Int. 2023, 5647758. doi:10.1155/2023/5647758
Yang, Q., Chen, S., Wang, X., Yang, X., Chen, L., Huang, T., et al. (2023b). Exercise mitigates endothelial pyroptosis and atherosclerosis by downregulating NEAT1 through N6-methyladenosine modifications. Arterioscler. Thromb. Vasc. Biol. 43, 910–926. doi:10.1161/atvbaha.123.319251
Yang, Y., Li, M., Liu, Y., Wang, Z., Fu, X., He, X., et al. (2022). The lncRNA punisher regulates apoptosis and mitochondrial homeostasis of vascular smooth muscle cells via targeting miR-664a-5p and OPA1. Oxid. Med. Cell Longev. 2022, 5477024. doi:10.1155/2022/5477024
Ye, B., Liang, X., Zhao, Y., Cai, X., Wang, Z., Lin, S., et al. (2022). Hsa_circ_0007478 aggravates NLRP3 inflammasome activation and lipid metabolism imbalance in ox-LDL-stimulated macrophage via miR-765/EFNA3 axis. Chem. Biol. Interact. 368, 110195. doi:10.1016/j.cbi.2022.110195
Ye, M., Ni, Q., Wang, H., Wang, Y., Yao, Y., Li, Y., et al. (2023). CircRNA circCOL1A1 acts as a sponge of miR-30a-5p to promote vascular smooth cell phenotype switch through regulation of Smad1 expression. Thromb. Haemost. 123 (1), 97–107. doi:10.1055/s-0042-1757875
You, L., Zheng, Y., Yang, J., Hou, Q., Wang, L., Zhang, Y., et al. (2022). LncRNA MDRL mitigates atherosclerosis through miR-361/SQSTM1/NLRP3 signaling. Mediat. Inflamm. 2022, 5463505. doi:10.1155/2022/5463505
Yuan, R., Xin, Q., Ma, X., Yu, M., Miao, Y., Chen, K., et al. (2023). Identification of a novel angiogenesis signalling circSCRG1/miR-1268b/NR4A1 pathway in atherosclerosis and the regulatory effects of TMP-PF in vitro. Molecules 28 (3), 1271. doi:10.3390/molecules28031271
Zealy, R. W., Wrenn, S. P., Davila, S., Min, K. W., and Yoon, J. H. (2017). microRNA-binding proteins: specificity and function. Wiley Interdiscip. Rev. RNA 8 (5), e1414. doi:10.1002/wrna.1414
Zhai, C., Cong, H., Hou, K., Hu, Y., Zhang, J., Zhang, Y., et al. (2020). Effects of miR-124-3p regulation of the p38MAPK signaling pathway via MEKK3 on apoptosis and proliferation of macrophages in mice with coronary atherosclerosis. Adv. Clin. Exp. Med. 29 (7), 803–812. doi:10.17219/acem/121926
Zhang, B., Zhang, Y. F., Li, R., Zhao, L., Qin, S. G., Pan, L. F., et al. (2020). MiR-217 inhibits apoptosis of atherosclerotic endothelial cells via the TLR4/PI3K/Akt/NF-κB pathway. Eur. Rev. Med. Pharmacol. Sci. 24 (24), 12867–12877. doi:10.26355/eurrev_202012_24190
Zhang, F., Zhang, R., Zhang, X., Wu, Y., Li, X., Zhang, S., et al. (2018). Comprehensive analysis of circRNA expression pattern and circRNA-miRNA-mRNA network in the pathogenesis of atherosclerosis in rabbits. Aging (Albany NY) 10 (9), 2266–2283. doi:10.18632/aging.101541
Zhang, M., Zhu, Y., Zhu, J., Xie, Y., Wu, R., Zhong, J., et al. (2022a). circ_0086296 induced atherosclerotic lesions via the IFIT1/STAT1 feedback loop by sponging miR-576-3p. Cell Mol. Biol. Lett. 27 (1), 80. doi:10.1186/s11658-022-00372-2
Zhang, P., Cao, L., Fan, P., Mei, Y., and Wu, M. (2016). LncRNA-MIF, a c-Myc-activated long non-coding RNA, suppresses glycolysis by promoting Fbxw7-mediated c-Myc degradation. EMBO Rep. 17 (8), 1204–1220. doi:10.15252/embr.201642067
Zhang, S., Sun, Y., Xiao, Q., Niu, M., Pan, X., and Zhu, X. (2023a). Lnc_000048 promotes histone H3K4 methylation of MAP2K2 to reduce plaque stability by recruiting KDM1A in carotid atherosclerosis. Mol. Neurobiol. 60 (5), 2572–2586. doi:10.1007/s12035-023-03214-0
Zhang, X., Rotllan, N., Canfrán-Duque, A., Sun, J., Toczek, J., Moshnikova, A., et al. (2022b). Targeted suppression of miRNA-33 using pHLIP improves atherosclerosis regression. Circ. Res. 131 (1), 77–90. doi:10.1161/circresaha.121.320296
Zhang, Y., Chen, J., He, S., Xiao, Y., Liu, A., Zhang, D., et al. (2022c). Systematic identification of aberrant non-coding RNAs and their mediated modules in rotator cuff tears. Front. Mol. Biosci. 9, 940290. doi:10.3389/fmolb.2022.940290
Zhang, Z., Qiu, Y., Li, W., Tang, A., Huang, H., Yao, W., et al. (2023b). Astaxanthin alleviates foam cell formation and promotes cholesterol efflux in ox-LDL-induced RAW264.7 cells via CircTPP2/miR-3073b-5p/ABCA1 pathway. Molecules 28 (4), 1701. doi:10.3390/molecules28041701
Zhao, K., Zhao, Q., Guo, Z., Chen, Z., Hu, Y., Su, J., et al. (2018a). Hsa_Circ_0001275: A potential novel diagnostic biomarker for postmenopausal osteoporosis. Cell Physiol. Biochem. 46 (6), 2508–2516. doi:10.1159/000489657
Zhao, S., Tang, X., Miao, Z., Chen, Y., Cao, J., Song, T., et al. (2022). Hsp90 S-nitrosylation at Cys521, as a conformational switch, modulates cycling of Hsp90-AHA1-CDC37 chaperone machine to aggravate atherosclerosis. Redox Biol. 52, 102290. doi:10.1016/j.redox.2022.102290
Zhao, S., Wang, H., Xu, H., Tan, Y., Zhang, C., Zeng, Q., et al. (2021). Targeting the microRNAs in exosome: A potential therapeutic strategy for alleviation of diabetes-related cardiovascular complication. Pharmacol. Res. 173, 105868. doi:10.1016/j.phrs.2021.105868
Zhao, W., Geng, D., Li, S., Chen, Z., and Sun, M. (2018b). LncRNA HOTAIR influences cell growth, migration, invasion, and apoptosis via the miR-20a-5p/HMGA2 axis in breast cancer. Cancer Med. 7 (3), 842–855. doi:10.1002/cam4.1353
Zhou, J., Niu, C., Huang, B., Chen, S., Yu, C., Cao, S., et al. (2022). Platelet membrane biomimetic nanoparticles combined with UTMD to improve the stability of atherosclerotic plaques. Front. Chem. 10, 868063. doi:10.3389/fchem.2022.868063
Zhu, F., Chang, G., Tang, X., Gao, L., and Zhang, N. (2023). Doxorubicin inhibits cholesterol efflux through the miR-33/ABCA1 pathway. Biochem. Biophys. Res. Commun. 644, 149–154. doi:10.1016/j.bbrc.2023.01.024
Zhu, F., and Ren, Z. (2022). Icariin inhibits the malignant progression of lung cancer by affecting the PI3K/Akt pathway through the miR-205-5p/PTEN axis. Oncol. Rep. 47 (6), 115. doi:10.3892/or.2022.8326
Zhu, J., Liu, B., Wang, Z., Wang, D., Ni, H., Zhang, L., et al. (2019a). Exosomes from nicotine-stimulated macrophages accelerate atherosclerosis through miR-21-3p/PTEN-mediated VSMC migration and proliferation. Theranostics 9 (23), 6901–6919. doi:10.7150/thno.37357
Keywords: atherosclerosis, ncRNAs, cardiovascular disease, targeted therapy of atherosclerosis, miRNAs
Citation: Yu Z, Yin J, Tang Z, Hu T, Wang Z, Chen Y, Liu T and Zhang W (2023) Non-coding RNAs are key players and promising therapeutic targets in atherosclerosis. Front. Cell Dev. Biol. 11:1237941. doi: 10.3389/fcell.2023.1237941
Received: 10 June 2023; Accepted: 22 August 2023;
Published: 01 September 2023.
Edited by:
Malene Lindholm, Stanford University, United StatesReviewed by:
Donato Santovito, Ludwig Maximilian University of Munich, GermanyBernardo Bonilauri, Stanford University, United States
Copyright © 2023 Yu, Yin, Tang, Hu, Wang, Chen, Liu and Zhang. This is an open-access article distributed under the terms of the Creative Commons Attribution License (CC BY). The use, distribution or reproduction in other forums is permitted, provided the original author(s) and the copyright owner(s) are credited and that the original publication in this journal is cited, in accordance with accepted academic practice. No use, distribution or reproduction is permitted which does not comply with these terms.
*Correspondence: Ying Chen, chenying66323@163.com; Tianjia Liu, 267089397@qq.com; Wei Zhang, 156696202@qq.com