- Departamento de Biología Molecular, Área de Microbiología, Universidad de León, León, Spain
Phosphate and calcium ions are nutrients that play key roles in growth, differentiation and the production of bioactive secondary metabolites in filamentous fungi. Phosphate concentration regulates the biosynthesis of hundreds of fungal metabolites. The central mechanisms of phosphate transport and regulation, mediated by the master Pho4 transcriptional factor are known, but many aspects of the control of gene expression need further research. High ATP concentration in the cells leads to inositol pyrophosphate molecules formation, such as IP3 and IP7, that act as phosphorylation status reporters. Calcium ions are intracellular messengers in eukaryotic organisms and calcium homeostasis follows elaborated patterns in response to different nutritional and environmental factors, including cross-talking with phosphate concentrations. A large part of the intracellular calcium is stored in vacuoles and other organelles forming complexes with polyphosphate. The free cytosolic calcium concentration is maintained by transport from the external medium or by release from the store organelles through calcium permeable transient receptor potential (TRP) ion channels. Calcium ions, particularly the free cytosolic calcium levels, control the biosynthesis of fungal metabolites by two mechanisms, 1) direct interaction of calcium-bound calmodulin with antibiotic synthesizing enzymes, and 2) by the calmodulin-calcineurin signaling cascade. Control of very different secondary metabolites, including pathogenicity determinants, are mediated by calcium through the Crz1 factor. Several interactions between calcium homeostasis and phosphate have been demonstrated in the last decade: 1) The inositol pyrophosphate IP3 triggers the release of calcium ions from internal stores into the cytosol, 2) Expression of the high affinity phosphate transporter Pho89, a Na+/phosphate symporter, is controlled by Crz1. Also, mutants defective in the calcium permeable TRPCa7-like of Saccharomyces cerevisiae shown impaired expression of Pho89. This information suggests that CrzA and Pho89 play key roles in the interaction of phosphate and calcium regulatory pathways, 3) Finally, acidocalcisomes organelles have been found in mycorrhiza and in some melanin producing fungi that show similar characteristics as protozoa calcisomes. In these organelles there is a close interaction between orthophosphate, pyrophosphate and polyphosphate and calcium ions that are absorbed in the polyanionic polyphosphate matrix. These advances open new perspectives for the control of fungal metabolism.
1 Introduction
The ability to respond to multiple environmental conditions and different nutritional factors is critically important for the growth and differentiation of bacteria and fungi. This requires the integration of numerous sensing mechanisms and signaling cascades that respond to different inputs signals. Calcium is a well-known signaling molecule in the metabolism of filamentous fungi and many other eukaryotic cells. The calcium ions signaling process and its regulation has been studied in the yeast Saccharomyces cerevisiae but there is less information in filamentous fungi of the signaling mechanisms and their interaction with biotic and abiotic stress conditions (Martín and Liras, 2021a). Transient receptor potential (TRP) ion channels are non-selective calcium permeable cation transporters that have been very well studied in animal cells but the information in filamentous fungi is scarce (Venkatachalam and Montell, 2007; Martín and Liras, 2022b). The TRPs are related to members of the multiple facilitator superfamily (MFS) proteins which have 12 transmembrane domains, inserted in either plasma or organelle membranes, e.g. the Pho84 and Pho89 inorganic phosphate transporters of the multiple facilitator superfamily. In addition to their role in cation transport, TRP serve as cell sensors for physical and chemical signals such as osmolarity, high temperature and a variety of chemical substances (Clapham, 2003). Many canonical TRPs contain calmodulin binding domains (Zhu, 2005), EF-hand motives for calcium binding (Zurborg et al., 2007) and ankyrin repeats that appear to serve as a bite for interaction with a variety of stimuli. The presence of calmodulin-binding domains indicates that TRPs play an important role in the overall regulation of calcium homeostasis (see below). Several TRP channels have been identified in S. cerevisiae, Candida albicans and in some fungi, e.g. the TRP1 channel in Giberella zeae (Ihara et al., 2013) or those related to transport of intermediates or external stimuli in β-lactam antibiotic producing fungi such as Pen V in Penicillium chrysogenum and CefP in Acremonium chrysogenum (Martín and Liras, 2021b). The CaPhm7 gene, encoding a TRP in C. albicans, is a member of the calcium-permeable stress-gated cation transporters (CSC) and is involved in filamentation of this yeast, ion homeostasis, drug resistance and pathogenicity (Jiang and Pan, 2018). The homologous gene in S. cerevisiae, ScPhm7, has been found to be closely related with the PHO regulon members (Ogawa et al., 2000). Based on the available information it has been proposed that the phm7 genes, both in S. cerevisiae and C. albicans, and the penV and cefP of P. chrysogenum and A. chrysogenum, respectively, play a role in calcium ion transport, connecting the effect of phosphate concentration with calcium homeostasis (see Section 3.3). This suggests that in yeasts there is an important connection between phosphate and calcium transport and signaling (Box 1). This hypothesis is supported by the finding that several genes of the PHO regulon are controlled by calcium through the calmodulin-calcineurin signaling cascade. Taking into account the previous knowledge this article is focused on the characterization of the interactions between calcium transport and signaling and phosphate regulation of fungal metabolism with special attention to the biosynthesis of bioactive secondary metabolites and the virulence of pathogenic fungi. First, the molecular mechanisms of phosphate control in filamentous fungi and their regulation by external stressing factors are discussed (Section 2), the second part of the article (Section 3) covers the molecular mechanism of calcium uptake and signaling, and finally Section 4 revises the interaction between phosphate and calcium regulation at the cell biology level, including a comparison of the roles of vacuoles and acidocalcisomes in these processes.
BOX 1 Examples of cross talking between phosphate concentration and calcium homeostasis in filamentous fungi.
1- A significant part of calcium in vacuoles, endoplasmic reticulum, and other organelles is stored forming complex with polyphosphate (Bootman et al., 2001; Martín and Liras, 2021a).
2- Inhibition of the Aspergillus fumigatus calcineurin activity increases expression of six putative phosphate transport genes (Silva-Ferrieiro et al., 2007).
3- Mutants of Aspergillus fumigatus defective in the calcineurin catalytic subunit A are impaired in phosphate transport. This effect is reversed by addition of high phosphate concentration (Silva-Ferreira et al., 2007).
4- The phosphate/sodium symporter Pho89 is controlled by the CrzA transcriptional regulator that governs calcium metabolism (Serrano et al., 2002; Serra-Cardona et al., 2014).
5- The transient receptor potential calcium channel CaPhm7 of Candida albicans, homologous of the ScPhm7 in Saccharomyces cerevisiae, controls expression of the Pho89 symporter (Jiang and Pan, 2018).
6- High intracelullar ATP concentration iresults in the formation of inositol polyphosphate derivatives IP3, IP7 and higher members of this family. IP3 determines the release of calcium from internal stores to the cytosol.
7- Some fungi contain acidocalcisomes organelles that accumulate large amounts of orthophosphate, pyrophosphate and polyphosphate combined with calcium and monovalent cations. Acidocalcisome-like vacuoles are present also in Saccharomyces cerevisiae (Seufferheld et al., 2011; Kikuchi et al., 2014; Vila et al., 2022).
8- Phosphorylation of the IP3R enhances calcium release mediated by IP3 (Betzenhauser, and Yule, 2010).
9-The activity of calcium/calmodulin-dependent protein kinases is modulated by protein phosphorylation in response to the intracellular calcium concentration (Tamuli et al., 2011; Jiang et al., 2023).
2 Phosphate control of fungal metabolite biosynthesis
Phosphorous in the form of inorganic (Pi) and organic phosphate is an essential nutrient for all living being. Phosphate is a cellular component of nucleic acids, ATP, cyclic AMP (cAMP), and other nucleotides, highly phosphorylated inositol-derivatives and phospholipids. It plays an important role in the oxidative phosphorylation and respiratory chains. Polyphosphate is a phosphate and energy nutrient reserve and polyphosphate synthesizing and solubilizing enzyme systems exerted a relevant function in the growth of mycorrhiza and mycorrhiza-associated plants (Das et al., 2022). Penicillium oxalicum has been reported to be very efficient in the release of inorganic phosphate from complex phosphate salts in soil, so called rock phosphate, and this fungus may be used as biofertilizer (Wang et al., 2021). In addition, phosphate participates in numerous regulatory and signaling mechanisms mediated by phosphorylation/dephosphorylation of proteins.
Phosphate regulates the biosynthesis of hundreds of antibiotics in bacteria and filamentous fungi. Early studies in filamentous fungi (Martín, 1977; Martín and Demain, 1980) showed that phosphate inhibits the biosynthesis of important bioactive metabolites. One example is the phosphate inhibition of ergot alkaloids in Claviceps purpurea that is exerted at the dimethyl-allyltryptophan synthesis level. The first step of the alkaloid pathway (Rao and Gupta, 1975). Later, it was established that the penicillin biosynthesis in P. chrysogenum is also regulated by the phosphate concentration in the culture medium in concert with carbon catabolite regulation (Martín et al., 1999). The concerted action of glucose on phosphate regulation of fungal metabolism is supported by the finding that the phosphate sensing and uptake in S. cerevisiae requires the presence of glucose in the culture medium even though this sugar is sensed and transported by systems different from the phosphate transporters (Giots et al., 2003); the interaction of glucose sensing and transport with phosphate uptake is an interesting example of coordination between nutrients assimilation in benefit of fungal metabolism, although the precise mechanism of this interaction needs to be further studied. However, in spite of the relevance of phosphate in bioactive secondary metabolites production there was very little progress until the end of the 20th century. Many of these metabolites are secreted and are known to be associated with the formation of sclerotia and sexual and asexual spores (Lim and Keller, 2014; Mulinti et al., 2014; Calvo and Cary, 2015) but there is little information about whether the biosynthesis of most of these metabolites is regulated by phosphate at the transcriptional, translational or posttranslational level. Parallel studies in bacteria achieved significant progress on the characterization of mechanisms of control of secondary metabolites biosynthesis by phosphate in Streptomyces and related actinobacteria (Sola-Landa et al., 2003; Martín, 2004).
2.1 The PHO regulon in fungi
In fungi the PHO regulon includes genes that respond to phosphate starvation or sufficiency; initially it was reported that the PHO regulon in S. cerevisiae is formed by 22 genes, nine of which are regulatory genes (Ogawa et al., 2000). Similarly, nine genes homologous to those of the yeasts PHO regulon were identified later in the genome of Aspergillus fumigatus (Serra-Cardona et al., 2014), however, recent studies have reported a larger number of genes controlled by phosphate in the genome of some fungi (Tomar and Sinha, 2014). A study of Fusarium graminearum phosphatome identified up to 82 phosphatase genes (Yun et al., 2015). Eleven of these genes were found to be essential but the remaining 71 phosphatase genes have also impact in fungal development. These phosphatases were shown to be involved in fungal growth and differentiation, in the biosynthesis of secondary metabolites, pathogenesis and virulence.
The proteins encoded by the PHO regulon genes are located in the plasma membrane, the periplasmic space, vacuoles, mitochondria or the cytosol. Several genes were found to encode proteins for scavenging phosphate from the extracellular medium, for polyphosphate hydrolysis and transport across vacuole or mitochondria membrane systems (Persson et al., 2003). Phosphate transport and metabolism are important factors in fungal virulence and pathogenesis. Mutants of Cryptococcus neoformans defective in phosphate acquisition and storage showed altered virulence and pathogenicity associated with the production of bioactive metabolites (Kretschmer et al., 2014).
Particularly relevant was the finding in fungi of genes encoding proteins for the transport of inorganic phosphate and regulatory genes that control inorganic phosphate homeostasis. Two of the PHO regulon proteins, encoded by pho4 and pho2, are master regulators that control expression of many pho genes. The S. cerevisiae Pho4 is a 312 amino acids transcriptional regulator that belongs to the basic helix-loop-helix (bHlH) family (Persson et al., 2003). Pho4 is a dimer that works in collaboration with Pho2 controlling the transcription of the PHO regulon genes and its action is modulated by phosphorylation at serine-threonine residues, that is catalysed by Pho85/Pho80 cyclin-kinase complex (see below) (Komeili, 1999; Secco et al., 2012a; Tomar and Sinha, 2014). Initial studies on the control of gene expression by this transcriptional regulator was made by studying the expression of pho5, a gene that encodes the yeast secreted acid phosphatase, an easy to measure reporter of the Pho4-mediated regulon genes. Two activating sequences Uasp1 and Uasp2 that bind Pho4 were found in the region upstream of the pho5 promoter (Rudolph and Hinnen, 1987; Ogawa et al., 2000). The binding sequences of the Pho4 protein are CACGTG or CACGTT, the Uasp2 sequence is protected by the nucleosome structure and therefore only the Uasp1 sequence is accessible unless the chromatin is remodelled by different protein modifications including acetylation/deacetylation or phosphorylation (Steger et al., 2003). Pho4 binding sequences have been found in almost all the promoters of the PHO regulon genes (Ogawa et al., 2000). Pho4 plays also an important role in the control of pathogenicity and virulence of pathogenic fungi. Studies using a C. neoformans mutant defective in Pho4 showed that this regulatory protein is required for phosphate uptake, growth and C. neoformans dissemination into the brain (Lev et al., 2017; Bhallan et al., 2022).
2.2 Inositol pyrophosphates signaling molecules as reporters in phosphate homeostasis
The mechanism by which fungal cells sense inorganic phosphate is poorly characterized. Inositol pyrophosphates are a family of metabolic messengers characterized by the presence of one or more pyrophosphate (PPi) groups attached to a mio-inositol ring (Wera et al., 2001). The presence of energy rich phosphoanhydre bonds in these molecules is one of the characteristic features that explain their important role in regulation of phosphate metabolism. The intracellular messenger IP3 (1,4,5 inositol-triphosphate) is formed by phospholipase C that cleaves phosphatidyl-inositol-4,5 diphosphate yielding IP3 and diacylglycerol. In yeasts, higher members of this family e.g. mio-inositolheptakisphosphate (IP7) are formed by sequential phosphorylation of IP3 by pyrophosphate kinases and higher members that contain more phosphate groups than carbon atoms reflects the energetic and phosphorylation status of the cell. Particularly, the ATP concentration required for the conversion of mio-inositol hexakisphosphate (IP6) to IP7 since the IP6 kinase has a Km for ATP in the millimolar range (Azevedo and Saiardi, 2017).
In this respect an important finding was the observation that the intracellular concentration of inositol pyrophosphate changes in response to the phosphate concentration in the medium. Proteins involved in cellular reactions of phosphate metabolism, e.g. phosphate transporters, phosphate signaling proteins or polyphosphate polymerases contain a so called SPX domain that interact with inositol pyrophosphates signaling molecules (Secco et al., 2012b). These domains consist of 135–380 amino acid residues and are located in the amino terminal region of the corresponding protein. Initial studies showed that the SPX domain serves as substrate for the interaction of inositol pyrophosphate signaling molecules in plants and animals. In S. cerevisiae the pho90 and pho87 phosphate transporters interact with the SPL2 protein at its SPX domain and this decreases the phosphate uptake (Wykoff et al., 2007; Hürliman et al., 2009). Three SPX domains of different organisms, including S. cerevisiae (Hothorn et al., 2009) were crystalized, providing evidence of the SPX surface that interacts with inositol pyrophosphate (Samyn et al., 2012; Wild et al., 2016). Furthermore, IP7 and higher inositol pyrophosphates act as donors of orthophosphate in protein modifications catalysed by pyrophosphorylases that result in the introduction of an additional phosphate group in the serine phosphate residues in proteins to form serine pyrophosphate units. Therefore, increments in the ATP levels of the cell raises the intracellular level of IP7. In summary, inositol pyrophosphate molecules communicate the inorganic phosphate extracellular level to the SPX domain of the phosphate transporters and other proteins involved in phosphate metabolism, regulating their activity.
2.3 Mechanisms of phosphate sensing and transport in fungi
Phosphate availability is extremely important to fungi for growth. Inorganic phosphate is taken up in yeasts and filamentous fungi by the high affinity transporters systems encoded by pho84 and pho89 (Bun-ya et al., 1991; Martínez and Persson, 1998; Persson et al., 1999) and low affinity transporters encoded by pho87 and pho90 (Bun-ya et al., 1996; Ghillebert et al., 2011; Wykoff and O´Shea, 2001). When the phosphate concentration in the medium approaches the Km of the low affinity system then the cells trigger the formation of high affinity transporters. This provides a time lapse for the cell to survive using the phosphate low affinity transport system until the high affinity transport is fully induced. The importance of phosphate availability in fungal nutrition is highlighted by the presence of five phosphate transport genes pho84, pho87, pho89, pho90 and pho91 in S. cerevisiae; only quintuple mutants defective in the five transporters are unable to grow, indicating that all of them are active phosphate transporters (Wykoff and O’Shea, 2001).
Analysis of the hydrophobicity of the encoded proteins in yeast showed that both Pho84 and Pho89, belong to the multiple facilitator superfamily (MFS transporters) and have 587 and 574 amino acids respectively, have 12 transmembrane spanning domains with an intervening hydrophilic loop of 74 amino acids between the transmembrane domains TMS6 and TMS7 in Pho84, and a loop of 110 amino acids located between TMS7 and TMS8 in Pho89 (Bottger and Petterson, 2002; Persson et al., 2003). The MFS proteins include many transporters for several primary or secondary metabolites that are involved in communication between cells in pure cultures and/or between different species (Martín et al., 2005). Pho84 and Pho89 are symporters that introduce inorganic phosphate and either protons or Na+, respectively. The S. cerevisiae Pho89 high affinity symporter is functionally similar to other sodium transporters in mammals and Neurospora crassa (Bottger and Petersson, 2002). The similarity between Pho84 and Pho89 sequences is low (15% amino acids identity) and there are important differences between them. Pho84 has an optimal pH of 4.5 while the optimal pH for Pho89 is 9.5 (Persson et al., 2003). These transporters are under strict phosphate control and are only derepressed at low phosphate concentration. In addition to its control by phosphate starvation, expression of pho84 and pho89 increases at alkaline pH, particularly the expression of pho89 is enhanced by alkaline pH even in mutants defective in the transcriptional activator Pho4 (Lamb et al., 2001; Serrano et al., 2002). The evolutive acquisition of high affinity and low affinity transport systems appears to confer metabolic advantage to yeasts and filamentous fungi (Levy et al., 2011) as it is also well documented in bacteria (Martín and Liras, 2021a).
2.4 Transport related nutrient effectors: Pho84
Transport related nutrient effectors (named transfectors) have been discovered in S. cerevisiae and in several other eukaryotic cells. These proteins serve as nutrient sensors and are also involved in the nutrient transport mechanisms. In S. cerevisiae Pho84 is a transfector that is involved in phosphate sensing and transport, and as effector rapidly activates the protein kinase A pathway in concert with glucose (Giots et al., 2003). Arsenate, is a non-metabolizable structural analogue of phosphate that inhibits growth of fungi suggesting that arsenate binds to phosphate receptors. Mutants defective in the high affinity Pho84 transporter are unsensitive to arsenate suggesting that Pho4 is involved in both phosphate sensing and transport into the fungal cells (Giots et al., 2003; Wykoff et al., 2007; Popova et al., 2010). Phosphate containing molecules that interact with the Pho84 transporter can trigger the phosphate signaling without been transported, as is the case with glycerol-3-phosphate (Popova et al., 2010). These compounds behave as phosphate agonists of Pho84 and, therefore, affect the signaling activity of phosphate. In summary, this information indicates that agonists exert their function by interacting with the Pho84 phosphate binding site but do not require a complete transport into the cell.
2.5 Characteristics of the Pho89 phosphate transporter: evidence for interaction of phosphate and calcium metabolism
Remarkably, the Pho89 symporter is under the control of a calcium TRP (Jiang and Pan, 2018) providing evidence of a close interaction between calcium transport and phosphate regulation. The Pho89 phosphate transporter in yeasts is a high affinity transporter functionally similar to the Pit transporter in bacteria (Martín and Liras, 2021) and to the mammalian Na+ P3, and is conserved from bacteria to vertebrates (Werner and Kinne, 2001). This protein has 10–12 transmembrane domains, depending on the species, and a large hydrophilic loop between regions TMS7 and TMS8 that has been shown to be located in the cytosolic side of the cell membrane (Chien et al., 1997; Persson et al., 1999). The Pho89 protein of S. cerevisiae has a Km for Pi of between 0.4 and 0.6 μM. This transporter is active at alkaline pH but lacks affinity for the substrate at pH below 4.5 in contrast to the proton dependent Pho84 that is very active at a wide pH range (Martínez and Persson, 1998).
In S. cerevisiae the Pho89 encoding gene is governed by a Crz zinc finger type transcriptional factor that is activated by the calcium-calcineurin system; accordingly, the pho89 gene is no expressed in crz1 mutants (Serrano et al., 2002). Notably, in S. cerevisiae expression of pho89 transporter gene is controlled by Crz1 transcriptional activator of calcium metabolism genes under conditions of alkaline pH providing evidence for another interaction between phosphate metabolism and calcium homeostasis (Serra-Cardona et al., 2014, Box 1). These findings agree with recent observations in C. albicans, in which the calcium permeable stress gated CaPhm7 affects the expression of pho89 (Jiang and Pan, 2018). These results suggests that Pho89 has a clearly distinct role than Pho84 and connects phosphate transport with calcium metabolism (Secco et al., 2012a).
2.6 Signaling molecular mechanism and circuits of control regulated by the Pho4 transcriptional factor
The mechanisms of regulation of phosphate transport and metabolism by the master transcriptional regulator Pho4 are well documented. As indicated above the activation or inactivation of the pho genes takes place by phosphorylation or dephosphorylation of the Pho4 regulator. In phosphate sufficient conditions Pho4 is phosphorylated by protein kinases and then excluded from the nucleus to the cytosol, therefore, the PHO regulon genes remain unexpressed (Secco et al., 2012a). In phosphate depleted conditions Pho4 is unphosphorylated, binds to a nuclear import receptor and is introduced in the nucleus where attach to response elements (PRE) and activates different genes of the PHO regulon, including pho84 and pho89 (Mouillon and Persson, 2006; Tomar and Sinha, 2014). The phosphorylation of Pho4 is exerted by the cyclin-dependent kinase (CDK) complex Pho80-Pho85. This CDK complex phosphorylates serine/threonine residues in different sites of the Pho4 transcriptional factor and this causes distinct cellular localization (Komeili, 1999; Secco et al., 2012a; Secco et al., 2012b). The activity of the cyclin-dependent kinase complex CDK is controlled by Pho81, which is a CDK inhibitor. Under phosphate limiting condition the cyclin-dependent protein kinase complex is inhibited, resulting in a unphosphorylated active Pho4 and thus the genes of the PHO regulon are activated; vice versa, under phosphate sufficiency conditions the Pho81 activity is null and the cyclin-dependent protein kinase is active, resulting in a phosphorylated Pho4 that remains in the cytosol, and therefore the PHO regulon genes are not expressed (Schneider et al., 1994; O´Neill et al., 1996) (Figure 1).
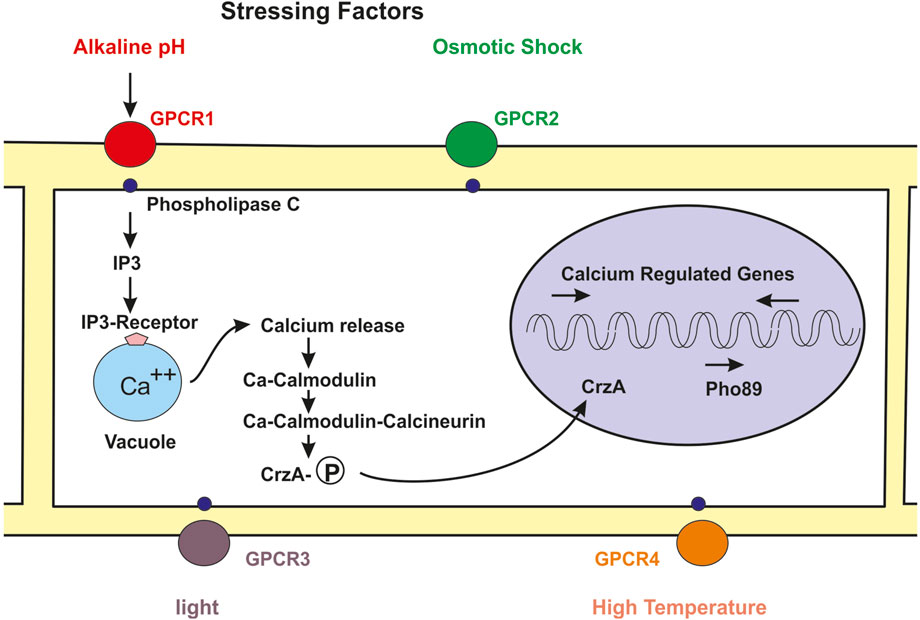
FIGURE 1. Skeme of the molecular mechanism of regulation of fungal metabolism by the calcium-calmodulin-calcineurin pathway. Numerous stressing signals interact with GPCRs receptors in the cell membrane (circles red, green, purple, orange). The GPCR bind to the phospholypase C (small blue circles) and this protein forms IP3. The binding of IP3 to its receptor in the vacuole (blue clear circle) membrane triggers the release of calcium to the cytosol. Free cytosolic calcium binds to calmodulin and the calcium-calmodulin complex activates the calcineurin phosphatase activity. The phosphatase activity removes phosphate from the phosphorylated Crz1 transcriptional factor and the unphosphorylated Crz1 protein enters into the nucleus (pink circle) and activates expression of the high affinity phosphate pho89 transport gene and of numerous genes regulated by calcium.
In addition to phosphorylation by the CDK complex the action of Pho4 is affected by trans-acting factor; they include the cooperation with the Pho2 homeodomain (Ogawa et al., 2000; Zhou and O´Shea, 2011). According to these authors Pho2 bind to a sequence distant 15 bp from the Pho4 binding site. When attached to this position Pho2 exert an activation of Pho4 binding to its target DNA. Furthermore, the interaction of Pho4 with its target sequence is controlled by the nucleosome in that DNA region. In S. cerevisiae nucleosome depleted regions there is a competition with the Cbf1 factor that recognize the same DNA sequence as Pho4, the relative abundance in the nucleus of these two proteins, Pho4 and ScBf1, determines the occupancy of the Pho4 binding site in the DNA and therefore the expression of the PHO regulon genes (Zhou and O´Shea, 2011). Detailed studies of the genes encoding enzymes of the PHO regulon have been performed only in a few model filamentous fungi, for example N. crassa (Tomar and Sinha, 2014). The core of the PHO regulon enzymes is conserved in the studied yeasts and most filamentous fungi but there are significant differences among them. The designation of functionally similar genes is sometimes different, e.g. the core PHO regulon genes in N. crassa are named nuc-1, preg, pgov and nuc-2 that correspond to the equivalent pho4, pho80, pho85 and pho81 genes of S. cerevisiae, respectively (Peleg et al., 1996; Gras et al., 2007; Tomar and Sinha, 2014). Although the core components of the S. cerevisiae PHO regulon are conserved in N. crassa it is unclear whether additional components occur in other filamentous fungi.
3 Calcium in fungi metabolism, an overview
Calcium is an essential nutrient that plays very important roles in fungal metabolism, affecting growth and differentiation (Tisi et al., 2016). Calcium ions serve as intracellular messengers common to all eukaryotic cells. Several extracellular signals, including divalent cations, lithium, ethanol, caffeine, some nitrogen sources, stressing pH and temperatures and antifungal drugs increase the intracellular calcium concentration (Thewes, 2014).
Initial studies on the regulation of calcium metabolism were performed in S. cerevisiae and N. crassa. At the end of the last century research work on the model filamentous fungi N. crassa, showed that fungi establish a calcium gradient in the hyphae that is important for apical fungal growth and development (Jackson and Heath, 1993; Kothe and Free, 1998), and for the secretion of secondary metabolites (Prokisch et al., 1997). In addition, calcium affects the production of bioactive secondary metabolites in many filamentous fungi. One example is the control by calcium of the production of penicillin and the expression of the penicillin biosynthetic genes (Domínguez-Santos et al., 2017). Vacuoles, peroxisomes and related microbodies involved in penicillin biosynthesis and secretion are concentrated in a hyphal region determined by the calcium gradient (reviewed by Martín and Liras, 2022b).
3.1 Tolerance to calcium stress: the neuronal calcium sensor
Calcium is taken up by the cells using different P-type membrane calcium translocases and calcium ATPase pumps (reviewed by Espeso, 2016). Excessive accumulation of calcium in the cells creates a calcium stress problem since high intracellular concentration of calcium interfers with nucleotide, polyphosphate and other macromolecules function. To avoid the calcium toxicity fungi and other eukaryotic cells have a system for calcium tolerance/sensitivity, the neuronal-calcium sensor (NCS). Transient increase in calcium causes activation of various Ca2+ binding proteins, including the neuronal calcium sensor-1 (NCS-1). Members of the NCS family contain an N-terminal myristoylation domain and four Ca2+ binding EF-hand domains (Burgoyne et al., 2004; Burgoyne, 2007). The NCSs are small proteins evolutively conserved across the species, and homologue proteins have been identified in yeast, filamentous fungi and mammals among other living beings (Sánchez-Gracia et al., 2010; Tamuli et al., 2011) In S. cerevisiae the NCS-1 protein is essential for growth and it is involved in the regulation of the activity and cellular location of the phosphatidyl-inositol-4-kinase (Huttner et al., 2003; Strahl et al., 2007). A knock-out mutant of N. crassa NCS-1 shows increased sensitivity to high calcium concentration and UV light (Tamuli et al., 2011) suggesting that NCS-1 is involved in calcium tolerance in this fungus. Similar increase sensitivity to calcium concentration has been observed in a NCS mutant of Magnaporthe grisea (Saitoh et al., 2003); however, the NCS null mutant of A. fumigatus does not show increased sensitivity to calcium concentration, indicating that the behaviour of NCS in A. fumigatus is different from that of other fungi (Mota et al., 2008). The NCS-1 homologous of A. fumigatus (NCS-A) is not essential for growth but plays an important role in sterols distribution in membrane domains and polar growth (Mota et al., 2008). The mechanism of NCS-mediated stress tolerance has been studied in N. crassa (Gohain and Tamuli, 2019). Increasing calcium levels enhances expression of the NCS-1 encoding gene. Importantly, these authors observed that the expression of the NCS-1 encoding gene is controlled by the calcineurin responsive zinc finger Crz1 transcriptional regulator (see below) indicating that there is a clear regulation of the NCS-1 protein by the calmodulin-calcineurin signaling pathway.
3.2 The calcium sensor calmodulin and its interaction with proteins
Intracellular calcium binds to calmodulin, a protein encoded by the cmd1 gene (Cyert, 2001). Calmodulin is a small protein, 17 kDa, that is highly conserved in all eukaryotic organisms, and binds Ca2+ ions with high affinity (Kd 10−6 to 10−5 M). In S. cerevisiae it contains four amino acid motives, named EF-hands for calcium binding, although only three of the EF-hands in the calmodulin molecule are functional, the fourth lacks the Ca2+ binding loop (Kraus and Heitman, 2003). These motives consist in 12 amino acid loops that connect alpha helices and contain glutamic acid and aspartic acid residues that chelate the Ca2+ ion (Davis et al., 1986; Cyert, 2001).
Calmodulin, usually activated by Ca2+ acts by at least three different mechanisms: 1) interacting directly with proteins involved in the biosynthesis of bioactive metabolites, differentiation and pathogenicity, 2) Activating the calcium-calmodulin complex a class of protein kinases named calcium/calmodulin-dependent protein kinases, and 3) binding to the protein calcineurin what triggers the calmodulin-calcineurin cascade that regulates numerous genes in the cell (Figure 1).
3.3 Direct interaction of calmodulin with secondary metabolites biosynthetic enzymes
Calmodulin interacts directly with many proteins in fungal cells. In the calcium-dependent processes calmodulin binds calcium through the EF hands what results in a change of calmodulin configuration that modulates its interaction with other proteins.
The direct interaction of calmodulin with secondary metabolites synthesizing enzymes has been studied in the insect pathogen Beauveria bassiana; this fungus produces several secondary metabolites, including compounds derived from the phenylpropanoid pathway and non-ribosomal peptides among others. The phenylpropanoid pathway starts from phenylalanine that is converted to cinnamic acid by the phenylalanine ammonia lyase (PAL) or a related tyrosine ammonia lyase (TAL) that forms coumaric acid (Figure 2A) (Martín and Liras, 2022a). Calmodulin interacts directly with the PAL enzyme of B. bassiana and inhibits its activity (Kim et al., 2015). Other interesting example is the formation of beauvericin, a product of B. bassiana with antimicrobial, antiviral and insecticidal activities. One structural component of beauvericin is 2-hydroxyisovaleric acid that is formed from 2-ketoisovaleric acid by the 2-ketoisovaleric acid reductase. Then a 325 kDa non-ribosomal peptide synthetase (NRPS) forms beauvericin (Figure 2B). Calmodulin binds the 2-ketoisovalerate reductase in vivo and in vitro and inhibits the biosynthesis of this precursor and of the final product, beauvericin (Kim et al., 2016). Salt stress and light inhibit the biosynthesis of 2-ketoisovalerate and beauvericin and this inhibition is reversed by calmodulin inhibitors indicating that the effect of these stressing factors is mediated by the interaction of calmodulin with the 2-ketoisovalerate reductase. In addition, calmodulin interact directly with the beauvericin synthetase. Calmodulin binding sequences have been found in the PAL, the 2-ketoisovalerate reductase and the beauvericin synthetase of B. bassiana (Figure 2) and similar sequences occur in many calmodulin binding proteins (Kim and Sung, 2018). The calmodulin binding domain consist in stretches of 16–35 amino acids that form a basic amphipathic α-helix (O´Day, 2003). The beauvericin synthetase contains a calmodulin-binding domain in the C-terminal region; binding of calmodulin to this region was confirmed by experiments using a synthetic binding domain and the formation of a complex was demonstrated by non-denaturing polyacrylamide gel electrophoresis (Kim and Sung, 2018). Formation of this complex takes place in presence of calcium ions but not in their absence. The direct interaction of calmodulin with enzymes involved in the biosynthesis of bioactive metabolites is of great interest although much more research needs to be done to advance in this field.
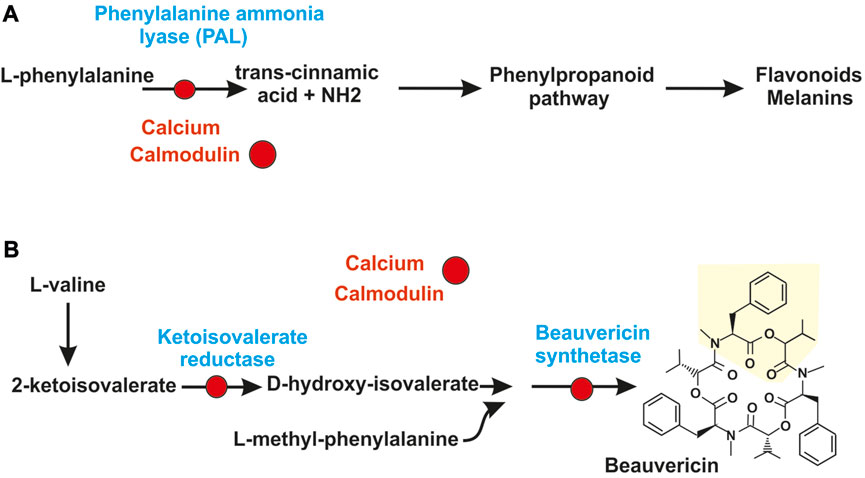
FIGURE 2. Calcium control of secondary metabolites by direct interaction of calmodulin with secondary metabolites synthesizing enzymes. (A) Regulation by calcium of the phenylpropanoid pathway. The calcium-calmodulin complex (red circles) binds and inactivate the phenylalanine ammonia lyase (PAL), first enzyme of the phenylpropanoid pathway. This inactivation blocks the pathway and the formation of secondary metabolites derived from it, as flavonoids and melanins. (B) Skeme of biosynthesis of beauvericin in Beauveria bassiana. The calcium-calmodulin complex (red circles) binds and inactivate: 1) the 2-ketoisovalerate reductase converting 2-ketoisovaleric acid in d-hydroxyisovalerate and 2) the non-ribosomal peptide synthetase of Beauveria bassiana that condenses three units of L-methyl-phenylalanine-d-hydroxyisovalerate depsipeptide to form beauvericin.
3.4 Calcium and calmodulin-dependent protein kinases
Very small increases in cytosolic calcium content triggers the rapid formation of several protein kinases, including the calcium/calmodulin-dependent protein kinases (named CamKs). These enzymes are serine/threonine protein kinases that are involved in distinct mechanisms of control of calcium homeostasis in eukaryotic cells. Most of these calcium/calmodulin dependent protein kinases have been characterized in mammals but there is little information of this group of proteins in filamentous fungi (Tamuli et al., 2011). Studies on the structure of these proteins indicate that they contain an amino terminal protein kinase domain linked to an autoinhibitory domain that overlaps with the calmodulin-binding domain (Hook and Means, 2001). These enzymes show an elaborated mode of action: the autoinhibitory domain binds to the catalytic centre and maintains the protein kinase in an inactive state until calcium and calmodulin bind to the calmodulin binding site and, therefore, activate the protein kinase activity. These calcium/calmodulin protein kinases differ in their range of substrate specificity (Tamuli et al., 2011). Protein kinases of this class were found in S. cerevisiae, Schizosaccharomyces pombe (Melcher and Thorner, 1996; Rasmussen, 2000) and also in several filamentous fungi (Table 1). In N. crassa two different CamKs protein kinases have been identified that regulate the circadian clock (Yang et al., 2001) and sexual development of ascospores (Tamuli et al., 2011). Recently, three calmodulin-dependent protein kinases have been studied in Alternaria alternata, a fungus that causes pears infection. Gene disruption studies indicated that mutation of one of the kinases gene does not affect the overall growth but reduces hyphal differentiation, sporulation and melanin formation (Jiang et al., 2023). Expression of the three kinases is intense during the infection process; in addition inhibition of CamKs activity supress the infection process indicating that these kinases play key roles in pathogenicity. Some of these enzymes are also important in the development of invasion structures in pathogenic fungi. In C. gloeosporioides an inhibition of the CamKs delays spore germination and formation of the appressorium. Treatment of C. gloeosporioides with inhibitors of these protein kinases reduces also the formation of melanin associated with the penetration of the appressorium during infection of the hosts (Kim et al., 1998). The available information suggests that there are different roles played by these calcium/calmodulin protein kinases in fungi. In summary, phosphorylation of proteins by these calcium calmodulin-dependent protein kinases provides a novel example of interaction between calcium homeostasis and phosphate regulation (Box 1).
3.5 The calmodulin-calcineurin signaling cascade
Fungi sense different nutrients in the medium and transduce the signal by cascades that produce adequate responses in the cell, some of them mediated by the calmodulin-calcineurin pathway (Martín et al., 2019). In contrast with the little information available on regulation of fungal metabolites by direct interaction of calmodulin with the antibiotic synthetases, the molecular mechanism of calcineurin-mediated regulation has received much attention in several fungi. Calcineurin is a heterodimeric protein phosphatase that consist in a catalytic subunit CnA and a regulatory subunit CnB. The catalytic subunit CnA contains the phosphatase active center and an associate regulatory region which are separated by a small amino acid stretch. This regulatory region serves to link together the CnA and CnB subunits and, in addition, contains domains for interaction with calmodulin and for self-inhibition (Klee et al., 1998). Calcineurin is the target of the immunosuppressants cyclosporin and FK506 (tachrolimus) and these compounds have been extensively used in research to block the activity of calcineurin in vivo. The calcineurin in S. cerevisiae regulates expression of numerous genes through the transcriptional factor Crz1 (calcineurin responsive zinc finger). For example, it regulates cell wall biosynthesis, ion homeostasis, vesicles traffic and protein degradation (Yoshimoto et al., 2002).
The calmodulin-calcineurin signaling pathway in filamentous fungi and yeasts is triggered by external stressing factors that are recognized at the membrane level by G protein couple receptors (GPCR) (Krishnan et al., 2012; Martín et al., 2019). Some phospholipases, particularly phospholipase C and the secretory phospholipase La2 (SPLa2) exert important roles in calcium homeostasis (Barman and Tamuli, 2015). In mammals, the GPCRs activate the membrane phospholipase C that forms inositol-1,4,5 triphosphate (IP3, so-called calcium releasing factor); this factor interacts with specific receptors resulting in cytosolic accumulation of Ca2+. Interaction of IP3 with the IP3 receptor (IP3R) changes the configuration of this receptor opening calcium channels (Prole and Taylor, 2011, 2019; Taylor and Machaca, 2019); as a result, calcium is released from the endoplasmic reticulum and is redistributed to the cytosol and different organelles. The IP3 receptors of mammals are phosphorylated by the cAMP-dependent protein kinase A (Taylor, 2017). This phosphorylation of IP3R is another example of the interaction of phosphate modification of proteins in calcium homeostasis. Phosphorylation of the IP3 receptors in humans enhance the IP3-mediated calcium release (Betzenhauser et al., 2010; Taylor, 2017) (Box 1). The IP3 receptor contain an ATP-binding site and it has been shown that ATP regulates IP3-mediated calcium release. In addition to ATP other adenine nucleotides and also GTP appears to exert a regulatory function on IP3R affinity, although the selectivity of the nucleotide binding is still unclear.
3.5.1 IP3 receptors in eukaryotes: do IP3 receptors exist in fungi?
IP3 receptors are expressed in most animal cells and protozoa (Prole and Taylor, 2011). This type of receptors has been described in several insects following the initial identification in Drosophila melanogaster (Yoshikawa et al., 1992). The IP3R of insects has about 60% identity to those of mammals suggesting that they are relatively well conserved (Toprak et al., 2021). Enzymes involved in the biosynthesis and turnover of IP3 have been found in endoparasites of the Apicomplexa group; however, there is no clear evidence of the presence of canonical IP3R in these parasites and has been suggested that they may harbour a primitive non-canonical type of IP3 receptor (Garcia et al., 2017). A similar non-canonical IP3R may occur in filamentous fungi, since the biosynthesis of IP3 and other enzymes related to IP3 metabolism occurs in fungi (see Section 2.2); however, additional research is needed to clarify this hypothesis.
The increase of intracellular calcium is sensed by calmodulin which upon calcium binding interacts with the calcineurin activating its phosphatase activity, this start the calmodulin-calcineurin cascade and results in the dephosphorylation of CrzA (Crz1) that is then transported to the nucleus by a nuclear membrane import receptor (Figure 1).
The fungal calcineurin is highly similar to the homologous phosphatase in animal cells, which plays a very important role in their metabolism. Pioneer studies in human cells indicated that calcineurin exert its function by dephosphorylating the nuclear factor of activating T cells (NFAT) what results in introduction of the dephosphorylated NFAT to the nucleus and subsequent activation of the calcineurin-dependent genes (Crabtree, 2001). A similar mechanism was found in filamentous fungi involving the CrzA transcriptional factor.
3.6 The calcineurin role in the calcium signaling pathway
In fungi calcineurin works through regulation of different transcriptional factors, of which the best known is Crz1 in S. cerevisiae, named CrzA in some filamentous fungi (Stathopoulos and Cyert, 1997). Crz1 contains a C2H2 zinc finger motif that binds to calcineurin-dependent regulatory elements (CDRE) (Fedotova et al., 2017; Gohain and Tamuli, 2019). The nuclear concentration of CrzA changes in a pulsatile mode rather than maintaining a constant level. This means that the CrzA-mediated calcineurin signaling is modulated by the frequency, duration and amplitude of the pulses (Dalal et al., 2014).
Following the identification of Crz1 in S. cerevisiae, the crzA gene was cloned from A. fumigatus and several other filamentous fungi (Soriani et al., 2008). Disruption and complementation of crzA in A. fumigatus proved that this gene is involved in cell tolerance to high calcium and manganese concentrations. In addition, the crzA mutant showed an altered expression of several genes encoding calcium transport at high calcium concentration, and decreased virulence. A detailed characterization of the role of CrzA has been made in A. nidulans using crzA defective mutants (Hagiwara et al., 2008; Spielvogel et al., 2008) demonstrating that CrzA was the regulator of calcium homeostasis. The crzA-defective mutant was highly sensitive to external calcium or manganese concentrations and to alkaline pH. This mutant was also altered in a vacuole calcium exchanger (Hagiwara et al., 2008). Spielvogel et al. (2008) observed that the crzA mutant had an aberrant morphology related to decreased expression of the chitin synthetase gene. Interestingly, GFP fluorescent protein labelled CrzA was located in the cytosol when the cells were cultured in low calcium concentration but upon calcium addition was rapidly internalized into the nucleus and therefore exerts its regulation by binding to CDRE sequences (Figure 1). The CrzA gene of Aspergillus parasiticus is required for the production of aflatoxin under calcium-stress conditions (Chang, 2008) and in Fusarium oxysporum CrzA is needed for the production of deoxynivalenol (DON) and for virulence (Chen L. et al., 2019) (see Section 3.7).
3.7 Ambient pH, osmolarity and heat shock stresses effect on the calcineurin mediated regulatory cascade
Several authors have studied the effect of alkaline pH or high calcium concentration on the control of the calmodulin-calcineurin cascade. It is well known that in S. cerevisiae alkaline pH triggers a rapid uptake of calcium and the calcium increment in the cytosol triggers the calcineurin mediated cascade (Serrano et al., 2002; Yoshimoto et al., 2002; Viladevall et al., 2004).
The role of Aspergillus oryzae calcineurin in response to stress has been studied in detail by Juvvadi et al. (2003). In A. oryzae sequences for binding stress response regulatory elements (STRE) and for heat stress factor binding have been found in the upstream region of the cnA gene of A. oryzae, encoding the calcineurin catalytic subunit CnA. The calcineurin activity in the wild type A. oryzae increased in response to alkaline pH, salt concentration, and high temperatures. Blocking the cnA mRNA by expression of the antisense RNA decreases the activity of the calcineurin under stressing conditions and caused reduced growth. In contrast overexpression of cnA resulted in an increased calcineurin activity under stressing conditions and produces tolerance to the calcineurin inhibitor FK506. These results support the conclusion that adaptation to different stressing factors in this fungus is mediated by the calcineurin signaling pathway.
3.8 Impact of the calmodulin-calcineurin pathway in expression of genes related to antifungal activity
There are many examples of the effect of stressing factors and alkaline pH on the production of antimicrobial fungal products. One of them is the formation of the cysteine rich antifungal protein AFP that is produced by several species of Aspergillus and Penicillium. The AFP protein is of interest because it shows antifungal activity against pathogenic yeasts and filamentous fungi (Huber et al., 2018).
The AFP protein is a small protein (6.2 kDa in P. chrysogenum) with potent antifungal activity toward Fusarium and Aspergillus species (Theis et al., 2005), some of which are plant pathogens. Therefore, it has been suggested to use AFP in the protection of vegetables such as tomato, grape and rice plant infections by Fusarium oxysporum and Botrytis cinerea. Expression of the AFP encoding gene is upregulated at alkaline pH (Meyer and Stahl, 2002a; Mayre et al., 2002b) and is abolished by the calcineurin inhibitor FK506 suggesting that transcription of the encoding gene is regulated by the calmodulin/calcineurin cascade (Meyer et al., 2005). This hypothesis is supported by the finding in the upstream region of the AFP encoding gene of a CDRE sequence that is the binding site for the CrzA transcriptional regulator. The mode of action of the AFP protein in relation to calcium metabolism has been elucidated in N. crassa. The AFP protein was shown to increase significantly the intracellular calcium concentration of N. crassa resting cells. The response of calcium metabolism to the addition of AFP change by chelating the extracellular calcium with EDTA, or other calcium specific chelators; calcium chelation counteract the AFP perturbation of calcium homeostasis indicating that calcium ions are involved and required for the AFP toxicity effect. The calcium transport blocker diltiazem exerts the same effect on calcium homeostasis as the addition of AFP (Binder et al., 2010). The addition of both AFP and diltiazen exert an cumulative effect on calcium homeostasis. In summary, in N. crassa the effect of AFP is due to the transport of calcium into the cells or its release from internal stores into the cytosol, where it triggers the regulation of calcium homeostasis, although the molecular mechanism by which AFP affects the calcium sensing and/or transport is not yet clear.
3.9 Calcineurin in fungal pathogenesis related to production of bioactive secondary metabolites
The infection and virulence of several animal and plant pathogenic fungi is regulated by calcium. Early studies on calcineurin mediated regulation in the pathogenic filamentous fungi M. grisea and B. cinerea indicated that calcineurin controls the formation of invasion structures. The development of these structures and the infection in plants is inhibited by cyclosporin (Viaud et al., 2002, 2003; Schumacher et al., 2008). In these infections, calcium regulates growth rate, morphology, differentiation of the filamentous fungus and attachment and penetration in the plant (Gupta et al., 2022). This is a very complex phenomenon that requires many enzymes, and bioactive metabolites that play important roles in intercellular communications (Table 2), however, in only a few fungal infections there is clear evidence of the involvement of calcineurin regulation in secondary metabolites production. Here we describe a few of the more representative examples. A. parasiticus produces several toxins, including aflatoxin. Mutants of two strains deficient in crzA, grown in media supplemented with calcium produce very low amounts of aflatoxin and O-methylsterigmatocystin. Transcriptional studies of the aflatoxin biosynthetic genes revealed that three of them, nor1, ver1 and omtA were very poorly transcribed in a crzA defective mutant indicating that expression of the aflatoxin genes was under the control of the calcium/calcineurin signaling pathway in calcium stressing conditions (Chang, 2008).
An important plant pathogen is Fusarium graminearum that produces the Fusarium head blight disease in wheat and other cereals (Chen et al., 2019). This fungus synthesizes several trichothecenes which are highly toxic and carcinogenic to humans and other mammals. The trichothecenes include the toxins deoxynivalenol (DON), nivalenol, and zearalenone (Goswami and Kistler, 2004; Pestka and Smolinski, 2005). DON and its acetylated derivatives three acetyl deoxynivalenol and 15 acetyl deoxynivalenol are highly toxic compounds.
Recently, Chen et al. (2019a, b) studied the role of Crz1 of F. graminearum and observed that in addition to the effect on growth and differentiation, the Crz1 mediated regulation reduces drastically the trichothecenes formation. Importantly, expression studies of the F. graminearum crz1 mutant compared to the parental strain revealed that twelve trichothecene try genes involved in the biosynthesis of DON have significant reduced expression (Chen et al., 2019). These results connect previous studies on regulation of DON biosynthesis (Hu et al., 2014; Yu et al., 2014; Zheng et al., 2016) with the calcium-calcineurin mediated control of this toxin (Imazaki et al., 2010).
Melanin is a dark pigment that has important roles in protecting the fungal cells against UV irradiation and in the resistance of different microorganisms to antimicrobial agents (Nosanchuk and Casadevall., 2006). In Verticillium dahliae, that causes the Verticillium wilt in trees, the crzA gene is involved in the biosynthesis of microconidia melanin which is important for the pathogenesis of this fungus (Xiong et al., 2015). Similar observations were also made in the mutants defective in crzA of Penicillium digitatum that also forms melanized microconidia (Zhang et al., 2009). Another important fungal pathogen is the citrus pathogen Alternaria alternata that synthesizes the polyketide AK toxin. This fungus contains a PLC-phospholipase similar to the well characterized enzyme of N. crassa that forms IP3. IP3 diffuses and binds to IP3 receptors and induces release of calcium ions from the vacuoles. The phospholipase of A. alternata is involved in calcium homeostasis, pathogenicity and virulence (Tsai and Chung, 2014).
Another example of calcium-calcineurin Crz-mediated regulation of pathogenicity is the production of virulence factors by C. neoformans. This fungus is a basidiomycete that causes meningoencefalitis in humans, a severe disease with high mortality (Fu et al., 2018). During the infection C. neoformans release pigments, enzymes and several virulence factors that are secreted in vesicles, one of the major virulence factors is glucuronosyl-manan that is released forming the extracellular capsule, a pathogenicity determinant that has potent immunosuppressive activity (Rodrígues et al., 2008).
In N. crassa there are detailed studies on the effect of calcineurin on the sexual and asexual spore development (Tamuli et al., 2016) and on the formation of some secondary metabolites, e.g. carotenoids (Barman and Tamuli, 2015). The N. crassa carotenoids include the neurosporene astaxanthin and some carotenoid precursors (Avalos et al., 2013) that are responsible of the orange colour of N crassa. These carotenoids prevent damage produced by UV light irradiation (Barman and Tamuli, 2015).
3.10 Regulators of calcineurin biosynthesis: effect of Rcn regulators on the biosynthesis of secondary metabolites
Calcineurin affects many reactions in the cell and therefore its activity is likely to be regulated by different cell factors. A family of these factors, named calcipressins, bind the calcineurin CnA subunit inhibiting its phosphatase activity (Juvvadi et al., 2014, 2015). In yeasts one of the Crz regulated genes encodes a calcineurin modulator, Rcn1 (RcnA in some fungi), named for regulator of calcineurin, that belongs to the calcipressins family. These are cytosolic proteins that do not enter in the nucleus and, therefore, appear to modulate the calcineurin activity by direct or indirect interaction with this phosphatase. The CrzA protein of A. fumigatus affects also expression of some genes involved in different aspects of calcium metabolism (Soriani et al., 2010). A. fumigatus rcnA null mutants are affected in the expression of several genes, including the gene for the calcineurin subunit A (Pinchai et al., 2009) and, therefore, these authors proposed that RcnA might exert a feedback regulation of the calcineurin-mediated regulatory cascade.
Members of the Rcn family are conserved in all eukaryotes from yeast to humans (Kingsbury and Cunningham, 2000). Several studies provide evidence on the role of calcipressing in fungal biology including S. cerevisiae (Mehta et al., 2009) and in the fungi A. fumigatus, A. nidulans, C. neoformans and Magnaporte oryzae (Pinchai et al., 2009; Liu et al., 2022). The exact mechanism of regulation of calcineurin by Rcn1 is complex. This protein contains a proline-serine repeated motif which seems to be phosphorylated in two different serine residues by the mitogen-activated protein kinase (MAPK). As a consequence of the phosphorylation of Rcn1 the interaction with the calcineurin subunit A is modified and calcineurin phosphatase activity is modulated; when Rcn1 is unphosphorylated the regulator exert a negative effect on calcineurin while when Rcn1 is phosphorylated the regulator does not inhibit the calcineurin phosphatase activity (Hilioti et al., 2004; Kishi et al., 2007; Li et al., 2011).
Recently the mechanism of regulators of calcineurin on the biosynthesis of secondary metabolites has been elucidated in M. oryzae, a fungus that causes the rice blight disease (Zhang et al., 2016b, 2019). During infection M. oryzae attaches to the rice plant cells and form a melanin rich appressorium that penetrates the plant tissue (Zhang et al., 2019). Deletion of the MoRCN1 gene causes a decrease in virulence and reduces the formation of several secondary metabolites. Transcriptomic analysis of this M. oryzae mutant has revealed that the Rcn1 regulator enhances expression of 491 genes and causes downregulation of 377 genes. The Rcn1 regulated genes encode enzymes involved in the biosynthesis of lysine, serine, threonine and the aromatic amino acids, and several genes involved in lipids biosynthesis and fatty acid degradation that may provide precursors for secondary metabolites, including genes for the biosynthesis of staurosporin, indolditerpenoids, meroterpenoids and aflatoxins. The affected genes encode also an ABC transporter; these transporters are frequent in many secondary metabolite gene clusters (García-Estrada et al., 2011; Abu Ammar et al., 2013; Martín, 2020).
4 Interaction of phosphate and calcium regulation: vacuoles and acidocalcisomes
Acidocalcisomes are membrane bound acidic calcium store organelles rich in orthophosphate, pyrophosphate and polyphosphate bound to calcium, magnesium and sodium. In these organelles calcium is bound to a polyanionic matrix of polyphosphate although calcium may be released by alkalinization of these vesicles (Docampo and Moreno, 2011). Acidocalcisomes have been found in all kingdoms of life from bacteria to humans. In some bacteria aggregates of polyphosphate (called volutine granules) have been found to be surrounded by a membrane. These vesicles were initially characterized in Trypanosomes but since then they have been studied in different eukaryotic cells including some fungi (Docampo et al., 2010; Seufferheld et al., 2011). Acidocalcisomes have been found in arbuscular mycorrhizae, and acidocalcisome-like vacuoles occur in S. cerevisiae (Kikuchi et al., 2014; Vila et al., 2022). Acidocalcisome-like vacuoles are characterized by their biochemical features, mainly acidic character, storage of polyphosphate and calcium ions. One or two proton pumps and transporters maintain the acidity of these organelles including the proton vacuole ATPase in yeasts and/or the vacuole proton pyrophosphatase (VP1) in other eukaryotic cells. The synthesis of polyphosphate and its transport to these organelles is performed by a vacuole transporter chaperone complex (VTC) that is well characterized in yeasts and Trypanosomes (Lander et al., 2016). Regarding calcium transport, acidocalcisomes contain a calcium ATPse involved in calcium uptake and some eukaryotic organisms contain additional ATPses that transport magnesium, zinc, inorganic phosphate and polyamines (Huang et al., 2014). Indeed, four polyamine transporters named TPO1 to TPO4 have been found in the vacuole membrane of S. cerevisiae (Tomitori et al., 2001). They belong to the MFS superfamily and contain 12 transmembrane domains. Disruption of the tpo genes revealed that these polyamine transporters are involved in uptake of several polyamines and its accumulation in the vacuoles. The disrupted mutants showed increase sensitivity to polyamines, indicating that these transporters participate in polyamine tolerant mechanisms. Noteworthy, fungal vacuoles have similar behaviour as acidocalcisomes, including the ability to acidify vacuoles by calcium ATPses.
Acidocalcisomes are rich in pumps and membrane transporters involved in cation and phosphate homeostasis and calcium signaling. Acidic pH conditions are required to bind these metals to polyphosphate. The biosynthesis of polyphosphate by VTC in filamentous fungi vacuoles and protozoa acidocalcisomes support the proposal that these two organelles are somehow functionally similar. Specialized acidocalcisomes have been found in the tropical pathogenic fungus Fonsecaea pedrosoi (Franzen et al., 2008). This fungus contains melanosomes which are membrane bound organelles that contain high levels of polyphosphate, calcium, metals and particularly melanin. The internal pH of eukaryotic melanosomes is very acid and this acidity favours the binding of metals to polyphosphate. The pathogenicity of this fungus is associated to its high level of melanin (see above Section 3.7). Electron microscopy studies, biochemical and immunochemical analysis of the content of melanosomes revealed that melanin is accumulated in sequential stages of development of this structure, suggesting that these organelles are specialized in melanin accumulation (Huang et al., 2014).
5 Summary and future outlook
During the last decades it has been established that the biosynthesis of secondary metabolites in filamentous fungi is controlled by elaborated interactions between the phosphate concentration in the culture medium and calcium ions that serve as intracellular messengers in numerous reactions in the fungal metabolism. The biosynthesis of hundreds of secondary metabolites is controlled by phosphate in filamentous fungi and in bacteria; notable advances have been made in our understanding of the mechanisms of phosphate control of secondary metabolites in actinobacteria (Martín and Liras, 2021a) but there is less information on equivalent control in filamentous fungi. Twenty-two genes were initially reported in the PHO regulon in S. cerevisiae, nine of them regulatory genes; but many more genes are regulated by Pho4 in some filamentous fungi. Noteworthy, eighty-two phosphatase genes have been reported recently in the genome of F. graminearum (Yun et al., 2015). The homeostasis of phosphate in fungi and its role is more elaborate that it was previously reported. The importance of phosphate availability in fungal metabolism is reflected by the presence of two well characterized high affinity phosphate transporters in S. cerevisiae, Pho84 and Pho89, and two low affinity transporters, Pho87 and Pho90. Pho84 is a well know transfector (nutrient-related transport effector) that performs both phosphate sensing and phosphate transport (Popova et al., 2010). Some phosphate analogues interact with phosphate receptors exerting a regulatory function although they are not transported into the cells. These findings open new fields for studying the interaction of transporting and sensing mechanisms that should be elucidated in the near future. In contrasts to Pho84, that is a phosphate and proton symporter, Pho89 is a phosphate/sodium symporter that in C. albicans is regulated by a TRP calcium ion channels providing evidence that there is a close connection between phosphate transport and calcium metabolism (Jiang and Yang, 2018). Regulation of the core components of the PHO regulon is exerted by dephosphorylation of the master regulator Pho4 that causes its entry into the nucleus where it exerts the regulation of the PHO regulon genes or its exclusion from the nucleus. However, how the degree of phosphorylation/dephosphorylation of Pho4 does affects the biosynthesis of phosphate regulated secondary metabolites? Activation of the PHO regulon genes by the master Pho4 regulator is modulated by competitive binding of other regulatory proteins such as Pho2 and Cbf1, that recognize the same Pho4 binding sequences in the promoter of phosphate regulated genes (Zhou and O´Shea, 2011). It is likely that other still unknown transactivating or competing factors may modulate the interaction of Pho4 with its target sequences. Phosphate regulation of the biosynthesis of secondary metabolites, e.g. penicillin biosynthesis, is exerted in collaboration with carbon catabolite regulatory factors (Martín et al., 1999) but further studies on the mechanism of this concerted regulation still needs to be fully elucidated (Giots et al., 2003). Phosphate homeostasis in fungi is known to be related to the energetic and phosphorylation status of the cells that responds to the intracellular ATP versus AMP ratio and this is transmitted in the cells by a family of highly phosphorylated inositol pyrophosphate molecules (IP6, IP7 and higher members of the family); this provides an interesting information on how the overall phosphorylation and energetic metabolism of the cell is modulated (Secco et al., 2012; Wild et al., 2016; Azevedo and Saiardi, 2017). Regarding calcium homeostasis in fungi it is well know that calcium serves as intracellular messenger in all eukaryotic cells. The intracellular calcium concentration in fungi responds to a large variety of input signals including divalent cations, lithium, stressing pH and temperature, ethanol, caffeine, and some antifungal agents (Thewes, 2014). Filamentous fungi establish a gradient of calcium along the hyphae and this is related to the targeting of vesicles involved in secondary metabolism biosynthesis that accumulated in distal section of the hyphal tips, therefore, molecular mechanism related to calcium homeostasis and calcium gradients within hyphae are important aspects of frontier research to elucidate the secretion mechanism of bioactive metabolites. Calcium regulation of fungal metabolism is mediated by the calcium binding protein calmodulin and by its interaction with the phosphatase calcineurin. Noteworthy, calmodulin in response to calcium binding interacts directly with three different secondary metabolite biosynthesis enzymes in B. bassiana, particularly with the beauvericin synthetase, a non-ribosomal peptide synthetase (Kim and Sung, 2018). However, there are very few studies on this direct interaction of calmodulin with secondary metabolism biosynthesis enzymes and this field needs to be supported by additional research. A central role in the regulation of calcium homeostasis is exerted by the calcium-calmodulin-calcineurin signaling cascade in response to external stressing factors recognized by G proteins coupled receptors (GPCR). The membrane phospholipase C triggers the formation of IP3 calcium releasing factor that, therefore, increases the cytosolic concentration of this ion; the increased calcium ions bind calmodulin and triggers the calmodulin-calcineurin cascade (Figure 1). The zinc finger Crz1 (CrzA) factor in response to calcium concentration is dephosphorylated entering into the nucleus where it controls the expression of numerous genes by binding the calcineurin dependent regulatory elements. An important interaction occurs between the Crz1 factor and the phosphate metabolism as shown by the fact that Crz1 defective mutants of S. cerevisiae are unable to express the Pho89 transporter gene. In other word, calcium concentration in the cell controls phosphate uptake and metabolism by the Pho89 transporter in a Crz1-dependent manner. An impressive amount of fungal metabolites that include melanin and carotenoid pigments, antibiotics, antitumor agents and aflatoxins are regulated by calcium ions through CrzA (Table 2).
A third example of link between calcium and phosphate metabolism is the presence in fungi of calcisome-like vacuoles; calcisomes are membrane-bound typical calcium stores rich in polyphosphate. In these organelles calcium is bound to a polyanionic matrix of orthophosphate, pyrophosphate and polyphosphate; calcium ions may be released by alkalinization of the cells. In protozoa the cell biology of acidocalcisomes is well known; therefore, information that serves as model for these fungal calcisome-like vacuoles might be obtained by comparison of the calcium and phosphate effect on stress in these organisms. Progress in the characterization of acidocalcisomes in filamentous fungi will significantly improve our understanding of the molecular mechanism of interaction between phosphate control and calcium homeostasis.
Author contributions
JM was the only contributor in interpreting the literature data and writing the manuscript.
Acknowledgments
I acknowledge the University of León for allowing informatic connections through the institutional Web. I thank Prof. Paloma Liras for the helpful scientific discussions.
Conflict of interest
The author declares that the research was conducted in the absence of any commercial or financial relationships that could be construed as a potential conflict of interest.
Publisher’s note
All claims expressed in this article are solely those of the authors and do not necessarily represent those of their affiliated organizations, or those of the publisher, the editors and the reviewers. Any product that may be evaluated in this article, or claim that may be made by its manufacturer, is not guaranteed or endorsed by the publisher.
References
Abu-Ammar, G., Tryono, R., Döll, K., Karlovsky, P., Deising, H. B., and Wirsel, S. G. R. (2013). Identification of ABC transporter genes of Fusarium graminearum with roles in azole tolerance and/or virulence. PLoS One 8, e79042. doi:10.1371/journal.pone.0079042
Avalos, J., Luis, M., and Corrochano, L. M. (2013). “Carotenoid biosynthesis in Neurospora,” in Neurospora: Genomics and molecular biology Editors D. P. Kasbekar, and K. McCluskey (Norfolk, UK: Caister Academic Press), 227–241.
Azevedo, C., and Saiardi, A. (2017). Eukaryotic phosphate homeostasis: The inositol pyrophosphate perspective. Trends biochem. Sci. 42, 219–231. doi:10.1016/j.tibs.2016.10.008
Barman, A., and Tamuli, R. (2015). Multiple cellular roles of Neurospora crassa plc-1, splA2, and cpe-1 in regulation of cytosolic free calcium, carotenoid accumulation, stress responses, and acquisition of thermotolerance. J. Microbiol. 53, 226–235. doi:10.1007/s12275-015-4465-1
Betzenhauser, M. J., and Yule, D. I. (2010). Regulation of inositol 1,4,5-trisphosphate receptors by phosphorylation and adenine nucleotides. Curr. Top. Membr. 66, 273–298. doi:10.1016/S1063-5823(10)66012-7
Bhallan, K., Qu, X., Kretschmer, M., and Kronstad, J. W. (2022). The phosphate language of fungi. Trends Microbiol. 30, 338–349. doi:10.1016/j.tim.2021.08.002
Binder, U., Chu, M., Read, N. D., and Marx, F. (2010). The antifungal activity of the Penicillium chrysogenum protein PAF disrupts calcium homeostasis in Neurospora crassa. Eukaryot. Cell. 9, 1374–1382. doi:10.1128/EC.00050-10
Bootman, M. D., Collins, T. J., Peppiatt, C. M., Prothero, L. S., MacKenzie, L., De Smet, P., et al. (2001). Calcium signalling-an overview. Semin. Cell Dev. Biol. 12, 3–10. doi:10.1006/scdb.2000.0211
Bottger, P., and Pedersen, L. (2002). Two highly conserved glutamate residues critical for type III sodium-dependent phosphate transport revealed by uncoupling transport function from retroviral receptor function. J. Biol. Chem. 277, 42741–42747. doi:10.1074/jbc.M207096200
Bun-ya, M., Nishimura, M., Harashima, S., and Oshima, Y. (1991). The PHO84 gene of Saccharomyces cerevisiae encodes an inorganic phosphate transporter. Mol. Cell. Biol. 11, 3229–3238. doi:10.1128/mcb.11.6.3229-3238.1991
Bun-ya, M., Shikata, K., Nakade, S., Yompakdee, C., Harashima, S., and Oshima, Y. (1996). Two new genes, PHO86 and PHO87, involved in inorganic phosphate uptake in Saccharomyces cerevisiae. Curr. Genet. 29, 344–351. doi:10.1007/s002940050055
Burgoyne, R. D. (2007). Neuronal calcium sensor proteins: Generating diversity in neuronal Ca2+ signalling. Nat. Rev. Neurosci. 8 (3), 182–193. doi:10.1038/nrn2093
Burgoyne, R. D., O′Callaghan, D. W., Hasdemir, B., Haynes, L. P., and Tepikin, A. V. (2004). Neuronal Ca2+-sensor proteins: Multitalented regulators of neuronal function. Trends Neurosci. 27, 203–209. doi:10.1016/j.tins.2004.01.010
Calvo, A. M., and Cary, J. W. (2015). Association of fungal secondary metabolism and sclerotial biology. Front. Microbiol. 16, 62. doi:10.3389/fmicb.2015.00062
Chang, P-K. (2008). Aspergillus parasiticus crzA, which encodes calcineurin response zinc-finger protein, is required for aflatoxin production under calcium stress. Int. J. Mol. Sci. 9, 2027–2043. doi:10.3390/ijms9102027
Chen, L., Tong, Q., Zhang, C., and Ding, K. (2019a). The transcription factor FgCrz1A is essential for fungal development, virulence, deoxynivalenol biosynthesis and stress responses in Fusarium graminearum. Curr. Genet. 65, 153–166. doi:10.1007/s00294-018-0853-5
Chen, Y., Kistler, H. C., and Ma, Z. (2019b). Fusarium graminearum trichothecene mycotoxins: Biosynthesis, regulation, and management. Annu. Rev. Phytopathol. 57, 15–39. doi:10.1146/annurev-phyto-082718-100318
Chien, M. L., Foster, J. L., Douglas, J. L., and García, J. V. (1997). The amphotropic murine leukemia virus receptor gene encodes a 71-kilodalton protein that is induced by phosphate depletion. J. Virol. 71, 4564–4570. doi:10.1128/JVI.71.6.4564-4570.1997
Clapham, D. E. (2003). TRP channels as cellular sensors. Nature 426, 517–524. doi:10.1038/nature02196
Crabtree, G. R. (2001). Calcium, calcineurin, and the control of transcription. J. Biol. Chem. 276, 2313–2316. doi:10.1074/jbc.R000024200
Cyert, M. S. (2001). Genetic analysis of calmodulin and its targets in Saccharomyces cerevisiae. Annu. Rev. Genet. 35, 647–672. doi:10.1146/annurev.genet.35.102401.091302
Dalal, C. K., Cai, L., Lin, Y., Rahbar, K., and Elowitz, M. B. (2014). Pulsatile dynamics in the yeast proteome. Curr. Biol. 24, 2189–2194. doi:10.1016/j.cub.2014.07.076
Das, D., Paries, M., Hobecker, K., Gigl, M., Dawid, C., Lam, H. M., et al. (2022). Phosphate starvation response transcription factors enable arbuscular mycorrhiza symbiosis. Nat. Commun. 13, 477–488. doi:10.1038/s41467-022-27976-8
Davis, T. N., Urdea, M. S., Masiarz, F. R., and Thorner, J. (1986). Isolation of the yeast calmodulin gene: Calmodulin is an essential protein. Cell 47, 423–431. doi:10.1016/0092-8674(86)90599-4
Docampo, R., and Moreno, S. N. (2011). Acidocalcisomes. Cell Calcium 50, 113–119. doi:10.1016/j.ceca.2011.05.012
Docampo, R., Ulrich, P., and Moreno, S. N. (2010). Evolution of acidocalcisomes and their role in polyphosphate storage and osmoregulation in eukaryotic microbes. Phil. Trans. R. Soc. Lond. Ser. B, Biol. Sci. 365, 775–784. doi:10.1098/rstb.2009.0179
Domínguez-Santos, R., Kosalková, K., García-Estrada, C., Barreiro, C., Ibáñez, A., Morales, A., et al. (2017). Casein phosphopeptides and CaCl2 increase penicillin production and cause an increment in microbody/peroxisome proteins in Penicillium chrysogenum. J. Proteomics 156, 52–62. doi:10.1016/j.jprot.2016.12.021
Espeso, E. A. (2016). The CRaZy calcium cycle. Adv. Exp. Med. Biol. 892, 169–186. doi:10.1007/978-3-319-25304-6_7
Fedotova, A. A., Bonchuk, A. N., Mogila, V. A., and Georgiev, P. G. (2017). C2H2 zinc finger proteins: The largest but poorly explored family of higher eukaryotic transcription factors. Acta Nat. 9, 47–58. doi:10.32607/20758251-2017-9-2-47-58
Franzen, A. J., Cunha, M. M. L., Miranda, K., Hentschel, J., Plattner, H., Silva, M. B., et al. (2008). Ultrastructural characterization of melanosomes of the human pathogenic fungus Fonsecaea pedrosoi. J. Struct. Biol. 162, 75–84. doi:10.1016/j.jsb.2007.11.004
Fu, C., Donadio, N., Cardenas, M. E., and Heitman, J. (2018). Dissecting the roles of the calcineurin pathway in unisexual reproduction, stress responses, and virulence in Cryptococcus neoformans. Genetics 208, 639–653. doi:10.1534/genetics.117.300422
Garcia, C. R. S., Alves, E., Pereira, P. H. S., Bartlett, P. J., Thomas, A. P., Mikoshiba, K., et al. (2017). InsP3 signaling in apicomplexan parasites. Curr. Top. Med. Chem. 17 (19), 2158–2165.
García-Estrada, C., Ullán, R. V., Albillos, S. M., Fernández-Bodega, A., Durek, P., von Döhren, H., et al. (2011). A single cluster of coregulated genes encodes the biosynthesis of the mycotoxins roquefortine C and meleagrin in Penicillium chrysogenum. Chem. Biol. 18, 1499–1512. doi:10.1016/j.chembiol.2011.08.012
Ghillebert, R., Swinnen, E., De Snijder, P., Smets, B., and Winderickx, J. (2011). Differential roles for the low-affinity phosphate transporters Pho87 and Pho90 in Saccharomyces cerevisiae. Biochem. J. 434, 243–251. doi:10.1042/BJ20101118
Giots, F., Donaton, M. C. V., and Thevelein, J. M. (2003). Inorganic phosphate is sensed by specific phosphate carriers and acts in concert with glucose as a nutrient signal for activation of the protein kinase A pathway in the yeast Saccharomyces cerevisiae. Mol. Microbiol. 47, 1163–1181. doi:10.1046/j.1365-2958.2003.03365.x
Gohain, D., and Tamuli, R. (2019). Calcineurin responsive zinc-finger-1 binds to a unique promoter sequence to upregulate neuronal calcium sensor-1, whose interaction with MID-1 increases tolerance to calcium stress in Neurospora crassa. Mol. Microbiol. 111, 1510–1528. doi:10.1111/mmi.14234
Goswani, R. S., and Kistler, H. C. (2004). Heading for disaster: Fusarium graminearum on cereal crops. Mol. Plant Pathol. 5, 515–525. doi:10.1111/j.1364-3703.2004.00252.x
Gras, D. E., Silveira, H. C. S., Martínez-Rossi, N. M., and Rossi, A. (2007). Identification of genes displaying differential expression in the nuc-2 mutant strain of the mold Neurospora crassa grown under phosphate starvation. FEMS Microbiol. Lett. 269, 196–200. doi:10.1111/j.1574-6968.2006.00613.x
Gupta, S., Kumar, A., and Tamuli, R. (2022). CRZ1 transcription factor is involved in cell survival, stress tolerance, and virulence in fungi. J. Biosci. 47, 66–86. doi:10.1007/s12038-022-00294-3
Hagiwara, D., Kondo, A., Fujioka, T., and Abe, K. (2008). Functional analysis of C2H2 zinc finger transcription factor CrzA involved in calcium signaling in Aspergillus nidulans. Curr. Genet. 54, 325–338. doi:10.1007/s00294-008-0220-z
Hilioti, Z., Gallagher, D. A., Low-Nam, S. T., Ramaswamy, P., Gajer, P., Kingsbury, T. J., et al. (2004). GSK-3 kinases enhance calcineurin signaling by phosphorylation of RCNs. Genes Dev. 18, 35–47. doi:10.1101/gad.1159204
Hook, S. S., and Means, A. R. (2001). Ca2+/CaM-dependent kinases: From activation to function. Annu. Rev. Pharmacol. Toxicol. 41, 471–505. doi:10.1146/annurev.pharmtox.41.1.471
Hothorn, M., Neumann, H., Lenherr, E. D., Wehner, M., Rybin, V., HassaUttenweiler, A., et al. (2009). Catalytic core of a membrane-associated eukaryotic polyphosphate polymerase polyphosphate polymerase. Science 324, 513–516. doi:10.1126/science.1168120
Hu, S., Zhou, X., Gu, X., Cao, S., Wang, C., and Xu, J. (2014). The cAMP-PKA pathway regulates growth, sexual and asexual differentiation, and pathogenesis in Fusarium graminearum. Mol. Plant Microbe Interact. 27, 557–566. doi:10.1094/MPMI-10-13-0306-R
Huang, G., Ulrich, P. N., Storey, M., Johnson, D., Tischer, J., Tovar, J. A., et al. (2014). Proteomic analysis of the acidocalcisome, an organelle conserved from bacteria to human cells. PLoS Pathog. 10, e1004555. doi:10.1371/journal.ppat.1004555
Huber, A., Hajdu, D., Rratschun-Khan, D., Gáspári, Z., Varbanov, M., Philippot, S., et al. (2018). New antimicrobial potential and structural properties of pafb: A cationic, cysteine-rich protein from Penicillium chrysogenum Q176. Sci. Rep. 8, 1751–1767. doi:10.1038/s41598-018-20002-2
Hürlimann, H, C., Pinson, B., Stadler-Waibel, M., Zeeman, S. C., and Freimoser, F. M. (2009). The SPX domain of the yeast low-affinity phosphate transporter Pho90 regulates transport activity. EMBO Rep. 10, 1003–1008. doi:10.1038/embor.2009.105
Huttner, I. G., Strahl, T., Osawa, M., King, D. S., Ames, J. B., and Thorner, J. (2003). Molecular interactions of yeast frequenin (frq1) with the phosphatidylinositol 4-kinase isoform Pik1. J. Biol. Chem. 278, 4862–4874. doi:10.1074/jbc.M207920200
Ihara, M., Hamamoto, S., Miyanoiri, Y., Takeda, M., Kainosho, M., Yabe, I., et al. (2013). Molecular bases of multimodal regulation of a fungal transient receptor potential (TRP) channel. J. Biol. Chem. 288, 15303–15317. doi:10.1074/jbc.M112.434795
Imazaki, A., Tanaka, A., Harimoto, Y., Yamamoto, M., Akimitsu, K., Park, P., et al. (2010). Contribution of peroxisomes to secondary metabolism and pathogenicity in the fungal plant pathogen Alternaria alternata. Eukaryot. Cell 9, 682–694. doi:10.1128/EC.00369-09
Jackson, S. L., and Heath, I. B. (1993). Roles of calcium ions in hyphal tip growth. Microbiol. Rev. 57, 367–382. doi:10.1128/mr.57.2.367-382.1993
Jiang, L., and Pan, H. (2018). Functions of CaPhm7 in the regulation of ion homeostasis, drug tolerance, filamentation and virulence in Candida albicans. BMC Microbiol. 18, 49–57. doi:10.1186/s12866-018-1193-9
Jiang, L., and Yang, Y. (2018). The putative transient receptor potential channel protein encoded by the orf19.4805 gene is involved in cation sensitivity, antifungal tolerance, and filamentation in Candida albicans. Can. J. Microbiol. 64, 727–731. doi:10.1139/cjm-2018-0048
Jiang, Q., Li, Y., Mao, R., Bi, Y., Liu, Y., Zhang, M., et al. (2023). AaCaMKs positively regulate development, infection structure differentiation and pathogenicity in Alternaria alternata, causal agent of pear black spot. Int. J. Mol. Sci. 24 (2), 1381.
Jiao, M., Yu, D., Tan, C. L., Guo, J., Lan, D. Y., Han, E., et al. (2017). Basidiomycete-specific PsCaMKL1 encoding a CaMK-like protein kinase is required for full virulence of Puccinia striiformis f. sp. tritici. Environ. Microbiol. 19, 4177–4189. doi:10.1111/1462-2920.13881
Joseph, J. D., and Means, A. R. (2000). Identification and characterization of two Ca2+/CaM-dependent protein kinases required for normal nuclear division in Aspergillus nidulans. J. Biol. Chem. 275, 38230–38238. doi:10.1074/jbc.M006422200
Juvvadi, P. R., Kuroki, Y., Arioka, M., Nakajima, H., and Kitamoto, K. (2003). Functional analysis of the calcineurin-encoding gene cnaA from Aspergillus oryzae: Evidence for its putative role in stress adaptation. Arch. Microbiol. 179, 416–422. doi:10.1007/s00203-003-0546-3
Juvvadi, P. R., Lamoth, F., and Steinbach, W. J. (2014). Calcineurin as a multifunctional regulator: Unraveling novel functions in fungal stress responses, hyphal growth, drug resistance, and pathogenesis. Fungal Biol. Rev. 28, 56–69. doi:10.1016/j.fbr.2014.02.004
Juvvadi, P. R., Ma, Y., Richards, A. D., Soderblom, E. J., Moseley, M. A., Lamoth, F., et al. (2015). Identification and mutational analyses of phosphorylation sites of the calcineurin-binding protein CbpA and the identification of domains required for calcineurin binding in Aspergillus fumigatus. Front. Microbiol. 6, 175–185. doi:10.3389/fmicb.2015.00175
Karunakaran, M., Nair, N. V., Rho, H. S., and Lee, Y. H. (2006). Effects of Ca2+/calmodulin mediated signaling on spore germination and appressorium development in Colletotrichum falcatum Went. Acta Phytopathol. Entomol. hung. 41, 249–260. doi:10.1556/aphyt.41.2006.3-4.8
Kikuchi, Y., Hijikata, N., Yokoyama, K., Ohtomo, R., Handa, Y., Kawaguchi, M., et al. (2014). Polyphosphate accumulation is driven by transcriptome alterations that lead to near-synchronous and near-equivalent uptake of inorganic cations in an arbuscular mycorrhizal fungus. New Phytol. 204, 638–649. doi:10.1111/nph.12937
Kim, J., Park, H., Han, J-G., Oh, J., Choi, H-K., Kim, S. H., et al. (2015). Regulation of a phenylalanine ammonia lyase (BbPAL) by calmodulin in response to environmental changes in the entomopathogenic fungus Beauveria bassiana. Environ. Microbiol. 17, 4484–4494. doi:10.1111/1462-2920.12898
Kim, J., and Sung, G. H. (2018). Beauvericin synthetase contains a calmodulin binding motif in the entomopathogenic fungus Beauveria bassiana. J. Gen. Appl. Microbiol. 64, 145–147. doi:10.2323/jgam.2017.09.003
Kim, J., Yoon, D. H., Oh, J., Hyun, M. W., Han, J. G., and Sung, G. H. (2016). Calmodulin-mediated suppression of 2-ketoisovalerate reductase in Beauveria bassiana beauvericin biosynthetic pathway. Environ. Microbiol. 18, 4136–4143. doi:10.1111/1462-2920.13461
Kim, Y., Li, D., and Kolattukudy, P. E. (1998). Induction of Ca2+-calmodulin signaling by hard-surface contact primes Colletotrichum gloeosporioides conidia to germinate and form appressoria. J. Bacteriol. 180, 5144–5150. doi:10.1128/JB.180.19.5144-5150.1998
Kingsbury, T. J., and Cunningham, K. W. (2000). A conserved family of calcineurin regulators. Genes Dev. 14, 1595–1604. doi:10.1101/gad.14.13.1595
Kishi, T., Ikeda, A., Nagao, R., and Koyama, N. (2007). The SCFCdc4 ubiquitin ligase regulates calcineurin signaling through degradation of phosphorylated Rcn1, an inhibitor of calcineurin. Proc. Natl. Acad. Sci. USA. 104, 17418–17423. doi:10.1073/pnas.0704951104
Klee, C., Ren, H., and Wang, X. (1998). Regulation of the calmodulin-stimulated protein phosphatase, calcineurin. J. Biol. Chem. 273, 13367–13370. doi:10.1074/jbc.273.22.13367
Komeili, A., and O'Shea, E. K. (1999). Roles of phosphorylation sites in regulating activity of the transcription factor Pho4. Science 284, 977–980. doi:10.1126/science.284.5416.977
Kothe, G. O., and Free, S. J. (1998). Calcineurin subunit B is required for normal vegetative growth in Neurospora crassa. Fungal Genet. Biol. 23, 248–258. doi:10.1006/fgbi.1998.1037
Kraus, P. R., and Heitman, J. (2003). Coping with stress: Calmodulin and calcineurin in model and pathogenic fungi. Biophys. Biochem. Res. Comm. 311, 1151–1157. doi:10.1016/s0006-291x(03)01528-6
Kretschmer, M., Reiner, E., Hu, G., Tam, N., Oliveira, D. L., Caza, M., et al. (2014). Defects in phosphate acquisition and storage influence virulence of Cryptococcus neoformans. Infect. Immun. 82, 2697–2712. doi:10.1128/IAI.01607-14
Krishnan, A., Almén, M. S., Fredriksson, R., and Schiöth, H. B. (2012). The origin of GPCRs: identification of mammalian like Rhodopsin, Adhesion, Glutamate and Frizzled GPCRs in fungi. PLoS One 7 (1), e29817.
Kumar, R., and Tamuli, R. (2014). Calcium/calmodulin-dependent kinases are involved in growth, thermotolerance, oxidative stress survival, and fertility in Neurospora crassa. Arch. Microbiol. 196 (4), 295–305. doi:10.1007/s00203-014-0966-2
Lamb, T. M., Xu, W., Diamond, A., and Mitchell, A. P. (2001). Alkaline response genes of Saccharomyces cerevisiae and their relationship to the RIM101 pathway. J. Biol. Chem. 276, 1850–1856. doi:10.1074/jbc.M008381200
Lander, N., Cordeiro, C., Huang, G., and Docampo, R. (2016). Polyphosphate and acidocalcisomes. Biochem. Soc. Trans. 44, 1–6. doi:10.1042/BST20150193
Lee, S. C., and Lee, Y. H. (1999). Calcium/calmodulin-dependent signaling for appressorium formation in the plant pathogenic fungus Magnaporthe grisea. Mol. Cells 8, 698–704.
Lev, S., Kaufman-Francis, K., Desmarini, D., Juillard, P. G., Li, C., Stifter, S. A., et al. (2017). Pho4 is essential for dissemination of Cryptococcus neoformans to the host brain by promoting phosphate uptake and growth at alkaline pH. mSphere 2, 003811–e416. doi:10.1128/mSphere.00381-16
Levy, S., Kafri, M., Carmi, M., and Barkai, N. (2011). The competitive advantage of a dual-transporter system. Science 334, 1408–1412. doi:10.1126/science.1207154
Li, H., Rao, A., and Hogan, P. G. (2011). Interaction of calcineurin with substrates and targeting proteins. Trends Cell Biol. 21, 91–103. doi:10.1016/j.tcb.2010.09.011
Lim, F. Y., and Keller, N. P. (2014). Spatial and temporal control of fungal natural product synthesis. Nat. Prod. Rep. 31, 1277–1286. doi:10.1039/c4np00083h
Liu, C., Liu, T., Lv, Z., Qin, M., Qu, Z., Zhang, Z., et al. (2022). A calcineurin regulator MoRCN1 is important for asexual development, stress response, and plant infection of Magnaporthe oryzae. Front. Plant Sci. 13, 925645–925659. doi:10.3389/fpls.2022.925645
Liu, X. H., Lu, J. P., Dong, B., Gu, Y., and Lin, F. C. (2009). Disruption of MoCMK1, encoding a putative calcium/calmodulin-dependent kinase in Magnaporthe oryzae. Microbiol. Res. 8, 7. doi:10.1016/j.micres.2009.08.007
Ma, X., Shi, J., Li, Y., and Han, Y. (2020). Ascospore germination characteristics and the ultrastructure observation of fruit body of Pseudopeziza medicaginis. Microbiology 47, 3564–3576.
Martín, J. F., Casqueiro, J., Kosalková, K., Marcos, A. T., and Gutiérrez, S. (1999). Penicillin and cephalosporin biosynthesis: Mechanism of carbon catabolite regulation of penicillin production. Leeuwen. 75, 21–31. doi:10.1023/a:1001820109140
Martín, J. F., Casqueiro, J., and Liras, P. (2005). Secretion systems for secondary metabolites: How producer cells send out messages of intercellular communication. Curr. Opin. Microbiol. 8, 282–293. doi:10.1016/j.mib.2005.04.009
Martín, J. F. (1977). “Control of the synthesis of antibiotics by phosphate,” in Advances in biochemical engineering Editors T. J. Ghose, A. Fietcher, and N. Blakebrough (Berlin: Springer-Verlag), 105–127.
Martín, J. F., and Demain, A. L. (1980). Control of antibiotic biosynthesis. Microbiol. Rev. 44, 230–251. doi:10.1128/mr.44.2.230-251.1980
Martín, J. F., and Liras, P. (2022a). Comparative molecular mechanisms of biosynthesis of naringenin and related chalcones in actinobacteria and plants: Relevance for the obtention of potent bioactive metabolites. Antibiotics 11, 82–103. doi:10.3390/antibiotics11010082
Martín, J. F., and Liras, P. (2021a). Molecular mechanisms of phosphate sensing, transport and signalling in Streptomyces and related actinobacteria. Int. J. Mol. Sci. 22, 1129–1149. doi:10.3390/ijms22031129
Martín, J. F., and Liras, P. (2021b). The PenV vacuolar membrane protein that controls penicillin biosynthesis is a putative member of a subfamily of stress-gated transient receptor calcium channels. Curr. Res. Biotechnol. 3, 317–322. doi:10.1016/j.crbiot.2021.11.004
Martín, J. F., and Liras, P. (2022b). Vacuolal and peroxisomal calcium ion transporters in yeasts and fungi: Key role in the translocation of intermediates in the biosynthesis of fungal metabolites. Genes 13, 1450–1467. doi:10.3390/genes13081450
Martín, J. F. (2004). Phosphate control of the biosynthesis of antibiotics and other secondary metabolites is mediated by the PhoR-PhoP system: An unfinished story. J. Bacteriol. 186, 5197–5201. doi:10.1128/JB.186.16.5197-5201.2004
Martín, J. F. (2020). Transport systems, intracellular traffic of intermediates and secretion of β-lactam antibiotics in fungi. Fungal Biol. Biotechnol. 7, 6–21. doi:10.1186/s40694-020-00096-y
Martín, J. F., van den Berg, M. A., ver Loren van Themaat, E., and Liras, P. (2019). Sensing and transduction of nutritional and chemical signals in filamentous fungi: Impact on cell development and secondary metabolites biosynthesis. Biotechnol. Adv. 37, 107392–107407. doi:10.1016/j.biotechadv.2019.04.014
Martínez, P., and Persson, B. L. (1998). Identification, cloning and characterization of a derepressible Na+-coupled phosphate transporter in Saccharomyces cerevisiae. Mol. Gen. Genet. 258, 628–638. doi:10.1007/s004380050776
Mehta, S., Li, H., Hogan, P. G., and Cunningham, K. W. (2009). Domain architecture of the regulators of calcineurin (RCANs) and identification of a divergent RCAN in yeast. Mol. Cell. Biol. 29, 2777–2793. doi:10.1128/MCB.01197-08
Melcher, L., and Thorner, J. (1996). Identification and characterization of the CLK1 gene product, a novel CaM kinase-like protein kinase from the yeast Saccharomyces cerevisiae. J. Biol. Chem. 271, 29958–29968. doi:10.1074/jbc.271.47.29958
Meyer, V., Spielvogel, A., Funk, L., Tilburn, J., Arst, H. N., and Stahl, U. (2005). Alkaline pH-induced up-regulation of the afp gene encoding the antifungal protein (AFP) of Aspergillus giganteus is not mediated by the transcription factor PacC: Possible involvement of calcineurin. Mol. Gen. Genomics 274, 295–306. doi:10.1007/s00438-005-0002-y
Meyer, V., and Stahl, U. (2002a). New insights in the regulation of the afp gene encoding the Antifungal Protein of Aspergillus giganteus. Curr. Genet. 42, 36–42. doi:10.1007/s00294-002-0324-9
Meyer, V., Wedde, M., and Stahl, U. (2002b). Transcriptional regulation of the antifungal protein in Aspergillus giganteus. Mol. Genet. Genomics 266, 747–757. doi:10.1007/s00438-001-0609-6
Mota, A. O., Malavazi, I., Soriani, F. M., Heinekamp, T., Jacobsen, I., Brakhage, A. A., et al. (2008). Molecular characterization of the Aspergillus fumigatus NCS-1 homologue. NcsA. Mol. Genet. Genomics. 280 (6), 483–495. doi:10.1007/s00438-008-0381
Mouillon, J-M., and Persson, B. L. (2006). New aspects on phosphate sensing and signalling in Saccharomyces cerevisiae. FEMS Yeast Res. 6, 171–176. doi:10.1111/j.1567-1364.2006.00036.x
Mulinti, P., Allen, N. A., Coyle, C. M., Gravelat, F. N., Sheppard, D. C., and Panaccione, D. G. (2014). Accumulation of ergot alkaloids during conidiophore development in Aspergillus fumigatus. Curr. Microbiol. 68, 1–5. doi:10.1007/s00284-013-0434-2
Nosanchuk, J. D., and Casadevall, A. (2006). Impact of melanin on microbial virulence and clinical resistance to antimicrobial compounds. Antimicrob. Agents Chemother. 50, 3519–3528. doi:10.1128/AAC.00545-06
O’Day, D. H. (2003). CaMBOT: Profiling and characterizing calmodulin-binding proteins. Cell. Signal. 15, 347–354. doi:10.1016/s0898-6568(02)00116-x
Ogawa, N., DeRisi, J., and Brown, P. O. (2000). New components of a system for phosphate accumulation and polyphosphate metabolism in Saccharomyces cerevisiae revealed by genomic expression analysis. Mol. Biol. Cell 11, 4309–4321. doi:10.1091/mbc.11.12.4309
O´Neill, E. M., Kaffman, A., Jolly, E. R., and O’Shea, E. K. (1996). Regulation of PHO4 nuclear localization by the PHO80–PHO85 cyclinCDK complex. Science 271, 209–212. doi:10.1126/science.271.5246.209
Peleg, Y., Addison, R., Aramayo, R., and Metzenberg, R. L. (1996). Translocation of Neurospora crassa transcription factor NUC-1 into the nucleus is induced by phosphorus limitation. Fungal Genet. Biol. 20, 185–191. doi:10.1006/fgbi.1996.0034
Persson, B. L., Lagerstedt, J. O., Pratt, J. R., Pattison-Granberg, J., Lundh, K., Shokrollahzadeh, S., et al. (2003). Regulation of phosphate acquisition in Saccharomyces cerevisiae. Curr. Genet. 43, 225–244. doi:10.1007/s00294-003-0400-9
Persson, B. L., Petersson, J., Fristedt, , Weinander, R., Berhe, A., and Pattison, J. (1999). Phosphate permeases of Saccharomyces cerevisiae: Structure, function and regulation. Biochim. Biophys. Acta 1422, 255–272. doi:10.1016/s0304-4157(99)00010-6
Pestka, J. J., and Smolinski, A. T. (2005). Deoxynivalenol: Toxicology and potential effects on humans. J. Toxicol. Env. Heal. B 8, 39–69. doi:10.1080/10937400590889458
Pinchai, N., Perfect, B. Z., Juvvadi, P. R., Fortwendel, J. R., Cramer, R. A., Asfaw, Y. G., et al. (2009). Aspergillus fumigatus calcipressin CbpA is involved in hyphal growth and calcium homeostasis. Eukaryot. Cells 8, 511–519. doi:10.1128/EC.00336-08
Popova, Y., Thayumanavan, P., Lonati, E., Agrochão, M., and Thevelein, J. M. (2010). Transport and signaling through the phosphate-binding site of the yeast Pho84 phosphate transceptor. Proc. Natl. Acad. Sci. U. S. A. 107, 2890–2895. doi:10.1073/pnas.0906546107
Prokisch, H., Yarden, O., Dieminger, M., Tropschug, M., and Barthelmess, I. B. (1997). Impairment of calcineurin function in Neurospora crassa reveals its essential role in hyphal growth, morphology and maintenance of the apical Ca2+ gradient. Mol. Gen. Genet. 256, 104–114. doi:10.1007/s004380050551
Prole, D. L., and Taylor, C. W. (2011). Identification of intracellular and plasma membrane calcium channel homologues in pathogenic parasites. PLoS One 6 (10), e26218. doi:10.1371/journal.pone.0026218
Prole, D. L., and Taylor, C. W. (2019). Structure and function of IP3 receptors. Cold Spring Harb. Perspect. Biol. 11(4), a035063. doi:10.1101/cshperspect.a035063
Rao, K. K., and Gupta, A. R. (1975). Inhibition of tryptophan synthetase by phosphate in alkaloid-producing culture of Aspergillus fumigatus. Naturwissenschaften 62, 394–395. doi:10.1007/BF00625354
Rasmussen, C. D. (2000). Cloning of a calmodulin kinase I homologue from Schizosaccharomyces pombe. J. Biol. Chem. 275, 685–690. doi:10.1074/jbc.275.1.685
Rodrígues, A., Roy, J., Martínez-Martínez, S., López-Maderuelo, D., Niño-Moreno, P., Ortí, L., et al. (2009). A conserved docking surface on calcineurin mediates interaction with substrates and immunosuppressants. Mol. Cell. 33, 616–626. doi:10.1016/j.molcel.2009.01.030
Rodrígues, M. L., Nakayasu, E. S., Oliveira, D. L., Nimrichter, L., Nosanchuk, J. D., Almeida, I. C., et al. (2008). Extracellular vesicles produced by Cryptococcus neoformans contain protein components associated with virulence. Eukaryot. Cell 7, 58–67. doi:10.1128/EC.00370-07
Rudolph, H., and Hinnen, A. (1987). The yeast PHO5 promoter: Phosphate-control elements and sequences mediating mRNA start-site selection. Proc. Natl. Acad. Sci. U. S. A. 84, 1340–1344. doi:10.1073/pnas.84.5.1340
Saitoh, K., Arie, T., Teraoka, T., Yamaguchi, I., and Kamakura, T. (2003). Targeted gene disruption of the neuronal calcium sensor 1 homologue in rice blast fungus, Magnaporthe grisea. Biosci. Biotechnol. Biochem. 67, 651–653. doi:10.1271/bbb.67.651
Samyn, D. R., Ruiz-Pávon, L., Andersson, M. R., Popova, Y., Thevelein, J. M., and Persson, B. L. (2012). Mutational analysis of putative phosphate- and proton-binding sites in the Saccharomyces cerevisiae Pho84 phosphate:H(+) transceptor and its effect on signalling to the PKA and PHO pathways. Biochem. J. 445, 413–422. doi:10.1042/BJ20112086
Sánchez-Gracia, A., Romero-Pozuelo, J., and Ferrús, A. (2010). Two frequenins in Drosophila: Unveiling the evolutionary history of an unusual neuronal calcium sensor (NCS) duplication. BMC Evol. Biol. 19 (10), 54. doi:10.1186/1471-2148-10-54
Schneider, K. R., Smith, R. L., and O’ Shea, E. K. (1994). Phosphate-regulated inactivation of the kinase PHO80–PHO85 by the CDK inhibitor PHO81. Science 266, 122–126. doi:10.1126/science.7939631
Schumacher, J., de Larrinoa, I. F., and Tudzynski, B. (2008). Calcineurin responsive zinc finger transcription factor CRZ1 of Botrytis cinerea is required for growth, development, and full virulence on bean plants. Eukaryot. Cell 7, 584–601. doi:10.1128/EC.00426-07
Secco, D., Wang, C., Arpat, B. A., Wang, Z., Poirier, Y., Tyerman, S. D., et al. (2012b). The emerging importance of the SPX domain-containing proteins in phosphate homeostasis. New Phytol. 193, 842–851. doi:10.1111/j.1469-8137.2011.04002.x
Secco, D., Wang, C., Shou, H., and Whelan, J. (2012a). Phosphate homeostasis in the yeast Saccharomyces cerevisiae, the key role of the SPX domain-containing proteins. FEBS Lett. 586, 289–295. doi:10.1016/j.febslet.2012.01.036
Serra-Cardona, A., Petrezsélyová, S., Canadell, D., Ramos, J., and Ariño, J. (2014). Coregulated expression of the Na+/phosphate Pho89 transporter and Ena1 Na+-ATPase allows their functional coupling under high-pH stress. Mol. Cell Biol. 4, 4420–4435. doi:10.1128/MCB.01089-14
Serrano, R., Ruiz, A., Bernal, D., Chambers, J. R., and Arino, J. (2002). The transcriptional response to alkaline pH in Saccharomyces cerevisiae: Evidence for calcium-mediated signalling. Mol. Microbiol. 46, 1319–1333. doi:10.1046/j.1365-2958.2002.03246.x
Seufferheld, M. J., Kim, K. M., Whitfield, J., Valerio, A., and Caetano-Anolles, G. (2011). Evolution of vacuolar proton pyrophosphatase domains and volutin granules: Clues into the early evolutionary origin of the acidocalcisome. Biol. Direct. 6, 50–65. doi:10.1186/1745-6150-6-50
Silva-Ferreira, M. E. de, Heinekamp, T., Härtl, A., Brakhage, A. A., Semighini, C. P., Harris, S. D., et al. (2007). Functional characterization of the Aspergillus fumigatus calcineurin. Fungal Gen. Biol. 44, 219–230. doi:10.1016/j.fgb.2006.08.004
Sola-Landa, A., Moura, R. S., and Martín, J. F. (2003). The two component PhoR-PhoP system controls both primary metabolism and secondary metabolite biosynthesis in Streptomyces lividans. Proc. Natl. Acad. Sci. USA. 100, 6133–6138. doi:10.1073/pnas.0931429100
Soriani, F. M., Malavazi, I., da Silva Ferreira, M. A., Savoldi, M., Von Zeska Kress, M. R., de Souza Goldman, M. H., et al. (2008). Functional characterization of the Aspergillus fumigatus CRZ1 homologue, CrzA. CrzA. Mol. Microbiol. 67, 1274–1291. doi:10.1111/j.1365-2958.2008.06122.x
Soriani, F. M., Malavazi, I., Savoldi, M., Espeso, E., Dinamarco, T. M., Bernardes, L. A. S., et al. (2010). Identification of possible targets of the Aspergillus fumigatus CRZ1 homologue, CrzA. BMC Microbiol. 15, 12. doi:10.1186/1471-2180-10-12
Spielvogel, A., Findon, H., Arst, H. N., Araújo-Bazán, L., Hernández-Ortíz, P., Stahl, U., et al. (2008). Two zinc finger transcription factors, CrzA and SltA, are involved in cation homoeostasis and detoxification in Aspergillus nidulans. Biochem. J. 414, 419–429. doi:10.1042/BJ20080344
Stathopoulos, A. M., and Cyert, M. S. (1997). Calcineurin acts through theCRZ1/TCN1-encoded transcription factor to regulate gene expression in yeast. Genes Dev. 11, 3432–3444. doi:10.1101/gad.11.24.3432
Steger, D. J., Haswell, E. S., Miller, A. L., Wente, S. R., and O’Shea, E. K. (2003). Regulation of chromatin remodeling by inositol polyphosphates. Science 299, 114–116. doi:10.1126/science.1078062
Strahl, T., Huttner, I. G., Lusin, J. D., Osawa, M., King, D., Thorner, J., et al. (2007). Structural insights into activation of phosphatidylinositol 4-kinase (Pik1) by yeast frequenin (Frq1). J. Biol. Chem. 82, 30949–30959. doi:10.1074/jbc.M705499200
Tamuli, R., Kumar, R., and Rekha Deka, R. (2011). Cellular roles of neuronal calcium sensor-1 and calcium/calmodulin-dependent kinases in fungi. J. Basic Microbiol. 511, 120–128.
Tamuli, R., Deka, R., and Borkovich, K. A. (2016). Calcineurin subunits A and B interact to regulate growth and asexual and sexual development in Neurospora crassa. PLoS One 11, e0151867. doi:10.1371/journal.pone.0151867
Taylor, C. W., and Machaca, K. (2019). IP3 receptors and store-operated Ca2+ entry: A license to fill. Curr. Opin. Cell Biol. 57, 1–7. doi:10.1016/j.ceb.2018.10.001
Taylor, C. W. (2017). Regulation of IP3 receptors by cyclic AMP. Cell Calcium 63, 48–52. doi:10.1016/j.ceca.2016.10.005
Theis, T., Marx, F., Salvenmoser, W., Stahl, U., and Meyer, V. (2005). New insights into the target site and mode of action of the antifungal protein of Aspergillus giganteus. Res. Microbiol. 156, 47–56. doi:10.1016/j.resmic.2004.08.006
Thewes, S. (2014). Calcineurin-Crz1 signaling in lower eukaryotes. Eukaryot. Cells 13, 694–705. doi:10.1128/EC.00038-14
Tisi, R., Rigamonti, M., Groppi, S., and Belotti, F. (2016). Calcium homeostasis and signaling in fungi and their relevance for pathogenicity of yeasts and filamentous fungi. AIMS Mol. Sci. 3, 505–549. doi:10.3934/molsci.2016.4.505
Tomar, P., and Sinha, H. (2014). Conservation of PHO pathway in ascomycetes and the role of Pho84. J. Biosci. 39, 525–536. doi:10.1007/s12038-014-9435-y
Tomitori, H., Kashiwagi, K., Asakawa, T., Kakinuma, Y., Michael, A. J., and Igarashi, K. (2001). Multiple polyamine transport systems on the vacuolar membrane in yeast. Biochem. J. 353, 681–688. doi:10.1042/0264-6021:3530681
Toprak, U., Doğan, C., and Hegedus, D. (2021). A comparative perspective on functionally-related, intracellular calcium channels: The insect ryanodine and inositol 1,4,5-trisphosphate receptors. Biomolecules 11 (7), 1031. doi:10.3390/biom11071031
Tsai, H. C., and Chung, K. R. (2014). Calcineurin phosphatase and phospholipase C are required for developmental and pathological functions in the citrus fungal pathogen Alternaria alternata. Microbiology 160, 1453–1465. doi:10.1099/mic.0.077818-0
Valle-Aviles, L., Valentin-Berrios, S., Gonzalez-Mendez and, R. R., and Rodriguez-del, V. N. (2007). Functional, genetic and bioinformatic characterization of a calcium/calmodulin kinase gene in Sporothrix schenckii. BMC Microbiol. 7, 107. doi:10.1186/1471-2180-7-107
Venkatachalam, K., and Montell, C. (2007). TRP channels. Annu. Rev. Biochem. 76, 387–417. doi:10.1146/annurev.biochem.75.103004.142819
Viaud, M., Brunet-Simon, A., Brypoo, Y., Pradier, J. M., and Levis, C. (2003). Cyclophilin A and calcineurin functions investigated by gene inactivation, cyclosporin A inhibition and cDNA arrays approaches in the phytopathogenic fungus Botrytis cinerea. Mol. Microbiol. 50, 1451–1465. doi:10.1046/j.1365-2958.2003.03798.x
Viaud, M. C., Balhadere, P. V., and Talbot, N. J. (2002). A Magnaporthe grisea cyclophilin acts as a virulence determinant during plant infection. Plant Cell 14, 917–930. doi:10.1105/tpc.010389
Vila, T., Frases, S., and Gomes, F. M. (2022). Lessons from protozoans: Phosphate sensing and polyphosphate storage in fungi. PLoS Pathog. 18, e1010298. doi:10.1371/journal.ppat.1010298
Viladevall, L., Serrano, R., Ruiz, A., Domenech, G., Giraldo, J., Barcelo, A., et al. (2004). Characterization of the calcium-mediated response to alkaline stress in Saccharomyces cerevisiae. J. Biol. Chem. 279, 43614–43624. doi:10.1074/jbc.M403606200
Wang, J., Xie, Y. X., Pu, J. J., Zhang, X., Qi, Y. X., and Huang, J. S. (2008). Calcium/calmodulin-dependent signaling for prepenetration development in Phyllosticta musarum. Chin. J. Trop. Crops 29, 641–647.
Wang, J., Zhao, Y. G., and Maqbool, F. (2021). Capability of Penicillium oxalicum y2 to release phosphate from different insoluble phosphorus sources and soil. Folia Microbiol. 66, 69–77. doi:10.1007/s12223-020-00822-4
Wera, S., Bergsma, J. C., and Thevelein, J. M. (2001). Phosphoinositides in yeast: Genetically tractable signalling. FEMS Yeast Res. 1 (1), 9–13. doi:10.1111/j.1567-1364.2001.tb00008.x
Werner, A., and Kinne, R. K. H. (2001). Evolution of the Na-Pi cotransport systems. Am. J. Physiol. Reg. Integ. Comp. Physiol. 280, R301–R312. doi:10.1152/ajpregu.2001.280.2.R301
Wild, R., Gerasimaite, R., Jung, J-Y., Truffault, V., Pavlovic, I., Schmidt, A., et al. (2016). Control of eukaryotic phosphate homeostasis by inositol polyphosphate sensor domains. Science 352, 986–990. doi:10.1126/science.aad9858
Wu, D., Zhao, Z. T., Li, Z. Y., Yang, Z., Zha, Y. H., Sheng, K., et al. (2015). Identification and expression pattern analysis of calcium/calmodulin-dependent protein kinases (CaMKs) gene family in Setosphaeria turcica. J. Agric. Biotechnol. 23, 1020–1030.
Wykoff, D. D., and O’Shea, E. K. (2001). Phosphate transport and sensing in Saccharomyces cerevisiae. Genetics 59, 1491–1499. doi:10.1093/genetics/159.4.1491
Wykoff, D. D., Rizvi, A. H., Raser, J. M., Margolin, B., and O’Shea, E. K. (2007). Positive feedback regulates switching of phosphate transporters in S. cerevisiae regulates switching of phosphate transporters in S. cerevisiae. Mol. Cell 27, 1005–1013. doi:10.1016/j.molcel.2007.07.022
Xiong, D., Wang, Y., Tang, C., Fang, Y., Zou, J., and Tian, C. (2015). VdCrz1 is involved in microsclerotia formation and required for full virulence in Verticillium dahliae. Fungal Genet. Biol. 82, 201–212. doi:10.1016/j.fgb.2015.07.011
Yang, Y., Cheng, P., Zhi, G., and Liu, Y. (2001). Identification of a calcium/calmodulin-dependent protein kinasethat phosphorylates the Neurospora circadian clock protein FREQUENCY. J. Biol. Chem. 276, 41064–41072. doi:10.1074/jbc.M106905200
Yoshikawa, S., Tanimura, T., Miyawaki, A., Nakamura, M., Yuzaki, M., Furuichi, T., et al. (1992). Molecular cloning and characterization of the inositol 1,4,5-trisphosphate receptor in Drosophila melanogaster. J. Biol. Chem. 267, 16613–16619. doi:10.1016/s0021-9258(18)42047-9
Yoshimoto, H., Saltsman, K., Gasch, A. P., Li, H. X., Ogawa, N., Botstein, D., et al. (2002). Genome-wide analysis of gene expression regulated by the calcineurin/Crz1p signaling pathway in Saccharomyces cerevisiae. J. Biol. Chem. 277, 31079–31088. doi:10.1074/jbc.M202718200
Yu, F., Gu, Q., Yun, Y., Yin, Y., Xu, J., Shim, W., et al. (2014). The TOR signaling pathway regulates vegetative development and virulence in Fusarium graminearum. New Phytol. 203, 219–232. doi:10.1111/nph.12776
Yun, Y., Liu, Z., Yin, Y., Jiang, J., Chen, Y., Xu, J. R., et al. (2015). Functional analysis of the Fusarium graminearum phosphatome. New Phytol. 207, 119–134. doi:10.1111/nph.13374
Zelter, A., Bencina, M., Bowman, B. J., Yarden, O., and Read, N. D. (2004). A comparative genomic analysis of the calcium signaling machinery in Neurospora crassa, Magnaporthe grisea, and Saccharomyces cerevisiae. Fungal Genet. Biol. 41 (9), 827–841. doi:10.1016/j.fgb.2004.05.001
Zhang, H. F., Zheng, X. B., and Zhang, Z. G. (2016b). The Magnaporthe grisea species complex and plant pathogenesis. Mol. Plant Pathol. 17, 796–804. doi:10.1111/mpp.12342
Zhang, S. P., Yang, L. N., Li, L. W., Zhong, K. L., Wang, W. H., Liu, M. X., et al. (2019). System-wide characterization of MoArf GTPase family proteins and adaptor protein MoGga1 involved in the development and pathogenicity of Magnaporthe oryzae. mBio 10, 023988–e2419. doi:10.1128/mBio.02398-19
Zhang, T., Xu, Q., Sun, X., Li, H., Wang, Y., and Zheng, X. (2009). MgCRZ1, a transcription factor of Magnaporte grisea, controls growth, development and is involved in full virulence. FEMS Microbiol. Lett. 293, 160–169. doi:10.1111/j.1574-6968.2009.01524.x
Zhen, Z., Zhang, G., Yang, L., Ma, N., Li, Q., Ma, Y., et al. (2019). Characterization and functional analysis of calcium/calmodulin-dependent protein kinases (CaMKs) in the nematode-trapping fungus Arthrobotrys oligospora. Appl. Microbiol. Biotechnol. 103 (2), 819–832. doi:10.1007/s00253-018-9504-5
Zheng, W., Zheng, H., Zhao, X., Zhang, Y., Xie, Q., Lin, X., et al. (2016). Retrograde trafficking from the endosome to the trans-Golgi network mediated by the retromer is required for fungal development and pathogenicity in Fusarium graminearum. New Phytol. 210, 1327–1343. doi:10.1111/nph.13867
Zhou, X., and O’Shea, E. K. (2011). Integrated approaches reveal determinants of genome-wide binding and function of the transcription factor Pho4. Mol. Cell 42, 826–836. doi:10.1016/j.molcel.2011.05.025
Zhu, M. X. (2005). Multiple roles of calmodulin and other Ca(2+)-binding proteins in the functional regulation of TRP channels. Pflugers Arch. 451, 105–115. doi:10.1007/s00424-005-1427-1
Keywords: filamentous fungi, secondary metabolites biosynthesis, phosphate control, Pho4 regulator, inositol pyrophosphates, calcium homeostasis, Crz1(CrzA) transcriptional factor, acidocalcisomes
Citation: Martín JF (2023) Interaction of calcium responsive proteins and transcriptional factors with the PHO regulon in yeasts and fungi. Front. Cell Dev. Biol. 11:1225774. doi: 10.3389/fcell.2023.1225774
Received: 19 May 2023; Accepted: 24 July 2023;
Published: 03 August 2023.
Edited by:
Daniel G. S. Capelluto, Virginia Tech, United StatesReviewed by:
Guanggan Hu, University of British Columbia, CanadaRenata Tisi, University of Milano-Bicocca, Italy
Copyright © 2023 Martín. This is an open-access article distributed under the terms of the Creative Commons Attribution License (CC BY). The use, distribution or reproduction in other forums is permitted, provided the original author(s) and the copyright owner(s) are credited and that the original publication in this journal is cited, in accordance with accepted academic practice. No use, distribution or reproduction is permitted which does not comply with these terms.
*Correspondence: Juan F. Martín, amYubWFydGluQHVuaWxlb24uZXM=