- 1Collaborative Innovation Center of Yangtze River Delta Region Green Pharmaceuticals, College of Pharmaceutical Sciences, Zhejiang University of Technology, Hangzhou, China
- 2Department of Molecular, Cellular, and Developmental Biology, University of Michigan, Ann Arbor, MI, United States
Macroautophagy/autophagy is a lysosome-dependent catabolic pathway for the degradation of intracellular proteins and organelles. Autophagy dysfunction is related to many diseases, including lysosomal storage diseases, cancer, neurodegenerative diseases, cardiomyopathy, and chronic metabolic diseases, in which increased reactive oxygen species (ROS) levels are also observed. ROS can randomly oxidize proteins, lipids, and DNA, causing oxidative stress and damage. Cells have developed various antioxidant pathways to reduce excessive ROS and maintain redox homeostasis. Treatment targeting only one aspect of diseases with autophagy dysfunction and oxidative stress shows very limited effects. Herein, identifying the bridging factors that can regulate both autophagy and antioxidant pathways is beneficial for dual-target therapies. This review intends to provide insights into the current identified bridging factors that connect autophagy and Nrf2 antioxidant pathway, as well as their tight interconnection with each other. These factors could be potential dual-purpose targets for the treatment of diseases implicated in both autophagy dysfunction and oxidative stress.
1 Introduction
Autophagy is a metabolic process that takes place in eukaryotic cells, which use lysosomes to degrade proteins and damaged organelles for self-eating and self-renewal (Wong et al., 2020). Autophagy dysfunction contributes to a variety of pathologies, including lysosomal storage diseases, neurodegenerative diseases, chronic metabolic diseases, and cancer (Klionsky et al., 2021). Of note, these diseases are usually accompanied by elevated levels of reactive oxygen species (ROS) (Giordano et al., 2014; Ryter et al., 2019; Wu W. et al., 2021).
ROS are highly reactive and diffusive molecules, and endogenous ROS mainly come from cellular respiration in mitochondria (Wang et al., 2021; Sies et al., 2022). In addition, various organelles (endoplasmic reticulum and peroxisome) and enzymes (NADPH oxidase and p450 cytochrome) also produce ROS during metabolic processes (Wang et al., 2021; Sies et al., 2022). ROS are regarded as a “double-edged sword.” Physiologically low levels of ROS serve as a signal, promoting cell viability and immune function and maintaining cell homeostasis (Sies and Jones, 2020; Sies et al., 2022). Conversely, excessive ROS oxidize and damage cellular components, leading to cellular death (Davalli et al., 2016), which is implicated in many diseases (Luo et al., 2020; Yang and Lian, 2020). To maintain ROS at low levels, cells are controlled by multiple antioxidant systems, including various non-enzymatic and enzymatic antioxidant pathways (Alkadi, 2020; Forman and Zhang, 2021), as well as several antioxidant transcription factors. Furthermore, these antioxidants have different subcellular localizations, therefore eliminating ROS in different compartments (Brieger et al., 2012).
Interestingly, ROS also interplay with autophagy (Gao et al., 2020). Autophagy can participate in the redox balance by clearing oxidative stress-damaged molecules and organelles (Yun et al., 2020). The Nrf2-Keap1-ARE pathway plays a central role in adaptive cellular redox response (Shaw and Chattopadhyay, 2020; Esteras and Abramov, 2022). Studies have reported that several proteins serve as bridges between the Nrf2 pathway and autophagy (Li et al., 2021a; Zhang et al., 2021). In this review, we focus on the bridging factors that establish networks between autophagy and the Nrf2 antioxidant pathway.
1.1 ROS, oxidative stress, and antioxidant pathways
ROS define several highly reactive, short-lived molecules, which are formed during the oxygen reduction process (Shadel and Horvath, 2015). Intracellular ROS are mainly produced by the electron transport chain of mitochondria and NADPH oxidase (Lennicke and Cochemé, 2021). Other sources that generate ROS include the endoplasmic reticulum, peroxisome, and nucleus (Lennicke and Cochemé, 2021). Endogenous ROS generally contain superoxide (O2−), hydrogen peroxide (H2O2), hydroxyl radicals, and oxygen anions (Shadel and Horvath, 2015). Physiological ROS act as signaling molecules, regulating physiological and metabolic functions and promoting cell proliferation and innate immune response (Mittler, 2017; Forrester et al., 2018); conversely, pathological or exogenous stimulus can induce ROS production and accumulation (Zorov et al., 2014; Yuan et al., 2019). To maintain redox homeostasis, cells have developed diverse antioxidant pathways to counteract ROS (Figure 1). However, in response to pathological or exogenous stimuli, antioxidant pathways are overloaded with excessive ROS, leading to oxidative stress, which causes damage to lipids, proteins, and DNA (Sies, 2015; Forman and Zhang, 2021). Various non-enzymatic and enzymatic antioxidant pathways are involved in reducing ROS and preventing oxidative stress, including superoxide dismutases, thioredoxin, catalases, peroxidases, and glutathione (Elias et al., 2008; He et al., 2017). Furthermore, multiple transcriptional antioxidant pathways, i.e., Nrf2, NF-κB, FoxO, and p53, make an important contribution to anti-oxidation (Marinho et al., 2014) (Figure 1). In particular, the Nrf2-Keap1-ARE pathway plays a central role in the regulation of antioxidant enzyme genes and cytoprotective defense (Lu et al., 2016; Liu S. et al., 2022).
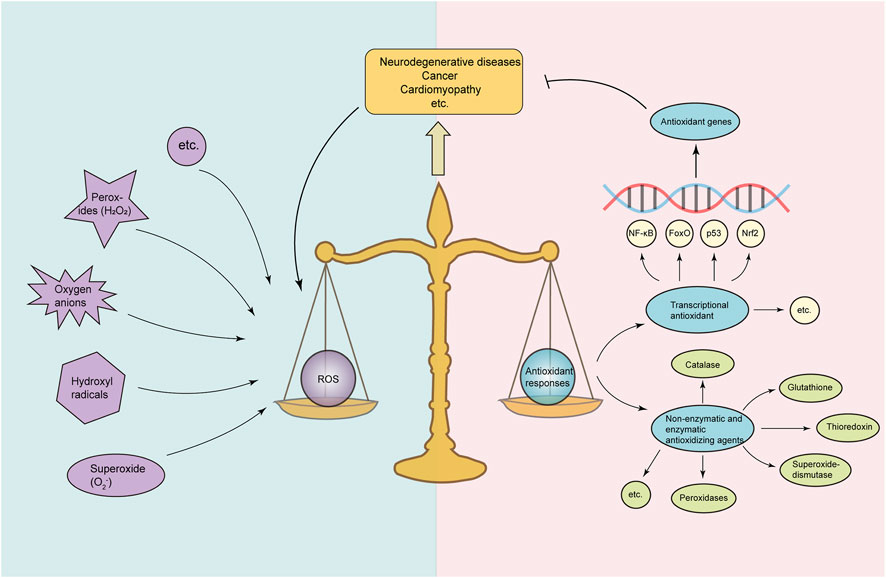
FIGURE 1. Balance between ROS and antioxidant responses. Under normal conditions, cells maintain redox homeostasis by balancing ROS levels and antioxidant activity. Cellular ROS including oxygen anions, superoxide (O2−), hydroxyl radicals, and hydrogen peroxides (H2O2) and antioxidant responses consisting of non-enzymatic and enzymatic antioxidant pathways (peroxidases, superoxide dismutase, thioredoxin, and glutathione) and transcriptional antioxidant pathways (including Nrf2, NF-κB, FoxO, and p53) form a balance. Under certain pathological conditions such as neurodegenerative diseases, cancer, and cardiomyopathy, excessive ROS accumulation overloads the antioxidant responses and breaks the cellular homeostasis, resulting in oxidative stress and mitochondrial dysfunction, contributing to the progression of diseases.
1.2 Nrf2-Keap1-ARE signaling pathway
Nuclear factor erythroid 2-related factor 2 (Nrf2) is a basic leucine zipper transcription factor belonging to the Cap ‘n’ Collar family. Under quiescent conditions, cytosolic Nrf2 directly binds to Kelch-like ECH-associated protein 1 (Keap1), a substrate adapter protein for the Cul3–E3–ligase complex, resulting in rapid degradation of Nrf2 (Ulasov et al., 2022). Keap1 is also a cysteine (Cys)-rich sensor of redox damage (Yamamoto et al., 2018). However, with exposure to stimuli such as oxidative/xenobiotic stress, Nrf2 escapes from Keap1-mediated repression and translocates to the nucleus, where it dimerizes with small musculoaponeurotic fibrosarcoma proteins (sMaf). The activated Nrf2-sMaf heterodimer binds to antioxidant response element (ARE) sequences in the promoter of a tandem of antioxidant genes, including heme oxygenase-1 (HMOX1), aldo-keto reductases, glutathione, and glutathione-S-transferase (Hayes and Dinkova-Kostova, 2014; Tonelli et al., 2018), to trigger their expression. Numerous studies have revealed the complexity and diversity of the Nrf2-Keap1 pathway in regulating biological processes, including cell proliferation, differentiation, anti-inflammation, and cytoprotection (Murakami and Motohashi, 2015; Yamamoto et al., 2018). Nrf2 has also been demonstrated to regulate mitochondrial biogenesis (Esteras and Abramov, 2022). Two key regulators of mitochondrial biogenesis, namely, proliferator-activated receptor gamma coactivator 1-α and nuclear respiratory factor 1, which are responsible for mitochondrial DNA transcription, are under the control of Nrf2 (Piantadosi et al., 2008; Baldelli et al., 2013; Merry and Ristow, 2016).
It is known that ROS also interact with autophagy. ROS could promote the formation of autophagy. Autophagy, in turn, could reduce oxidative damage by engulfing and degrading oxidized substances.
1.3 ROS and autophagy interplay
Autophagy is a catabolic process that is responsible for the degradation of intracellular proteins and damaged organelles in response to endogenous and exogenous stresses, including oxidative stress, endoplasmic reticulum stress, hypoxic stress, and nutrient and growth factor starvation (Galati et al., 2019). Promoting autophagy has been reported to have beneficial effects on longevity, anti-infection, and disease prevention, including diseases such as myocarditis, tumors, and neurodegenerative diseases (Petrache et al., 2008). Autophagy dysfunction has also been identified in many diseases, including Niemann–Pick type C (NPC) disease, atherosclerosis, and non-alcoholic steatohepatitis (Fischer et al., 2009). It is broadly recognized that excessive ROS can regulate autophagy (activation or inhibition) in various conditions (Gao et al., 2020; Ornatowski et al., 2020; Zhou et al., 2022). Autophagy, in turn, can also reduce oxidative damage by degrading oxidized substances (Li et al., 2015) (Figure 2). Thus, ROS and autophagy interact closely with each other. The process of autophagy is generally divided into the occurrence of phagophores, the formation of autophagosomes, the fusion of autophagosomes and lysosomes, and degradation. A variety of autophagy-related proteins (Atg) constantly regulate the whole process of autophagy (Levine and Kroemer, 2019).
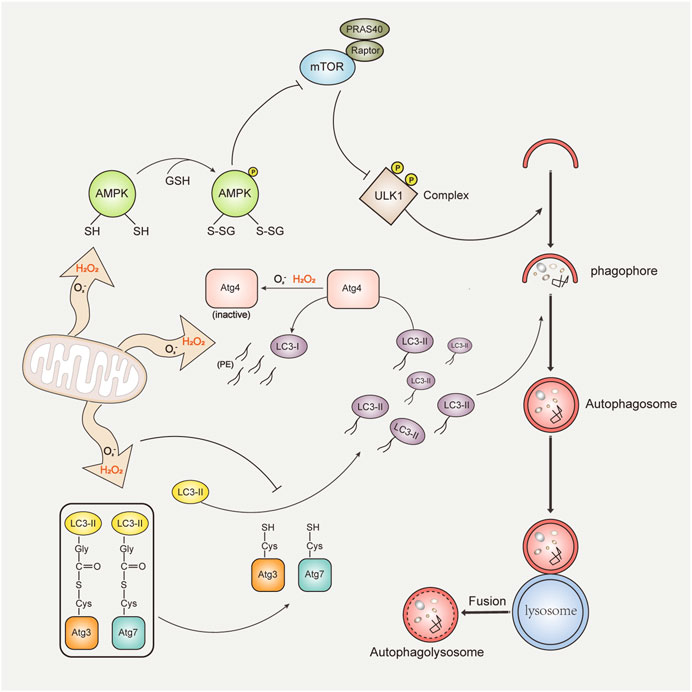
FIGURE 2. ROS and autophagy interplay. Mitochondria-released ROS (mainly H2O2 and O2−) regulate autophagy through three mechanisms: (1) ROS directly oxidize the cysteine residues of α and β subunits of AMPK, and activated AMPK inhibits the activity of the mTORC1 complex or phosphorylates the ULK1 complex, subsequently promoting autophagy (top). (2) Oxidation of Atg4 by ROS results in inactivation of LC3-PE deconjugation activity and accumulation of autophagic LC3-II isoforms, supporting autophagosome formation (middle). (3) The oxidation of catalytic thiols on Atg3 and Atg7 by ROS prevents the lipidation of LC3, thereby inhibiting autophagy (bottom). Autophagy, in turn, contributes to reduction of ROS and damaged organelles through pathways such as mitophagy. Damaged mitochondria are recruited into the autophagosome by binding with LC3. The mature autophagosome fuses with the lysosome to form the autolysosome, and the damaged mitochondria are subsequently degraded.
Previous studies have identified that H2O2 and O2− are major inducers of autophagy (Filomeni et al., 2015). H2O2 is relatively stable, long-lived, and highly selective to cysteine (Bienert and Chaumont, 2014). Instead, O2− is unstable and easily converted to H2O2 spontaneously or catalyzed by enzymes (Fujii et al., 2022). In the initiation stage of autophagy, ROS (H2O2 and O2−) promote autophagy mainly via regulating the mechanistic target of rapamycin (mTOR). mTOR is a receptor of amino acids and ATP that plays a gating role in autophagy. ROS can activate AMP-activated protein kinase (AMPK) or inhibit protein kinase B (Zhao et al., 2017), which represses mTORC1 complex activity and enhances autophagy (Li et al., 2019; Guo et al., 2022). In the autophagosome formation stage, Atg4, an essential protease in autophagy, has been identified as a direct target of H2O2 (Scherz-Shouval et al., 2007). H2O2 directly oxidizes the Cys81 of Atg4 (Scherz-Shouval et al., 2007), thus leading to the inactivation of Atg4 and promoting the lipidation of light chain 3 (LC3, also the mammalian homolog of Atg8), thereby supporting the formation of autophagosomes (Pérez-Pérez et al., 2021). Interestingly, ROS-induced autophagy is primarily caused by O2− during prolonged starvation (Chen et al., 2009). In contrast, H2O2 is produced immediately after starvation and regulates autophagy (Zhou et al., 2022). In addition, the crosstalk between autophagy and ROS also varies in different cell types. For example, in renal tubular cells, increased ROS can activate autophagy, leading to mitochondrial destruction and kidney damage (Duan et al., 2018). However, in tumor cells, ROS-induced autophagy activation can promote cell growth and cancer progression (Taucher et al., 2022).
Acute H2O2 can prevent LC3 lipidation, thereby inhibiting autophagy (Frudd et al., 2018). H2O2 oxidizes the cysteine residues of Atg3 and Atg7, thereby weakening their covalent interaction with LC3 (Frudd et al., 2018). Increased ROS can also inhibit autophagy by downregulating unc-51-like kinase 1 (ULK1) (Ci et al., 2014), an autophagy regulator. In addition, ROS also regulates autophagy through mitogen-activated protein kinase signaling pathways, including p38 kinase, extracellular signal-regulated kinase, and c-Jun amino-terminal kinase (Sui et al., 2014; Liu et al., 2015; Fan et al., 2020). Conversely, autophagy could regulate the levels of intracellular ROS. Selective autophagy, such as mitophagy and pexophagy, contributes to ROS reduction.
1.3.1 Autophagy regulates ROS
Mitochondria are the primary sites of ROS production (approximately 90% of cellular ROS) (Balaban et al., 2005). Thus, mitochondria also contain a large number of antioxidants (proteins and enzymes), including glutathione, glutathione reductase, peroxiredoxin, and superoxide dismutase (Yang et al., 2020). Mitochondrial antioxidants reduce oxidative damage to mitochondria and maintain their homeostasis (Liang et al., 2012; Ristić et al., 2015; Kang et al., 2020). In pathological conditions, excessive ROS leads to oxidative stress, causing mitochondrial dysfunction and cellular damage (Tirichen et al., 2021). The process of specific and selective degradation of damaged mitochondria is called mitophagy. Mitophagy generally consists of two steps: the initiation of general autophagy and the priming of damaged mitochondria for autophagic recognition (Figure 2). Recently, studies have shown that ROS, as cellular signaling molecules, could trigger autophagosome formation and autophagic degradation (Filomeni et al., 2015; Roca-Agujetas et al., 2019).
In the process of mitophagy, mitophagy receptors or ubiquitin-autophagy adapters directly involve themselves in forming autophagosomes surrounding mitochondria and further degrading damaged mitochondria in autolysosomes, which are produced by the fusion of autophagosomes and lysosomes (Onishi et al., 2021). Recognition of receptor-mediated mitophagy depends on receptor proteins such as Atg32 in yeast (Okamoto et al., 2009) and several proteins in mammals, including NIP3-like protein X (Novak et al., 2010), Bcl-2 19-kDa interacting protein 3 (Zhu et al., 2013), FUN14 domain containing 1 (Liu et al., 2012), FK506-binding protein 8 (Bhujabal et al., 2017), and Bcl2-like 13 (Murakawa et al., 2015). These receptors contain a conserved key domain, the LC3-interacting region (LIR) domain, which can directly bind to Atg8/LC3 or other proteins in this family and then trigger mitophagy. In ubiquitin-mediated mitophagy, PTEN-induced putative kinase 1 accumulates on the outer membrane of damaged mitochondria, which promotes the E3 ubiquitin ligase-Parkin to be recruited to the surface of mitochondria. Subsequently, autophagy adapters (Sulkshane et al., 2021) (e.g., Atg 8 family, SQSTM1, a neighbor of BRCA1 gene 1, optineurin, Tax1-binding protein 1, and nuclear dot protein 52 kDa) directly recognize poly-ubiquitin chains on damaged mitochondria and then anchor them to phagocytic vesicles (Sulkshane et al., 2021).
1.3.2 Pexophagy
Peroxisomes are small and highly dynamic organelles that are ubiquitous in eukaryotic cells and play a key role in cell metabolism and the regulation of redox homeostasis (Germain and Kim, 2020). Pexophagy, a process of selective autophagy for damaged peroxisomes, contributes to maintaining the intracellular redox balance (Germain and Kim, 2020). Under ROS stimulation, the peroxisome localization protein PEX5 (peroxisomal biogenesis factor 5) is phosphorylated at Ser141 by ataxia telangiectasia mutated kinase and subsequently ubiquitinated by E3 ligase (Zhang et al., 2015). SQSTM1 then recognizes the ubiquitinated PEX5 complex and triggers pexophagy (Zhang et al., 2015). In addition, PEX2 is identified as an E3 ubiquitin ligase during amino acid starvation (Sargent et al., 2016). PEX2 can ubiquitinate PEX5, thereby activating NBR1-dependent pexophagy (Sargent et al., 2016).
2 Bridging factors connecting autophagy and Nrf2-Keap1 pathway
Both autophagy dysfunction and oxidative stress have been implicated in many diseases. Treatment targeting one aspect shows limited effects. For example, N-acetylcysteine (NAC), a potent antioxidant, has been examined in clinical trials of NPC disease, which shows increased oxidative stress and dysfunctional autophagy (Fu et al., 2013; Wheeler and Sillence, 2020). Results show that NAC significantly reduces oxidative stress in NPC disease but has no effect on autophagic dysfunction-related cholesterol accumulation, thus failing in clinical trials (Fu et al., 2013). Of note, identifying the bridges that connect autophagy and antioxidant pathways could be extremely beneficial to novel dual-target therapies.
2.1 SQSTM1
SQSTM1 (sequestosome1, also called p62) is an autophagy adapter protein that participates in the degradation of protein aggregates and cytoplasmic bodies (Lin et al., 2013). Numerous studies have described the effects of SQSTM1 on a variety of diseases, such as Parkinson’s disease (PD) (Martins-Marques et al., 2015; Chu, 2019; Kumar et al., 2022). Under normal conditions, SQSTM1 recruits α-synuclein to form aggregates and eliminates them through autophagy. In contrast, in PD patients, dysfunctional SQSTM1 fails to recruit impaired α-synuclein, which accumulates in the central nervous system and aggravates PD progression (Shin et al., 2020). In addition, SQSTM1 can recognize ubiquitinated Parkin, a key protein of mitophagy, and disrupted SQSTM1 mediates mitophagy deficiency (Song et al., 2016), which is an important cause of PD.
SQSTM1 recruits ubiquitinated proteins and organelles to the autophagosome through its simultaneous interaction with multiple proteins, including protein kinase C and mTOR (Katsuragi et al., 2015). In autophagy-deficient cells, SQSTM1-induced Nrf2 activation is critical in tumor progression (Komatsu et al., 2010). SQSTM1 can directly bind to Keap1, disrupting Keap1-regulated Nrf2 degradation and promoting aberrant Nrf2-activation (Komatsu et al., 2010). The DPSTGE motif in the Keap1 interaction region (KIR) domain of SQSTM1 resembles the ETGE motif in Nrf2 (Komatsu et al., 2010) and illuminates the competitive binding of Keap1 between SQSTM1 and Nrf2. However, the binding ability of SQSTM1-KIR to Keap1 is much weaker than that of Nrf2-ETGE. Nevertheless, under exposure to ROS or other electrophilic stimuli, Ser351/349 within the DPSTGE motif in the KIR region will be phosphorylated, and consequently, the binding affinity of SQSTM1 to Keap1 can be drastically increased (Ichimura et al., 2013; Sánchez-Martín and Komatsu, 2018).
SQSTM1 can recruit Keap1 and LC3 to form the LC3–SQSTM1–Keap1 complex, which is then degraded by selective autophagy (Liao et al., 2019) (Figure 3). Nevertheless, the interaction between LC3, SQSTM1, and Keap1 still remains controversial, as the binding competition to SQSTM1 between Keap1 and LC3 can be attributed to the spatial adjacency of the LIR domain and KIR domain in SQSTM1 (Jain et al., 2010). Moreover, depleting SQSTM1 with siRNA leads to an almost twofold increase in the half-life of Keap1 (Copple et al., 2010), indicating that SQSTM1 could influence the basal level of Keap1 and regulate its degradation via clearance of the SQSTM1–Keap1 complex by the ubiquitin–proteasome system, which is triggered by binding of the ubiquitin-associated domain (UBA) of SQSTM1. Upon oxidative stress, the escape of Nrf2 from Keap1 also contributes to the formation of LC3–SQSTM1–Keap1 aggregates (Zhang et al., 2021). In addition, Nrf2 regulates SQSTM1 gene expression (Ho and Gorski, 2019). Overall, an SQSTM1–Keap1–Nrf2 positive-feedback loop (Zhang et al., 2021) represents the mutual regulatory relationship between the Nrf2 antioxidant system and autophagy (Figure 3).
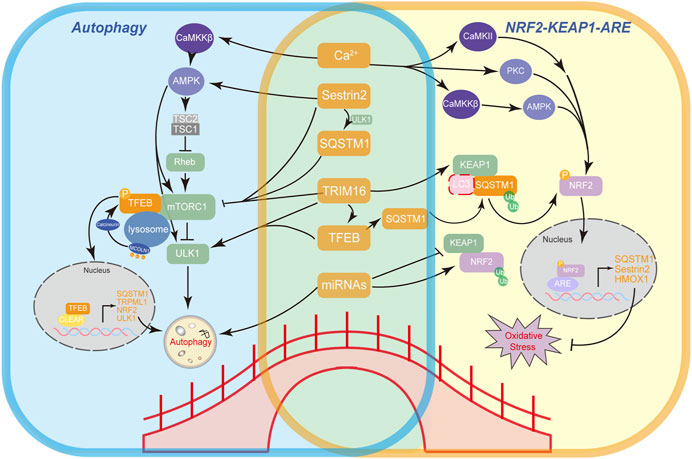
FIGURE 3. Bridging factors that connect autophagy and Nrf2 antioxidant pathway. A working model illustrates the mechanisms of bridging factors (SQSTM1, TFEB, Sestrin2, TRIM16, Ca2+, and miRNAs) connecting autophagy (left) and the main antioxidant Nrf2-Keap1-ARE pathway (right) and the feedback loops between these factors.
2.2 Transcription factor EB
Transcription factor EB (TFEB), a basic helix-loop-helix-leucine-zipper transcription factor, belongs to the MiT family (Napolitano and Ballabio, 2016). TFEB promotes the expression of a tandem of genes involved in autophagic and lysosomal biogenesis and function (Franco-Juárez et al., 2022; Tan et al., 2022). Thus, TFEB, as a potential therapeutic target, has been associated with many diseases, such as lysosomal storage diseases, neurodegenerative diseases, and cancer (Bajaj et al., 2019; Tan et al., 2022). TFEB agonist treatment has therapeutic effects on several animal models, including Batten disease (Palmieri et al., 2017), NPC disease (Argüello et al., 2021), and Pompe disease (Spampanato et al., 2013).
Overexpression of TFEB leads to degradation of bulk autophagic substrates such as damaged mitochondria (Nezich et al., 2015), long-lived protein aggregates (Martini-Stoica et al., 2016), and lipid droplets (Settembre et al., 2013), indicating the crucial role of TFEB in organelle-specific autophagy, such as mitophagy and lipophagy (Settembre et al., 2013; Nezich et al., 2015). Importantly, TFEB has no effect on the basal transcriptional levels of downstream target genes but rather regulates their transcriptional levels in response to stimuli. Under normal conditions, TFEB is phosphorylated by mTORC1 and exists mainly in an inactive state in the cytosol (Martina and Puertollano, 2013). Upon stimuli such as starvation or oxidative stress, TFEB is dephosphorylated, subsequently translocates to the nucleus, and promotes the activation of its target genes (Sancak et al., 2010; Medina et al., 2015).
Interestingly, Bo Pan et al., 2020 have delineated a relationship between SQSTM1 and TFEB. They proposed that the protein aggregates formed by SQSTM1 during systemic proteasome inhibition sequester mTOR and prevent its TFEB phosphorylation. Furthermore, SQSTM1 is capable of interacting with Raptor, a part of mTORC1, and colocalizing with Rag GTPases at the lysosomal membrane, hence being responsible for the activation of mTORC1 (Duran et al., 2011). SQSTM1 can also recruit the tumor necrosis factor receptor-associated factor 6 in an amino acid-dependent manner and activate mTOR as well (Linares et al., 2013). Notably, Nrf2/NFE2L2 is identified as a target gene of TFEB; thus, TFEB regulates redox homeostasis via targeting Nrf2 (Li et al., 2021b). Therefore, a network forms that connects Nrf2, SQSTM1, TFEB, and mTOR (Figure 3).
2.3 Other non-canonical bridging regulatory factors
Other non-canonical autophagy regulatory proteins like Sestrin2 (Bae et al., 2013) and tripartite motif-containing protein 16 (TRIM16) (Kimura et al., 2015) also participate in the regulation of Nrf2 and mTOR via direct or indirect interactions, providing novel targets for pondering the connection between autophagy and the Nrf2 antioxidant pathway.
2.3.1 Sestrin2
Sestrin2, a highly conserved protein belonging to the Sestrin family, is involved in multiple biological functions, including regulating redox reactions, metabolic homeostasis, and the aging process (Lee et al., 2013; Gong et al., 2021). Sestrin2 has been proposed to be the therapeutic target for various diseases, such as cardiac diseases, respiratory diseases, and non-alcoholic fatty liver disease (Ren D. et al., 2020; Sun et al., 2020; Wu D. et al., 2021). Sestrin2 has been reported to protect insulin-resistant cells by activating the AMPK pathway or upregulating the mTOR pathway (Gong et al., 2021), thus promoting a beneficial effect on diabetes (Tian et al., 2022).
Studies have shown that Sestrin2 overexpression can induce autophagic degradation of Keap1, leading to the upregulation of Nrf2 activity (Bae et al., 2013). In addition, this process requires the phosphorylation of the UBA domain of SQSTM1 at Ser409 (Polito et al., 2014; Rhee and Bae, 2015), resulting in the degradation of LC3–SQSTM1–Keap1 aggregates by selective autophagy.
Sestrin2 regulates autophagy mainly through its intervention with mTORC1 signaling (Wolfson et al., 2016). Upon stress, Sestrin2 activates AMPK, which promotes the phosphorylation of tuberous sclerosis complex 2, leading to mTORC1 inhibition (Wolfson et al., 2016). Sestrin2 can also regulate mTORC1 through GAP activity toward the Rags (n) 1/2 complex (Parmigiani et al., 2014; Rhee and Bae, 2015). GATOR1 inhibits mTORC1 signaling, while GATOR2 positively regulates mTORC1 and inhibits GATOR1 by forming a complex at the lysosomal membrane (Bar-Peled et al., 2013). Upon amino acid starvation, Sestrin2 interacts with GATOR2, thus impeding its interaction with GATOR1, which results in the inactivation of mTORC1 (Chantranupong et al., 2014).
2.3.2 TRIM16
TRIM16 has been identified as a regulator of oxidative-stress-responsive proteins (Ren X. et al., 2020) and is associated with tumor suppression (Kim et al., 2016), cell motility (Huo et al., 2015; Li et al., 2016), apoptosis (Kim et al., 2016), and autophagy (Chauhan et al., 2016; New and Thomas, 2019). TRIM16 deficiency significantly exacerbates cardiomyocyte hypertrophy, while overexpression of TRIM16 inhibits cardiac hypertrophy, suggesting that a TRIM16 inhibitor could be a novel inhibitor of pathological heart hypertrophy and heart failure (Liu J. et al., 2022). Jena et al. (2018) have unveiled the latent significance of TRIM16 in protecting cells from oxidative stress-induced cytotoxicity by interacting with Nrf2, Keap1, and SQSTM1. TRIM16, as an E3 ligase, promotes the SQSTM1-induced autophagic degradation of Keap1 and regulates Nrf2 activity (Jena et al., 2018).
TRIM16 associates with ULK1 and Beclin 1 to engage in autophagy (Chauhan et al., 2016). Furthermore, TRIM16 forms a protein complex with endogenous DEP domain-containing mTOR-interacting proteins, a mTOR inhibitor, and Rag B and Rag D factors, which are responsible for mTOR recruitment to the lysosome (Jena et al., 2018). This TRIM16 complex also interacts with TFEB and calcineurin (Chauhan et al., 2016) (the phosphatase that dephosphorylates TFEB (Medina et al., 2015)). Accumulating evidence illuminates the scaffolding role of TRIM16 in autophagy regulation. Interestingly, both Sestrin2 and TRIM16 are positively regulated by Nrf2 at the transcriptional level (Shin et al., 2012; Jena et al., 2018; Jena et al., 2019). Hence, SQSTM1, Sestrin2, TRIM16, and Nrf2 can also form a complex feedback loop (Figure 3).
2.4 Ions and miRNAs
2.4.1 Calcium
Ca2+ is the most widespread intracellular messenger (Glaser et al., 2019) whose role in autophagy has been studied extensively. For example, starvation induces lysosomal Ca2+ release through the TRPML1 channel, which activates TFEB by calcineurin and promotes autophagy (Medina et al., 2015). Meanwhile, the calcium/calmodulin-dependent protein kinase (CaMK) family, as the major Ca2+ signal transducer, plays a crucial role in a variety of autophagy-related signaling pathways, including Ca2+-CaMKKβ-AMPK (Woods et al., 2005)-induced autophagy (Kim et al., 2011; Hu et al., 2019). Furthermore, Ca2+ channels in various organelles, such as the lysosome or endoplasmic reticulum, have effects on autophagy (Decuypere et al., 2011a; Decuypere et al., 2011b), but this remains controversial.
A recent study has revealed that TRPV1-evoked Ca2+ influx promotes CaMKII phosphorylation and subsequently promotes Nrf2 nuclear translocation (Lv et al., 2021). In addition, the Ser558 site in the Neh1 domain of Nrf2 has been identified as a direct phosphorylation site of AMPK (Joo et al., 2016), which results in Nrf2 activation via the Ca2+-CaMKKβ-AMPK pathway. Moreover, Ca2+-dependent protein kinase C can phosphorylate the Ser40 site in the Neh2 domain of Nrf2 (Niture et al., 2014), thereby activating Nrf2.
2.4.2 miRNAs
At post-transcriptional level, microRNAs (miRNAs) have been reported to impact both the regulation of autophagy and Nrf2 antioxidant signaling. miR-144 is the first miRNA identified as an Nrf2 negative regulator (Sangokoya et al., 2010), and miR-144 can also promote autophagy (Chen et al., 2015). Conversely, Nrf2 can upregulate miR-129-3p, which inhibits mTOR and leads to the initiation of autophagy (Sun et al., 2019). In addition, a study investigated miR-93 and Nrf2 and demonstrated that miR-93 can regulate the mRNA and protein levels of Nrf2 (Singh et al., 2013), while it can target Atg16L to affect autophagy in breast cancer cells (Lu et al., 2014).
3 Conclusion
In summary, examining the crosstalk between Nrf2 antioxidant signaling and autophagy provides insights into how they are interconnected and the proteins that mediate their communication. In the aspect of autophagy and the Nrf2-Keap1 antioxidant pathway, SQSTM1, TFEB, Sestrin2, and TRIM16 are all involved in different conditions and act as the scaffolds connecting the two pathways (Table 1). Moreover, studies also reveal that intricate feedback loops form between these bridging proteins. These factors are potential therapeutic targets for diseases with both autophagy dysfunction and oxidative stress. However, since these regulatory proteins seem intricately entangled, potential side effects in practical scenarios should also be taken into consideration. Nevertheless, further studies on understanding the complex crosstalk between autophagy and antioxidant pathways are yet to be conducted.
Author contributions
Conceptualization, DL; data curation, DL, BN, and SH; writing—original draft preparation, BN, SH, and CM; writing—review and editing, DL, BN, SH, and WZ; supervision, DL; project, DL. All authors contributed to the article and approved the submitted version.
Acknowledgments
This work was supported by a grant from the National Natural Science Foundation of China (31600823 to DL).
Conflict of interest
The authors declare that the research was conducted in the absence of any commercial or financial relationships that could be construed as a potential conflict of interest.
Publisher’s note
All claims expressed in this article are solely those of the authors and do not necessarily represent those of their affiliated organizations, or those of the publisher, the editors, and the reviewers. Any product that may be evaluated in this article, or claim that may be made by its manufacturer, is not guaranteed or endorsed by the publisher.
References
Alkadi, H. (2020). A review on free radicals and antioxidants. Infect. Disord. drug targets 20 (1), 16–26. doi:10.2174/1871526518666180628124323
Argüello, G., Balboa, E., Tapia, P. J., Castro, J., Yañez, M. J., Mattar, P., et al. (2021). Genistein activates transcription factor EB and corrects niemann-pick C phenotype. Int. J. Mol. Sci. 22 (8), 4220. doi:10.3390/ijms22084220
Bae, S. H., Sung, S. H., Oh, S. Y., Lim, J. M., Lee, S. K., Park, Y. N., et al. (2013). Sestrins activate Nrf2 by promoting p62-dependent autophagic degradation of Keap1 and prevent oxidative liver damage. Cell Metab. 17 (1), 73–84. doi:10.1016/j.cmet.2012.12.002
Bajaj, L., Lotfi, P., Pal, R., Ronza, A. D., Sharma, J., and Sardiello, M. (2019). Lysosome biogenesis in health and disease. J. Neurochem. 148 (5), 573–589. doi:10.1111/jnc.14564
Balaban, R. S., Nemoto, S., and Finkel, T. (2005). Mitochondria, oxidants, and aging. Cell 120 (4), 483–495. doi:10.1016/j.cell.2005.02.001
Baldelli, S., Aquilano, K., and Ciriolo, M. R. (2013). Punctum on two different transcription factors regulated by PGC-1α: Nuclear factor erythroid-derived 2-like 2 and nuclear respiratory factor 2. Biochimica biophysica acta 1830 (8), 4137–4146. doi:10.1016/j.bbagen.2013.04.006
Bar-Peled, L., Chantranupong, L., Cherniack, A. D., Chen, W. W., Ottina, K. A., Grabiner, B. C., et al. (2013). A Tumor suppressor complex with GAP activity for the Rag GTPases that signal amino acid sufficiency to mTORC1. Science 340 (6136), 1100–1106. doi:10.1126/science.1232044
Bhujabal, Z., Birgisdottir Å, B., Sjøttem, E., Brenne, H. B., Øvervatn, A., Habisov, S., et al. (2017). FKBP8 recruits LC3A to mediate Parkin-independent mitophagy. EMBO Rep. 18 (6), 947–961. doi:10.15252/embr.201643147
Bienert, G. P., and Chaumont, F. (2014). Aquaporin-facilitated transmembrane diffusion of hydrogen peroxide. Biochimica biophysica acta 1840 (5), 1596–1604. doi:10.1016/j.bbagen.2013.09.017
Brieger, K., Schiavone, S., Miller, F. J., and Krause, K. H. (2012). Reactive oxygen species: From health to disease. Swiss Med. Wkly. 142, w13659. doi:10.4414/smw.2012.13659
Chantranupong, L., Wolfson, R. L., Orozco, J. M., Saxton, R. A., Scaria, S. M., Bar-Peled, L., et al. (2014). The Sestrins interact with GATOR2 to negatively regulate the amino-acid-sensing pathway upstream of mTORC1. Cell Rep. 9 (1), 1–8. doi:10.1016/j.celrep.2014.09.014
Chauhan, S., Kumar, S., Jain, A., Ponpuak, M., Mudd, M. H., Kimura, T., et al. (2016). TRIMs and galectins globally cooperate and TRIM16 and galectin-3 Co-direct autophagy in endomembrane damage homeostasis. Dev. Cell 39 (1), 13–27. doi:10.1016/j.devcel.2016.08.003
Chen, S., Li, P., Li, J., Wang, Y., Du, Y., Chen, X., et al. (2015). MiR-144 inhibits proliferation and induces apoptosis and autophagy in lung cancer cells by targeting TIGAR. Cell. physiology Biochem. Int. J. Exp. Cell. physiology, Biochem. Pharmacol. 35 (3), 997–1007. doi:10.1159/000369755
Chen, Y., Azad, M. B., Gibson, S. B., Hsu, M. H., Liang, W. M., and Cheng, F. C. (2009). Effects of magnesium on exercise performance and plasma glucose and lactate concentrations in rats using a novel blood-sampling technique. Cell death Differ. 16 (7), 1040–1047. doi:10.1139/H09-105
Chu, C. T. (2019). Mechanisms of selective autophagy and mitophagy: Implications for neurodegenerative diseases. Neurobiol. Dis. 122, 23–34. doi:10.1016/j.nbd.2018.07.015
Ci, Y., Shi, K., An, J., Yang, Y., Hui, K., Wu, P., et al. (2014). ROS inhibit autophagy by downregulating ULK1 mediated by the phosphorylation of p53 in selenite-treated NB4 cells. Cell death Dis. 5 (11), e1542. doi:10.1038/cddis.2014.506
Copple, I. M., Lister, A., Obeng, A. D., Kitteringham, N. R., Jenkins, R. E., Layfield, R., et al. (2010). Physical and functional interaction of sequestosome 1 with Keap1 regulates the keap1-nrf2 cell defense pathway. J. Biol. Chem. 285 (22), 16782–16788. doi:10.1074/jbc.M109.096545
Davalli, P., Mitic, T., Caporali, A., Lauriola, A., and D'Arca, D. (2016). ROS, cell senescence, and novel molecular mechanisms in aging and age-related diseases. Oxidative Med. Cell. Longev. 2016, 3565127. doi:10.1155/2016/3565127
Decuypere, J. P., Bultynck, G., and Parys, J. B. (2011a). A dual role for Ca(2+) in autophagy regulation. Cell calcium 50 (3), 242–250. doi:10.1016/j.ceca.2011.04.001
Decuypere, J. P., Monaco, G., Bultynck, G., Missiaen, L., De Smedt, H., and Parys, J. B. (2011b). The IP(3) receptor-mitochondria connection in apoptosis and autophagy. Biochimica biophysica acta 1813 (5), 1003–1013. doi:10.1016/j.bbamcr.2010.11.023
Duan, X., Kong, Z., Mai, X., Lan, Y., Liu, Y., Yang, Z., et al. (2018). Autophagy inhibition attenuates hyperoxaluria-induced renal tubular oxidative injury and calcium oxalate crystal depositions in the rat kidney. Redox Biol. 16, 414–425. doi:10.1016/j.redox.2018.03.019
Duran, A., Amanchy, R., Linares, J. F., Joshi, J., Abu-Baker, S., Porollo, A., et al. (2011). p62 is a key regulator of nutrient sensing in the mTORC1 pathway. Mol. Cell 44 (1), 134–146. doi:10.1016/j.molcel.2011.06.038
Elias, R. J., Kellerby, S. S., and Decker, E. A. (2008). Antioxidant activity of proteins and peptides. Crit. Rev. food Sci. Nutr. 48 (5), 430–441. doi:10.1080/10408390701425615
Esteras, N., and Abramov, A. Y. (2022). Nrf2 as a regulator of mitochondrial function: Energy metabolism and beyond. Free Radic. Biol. Med. 189, 136–153. doi:10.1016/j.freeradbiomed.2022.07.013
Fan, J., Ren, D., Wang, J., Liu, X., Zhang, H., Wu, M., et al. (2020). Bruceine D induces lung cancer cell apoptosis and autophagy via the ROS/MAPK signaling pathway in vitro and in vivo. Cell death Dis. 11 (2), 126. doi:10.1038/s41419-020-2317-3
Filomeni, G., De Zio, D., and Cecconi, F. (2015). Oxidative stress and autophagy: The clash between damage and metabolic needs. Cell death Differ. 22 (3), 377–388. doi:10.1038/cdd.2014.150
Fischer, B. M., Domowicz, D. A., Zheng, S., Carter, J. L., McElvaney, N. G., Taggart, C., et al. (2009). Neutrophil elastase increases airway epithelial nonheme iron levels. Clin. Transl. Sci. 2 (5), 333–339. doi:10.1111/j.1752-8062.2009.00151.x
Forman, H. J., and Zhang, H. (2021). Targeting oxidative stress in disease: Promise and limitations of antioxidant therapy. Nat. Rev. Drug Discov. 20 (9), 689–709. doi:10.1038/s41573-021-00233-1
Forrester, S. J., Kikuchi, D. S., Hernandes, M. S., Xu, Q., and Griendling, K. K. (2018). Reactive oxygen species in metabolic and inflammatory signaling. Circulation Res. 122 (6), 877–902. doi:10.1161/CIRCRESAHA.117.311401
Franco-Juárez, B., Coronel-Cruz, C., Hernández-Ochoa, B., Gómez-Manzo, S., Cárdenas-Rodríguez, N., Arreguin-Espinosa, R., et al. TFEB (2022). TFEB; beyond its role as an autophagy and lysosomes regulator. Cells 11 (19), 3153. doi:10.3390/cells11193153
Frudd, K., Burgoyne, T., and Burgoyne, J. R. (2018). Oxidation of Atg3 and Atg7 mediates inhibition of autophagy. Nat. Commun. 9 (1), 95. doi:10.1038/s41467-017-02352-z
Fu, R., Wassif, C. A., Yanjanin, N. M., Watkins-Chow, D. E., Baxter, L. L., Incao, A., et al. (2013). Efficacy of N-acetylcysteine in phenotypic suppression of mouse models of Niemann-Pick disease, type C1. Hum. Mol. Genet. 22 (17), 3508–3523. doi:10.1093/hmg/ddt206
Fujii, J., Homma, T., and Osaki, T. (2022). Superoxide radicals in the execution of cell death. Antioxidants (Basel, Switz. 11 (3), 501. doi:10.3390/antiox11030501
Galati, S., Boni, C., Gerra, M. C., Lazzaretti, M., and Buschini, A. (2019). Autophagy: A player in response to oxidative stress and DNA damage. Oxidative Med. Cell. Longev. 2019, 5692958. doi:10.1155/2019/5692958
Gao, L., Loveless, J., Shay, C., and Teng, Y. (2020). Targeting ROS-mediated crosstalk between autophagy and apoptosis in cancer. Adv. Exp. Med. Biol. 1260, 1–12. doi:10.1007/978-3-030-42667-5_1
Germain, K., and Kim, P. K. (2020). Pexophagy: A model for selective autophagy. Int. J. Mol. Sci. 21 (2), 578. doi:10.3390/ijms21020578
Giordano, S., Darley-Usmar, V., and Zhang, J. (2014). Autophagy as an essential cellular antioxidant pathway in neurodegenerative disease. Redox Biol. 2, 82–90. doi:10.1016/j.redox.2013.12.013
Glaser, T., Arnaud Sampaio, V. F., Lameu, C., and Ulrich, H. (2019). Calcium signalling: A common target in neurological disorders and neurogenesis. Seminars Cell & Dev. Biol. 95, 25–33. doi:10.1016/j.semcdb.2018.12.002
Gong, L., Wang, Z., Wang, Z., and Zhang, Z. (2021). Sestrin2 as a potential target for regulating metabolic-related diseases. Front. Endocrinol. 12, 751020. doi:10.3389/fendo.2021.751020
Guo, H., Ouyang, Y., Yin, H., Cui, H., Deng, H., Liu, H., et al. (2022). Induction of autophagy via the ROS-dependent AMPK-mTOR pathway protects copper-induced spermatogenesis disorder. Redox Biol. 49, 102227. doi:10.1016/j.redox.2021.102227
Hayes, J. D., and Dinkova-Kostova, A. T. (2014). The Nrf2 regulatory network provides an interface between redox and intermediary metabolism. Trends Biochem. Sci. 39 (4), 199–218. doi:10.1016/j.tibs.2014.02.002
He, L., He, T., Farrar, S., Ji, L., Liu, T., and Ma, X. (2017). Antioxidants maintain cellular redox homeostasis by elimination of reactive oxygen species. Cell. physiology Biochem. Int. J. Exp. Cell. physiology, Biochem. Pharmacol. 44 (2), 532–553. doi:10.1159/000485089
Ho, C. J., and Gorski, S. M. (2019). Molecular mechanisms underlying autophagy-mediated treatment resistance in cancer. Cancers (Basel) 11 (11), 1775. doi:10.3390/cancers11111775
Hu, Y. X., Han, X. S., and Jing, Q. (2019). Ca(2+) ion and autophagy. Adv. Exp. Med. Biol. 1206, 151–166. doi:10.1007/978-981-15-0602-4_7
Huo, X., Li, S., Shi, T., Suo, A., Ruan, Z., and Yao, Y. (2015). Tripartite motif 16 inhibits epithelial-mesenchymal transition and metastasis by down-regulating sonic hedgehog pathway in non-small cell lung cancer cells. Biochem. Biophys. Res. Commun. 460 (4), 1021–1028. doi:10.1016/j.bbrc.2015.03.144
Ichimura, Y., Waguri, S., Sou, Y. S., Kageyama, S., Hasegawa, J., Ishimura, R., et al. (2013). Phosphorylation of p62 activates the Keap1-Nrf2 pathway during selective autophagy. Mol. Cell 51 (5), 618–631. doi:10.1016/j.molcel.2013.08.003
Jain, A., Lamark, T., Sjottem, E., Larsen, K. B., Awuh, J. A., Overvatn, A., et al. (2010). p62/SQSTM1 is a target gene for transcription factor NRF2 and creates a positive feedback loop by inducing antioxidant response element-driven gene transcription. J. Biol. Chem. 285 (29), 22576–22591. doi:10.1074/jbc.M110.118976
Jena, K. K., Kolapalli, S. P., Mehto, S., Nath, P., Das, B., Sahoo, P. K., et al. (2018). TRIM16 controls assembly and degradation of protein aggregates by modulating the p62-NRF2 axis and autophagy. EMBO J. 37 (18), e98358. doi:10.15252/embj.201798358
Jena, K. K., Mehto, S., Kolapalli, S. P., Nath, P., Sahu, R., Chauhan, N. R., et al. (2019). TRIM16 governs the biogenesis and disposal of stress-induced protein aggregates to evade cytotoxicity: Implication for neurodegeneration and cancer. Autophagy 15 (5), 924–926. doi:10.1080/15548627.2019.1586251
Joo, M. S., Kim, W. D., Lee, K. Y., Kim, J. H., Koo, J. H., and Kim, S. G. (2016). AMPK facilitates nuclear accumulation of Nrf2 by phosphorylating at serine 550. Mol. Cell Biol. 36 (14), 1931–1942. doi:10.1128/MCB.00118-16
Kang, L., Liu, S., Li, J., Tian, Y., Xue, Y., and Liu, X. (2020). The mitochondria-targeted anti-oxidant MitoQ protects against intervertebral disc degeneration by ameliorating mitochondrial dysfunction and redox imbalance. Cell Prolif. 53 (3), e12779. doi:10.1111/cpr.12779
Katsuragi, Y., Ichimura, Y., and Komatsu, M. (2015). p62/SQSTM1 functions as a signaling hub and an autophagy adaptor. FEBS J. 282 (24), 4672–4678. doi:10.1111/febs.13540
Kim, J., Kundu, M., Viollet, B., and Guan, K. L. (2011). AMPK and mTOR regulate autophagy through direct phosphorylation of Ulk1. Nat. Cell Biol. 13 (2), 132–141. doi:10.1038/ncb2152
Kim, P. Y., Tan, O., Liu, B., Trahair, T., Liu, T., Haber, M., et al. (2016). High TDP43 expression is required for TRIM16-induced inhibition of cancer cell growth and correlated with good prognosis of neuroblastoma and breast cancer patients. Cancer Lett. 374 (2), 315–323. doi:10.1016/j.canlet.2016.02.021
Kimura, T., Jain, A., Choi, S. W., Mandell, M. A., Schroder, K., Johansen, T., et al. (2015). TRIM-mediated precision autophagy targets cytoplasmic regulators of innate immunity. J. Cell Biol. 210 (6), 973–989. doi:10.1083/jcb.201503023
Klionsky, D. J., Petroni, G., Amaravadi, R. K., Baehrecke, E. H., Ballabio, A., Boya, P., et al. (2021). Autophagy in major human diseases. EMBO J. 40 (19), e108863. doi:10.15252/embj.2021108863
Komatsu, M., Kurokawa, H., Waguri, S., Taguchi, K., Kobayashi, A., Ichimura, Y., et al. (2010). The selective autophagy substrate p62 activates the stress responsive transcription factor Nrf2 through inactivation of Keap1. Nat. Cell Biol. 12 (3), 213–223. doi:10.1038/ncb2021
Kumar, A. V., Mills, J., and Lapierre, L. R. (2022). Selective autophagy receptor p62/SQSTM1, a pivotal player in stress and aging. Front. Cell Dev. Biol. 10, 793328. doi:10.3389/fcell.2022.793328
Lee, J. H., Budanov, A. V., and Karin, M. (2013). Sestrins orchestrate cellular metabolism to attenuate aging. Cell Metab. 18 (6), 792–801. doi:10.1016/j.cmet.2013.08.018
Lennicke, C., and Cochemé, H. M. (2021). Redox metabolism: ROS as specific molecular regulators of cell signaling and function. Mol. Cell 81 (18), 3691–3707. doi:10.1016/j.molcel.2021.08.018
Levine, B., and Kroemer, G. (2019). Biological functions of autophagy genes: A disease perspective. Cell 176 (1-2), 11–42. doi:10.1016/j.cell.2018.09.048
Li, D., Ding, Z., Du, K., Ye, X., and Cheng, S. (2021a). Reactive oxygen species as a link between antioxidant pathways and autophagy. Oxid. Med. Cell Longev. 2021, 5583215. doi:10.1155/2021/5583215
Li, D., Shao, R., Wang, N., Zhou, N., Du, K., Shi, J., et al. (2021b). Sulforaphane Activates a lysosome-dependent transcriptional program to mitigate oxidative stress. Autophagy 17 (4), 872–887. doi:10.1080/15548627.2020.1739442
Li, L., Dong, L., Qu, X., Jin, S., Lv, X., and Tan, G. (2016). Tripartite motif 16 inhibits hepatocellular carcinoma cell migration and invasion. Int. J. Oncol. 48 (4), 1639–1649. doi:10.3892/ijo.2016.3398
Li, L., Tan, J., Miao, Y., Lei, P., and Zhang, Q. (2015). ROS and autophagy: Interactions and molecular regulatory mechanisms. Cell. Mol. Neurobiol. 35 (5), 615–621. doi:10.1007/s10571-015-0166-x
Li, M. Y., Zhu, X. L., Zhao, B. X., Shi, L., Wang, W., Hu, W., et al. (2019). Adrenomedullin alleviates the pyroptosis of Leydig cells by promoting autophagy via the ROS-AMPK-mTOR axis. Cell death Dis. 10 (7), 489. doi:10.1038/s41419-019-1728-5
Liang, L. P., Waldbaum, S., Rowley, S., Huang, T. T., Day, B. J., and Patel, M. (2012). Mitochondrial oxidative stress and epilepsy in SOD2 deficient mice: Attenuation by a lipophilic metalloporphyrin. Neurobiol. Dis. 45 (3), 1068–1076. doi:10.1016/j.nbd.2011.12.025
Liao, W., Wang, Z., Fu, Z., Ma, H., Jiang, M., Xu, A., et al. (2019). p62/SQSTM1 protects against cisplatin-induced oxidative stress in kidneys by mediating the cross talk between autophagy and the Keap1-Nrf2 signalling pathway. Free Radic. Res. 53 (7), 800–814. doi:10.1080/10715762.2019.1635251
Lin, X., Li, S., Zhao, Y., Ma, X., Zhang, K., He, X., et al. (2013). Interaction domains of p62: A bridge between p62 and selective autophagy. DNA Cell Biol. 32 (5), 220–227. doi:10.1089/dna.2012.1915
Linares, J. F., Duran, A., Yajima, T., Pasparakis, M., Moscat, J., and Diaz-Meco, M. T. (2013). K63 polyubiquitination and activation of mTOR by the p62-TRAF6 complex in nutrient-activated cells. Mol. Cell 51 (3), 283–296. doi:10.1016/j.molcel.2013.06.020
Liu, G. Y., Jiang, X. X., Zhu, X., He, W. Y., Kuang, Y. L., Ren, K., et al. (2015). ROS activates JNK-mediated autophagy to counteract apoptosis in mouse mesenchymal stem cells in vitro. Acta Pharmacol. Sin. 36 (12), 1473–1479. doi:10.1038/aps.2015.101
Liu, J., Li, W., Deng, K. Q., Tian, S., Liu, H., Shi, H., et al. (2022b). The E3 ligase TRIM16 is a key suppressor of pathological cardiac hypertrophy. Circulation Res. 130 (10), 1586–1600. doi:10.1161/CIRCRESAHA.121.318866
Liu, L., Feng, D., Chen, G., Chen, M., Zheng, Q., Song, P., et al. (2012). Mitochondrial outer-membrane protein FUNDC1 mediates hypoxia-induced mitophagy in mammalian cells. Nat. Cell Biol. 14 (2), 177–185. doi:10.1038/ncb2422
Liu, S., Pi, J., and Zhang, Q. (2022a). Signal amplification in the KEAP1-NRF2-ARE antioxidant response pathway. Redox Biol. 54, 102389. doi:10.1016/j.redox.2022.102389
Lu, C., Chen, J., Xu, H. G., Zhou, X., He, Q., Li, Y. L., et al. (2014). MIR106B and MIR93 prevent removal of bacteria from epithelial cells by disrupting ATG16L1-mediated autophagy. Gastroenterology 146 (1), 188–199. doi:10.1053/j.gastro.2013.09.006
Lu, M. C., Ji, J. A., Jiang, Z. Y., and You, Q. D. (2016). The keap1-nrf2-ARE pathway as a potential preventive and therapeutic target: An update. Med. Res. Rev. 36 (5), 924–963. doi:10.1002/med.21396
Luo, J., Mills, K., le Cessie, S., Noordam, R., and van Heemst, D. (2020). Ageing, age-related diseases and oxidative stress: What to do next? Ageing Res. Rev. 57, 100982. doi:10.1016/j.arr.2019.100982
Lv, Z., Xu, X., Sun, Z., Yang, Y. X., Guo, H., Li, J., et al. (2021). TRPV1 alleviates osteoarthritis by inhibiting M1 macrophage polarization via Ca(2+)/CaMKII/Nrf2 signaling pathway. Cell death Dis. 12 (6), 504. doi:10.1038/s41419-021-03792-8
Marinho, H. S., Real, C., Cyrne, L., Soares, H., and Antunes, F. (2014). Hydrogen peroxide sensing, signaling and regulation of transcription factors. Redox Biol. 2, 535–562. doi:10.1016/j.redox.2014.02.006
Martina, J. A., and Puertollano, R. (2013). Rag GTPases mediate amino acid-dependent recruitment of TFEB and MITF to lysosomes. J. Cell Biol. 200 (4), 475–491. doi:10.1083/jcb.201209135
Martini-Stoica, H., Xu, Y., Ballabio, A., and Zheng, H. (2016). The autophagy-lysosomal pathway in neurodegeneration: A TFEB perspective. Trends Neurosci. 39 (4), 221–234. doi:10.1016/j.tins.2016.02.002
Martins-Marques, T., Ribeiro-Rodrigues, T., Pereira, P., Codogno, P., and Girao, H. (2015). Autophagy and ubiquitination in cardiovascular diseases. DNA Cell Biol. 34 (4), 243–251. doi:10.1089/dna.2014.2765
Medina, D. L., Di Paola, S., Peluso, I., Armani, A., De Stefani, D., Venditti, R., et al. (2015). Lysosomal calcium signalling regulates autophagy through calcineurin and TFEB. Nat. Cell Biol. 17 (3), 288–299. doi:10.1038/ncb3114
Merry, T. L., and Ristow, M. (2016). Nuclear factor erythroid-derived 2-like 2 (NFE2L2, Nrf2) mediates exercise-induced mitochondrial biogenesis and the anti-oxidant response in mice. J. physiology 594 (18), 5195–5207. doi:10.1113/JP271957
Mittler, R. (2017). ROS are good. Trends Plant Sci. 22 (1), 11–19. doi:10.1016/j.tplants.2016.08.002
Murakami, S., and Motohashi, H. (2015). Roles of Nrf2 in cell proliferation and differentiation. Free Radic. Biol. Med. 88, 168–178. doi:10.1016/j.freeradbiomed.2015.06.030
Murakawa, T., Yamaguchi, O., Hashimoto, A., Hikoso, S., Takeda, T., Oka, T., et al. (2015). Bcl-2-like protein 13 is a mammalian Atg32 homologue that mediates mitophagy and mitochondrial fragmentation. Nat. Commun. 6, 7527. doi:10.1038/ncomms8527
Napolitano, G., and Ballabio, A. (2016). TFEB at a glance. J. Cell Sci. 129 (13), 2475–2481. doi:10.1242/jcs.146365
New, J., and Thomas, S. M. (2019). Autophagy-dependent secretion: Mechanism, factors secreted, and disease implications. Autophagy 15 (10), 1682–1693. doi:10.1080/15548627.2019.1596479
Nezich, C. L., Wang, C., Fogel, A. I., and Youle, R. J. (2015). MiT/TFE transcription factors are activated during mitophagy downstream of Parkin and Atg5. J. Cell Biol. 210 (3), 435–450. doi:10.1083/jcb.201501002
Niture, S. K., Khatri, R., and Jaiswal, A. K. (2014). Regulation of nrf2-an update. Free Radic. Biol. Med. 66, 36–44. doi:10.1016/j.freeradbiomed.2013.02.008
Novak, I., Kirkin, V., McEwan, D. G., Zhang, J., Wild, P., Rozenknop, A., et al. (2010). Nix is a selective autophagy receptor for mitochondrial clearance. EMBO Rep. 11 (1), 45–51. doi:10.1038/embor.2009.256
Okamoto, K., Kondo-Okamoto, N., and Ohsumi, Y. (2009). Mitochondria-anchored receptor Atg32 mediates degradation of mitochondria via selective autophagy. Dev. Cell 17 (1), 87–97. doi:10.1016/j.devcel.2009.06.013
Onishi, M., Yamano, K., Sato, M., Matsuda, N., and Okamoto, K. (2021). Molecular mechanisms and physiological functions of mitophagy. EMBO J. 40 (3), e104705. doi:10.15252/embj.2020104705
Ornatowski, W., Lu, Q., Yegambaram, M., Garcia, A. E., Zemskov, E. A., Maltepe, E., et al. (2020). Complex interplay between autophagy and oxidative stress in the development of pulmonary disease. Redox Biol. 36, 101679. doi:10.1016/j.redox.2020.101679
Palmieri, M., Pal, R., Nelvagal, H. R., Lotfi, P., Stinnett, G. R., Seymour, M. L., et al. (2017). mTORC1-independent TFEB activation via Akt inhibition promotes cellular clearance in neurodegenerative storage diseases. Nat. Commun. 8, 14338. doi:10.1038/ncomms14338
Pan, B., Li, J., Parajuli, N., Tian, Z., Wu, P., Lewno, M. T., et al. (2020). The calcineurin-TFEB-p62 pathway mediates the activation of cardiac macroautophagy by proteasomal malfunction. Circulation Res. 127 (4), 502–518. doi:10.1161/CIRCRESAHA.119.316007
Parmigiani, A., Nourbakhsh, A., Ding, B., Wang, W., Kim, Y. C., Akopiants, K., et al. (2014). Sestrins inhibit mTORC1 kinase activation through the GATOR complex. Cell Rep. 9 (4), 1281–1291. doi:10.1016/j.celrep.2014.10.019
Pérez-Pérez, M. E., Lemaire, S. D., and Crespo, J. L. (2021). The ATG4 protease integrates redox and stress signals to regulate autophagy. J. Exp. Bot. 72 (9), 3340–3351. doi:10.1093/jxb/erab063
Petrache, I., Medler, T. R., Richter, A. T., Kamocki, K., Chukwueke, U., Zhen, L., et al. (2008). Superoxide dismutase protects against apoptosis and alveolar enlargement induced by ceramide. Am. J. physiology. Lung Cell. Mol. physiology 295 (1), L44–L53. doi:10.1152/ajplung.00448.2007
Piantadosi, C. A., Carraway, M. S., Babiker, A., and Suliman, H. B. (2008). Heme oxygenase-1 regulates cardiac mitochondrial biogenesis via Nrf2-mediated transcriptional control of nuclear respiratory factor-1. Circulation Res. 103 (11), 1232–1240. doi:10.1161/01.RES.0000338597.71702.ad
Polito, V. A., Li, H., Martini-Stoica, H., Wang, B., Yang, L., Xu, Y., et al. (2014). Selective clearance of aberrant tau proteins and rescue of neurotoxicity by transcription factor EB. EMBO Mol. Med. 6 (9), 1142–1160. doi:10.15252/emmm.201303671
Ren, D., Quan, N., Fedorova, J., Zhang, J., He, Z., and Li, J. (2020a). Sestrin2 modulates cardiac inflammatory response through maintaining redox homeostasis during ischemia and reperfusion. Redox Biol. 34, 101556. doi:10.1016/j.redox.2020.101556
Ren, X., Yu, J., Guo, L., and Ma, H. (2020b). TRIM16 protects from OGD/R-induced oxidative stress in cultured hippocampal neurons by enhancing Nrf2/ARE antioxidant signaling via downregulation of Keap1. Exp. Cell Res. 391 (1), 111988. doi:10.1016/j.yexcr.2020.111988
Rhee, S. G., and Bae, S. H. (2015). The antioxidant function of sestrins is mediated by promotion of autophagic degradation of Keap1 and Nrf2 activation and by inhibition of mTORC1. Free Radic. Biol. Med. 88, 205–211. doi:10.1016/j.freeradbiomed.2015.06.007
Ristić, A. J., Savić, D., Sokić, D., Bogdanović Pristov, J., Nestorov, J., Baščarević, V., et al. (2015). Hippocampal antioxidative system in mesial temporal lobe epilepsy. Epilepsia 56 (5), 789–799. doi:10.1111/epi.12981
Roca-Agujetas, V., de Dios, C., Lestón, L., Marí, M., Morales, A., and Colell, A. (2019). Recent insights into the mitochondrial role in autophagy and its regulation by oxidative stress. Oxidative Med. Cell. Longev. 2019, 3809308. doi:10.1155/2019/3809308
Ryter, S. W., Bhatia, D., and Choi, M. E. (2019). Autophagy: A lysosome-dependent process with implications in cellular redox homeostasis and human disease. Antioxid. Redox Signal 30 (1), 138–159. doi:10.1089/ars.2018.7518
Sancak, Y., Bar-Peled, L., Zoncu, R., Markhard, A. L., Nada, S., and Sabatini, D. M. (2010). Ragulator-Rag complex targets mTORC1 to the lysosomal surface and is necessary for its activation by amino acids. Cell 141 (2), 290–303. doi:10.1016/j.cell.2010.02.024
Sánchez-Martín, P., and Komatsu, M. (2018). p62/SQSTM1 – steering the cell through health and disease. J. Cell Sci. 131 (21), jcs222836. doi:10.1242/jcs.222836
Sangokoya, C., Telen, M. J., and Chi, J. T. (2010). microRNA miR-144 modulates oxidative stress tolerance and associates with anemia severity in sickle cell disease. Blood 116 (20), 4338–4348. doi:10.1182/blood-2009-04-214817
Sargent, G., van Zutphen, T., Shatseva, T., Zhang, L., Di Giovanni, V., Bandsma, R., et al. (2016). PEX2 is the E3 ubiquitin ligase required for pexophagy during starvation. J. Cell Biol. 214 (6), 677–690. doi:10.1083/jcb.201511034
Scherz-Shouval, R., Shvets, E., Fass, E., Shorer, H., Gil, L., and Elazar, Z. (2007). Reactive oxygen species are essential for autophagy and specifically regulate the activity of Atg4. EMBO J. 26 (7), 1749–1760. doi:10.1038/sj.emboj.7601623
Settembre, C., De Cegli, R., Mansueto, G., Saha, P. K., Vetrini, F., Visvikis, O., et al. (2013). TFEB controls cellular lipid metabolism through a starvation-induced autoregulatory loop. Nat. Cell Biol. 15 (6), 647–658. doi:10.1038/ncb2718
Shadel, G. S., and Horvath, T. L. (2015). Mitochondrial ROS signaling in organismal homeostasis. Cell 163 (3), 560–569. doi:10.1016/j.cell.2015.10.001
Shaw, P., and Chattopadhyay, A. (2020). Nrf2-ARE signaling in cellular protection: Mechanism of action and the regulatory mechanisms. J. Cell. physiology 235 (4), 3119–3130. doi:10.1002/jcp.29219
Shin, B. Y., Jin, S. H., Cho, I. J., and Ki, S. H. (2012). Nrf2-ARE pathway regulates induction of Sestrin-2 expression. Free Radic. Biol. Med. 53 (4), 834–841. doi:10.1016/j.freeradbiomed.2012.06.026
Shin, W. H., Park, J. H., and Chung, K. C. (2020). The central regulator p62 between ubiquitin proteasome system and autophagy and its role in the mitophagy and Parkinson's disease. BMB Rep. 53 (1), 56–63. doi:10.5483/BMBRep.2020.53.1.283
Sies, H., Belousov, V. V., Chandel, N. S., Davies, M. J., Jones, D. P., Mann, G. E., et al. (2022). Defining roles of specific reactive oxygen species (ROS) in cell biology and physiology. Nat. Rev. Mol. Cell Biol. 23 (7), 499–515. doi:10.1038/s41580-022-00456-z
Sies, H., and Jones, D. P. (2020). Reactive oxygen species (ROS) as pleiotropic physiological signalling agents. Nat. Rev. Mol. Cell Biol. 21 (7), 363–383. doi:10.1038/s41580-020-0230-3
Sies, H. (2015). Oxidative stress: A concept in redox biology and medicine. Redox Biol. 4, 180–183. doi:10.1016/j.redox.2015.01.002
Singh, B., Ronghe, A. M., Chatterjee, A., Bhat, N. K., and Bhat, H. K. (2013). MicroRNA-93 regulates NRF2 expression and is associated with breast carcinogenesis. Carcinogenesis 34 (5), 1165–1172. doi:10.1093/carcin/bgt026
Song, P., Li, S., Wu, H., Gao, R., Rao, G., Wang, D., et al. (2016). Parkin promotes proteasomal degradation of p62: Implication of selective vulnerability of neuronal cells in the pathogenesis of Parkinson's disease. Protein & Cell 7 (2), 114–129. doi:10.1007/s13238-015-0230-9
Spampanato, C., Feeney, E., Li, L., Cardone, M., Lim, J. A., Annunziata, F., et al. (2013). Transcription factor EB (TFEB) is a new therapeutic target for Pompe disease. EMBO Mol. Med. 5 (5), 691–706. doi:10.1002/emmm.201202176
Sui, X., Kong, N., Ye, L., Han, W., Zhou, J., Zhang, Q., et al. (2014). p38 and JNK MAPK pathways control the balance of apoptosis and autophagy in response to chemotherapeutic agents. Cancer Lett. 344 (2), 174–179. doi:10.1016/j.canlet.2013.11.019
Sulkshane, P., Ram, J., Thakur, A., Reis, N., Kleifeld, O., and Glickman, M. H. (2021). Ubiquitination and receptor-mediated mitophagy converge to eliminate oxidation-damaged mitochondria during hypoxia. Redox Biol. 45, 102047. doi:10.1016/j.redox.2021.102047
Sun, W., Wang, Y., Zheng, Y., and Quan, N. (2020). The emerging role of Sestrin2 in cell metabolism, and cardiovascular and age-related diseases. Aging Dis. 11 (1), 154–163. doi:10.14336/AD.2019.0320
Sun, W., Yi, Y., Xia, G., Zhao, Y., Yu, Y., Li, L., et al. (2019). Nrf2-miR-129-3p-mTOR Axis controls an miRNA regulatory network involved in HDACi-induced autophagy. Mol. Ther. 27 (5), 1039–1050. doi:10.1016/j.ymthe.2019.02.010
Tan, A., Prasad, R., Lee, C., and Jho, E. H. (2022). Past, present, and future perspectives of transcription factor EB (TFEB): Mechanisms of regulation and association with disease. Cell death Differ. 29 (8), 1433–1449. doi:10.1038/s41418-022-01028-6
Taucher, E., Mykoliuk, I., Fediuk, M., and Smolle-Juettner, F. M. (2022). Autophagy, oxidative stress and cancer development. Cancers (Basel) 14 (7), 1637. doi:10.3390/cancers14071637
Tian, X., Gao, Y., Zhong, M., Kong, M., Zhao, L., Feng, Z., et al. (2022). The association between serum Sestrin2 and the risk of coronary heart disease in patients with type 2 diabetes mellitus. BMC Cardiovasc. Disord. 22 (1), 281. doi:10.1186/s12872-022-02727-1
Tirichen, H., Yaigoub, H., Xu, W., Wu, C., Li, R., and Li, Y. (2021). Mitochondrial reactive oxygen species and their contribution in chronic kidney disease progression through oxidative stress. Front. Physiol. 12, 627837. doi:10.3389/fphys.2021.627837
Tonelli, C., Chio, I. I. C., and Tuveson, D. A. (2018). Transcriptional regulation by Nrf2. Antioxid. Redox Signal 29 (17), 1727–1745. doi:10.1089/ars.2017.7342
Ulasov, A. V., Rosenkranz, A. A., Georgiev, G. P., and Sobolev, A. S. (2022). Nrf2/Keap1/ARE signaling: Towards specific regulation. Life Sci. 291, 120111. doi:10.1016/j.lfs.2021.120111
Wang, Y., Qi, H., Liu, Y., Duan, C., Liu, X., Xia, T., et al. (2021). The double-edged roles of ROS in cancer prevention and therapy. Theranostics 11 (10), 4839–4857. doi:10.7150/thno.56747
Wheeler, S., and Sillence, D. J. (2020). Niemann-Pick type C disease: Cellular pathology and pharmacotherapy. J. Neurochem. 153 (6), 674–692. doi:10.1111/jnc.14895
Wolfson, R. L., Chantranupong, L., Saxton, R. A., Shen, K., Scaria, S. M., Cantor, J. R., et al. (2016). Sestrin2 is a leucine sensor for the mTORC1 pathway. Science 351 (6268), 43–48. doi:10.1126/science.aab2674
Wong, S. Q., Kumar, A. V., Mills, J., and Lapierre, L. R. (2020). Autophagy in aging and longevity. Hum. Genet. 139 (3), 277–290. doi:10.1007/s00439-019-02031-7
Woods, A., Dickerson, K., Heath, R., Hong, S. P., Momcilovic, M., Johnstone, S. R., et al. (2005). Ca2+/calmodulin-dependent protein kinase kinase-beta acts upstream of AMP-activated protein kinase in mammalian cells. Cell Metab. 2 (1), 21–33. doi:10.1016/j.cmet.2005.06.005
Wu, D., Zhang, H., Wu, Q., Li, F., Wang, Y., Liu, S., et al. (2021b). Sestrin 2 protects against LPS-induced acute lung injury by inducing mitophagy in alveolar macrophages. Life Sci. 267, 118941. doi:10.1016/j.lfs.2020.118941
Wu, W., Qin, Q., Ding, Y., Zang, H., Li, D. S., Nagarkatti, M., et al. (2021a). Autophagy controls nrf2-mediated dichotomy in pressure overloaded hearts. Front. Physiol. 12, 673145. doi:10.3389/fphys.2021.673145
Yamamoto, M., Kensler, T. W., and Motohashi, H. (2018). The KEAP1-NRF2 system: A thiol-based sensor-effector apparatus for maintaining redox homeostasis. Physiol. Rev. 98 (3), 1169–1203. doi:10.1152/physrev.00023.2017
Yang, N., Guan, Q. W., Chen, F. H., Xia, Q. X., Yin, X. X., Zhou, H. H., et al. (2020). Antioxidants targeting mitochondrial oxidative stress: Promising neuroprotectants for epilepsy. Oxidative Med. Cell. Longev. 2020, 6687185. doi:10.1155/2020/6687185
Yang, S., and Lian, G. (2020). ROS and diseases: Role in metabolism and energy supply. Mol. Cell. Biochem. 467 (1-2), 1–12. doi:10.1007/s11010-019-03667-9
Yuan, T., Yang, T., Chen, H., Fu, D., Hu, Y., Wang, J., et al. (2019). New insights into oxidative stress and inflammation during diabetes mellitus-accelerated atherosclerosis. Redox Biol. 20, 247–260. doi:10.1016/j.redox.2018.09.025
Yun, H. R., Jo, Y. H., Kim, J., Shin, Y., Kim, S. S., and Choi, T. G. (2020). Roles of autophagy in oxidative stress. Int. J. Mol. Sci. 21 (9), 3289. doi:10.3390/ijms21093289
Zhang, J., Tripathi, D. N., Jing, J., Alexander, A., Kim, J., Powell, R. T., et al. (2015). ATM functions at the peroxisome to induce pexophagy in response to ROS. Nat. Cell Biol. 17 (10), 1259–1269. doi:10.1038/ncb3230
Zhang, W., Feng, C., and Jiang, H. (2021). Novel target for treating Alzheimer's Diseases: Crosstalk between the Nrf2 pathway and autophagy. Ageing Res. Rev. 65, 101207. doi:10.1016/j.arr.2020.101207
Zhao, Y., Hu, X., Liu, Y., Dong, S., Wen, Z., He, W., et al. (2017). ROS signaling under metabolic stress: Cross-talk between AMPK and AKT pathway. Mol. cancer 16 (1), 79. doi:10.1186/s12943-017-0648-1
Zhou, J., Li, X. Y., Liu, Y. J., Feng, J., Wu, Y., Shen, H. M., et al. (2022). Full-coverage regulations of autophagy by ROS: From induction to maturation. Autophagy 18 (6), 1240–1255. doi:10.1080/15548627.2021.1984656
Zhu, Y., Massen, S., Terenzio, M., Lang, V., Chen-Lindner, S., Eils, R., et al. (2013). Modulation of serines 17 and 24 in the LC3-interacting region of Bnip3 determines pro-survival mitophagy versus apoptosis. J. Biol. Chem. 288 (2), 1099–1113. doi:10.1074/jbc.M112.399345
Zorov, D. B., Juhaszova, M., and Sollott, S. J. (2014). Mitochondrial reactive oxygen species (ROS) and ROS-induced ROS release. Physiol. Rev. 94 (3), 909–950. doi:10.1152/physrev.00026.2013
Glossary
Keywords: bridging factors, autophagy, Nrf2-Keap1, TRIM16, SQSTM1, Sestrin2
Citation: Ning B, Hang S, Zhang W, Mao C and Li D (2023) An update on the bridging factors connecting autophagy and Nrf2 antioxidant pathway. Front. Cell Dev. Biol. 11:1232241. doi: 10.3389/fcell.2023.1232241
Received: 31 May 2023; Accepted: 24 July 2023;
Published: 09 August 2023.
Edited by:
Bilal Çiğ, Ahi Evran University Medicine Faculty Department of Physiology, TürkiyeReviewed by:
Sung Min Son, University of Cambridge, United KingdomFrançois Singh, University of Iceland, Iceland
Copyright © 2023 Ning, Hang, Zhang, Mao and Li. This is an open-access article distributed under the terms of the Creative Commons Attribution License (CC BY). The use, distribution or reproduction in other forums is permitted, provided the original author(s) and the copyright owner(s) are credited and that the original publication in this journal is cited, in accordance with accepted academic practice. No use, distribution or reproduction is permitted which does not comply with these terms.
*Correspondence: Dan Li, bGlkYW5Aemp1dC5lZHUuY24=
†These authors have contributed equally to this work and share first authorship